DOI:
10.1039/D3NR02035E
(Paper)
Nanoscale, 2023,
15, 10634-10641
Experimental observation of the role of countercations in modulating the electrical conductance of Preyssler-type polyoxometalate nanodevices†
Received
2nd May 2023
, Accepted 21st May 2023
First published on 22nd May 2023
Abstract
Polyoxometalates are nanoscale molecular oxides with promising properties that are currently explored for molecule-based memory devices. In this work, we synthesize a series of Preyssler polyoxometalates (POMs), [Na⊂P5W30O110]14−, stabilized with four different counterions, H+, K+, NH4+, and tetrabutylammonium (TBA+). We study the electron transport properties at the nanoscale (conductive atomic force microscopy, C-AFM) of molecular junctions formed by self-assembled monolayers (SAMs) of POMs electrostatically deposited on the ultraflat gold surface prefunctionalized with a positively charged SAM of amine-terminated alkylthiol chains. We report that the electron transport properties of P5W30-based molecular junctions depend on the nature of the counterions; the low-bias current (in the voltage range [−0.6 V; 0.6 V]) gradually increases by a factor of ∼100 by changing the counterion in the order: K+, NH4+, H+ and TBA+. From a statistical study (hundreds of current–voltage traces) using a simple analytical model for charge transport in nanoscale devices, we show that the energy position of the lowest unoccupied molecular orbital (LUMO) of P5W30 with respect to the Fermi energy of the electrodes increases from ∼0.4 eV to ∼0.7 eV and that the electrode coupling energy also increases from ∼0.05 to 1 meV in the same order from K+, NH4+, H+ to TBA+. We discuss several hypotheses on the possible origin of these features, such as a counterion-dependent dipole at the POM/electrode interface and counterion-modulated molecule/electrode hybridization, with, in both cases, the largest effect in the case of TBA+ counterions.
Introduction
Ions inserted (inadvertently or in a controlled way) into solid-state molecular junctions (MJs) play an important role in modulating or controlling the electron transport properties. For example, ions can modulate the conductance of MJs as theoretically predicted,1,2 and as observed in several MJs, this conductance modulation depends on the chemical nature of the ions and the details of the molecule–ion conformation.3–7 Not only the conductance of the MJs, but also the shape of the current–voltage (I–V) behavior can be in situ switched between a linear (symmetric) and a rectifying (asymmetric) behavior by inserting/removing the ions or moving the precise position (electrical field driven) of the ions in the MJs,8–11 opening the way toward molecular-scale programmable functional devices and synaptic (neuro-inspired) behavior.12,13 Polyoxometalates (POMs) are candidates of choice owing to their remarkable reversible multi-redox states14,15 and photo-stimulable redox properties,16,17 and they have attracted growing interest with several recent demonstrations of proof-of-principles such as silicon-integrated POM-based memory devices18 and unconventional (in-memory and neuromorphic) computing devices.19–21
In solution, it is well known that the nature of counterions has a strong impact on the redox potentials for the POMs, and hence on the electron transfer rates.22 In the specific case of POM-based molecular junctions, the interactions with unavoidable counterions also control many properties of POM-based materials and devices.23,24 For example, for a single POM on a metallic surface, the electron cloud density around the POM, as observed with a scanning tunneling microscope (STM), depends on the counterions that modify the hybridization between the POM and the metal surface.25 A recent theoretical study shows that the presence of a counterion (whatever its nature, here tetramethylammonium (TMA) vs. Cs+) moves the LUMO (lowest unoccupied molecular orbital) of W18O54(SO3)2− based MJs closer to the Fermi energy of the electrodes (∼0.08 eV above the Fermi energy in both the cases).26 Despite this similar position of the LUMO, the computed current–voltage (I–V) curves showed slight differences in the shape (more asymmetric I–V with Cs+). The authors suggest that this feature might be due to the small differences in the amplitude of the calculated transmission coefficient of electrons in the MJ, clearly calling for more joint experimental and theoretical work. They also noted that the presence of the counterions does not create additional new conduction channels in the MJs, but they modify the potential landscape “viewed” by the POMs that transmit the electrons through the junction.
Here, we synthesized a series of Preyssler polyoxometalates (POMs), [Na⊂P5W30O110]14−,27 P5W30 for short, stabilized with four different counterions, H+, K+, NH4+ and tetrabutylammonium (N(C4H9)4+ or TBA+). We formed, by electrostatic deposition, monolayers of these POMs on an ultraflat Au surface prefunctionalized with a positively charged self-assembled monolayer (SAM) of amine-terminated alkylthiol chains following the protocol already developed and reported in our previous work for other POMs.17,28 The electron transport (ET) properties of these POMs were measured by conductive-AFM (atomic force microscopy) and the current vs. voltage curves of these MJs were statistically analyzed (hundreds of I–V traces) to determine the energy position of the molecular orbitals involved in the ET and the electronic coupling between the molecules and the electrodes. We demonstrate that the energy position of the LUMO of P5W30 with respect to the Fermi energy of the electrodes increases from ∼0.4 eV to ∼0.7 eV and that the electrode coupling energy evolves from ∼0.05 to 1 meV depending on the nature of the counterion in the order of K+, NH4+, H+ and TBA+.
Synthesis and structural characterization
The synthesis of the P5W30 POMs with various counterions is schematized in Fig. 1a. The potassium salt of [Na⊂P5W30O110]14− can be obtained under hydrothermal conditions.29 The sample we used has the molecular formula K13.3Na0.7[Na⊂P5W30O110]·27H2O. The ammonium salt (NH4)14[Na⊂P5W30O110]·25H2O has been prepared according to ref. 30. The acid salt H14[Na⊂P5W30O110]·44H2O was prepared by ion exchange of a solution of the potassium salt in a Dowex 50W-X8 proton exchange resin, following a published procedure.31 Acido-basic titration of heteropolyacids with cation-hydroxides is a common route to introduce other cations and it has also been applied to this Preyssler-type phosphotungstic acid.32 In our hands, the acido-basic titration of H14[Na⊂P5W30O110] with tetrabutylammonium hydroxide was not complete, despite careful colorimetric monitoring of the equivalence. We ended up with the mixed salt TBA10H4[Na⊂P5W30O110]·24H2O.
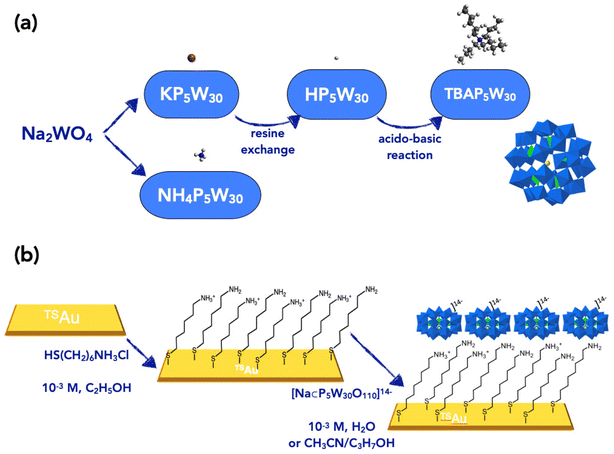 |
| Fig. 1 (a) Schematic of the synthesis routes (cations and POM not at the scale). (b) Schematic description of the two-step fabrication of the self-assembled monolayers. | |
We ended-up with 4 POMs: K13.3+Na0.7+[Na⊂P5W30O110]14− (KP5W30 for short); H14+[Na⊂P5W30O110]14− (HP5W30 for short); TBA10+H4+[Na⊂P5W30O110]14− (TBAP5W30 for short) and (NH4)14+[Na⊂P5W30O110]14− (NH4P5W30 for short). See more details, IR, 31P NMR characterization, TGA and elemental analysis, in the ESI (Section 1†).
These POMs (dissolved in H2O, or acetonitrile/isopropanol for HP5W30 and TBAP5W30) were electrostatically deposited on ultra-flat template-stripped TSAu substrates functionalized with a 6-aminohexane-1-thiol hydrochloride (HS–(CH2)6–NH3+/Cl−) self-assembled monolayer (SAM), or C6 SAM for short, following a protocol already developed and reported in our previous work for other POMs (Fig. 1b and also see Section 2 in the ESI†).17,28 The thicknesses (systematically measured by ellipsometry, see Section 3 in the ESI†) are summarized in Table 1.
Table 1 Thickness measured by ellipsometry at each step of the fabrication of the molecular junction
Thickness (Å) |
KP5W30 |
HP5W30 |
NH4P5W30 |
TBAP5W30 |
C6-SAM |
11 ± 2 |
POM layer |
9 ± 2 |
10 ± 2 |
9 ± 2 |
14 ± 2 |
We note that the P5W30 POM has an oval shape with a long axis of 1.8 nm and a short axis of 1.3 nm. Thus the 1.4 nm measured thickness of the TBAP5W30 sample indicates a denser monolayer with possibly a small percentage of the TBAP5W30 molecule standing upright on the surface (vertical POM orientation), while the thinner values for the 3 other samples can be ascribed to a less compact monolayer regardless of the POM orientation (disordered monolayers with various POM orientations). The thicker TBAP5W30 SAM may also be caused by the bulky TBA ions (diameter ≈ 0.5–1 nm depending on the conformation of the butyl chains) mainly intercalated between the POMs and the electrode, while small cations can be distributed around the POM.
Electron transport properties
Fig. 2 shows the 2D-histograms (or “heat map”) of the I–V curves (between 400 and 500) acquired by C-AFM at different locations on the POM monolayer for the four TSAu–C6/POM//Pt MJs (“–” denotes a chemical bond, “/” electrostatic contact and “//” mechanical contact). The main feature is that these I–V distributions have almost the same level of currents at large voltages, i.e. |V| > 1 V, while the currents in the voltage range [−0.6 V; 0.6 V] gradually increase with changing the counterion in the order from K+, NH4+, H+ to TBA+. This is clearly seen in Fig. 3a, showing the mean current–voltage (Ī–V) plots for the 4 samples and their counterion dependence at various voltages (Fig. 3b). The mean current Ī is around 10−8 A at ±1.5 V, while it varies over 2 decades at ±0.5 V. This observation is also supported by plotting and comparing the current histograms at given voltages (here at ±0.5 V and ±1 V), Fig. S9 and S10 in the ESI.†
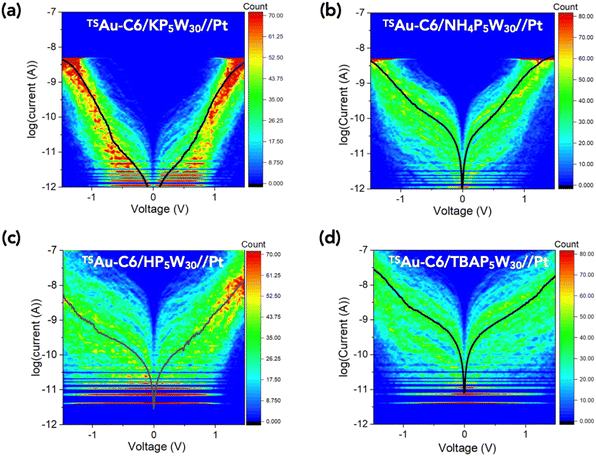 |
| Fig. 2 2D histograms (400–500 I–V traces) of the four samples in a semi-log|I|–V plot. The solid black lines are the calculated mean Ī–V curves. (a) KP5W30 MJs, (b) NH4P5W30 MJs, (c) HP5W30 MJs and (d) TBAP5W30 MJs. | |
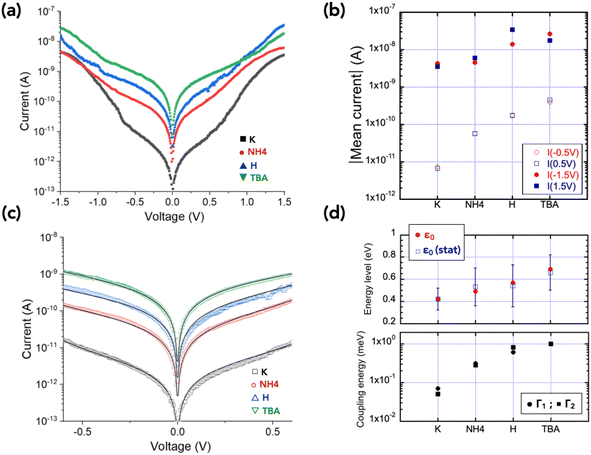 |
| Fig. 3 (a) Mean Ī–V curves for the four samples (from the datasets shown in Fig. 2), (b) counterion dependence of the mean current Ī at ±1.5 V and ±0.5 V, (c) fits (solid lines) of the SEL model in the voltage window [−0.6 V; 0.6 V] (fit R2 = 0.995 for K+, 0.998 for NH4+, 0.995 for H+, 0.999 for TBA+), (d) evolution of the SEL model parameters ε0, Γ1 and Γ2 with the counterions. | |
The Ī–V shapes for all samples show a bump at large voltages, i.e. |V| > 0.7–1 V, while the low-voltage Ī–V's are well fitted between −0.6 and 0.6 V with a single-energy level (SEL) model given by the following analytical expression:33,34
| 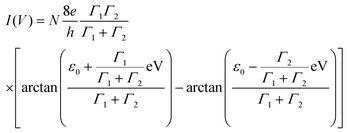 | (1) |
where
ε0 is the energy of the molecular orbital (MO), here the LUMO, involved in the transport (with respect to the Fermi energy of the electrodes),
Γ1 and
Γ2 are the electronic coupling energies between the MO and the electron clouds in the two electrodes,
e is the elementary electron charge,
h is the Planck constant and
N is the number of molecules contributing to the ET in the molecular junction (see details in Section 6 in the ESI
†). Among several limitations (Section 6 in the ESI
†), the SEL model is a low temperature approximation and the temperature broadening of the Fermi function in the electrodes is not taken into account. However, it was shown that it can be reasonably used at room temperature for voltages below the sharpened increase of the current (not observed here in the −0.6/0.6 V window) characterizing the transition between the off-resonance and resonant transport conditions at which the broadening of the Fermi function modifies the
I–
V shape.
35–37
Fig. 3c shows typical examples of the SEL fits on the mean Ī–V and Fig. 3d shows the evolution of the fitted parameters versus the nature of the counterions, clearly revealing an increase of the molecular energy level ε0 from 0.42 eV (for KP5W30) to 0.69 eV (for TBAP5W30). The electrode coupling energies, Γ1 and Γ2, follow the same trend, increasing from ∼0.08 to 1–2 meV in the same counterion order (Fig. 3d and Table 2). The same trend is confirmed from a statistical analysis by fitting the SEL model with all the individual I–V's of the datasets shown in Fig. 2. The statistical distributions of ε0, Γ1 and Γ2 for the 4 MJs are shown in Fig. S12 and S13 (ESI†) and the mean values of these distributions are summarized in Fig. 3d and Table 2.
Table 2 SEL parameters ε0, Γ1 and Γ2 fitted on the mean Ī–V (Fig. 3a) and mean values 〈ε0〉, 〈Γ1〉 and 〈Γ2〉 deduced from the statistical analysis (see text). For the energy level 〈ε0〉, the error is the standard deviation of the normal distribution (Fig. S12†). For the 〈Γ1〉 and 〈Γ2〉 parameters, the values in parentheses are the min and max values at the FWHM of the log–normal distributions (Fig. S13†)
|
ε
0 (eV) |
Γ
1 (meV) |
Γ
2 (meV) |
〈ε0〉 (eV) |
〈Γ1〉 (meV) |
〈Γ2〉 (meV) |
KP5W30 |
0.42 |
0.085 |
0.079 |
0.42 ± 0.10 |
0.07 (0.025/0.2) |
0.05 (0.02/0.13) |
NH4P5W30 |
0.49 |
0.33 |
0.39 |
0.53 ± 0.17 |
0.31 (0.1/1) |
0.28 (0.09/0.83) |
HP5W30 |
0.57 |
0.68 |
0.81 |
0.54 ± 0.19 |
0.61 (0.15/2.4) |
0.51 (0.14/1.86) |
TBAP5W30 |
0.69 |
2.0 |
1.0 |
0.66 ± 0.16 |
1 (0.24/4.3) |
0.99 (0.25/3.8) |
The same results are obtained by analyzing the I–V curves with the transition voltage spectroscopy (TVS) method.38–42 The energy level and the electrode coupling energy determined by the fit of the SEL model and by the TVS method are in very good agreement (Section 7, Fig. S14 and S15 in the ESI†).
Although the statistical analysis shows a large distribution of values (e.g. a factor ≈ 100 for the parameters Γ1 and Γ2 – Fig. S13 in the ESI,† and Table 2), the same trends and order of magnitude of these parameters are observed from the analysis of the mean Ī–V, the statistical analysis of the full dataset, and using both the SEL and TVS methods, making the experimental observations conclusive.
Discussion
The low-bias conductance of the P5W30-based MJs increases by a factor of ∼100 from the K+ to the TBA+ cations (Fig. 3b and c). However, the weak increase of the energy position of the LUMO (by a factor of ∼1.6, Fig. 3d and Table 2) should have induced a decrease of the current due to a higher electron energy barrier (ε0 − εF) at the molecule/electrode interface. A factor of ∼4.5 is estimated from eqn (1) assuming the same molecule/electrode coupling energy for simplicity (Γ1 = Γ2 = 0.1 meV) in the simulated I–V curves (Fig. S16 in the ESI†). The increase of the measured current is clearly related to the large increase (a factor of ∼20, Fig. 3d and Table 2) of the molecule/electrode coupling energy. We examine several hypotheses to explain these results. The trend observed in solution for the size-depending behavior of the alkali salts of POMs is barely transposable: indeed, a positive shift of the reduction potential (which would correspond here to a stabilization of the LUMO and a decrease of ε0) and a greater electron transfer rate to POMs associated with larger alkali metal cations were ascribed to an increase of the ion pairing energy related to a decrease of the cation solvation and the formation of more intimate cation/anion pairs.22 Although we cannot completely exclude the presence of some remaining solvent molecules in our MJs, solvation is not really relevant here. In a recent theoretical report, the efficiency of cations to compensate for the charging of a hexavanadate POM has been addressed by simultaneous addition of extra cations and electrons: in the gas phase, Li+ and H+ were found to be the most efficient, followed by NH4+ and K+.43 Although the origin of this effect was not further discussed, it could similarly impact the electron transport across the MJs. Last but not least, the tetrabutylammonium cation is very different from the other cations used in this study, and because of its volume and lower charge density, it is the softest cation of the series.
Among many factors, the energy level alignment in the MJs (the LUMO of the P5W30 POM with respect to the electrode Fermi energy) may depend on the interface dipole. A larger TBA counterion could probably induce a larger local dipole (larger distance between the positive and negative charges), and if we assume that the counterions are mostly inserted between the POM monolayer and the electrode (due to steric hindrance, vide supra the thickness measurements), then a global dipole could exist at the interface with an orientation leading to an upward larger LUMO offset from the Fermi level due to the dipole-induced vacuum level shift (Fig. S17 in the ESI†). In contrast, smaller cations create weaker dipoles randomly arranged around the POM,44 resulting in a weaker (or even negligible) average dipole at the interface, and thus a weaker LUMO shift. Note that we cannot exclude, at this stage without further theoretical explorations, that the atomistic details of the POM/electrode contact configuration also play a role (as also known in many other MJs). In the case of the W18O54(SO3)2− based MJs,26 a change by a factor of ca. 4 in the amplitude of the current and a variation of the LUMO between ca. 0.4 and 1.2 eV were theoretically predicted with respect to whether the POM was oriented with its long axis perpendicular or horizontal between the electrodes and how (atomic configuration) the POM was connected to the Au electrode. From the thickness measurements (vide supra), we also suggest possible different orientations of the POMs, and thus further detailed calculations (out of the scope of this work) are needed to reach a conclusion.
The evolution of the molecule/electrode coupling energy is most intriguing. Nevertheless, it has been recently demonstrated that the counterion can mediate the intermolecular electron transfer between two adjacent and nearby POMs,45 leading to a possible POM–cation–POM electron conduction channel. In the situation schematically depicted above for the case of the TBA+ counterion (Fig. S17 in the ESI†), we extrapolate that the same kind of mechanism could enhance the POM–cation–electrode electronic coupling, compared to a more random organization around the POMs for the other counterions (Fig. S17†). Moreover, the fact that the POM/electrode interaction also depends on the nature of the counterions, was observed from the STM images of a single POM deposited on a metal surface. In this experiment, the size of the electron cloud density measured by STM largely extends the geometric size of the POM due to the hybridization of the POM and the counterion with the metal surface, with a stronger hybridization with a TBA+ cation rather than the POM encapsulated in a cyclodextrin cage (cation free).25 This hybridization is also the key factor for fixing the molecule/electrode energy coupling in the SEL model (eqn (1)). Thus, we hypothesize that the TBA+ counterion could lead to a stronger POM/electrode hybridization than the other ones. Finally, we note that our energy values with the TBA+ counterion (ε0 = 0.66–0.69 eV, Γ1 and Γ2 ≈ 1–2 meV) are of the same order as the values determined by STM experiments on a single similar Preyssler POM ([DyP5W30O110]12−) in the case of a strong molecule/electrode coupling (established by moving the STM tip in close contact to the molecule in that case): ε0 ≈ 0.7 eV, Γ1 and Γ2 ≈ 1–10 meV.46
At higher voltages (|V| > 0.7 V), the bump of the current cannot be fitted with the SEL model. We hypothesize that this “additional” current can be due to a second level entering in the energy window at higher voltages (Fig. S11 in the ESI†). Such a behavior was theoretically simulated in the case of a two-channel electron transmission in the MJs.47 This second level is tentatively attributed to the LUMO+1 of the POMs. Our results suggest that the LUMO+1 is less sensitive to the counterions, since the currents at |V| > 0.7 V are less dependent on them (Fig. 3b and Fig. S10 in the ESI†). We also note that the POM samples with the NH4+ and H+ countercations display slightly higher currents at +1 V than those at −1 V (Fig. S10 and Table S1 in the ESI†), while no voltage dependence asymmetry is observed at lower voltages (Fig. S9†). The mean asymmetry ratio (also known as the rectification ratio) R = Ī(+1 V)/Ī(−1 V) is weak (≈1.7 and ≈3.2 for HP5W30 and NH4P5W30, respectively) with a difference in log-Ī (log-mean current) of 0.24 for HP5W30 and 0.5 for NH4P5W30, smaller than log-σ (log standard deviation, in the range of 0.84–1.1). It has been pointed out that weak R values must be considered with caution without a solid statistical analysis.48 A two-sample t-test on the current datasets at +1 and −1 V was used to assess if these datasets statistically and significantly differ from each other (see Section 9 in the ESI†). The t-test results show that the null hypothesis (the same mean value for the two datasets) can be rejected and this weak current asymmetry is statistically significant. The origin of this effect may be due to different contact geometries of the POM to the electrodes (at the atomic level) in the presence of the different counterions, with an asymmetric hybridization between the POM and the two electrodes for the LUMO+1 in the case of H+ and NH4+ counterions (no asymmetry is observed at lower bias, −0.6/0.6 V, involving only the LUMO in the electron transport mechanism (Fig. 3c and Fig. S9 in the ESI†)). A similar effect was theoretically predicted for the W18O54(SO3)2− POM with TMA (symmetric I–V) or Cs+ (asymmetric I–V) counterions.26 Further theoretical work (out of the scope of this work) would be necessary to clarify this point.
Conclusion
In conclusion, we have demonstrated that the electron transport properties, at the nanoscale, of P5W30-based molecular junctions depend on the nature of the counterions. From a statistical study (hundreds of I–V traces) using a simple analytical model derived from the Landauer–Imry–Büttiker formalism for charge transport in nanoscale devices, we have found that the energy position of the LUMO of P5W30 with respect to the Fermi energy of the electrodes increases from ∼0.4 eV to ∼0.7 eV and that the electrode coupling energy evolves from ∼0.05 to 1 meV depending on the nature of the counterion in the order from K+, NH4+, H+ to TBA+. We have suggested that these variations could be due to a counterion-dependent POM/electrode interface dipole and a molecule/electrode hybridization mediated by the counterions. These non-trivial POM–counterion–metal electrode interactions clearly require further theoretical studies to elucidate the influence of the counterions on the electron transport properties of POM-based molecular devices.
Methods
Sample fabrication
Bottom metal electrode fabrication.
Template-stripped gold (TSAu) substrates were prepared according to a method previously reported49–51 (Section 2 in the ESI†).
Self-assembled monolayers.
The SAMs on TSAu were fabricated following a protocol developed and optimized in our previous works for the electrostatic immobilization of POMs on amine-terminated SAMs17,28 (Section 2 in the ESI†).
Spectroscopic ellipsometry
The thickness of the SAMs was measured by spectroscopic ellipsometry (UVISEL ellipsometer (HORIBA), Section 3 in the ESI†).
CAFM under ambient conditions.
We measured the electron transport properties at the nanoscale by CAFM (ICON, Bruker) at room temperature using a tip probe in platinum/iridium (see Section 4 in the ESI†).
Author contributions
C. H., S. R., F. V. and A. P. synthesized and characterized the POMs and fabricated the SAMs. C. H. did all the C-AFM measurements, C. H. and D. V. analyzed the data. A. P. and D. V. conceived and supervised the project. The manuscript was written by D. V. with the contributions of all the authors. All authors have given approval to the final version of the manuscript.
Conflicts of interest
The authors declare no competing financial interest.
Acknowledgements
We acknowledge the support of the CNRS (France), project neuroPOM, under a grant of the 80PRIME program. We are thankful to Dr Masahiro Sadakane for sharing his knowledge about the preparation of the tetrabutylammonium salt of the Preyssler anion.
References
- G. Heimel, E. Zojer, L. Romaner, J. L. Brédas and F. Stellacci, Doping molecular wires, Nano Lett., 2009, 9(7), 2559–2564 CrossRef CAS PubMed.
- C. J. Chen, M. Smeu and M. A. Ratner, Modeling ion sensing in molecular electronics, J. Chem. Phys., 2014, 140(5), 054709 CrossRef PubMed.
- X. Xiao, B. Xu and N. Tao, Conductance titration of single peptide molecules, J. Am. Chem. Soc., 2004, 126, 5370–5371 CrossRef CAS PubMed.
- F. Chen, X. Li, J. Hihath, Z. Huang and N. J. Tao, Effect of anchoring groups on single-molecule conductance: comparative study of thiom-, amine-, and carboxylic-acid-terminated molecules, J. Am. Chem. Soc., 2006, 128(49), 15874–15881 CrossRef CAS PubMed.
- T. K. Tran, K. Smaali, M. Hardouin, Q. Bricaud, M. Ocafrain, P. Blanchard, S. Lenfant, S. Godey, J. Roncali and D. Vuillaume, A Crown-Ether Loop-Derivatized Oligothiophene Doubly Attached on Gold Surface as Cation-Binding Switchable Molecular Junction, Adv. Mater., 2013, 25(3), 427–431 CrossRef CAS PubMed.
- E. Mervinetsky, I. Alshanski, S. Lenfant, D. Guerin, L. Medrano Sandonas, A. Dianat, R. Gutierrez, G. Cuniberti, M. Hurevich and S. Yitzchaik,
et al., Electron Transport through Self-Assembled Monolayers of Tripeptides, J. Phys. Chem. C, 2019, 123(14), 9600–9608 CrossRef CAS.
- H. Audi, Y. Viero, N. Alwhaibi, Z. Chen, M. Iazykov, A. Heynderickx, F. Xiao, D. Guerin, C. Krzeminski and I. M. Grace,
et al., Electrical molecular switch addressed by chemical stimuli, Nanoscale, 2020, 12(18), 10127–10139 RSC.
- B. Capozzi, J. Xia, O. Adak, E. J. Dell, Z. F. Liu, J. C. Taylor, J. B. Neaton, L. M. Campos and L. Venkataraman, Single-molecule diodes with high rectification ratios through environmental control, Nat. Nanotechnol., 2015, 10(6), 522–527 CrossRef CAS PubMed.
- Y. Ai, A. Kovalchuk, X. Qiu, Y. Zhang, S. Kumar, X. Wang, M. Kuhnel, K. Norgaard and R. C. Chiechi, In-Place Modulation of Rectification in Tunneling Junctions Comprising Self-Assembled Monolayers, Nano Lett., 2018, 18(12), 7552–7559 CrossRef CAS PubMed.
- P. Chandra Mondal, U. M. Tefashe and R. L. McCreery, Internal Electric Field Modulation in Molecular Electronic Devices by Atmosphere and Mobile Ions, J. Am. Chem. Soc., 2018, 140(23), 7239–7247 CrossRef CAS PubMed.
- Y. Han, C. Nickle, Z. Zhang, H. Astier, T. J. Duffin, D. Qi, Z. Wang, E. Del Barco, D. Thompson and C. A. Nijhuis, Electric-field-driven dual-functional molecular switches in tunnel junctions, Nat. Mater., 2020, 19(8), 843–848 CrossRef CAS PubMed.
- Y. Wang, Q. Zhang, H. P. A. G. Astier, C. Nickle, S. Soni, F. A. Alami, A. Borrini, Z. Zhang, C. Honnigfort and B. Braunschweig,
et al., Dynamic molecular switches with hysteretic negative differential conductance emulating synaptic behaviour, Nat. Mater., 2022, 21, 1403–1411 CrossRef CAS PubMed.
- Y. Zhang, L. Liu, B. Tu, B. Cui, J. Guo, X. Zhao, J. Wang and Y. Yan, An artificial synapse based on molecular junctions, Nat. Commun., 2023, 14, 247 CrossRef CAS PubMed.
- D. L. Long, R. Tsunashima and L. Cronin, Polyoxometalates: building blocks for functional nanoscale systems, Angew. Chem., Int. Ed., 2010, 49(10), 1736–1758 CrossRef CAS PubMed.
- O. Linnenberg, M. Moors, A. Notario-Estevez, X. Lopez, C. de Graaf, S. Peter, C. Baeumer, R. Waser and K. Y. Monakhov, Addressing Multiple Resistive States of Polyoxovanadates: Conductivity as a Function of Individual Molecular Redox States, J. Am. Chem. Soc., 2018, 140(48), 16635–16640 CrossRef CAS PubMed.
- M. J. Turo, L. Chen, C. E. Moore and A. M. Schimpf, Co2+-Linked [NaP5W30O110]14−: A Redox-Active Metal Oxide Framework with High Electron Density, J. Am. Chem. Soc., 2019, 141(11), 4553–4557 CrossRef CAS PubMed.
- C. Huez, D. Guérin, S. Lenfant, F. Volatron, M. Calame, M. L. Perrin, A. Proust and D. Vuillaume, Redox-controlled conductance of polyoxometalate molecular junctions, Nanoscale, 2022, 14, 13790 RSC.
- C. Busche, L. Vila-Nadal, J. Yan, H. N. Miras, D. L. Long, V. P. Georgiev, A. Asenov, R. H. Pedersen, N. Gadegaard and M. M. Mirza,
et al., Design and fabrication of memory devices based on nanoscale polyoxometalate clusters, Nature, 2014, 515(7528), 545–549 CrossRef CAS PubMed.
- H. Tanaka, M. Akai-Kasaya, A. TermehYousefi, L. Hong, L. Fu, H. Tamukoh, D. Tanaka, T. Asai and T. Ogawa, A molecular neuromorphic network device consisting of single-walled carbon nanotubes complexed with polyoxometalate, Nat. Commun., 2018, 9(1), 2693 CrossRef PubMed.
- D. Banerjee, T. Kotooka, S. Azhari, Y. Usami, T. Ogawa, J. K. Gimzewski, H. Tamukoh and H. Tanaka, Emergence of In-Materio Intelligence from an Incidental Structure of a Single-Walled Carbon Nanotube–Porphyrin Polyoxometalate Random Network, Adv. Intell. Syst., 2022, 4(4), 2100145 CrossRef.
- G. Zhang, Z. Y. Xiong, Y. Gong, Z. Zhu, Z. Lv, Y. Wang, J. Q. Yang, X. Xing, Z. P. Wang and J. Qin,
et al., Polyoxometalate Accelerated Cationic Migration for Reservoir Computing, Adv. Funct. Mater., 2022, 32, 2204721 CrossRef CAS.
- V. A. Grigoriev, D. Cheng, C. L. Hill and I. A. Weinstock, Role of Alkali Metal Cation Size in the Energy and Rate of Electron Transfer to Solvent-Separated 1:1 [(M+)(Acceptor)] (M+ = Li+, Na+, K+) Ion Pairs, J. Am. Chem. Soc., 2001, 123(22), 5292–5307 CrossRef CAS PubMed.
- A. Misra, K. Kozma, C. Streb and M. Nyman, Beyond Charge Balance: Counter-Cations in Polyoxometalate Chemistry, Angew. Chem., Int. Ed., 2020, 59(2), 596–612 CrossRef CAS PubMed.
- K. Y. Monakhov, Implication of counter-cations for polyoxometalate-based nano-electronics, Comments Inorg. Chem., 2022, 1–10 CrossRef.
- F. Yang, M. Moors, D. A. Hoang, S. Schmitz, M. Rohdenburg, H. Knorke, A. Charvat, X.-B. Wang, K. Y. Monakhov and J. Warneke, On-Surface Single-Molecule Identification of Mass-Selected Cyclodextrin-Supported Polyoxovanadates for Multistate Resistive-Switching Memory Applications, ACS Appl. Nano Mater., 2022, 5(10), 14216–14220 CrossRef CAS.
- P. Lapham, L. Vila-Nadal, L. Cronin and V. P. Georgiev, Influence of the Contact Geometry and Counterions on the Current Flow and Charge Transfer in Polyoxometalate Molecular Junctions: A Density Functional Theory Study, J. Phys. Chem. C, 2021, 125(6), 3599–3610 CrossRef CAS PubMed.
- M. H. Alizadeh, S. P. Harmalker, Y. Jeannin, J. Martin-Frere and M. T. Pope, A heteropolyanion with fivefold molecular symmetry that contains a nonlabile encapsulated sodium ion. The structure and chemistry of [NaP5W30O110]14, J. Am. Chem. Soc., 1985, 107(9), 2662–2669 CrossRef CAS.
- K. Dalla Francesca, S. Lenfant, M. Laurans, F. Volatron, G. Izzet, V. Humblot, C. Methivier, D. Guerin, A. Proust and D. Vuillaume, Charge transport through redox active [H7P8W48O184]33− polyoxometalates self-assembled onto gold surfaces and gold nanodots, Nanoscale, 2019, 11(4), 1863–1878 RSC.
- I. Creaser, M. C. Heckel, R. J. Neitz and M. T. Pope, Rigid nonlabile polyoxometalate cryptates [ZP5W30O110](15−n)− that exhibit unprecedented selectivity for certain lanthanide and other multivalent cations, Inorg. Chem., 1993, 32(9), 1573–1578 CrossRef CAS.
-
Y. Jeannin, J. Martin-Frere, D. J. Choi and M. T. Pope, The Sodium Pentaphosphato(V)-Triacontatungstate Anion Isolated as the Ammonium Salt, in Inorganic Syntheses, ed. A. P. Ginsberg, Wiley & Son, 1990, vol. 27, pp. 115–118 Search PubMed.
- M. Sadakane, Y. Ichi, Y. Ide and T. Sano, Thermal Stability and Acidic Strength of Preyssler-Type Phosphotungstic Acid, H14[P5W30O110Na] and It's Catalytic Activity for Hydrolysis of Alkyl Acetates, Z. Anorg. Allg. Chem., 2011, 637(14–15), 2120–2124 CrossRef CAS.
- K. Sahiro, Y. Kawato, K. Koike, T. Sano, T. Nakai and M. Sadakane, Preyssler-type phosphotungstate is a new family of negative-staining reagents for the TEM observation of viruses, Sci. Rep., 2022, 12(1), 7554 CrossRef PubMed.
-
S. Datta, Electronic transport in mesoscopic systems, Cambridge University Press, 1995 Search PubMed.
-
J. C. Cuevas and E. Scheer, Molecular electronics: an introduction to theory and experiment, World Scientific, 2010 Search PubMed.
- L. Grüter, F. Cheng, T. T. Heikkilä, M. T. González, F. Diederich, C. Schönenberger and M. Calame, Resonant tunnelling through a C60 molecular junction in a liquid environment, Nanotechnology, 2005, 16(10), 2143–2148 CrossRef PubMed.
-
J. P. Bourgoin, D. Vuillaume, M. Goffman and A. Filoramo, Molecular Electronics, in Nanoscience, ed. C. Dupas, P. Houdy and M. Lahmani, Springer, 2007 Search PubMed.
-
J. Brunner, Gaining Microscopic Insight into Molecular Junctions by Transport Experiments, PhD thesis, Basel University, 2013 Search PubMed.
- J. M. Beebe, B. Kim, J. W. Gadzuk, C. Daniel Frisbie and J. G. Kushmerick, Transition from Direct Tunneling to Field Emission in Metal-Molecule-Metal Junctions, Phys. Rev. Lett., 2006, 97(2), 026801 CrossRef PubMed.
- J. M. Beebe, B. Kim, C. D. Frisbie and J. G. Kushmerick, Measuring Relative Barrier Heights in Molecular Electronic Junctions with Transition Voltage Spectroscopy, ACS Nano, 2008, 2(5), 827–832 CrossRef CAS PubMed.
- E. H. Huisman, C. M. Guédon, B. J. van Wees and S. J. van der Molen, Interpretation of Transition Voltage Spectroscopy, Nano Lett., 2009, 9(11), 3909–3913 CrossRef CAS PubMed.
- F. Mirjani, J. M. Thijssen and S. J. van der Molen, Advantages and limitations of transition voltage spectroscopy: A theoretical analysis, Phys. Rev. B: Condens. Matter Mater. Phys., 2011, 84(11), 115402 CrossRef.
- I. Bâldea, Ambipolar transition voltage spectroscopy: Analytical results and experimental agreement, Phys. Rev. B: Condens. Matter Mater. Phys., 2012, 85(3), 035442 CrossRef.
- A. S. Sorokina, D. A. Ryndyk, K. Y. Monakhov and T. Heine, What is the maximum charge uptake of Lindqvist-type polyoxovanadates in organic-inorganic heterostructures?, Phys. Chem. Chem. Phys., 2022, 24(43), 26848–26852 RSC.
- J. A. Fernández, X. López, C. Bo, C. de Graaf, E. J. Baerends and J. M. Poblet, Polyoxometalates with Internal Cavities: Redox Activity, Basicity, and Cation Encapsulation in [Xn+P5W30O110](15−n)− Preyssler Complexes, with X = Na+, Ca2+, Y3+, La3+, Ce3+, and Th4+, J. Am. Chem. Soc., 2007, 129(40), 12244–12253 CrossRef PubMed.
- I. Werner, J. Griebel, A. Masip-Sanchez, X. Lopez, K. Zaleski, P. Kozlowski, A. Kahnt, M. Boerner, Z. Warneke and J. Warneke,
et al., Hybrid Molecular Magnets with Lanthanide- and Countercation-Mediated Interfacial Electron Transfer between Phthalocyanine and Polyoxovanadate, Inorg. Chem., 2023, 62, 3761–3775 CrossRef CAS PubMed.
- S. Sherif, G. Rubio-Bollinger, E. Pinilla-Cienfuegos, E. Coronado, J. C. Cuevas and N. Agrait, Current rectification in a single molecule diode: the role of electrode coupling, Nanotechnology, 2015, 26(29), 291001 CrossRef PubMed.
- A. Migliore and A. Nitzan, Nonlinear Charge Transport in Redox Molecular Junctions: A Marcus Perspective, ACS Nano, 2011, 5(8), 6669–6685 CrossRef CAS PubMed.
- C. Nijhuis, W. Reus and G. Whitesides, Molecular Rectification in Metal–SAM–Metal Oxide–Metal Junctions, J. Am. Chem. Soc., 2009, 131(49), 17814–17827 CrossRef CAS PubMed.
- M. Hegner, P. Wagner and G. Semenza, Ultralarge atomically flat template-stripped Au surfaces for scanning probe microscopy, Surf. Sci., 1993, 291, 39–46 CrossRef CAS.
- E. Weiss, R. Chiechi, G. Kaufman, J. Kriebel, Z. Li, M. Duati, M. Rampi and G. Whitesides, Influence of defects on the electrical characteristics of mercury-drop junctions: self-assembled monolayers of n-alkanethiolates on rough and smooth silver, J. Am. Chem. Soc., 2007, 129(14), 4336–4349 CrossRef CAS PubMed.
- E. A. Weiss, G. K. Kaufman, J. K. Kriebel, Z. Li, R. Schalek and G. M. Whitesides, Si/SiO2-Templated Formation of Ultraflat Metal Surfaces on Glass, Polymer, and Solder Supports: Their Use as Substrates for Self-Assembled Monolayers, Langmuir, 2007, 23(19), 9686–9694 CrossRef CAS PubMed.
Footnotes |
† Electronic supplementary information (ESI) available: Synthesis details; IR and NMR characterization; fabrication of self-assembled monolayers; ellipsometry measurements; the protocol of C-AFM measurements; supplemental details on the I–V measurements (statistical data); details on the single energy level (SEL) model and on the statistical distribution of the model parameters; transition voltage spectroscopy (details on the technique and results); supplementary figures for the discussion. See DOI: https://doi.org/10.1039/d3nr02035e |
‡ Now at: Instituto de Ciencia Molecular (ICMoL), University of Valencia, Spain. |
|
This journal is © The Royal Society of Chemistry 2023 |