DOI:
10.1039/D3SC01036H
(Review Article)
Chem. Sci., 2023,
14, 5842-5871
Organelle-targeting ratiometric fluorescent probes: design principles, detection mechanisms, bio-applications, and challenges
Received
23rd February 2023
, Accepted 27th April 2023
First published on 12th May 2023
Abstract
Biological species, including reactive oxygen species (ROS), reactive sulfur species (RSS), reactive nitrogen species (RNS), F−, Pd2+, Cu2+, Hg2+, and others, are crucial for the healthy functioning of cells in living organisms. However, their aberrant concentration can result in various serious diseases. Therefore, it is essential to monitor biological species in cellular organelles such as the cell membrane, mitochondria, lysosome, endoplasmic reticulum, Golgi apparatus, and nucleus. Among various fluorescent probes for species detection within the organelles, ratiometric fluorescent probes have drawn special attention as a potential way to get beyond the drawbacks of intensity-based probes. This method depends on measuring the intensity change of two emission bands (caused by an analyte), which produces an efficient internal referencing that increases the detection's sensitivity. This review article discusses the literature publications (from 2015 to 2022) on organelle-targeting ratiometric fluorescent probes, the general strategies, the detecting mechanisms, the broad scope, and the challenges currently faced by fluorescent probes.
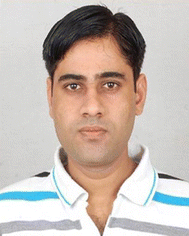 Manoj Kumar Goshisht | Dr Manoj Kumar Goshisht is an Assistant Professor of Chemistry at Govt Naveen College Tokapal, Bastar, Chhattisgarh, India. He obtained his masters degree from Guru Jambheshwar University of Science & Technology, Hisar, Haryana, and PhD degree from Dr B. R. Ambedkar National Institute of Technology, Jalandhar, Punjab, India. He has cleared the National Eligibility Test (CSIR-UGC NET) for Assistant Professor in the subject of Chemical Sciences. He is a winner of the Editors Pick Award of the ‘‘9th DST & ACS Workshop.’’ Currently, he is a visiting scientist at University of Wisconsin—Green Bay, USA. His research interests include organic chemistry, materials chemistry, supramolecular chemistry, and nanomaterials. He has published various research papers in reputed international peer-reviewed journals of The American Chemical Society, The Royal Society of Chemistry, and Springer Nature. He also has a book to his credit published by CRC Press (an imprint of the Taylor & Francis group). |
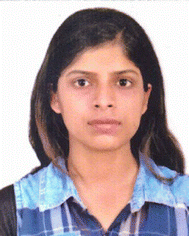 Neetu Tripathi | Dr Neetu Tripathi obtained her PhD from Guru Nanak Dev University, Amritsar, India. She completed her masters with 1st rank (Gold medal) in MSc Chemistry (Instrumental Analysis). She has been a recipient of the prestigious INSPIRE fellowship of the Government of India during her PhD She has also cleared the National Eligibility Test for Assistant Professor (CSIR-UGC NET) and the Graduate Aptitude test (GATE) in the subject of chemical sciences. Her research interest includes organic chemistry, materials chemistry, supramolecular chemistry, and nanomaterials. She has published various research papers in international peer-reviewed journals (Royal Society of Chemistry, Elsevier journals and Springer journal). She is also an author of a book published by CRC press (an imprint of the Taylor & Francis group). |
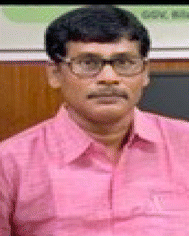 Goutam Kumar Patra | Prof. Goutam Kumar Patra, Head of the Department of Chemistry, Guru Ghasidas Central University, Bilaspur did PhD from Jadavpur University, under the supervision of Prof. Dipankar Datta at Indian Association for the Cultivation of Science, Kolkata. Then he joined the Tel Aviv University, Israel, as a postdoctoral research fellow with Prof. Israel Goldberg (2000–2002). Subsequently he moved to the Carnegie Mellon University, USA where he worked with Prof. Catalina Achim. Successively he joined as Asst. Prof. in Vijoygarh Jyotish Ray College, Kolkata in December 2003. He visited Max Planck Institute of Bioinorganic Chemistry, Mülheim, Germany as a BOYSCAST fellow during 2006–07 and worked in the group of the then Director, Prof. Karl Wieghardt. He has been a Professor in Guru Ghasidas Central University, Bilaspur since 2012 and former Dean, School of Physical Science. His research interests include chemosensors, redox activity, aza macrocyclic chemistry, crystal engineering, porphyrin and supramolecular chemistry, peptide nucleic acids (PNAs) and free radical chemistry. So far, he has published more than hundred research papers in journals of national and international repute and guided eight doctoral students and one post-doctoral student. |
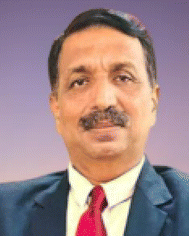 Manohar Chaskar | Dr Manohar Chaskar is the Dean of the Science and Technology Faulty of Savitribai Phule Pune University. Dr Chaskar completed his PhD in materials science, and his post-doc from Nagoya University, Japan in photocatalysis. Dr Chaskar's research interests include the use of nanomaterials in catalyzing organic reactions. Dr Chaskar has published several high impact research articles in reputed national and international journals and has one patent to his credit while two are in the process. He is also a visiting scientist in Germany and Japan. |
1 Introduction
In this modern era, everyone is interconnected. Similarly, in living beings, cells, organelles and biological species are interconnected and act cooperatively for the normal functioning of living systems.1,2 For instance, biological species (anions, cations, thiols, nitrogen and oxygen) perform a variety of role in organelles such as signaling molecules, enzyme cofactors, etc. However, their chemical imbalance can cause cellular malfunction.3 Therefore, smart research work on organelles and biological species is essential, which is challenging.
Over the years, significant studies and research work have been done on organelles and biological species present within the organelles. For example, organelle-targeting medicine has been developed for curing various diaeases.4,5 Bioimaging technique has been developed for examining biomolecules in living cells and tissues.6 Choi et al. studied fluorescent probes for organelles with respect to recent advances and bio-applications.7
Nowadays, fluorescent probes for targeting organelles have gained more attention due to their excellent photo-physical characteristics, high sensitivity, rapid response, low cost, non-invasiveness, and real-time imaging.8 Notably, many single emission probes are unable to withstand environmental interference. Through a built-in correction of two emissions at different wavelengths, ratiometric fluorescence probes could eliminate background interference.9 Additionally, ratiometric fluorescence imaging has an even better resolution due to the two clearly defined emission peaks.10 Therefore, the development of ratiometric fluorescent probes is a winning strategy for the sensitive detection of small molecules, thanks to its reduced environmental effect.
Although there are several outstanding reviews in the literature based on ratiometric probes with sensing applications, each one has concentrated on a single aspect, such as the optical processes, a particular class of fluorophores, or a specific subset of target analytes. In 2018, Huang et al. reported ratiometric optical nanoprobes for molecular detection and imaging.10 In an excellent review, the design principles and applications of fluorescent probes in the ratiometric detection of anions, cations, and biological molecules has been beautifully demonstrated.11 Another review article highlighted various chemo/probe-based semiconductor quantum dots (QDs).12
In 2020, Wu et al. summarized recent advancements in the metal–organic framework (MOF)-based ratiometric fluorescent probes.13 Recently, Bigdeli et al. published a review article on fluorescent nanoprobes for visual detection.8
We illustrate the design principles, fundamental detection mechanisms, and applications of organelle-targeting ratiometric fluorescent probes. The current limitations and prospective future directions, which would spur additional research interest and bring up fresh opportunities for biological analysis, are also covered.
2 Design principles
In designing fluorescent probes, photo-physical parameters such as photo-induced electron transfer (PET), internal charge transfer (ICT), monomer–excimer formation, Förster resonance energy transfer (FRET), and excited state intramolecular proton transfer (ESIPT) are frequently used. FRET is a relatively excellent strategy for designing ratiometric fluorescent probes and increasing the Stokes shift.14
Generally, the design of ratiometric probes involves the combination of two fluorophores, one reference fluorophore (may or may not show a change in emission intensity upon interaction with the analyte) and another dynamic fluorophore (always shows a change in emission intensity upon interaction with the analyte).8 Generally, ratiometric changes in the emission spectrum are of the following type:
(I) Static + dynamic change: herein, upon the addition of an analyte, the emission intensity of one fluorophore is almost kept unchanged, whereas other fluorophores may undergo an increase/decrease/shift in emission intensity (Fig. 1A).15,16
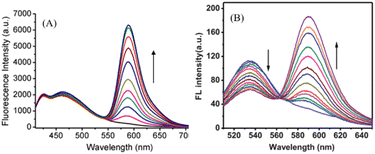 |
| Fig. 1 Schematic representation of the ratiometric fluorescence change when (A) static + dynamic fluorophores (reprinted from ref. 15, copyright 2019, Elsevier) and (B) dynamic + dynamic fluorophores (reprinted from ref. 17, copyright 2012, The Royal Society of Chemistry) are utilized. | |
(II) Dynamic + dynamic change: herein, both the fluorophores change (increase/decrease/or shift in emission intensity), but in opposite directions (Fig. 1B).17
Generally, organelle-targeting ratiometric fluorescent probes contain a fluorophore, recognition units, and targeting moieties (Fig. 2). The organelle-targeting groups are selective to specific organelles. For example, due to its alkalinity, the morpholine group is a conventional lysosome-targeting moiety.18 In addition, the polarity-dependent approach helps create various biological probes, which are helpful for imaging multiple organelles.19
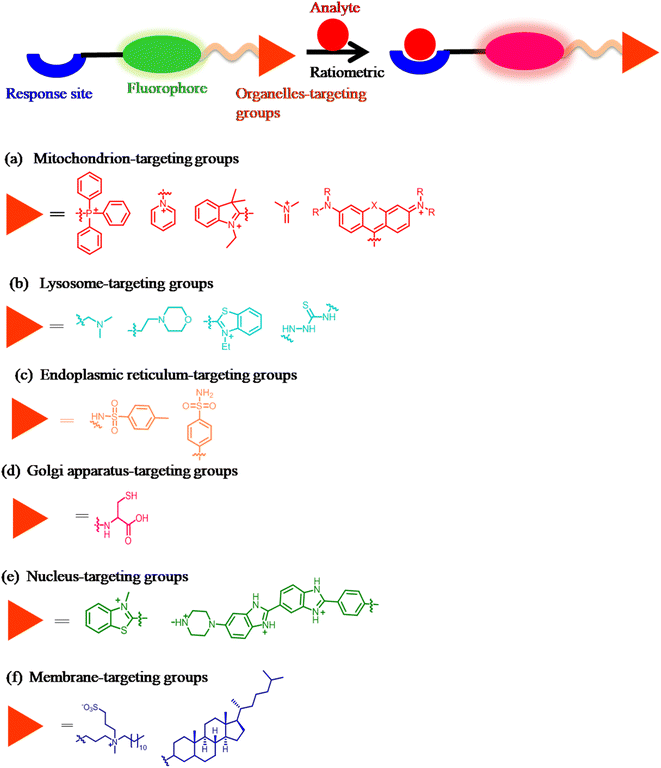 |
| Fig. 2 General strategies for designing organelle-targeting ratiometric fluorescent probes and common organelle-targeting groups for (a) mitochondria; (b) lysosome; (c) endoplasmic reticulum; (d) Golgi apparatus; (e) nucleus; (f) membrane. | |
2.1 Designing mitochondria-targeting ratiometric fluorescent probes
Since the mitochondrial membrane typically has a potential of −180 mV, this property is employed to direct lipophilic positively charged probes into the mitochondria.20 The common mitochondrial-targeting units include the quaternized pyridine moiety,21 triphenylphosphonium (TPP),22,23 indole,24 cyanine,25,26 pyridinium,27,28 and rhodamine29 (Fig. 2a).30–33 Furthermore, to ascertain the mitochondria localization of probes in cells, the co-localization experiment was performed using commercially available mitochondrion-specific dyes such as MitoTracker Green FM, MitoTracker Red, and MitoTracker Orange.30 Generally, mitochondrion-targeting fluorescent probes consist of a fluorophore linked to the mitochondrion-targeting moiety and an activation unit.
2.2 Designing lysosome-targeting ratiometric fluorescent probes
Modifying them with lipophilic amines is the most popular method for directing probes into lysosomes.34–36 Due to the membrane-impermeable protonated amines in lysosomes, selective probe trapping occurs. The pyridine group,37 monothio-bishydrazide moiety,14 and morpholine15 are the common lysosome-targeting groups (Fig. 2b). Typically, the design of the probe for lysosome requires linking of the fluorophore with the lysosome-targeting group and an activation unit.
2.3 Designing endoplasmic reticulum-targeting ratiometric fluorescent probes
The commonly used ER-targeting moieties include glibenclamide,38 methyl sulphonamide,39 and the p-toluenesulfonamide group (Fig. 2c).40,41 ER-targeting fluorescent probes generally have (1) a moderate size (conjugated band numbers (CBN < 40)), (2) a cationic character, and (3) an appropriate lipophilicity (+6 > log
Poct > 0).42 The ER-targeting fluorescent probes mainly track cellular concentrations of stress-responsive substances like NO, H2S, H2O2, and HOCl.43,44
2.4 Designing Golgi-apparatus-targeting ratiometric fluorescent probes
Motivated by the abundance of cysteine residues in the Golgi apparatus, Huang and coworkers proved L-cysteine as an effective Golgi apparatus targeting ligand. They created various probes using this technique (Fig. 2d).45–48
2.5 Designing nucleus-targeting ratiometric fluorescent probes
The nuclear envelope is a highly controlled membrane barrier. Therefore, passive diffusion or active transport uses the nuclear pore complex (NPC) to target the nucleus.49 Small fluorescent probes with cationic centers and hydrophobic planar aromatic structures can selectively label DNA molecules by focusing on the minor grooves in DNA (negatively charged double strands). Some of them have been made available for purchase.50,51 From a molecular docking experiment, Ma et al. demonstrated electrostatic binding between a positively charged probe and negatively charged nucleus RNA major groove (affinity energy = −5.78 kcal mol−1).52 Nucleus-targeting unit functionalization is an excellent strategy for delivering fluorescent functional probes into the nucleus of living cells (Fig. 2e).53,54
2.6 Designing membrane-targeting ratiometric fluorescent probes
Currently, the available probes for the membrane share a common approach: the conjugation of an environment-sensitive fluorophore to generate membrane-specific signals and a membrane-anchoring moiety to minimize the diffusion of the probe (Fig. 2f).7
3 Detection mechanism
The various fluorescence-based sensing mechanisms include photo-induced electron transfer (PET), internal charge transfer (ICT), monomer–excimer formation, Förster resonance energy transfer (FRET), and excited state intra-molecular proton transfer (ESIPT). These mechanisms are available in detail in our recent publications55–57 and some excellent reviews.9,58 In this section, we explain the fluorescence-based sensing mechanisms briefly. The HOMO localizes on the donor moieties in the ICT-based fluorescent probe. The LUMO is centered on acceptor moieties, thus creating a solid dipole with a charge transfer phenomenon upon excitation. The preferential interaction of the analyte at either the donor or acceptor results in a change in dipole strengths and, consequently, spectral shifts (Fig. 3). To effectively measure ratiometrically, an ICT-based probe should exhibit a visible difference in fluorescence intensity as well as a significant change in emission wavelength. In the PET process (Fig. 4), upon excitation, an electron is transferred from the HOMO (highest occupied molecular orbital) of the receptor (donor) to the LUMO (lowest unoccupied molecular orbital) of the fluorophore (acceptor). However, in the case of the guest-bounded receptor, the HOMO energy levels become lower than those of the fluorophore, inhibiting the PET process and fluorescence change. In the monomer–excimer-based sensing mechanism, upon the addition of an analyte, generally excited state complex formation (excimer) occurs by the interaction between the excited states of one fluorophore and the ground state of another molecule (Fig. 5). The probes that include polyaromatic hydrocarbon (PAH) moieties, such as pyrene, anthracene, etc., typically display this type of sensing mechanism. In the FRET process, energy is transferred from the excited donor molecule to the ground-state acceptor molecule. The critical parameter which governs the FRET phenomenon is spectral overlaps (donor emission spectrum and acceptor absorption spectrum) (Fig. 6). FRET-based probes prove to be an excellent tool for ratiometric imaging due to the stoichiometric relationship between the donor (D) and acceptor (A). In the ESIPT process, proton transfer occurs from the preferred enol-form to the excited state keto-form upon excitation. During relaxation, the excited state keto-form converts back to the enol form by reverse proton transfer. Interestingly, intense fluorescence, large Stokes-shift, and photo-stability are the various unique features of ESIPT-based probes (Fig. 7).
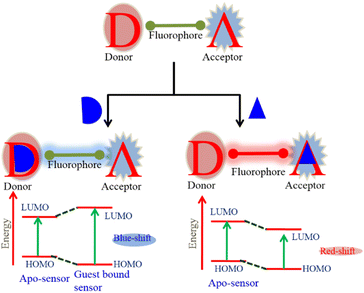 |
| Fig. 3 Schematic representation of the internal charge transfer (ICT)-based sensing mechanism in ratiometric design. | |
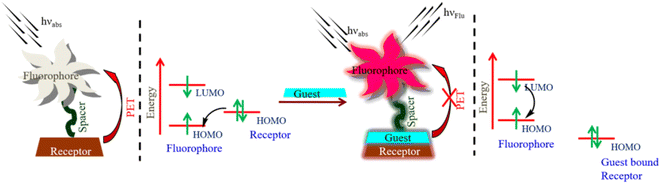 |
| Fig. 4 Schematic representation of the photo-induced electron transfer (PET)-based sensing mechanism in ratiometric design. | |
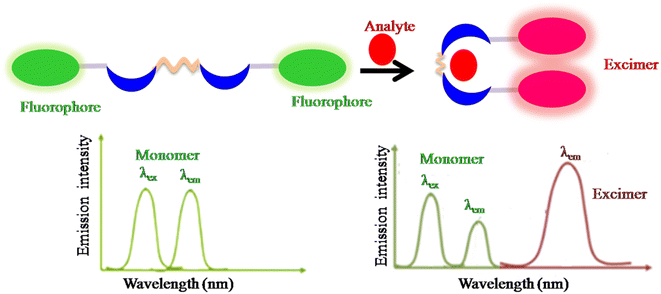 |
| Fig. 5 Schematic representation of the monomer–excimer formation-based sensing mechanism in ratiometric design. | |
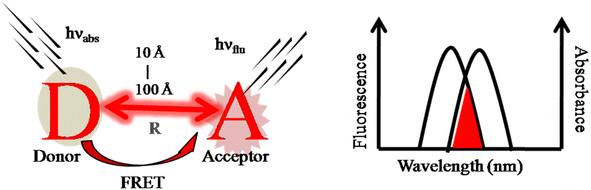 |
| Fig. 6 Schematic representation of the Förster resonance energy transfer (FRET)-based sensing mechanism in ratiometric design. | |
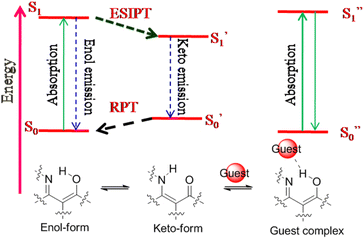 |
| Fig. 7 Schematic representation of the excited state intra-molecular proton transfer (ESIPT)-based sensing mechanism in ratiometric design. | |
4 Mitochondria-targeting ratiometric fluorescent probes
Mitochondria are organelles that play a vital role in cell physiology, including oxidative respiration, ATP production, and signal transduction. Mitochondria are widely known as the main generators of various ROS, RSS and RNS. Thus, probes that can specifically target mitochondria play a key role in monitoring multiple functions of mitochondria and mitochondrion-related illnesses.59–62
4.1 Reactive oxygen species (ROS) detection in mitochondria
The reactive oxygen species H2O2 and HOCl are potent oxidants with antibacterial capabilities.63,64 However, the aberrant synthesis of ROS species in vivo has been linked to several illnesses, including lung damage, atherosclerosis, osteoarthritis, and rheumatoid arthritis.27 Therefore, real-time and on-site detection of ROS is an exciting research topic.
Hu et al. constructed probe 1 with far-red emission by combining a pyrene unit (electron donor, high quantum yield, ability to form a complex in the excited state) with a benzo[e]indolium unit (electron acceptor, extended π-conjugation, mitochondrion-targeting group) linked by an ethylene bridge (Fig. 8, Table 1).65 In EtOH/PBS solution, the free probe exhibited emission at 632 nm (λex = 525 nm). Upon the addition of ClO−, emission at 632 nm gradually fades away with a concomitant increase in fluorescence intensity at 455 nm (blue emission). Probe 1 with excellent mitochondrial targeting features, such as high selectivity (detection limit = 182 nM), fast response time, significant Stokes–Stokes shift (107 nm), photostability, and live cell membrane permeability, displayed potential for detecting ClO− in mitochondria.
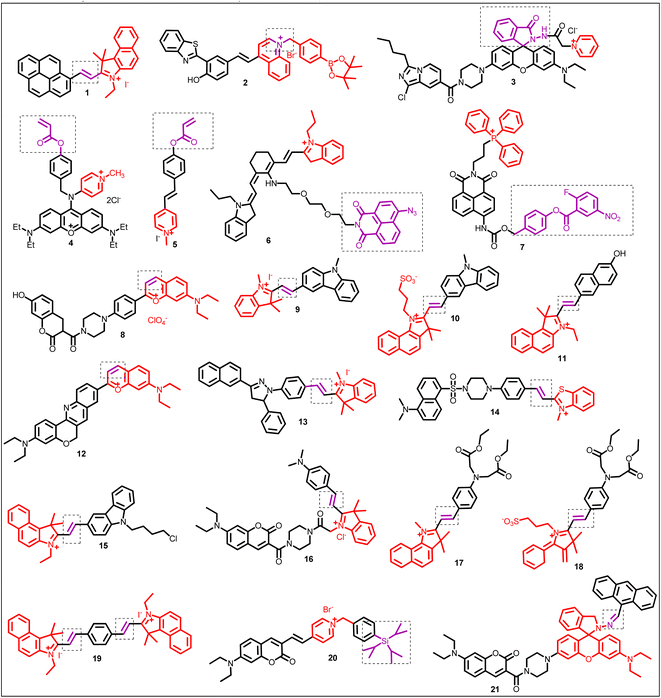 |
| Fig. 8 Chemical structure of mitochondrion-targeting ratiometric fluorescent probes (1–21) (red color: mitochondrion-targeting unit; purple color with dotted box: response site). | |
Table 1 Summary of organelle-targeting ratiometric fluorescent probes
BS |
Organelle |
Targeting moiety |
Analyte |
Λ
ex (nm) |
Λ
em (nm) |
Stokes shift (nm) |
LOD |
Sensing mechanism |
Application |
Ref. |
1 |
Mitochondria |
Benzo[e]indolium group |
ClO− |
525 |
I632/I455 |
107 |
182 nM |
Reaction based and ICT process |
HOCl investigation in biological samples |
65
|
2 |
Mitochondria |
Positively charged probe |
H2O2 |
440 |
I594/I666 |
102–254 |
23.1 nM |
ICT and ESIPT mechanisms |
H2O2 detection in living HeLa cells |
70
|
3 |
Mitochondria |
Quaternized pyridine moiety |
ClO− |
370 |
I575/I467 |
— |
10.2 nM |
FRET process |
Mitochondrial OCl− related studies |
71
|
4 |
Mitochondria |
Positively charged fluorophore |
Cysteine |
514 |
I540/I605 |
90 |
33.7 nM |
Reaction-based |
Quantitatively tracking the Cys distributions in mitochondria |
21
|
5 |
Mitochondria |
Positively charged fluorophore |
Cysteine |
350 |
I518/I452 |
— |
— |
Reaction based |
Monitoring cysteine level in live cells and in living tissue |
76
|
6 |
Mitochondria |
Cationic cyanine moiety |
H2S |
428 and 636 |
I530/I733 |
— |
1.31 μM |
ICT process and reaction based mechanism |
Monitoring mitochondrial H2S in living cells |
24
|
7 |
Mitochondria |
Triphenylphosphonium group |
H2Sn |
405 |
I550/I485 |
109 |
20 nM |
Reaction based |
To study H2S-linked physiological and pathological processes |
81
|
8 |
Mitochondria |
Benzopyrylium structure |
SO32− |
405 |
I455/I635 |
230 |
0.078 μM |
Nucleophilic addition reaction and FRET process |
SO32− imaging in biological systems |
88
|
9 |
Mitochondria |
Indolium group |
HSO3− |
350 |
I490/I590 |
— |
0.15 μM |
Nucleophilic addition reaction |
Monitoring SO2 derivatives in living cells |
89
|
10 |
Mitochondria |
Benzoindole group |
SO2 derivatives |
405 |
I463/I625 |
— |
58 nM |
Nucleophilic addition reaction |
Monitoring SO2 derivatives in living cells |
90
|
11 |
Mitochondria |
Cyanine dyes |
SO32−/HSO3− |
404 |
I467/I593 |
— |
3.6 nM |
1,4-Addition reaction |
Monitoring SO2 derivatives in living cells |
91
|
12 |
Mitochondria |
Positively charged fluorophore |
NaHSO3 |
405 and 580 |
I514/I613 |
— |
103 nM (λex = 580 nm) and 17 nM |
Nucleophilic addition reaction and ICT process |
Detection of SO2 derivatives in mitochondria under one-photon and two-photo absorption |
92
|
(λex = 405 nm), separately |
13 |
Mitochondria |
Hemi-cyanine dyes |
SO2 derivatives |
380 and 558 |
I480/I640 |
— |
80 nM |
Nucleophilic addition reaction and ICT process |
Detection of SO2 derivatives in mitochondria |
93
|
14 |
Mitochondria |
Benzothiazolium moiety |
Bisulfite |
390 |
I540/I590 |
|
69 nM |
Nucleophilic addition reaction and FRET process |
Quantitative detection of HSO3− in mitochondria |
94
|
15 |
Mitochondria |
Lipophilic cationic dye probe |
HSO3− |
322 and 510 |
I462/I588 |
— |
10 nM |
1,4-Nucleophilic addition reaction |
Detection of SO2 derivatives in living cells |
95
|
16 |
Mitochondria |
Indole moiety |
SO32− |
430 |
I476/I589 |
— |
12.85 nM |
Nucleophilic addition reaction, ICT process and FRET effect |
SO32− imaging in living cells |
96
|
17 and 18 |
Mitochondria |
Cyanine dye, 1H-benzo[e]indolium |
HSO3−/SO32− |
840 |
I476/I579 and I468/I586 for probes 17 and 18, respectively |
169 and 166 for probes 17 and 18, respectively |
0.20 μM and 0.11 μM for probes 17 and 18, respectively |
Nucleophilic addition mechanisms, ICT process |
SO32− imaging in living cells |
97
|
19 |
Mitochondria |
Benz[e]indole moiety |
HSO3−/SO32− |
380 |
I480/I600 |
— |
0.1 μM |
Nucleophilic addition mechanisms, ICT process |
SO32− imaging in living cells |
98
|
20 |
Mitochondria |
Pyridinium salt |
F− |
490 |
I539/I639 |
— |
12 nM |
Si–O bond cleavage and ICT process |
F− investigation in biological samples |
100
|
21 |
Mitochondria |
Rhodamine dyes as fluorescent lipophilic cations |
Pd2+ |
400 and 550 |
I594/I472 |
— |
— |
Chelation induced ring opening of rhodamine spirolactam and FRET process |
Ratiometric visualization of Pd2+ in mitochondria |
29
|
22 |
Lysosome |
Pyridine group |
H2O2 |
375 |
I550/I425 |
125 |
12.8 nM |
ICT process |
Lysosomal H2O2 detection |
37
|
23 |
Lysosome |
Morpholine group |
HOCl |
370 |
I589/I462 |
— |
10.2 nM |
PET process, TBET process and HOCl induced ring opening of rhodamine spirocyclic form |
To monitor HOCl changes in lysosome |
15
|
24 |
Lysosome |
Monothio-bishydrazide moiety |
HOCl |
410 |
I580/I480 |
— |
0.66 μM |
FRET process |
HOCl detection in living cells |
14
|
25 |
Lysosome |
Morpholine |
HOCl |
440 |
I610/I535 |
— |
0.58 μM |
ICT process |
Lysosomal HOCl detection in living cells and zebrafish |
109
|
26 |
Lysosome |
Morpholine moiety |
Bisulfite |
480 |
I512/I704 |
— |
0.09 μM |
1,6-Conjugate addition reaction |
Ratiometric fluorescence imaging of lysosomal bisulfite |
114
|
27 |
Lysosome |
Morpholine moiety |
Cysteine |
510 |
I510/I685 |
— |
0.28 μM |
1,6-Conjugate addition |
Fluorescence imaging of the lysosomal cysteine |
117
|
28 |
Lysosome |
Morpholine |
H2S |
350 |
I415/I560 |
— |
0.43 μM |
FRET-based |
H2S detection in lysosome |
118
|
29 |
Lysosome |
Morpholine |
Cu2+ |
440 |
I580/I519 |
|
1.45 nM |
FRET process |
Lysosomal Cu2+ detection in living cells |
123
|
30 |
Lysosome |
Morpholine |
Cu2+ |
360 nm |
I552/I486 |
|
105 nM |
FRET process |
Lysosomal Cu2+ detection in living cells |
125
|
31 |
Lysosome |
Morpholine |
Hg2+ |
480 nm |
535 to 595 nm |
|
0.23 μM |
PET process |
Lysosomal Hg2+ detection in living cells |
127
|
32 |
ER |
— |
H2O2 |
395 |
I540/I465 |
— |
38 nM |
Reaction based mechanism |
H2O2 detection in ER |
131
|
33 |
ER |
Methyl sulfonamide |
HClO |
For λem = 554, λex = 425; for λem = 588, λex = 500 |
I480/I554 |
— |
0.59 μM |
Reaction-based |
HClO detection in the ER of living cells |
39
|
the |
ER |
p-Toluenesulfonamide group |
HClO |
380 |
I484/I533 |
|
0.1 μM |
ICT process and reaction-based |
HClO detection in the ER of living cells |
41
|
35 |
ER |
— |
HOCl |
326 |
I450/I361 |
— |
3.6 μM |
Nucleophilic borono-Dakin oxidation mechanism |
Study of ER signaling and function under oxidative stress |
18
|
36 |
ER |
|
H2S |
480 |
I650/I560 |
(∼150 nm) |
39.1 nM |
Reaction based mechanism |
H2S detection in living cells and zebrafish |
144
|
37 |
ER |
— |
SO2 derivatives |
440 |
I534/I610 |
|
16.2 nM |
FRET process |
Fluorescence imaging of HSO3−/SO32− in living cells |
147
|
38 |
ER |
— |
Cu2+ |
410 |
I545/I480 |
|
1.1 μM and 0.7 μM for Cu2+ and Cu+, respectively |
Copper-promoted hydrolysis |
Imaging copper accumulation in the ER of live cells |
150
|
39 |
ER |
p-Toluenesulfonamide |
pH |
405 |
I446/I527 |
|
Response to pH in the range 5.0–7.2 |
ICT–PET–FRET mechanism |
Quantitative measurement of pH values in endoplasmic reticulum (ER) |
40
|
40 |
ER |
p-Toluenesulfonamide |
Carboxylesterase 2 detection |
380 |
I560/I414 |
— |
— |
Reaction-based |
Carboxylesterase 2 detection in drug-induced acute liver injury |
158
|
41 |
Golgi apparatus |
Phenylsulfonamide group |
H2O2 |
— |
I560/I470 |
— |
0.20 μM |
Reaction-based |
To monitor Golgi oxidative stress and novel drug targets |
162
|
42 |
Golgi apparatus |
Sulfanilamide |
ONOO− |
— |
I650/I477 |
— |
— |
Reaction-based |
To monitor Golgi oxidative stress and to evaluate drug-induced liver injury |
163
|
43 |
Golgi apparatus |
Aminoquinoline derivative |
CO |
360 |
I520/I425 |
— |
41 nM |
Pd0-mediated Tsuji–Trost reaction |
Fluorescence imaging of CO in cells and zebrafish |
164
|
44 |
Golgi apparatus |
4-CF3-7-aminoquinoline |
Glutathione (GSH) |
320 |
I510/I425 |
— |
0.49 μM |
Reaction-based |
GSH detection and organelle-targeted therapy |
165
|
45 |
Nucleolus |
— |
SO2 and formaldehyde |
365 |
I550/I635 |
|
0.17 μM |
FRET process and Michael addition reaction |
Living mice imaging |
52
|
46 |
Nucleus |
— |
NO |
370 |
I530/I424 |
|
20 nM |
Reaction based |
Excellent candidate for use as a green fluorescent nucleus probe or a nucleic acid permanent stain |
168
|
47 |
Nucleus |
N-Methyl benzothiazole cations |
Nucleus viscosity and G-QDNA |
340 |
I650/I407 |
— |
— |
Twisted internal charge transfer (TICT) |
Living cells |
169
|
48 |
Lipid droplets and nucleus |
Coumarin unit for lipid droplet and quinolinium unit for nucleic acid |
Polarity change in the cellular environment |
405 |
I470/I670 |
|
— |
ICT process |
Study of ferroptosis and ferroptosis-linked diseases through fluorescence imaging |
170
|
49 |
Nucleus |
Hoechst unit |
DNA |
345 nm |
I505/I450 |
|
72 nM |
FRET process |
Excellent probe for the monitoring of nucleus DNA damage |
171
|
50 and 51 |
Cytomembrane |
Alkyl side chain of the pyridine salt |
SO2 |
415 |
I644/I486 |
— |
— |
Michael addition |
Bio-application in a mice model |
178
|
52 |
Plasma membrane |
CAZ |
pH |
380 (basic form) and 480 (acidic form) |
496 (basic form) and 566 (acidic form) |
— |
— |
— |
Imaging and measuring vesicular acidification |
182
|
53 |
Mitochondria and lysosome |
Semi-cyanine unit for targeting mitochondria and morpholine group for targeting lysosome |
SO2 |
400 |
I530/I600 |
|
0.82 μM |
FRET process |
Simultaneous detection of endogenous SO2 in lysosome and mitochondria by one and two-photon modes. |
183
|
54 |
Mitochondria and lipid droplets (LDs) |
Hemi-cyanine to target mitochondria and neutral form of probe 54 to target LDs |
pH |
380 |
I450/I580 |
— |
— |
Reaction-based and ICT process |
Furthermore, probe 54 was successfully applied for monitoring the pH fluctuation in living cells under different exotic chemicals |
19
|
An important ROS, endogenous H2O2, functions as a signaling molecule to control various cellular processes, such as cell division, proliferation, and migration.66,67 However, high H2O2 concentrations can harm proteins and nucleic acids, which are strongly related to many disorders such as malignancies, diabetes, and Alzheimer's disease.68,69 Therefore, monitoring of H2O2 concentration is essential. He et al. designed benzothiazole dye (adequate stability, large Stokes-shift, large quantum yield, tunable emission) based probe 2 containing an aromatic boronic ester moiety (H2O2 recognition group) (Fig. 8, Table 1).70 The fluorescence titration of probe 2 with H2O2 revealed an increase in new emission maxima at 594 nm at the expense of emission at 666 nm. The limit of detection was 23.1 nM. The sensing mechanism was the H2O2 triggered aromatic boronic ester moiety removal. Furthermore, probe 2, with excellent mitochondrial targeting properties, such as significant Stokes shift (152 nm), photostability, and Pearson's colonization coefficient (0.94), displayed potential for detecting H2O2 in mitochondria.
Shen et al. designed probe 3 based on the FRET platform for detecting OCl− (Fig. 8, Table 1).71 In the presence of OCl−, probe 3 displayed ratiometric fluorescence change, and the plot of intensity ratio (I575/I467) with the concentration of OCl− was linear in the range of 0 to 5 μM. The limit of detection was 10.2 nM. From the fluorescence imaging experiment, the applied probe 3 successfully examined endogenous OCl− in Murine RAW 264.7 cells.
4.2 Reactive sulfur species (RSS) detection in mitochondria
Reactive sulfur species (RSS), such as cysteine (Cys), hydrogen sulfide (H2S), and hydrogen polysulfides (H2Sn), are produced in large quantities in mitochondria and are associated with critical mitochondrial-related pathological and physiological processes.7 Cys can act as an antioxidant in mitochondria by removing various ROS from mitochondria to stop oxidative damage.72 Additionally, Cys is necessary for the mitochondrial process of protein turnover.73–75 Therefore, it is essential to identify and measure Cys in real-time within cells, particularly in the mitochondria, to understand the pathological and physiological processes properly.
Yang et al. designed xanthylene-based fluorescent probe 4 for the ratiometric detection of cysteine levels in the mitochondria (Fig. 8, Table 1).21 The probe 4 containing an acryloyl moiety (responsive site for cysteine) and a benzyl group (for easy distribution in the mitochondria) showed strong red fluorescence at λem = 605 nm (λex = 490 nm). In the presence of cysteine, probe 4 underwent ratiometric fluorescence change with the formation of new emission maxima at 540 nm and the simultaneous decrease in emission intensity at 605 nm. The limit of detection was 33.7 nM for cysteine. Applied probe 4 examined endogenous cysteine levels in HeLa cells through bioimaging, demonstrating potential applications in natural areas.
Considering the advantage of two-photon fluorescence microscopy and ratiometric detection, Niu et al. developed probe 5 for detecting cysteine over other biothiols (Fig. 8, Table 1).76 In the presence of cysteine, the fluorescence spectrum of probe 5 exhibited ratiometric change (I518/I452), linear in the range between 0.5 and 40 μM. Interestingly, the merocyanine fluorophore and probe 5 exhibited a large two-photon cross-section (Φσmax) of 72.6 GM (λex = 760 nm) and 65.2 GM (λex = 740 nm), respectively, favorable in producing bright and high contrast images of living samples. In addition to detecting cysteine in live cells and mitochondria, probe 5 exhibited promising application for monitoring cysteine concentration in living tissues (down to 150 μm depth) using two-photon fluorescence microscopy.
H2S plays essential roles in mitochondria, such as scavengers for reactive oxygen species,77 and is associated with various mitochondrial-related pathological and physiological processes.78,79 Therefore, monitoring of H2S level in mitochondria is crucial. Liu et al. proposed probe 6 integrated with cyanine (mitochondria-targeting group) and naphthalimide group (responsive to H2S) for ratiometric detection of H2S in mitochondria (Fig. 8, Table 1).24 In the CTAB solution, upon the addition of Na2S, probe 6 displayed ratiometric fluorescence response, and the intensity ratio (I530/I733) showed good linearity in the range of 1–9 μM. The detection limit was 1.31 μM for Na2S. In the presence of Na2S, reduction of the azide group to the amino group (electron donating) occurs, activating the ICT process and turn-on fluorescence of the naphthalimide moiety. Probe 6 found applications for fluorescence imaging and ratiometric detection of H2S in live cells.
Han et al. engineered probe 7 integrated with triphenylphosphonium (mitochondria-targeting group), 2-fluoro-5-nitrobenzoic unit, and 1,8-naphthalimide fluorophore (well-known ICT fluorophore)80 for the detection of H2Sn (Fig. 8, Table 1).81 In the phosphate-buffered saline (PBS) solution, fluorescence titration of probe 7 with Na2S2 revealed a decrease in emission intensity at 485 nm. A new emission maximum at 550 nm emerged and increased (Stokes-shift = 109 nm). Probe 7 successfully demonstrated its application in imaging intracellular H2Sn with good selectivity and sensitivity.
SO2 is an essential endogenous signaling molecule that performs significant roles in many physiological processes. However, increased SO2 concentration is associated with severe lung cancer, nervous system diseases, and respiratory problems.82–85 Therefore, detecting SO2 and its derivatives in living systems becomes a high priority. Notably, a platform with fluorophores attached to aromatic heterocycles through C–C bonds was frequently used as a Michael addition receptor. The Michael-addition principle allows nucleophiles, such as bisulfite, to attack the C–C double bond.86,87 In this section, most probes for bisulfite detection follow the nucleophilic addition reaction mechanism.
Taking advantage of the merits of FRET-based systems such as a large Stokes-shift, Huang et al. developed near-infrared (NIR) fluorescent probe 8 by combining a coumarin carboxylic acid group with a piperazine substituted benzopyrylium salt (mitochondrion-targeting group) (Fig. 8, Table 1).88 In PBS solution, the fluorescence spectrum of probe 8 exhibited emission maxima at 635 nm and a large Stokes-shift (230 nm). The nucleophilic addition reaction of SO32− at the double bond of the benzopyrylium unit resulted in the interruption of the conjugated π-electron cloud, and the fluorescence at 635 nm decreased, accompanied by the simultaneous increase in the new emission peak at 455 nm. From the co-localization experiment, probe 8 efficiently targeted mitochondria (Pearson's co-localization coefficient = 0.84), and further investigations in HeLa cells and the nude mice experiment showed the application of probe 8 in biological systems for detecting SO32− (Fig. 9).
 |
| Fig. 9
In vivo photos of nude mice (A) 8 (20 μM); (B–E) 8 (20 μM) + different concentrations of SO32− (5–20 μM). λex = 530 nm, λem = 600–700 nm. Reprinted from ref. 88, copyright 2021 Elsevier. | |
Liu et al. exploited probe 9, prepared by the condensation reaction between indolium or pyridinium and 3-formyl-9-methyl carbazole unit (Fig. 8).89 In the presence of HSO3−, probe 9 displayed ratiometric fluorescence change and the ratio of emission intensity (I490/I590) varied from 0.0383 to 3.8769 (101-fold enhancement). The in vitro imaging experiment proved the application of probe 9 for the quantification of SO2 derivatives in the mitochondria.
The same group presented another probe, probe 10, containing a carbazole and an alkyl sulfonated benzoindole (water soluble, mitochondria-targeting group) as the basic skeleton (Fig. 8, Table 1).90 In the presence of HSO3−, the emission spectrum of probe 10 revealed a blue shift of 162 nm, and the emission intensity ratio (I463/I625) was 56 (Fig. 10). The change in proton signals from 8.03–8.78 to 5.15 after the addition of HSO3− in the 1H NMR spectrum, together with NOESY and COSY results, proved a 1,4-addition reaction-based detection mechanism. Unlike other reported probes for mitochondrial SO2 detection, probe 10 could monitor the mitochondrial SO2 level variation stimulated by carbonyl cyanide m-chlorophenyl hydrazone (CCCP) or by drugs.
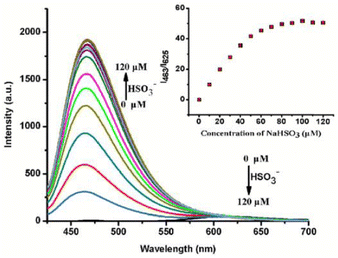 |
| Fig. 10 Fluorescence titration spectrum of probe 10 with HSO3−. Inset: plot of intensity ratio (I463/I625) vs. [HSO3−]. Reprinted from ref. 90, copyright 2016 Royal Society of Chemistry. | |
Based on the 1,4-addition reaction between the polymethine chain of hemicyanine and SO32− or HSO3−, Wang et al. developed probe 11 to detect SO2 derivatives in aqueous medium and the mitochondria of living cells (Fig. 8, Table 1).91 Upon the addition of HSO3−, the fluorescence spectrum of probe 11 showed ratiometric fluorescence change and the plot of their ratio (I467/I593) vs. the concentration of HSO3− was linear in the range of 1–9 μM. The sensing mechanism could be attributed to the interruption of the π-conjugation of probe 11 upon the addition of HSO3− at the responsive site of probe 11, leading to a ratiometric change in the absorption and fluorescence spectrum. The fluorescence co-localization experiment revealed the unique distribution of probe 11 within the mitochondria of live MCF-cells (Pearson's correlation coefficients = 0.931, and Mander's overlap = 0.872). Furthermore, the applied probe 11 examined mitochondrial SO2 derivatives quantitatively through a fluorescence imaging experiment.
Wang et al. applied the strategy of combining two classical dyes to construct long-wavelength probe 12, prepared from benzopyrylium and chromenoquinoline dyes (Fig. 8, Table 1).92 The emission spectrum of the two-photon fluorescent probe showed a gradual decrease in red fluorescence at 613 nm (λex = 580 nm) and an increase in blue fluorescence at 514 nm (λex = 405 nm) upon the addition of HSO3−. The detection limit was 103 nM and 17 nM for the red and green channels, respectively. The nucleophilic addition reaction of HSO3− at the C
C bond of probe 12 resulted in the interruption of π-conjugation/inhibition of the ICT process from the chromenoquinoline to the benzopyrylium group. Thus, a significant blue shift (99 nm) in the emission spectrum was observed. Furthermore, probe 12 displayed application for detecting SO2 derivatives in the solid state.
Wang et al. developed probe 13 based on pyrazoline (high quantum yield, cell permeability, and low cytotoxicity) and hemicyanine dyes (water soluble, mitochondrion-targeting group) (Fig. 8, Table 1).93 The fluorescence titration of probe 13 with SO32− revealed ratiometric fluorescence change, and the intensity ratio (I480/I640) changed from 0.45 to 445 (989 times). Intriguingly, probe 13 demonstrated an application for the ratiometric imaging of mitochondrial SO2 derivatives in living cells.
Keeping in mind Michael's addition principle and the FRET process, Wu et al. designed probe 14 based on the conjugated platform of dansyl, piperazine, and benzothiazolium, in which the benzothiazole moiety acts as a recognition unit and mitochondrion-targeting group (Fig. 8, Table 1).94 Upon the incremental addition of HSO3−, the fluorescence intensity ratio (I540/I590) of probe 14 showed a change from 0.3 to 1.5 (5-fold), and the limit of detection was 69 nM. Furthermore, probe 14 demonstrated a successful application for detecting HSO3− in the mitochondria of living cells through fluorescence imaging (Fig. 11).
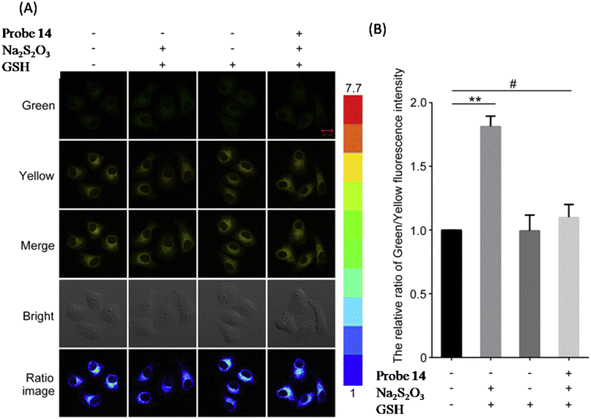 |
| Fig. 11 (A) Confocal microscopic images of HepG2 cells demonstrating the application of probe 14 for HSO3− detection. (B) The relative intensity ratio (yellow/green) of columns 1, 2, 3 and 4 in (A). Reprinted from ref. 94, copyright 2017 Elsevier. | |
α,β-Unsaturated compounds are prone to a nucleophilic addition reaction by HSO3−. With this in mind, Xu et al. prepared probe 15 by the condensation of 1H-benzo[e]indolium (water soluble, high quantum yield, significant Stokes shift (>100 nm), and mitochondrion-targeting group) with carbazole-3-aldehyde (Fig. 8, Table 1).95 Upon the addition of HSO3−, the fluorescence spectrum of probe 15 underwent ratiometric fluorescence change with a decrease in the emission band at 588 nm and an increase in the new emission band at 462 nm, simultaneously. Interestingly, the cell staining experiment revealed probe 15 as cell-permeable and mitochondria-targetable, and it can monitor the intracellular SO2 derivatives in live cells (HeLa cells).
Zhang et al. constructed probe 16 composed of the coumarin-hemicyanine skeleton, in which the FRET process occurs from coumarin to hemicyanine and the ICT process occurs from the styryl to the indolium group (mitochondrion-targeting) (Fig. 8, Table 1).96 The nucleophilic approach of SO32− towards the double bond of hemicyanine resulted in disruption of π-conjugation and interruption in the ICT/FRET process. This dual emission signal of probe 16 changed in a see-saw manner with a significant emission shift (113 nm). The staining experiment revealed probe 16 as a specific mitochondrion-targeting probe and can detect endogenous SO32− in living cells such as HepG2 and L02 cells.
Zhao et al. developed probes 17 and 18 by using diethyl 2,2′-(phenylazanediyl)diacetate (electron-donor) and 1H-benzo[e]indolium (water soluble, high quantum yields, NIR emission property, and mitochondrion-targeting group) (Fig. 8, Table 1).97 In the presence of HSO3−/SO32−, probe 17 and 18 displayed ratiometric fluorescence change, blue-shift in emission (over 100 nm), and high selectivity and sensitivity (LOD = 0.20 μM and 0.11 μM for probes 17 and 18, respectively). The sensing mechanism for both probes was a nucleophilic addition reaction. However, the path of the sensing mechanism was different. Interestingly, both the probes displayed the capability of quantifiable tracking and fluorescence imaging of HSO3−/SO32− in the mitochondria of living cells.
Zheng et al. constructed probe 19 by the condensation reaction between trimethylbenzoindolium (mitochondrion-targeting moiety) and p-diphthalaldehyde (Fig. 8, Table 1).98 The fluorescence response of probe 19 toward HSO3− was initially ratiometric (at low concentrations) and then turn-on emission (at high concentrations). The sensing mechanism was a twice nucleophilic addition reaction. The cell imaging revealed that the probe 19 could specifically detect HSO3− in the mitochondria of living cells (HepG2 cells).
4.3 Fluoride detection in mitochondria
The fluoride ion is one of the most significant anions crucial to many biological and medicinal processes. According to literature reports, a high fluoride ion concentration can damage mitochondria through oxidative stress and reduce the mitochondrial respiratory chain's efficiency, resulting in mitochondrial malfunction and the development of neurodegenerative disorders.99 As a result, it is essential and beneficial to monitor fluoride ions in mitochondria.
Shen et al. applied ICT-modulated strategies for constructing probe 20 based on a diethylaminocoumarin derivative (a well-known ICT fluorophore) in which a pyridinium salt behaves as a mitochondrion-targeting group (Fig. 8, Table 1).100 Initially, probe 20 exhibited red fluorescence at 639 nm (λex = 490 nm) attributed to the ICT process between the pyridinium cation and 7-diethylamino-coumarin unit. Upon the addition of F−, a new emission band at 539 nm emerged and increased at the expense of a decrease in emission at 639 nm. The sensing mechanism was F−-induced cleavage of the Si–O bond between the phenyl and triisopropylsilyl groups. Furthermore, the applied probe 20 successfully examined mitochondrial F− in living cells.
4.4 Palladium ion (Pd2+) detection in mitochondria
Due to its high stability and reliability characteristics, palladium has been extensively employed in various applications, including catalysis, synthesis of organic compounds, medicine, dental crowns, fuel cells, and electronics production. However, palladium ion enrichment in living organisms can cause severe health-related diseases.101,102 Therefore, developing an efficient tool for palladium ion detection in a biological system is necessary.
Wang et al. developed probe 21 to detect Pd2+ in living cells based on the FRET process and rhodamine ring-opening mechanisms (Fig. 8, Table 1).29 In PBS buffer solution, free probe 21 exhibited emission maxima at 472 nm (λex = 400 nm). Upon adding Pb2+, a decrease in emission at 472 nm and an increase in the new emission band at 594 nm were observed. Moreover, the fluorescence imaging experiment revealed the application of probe 21 for the ratiometric visualization of Pd2+ in the mitochondria of living cells.
4.5 Challenges
Most organelle-targeting methods discussed above focus on lipophilic cationic fluorescent probes that selectively target mitochondria. However, problems with cationic probes, such as the effect on membrane potential and cellular toxicity, are yet to be overcome. Furthermore, to explain the probe's potential to target mitochondria, mostly co-localization experiments have been performed using commercially available mitochondria-specific dyes such as MitoTracker Green FM, MitoTracker Red, and MitoTracker Orange. However, there can be several other contributing factors and principles behind the probe's potential to target mitochondria, and these factors need a clear discussion.
In addition, mitochondria contain hundreds of biomolecules, such as anions, cations, enzymes, mitochondrial DNA, RNA, lipids, and so forth. Using fluorescent probes, it is still challenging to selectively label bioactive compounds at low concentrations (often nanomolar levels). No doubt, ratiometric fluorescent probes hold promise for removing various background interferences. However, nonetheless, just a few mitochondrion-targeting ratiometric fluorescent probes have been developed to date.
5 Lysosome-targeting ratiometric fluorescent probes
Lysosomes, an essential subcellular organelle, which can function as a digestive compartment in eukaryotic cells and include a variety of enzymes and proteins, are critical regulators in metabolic processes under acidic pH circumstances.103 As key indicators of lysosome function and oxidative stress, reactive oxygen species (ROS) such as HClO and H2O2 and reactive sulphur species (RSS) such as H2S have been the focus of numerous probe designs. Since the lysosome lumen is acidic, developing probes that can detect HOCl in an acidic medium is challenging.4,30
5.1 Reactive oxygen species (ROS) detection in the lysosome
H2O2 is a significant reactive oxygen species (ROS) with distinct destructive oxidation characteristics. Lysosomes can produce hydrogen peroxide (H2O2) to combat pathogens.104–106 Therefore, it's crucial to create a reliable method for measuring H2O2 in inflammatory tissues to assess the physiological and pathological link between lysosomal H2O2 and inflammation.
Inspired by the excellent optical properties of naphthalimide derivatives (donor–π-acceptor structured), Zhou et al. constructed probe 22 based on naphthalimide, benzylboric acid (H2O2 responsive group), and pyridine group (lysosome-targeting group) for monitoring H2O2 in living tissue and in inflamed tissue (Fig. 12, Table 1).37 In the presence of H2O2, probe 22 displayed ratiometric fluorescence change, assigned to H2O2 mediated removal of the boric acid group from probe 22 and ICT effect. The fluorescence color of the solution changed from bright blue to light yellow. Furthermore, tissue imaging experiments using a confocal microscope demonstrated a potential application for H2O2 detection in inflamed tissues.
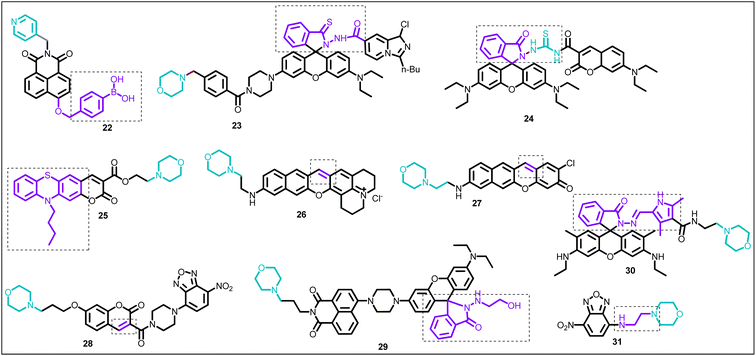 |
| Fig. 12 Chemical structure of lysosome-targeting ratiometric fluorescent probes (22–31), (brown color: lysosome-targeting unit; purple color with dotted box: response site). | |
TBET-based probes offer several advantages, such as high energy transfer efficiencies, improved imaging resolution, and a large Stokes-shift.107,108 With this in mind, Shen et al. synthesized probe 23 based on the imidazo[1,5-a]pyridine moiety (donor) and rhodamine moiety (acceptor) (Fig. 12, Table 1).15 The free probe 23 exhibited emission at 462 nm attributed to the emission of the imidazo[1,5-a]pyridine fluorophore. Upon the gradual addition of aliquots of HOCl, the emission intensity at 462 nm almost remained constant, while a new emission maximum at 589 nm emerged and increased, assigned to the rhodamine moiety. The sensing mechanism was the change of the rhodamine spiro form to the ring-open state in the presence of HOCl and the TBET process between the rhodamine unit and imidazo[1,5-a]pyridine. Applied probe 23 successfully monitored the HOCl changes in the lysosomes.
Using a similar mechanism to the one described above, which converts the rhodamine spiro-form into the ring-open form when HOCl is present, Yuan et al. provided the coumarin and rhodamine based FRET platform 24 for HOCl detection in living cells (Fig. 12, Table 1).14 Upon excitation at 410 nm, probe 24 displayed emission at 480 nm (which belonged to the coumarin moiety). However, in the presence of HOCl, the emission spectrum of probe 24 showed a decrease in intensity at 480 nm and an increase in new maxima at 580 nm (which belonged to rhodamine), assigned to a ring-opening and FRET (FRET efficiency = 93.75%) based detecting mechanism.
Liu et al. constructed probe 25 from phenothiazine coumarin and a morpholine unit for hypochlorite detection (Fig. 12, Table 1).109 The fluorescence titration of probe 25 with ClO− showed a blue shift in emission from 610 to 535 nm. The sensing mechanism was inhibition of the ICT process due to the oxidation of the phenothiazine moiety. Furthermore, the fluorescence imaging experiment demonstrated the application of probe 25 for detecting ClO− in living cells (RAW264.7 cells) and zebrafish (Fig. 13).
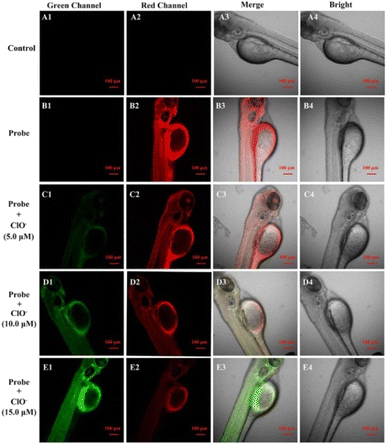 |
| Fig. 13 Fluorescence images of zebrafish. The control group (A1–A4). Zebrafish co-cultured with probe 25 (10 μM) (B1–B4), followed by incubation with ClO− 5 μM (C1–C4), 10 μM ClO− (D1–D4) and 15 μM ClO− (E1–E4). Reprinted from ref. 109, copyright 2021 Elsevier. | |
5.2 Reactive sulfur species (RSS) detection in lysosome
The endogenous oxidation of hydrogen sulfide or sulfur-containing amino acids produces bisulfite, which remains in equilibrium with sulfur dioxide and sulfite in aqueous media.110–113 As a result, the discovery of bisulfite in lysosomes is of great interest.
Tamima et al. pioneered probe 26 by introducing a morpholine moiety (targeting group) to the benzopyronin dye (Fig. 12, Table 1).114 After adding bisulfite, the absorption and fluorescence spectrum of probe 26 displayed complete peak separation from 613 nm to 426 nm (spectral shift = 187 nm) and from 704 nm to 512 nm (spectral shift = 192 nm), respectively. The sensing mechanism was the 1,6-conjugate addition reaction. Probe 26 specifically targeted lysosomes and displayed application for monitoring intracellular bisulfite levels.
Cysteine, homocysteine, hydrogen sulfide, and glutathione are examples of cellular thiols frequently studied employing lysosome-targeting probes.115 These biothiols are significant indications of lysosomal function because they are produced by lysosomal proteolysis.116
Tamima et al. devoted benzo[b]xanthene-derived probe 27 for the two-photon ratiometric fluorescence-based detection of cysteine (Fig. 12, Table 1).117 The detecting mechanism of cysteine was a 1,6-conjugate addition reaction to the benzoxanthene core, resulting in the formation of a cysteine adduct that emitted red. However, in the presence of hydrogen peroxide, the 1-cysteine adduct was reverted to 27. Furthermore, probe 27 demonstrated practical application to quantify cysteine levels in biological samples (human blood plasma).
Zhang et al. applied a cleavable FRET-based strategy for designing a lysosome-targeting probe 28, based on a coumarin–NBD (nitrobenzofurazan) cassette (Fig. 12, Table 1).118 Free probe 28 showed two emission maxima at 415 nm and 560 nm, belonging to the coumarin and NBD groups. Upon treatment with H2S, probe 28 underwent ratiometric fluorescence change, the solution's emission color changed from yellow to blue, and the intensity ratio increased from 0.22 to 67.7 (300-fold approx.). Furthermore, probe 28 preferentially targeted lysosomes and displayed potential application for detecting H2S in lysosome.
5.3 Cu2+ detection in the lysosome
Many essential physiological processes involve the role of copper. Copper plays important roles in a variety of fundamental physiological processes.119 At the organelle level, improper copper homeostasis can cause several severe illnesses.120–122 As a result, it is still essential to monitor the concentration of copper levels in cells, particularly in lysosomes.
Liu et al. applied FRET-based strategies for constructing ratiometric probe 29 (Fig. 12, Table 1).123 The probe 29 displayed high selectivity, sensitivity (detection limit = 1.45 nM), and ratiometric fluorescence response towards Cu2+. From fluorescence imaging experiments, live cells (L929 cells) incubated with probe 29 showed blue and weak green fluorescence. However, cells pre-treated with Cu2+ and then incubated with probe 29 showed solid green and diminished blue fluorescence. In addition, probe 29 was applied for fluorescence imaging of Cu2+ in the lysosome of living cells.
Inspired by Czarnik's report on Cu2+-induced ring opening of rhodamine,124 Wu et al. synthesized probe 30 for Cu2+ detection (Fig. 12, Table 1).125 In CH3CN–H2O (8
:
2) solution, probe 30 behaved as a selective and sensitive probe for Cu2+ over other tested ions. The sensing mechanism was Cu2+-induced ring opening and the FRET process from pyrrole to rhodamine.
5.4 Hg2+ detection in the lysosome
Mercury is a hazardous and pervasive heavy metal that can result in numerous serious health issues, including renal failure, damage to the central nervous system, etc.126 Therefore, it is crucial to have an effective method for detecting mercury ions.
Zhang et al. developed an NBD-based probe 31 by introducing a morpholine moiety to the NBD fluorophore for Hg2+ detection (Fig. 12, Table 1).127 In the presence of Hg2+, the absorption spectrum of probe 31 showed a red shift of the peak from 470 nm to 494 nm, and the color detected by the naked eye changed from light yellow to red. Under similar conditions, the fluorescence spectrum also revealed a red shift of emission maxima from 535 nm to 595 nm. Herein, the morpholine unit played dual roles as a ligand for Hg2+ and lysosome-targeting group. The sensing mechanism was assigned to the inhibition of the PET process from the nitrogen atom of morpholine to the NBD fluorophore upon coordination with Hg2+.
5.5 Challenges
Lysosome-based research has advanced significantly in recent years. Nevertheless, there are still several challenges for these probes. The synthetic probe cannot differentiate between autolysosomes, autophagosomes, endosomes, and other acidic compartments. These probes are harmful to live cells and inappropriate for long-term detection because they make lysosome alkaline. As a result, lysosome-specific probes devoid of the alkalization effect are necessary. Most of the developed probes have emissions in the visible region, thus, cannot be used for deep-tissue imaging due to the poor penetration power.
6 Endoplasmic reticulum-targeting ratiometric fluorescent probes
The most prominent organelle in a cell, the endoplasmic reticulum (ER), is crucial for protein synthesis, folding, distribution, and calcium ion storage. Literature reports revealed that ER stress, which is linked to significant diseases, can cause autophagy and even cell death. Therefore, studying ER is an exciting field of research.128,129
6.1 Reactive oxygen species (ROS) detection in endoplasmic reticulum
H2O2, an important reactive oxygen species, plays a crucial role in various pathological and physiological processes. During ER stress, the increased concentration of H2O2 can damage cellular proteins and may result in cancer, metabolic diseases, cardiovascular diseases, neurodegenerative diseases, etc.130,131 Therefore, developing efficient analytical methods for H2O2 in ER is crucial.
In the past, researchers developed various probes for detecting exogenous H2O2 in ER. However, quantitative detection of endogenous H2O2 remains challenging. To solve this issue, Gao et al. synthesized probe 32 based on α-ketoamide and naphthalimide groups (Fig. 14, Table 1).131 Upon adding H2O2, the fluorescence spectrum of probe 32 revealed ratiometric fluorescence change, attributed to the reaction between H2O2 and α-ketoamide and subsequent hydrolysis of amido linkage. The limit of detection was 38 nM for H2O2. Furthermore, applied probe 32 found application for quantitative measurement of endogenous H2O2 in ER of living cells (HeLa cells), both under normal conditions (0.692 μM H2O2) and under ER stress (1.26 μM H2O2).
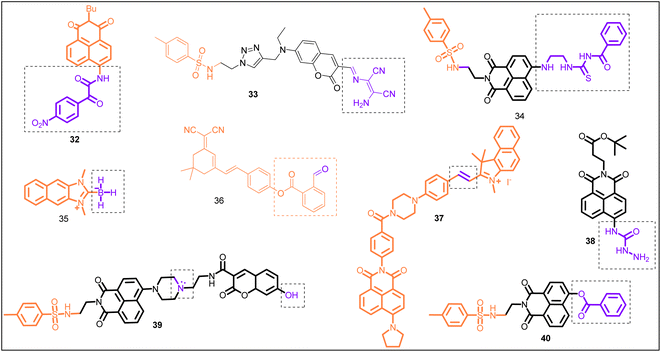 |
| Fig. 14 Chemical structure of endoplasmic reticulum-targeting ratiometric fluorescent probe (32–40), (orange color: ER-targeting unit; purple color with dotted box: response site). | |
In the past, the diaminomalenonitrile-based Schiff base has been used by several researchers for developing ClO− specific sensors.132–134 On the other hand, the sulfonamide group has been known to target ER.135 Taken together, Hou et al. constructed biosensor 33 for ClO− detection in ER (Fig. 14, Table 1).39 Upon the addition of ClO−, the fluorescence spectrum of biosensor 33 displayed a blue shift in emission wavelength from 554 nm to 480 nm, attributed to the reaction-based sensing mechanism. The plot of emission intensity ratio (I480/I554) vs. ClO− concentration was linear between 0 and 120 μM concentrations. The detection limit was 0.59 μM for ClO−. The applied biosensor 33 examined exo/endogenous ClO− in the ER of living cells.
Inspired by the excellent optical properties and ICT process of 4-aminonaphthalimide moiety,136 Ma et al. constructed biosensor 34 using a 4-aminonaphthalimide moiety (fluorescent group), (2-aminoethyl) thiourea unit (HClO recognition site), and p-toluenesulfonamide unit (ER-targeting group) (Fig. 14, Table 1).41 In ethanol–H2O (1
:
1, v/v) solution, free sensor 34 showed emission at 533 nm (green emission), attributed to the ICT process. After adding HOCl, biosensor 34 revealed ratiometric fluorescence change with blue-shift (49 nm) of the emission maxima from 533 nm to 484 nm, which was assigned to inhibition of the ICT process. Furthermore, biosensor 34 demonstrated an application for HOCl detection in the ER of PC-12 cells.
In the past, aryl boronic acid has been widely utilized in designing fluorescent sensors for biological species,137 such as peroxynitrite (ONOO−),138 hypochlorite (OCl−)139 and hydrogen peroxide.140,141 Pak et al. designed NHC-borane-based fluorescent sensor 35, constructed from a naphthoimidazolium precursor (HOCl responsive unit) for specific detection of HOCl over other ROS (Fig. 14, Table 1).18 Sensor 35 followed an electrophilic oxidation mechanism that involved B–H bond cleavage. Sensor 35 successfully targeted ER and demonstrated an application for monitoring HOCl in living cells (Raw 264.7 cells) and rat hippocampal slices by a two-photon fluorescence microscopy experiment.
6.2 Reactive sulfur species (RSS) detection in endoplasmic reticulum
Taking into account the essential role of H2S during ER stress and ER functions,142,143 Shu et al. pioneered ratiometric NIR-fluorescent biosensor 36 based on dicyano-isophorone (NIR emission, large Stokes-shift) and an O-carboxybenzaldehyde unit (H2S recognition site) for H2S detection in ER (Fig. 14, Table 1).144 Sensor 36 showed fluorescence emission at 560 nm due to the ICT effect. Upon the gradual addition of H2S, a new emission peak at 650 nm appeared and increased with the simultaneous decrease in λem at 560 nm. The sensing mechanism was a nucleophilic addition reaction. Furthermore, sensor 36 demonstrated an application for monitoring H2S in living cells (HeLa cells) and zebrafish. Interestingly, sensor 36 successfully detected endogenous H2S produced during ER stress incited by Tunicamycin.
Nowadays, physiological functions of SO2 and its derivatives are gaining increasing attention. According to literature reports, SO2 is vital in cardiovascular processes145 and can regulate hippocampal neuron apoptosis.146 Thus, SO2 detection is essential. Li et al. developed a FRET-based platform 37, constructed from benzoindole-based hemicyanine (acceptor) and naphthalimide derivatives (donor), for the detection of SO2 derivatives in ER (Fig. 14, Table 1).147 Sensor 37 exhibited emission maxima in buffer solution at 610 nm (λex = 440 nm), attributed to the FRET process. The sensing mechanism was a nucleophilic addition reaction and inhibition of the FRET process. Furthermore, sensor 37 successfully targeted ER and demonstrated an application for imaging exogenous and endogenous SO2 derivatives in living cells.
6.3 Cu2+ detection in endoplasmic reticulum
Mitochondria and lysosomes implicate the cellular homeostasis of copper.148 Furthermore, lysosomes in damaged tissue contained high concentrations of copper ions.149 However, it is unclear whether or not the copper ions in these organelles are the cause of the ER's harmful actions. Thus, to understand copper-related diseases, a reliable technique for imaging copper at the level of organelles is crucial.
Park et al. presented a naphthalimide and hydrazide based biosensor 38 for copper ion detection in living cells (Fig. 14, Table 1).150 Upon the addition of copper ion to the solution of sensor 38 in HEPES buffer, the emission maxima were red-shifted (380 nm to 440 nm), and solution color changed from blue to yellowish-green. The detecting mechanism was assigned to the copper-mediated hydrolytic reaction of sensor 38, forming aminonaphthalimide. Additionally, the biocompatible sensor 38 specifically targeted the ER of living cells and displayed potential for qualitative and quantitative detection of Cu+/Cu2+ under physiological conditions.
6.4 Monitoring pH in endoplasmic reticulum
The physiological functions of the ER, such as targeting during secretion, protein sorting, and retrieving resident chaperones, are regulated by the pH of the ER, which serves as a crucial parameter.151 The ER pH is the same as that of the cytoplasm under normal physiological conditions.152 Recent research has shown that ER stress potently stimulates autophagy, strongly linked to many diseases (such as cancer, infectious disorders, and neurodegeneration), causing the ER pH to drop.153,154 Therefore, it is crucial to quantitatively evaluate the pH change in ER to explain the biological functions of ER fully.
Fluorescent probes with dual responsive sites have shown improved sensitivity to pH and enhanced emission wavelength.155 With this in mind, Dong et al. pioneered probe 39 based on the naphthalimide–coumarin platform and employed hydroxyl and morpholine groups as the pH-responsive sites (Fig. 14, Table 1).40 On excitation at 405 nm, the fluorescence spectrum of probe 39 showed ratiometric change with a single emission band at 527 nm (acidic pH, 4.09–5.08) and two emission maxima at 446 nm and 527 nm between pH 5.08 and 7.73, and further single emission maxima at 446 nm at pH 7.73 (basic pH). The sensing mechanism was assigned to the FRET–PET–ICT process (Fig. 15). Furthermore, due to the presence of the p-toluenesulfonamide group (ER-targeting group), probe 39 successfully targeted ER. It demonstrated an application for quantitatively detecting the pH changes in the dexamethanose-treated cell and during ER stress.
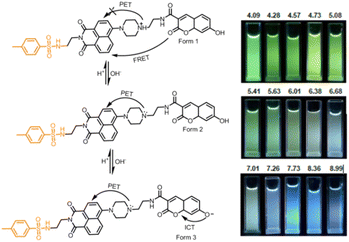 |
| Fig. 15 (A) The sensing mechanism of probe 39 to pH. (B) Fluorescence images of probe 39 at different pH under illumination at 365 nm. Reprinted from ref. 40, copyright 2019 Royal Society of Chemistry. | |
6.5 Carboxylesterase 2 (CES2) detection in endoplasmic reticulum
Carboxylesterase 2 (CES2) has critical roles in ER, such as metabolic, drug detoxification, molecular target for the prodrug design, and ER stress-associated diseases.156,157 Tian et al. developed ratiometric fluorescent probe 40 based on p-toluenesulfonamide (ER-targeting group) for CES2 detection (Fig. 14, Table 1).158 Among various hydrolases, only CES2 catalyzed the hydrolysis of probe 40. Probe 40 was applied for imaging CES2 in living cells (HepG2 cells) and tumor tissue (Fig. 16). Notably, Probe 40 revealed a significant decrease in CES2 activity under ER stress and drug (acetaminophen (APAP))-induced liver injury model.
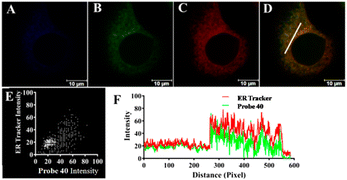 |
| Fig. 16 Fluorescence images of probe 40 in HepG2 at the (A) blue channel (425–475 nm); (B) at the green channel (535–585 nm); (C) ER-Tracker (red); (D) merged image (green channel and ER-Tracker channel (red)); (E) intensity scatter plot; and (F) intensity plot for the region of interest (white line in panel D). Reprinted from ref. 158, copyright 2019 American Chemical Society. | |
6.6 Challenges
Over the past few years, scientists engineered several ER-targeting fluorescent probes for the selective detection of various substances like NO, H2S, H2O2, and HOCl. However, the mechanism behind ER selectivity still needs to be clarified. Many biological species present in ER still need to be detected due to a lack of efficient probes. In addition, most of the probes discussed above emit visible light. Thus, their in vivo applicability is restricted.
7 Golgi apparatus-targeting ratiometric fluorescent probes
The Golgi apparatus is an organelle with a phospholipid membrane composed of cisterna. It transforms proteins from the rough ER, then separates them into vesicles for transport to other cell regions. According to recent findings, CO is crucial for the Golgi apparatus.159–161 Thus, to study in depth various functions of the Golgi apparatus, it is essential to develop efficient Golgi apparatus-targeting probes.
7.1 Reactive oxygen species (ROS) detection in Golgi apparatus
Golgi oxidative stress is closely linked to the occurrence and progression of hypertension, and the concentration of hydrogen peroxide (H2O2) plays a critical role in this process. To address this issue, Wang et al. developed a two-photon fluorescent probe 41, which targets the Golgi apparatus with the aid of a phenylsulfonamide group. The response mechanism was a reaction-based process (Fig. 17).162 Upon reacting with H2O2, the boric acid ester is transformed into a hydroxyl group that donates electrons, thereby promoting the push–pull electron effect of the naphthalimide-conjugated system. This leads to the production of strong fluorescence emission. The probe 41 enables in situ H2O2 ratiometric imaging in living systems and provides a highly effective means to monitor Golgi oxidative stress. The probe 41 was able to identify the generation of H2O2 during Golgi oxidative stress and demonstrated increased levels of Golgi H2O2 in the kidneys of hypertensive mice.
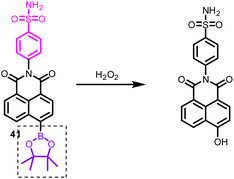 |
| Fig. 17 Reaction-based detection mechanism of probe 41 for H2O2. | |
7.2 Reactive nitrogen species (RNS) detection in Golgi apparatus
The detection of peroxynitrite (ONOO−) is essential for the study and treatment of drug-induced liver injury (DILI) associated with oxidative stress. Targeting the Golgi apparatus has emerged as a new approach for DILI research and treatment. Feng et al. developed a new probe, 42, by conjugating a sulfanilamide moiety to a coumarin–hemi-cyanine conjugated system (Fig. 18).163 The probe displayed high sensitivity, selectivity, and low cytotoxicity, and showed a rapid ratiometric fluorescence response to ONOO−. Unexpectedly, probe 42 also displays unique targeting properties in living cells, with the ability to label the cell membrane first and then the Golgi. Imaging experiments with probe 42 showed it to be effective in monitoring ONOO− under Golgi oxidative stress and in DILI using mice models.
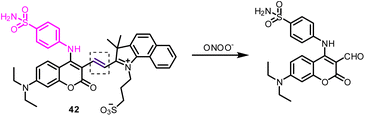 |
| Fig. 18 Reaction-based detection mechanism of probe 42 for ONOO−. | |
7.3 CO monitoring in Golgi apparatus
Inspired by the recent reports on the fluorescent probe for CO detection based on the Tsuji–Trost reaction and to understand in depth the role of CO in subcellular organelles, Zheng et al. constructed probe 43 (Fig. 19, Table 1). On excitation at 360 nm, probe 43 exhibited emission at 425 nm.164 Upon the addition of CORM-3 (CO donor), probe 43 showed a significant 95 nm red shift with a decrease and increased emission maxima at 425 nm and 560 nm, respectively. The detecting mechanism was attributed to the cleavage of the allycarbamate group of probe 43 to form 43a mediated by a Pd0 Tsuji–Trost reaction. Furthermore, probe 43 found application for CO imaging in cells and zebrafish and to visualize CO levels during cellular oxidative stress stimulated by lipopolysaccharide (Fig. 20).
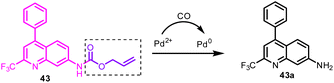 |
| Fig. 19 Sensing mechanism of probe 43 for CO detection based on Tsuji–Trost reaction. | |
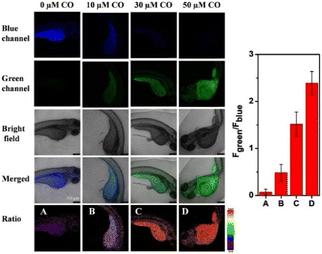 |
| Fig. 20 CO fluorescence imaging in zebrafish with probe 43. The right figure exhibits the fluorescence intensity ratio value (green/blue). Reprinted from ref. 164, copyright 2021 Elsevier. | |
7.4 Glutathione (GSH) detection in Golgi apparatus
The high expression of antioxidants like glutathione in cancer cells helps them withstand oxidative stress within the Golgi apparatus. Thus, monitoring changes in glutathione concentration within the Golgi could serve as an effective way to track the occurrence and progression of tumor cells. Rong et al. created a Golgi-targeting probe 44 that could detect GSH with high accuracy.165 The fluorescence titration experiment of probe 44 with GSH revealed a decrease in intensity at 425 nm and emergence of a new peak at 510 nm. The detection limit was 0.49 μM for GSH. The response mechanism was a reaction-based process (Fig. 21). Furthermore, the Golgi stress response experiment revealed the ability of probe 44 for in situ endogenous GSH detection in the Golgi apparatus during oxidative stress.
 |
| Fig. 21 Reaction-based detection mechanism of probe 44 for GSH. | |
7.5 Challenges
The Golgi apparatus-targeting ratiometric fluorescent probes are rare in the literature. Furthermore, the targeting mechanism is not clear.
8 Nucleus-targeting ratiometric fluorescent probes
For both cancer treatment and genetic engineering, the cell nucleus has been a primary target since it stores the genetic material that is protected by the nuclear envelope, which is made up of two lipid bilayer membranes.166
8.1 Reactive sulphur species (RSS) detection in the nucleus
Organisms' excessive generation of formaldehyde (FA) and sulfur dioxide (SO2) is directly linked to several ailments, such as genotoxicity, respiratory disease, and neurological abnormalities. However, the protective barrier of the cell nucleus membrane makes it challenging for fluorescent probes to investigate the correlation of FA and SO2 in the nucleolus regions. Ma et al. took this challenge and constructed probe 45 based on the benzopyryllium-dansyl FRET platform (Fig. 23, Table 1).52 The probe 45 was used for SO2 reversible sensing and recovered by FA. The sensing mechanism of SO2 was the interruption of the FRET process between dansyl (donor) and benzopyryllium (acceptor). The FA addition restored the FRET process because of the reversible Michael addition reaction. Probe 45 found application for quantitatively monitoring endogenous SO2/FA in the nucleus region of live cells and living animals.
8.2 Reactive nitrogen species (RNS) detection in the nucleus
Nitric oxide (NO) is a significant signal molecule involved in a variety of physiological and pathological processes. Thus, to understand these processes, real-time detection of short-living NO in the biological medium is crucial. Based on their previous reports on NO detection,167 Li et al. developed probe 46 for NO detection in living cells.168 The fluorescence spectrum of probe 46 showed a ratiometric change in fluorescence with NO, and the plot of intensity ratio I530/I424vs. NO concentration was linear between 0 and 40 μM. The sensing mechanism was a reaction-based process in which 46m reacted with NO to generate product 46p (Fig. 22, Table 1). In living RAW 264.7 cells, 46m can detect both exogenous and endogenous NO. It's interesting to note that 46m and its sensing product 46p both show localization to the nucleus and the mitochondria, respectively. In the presence of ctDNA, 46m showed high sensitivity to NO (LOD = 2.8 nM). However, due to nucleus localization, 46p could be an excellent green-fluorescent probe for the nucleus.
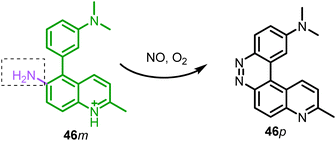 |
| Fig. 22 Reaction-based sensing mechanism of probe 46 for NO detection. | |
8.3 Nucleus DNA detection
The nucleus viscosity and G-quadruplex have several critical roles in biological processes, such as controlling gene expression, preventing tumorigenesis, etc. Thus, to better understand their molecular process and function, an efficient tool that can target the nucleus is in demand. Sun et al. developed D–π–A type probe 47 based on triphenylamine (donor) and N-methyl benzothiazole (acceptor and nucleus-targeting group) (Fig. 23, Table 1).169 With a considerable ratiometric increase in fluorescence, probe 47 revealed strong selectivity to G-quadruplex DNA and viscosity inside the nucleus. However, the notable limitation of probe 47 is that it cannot distinguish between G-quadruplex DNA and viscosity change.
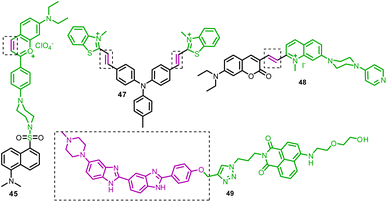 |
| Fig. 23 Chemical structure of nucleus-targeting ratiometric fluorescent probes 45–49. | |
Ferroptosis controls cell death by accumulating lipid peroxide-associated reactive oxygen species, which is predicted to change the shape and polarity of lipid droplets (LDs). However, there needs to be concrete proof of this. Wang et al. reported dual-organelle targeting (LD and nucleus) fluorescent probe 48 for monitoring cellular microenvironment polarity change (Fig. 23, Table 1).170 The fluorescence titration of probe 48 with ds26DNA revealed ∼11-fold increase in emission intensity at 670 nm, while emission maxima at 470 nm remained constant. From molecular modeling calculations, the selectivity of probe 48 to DNA could be due to its binding to the minor grooves of DNA through hydrogen bonding and electrostatic interactions.
Yang et al. developed a ratiometric fluorescent probe 49 based on a naphthalimide dye and a Hoechst (nucleus-targeting unit) (Fig. 23, Table 1).171 Upon gradually increasing the concentration of ctDNA, the emission maxima of probe 49 at 450 nm and 505 nm increased significantly, ascribed to the FRET process from the Hoescht to the naphthalimide unit. Probe 49's remarkable benefit is its ability to provide precise and wash-free nuclear DNA staining in living cells, including normal cells COS-7 and L02, as well as cancer cells MCF-7, SMMC-7721, and HeLa. Furthermore, probe 49 found a potential application for monitoring nucleus DNA damage induced by the anticancer drug etoposide and hydroxyl radicals.
8.4 Challenges
For chemical biology purposes, nucleus-targeting systems have been thoroughly investigated. However, commercially available nucleus-targeting fluorescent probes are limited to staining DNAs.172 Nowadays, newly developed nucleus-targeting probes are more specific to a particular analyte but are still rare. Furthermore, each developed method needs a clear explanation of organelle-specific/targeting mechanism, cellular toxicity, targeting ability in the altered environment, probe stability, response time, etc.
9 Membrane-targeting ratiometric fluorescent probes
Cytomembrane is an essential target for the study of dynamics and morphology due to the recent discovery of membrane microdomains (rafts) in cancer173 and viral infection.174,175 The cytomembrane also contributes to amyloid formation in neurodegenerative diseases.176,177
9.1 Reactive sulphur species (RSS) detection in the membrane
Zhang et al. developed probes 50 and 51 based on coumarin derivatives (Fig. 24, Table 1).178 Notably, the fluorescence spectrum of probe 50 and 51 exhibited ratiometric response with SO2 and visible color change of the solution from dark purple to colorless. The nucleophilic addition reaction was the sensing mechanism of probes 50 and 51 for SO2. From cell imaging experiments, probes 50 and 51 exhibited good cytomembrane-targeting and mitochondrion-targeting ability and can detect SO2 in mice. Due to the negatively charged cell inner membrane, the cationic property of probe 50 allowed it to target the cell membrane through electrostatic interactions. However, the probe 51 can selectively accumulate in mitochondria because of its positively charged nature, long alkyl chain, and appropriate hydrophobic characteristic (Fig. 25).
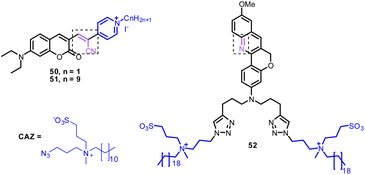 |
| Fig. 24 Chemical structure of membrane-targeting ratiometric fluorescent probes 50–52. | |
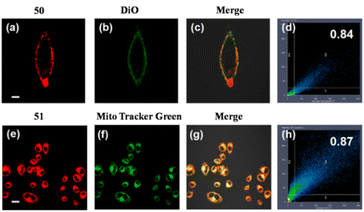 |
| Fig. 25 Co-localization imaging of 50 and 51. Fluorescence images of (a, e) 50 and 51 in the red channel; (b, f) MitoTracker Green in the green channel; (c, g) overlay image; (d, h) 50 and 51 intensity correlation plot. Reprinted from ref. 178, copyright 2021 Elsevier. | |
9.2 Monitoring pH changes in the membrane
The cell’s various biomolecules, pathogens, and fluids are regulated by intracellular vesicles that the plasma membrane produces.179 Intravesicular pH changes during trafficking depend on endosomal maturation and signaling.180 The cell’s various biomolecules, pathogens, and fluids are regulated by intracellular vesicles that the plasma membrane produces. Inspired by the work of Liu et al. on red-shifted chromenoquinoline based probes,181 Michelis et al. designed probe 52 based on chromenoquinoline for imaging the distribution and acidification of intracellular vesicles and to measure the pH of individual vesicles (Fig. 24, Table 1).182 From the fluorescence experiment, probe 52 underwent an increase in emission intensity upon binding with the plasma membrane (10-fold). Furthermore, probe 52 monitored the acidification of the vesicles throughout the endocytic pathway. The main limitation associated with probe 52 is that we cannot use it for long-term tracking due to the instability of the basic form.
9.3 Challenges
Despite several efforts in designing membrane-targeting ratiometric fluorescent probes, literature reports are rare. To date, no commercially available membrane-targeting fluorescent probes are available. In the future, researchers must consider several parameters for designing efficient membrane-targeting fluorescent probes, such as probe stability, selectivity, sensitivity, response time, orientation/location in the membrane, and detailed mechanism of interaction with the membrane.
10 Multi organelle-targeting ratiometric fluorescent probes
Developing a powerful molecular tool that can target other organelles simultaneously is necessary to study the relationship between different organelles.
10.1 Reactive sulphur species (RSS) detection in lysosome and mitochondria
From a recent study, SO2 is a critical gas messenger that plays a vital role in many cellular processes, including apoptosis in lysosomes and mitochondria. Therefore, to know the relationship between lysosome and mitochondria in regulating SO2-related cellular activities, Kong et al. pioneered probe 53 (Fig. 26, Table 1).183 Free probe 53 showed emission at 600 nm (red fluorescence) owing to the FRET process between the naphthalimide unit and the semi-cyanine unit. Upon the addition of SO2, the emission intensity at 600 nm decreased. In comparison, a new emission maximum at 530 nm appeared and increased, assigned to Michael's addition reaction of SO2 in the semi-cyanine unit and inhibition of the FRET process. In living cells, probe 53 found an application for simultaneously detecting endogenous SO2 in lysosome and mitochondria by one and two-photon modes.
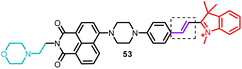 |
| Fig. 26 Chemical structure of dual organelle-targeting ratiometric fluorescent probe 53. | |
10.2 Monitoring pH fluctuation in mitochondria and lipid droplets (LDs)
Understanding the fundamental connection between fluctuating mitochondrial pH and lipid droplet (LD) generation is crucial for understanding cell physiology. Bai et al. developed probe 54 based on hemicyanine and rhodamine dyes for selectively monitoring mitochondria and lipid droplets under different pH values through a dual-emission channel (Fig. 27 and 28, Table 1).19 The pH (from 2.52 to 10.50) sensing behavior of probe 54 revealed a notable decrease in the emission band at 580 nm, accompanied by the increase in a new emission peak at 450 nm, attributed to two different structural forms under acidic and basic medium. Under acidic conditions, the ring-open form of probe 54 targeted mitochondria and displayed solid red emission. In contrast, the ring-closed form of probe 54 targeted LDs and gave blue emission. Furthermore, applied probe 54 monitored the pH fluctuation in living cells in the presence of different exotic chemicals.
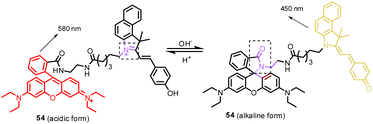 |
| Fig. 27 Chemical structure of probe 54 and the proposed pH sensing mechanism. | |
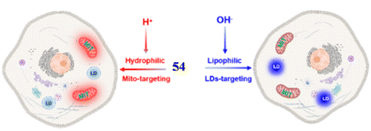 |
| Fig. 28 Organelle-targeting mechanism of probe 54. Reprinted from ref. 19, copyright 2022 American Chemical Society. | |
10.3 Challenges
Dual organelle-targeting probes hold great promise for elucidating the relationship between organelles and deepening our understanding of the biological processes behind the biological species. However, building molecular probes with two or more sensitive moieties is challenging. Furthermore, dual organelle-targeting probes are rare in the literature.
11 Conclusion and future outlook
This review article highlighted recent advances in organelle-targeting ratiometric fluorescent probes reported since 2015. In particular, we discussed synthetic fluorescent probes with potential applications in biological systems such as biological species detection, fluorescence imaging, studying physiological and pathological processes, etc. Additionally, we highlighted the methods utilized to construct these probes and sensing mechanisms for their response to particular species.
Nowadays, significant research has been put into developing organelle-targeting fluorescent probes to create fluorescent tools with improved resolution and sensitivity and a better understanding of the molecular mechanisms behind various biological processes. In conclusion, high selectivity, high reactivity, fast response time, low detection limit, good solubility, and organelle-targeting ability are the multiple advantages of the fluorescent probe. Currently, ratiometric probes that meet all the above properties are rare in the literature.
Researchers will always be very interested in the advancement of ratiometric probes and their commercial applications. Despite the abundance of current outcomes, the following areas require additional work in the future. First, compared to traditional one-photon fluorescence probes, two-photon fluorescent probes provide several advantages, such as deep visualization, reduced photo-toxicity, minimal light scattering, and highly bright and contrast images.184 Thus, the development of ratiometric probes with two-photon properties is greatly needed. Second, for in vivo application, near-infra-red (NIR) probes provide several advantages, such as deep tissue penetration, reduced photon scattering, and reduced photodamage to the living organism.185 Thus, ratiometric probes with emissions in the NIR region are in demand. Third, Reversible probes hold great promise for revealing the dynamic states of relevant analytes in various processes.52 Thus, developing ratiometric probes for biological species detection based on reversible reactions is essential. Fourth, probes targeting organelles such as the nucleus, membrane, lipid droplet (LD), melanosome, and Golgi apparatus are rare in the literature (Table 1). Fifth, there are several species in biological systems. However, only a limited number of species, such as ROS, RNS, RSS, metal ions, and pH, have been the subject of interest (Table 1). Sixth, most of the discussed ratiometric probes have found applications for the bio-imaging of biological species in living cells and mice. To date, no commercially available ratiometric probes are available. By taking the factors mentioned above into account in the probe design, fluorescent probes can be utilized as significant materials in the coming days, and their commercial availability will be anticipated in the future.
Data availability
All the data were collected freely from websites such as https://scholar.google.com/ and https://www.sci-hub.se/. Microsoft Office Word 2007 was used for writing the article, while Microsoft Office PowerPoint 2007 was used for graphical presentation. ChemBioDraw was used for chemical structure drawing.
Author contributions
Dr Manoj Kumar Goshisht and Dr Neetu Tripathi contributed equally to writing the original draft and to reviewing and editing the manuscript. Dr Goutam Kumar Patra and Dr Manohar Chaskar contributed to reviewing and editing the manuscript.
Conflicts of interest
The authors declare that they have no known competing financial interests or personal relationships that could have appeared to influence the work reported in this paper.
References
- X. Chen, R. Kang, G. Kroeme and D. Tang, Organelle-specific regulation of ferroptosis, Cell Death Differ., 2021, 28, 2843–2856 CrossRef CAS PubMed
.
- N. Trinh, K. A. Jolliffe and E. New, Dual-Functionalisation of Fluorophores for the Preparation of Targeted and Selective Probes, Angew. Chem., Int. Ed., 2020, 59, 20290–20301 CrossRef CAS PubMed
.
- M. Schrader, M. Kamoshita and M. Islinger, Organelle interplay-peroxisome interactions in health and disease, J. Inherited Metab. Dis., 2020, 43, 71–89 CrossRef PubMed
.
- J. Lin, K. Yang and E. J. New, Strategies for organelle targeting of fluorescent probes, Org. Biomol. Chem., 2021, 19, 9339–9357 RSC
.
- Z. He, Y. Zhang, A. R. Khan, J. Ji, A. Yu and G. Zhai, A novel progress of drug delivery system for organelle targeting in tumour cells, J. Drug Targeting, 2020, 29, 12–28 CrossRef PubMed
.
- Y. Chen, Y. Bai, Z. Han, W. He and Z. Guo, Photoluminescence imaging of Zn2+ in living systems, Chem. Soc. Rev., 2015, 44, 4517–4546 RSC
.
- N.-E. Choi, J.-Y. Lee, E.-C. Park, J.-H. Lee and J. Lee, Recent Advances in Organelle-Targeted Fluorescent Probes, Molecules, 2021, 26, 217 CrossRef CAS PubMed
.
- A. Bigdeli, F. Ghasemi, S. Abbasi-Moayed, M. Shahrajabian, N. Fahimi-Kashani, S. Jafarinejad, M. A. F. Nejad and M. R. Hormozi-Nezhad, Ratiometric fluorescent nanoprobes for visual detection: design principles and recent advances – a review, Anal. Chim. Acta, 2019, 1079, 30–58 CrossRef CAS PubMed
.
- S.-H. Park, N. Kwon, J.-H. Lee, J. Yoon and I. Shin, Synthetic ratiometric fluorescent probes for detection of ions, Chem. Soc. Rev., 2020, 49, 143–179 RSC
.
- X. Huang, J. Song, B. C. Yung, X. Huang, Y. Xiong and X. Chen, Ratiometric optical nanoprobes enable accurate molecular detection and imaging, Chem. Soc. Rev., 2018, 47, 2873–2920 RSC
.
- M. H. Lee, J. S. Kim and J. L. Sessler, Small molecule-based ratiometric fluorescence probes for cations, anions, and biomolecules, Chem. Soc. Rev., 2015, 44, 4185–4191 RSC
.
- P. Wu, X. Hou, J.-J. Xu and H.-Y. Chen, Ratiometric fluorescence, electrochemiluminescence, and photoelectrochemical chemo/biosensing based on semiconductor quantum dots, Nanoscale, 2016, 8, 8427–8442 RSC
.
- S. Wu, H. Min, W. Shi and P. Cheng, Multicenter Metal–Organic Framework-Based Ratiometric Fluorescent Probes, Adv. Mater., 2020, 32, 1805871 CrossRef CAS PubMed
.
- Q. Yuan, Z.-M. Zhao, Y.-R. Zhang, L. Su, J.-Y. Miao and B.-X. Zhao, A lysosome-targeted ratiometric fluorescent probe for detection of hypochlorous acid in living cells, Sens. Actuators, B, 2017, 247, 736–741 CrossRef CAS
.
- S.-L. Shen, X.-Q. Huang, X.-H. Lin and X.-Q. Cao, A ratiometric fluorescent probe for lysosomal hypochlorous acid based on through-bond energy transfer strategy, Anal. Chim. Acta, 2019, 1052, 124–130 CrossRef CAS PubMed
.
- F. Ghasemi, M. R. Hormozi-Nezhad and M. Mahmoudi, A new strategy to design colorful ratiometric probes and its application to fluorescent detection of Hg(II), Sens. Actuators, B, 2018, 259, 894–899 CrossRef CAS
.
- B. Liu, F. Zeng, G. Wu and S. Wu, Nanoparticles as scaffolds for FRET-based ratiometric detection of mercury ions in water with QDs as donors, Analyst, 2012, 137, 3717–3724 RSC
.
- Y. L. Pak, S. J. Park, G. Song, Y. Yim, H. Kang, H. M. Kim, J. Bouffard and J. Yoon, Endoplasmic Reticulum-Targeted
Ratiometric N-Heterocyclic Carbene Borane Probe for Two-Photon Microscopic Imaging of Hypochlorous Acid, Anal. Chem., 2018, 90, 12937–12943 CrossRef CAS
.
- Q. Bai, C. Yang, M. Yang, Z. Pei, X. Zhou, J. Liu, H. Ji, G. Li, M. Wu, Y. Qin, Q. Wang and L. Wu, pH-Dominated Selective Imaging of Lipid Droplets and Mitochondria via a Polarity-Reversible Ratiometric Fluorescent Probe, Anal. Chem., 2022, 94, 2901–2911 CrossRef CAS
.
- M. Ren, K. Zhou, L. He and W. Lin, Mitochondria and lysosome-targetable fluorescent probes for HOCl: recent advances and perspectives, J. Mater. Chem. B, 2018, 6, 1716–1733 RSC
.
- X.-Z. Yang, X.-R. Wei, R. Sun, Y.-J. Xu and J.-F. Ge, A novel xanthylene-based effective mitochondria-targeting ratiometric cysteine probe and its bioimaging in living cells, Talanta, 2020, 209, 120580 CrossRef CAS PubMed
.
- G. Cheng, J. Fan, W. Sun, K. Sui, X. Jin, J. Wang and X. Peng, A highly specific BODIPY-based probe localized in mitochondria for HClO imaging, Analyst, 2013, 138, 6091–6096 RSC
.
- Q. Huang, Y. Zhou, Q. Zhang, E. Wang, Y. Min, H. Qiao, J. Zhang and T. Ma, A new “off–on” fluorescent probe for Pd2+ in aqueous solution and live-cell based on spirolactam ring-opening reaction, Sens. Actuators, B, 2015, 208, 22–29 CrossRef CAS
.
- J. Liu, X. Liu, S. Lu, L. Zhang, L. Feng, S. Zhong, N. Zhang, T. Bing and D. Shangguan, Ratiometric detection and imaging of hydrogen sulfide in mitochondria based on a cyanine/naphthalimide hybrid fluorescent probe, Analyst, 2020, 145, 6549–6555 RSC
.
- X. Zhang, G. J. Song, X. J. Cao, J. T. Liu, M. Y. Chen, X. Q. Cao and B.-X. Zhao, A new fluorescent pH probe for acidic conditions, RSC Adv., 2015, 5, 89827–89832 RSC
.
- J. Yang, K. Li, J.-T. Hou, L.-L. Li, C.-Y. Lu, Y.-M. Xie, X. Wang and X.-Q. Yu, Novel Tumor-Specific and Mitochondria-Targeted near-Infrared-Emission Fluorescent Probe for SO2 Derivatives in Living Cells, ACS Sens, 2016, 2, 166–172 CrossRef
.
- J.-T. Hou, M.-Y. Wu, K. Li, J. Yang, K.-K. Yu, Y.-M. Xie and X.-Q. Yu, Mitochondria-targeted colorimetric and fluorescent probes for hypochlorite and their applications for in vivo imaging, Chem. Commun., 2014, 50, 8640–8643 RSC
.
- L. Zang, C. Liang, Y. Wang, W. Bu, H. Sun and S. Jiang, A highly specific pyrene-based fluorescent probe for hypochlorite and its application in cell imaging, Sens. Actuators, B, 2015, 211, 164–169 CrossRef CAS
.
- L. Wang, M. Ren, Z. Li, L. Dai and W. Lin, Development of a FRET-based ratiometric fluorescent probe to monitor the changes in palladium(II) in aqueous solution and living cells, New J. Chem., 2019, 43, 552 RSC
.
- P. Gao, W. Pan, N. Li and B. Tang, Fluorescent probes for organelle-targeted biological species imaging, Chem. Sci., 2019, 10, 6035–6071 RSC
.
- Z. Xue, R. Zhu, S. Wang, J. Li, J. Han, J. Liu and S. Han, Organelle-Directed Staudinger Reaction Enabling Fluorescence-on Resolution of Mitochondrial Electropotentials via a Self-Immolative Charge Reversal Probe, Anal. Chem., 2018, 90, 2954–2962 CrossRef CAS PubMed
.
- T. B. Ren, Q. L. Zhang, D. Su, X. X. Zhang, L. Yuan and X. B. Zhang, Detection of analytes in mitochondria without interference from other sites based on an innovative
ratiometric fluorophore, Chem. Sci., 2018, 9, 5461–5466 RSC
.
- Y. Chen, C. Zhu, J. Cen, Y. Bai, W. He and Z. Guo, Ratiometric detection of pH fluctuation in mitochondria with a new fluorescein/cyanine hybrid probe, Chem. Sci., 2015, 6, 3187–3194 RSC
.
- X. Zhang, B. Wang, C. Wang, L. Chen and Y. Xiao, Monitoring Lipid Peroxidation within Foam Cells by Lysosome-Targetable and Ratiometric Probe, Anal. Chem., 2015, 87, 8292–8300 CrossRef CAS PubMed
.
- Z. Wu, D. Liang and X. Tang, Visualizing Hydrogen Sulfide in Mitochondria and Lysosome of Living Cells and in Tumors of Living Mice with Positively Charged Fluorescent Chemoprobes, Anal. Chem., 2016, 88, 9213–9218 CrossRef CAS
.
- Y. Cai, C. Gui, K. Samedov, H. Su, X. Gu, S. Li, W. Luo, H. H. Y. Sung, J. W. Y. Lam, R. T. K. Kwok, I. D. Williams, A. Qin and B. Z. Tang, An acidic pH independent piperazine-TPE AIEgen as a unique bioprobe for lysosome tracing, Chem. Sci., 2017, 8, 7593 RSC
.
- R. Zhou, Q. Peng, D. Wan, C. Yu, Y. Zhang, Y. Hou, Q. Luo, X. Li, S. Zhang, L. Xie, P. Ou and Y. Peng, Construction of a lysosome-targetable ratiometric fluorescent probe for H2O2 tracing and imaging in living cells and an inflamed model, RSC Adv., 2021, 11, 24032 RSC
.
- L. Fang, G. Trigiante, R. Crespo-Otero, C. S. Hawes, M. P. Philpott, C. R. Jones and M. Watkinson, Endoplasmic reticulum targeting fluorescent probes to image mobile Zn2+, Chem. Sci., 2019, 10, 10881–10887 RSC
.
- J.-T. Hou, H. S. Kim, C. Duan, M. S. Ji, S. Wang, L. Zeng, W. X. Ren and J. S. Kim, A ratiometric fluorescent probe for detecting hypochlorite in the endoplasmic reticulum, Chem. Commun., 2019, 55, 2533–2536 RSC
.
- B. Dong, W. Song, Y. Lu, X. Kong, A. H. Mehmood and W. Lin, An ultrasensitive ratiometric fluorescent probe based on the ICT-PET-FRET mechanism for the quantitative measurement of pH values in the endoplasmic reticulum (ER), Chem. Commun., 2019, 55, 10776–10779 RSC
.
- Q. Ma, C. Wang, G. Mao, M. Tian, J. Suna and S. Feng, An endoplasmic reticulum-targeting and ratiometric fluorescent probe for hypochlorous acid in living cells based on a 1,8-naphthalimide derivative, New J. Chem., 2020, 44, 18389–18398 RSC
.
- J. Colston, R. Horobin, F. Rashid-Doubell, J. Pediani and K. K. Johal, Why fluorescent probes for endoplasmic reticulum are selective: an experimental and QSAR-modelling study, Biotech. Histochem., 2003, 78, 323–332 CrossRef CAS PubMed
.
- L. Zhou, Z.-Q. Cheng, N. Li, Y.-X. Gea, H.-X. Xie, K. Zhu, A. Zhou, J. Zhang, K.-M. Wang and C.-S. Jiang, A highly sensitive endoplasmic reticulum-targeting fluorescent probe for the imaging of endogenous H2S in live cells, Spectrochim. Acta, Part A, 2020, 240, 118578 CrossRef CAS
.
- T. Yang, J. Sun, W. Yao and F. Gao, A two-photon fluorescent probe for turn-on monitoring HOCl level in endoplasmic reticulum, Dyes Pigm., 2020, 180, 108435 CrossRef CAS
.
- W. Zhang, J. Zhang, P. Li, J. Liu, D. Su and B. Tang, Two-photon fluorescence imaging reveals a Golgi apparatus superoxide anion-mediated hepatic ischaemia-reperfusion signalling pathway, Chem. Sci., 2018, 10, 879–883 RSC
.
- R. S. Li, P. F. Gao, H. Z. Zhang, L. L. Zheng, C. M. Li, J. Wang, Y. F. Li, F. Liu, N. Li and C. Z. Huang, Chiral nanoprobes for targeting and long-term imaging of the Golgi apparatus, Chem. Sci., 2017, 8, 6829–6835 RSC
.
- P. Li, X. Guo, X. Bai, X. Wang, Q. Ding, W. Zhang, W. Zhang and B. Tang, Golgi Apparatus Polarity Indicates Depression-Like Behaviors of Mice Using in Vivo Fluorescence Imaging, Anal. Chem., 2019, 91, 3382–3388 CrossRef CAS PubMed
.
- T. Hirayama, M. Inden, H. Tsuboi, M. Niwa, Y. Uchida, Y. Naka, I. Hozumi and H. Nagasawa, A Golgi-targeting fluorescent probe for labile Fe(II) to reveal an abnormal cellular iron distribution induced by dysfunction of VPS35, Chem. Sci., 2019, 10, 1514–1521 RSC
.
- M. W. Hetzer, The Nuclear Envelope, Perspect. Biol., 2010, 2, a000539 Search PubMed
.
- A. Nakamura, K. Takigawa, Y. Kurishita, K. Kuwata, M. Ishida, Y. Shimoda, I. Hamachi and S. Tsukiji, Hoechst tagging: a modular strategy to design synthetic fluorescent probes for live-cell nucleus imaging, Chem. Commun., 2014, 50, 6149–6152 RSC
.
- G. Lukinavicius, C. Blaukopf, E. Pershagen, A. Schena, L. Reymond, E. Derivery, M. Gonzalez-Gaitan, E. D'Este, S. W. Hell, D. W. Gerlich and K. Johnsson, SiR–Hoechst is a far-red DNA stain for live-cell nanoscopy, Nat. Commun., 2015, 6, 8497 CrossRef CAS PubMed
.
- Y. Ma, W. Gao, L. Zhu, Y. Zhao and W. Lin, A ratiometric fluorescent probe for reversible monitoring of endogenous SO2/formaldehyde in cytoplasm and nucleoli regions and its applications in living mice, Analyst, 2020, 145, 1865–1870 RSC
.
- S. Zhang, H. Sun, H. Chen, Q. Li, A. Guan, L. Wang, Y. Shi, S. Xu, M. Liu and Y. Tang, Direct visualization of nucleolar G-quadruplexes in live cells by using a fluorescent light-up probe, Biochim. Biophys. Acta, Gen. Subj., 2018, 1862, 1101–1106 CrossRef CAS PubMed
.
- N. Sato, G. Tsuji, Y. Sasaki, A. Usami, T. Moki, K. Onizuka, K. Yamada and F. Nagatsugi, A new strategy for site-specific alkylation of DNA using oligonucleotides containing an abasic site and alkylating probes, Chem. Commun., 2015, 51, 14885–14888 RSC
.
- M. K. Goshisht and N. Tripathi, Fluorescence-based probes as an emerging tool for anion detection: mechanism, probey materials and applications, J. Mater. Chem. C, 2021, 9, 9820–9850 RSC
.
- M. K. Goshisht, G. K. Patra and N. Tripathi, Fluorescent Schiff base probes as a versatile tool for metal ion detection: strategies, mechanistic insights, and applications, Mater. Adv., 2022, 3, 2612–2669 RSC
.
-
N. Tripathi and M. K. Goshisht, Aggregation of Luminophores in Supramolecular Systems: From Mechanisms to Applications, CRC Press, Taylor Fr., 2020, pp. 1–220 Search PubMed
.
- Z. Liu, W. He and Z. Guo, Metal coordination in photoluminescent sensing, Chem. Soc. Rev., 2013, 42, 1568–1600 RSC
.
- J. Gong, C. Liu, X. Jiao, S. He, L. Zhao and X. Zeng, Novel mitochondria-targeted viscosity probe based on a fluorescent rotatable xanthene-hemicyanine dyad, Microchem. J., 2020, 158, 105191 CrossRef CAS
.
- W. Sun, Y.-D. Shi, A.-X. Ding, Z.-L. Tan, H. Chen, R. Liu, R. Wang and Z.-L. Lu, Imaging viscosity and peroxynitrite by a mitochondria-targeting two-photon ratiometric fluorescent probe, Sens. Actuators, B, 2018, 276, 238–246 CrossRef CAS
.
- K. Yang, J. L. Kolanowski and E. J. New, Mitochondrially targeted fluorescent redox probes, Interface Focus, 2017, 7, 20160105 CrossRef PubMed
.
- G. Zhang, J. J. Gruskos, M. S. Afzal and D. Buccella, Visualizing changes in mitochondrial Mg2+ during apoptosis with organelle-targeted triazole-based ratiometric fluorescent probes, Chem. Sci., 2015, 6, 6841 RSC
.
- Z. M. Prokopowicz, F. Arce, R. Biedron, C. L. Chiang, M. Ciszek, D. R. Katz, M. Nowakowska, S. Zapotoczny, J. Marcinkiewic and B. M. Chain, Hypochlorous Acid: A Natural Adjuvant That Facilitates Antigen Processing, Cross-Priming, and the Induction of Adaptive Immunity, J. Immunol., 2010, 184, 824–835 CrossRef CAS PubMed
.
- N. Tripathi and M. K. Goshisht, Recent Advances and Mechanistic Insights into Antibacterial Activity, Antibiofilm Activity, and Cytotoxicity of Silver Nanoparticles, ACS Appl. Bio Mater., 2022, 5, 1391–1463 CrossRef CAS PubMed
.
- Q. Hu, C. Qin, L. Huang, H. Wang, Q. Liu and L. Zeng, Selective visualization of hypochlorite and its fluctuation in cancer cells by a mitochondria-targeting ratiometric fluorescent probe, Dyes Pigm., 2018, 149, 253–260 CrossRef CAS
.
- M. Valko, D. Leibfritz, J. Moncol, M. T. Cronin, M. Mazur and J. Telser, Free radicals and antioxidants in normal physiological functions and human disease, Int. J. Biochem. Cell Biol., 2007, 39, 44 CrossRef CAS PubMed
.
- B. D'Autréaux and M. B. Toledano, ROS as signalling molecules: mechanisms that generate specificity in ROS homeostasis, Nat. Rev. Mol. Cell Biol., 2007, 8, 813–824 CrossRef PubMed
.
- N. Li, J. Huang, Q. Wang, Y. Gu and P. Wang, A reaction based one- and two-photon fluorescent probe for selective imaging H2O2 in living cells and tissues, Sens. Actuators, B, 2018, 254, 411–416 CrossRef CAS
.
- A. M. Shah and K. M. Channon, Free radicals and redox signalling in cardiovascular disease, Heart, 2004, 90, 486–487 CrossRef CAS PubMed
.
- L. He, X. Liu, Y. Zhang, L. Yang, Q. Fang, Y. Geng, W. Chen and X. Song, A mitochondria-targeting ratiometric fluorescent probe for imaging hydrogen peroxide with long-wavelength emission and large Stokes shift, Sens. Actuators, B, 2018, 276, 247–253 CrossRef CAS
.
- S.-L. Shen, X.-F. Zhang, Y.-Q. Ge, Y. Zhua and X.-Q. Cao, A mitochondria-targeting ratiometric fluorescent probe for the detection of hypochlorite based on the FRET strategy, RSC Adv., 2017, 7, 55296 RSC
.
- C. Han, H. Yang, M. Chen, Q. Su, W. Feng and F. Li, Mitochondria-Targeted Near-Infrared Fluorescent Off–On Probe for Selective Detection of Cysteine in Living Cells and in vivo, ACS Appl. Mater. Interfaces, 2015, 7, 27968–27975 CrossRef CAS PubMed
.
- Y. Zhou and J. Yoon, Recent progress in fluorescent and colorimetric chemoprobes for detection of amino acids, Chem. Soc. Rev., 2012, 41, 52–67 RSC
.
- D. W. Bak and E. Weerapana, Cysteine-mediated redox signalling in the mitochondria, Mol. BioSyst., 2015, 11, 678–697 RSC
.
- S. Y. Lim, K. H. Hong, D. I. Kim, H. Kwon and H. J. Kim, Tunable heptamethine-azo dye conjugate as an NIR fluorescent probe for the selective detection of mitochondrial glutathione over cysteine and homocysteine, J. Am. Chem. Soc., 2014, 136, 7018–7025 CrossRef CAS PubMed
.
- W. Niu, L. Guo, Y. Li, S. Shuang, C. Dong and M. S. Wong, Highly Selective Two-Photon Fluorescent Probe for Ratiometric Sensing and Imaging Cysteine in Mitochondria, Anal. Chem., 2016, 88, 1908–1914 CrossRef CAS PubMed
.
- K. R. Olson, Hydrogen sulfide, reactive sulfur species and coping with reactive oxygen species, Free Radical Biol. Med., 2019, 140, 74–83 CrossRef CAS PubMed
.
- J. Beltowski, G. Wojcicka and A. Jamroz-Wisniewska, Hydrogen sulfide in the regulation of insulin secretion and insulin sensitivity: implications for the pathogenesis and treatment of diabetes mellitus, Biochem. Pharmacol., 2018, 149, 60–76 CrossRef CAS PubMed
.
- C. Szabo, C. Coletta, C. Chao, K. Modis, B. Szczesny, A. Papapetropoulos and M. R. Hellmich, Tumor-derived hydrogen sulfide, produced by cystathionine-β-synthase, stimulates bioenergetics, cell proliferation, and angiogenesis in colon cancer, Proc. Natl. Acad. Sci. U. S. A., 2013, 110, 12474–12479 CrossRef CAS PubMed
.
- L. Zhou, L. Xie, C. Liu and Y. Xiao, New trends of molecular probes based on the fluorophore 4-amino-1,8-naphthalimide, Chin. Chem. Lett., 2019, 30, 1799–1808 CrossRef CAS
.
- Q. Han, L. Yang, Y. Song, J. Ru, H. Zhang, H. Jiang and X. Wang, A ratiometric fluorescent probe for monitoring the changes in the level of hydrogen polysulfides in mitochondria during stimulus-induced redox imbalance, Dyes Pigm., 2021, 188, 109190 CrossRef CAS
.
- F. Pannullo, D. Lee, L. Neal, M. Dalvi, P. Agnew, F. M. Connor and C. Sarran, Scoping the proximal and distal dimensions of climate change on health and wellbeing, Environ. Health, 2017, 16, 29 CrossRef PubMed
.
- A. Salurcan, M. Turkyilmaz and M. Ozkan, Effects of sulfur dioxide concentration on organic acids and β-carotene in dried apricots during storage, Food Chem., 2017, 221, 412–421 CrossRef CAS PubMed
.
- S. C. Morgan, C. M. Scholl, N. L. Benson, M. L. Stone and D. M. Durall, Sulfur dioxide addition at crush alters Saccharomyces cerevisiae strain composition in spontaneous fermentations at two Canadian wineries, Int. J. Food Microbiol., 2017, 244, 96–102 CrossRef CAS PubMed
.
- R. Nassar, A. Trivella, S. Mokh, M. Al-Iskandarani, H. Budzinski and P. Mazellier, Photodegradation of sulfamethazine, sulfamethoxypiridazine, amitriptyline, and clomipramine drugs in aqueous media, J. Photochem. Photobiol., A, 2017, 336, 176–182 CrossRef CAS
.
- Y. Zhang, L. Guan, H. Yu, Y. Yan, L. Du, Y. Liu, M. Sun, D. Huang and S. Wang, Reversible Fluorescent Probe for Selective Detection and Cell Imaging of Oxidative Stress Indicator Bisulfite, Anal. Chem., 2016, 88, 4426–4431 CrossRef CAS PubMed
.
- Y. Sun, S. Fan, S. Zhang, D. Zhao, L. Duan and R. Li, A fluorescent turn-on probe based on benzo[e]indolium for bisulfite through 1,4-addition reaction, Sens. Actuators, B, 2014, 193, 173–177 CrossRef CAS
.
- Y. Huang, Y. Zhang, F. Huo and C. Yin, FRET-dependent single/two-channel switch endowing a dual detection for sulfite and its organelle targeting applications, Dyes Pigm., 2021, 184, 108869 CrossRef CAS
.
- Y. Liu, K. Li, M. -Y. Wu, Y. -H. Liu, Y. -M. Xie and X. -Q. Yu, A mitochondria-targeted colorimetric and ratiometric fluorescent probe for biological SO2 derivatives in living cells, Chem. Commun., 2015, 51, 10236–10239 RSC
.
- Y. Liu, K. Li, K.-X. Xie, L.-L. Li, K.-K. Yu, X. Wang and X.-Q. Yu, A water-soluble and fast-response mitochondria-targeted fluorescent probe for colorimetric and ratiometric sensing of endogenously generated SO2 derivatives in living cells, Chem. Commun., 2016, 52, 3430–3433 RSC
.
- Y. Wang, Q. Meng, R. Zhang, H. Jia, X. Zhang and Z. Zhang, A ratiometric fluorescence probe for imaging sulfur dioxide derivatives in the mitochondria of living cells, Org. Biomol. Chem., 2017, 15, 2734–2739 RSC
.
- X.-B. Wang, H.-J. Li, Z. Chi, X. Zhao and Y.-C. Wu, A novel mitochondrial-targeted two-photon fluorescent probe for ultrafast monitoring of SO2 derivatives and its applications, Talanta, 2020, 217, 121086 CrossRef CAS PubMed
.
- X.-B. Wang, H.-J. Li, Z. Chi, X. Zeng, L.-J. Wang, Y.-F. Cheng and Y.-C. Wu, A novel mitochondrial targeting fluorescent probe for ratiometric imaging SO2 derivatives in living
cells, J. Photochem. Photobiol., A, 2020, 390, 112339 CrossRef CAS
.
- W.-L. Wu, H.-L. Ma, M.-F. Huang, J.-Y. Miao and B.-X. Zhao, Mitochondria-targeted ratiometric fluorescent probe based on FRET for bisulfite, Sens. Actuators, B, 2017, 241, 239–244 CrossRef CAS
.
- J. Xu, J. Pan, X. Jiang, C. Qin, L. Zeng, H. Zhang and J. F. Zhang, A mitochondria-targeted ratiometric fluorescent probe for rapid, sensitive and specific detection of biological SO2 derivatives in living cells, Biosens. Bioelectron., 2016, 77, 725–732 CrossRef CAS PubMed
.
- L.-J. Zhang, Z.-Y. Wang, J.-T. Liu, J.-Y. Miao and B.-X. Zhao, A rational design of ratiometric fluorescent probes based on new ICT/FRET platform and imaging of endogenous sulfite in living cell, Sens. Actuators, B, 2017, 253, 19–26 CrossRef CAS
.
- M. Zhao, D. Liu, L. Zhou, B. Wu, X. Tian, Q. Zhang, H. Zhou, J. Yang, J. Wu and Y. Tian, Two water-soluble two-photon fluorescence probes for ratiometric imaging endogenous SO2 derivatives in mitochondria, Sens. Actuators, B, 2018, 255, 1228–1237 CrossRef CAS
.
- X.-L. Zheng, H. Li, W. Feng, H.-C. Xia and Q.-H. Song, Two-Step Sensing, Colorimetric and Ratiometric Fluorescent Probe for Rapid Detection of Bisulfite in Aqueous Solutions and in Living Cells, ACS Omega, 2018, 3, 11831–11837 CrossRef CAS PubMed
.
- P. B. Mahaboob and S. M. Saumya, Suppression of mitochondrial oxidative phosphorylation and TCA enzymes in discrete brain regions of mice exposed to high fluoride: Amelioration by Panax ginseng (Ginseng) and Lagerstroemia speciosa (Banaba) extracts, Cell. Mol. Neurobiol., 2013, 33, 453–464 CrossRef PubMed
.
- Y. Shen, X. Zhang, Y. Zhang, H. Li and Y. Chen, An ICT-Modulated strategy to construct colorimetric and ratiometric fluorescent probe for mitochondria-targeted fluoride ion in cell living, Sens. Actuators, B, 2018, 258, 544–549 CrossRef CAS
.
- R. Balamurugan, J.-H. Liu and B.-T. Liu, A review of recent developments in fluorescent probes for the selective detection of palladium ions, Coord. Chem. Rev., 2018, 376, 196–224 CrossRef CAS
.
- L. Zhang, Y. Wang, J. Yu, G. Zhang, X. Cai, Y. Wu and L. Wang, A colorimetric and fluorescent probe based on PBIs for palladium detection, Tetrahedron Lett., 2013, 54, 4019–4022 CrossRef CAS
.
- R. M. Perera and R. Zoncu, The Lysosome as a Regulatory Hub, Annu. Rev. Cell Dev. Biol., 2016, 32, 223–253 CrossRef CAS PubMed
.
- M. Ren, B. Deng, K. Zhou, X. Kong, J. Y. Wang and W. Lin, Single Fluorescent Probe for Dual-Imaging Viscosity and H2O2 in Mitochondria with Different Fluorescence Signals in Living Cells, Anal. Chem., 2017, 89, 552 CrossRef CAS PubMed
.
- J. Liu, S. Zhou, J. Ren, C. Wu and Y. Zhao, A lysosome-locating and acidic pH-activatable fluorescent probe for visualizing endogenous H2O2 in lysosomes, Analyst, 2017, 142, 4522 RSC
.
- Z. Wu, M. Liu, Z. Liu and Y. Tian, Real-Time Imaging and Simultaneous Quantification of Mitochondrial H2O2 and ATP in Neurons with a Single Two-Photon Fluorescence-Lifetime-Based Probe, J. Am. Chem. Soc., 2020, 142, 7532 CrossRef CAS PubMed
.
- Y. R. Zhang, N. Meng, J. Y. Miao and B. X. Zhao, A Ratiometric Fluorescent Probe Based on a Through-Bond Energy Transfer (TBET) System for Imaging HOCl in Living Cells, Chem.–Eur. J., 2015, 21, 19058–19063 CrossRef CAS PubMed
.
- S. L. Shen, J. Y. Ning, X. F. Zhang, J. Y. Miao and B. X. Zhao, Through-bond energy transfer-based ratiometric fluorescent probe for the imaging of HOCl in living cells, Sens. Actuators, B, 2017, 244, 907–913 CrossRef CAS
.
- J. Liu, P. Niu, Y. Rong, W. Chen, X. Liu, L. Wei and X. Song, A phenothiazine coumarin based
ratiometric fluorescent probe for real-time detection of lysosomal hypochlorite in living cell and zebra fish, Spectrochim. Acta, Part A, 2021, 261, 120024 CrossRef CAS PubMed
.
- D. Q. Rich, J. Schwartz, M. A. Mittleman, M. Link, H. Luttmann-Gibson, P. J. Catalano, F. E. Speizer and D. W. Dockery, Association of short-term ambient air pollution concentrations and ventricular arrhythmias, Am. J. Epidemiol., 2005, 161, 1123 CrossRef PubMed
.
- T.-M. Chen, W. G. Kuschner, J. Gokhale and S. Shofer, Outdoor air pollution: nitrogen dioxide, sulfur dioxide, and carbon monoxide health effects, Am. J. Med. Sci., 2007, 333, 249 CrossRef PubMed
.
- J. Chao, Y. Zhang, H. Wang, Y. Zhang, F. Huo, C. Yin, L. Qin and Y. Wang, Fluorescent Red GK as a fluorescent probe for selective detection of bisulfite anions, Sens. Actuators, B, 2013, 188, 200–206 CrossRef CAS
.
- Y.-Q. Sun, J. Liu, J. Zhang, T. Yang and W. Guo, Fluorescent probe for biological gas SO2 derivatives bisulfite and sulfite, Chem. Commun., 2013, 49, 2637 RSC
.
- U. Tamima, M. Santra, C. W. Song, Y. J. Reo and K. H. Ahn, A Benzopyronin-Based Two-Photon Fluorescent Probe for Ratiometric Imaging of Lysosomal Bisulfite with Complete Spectral Separation, Anal. Chem., 2019, 91, 10779–10785 CrossRef CAS PubMed
.
- V. S. Lin, W. Chen, M. Xian and C. J. Chang, Chemical probes for molecular imaging and detection of hydrogen sulfide and reactive sulfur species in biological systems, Chem. Soc. Rev., 2015, 44, 4596–4618 RSC
.
- B. Arunachalam, U. T. Phan, H. J. Geuze and P. Cresswell, Enzymatic reduction of disulfide bonds in lysosomes: Characterization of a Gamma-interferon-inducible lysosomal thiol reductase (GILT), Proc. Natl. Acad. Sci. U. S. A., 2000, 97, 745–750 CrossRef CAS PubMed
.
- U. Tamima, C. W. Song, M. Santra, Y. J. Reo, H. Bannac, M. R. Islamd and K. H. Ahna, A benzo[b]xanthene-derived fluorescent probe capable of two-photon ratiometric imaging of lysosomal cysteine with high specificity, Sens. Actuators, B, 2020, 322, 128588 CrossRef CAS
.
- J. Zhang, R. Wang, Z. Zhu, L. Yi and Z. Xi, A FRET-based ratiometric fluorescent probe for visualizing H2S in lysosomes, Tetrahedron, 2015, 71, 8572–8576 CrossRef CAS
.
- P. Verwilst, K. Sunwoo and J. S. Kim, The role of copper ions in pathophysiology and fluorescent probes for the detection thereof, Chem. Commun., 2015, 51, 5556 RSC
.
- S. G. Kaler, ATP7A-related copper transport diseases-emerging concepts and future trends, Nat. Rev. Neurol., 2011, 7, 15 CrossRef CAS PubMed
.
- S. Lutsenko, A. Gupta, J. L. Burkhead and V. Zuzel, Cellular multitasking: the dual role of human Cu-ATPases in cofactor delivery and intracellular copper balance, Arch. Biochem. Biophys., 2008, 476, 22 CrossRef CAS PubMed
.
- D. G. Barceloux, Copper, Clin. Toxicol., 1999, 37, 217 CAS
.
- C. Liu, X. Jiao, S. He, L. Zhao and X. Zeng, A highly selective and sensitive fluorescent probe for Cu2+ based on a novel naphthalimide–rhodamine platform and its application in live cell imaging, Org. Biomol. Chem., 2017, 15, 3947 RSC
.
- V. Dujols, F. Ford and A. W. Czarnik, A Long-Wavelength Fluorescent Chemodosimeter Selective for Cu(II) Ion in Water, J. Am. Chem. Soc., 1997, 119, 7386–7387 CrossRef CAS
.
- W.-N. Wu, H. Wu, R.-B. Zhong, Y. Wang, Z.-H. Xu, X.-L. Zhao, Z.-Q. Xu and Y.-C. Fan, Ratiometric fluorescent probe based on pyrrole-modified rhodamine 6G hydrazone for the imaging of Cu2+ in lysosomes, Spectrochim. Acta, Part A, 2019, 212, 121–127 CrossRef CAS PubMed
.
- Y. Wang, L. Zhang, X. Han, L. Zhang, X. Wang and L. Chen, Fluorescent probe for mercury ion imaging
analysis: strategies and applications, Chem. Eng. J., 2021, 406, 127166 CrossRef CAS
.
- Y. Zhang, H. Chen, D. Chen, D. Wu, Z. Chen, J. Zhang, X. Chen, S. H. Liu and J. Yin, A colorimetric and ratiometric fluorescent probe for mercury (II) in lysosome, Sens. Actuators, B, 2016, 224, 907–914 CrossRef CAS
.
- Q. Xia, X. Wang, Y. Liu, Z. Shen, Z. Ge, H. Huang, X. Li and Y. Wang, An endoplasmic reticulum-targeted two-photon fluorescent probe for bioimaging of HClO generated during sleep deprivation, Spectrochim. Acta, Part A, 2020, 229, 117992 CrossRef CAS PubMed
.
- M. Yan, H. Fang, X. Wang, J. Xu, C. Zhang, L. Xu and L. Li, A two-photon fluorescent probe for visualizing endoplasmic reticulum peroxynitrite in Parkinson's disease models, Sens. Actuators, B, 2021, 328, 129003 CrossRef CAS
.
- H. Xiao, P. Li, X. Hu, X. Shi, W. Zhang and B. Tang, Simultaneous fluorescence imaging of hydrogen peroxide in mitochondria and endoplasmic reticulum during apoptosis, Chem. Sci., 2016, 7, 6153–6159 RSC
.
- C. Gao, Y. Tian, R. Zhang, J. Jing and X. Zhang, Endoplasmic Reticulum-Directed Ratiometric Fluorescent Probe for quantitative Detection of Basal H2O2, Anal. Chem., 2017, 89, 12945–12950 CrossRef CAS PubMed
.
- L. Yuan, W. Lin, J. Song and Y. Yang, Development of an ICT-based ratiometric fluorescent hypochlorite probe suitable for living cell imaging, Chem. Commun., 2011, 47, 12691–12693 RSC
.
- G. Li, Q. Lin, L. Sun, C. Feng, P. Zhang, B. Yu, Y. Chen, Y. Wen, H. Wang, L. Ji and H. Chao, A mitochondrial targeted two-photon iridium(III) phosphorescent probe for selective detection of hypochlorite in live cells and in vivo, Biomaterials, 2015, 53, 285–295 CrossRef CAS PubMed
.
- H. Feng, Z. Zhang, Q. Meng, H. Jia, Y. Wang and R. Zhang, Rapid Response Fluorescence Probe Enabled In Vivo Diagnosis and Assessing Treatment Response of Hypochlorous Acid-Mediated Rheumatoid Arthritis, Adv. Sci., 2018, 5, 1800397 CrossRef PubMed
.
- S. Xu, H.-W. Liu, X.-X. Hu, S.-Y. Huan, J. Zhang, Y.-C. Liu, L. Yuan, F.-L. Qu, X.-B. Zhang and W. Tan, Visualization of Endoplasmic Reticulum Aminopeptidase 1 under Different Redox Conditions with a Two-Photon Fluorescent Probe, Anal. Chem., 2017, 89, 7641–7648 CrossRef CAS PubMed
.
- Q. Ma, C. Wang, Y. Bai, J. Xu, J. Zhang, Z. Li and X. Guo, A lysosome-targetable and ratiometric fluorescent probe for hypochlorous acid in living cells based on a 1,8-naphthalimide derivative, Spectrochim. Acta, Part A, 2019, 223, 117334 CrossRef CAS PubMed
.
- Z. Guo, I. Shin and J. Yoon, Recognition and sensing of various species using boronic acid derivatives, Chem. Commun., 2012, 48, 5956–5967 RSC
.
- X. Sun, Q. Xu, G. Kim, S. E. Flower, J. P. Lowe, J. Yoon, J. S. Fossey, X. Qian, S. D. Bull and T. D. James, A water-soluble boronate-based fluorescent probe for the selective detection of peroxynitrite and imaging in living cells, Chem. Sci., 2014, 5, 3368–3373 RSC
.
- Q. Xu, K.-A. Lee, S. Lee, K. M. Lee, W.-J. Lee and J. Yoon, A Highly Specific Fluorescent Probe for Hypochlorous Acid and Its Application in Imaging Microbe-Induced HOCl Production, J. Am. Chem. Soc., 2013, 135, 9944–9949 CrossRef CAS PubMed
.
- H. Li, Q. Yao, J. Fan, J. Du, J. Wang and X. Peng, A two-photon NIR-to-NIR fluorescent probe for imaging hydrogen peroxide in living cells, Biosens. Bioelectron., 2017, 94, 536–543 CrossRef CAS PubMed
.
- R. D. Hanna, Y. Naro, A. Dieters and P. E. Floreancig, Alcohol, Aldehyde, and Ketone Liberation and Intracellular Cargo Release through Peroxide-Mediated α-Boryl Ether Fragmentation, J. Am. Chem. Soc., 2016, 138, 13353–13360 CrossRef CAS PubMed
.
- Z. F. Chen, B. Zhao, X. Y. Tang, W. Li, L. L. Zhu, C. S. Tang, J. B. Du and H. F. Jin, Red yeast rice prevents atherosclerosis through regulating inflammatory signaling pathways, Chin. Med. J., 2011, 124, 3460–3467 CAS
.
- R. Ying, X. Q. Wang, Y. Yang, Z. J. Gu, J. T. Mai, Q. Qiu, Y. X. Chen and J. F. Wang, Hydrogen sulfide suppresses endoplasmic reticulum stress-induced endothelial-to-mesenchymal transition through Src pathway, Life Sci., 2016, 144, 208–217 CrossRef CAS PubMed
.
- W. Shu, S. Zang, C. Wang, M. Gao, J. Jing and X. Zhang, An Endoplasmic Reticulum-Targeted Ratiometric Fluorescent Probe for the Sensing of Hydrogen Sulfide in Living Cells and Zebrafish, Anal. Chem., 2020, 92, 9982–9988 CrossRef CAS PubMed
.
- W. Yu, H. Jin, C. Tang, J. Du and Z. Zhang, Sulfur-containing gaseous signal molecules, ion channels and cardiovascular diseases, Br. J. Pharmacol., 2018, 175, 1114–1125 CrossRef CAS PubMed
.
- M. Niu, Y. Han, Q. Li and J. Zhang, Endogenous sulfur dioxide regulates hippocampal neuron apoptosis in developing epileptic rats and is associated with the PERK signaling pathway, Neurosci. Lett., 2018, 665, 22–28 CrossRef CAS PubMed
.
- Z.-Y. Li, X.-L. Cui, Y.-H. Yan, Q.-L. Che, J.-Y. Miao, B.-X. Zhao and Z.-M. Lin, A novel endoplasmic reticulum-targeted ratiometric fluorescent probe based on FRET for the detection of SO2 derivatives, Dyes Pigm., 2021, 188, 109180 CrossRef CAS
.
- J. A. Cotruvo, A. T. Aron, K. M. Ramos-Torres and C. J. Chang, Synthetic fluorescent probes for studying copper in biological systems, Chem. Soc. Rev., 2015, 44, 4400 RSC
.
- H. Hayashi, A. Hattori, Y. Tatsumi, K. Hayashi, Y. Katano, J. Ueyama, S. Wakusawa, M. Yano and H. Goto, Various copper and iron overload patterns in the livers of patients with Wilson disease and idiopathic copper toxicosis, Med. Mol. Morphol., 2013, 46, 133 CrossRef PubMed
.
- S. Y. Park, W. Kim, S.-H. Park, J. Han, J. Lee, C. Kang and M. H. Lee, An endoplasmic reticulum-selective ratiometric fluorescent probe for imaging a copper pool, Chem. Commun., 2017, 53, 4457–4460 RSC
.
- A. Deschamps, A.-S. Colinet, O. Zimmermannova, H. Sychrova and P. Morsomme, A new pH probe localized in the Golgi apparatus of Saccharomyces cerevisiae reveals unexpected roles of Vph1p and Stv1p isoforms, Sci. Rep., 2020, 10, 1881 CrossRef CAS PubMed
.
- M. M. Wu, J. Llopis, S. Adams, J. M. McCaffery, M. S. Kulomaa, T. E. Machen, H.-P. H. Moore and R. Y. Tsien, Organelle pH studies using targeted avidin and fluorescein-biotin, Chem. Biol., 2000, 7, 197 CrossRef CAS PubMed
.
- S. Bernales, S. Schuck and P. Walter, ER-phagy: selective autophagy of the endoplasmic reticulum, Autophagy, 2007, 3, 285 CrossRef PubMed
.
- R. Sano and J. C. Reed, ER stress-induced cell death mechanisms, Biochim. Biophys. Acta, 2013, 1833, 3460 CrossRef CAS PubMed
.
- L. He, B. Dong, Y. Liu and W. Lin, Fluorescent chemoprobes manipulated by dual/triple interplaying sensing mechanisms, Chem. Soc. Rev., 2016, 45, 6449 RSC
.
- M. A. Ruby, J. Massart, D. M. Hunerdosse, M. Schonke, J. C. Correia, S. M. Louie, J. L. Ruas, E. Naslund, D. K. Nomura and J. R. Zierath, Human Carboxylesterase 2 Reverses Obesity-Induced Diacylglycerol Accumulation and Glucose Intolerance, Cell Rep., 2017, 18, 636–646 CrossRef CAS PubMed
.
- Y. Li, M. Zalzala, K. Jadhav, Y. Xu, T. Kasumov, L. Yin and Y. Zhang, Carboxylesterase 2 prevents liver steatosis by modulating lipolysis, endoplasmic reticulum stress, and lipogenesis and is regulated by hepatocyte nuclear factor 4 alpha in mice, Hepatology, 2016, 63, 1860–1874 CrossRef CAS PubMed
.
- X. Tian, F. Yan, J. Zheng, X. Cui, L. Feng, S. Li, L. Jin, T. D. James and X. Ma, Endoplasmic Reticulum Targeting Ratiometric Fluorescent Probe for Carboxylesterase 2 Detection in Drug-Induced Acute Liver Injury, Anal. Chem., 2019, 91, 15840–15845 CrossRef CAS PubMed
.
- X. Li, J. Yu, L. Gong, Y. Zhang, S. Dong, J. Shi, C. Li, Y. Li, Y. Zhang and H. Li, Heme oxygenase-1(HO-1) regulates Golgi stress and attenuates endotoxin-induced acute lung injury through hypoxia inducible factor-1α (HIF-1α)/HO-1 signaling pathway, Free Radical Biol. Med., 2021, 165, 243–253 CrossRef CAS PubMed
.
- F. Coceani, L. Kelsey, E. Seidlitz, G. S. Marks, B. E. McLaughlin, H. J. Vreman, D. K. Stevenson, M. Rabinovitch and C. Ackerley, Carbon monoxide formation in the ductus arteriosus in the lamb: implications for the regulation of muscle tone, Br. J. Pharmacol., 1997, 120, 599–608 CrossRef CAS PubMed
.
- X. Wang, Y. Wang, H. P. Kim, K. Nakahira, S. W. Ryter and A. M. K. Choi, Carbon Monoxide Protects against Hyperoxia-induced Endothelial Cell Apoptosis by Inhibiting Reactive Oxygen Species Formation, J. Biol. Chem., 2007, 282, 1718–1726 CrossRef CAS PubMed
.
- H. Wang, Z. He, Y. Yang, J. Zhang, W. Zhang, W. Zhang, P. Li and B. Tang, Ratiometric fluorescence imaging of Golgi H2O2 reveals a correlation between Golgi oxidative stress and hypertension, Chem. Sci., 2019, 10, 10876 RSC
.
- S. Seng, Z. Zheng, S. Gong and G. Feng, A unique probe enables labeling cell membrane and Golgi apparatus and tracking peroxynitrite in Golgi oxidative stress and drug-induced liver injury, Sens. Actuators, B, 2022, 136, 131751 Search PubMed
.
- Z. Zheng, S. Feng, S. Gong and G. Feng, Golgi-targetable fluorescent probe for ratiometric imaging of CO in cells and zebrafish, Sens. Actuators, B, 2021, 347, 130631 CrossRef CAS
.
- X. Rong, C. Liu, M. Li, H. Zhu, Y. Zhang, M. Su, X. Wang, X. Li, K. Wang, M. Yu, W. Sheng and B. Zhu, An Integrated Fluorescent Probe for Ratiometric Detection of Glutathione in the Golgi Apparatus and Activated Organelle Targeted Therapy, Anal. Chem., 2021, 93, 16105–16112 CrossRef CAS PubMed
.
- S.-S. Wang, S.-Y. Du, X. He, Y.-M. Qi, X.-L. Li, R.-X. Rong, Z.-R. Cao and K.-R. Wang, Nucleus-targeting imaging and enhanced cytotoxicity based on naphthalimide derivatives, Bioorg. Chem., 2021, 115, 105188 CrossRef CAS PubMed
.
- C.-G. Dai, J.-L. Wang, Y.-L. Fu, H.-P. Zhou and Q.-H. Song, Selective and Real-Time Detection of Nitric Oxide by a Two-Photon Fluorescent Probe in Live Cells and Tissue Slices, Anal. Chem., 2017, 89, 10511–10519 CrossRef CAS PubMed
.
- C. Li, W.-J. Tang, W. Feng, C. Liu and Q.-H. Song, A rapid-response and ratiometric fluorescent probe for nitric oxide: From the mitochondria to the nucleus in live cells, Anal. Chim. Acta, 2020, 1096, 148–158 CrossRef CAS PubMed
.
- W. Sun, J.-X. Cui, L.-L. Ma, Z.-L. Lu, B. Gong, L. He and R. Wang, Imaging nucleus viscosity and G-quadruplex DNA in living cells using a nucleus-targeting two-photon fluorescent probe, Analyst, 2018, 143, 5799 RSC
.
- K.-N. Wang, L.-Y. Liu, D. Mao, S. Xu, C.-P. Tan, Q. Cao, Z.-W. Mao and B. Liu, A Polarity-Sensitive Ratiometric Fluorescence Probe for Monitoring Changes in Lipid Droplets and Nucleus during Ferroptosis, Angew. Chem., Int. Ed., 2021, 60, 15095 CrossRef CAS PubMed
.
- F. Yang, C. Wang, L. Wang, Z.-W. Ye, X.-B. Song and Y. Xiao, Hoechst-naphthalimide dyad with dual emissions as specific and ratiometric probe for nucleus DNA damage, Chin. Chem. Lett., 2017, 28, 2019–2022 CrossRef CAS
.
- J. Bucevicius, J. Keller-Findeisen, T. Gilat, S. W. Hell and G. Lukinavicius, Rhodamine–Hoechst positional isomers for highly efficient staining of heterochromatin, Chem. Sci., 2019, 10, 1962–1970 RSC
.
- S. Staubach and F.-G. Hanisch, Lipid rafts: signaling and sorting platforms of cells and their roles in cancer, Expert Rev. Proteomics, 2011, 8, 263–277 CrossRef CAS PubMed
.
- P. Sengupta, A. Y. Seo, H. A. Pasolli, Y. E. Song, M. C. Johnson and J. Lippincott-Schwartz, A lipid-based partitioning mechanism for selective incorporation of proteins into membranes of HIV particles, Nat. Cell Biol., 2019, 21, 452–461 CrossRef CAS PubMed
.
- S. T. Yang, A. J. B. Kreutzberger, V. Kiessling, B. K. Ganser-Pornillos, J. M. White and L. K. Tamm, HIV virions sense plasma membrane heterogeneity for cell entry, Sci. Adv., 2017, 3, 1700338 CrossRef PubMed
.
- G. Di Paolo and T. W. Kim, Linking lipids to Alzheimer's disease: cholesterol and beyond, Nat. Rev. Neurosci., 2011, 12, 284–296 CrossRef CAS PubMed
.
- C. L. Schengrund, Lipid rafts: Keys to neurodegeneration, Lipid rafts: Keys to neurodegeneration, Brain Res. Bull., 2010, 82, 7–17 CrossRef CAS PubMed
.
- T. Zhang, L. Li, F. Huo, W. Zhang, J. Chao and C. Yin, Alkyl chain regulated cytomembrane and mitochondria targeting fluorescent probes for ratiometric sensing SO2 and its bio-application in mice model, Sens. Actuators, B, 2021, 342, 130041 CrossRef CAS
.
- S. Sigismund, S. Confalonieri, A. Ciliberto, S. Polo, G. Scita and P. P. Di Fiore, Endocytosis and signaling: cell logistics shape the eukaryotic cell plan, Physiol. Rev., 2012, 92, 273–366 CrossRef CAS PubMed
.
- J. R. Casey, S. Grinstein and J. Orlowski, Probes and regulators of intracellular pH, Nat. Rev. Mol. Cell Biol., 2010, 11, 50–61 CrossRef CAS PubMed
.
- X. Liu, Y. Su, H. Tian, L. Yang, H. Zhang, X. Song and J. W. Foley, Ratiometric Fluorescent Probe for Lysosomal pH Measurement and Imaging in Living Cells Using Single-Wavelength Excitation, Anal. Chem., 2017, 89, 7038–7045 CrossRef CAS PubMed
.
- S. Michelis, L. Danglot, R. Vauchelles, A. S. Klymchenko and M. Collot, Imaging and Measuring Vesicular Acidification with a Plasma Membrane-Targeted Ratiometric pH Probe, Anal. Chem., 2022, 94, 5996–6003 CrossRef CAS PubMed
.
- X. Kong, J. Yin, M. Li, L. Zhu, B. Dong, Y. Ma and W. Lin, Simultaneously imaging of SO2 in lysosomes and mitochondria based on a dual organelle-targeted fluorescent probe, Sens. Actuators, B, 2019, 292, 80–87 CrossRef CAS
.
- V. Juvekar, S. J. Park, J. Yoon and H. M. Kim, Recent progress in the two-photon fluorescent probes for metal ions, Coord. Chem. Rev., 2021, 427, 213574 CrossRef CAS
.
- S. I. Reja, M. Minoshima, Y. Hori and K. Kikuchi, Near-infrared fluorescent probes: a next-generation tool for protein-labeling applications, Chem. Sci., 2021, 12, 3437 RSC
.
|
This journal is © The Royal Society of Chemistry 2023 |