Syngas fermentation to ethanol: CODH/AdhE1 gene expression and microbial community dynamics†
Received
7th April 2023
, Accepted 14th July 2023
First published on 10th August 2023
Abstract
Biosynthesis of ethanol through syngas fermentation employing enriched microbial consortia offers resilience to operate under non-sterile conditions with energy efficiency. Gas fermentation was performed by varying C1 feedstock with reference to selective enrichment of microbial consortia to maximize ethanol production with simultaneous process parameter optimization. Three different operating conditions (R1-CO2 + HCO3−, R2-syngas, and R3-syngas + HCO3−) were evaluated using diverse shock-based enrichment strategies (heat-shock, acid-shock and BESA). R2 and R3 systems showed potential for ethanologenic conversion of C1 gases. The highest ethanol (0.38 g L−1) and acetic acid (2.1 g L−1) yields were obtained in the R3 conditions using BESA-treated culture. Upregulation of genes involved in the Wood–Ljungdahl pathway, specifically alcohol dehydrogenase (AdhE1), under the treated conditions correlated with ethanol synthesis. Over-expression of the Ni-protein subunit (CODH) and Fdhl in the treated conditions demonstrated their role in the selective production of ethanol and acetate. Analysis of the microbial community revealed that the R3 conditions showed abundant phyla such as Firmicutes and Actinobacteria, and members of the carboxydotrophs, which possess the coding sequences of the upregulated genes. The treated conditions exhibited a three-fold increase in ethanol biosynthesis with an inorganic carbon fixation rate of 66 mg L−1 h−1, indicating efficient conversion of C1 substrate by the enriched biocatalyst. Higher relative expression and dominance of carboxydotrophs in the microbial diversity depicted the metabolic inclination of enriched biocatalysts towards the conversion of C1 gases.
Environmental significance
The current study holds significant environmental importance, as it focuses on utilizing biological strategies to sequester greenhouse gases. The process involves converting C1 gases into green fuel, which has the potential to effectively reduce greenhouse gas emissions by offering an alternative to fossil fuel usage. The study demonstrates sustainable energy production by using waste gas streams as feedstock for synthesizing acetic acid and ethanol. This approach relies on enriched microbial consortia, which provides several environmental benefits, such as increased resilience and adaptive capacity, as well as non-sterile operation, promoting more sustainable approaches to biochemical production. The research also looks into the microbial community and highlighted the upregulation of specific genes involved in ethanol synthesis, indicating the potential of genetic engineering and biotechnological advancements to improve the productivity.
|
1. Introduction
The escalating energy demands have resulted in depletion of non-renewable resources, necessitating the utilization of conventional, first-generation fuels and consequently leading to the inevitable release of carbon emissions in the form of CO2/CO. Over the past decade, there has been a rapid expansion in the demand for fuel, soaring from less than 20 billion liters annually to over 100 billion liters.1,2 This surge in anthropocentric activities, driven by a global population of 8 billion, poses a detrimental impact on the ecosystem and environment, including the challenges of global warming and resource scarcity.3–5 One potential approach to meet the increasing fuel demand while mitigating C1 emissions involves the synthesis of fuels and chemicals using synthesis gas (syngas) as a feedstock. Syngas, primarily composed of CO, CO2, and H2, which predominantly generated through gasification processes of wood, municipal solid waste, cellulosic biomass, and other sources, which contribute significantly to carbon emissions.6,7
Various strategies have been implemented to capture these carbon emissions and simultaneously synthesized second-generation fuels through biological conversion of C1 gases, which competes with the conventional routes of carbon capture, utilization, and storage making it an important tool for achieving the sustainable development goal (SDG) related to climate action (SDG 13).8 Biological C1 sequestration offers significant ecological restoration benefits and provides an alternative solution to the limitations of conventional mitigation and fuel production processes.1,9,10 The biological C1 gas fermentation processes may be slower than chemical reactions, but they have several advantages such as exclusion of catalyst costs, higher specificity, lower energy consumption, greater resistance to adverse conditions, and independence of H2/CO purity ratio.6,11 Microbial-mediated syngas fermentation was carried by facultative and anaerobic carboxydotrophs through Wood–Ljungdhal pathway (WLP), resulting in the production of carboxylic acids.12,14
The C1 gas fermentation was studied using single strains, including Clostridium carboxidivorans, Clostridium ljungdahlii, Clostridium ragsdale, and other Clostridium species.14,15 The utilization of pure cultures has enabled the assessment of relative gene expression of the Wood–Ljungdahl pathway, specific product profiles, and fermentation kinetics. However, some studies suggested that the use of a single culture in CO fermentation can impede microbial metabolism due to the mildly toxic nature of CO at the active sites of metabolic enzymes, resulting in lower fermentation efficiencies.16 Additionally, single culture fermentation has limitations such as sensitivity to operation, the need for careful selection of potential strains, and cross-contamination by unwanted microbiomes.17,18 An alternative approach to this is the use of mixed microbial consortia for C1 gas fermentation.15,19–21
Notable advantages of mixed culture gas fermentation include the presence of diversified C1 gas-consuming microbes, the ability to allow mild sterilization, robust operation facilities, and a higher capacity for continuous C1 gas exposure.22 Carboxydotrophic acetogens can utilize C1 gases as a carbon source and produce fatty acids. To achieve successful microbial C1 conversion with mixed biocatalysts, various pre-treatment and enrichment strategies have been employed to enhance the sequester efficiency of the consortia.20,23 Several C1 fermentation strategies including heat and chemical pre-treatment (alkali, acid, 2-bromoethano-sulfonic acid, chloroform, etc.), followed by higher bicarbonate and CO2 supplementation, have been performed and evaluated to improve conversion rates and product titres.24,25 Despite the production of the primary metabolite (acetate) in gas fermentation, the biosynthesis of ethanol through syngas fermentation remains a considerable challenge.
The primary objective of this study is to elucidate the ethanologenic potential of gas fermentation by varying C1 feedstock, selectively enriching consortia by evaluating selective gene (CODH/AdhE1) expression, and microbial community distributions. Inorganic carbon fixation and WLP metabolic intermediate products were monitored during the process of C1 gas fixation with 12 experimental variations including 3 control systems. The optimized conditions for ethanol production were discussed with reference to bioprocess parameters, fermentation product yield, selective gene expressions and microbial profiles.
2 Materials and methods
2.1 Parent micro-biome
The anaerobic mixed microbial consortia used in the experiment were collected from sewage treatment plant (STP) located at Amberpet, Hyderabad, Telangana. To remove larger coasre particles, collected sludge was filtered through a stainless-steel mesh sieve (304 grade quality, 350 mm diameter aperture size ∼0.21–0.24 mm) and then the filtered sludge was used for experimental purpose.
2.2 Enrichment of carboxydotrophs
To prevent methanogenic interference in the enrichment of C1 gas-consuming carboxydotrophs, the anaerobic consortia were pre-treated using thermal and chemical shocks. The mixed cultures were treated with heat shock (80 °C for 2 hours) or acid shock (H3PO4, pH 3 for 12 hours), or 2-bromoethanesulfonic acid (BESA; 2 g L−1)5,26,27 to inhibit methanogenic microbiomes. After treatment, the heat-treated culture was allowed to cool down to the ambient temperature, while the acid-treated culture was adjusted to near neutral pH using 1 N NaOH. The treated inoculum was inoculated into separate reactors at a ratio of 15% v/v. Growth medium employed under all the experimental conditions was composed of 0.1 M phosphate buffer (pH 7.0 ± 0.02) with 0.1% resazurin, and 2% L-cysteine as reducing agents.
2.3 Experimental methods
For experimentation, Borosil® screwcap reagent bottles with a total/working volume of 250/200 ml were utilized. To establish an anaerobic environment, a butyl rubber stopper was inserted into the opening of each bottle. After pre-treatment, selective enrichment of carboxydotrophs in consortia was carried out by sparging CO2, syngas (consisting of 35% CO, 30% CO2, 20% H2, and the remaining N2), and bicarbonate (HCO3−) as an additional inorganic substrate (5 g L−1) in different combinations. A total of twelve sets of experimental conditions (three different conditions for each treated culture) were designed and optimized to attain the biosynthesis of alcohol. The twelve experimental conditions were as follows: H-R1 (H + CO2 + HCO3−); H-R2 (H + syn); H-R3 (H + syn + HCO3−) for heat shock culture, A-R1 (A + CO2 + HCO3−); A-R2 (A + syn); A-R3 (A + syn + HCO3−) for acid shock culture, and B-R1 (B + CO2 + HCO3−); B-R2 (B + syn); B-R3 (B + syn + HCO3−) for the BESA culture. A control set of experiments was carried out with untreated cultures, following the C-R1 (control + CO2 + HCO3−); C-R2 (control + syn); C-R3 (control + syn + HCO3−) conditions. CO2, syngas, and hydrogen gases were sparged into each reactor at a flow rate of 0.6 standard cubic centimetres per minute (sccm). A total of seven cycles were performed, with a retention time of seven days for each cycle and the average of seven-cycle data is presented here for better understanding.
2.4 Performance monitoring/analysis
The process parameters such pH, substrate concentration, product formation were assessed at 24-hour intervals to evaluate the system performance. The pH of the samples was monitored using a pH meter (Hanna Instruments; USA). To measure the concentration of total inorganic carbon (TIC), Shimadzu TOC-L Analyzer was used, which detects TIC in terms of parts per million (ppm) using the 680 °C combustion catalytic oxidation method with nitrogen as a carrier gas. The fermentation product profiles (ethanol and volatile fatty acids/VFA) were determined using a Shimadzu LC20A HPLC system having a refractive index detector (RID20A; Shimadzu) and a RezexTM RHM-Monosaccharide H+ column (Phenomenex, India).19,20 Prior to analysis, samples were filtered using 0.2 micron filters and diluted. The eluent used in the HPLC was ultrapure water, with a flow rate of 0.5 mL min−1 in isocratic mode and an injection sample volume of 20 μL.
To comprehend the fixation and fermentation efficiency of the biocatalyst, the rate of inorganic carbon (IC) fixation into the respective fermentation products (VFA, ethanol) was analysed. The amount of IC fixation rate (ICFR; mg L−1 h−1) in the enriched culture with CO2/CO was computed using the following eqn (1).13
| 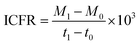 | (1) |
where
M1 is the acetic acid (g L
−1) at
t1 (h) and
M0 is the acetic acid (g L
−1) at
t0 (h).
2.5 Microbial diversity analysis
Denaturing gradient gel electrophoresis (DGGE) was employed for the analysis of microbial diversity.19,20 At the end of the operation, enriched cultures and controls were collected and genomic DNA (gDNA) was extracted using a HiPurA® soil DNA purification kit (HiGenoMB, HiMEDIA, India). The highly conserved region (V3) of the 16S rRNA gene was amplified using a universal forward primer (341F, 5′-CTACGGGAGGCAGCAG-3′) and reverse primer (517R, 5′-ATTACCGCGGCTGCTGG-3′) and a thermal cycler (Applied Biosystems, United States). A GC clamp was added to the forward primer in the PCR reaction for effective separation in the gel. The amplified PCR product was purified using a quick PCR purification kit (Qiagen, USA). Electrophoresis was performed using an 8% polyacrylamide gel with a 30 to 70% denaturant gradient for 8 hours at a constant voltage of 100 V using a Bio-Rad DCode system. Ethidium bromide staining was performed on the casted gel to visualize the separated DNA bands, which were then collected and incubated overnight in 0.5 ml Eppendorf tubes for DNA elution in nuclease-free water. The eluted DNA was used as a template for PCR without the GC clamp in the forward primer to create a specific DNA library by re-amplification. The resulting PCR product was sequenced by the Sanger sequencing method, and the raw FASTA files were blasted using NCBI BLAST program to identify the closest taxonomic relatives. A phylogenetic tree was constructed using MEGA 6.0 software with the neighbour-joining statistical method.28,29
2.6 Relative quantification of gene expression
Genes of the WLP were investigated and identified using their respective locus tags: cooC2 (CLJU_c37660) encodes the CODH nickel-insertion accessory protein, adhE1 codes for alcohol dehydrogenase and Fdhl (CLJU_c08930) codes for formate dehydrogenase subunit alpha. Primer sequences for Fdhl and adhE1 were obtained from Xie et al.30 while the primer sequence for cooC2 was designed using the primer blast tool. All primers were purchased in standard desalted form. Sequences of the primes are as follows:
Primer |
5′ to 3′ sequence |
FdhI-F |
AGCAGTTAGCCGATGAACCA |
FdhI-R |
GGCAACCACAGAACCTCTTC |
adhE1-F |
ATCAGTTGGTGGTGGCTCAG |
adhE1-R |
TTCCTGCGGATGTTGCTACT |
cooC2-F |
ATCTACACCTTGTGCTGTTCC |
cooC2-R |
TTGTTTCTGCCCGGAAAATG |
A quantitative reverse transcription using a dye-based method was carried out with RNA templates, where the volume was adjusted between 1 μL and 3 μL to ensure the use of a constant amount of 10 ng of RNA in each reaction. To verify target specificity, a melt curve was recorded under the recommended conditions for a real-time detection instrument (Light Cycler-96), which involved a ramp in the annealing temperature. In addition, a no template control (NTC) was included and all measurements were conducted in duplicate at least. qPCR data was normalized to the housekeeping gene and the upregulated expressions analyzed using the formula in eqn (2):
3. Results and discussion
3.1 Gas fermentation with ‘N + CO2 + HCO3−’
To synthesize ethanol from C1 gases using mixed consortia, R1 experimental conditions were designed with supplementation of CO2 and bicarbonate. CO2 gas sparging to the R1 systems (heat, acid, BESA, and control) enabled the enrichment of carboxydotrophs. Acetate was found to be the primary metabolite product in the acetyl-CoA reduction pathway, with varying concentrations observed under all R1 conditions. However, ethanol synthesis was not observed under the R1 conditions, indicating limitations in the solventogenic metabolism of carboxydotrophs. During the seven-day fermentation/retention time (RT). The concentration of metabolites, specifically acetate, showed a linear increase until the 4th and 5th days of cycle operation along with cell growth. A maximum concentration of acetic acid (0.44 g L−1) was observed under the R1 conditions of the control system (C-R1) on the 5th day (Fig. 1a). The average acetic acid production efficiency under the C-R1 conditions was found to be 11 mg L−1 h−1 across all cycles. All R1 conditions showed an increase in the acetate concentration during the exponential phase, followed by stability and a decrement. A similar trend in fermentation metabolite concentrations was previously observed in other gas fermentation studies.31,32 Under H-R1 conditions, the rate of acetic acid production was 33 mg L−1 h−1, with a maximum volumetric production of 0.97 g L−1 (Fig. 1b). The acetic acid concentration in H-R1 was 2.2-fold higher than that in C-R1. The acid-treated culture in R1 exhibited a maximum acetic acid concentration of 0.87 g L−1, which was significantly higher than that of the control (C-R1) and marginally lower than that of H-R1 (Fig. 1c). The average acetate productivity of A-R1, which reflects the biocatalyst's fermentation capability, was 29 mg L−1 h−1. In the case of B-R1, the maximum acetic acid production (0.81 g L−1) occurred on the 5th day of operation, with an average volumetric production rate of 26 mg L−1 h−1 (Fig. 1d). Compared to the control, the R1 treated systems showed a higher product titer (Fig. 1e). Thermal treatment (H-R) resulted in higher product formation and increased fermentation rates compared to the untreated control culture; similarly, acid-treated conditions (A-R) also showed enhanced fermentation efficiency with 2-3-fold higher product formation compared to the control. All the R1 systems did not show alcohol presence due to the absence of chain elongation. The supplemented bicarbonate concentration might not be sufficient enough to trigger solventogenesis in the R1 systems.32 The stoichiometry of the Wood–Ljungdahl pathway for syngas to acetate and ethanol is as follows in eqn (3) and (4). | 4CO + 2H2O + 2CO2 + 4H2 → 2CH3COOH + 2CO2 + 2H2O | (3) |
| 6CO + 3H2O + 2CO2 + 6H2 → 2CH3CH2OH + 4CO2 + 3H2O | (4) |
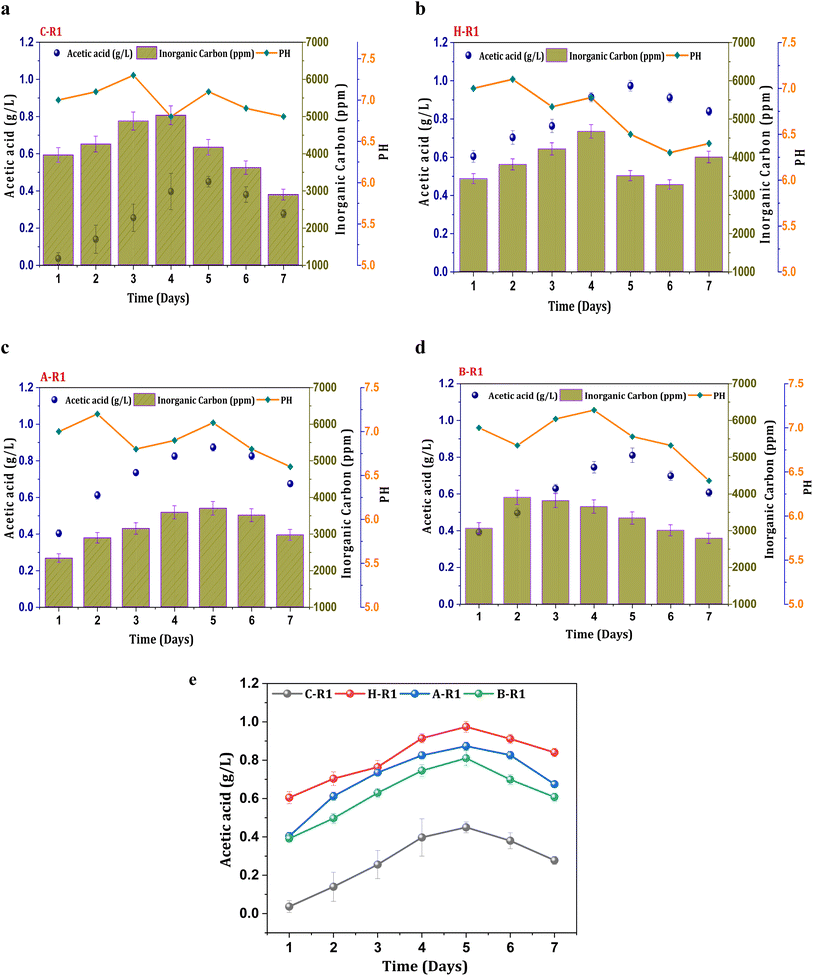 |
| Fig. 1 Profile of acetic acid, pH and IC in R1 systems (a) C-R1; (b) H-R1; (c) A-R1; (d) B-R1 and (e) comparative acetic acid profile among the R1 conditions. | |
3.2 Syngas fermentation – ethanologenic potential ‘N + syn’
Fig. 2a illustrates control conditions with R2 (syngas), which resulted in a noticeable increase in the concentration of the primary metabolite (acetic acid), exhibiting a maximum of 0.55 g L−1 acetic acid and an average productivity of 13 mg L−1 h−1. The profile of carboxylic acids increased consistently until the mid phase of cycle operation and follows a declining trend. A decrease in the acetate concentration after the 5th day of operation might be due to mild toxic effect of carbon monoxide on metabolic activity and metabolic phase shift.22 The biosynthesis of alcohols was not observed in the untreated culture (C-R2) due to unwanted microbial interactions in the system which hinders the acetaldehyde conversions. However, relatively improved product synthesis was observed under the R2 conditions with treated consortia compared to C-R2. Syngas fermentation under the H-R2 conditions demonstrated an increase in ethanologenic potential, suggesting the activation of the solventogenic activity with enhanced ethanol synthesis (0.21 g L−1) (Fig. 2e). Among all the R2 conditions, the B-R2 system showed relatively higher ethanol production (0.38 g L−1) followed by A-R2 (0.32 g L−1). The presence of the H2 fraction in the syngas assisted the solventogenic phase activations under R2 conditions leading towards the ethanologenic potential. The syngas used in the process contains a hydrogen fraction (20% H2), which acts as an electron donor in the acetyl-CoA reduction pathway, assisting in the reduction of CO2/CO. In the seven cycles of operation, the ethanol fraction was observed after the third cycle, indicating the dormant solventogenic activity of the culture during the initial stages (Fig. 2e). The synthesis of ethanol from the fourth cycle onwards depicts the early entry of actively growing cells into the exponential phase, which enhances the metabolic activity towards ethanol production. The A-R2 system showed a higher acetic acid titre (1.15 g L−1) with an average volumetric production of 40 mg L−1 h−1 (Fig. 2b) followed by H-R2 (39 mg L−1 h−1; 1.12 g L−1) (Fig. 2c) and B-R2 (1 g L−1; 33 mg L−1 h−1) (Fig. 2d). After the 4th day, acetic acid showed a decreased concentration. The conversion of acetogenic products to the solventogenic products may be the reason for the decrease in the acetate concentration. The increase in the fraction of products under the treated culture conditions (H-R2, A-R2, and BR2) indicates the active growth of carboxydotrophs in the consortia, which is suppressing other microbial interventions during the gas fermentation towards an active solventogenic phase.
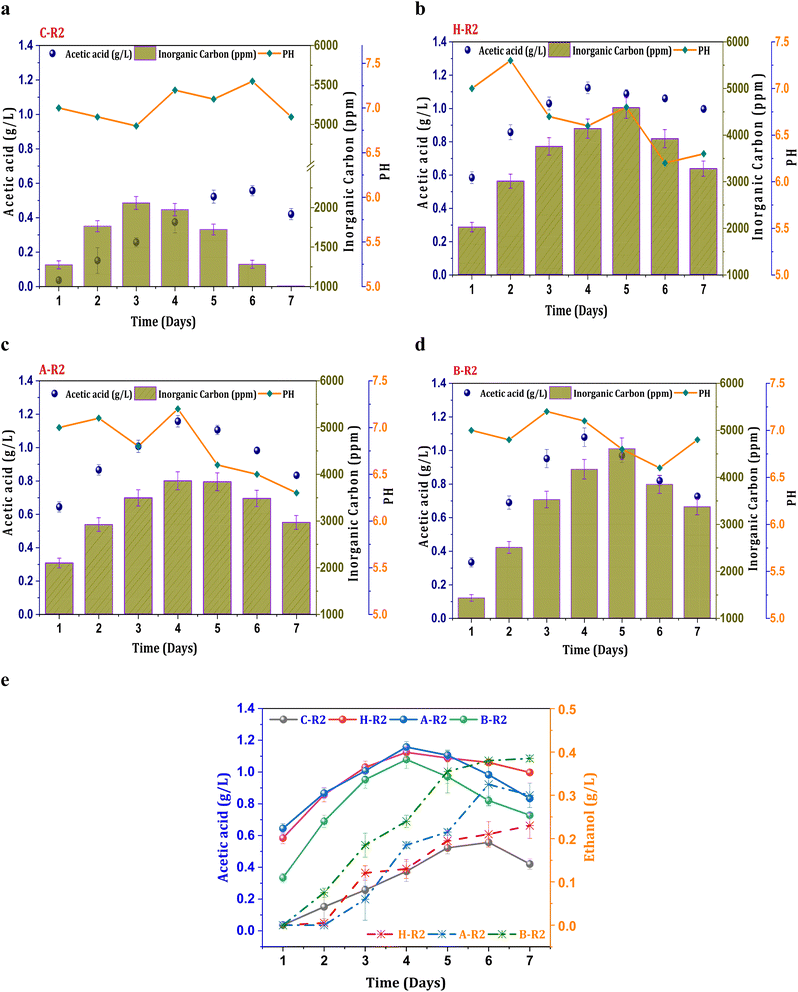 |
| Fig. 2 Profile of acetic acid, pH and IC under R2 conditions, (a) C-R2; (b) H-R2; (c) A-R2; (d) B-R2 and (e) comparative acetic acid and ethanol profiles. | |
3.3 Bicarbonate effect on syngas fermentation – ethanologenic potential of ‘N + syn + HCO3’
When 5 g L−1 of bicarbonate was added to the R3 conditions before each cycle operation, fermentation metabolites were produced in higher fractions compared to R2 systems. The production of acetate under the untreated C-R3 conditions was 0.75 g L−1, which was 1.7 times higher than that of the C-R1 and C-R2 conditions (Fig. 3a). The rate of acetate production in C-R3 was 18 mg L−1 h−1, indicating improved fermentation rates even in untreated cultures in the presence of syngas and bicarbonate as the feedstock. However, solventogenesis was not observed in the control cultures, possibly due to utilization of the electron donor (H2) by undesired microbiomes especially hydrogenotrophic methanogens. It has been reported that cross-contamination of undesired microbes in syngas fermentation hinders the production rate through metabolic competition.16 Though there was no presence of ethanol in the control system, remaining R3 systems depicted ethanol during the gas fermentation. The B-R3 (0.38 g L−1) conditions showed relatively higher production followed by A-R3 (0.34 g L−1) and H-R3 (0.29 g L−1) (Fig. 3e). Along with H2, additional supplementation of bicarbonate in R3 systems assisted the metabolic shift to solventogenesis. As a result of suppressed methanogenic activity, H2 gas is not used by hydrogenotrophic methanogens to produce CH4 resulting in chain elongation towards ethanol. Nearly, a ∼0.6 fold increment in the ethanol concentrations from the R2 to R3 systems was observed suggesting the positive impact of the bicarbonate addition on the acetaldehyde conversion. The supplementation of bicarbonate also assisted in the rate of product formation by providing an additional carbon source. A nearly 0.86-fold increment in the acetic acid synthesis was observed under the R3 conditions compared with other R2 conditions, suggesting improved fermentation productivity. The B-R3 system documented relatively higher acetic acid production (2 g L−1) (Fig. 3d) followed by A-R3 (1.9 g L−1) Fig. 3c and H-R3 (1.7 g L−1) (Fig. 3b). The average production rate of acetic acid was 56, 53, and 55 mg L−1 h−1 in BR-3, H-R3, and C-R3, respectively. The presence of bicarbonate under R1 conditions, along with CO2, did not result in solventogenesis due to the slower metabolic rate. The addition of syngas to the biocatalyst enables the active growth of carboxydotrophs, which utilize CO and CO2 as energy sources and H2 as a reducing agent via the acetyl-CoA reduction pathway. However, excessive CO concentrations can inhibit the fermentation efficiency of carboxydotrophs, which inhibits electron donor uptake.34,35 The synthesis of alcohols under R3 conditions suggests that the given concentration of syngas is non-toxic for fermentation. The biological water–gas shift reaction between CO and H2O increases the liquid–gas interferences in R2 and R3, which enhances CO intake by carboxydotrophs. The supplemented fraction of H2 in R2 and R3 promotes the biological reduction of C1 by providing reducing equivalents.2,35
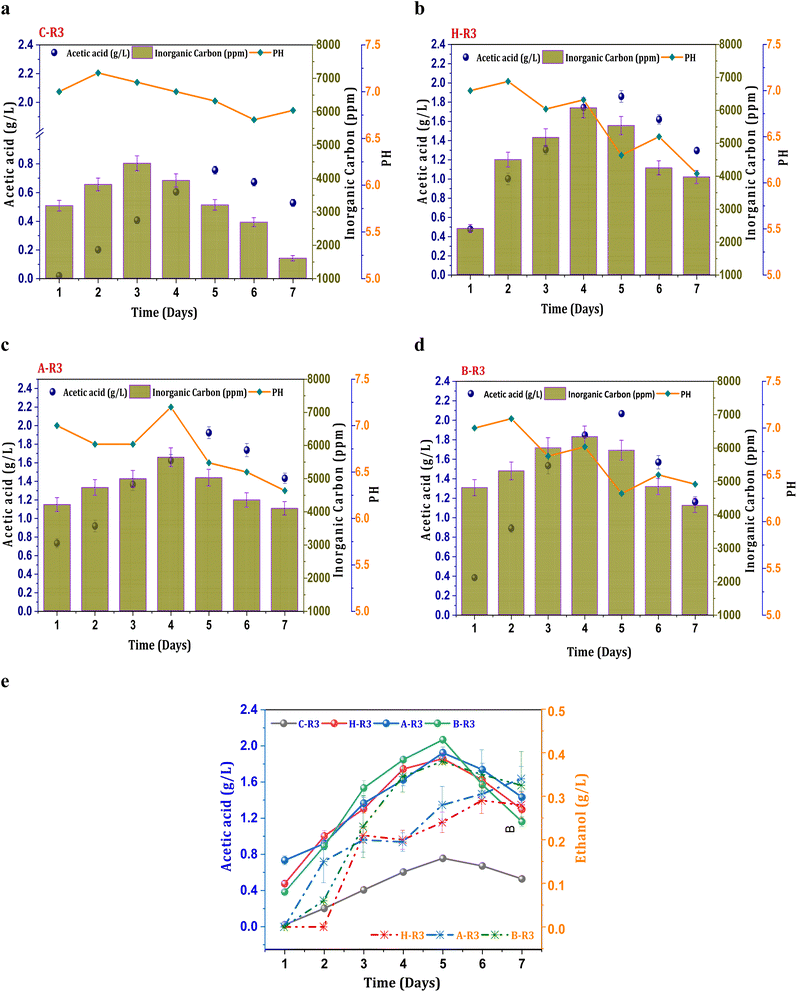 |
| Fig. 3 Profile of acetic acid, pH and IC under R3 conditions, (a) C-R3; (b) H-R3; (c) A-R3; (d) B-R3 and (e) comparative acetic acid and ethanol profiles. | |
3.4 Relative expression of the WLP genes
The metabolic rates of the consortia were studied through the relative expression of WLP coding genes during the peak phase of experimental operation (Fig. 4). The expression levels of the Ni-containing protein subunit of CODH (cooC2) indicate the activity of CODH/ACS in taking up the C1 gas into the cell. Under the R1 conditions, cooC2 expression was normalized with the housekeeping gene (16s) expression (Fig. 5a). FASTA files including the partial sequences of the expressed genes are provided in the ESI.† Enriched cultures exhibited higher cooC2 expression levels compared to the control. Although the formate dehydrogenase alpha subunit (Fdhl) showed expression levels under all R1 conditions, the detection of formic acid in the fermentation medium was irregular and unspecific. This might suggest that formate is rapidly converted into subsequent products in the methyl branch of the WLP. Under the R1 conditions, no fractions of ethanol (EtOH) were detected, which aligns with the non-expression of alcohol dehydrogenase genes. However, in the R2 systems, the fermentation efficiency shifted towards the production of fatty acids and ethanol (Fig. 5b). Notably, genes encoding CODH, adhE1, and Fdhl exhibited higher levels of relative upregulation under the R2 conditions compared to the R1 conditions. R3 conditions with syngas and bicarbonate showed relatively higher upregulations in AdhE1 when compared with the control, R1 and even R2 systems. The 10-fold overexpression of the CODH nickel subunit and 8.6-fold overexpression of adhE1 genes under the treated conditions of the R2 and R3 systems indicate that intracellular metabolism is directed towards solventogenesis. The increased expression of alcohol dehydrogenase genes in both R2 and R3 systems suggests that the addition of syngas and bicarbonate supplementation specifically promotes the reduction of C1 compounds into ethanol (Fig. 5c). The upregulation of adhE1 did not show significant variations among the treated cultures under the same conditions (R2 and R3), suggesting that the presence of the electron donor H2 and bicarbonate facilitates the reduction of CO2 and CO into fermentation products. Chain elongation under the R3 conditions correlates with the upregulation of alcohol dehydrogenase. Notably, the higher fold change in the upregulation of adhE1 under R3 conditions, where bicarbonate supplementation was present, suggests the dominance of the solventogenic carboxydotrophic community within the mixed consortia. The observed metabolic shift in this study, resulting from changes in the feed composition, is consistent with previous findings for C. ljungdahlii, under different fermentation conditions.30 Analysis of the microbial community revealed correlations between the presence of dominant phyla encoding Wood–Ljundahl pathway (WLP) genes and the upregulation of selected genes.
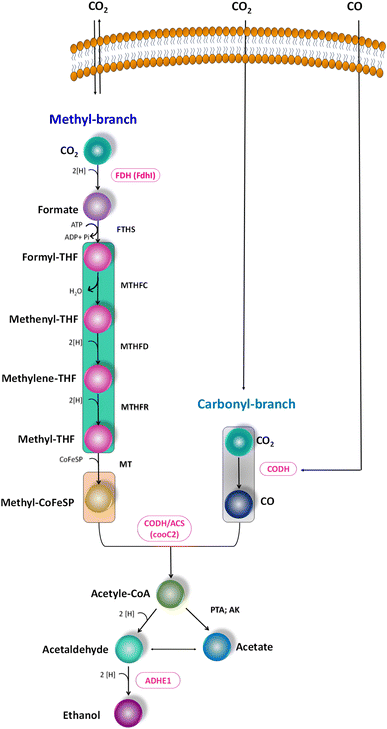 |
| Fig. 4 Scheme of the WLP, functional genes encoding for the C1 conversion. Targeted genes are highlighted (pink colour). | |
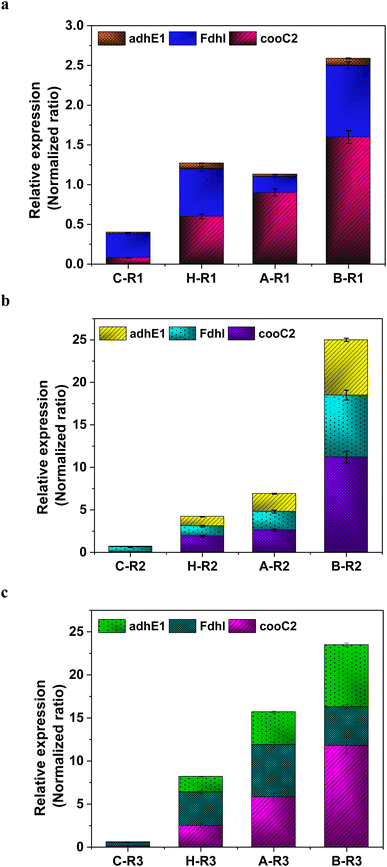 |
| Fig. 5 Relative expression of the WLP genes under (a) R1 conditions; (b) R2 and (c) R3 conditions. | |
3.5 Microbial community distribution
In order to assess the microbial diversity under the best-performing conditions, B-R1 and B-R3 along with C were subjected to comparative microbial community analysis. The analysis revealed a total of 25 operational taxonomic units (OTUs) present in all three cultures, illustrating a diverse distribution of different phyla (Fig. 6a). To construct a phylogenetic tree, the three closest relatives of the OTUs were identified and represented (Fig. 6b–d) and ESI,† including details about the closest relatives of the obtained OTUs and a Venn diagram illustrating the common OTUs shared among the three cultures (Table S1 and Fig. S1†). In the untreated control culture, 12 OTUs were identified, representing a broad range of phylum abundances. Among these, Proteobacteria (69%) emerged as the dominant phylum, followed by Firmicutes (11%), Actinobacteria (8%), and other uncultured bacteria. However, in the treated cultures, where syngas and CO2 were supplemented, the abundance of phyla displayed a significant diversification towards C1-consuming microbes, aligning with previous studies that reported the presence of such microflora from families such as Peptococcaceae, Ruminococcaceae, Lachnospiraceae, etc.20,28
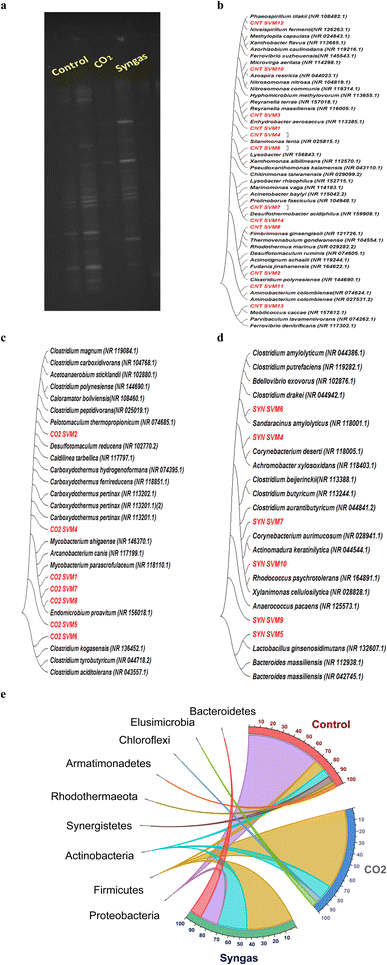 |
| Fig. 6 (a) DGGE gel with separated DNA bands against the gradient; (b) phylogenetic trees of cultures with OTUs obtained in the untreated control, (c) CO2 supplemented and (d) syngas; microbial community profiling of the cultures. (e) Chord diagram of relative abundance of phyla representing the community distributions. | |
In cultures supplemented with CO2, Firmicutes were found to be highly enriched, comprising 76% of the microbial composition, while Actinobacteria represented the second largest phylum at 14%. This enrichment of Firmicutes continued in cultures supplemented with syngas, although at a slightly reduced abundance of 74%. Additionally, Bacteroidetes (11%) were also present in these cultures, known for their ability to produce fatty acids and alcohols from inorganic carbon sources. The continuous sparging of C1 gases in the culture medium had a significant impact on the diversity of species. Syngas supplementation (R2 and R3) selectively enriched the relative abundance of Clostridium genera, including well-known carboxydotrophic acetogens and organisms involved in solventogenesis from inorganic carbon. Examples of such Clostridium species enriched in the syngas-supplemented cultures include C. beijerinckii, C. aurantibutyricum, and C. amylolyticum. These species have the capacity to efficiently utilize C1 gases and participate in the production of valuable fermentation products.
Clostridium species were also found to dominate in CO2-supplemented cultures, such as C. peptidivorans, C. polynesiense, C. magnum, and Carboxydothermus ferrireducens. These species are known for their ability to valorise the gaseous feedstock, contributing to the conversion of inorganic carbon sources into fermentation products. Importantly, all the microbial communities depicted under the R2 and R3 conditions were found to possess coding sequences of AdhE1 suggesting potential for solventogenesis. The enrichment of Clostridium species, along with the presence of AdhE1 coding sequences, in the microbial communities of R2 and R3 conditions highlights their crucial role in enhanced solventogenesis and the production of short-chain carboxylic acids from inorganic gaseous carbon sources.
The microbial community composition under the three conditions demonstrated that the continuous C1 gas supplementation resulted in the preferential growth of carboxydotrophs (Fig. 6e). The prolonged enrichment of the cultures created specific environmental conditions that favoured the proliferation of carboxydotrophs while inhibiting the growth of undesired bacteria. Notably, the cultures treated with BESA exhibited a significant enrichment of Actinobacteria (28%) and Chloroflexi (5%), which are well-known for their ability to ferment carbohydrates. The higher abundance of microorganisms capable of fixing C1 gases in the treated culture (BESA) enhanced the growth of carboxydotrophs, resulting in conversion of C1 gases. In contrast, the untreated culture supplemented with syngas did not show a significant enrichment of carboxydotrophs, highlighting the importance of pre-treatment as a crucial factor influencing the diversity of microorganisms required for ethanol biosynthesis and fermentation specificity, while avoiding the presence of methanogenic microbiota.
3.6 Inorganic carbon utilization/fixation
In order to assess the substrate consumption capacity of the biocatalyst, the concentration of the substrate in the systems was measured in terms of ppm of inorganic carbon (IC). The profile of IC concentration under all conditions (R1, R2 and R3) showed a proportional trend with product formation, as depicted in Fig. 1–3. Typically, the relationship between reactants and products during fermentation is inverse as the substrate is converted to fermentation products.19,33 However, in the present study, a linear increase in the IC concentration in the initial days of operation followed by a declining trend was observed, which might suggest a slower rate of gas–liquid shift in the early phase. After reaching the maximum state, IC concentrations tend to decline in the later days of the cycle operation. The fermentation efficiency of the biocatalyst is primarily influenced by the utilization of the substrate.5,16 The inorganic carbon fixation rate (ICFR; mg L−1 h−1), which accounts for the production of cumulative fermentation products (acetate and ethanol), was calculated (Fig. 7). The fixation of IC fractions from syngas by the biocatalyst is dependent on its metabolic activity and growth rate. Fixation with respect to the products was observed under all conditions, suggesting that prolonged exposure to C1 gases causes a metabolic shift of the microbes towards C1 reduction pathways.12 Under R1 conditions where CO2 and bicarbonate were supplemented, the ICFR was observed to be 12 mg L−1 h−1 with the untreated culture (C-R1), whereas treated cultures showed a comparatively higher fixation rate of 34 mg L−1 h−1 and 29 mg L−1 h−1 in H-R1 and A-R1, respectively. The higher fixation rate under the R1 conditions of treated cultures suggested a positive influence of pre-treatment.
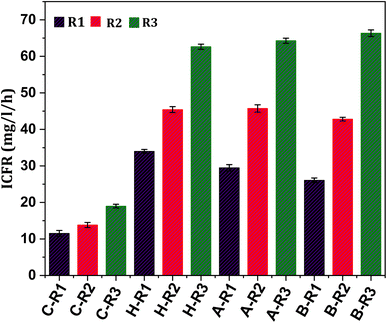 |
| Fig. 7 Inorganic carbon fixation rates under all experimental conditions operated. | |
After changing the gaseous feedstock under R2 conditions, the ICFR was triggered, resulting in increased carbon fixation. The untreated culture (C-R2) had an ICFR of 14 mg L−1 h−1, while the treated R2 conditions (B-R2, H-R2, and A-R2) had ICFR values of 43, 45, and 46 mg L−1 h−1, respectively. The use of syngas as a feedstock and changes in operational conditions helped the biocatalyst enter the early active growth phase, which enhanced carbon fixation. Additionally, supplementing bicarbonate along with syngas under R3 conditions significantly increased carbon intake by the biocatalyst, as reflected in the ICFR and product formation. The ICFR value under R3 conditions with untreated culture (C-R3) was 19 mg L−1 h−1, which was higher than those under R2 and R1 conditions of the control. The H-R3 and A-R3 reactors had ICFR values of 63 and 64 mg L−1 h−1, respectively, indicating the suppression of non-gas-consuming microbiomes as a key factor in C1 gas fermentation. B-R3 had the highest ICFR of 66 mg L−1 h−1 compared to other systems. The ICFR values of all conditions correlated with the formation of fermentation products. The active reduction metabolism (WLP) of the carboxydotrophs in the treated cultures accounted to produce higher product fractions with a simultaneously increased ICFR, which was 3-fold higher under R3 treated conditions than that under untreated R1 conditions. These results demonstrate that changes in feedstock and operational conditions can significantly enhance carbon fixation by the biocatalyst.5,36
3.7 Fermentation efficiency and pH profile
The biocatalyst was found to have higher ethanologenic activity under syngas feedstock conditions (Table 1). The rate of fermentation was significantly impacted by sparging of C1 gases into the medium under all conditions. The productivity of acetic acid increased slowly, reaching a maximum productivity between the 4th and 5th days of operation. Higher productivity of acetic acid was observed in heat (257 mg L−1 d−1; H-R3), acid (274 mg L−1 d−1; A-R3), and BESA (298 mg L−1 d−1; B-R3) treated cultures. The productivity of acetic acid appeared to be higher under all R3 conditions when compared to the R1 and R2. Within the single treated conditions, the highest productivity was observed with syngas in the presence of additional bicarbonate (R3), while CO2 and HCO3− supplementation to all pre-treated cultures (H-R1, B-R1, and A-R1) resulted in comparatively lower fermentation efficiency compared to syngas sparging (R2). This may be due to the rapid metabolic uptake of CO present in syngas. The maximum productivity obtained in each treated culture was taken into consideration for comparison (R3 conditions) of the acetic acid and ethanol profiles (Fig. 3e). Among the R3 conditions, the highest productivity was observed in B-R3, with a maximum of 2.03 g L−1, followed by acid-treated (A-R3; 1.9 g L−1) and heat-treated (H-R3; 1.86 g L−1) conditions.
Table 1 Comparative outcomes of operating conditions and respective product yields
Pre-treatment |
Condition |
Substrate |
Acetic acid (g L−1) |
Acetic acid (mg L−1 d−1) |
Ethanol (g L−1) |
Ethanol (mg L−1 d−1) |
ICFR (mg L−1 h−1) |
pH drops |
Retention time (days) |
Control (untreated) |
C-R1 |
CO2 + HCO3− |
0.449 ± 0.02 |
64 ± 0.05 |
— |
— |
12 ± 0.8 |
∼6.8 |
7 |
C-R2 |
Syngas |
0.556 ± 0.03 |
79 ± 0.06 |
— |
— |
14 ± 0.7 |
∼6.9 |
C-R3 |
Syngas + HCO3− |
0.756 ± 0.02 |
108 ± 0.08 |
— |
— |
19 ± 0.5 |
∼6.8 |
Heat treatment |
H-R1 |
CO2 + HCO3− |
0.974 ± 0.03 |
139 ± 0.03 |
— |
— |
34 ± 0.5 |
∼6.4 |
7 |
H-R2 |
Syngas |
1.124 ± 0.03 |
160 ± 0.06 |
0.23 ± 0.02 |
32 ± 0.08 |
45 ± 0.8 |
∼6.3 |
H-R3 |
Syngas + HCO3− |
1.86 ± 0.06 |
265 ± 0.07 |
0.29 ± 0.02 |
41 ± 0.04 |
63 ± 0.7 |
∼6.1 |
Acid treatment |
A-R1 |
CO2 + HCO3− |
0.87 ± 0.02 |
124 ± 0.09 |
— |
— |
29 ± 0.9 |
∼6.6 |
7 |
A-R2 |
Syngas |
1.15 ± 0.02 |
164 ± 0.14 |
0.32 ± 0.04 |
45 ± 0.07 |
46 ± 0.9 |
∼6.3 |
A-R3 |
Syngas + HCO3− |
1.92 ± 0.06 |
274 ± 0.18 |
0.34 ± 0.02 |
48 ± 0.05 |
64 ± 0.7 |
∼6.3 |
BESA treatment |
B-R1 |
CO2 + HCO3− |
0.81 ± 0.06 |
115 ± 0.2 |
— |
— |
26 ± 0.12 |
∼6.5 |
7 |
B-R2 |
Syngas |
1.12 ± 0.01 |
171 ± 0.13 |
0.38 ± 0.09 |
54 ± 0.08 |
43 ± 0.71 |
∼6.1 |
B-R3 |
Syngas + HCO3− |
2.1 ± 0.08 |
298 ± 0.21 |
0.386 ± 0.1 |
55 ± 0.03 |
66 ± 0.45 |
∼6.2 |
Microbial syngas fermentation is influenced by pH, which affects cell physiology, ionic transport, proton motive force, and metabolic activity.37–39 The pH level plays a crucial role in fermentation capabilities as it impacts the reduction of C1 gases (Fig. 1–3). In this experiment, the pH of the medium was adjusted to 7.0 ± 0.02, but it became acidic upon introduction of CO2 and CO gases. The pH showed irregular trends attributed to reversible reactions of C1 gas into bicarbonate. Accumulation of primary metabolites led to a slight decrease in pH, with the maximum drop observed around 6.0 ± 0.02, consistent with earlier studies.5,19,40 Despite unusual daily pH trends, all reactors exhibited an overall acidic inclination, indicating accumulation of fermented products in the medium.
4. Conclusion
The simultaneous addition of syngas and bicarbonate under R3 conditions resulted in improved utilization of inorganic carbon for the production of ethanol in the gas fermentation system. The enrichment of consortia prior to the process played an influencing role in the rate of fermentation and carbon uptake, thereby product selectivity. The highest ethanol and acetic acid yields were obtained under the R3 conditions using BESA-treated culture followed by R2 and R1 systems. The microbial community analysis showed an abundance of Firmicutes in the enriched cultures. Specific microorganisms (Clostridium sp.) capable of consuming C1 compounds have been selectively enriched, which were responsible for the production of acetic acid and ethanol. The upregulation of cooC2, Fdhl, and adhE1 genes was noticed in the R2 and R3 systems indicating the enrichment of solventogenic carboxydotrophs within the consortia. Provision of an electron donor in the form of hydrogen in the R2 and R3 systems assisted in the fatty acid chain elongation leading to the ethanol synthesis. This study provided an effective strategy to enrich the biocatalyst with ethanologenic potential by addressing the mitigation of C1 emissions sustainably.
Conflicts of interest
There are no conflicts to declare.
Acknowledgements
The research was sponsored by a research grant from the Department of Biotechnology (DBT) of the Government of India (No. BT/PR20759/BCE/8/1218/2016). The authors would like to thank the Director of CSIR-IICT for providing the facilities to perform the study (Manuscript no. IICT/Pubs./2022/032).
References
- A. Grimalt-Alemany, M. Łężyk, L. Lange, I. V. Skiadas and H. N. Gavala, Enrichment of syngas-converting mixed microbial consortia for ethanol production and thermodynamics-based design of enrichment strategies, Biotechnol. Biofuels., 2018, 11(1), 1–22, DOI:10.1186/s13068-018-1189-6.
- A. Tharak, R. Katakojwala, S. Kajla and S. Venkata Mohan, Chemolithoautotrophic reduction of CO2 to acetic acid in gas and gas-electro fermentation systems: Enrichment, microbial dynamics, and sustainability assessment, Chem. Eng. J., 2023, 454, 140200, DOI:10.1016/j.cej.2022.140200.
- O. Sarkar, R. Katakojwala and S. Venkata Mohan, Low carbon hydrogen production from a waste-based biorefinery system and environmental sustainability assessment, Green Chem., 2021, 23(1), 561–574, 10.1039/D0GC03063E.
- M. Balat, Status of fossil energy resources: A global perspective, Energy Sources, Part B, 2007, 2(1), 31–47, DOI:10.1080/15567240500400895.
- J. A. Modestra, R. Katakojwala and S. Venkata Mohan, CO2 fermentation to short chain fatty acids using selectively enriched chemolithoautotrophic acetogenic bacteria, Chem. Eng. J., 2020, 394, 124759, DOI:10.1016/j.cej.2020.124759.
- F. Lanzillo, G. Ruggiero, F. Raganati, M. E. Russo and A. Marzocchella, Batch syngas fermentation by Clostridium carboxidivorans for production of acids and alcohols, Processes, 2020, 8(9), 1075, DOI:10.3390/pr8091075.
- J. R. Phillips, K. T. Klasson, E. C. Clausen and J. L. Gaddy, Biological production of ethanol from coal synthesis gas, Appl. Biochem. Biotechnol., 1993, 39(1), 559–571, DOI:10.1007/BF02919018.
- T. A. Ewing, N. Nouse, M. van Lint, J. van Haveren, J. Hugenholtz and D. S. van Es, Fermentation for the production of biobased chemicals in a circular economy: a perspective for the period 2022–2050, Green Chem., 2022, 24(17), 6373–6405, 10.1039/D1GC04758B.
- S. Dey, A. Bhattacharya, P. Kumar and A. Malik, High-rate CO2 sequestration using a novel venturi integrated photobioreactor and subsequent valorization to microalgal lipids, Green Chem., 2020, 22(22), 7962–7973, 10.1039/D0GC02552F.
- A. Grimalt-Alemany, M. Łężyk, K. Asimakopoulos, I. V. Skiadas and H. N. Gavala, Cryopreservation and fast recovery of enriched syngas-converting microbial communities, Water res, 2020, 177, 115747, DOI:10.1016/j.watres.2020.115747.
-
E. J. Wolfrum and A. S. Watt, Bioreactor design studies for a hydrogen-producing bacterium, in Biotechnology for Fuels and Chemicals, Humana Press, Totowa, NJ, 2002, DOI:10.1007/978-1-4612-0119-9_50.
- A. Tharak and S. Venkata Mohan, Electrotrophy of biocathodes regulates microbial-electro-catalyzation of CO2 to fatty acids in single chambered system, Bioresour. Technol., 2021, 320, 124272, DOI:10.1016/j.biortech.2020.124272.
- A. Tharak and S. Venkata Mohan, Syngas fermentation to acetate and ethanol with adaptative electroactive Carboxydotrophs in single chambered microbial electrochemical system, Micromachines, 2022, 13(7), 980, DOI:10.3390/mi13070980.
- C. Cheng, W. Li, M. Lin and S. T. Yang, Metabolic engineering of Clostridium carboxidivorans for enhanced ethanol and butanol production from syngas and glucose, Bioresour. Technol., 2019, 284, 415–423, DOI:10.1016/j.biortech.2019.03.145.
- S. Esquivel-Elizondo, A. G. Delgado, B. E. Rittmann and R. Krajmalnik-Brown, The effects of CO2 and H2 on CO metabolism by pure and mixed microbial cultures, Biotechnol. Biofuels, 2017, 10(1), 1–13, DOI:10.1186/s13068-017-0910-1.
- S. Chakraborty, E. R. Rene, P. N. Lens, M. C. Veiga and C. Kennes, Enrichment of a solventogenic anaerobic sludge converting carbon monoxide and syngas into acids and alcohols, Bioresour. Technol., 2019, 272, 130–136, DOI:10.1016/j.biortech.2018.10.002.
- J. Chen and M. A. Henson, In silico metabolic engineering of Clostridium ljungdahlii for synthesis gas fermentation, Metab. Eng., 2016, 38, 389–400, DOI:10.1016/j.ymben.2016.10.002.
- B. T. Maru, P. C. Munasinghe, H. Gilary, S. W. Jones and B. P. Tracy, Fixation of CO2 and CO on a diverse range of carbohydrates using anaerobic, non-photosynthetic mixotrophy, FEMS Microbiol. Lett., 2018, 365(8), fny039, DOI:10.1093/femsle/fny039.
- R. Katakojwala, A. Tharak, O. Sarkar and S. Venkata Mohan, Design and evaluation of gas fermentation systems for CO2 reduction to C2 and C4 fatty acids: Non-genetic metabolic regulation with pressure, pH and reaction time, Bioresour. Technol., 2022, 351, 126937, DOI:10.1016/j.biortech.2022.126937.
- J. A. Modestra and S. Venkata Mohan, Microbial electrosynthesis of carboxylic acids through CO2 reduction with selectively enriched biocatalyst: microbial dynamics, J. CO2 Util., 2017, 20, 190–199, DOI:10.1016/j.jcou.2017.05.011.
- R. Ganigué Pagès, P. Sánchez Paredes, L. Bañeras Vives and J. Colprim Galceran, Low Fermentation pH Is a Trigger to Alcohol Production, but a Killer to Chain Elongation, Front. Microbiol, 2016, 7, 702, DOI:10.3389/fmicb.2016.00702.
- A. Singla, D. Verma, B. Lal and P. M. Sarma, Enrichment and optimization of anaerobic bacterial mixed culture for conversion of syngas to ethanol, Bioresour. Technol., 2014, 172, 41–49, DOI:10.1016/j.biortech.2014.08.083.
- M. El-Gammal, R. Abou-Shanab, I. Angelidaki, B. Omar, P. V. Sveding, D. B. Karakashev and Y. Zhang, High efficient ethanol and VFA production from gas fermentation: Effect of acetate, gas and inoculum microbial composition, Biomass Bioenergy, 2017, 105, 32–40, DOI:10.1016/j.biombioe.2017.06.020.
- G. Mohanakrishna, I. M. A. Reesh, K. Vanbroekhoven and D. Pant, Microbial electrosynthesis feasibility evaluation at high bicarbonate concentrations with enriched homoacetogenic biocathode, Sci. Total Environ., 2020, 715, 137003, DOI:10.1016/j.scitotenv.2020.137003.
- N. Shen, K. Dai, X. Y. Xia, R. J. Zeng and F. Zhang, Conversion of syngas (CO and H2) to biochemicals by mixed culture fermentation in mesophilic and thermophilic hollow-fiber membrane biofilm reactors, J. Clean. Prod., 2018, 202, 536–542, DOI:10.1016/j.jclepro.2018.08.162.
- S. Venkata Mohan, V. L. Babu and P. N. Sarma, Effect of various pretreatment methods on anaerobic mixed microflora to enhance biohydrogen production utilizing dairy wastewater as substrate, Bioresour. Technol., 2008, 99(1), 59–67, DOI:10.1016/j.biortech.2006.12.004.
- K. V. Krishna and S. Venkata Mohan, Selective enrichment of electrogenic bacteria for fuel cell application: Enumerating microbial dynamics using MiSeq platform, Bioresour. Technol., 2016, 213, 146–154, DOI:10.1016/j.biortech.2016.03.117.
- O. Sarkar, A. N. Kumar, S. Dahiya, K. V. Krishna, D. K. Yeruva and S. Venkata Mohan, Regulation of acidogenic metabolism towards enhanced short chain fatty acid biosynthesis from waste: metagenomic profiling, RSC Adv., 2016, 6(22), 18641–18653, 10.1039/C5RA24254A.
- J. S. Sravan, S. K. Butti, O. Sarkar, K. V. Krishna and S. Venkata Mohan, Electrofermentation of food waste–regulating acidogenesis towards enhanced volatile fatty acids production, Chem. Eng. J., 2018, 334, 1709–1718, DOI:10.1016/j.cej.2017.11.005.
- B. T. Xie, Z. Y. Liu, L. Tian, F. L. Li and X. H. Chen, Physiological response of Clostridium ljungdahlii DSM 13528 of ethanol production under different fermentation conditions, Bioresour. Technol., 2015, 177, 302–307, DOI:10.1016/j.biortech.2014.11.101.
- A. Panneerselvam, M. R. Wilkins, M. J. M. DeLorme, H. K. Atiyeh and R. L. Huhnke, Effects of Various Reducing Agents on Syngas Fermentation by, Biol. Eng. Trans., 2010, 2(3), 135–144, DOI:10.13031/2013.34831.
- K. M. Hurst and R. S. Lewis, Carbon monoxide partial pressure effects on the metabolic process of syngas fermentation, Biochem. Eng. J., 2010, 48(2), 159–165, DOI:10.1016/j.bej.2009.09.004.
- S. G. Barbosa, L. Peixoto, J. I. Alves and M. M. Alves, Bioelectrochemical systems (BESs) towards conversion of carbon monoxide/syngas:
A mini-review, Renew. Sust. Energ. Rev., 2021, 135, 110358, DOI:10.1016/j.rser.2020.110358.
- B. Bennett, B. J. Lemon and J. W. Peters, Reversible carbon monoxide binding and inhibition at the active site of the Fe-only hydrogenase, Biochemistry, 2000, 39(25), 7455–7460, DOI:10.1021/bi992583z.
- Y. Tang, Y. Huang, W. Gan, A. Xia, Q. Liao and X. Zhu, Ethanol production from gas fermentation: Rapid enrichment and domestication of bacterial community with continuous CO/CO2 gas, Renewable Energy, 2021, 175, 337–344, DOI:10.1016/j.renene.2021.04.134.
- J. Jeong, J. Y. Kim, B. Park, I. G. Choi and I. S. Chang, Genetic engineering system for syngas-utilizing acetogen, Eubacterium limosum KIST612, Bioresour. Technol. Rep., 2020, 11, 100452, DOI:10.1016/j.biteb.2020.100452.
- H. Argun and F. Kargi, Effects of sludge pre-treatment method on bio-hydrogen production by dark fermentation of waste ground wheat, Int. J. Hydrog. Energy, 2009, 34(20), 8543–8548, DOI:10.1016/j.ijhydene.2009.08.049.
- L. Zhuang, Q. Chen, S. Zhou, Y. Yuan and H. Yuan, Methanogenesis control using 2-bromoethanesulfonate for enhanced power recovery from sewage sludge in air-cathode microbial fuel cells, Int. J. Electrochem. Sci., 2012, 7, 6512–6523 CrossRef CAS.
- P. Mohammadi, S. Ibrahim and M. S. M. Annuar, Comparative study on the effect of various pretreatment methods on the enrichment of hydrogen producing bacteria in anaerobic granulated sludge from brewery wastewater, Korean J. Chem. Eng., 2012, 29(10), 1347–1351, DOI:10.1007/s11814-012-0018-z.
- Y. M. Wong, T. Y. Wu and J. C. Juan, A review of sustainable hydrogen production using seed sludge via dark fermentation, Renew. Sust. Energ. Rev, 2014, 34, 471–482, DOI:10.1016/j.rser.2014.03.008.
|
This journal is © The Royal Society of Chemistry 2023 |