DOI:
10.1039/D3QI00193H
(Review Article)
Inorg. Chem. Front., 2023,
10, 4322-4357
Magnetic materials based on heterometallic CrII/III–LnIII complexes
Received
31st January 2023
, Accepted 28th May 2023
First published on 31st May 2023
Abstract
Accommodating disparate spin centres within a single ensemble is a valuable synthetic strategy to obtain interesting molecular magnetic materials. 3d–4f heterometallic complexes are one such subclass that has been extensively explored for the last three decades owing to their interesting magnetic properties. The primary reason is that the bridging ligand mediates magnetic super-exchange between 3d and 4f ions to considerably reduce the prevalent quantum tunnelling of magnetisation (QTM) in such complexes. Among the 3d–4f family, CrIII–LnIII assemblies nicely demonstrate the pivotal role played by strong exchange interactions between these two individual spin carriers to engender interesting single-molecule magnet (SMM) properties. In fact, this part of heterometallic chemistry has flourished greatly in the last decade despite its late development. In this review we provide an extensive synthetic, structural, magnetic, and magnetocaloric description of various CrIII–LnIII complexes along with their fundamental mechanisms of exchange interaction. Finally, we provide some possible solutions to improve the SMM properties of the CrIII–LnIII and other heterometallic complexes.
1. Introduction
In the last few decades, the single-molecule magnet (SMM) field has drawn increasing attention and started blossoming since the seminal discovery by Christou and co-workers in 1993 that magnetic memory can be entangled within a discrete mixed-valence complex [MnIII8MnIV4O12(OAc)16(H2O)4]·2AcOH·4H2O (1), famously known as Mn12ac.1,2 This example launched an entirely new research direction in the molecular magnetism field, which soon turned from a solely coordination chemistry context into the centrepiece theme of a multidisciplinary research field.3–5 The SMM can be defined as a discrete coordination or organometallic molecular entity that can retain its magnetization even in the absence of an external dc magnetic field below a certain temperature. Unlike conventional bulk magnets, they behave as quantized systems, and their magnetization dynamics are solely of molecular origin instead of a collective phenomenon.6,7 These molecules are characterized by two important parameters: the first is the energy barrier for magnetization reversal (Ueff), which is related to the axial zero-field splitting parameter (ZFS), D and total ground-state spin, S through the equation Ueff = S2|D| for integer and
for half-integer spin systems (Fig. 1). The second is the blocking temperature (TB) below which these complexes show open hysteresis in the magnetization (M) vs. field (H) plot, a direct signature of permanent magnet behaviour. Moreover, these nanomagnets also exhibit interesting physics, such as quantum tunnelling of magnetization (QTM), quantum phase interference (QIP), etc.,8–10 which endows them with the potential to be promising candidates for numerous potential technological applications such as high-density data storage device fabrication, quantum computation, and molecular spintronic materials.4,11–13 Therefore, harnessing a system with TB above room temperature is the holy grail of the molecular magnetism field. Based on these backgrounds, varieties of molecular complexes have been synthesized; depending upon composition, they can be divided into three major subcategories: mono or polynuclear 3d transition metal complexes,14–21 mononuclear or polynuclear rare earth 4f complexes22–31 and heterometallic 3d–4f32–40 or 4d–4f complexes.41,42 Initially, the main quest in this field was to harness high nuclearity complexes composed of paramagnetic first-row transition metal ions (MnII/III, FeII/III, CoII, and NiII),15,43 inspired by some of the groundbreaking works reported earlier in the literature such as {MnIII8MnIV4},1 {MnIIMnIII7MnIV4},44 {FeIII8},45 {FeIII19},46 {FeIII9},47 and {VIII4}.46,48,49–52 The main objective was to maximize the total ground state spin (S) as the formula for the thermal energy barrier (Ueff) to magnetization-reversal has quadratic dependence on S, mentioned earlier (vide supra). However, later it was conceived from the combination of various systematic experimental investigations and multi-reference ab initio calculations that the incorporation of a large number of paramagnetic metal ions in a single molecule to maximize the thermal energy barrier by enhancing the total ground state S might be counterproductive. This is because the uniaxial magneto-anisotropy parameter (D) usually shares an inverse relationship with S2, making Ueff virtually invariant to S.53–55 As a proof of principle, a Mn19 complex [MnIII12MnII7(μ4-O)8(μ3,η1-N3)8(HL1)12(MeCN)6]Cl2 (2) possesses a remarkably large ground state spin of 83/2, but surprisingly did not show any SMM behaviour due to its overall negligible anisotropy.56 On the contrary, interesting SMM behaviour can be retrieved successfully just by invoking a highly anisotropic DyIII ion through the replacement of the central isotropic MnII centre in the resultant complex [MnIII12MnII6DyIII(μ4-O)8(μ3-Cl)6.5(μ3-N3)1.5(HL1)12(MeOH)6]Cl3 (3) [L1H3 = 2,6-bis(hydroxymethyl)-4-methylphenol].57 Subsequently, the LnIII-based SMM field has started blooming since the seminal discovery of double-decker mononuclear phthalocyanine (Pc) lanthanide complexes [LnIIIPc2]n (LnIII = Tb, Dy, Ho; H2Pc = phthalocyanine; n = −1, 0, +1) (4), by the Ishikawa group, portraying interesting SMM behaviour.58 The heterometallic 3d–4f-based SMM chemistry began with the report of the tetranuclear {CuII2TbIII2} (Ueff = 14.7 cm−1; TB = 1.2 K) complex by Osa and co-workers in 2004.59 From then, a plethora of such complexes appeared in the literature, mainly composed of first-row transition metals such as CoII, MnIII, NiII and CuII ions, primarily due to the significant molecular anisotropy in their various coordination geometries, either through first (CoII ion), second-order spin orbit coupling (NiII ion) or via Jahn–Teller distortion (MnIII octahedral).19,21,60–66 In fact, the chemistry of such a mixed 3d–4f class of complexes had been further extended to earlier transition metals such as CrIII ion, and CrIII–LnIII-based SMM chemistry witnessed considerable growth in the last decade. Up to now, using various multidentate chelating or open-arm flexible ligand systems, more than forty Cr–Ln heterometallic complexes of varying nuclearities (between 2–16) have been isolated.
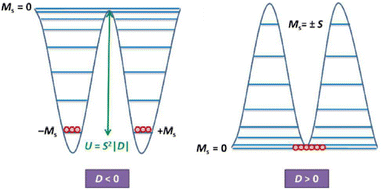 |
| Fig. 1 Double-well energy diagram for a SMM system where spin is a good quantum number to define the system.19 Reprinted with permission from ref. 19. Copyright 2016 Royal Society of Chemistry. | |
The typical examples are {CrLn2},67,68{Cr2-Ln2},69,70{Cr2Ln3},71,72{Cr4Ln},73 {Cr2Ln4},74,75 {Cr3Ln3},76,77 {Cr4Ln4},78–80 {Cr3Ln6},81 {Cr6Ln6}82 and {Cr8Ln8}.83 All these compounds unveiled interesting magnetic properties, and some even turned out to have excellent SMMs. Despite such a significant number of reports of CrIII–LnIII-based complexes with intriguing magnetic properties, to date not a single review has been dedicated to this particular field of coordination chemistry. Therefore, it is high time to deliver a meaningful comprehensive literature review based on these heterometallic assemblies, which eventually will help the readers to have a clear understanding regarding the various aspects of the magnetic properties of these complexes. We strongly believe that this kind of account will set the tone for further augmenting this interesting subcategory of heterometallic SMM chemistry with its full potential in forthcoming days. To do so, we describe this review in three parts: (i) the importance of 3d–4f magnetic exchange interaction in articulating the SMM behaviour with particular emphasis on the mechanism of CrIII–LnIII exchange coupling; (ii) the origin of magnetic anisotropy and role played by both CrII and CrIII ions in the synthesis of SMMs; and finally (iii) detailed synthetic and structural aspects of different heterometallic Cr–Ln-based complexes of varying structural topologies reported to date, with a major focus on elaborative discussion of magneto-structural correlations including the SMM behaviour and magnetocaloric effect.
2. General mechanism of CrIII-4f magnetic exchange interactions
In general, 3d–4f magnetic exchange plays a vital role in mitigating the low-temperature QTM (quantum tunnelling of magnetization) process by which a molecule demagnetizes fast upon penetrating through the imposed energy barrier via ground or low-lying excited states (thermal-assisted tunnelling).23,84 Therefore, it is necessary to have a discussion on the mechanism of magnetic exchange and its overwhelming influence in augmenting the SMM performance of various 3d–4f coordination complexes. From the experimental point of view, appropriate calculation and analysis of intramolecular magnetic exchange coupling constants is tremendously difficult for systems containing 4f ions due to the presence of large magnetic anisotropy and weak exchange interactions arising from the high degree of localization of 4f orbitals. However, the magnetically isotropic GdIII ion (f7) provides a much easier way to evaluate the exchange interactions in 3d-Gd systems, which do not have many complications from overparameterization, and finally to extend the conclusion to other isostructural 3d–4f complexes containing highly anisotropic LnIII ions. In 1985, the synthesis of the seminal heterometallic trinuclear complex {CuII2Gd} by Gatteschi and co-workers, employing a multidentate chelating Schiff base ligand N,N′-ethylene- bis (o-hydroxyacetophenoneiminato), showed strong CuII–GdIII ferromagnetic exchange interactions [JCu–Gd = 5.32(5) and 7.38(7) cm−1].85 In fact, the further assertion that the ferromagnetic interaction between CuII and GdIII ion is independent of the topology and nuclearity of the final complex opens the avenue to explore the magnetic properties of various other 3d–4f-based heterometallic complexes.32,60,65,66,86 Although the ferromagnetic interactions between {3d–4f} pairs are not intrinsic, several factors such as the nature of both 3d and 4f ions, coordination geometry and hence local symmetry around these ions, the nature and number of bridging ligands between the metal ions, and the dihedral angle etc., control the magnitude and sign of overall magnetic interaction.62,69,87–95 In fact, the sign of magnetic exchange interaction is crucial, since a ferromagnetic coupling surmounts a large ground state total spin (S) which is very important for constructing interesting SMMs and magnetic refrigerators. To understand the actual mechanism of such dominant ferromagnetic exchange interactions in the {CuII–GdIII} pair, several qualitative explanations have been proposed. Gatteschi suggested transfer of electron density from the 3dx2–y2 orbital of CuII to the 5d orbital of GdIII, while Kahn proposed the spin polarization mechanism where interaction takes place between the tails of the Cu 3d orbital with the empty Gd 6s orbital.96,97 They explained that the diffuse nature of 5d orbitals makes them likely to be delocalized on the bridging atoms and thereby mediates the exchange interactions. In order to get further insight into the general mechanism of exchange coupling in {CuII–4f}as well as other{3d–4f} pairs, Ruiz and Rajaraman's group independently documented a series of extensive CASSCF and DFT calculations on various heterometallic complexes.87,94,98,99 From their calculations, it is apparent that the overall magnetic exchange interactions (J3d−4f) can be considered as a cumulative effect of both ferro (JF) and antiferromagnetic (JAF) interactions involving both types of metal ions, i.e. JT = JF + JAF (Fig. 2). The antiferromagnetic (AFM) part (JAF) of JT arises from the direct non-vanishing overlap of magnetic orbitals between 4f and 3d ions of suitable symmetry, while the ferromagnetic (FM) contributions (JF) come from the combined effect of interactions between remaining orthogonal 4f and the magnetic 3d orbitals, and charge transfer (CT) transition from the σ-type d orbitals (dx2–y2 & dz2) of 3d metal ion to the empty 5d/6s/6p orbitals of lanthanides. Moreover, the 5d orbitals also get electronic contribution from the local 4f → 5d orbital excitation (Fig. 2). However, no CT takes place from π-type d orbitals such as dxy, dxz or dyz containing unpaired electrons. It is important to note that the level of electronic occupation of 5d orbitals plays a pivotal role in controlling the sign of the coupling constant, with larger occupancies leading to more ferromagnetic interaction in structurally related compounds. To get more information regarding the Cu–Ln magnetic interactions which are outside of the scope of this review, readers are recommended to go through the recent comprehensive review published by Chandrasekhar and co-workers.32
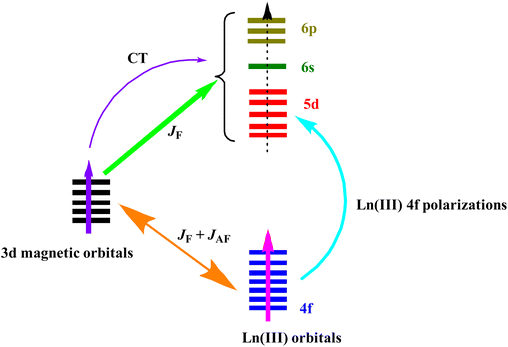 |
| Fig. 2 Schematic representation of probable general 3d–4f magnetic exchange interactions in heterometallic complexes.94 Reprinted with permission from ref. 94. Copyright 2016 American Chemical Society. | |
With this brief background, we shall consider the nature of {CrIII–LnIII} interactions, which is the focus of this review. Literature precedents showed that generally JCr(III)–Ln(III) interactions are antiferromagnetic, which is contrary to {CuII–Ln/GdIII} interactions (Table 2). In this regard, Rajaraman and co-workers provided a deeper understanding regarding the mechanism of coupling involved in {Cr–Gd} systems through their extensive calculation published in a couple of papers.71,100 From their calculations, it was very evident that unlike the late 3d transition metal ions, the lack of unpaired electron(s) in σ-type orbitals (dx2–y2 & dz2) completely attenuates the charge transfer from 3d orbital of CrIII to the 5d orbitals of GdIII ion, resulting in a significant reduction in the JF contribution. On the other hand, substantial JAF contributions come from the relatively strong direct overlap between the two sets of CrIII-3d and GdIII-4f magnetic orbitals. This agrees well with the overall CrIII–GdIII AFM interactions found in most of the CrIII–LnIII complexes. Furthermore, magnetostructural correlation had been developed by variation of ∠Cr–F–Gd bridging angle (130–180°) as a quality check parameter on a series of complexes to gain insight into the nature of magnetic exchange, which revealed that the magnitude of ferromagnetic JCr(III)–Gd(III) interaction decreases with increase in ∠Cr–F–Gd angle (Fig. 3), and in fact a switch from ferro- to antiferromagnetic interaction is predicted at a critical bridging angle of 138°.100
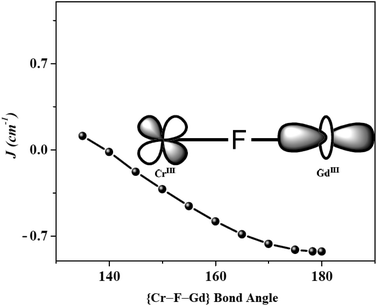 |
| Fig. 3 Angular dependence of J parameter on varying ∠Cr–F–Gd bridging angle.71,100 Reprinted with permission from ref. 117. Copyright 2013 Royal Society of Chemistry. | |
Such increase in antiferromagnetic interactions at a larger ∠Cr–F–Gd bridging angle was interpreted as follows: at a lower angle, there is a significant decrease in the CrIII–GdIII orbital direct overlap leading to less JAF contribution, whereas a moderate increase in spin densities on metal ions and concomitant proportional decrease in spin densities on bridging fluoride ions through charge transfer leads to predicted overall ferromagnetic coupling. On the other hand, at a larger angle, the orthogonal arrangement of the spin-bearing 3d orbitals of CrIII and the GdIII 5d-orbitals leads to a reduction in the ferromagnetic interaction and a concomitant increase in the antiferromagnetic coupling, resulting in an overall increase in JAF contribution.71,100 From the magnetostructural data in Table 1, it is also evident that the magnitude of {CrIII–LnIII} interaction in hydroxide or methoxide bridge complexes is somewhat stronger compared to the fluoride bridge complexes, presumably due to the short CrIII–LnIII distance or the low <Cr–O–Ln bridging angle.101
Table 1 Representative magneto-structural correlation data of some important CrIII–LnIII-based complexes
Complex |
Cr⋯Ln distance (Å) |
Bridging angle (°) |
J
Cr(III)–Ln(III) (cm−1) |
U
eff (cm−1) & TB(K) |
Ref. |
Theoretically calculated value.
Corresponding structural data for gadolinium are missing.
|
trans-CrF2(py)4Gd(hfac)4 (5) py = pyridine, hfac = hexafluroacetyalacetonatao |
Cr⋯Gd 4.253(6) |
<Cr−F−Gd = 178.32(39) |
J
Cr(III)–Gd(III) = −0.84(4)(−0.80)a |
— |
100
|
Cyclo-{mer-[CrF(μ-F)2 (py)3]Gd(hfac)3}2(6b) |
Cr⋯Tbb 4.154(8), 4.099(6) |
<Cr−F−Tbb = 156.21(5)° and 165.32(5) |
J
Cr(III)–Gd(III) = −0.57(7) |
— |
102
|
{fac-[Cr(μ-F)3(Me3tame)]2Gd3(hfac)6 (μ-F)3} (7) tame = 1,1,1-tris-((methylamino)methylethane) |
Cr⋯Gd 3.989(2) & 4.050(2) |
<Cr−F−Gd = 142.8(3)−146.6(3) |
J
Cr(III)–Gd(III) = −0.14(0) |
— |
103
|
[{CrF3(Me3tacn)}2Gd3F2(NO3)7(OH2)(CH3CN)] (8) Me3tacn = N,N′,N′′-trimethyl-1,4,7-triazacyclononane |
3.8895, 3.9815 & 4.0234 |
<Cr−F−Gd = 142.028,137.148 & 144.318 |
JCrIII–GdIII = +0.046 and +0.036 |
— |
71
|
[Cr2Dy2(OMe)2(O2CPh)4(mdea)2(NO3)2] (9) mdeaH2 = N-methyldiethanolamine |
Cr⋯Dy 3.3025 |
<Cr−O−Dy = 103.3010 & 95.809 |
JCrIII–DyIII = −20.5–26.0a |
54 & 3.5 |
70
|
[Cr4Dy4(μ3-OH)4 (μ-N3)4(mdea)4(piv)4] (10) |
Cr⋯Dy 3.3334 |
<Cr−O−Dy = 98.709 |
JCrIII–DyIII = −4.5 |
10.5 & 0.04 |
80
|
[Cr4Dy4(μ-F4)(μ3-OMe)1.25(μ3-OH)2.75(O2CPh)8(mdea)4] (11) |
Cr⋯Dy 3.30111 |
<Cr−O−Dy = 103.2716 & 96.6615 |
JCrIII–DyIII = −1.8 &−0.8b |
55 &3.5 |
78
|
{Et3NH[Cr2Gd3(OH)6(piv)10 (H2O)2]} (12)pivH = pivalic acid |
Cr⋯Gd 3.4537(15) & 3.4502(18) |
<Cr−O−Gd = 103.35(22), & 104.10(1) |
J
Cr(III)–Gd(III) = +0.215 |
— |
104
|
3. Origin of magnetic anisotropy and importance of CrII/III ion in fabricating Cr–Ln-based SMMs
Knowledge regarding the uniaxial magnetic anisotropy, which has a direct relationship with the ground state electronic structure of any paramagnetic metal ion under various ligand/crystal field environments, is very crucial and fundamental to fine tune the nature of SMMs. As far as heterometallic SMMs are concerned, literature precedents highlight that the initial development of Cr–Ln-based combinations was quite sluggish and sparse compared to other 3d metal counterparts such as MnIII,105–107 CoII,108–111 NiII,38 FeII/III,112–115 and CuII ion.32,86,116 In fact, the use of chromium as a constituent ion is more often than not overlooked despite the added advantage that the majority of chromium isotopes (50Cr, 52Cr, 54Cr, natural abundance more than 90.0%) are devoid of nuclear spin (I = 0), which contributes significantly to the electron–nucleus hyperfine interaction-mediated fast QTM efficiency.117,118 The various reasons for such sluggish and late development of Cr–Ln-based systems are: (i) the inert nature and rigid coordination characteristics of the Cr3+ion;79 (ii) this ion also shows weak anisotropy (the |D| values usually lie in the range of 0.2 to 0.5 cm−1)119 compared with late transition metal ions such as FeII, NiII, CoII ions due to isotropic electronic distribution; and (iii) the lack of unpaired electron in the σ-bonding dx2–y2 or dz2-orbital under various crystal field environments enables CrIII to be involved in intramolecular antiferromagnetic exchange interactions with the LnIII ions. Nonetheless, in 2010 Powell and co-workers successfully isolated heterometallic octanuclear complex [Cr4Dy4(μ3-OH)4(μ-N3)4(mdea)4(piv)4]·3CH2Cl2 (10) (H2mdea = methyldiethanolamine, PivH = pivalic acid) featuring a 4f-square in a 3d-square structural topology, which revealed interesting SMM behaviour with effective energy barrier (Ueff) = 10.5 cm−1 and open hysteresis loop up to ∼1 K (sweep rate 0.035 Ts−1).80 A couple of years later, a seminal report of the tetranuclear complex [CrIII2DyIII2(OMe)2(O2CPh)4(mdea)2(NO3)2] (9) by Murray and co-workers showed Ueff = 54 cm−1 and TB = 3.5 K (sweep rate 0.035 Ts−1). Detailed analysis of magnetic data revealed that strong antiferromagnetic interactions (JCr(III)–Dy(III) = −16.7 to −20.3 cm−1) exist between the DyIII and CrIII ions in 9 which are mainly of the collinear ising-type, i.e. the chromium spins are always aligned along the Z axis. The utmost importance of strong CrIII–LnIII exchange interaction was further nicely illustrated by the Rajaraman and Murray group together through the isolation of a couple of structurally similar complexes [CoIII4DyIII4(μ-F)4(μ3-OH)4(o-tol)8(mdea)4]·3H2O (13) and [CrIII4DyIII4(μ-F4)(μ3-OMe)1.25(μ3-OH)2.75(O2CPh)8(mdea)4] (11), where they have shown that purposeful replacement of the diamagnetic CoIII ion in 13 by a paramagnetic CrIII ion in 11 (JCr(III)–Dy(III) = −1.8 cm−1 by POLY_ANISO) has led to an increase in Ueff from 39.0 to 55.0 cm−1 along with the significant suppression of QTM at low temperature in the chromium analogue, with the observation of highly coercive magnetic hysteresis loops up to ∼2 K.78 These examples clearly highlight that the CrIII–LnIII magnetic exchange interaction alone is sufficient to engender interesting magnetic properties in Cr–Ln-based systems despite the isotropic/weakly anisotropic nature of the CrIII ion. Moreover, Long's group's seminal work on a series of mononuclear complexes of general formula [Cr(dmpe)2(CN)X](PF6) (X = Cl (14a), Br (14b), I (14c)), possessing CrIII ion in a pseudo-octahedral geometry, has shown that a considerable amount of axial ZFS (|D| = 0.11–2.30 cm−1) can be installed even within the CrIII ion through the spin–orbit coupling imparted by varying the coordinated ligands from lighter chlorine to heavier iodine atom.120 Based on those landmark reports, SMM chemists were enticed enough to embark on the voyage of exploring heterometallic assemblies based on the combination of a highly anisotropic 4f ion with CrIII in the quest for molecular systems possessing fascinating SMM properties. Consequently, to date, more than forty CrIII–LnIII-based complexes have been reported in the literature, and their synthetic methods, including their detailed magnetic properties, will be discussed in the subsequent sections. As far as the nature of bridging ligands is concerned, these complexes can be divided into two major subcategories: either single or doubly fluoride-bridged complexes of the general formula {CrIII–F–LnIII};71 or based on the oxygen atom-bridged complexes {CrIII–X–LnIII} (X = O, OH or OR),78,80 of which the latter group arguably contributes the most in this specific family of heterometallic assemblies.
On the other hand, literature precedents lend support that CrII ions often could be a good source of easy-axis anisotropy (D < 0) under various geometries such as octahedral, tetragonal pyramidal, seesaw and square planar121 (see also other references in Table 1), and hence could be a potential component for developing CrII–LnIII-based SMM systems. A qualitative d orbital splitting diagram along with the electron distribution of CrII ion in various geometries is shown in Fig. 4. A close inspection of the octahedral ligand field splitting of the free CrII ion revealed that a low-lying orbital ground doublet 5Eg and a high-lying orbital triplet 5T2g arise from the electronic configuration due to the promotion of an electron from t2g to eg. The 5Eg, being highly unstable towards Jahn–Teller distortion, undergoes tetragonal elongation and significantly contributes to the ZFS akin to the isoelectronic MnIII ion. In other geometries, the axial anisotropy arises presumably from ligand field symmetry-induced mixing of non-degenerate levels through second-order spin orbit coupling (SOC).62 A high-frequency/field electron paramagnetic resonance (HFEPR) spectroscopy measurement has been used to investigate the ZFS parameters of the CrII ion which divulged significant negative values of D in the range of −1.5 to −3.7 cm−1 (Table 2). Moreover, the presence of an unpaired electron in the σ-bonding dz2 orbital under various ligand field environments paves the way for ferromagnetic interaction with the LnIII ions through the spin-delocalization mechanism.87 Therefore, combining the CrII ion with highly anisotropic 4f metal ions such as DyIII and TbIII could be helpful to isolate heterometallic complexes with interesting SMM properties. Nonetheless, to date not a single CrII–LnIII-based system has been isolated, presumably due to the strong redox-unstable nature of the CrII with respect to oxidation towards CrIII under open atmospheric condition and the lack of an appropriate synthetic design.122
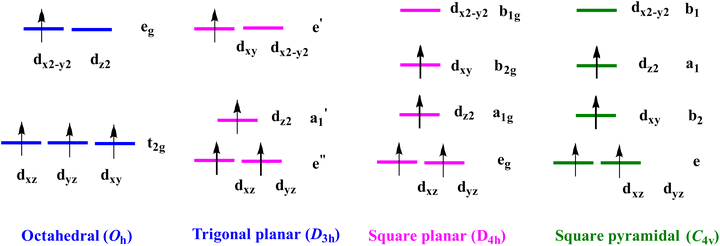 |
| Fig. 4 Qualitative d-orbital splitting energy diagram of CrII ion under various geometries reported in the literature. | |
Table 2 Axial (D) and rhombic (E) ZFS parameters of CrII ion in some representative complexes under different coordination number and geometries from HF-EPR data
Complex |
Coordination number |
Geometry (valence electronic configuration) |
D
exp (cm−1) |
E
exp (cm−1) |
Ref. |
Theoretical value from CASSCF/NEVPT2 calculations.
|
[K(18-crown-6) (Et2O)2] [Cr{N-(SiMe3)2}3] (15) |
3 |
Trigonal planar (e′′2a1′1e′1) |
−15.10a |
0.0453a |
123
|
[Cr(N(TMS)2)2(X)2] TMS = SiMe3, X = pyridine (16a) X = THF (16b) |
4 |
Square planar (eg2a1g1b2g1) |
−2.01 for 16a |
0.02 |
117
|
−2.54 for 16b |
0.01 |
Cr(RNC(CH3)NR)2 |
4 |
Square planar |
−1.74 |
0.07 |
124
|
R = iPr (17a) = Cy (17b) |
Square planar |
−1.82 |
0.09 |
(Cyclohexyl) = Dipp (17c) |
Seesaw |
−1.71 |
0.06 |
(2,6-Diisopropylphenyl) = tBu (17d) |
|
−1.94 |
0.03 |
[Cr5(tpda)4Cl2] (18) (H2tpda = N2,N6-di(pyridin-2-yl)-pyridine-2,6-diamine) |
5 |
Square-pyramidal (e2b21a11) |
−1.53 (SMM) |
0.0092 |
125
|
[Cr3(dpa)4Cl2] (19) Hdpa = dipyridin-2-ylamine |
5 |
Square-pyramidal |
−1.643 (SMM) |
0.034 |
126
|
Mo2Cr(dpa)4Cl2 (20) |
5 |
Square-pyramidal |
−2.187 (SMM) |
0.024 |
127
|
W2Cr(dpa)4Cl2 (21) |
5 |
Square-pyramidal |
−3.617 (SMM) |
0.0385 |
127
|
(NH4)2Cr(H2O)6(SO4)2 (22) |
6 |
Octahedral (t2g3eg1) |
−2.43 |
0.089 |
128
|
Cr(dmpe)2-(CN)X X = Cl (14a), I (14c) |
6 |
Pseudo-octahedral (t2g4) |
−3.97 (14a) and −6.26 (14c) |
— |
120
|
4. CrIII–LnIII complexes as magnetic refrigerants
In addition to their exciting magnetic properties, heterometallic CrIII–LnIII complexes have gained tremendous interest owing to the magnetocaloric effect (MCE), which forms the basis of molecular magnetic coolers.129–132 Molecular magnetic cooling is a kind of magneto-thermodynamic phenomenon that denotes the change in entropy (−ΔSmaxm) of a magnetically active substrate under a variable temperature and magnetic field. They have great application in refrigeration under ultralow temperature environments, and have great environmentally friendly advantages. For a large MCE value, complexes must possess a combination of a large-spin ground state, insignificant anisotropy (D = 0), high magnetic density, and weak ferromagnetic interaction.98 Recently, cryogenic magneto-refrigerants based on 3d-GdIII complexes formed with the rational incorporation of an isotropic 3d ion and GdIII ion (isotropic with the highest possible spin state of S = 7/2) have emerged as a promising candidate for enhancing the MCE value.133,134 Among the 3d metal ions, one of the best candidates may be the isotropic CrIII ion, with a maximum entropy of 222 J kg−1 K−1.135 However, due to the inert nature of the CrIII ion it becomes challenging to stitch it with LnIII ions to synthesize polynuclear complexes with a high MCE value. Thus, CrIII–LnIII complexes as magneto-refrigerants are seldom explored and need more investigation to harness the effective potential of these complexes.
Pedersen and group have demonstrated a few examples of the magnetocaloric effect through the use of fluoride-bridged CrIII–LnIII complexes, which lead to weak exchange interactions and show relevant magnetic entropy changes.71,100,102 Recently, Zhao and group have displayed a significant magnetocaloric effect in a ferromagnetic {CrIII2GdIII3} cluster having large magnetic entropy change of 27.0 J kg−1 K−1 at 4.0 K.104 Zhai and group have synthesized a 3-dimensional sulphate framework-based unit having a large MCE of −ΔSmaxm = 38.33 J kg−1 K−1 at 2 K.136 Shao and group have employed a building block strategy to generate a neutrally layered 2-dimensional metal coordination polymer consisting of an octanuclear {CrIII4GdIII4} metal cluster core and an organic ligand as a linker. The complex exhibits a large magnetic entropy of 28.8 J kg−1 K−1 at 3.5 K.137 Xu and group have constructed a {LnIII8CrIII4} motif with a one-dimensional chain structure displaying a magnetic entropy change of −ΔSmaxm = 23.40 J kg−1 K−1 at 3 K.138 Wen and group have reported the first example of an isolated 3D two-fold interpenetrating CrIII–GdIII framework using a carboxylate ligand having a large magnetocaloric effect of 39.86 J kg−1 K−1 at T = 2 K.135 There are substantially fewer polynuclear CrIII–LnIII compounds with giant MCE, such as {Cr4Ln},73 {Cr6Ln2},139 {Cr2Ln4},140 {Cr4Ln4},141 {Cr3Ln7},142 {Cr12Ln4},139 {Cr2Ln5},140 {Cr4Ln8}.140 Thus, CrIII–LnIII compounds could be a potential candidate for magnetic refrigerants with large magnetic entropy change; however, CrIII–LnIII compounds are rarely investigated, and thorough and detailed study is needed to establish their fruitfulness as magnetic coolers.
5. CrIII–LnIII heterometallic complexes of varying nuclearities
5.1 Dinuclear CrIII–LnIII complexes
The use of 2,2′-bipyridine ligand afforded two hydroxo-bridged iso-structural discrete dinuclear heterometallic CrIII/LnIII complexes [LnCr(bipy)2(μ-OH)2(H2O)6](ClO4)4·2H2O {Ln = Gd (23a) or Dy (23b)} by heating an aqueous solution of 2,2′-bipyridine and CrCl3 with a molar ratio of 1
:
1 followed by the addition of Ln(ClO4)3.143 Interestingly, it was found that the stoichiometry of the added lanthanide perchlorate salt had an impact on the nuclearity of the final outcome. The use of 24 equivalents of Ln(ClO4)3 yielded dinuclear [CrIIILnIII] (Ln = Gd (23a) and Dy (23b)) complexes, whereas 12 equivalents of Ln(ClO4)3 led to the formation of trinuclear [CrIII2LnIII] (Ln = Gd (24a), Dy (24b), and Tb (24c)) species (discussed in the next section).
Structural inspection showed two 2,2′-bipyridine ligand chelated with CrIII ion, two μ-OH anions bridges between GdIII and CrIII ions with a Gd⋯Cr distance of 3.417(1) Å and six terminal aqua ligands bound with GdIII to fulfill the distorted square antiprism geometry (Fig. 5). Magnetic data analysis revealed antiferromagnetic interaction between metal centers (Ln = GdIII (23a) and DyIII (23b)) with JCr–Gd = –0.102 cm−1.143
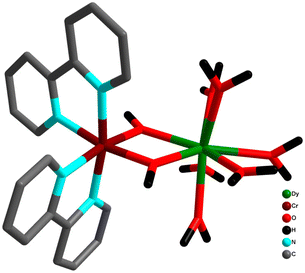 |
| Fig. 5 Molecular structure for the cationic core of 23. | |
The reaction of CrO3 and Gd2O3 in the presence of iminodiacetic acid (H2IDA) under hydrothermal conditions constructed a three-dimensional (3D) two-fold interpenetrated coordination polymer [CrIIIGd(IDA)2(C2O4)]∞ (25). Here the oxalate anion was generated in situ from the dicarboxylate ligand under hydrothermal conditions in the presence of an oxidant like CrO3.144,145
The dinuclear asymmetric unit consists of one GdIII ion and CrIII ion held together by two IDA2− anions and one oxalate anion (ox2−) anion. The GdIII ion is eight coordinated, surrounded by four O atoms from two ox2− anions and four O atoms of IDA2− anions, while the CrIII ion, six coordinated, forms a tetradentate metalloligand by the chelation of two IDA2− anions.
An interpenetrating 3D network is obtained by {Gd(ox)}∞ chains connected to metalloligands formed by adjacent GdIII ion and CrIII ions bridged by syn, anti carboxylate groups. Magnetic data analysis revealed the presence of antiferromagnetic interaction between the GdIII ion and CrIII ion. A large magnetocaloric value −ΔSmaxm = 39.86 J kg−1 K−1 suggests that the Cr–Gd complex could be a promising cryogenic magneto-refrigerant candidate.135
5.2 Trinuclear complexes
Trinuclear isostructural [CrIII2Ln] (Ln = Gd (24a), Dy (24b), or Tb (24c)) species consist of a CrLnCr core formed by linkage of each CrIII ion to LnIII ion with the aid of two μ-OH− groups. Similar to the complexes 23a and 23b, LnIII ions in all the complexes are eight coordinated, in a distorted square antiprism geometry and CrIII ions, six coordinated, distorted octahedral geometry (Fig. 6).143 The [Cr2Ln] core is not linear and angle ∠Cr–Gd–Cr is 111.27(4)° with an average Gd⋯Cr separation of 3.449(7) Å.
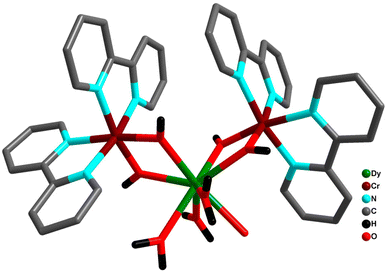 |
| Fig. 6 Molecular structure for the cationic core of 24. | |
Antiferromagnetic interaction predominates between CrIII and GdIII ions in complex 24a. AC susceptibility data reveal no frequency-dependent out-of-phase maxima for all the complexes.
Another example of a linear trinuclear complex is the fluoride-bridged asymmetric [Dy(hfac)3(H2O)–CrF2(py)4–Dy(hfac)3(NO3)] (26) cluster (Fig. 7).67 The structural parameters reveal that the angles ∠Cr–F–Dy and ∠Dy–Cr–Dy are 177.74(7)° and 177.80(5)°, respectively, with a Cr–Dy separation of 4.223(5) Å. The bond lengths associated with Cr–F and Dy–F of the bridging fluorides are as follows: bridging Cr–F lengths are significantly elongated [1.915(1) Å and 1.905(1) Å] compared to the non-bridging fluorides [1.844(2) Å and 1.845(2) Å] as in {CrIIIF2(py)4}+. The Dy–F distances (2.347(2) Å and 2.325(1) Å) are shorter compared to the Dy–O bond lengths ∼2.369(2) Å to 2.45(2) Å.
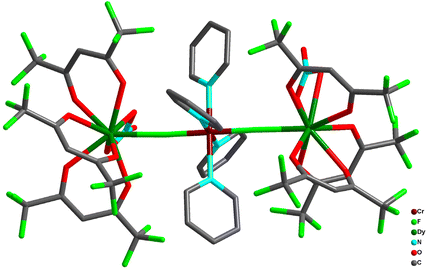 |
| Fig. 7 Molecular structure of 26. | |
Interestingly, a ferrimagnetic to a ferromagnetic arrangement of magnetic moments of both metal ions were recorded by X-ray magnetic circular dichroism (XMCD). Fitting XMCD data to a spin-Hamiltonian mode, a weak antiferromagnetic interaction of J = −0.18 cm−1 between both metal ions was obtained. In this context, it is important to mention that XMCD is a fantastic tool for the exact determination of exchange parameters even when the interaction is weak and strong anisotropy effects of the 4f ion are present.146 Detailed magnetic analysis reveals the SMM behavior of 26 with effective energy barrier of 3 cm−1. DFT calculations demonstrate the antiferromagnetic interaction between DyIII–CrIII ions originates from a superexchange mechanism via the fluoride bridge.67
A trinuclear methoxo-bridged complex [CrIIIDy2(OCH3)4(dpm)5(CH3OH)]·CH3OH (27) was synthesized from the reaction of CrCl3·(THF)3, DyCl3·CH3OH, (1,1,1-tris(hydroxymethyl)-ethane (H3L) and dipivaloymethane (dpm) ligand. The complex 27 has an overall star-shaped topology involving bridging methanol-methoxide units and beta-diketonates where a central CrIII ion is coordinated to three DyIII ion with the help of two tripodal trianionic ligand L3− (Fig. 8).
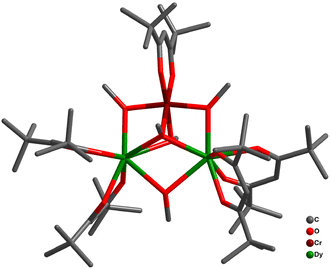 |
| Fig. 8 Molecular structure of complex 27. | |
The core consists of two μ3-methoxy bridges above and below the plane of metal ions, while the edges of the triangle are connected by μ-bridged two methoxide ligands that link between CrIII and DyIII ions. Another neutral methanol molecule participates in bridging two DyIII ions. To gain insight into the dynamics of magnetization, ac susceptibility measurements were performed revealing SMM behavior in zero dc field with effective energy barrier of 13.8 cm−1 and τ0 = 8.4 × 10−9 s.68
5.3 Tetranuclear complexes
Bendix and co-workers reported a series of isostructural tetranuclear molecular square complexes, [{CrIIIF2(NN)2}2{Ln(NO3)4}2] (Ln = Ce–Nd, Sm–Ho (28a–28i)) by the 1
:
1 reaction of Ln(NO3)3(aq.) with difluorido complex cis-[CrIIIF2(NN)2](NO3) [NN = 1,10-phenanthroline (phen) or 2,2′-bipyridine (bpy)]147 as building blocks in methanol (Scheme 1). In these complexes, cis-coordination by the two fluoride ions from cis-[CrIIIF2(NN)2]+ facilitates the formation of the square motif through an almost linear fluoride-bridge between CrIII and LnIII (∠Ln–F–Cr ∼ 169°).
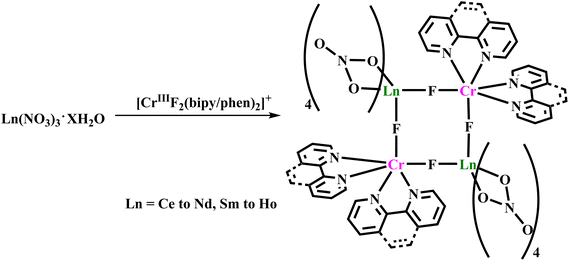 |
| Scheme 1 Line draw structure for complexes 28a–28i. | |
This is the first example of unsupported fluoride-bridges between a paramagnetic transition metal ion and lanthanide ion which have exhibited interesting magnetic properties. The structural simplicity allowed the theoretical modelling and quantification of magnetic properties of fluoride-mediated gadolinium analogue which displays overall antiferromagnetic Gd–Cr exchange interactions with JGd–Cr∼ between −0.14 cm−1 and −0.71 cm−1. However, for some compounds, the temperature dependencies of the χT value drop slightly with decreasing temperature because of the progressive depopulations of Stark sublevels of LnIII ions which basically mask the weak ferromagnetic Ln–Cr (Ln = Nd, Sm) interactions. The lack of any out-of-phase (χ′′) signal in ac susceptibility measurements with and/or without static field precludes the possibility of SMM behaviour in these complexes.103
With the aim of expanding the family of fluoride-bridged CrIII–LnIII clusters, Bendix and co-workers utilized trifluorido complex mer-[CrF3(py)3] (py = pyridine) as synthon. This upon reaction with [Ln(hfac)3(H2O)2] in the presence of different solvents has led different tetranuclear complexes, [Cr2Ln2(μ-F)4(μ-OH)2(py)4(hfac)6], (Ln = Y(29a), Gd(29b), Tb(29c), Dy(29d), Ho(29e), and Er(29f); hfacH = 1,1,1,5,5,5-hexafluoroacetylacetone), and [Cr2Ln2(μ-F)4F2(py)6(hfac)6], ((Ln = Y(6a), Gd(6b), Tb(6c), Dy(6d), Ho(6e), and Er(6f); hfacH = 1,1,1,5,5,5-hexafluoroacetylacetone), in acetonitrile and 1,2-dichloroethane, respectively (Scheme 2). The structural analysis of 29 revealed two octahedrally coordinated CrIII ions bridged by two hydroxide ions (<Cr–O–Cr ∼ 96°). Other positions of the octahedron were occupied by two pyridine (trans to hydroxide group) and two trans fluoride ions. Each fluoride ion forms a bridge to the LnIII ion (<Cr–F–Ln ∼ 140°). LnIII displayed eight coordination which is fulfilled by two bridging fluoride ions and three bidentate [hfac]− ligands. Here it is important to mention that partial hydrolysis of less robust mer-[CrF3(py)3] complexes assisted in the construction of the aforementioned structures of complexes 29. On the other hand, complexes 6 contain two mer-[CrF3(py)3] moieties where two cis-fluoride ions coordinated to LnIII ions forming a fluoride-bridged square of alternating CrIII and LnIII metal centres. The bridging angles for the fluoride ions vary in the range of 156° to 166°.
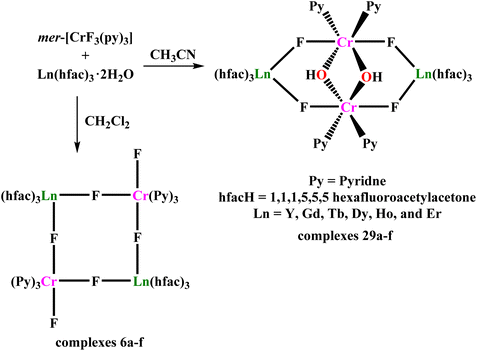 |
| Scheme 2 Line draw structure for complexes 29a–f and 6a–f. | |
Analysis of the magnetic data for 29b and 6b revealed that fluoride-mediated CrIII–GdIII exchange interactions were 0.43(5) cm−1 and 0.57(7) cm−1, respectively, which is consistent with the earlier reports.100 Variable-temperature and frequency dependent ac susceptibility measurements in the presence of a static field showed that 29a is a typical SMM with Ueff = 4.59(18) cm−1 as the thermal barrier for relaxation with τ0 = 2.8(3) × 10−5 s (Fig. 9). On the other hand, the temperature variation of χ′′ for 6a, obtained in a 1000 Oe static field without peak maxima, is indicative of a slow relaxation phenomenon without measurable energy barrier. Interestingly, 6b displayed a moderate cooling power with maximum magnetic entropy change −ΔSmaxm of 11.4 J kg−1 K−1 for ΔH = 9 T at T = 4.1 K.102
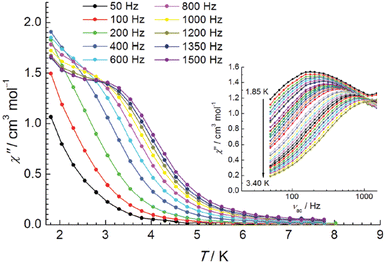 |
| Fig. 9 Out-of-phase (χ′′(T)) component of the ac susceptibility obtained on 29a in a dc field of Hdc = 1200 Oe (Hac = 3.8 Oe). Inset: χ′′(νac) of 29a at selected temperatures from 1.85 K to 3.40 K at Hdc = 1600.102 Reprinted with permission from ref. 102. Copyright 2012 Royal Society of Chemistry. | |
Apart from the aforementioned structural motifs in the tetranuclear Cr–Ln system, other well-studied motifs are “diamond”, “butterfly” or “defective dicubane” arrangements. In general, there are two possible variances for the abovementioned 3d/4f cores: in Type I arrangement, two 4f metal ions present in the body positions and the 3d transition metal ions occupy the outer wingtip sites; in Type II exactly the opposite situation prevails, where 3d metal ions occupy the body positions and 4f metal ions are at the wingtips (Scheme 3). Murray and co-workers have reported extensive magnetic studies for complexes consisting of Type I core based {MIII2LnIII2}, (M = Co, Cr and Ln = Dy, Tb, Ho).69,70,148–155
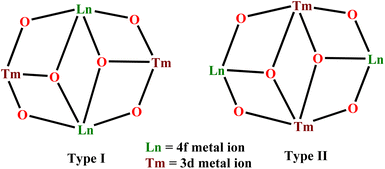 |
| Scheme 3 Schematic diagram of Type I and Type II compound. | |
Systematic study has revealed that the Type I complexes can be subdivided into three families depending on the metal and co-ligands used in that particular system. The first type of such family assembled with benzoate and amine-polyalcohol co-ligands and resulted in {CoIII2DyIII2}-benzoate butterfly complexes; [CoIII2DyIII2(OMe)2(O2CPh)4(RN{(CH2)2O}2)2(L)2][X]2 (R = H (30a), Me (30b), Bu (30c), (CH2)2OH) (30d), L = MeOH or NO3 and X = NO3.154,155 This class of compounds displays magnetic behaviour analogous to Dy2 derivative as CoIII is a diamagnetic substance. It was found that all the complexes displayed SMM behaviour and a thermal energy barrier ranging from 54 to 80 cm−1.155 This wide range of thermal energy barrier for the spin reversal is due to the use of electronically different N-substituted diethanolamine ligands in the formation of different complexes. Another example of this category consists of double cluster compounds [CoIII2DyIII2(OMe)2(teaH)2(O2CPh)4(MeOH)4](NO3)2·MeOH·H2O (31a) and [CoIII2DyIII2(OMe)2(teaH)2(O2CPh)4(MeOH)2(NO3)2]·MeOH·H2O.(31b).154 The magnetization relaxation for this category of complexes exhibits temperature dependency below 2.5 K, suggesting quantum tunnelling of magnetization (QTM) below this temperature. Importantly, the extent of QTM has been reduced in these classes of complexes compared to the analogous Dy SMMs (binuclear Dy2 SMMs) due to the presence of nonmagnetic exchange ground state that arises from the antiferromagnetic dipolar coupling. This observed phenomenon was corroborated using ab initio calculations. Theoretical calculations explain the suppressed QTM due to the following reasons: the ground doublet has a small splitting (10−6 cm−1), due to the non-Kramer's state resulting from two coupled DyIII ions, which reduces QT. A second reason is the non-magnetic ground state, as the dipolar coupling is much stronger than the exchange interaction and antiferromagnetic in nature, which reduces the transverse influence from the neighbouring molecules. The ground state being non-magnetic, the magnetic field from the neighbouring complexes will vanish on lowering temperature, and thereby only the ground of each molecule remains populated at T ∼ 0 K, decreasing the possibility for QTM. This was further supported by the experimental magnetic dilution technique for the analogous [CoIII2YIII2] analogue. The relaxation mechanism was dominated by a single-ion character and overall weak dipolar antiferromagnetic interactions for these complexes, and these phenomena play a pivotal role in the suppression of QTM at zero-field.
In the follow-up work, the investigators were able to isolate a structurally nearly similar second set of {CoIII2DyIII2}-acac butterfly complexes, [CoIII2DyIII2(OR)2(R′N{(CH2)2O}2)2(acac)4(NO3)2] (R = H, Me and R′ = Me, (CH2)2OH) (32), utilizing acetylacetone (acacH) instead of benzoate. Both types of complex displayed square anti-prismatic (SAP) geometry with a near similar primary coordination sphere around DyIII ions, although, as terminal substituents around the DyIII ions differ, consequently the low-temperature spin dynamics are also different in these complexes.152,153
A third set of compounds was isolated by substituting CoIII with CrIII in the aforementioned families to construct structurally related {CrIII2DyIII2}-benzoate and {CrIII2DyIII2}-acac complexes.69,70,148–151 In 2013, Murray and co-workers reported the isolation of [CrIII2DyIII2(OMe)2(O2CPh)4(mdea)2(NO3)2] (9) (mdeaH2 = N-methyldiethanolamine)70 (Fig. 10) which showed different and distinct low-temperature spin dynamics compared to the analogous CoIII counterpart; although both have comparable relaxation energy barriers of 77 K, the activation barriers in 31b and 9 are of different origins.
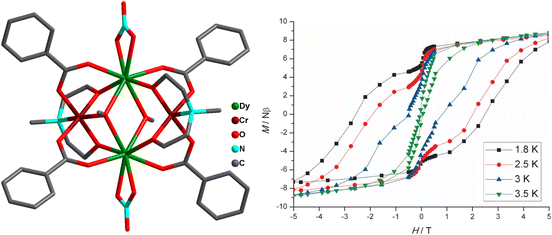 |
| Fig. 10 Molecular structure of 9 (left) and hysteresis plot for 9, with an average sweep rate of 0.003 Ts−1, at different temperatures indicated (right).70 Reprinted with permission from ref. 70. Copyright 2013 John Wiley and Sons. | |
As a result, at very low temperature a significant increase in relaxation time was observed for 9 compared to 31b.
Ab initio calculations corroborate that the magnetic properties in 9 arise as a consequence of the magnetic interactions between the DyIII and CrIII ions (JDy–Cr = |8|−|10| cm−1). Therefore, the energy barrier in 9 is of a multilevel exchange type (Fig. 11), with significantly reduced QTM and considerably longer relaxation times. On the contrary, in 31b, the barrier originates from one excited state of individual DyIII ions, and therefore shorter relaxation times are observed. As a result of reduced efficiency of QTM, the CrIII analogue displayed magnetic hysteresis up to 3.5 K with a large coercive field (coercive field of ca. 2.8 T at 1.8 K), whereas for the CoIII analogue (31b) no hysteresis was observed above 1.8 K using a conventional SQUID magnetometer. The comparison of the {MIII2LnIII2}, (M = Co, Cr) cores for 31b and 9 is displayed in Fig. 12.
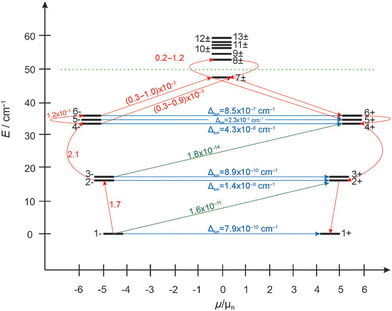 |
| Fig. 11
Ab initio calculated exchange spin states and the plausible relaxation dynamics in 9, plausible anisotropy energy barrier (dashed green line), QTM (blue line), spin–phonon transitions (red and dark-green).70 Reprinted with permission from ref. 70. Copyright 2013 John Wiley and Sons. | |
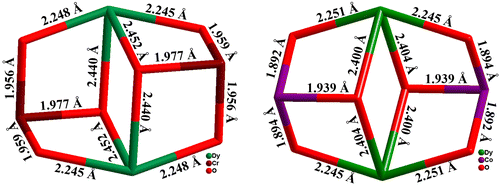 |
| Fig. 12 Comparison of {MIII2LnIII2} cores, (M = Co, Cr) for 31b and 9. | |
In their subsequent efforts, the authors prepared several iso-structural [CrIII2DyIII2] analogues containing a similar tetranuclear metallic core by varying the amine–diolate ligands.151 By utilizing diethanolamine (deaH2), N-ethyldiethanolamine (edeaH2), butyldiethanolamine (bdeaH2), and triethanolamine (teaH3) they have reported four new 3d/4f complexes of formulae [CrIII2DyIII2(OMe)2(O2CPh)4(dea)2(MeOH)4](NO3)2 (33a), [CrIII2DyIII2(OMe)(OH)(O2CPh)4(edea)2(NO3)2]·MeOH·Et2O (33b) [CrIII2DyIII2(OMe)2(O2CPh)4(bdea)2(NO3)2] (33c), and [CrIII2DyIII2(OMe)2(O2CPh)4(teaH)2(NO3)2(MeOH)2] (33d). All the compounds 33a–d distinctly displayed SMM behaviour as characterized by ac susceptibility and variable field magnetization/hysteresis measurements. Ac susceptibility analysis yields anisotropy barriers of 43.2, 55, 42.8, and 44 cm−1 for 33a, 33b, 33c, and 33d, respectively. These values are comparable to the value obtained for the analogous {CrIII2DyIII2}-mdea complex 9, of 53.5 cm−1. This indicates that replacement of amine–diolate ligands around the Dy ions in 33a–d has trivial influence on the energy barrier. Nonetheless, the strength of the magnetic exchange interaction determines the energy barrier for the magnetization reversal in these tetranuclear complexes, as corroborated theoretically in analogous {CrIII2DyIII2}-mdea complex.70
As well as the DyIII analogue (9), the author also prepared isostructural [CrIII2LnIII2(OMe)2(O2CPh)4(mdea)2(NO3)2]69 where Ln = Gd (34a), Er (34b),Tb (34c) and Ho (34d) by replacing DyIII in 9 to compare the role played by LnIII ions in magnetic behavior. Additionally, [CrIII2LnIII2(OMe)2(O2CPh)4(teaH)2(NO3)2(MeOH)2] [Ln = Pr (34e) and Nd (34f)] were also synthesized using triethanolamine and benzoic acid. The Gd derivative (34a) did not respond to the ac measurement owing to the isotropic nature of the GdIII ion. Erbium derivative (34b) displayed only tails without any maxima in the frequency-dependent out-of-phase ac susceptibility measurement, which confirmed the occurrence of very fast QTM in ground exchange states, clearly indicating the system's inability to generate single-ion bistability. The Tb (34c) and Ho (34d) analogues responded to ac susceptibility at zero dc field with anisotropy barriers of 45 and 36 cm−1, respectively, which are relatively small compared with 31b (54 cm−1), indicating fast QTM in 34c and 34d with respect to 31b. Moreover, 31b opens up a hysteresis loop up to 3.5 K with a large coercive field (sweep rate of 0.003 Ts−1), whereas Tb (34c) and Ho (34d) analogues display a magnetic hysteresis loop up to 2.5 (34c) and 1.8 K (34d), respectively, (sweep rate of 0.003 Ts−1) with a large loss of magnetization at zero-field due to strong ground state tunnelling of magnetization (Fig. 13). These differences might be due to the following reasons: DyIII, TbIII and HoIII possess oblate-spheroidal electron densities in their ground state. So, the ligand field from the axial/pseudo-axial direction may stabilize the largest angular momentum projections of the ground spin–orbit multiplet, which results in magnetic bistability and Ueff to magnetic reorientation in these complexes. Although all the systems have magnetic bistability the non-Kramers nature of TbIII and HoIII leads to greater tendency for QTM at the ground state and also causes inferior hysteresis for 34c and 34d analogues compared with 31b.
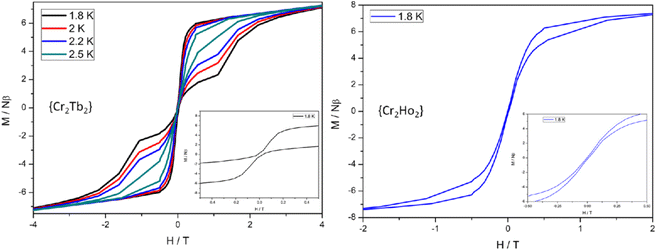 |
| Fig. 13 Hysteresis plots for 34c (left) and 34d (right) with a sweep rate of 0.003 Ts−1, at the temperatures indicated.69 Reprinted with permission from ref. 69. Copyright 2015 American Chemical Society. | |
The same group reported a series of tetranuclear 3d–4f complexes with the general formula of [CrIII2DyIII2(OMe)2(RN{(CH2)2O}2)2(acac)4(NO3)2] [R = Me (35a), Et (35b), nBu (35c)]150 (Fig. 14) with a Type 1 butterfly core arrangement. These complexes possess anisotropy barriers of [23.6 (35a), 25.7 (35b) and 28.5 (35c) cm−1] with open hysteresis loops up to 1.8 K for 35a, 2.2 K for 35b and 35c. From the previous discussion it is very much evident that the energy barrier and the relaxation time for the CrIII–LnIII complexes are better compared to the CoIII–LnIII analogues due to the significant magnetic exchange interaction between CrIII and LnIII ions to suppress the QTM.154
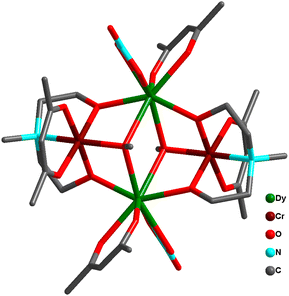 |
| Fig. 14 The molecular structure of compound 35a. | |
Importantly, in spite of nearly similar core structures, the energy barrier for 35a–c was approximately half of that of 31b. The above phenomenon was explained with the help of theoretical calculations which showed a weaker magnetic exchange interaction in 35a–c which is roughly half in magnitude to that of 31b. This result demonstrated that the strength of the Cr–Dy interaction directly played a crucial role in determining the effective energy barrier. Here it is noteworthy to mention that although the local environments around the DyIII centre in complexes 31b and 35a–c are significantly different, this plays a only minor role in manipulating the low-temperature dynamic behaviour in these complexes (Fig. 15). The exchange interactions between the metal ions are more important than the coordination environment around the DyIII ion as long as the change in local geometry does not drastically alter the ground state, gz ∼ 20, of the DyIII ion and the exchange pathways are not significantly weakened.
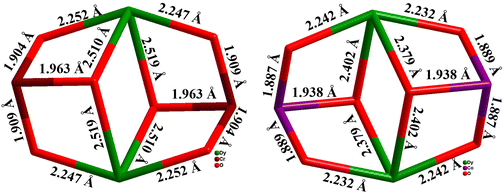 |
| Fig. 15 Comparison of coordination environments for core structures of 35a and 31b.150,154 | |
In another work the authors synthesized butterfly-cored {CrIII2DyIII2}-hfacac (Hhfacac = hexafluoroacetoacetone), i.e [CrIII2DyIII2(OMe)2(mdea)2(hfacac)6] (36).149 This complex displayed a barrier of 27.8 cm−1 which was slightly larger than the analogous complex, [CrIII2DyIII2(OMe)2(mdea)2(acac)4(NO3)2] (35a). A plausible reason might be the consequence of replacing [acac]− and nitrate ligands with strongly electron-withdrawing [hfacac]− ligands that imposed stronger exchange interactions among the ions in 36 compared with 35a. As a result, the blocking temperature increases from 1.8 to 2.2 K for 36 compared with 35a, which is not observed in the corresponding [CoIII2DyIII2] case due to weaker exchange interaction. This work represents an example in which the incorporation of an electron-withdrawing group in a complex can enhance the effective energy barrier either by stabilizing the ground Kramers doublet or by manipulating the exchange interaction. Utilizing this concept, Murray and co-workers have shown significant improvements in magnetic relaxation time, magnetic hysteresis blocking temperature and magnetic coercivity of {CrIII2DyIII2}-benzoate complexes via replacing bridging benzoate ligands with substituted benzoate ligand. Varying the benzoate ligand from electron-withdrawing (halogen) to electron-donating groups (tert-butyl), they have synthesized a variety of complexes, [CrIII2LnIII2(OMe)2–x(OH)x(2-Cl-4,5-F-benz)4(mdea)2(NO3)2]·xMeOH [Ln = Tb (37a), Dy (37b) and Ho (37c)] (2-Cl-4,5-F-benzH = 2-chloro-4,5-fluorobenzoic acid) and [CrIII2DyIII2(OMe)(OH)(4-tBu-benz)4(tBudea)2(NO3)2]·MeOH·2Et2O (38) (tBudeaH2 = N-tert-butyldiethanolamine, 4-tert-benzoic acid = 4-tBu-benzH).148 All these complexes display SMM behaviour. Most importantly, the observed magnetic hysteresis loops of all samples are temperature dependent, with open loops observed up to 3.5, 4.7 and 2.6 K, for 37a, 37b and 37c, respectively. Alternatively, the benzoate analogue showed hysteresis loops up to 2.5 (34c), 3.5 (9) and 1.8 (34d). Comparison of the hysteresis loops also indicates a substantial increase in the coercive field observed for 37a, 37b and 37c compared with 34c, 9 and 34d (Fig. 16). Most importantly, 9, 34a and 34d show large loss of magnetization at zero field in M vs. H hysteresis plot, whereas complexes 37a–c display a considerable reduction of magnetization indicating significant suppression of zero-field QTM. Notably, there is no loss of magnetization at zero field for 37b compared with 9.
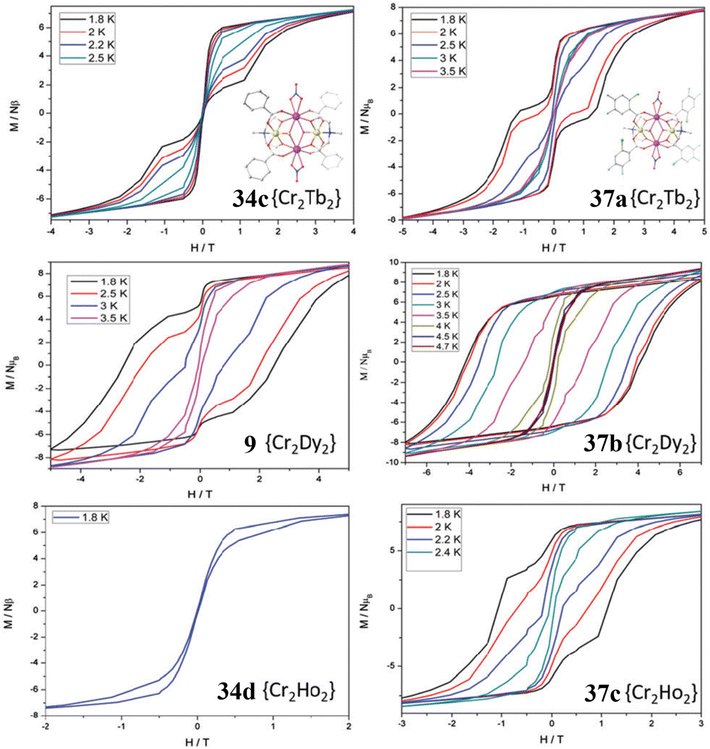 |
| Fig. 16 Comparison of the hysteresis curves of (at sweep rate of 0.003 Ts−1) (34c and 37a), (9 and 37b) and (34d and 37c).148 Reprinted with permission from ref. 148. Copyright 2016 Royal Society of Chemistry. | |
Powell and co-workers reported Type II butterfly-cored complexes: [MIII2LnIII2(μ3-OH)2(pMe-PhCO2)6(L)2] [H2L = 2,2′-((pyridin-2-ylmethyl)azanediyl)bis(ethan-1-ol), M = Cr, Ln = Dy (39a) or Y (39b); M = Mn, Ln = Dy (40a) or Y (40b); M = Fe, Ln = Dy (41); M = Al, Ln = Dy (42)] and the diluted complex [AlIII2DyIII2]: [AlIII2DyIII0.18Y1.82] (43) (Fig. 17).88,156 No out-of-phase signal was observed even after using a static dc field, indicating the absence of SMM behaviour for CrIII analogue (39a). 40a {Mn2Dy2} displayed an anisotropy barrier Ueff of 13.35 cm−1 at zero dc field. For 41 {Fe2Dy2}, 42 {Al2Dy2} and 43 {AlIII2DyIII0.18Y1.82} the anisotropic energy barrier was found to be 11.23, 28.84 and 44.41 cm−1 under 1000 Oe applied dc field. The SMM behaviour for the four complexes follows the trend: {Al2Dy2} > {Mn2Dy2} > {Fe2Dy2} > {Cr2Dy2}. The combined experimental and theoretical calculations suggested that altering the 3dIII ions can affect the single-ion properties, the nature and the magnitude of the 3dIII–3dIII, 3dIII–DyIII and DyIII–DyIII magnetic coupling, thus quenching the quantum tunnelling of magnetization (QTM) significantly, thereby improving the SMM properties within these motifs (Fig. 18).
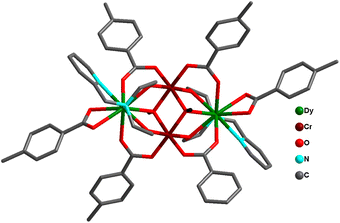 |
| Fig. 17 Molecular structure of 39a. | |
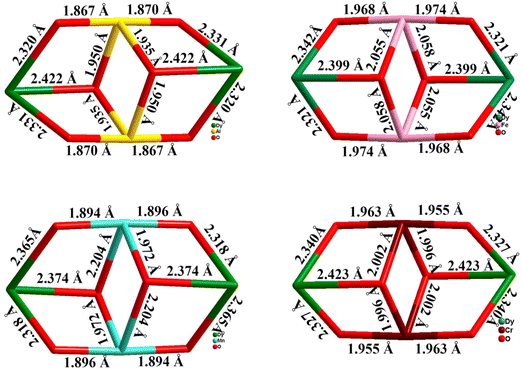 |
| Fig. 18 Comparison of the core structures in complexes 39a, 40a, 41 and 42.88,156 | |
It has been noted that the tetranuclear [Cr2Ln2] of diverse structures represent most of the literature precedents in the family of Cr–Ln-based heterometallic complexes. We have provided the detailed magnetic properties of these analogues in Table 3.
Table 3 Representative examples of tetranuclear CrIII–LnIII complexes
Compounds |
Bridging ligand |
J
CrGd [cm−1] |
Energy barrier (Ueff) time constant (τ0) |
Magnetocaloric effect (−ΔSm) |
Ref. |
[CrIII2LnIII2(μ-F)4(μ-OH)2(py)4 (hfac)6] (LnIII = Y, Gd, Tb, Dy, Ho, Er) hfacH = 1,1,1,5,5,5-hexafluoroacetylacetone, py = pyridine (29a–f) |
Ln⋯F⋯Cr |
J
CrGd = 0.43 |
U
eff = 4.59 cm−1 and τ0 = 2.8 × 10−5 s for LnIII = Dy |
— |
119
|
[CrIII2LnIII2(μ-F)4F2(py)6(hfac)6] (LnIII = Y, Gd, Tb, Dy, Ho, Er); hfacH = 1,1,1,5,5,5-hexafluoroacetylacetone, py = pyridine (6a–f) |
Ln⋯F⋯Cr |
J
CrGd = 0.57 |
— |
−ΔSm = 11.4 J kg−1 K−1 |
119
|
[CrIII2LnIII2(OMe)2(O2CPh)4(mdea)2(NO3)2] (LnIII = Dy); mdeaH2 = N-methyldiethanolamine (9) |
Ln⋯O⋯Cr (O from mdea) |
J
CrDy = |8|−|10| |
U
eff = 53.5 cm−1 and τ0 = 5.1 × 10−8 s for LnIII = Dy |
— |
55
|
[CrIII2Dy2(OMe)2(O2CPh)4(dea)2(MeOH)4](NO3)2 (LnIII = Dy); deaH2 = diethanolamine (33a) |
Ln⋯O⋯Cr (O from dea) |
— |
U
eff = 43.2 cm−1 and τ0 = 2.3 × 10−7 s for LnIII = Dy |
— |
167
|
[CrIII2Dy2(OMe)(OH)(O2CPh)4(edea)2(NO3)2]·MeOH·Et2O (33b) (LnIII = Dy); edeaH2 = N-ethyldiethanolamine |
Ln⋯O⋯Cr (O from edea) |
— |
U
eff = 55 cm−1 and τ0 = 3.4 × 10−8 s for LnIII = Dy |
— |
167
|
[CrIII2Dy2(OMe)2(O2CPh)4(bdea)2(NO3)2] (33c) (LnIII = Dy); bdeaH2 = N-n-butyldiethanolamine |
Ln⋯O⋯Cr (O from bdea) |
— |
U
eff = 42.8 cm−1 and τ0 = 1.1 × 10−7 s for LnIII = Dy |
— |
167
|
[CrIII2Dy2(OMe)2(O2CPh)4(teaH)2(NO3)2(MeOH)2] (33d)(LnIII = Dy); teaH2 = triethanolamine |
Ln⋯O⋯Cr (O from teaH) |
— |
U
eff = 44 cm−1 and τ0 = 8.3 × 10−7 s for LnIII = Dy |
— |
167
|
[CrIII2LnIII2(OMe)2−x(OH)x(O2CPh)4 (mdea)2(NO3)2] (34a–d) (LnIII = Gd, Tb, Ho, Er); mdeaH2 = N-methyldiethanolamine |
Ln⋯O⋯Cr (O from mdea) |
J
CrGd = −0.960 |
U
eff = 44.5 cm−1 and τ0 = 1.7 × 10−9 s for Tb; Ueff = 36.1 cm−1 and τ0 = 1.1 × 10−9 s for Ho |
— |
54
|
[CrIII2LnIII2−(OMe)2(O2CPh)4(teaH)2 (NO3)2(MeOH)2] (LnIII = Pr, Nd); tea = triethanolamine (34e–f) |
Ln⋯O⋯Cr (O from teaH) |
— |
— |
— |
54
|
[CrIII2LnIII2(OMe)2(MeN{(CH2)2OH}2)2(acac)4(NO3)2] (35a) (LnIII = Dy); acacH = acetylacetone |
Ln⋯O⋯Cr (O from acac) |
|
U
eff = 23.6 cm−1 and τ0 = 1.2 × 10−7 s for LnIII = Dy |
— |
166
|
[CrIII2LnIII2(OMe)2(EtN{(CH2)2OH}2)2(acac)4(NO3)2] (35b) (LnIII = Dy); acacH = acetylacetone |
Ln⋯O⋯Cr (O from acac) |
|
U
eff = 25.7 cm−1 and τ0 = 9.2 × 10−8 s for LnIII = Dy |
— |
166
|
[CrIII2LnIII2(OMe)2(nBuN{(CH2)2OH}2)2(acac)4(NO3)2] (35c) (LnIII = Dy) acacH = acetylacetone |
Ln⋯O⋯Cr (O from acac) |
|
U
eff = 28.5 cm−1 and τ0 = 3.1 × 10−7 s for LnIII = Dy |
— |
166
|
[CrIII2LnIII2(OMe)2−(mdea)2 (hfacac)6] (LnIII = Dy) (36); mdeaH2 = N-methyldiethanolamine, hfacacH = hexafluoroacetylacetone |
Ln⋯O⋯Cr (O from mdea) |
|
U
eff = 28.6 cm−1 and τ0 = 1.6 × 10−7 s for LnIII = Dy |
— |
165
|
[CrIII2LnIII2(OMe)2−x(OH)x(2-Cl-4,5-F-benz)4(mdea)2(NO3)2] ·xMeOH (LnIII = Tb, Dy and Ho) (37a–c); mdeaH2 = N-methyldiethanolamine, 2-Cl-4,5-F-benz = 2-chloro-4,5-fluorobenzoic acid |
Ln⋯O⋯Cr (O from mdea and Ln⋯O⋯Cr) from 2-Cl-4,5-F-benz |
|
U
eff = 44 cm−1 and τ0 = 7.7 × 10−9 s for LnIII = Tb; Ueff = 61 cm−1 and τ0 = 2.1 × 10−7 s for LnIII = Dy; Ueff = 36 cm−1 and τ0 = 6.8 × 10−9 s for LnIII = Ho |
|
164
|
[CrIII2Dy2(OMe)(OH)(4-tBu-benz)4(tBudea)2(NO3)2]·MeOH·2Et2O (LnIII = Dy) (38); (tBudeaH2 = N-tert-butyldiethanolamine), 4-tBu-benz = 4-tert-benzoic acid |
Ln⋯O⋯Cr (O from mdea and bridging ligand from 4-tBu-benz |
|
U
eff = 45 cm−1 and τ0 = 7.7 × 10−8 s |
|
164
|
[CrIII2LnIII2(μ3-OH)2(p-Me-PhCO2)6(L)2] (LnIII = Dy, Y) (39a–b); H2L = 2,2′-((pyridin-2-ylmethyl)azanediyl)bis(ethan-1-ol), p-Me-PhCO2 = 4-methylbenzoic acid |
Ln⋯O⋯Cr (O from L and bridging O atom from p-Me-PhCO2 |
|
— |
|
105
|
[CrIII2DyIII2(teaH)2(OCH3)2(piv)6] (44); teaH = triethanolamine, piv = pivalic acid |
Ln⋯O⋯Cr (O from tea and bridging ligand from piv |
J
CrDy = −1.5 |
U
eff = 50 cm−1 and τ0 = 1.2 × 10−7 s |
|
157
|
[CrIII2TbIII2(OH)2(FcCO2)4(NO3)2(Htea)2] (45a) Fc = (η5-C5H4)(η5-C5H5)Fe and tea = triethanolamine |
Ln⋯O⋯Cr (O from tea) |
|
U
eff = 37.5 cm−1 and τ0 = 21 × 10−9 s |
|
158
|
[CrIII2DyIII2(OH)2(FcCO2)4(NO3)2(Htea)2] (45b) Fc = (η5-C5H4)(η5-C5H5)Fe and tea = triethanolamine |
Ln⋯O⋯Cr (O from tea) |
|
U
eff = 52.1 cm−1 and τ0 = 26 × 10−9 s |
|
158
|
[CrIII2HoIII2(OH)2(FcCO2)4(NO3)2(Htea)2] (45c) Fc = (η5-C5H4)(η5-C5H5)Fe and tea = triethanolamine |
Ln⋯O⋯Cr (O from tea) |
|
U
eff = 32.6 cm−1 and τ0 = 2 × 10−9 s |
|
158
|
5.4 Pentanuclear complexes
Bendix and co-workers utilized synthons fac-[CrF3(Me3tame)] and fac-[CrF3(Me3tacn)], where Me3tame = 1,1,1-tris-((methylamino)methylethane, Me3tacn = N,N′,N′′-trimethyl-1,4,7 triazacyclononane (Me3tacn), as building blocks to synthesize pentanuclear [Cr2Ln3] complexes [Ln = Gd (7a), Ho (7b), Yb (7c), Nd (7d)]. The pentanuclear complexes are in trigonal bipyramidal geometry where the equatorial plane consists of LnIII ions which are bridged by bidentate/bridging nitrate (μ-nitrato-1,2 κO:1 κO) ligands or fluoride ligands. CrIII ions are placed in the apical position. The NdIII derivative consisted of the most unusual μ3-fluorido ligand bridging in all three metal centres of the equatorial plane symmetrically. The structural simplicity allowed the modelling of the magnetic properties and quantification of magnetic coupling in 7a. Gd–Cr exchange interaction was found to be antiferromagnetic with JCr–Gd = −0.14 cm−1 and negligible JGd–Gd = 0.06 cm−1. The weak intra cluster interactions, large net magnetic moment and negligible ZFS in 7 indicates a large number of electronic states that are almost degenerate with the ground state. This phenomenon made them suitable candidates for evaluating the magneto-caloric effect. Experimentally 7a displayed large magneto-caloric effect with maximum value of 28.7 J kg−1 K−1 at 2.2 K for an applied field of 90 kOe.103
The authors further extended this work for the other 3d analogues. Similar synthetic protocols with the precursor fac-[MIIIF3(Me3-tacn)3]·4H2O [M = Cr, Fe, Ga] led to the isostructural [{MF3(Me3−tacn)}2Gd3F2(NO3)7(H2O)(CH3CN)] complexes [M = Cr (8a), Fe (8b), Ga (8c)] (Fig. 19). Weak exchange interactions in these complexes were rationalized computationally. Cr and Fe analogues reveal large magnetic entropy changes of 38.3 J kg−1 K−1 for 8a at 2.0 K and 33.1 J kg−1 K−1 for 8b at 4.2 K with ΔH = 7 T. Interestingly, the larger spin ground state was present in 8b through intramolecular Fe–Gd ferromagnetic interactions. However, the presence of close-lying excited states through weaker coupling in 8a makes them more prominent in achieving high magneto-caloric value.71 This complementary exchange interaction (J) in 8a and 8b can nicely be correlated in terms of the d electronic configuration of the corresponding 3d metals. The CrIII ion with (t2g)3 electronic configuration induces relatively smaller interaction with the GdIII 5d orbitals compared to the FeIII ion in (t2g)3(eg)2 configuration. The dz2 orbital of FeIII accelerates the stronger overlap with the GdIII 5d orbitals generating stronger ferromagnetic coupling. Theoretically it has been postulated that the extent of JM–Gd interaction depends on the {MIII–F–GdIII} angle. The extent of ferromagnetic JFe–Gd interaction increases with the increase in {FeIII–F–GdIII} bridging angle, whereas an opposite trend was found for {CrIII–F–GdIII} angles.
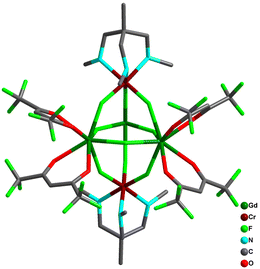 |
| Fig. 19 Molecular structure of complex 8a.71 | |
Wang and co-workers synthesized pentanuclear trigonal bipyramidal {Cr2Ln3} complexes Na3[Dy3Cr2(HGly)6(μ3-OH)6(H2O)9]·(ClO4)8·Cl4·14H2O (46a) and Na3[Tb3Cr2(HGly)6(μ3-OH)6(H2O)9]·(ClO4)6·Cl6·6H2O (46b) using glycine (HGly).72 Subsequently the reaction of 46a with a ditopic linker 4,4′-dipyridyl N,N′-dioxide (dpyo) afforded [Dy3Cr2(HGly)6(dpyo)2(μ3-OH)6(H2O)7]·(ClO4)9·15H2O (47). From the structural point of view, {Cr2Ln3} adopted a trigonal–bipyramidal configuration where the triangular equatorial plane defined by the three LnIII ions and CrIII ion occupied axial positions. Each Ln2Cr triangular face in trigonal–bipyramidal geometry was capped by one μ3-OH− group forming the [CrIII2(OH)6LnIII2]9+ core, and furthermore adjacent LnIII and CrIII were connected by acid groups of glycinato ligand in syn–syn mode. Compound 47 has the core structure similar to that of 46a where two terminal water molecules of two DyIII ions are replaced by two mono-coordinated dpyo ligands. In the quest for a polymeric network, the authors ended with a zero-dimensional molecular complex. A 3D supramolecular cds network was formed through hydrogen-bonding and π–π stacking interactions. All the three analogues displayed SMM behaviour with the following characteristics: Ueff ≈ 9.8 cm−1 and τ0 = 4.2 × 10−8 s for 46a; Ueff ≈ 9.0 cm−1 and τ0 = 6.4 × 10−7 s for 46b; and Ueff ≈ 9.2 cm−1 and τ0 = 9.16 × 10−8 s for 47. These results indicated that there is no significant impact of lanthanide ion or ligation by the dpyo ligands on the SMM behaviour in these complexes.
Zhao and co-workers reported trigonal bipyramidal {Cr2Ln3} complexes with the formula of [{CrIII2LnIII3L10(OH)6(H2O)2}(Et3NH)] [Ln = Tb (12a), Dy (12b), Y(12c); Gd (12d) HL = pivalic acid, Et3N = triethylamine]. The metal core of each complex is analogous to 12a, consisting of trigonal bipyramidal topology with three LnIII ions (equatorial plane) and two CrIII ions (axial position) held together by six μ3-OH bridges. Around the periphery ten L− ligands and two terminal water molecules further strengthen the [CrIII2(OH)6LnIII2]9+ core where six pivalate ligands adopt a syn–syn mode to bridge adjacent CrIII and LnIII ions, two bidentately stitch two LnIII centers, and two act as monodentate ligands to coordinate with one LnIII ion.
In order to understand the nature of the CrIII–LnIII magnetic interaction the authors prepared an analogous AlIII–LnIII derivative with the formula [{AlIII2LnIII3L10(OH)6(H2O)2}Et3NH·H2O] [Ln = Tb (48a), Dy (48b)].159 Predominant ferromagnetic interactions were found to prevail between CrIII and LnIII ions. AC magnetic susceptibility confirmed SMM behavior for [CrIII2TbIII3] (12a), [CrIII2DyIII3] (12b), and [AlIII2DyIII3] (48b) without expected maxima until 1.8 K, mainly due to fast QTM. The authors were able to extract the parameters as follows: Ueff ≈ 11.8 cm−1, τ0 = 7 × 10−9 s for 12a and Ueff ≈ 7 cm−1, τ0 = 1.3 × 10−9 s for 12b.160 The single-ion behavior of LnIII ions and/or the CrIII–LnIII ferromagnetic interactions are the origin of slow relaxation of the magnetization behaviour in the abovementioned complexes. Due to isotropic CrIII and GdIII ions, large magnetization value, and dominant ferromagnetic exchange interaction between CrIII and GdIII ions, 12d showed large MCE with −ΔSmaxm of 27.0 J kg−1 K−1 at 4.0 K and ΔH = 7 T.104
Very recently, Rajaraman and co-workers reported similar pentanuclear trigonal bipyramidal {Cr2Ln3} complexes, [CrIII2LnIII3(PhCO2)7(OH)6(iPrO)(NO3)(H2O)3] (iPrO = isopropoxide) [where Ln = Dy (49a), Gd (49b)] (Fig. 20).161 It has been well demonstrated with the aid of theoretical investigations that the stronger ferromagnetic {CrIII–DyIII} exchange interaction with JCrIII–DyIII = +1.20 cm−1 facilitates reducing the ground state QTM to generate the SMM behaviour in the Dy analogue, with Ueff value of 30.9 K (21.4 cm−1) and τ0 = 4.09 × 10−10 s.
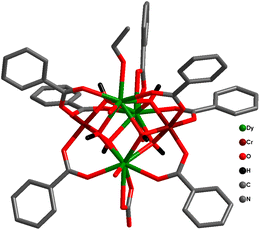 |
| Fig. 20 Molecular structure for 49a.161 | |
Car and co-workers prepared {CrIII4Ln} complexes [Cr4Ln(CH3COO)(pyCOO)((py)2COO)4]·(NO3)2 (LnIII = Gd (50a), Tb (50b) and Dy (50c) through hydrothermal reactions involving di-(2-pyridyl)ketone ligand. In the reaction condition di-(2-pyridyl)ketone gets reduced to gem-diol dianion (Py2COO2−) and decomposed to simplified anion of pyridine 2-carboxylic acid. Collective coordination modes of these ligands led to a diamond-shaped type topology of the central metal {CrIII4Ln} core. The presence of competing ferro- and antiferromagnetic exchange coupling between CrIII–LnIII and LnIII–LnIII centers, respectively, below 50 K was predominant (JGd–Cr = 0.25 cm−1 and JCr–Cr = −0.55 cm−1). None of the compounds responded towards ac susceptibility signals, but the gadolinium derivative exhibited interesting magnetocaloric properties with −ΔSmaxm of 18.31 J kg−1 K−1 at 4.0 K with ΔH = 8 T.73
5.5 Hexanuclear complexes
Sequential reaction of 1,3-diamino-2-hydroxy-propane-N,N,N′,N′′-tetraacetic acid (H5hdpta), sodium acetate and CrCl3 yielded Na[CrIII2(hdpta)(AcO)2]. It is a connected ion framework containing Na+ nodes and dinuclear [Cr2(hdpta)(Ac)2]− complex ligands where two CrIII ions are chelated by one hdpta5− and connected simultaneously by two acetate auxiliary ligands. Further reaction of Na[Cr2(hdpta)(AcO)2] with Gd(NO3)3·6H2O afforded complex [Gd(NO3)3(H2O)4][Cr4Gd(hdpta)2(OH)4(H2O)5]·(NO3)·32H2O (51). The cationic part [Cr4Gd(hdpta)2(OH)4(H2O)5]+ forms a 1D chain based on GdIII nodes and [Cr4(hdpta)2(OH)4] complex ligands. CrIII ions are anti-ferromagnetically coupled in this complex.162
As already discussed in the tetranuclear section (vide supra), N-substituted diethanolamine ligand N-n-butyldiethanolamine (H2bdea) in the presence of different co-ligands yielded three different types of coordination cluster ranging from octanuclear “square-in-square” (Ln = La–Tb), hexanuclear “triangle-in-triangle” (Ln = Dy, Ho, Y) and tetranuclear “butterfly” or “defect dicubane” (Ln = Er–Lu) core topologies. In this section we will discuss the hexanuclear “triangle-in-triangle”-based complexes [CrIII3DyIII3(μ3-N3)0.36(μ3-OH)3.64(bdea)3(μ-piv)6(η1-piv)2(OH2)] (52a), [CrIII3HoIII3(μ3-OH)4(bdea)3(μ-piv)6(OH2)3]Cl2 (52b), and [CrIII3YIII3(μ3-OH)4(bdea)3(μ-piv)6(η1-piv)2(OH2)] (52c). The core structure in 52a (Fig. 21) consisted of a disordered mixture of azide and hydroxide present in the centre with μ3-bridging mode. Similarly, reaction with HoIII also forms a similar hexanuclear “triangle-in-triangle” topology. But the structure is not iso-structural to the DyIII analogue. This is due to the presence of charge-balancing pivalate moieties taking part in direct coordination with the DyIII ion in 52a, whereas in HoIII analogue (52b) chloride ions act as charge-balancing counter ions to generate a more symmetrical core for {Cr3Dy3} complex compared to the {Cr3Ho3} complex. Unfortunately, none of these complexes display SMM behavior.77 Furthermore, changing the ligand from more sterically bulky N-n-butyldiethanolamine (H2bdea) to less sterically encumbered N-methyldiethanolamine (H2mdea) generated a couple of analogous hexanuclear complexes [CrIII3LnIII3(mdea)3(piv)8(OH)4(H2O)], [LnIII = Gd (53a) and Dy (53b)] (Fig. 21). Surprisingly, unlike 52a, the DyIII analogue 53b displayed SMM behaviour at zero dc field below 4 K. This example nicely showcased that subtle changes in the peripheral ligand backbone create a major impact in altering the magnetic properties.76
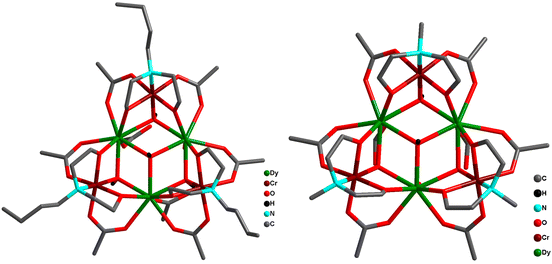 |
| Fig. 21 Molecular structure of complex 52a (left; N-n-butyl) and 53b (right; N-methyl). | |
Another unique compressed octahedral complex [(pipzH2){Cr2Dy4(μ4-O)2(μ3-OH)4(H2O)10(μ3-SO4)4(SO4)2}]·2H2O (54) was formed by hydrothermal reaction of Dy(NO3)3·6H2O, Cr(NO3)3·9H2O, Na2SO4 and piperazine. The {CrIII2DyIII4}18+ core consisted of an octahedral skeleton having four Dy ions in the equatorial plane and two Cr ions present at axial positions held together by two μ4-O2− atoms (Fig. 22). Also, it was noted that Dy⋯··Dy separations are ∼4.0 Å while Cr⋯··Dy separations range from 3.30 to 3.37 Å, and the Cr⋯Cr distance is 2.91 Å, indicating a compressed octahedron {Cr2Dy4} core. Ac magnetic studies revealed SMM behavior in complex 54 with Ueff = 27.6 cm−1 and τ0 = 2.9 × 10−9 s. Interestingly, 54 represented the first sulphate-based SMM.75
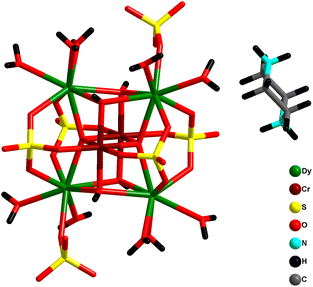 |
| Fig. 22 Molecular structure of 54. | |
To further investigate, iso-structural analogues (pipzH2)[CrIII2LnIII4(μ4-O)2(μ3-OH)4(H2O)10(μ3-SO4)4(SO4)2]·2H2O [Ln = Tb (55a), Ho (55b), Er (55c), Yb (55d), and Y (55e)] were synthesized utilizing similar synthetic protocols. The core [CrIII2TbIII4(μ4-O2−)2(μ3-OH)4]10+ is a double cubane composed of two Cr ions and four Tb ions which are bridged by two μ4-O2− atoms and four individual hydroxido μ3-OH− groups. Magnetic analysis revealed the antiferromagnetic interaction between metal centers for Tb, Ho, Er analogues. The Cr–Cr exchange interaction obtained for 55e analogue was found to be ferromagnetic with the value of 3.61(11) cm−1; however, Cr–Ln interactions were antiferromagnetic. Dynamic magnetic measurements revealed slow magnetic relaxation in the Cr2Tb4 and Cr2Er4 analogues, indicating SMM behavior. However, the energy barrier (16.75 cm−1) and pre-exponential factor τ0 (4.0 × 10−9 s) could be only retrieved for the Cr2Tb4 analogue.74
With the help of a sulfate framework, three [Ln4Cr2] cluster-based 3D complexes [Ln4Cr2(μ4-O)2(μ3-OH)4(H2O)9(SO4)5]·3H2O [LnIII = GdIII (56a), TbIII (56b), and DyIII (56c)] were synthesized. The asymmetric unit of 56a consisted of two Gd metal ions, and one Cr ion along with two and half sulfate anions, two μ3-OH− groups, and one μ4-O2−. The hexanuclear [Gd4Cr2] cluster moiety was formed with two such symmetry-linked units to generate a bi-cubic Gd4Cr2O6 core (Fig. 23). 56a analogue displayed MCE of −ΔSmaxm = 38.33 J kg−1 K−1 under ΔH = 7 T at 2.0 K. Tb and Dy analogues exhibited slow relaxation of magnetization.136
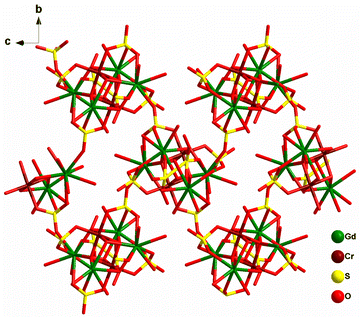 |
| Fig. 23 Three-dimensional view of 56a. | |
Other examples of sulfate-bridged 3D inorganic polymers containing {Cr2Dy4} core were described as [Cr2Dy4(μ4-O)2(μ3-OH)4(H2O)5(μ4-SO4)4(μ3-SO4)]·9H2O (57a) and [Cr2Dy4(μ4-O)2(μ3-OH)4(H2O)8(μ4-SO4)2(μ3-SO4)(ter-η3-SO4)2]·2H2O (57b).163 Despite having similar chemical components, the two compounds displayed structural divergence due to a difference in the binding modes of sulfate anions. With two CrIII ions at axial positions and four other DyIII ions at equatorial positions bonded together by two μ4-O2− and four μ3-OH− anions, a remarkable {Cr2Dy4} metallic octahedron is formed. In 57a, two types of sulfate bridging are present: firstly μ4-SO42− anion bridges two DyIII and CrIII ions from the [Cr2Dy4(μ4-O)2(μ3-OH)4]10+ core by three oxygens along with a DyIII ion from the neighboring [Cr2Dy4(μ4-O)2(μ3-OH)4]10+ motif by the fourth oxygen atom. Secondly, μ3-SO42− anion binds three Dy atoms from two adjacent [Cr2Dy4(μ4-O)2(μ3-OH)4]10+ motifs by three oxygen atoms. Thus, each [Cr2Dy4(μ4-O)2(μ3-OH)4]10+ core is connected to six adjacent neighbors and each layer parallel to ab plane, forming a 3D extended network. In complex 57b the same type of bridging is present; however, along with it another bridging mode is also present. The new sulfate bridging ligand, ter-η3-SO42− binds two DyIII and one CrIII from the same [Cr2Dy4(μ4-O)2(μ3-OH)4]10+ core with three oxygen atoms. Structural inspection of 57b shows that the individual units of this complex are analogous to 57a. However, the 3D network in 57b is formed by connecting the individual units of 57a through sulphate bridges (Fig. 24). SMM behavior is observed for both 57a (Ueff of 26 cm−1 and τ0 of 6.7 × 10−8 s) and 57b (Ueff of 18 cm−1 and τ0 = 7.3 × 10−9 s). It was noted that the SMM performance of 57a was better than 57b due to the orientation of the clusters, which are closely linked with permutation of magnetic moment among SMM-like clusters, thereby influencing the spin dynamics.
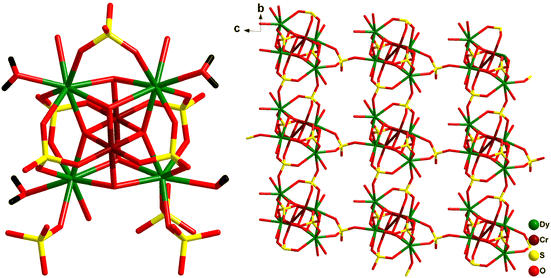 |
| Fig. 24 Molecular structure of complex 57a (simplified view; left) and 3-dimensional view (right). | |
5.6 Heptanuclear complexes
Reaction of 2,2-dimethylolpropionic acid (H3L), Ln(ClO4)3 and chromium salts utilizing different anions reacted under high pH conditions yielded a series of Ln–Cr clusters with formula [Ln5Cr2(H2L)2(OAc)6(μ3-OH)6(H2O)15](ClO4)7 (Ln = Gd 58a and Ln = Dy 58b) and [Ln8Cr4(H2L)4(OAc)8(μ3-OH)16(μ4-O)(H2O)8](Cl)(ClO4)5·10H2O (Ln = Gd for 59a and Ln = Dy for 59b) (Fig. 25). This is a unique example of anion-dependent assembly, templated by mixed anions.
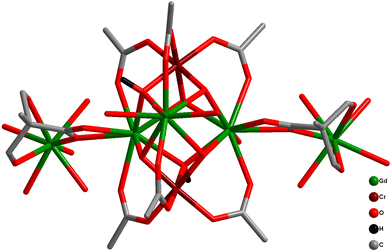 |
| Fig. 25 Molecular structure of complex 58a. | |
Furthermore, the cationic cluster comprises a unit [Gd(H2L)]2+ and another pentanuclear cationic cluster [Gd3Cr2(μ3-OH)6]9+ which is surrounded by fifteen water molecules and six acetate ligands. Mononuclear cationic cluster [Gd(H2L)]2+ is formed by one Gd(III) ion and chelating ligand H2L−, while pentanuclear cationic unit [Gd3Cr2(μ3-OH)6]9+ is formed by three GdIII ions and two CrIII ions linked together by six μ3-OH groups. Overall, the cationic core of [Gd5Cr2(H2L)2(μ3-OH)6]7+ is formed by the cumulative action of both the mononuclear cationic core [Gd(H2L)]2+ and the pentanuclear core [Gd3Cr2(μ3-OH)6]9+ joined by a carboxyl group from H2L−. Gd⋯Cr and Gd⋯Gd interactions are weak antiferromagnetic and ferromagnetic, respectively. 58a displayed the magnetocaloric effect with −ΔSmaxm value of 25.2 J kg−1 K−1 at 5 K and 7 T.140
Vignesh et al. reported the heptanuclear complex [CrIIIDyIII6(OH)8(o-tol)12(NO3)(MeOH)5]·3MeOH (60a), (o-tol = ortho-toluate).164 The metal core is formed by two triangular DyIII motifs lying above and below a single CrIII ion producing a common vertex sharing a trigonal pyramidal structure. It is further stabilized by eight μ3-OH, twelve ortho-tol, nitrate ions and methanol ligands. CrIII ions are six coordinated to form octahedral geometry, and DyIII ions are eight coordinated and in triangular dodecahedron geometry (Fig. 26). Antiferromagnetic interaction was dominant between the metal centers. M vs. H plots based on the single crystals in micro-SQUID revealed steps in the hysteresis curve analogous to the first Dy3-based triangular toroidal system. In fact, complex 60a displayed better coercivity compared with the Dy3 analogue, which was due to the coupling between the two {DyIII3} triangles.165,166 Interestingly, it was found that the CrIII ion did not play any role in the coupling of the two toroidal wheels and the coupling was due to the dipolar interactions between the two DyIII3 wheels. This phenomenon was supported by theoretical investigations.
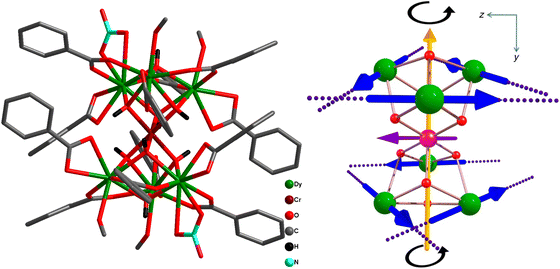 |
| Fig. 26 Molecular structure of complex 60a (left) and orientation of the individual magnetic anisotropy axes. Black arrows denote the rotation of the toroidal magnetic moment and the yellow arrow depicts S6 symmetry axis (right).164 Reprinted with permission from ref. 164. Copyright 2017 Nature. | |
For further analysis, toroidal and ferrotoroidal systems consisting of different LnIII centers, [CrIIILnIII6(OH)8(o-tol)12(NO3)(MeOH)5]·2MeOH (Ln = Tb (60b), Ho (60c) and Er (60d), o-tol = o-toluate) were synthesized and studied. Structural parameters were analogous to the 60a analogue, where a heptanuclear complex comprising two Ln3 triangular motifs was joined together by a common CrIII ion. {CrTb6} and {CrEr6} analogues revealed slow relaxation of magnetization under 3000 Oe static magnetic field. Magnetization measurements based on single crystals using μ-SQUID showed a hysteresis loop below 0.03 K for {CrTb6} and {CrHo6} analogues (sweep rate = 0.14 Ts−1). Ab initio calculations confirmed toroidal magnetic moments for Tb and Ho analogues. The two Ln3 triangles coupled together to stabilize the con-rotating ferrotoroidal ground state in these complexes. Interestingly, this represented the first toroidal behavior in non-DyIII complexes.167
Under solvothermal conditions, four isostructural LnIII6CrIII complexes {(Ln6Cr)(C8A)2} compounds [Ln = Gd (61a), Tb (61b), Dy (61c) and Tm (61d)] (H8C8A = p-tert-butylcalix[8]arene) were synthesized. A sandwich-like structure was formed where the GdIII6CrIII core was capped between two tail-to-tail calix[8]arene molecules. This double-cone arrangement binds a GdIII ion utilizing the four phenolic oxygen atoms at the lower rim such that a secondary building unit (SBU) of Gd2-C8A is formed. An additional GdIII ion placed at the joint of two conic units and coordinated by two phenolic oxygen atoms of both conic units forms a Gd3-C8A unit. One CrIII ion shares the common vertex of these two Gd3-C8A entities, forming a Gd3Cr-C8A motif. Overall, the heptanuclear core (Gd6Cr)-(C8A)2 is formed with the collective action of the two water molecules and two μ4-O2− bridging the Gd3-C8A and Gd3Cr-C8A motif. 61b shows SMM behavior due to the presence of temperature-dependent and frequency-dependent out-of-phase ac signals, with Ueff of 12.6 cm−1 and τ0 being 7.52 × 10−8 s.168
Representative examples of the heterometallic heptanuclear complexes and their magnetic responses are depicted in Fig. 27.169–172
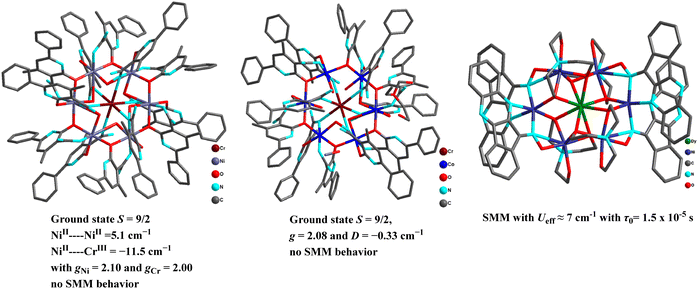 |
| Fig. 27 Representative examples of heterometallic heptanuclear complexes and their magnetic properties.169–171 | |
Utilizing maleic acid as a ligand, a heterometallic complex [{Na(H2O)][Na2Cr2Tb3(μ3-OH)6(L)6(H2O)17}]·16H2O (where H2L = maleic acid) (62) was synthesized. The maleic ligand adopted two coordination modes: μ3–κ1:κ1:κ1 and μ4–κ1:κ1:κ1:κ1 which coordinates to three and four metal ions, respectively. In μ3-mode, one carboxylate group binds to TbIII, CrIII and NaI ions while the other carboxylate group remains free. In μ4-mode, the same coordination mode as that of μ3 is present except the second carboxylate group coordinates to one mononuclear half-occupied NaI ion. It was found that each [Na2Cr2Tb3(μ3-OH)6(L)6(H2O)17]− anion moiety acts as linker and connects to three mononuclear NaI nodes. Dc magnetic studies revealed ferromagnetic interaction between the metal ions. Slow magnetic relaxation for this analogue was observed below 3 K without achieving any maxima in out-of-phase signals.173
5.7 Octanuclear complexes
In 2010, Powell and coworkers reported the first CrIII-containing 3d–4f SMM, [CrIII4DyIII4(μ3-OH)4(μ-N3)4(mdea)4(piv)4]·3CH2Cl2 (10) (H2mdea = N-methyl diethanolamine, HPiv = pivalic acid). This was synthesized by a one-pot reaction involving H2mdea and NaN3 with CrCl2 in CH3CN under an inert atmosphere, followed by addition of Dy(NO3)3·6H2O, pivalic acid, and CH2Cl2. The basic structural motif was composed of a Dy4-square within-a-Cr4-square, where the central core consisted of four DyIII ions to form an approximate square. Each pair of adjacent DyIII cations was bridged by a hydroxo ligand, which also coordinated with a CrIII ion on each edge of the Dy4 square. The opposite face of μ3-OH bridge was occupied by an azide ligand with end-on bridging mode to connect each Dy⋯Dy edge. Each CrIII was chelated by a doubly deprotonated (mdea)2−, the oxygen atoms of which bridged to the adjacent DyIII cations. Peripheral ligation was provided by the eight syn,syn-bridging pivalate ligands, which linked adjacent pairs of CrIII and DyIII cations (Fig. 28).
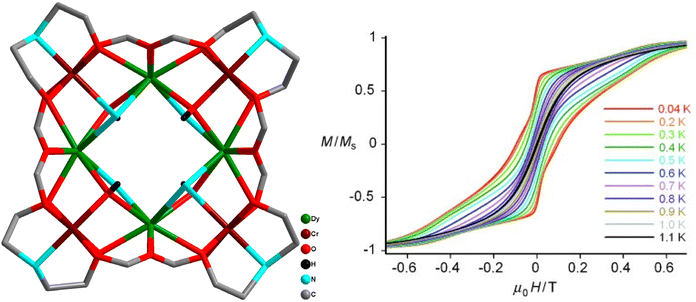 |
| Fig. 28 Molecular structure of 10 (left) and hysteresis plot at different temperatures with a sweep rate of 0.035 Ts−1 (right).80 Reprinted with permission from ref. 80. Copyright 2010 John Wiley and Sons. | |
The complex 10 displayed SMM behaviour with the energy barrier of 15 K and pre-exponential factor τ0 = 1.9 × 10−7 s. The hysteresis loops for 10 were obtained in micro-SQUID analysis for single crystals in the range of 0.04–1.1 K (Fig. 28). Cole–Cole plot indicated (α ≈ 0.5) more than one relaxation process in the range of 1.9–2.2 K. However, the relaxation dynamics remains almost unchanged with the application of a small dc field, indicating the relaxation processes are not influenced by QTM above 1.8 K. Ab initio calculations suggested that axial-type strong magnetic anisotropy was present on four DyIII ions, responsible for SMM behaviour of 10 (CrIII ions are almost isotropic). The calculations also demonstrated that the steps in the hysteresis loops in this molecule were due to the crossing of low-lying energy spectrum arising from the exchange multiplets involving Dy–Cr, Dy–Dy, and Cr–Cr exchange interactions. These low-lying energy states allowed the QTM among them to feature steps in the hysteresis curve.80,174 Powell and co-workers carried out a detailed analysis based on single-crystal SQUID and torque magnetometry to locate the anisotropy axes for this complex in 2019. These experiments allowed them to find the easy axis anisotropy on DyIII sites.174
The same group has extended their work replacing the N-methyldiethanolamine (H2mdea) ligand with N-n-butyldiethanolamine (H2bdea) to produce the analogous square-in-square topologies, [CrIII4LnIII4(bdea)4(μ3-N3)x(μ3-OH)4−x(μ-NO3)2(μ,η2-piv)2(piv)8]; Ln = La, x = 0.9, (63a); Ln = Ce, x = 1, (63b); Ln = Pr, (63c); Ln = Nd, x = 0.9, (63d); Ln = Sm, x = 0.7, (63e); Ln = Eu, x = 0.6, (63f); Ln = Gd, (63g). Here it is noteworthy to mention that size of the LnIII ions can direct the self-assembly of CrIIInLnIIIn complexes. It is evident that 63a–g were obtained for the light to middleweight lanthanides with comparatively large radius. The smaller radius LnIII ions such as Dy, Y, Ho, and Er, lead to {Cr3Ln3} hexanuclear “triangle-in-triangle” complexes, which has been discussed in hexanuclear section. Moreover, the smallest lanthanides such as TmIII and YbIII led to tetranuclear {Cr2Ln2} butterfly complexes. DC magnetic measurements exhibited ferromagnetic behaviour below 10 K for 63b, 63d and 63e, while antiferromagnetic interaction prevailed for 63c at low temperature. For 63aχT drops below 5 K, indicating weak antiferromagnetic exchange interaction among the CrIII ions as La is diamagnetic. The authors have speculated that for late lanthanides the CrIII–LnIII interaction is antiferromagnetic, whereas for the early lanthanides it is ferromagnetic irrespective of their arrangements (square, triangular or dimeric).77
Murray and co-workers reported another octanuclear “square-in-square” motif containing a heterometallic {Cr4Dy4} core (64) analogous to 10 through the reaction of CrCl3·6H2O, Dy(NO3)3·6H2O, benzoic acid, mdeaH2 (N-methyldiethanolamine), NaF and NEt3 in MeCN.175 The basic structural motif of [CrIII4DyIII4F4(OMe)1.12(OH)2.88(O2CPh)8(mdea)4] (64) is analogous to that of [CrIII4DyIII4(μ3-OH)4(N3)4(mdea)4(piv)4] (10) with some minor changes in the coordinating ligand (Fig. 29). The μ3-bridged anions throughout the crystal are disordered and modelled as MeO− and HO− in the ratio of 0.28 (MeO−):0.72(HO−). Here it is important to mention that the structural differences in 64 were due to the coordination of benzoic acid and F− anions, whereas in 10 it was pivalic acid and N3− anions. It is noteworthy that the authors attempted to replace the μ3-OH bridges with F− anions in their butterfly {CrIII2DyIII2} families in order to study the effect of anions on the magnetic relaxation, but during the self-assembly process they ended up with the octanuclear {CrIII4DyIII4} cluster.
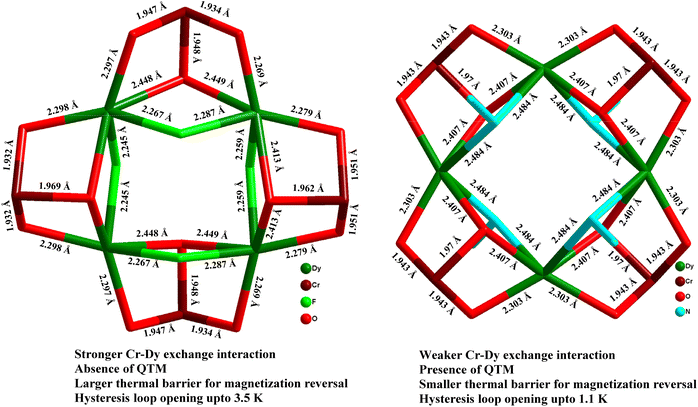 |
| Fig. 29 Comparison of bond lengths and magnetic properties in complexes 64 and 10.77,175 | |
The anions played a pivotal role in modulating the magnetic properties of 64 compared with 10. The magnetic properties were altered in the following ways: (i) CrIII⋯DyIII exchange interaction was found to be stronger in 64, and as a consequence the effect of QTM was less effective in 64; (ii) these combined effects essentially led to the larger thermal barrier for the magnetization reversal in 64 (Ueff = 54.9 cm−1 with τ0 = 6.1 × 10−8 s) over 39 (Ueff = 10.4 cm−1 and τ0 = 1.9 × 10−7 s); (iii) the hysteresis loop opening for 64 was up to 3.5 K using a conventional magnetometer (scan rate 0.003 Ts−1), whereas for 10 it was up to 1.1 K in micro-SQUID (scan rate 0.035 Ts−1); (iv) complex 64 displayed a blocking temperature (relaxation of 100 s) of 3.8 K, which is greater than that of complex 10 (∼1 K). From these instances, it is evident that electronegative bridging fluoride ions have significant influence on the anisotropy barrier and as well as magnetization relaxation. This phenomenon has also been reflected in other fluoride-bridged lanthanide analogues.101,176–180
With extension of their previous work, Murray and co-workers reported isostructural “square-in-square” topology with different co-ligands; [CoIII4DyIII4(μ-OH)4(μ3-OMe)4(O2CC(CH3)3)4(tea)4(H2O)4]·4H2O (65) and [CoIII4DyIII4(μ-F)4(μ3-OH)4(o-tol)8(mdea)4]3H2O·EtOH·MeOH (13), [CrIII4DyIII4(μ-F4)(μ3-OMe)1.25(μ3-OH)2.75(O2CPh)8(mdea)4] (11) [CrIII4DyIII4(μ3-OH)4(μ-N3)4(mdea)4(piv)4] (10) (tea3− = triply deprotonated triethanolamine; mdea2− = doubly deprotonated N-methyldiethanolamine; o-tol = o-toluate).78 Apart from 65, the other three compounds 13, 11 and 10 displayed SMM behaviour with an energy barrier Ueff of 39.0 cm−1, 55.0 cm−1 and 10.4 cm−1, respectively. In order to understand the differences observed in the energy barrier the authors performed CASSCF/RASSI-SO/POLY_ANISO calculations on the complexes to understand the role played by exchange interaction as well as the mechanism of the magnetic relaxation phenomenon. The calculations revealed that the exchange interaction (JDy–Dy) is −0.16, 1.6 and 2.8 cm−1 for 65, 13 and 11, respectively, whereas the JDy–Cr interaction is 1.8 cm−1 for complex 11. The substitution of the hydroxide ion by fluoride ion quenches the QTM significantly, leading to better-quality SMM properties for 13 in comparison with 65. Additionally, the incorporation of paramagnetic CrIII instead of diamagnetic CoIII leads to strengthening of the CrIII⋯DyIII coupling, resulting in quenching of QTM at low temperatures for complexes 11 and 10. Complex 11 exhibited the best SMM behavior due to the presence of highly electronegative F− ions and stronger CrIII⋯DyIII exchange interaction to quench the QTM. The presence of the most electronegative F− ions pushes the excited states higher in energy due to prominent electrostatic repulsion, and therefore the relaxation of magnetization occurs through the higher energy barrier.100 As a consequence, magnetic hysteresis loops above 2 K (sweep rate 0.003 Ts−1) were observed for 11.
Analogous “Dy4-square inscribed in a M4-square” topology can be observed with other 3d metal ions i.e. [MnIII4DyIII4(μ3-OH)4(μ-X)4(O2CBut)8(tBu-dea)4], X = N3− (66a), OCN− (66b), NO3− (66c);181 [FeIII4DyIII4(μ3-OH)4(O2CC6H4CH3)12(n-bdea)4] (67).182 The MnIII4DyIII4 analogue displayed frequency-dependent in-phase and out-of-phase signals below 4 K without maxima, indicating slow relaxation of the magnetization. On the contrary, complex 67 did not display any ac susceptibility responses either in zero or nonzero (500–3000 Oe) dc field, indicating the non-SMM nature of the same. In fact, the change of bridging ligand X− (N3− → OCN− → NO3−) also did not significantly affect the magnetic properties of the [FeIII4DyIII4] analogue. Chandrasekhar and co-workers reported analogous topology for a series of NiII–LnIII complexes [Ln4Ni4(H3L)4(μ3-OH)4(μ2-OH)4](Cl)4 using N1,N3-bis(6-formyl-2-(hydroxymethyl)-4-methylphenol)diethylenetriamine (H5L).183 Similar “square-in-square”-based structures have also been found in a series of complexes [MnIII4LnIII4(OH)4(Calix)4(NO3)2(DMF)6(H2O)6], Ln = Gd (68a), Tb (68b), Dy (68c) by using methylene-bridged calix[4]arenes.184,185
Apart from the aforementioned “square-in-square” structural topology, Xu and co-workers reported two couples of chiral “tower-like” Ln4Cr4 complexes formulated as L- and D-[Gd4Cr4(IN)10(μ3-OH)4(μ4-O)4(H2O)12]·[IN]2·8H2O (69a and 69b), L- and D-[Dy4Cr4(IN)11(μ3-OH)4(μ4-O)4(H2O)8]·[IN]·1.5H2O (70a and 70b) (HIN = isonicotinic acid). Four μ3-OH and four μ4-O connect four LnIII ions and four CrIII ions to form a “tower-like” [Ln4Cr4] (Ln = Gd, Dy) metal skeleton. The Gd analogue (the mixture of 69a and 69b) showed the magnetocaloric effect with −ΔSm = 18.08 J kg−1 K−1 at 3 K with ΔH = 7 T.141
To explore the effect of nicotinic acid (HNA) instead of isonicotinic acid (HIN) as ligand, Xu and co-workers reported three-dimensional 3d–4f heterometallic cluster-based coordination polymers, [Ln4Cr4(μ3-O)4(μ4-O)4(NA)8(H2O)12], [Ln = Gd (71a), Tb (71b), Er (71c)] through hydrothermal synthesis. Structural analysis showed that the basic unit of 71a was composed of butterfly-shaped Gd4Cr4 clusters where four Gd atoms and four Cr atoms were interconnected by four μ3-O2− and four μ4-O2−to generate a Gd4Cr4O8 metal skeleton. Further analysis of the core structure revealed that the cubane-like core Cr4O4 connects with two distorted cubane-like subunits Cr2Gd2O4via a shared face. The adjacent Gd4Cr4 units are interconnected through NA− ligands to generate a 3D structure. The Gd analogue displayed the magnetic entropy change (−ΔSmaxm) value of 22.05 J K−1 kg−1 at 2.5 K for the applied field of 70 kOe.186
Zheng and co-workers utilized trilacunary Keggin-type polyoxometalate Na10[α-SiW9O34]·16H2O to assemble a series of {Cr4Ln4} heterometallic clusters with the formula of Cs2[Cr4Ln4(μ4-O)4(μ3-O)4(C8H4O4)4(H2O)12](H3SiW12O40)Cl·23H2O [Ln = Ce (72a), Pr (72b), Nd (72c)] and [Cr4Ln4(μ4-O)4(μ3-O)4(C8H4O4)4(H2O)10](H6SiW12O40)Cl2·18H2O, (C8H4O4 = dianion of phthalic acid), [Ln = Sm (73a), Eu (73b), Gd (73c), Tb (73d), Dy (73e), Ho (73f), Er (73g)].79 These compounds were composed of four-layered prismatic[Cr4Ln4] cores where Cr–Ln heterometallic clusters were stabilized by both organic ligands (phthalic acid) and inorganic POM species like Na10[α-SiW9O34]·16H2O. It is notable that Xu and co-workers also reported CrIII–LnIII discrete as well as 3-D polymeric clusters containing similar four-layered [Cr4Ln4(μ4-O)4(μ3-O)4] (Ln = Gd, Dy) cores. The authors selectively measured the magnetic properties of the Tb (73d) and Dy (73e) analogue. Only analogue 73e showed a signature of slow relaxation of magnetization behaviour without peak maxima in variable-temperature ac susceptibility measurements.
5.8 Dodecanuclear complexes
As mentioned in the heptanuclear section (vide supra), [Gd8Cr4(H2L)4(OAc)8(μ3-OH)16(μ4-O)1(H2O)8](Cl)(ClO4)5·10H2O (59a) and [Dy8Cr4(H2L)4(OAc)8(μ3-OH)16(μ4-O)1(H2O)8](Cl)(ClO4)5·10H2O (59b) were formed utilizing a procedure similar to complexes 58a–b but using CrCl3 instead of Cr(OAc)3.140 Incorporation of Cl− ions in combination with ClO4− ions as a mixed anion template led to the high-nuclearity Ln8Cr4 clusters. Complex 59a consisted of one quadrangular cationic core of [Gd8Cr4(H2L)4(OAc)8(μ3-OH)16(μ4-O)1(H2O)8]6+ which can be described as four cubane-like units [Gd3Cr(μ3-OH)4]8+ templated by Cl− and ClO4− anion through the sharing of the GdIII vertex center with a central μ4-O group (Fig. 30). Weak antiferromagnetic interaction for both Gd⋯Cr and Gd⋯Gd was found for complex 59a. Magnetothermal analysis revealed magnetic entropy changes of 33.8 J kg−1 K−1 at 2 K and ΔH = 7 T for this complex 59a.
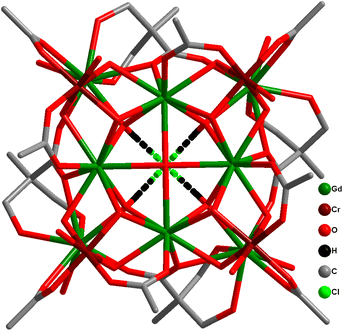 |
| Fig. 30 Molecular structure of complex 59a.140 | |
Solvothermal conditions using N-substituted diethanolamine ligand yielded dodecanuclear CrIII–LnIII-based coordination clusters, [CrIII6LnIII6(μ3-OH)8(tbdea)6(C6H5COO)16]·2H2O [Ln = Dy (74a), Y (74b)].82 To rationalize the role of the diamagnetic metal ion in the vicinity of LnIII centers on the magnetic properties, the analogous cobalt complex [CoIII6DyIII6(μ3-OH)8(nbdea)6(m-CH3C6H4COO)16]·2H2O·2CH3CN (75) was synthesized. All three compounds have an analogous dodecanuclear {MIII6LnIII6} core (M = Cr, Co; Ln = Dy, Y), differing only in the ligand and coligand. For 74a, the {CrIII6DyIII6} core was formed by two {CrIII3DyIII3} sub-units in the form of a “Dy3 triangle within a Cr3 triangle” geometry where Cr1 and Cr2 are placed on one side of the Dy3 triangle with Cr3 on the other side. In the {CrIII3DyIII3} subunit, three Dy atoms are bridged by μ3-hydroxide in the central position. Each CrIII ion is chelated with a doubly deprotonated ligand along with two alkoxide oxygens, forming an alkoxo bridge to an adjacent DyIII ion. Six syn–syn bridging benzoate ligands connect the adjacent DyIII and CrIII centers around the edge of the subunits (Fig. 31). Weak ferromagnetic CrIII⋯DyIII interaction dominates in the [CrIII6DyIII6] complex, whereas an intramolecular antiferromagnetic interaction between the adjacent DyIII centers is found in the [CoIII6DyIII6] analogue. The ac magnetic susceptibilities for [CrIII6DyIII6] (74a) and [CoIII6DyIII6] (75) exhibited slow relaxation of magnetization with the energy gap of 8.9 cm−1 and 14.46 cm−1, respectively, which indicated that the replacement of paramagnetic 3d ions with diamagnetic CoIII ion led to quenching of the QTM and enhanced the energy barrier. A similar improvement of SMM behavior by incorporating diamagnetic metal ions adjacent to lanthanide ions was also found in other 3d–4f heterometallic complexes.37,187–189
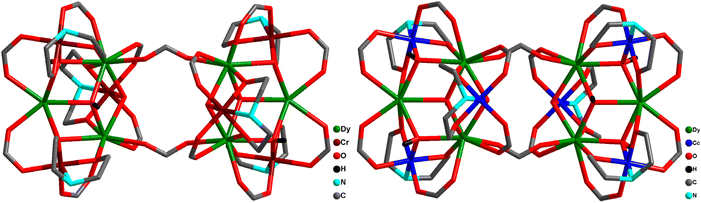 |
| Fig. 31 Molecular structure of complexes with [CrIII6DyIII6] (left) and [CoIII6DyIII6] (right) cores.82 | |
In the category of [M6Ln6]-based complexes, literature reports show two reports, [Zn6Dy6] (76)-based core using 2-(hydroxy-N-(2-(2-hydroxybenzylidene)amino)ethyl)benzamide (H3L) and [Ni6Gd6] complex (77) using phosphonic acid (Fig. 32). Complex [Zn6Dy6] (76) exhibited SMM behaviour without reaching peak maxima in dynamic magnetic measurements,190 whereas [Ni6Gd6] complex (77) displayed MCE with −ΔSmaxm is 26.5 J kg−1 K−1 at 3 K and ΔH = 7 T.191
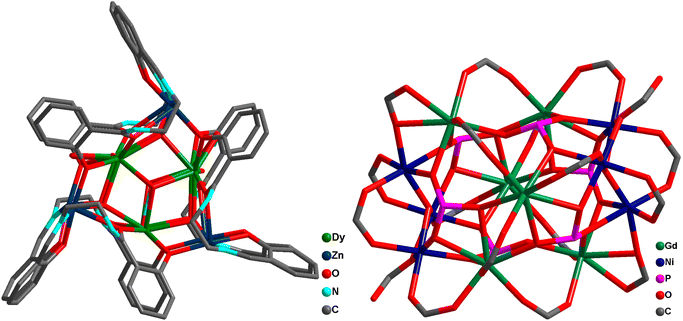 |
| Fig. 32 Simplified view of structural cores for [Zn6Dy6] (left) and [Ni6Gd6] (right).190,191 | |
Hydrothermal conditions afforded two isomorphic heterometallic {Ln8Cr4} complexes formulated as [Gd8Cr4(IN)18(μ3-O)2(μ3-OH)6(μ3-O)4(H2O)10]·13H2O (78a) and [Tb8Cr4(IN)18(μ3-O)2(μ3-OH)6(μ3-O)4(H2O)10]·13H2O (78b); (HIN = isonicotinic acid). The structural unit of {Gd8Cr4} is composed of two {Ln4Cr2} “drumlike” cores linked by ligand HIN. Even though the two building blocks {Ln4Cr2} share the same metallic framework, they differ in the coordination mode provided by the ligand (Fig. 33). Each unit {Gd8Cr4} is linked by ligand HIN, forming a rare one-dimensional wave-like structure. Antiferromagnetic interaction is dominant between the LnIII ion and CrIII ion. Also, the entropy change for 78a was found to be −ΔSmaxm = 23.40 J kg−1 K−1 at 3 K and ΔH = 7 T.138
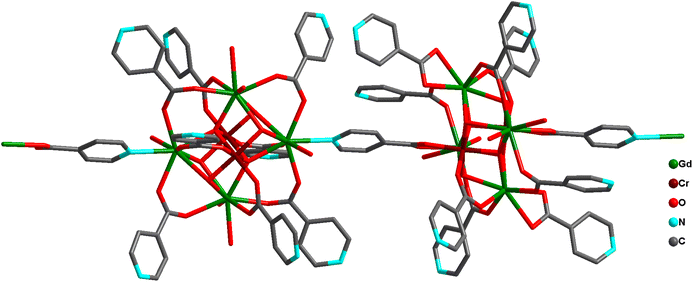 |
| Fig. 33 Molecular structure of complex 78a. | |
Representative examples in the family of heterometallic dodecanuclear complexes and the magnetic properties are listed in Fig. 34.192,193
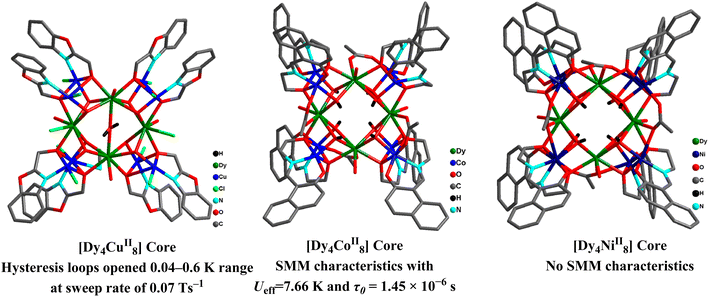 |
| Fig. 34 Representative examples of heterometallic dodecanuclear complexes and their magnetic properties.192,193 | |
5.9 Hexadecanuclear complexes
The largest CrIII–LnIII complex [Cr8Ln8(mdea)16(CH3COO)8(NO3)8] (mdeaH2 = N-methyldiethanolamine, LnIII = Gd (79a), Dy (79b), and Y (79c), respectively) consists of sixteen alternating Cr and Ln metal centers. The solvothermal conditions involving N-methyldiethnanolamine, Cr(acac)3, Ln(NO3)3 in actonitrile at 130 °C allowed the formation of hexadecanculear complexes. All eight Cr ions and eight Gd ions are arranged alternately to form a sixteen-membered ring (Fig. 35). Dc magnetic studies showed dominant antiferromagnetic interaction in Gd and Dy analogues and weak ferromagnetic interaction in the Y analogue. The presence of a large ground spin state (ST = 16) as well as the antiferromagnetic exchange interaction ∼1.5 cm−1 for the [Cr8Gd8] analogue is confirmed by Quantum Monte Carlo (QMC) simulation. Moreover, ac magnetic studies show slow relaxation of magnetization for 79b with Ueff of 13.2 cm−1 and τ0 = 3.5 × 10−8 s.83
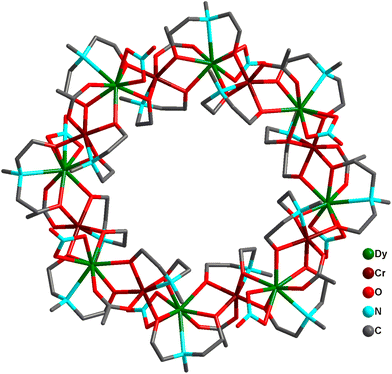 |
| Fig. 35 Molecular structure of complex 79b. | |
In the family of heterometallic hexadecanuclear 3d–4f complexes, Winpenny and coworkers reported [CoII8GdIII8] complexes, [CoII8GdIII8(μ3-OH)4(NO3)4(O3PtBu)8(O2CtBu)16] (80) using both phosphonic acid and carboxylic acid ligands.194,195 This complex displayed the MCE value of −ΔSmaxm as 20.40 J kg−1 K−1 at 3 K and ΔH = 7 T.
5.10 Heterotrimetallic complexes
Cyano-bridged hetero-di/trimetllic complexes have been reported using [Cr(CN)6]3− as the connector to generate the multi-dimensional coordination networks.196 Ohba and coworkers have reported trimetallic 3-dimensional pillared-layered complexes [Co2Ln(L)2(H2O)4][Cr(CN)6] [LaIII (81a) and GdIII (81b)] by connecting preformed CoII–LnIII complexes with K3[Cr(CN)6] (Fig. 36). Complex LaIII (81a) shows metamagnetic (Tc = 7.4 K) and complex GdIII (81b) shows ferromagnetic (Tc = 15.4 K) interaction.197
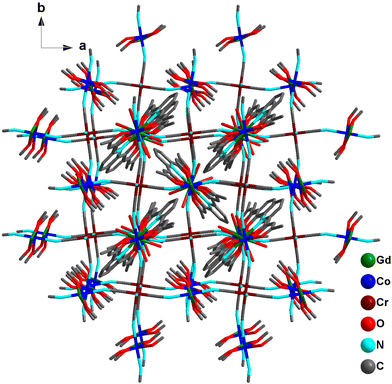 |
| Fig. 36 Three-dimensional coordination network for complex 81b along crystallographic c-axis. | |
6. Conclusion and outlook
In this review, we have attempted to demonstrate the magnetic and magnetocaloric aspects of CrIII–LnIII-based heterometallic complexes. To do so, we have categorically presented the importance of CrII and CrIII ions as sources of anisotropy and their coupling mechanism with LnIII ions. Moreover, we have also demonstrated the detailed synthetic, structural and SMM behaviour for most of the complexes. From the synthesis and structural discussion part, it is very obvious that all the CrIII–LnIII heterometallic assemblies were isolated using a serendipity-based approach where the multidentate ligands (Chart 1) have been employed to bridge the CrIII and LnIII centers to generate the multimetallic architectures. Furthermore, the magnetic property analysis divulged how the exchange interaction between CrIII and LnIII ions, through either the fluoride or oxide/hydroxide bridge, played a pivotal role in shaping the interesting SMM behaviour of those heterometallic complexes by minimizing the effect of QTM. In fact, the tetranuclear complex [CrIII2DyIII2(OMe)2(O2CPh)4(mdea)2(NO3)2]70 turns out to be an excellent SMM, having the strongest exchange interactions of −16.7 to −20.3 cm−1 and the highest blocking temperature (TB) of 3.7 K among the 3d–4f family of complexes reported to date. Despite the fact that some of the complexes exhibit impressive magnetic dynamics, it should be noted that the blocking barrier as well as temperature are still much lower than for the lanthanide-based single-ion magnets. Therefore, the main challenge remains in this field is to develop systems with a considerably higher blocking temperature (TB) and Ueff. We believe that these problems can be countered through strong exchange interactions between 3d and 4f metal ions as well as by preserving the high symmetry such as C∞, Cn (n ≥ 7), S8, D4d, D5h, D6hetc. around LnIII ions to lower the effect of problematic QTM.23,198 This essence and art of coupling between CrIII as well as other paramagnetic transition metals with LnIII spin centers can be modulated through the selection of specific coordination compartments ligands along with the preferential choice of coordinating entities such as more polarizable sulfur or selenium rather than hard fluoride or oxides (hydroxides).
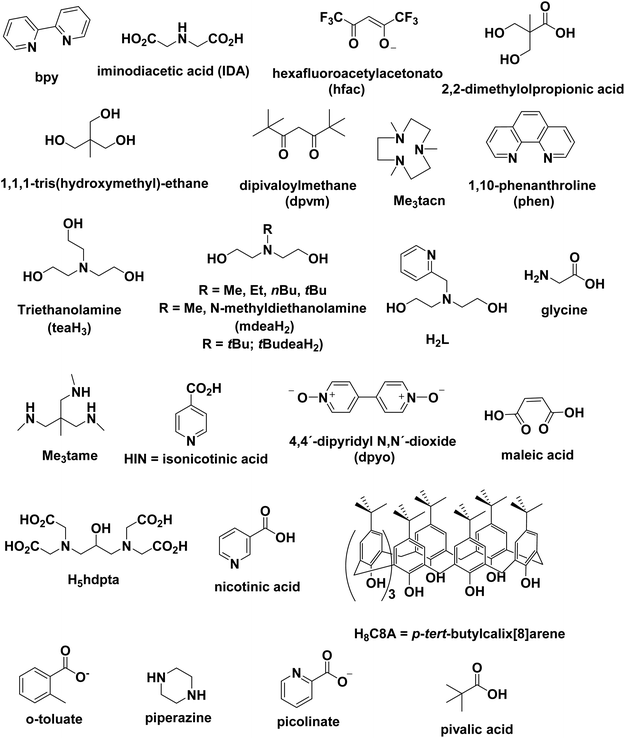 |
| Chart 1 Library of ligands used to synthesize CrIII–LnIII complexes. | |
The higher extent of polarizability associated with sulphur/selenium leads to larger degree of covalency in the bond. This essentially leads to more overlap between transition- and lanthanide-metal ions and leads to better exchange interactions between them. Moreover, the use of a radical ligand system as bridge could also mediate stronger exchange coupling between chromium and lanthanides, and hence a higher blocking barrier.199 Additionally, the judicious choice of ligands with specific coordination pockets for CrIII as well as LnIII centres could lead to the synthesis of interesting dinuclear/trinuclear heterometallic complexes which would provide a better platform to understand and manipulate the magnetization dynamics, rather than aesthetically pleasing bigger complexes often characterized by low Ueff values.109,200–206 In fact, to date, the SMM properties of the CrII–LnIII-based systems and surface-grafted CrIII–LnIII-based systems are yet to be explored. Therefore, stabilization of such systems through chemical modification also could unleash numerous potential avenues in heterometallic SMM chemistry.207–214 So, with all sorts of possibilities, we are expecting more exciting developments in the near future from the chemical community to address some of those unsolved problems regarding both chromium-containing and other transition metal–lanthanide heterometallic assemblies.
Conflicts of interest
There are no conflicts to declare.
Acknowledgements
A. D and P. B are grateful to the Gandhi Institute of Technology and Management (GITAM) and Panskura Banamali College (Autonomous), respectively. S. D acknowledges the financial support from the SERB-DST Early Career Research Award (ECR) with Project Number ECR/2016/001746.
References
- R. Sessoli, H. L. Tsai, A. R. Schake, S. Wang, J. B. Vincent, K. Folting, D. Gatteschi, G. Christou and D. N. Hendrickson, High-spin molecules: [Mn12O12(O2CR)16(H2O)4], J. Am. Chem. Soc., 1993, 115, 1804–1816 CrossRef CAS
.
- R. Sessoli, D. Gatteschi, A. Caneschi and M. A. Novak, Magnetic bistability in a metal-ion cluster, Nature, 1993, 365, 141–143 CrossRef CAS
.
-
M. Holynska, Single-Molecule Magnets: Molecular Architectures and Building Blocks for Spintronics, Wiley–VCH Verlag GmbH & Co. KGaA, 2015 Search PubMed
.
- G. Aromí, D. Aguilà, P. Gamez, F. Luis and O. Roubeau, Design of magnetic coordination complexes for quantum computing, Chem. Soc. Rev., 2012, 41, 537–546 RSC
.
- A. Gaita-Ariño, F. Luis, S. Hill and E. Coronado, Molecular spins for quantum computation, Nat. Chem., 2019, 11, 301–309 CrossRef PubMed
.
-
D. Gatteschi, R. Sessoli and J. Villain, Molecular Nanomagnets, Oxford University Press, Oxford, New York, 2006 Search PubMed
.
-
G. Aromí, E. K. Brechin and R. E. P. Winpenny, Structure and Bonding, Springer-Verlag, Berlin Heidelberg, 2006 Search PubMed
.
- W. Wernsdorfer and R. Sessoli, Quantum Phase Interference and Parity Effects in Magnetic Molecular Clusters, Science, 1999, 284, 133–135 CrossRef CAS
.
- T. Leviant, A. Keren, E. Zeldov and Y. Myasoedov, Quantum ignition of deflagration in the Fe8 molecular magnet, Phys. Rev. B: Condens. Matter Mater. Phys., 2014, 90, 134405 CrossRef
.
- R. E. P. Winpenny, Quantum Information Processing Using Molecular Nanomagnets As Qubits, Angew. Chem., Int. Ed., 2008, 47, 7992–7994 CrossRef CAS PubMed
.
- E. Moreno-Pineda and W. Wernsdorfer, Measuring molecular magnets for quantum technologies, Nat. Rev. Phys., 2021, 3, 645–659 CrossRef CAS
.
- M. Shiddiq, D. Komijani, Y. Duan, A. Gaita-Ariño, E. Coronado and S. Hill, Enhancing coherence in molecular spin qubits via atomic clock transitions, Nature, 2016, 531, 348–351 CrossRef CAS PubMed
.
- L. Bogani and W. Wernsdorfer, Molecular spintronics using single-molecule magnets, Nat. Mater., 2008, 7, 179–186 CrossRef CAS PubMed
.
- P. Kumar Sahu, R. Kharel, S. Shome, S. Goswami and S. Konar, Understanding the unceasing evolution of Co(II) based single-ion magnets, Coord. Chem. Rev., 2023, 475, 214871 CrossRef CAS
.
- C. Papatriantafyllopoulou, E. E. Moushi, G. Christou and A. J. Tasiopoulos, Filling the gap between the quantum and classical worlds of nanoscale magnetism: giant molecular aggregates based on paramagnetic 3d metal ions, Chem. Soc. Rev., 2016, 45, 1597–1628 RSC
.
- S. Gómez-Coca, D. Aravena, R. Morales and E. Ruiz, Large magnetic anisotropy in mononuclear metal complexes, Coord. Chem. Rev., 2015, 289–290, 379–392 CrossRef
.
- G. A. Craig and M. Murrie, 3d single-ion magnets, Chem. Soc. Rev., 2015, 44, 2135–2147 RSC
.
- M. Murrie, Cobalt(II) single-molecule magnets, Chem. Soc. Rev., 2010, 39, 1986–1995 RSC
.
- J. M. Frost, K. L. M. Harriman and M. Murugesu, The rise of 3-d single-ion magnets in molecular magnetism: towards materials from molecules?, Chem. Sci., 2016, 7, 2470–2491 RSC
.
- Y.-S. Meng, S.-D. Jiang, B.-W. Wang and S. Gao, Understanding the Magnetic Anisotropy toward Single-Ion Magnets, Acc. Chem. Res., 2016, 49, 2381–2389 CrossRef CAS
.
- A. K. Bar, C. Pichon and J.-P. Sutter, Magnetic anisotropy in two- to eight-coordinated transition–metal complexes: Recent developments in molecular magnetism, Coord. Chem. Rev., 2016, 308, 346–380 CrossRef CAS
.
- A. Borah and R. Murugavel, Magnetic relaxation in single-ion magnets formed by less-studied lanthanide ions Ce(III), Nd(III), Gd(III), Ho(III), Tm(II/III) and Yb(III), Coord. Chem. Rev., 2022, 453, 214288 CrossRef CAS
.
- J.-L. Liu, Y.-C. Chen and M.-L. Tong, Symmetry strategies for high performance lanthanide-based single-molecule magnets, Chem. Soc. Rev., 2018, 47, 2431–2453 RSC
.
- F.-S. Guo, A. K. Bar and R. A. Layfield, Main Group Chemistry at the Interface with Molecular Magnetism, Chem. Rev., 2019, 119, 8479–8505 CrossRef CAS
.
- F. Pointillart, O. Cador, B. Le Guennic and L. Ouahab, Uncommon lanthanide ions in purely 4f Single Molecule Magnets, Coord. Chem. Rev., 2017, 346, 150–175 CrossRef CAS
.
- S. G. McAdams, A.-M. Ariciu, A. K. Kostopoulos, J. P. S. Walsh and F. Tuna, Molecular single-ion magnets based on lanthanides and actinides: Design considerations and new advances in the context of quantum technologies, Coord. Chem. Rev., 2017, 346, 216–239 CrossRef CAS
.
- Z. Zhu and J. Tang, Metal–metal bond in lanthanide single-molecule magnets, Chem. Soc. Rev., 2022, 51, 9469–9481 RSC
.
- F. Habib and M. Murugesu, Lessons learned from dinuclear lanthanide nano-magnets, Chem. Soc. Rev., 2013, 42, 3278–3288 RSC
.
- M. Feng and M.-L. Tong, Single Ion Magnets from 3d to 5f: Developments and Strategies, Chem. – Eur. J., 2018, 24, 7574–7594 CrossRef CAS PubMed
.
- Z. Zhu, M. Guo, X.-L. Li and J. Tang, Molecular magnetism of lanthanide: Advances and perspectives, Coord. Chem. Rev., 2019, 378, 350–364 CrossRef CAS
.
- D. N. Woodruff, R. E. P. Winpenny and R. A. Layfield, Lanthanide Single-Molecule Magnets, Chem. Rev., 2013, 113, 5110–5148 CrossRef CAS PubMed
.
- A. Dey, P. Bag, P. Kalita and V. Chandrasekhar, Heterometallic CuII–LnIII complexes: Single molecule magnets and magnetic refrigerants, Coord. Chem. Rev., 2021, 432, 213707 CrossRef CAS
.
- J. Wang, M. Feng, M. N. Akhtar and M.-L. Tong, Recent advance in heterometallic nanomagnets based on TMxLn4−x cubane subunits, Coord. Chem. Rev., 2019, 387, 129–153 CrossRef CAS
.
- L. K. Thompson and L. N. Dawe, Magnetic properties of transition metal (Mn(II), Mn(III), Ni(II), Cu(II)) and lanthanide (Gd(III), Dy(III), Tb(III), Eu(III), Ho(III), Yb(III)) clusters and [nxn] grids: Isotropic exchange and SMM behaviour, Coord. Chem. Rev., 2015, 289–290, 13–31 CrossRef CAS
.
- M. Andruh, J.-P. Costes, C. Diaz and S. Gao, 3d−4f Combined Chemistry: Synthetic Strategies and Magnetic Properties, Inorg. Chem., 2009, 48, 3342–3359 CrossRef CAS PubMed
.
- C. Benelli and D. Gatteschi, Magnetism of Lanthanides in Molecular Materials with Transition-Metal Ions and Organic Radicals, Chem. Rev., 2002, 102, 2369–2388 CrossRef CAS PubMed
.
- A. Chakraborty, J. Goura, P. Kalita, A. Swain, G. Rajaraman and V. Chandrasekhar, Heterometallic 3d–4f single molecule magnets containing diamagnetic metal ions, Dalton Trans., 2018, 47, 8841–8864 RSC
.
- A. Chakraborty, J. Goura, P. Bag and V. Chandrasekhar, NiII-LnIII Heterometallic Complexes as Single-Molecule Magnets, Eur. J. Inorg. Chem., 2019, 2019, 1180–1200 CrossRef CAS
.
- A. Swain, A. Sen and G. Rajaraman, Are lanthanide-transition metal direct bonds a route to achieving new generation {3d–4f} SMMs?, Dalton Trans., 2021, 50, 16099–16109 RSC
.
- M. G. F. Vaz and M. Andruh, Molecule-based magnetic materials constructed from paramagnetic organic ligands and two different metal ions, Coord. Chem. Rev., 2021, 427, 213611 CrossRef CAS
.
- S. K. Langley, D. P. Wielechowski, V. Vieru, N. F. Chilton, B. Moubaraki, L. F. Chibotaru and K. S. Murray, The first 4d/4f single-molecule magnet containing a {RuIII2DyIII2} core, Chem. Commun., 2015, 51, 2044–2047 RSC
.
- A. Swain, R. Martin, K. R. Vignesh, G. Rajaraman, K. S. Murray and S. K. Langley, Enhancing the barrier height for magnetization reversal in 4d/4f RuIII2LnIII2 “butterfly” single molecule magnets (Ln = Gd, Dy) via targeted structural alterations, Dalton Trans., 2021, 50, 12265–12274 RSC
.
- A. Zabala-Lekuona, J. M. Seco and E. Colacio, Single-Molecule Magnets: From Mn12-ac to dysprosium metallocenes, a travel in time, Coord. Chem. Rev., 2021, 441, 213984 CrossRef CAS
.
- H.-L. Tsai, H. J. Eppley, N. de Vries, K. Folting, G. Christou and D. N. Hendrickson, Superparamagnetic-like properties of the valence-trapped MnMn7Mn4 anion in the salt (PPh4)[Mn12O12(O2CEt)16(H2O)4], J. Chem. Soc., Chem. Commun., 1994, 1745–1746, 10.1039/C39940001745
.
- A. L. Barra, P. Debrunner, D. Gatteschi, E. S. Ch and R. Sessoli, Superparamagnetic-like behavior in an octanuclear iron cluster, EPL, 1996, 35, 133 CrossRef CAS
.
- J. C. Goodwin, R. Sessoli, D. Gatteschi, W. Wernsdorfer, A. K. Powell and S. L. Heath, Towards nanostructured arrays of single molecule magnets: new Fe19 oxyhydroxide clusters displaying high ground state spins and hysteresis, J. Chem. Soc., Dalton Trans., 2000, 1835–1840, 10.1039/B002135K
.
- A. K. Boudalis, B. Donnadieu, V. Nastopoulos, J. M. Clemente-Juan, A. Mari, Y. Sanakis, J.-P. Tuchagues and S. P. Perlepes, A Nonanuclear Iron(II) Single-Molecule Magnet, Angew. Chem., Int. Ed., 2004, 43, 2266–2270 CrossRef CAS PubMed
.
- S. L. Castro, Z. Sun, C. M. Grant, J. C. Bollinger, D. N. Hendrickson and G. Christou, Single-Molecule Magnets: Tetranuclear Vanadium(III) Complexes with a Butterfly Structure and an S = 3 Ground State, J. Am. Chem. Soc., 1998, 120, 2365–2375 CrossRef CAS
.
- A. K. Powell, S. L. Heath, D. Gatteschi, L. Pardi, R. Sessoli, G. Spina, F. Del Giallo and F. Pieralli, Synthesis, Structures, and Magnetic Properties of Fe2, Fe17, and Fe19 Oxo-Bridged Iron Clusters: The Stabilization of High Ground State Spins by Cluster Aggregates, J. Am. Chem. Soc., 1995, 117, 2491–2502 CrossRef CAS
.
- S. Mukherjee, R. Bagai, K. A. Abboud and G. Christou, Raising the Spin of FeIII7 Disklike Clusters: The Power of Molecular Spin Frustration, Inorg. Chem., 2011, 50, 3849–3851 CrossRef CAS PubMed
.
- R. Bagai and G. Christou, The Drosophila of single-molecule magnetism: [Mn12O12(O2CR)16(H2O)4], Chem. Soc. Rev., 2009, 38, 1011–1026 RSC
.
- K. Weighardt, K. Pohl, I. Jibril and G. Huttner, Hydrolysis Products of the Monomeric Amine Complex (C6H15N3)FeCl3: The Structure of the Octameric Iron(III) Cation of {[(C6H15N3)6Fe8(μ3-O)2(μ2-OH)12]Br7(H2O)}Br·8H2O, Angew. Chem., Int. Ed. Engl., 1984, 23, 77–78 CrossRef
.
- F. Neese and D. A. Pantazis, What is not required to make a single molecule magnet, Faraday Discuss., 2011, 148, 229–238 RSC
.
- E. Ruiz, J. Cirera, J. Cano, S. Alvarez, C. Loose and J. Kortus, Can large magnetic anisotropy and high spin really coexist?, Chem. Commun., 2008, 52–54, 10.1039/B714715E
.
- O. Waldmann, A Criterion for the Anisotropy Barrier in Single-Molecule Magnets, Inorg. Chem., 2007, 46, 10035–10037 CrossRef CAS PubMed
.
- A. M. Ako, I. J. Hewitt, V. Mereacre, R. Clérac, W. Wernsdorfer, C. E. Anson and A. K. Powell, A Ferromagnetically Coupled Mn19 Aggregate with a Record S = 83/2 Ground Spin State, Angew. Chem., Int. Ed., 2006, 45, 4926–4929 CrossRef CAS PubMed
.
- A. M. Ako, V. Mereacre, R. Clérac, W. Wernsdorfer, I. J. Hewitt, C. E. Anson and A. K. Powell, A [Mn18Dy] SMM resulting from the targeted replacement of the central MnII in the S = 83/2 [Mn19]-aggregate with DyIII, Chem. Commun., 2009, 544–546, 10.1039/B814614D
.
- N. Ishikawa, M. Sugita, T. Ishikawa, S.-y. Koshihara and Y. Kaizu, Lanthanide Double-Decker Complexes Functioning as Magnets at the Single-Molecular Level, J. Am. Chem. Soc., 2003, 125, 8694–8695 CrossRef CAS PubMed
.
- S. Osa, T. Kido, N. Matsumoto, N. Re, A. Pochaba and J. Mrozinski, A Tetranuclear 3d−4f Single Molecule Magnet: [CuIILTbIII(hfac)2]2, J. Am. Chem. Soc., 2004, 126, 420–421 CrossRef CAS PubMed
.
- K. Liu, W. Shi and P. Cheng, Toward heterometallic single-molecule magnets: Synthetic strategy, structures and properties of 3d–4f discrete complexes, Coord. Chem. Rev., 2015, 289–290, 74–122 CrossRef CAS
.
- J.-P. Sutter, V. Béreau, V. Jubault, K. Bretosh, C. Pichon and C. Duhayon, Magnetic anisotropy of transition metal and lanthanide ions in pentagonal bipyramidal geometry, Chem. Soc. Rev., 2022, 51, 3280–3313 RSC
.
- S. Gomez-Coca, E. Cremades, N. Aliaga-Alcalde and E. Ruiz, Mononuclear Single-Molecule Magnets: Tailoring the Magnetic Anisotropy of First-Row Transition-Metal Complexes, J. Am. Chem. Soc., 2013, 135, 7010–7018 CrossRef CAS PubMed
.
- H. L. C. Feltham and S. Brooker, Review of purely 4f and mixed-metal nd-4f single-molecule magnets containing only one lanthanide ion, Coord. Chem. Rev., 2014, 276, 1–33 CrossRef CAS
.
- L. Rosado Piquer and E. C. Sañudo, Heterometallic 3d–4f single-molecule magnets, Dalton Trans., 2015, 44, 8771–8780 RSC
.
- H.-S. Wang, K. Zhang, Y. Song and Z.-Q. Pan, Recent advances in 3d-4f magnetic complexes with several types of non-carboxylate organic ligands, Inorg. Chim. Acta, 2021, 521, 120318 CrossRef CAS
.
- A. Dey, J. Acharya and V. Chandrasekhar, Heterometallic 3d–4f Complexes as Single-Molecule Magnets, Chem. – Asian J., 2019, 14, 4433–4453 CrossRef CAS PubMed
.
- J. Dreiser, K. S. Pedersen, C. Piamonteze, S. Rusponi, Z. Salman, M. E. Ali, M. Schau-Magnussen, C. A. Thuesen, S. Piligkos, H. Weihe, H. Mutka, O. Waldmann, P. Oppeneer, J. Bendix, F. Nolting and H. Brune, Direct observation of a ferri-to-ferromagnetic transition in a fluoride-bridged 3d–4f molecular cluster, Chem. Sci., 2012, 3, 1024–1032 RSC
.
- P.-E. Car, A. Favre, A. Caneschi and R. Sessoli, Single molecule magnet behaviour in a rare trinuclear {CrIIIDyIII2} methoxo-bridged complex, Dalton Trans., 2015, 44, 15769–15773 RSC
.
- S. K. Langley, D. P. Wielechowski, N. F. Chilton, B. Moubaraki and K. S. Murray, A Family of {CrIII2LnIII2} Butterfly Complexes: Effect of the Lanthanide Ion on the Single-Molecule Magnet Properties, Inorg. Chem., 2015, 54, 10497–10503 CrossRef CAS PubMed
.
- S. K. Langley, D. P. Wielechowski, V. Vieru, N. F. Chilton, B. Moubaraki, B. F. Abrahams, L. F. Chibotaru and K. S. Murray, A {CrIII2DyIII2} Single-Molecule Magnet: Enhancing the Blocking Temperature through 3d Magnetic Exchange, Angew. Chem., Int. Ed., 2013, 52, 12014–12019 CrossRef CAS PubMed
.
- K. S. Pedersen, G. Lorusso, J. J. Morales, T. Weyhermüller, S. Piligkos, S. K. Singh, D. Larsen, M. Schau-Magnussen, G. Rajaraman, M. Evangelisti and J. Bendix, Fluoride-Bridged {GdIII3MIII2} (M=Cr, Fe, Ga) Molecular Magnetic Refrigerants, Angew. Chem., Int. Ed., 2014, 53, 2394–2397 CrossRef CAS PubMed
.
- X.-Q. Wang, Z.-Y. Li, Z.-X. Zhu, J. Zhu, S.-Q. Liu, J. Ni and J.-J. Zhang, Pentanuclear {Cr2Ln3} (Ln = Dy, Tb) Heterometallic Clusters Based on an Amino Acid Ligand: Slow Relaxation of Magnetization and Substitution Reactions, Eur. J. Inorg. Chem., 2013, 2013, 5153–5160 CrossRef CAS
.
- O. Blacque, A. Amjad, A. Caneschi, L. Sorace and P.-E. Car, Synthesis, structure, magnetic and magnetocaloric properties of a series of {CrIII4LnIII} complexes, New J. Chem., 2016, 40, 3571–3577 RSC
.
- H. Xiang, W.-G. Lu, L. Jiang, W.-X. Zhang and Y. Lan, A Family of Double Cubanes {CrIII2LnIII4O6} (Ln = Tb, Ho, Er, Yb, Y) Based on Sulfate: Single-Molecule Magnet Behavior in the Terbium and Erbium Analogues, Eur. J. Inorg. Chem., 2016, 2016, 907–912 CrossRef CAS
.
- H. Xiang, W.-G. Lu, W.-X. Zhang and L. Jiang, A {Cr2Dy4} compressed octahedron: the first sulfate-based single-molecule magnet, Dalton Trans., 2013, 42, 867–870 RSC
.
- J. H. Mecchia Ortiz, D. Cabrosi, C. Cruz, V. Paredes-García and P. Alborés, Synthesis, structural characterization, and magnetic property study of {Cr3Ln3}, Ln = Gd and Dy complexes, Dalton Trans., 2022, 51, 624–637 RSC
.
- J. Rinck, Y. Lan, C. E. Anson and A. K. Powell, Coordination Cluster Nuclearity Decreases with Decreasing Rare Earth Ionic Radius in 1
:
1 Cr/Ln N-Butyldiethanolamine Compounds: A Journey across the Lanthanide Series from Cr4IIILa4−Cr4IIITb4 via Cr3IIIDy3 and Cr3IIIHo3 to Cr2IIIEr2−Cr2IIILu2, Inorg. Chem., 2015, 54, 3107–3117 CrossRef CAS PubMed
.
- K. R. Vignesh, S. K. Langley, K. S. Murray and G. Rajaraman, Quenching the Quantum Tunneling of Magnetization in Heterometallic Octanuclear {TMIII4DyIII4} (TM=Co and Cr) Single-Molecule Magnets by Modification of the Bridging Ligands and Enhancing the Magnetic Exchange Coupling, Chem. – Eur. J., 2017, 23, 1654–1666 CrossRef CAS PubMed
.
- Y.-N. Gu, D. Zhao, H. Yu, R. Ge, Z. Li, C.-B. Tian, X.-X. Li, Y.-Q. Sun and S.-T. Zheng, Incorporating polyoxometalates and organic ligands to pursue 3d–4f heterometallic clusters: a series of {Cr4Ln4} clusters stabilized by phthalic acid and [SiW12O40]4−, RSC Adv., 2019, 9, 13543–13549 RSC
.
- J. Rinck, G. Novitchi, W. Van denHeuvel, L. Ungur, Y. Lan, W. Wernsdorfer, C. E. Anson, L. F. Chibotaru and A. K. Powell, An Octanuclear [CrIII4DyIII4] 3d–4f Single-Molecule Magnet, Angew. Chem., Int. Ed., 2010, 49, 7583–7587 CrossRef CAS PubMed
.
- S. Schmitz, J. van Leusen, N. V. Izarova, Y. Lan, W. Wernsdorfer, P. Kögerler and K. Y. Monakhov, Supramolecular 3d–4f single-molecule magnet architectures, Dalton Trans., 2016, 45, 16148–16152 RSC
.
- S. Chen, V. Mereacre, Z. Zhao, W. Zhang, M. Zhang and Z. He, Targeted replacement: systematic studies of dodecanuclear {MIII6LnIII6} coordination clusters (M = Cr, Co; Ln = Dy, Y), Dalton Trans., 2018, 47, 7456–7462 RSC
.
- L. Qin, J. Singleton, W.-P. Chen, H. Nojiri, L. Engelhardt, R. E. P. Winpenny and Y.-Z. Zheng, Quantum Monte Carlo Simulations and High-Field Magnetization Studies of Antiferromagnetic Interactions in a Giant Hetero-Spin Ring, Angew. Chem., Int. Ed., 2017, 56, 16571–16574 CrossRef CAS PubMed
.
- C. E. Jackson, I. P. Moseley, R. Martinez, S. Sung and J. M. Zadrozny, A reaction-coordinate perspective of magnetic relaxation, Chem. Soc. Rev., 2021, 50, 6684–6699 RSC
.
- A. Bencini, C. Benelli, A. Caneschi, R. L. Carlin, A. Dei and D. Gatteschi, Crystal and molecular structure of and magnetic coupling in two complexes containing gadolinium(III) and copper(II) ions, J. Am. Chem. Soc., 1985, 107, 8128–8136 CrossRef CAS
.
- Y. Peng and A. K. Powell, What do 3d-4f butterflies tell us?, Coord. Chem. Rev., 2021, 426, 213490 CrossRef CAS
.
- T. Gupta and G. Rajaraman, Modelling spin Hamiltonian parameters of molecular nanomagnets, Chem. Commun., 2016, 52, 8972–9008 RSC
.
- Y. Peng, M. K. Singh, V. Mereacre, C. E. Anson, G. Rajaraman and A. K. Powell, Mechanism of magnetisation relaxation in {MIII2DyIII2} (M = Cr, Mn, Fe, Al) “Butterfly” complexes: how important are the transition metal ions here?, Chem. Sci., 2019, 10, 5528–5538 RSC
.
- J.-P. Costes, C. Duhayon, S. Mallet-Ladeira, S. Shova and L. Vendier, Does the Sign of the Cu–Gd Magnetic Interaction Depend on the Number of Atoms in the Bridge?, Chem. – Eur. J., 2016, 22, 2171–2180 CrossRef CAS PubMed
.
- G. Rajaraman, F. Totti, A. Bencini, A. Caneschi, R. Sessoli and D. Gatteschi, Density functional studies on the exchange interaction of a dinuclear Gd(iii)–Cu(ii) complex: method assessment, magnetic coupling mechanism and magneto-structural correlations, Dalton Trans., 2009, 3153–3161, 10.1039/B817540C
.
- M. K. Singh, T. Rajeshkumar, R. Kumar, S. K. Singh and G. Rajaraman, Role of (1,3) {Cu-Cu} Interaction on the Magneto-Caloric Effect of Trinuclear {CuII-GdIII-CuII} Complexes: Combined DFT and Experimental Studies, Inorg. Chem., 2018, 57, 1846–1858 CrossRef CAS PubMed
.
- A. Upadhyay, K. R. Vignesh, C. Das, S. K. Singh, G. Rajaraman and M. Shanmugam, Influence of the Ligand Field on the Slow Relaxation of Magnetization of Unsymmetrical Monomeric Lanthanide Complexes: Synthesis and Theoretical Studies, Inorg. Chem., 2017, 56, 14260–14276 CrossRef CAS PubMed
.
- K. R. Vignesh, S. K. Langley, C. J. Gartshore, B. Moubaraki, K. S. Murray and G. Rajaraman, What Controls the Magnetic Exchange and Anisotropy in a Family of Tetranuclear {Mn2IIMn2III} Single-Molecule Magnets?, Inorg. Chem., 2017, 56, 1932–1949 CrossRef CAS PubMed
.
- T. Gupta, M. F. Beg and G. Rajaraman, Role of Single-Ion Anisotropy and Magnetic Exchange Interactions in Suppressing Zero-Field Tunnelling in {3d-4f} Single Molecule Magnets, Inorg. Chem., 2016, 55, 11201–11215 CrossRef CAS PubMed
.
- N. Ahmed, C. Das, S. Vaidya, S. K. Langley, K. S. Murray and M. Shanmugam, Nickel(II)–Lanthanide(III) Magnetic Exchange Coupling Influencing Single-Molecule Magnetic Features in {Ni2Ln2} Complexes, Chem. – Eur. J., 2014, 20, 14235–14239 CrossRef CAS PubMed
.
- O. Kahn, Dinuclear Complexes with Predictable Magnetic Properties, Angew. Chem., Int. Ed. Engl., 1985, 24, 834–850 CrossRef
.
- C. Benelli, A. Caneschi, D. Gatteschi, O. Guillou and L. Pardi, Synthesis, crystal structure, and magnetic properties of tetranuclear complexes containing exchange-coupled dilanthanide-dicopper(lanthanide = gadolinium, dysprosium) species, Inorg. Chem., 1990, 29, 1750–1755 CrossRef CAS
.
- E. Cremades, S. Gómez-Coca, D. Aravena, S. Alvarez and E. Ruiz, Theoretical Study of Exchange Coupling in 3d-Gd Complexes: Large Magnetocaloric Effect Systems, J. Am. Chem. Soc., 2012, 134, 10532–10542 CrossRef CAS PubMed
.
- S. K. Singh, N. K. Tibrewal and G. Rajaraman, Density functional studies on dinuclear {NiIIGdIII} and trinuclear {NiIIGdIIINiII} complexes: magnetic exchange and magneto-structural maps, Dalton Trans., 2011, 40, 10897–10906 RSC
.
- S. K. Singh, K. S. Pedersen, M. Sigrist, C. A. Thuesen, M. Schau-Magnussen, H. Mutka, S. Piligkos, H. Weihe, G. Rajaraman and J. Bendix, Angular dependence of the exchange interaction in fluoride-bridged GdIII–CrIII complexes, Chem. Commun., 2013, 49, 5583–5585 RSC
.
- K. S. Pedersen, M. A. Sørensen and J. Bendix, Fluoride-coordination chemistry in molecular and low-dimensional
magnetism, Coord. Chem. Rev., 2015, 299, 1–21 CrossRef CAS
.
- C. A. Thuesen, K. S. Pedersen, M. Schau-Magnussen, M. Evangelisti, J. Vibenholt, S. Piligkos, H. Weihe and J. Bendix, Fluoride-bridged {Ln2Cr2} polynuclear complexes from semi-labile mer-[CrF3(py)3] and [Ln(hfac)3(H2O)2], Dalton Trans., 2012, 41, 11284–11292 RSC
.
- T. Birk, K. S. Pedersen, C. A. Thuesen, T. Weyhermüller, M. Schau-Magnussen, S. Piligkos, H. Weihe, S. Mossin, M. Evangelisti and J. Bendix, Fluoride Bridges as Structure-Directing Motifs in 3d-4f Cluster Chemistry, Inorg. Chem., 2012, 51, 5435–5443 CrossRef CAS PubMed
.
- X.-Q. Zhao, J. Wang, F.-H. Zhang, M.-M. Sun, Y.-C. Li, M.-M. Wang and Y.-F. Tang, Significant magnetocaloric effect in a ferromagnetic {CrIII2GdIII3} cluster, Polyhedron, 2020, 179, 114385 CrossRef CAS
.
- A. Saha, M. Thompson, K. A. Abboud, W. Wernsdorfer and G. Christou, Family of Double-Cubane Mn4Ln2 (Ln = Gd, Tb, Dy, Ho) and Mn4Y2 Complexes: A New Mn4Tb2 Single-Molecule Magnet, Inorg. Chem., 2011, 50, 10476–10485 CrossRef CAS PubMed
.
- K. R. Vignesh, S. K. Langley, B. Moubaraki, K. S. Murray and G. Rajaraman, Understanding the Mechanism of Magnetic Relaxation in Pentanuclear {MnIVMnIII2LnIII2} Single-Molecule Magnets, Inorg. Chem., 2018, 57, 1158–1170 CrossRef CAS PubMed
.
- M. M. Hänninen, A. J. Mota, R. Sillanpää, S. Dey, G. Velmurugan, G. Rajaraman and E. Colacio, Magneto-Structural Properties and Theoretical Studies of a Family of Simple Heterodinuclear Phenoxide/Alkoxide Bridged MnIIILnIII Complexes: On the Nature of the Magnetic Exchange and Magnetic Anisotropy, Inorg. Chem., 2018, 57, 3683–3698 CrossRef PubMed
.
-
A. Dey, S. Tripathi, M. Shanmugam, R. S. Narayanan and V. Chandrasekhar, in Organometallic Magnets, ed. V. Chandrasekhar and F. Pointillart, Springer International Publishing, Cham, 2019, pp. 77–100, DOI:10.1007/3418_2018_9
.
- V. Chandrasekhar, B. M. Pandian, R. Azhakar, J. J. Vittal and R. Clérac, Linear Trinuclear Mixed-Metal CoII−GdIII−CoII Single-Molecule Magnet: [L2Co2Gd][NO3]·2CHCl3 (LH3 = (S)P[N(Me)NCH−C6H3–2-OH-3-OMe]3), Inorg. Chem., 2007, 46, 5140–5142 CrossRef CAS PubMed
.
- V. Chandrasekhar, B. M. Pandian, J. J. Vittal and R. Clérac, Synthesis, Structure, and Magnetism of Heterobimetallic Trinuclear Complexes {[L2Co2Ln][X]} [Ln = Eu, X = Cl; Ln = Tb, Dy, Ho, X = NO3; LH3 = (S)P[N(Me)N
CH−C6H3–2-OH-3-OMe]3]: A 3d−4f Family of Single-Molecule Magnets, Inorg. Chem., 2009, 48, 1148–1157 CrossRef CAS PubMed
.
- A. V. Funes and P. Alborés, Recent Developments in CoIII2LnIII2 Single-Molecule Magnets, Eur. J. Inorg. Chem., 2018, 2018, 2067–2089 CrossRef CAS
.
- J.-L. Liu, J.-Y. Wu, Y.-C. Chen, V. Mereacre, A. K. Powell, L. Ungur, L. F. Chibotaru, X.-M. Chen and M.-L. Tong, A Heterometallic FeII–DyIII Single-Molecule Magnet with a Record Anisotropy Barrier, Angew. Chem., Int. Ed., 2014, 53, 12966–12970 CrossRef CAS PubMed
.
- D. Prodius, V. Mereacre, P. Singh, Y. Lan, S. Mameri, D. D. Johnson, W. Wernsdorfer, C. E. Anson and A. K. Powell, Influence of lanthanides on spin-relaxation and spin-structure in a family of Fe7Ln4 single molecule magnets, J. Mater. Chem. C, 2018, 6, 2862–2872 RSC
.
- P. Bag, J. Goura, V. Mereacre, G. Novitchi, A. K. Powell and V. Chandrasekhar, Synthesis, magnetism and Mössbauer studies of tetranuclear heterometallic {FeIII2Ln2}(Ln = Gd, Dy, Tb) complexes: evidence of slow relaxation of magnetization in the terbium analogue, Dalton Trans., 2014, 43, 16366–16376 RSC
.
- Y. Peng, H. Kaemmerer and A. K. Powell, From the {FeIII2Ln2} Butterfly's Perspective: the Magnetic Benefits and Challenges of Cooperativity within 3d–4f Based Coordination Clusters, Chem. – Eur. J., 2021, 27, 15044–15066 CrossRef PubMed
.
- J. Wu, L. Zhao, L. Zhang, X.-L. Li, M. Guo, A. K. Powell and J. Tang, Macroscopic Hexagonal Tubes of 3d–4f Metallocycles, Angew. Chem., Int. Ed., 2016, 55, 15574–15578 CrossRef CAS PubMed
.
- Y.-F. Deng, T. Han, Z. Wang, Z. Ouyang, B. Yin, Z. Zheng, J. Krzystek and Y.-Z. Zheng, Uniaxial magnetic anisotropy of square-planar chromium(II) complexes revealed by magnetic and HF-EPR studies, Chem. Commun., 2015, 51, 17688–17691 RSC
.
- J. Flores Gonzalez, F. Pointillart and O. Cador, Hyperfine coupling and slow magnetic relaxation in isotopically enriched DyIII mononuclear single-molecule magnets, Inorg. Chem. Front., 2019, 6, 1081–1086 RSC
.
- J. Krzystek, A. Ozarowski and J. Telser, Multi-frequency, high-field EPR as a powerful tool to accurately determine zero-field splitting in high-spin transition metal coordination complexes, Coord. Chem. Rev., 2006, 250, 2308–2324 CrossRef CAS
.
- H. I. Karunadasa, K. D. Arquero, L. A. Berben and J. R. Long, Enhancing the Magnetic Anisotropy of Cyano-Ligated Chromium(II) and Chromium(III) Complexes via Heavy Halide Ligand Effects, Inorg. Chem., 2010, 49, 4738–4740 CrossRef CAS PubMed
.
- J. Su, L. Yin, Z. Ouyang, Z. Wang and W. Zheng, 1,2-Diaza-4-phospholide complexes of chromium(II): dipotassium organochromates behaving as single-molecule magnets, Dalton Trans., 2020, 49, 6945–6949 RSC
.
-
F. A. Cotton, G. Wilkinson, C. A. Murillo and M. Bochmann, Advanced Inorganic Chemistry, 6th edn, 1999 Search PubMed
.
- A. Sarkar, R. Jose, H. Ghosh and G. Rajaraman, Record High Magnetic Anisotropy in Three-Coordinate MnIII and CrII Complexes: A Theoretical Perspective, Inorg. Chem., 2021, 60, 9680–9687 CrossRef CAS PubMed
.
- Y.-Q. Zhai, N. Ge, Z.-H. Li, W.-P. Chen, T. Han, Z.-W. Ouyang, Z. Wang and Y.-Z. Zheng, Magnetic Anisotropy: Structural Correlation of a Series of Chromium(II)–Amidinate Complexes, Inorg. Chem., 2021, 60, 1344–1351 CrossRef CAS PubMed
.
- A. Cornia, L. Rigamonti, S. Boccedi, R. Clérac, M. Rouzières and L. Sorace, Magnetic blocking in extended metal atom chains: a pentachromium(II) complex behaving as a single-molecule magnet, Chem. Commun., 2014, 50, 15191–15194 RSC
.
- J. Wang, Z. Wang, R. J. Clark, A. Ozarowski, J. van Tol and N. S. Dalal, A high-frequency EPR characterization of the S = 2 linear tri-atomic chain in Cr3(dpa)4Cl2·CH2Cl2, Polyhedron, 2011, 30, 3058–3061 CrossRef CAS
.
- J. H. Christian, D. W. Brogden, J. K. Bindra, J. S. Kinyon, J. van Tol, J. Wang, J. F. Berry and N. S. Dalal, Enhancing the Magnetic Anisotropy of Linear Cr(II) Chain Compounds Using Heavy Metal Substitutions, Inorg. Chem., 2016, 55, 6376–6383 CrossRef CAS PubMed
.
- C. Dobe, C. Noble, G. Carver, P. L. W. Tregenna-Piggott, G. J. McIntyre, A.-L. Barra, A. Neels, S. Janssen and F. Juranyi, Electronic and Molecular Structure of High-Spin d4 Complexes: Experimental and Theoretical Study of the [Cr(D2O)6]2+ Cation in Tutton's Salts, J. Am. Chem. Soc., 2004, 126, 16639–16652 CrossRef CAS PubMed
.
- S.-J. Liu, S.-D. Han, J.-P. Zhao, J. Xu and X.-H. Bu, In situ synthesis of molecular magnetorefrigerant materials, Coord. Chem. Rev., 2019, 394, 39–52 CrossRef CAS
.
- X.-M. Luo, Y.-K. Li, X.-Y. Dong and S.-Q. Zang, Platonic and Archimedean solids in discrete metal-containing clusters, Chem. Soc. Rev., 2023, 52, 383–444 RSC
.
- Y.-Z. Zheng, G.-J. Zhou, Z. Zheng and R. E. P. Winpenny, Molecule-based magnetic coolers, Chem. Soc. Rev., 2014, 43, 1462–1475 RSC
.
- J.-L. Liu, Y.-C. Chen, F.-S. Guo and M.-L. Tong, Recent advances in the design of magnetic molecules for use as cryogenic magnetic coolants, Coord. Chem. Rev., 2014, 281, 26–49 CrossRef CAS
.
- M. Evangelisti and E. K. Brechin, Recipes for enhanced molecular cooling, Dalton Trans., 2010, 39, 4672–4676 RSC
.
- M. Evangelisti, F. Luis, L. J. de Jongh and M. Affronte, Magnetothermal properties of molecule-based materials, J. Mater. Chem., 2006, 16, 2534–2549 RSC
.
- S.-J. Liu, X.-R. Xie, T.-F. Zheng, J. Bao, J.-S. Liao, J.-L. Chen and H.-R. Wen, Three-dimensional two-fold interpenetrated CrIII–GdIII heterometallic framework as an attractive cryogenic magnetorefrigerant, CrystEngComm, 2015, 17, 7270–7275 RSC
.
- Z.-Y. Li, J.-J. Zhang, S.-Q. Liu, H. Zhang, Y.-J. Sun, X.-Y. Liu and B. Zhai, Heterometallic Hexanuclear [Ln4Cr2] Cluster-Based Three-Dimensional Sulfate Frameworks as a Magnetic Refrigerant and Single Molecular Magnet, Cryst. Growth Des., 2018, 18, 7335–7342 CrossRef CAS
.
- J.-J. Yin, T.-Q. Lu, C. Chen, G.-L. Zhuang, J. Zheng, X.-Y. Zheng and F. Shao, Magnetocaloric Effect and Slow Magnetic Relaxation on Two-Dimensional Layered 3d-4f Cluster-Based Metal–Organic Frameworks, Cryst. Growth Des., 2020, 20, 4005–4012 CrossRef CAS
.
- Y.-M. Han, N.-F. Li, Y.-Z. Yu, J.-P. Cao, M.-X. Yang, Y.-L. Hong, R.-K. Kang, P. Yuan and Y. Xu, Synthesis, crystal structures and magnetic properties of two heterometallic {Ln8Cr4} (Ln=Gd3+ and Tb3+) complexes with one-dimensional wave chain structure, RSC Adv., 2020, 10, 11365–11370 RSC
.
- A. McRobbie, A. R. Sarwar, S. Yeninas, H. Nowell, M. L. Baker, D. Allan, M. Luban, C. A. Muryn, R. G. Pritchard, R. Prozorov, G. A. Timco, F. Tuna, G. F. S. Whitehead and R. E. P. Winpenny, Chromium chains as polydentate fluoride ligands for lanthanides, Chem. Commun., 2011, 47, 6251–6253 RSC
.
- J.-J. Yin, C. Chen, G.-L. Zhuang, J. Zheng, X.-Y. Zheng and X.-J. Kong, Anion-Dependent Assembly of 3d–4f Heterometallic Clusters Ln5Cr2 and Ln8Cr4, Inorg. Chem., 2020, 59, 1959–1966 CrossRef CAS PubMed
.
- C. Cui, J.-P. Cao, X.-M. Luo, Q.-F. Lin and Y. Xu, Two Pairs of Chiral “Tower-Like” Ln4Cr4 (Ln=Gd, Dy) Clusters: Syntheses, Structure, and Magnetocaloric Effect, Chem. – Eur. J., 2018, 24, 15295–15302 CrossRef CAS PubMed
.
- J.-J. Yin, T.-Q. Lu, C. Chen, H.-Y. Shi, G.-L. Zhuang, J. Zheng, X. Fang and X.-Y. Zheng, A new family of decanuclear Ln7Cr3 clusters exhibiting a magnetocaloric effect, RSC Adv., 2021, 11, 17346–17351 RSC
.
- Z.-Y. Li, X.-Q. Wang, J.-J. Zhang, S.-Q. Liu, J. Ni and Y.-J. Sun, Synthesis, Structures, and Magnetic Properties of Binuclear [CrLn] (Ln=Gd or Dy) and Trinuclear [Cr2Ln] (Ln=Gd, Dy, or Tb) Heterometallic Clusters with 2,2′-Bipyridine as Ligand, Eur. J. Inorg. Chem., 2015, 2015, 5702–5707 CrossRef CAS
.
- K. E. Knope, H. Kimura, Y. Yasaka, M. Nakahara, M. B. Andrews and C. L. Cahill, Investigation of in Situ Oxalate Formation from 2,3-Pyrazinedicarboxylate under Hydrothermal Conditions Using Nuclear Magnetic Resonance Spectroscopy, Inorg. Chem., 2012, 51, 3883–3890 CrossRef CAS PubMed
.
- B. Zhai, L. Yi, H.-S. Wang, B. Zhao, P. Cheng, D.-Z. Liao and S.-P. Yan, First 3D 3d−4f Interpenetrating Structure: Synthesis, Reaction, and Characterization of {[LnCr(IDA)2(C2O4)]}n, Inorg. Chem., 2006, 45, 8471–8473 CrossRef CAS PubMed
.
- T. Funk, A. Deb, S. J. George, H. Wang and S. P. Cramer, X-ray magnetic circular dichroism—a high energy probe of magnetic properties, Coord. Chem. Rev., 2005, 249, 3–30 CrossRef CAS
.
- T. Birk, M. J. Magnussen, S. Piligkos, H. Weihe, A. Holten and J. Bendix, Alkali metal cation complexation and solvent interactions by robust chromium(III) fluoride complexes, J. Fluor. Chem., 2010, 131, 898–906 CrossRef CAS
.
- S. K. Langley, D. P. Wielechowski, B. Moubaraki and K. S. Murray, Enhancing the magnetic blocking temperature and magnetic coercivity of {CrIII2LnIII2} single-molecule magnets via bridging ligand modification, Chem. Commun., 2016, 52, 10976–10979 RSC
.
- S. K. Langley, C. Le, L. Ungur, B. Moubaraki, B. F. Abrahams, L. F. Chibotaru and K. S. Murray, Heterometallic 3d–4f Single-Molecule Magnets: Ligand and Metal Ion Influences on the Magnetic Relaxation, Inorg. Chem., 2015, 54, 3631–3642 CrossRef CAS PubMed
.
- S. K. Langley, D. P. Wielechowski, V. Vieru, N. F. Chilton, B. Moubaraki, L. F. Chibotaru and K. S. Murray, Modulation of slow magnetic relaxation by tuning magnetic exchange in {Cr2Dy2} single molecule magnets, Chem. Sci., 2014, 5, 3246–3256 RSC
.
- S. K. Langley, D. P. Wielechowski, B. Moubaraki, B. F. Abrahams and K. S. Murray, Magnetic Exchange Effects in {CrIII2DyIII2} Single Molecule Magnets Containing Alcoholamine Ligands, Aust. J. Chem., 2014, 67, 1581–1587 CrossRef CAS
.
- S. K. Langley, N. F. Chilton, B. Moubaraki and K. S. Murray, Anisotropy barrier enhancement via ligand substitution in tetranuclear {CoIII2LnIII2} single molecule magnets, Chem. Commun., 2013, 49, 6965–6967 RSC
.
- S. K. Langley, N. F. Chilton, B. Moubaraki and K. S. Murray, Single-Molecule Magnetism in Three Related {CoIII2DyIII2}-Acetylacetonate Complexes with Multiple Relaxation Mechanisms, Inorg. Chem., 2013, 52, 7183–7192 CrossRef CAS PubMed
.
- S. K. Langley, N. F. Chilton, L. Ungur, B. Moubaraki, L. F. Chibotaru and K. S. Murray, Heterometallic Tetranuclear [LnIII2CoIII2] Complexes Including Suppression of Quantum Tunneling of Magnetization in the [DyIII2CoIII2] Single Molecule Magnet, Inorg. Chem., 2012, 51, 11873–11881 CrossRef CAS PubMed
.
- S. K. Langley, L. Ungur, N. F. Chilton, B. Moubaraki, L. F. Chibotaru and K. S. Murray, Single-Molecule Magnetism in a Family of {CoIII2DyIII2} Butterfly Complexes: Effects of Ligand Replacement on the Dynamics of Magnetic Relaxation, Inorg. Chem., 2014, 53, 4303–4315 CrossRef CAS PubMed
.
- Y. Peng, V. Mereacre, C. E. Anson and A. K. Powell, Multiple superhyperfine fields in a {DyFe2Dy} coordination cluster revealed using bulk susceptibility and 57Fe Mössbauer studies, Phys. Chem. Chem. Phys., 2016, 18, 21469–21480 RSC
.
- J. H. Mecchia Ortiz, D. Cabrosi, L. M. Carrella, E. Rentschler and P. Alborés, SMM Behaviour of the Butterfly {CrIII2DyIII2} Pivalate Complex and Magneto-structurally Correlated Relaxation Thermal Barrier, Chem. – Eur. J., 2022, 28, e202201450 CrossRef CAS PubMed
.
- P. S. Koroteev, Z. V. Dobrokhotova, A. B. Ilyukhin, E. V. Belova, A. D. Yapryntsev, M. Rouzières, R. Clérac and N. N. Efimov, Tetranuclear Cr–Ln ferrocenecarboxylate complexes with a defect-dicubane structure: synthesis, magnetism, and thermolysis, Dalton Trans., 2021, 50, 16990–16999 RSC
.
- X.-Q. Zhao, S. Xiang, J. Wang, D.-X. Bao and Y.-C. Li, Magnetic Nature of the CrIII–LnIII Interactions in [CrIII2LnIII3] Clusters with Slow Magnetic Relaxation, ChemistryOpen, 2018, 7, 192–200 CrossRef CAS PubMed
.
- J. Bartolomé, G. Filoti, V. Kuncser, G. Schinteie, V. Mereacre, C. E. Anson, A. K. Powell, D. Prodius and C. Turta, Magnetostructural correlations in the tetranuclear series of {Fe3LnO2} butterfly core clusters: Magnetic and Mössbauer spectroscopic study, Phys. Rev. B: Condens. Matter Mater. Phys., 2009, 80, 014430 CrossRef
.
- D. Chauhan, K. R. Vignesh, A. Swain, S. K. Langley, K. S. Murray, M. Shanmugam and G. Rajaraman, Exploiting Strong {CrIII–DyIII} Ferromagnetic Exchange Coupling to Quench Quantum Tunneling of Magnetization in a Novel {CrIII2DyIII3} Single-Molecule Magnet, Cryst. Growth Des., 2023, 23, 197–206 CrossRef CAS
.
- S.-Q. Liu, C.-L. Dong, H. Zhao, H. Zhang, J. Ni, X.-Y. Liu and J.-J. Zhang, A One Dimensional 3d–4f Heterometallic Chain Based on Gd3 + Nodes and Tetranuclear {Cr4(hdpta)2} Complex Ligands: Synthesis, Structure and Magnetic Properties, J. Cluster Sci., 2016, 27, 883–894 CrossRef CAS
.
- C.-M. Liu, D.-Q. Zhang, X. Hao and D.-B. Zhu, Arraying Octahedral {Cr2Dy4} Units into 3D Single-Molecule-Magnet-Like Inorganic Compounds with Sulfate Bridges, Inorg. Chem., 2018, 57, 6803–6806 CrossRef CAS PubMed
.
- K. R. Vignesh, A. Soncini, S. K. Langley, W. Wernsdorfer, K. S. Murray and G. Rajaraman, Ferrotoroidic ground state in a heterometallic {CrIIIDyIII6} complex displaying slow magnetic relaxation, Nat. Commun., 2017, 8, 1023 CrossRef PubMed
.
- L. Ungur, S.-Y. Lin, J. Tang and L. F. Chibotaru, Single-molecule toroics in Ising-type lanthanide molecular clusters, Chem. Soc. Rev., 2014, 43, 6894–6905 RSC
.
- J. Tang, I. Hewitt, N. T. Madhu, G. Chastanet, W. Wernsdorfer, C. E. Anson, C. Benelli, R. Sessoli and A. K. Powell, Dysprosium Triangles Showing Single-Molecule Magnet Behavior of Thermally Excited Spin States, Angew. Chem., Int. Ed., 2006, 45, 1729–1733 CrossRef CAS PubMed
.
- K. R. Vignesh, S. K. Langley, A. Swain, B. Moubaraki, M. Damjanović, W. Wernsdorfer, G. Rajaraman and K. S. Murray, Slow Magnetic Relaxation and Single-Molecule Toroidal Behaviour in a Family of Heptanuclear {CrIIILnIII6} (Ln=Tb, Ho, Er) Complexes, Angew. Chem., Int. Ed., 2018, 57, 779–784 CrossRef CAS PubMed
.
- H. Han, X. Li, X. Zhu, G. Zhang, S. Wang, X. Hang, J. Tang and W. Liao, Single-Molecule-Magnet Behavior in a Calix[8]arene-Capped {Tb6IIICrIII} Cluster, Eur. J. Inorg. Chem., 2017, 2017, 2088–2093 CrossRef CAS
.
- F. Kobayashi, R. Ohtani, S. Kusumoto, L. F. Lindoy, S. Hayami and M. Nakamura, Wheel-type heterometallic ferromagnetic clusters: [Ni7−xMx(HL)6(μ3-OMe)4(μ3-OH)2]Cl2 (M=Zn, Co, Mn; x=1, 3), Dalton Trans., 2018, 47, 16422–16428 RSC
.
- A. B. Canaj, D. I. Tzimopoulos, D. A. Kalofolias, M. Siczek, T. Lis, M. Murrie and C. J. Milios, Heterometallic lanthanide-centred [NiII6LnIII] rings, Dalton Trans., 2018, 47, 12863–12867 RSC
.
- F. E. Kakaroni, A. Collet, E. Sakellari, D. I. Tzimopoulos, M. Siczek, T. Lis, M. Murrie and C. J. Milios, Constructing CrIII-centered heterometallic complexes: [NiII6CrIII] and [CoII6CrIII] wheels, Dalton Trans., 2018, 47, 58–61 RSC
.
- N. Dohmeier, A. Helmstedt, N. Müller, A. Gryzia, A. Brechling, U. Heinzmann, M. Heidemeier, E. Krickemeier, A. Stammler, H. Bögge, T. Glaser, L. Joly and K. Kuepper, Heptanuclear [FeIII6CrIII]3+ Complexes Experimentally Studied by Means of Magnetometry, X-ray Diffraction, XAS, XMCD and Spin-Polarized Electron Spectroscopy in Cross-Comparison with [MnIII6CrIII]3+ Single-Molecule Magnets, Magnetochemistry, 2016, 2, 5 CrossRef
.
- H. Zhang, Z.-Y. Li, J. Zhu, Y.-X. Yu, J. Ni, B.-J. Ding, D.-Z. Liu and J.-J. Zhang, A heptanuclear heterotrimetallic [Na2Cr2Tb3] cluster exhibiting single-molecule magnet behavior, Inorg. Chem. Commun., 2013, 37, 202–205 CrossRef CAS
.
- M. Perfetti, J. Rinck, G. Cucinotta, C. E. Anson, X. Gong, L. Ungur, L. Chibotaru, M.-E. Boulon, A. K. Powell and R. Sessoli, Single Crystal Investigations Unravel the Magnetic Anisotropy of the “Square-In Square” Cr4Dy4 SMM Coordination Cluster, Front. Chem., 2019, 7 DOI:10.3389/fchem.2019.00006
.
- S. K. Langley, C. M. Forsyth, B. Moubaraki and K. S. Murray, A fluoride bridged {CrIII4DyIII4} single molecule magnet, Dalton Trans., 2015, 44, 912–915 RSC
.
- B.-K. Ling, Y.-Q. Zhai, P.-B. Jin, H.-F. Ding, X.-F. Zhang, Y. Lv, Z. Fu, J. Deng, M. Schulze, W. Wernsdorfer and Y.-Z. Zheng, Suppression of zero-field quantum tunneling of magnetization by a fluorido bridge for a “very hard” 3d-4f single-molecule magnet, Matter, 2022, 5, 3485–3498 CrossRef CAS
.
- G. Brunet, F. Habib, I. Korobkov and M. Murugesu, Slow Magnetic Relaxation Observed in Dysprosium Compounds Containing Unsupported Near-Linear Hydroxo- and Fluoro-Bridges, Inorg. Chem., 2015, 54, 6195–6202 CrossRef CAS PubMed
.
- N. Jiang and H. S. La Pierre, Frustrated Magnetism in a 2-D Ytterbium Fluoride, Inorg. Chem., 2019, 58, 12152–12156 CrossRef CAS PubMed
.
- J. Corredoira-Vázquez, C. González-Barreira, M. Fondo, A. M. García-Deibe, J. Sanmartín-Matalobos, S. Gómez-Coca, E. Ruiz and E. Colacio, Dinuclear Fluoride Single-Bridged Lanthanoid Complexes as Molecule Magnets: Unprecedented Coupling Constant in a Fluoride-Bridged Gadolinium Compound, Inorg. Chem., 2022, 61, 9946–9959 CrossRef PubMed
.
- Q. Zhou, F. Yang, D. Liu, Y. Peng, G. Li, Z. Shi and S. Feng, Synthesis, Structures, and Magnetic Properties of Three Fluoride-Bridged Lanthanide Compounds: Effect of Bridging Fluoride Ions on Magnetic Behaviors, Inorg. Chem., 2012, 51, 7529–7536 CrossRef CAS PubMed
.
- V. Mereacre, M. N. Akhtar, Y. Lan, A. M. Ako, R. Clérac, C. E. Anson and A. K. Powell, Structures and magnetic properties of MnIII4LnIII4 aggregates with a “square-in-square” topology, Dalton Trans., 2010, 39, 4918–4927 RSC
.
- S. Chen, V. Mereacre, D. Prodius, G. E. Kostakis and A. K. Powell, Developing a “Highway Code” To Steer the Structural and Electronic Properties of FeIII/DyIII Coordination Clusters, Inorg. Chem., 2015, 54, 3218–3227 CrossRef CAS PubMed
.
- S. Biswas, J. Goura, S. Das, C. V. Topping, J. Brambleby, P. A. Goddard and V. Chandrasekhar, Octanuclear Heterobimetallic {Ni4Ln4} Assemblies Possessing Ln4 Square Grid [2 × 2] Motifs: Synthesis, Structure, and Magnetism, Inorg. Chem., 2016, 55, 8422–8436 CrossRef CAS PubMed
.
- G. Karotsis, S. Kennedy, S. J. Teat, C. M. Beavers, D. A. Fowler, J. J. Morales, M. Evangelisti, S. J. Dalgarno and E. K. Brechin, [MnIII4LnIII4] Calix[4]arene Clusters as Enhanced Magnetic Coolers and Molecular Magnets, J. Am. Chem. Soc., 2010, 132, 12983–12990 CrossRef CAS PubMed
.
- G. Karotsis, M. Evangelisti, S. J. Dalgarno and E. K. Brechin, A Calix[4]arene 3d/4f Magnetic Cooler, Angew. Chem., Int. Ed., 2009, 48, 9928–9931 CrossRef CAS PubMed
.
- Y. Yu, X. Pan, C. Cui, X. Luo, N. Li, H. Mei and Y. Xu, A Series Three-Dimensional Ln4Cr4 (Ln = Gd, Tb, Er) Heterometallic Cluster-Based Coordination Polymers Containing Interesting Nanotubes Exhibiting High Magnetic Entropy, Inorg. Chem., 2020, 59, 5593–5599 CrossRef CAS PubMed
.
- S. Das, K. S. Bejoymohandas, A. Dey, S. Biswas, M. L. P. Reddy, R. Morales, E. Ruiz, S. Titos-Padilla, E. Colacio and V. Chandrasekhar, Amending the Anisotropy Barrier and Luminescence Behavior of Heterometallic Trinuclear Linear [MII-LnIII-MII] (LnIII=Gd, Tb, Dy; MII=Mg/Zn) Complexes by Change from Divalent Paramagnetic to Diamagnetic Metal Ions, Chem. – Eur. J., 2015, 21, 6449–6464 CrossRef CAS PubMed
.
- A. Upadhyay, C. Das, S. Vaidya, S. K. Singh, T. Gupta, R. Mondol, S. K. Langley, K. S. Murray, G. Rajaraman and M. Shanmugam, Role of the Diamagnetic Zinc(II) Ion in Determining the Electronic Structure of Lanthanide Single-Ion Magnets, Chem. – Eur. J., 2017, 23, 4903–4916 CrossRef CAS PubMed
.
- A. Upadhyay, S. K. Singh, C. Das, R. Mondol, S. K. Langley, K. S. Murray, G. Rajaraman and M. Shanmugam, Enhancing the effective energy barrier of a Dy(iii) SMM using a bridged diamagnetic Zn(ii) ion, Chem. Commun., 2014, 50, 8838–8841 RSC
.
- X.-Q. Song, P.-P. Liu, Y.-A. Liu, J.-J. Zhou and X.-L. Wang, Two dodecanuclear heterometallic [Zn6Ln6] clusters constructed by a multidentate salicylamide salen-like ligand: synthesis, structure, luminescence and magnetic properties, Dalton Trans., 2016, 45, 8154–8163 RSC
.
- Y.-Z. Zheng, M. Evangelisti and R. E. P. Winpenny, Large Magnetocaloric Effect in a Wells–Dawson Type {Ni6Gd6P6} Cage, Angew. Chem., Int. Ed., 2011, 50, 3692–3695 CrossRef CAS PubMed
.
- O. Iasco, G. Novitchi, E. Jeanneau, W. Wernsdorfer and D. Luneau, Benzoxazole-Based Heterometallic Dodecanuclear Complex [DyIII4CuII8] with Single-Molecule-Magnet Behavior, Inorg. Chem., 2011, 50, 7373–7375 CrossRef CAS PubMed
.
- S. Yu, Q.-H. Zhang, Z. Chen, H.-H. Zou, H. Hu, D. Liu and F.-P. Liang, Structure, assembly mechanism and magnetic properties of heterometallic dodecanuclear nanoclusters DyIII4MII8 (M = Ni, Co), Inorg. Chem. Front., 2021, 8, 5214–5224 RSC
.
- Y.-Z. Zheng, M. Evangelisti and R. E. P. Winpenny, Co–Gd phosphonate complexes as magnetic refrigerants, Chem. Sci., 2011, 2, 99–102 RSC
.
- Y.-Z. Zheng, M. Evangelisti, F. Tuna and R. E. P. Winpenny, Co–Ln Mixed-Metal Phosphonate Grids and Cages as Molecular Magnetic Refrigerants, J. Am. Chem. Soc., 2012, 134, 1057–1065 CrossRef CAS PubMed
.
- M. Estrader, J. Ribas, V. Tangoulis, X. Solans, M. Font-Bardía, M. Maestro and C. Diaz, Synthesis, Crystal Structure, and Magnetic Studies of One-Dimensional Cyano-Bridged Ln3 + −Cr3 + Complexes with bpy as a Blocking Ligand, Inorg. Chem., 2006, 45, 8239–8250 CrossRef CAS PubMed
.
- T. Shiga, H. Ōkawa, S. Kitagawa and M. Ohba, Stepwise Synthesis and Magnetic Control of Trimetallic Magnets [Co2Ln(L)2(H2O)4][Cr(CN)6]·nH2O (Ln = La, Gd; H2L = 2,6-Di(acetoacetyl)pyridine) with 3-D Pillared-Layer Structure, J. Am. Chem. Soc., 2006, 128, 16426–16427 CrossRef CAS PubMed
.
- J.-L. Liu, J.-Y. Wu, G.-Z. Huang, Y.-C. Chen, J.-H. Jia, L. Ungur, L. F. Chibotaru, X.-M. Chen and M.-L. Tong, Desolvation-Driven 100-Fold Slow-down of Tunneling Relaxation Rate in Co(II)-Dy(III) Single-Molecule
Magnets through a Single-Crystal-to-Single-Crystal Process, Sci. Rep., 2015, 5, 16621 CrossRef CAS PubMed
.
- S. Demir, I.-R. Jeon, J. R. Long and T. D. Harris, Radical ligand-containing single-molecule magnets, Coord. Chem. Rev., 2015, 289–290, 149–176 CrossRef CAS
.
- T. Shimada, A. Okazawa, N. Kojima, S. Yoshii, H. Nojiri and T. Ishida, Ferromagnetic Exchange Couplings Showing a Chemical Trend in Cu–Ln–Cu Complexes (Ln=Gd, Tb, Dy, Ho, Er), Inorg. Chem., 2011, 50, 10555–10557 CrossRef CAS PubMed
.
- V. Chandrasekhar and B. Murugesapandian, Phosphorus-Supported Ligands for the Assembly of Multimetal Architectures, Acc. Chem. Res., 2009, 42, 1047–1062 CrossRef CAS PubMed
.
- V. Chandrasekhar, B. M. Pandian, R. Boomishankar, A. Steiner, J. J. Vittal, A. Houri and R. Clérac, Trinuclear Heterobimetallic Ni2Ln complexes [L2Ni2Ln][ClO4] (Ln=La, Ce, Pr, Nd, Sm, Eu, Gd, Tb, Dy, Ho, and Er; LH3=(S)P[N(Me)N
CH−C6H3–2-OH-3-OMe]3): From Simple Paramagnetic Complexes to Single-Molecule Magnet Behavior, Inorg. Chem., 2008, 47, 4918–4929 CrossRef CAS PubMed
.
- A. Chakraborty, P. Bag, E. Rivière, T. Mallah and V. Chandrasekhar, Assembly of heterobimetallic NiII–LnIII (LnIII=DyIII, TbIII, GdIII, HoIII, ErIII, YIII) complexes using a ferrocene ligand: slow relaxation of the magnetization in DyIII, TbIII and HoIII analogues, Dalton Trans., 2014, 43, 8921–8932 RSC
.
- J. Acharya, A. Swain, A. Chakraborty, V. Kumar, P. Kumar, J. F. Gonzalez, O. Cador, F. Pointillart, G. Rajaraman and V. Chandrasekhar, Slow Magnetic Relaxation in Dinuclear CoIIYIII Complexes, Inorg. Chem., 2019, 58, 10725–10735 CrossRef CAS PubMed
.
- J.-P. Costes, G. Novitchi, V. Vieru, L. F. Chibotaru, C. Duhayon, L. Vendier, J.-P. Majoral and W. Wernsdorfer, Effects of the Exchange Coupling on Dynamic Properties in a Series of CoGdCo Complexes, Inorg. Chem., 2019, 58, 756–768 CrossRef CAS PubMed
.
- J.-W. Yang, Y.-M. Tian, J. Tao, P. Chen, H.-F. Li, Y.-Q. Zhang, P.-F. Yan and W.-B. Sun, Modulation of the Coordination Environment around the Magnetic Easy Axis Leads to Significant Magnetic Relaxations in a Series of 3d-4f Schiff Complexes, Inorg. Chem., 2018, 57, 8065–8077 CrossRef CAS PubMed
.
- E. Kiefl, M. Mannini, K. Bernot, X. Yi, A. Amato, T. Leviant, A. Magnani, T. Prokscha, A. Suter, R. Sessoli and Z. Salman, Robust Magnetic Properties of a Sublimable Single-Molecule Magnet, ACS Nano, 2016, 10, 5663–5669 CrossRef CAS PubMed
.
- D. R. Talham and M. W. Meisel, Thin films of coordination polymer magnets, Chem. Soc. Rev., 2011, 40, 3356–3365 RSC
.
- M. Cavallini, J. Gomez-Segura, D. Ruiz-Molina, M. Massi, C. Albonetti, C. Rovira, J. Veciana and F. Biscarini, Magnetic Information Storage on Polymers by Using Patterned Single-Molecule Magnets, Angew. Chem., Int. Ed., 2005, 44, 888–892 CrossRef CAS PubMed
.
- M. Cavallini, M. Facchini, C. Albonetti and F. Biscarini, Single molecule magnets: from thin films to nano-patterns, Phys. Chem. Chem. Phys., 2008, 10, 784–793 RSC
.
- R. Westerström, A.-C. Uldry, R. Stania, J. Dreiser, C. Piamonteze, M. Muntwiler, F. Matsui, S. Rusponi, H. Brune, S. Yang, A. Popov, B. Büchner, B. Delley and T. Greber, Surface Aligned Magnetic Moments and Hysteresis of an Endohedral Single-Molecule Magnet on a Metal, Phys. Rev. Lett., 2015, 114, 087201 CrossRef PubMed
.
- X.-Y. Zheng, H. Zhang, Z. Wang, P. Liu, M.-H. Du, Y.-Z. Han, R.-J. Wei, Z.-W. Ouyang, X.-J. Kong, G.-L. Zhuang, L.-S. Long and L.-S. Zheng, Insights into Magnetic Interactions in a Monodisperse Gd12Fe14 Metal Cluster, Angew. Chem., Int. Ed., 2017, 56, 11475–11479 CrossRef CAS PubMed
.
- J. Gómez-Segura, J. Veciana and D. Ruiz-Molina, Advances on the nanostructuration of magnetic molecules on surfaces: the case of single-molecule magnets (SMM), Chem. Commun., 2007, 3699–3707, 10.1039/B616352A
.
- A. Cornia, M. Mannini, P. Sainctavit and R. Sessoli, Chemical strategies and characterization tools for the organization of single molecule magnets on surfaces, Chem. Soc. Rev., 2011, 40, 3076–3091 RSC
.
|
This journal is © the Partner Organisations 2023 |