Balancing photosynthesis, O2 consumption, and H2 recycling for sustained H2 photoproduction in pulse-illuminated algal cultures†
Received
8th November 2022
, Accepted 3rd March 2023
First published on 3rd March 2023
Abstract
Photosynthetic H2 production in unicellular green alga Chlamydomonas reinhardtii is catalysed by O2-sensitive [Fe–Fe]-hydrogenase (H2ase) enzymes located in the chloroplast. The process is difficult to sustain due to (i) the inactivation of H2ase enzymes by O2 coevolved in photosynthesis and (ii) the competition of H2ases with the Calvin–Benson–Bassham (CBB) cycle for photosynthetic reductants. Our previous studies revealed that H2 production in nutrient-replete algal cultures could be sustained by applying a train of strong but short (1–5 s) light pulses interrupted by longer (3–9 s) dark periods. This limits O2 accumulation produced by photosystem II, prevents activation of the CBB cycle and redirects photosynthetic electrons to H2ase. In the present research, we demonstrate that the combination of strong light pulses with continuous low background illumination gives a significant gain in the net H2 photoproduction yield by pulse-illuminated algae but only for the first 24 h. We bring evidence that the attenuation of H2 evolution is primarily caused by the accumulation of H2 in the headspace of vials rather than O2 inhibition of the H2ase, whereas an increase in the H2 partial pressure leads to activation of H2 recycling and noticeable H2 uptake, which is accelerated by O2. We predicted that sustained H2 production in pulse-illuminated algae, which are additionally exposed to continuous low background light, could be achieved by decreasing the H2 partial pressure in cultures and preventing excessive accumulation of O2. Indeed, the application of periodic refreshments of a headspace atmosphere with argon and the introduction of O2 scavenger L-cysteine allowed the H2 photoproduction activity in algal cultures to be sustained for more than 10 days both under photoheterotrophic and photoautotrophic conditions, and yielding at least 6-times more H2 per litre of the culture than the standard pulse-illumination protocol.
Introduction
Molecular hydrogen (H2), when produced from renewable sources, is regarded as the cleanest energy carrier for the future circular economy with significant demands from the global fuel market.1 In addition, it serves as a crucial feedstock for a variety of industrial activities, including the manufacturing of fertilisers and the refining of petroleum. The photobiological water splitting (water biophotolysis) process, which is inherent to many species of cyanobacteria and green algae, is the most promising and environmentally friendly way for the generation of H2.2–4 In comparison with inorganic photocatalysts for water oxidation, CO2 reduction and H2 generation,5–7 the photobiological approach considers the natural and engineered photosynthetic organisms as whole-cell biocatalysts, which provide a fully renewable alternative to the traditional chemical synthesis and which are capable of self-repairing, operating in a wide range of the light spectrum, and utilizing cheap and abundant raw materials such as water, mineral nutrients, CO2 and some organic substrates.8–10
In green algae, H2 photoproduction occurs in two steps with the involvement of the photosystem II (PSII) water oxidizing complex and the proton-reducing [Fe–Fe]-hydrogenase (H2ase) enzyme associated with the photosynthetic electron-transport chain:
| 2H2O → 4H+ + O2 + 4e−; (Step 1, PSII-dependent reaction) | (1) |
| 4H+ + 4e− ⇄ 2H2; (Step 2, H2ase-dependent reaction) | (2) |
Green algae typically produce H2 on exposure to light after a period of dark anaerobic adaptation. The process is very efficient but short due to a fast accumulation of O2 co-evolved in step 1.11,12 Accumulated O2 leads to the inactivation of O2-sensitive H2ase in cells.13,14 Another reason for the fast termination of H2 evolution in algae is a competition of H2ase with the Calvin–Benson–Bassham (CBB) cycle for photosynthetic reductants, which occurs just before the inactivation of H2ase by O2.15,16 Activation of CO2 fixation coincides with a pronounced H2 uptake that also terminates with the accumulation of O2 in cells.17
Sustained H2 photoproduction in green algae is typically achieved by nutrient deprivation.18 In this approach, algal cultures are transferred to the medium depleted of an essential nutrient such as sulfur, nitrogen, phosphorus, or magnesium.19–22 Typically, under nutrient deprivation cells stop dividing, accumulate significant amounts of starch and partially degrade PSII reaction centres. The partial loss of water-oxidizing activity in algae results in the establishment of anaerobiosis in cultures due to efficient respiration and expression of H2ase enzymes in cells leading to sustained H2 photoproduction for a few days.23,24 Despite substantial improvement in the H2 production yield, the nutrient deprivation approach is not scalable for commercial purposes due to its low photosynthetic efficiency.25 Therefore, the current research efforts are primarily concentrated on the improvement of H2 photoproduction activity in nutrient-replete algae.26
Our previous studies revealed that H2 production in nutrient-replete Chlamydomonas reinhardtii algae could be sustained by a train of strong white-light pulses interrupted by longer dark phases.17,27,28 In this protocol, the duration of each light pulse in the light/dark sequence is short enough to prevent activation of the CBB cycle and limit the accumulation of O2 in cells. The pulse-illumination protocol represents a way to redirect photosynthetic electron flow to the green algal H2ase, thus resulting in increased H2 photoproduction yields. Under these conditions, algae produce H2via the most efficient mechanism of direct water biophotolysis, which has a theoretical light energy to H2 energy conversion efficiency (LHCE) of around 11–13%.29,30 Although in the best case up to 8% LHCE has been achieved during a short period in photoheterotrophic algal cultures31 and up to 4% under photoautotrophic conditions,16 photosynthetic H2 production in the current state is not yet efficient enough for industrial applications. There are a number of physiological, biochemical and technological barriers that limit the H2 production yield in algal cultures.32 Thus, further research efforts on improving the algal capacity to produce molecular hydrogen are indispensable.
In the present study, we identify the key factors affecting H2 photoproduction in green alga C. reinhardtii exposed to pulse-illumination under photoautotrophic and photoheterotrophic conditions and determine the major requirements for algae cultivation, which are important for supporting the long-term H2 photoproduction activity in nutrient-replete algal cultures and leading to improved H2 production yields.
Experimental
Algal growth conditions
The wild-type green alga Chlamydomonas reinhardtii strain CC-124 (mt−, nit−), which is a common model organism for studying photobiological H2 production, was obtained from the Chlamydomonas Resource Center at the University of Minnesota, USA. The stock cultures were grown in 150 ml Erlenmeyer flasks containing 50 ml of either Tris-acetate-phosphate (TAP) medium (pH 7.0) for photoheterotrophic growth or Tris-phosphate-HCl (TP) medium (pH 7.0) for photoautotrophic growth. The culture flasks were placed in a growth chamber at 25 °C on a rotary shaker (120 rpm) and cultivated under 70–75 μmol photons m−2 s−1 of photosynthetically active radiation (hereafter μmol m−2 s−1) provided by cool-white, fluorescent lamps (Philips Master TL-D T8 15W/840) and a 14 h photoperiod. The stock cultures were maintained by weekly dilutions. The experimental cultures were grown under the same growth conditions in 500 ml conical flasks containing 200 ml of TAP medium. These cultures were continuously sparged with sterile filtered air (filter with a pore-size 0.2 μm, Acro 37 TF, Gelman Sciences, USA).
Hydrogen photoproduction experiments
All H2 photoproduction experiments were performed with non-stressed nutrient-replete algae. No centrifugation was applied for cell harvesting. Simply, 10 ml cell suspensions were transferred from cultivation flasks into 73.5 ml gas-tight vials within 6 h from the start of the photoperiod on the 2nd, 3rd, and 4th day of growth depending on the required cell density. The vials were sealed with butyl rubber stoppers, flashed with argon (Ar) for 30 min and placed on a rotary shaker (120 rpm) in a growth chamber (AlgaeTron AG 130-ECO, PSI) at 25 °C. To initiate H2 photoproduction in algal suspensions, a train of 1 s white light pulses (280 μmol m−2 s−1) was applied to the surface of the vials.27 The pulses were either interrupted by 9 s dark periods (the control sample) or superimposed at the same 9 s intervals on white background illumination of different intensities: 2, 4, 12, 20 and 30 μmol m−2 s−1. Pulses and background illumination were provided by the top LED panel of the growth chamber. For long-term H2 production, the headspace of culture vials was replaced with pure Ar every 24 h by purging the gas through the suspensions for 30 min. For O2 scavenging, a fresh solution of 500 mM L-cysteine was prepared with deoxygenated water, filter sterilized and then introduced into the vials in the final concentration of 4 and 8 mM at the beginning of the experiment. The amounts of H2 and O2 in the headspace of vials were monitored once a day by injecting 150 μl gas samples into a gas chromatograph (Clarus 500, PerkinElmer, Inc.) equipped with a thermal conductivity detector and a molecular sieve 5A column (60/80 mesh). Ar was used as a carrier gas. The amounts of H2 and O2 dissolved in the liquid phase were calculated based on the partial pressure of the corresponding gas at the time of sampling and the solubility coefficients, which are 713 μmol H2 l−1 H2O (0.01744 ml ml−1) under 1 atm and 258 μmol O2 l−1 H2O (0.00632 ml ml−1) under 0.21 atm at 25 °C.33,34 These values as well as amounts of gases withdrawn for sampling were considered in the final production yields. The H2 and O2 yields in the cultures with daily renewals of the headspace atmosphere to Ar are shown as cumulative yields. The specific yields were calculated based on the initial total (a + b) chlorophyll (Chl) content in the samples. The changes in the Chl content throughout long-term (240 h) experiments, if any, have not been considered in these calculations. The Chl content in algal suspensions was assayed spectrophotometrically in 95% ethanol extracts by the method of Spreitzer.35
Hydrogenase activity assay
In vitro hydrogenase activity during H2 photoproduction was determined in photoheterotrophic and photoautotrophic algal samples. The assay was performed in 10 ml serum vials containing 900 μl of the reaction mixture consisting of 50 mM potassium-phosphate buffer (pH 6.9), 10 mM oxidized methyl viologen and 0.2% (w/v) Triton X-100. The vials were tightly sealed and flushed with Ar for 30 min. Then, 100 μl of anaerobic 100 mM Na-dithionite solution (prepared by adding anaerobic water to anaerobic vials with the Na-dithionite salt) was introduced into the vials to reduce the methyl viologen. The reaction was started by injecting 1 ml of the cell suspension into the reaction mixture and performed at 37 °C. The level of H2 in the headspace of the vials was measured by gas chromatography (as described above) for 40 min and the activity (μmol H2 (mg Chl h)−1) was calculated for the maximum H2 production rate based on the total Chl content in the sample.
Membrane inlet mass spectrometry measurements
In vivo CO2 (m/z = 44) exchange in algal cultures was measured by membrane inlet mass spectrometry (MIMS) using a modified DW1 (Hansatech Instruments) electrode chamber connected to a Prima PRO mass spectrometer (Thermo Scientific™) via a refrigerated cooling trap (−65 °C; EtOH; Julabo FT 902) as previously described.17,36 Briefly, 2 ml algal samples were placed in an MIMS chamber, and a microoxic environment inside the chamber was achieved by purging Ar gas through the suspension for about 2–3 min in the dark. The white light-emitting diode light pulses (∼1000 μmol m−2 s−1) were applied using an STM32F103 micro-controller board. The final curves were obtained after correction for gas consumption by using the mass spectrometer during the dark periods in the beginning and at the end of each experiment. This correction also included the CO2 release by the culture in the dark.
Results and discussion
Effect of low background illumination on H2 photoproduction by algal cultures exposed to the pulse-illumination protocol
Recently, we demonstrated that the induction of sustained H2 photoproduction in anaerobic C. reinhardtii algae under pulse-illumination occurs not only when the train of strong light pulses is superimposed on darkness (1 s light-on/9 s light-off regime, hereafter 1 s/9 s) but also when 1 s/9 s pulses are applied atop a very low (3 μmol m−2 s−1) background illumination.27 The appearance of H2 in pulse-illuminated cultures exposed to 3 μmol m−2 s−1 continuous background light was not surprising since even if this condition activates the CCB cycle, co-produced O2 is efficiently removed by respiration. Hydrogen photoproduction in green algae depends on photosynthetic activity and increases with an increase in light intensity.37 The increase in light intensity, though, enhances O2 accumulation. Therefore, we proposed that an increase in the intensity of the background illumination should also enhance H2 photoproduction activity in algal cultures, until the point where respiration could not adequately cope with the accelerated evolution of O2 leading to the inactivation of H2ase enzymes in algae.
To verify this hypothesis, 1 s light pulses were superimposed with a 10 s frequency either on the dark background (the control cultures) or on the low background illumination (2–20 μmol m−2 s−1). As shown in Fig. 1A, the control cultures produced around 140 μmol H2 (mg Chl)−1 in 24 h. Increasing the background illumination from 2 to 12 μmol m−2 s−1 resulted in a gradual increase in the maximum net H2 photoproduction yield from ∼200 to ∼380 μmol H2 (mg Chl)−1, while cultures exposed to 20 μmol m−2 s−1 showed a slight decrease in the net H2 production yield compared to cells illuminated by 12 μmol m−2 s−1 (Fig. 1A). The inhibition of the H2 production rate under 20 μmol m−2 s−1 light was likely caused by the excess content of intracellular O2 in algae, even though the net release of O2 in the headspace of vials occurred only after 24 h (Fig. 1B).
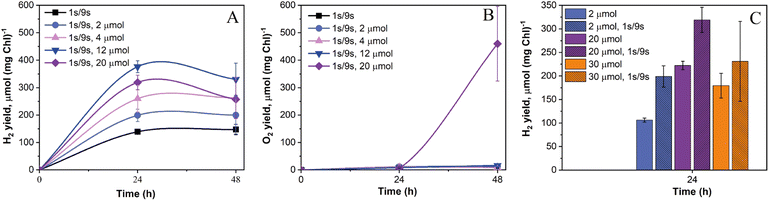 |
| Fig. 1 Effect of low background illumination on net H2 photoproduction and net O2 evolution by wild-type C. reinhardtii cells exposed to the pulse-illumination protocol. (A) Net H2 photoproduction and (B) net O2 evolution yields under 1 s/9 s pulse illumination (280 μmol m−2 s−1) applied atop darkness (the control sample) or continuous background illumination of 2 to 20 μmol m−2 s−1. (C) Comparison of net H2 photoproduction yields after 24 h illumination under continuous light and light pulses superimposed on continuous light. The initial experimental cultures contained ∼10 (A and B) and ∼8 (C) μg Chl (a + b) ml−1. The H2 and O2 yields were normalized to the initial total Chl content. The experiments were performed under photoheterotrophic conditions. Values are the mean of 6 to 15 independent replicates ± SD. | |
In line with our previous experiments,27,28 pulse-illuminated cultures produced the most H2 during the first 24 h. The application of background illumination resulted in a noticeable H2 uptake after 24 h, which became very pronounced at the light intensities above 4 μmol m−2 s−1 (Fig. 1A). This experiment demonstrated that the application of low background light in the pulse-illumination protocol improves the yield of H2 photoproduction, while still preventing O2 accumulation. In fact, most of the cultures yielded around 10 μmol O2 (mg Chl)−1 by the end of the experiment, except algae exposed to 20 μmol m−2 s−1 light where they produced above 440 μmol O2 (mg Chl)−1 (Fig. 1B).
To check whether H2 uptake in pulse-illuminated algae is linked to CO2 photoreduction in the CBB cycle, we introduced 20 mM NaHCO3 into the cultures at the beginning of the experiment. As shown in ESI Fig. 1,† the supplementation of pulse-illuminated algae with 20 mM NaHCO3 did not affect net H2 and O2 production yields in the control cultures throughout the experiment, and during the first 24 h in cultures exposed to 20 μmol m−2 s−1 background light (ESI Fig. 1†). After 24 h, the algae exposed to 20 μmol m−2 s−1 background illumination accelerated O2 production in the presence of bicarbonate compared to the cultures without added bicarbonate (ESI Fig. 1B†). The appearance of O2 in both cultures exposed to 20 μmol m−2 s−1 light indicated acceleration of the CBB cycle at around 24 h. This resulted in the pronounced H2 uptake (ESI Fig. 1A†). Interestingly, the addition of bicarbonate reduced H2 uptake under 20 μmol m−2 s−1 background illumination most probably due to the excessive production and accumulation of O2, leading to inhibition of the H2ase. Thus, the response of H2 uptake to the acceleration of the CBB cycle is rather complex. The enhanced CO2 fixation may indeed enhance H2 uptake (depending on the H2 partial pressure) but leads to excessive production of O2 that inactivates the H2ase enzyme.
Substantial H2 production during the first 24 h also occurred in cultures exposed to continuous light (without pulses) under pre-established anaerobic conditions (Fig. 1C). Similar observations of prolonged H2 production under low light intensities have been previously reported.38,39 However, it should be noted that the application of strong light pulses to cultures exposed to low continuous light always led to improved net H2 production yields in the range of tested conditions (Fig. 1C). It is important to note here that 280 μmol m−2 s−1 light pulses, even if applied only for 1 s in the 10 s pulse-illumination sequence, bring a significant fraction of light energy to drive photosynthetic H2 production, especially under the low background illumination. For example, under 2 μmol m−2 s−1 of continuous background light the applied 280 μmol m−2 s−1 light pulses bring up to 93% of the total light energy, while under 30 μmol m−2 s−1 background light, this value is around 48%. As shown in Fig. 1C, the net H2 photoproduction yield in algal cultures started decreasing after their exposure to 30 μmol m−2 s−1 light, which is just below the compensation point (when the rate of photosynthesis becomes equal to the rate of respiration) in the wild-type culture grown under photoheterotrophic conditions.40 Thus, the results revealed that efficient photosynthetic H2 production occurs when cellular respiration overtakes or minimizes accumulation of photosynthetic O2 and creates a microoxic environment inside algal chloroplasts. The latter is important for preventing inactivation of the O2-sensitive H2ase enzymes.41 Overall, the highest net H2 production yield in pulse-illuminated algae could be observed in cultures exposed additionally to around 10–12 μmol m−2 s−1 of background light (Fig. 1).
Thus, despite the limited accumulation of O2 in most of the cultures, the application of low intensity background light in the pulse-illumination protocol did not allow us to sustain H2 photoproduction for more than 24 h mainly due to activation of H2 uptake after that point.
Impact of cultivation conditions on pulse-illuminated algae under low background illumination
Similar to photoheterotrophic cultures, algae pre-grown under strict photoautotrophic conditions showed the capacity to sustain H2 photoproduction under pulse-illumination.27,28 Therefore, it was of interest to check whether pulse-illuminated photoautotrophic algae could also produce H2 under low background illumination. For this purpose, low cell density C. reinhardtii cultures (∼5 μg total Chl ml−1) were exposed to 1 s/9 s pulses superimposed on 12 μmol m−2 s−1 of continuous background light. The low cell density cultures were applied to minimize the self-shading effect, which extends the “dark zone” in algal suspensions, leading to the decreased photosynthetic O2 production and the enhanced O2 consumption by cells.39,42 As shown in Fig. 2A, photoautotrophic algal cells could produce H2 under this condition, albeit giving much lower yields compared to photoheterotrophic cultures (0.46 vs. 2.9 mmol H2 l−1, respectively). Predictably, H2 production in photoautotrophic algae proceeded with a noticeable release of O2 into the headspace of the vials (Fig. 2A), though the O2 content in vials did not exceed 0.1% by the end of the experiment (as calculated from the original GC data). Slight accumulation of O2 in cultures resulted in very low activities of the H2ase enzyme in cells (Fig. 2B). Similarly, Lee and Greenbaum observed 50% inhibition of H2 photoproduction in photoautotrophic algae after the introduction of 0.05% O2 in vials.43 However, the intracellular H2ase could tolerate up to 0.5% O2.43 Thus, the low level of the H2ase activity in photoautotrophic algae, which is observed in our case (Fig. 2B), could be caused not only by the inactivation of the H2ase enzyme itself but by its impaired biosynthesis. Indeed, Forestier et al. observed a much slower accumulation of H2ase transcripts and induction of the H2 photoproduction activity in photoautotrophically pre-grown algae compared to in photoheterotrophic cultures.44 In the presence of acetate in the medium (Fig. 2A), the concentration of O2 in vials was noticeably lower than that in photoautotrophic algae and is retained at a level sufficient for preventing H2ase enzyme inactivation by intracellular respiration (Fig. 2B).
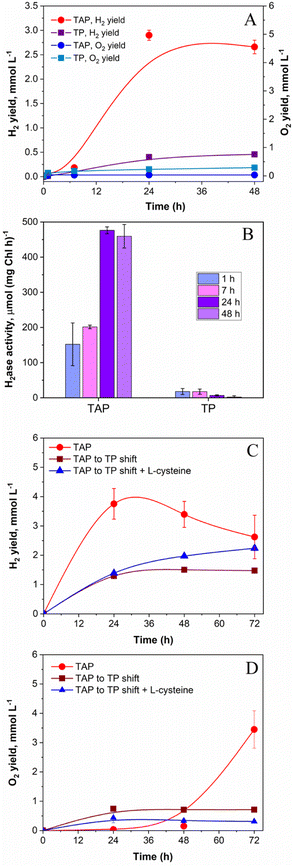 |
| Fig. 2 The effect of cultivation conditions on net H2 photoproduction, net O2 evolution and the H2ase activity in pulse-illuminated algae exposed to 12 μmol m−2 s−1 of background illumination. (A) Net H2 and O2 yields in photoheterotrophic (TAP) and photoautotrophic (TP) cultures. (B) Changes in the in vitro H2ase activity in the same cultures throughout the experiment. A comparison of net H2 photoproduction (C) and net O2 evolution (D) yields in photoheterotrophic cultures (TAP) and photoheterotrophically pre-grown cultures after their shift at t = 0 h to photoautotrophic conditions (TAP to TP shift) in the presence and absence of 4 mM L-cysteine. Experimental cultures contained around 5 (A, B) and 40 (C, D) μg Chl (a + b) ml−1 at the beginning of the experiment. Values are the mean of 3 independent replicates ± SD. | |
In our experimental setup, the highest level (476 μmol H2 (mg Chl h)−1) of the in vitro H2ase activity in photoheterotrophic algae was detected 24 h after application of the pulse-illumination protocol (Fig. 2B), and close to the point where cells began consuming H2 (Fig. 2A). The H2ase activity in photoheterotrophic algae was comparable to the one observed in S-deprived cells during the period of efficient H2 production.23,45 Even at 7 h, the H2ase activity was around 200 μmol H2 (mg Chl h)−1 (Fig. 2B; the 7 h TAP sample), which significantly exceeded the rate of photosynthetic H2 production (42 μmol H2 (mg Chl h)−1) determined for the interval between 7 and 24 h (Fig. 2A; the TAP sample). Thus, the H2ase activity could not be the limiting factor for H2 production in photoheterotrophic algae. It is important to note that the H2ase assay evaluates the maximum enzymatic capacity in vitro, while the situation in vivo might be more complex. On the other hand, photoautotrophic algae possessed a very low H2ase activity (maximum 18 μmol H2 (mg Chl h)−1 at 7 h) that led to the low net H2 photoproduction yield in cultures (Fig. 2, panels A and B; TP samples).
The presence of acetate in the medium during algae growth results in the accumulation of intracellular storage reserves in the form of starch and proteins.46 During H2 production, these reserves can be further utilized either for supporting anaerobiosis in algal cultures as substrates for respiration or for providing reductants to H2ase via the indirect H2 production pathway.47,48 Considering this information and attempting to improve the H2 photoproduction yield under photoautotrophic conditions, we pre-grew algae in the presence of acetate and before the investigation shifted them into photoautotrophic conditions. To ensure high respiratory activity in cultures, experiments were performed at high cell density (∼40 μg Chl (a + b) ml−1). This approach, though, did not enhance the net H2 photoproduction yield in the absence of acetate. As shown in Fig. 2C, the shifted algae produced ∼2.5 times lower H2 than the non-shifted cultures (1.5 vs. 3.75 mmol l−1, respectively). The shifted photoautotrophic algae, even at high cell density, still suffered from the excessive production of O2 (Fig. 2D). For reducing the O2 content in the medium, we applied L-cysteine, which is an efficient O2 scavenger. It shows reducing properties attributed to its thiol group, which in the presence of O2 reacts with the thiol group of another cysteine molecule to generate a disulphide bond and water. Furthermore, L-cysteine does not have inhibitory effects on algal growth and even slightly stimulates it.49 As shown in ESI Fig. 2,† the introduction of 4–8 mM L-cysteine into the cultures is sufficient for supporting H2 photoproduction in pulse-illuminated photoheterotrophic cultures under 12 μmol m−2 s−1 of background illumination. Similarly, the addition of 4 mM L-cysteine in photoautotrophic cultures slightly improved the net H2 photoproduction yield (Fig. 2C). These results indicated the essential role of acetate in supporting anaerobiosis and sustaining H2 production in pulse-illuminated algae under low background illumination. In the absence of acetate, the application of extra O2 scavengers is required for sustaining the H2 production activity in algal cultures.
Effect of H2 partial pressure on the photosynthetic production of H2 by pulse-illuminated algae
Vials with pulse-illuminated algae, which were additionally exposed to low background light, showed a pronounced H2 uptake after 24 h of the experiment (Fig. 1A). The effect was more pronounced in cultures that produced considerable amounts of H2 during the first 24 h (Fig. 1A, 12 and 20 μmol m−2 s−1 trends) just before H2 started to decline. Since the experiments were performed in gas-tight vials, it is unlikely that the effect is caused by the inhibition of H2ase in the presence of co-evolved O2 and the passive leak of H2 from the vials. We suggested the catalytic nature of H2 uptake in algae that occurs simultaneously with H2 release and becomes more pronounced under high H2 partial pressure. Indeed, the reaction of H2 production driven by algal H2ases is highly reversible.50–52 Depending on the redox state of the cells and the partial pressure of H2 in the environment, algae could either produce or oxidize H2. Hydrogen assimilation could be either the result of the oxy-hydrogen reaction, CO2 photoreduction in the CBB cycle or another unknown pathway.26,53,54
To confirm the catalytic nature of H2 uptake in pulse-illuminated algae, we first checked the ability of algal cultures that were exposed to the standard 1 s/9 s pulse-illumination protocol (without background illumination) to photoproduce H2 in the presence of 3% H2 in the headspace of vials. As shown in Fig. 3, under these conditions a very minor H2 production was observed. In the next step, we checked if periodic replacements of headspace gases with Ar without changing the medium could improve H2 photoproduction yield. This approach indeed extended the period of efficient H2 photoproduction by pulse-illuminated C. reinhardtii from 48 h (observed in the control) to above 120 h, yielding 440 μmol H2 (mg Chl)−1 at that point. It should be noted, however, that periodic replacements of headspace gases with Ar remove not only H2 but O2 as well. As we demonstrated above (ESI Fig. 1†), accumulation of O2 in vials may either accelerate H2 uptake by algae or inhibit the H2ase activity in cells depending on the produced O2 level. The addition of an O2 scavenger (8 mM L-cysteine) to the cultures stopped H2 uptake, though without any improvement in the H2 yield (Fig. 3; ESI Fig. 2†), while the addition of the O2 scavenger under low background illumination decreased the rate of H2 consumption by the cultures (ESI Fig. 2†). This indicates that the H2 uptake component depends on the H2 partial pressure in vials and the level of intracellular O2. The dependence of H2 uptake on O2 has also been demonstrated by Milrad et al.54 who observed elevated consumption of H2 in the absence of an O2-scavenging system in contrast to the sample with added glucose, glucose oxidase and catalase. Though, it should be noted that the excessive production of O2 inhibits H2 uptake (ESI Fig. 1†).
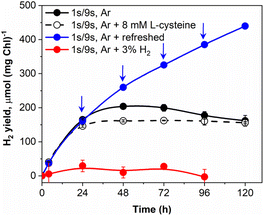 |
| Fig. 3 Effect of H2 partial pressure on net H2 photoproduction under pulse-illumination. The following conditions were tested: the control sample (1 s/9 s, Ar); 8 mM L-cysteine was introduced into anaerobic vials at t = 0 h (1 s/9 s, Ar + 8 mM L-cysteine); headspace gases in the vials were replaced with Ar every 24 h (1 s/9 s, Ar + refreshed); 3% H2 was introduced into the headspace of anaerobic vials at t = 0 h (1 s/9 s, Ar + 3% H2). Arrows indicate the points where the atmosphere of vials was replaced with Ar. The H2 yields were normalized to the initial total Chl content, which was around 9 μg ml−1. The H2 yield in the culture with daily renewals of headspace gases (1 s/9 s, Ar + refreshed) is represented as the cumulative yield. Values are the mean of 3 independent replicates ± SD. | |
Maximizing H2 photoproduction yields in pulse-illuminated cultures under photoheterotrophic and photoautotrophic conditions
As discussed above, the application of the low-intensity background light to pulse-illuminated C. reinhardtii cultures dramatically improves the H2 photoproduction rate but the process could not be sustained for longer than 24 h (Fig. 1A) due to the activation of H2 uptake under increasing H2 partial pressure (Fig. 3). Meanwhile, simultaneous accumulation of O2 in vials due to water-oxidation in PSII may partly accelerate H2 assimilation by the cells (ESI Fig. 1 and 2†) and lead to inactivation of the H2ase enzyme if the O2 content reaches the critical level. Since daily re-flushing of cultures with Ar replaces both H2 and O2 produced by algae, this approach should improve H2 photoproduction not only in cultures exposed to the standard 1 s/9 s pulse-illumination protocol (Fig. 3) but also in algae exposed to the same protocol under low background illumination. To check this, we applied 12 μmol m−2 s−1 background light. As expected, daily replacements of headspace gases to Ar indeed allowed the process to be sustained for longer than 200 h (Fig. 4A). Moreover, the pulse-illuminated algae exposed to 12 μmol m−2 s−1 continuous light significantly outperformed (P < 0.001) cultures without background illumination; H2 photoproduction yields at 216 h were 2.7 and 1.3 mmol H2 (mg Chl)−1, respectively, whereas the pulse-illuminated culture exposed to background light but without headspace refreshments could not sustain the process (Fig. 4A). As shown in the figure, the short (24 h) period of initial H2 production was followed by a long period of H2 uptake.
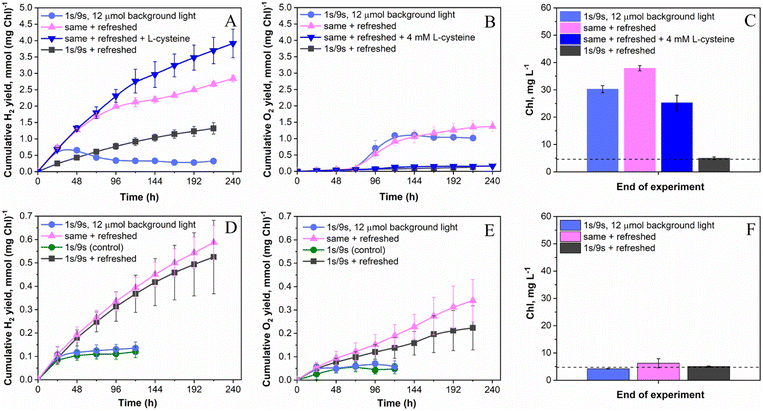 |
| Fig. 4 Sustaining H2 photoproduction in pulse-illuminated algae by the periodic replacement of headspace gases. The experiments were performed under photoheterotrophic (A–C) and photoautotrophic (D–F) conditions. The panels show: (A and D) the cumulative H2 photoproduction yield, (B and E) the cumulative O2 evolution yield, and (C and F) the total Chl (a + b) contents at the end of the experiment compared to the initial total Chl (a + b) contents (shown as dashed lines). For sustained H2 photoproduction, the atmosphere of the culture vials was replaced with Ar every 24 h. For O2 scavenging, 4 mM L-cysteine was introduced directly into the medium at the beginning of the experiment. The H2 and O2 yields were normalized to the initial total Chl content, which was around 5 μg ml−1. The H2 and O2 yields of periodically refreshed cultures are represented as cumulative yields. Values are the mean of 3–9 independent replicates ± SD. | |
The net release of O2 in pulse-illuminated algal cultures, which were exposed to continuous 12 μmol m−2 s−1 background light, occurred only after 72 h of cultivation (Fig. 4B, except the sample with 4 mM L-cysteine). To check whether the effect is linked to activation of the CBB cycle in cells at that point, real-time CO2 exchange measurements using MIMS were performed. As expected, the application of continuous light to pulse-illuminated algae at any stage of the long-term experiment led to activation of CO2 fixation in the CBB cycle (Fig. 5B). In contrast, the control algae that were exposed only to pulse-illumination release CO2. The CO2 release under pulse-illumination has been already explained,17,55 and therefore, will not be discussed in this work. Since the pulse-illuminated algae perform CO2 fixation under continuous low background light from the beginning of the experiment, the net release of O2 observed in cultures after 72 h (Fig. 4B) is likely caused by activation of aerobic metabolism in algal cells at that point leading to enhanced consumption of acetate and further acceleration of CO2 fixation. The latter resulted in a significant culture growth noticed from the increased Chl content (Fig. 4C). In contrast, algal cultures exposed to the standard pulse-illumination protocol did not show any noticeable growth (Fig. 4F). These data bring another piece of evidence on the absence of CO2 fixation in algal cultures just under the train of 1 s/9 s pulses, now demonstrated in the long-term (240 h) process.
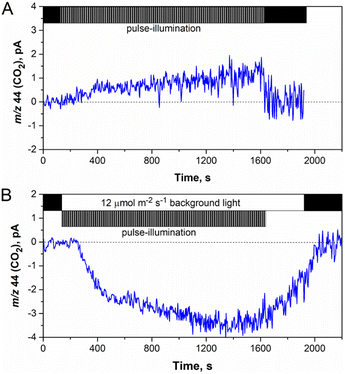 |
| Fig. 5 The effect of background illumination on CO2 exchange in pulse-illuminated algae. (A) The standard (1 s light/9 s dark) pulse-illumination protocol was applied to cultures after 2 min of dark adaptation. (B) The same pulse-illumination was employed simultaneously with continuous 12 μmol m−2 s−1 background light but background light was stopped 5 min before exposure of the sample to darkness. Both protocols were applied to microoxic algal samples, which were handled as described in the Experimental section. | |
To determine the impact of O2 on H2 photoproduction by pulse-illuminated algae during periods between replacements of headspace gases with Ar, we introduced 4 mM L-cysteine into the cultures at the beginning of the experiment. This amount of L-cysteine was sufficient for retaining a microoxic environment in the cultures exposed to background light at least for 240 h (Fig. 4B). The final cumulative O2 yield was ∼8-times lower than that in the similar cultures without L-cysteine (0.16 vs. 1.37 mmol O2 (mg Chl)−1, respectively). Daily levels of O2 in L-cysteine-treated vials were stable and did not exceed 0.03% by the end of each cycle on an average basis. As a result, L-cysteine-treated algae further improved the H2 photoproduction yield to above 3.9 mmol H2 (mg Chl)−1 (Fig. 4A) or ∼20 mmol l−1. This amount of H2 was at least 6-times higher than that in the standard 1 s/9 s pulse-illumination protocol (1.5–3 mmol l−1) as demonstrated previously27 and calculated from data shown in Fig. 1A, 2A and 3. Due to excessive growth (Fig. 4C), the H2 photoproduction activity in L-cysteine-treated cultures still suffered from competition with the CBB cycle. Therefore, we expect that the improved H2 production yield under this condition can be achieved by further limitation of photosynthetic electron flow to the CBB cycle, for example, in Rubisco-deficient C. reinhardtii mutants with impaired CO2 fixation capacity. Working with the Rubisco-deficient CC-2803 strain, Hemschemeier and co-authors48 demonstrated that this mutant can produce H2 without S-deprivation, but alga experiences enhanced photoinhibition under H2 production conditions. The latter might be resolved by the application of the pulse-illumination approach.
Thus, the major trigger for the induction of efficient H2 photoproduction in anaerobic algae seems to be the limitation of the CBB cycle. In the absence of CO2 fixation, H2 generation is one of the major electron sinks for supporting the PSII activity in an anaerobic environment.48 For example, in S-deprived algae, a major degradation of the Rubisco enzyme occurs before the onset of efficient H2 production,24,56 while restoration of the linear photosynthetic electron flow from PSII coincides with the appearance of H2 in cultures.57,58 In nutrient-replete dark-adapted algae, the activation of CO2 fixation results in the loss of H2 production activity and initiates H2 uptake.15 If activation of the CBB cycle is prevented by substrate-limitation16 or pulse-illumination (as discussed in this paper), the cultures are capable of sustaining H2 photoproduction for a prolonged period. All these data indicate the strong competition between these two metabolic pathways. Nevertheless, our current experimental data suggest the existence of the steady-state condition in which H2 photoproduction and CO2 fixation operate simultaneously (Fig. 4, A–C panels). Here, algal growth is supported by the assimilation of acetate in the presence of photosynthetically produced O2. On the other hand, respiration of acetate retains an anaerobic environment in vials, and protects H2ase from O2 inactivation.59–61
H2 photoproduction, O2 evolution and Chl content profiles noticeably changed when the same experiments were performed in photoautotrophic algae (Fig. 4, D–F panels). First, all cultures produced significantly (P < 0.01 for the worst pair) less H2 than that under photoheterotrophic conditions (Fig. 4, A and D panels). Second, in the absence of acetate, the pulse-illuminated algae released H2 and O2 in a stoichiometry close to that of direct water biophotolysis (2 mol H2 to 1 mol O2) in all daily refreshed cultures throughout the experiment (Fig. 4, compare D and E panels). Third, all cultures did not show any pronounced growth under any condition tested (Fig. 4F). As previously mentioned, the H2 photoproduction activity in photoautotrophic algae is limited by the H2ase activity (Fig. 2B), which suffers from the excessive net O2 produced by algae. As a result, the application of background illumination did not show any significant effect on H2 photoproduction yield both in daily refreshed and non-refreshed algae (Fig. 4D), though daily replacement of headspace gases with Ar did improve H2 photoproduction, as expected. The final H2 photoproduction yields in these cultures were around 0.5 and 0.6 mmol (mg Chl)−1 or 2.5 and 2.9 mmol l−1 under the standard pulse-illumination protocol and the same protocol with 12 μmol m−2 s−1 of background light, respectively. The corresponding non-refreshed cultures produced approximately the same amount of H2 of around 0.12 mmol H2 (mg Chl)−1 or around 0.6 mmol H2 l−1. In contrast to photoheterotrophic algae, photoautotrophic cultures placed in sealed vials under an Ar atmosphere were limited not only by acetate but CO2 as well. As a result, they did not show any significant growth. The conditions were similar to ones applied in the substrate (CO2) limitation protocol for initiation of sustained H2 photoproduction in photoautotrophic cultures under continuous light.16 It is important to note though, that the original CO2 limitation protocol is performed at high cell density (up to 150 μg total Chl l−1) and initiated by the exposure of algae to light after dark anaerobic adaptation for up to 4 h.16,62 The latter creates conditions for full expression of H2ase in algal cells, while the former prevents the inactivation of H2ase by O2 throughout H2 production due to the high respiratory activity in the dense algal cultures.
In the absence of CO2 and acetate, anaerobic algae could not perform normal photosynthesis that links the water oxidation reaction to CO2 fixation, and therefore algal cells predominantly derive O2via the water-splitting reaction (see eqn (1) and (2) in the Introduction section). Since respiration is also limited by the microoxic environment (photosynthetically produced O2 is diluted in an Ar atmosphere), it is not surprising that under these conditions we observed the stoichiometry of 2 mol H2 to 1 mol O2 during almost the whole period of H2 production. It is clear that H2 photoproduction in photoautotrophic cultures could only be sustained if O2 is efficiently removed from the cultures by periodic purging with Ar (Fig. 4D) and/or introduction of O2 scavengers and O2 absorbents.62–64 Nevertheless, our data show that substantial amounts of H2 can be produced by photoautotrophic algae in the long-term process since the water-splitting reaction, driven by the photosynthetic apparatus in tight connection with the H2ase enzyme, seems to provide cells with the energy for supporting their metabolic activity at a minimal level.
Conclusions
The factors affecting the long-term H2 photoproduction activity in pulse-illuminated green algae are summarized in Fig. 6. Among positive factors leading to the increased H2 photoproduction yield are inactivation of CO2 fixation by algal cells, no (or moderate) H2 recycling by the cells under high H2 partial pressure, and an anaerobic or microoxic environment preventing inactivation of the H2ase in algal cells. Non-compliance with these factors causes the activation of H2 uptake by algae and, in the worst case, the termination of H2 photoproduction in algal cultures due to activation of CO2 fixation and/or inactivation of the H2ase enzyme in cells.
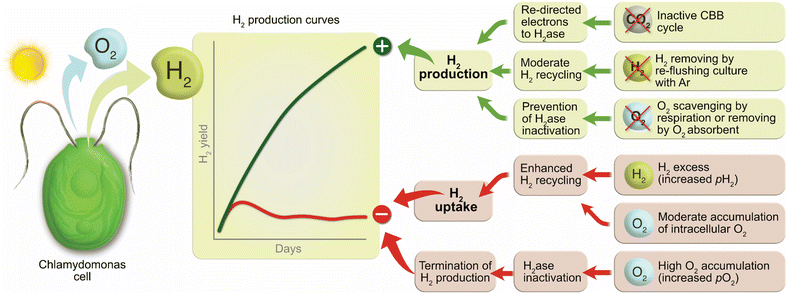 |
| Fig. 6 Factors affecting H2 photoproduction in pulse-illuminated algal cultures. | |
Thus, for achieving efficient and sustained H2 photoproduction in nutrient-replete pulse-illuminated algae, our research suggests the accomplishment of the following three requirements:
(1) O2 accumulation in cultures should be contained within the levels favourable to the induction and operation of the H2ase enzyme in algal cells. Under photoautotrophic conditions, this requirement could be satisfied by periodic purging of the cultures with Ar and/or by introducing O2 scavengers and O2 absorbents into the cultures. In photoheterotrophic algae, respiratory activity in cultures should be sufficient for removing photosynthetically produced O2. Thus, the limitation of respiratory activity by substrates (acetate in the case of C. reinhardtii) should be avoided.
(2) The produced H2 should be kept at sufficiently low levels for preventing H2 recycling by algal cells. For example, the produced H2 can be re-utilized by coupling H2-producing algal cultures to a H2-consuming fuel cell in a hybrid system to produce power.
(3) H2 photoproduction should not compete with CO2 fixation. In the future, this requirement could be resolved by metabolic engineering of production strains, while in the current state, pulse-illumination, and substrate (CO2) limitation protocols could be sufficient for preventing competition with the CBB cycle.
Author contributions
S. K. and Y. A.: conceptualization, methodology, funding acquisition and project management; S. V. and S. K.: investigation and formal analysis; S. K. and Y. A.: supervision and data validation; S. V. and S. K.: writing – original draft; S. K. and Y. A.: writing – review and editing.
Conflicts of interest
The authors declare that they have no known competing financial interests or personal relationships that could have appeared to influence the work reported in this paper.
Acknowledgements
This work was supported by the Kone Foundation (project no. 201608799), the NordForsk Nordic Center of Excellence “NordAqua” (project no. 82845), the EU FET Open Project FuturoLEAF under grant agreement no. 899576, and the Academy of Finland (AlgaLEAF, project no. 322754). The research was performed in the PhotoSYN Finnish Infrastructure for Photosynthesis Research.
References
- R. W. Howarth and M. Z. Jacobson, Energy Sci. Eng., 2021, 9, 1676–1687 CrossRef CAS.
-
V. A. Boichenko, E. Greenbaum and M. Seibert, in Molecular to Global Photosynthesis: Photoconversion of Solar Energy, ed. J. Barber and M. D. Archer, Imperial College Press, London, 2004, pp. 397–451 Search PubMed.
- S. Z. Tóth and I. Yacoby, Trends Biotechnol., 2019, 37, 1159–1163 CrossRef PubMed.
- A. Magnuson, F. Mamedov and J. Messinger, Joule, 2020, 4, 1157–1159 CrossRef CAS.
- A. Li, X. Chang, Z. Huang, C. Li, Y. Wei, L. Zhang, T. Wang and J. Gong, Angew. Chem., Int. Ed., 2016, 55, 13734–13738 CrossRef CAS PubMed.
- H. Huo, D. Liu, H. Feng, Z. Tian, X. Liu and A. Li, Nanoscale, 2020, 12, 13912–13917 RSC.
- E. Fudo, A. Tanaka, S. Iguchi and H. Kominami, Sustain. Energy Fuels, 2021, 5, 3303–3311 RSC.
- L. Nikkanen, D. Solymosi, M. Jokel and Y. Allahverdiyeva, Physiol. Plant., 2021, 173, 514–525 CrossRef CAS PubMed.
-
L. Nikkanen, M. Hubacek and Y. Allahverdiyeva, in Photosynthesis. Biotechnological Applications with Microalgae, De Gruyter, 2021, pp. 257–278 Search PubMed.
- Y. Allahverdiyeva, E.-M. Aro, B. van Bavel, C. Escudero, C. Funk, J. Heinonen, L. Herfindal, P. Lindblad, S. Mäkinen, M. Penttilä, K. Sivonen, M. Skogen Chauton, H. Skomedal and J. Skjermo, Physiol. Plant., 2021, 173, 507–513 CrossRef CAS PubMed.
- E. Greenbaum, Biophys. J., 1988, 54, 365–368 CrossRef CAS PubMed.
-
A. A. Tsygankov, in Microbial Technologies in Advanced Biofuels Production, ed. P. C. Hallenbeck, Springer US, Boston, MA, 2012, pp. 29–51 Search PubMed.
- M. L. Ghirardi, R. K. Togasaki and M. Seibert, Appl. Biochem. Biotechnol., 1997, 63–65, 141–151 CrossRef CAS PubMed.
- M. C. Posewitz, P. W. King, S. L. Smolinski, R. D. Smith, A. R. Ginley, M. L. Ghirardi and M. Seibert, Biochem. Soc. Trans., 2005, 33, 102–104 CrossRef CAS PubMed.
- Y. Milrad, S. Schweitzer, Y. Feldman and I. Yacoby, Plant Physiol., 2018, 177, 918–926 CrossRef CAS PubMed.
- V. Nagy, A. Podmaniczki, A. Vidal-Meireles, R. Tengölics, L. Kovács, G. Rákhely, A. Scoma and S. Z. Tóth, Biotechnol. Biofuels, 2018, 11, 69 CrossRef PubMed.
- S. Kosourov, V. Nagy, D. Shevela, M. Jokel, J. Messinger and Y. Allahverdiyeva, Proc. Natl. Acad. Sci. U.S.A, 2020, 117, 29629–29636 CrossRef CAS PubMed.
- A. Melis and T. Happe, Plant Physiol., 2001, 127, 740–748 CrossRef CAS PubMed.
- A. Melis, L. Zhang, M. Forestier, M. L. Ghirardi and M. Seibert, Plant Physiol., 2000, 122, 127–136 CrossRef CAS PubMed.
- K. A. Batyrova, A. A. Tsygankov and S. N. Kosourov, Int. J. Hydrogen Energy, 2012, 37, 8834–8839 CrossRef CAS.
- M. He, L. Li, L. Zhang and J. Liu, Int. J. Hydrogen Energy, 2012, 37, 16903–16915 CrossRef CAS.
- A. Volgusheva, G. Kukarskikh, T. Krendeleva, A. Rubin and F. Mamedov, RSC Adv., 2015, 5, 5633–5637 RSC.
- S. Kosourov, A. Tsygankov, M. Seibert and M. L. Ghirardi, Biotechnol. Bioeng., 2002, 78, 731–740 CrossRef CAS PubMed.
- L. Zhang, T. Happe and A. Melis, Planta, 2002, 214, 552–561 CrossRef CAS PubMed.
- A. Dubini and M. L. Ghirardi, Photosynth. Res., 2015, 123, 241–253 CrossRef CAS PubMed.
- S. Kosourov, M. Böhm, M. Senger, G. Berggren, K. Stensjö, F. Mamedov, P. Lindblad and Y. Allahverdiyeva, Physiol. Plant., 2021, 173, 555–567 CrossRef CAS PubMed.
- S. Kosourov, M. Jokel, E.-M. Aro and Y. Allahverdiyeva, Energy Environ. Sci., 2018, 11, 1431–1436 RSC.
- M. Jokel, V. Nagy, S. Z. Tóth, S. Kosourov and Y. Allahverdiyeva, Biotechnol. Biofuels, 2019, 12, 280 CrossRef CAS PubMed.
- J. R. Bolton and D. O. Hall, Photochem. Photobiol., 1991, 53, 545–548 CrossRef CAS.
- R. E. Blankenship, D. M. Tiede, J. Barber, G. W. Brudvig, G. Fleming, M. Ghirardi, M. R. Gunner, W. Junge, D. M. Kramer, A. Melis, T. A. Moore, C. C. Moser, D. G. Nocera, A. J. Nozik, D. R. Ort, W. W. Parson, R. C. Prince and R. T. Sayre, Science, 2011, 332, 805–809 CrossRef CAS PubMed.
- E. Touloupakis, C. Faraloni, A. M. Silva Benavides, J. Masojídek and G. Torzillo, Int. J. Hydrogen Energy, 2021, 46, 3684–3694 CrossRef CAS.
- Y. Chen, Energy Environ. Sci., 2022, 15, 2843–2857 RSC.
- T. E. Crozier and S. Yamamoto, J. Chem., 1974, 19, 242–244 CAS.
- H. E. Garcia and L. I. Gordon, Limnol. Oceanogr., 1992, 37, 1307–1312 CrossRef CAS.
-
E. H. Harris, The Chlamydomonas Sourcebook: a Comprehensive Guide to Biology and Laboratory Use, Academic Press, San Diego, 1989 Search PubMed.
- A. Santana-Sánchez, L. Nikkanen, E. Werner, G. Tóth, M. Ermakova, S. Kosourov, J. Walter, M. He, E.-M. Aro and Y. Allahverdiyeva, New Phytol., 2023, 237, 126–139 CrossRef PubMed.
- F. P. Healey, Plant Physiol., 1970, 45, 153–159 CrossRef CAS PubMed.
- P. J. Aparicio, M. P. Azuara, A. Ballesteros and V. M. Fernandez, Plant Physiol., 1985, 78, 803–806 CrossRef CAS PubMed.
- B. Degrenne, J. Pruvost and J. Legrand, Bioresour. Technol., 2011, 102, 1035–1043 CrossRef CAS PubMed.
- T. Rühle, A. Hemschemeier, A. Melis and T. Happe, BMC Plant Biol., 2008, 8, 107 CrossRef PubMed.
- S. T. Stripp, G. Goldet, C. Brandmayr, O. Sanganas, K. A. Vincent, M. Haumann, F. A. Armstrong and T. Happe, Proc. Natl. Acad. Sci. U. S. A., 2009, 106, 17331–17336 CrossRef CAS PubMed.
- A. Scoma, L. Durante, L. Bertin and F. Fava, New Phytol., 2014, 204, 890–900 CrossRef CAS PubMed.
- J. W. Lee and E. Greenbaum, Appl. Biochem. Biotechnol., 2003, 105–108, 303–313 CrossRef CAS PubMed.
- M. Forestier, P. King, L. Zhang, M. Posewitz, S. Schwarzer, T. Happe, M. L. Ghirardi and M. Seibert, Eur. J. Biochem., 2003, 270, 2750–2758 CrossRef CAS PubMed.
- L. Zhang and A. Melis, Philos. Trans. R. Soc. London, 2002, 357, 1499–1507 CrossRef CAS PubMed.
- G. Zamzam, C. W. J. Lee, F. Milne, J. Etsell and D. G. Durnford, Algal Res., 2022, 64, 102676 CrossRef.
- S. Fouchard, J. Pruvost, B. Degrenne and J. Legrand, Int. J. Hydrogen Energy, 2008, 33, 3302–3310 CrossRef CAS.
- A. Hemschemeier, S. Fouchard, L. Cournac, G. Peltier and T. Happe, Planta, 2008, 227, 397–407 CrossRef CAS PubMed.
- L. A. Márquez-Reyes, M. del P. Sánchez-Saavedra and I. Valdez-Vazquez, Int. J. Hydrogen Energy, 2015, 40, 7291–7300 CrossRef.
- S. N. Kosourov, K. A. Batyrova, E. P. Petushkova, A. A. Tsygankov, M. L. Ghirardi and M. Seibert, Int. J. Hydrogen Energy, 2012, 37, 8850–8858 CrossRef CAS.
- S. Noone, K. Ratcliff, R. Davis, V. Subramanian, J. Meuser, M. C. Posewitz, P. W. King and M. L. Ghirardi, Algal Res., 2017, 22, 116–121 CrossRef.
- A. Scoma and A. Hemschemeier, Algal Res., 2017, 26, 341–347 CrossRef.
- T. E. Maione and M. Gibbs, Plant Physiol., 1986, 80, 364–368 CrossRef CAS PubMed.
- Y. Milrad, S. Schweitzer, Y. Feldman and I. Yacoby, Plant Physiol., 2021, 186, 168–179 CrossRef CAS PubMed.
- D. Shevela, H.-N. Do, A. Fantuzzi, A. W. Rutherford and J. Messinger, Biochemistry, 2020, 59, 2442–2449 CrossRef CAS PubMed.
- V. Nagy, A. Vidal-Meireles, A. Podmaniczki, K. Szentmihályi, G. Rákhely, L. Zsigmond, L. Kovács and S. Z. Tóth, Plant J., 2018, 94, 548–561 CrossRef CAS PubMed.
- T. K. Antal, T. E. Krendeleva, T. V. Laurinavichene, V. V. Makarova, M. L. Ghirardi, A. B. Rubin, A. A. Tsygankov and M. Seibert, Biochim. Biophys. Acta – Bioenerg., 2003, 1607, 153–160 CrossRef CAS PubMed.
- A. A. Volgusheva, M. Jokel, Y. Allahverdiyeva, G. P. Kukarskikh, E. P. Lukashev, M. D. Lambreva, T. E. Krendeleva and T. K. Antal, Physiol. Plant., 2017, 161, 124–137 CrossRef CAS PubMed.
- S. Fouchard, A. Hemschemeier, A. Caruana, J. Pruvost, J. Legrand, T. Happe, G. Peltier and L. Cournac, Appl. Environ. Microbiol., 2005, 71, 6199–6205 CrossRef CAS PubMed.
- S. Kosourov, E. Patrusheva, M. L. Ghirardi, M. Seibert and A. Tsygankov, J. Biotechnol., 2007, 128, 776–787 CrossRef CAS PubMed.
- B. Degrenne, J. Pruvost, G. Christophe, J. F. Cornet, G. Cogne and J. Legrand, Int. J. Hydrogen Energy, 2010, 35, 10741–10749 CrossRef CAS.
- V. Nagy, A. Podmaniczki, A. Vidal-Meireles, S. Kuntam, É. Herman, L. Kovács, D. Tóth, A. Scoma and S. Z. Tóth, Bioresour. Technol., 2021, 333, 125217 CrossRef CAS PubMed.
- W. Ma, M. Chen, L. Wang, L. Wei and Q. Wang, Bioresour. Technol., 2011, 102, 8635–8638 CrossRef CAS PubMed.
- F. Khosravitabar and M. Hippler, Int. J. Hydrogen Energy, 2019, 44, 17835–17844 CrossRef CAS.
|
This journal is © The Royal Society of Chemistry 2023 |
Click here to see how this site uses Cookies. View our privacy policy here.