DOI:
10.1039/D2MA01075E
(Review Article)
Mater. Adv., 2023,
4, 2042-2061
Recent advancement in nanomaterial-encapsulated drug delivery vehicles for combating cancer, COVID-19, and HIV-like chronic diseases
Received
10th December 2022
, Accepted 22nd March 2023
First published on 30th March 2023
Abstract
Nanotechnology has gained immense attention owing to its multidimensional advantages in the scientific world. Recent progress has confirmed that nanostructured architectures possess promising medicinal applications and have triggered investigation as nanodrug delivery vehicles. These engineered vehicles offer an unprecedented platform for the controlled release of encapsulated drug to targeted site with higher effectuality and reduced toxicity by overcoming the loopholes allied with conventional drug delivery systems. This review provides a systematic overview of specific properties of nanostructured materials, viz., inorganic nanoparticles, polymeric micelles, chitosan, liposomes, dendrimers, carbon nanotubes, quantum dots, and niosomes, and consolidates their therapeutic approaches in the diagnosis and treatment of chronic diseases such as cancer, COVID-19, and HIV/AIDS. Factors including interactions of nanomaterials with physiological environment, mode of drug administration, stability of therapeutic agents, and mechanism of action have been summarized for attaining efficacious drug delivery. In addition to opportunities, the challenges of nanomedicines in drug delivery have also been discussed. In the futuristic perspective, further advancement is necessitated in the domain of nanotechnology mediated advanced drug delivery system by combining newer treatment approaches such as gene therapy and immunotherapy with the existing nanotechnology to improve the performance of drugs and maximize the efficiency of targeted drug distribution.
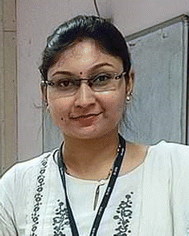
Suparna Paul
| Dr Suparna Paul has received MSc Chemistry degree with an award of Gold Medal in 2015 by National Institute of Technology, Durgapur, West Bengal, India. Thereafter, she successfully completed her PhD (Chemical Sciences) in 2022 with DST-INSPIRE Fellowship from Academy of Scientific and Innovative Research (AcSIR) under the joint supervision of Dr Priyabrata Banerjee, Pr. Scientist, CSIR-CMERI and Dr Naresh Chandra Murmu, Director, CSIR-CMERI, Durgapur. Her research has primarily been focused on the development, characterization, and application of sensory probes for the expeditious chromo-fluorogenic detection of heavy, toxic cationic contaminants, and lethal anions. As a research outcome, Dr Paul has published over 15 research papers in international SCI journals, 3 book chapters, and also has 2 patents and 2 copyrights. Currently, she is working as Assistant Professor in the Department of Chemistry, Seacom Skills University, Birbhum, West Bengal, India. |
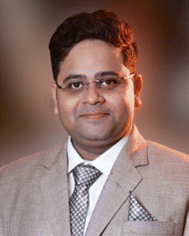
Subhajit Mukherjee
| Dr Subhajit Mukherjee is presently working as Assistant Professor in the Department of Chemistry, Seacom Skills University, Birbhum, West Bengal, India. Dr Mukherjee was awarded the Gold Medal in MSc Chemistry by the National Institute of Technology, Durgapur, West Bengal, India in 2013. He then acquired PhD in Chemistry from Department of Chemistry, NIT Durgapur in 2018 on the design and synthesis of metal-based anticancer drugs. Post PhD, he completed post-doctoral research at Shanghai Jiao Tong University School of Medicine, Shanghai, China with Prof. Jinyao Liu Dr Mukherjee also actively worked as Indo-Austria Cooperation Research Fellow at the Department of Chemistry Vienna University of Technology,” Vienna, Austria, Europe. Dr Mukherjee has published over 35 research papers in different SCI journals and has also received many prestigious awards. |
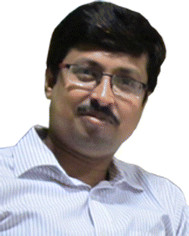
Priyabrata Banerjee
| Dr Priyabrata Banerjee is Principal Scientist and Associate Professor (AcSIR), Electric Mobility And Tribology Research Group, CSIR-CMERI, Durgapur, West Bengal, India. He received his PhD degree from IACS, Jadavpur, Kolkata, India in 2007. He did his post-doctoral research at Max Planck Institute for Bio-Inorganic Chemistry, Müelheim, Germany. He was a visiting fellow at HTW Dresden, Germany, and he has recently been selected as CSIR-Raman Research Fellow at Ghent University, Belgium. He has published 162 research papers (7244 citations, h-index: 46, i-index: 137) in several international SCI journals, 19 book chapters, and 15 magazines. He is the Editor of Advances in Materials and Processing Technologies, Taylor & Francis online and Guest Editor of Frontiers in Chemistry and Molecules [MDPI]. His current research interest broadly covers selective bio-relevant cation-anion detection, corrosion science, solid waste management, wastewater treatment, metal-mediated C-heteroatom bond fusion, renewable energy, metal complexes and Porous Frameworks (MOFs and COFs) and exploration of their functional chemistry. |
1. Introduction
In recent days, nanotechnology has played a tremendous role in the successful design and delivery of a drug to its target and has unveiled a new dimension in the field of medicine. Majority of drugs utilized for therapeutic applications including cancer, diabetes, cardiovascular disease treatment, and antiviral medication to combat HIV and COVID-19 lose their efficacy owing to lack of specificity. On the other hand, nanoparticle-encapsulated drugs lead to better target specificity and drug efficacy.1–3 Most of these drugs no longer remain active at their target sites due to various factors including lack of target specificity, aiming at other biological sites concomitantly with the actual site of their action, instability of the drug molecules inside the target cells or drug degradation before reaching the target, modification in the genetic environment of cell-surface receptors, and alteration in signalling allied with the evolution of diseases.4–6 For instance, many anticancer drugs such as cisplatin, carboplatin, oxaliplatin, or doxorubicin demonstrate reduced efficacy owing to these shortcomings.7 Nanoencapsulation provides protection to these drugs and makes them more specific toward their targeted site of application.
Nanoparticles with <100 nm diameter in at least one dimension containing metals, various biomaterials such as protein, lipids, or different biodegradable polymers can be dynamically utilized as a drug delivery vehicle. Cells tend to accept nanomaterials more efficiently than a macromolecule, and this is the primary reason underlying the medicinal activity of nanoparticles as drug carriers. An effective way to develop a nanovehicle for a particular drug is reliant on the nature of its interaction with the target, cell receptors present in the target cell surface, target cell population, drug action mechanism, and the pathology of the disease.8 In addition, an understanding of the mechanistic pathway of the cellular uptake of drug and the nanoencapsulated form, intracellular trafficking, drug retention, and the technique of protection against drug degradation is significant for enhancing drug activity post nanoparticle encapsulation. For adjuvating the drug with a nanoparticle, either the drug molecule is to be completely encapsulated within the inner matrix of the particle or the nanoparticle must adhere to the drug on its surface.
In previous literature, there are plentiful reports on nanoparticle-based materials as effective drug delivery vehicles for targeted therapeutic applications (chronic diseases).9,10 Ding et al. have unveiled self-adaptive nanomaterials toward rational drug delivery in cancer treatment. In comparison to trivial ones, self-adaptive nanomaterials exhibit promising reduction in drug release doses within the normal tissues and thereby maintain the concentration of the drug within the infected (tumor) cells for a prolonged time.11 In another work Sayyad et al., demonstrated and provided a deep insight regarding the application of nanomaterial-functionalized drug delivery systems for increasing the bioavailability of recently used drugs with reduced toxicity and increasing efficacy for COVID-19 treatment.12 Likewise, Kang et al., have reported the recent nanotechnological advancements including the stabilization of surface and stimuli-responsive functionalization, which appreciably improved the capacity of targeting along with therapeutic efficiency of the nanocarrier-modified drug delivery system.13 Das et al., reported an unprecedented and promising new class of G-quadruplex materials derived from imino-boronate, boronate esters, peptides, etc. These self-assembled nanostructured architectures display manifold bio-applications including drug delivery (doxorubicin), sustained release of vitamins, antimicrobial, antiinflammatory, wound healing, anticancer, bioimaging, and 3D bioprinting applications.14–16
In this consequence, this review systematically focuses on the progress of nanotechnology-based drug delivery systems for therapeutic applications along with their bottlenecks and the rationale for the codelivery of drugs to overcome these confinements. As a step ahead, the role of multifunctional nanocarriers (MNCs) for real-time combination therapy and targeting has been considered. The current findings of nanocarriers-assisted drug distribution in clinical trials and commercialized nanoformulations is highlighted herein. In particular, the authors have focused on highlighting the recent advancement in nanomedicine primarily for the treatment of COVID-19, cancer, and HIV-like life-threatening ailments, which are amongst the most important threats to mankind in the present era.
2. Advantages of nanoparticles-encapsulated drug delivery system
The conventional systems of drug delivery that includes tablets, capsules, syrups, and ointments often suffer from reduced biological availability along with undulations in the plasma drug level and are incompetent of achieving sustained drug release. In addition, the drug is required to be delivered at a definite controlled rate at the target site with acute precision to attain maximum efficiency and protection. With this consideration, there arises an exigency for developing controlled drug delivery systems for combating the difficulties related to conventional drug delivery systems.17 The controlled release of drug is required to maintain the plasma drug levels at a persistent rate within the therapeutic window and offer the anticipated therapeutic effect for a prolonged period. Without an efficient delivery mechanism, the whole therapeutic process can be rendered useless. The advantage of using a nanovehicle for an active drug delivery mostly arises from their controlled drug releasing activity in its specific delivery site, causing reduced drug toxicity, increased solubility of a poorly soluble drug, and improved bioavailability and pharmacokinetics of a medicine.18,19 Sometimes, nanoparticle encapsulation causes release of a drug in a controlled way and lowers the toxicity. A comparative investigation between a targeted and untargeted drug delivery system is schematically represented in Scheme 1. Considering the targeted drug delivery, the targeting strategies of the nanodrug plays a pivotal role in overcoming the commonly encountered challenges, drug instability, low absorption and specificity, reduced half-life, large volume of distribution along with low therapeutic index. Some frequently adopted approaches for attaining targeted drug delivery include controlling the distribution of drug by incorporating it within a carrier system. At times molecular level alteration in the drug structure is performed along with control of the drug input into the bioenvironment for ensuring a desirable drug distribution at the targeted site.
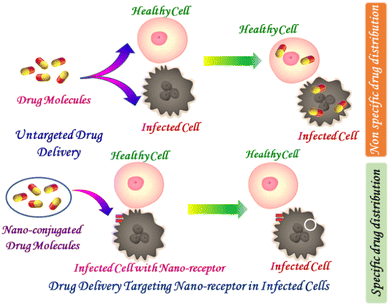 |
| Scheme 1 Schematic representation of untargeted and targeted drug delivery systems. | |
Panyam et al., unveiled the efficiency of biodegradable NPs for sustained intracellular (cytoplasmic) delivery of dexamethasone, whose site of action is intracellular. The chemotherapeutic agent dexamethasone is found to have some antiproliferative and antiinflammatory action. Dexamethasone interacts with cytoplasmic receptors to form a complex, and then this whole complex is transported to the inner core of the nucleus of the cell. This triggers the activation of some specific genes, which is behind cell proliferation. When it is loaded into polymeric NPs, polylactic/glycolic acid (PLGA), and polylactic acid (PLA), its antiproliferative activity for vascular smooth muscle cells is improved due to the controlled release of the drug with extended time period in a higher dose.20 The delivery vehicle for other anticancer drugs such as paclitaxel, which has been intended to be intravenously administered, doxorubicin, and 5-fluorouracil has also been formulated with this nanoparticle to achieve better efficacy of these drugs.21–24
In some cases of cancer, chemotherapeutics lose their activity due the development of resistance against multiple anticancer drugs. These drug resistances are mainly caused owing to the activation of a protein called p-glycoprotein. When an anticancer drug enters the cell after penetrating the cell membrane, the protein becomes activated and pumps out the drug from the cell as quickly it enters.22 To overcome this problem, nanotechnology has been used where no triggering of such protein such as p-glycoprotein has still been encountered.25 Paclitaxel has resistance over human colorectal tumors. However, the delivery of paclitaxel encapsulated in emulsifying wax nanoparticles is found to be efficient on human colon adenocarcinoma cell line (HCT-15). Again, the poor solubility of some drugs in biological fluids sometimes creates hindrance in their use in specific medications. NP-functionalized drug delivery vehicles takes advantage of enhanced permeability and retention (EPR) effect toward passive tumor targeting and are promising contenders for improving the therapeutic index and thereby reduce the side effects of drugs. As an example, the activity of paclitaxel (natural source of cancer drug) was increased after increasing its solubility via albumin conjugation. This albumin-paclitaxel composite in the injectable nanosuspension form was approved for breast cancer treatment. Interestingly, the above formulation had showcased lesser lethality in comparison to the chemotherapy drug Taxol.26
Drug intake, its circulations, metabolism, and removal from the body of an antiHIV or antiCOVID-19 drug can be enhanced by nanoparticle encapsulation or conjugation.27,28 The nanocomposite modifies the physical and chemical properties, which modifies the tissue distribution of the HIV affected organs in the human body. Targeted tissues achieve the therapeutic level of the dose by repetitive drug administration. However, this repetitive drug administration leads to serious toxicity in the patient's body. Nanotechnology-based therapy against HIV-AIDS opens a new way of medication to eliminate HIV, mostly by the enhancement of drug permeability through biological barriers such as blood brain barrier, reducing dose amount, and frequency; thus, the side effect of the HIV drugs is reduced. Deutsc et al., (1993) studied the effect of an antiretroviral medicine azidothymidine (AZT) over seven male HIV patient of the same age group. The study revealed that the liposomal-AZT showed better efficacy with almost zero side effect in comparison with AZT alone at the same level of dosage.29
In case of COVID-19 prevention, nanotechnology plays an important role and is an important topic of research nowadays. It can be used to build personal protective equipment or disinfectants30 or in designing nanomaterial-based vaccines to prevent the attack of SARS-CoV-2.31 In case of detection of the virus, the nanomaterial can also be used to develop simple, rapid, and cost-effective equipment or kit.
3. Classification of nanomaterials-based drug delivery systems
Depending upon the composition of smart nanostructured materials, these are broadly classified into two categories—organic (& polymer) and inorganic (& metallic) nanocarriers. The primary organic nanocarriers includes polymer-functionalized micelles, liposomes, vesicles, and dendrimers whilst the inorganic nanocarriers comprises of carbon nanotubes (CNYs), gold nanoparticles (AuNPs), silver nanoparticles (AgNPs), and quantum dots (Fig. 1).32 These nanocarriers have been clinically accepted for the treatment of various life-threatening ailments in association with varying stages of medical investigations.33,34 The main properties of a few commonly explored nanomaterials have been reviewed herein.
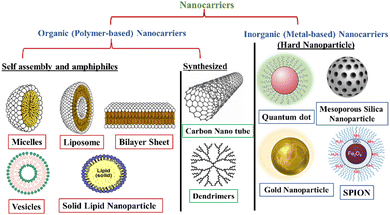 |
| Fig. 1 The most employed organic and inorganic nanocarriers for targeted drug delivery application. | |
3.1. Organic and polymer-based nanocarriers
Carbon-functionalized nanomaterials form the basis of organic nanocarriers, which are primarily characterized by extensive biocompatibility and enhanced drug loading ability. These permit a versatile regulation of surface morphology as well as chemical constituency. Most significantly, the colloidal stability and fairly large size facilitates the loading and delivery of a broad range of hydrophilic/hydrophobic drugs.35,36
Polymer-based nanocarriers represent another class of therapeutic nanocarriers that can be further classified into biodegradable and nonbiodegradable materials.37,38 A majority of biodegradable polymeric nanocarriers demonstrate excellent drug delivery applications since these provide extensive control in comparison to the complicated relationship amongst structure-function39–42 with a controlled drug release by overcoming physiological and pathological hurdles of living systems. Owing to the stable polymeric structure, these nanocarriers offer uniformity of particle size along with controlled drug delivery, which efficaciously reduces the effect of gastrointestinal environment subjected to oral administration. Some of the naturally-occurring polymeric substances, such as chitosan, dextran, heparin, and hyaluronan, have been widely explored as drug carriers for therapeutic applications.43,44 However, recently, the rational engineering of synthetic polymers including polyesters, polycarbonates, polycaprolactones (PCL), poly(lactic-co-glycolic acid) (PLGA), polyamides, polyvinyl imine (PEI), polyvinyl alcohol (PVA), and polypeptides have lured increasing magnetism in the nanomedicine-assisted functional world owing to their biocompatibility, nonteratogenicity, nontoxicity, and tunable biodegradation kinetics in vivo.45,46 For, instance, the PLGA's biodegradation is reliant on the hydrolysis prompted by the de-esterification of polymeric backbone, thereby generating monomeric lactic and glycolic acid, which are thereafter metabolically stabilized and finally eliminated by the body via Kreb's cycle-like natural pathway (Fig. 2). Owing to the excellent properties of these polymers, the US Food and Drug Administration (FDA) and European Medicines Agency (EMA) have clinically approved these polymeric substances as promising drug delivery vehicles in humans.
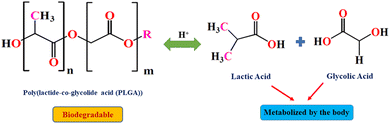 |
| Fig. 2 Biodegradation of PLGA polymer based on the hydrolytic cleavage of the copolymer and its subsequent metabolization by the body. | |
Several commonly studied organic and polymeric nanocarriers have been systematically discussed herein.
3.1.1. Micelle and vesicle-based nanocarriers.
Micelle-like nanocarriers is synthesized via the self-assembly of amphipathic polymers, which have gained increasing importance for utilization in drug delivery.47 The “water repelling” interior of the micelles produces a microenvironment toward the successful loading of lipophilic compounds and drugs. This causes a substantial improvement in the solubility of the hydrophobic drugs for attaining enhanced bioavailability. Likewise, the deliquescent core creates a stable interface amidst the hydrophobic core and water medium to improve the stability of colloid, thereby inhibiting aggregation and other undesirable connections with other components (Fig. 3).
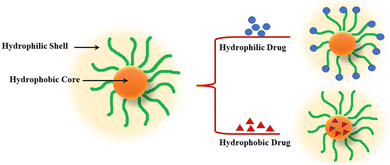 |
| Fig. 3 Structural representation of (a) Micellar self-assembly; (b) characteristics of encapsulated hydrophilic/hydrophobic drug. | |
Vesicles, on the other hand, are derived from amphiphilic polymers (also known as polymerosomes), which exhibit a unique bilayer structure possessing a hydrophilic central core. Most significantly, the “water-repelling” drug molecules are encapsulated within the hydrophilic core existing in the interior region of the double-layered membrane. In a recent report by Han and Wang et al., tumor and intracellular microenvironment receptive amphiphilic polymers bearing structural, functional diversity, as well as self-assembling surface morphology have been briefly highlighted.48,49 Owing to the unique structural characteristics, micelles and liposomes (vehicles) provide unusual safety to combat against degradation and diverse possibilities for targeted functionalization allied with combined therapeutic approaches.50–52
3.1.2. Liposomes.
Liposomes are usually lipid vesicles generated by an ordered arrangement of double layered phospholipid bearing cell-like structural properties.53 Despite the fact that polymer-functionalized nanocarriers possess many interesting characteristics toward in vitro/in vivo applicability, this type of lipid-derived drug release vesicles till date is consistent in maintaining its superiority in medicinal applications. In particular, the vesicles comprising of naturally-obtained or synthetically-derived lipids (liposomes) showcase an absolute nanomaterial-functionalized platform toward the rational engineering of advanced drug delivery vehicles in the biotechnology and nanomedicine-based multidimensional world.54,55 As a type of drug carrier, liposomes offer many advantages including nonimmunogenicity, nontoxicity, controlled drug release, extended time for drug activity, varying distribution of drug in vivo, enhancement in index of drug treatment, and reduced drug induced side effects.56 All these properties are associated with the ability of liposomes for undergoing versatile self-assembly,57–59 which is controlled by particular weak interactions that ultimately govern the colloidal constancy of these therapeutic drugs under extreme bioenvironmental conditions of ailing tissues.60–63 Considering the different types of liposomes, the cationic liposomes are positively charged, which clearly indicates that they might plausibly induce dose reliant cytotoxicity along with an inflammatory outcome. As complexes, these might undergo nonspecific interaction with negatively-charged serum proteins. In this consideration, neutral lipids64 as well as pH-responsive liposomes65 provide absolute ways for resolving the aforementioned issues.
Liposomes can be explored toward active drug molecules released at their targeted sites within the physiological systems, in particular ailing tissues or tumors. The inclusion of varying ligands, namely, peptides, monoclonal antibodies, aptamers, and growth factors, substantially advances the specific interaction of liposome during the process of drug release.66,67
3.1.3. Dendritic nanocarriers.
Dendrimers are usually 3D, intensely bifurcated nanomaterials that comprises of polymeric branched units that are covalently linked to the interior core and thereafter rearranged in concentric layers (namely, generations), which ultimately ends up in numerous external surface functionalities (Fig. 4(a)).68,69 Dendritic macromolecules can be organized in monodispersed space, which accounts for the facile administration and dissolution of insoluble drug components. Most importantly, these possess the propensity to enhance the soluble and bioavailable nature of hydrophobic drugs, which can be encapsulated within the intramolecular cavity or coupled to their surface-functionalized entities (Fig. 4(b) and (c)). As a step ahead, the prevailing numerous convenient surface functionalization along with nonaqueous environment within the packaging makes dendrimers efficacious drug delivery vehicles.70
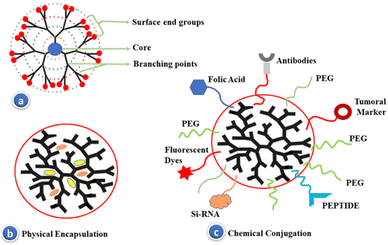 |
| Fig. 4 (a) Primary structural properties of dendrimers (b) dendrimer nanocarriers wherein the active components (including drug molecules) are entrapped within the internal cavity of dendrimers; (c) or conjugation to their surface-functionalized groups. | |
The most significant application of dendritic nanostructures involves the binding of appropriate chemical species into the surface. This phenomenon induces the growth of novel unprecedented prototypes that function as discrete sensing ligands and target specific components or imaging agents, while the targeted drug release application of dendrimers implicates a proficient exploration of dendritic nanocarriers toward the in vitro circulation of genetic material into the cells.71–73 The overall dendritic structure in solution is governed by a number of factors, including generation, length of spacer, surface functionalization, ionic strength, solution, as well as temperature.74,75 Although, the outstanding biological properties of the dendrimer-functionalized macromolecules make it extensively explored in biomedical and pharmaceutical domains, nevertheless, the presence of surface positive charges along with noncovalent interactions (electrostatic forces) exhibit a governing role in the targeted drug release process.
3.2. Inorganic nanocarriers
Recently, inorganic nanomaterials are extensively exploited for developing efficacious nanostructured systems toward application in drug delivery.76 These nanostructures usually comprise of two regions—an inner core comprising of the inorganic element, namely, gold, silver, quantum dots, silica, or Fe2O3 and an outer shell mostly consisting of an organic polymeric scaffold (or metals), which offer an appropriate substrate for conjugating biomacromolecules or safeguarding the inner core from undesirable physiochemical connections with the external biotic microenvironment.77 Amongst the various types of metal-functionalized nanocarriers, gold and silver are the most frequently used, which appear in varying structures that can be further classified into nanoparticles, nanorods, nanocapsules, nanocuboid, and nanowire.78 Silver and gold nanoparticles possess distinct properties such as SPR (surface plasmon resonance) and can be further explored for photothermal-assisted treatment of tumors and rheumatoid arthritis. The unprecedented magnetic along with plasmonic properties possessed by inorganic nanomaterials makes them ideal candidates for the indicative imaging of the ailing area in magnetic resonance (MR), computed tomography (CT), or positron emission tomography. Despite several advantages of inorganic nanomaterials including excellent biocompatibility and diversity, these display restricted achievement while treating chronic tissues owing to limited drug loading capacity and acute toxicity induced in vivo by gold and silver nanoparticles (in particular).79
Several commonly studied inorganic and metallic nanocarriers have been systematically discussed herein.
3.2.1. Carbon nanotubes.
Considering the varying allotropes of carbon, carbon nanotubes (CNTs) belong to the fullerene family that consists of either single or multiple sheets of graphene assembled into a cylinder-like tubular structure to give rise to single-walled carbon nanotubes (SWCNT) or multiwalled assembly, namely, multiwalled carbon nanotubes (MWCNT)80–82 (Fig. 5). CNTs are endowed with distinct physiochemical and biological properties along with their extensive ability of surface functionalization, which makes them a proficient contender as drug delivery vehicles. The SWNTs and MWNTs penetrate the cell via endocytosis or by direct insertion through the cell membrane. In addition to cell permeability, their inherent stability, tunable structure, and extensive drug loading ability, these are an extensively investigated class of nanocarriers toward the treatment of cancer-like life threatening ailments.83,84
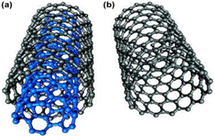 |
| Fig. 5 Structural representation of (a) MWCNTs, (b) SWCNTs (adopted with permission from ref. 73). | |
Anticancer drugs are either encapsulated within the central cavity or adhered to the CNT surface via covalent or noncovalent functionalization. In a literature report, it was perceived that the anticancer drugs are entrapped in the inner hollow core of the MWCNT based on an unreactive and nonaqueous platinum(IV) complex via hydrophobic–hydrophobic interactions. When subjected to chemical reduction, the anticancer drug was transformed into its cytotoxic and hydrophilic arrangement and thereafter detached from its respective transporter (Fig. 6).85
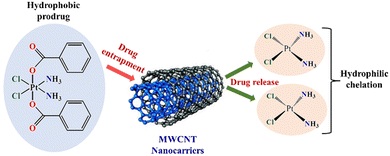 |
| Fig. 6 Entrapment of hydrophobic platinum(IV) prodrug within the cavities of multiwalled carbon nanotubes. Release from the CNT carrier of hydrophilic anticancer drugs (cisplatin) upon chemical reduction and hydrophobicity reversal. Adapted from ref. 86. | |
3.2.2. Metallic nanoparticles.
Metallic nanoparticles (NPs) having dimension 1–100 nm are extensively explored in medicinal applications and are comprised of cobalt, nickel, iron, gold, along with their oxides, namely, magnetite, maghemite, cobalt ferrite, and chromium dioxide. These are primarily synthesized and chemically tuned with diverse functionalities, which permit them to be adorned with different molecular entities, namely, therapeutic agents and biological molecules. The unprecedented magnetic properties, stability, and bio-adaptability of magnetic NPs enable them to undergo targeted drug delivery within the body, utilizing an externally applied magnetic field.87 For instance, superparamagnetic properties cause therapeutic agents to be stably delivered to the specific cell, thus facilitating the appropriate accumulation at the targeted tissue for delivering a safe treatment approach.88,89 Likewise, when metallic NPs are exposed to a varying magnetic field, these generate heat and magnetic hyperthermia, which makes it effective for tumor removal and treatment of cancer.90,91
Amongst the various types of metallic NPs, gold nanoparticles (AuNPs) have been extensively tailored for varying applications, including imaging agents (preliminary diagnostics), chronic diseases treatment (tumor therapy), as well as targeted drug delivery owing to their unusual electronic, optical, recognition, and biochemical functionalities.92 AuNPs comprise of an interior gold atom, which is enclosed by negatively charged reactive groups present on the surface, which can be readily functionalized by the addition of monolayered surface-active targeting ligands. The existence of negative charge makes them readily biofunctionalized via ionic, covalent, or physisorption. The AuNPs particularly used for therapeutic applications are prepared by the colloidal synthesis process route that involves a metal precursor, a reducing and stabilizing agent. Upon the specific tuning of electrical and optical properties, varying gold nanostructured particles can be generated with different shapes (nanosphere, nanorod, nanocage, and nanoshell) and sizes (1–100 nm) (Fig. 7). These are predominantly lucrative owing to the existence of surface plasmon resonance (SPR) bands,93,94 which assists them in converting light to heat and thereby scattering the as-generated heat for destroying the cancerous cells.
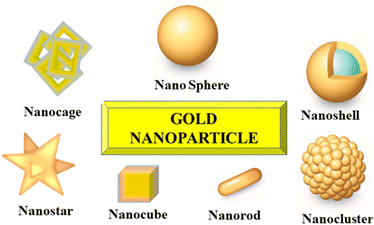 |
| Fig. 7 Examples of varying morphologies of AuNPs synthesized nanostructured architectures. | |
3.2.3. Quantum dots.
Quantum dots (QDs) are a class of semiconductor-based nanocrystals that are specifically aimed at fluorescence imaging owing to their unique luminous properties. Their fluorescent nature makes them ideal for innumerable biomedical applications, including drug delivery and cellular imaging.95 These consist of Group II and Group VI atoms, namely, CdS, CdTe, and ZnS. The synthesis of QDs involve either top-down methodology (mediated by X-ray lithography, ion-implantation, e-beam) or bottom-up process route (involving solution state self-assembly, followed by chemical reduction).96 Structurally, QDs comprises of three parts, an immensely small interior core of semiconducting compound (CdSe) having 2–10 nm diameter, encapsulated by a second semiconducting material, ZnS. Ultimately, this dual-layered structure is surrounded by a lid comprising of different materials (Fig. 8). Owing to the small size along with quantum effects, QDs exhibit unprecedented photophysical properties that permit the visualization of tumor during real-day assessment, drug loading, and finally its release at the specific location within the body. A majority of the traditionally used organic labelled dyes are unable to exhibit emission in the near-IR region (>650 nm), while QDs with their tunable emission characteristics are substantially superior in comparison to organic fluorophores and therefore particularly explored as biomarkers for the diagnostic imaging of cancer cells. The literature is replete with several reports wherein QDs are encapsulated in phospholipid micelles for passivating QDs toward biological applications.97 Interestingly, different types of ligands including peptides, vitamin B9 (folate), and macro proteins such as mAbs (monoclonal antibodies) can be readily implanted on the surface of QD, which triggers the growing interest in the rational engineering of nanotheranostic platforms toward concomitant detection, imaging, and therapeutic applications.98
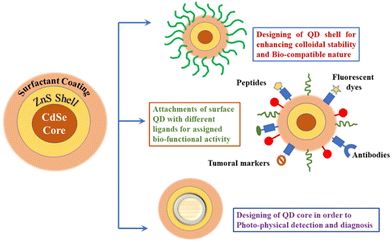 |
| Fig. 8 Designing approaches of QD-functionalized nanocarriers for effectual therapeutic applications. | |
3.2.4. Mesoporous silica-functionalized nanoparticles.
Silica (SiO2)-based nanoparticles deliver substantial advantages toward the designing of cost-effective complex systems via straightforward synthetic process route. In particular, their definite surface properties, porous architecture, and effective functionalization makes them potential candidates for increasing application in nanomedicine-based therapeutic delivery.99 SiO2 NPs possess extensive surface area, which are concealed with polar silanol moieties that promote a large amount of drug loading, improving the stability and biodistribution ability of the therapeutic drugs.100,101 In addition, these mesoporous silica-based nanocarriers (MSNs) undergo interaction with biomolecules such as nucleic acids, which permits it to be utilized as specific drug-releasing vehicles.102 Interestingly, their nanoporous dimension along with density can be readily tuned for attaining a persistent rate of drug delivery, making them potential nanoscale level drug carriers. Furthermore, the appropriate functionalization of MSN surface via chemical transformation of the surface active silanol moieties with varying location specific targeted agents facilitates the treatment of diseased tissues (such as tumor) mediated by the mechanism of active targeting.103,104 In this deliberation, various kinds of anticancer drugs, namely, paclitaxel, doxorubicin, and methotrexate, have been efficaciously transported to their targeted site by MSNs. Interestingly, different stimuli receptive molecular entities are capped within MSNs pores for inducing controlled and targeted drug release. For example, for delivering an encapsulated drug at the acidic tumor location, MSNs surface were known to be covered with B-cyclodextrin.105 Upon the further combination of these nanocarriers with contrasting agents such as gold, silver, metallic oxides (ferric oxide), organic coloring pigments, as well as quantum dots, its in vivo tracking is facilitated in living systems.106 Thus, MSNs are predominantly appropriate for a diagnostic purpose, specific drug release, biosensing, and cellular level uptake, in the therapeutic domain of application.
3.2.5. Stimuli-responsive nanoparticles (srNPs).
The stimuli-responsive nanoparticles (srNPs) are primarily functionalized for delivery, release, and activating the cargos in definite regions (for instance tumor microenvironments or intracellular regions of cancerous cells) by responding to internal/external stimuli, e.g., pH and enzymes.107,108 These have been rationally engineered by considering various pathological profiles in the normal tissues, intracellular compartment, and disease microenvironment to enhance the specificity, efficacy of drug delivery, as well as other biological activities. The appropriate design of nanocarrier and its structural optimization are amongst the few critical challenges to stimuli-responsive nanodrug delivery systems. There are several stimuli-responsive entities, including amide bond, diselenium bond, and ester bond, that have been purposefully introduced to varying materials for assembling the srNPs, which demonstrated an exceptional advancement on the synergistic therapy by controlling drug release.109 In addition, some inorganic materials of MnO2, Au, Ag, and Fe3O4 have also been extensively utilized as stimuli-responsive nanocarriers.110,111 Likewise, Banerjee et al., have unleased smart antiretroviral nanoparticles, which is strategically optimized for undergoing active and passive targeting in cancer chemotherapy. These nanocarriers are responsive to various triggers (internal and external). In case of antiretroviral therapy, the appropriate triggers for stimuli responsive drugs release include semen, enzymes, endosomal escape, temperature, and magnetic field. On the other hand, for chemotherapeutic application, further probable triggers include light and ultrasound, which are hitherto unexplored in HIV therapy.112
4. Therapeutic applications of nanoparticles in targeted delivery
The primary role of a therapeutic agent is related to “successful and targeted delivery” and its subsequent accumulation within the specific diseased site. to undergo an effective drug release, the therapeutic agent loaded vehicle must be reserved within the biological system for an appreciable duration, avoid the immunological system, target the specific diseased cell or tissue, and finally release the drug-loaded therapeutic agent.113 Nanoparticles with targeted delivery have been extensively explored for therapeutic application in the treatment of carcinogenic diseases. However, nowadays, in addition to cancer treatment, therapeutic applications of nanoparticles for curing other chronic ailments including Covid, cardiovascular disease, and neurodegenerative diseases, diabetes, HIV, etc., have attained great research efficacy. In this section, the therapeutic applications of nanoparticles in targeted delivery have been briefly outlined.
4.1. Insights of nanotechnology in therapeutic application against COVID-19
Coronavirus disease, more popularly, COVID-19 or SARS-CoV-2 infection, is an enduring worldwide pandemic, which is triggered by a virus belonging to the Coronaviridae family.114 The preliminary symptoms of this disease include fever, dry cough, body ache, mild to acute respiratory distress, loss of smell and taste, trauma, or failure of vital organs. Older people and the people already suffering from chronic diseases have a predominantly higher chance of evolving life-threatening complexities.115 The virus spreads implacably and severely, causing widespread socio-economic and health disruptions, which ultimately affects the overall safety and well-being of people and society. In this consideration, substantial efforts have been dedicated toward the development of preventive measures, diagnosis, and treatment methods for combating the distressing COVID-19. Unfortunately, at present, there is no medically approved technique for fighting this disease; however, the therapeutic approaches existing currently are handling the related symptoms.
In this consideration, nanotechnology-based treatment is a powerful diagnostic tool bearing important advantages in association with preventing, diagnosing, and delivering therapeutic approaches for managing viral diseases such as COVID-19.116,117 In the development of nanotechnology-based promising analysis, nanosensor-functionalized diagnostic approaches with improved drug delivery along with prolonged span of drug circulation for ensuring targeted outcome are of current research interest. Herein, the overall efficacy and performance of utilizing nanomaterials for preventing the spread of this global pandemic, speedy diagnosis of the disease, and related therapeutic methodologies have been systematically consolidated.
4.1.1. Use of nanotechnology toward the prevention of COVID-19.
The outbreak of this global pandemic has been increasing at an alarming rate, which necessitated proper preventive measures to be adopted. In this regard, nanotechnology have been extensively used as it delivers unprecedented new approaches for combating the spread of COVID-19. Therefore, the use of nanomaterials in disinfecting agents, gloves, masks, personal protective equipment (PPEs), and therapeutic agents is widely acclaimed.
(i) Nanomaterials in disinfectants.
Amongst the nanomaterials, metallic NPs, primarily TiO2 and AgNPs, along with rationally engineered hydrophilic nanocarriers, possess antiviral characteristics that would assist in safeguarding against COVID-19.118 Henceforth, various products utilize nanomaterials possessing antimicrobial property for disinfecting purposes. CAC-717 is an example of a product comprising of nanostructured materials that shows efficacy toward inactivating envelope and unencapsulated viruses.119,120 It has also been observed that the development of self-cleaning surfaces coated with metallic NPs (Ag, ZnO, Cu), which upon oxidation releases ions possessing antimicrobial properties, would be another approach for preventing the outbreak of this pandemic.121,122 Furthermore, nanodimensional TiO2 is a major constituent of UV-irradiated photosensitizers, which is extensively used for disinfecting hospitals and health centers such as indoor places.123 In addition to the use of disinfecting agents, practicing appropriate personal and interpersonal health hygiene, which includes the frequent washing of hands, especially after sneezing or coughing, would also held reduce its spread. Keeping this in mind, a few researchers have meticulously developed a nanometal-derived compound along with an aromatic therapeutic herb stoichiometrically formulated in an indigenously developed, environmentally safe, innocuous handwash-possessing disinfecting properties, and suitable for cleansing clothes.124 At present, carbon nanoparticles-based air and membrane filtration units are widely used for the removal and destruction of coronavirus carrying pathogens in air and water, respectively, to inhibit the further spread of COVID-19.
(ii) Nanomaterials in personal protective equipment (PPEs) such as masks and gloves.
Nanofibers and nanofiber-derived webs are frequently utilized as chief component of masks for minimizing the dispersion of macrorespiratory particles and also safeguard against the transmission of droplets by patients.125 It is well known that during the global pandemic, N95 respirators were extensively used, which demonstrated around 85% efficacy of filtering sub-nm particles, preventing SARS-CoV-2-induced contamination.126 However, the filtering ability of N95 respirators can be further improved by the incorporation of disposable tunable nanoporous silicon-functionalized membranes. In a current investigation, Si et al. established a bio-benign nanofibrous membrane, which possessed reusable antibacterial and antiviral properties which were potent for generating biocidal reactive oxygen species (ROS) upon exposure to sunlight. These membranes possess high bactericidal (>99.999%) and virucidal (>99.999%) activity and demonstrated immense ability to absorb >99% of fine particles.127 Interestingly, not only in masks but these nanomaterials have also been exploited for medical/surgical gloves fabrication to protect against COVID-19. Owing to the virucidal property, silver NPs are a major component of these gloves. As a step ahead, it has been investigated that the COVID-19 virus penetrates into the cells by the angiotensin-converting enzyme 2 (ACE2) receptors. Thus, reducing the levels of ACE2 within the body may plausibly aid in minimizing the rate of infection.128 This is exactly performed by the gloves indigenously made up of nanomaterials-encapsulated ACE2 proteins. These gloves could readily prevent the viruses from passing through the host cellular membrane by the neutralization of the same.129 These outcomes reveal that the use of engineered nanoarchitecture membranes in PPEs could readily stop the rapid outspread of SARS-CoV-2.
4.1.2. Use of nanotechnology toward the diagnosis of COVID-19.
Diagnostics exhibit the chief function in inhibiting COVID-19 by restricting the outspread of this virus by recognizing the infected person, followed by isolation. Although a handful of diagnostic strategies are practiced, yet the development of ultrasensitive and speedy diagnosis of COVID-19 is still quite tricky and challenging.130,131
At the primary level, chest computerized tomography (CT) scans along with molecular analysis have been implemented for the screening and diagnosis of COVID-19.132 Likewise, serological tests are straightforward and efficacious in diagnosing COVID-19, yet their application is limited owing to the prolonged time required for adaptive immune activation.133 In this regard, a wide range of nanostructured materials such as gold and magnetic nanoparticles, CNTs, QDs, and nanozymes have been widely explored for the diagnosis of this disease.
4.1.2.1. Gold nanoparticles.
Amongst the metallic nanoparticles, AuNPs have attained acute research significance owing to its optoelectronic properties that can be utilized by varying the types of biosensors for rapid chromogenic, electrochemical, and plasmonic sensing.134 An important application of AuNPs is in Lateral Flow Assays (LFA), wherein the targeted molecules are labelled and which induces color transition in the test zone, which can either be qualitatively estimated by bare eye or quantitatively analyzed via smartphone for assessing the target concentration.135 LFAs are frequently used in POC devices for the rapid detection (5–30 min) of antibodies and proteins present in complex biomatrices including urine, blood, and saliva and could be readily stored at ambient temperature without depriving biomolecular functions.136 Owing to this, IFA-based biosensors were distributed at kiosks in healthcare systems for the speedy decentralized diagnosis of COVID-19. LFAs undergoes the detection of immunoglobulin G (IgG) and immunoglobulin M (IgM) antibodies for the recognition of SARS-CoV-2 proteins and thereby identifies the infected patient.137 These approaches clearly reflect the ability of AuNPs toward the rapid detection of COVID-19.
4.1.2.2. Magnetic NPs (MNPs).
Magnetic NPs (MNPs) are particularly utilized in biosensors owing to signal intensification, preconcentration of targeted analytes, and, most significantly, magnetic separation prior to its recognition of analytes.138–140 In this regard, Somvanshi et al. fabricated a surface-modified MNPs and its subsequent protocol for extracting viral RNA for promising application in diagnosis of COVID-19.141 Zn(II)–Fe(III) NPs were prepared by the combustion process route; thereafter, the surface of as-generated NPs were chemically functionalized with SiO2 and –COO−-modified PVA. This NP provides an absolute platform for the automatic extraction of viral RNA, the dispersed sample and curtails the overall operational procedure, which delivers an immense ability toward the molecular level diagnosis of COVID-19. The traditional methods of analyte recognition from complex matrices including blood and urine offers some gaps and challenges, which includes exceptionally low analyte concentration and co-interference of other biomolecules in signaling response that appreciably diminishes the precision of analysis. MNP-functionalized biomolecular receptors readily overcome these lacuna and improve the performance of biosensors.142
4.1.2.3. Quantum dots (QDs) or semiconductor materials.
Owing to the excellent photophysical properties of QDs, it is efficaciously used as a fluorescent taggant in chemosensors and biosensors.143 Ashiba et al. unveiled an ultrasensitive biosensor for the detection as well as prevention of spreading of the virus. A surface plasmon resonance (SPR)-mediated luminescent immunosensor was developed wherein QD was used as an emissive dye.144 Background signaling was controlled by optimizing various parameters, including the excitation efficacy of QDs, extent of electric field improvement by SPR, and optimization of self-induced fluorescence. Consequently, the as-prepared sensor could attain a detection threshold of 0.01 ng mL−1 of the virus, which corresponds to the existence of hundreds of virus particles. In another investigation, QDs were linked with RNA aptamer-functionalized chip toward the ultrasensitive and speedy recognition of SARS-CoV N protein.145 The QD-ligated RNA aptamer could explicitly bind with the SARS-CoV N protein immobilized on the chip and thereby induce a visual response. The limit of detection (LOD) was obtained to be as low as 0.1 pg mL−1. This clearly reflects the usefulness of QD-functionalized probes for attaining the ultrasensitive diagnosis of COVID-19.146
4.1.2.4. Carbon-based nanomaterials.
Amongst the carbon-based nanomaterials, carbon nanotubes (CNTs), graphene, and carbon dots (CDs), broadly considered as zero-(0D), one-(1D), and two-(2D) nanomaterials, are potential contenders in the diagnosis of COVID-19.147 The literature reports the utilization of CNTs for the diagnosis of SARS-CoV-1 and SARS-CoV-2–like respirational viruses. A nanobiosensor was developed consisting of SWCNTs, which was chemically modified with ACE2 via noncovalent interaction and demonstrated immense binding propensity toward SARS-CoV-2-spiked protein. Interestingly, SWCNT induced two-fold emission enhancement in the existence of the targeted virus.148 Likewise, Seo et al. developed sheets of graphene improved with antibodies to fight against the S protein of SARS-CoV-2 and thereby produce a field-effect transistors (FET)-derived biosensor for the effective diagnosis of COVID-19.149 Together, these investigations clearly propose carbon-functionalized nanomaterials as promising tools for the decentralized diagnostic analysis of COVID-19.
4.1.2.5. Nanozymes.
These are artificially produced enzymes comprising of nanostructured materials possessing analogous efficacy as that of naturally-obtained enzymes.150 These are extensively used for the diagnosis and treatment of diseases owing to its exceptional catalytic property and rapid response along with self-assembling ability.151,152 For instance, an unprecedented nanozyme-assisted chemiluminescent paper-functionalized biosensor for the diagnosis of COVID-19. Usually, the conventional chemiluminescent-based immunodiagnostic approach exploits naturally-occurring proteases such as horseradish peroxidase (HRP) or alkaline phosphatase, which are related to low storage stability, tedious sampling procedure, and is economically unfeasible, which restricts their practical applicability. On the contrary, the anticipated biosensor utilized peroxidase imitating Co–Fe@hemin nanozyme over normal HRP, which substantially amplified the chemiluminescent response, thereby attaining a low detection threshold of 0.1 ng mL−1. Impressively, the synthetically-prepared nanozyme exhibited improved thermal and pH stability over its natural analogue. Consequently, the biosensor could be successfully explored for the diagnosis of COVID-19 in a resource-constrained environment.153
4.1.3. Use of nanotechnology toward the treatment of COVID-19.
The rapid outspread of new viruses along with their heterogeneity necessitates the development of novel therapeutic agents.154 The conventional antiviral treatment strategies suffer from severe challenges including poor selectivity, which results in the cytotoxicity of the host cells. In this regard, nanotechnology opens a new arena as an antiviral agent in therapeutic applications. Most importantly, the flexible property of NPs enables them to tunably undergo drug delivery as well as inactivate the targeted virus, owing to which it plays an important role in combating against SARS-CoV-2 in its different stages.155 Inhibiting the activity of proteins present on the viral surface eventually causes the death of the virus; thus, the NP could successfully reduce the internalization of the virus.156 Amongst the antiviral drugs, lopinavir, chloroquine, remdesivir, ritonavir, and ribavirim have showcased outstanding therapeutic action against SARS-CoV-2.157
The advancement of nanotechnology in COVID-19 drug delivery are owing to these advantages. (i) The surface morphology and dimension of nanocarriers allow the delivery of drugs to biologically-unavailable regions incapable of inducing an immune response,158 (ii) greater relative ratio of surface-to-volume, which improves the drug loading,159 (iii) the ability of NPs to cross the cell membrane possessing negative surface charge,160 (iv) metallic NPs (AgNPs, AuNPs) possess inherent virucidal property.161 In this regard, various nanostructured materials including metallic, metal oxide nanoparticles, Exosomes, CNTs, and QDs have been extensively explored for the treatment of CoVs.
4.1.3.1. Metallic nanoparticles.
Amongst the metallic NPs, AuNPs have attracted immense research interest in the diagnostic and therapeutic application of COVID-19. The facile functionalization of AuNPs utilizing the strong bond amidst gold and thiol (–SH) ligands is the chief driving force underlying its application in diagnosis and treatment of CoVs.162 In a recent investigation, it has been observed that upon the linking of AuNPs with the extended chain of sulfonate mercaptoethanesulfonate (MES) and undecanesulfonic acid (MUS) triggered an irreversible distortion in various viruses, in particular the respiratory syncytial virus along with in vitro and in vivo analysis. The plausible mechanistic course of interaction demonstrated that the chemical modification of AuNPs with MUS exhibited polyvalent binding interaction with the virus that destroys the protein-coated structure. Henceforth, such a type of polyvalent binding interaction might be a plausible unprecedented strategy for the treatment of COVID-19.163
It has been experimentally observed that AgNPs within 2–15 nm diameter displays anti-SARS-CoV-2 activity. Likewise, immunofluorescence analysis established that encapsulating polyvinylpyrrolidone with 10 nm dimension AgNPs causes complete inhibitory action of SARS-CoV-2, while 100 nm AgNPs did not induce any promising effect.164,165 A variety of inhalation and ingestion-based formulations comprising of AgNPs are commercialized as effective therapeutic agents.166
4.1.3.2. Metal oxide nanoparticles.
Metal oxide nanoparticles (MONP) are primarily investigated owing to their antimicrobial activity,167 which is governed by reactive oxygen species (ROS) production. For instance, iron oxide nanoparticles (IONPs) are innocuous and efficacious contenders for the therapeutic application in COVID-19 in consideration of its iron dysregulation. In a current investigation, it was perceived that interaction between SARS-CoV-2 and other receptors such as hemoglobin occurred in the RBC precursors, which resulted in the denaturing of hemoglobin and dysregulation of iron. In addition to IONPs, Zn ONPs are also explored owing to its biocompatibility, noncytotoxicity, and abundant nature. In another study, the antiviral property of ZnO NPs against H1N1 virus displayed that PEG (polyethylene glycol)-encapsulated ZnO NPs demonstrated superior antiviral action and reduced cytotoxicity over the unmodified ZnO surface. Henceforth, ZnO NPs can be effectively used as an antiviral agent for combating COVID-19.168,169
4.1.3.3. Carbon-functionalized nanomaterials.
Carbon-functionalized nanomaterials, including CNTs and graphene, exhibit outstanding physicochemical properties, which enable them for being utilized in scientific and technological interventions. In terms of COVID-19 treatment, CNTs are related to certain disadvantages including toxicity and fibrosis.170 In an in vitro study, it was observed that the intranasal administration of CNTs caused an increase in the infectivity of influenza H1N1 virus within the lung epithelial cells.171 In this regard, the surface modification of CNTs using lactones, carboxylic acid groups, as well as hydroxyls could plausibly be a promising strategy for overcoming these challenges and providing sustainable strategies for improving the effectiveness of COVID-19-treating therapeutic agents.172 Similarly, graphene and its derivatives are excellent nanoplatform for fighting against viral induced diseases. Reduced graphene is known to inhibit viral infections by inactivating the virus activity just before its entry within the host cell. Interestingly, silver-encapsulated graphene nanostructured composites tend to block the CoV in a concentration reliant way against infections induced by both encapsulated and nonencapsulated viruses. Such nanocomposites exhibit greater inhibition of CoV over graphene oxide (GO).173
4.1.3.4. Quantum dots (QDs).
QDs demonstrate an important function in treating human CoV infections. In a recent study, the antiviral activity of seven types of functional carbon quantum dots (CQDs) were explored for treating of human CoV HCoV-229E. The CQDs were generated obeying the hydrothermal carbonation of the precursory materials (carbon), Vitamin C, followed by post synthetic modification utilizing boronic acids. Upon undergoing QD treatment, the concentration-dependant inactivation of the virus was speculated.174 The entry of HCoV-229E within the host cell was prevented due to the plausible interaction of CQDs functionalities with the entry receptors of HCoV-229E along with the inhibition of virus replication phase.175 Currently, cationic CDs hydrothermally derived from natural sources such as curcumin have also been exhibited for successfully combating against coronavirus.176 The combined applications of nanotechnology toward prevention, diagnostics, and treatment of COVID-19 has been demonstrated in Fig. 9.
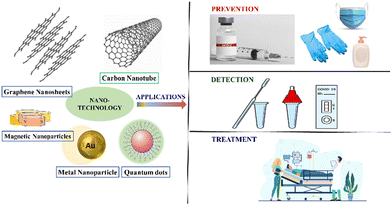 |
| Fig. 9 Combined applications of nanotechnology toward prevention, diagnostics, and treatment of COVID-19. | |
4.2. Insights of nanotechnology in therapeutic application against cancer
Cancer is one of the prime reasons of mortality and chemotherapeutic treatment is the most explored approach for dealing with this life-threatening ailment. Nevertheless, there are several disadvantages related with chemotherapeutic agents including poor aqueous solubility, dose-reliant toxic effect, and lack of tumor specificity.177 In addition, resistance to multiple drugs is another difficulty encountered in chemotherapy, which primarily occurs owing to enhanced efflux pumps that are accountable for readily exporting the anticancer drugs across the cell membrane.178 In this relevance, the advancement of nanostructured materials could plausibly overcome these challenges by the precise delivery of the drug at the targeted cancerous tissue with an optimal rate, promising efficacy, and minimal side effects.179 Passive and active targeting are the two major mechanisms adopted by the as-generated nanoparticles that govern the delivery of anticancer drugs at the targeted tumor site.180 Passive targeting is governed by the accumulation of the nanoparticle-based therapeutic agent within the tumor tissue to improve the imaging owing to the permeability tension effect (EPR) in the diseased tissues.181 On the other hand, active targeting is based on the principle of coupled interaction of the nanoparticle with the anticancer drug, viz., epidermal growth factor receptor (EGFR) for attaining targeted drug delivery. Several nanostructured materials including liposomes, AuNPs, CNTs, dendritic structures, micelles, and QDs have been extensively exploited in the treatment of cancer.
4.2.1. Liposomes.
Liposomes are the first nanoscale drug that have been clinically approved for therapeutic applications.182 These nanospheres comprise of naturally occurring or artificially generated outer phospholipid membrane and aqueous state nuclei encapsulating the drugs.183 Hydrophilic, hydrophobic, as well as neutral drugs can be readily transported in liposomes. It has been investigated that up to 100 nm-sized liposome can readily penetrate into the tumor and possess a longer half-life period while liposomes possessing larger size have shorter half-life since these are readily identified and removed by the mononuclear phagocyte system.184 In a recent study conducted by Kelley and Liu et al., it has been unveiled that the liposomal form of chemotherapeutic drug, Adriamycin, has been widely utilized for controlling metastatic ovarian cancer, wherein it has demonstrated substantial therapeutic advantages.185,186 The application of liposomes in the treatment of breast and prostate cancer have also gained increasing importance.187,188 Liposome-mediated nanocarriers have delivered a unique drug combination that improves the therapeutic applications and, most significantly, it can reverse the resistance induced by drugs.189
4.2.2. Gold nanoparticles (AuNPs).
AuNPs are broadly explored inorganic NPs wherein mixed single-layer encapsulated clusters are present, which are primarily made of gold core and a potential contender in drug delivery.190 The gold in the interior is unreactive and nontoxic in nature. As a consequence, surface-modified AuNPs are confirmed to augment the accumulation of the drug in tumor cells and thereby reverse the drug resistance.191 The mechanistic action of AuNPs involves both active and passive targeting pathways (Fig. 10). AuNPs have also been actively involved in multimodal ways of treating cancer, which includes gene therapy and photothermal therapy along with immunotherapy.192,193
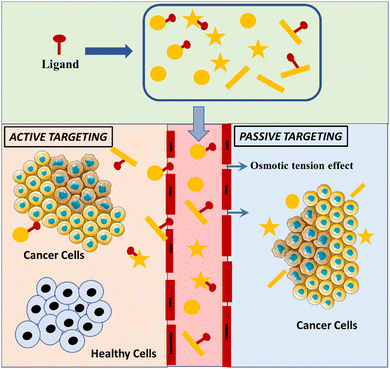 |
| Fig. 10 Different types of gold nanoparticles (varying sizes, morphologies, and ligands) accumulate within tumor tissues via osmotic tension effect (Passive targeting) or localize within definite cancer cells in a ligand–receptor binding way (Active targeting). | |
Carbon nanotubes (CNTs).
The unique and unprecedented physicochemical and biological properties of CNTs including extensive surface area, mechanical strength, electrical and thermal conductivity have made CNTs a promising contender in expansive therapeutic applications.194 Impressively, CNTs can readily absorb light in the near-infrared (NIR) region, which causes the NTs to be heated up via thermal ablation and ultimately targets the tumor cells.195,196 The natural forms of CNTs prompts the biofilms to be noninvasively penetrated and are considered as extremely capable carriers of varying drug molecules into the living cells.197 Consequently, CNTs have been widely used for the targeted delivery of doxorubicin, paclitaxel, and methotrexate siRNA by assembling with these drugs and administrating the same for the in vitro as well as in vivo treatment of various cancers.198
4.2.3. Dendritic structures.
Dendrimers are considered to be nanostructured materials possessing a spherical polymeric core with uniformly spaced branches.199 Various types of molecules, which include polyacrylamide, polyglycerol-succinic acid, polylysine, and melamine are frequently used for preparing dendritic structures.200 Dendrimers display varying chemical morphology and characteristics including alkalinity, hydrogen bond forming ability, and charge that can be monitored by either the growth of dendrimers or by tuning the surface functionalization of dendrimers. The dendrimer-functionalized drugs are prepared by the covalent interaction of anticancer drugs with the peripheral groups of dendrimers.201 The drug molecules initially adhere to the individual dendrimer, and the drug is controllably delivered by virtue of this binding. An additional advantage of dendritic structures lies in its DNA binding ability, as observed in DNA-polyamides clustered DNA-poly(amidoamine) (DNAPAMAM), which makes them immensely proficient in destroying cancerous cells, which prompt the folate receptor.202
4.2.4. Polymeric micelles.
Polymeric Micelles (PNPs) are defined as solid micelle-possessing particle dimension within the range of 10–1000 nm.203 Polymer NPs, nanospheres, nanocapsules, or polymer micelles are unanimously termed as Polymeric Micelles (PNPs) and these are reported to be the first polymer-based drug delivery vehicles.204 PNPs are known to seize hydrophobic drugs via covalent bonding or hydrophobic interaction with the core. Upon the oral and parenteral administration of these drugs, it reaches the diseased cells by varying ways and possibly offer an alternative platform for reducing the cytotoxicity of healthy tissues over the cancerous cells. Nevertheless, the major setback allied with PNPs in the domain of cancer-treating nanomedicine lies on the extent of effectivity of delivering the drugs at the targeted site with minimal side effects. For instance, Adriamycin (a conjugated nanostructured material) has been extensively used for treating varying types of cancer, wherein it attained a decent extent of therapeutic activity. However, it exhibited numerous side-effects including toxicity and heart-related problems. Nevertheless, there is still room for advancement using Doxil, which represents the liposomal form of doxorubicin and is related to less cardiotoxicity in infected persons, and henceforth offers a safer nanostructured alternative for scientists and researchers in the long run.205,206
4.2.5. Quantum dots.
Over the years, different types of QD-functionalized techniques including modifying of QD conjugates and immunostaining of QDs have emerged. With the advancement of multiplexing ability, the conjugation of QDs immensely surpasses the monochromatic analysis in terms of time as well as cost-efficacy.207 Interestingly, the immunostaining of QD serves as a probable tool in the diagnosis of cancer by detecting several biomarkers of tumor, which includes a cell protein or other parts of an assorted sample of tumor.208 Most importantly, the potentiality of QDs to be internalized within a single targeted organ enables it to be a potent solution in opposition to untargeted drug delivery and also circumvents the toxic effects of chemotherapeutic treatment. In recent days, the surface functionalization of QDs with peptides and antibodies such as biomolecules in the in vivo approach can be explored for targeting tumors and thereby provide sustainable solutions in cancer imaging and its treatment. Likewise, certain extremely sensitive QD-functionalized probes have been reported, which exhibits the polychromic luminescence imaging of cancerous cells in vivo and it recognizes ovarian cancer marker cancer antigen 125 (CA125) in varying specimens, viz., fixed cells, sections of tissue, and tissue graft.209 In a report investigated by Chen et al., it was observed that QD-based probes could readily detect breast cancer and established that in contrast to conventional immunohistochemistry, QD immunohistochemistry (IHC) could readily detect Human Epidermal Growth Factor Receptor 2 (HER2) in extremely low expressions.210,211
4.3. Insights of nanotechnology in therapeutic application against HIV/AIDS
Human immunodeficiency virus (HIV) is a part of Lentivirus genus, subcategory of Retroviridae family, which is responsible for causing acquired immunodeficiency syndrome (AIDS). It is usually transmitted by the means of sexual intercourse, exposure to contaminated intravenous drug injection paraphernalia, and transmission from mother to child.212 HIV is the worst adversity that not only affects the global health but also influences financial advancement as well as social constancy. In this consideration, the prevention and treatment of HIV-induced infection is a challenging task. Unfortunately, to date, there is a lack of efficacious HIV vaccine for therapeutic applications. Impressively, owing to the unique properties, nanomaterials and nanotechnology provide a sustainable solution for dealing with the bottlenecks related to the conventional HIV vaccines. In particular, the physiological function of the HIV vaccines is stimulated with the assistance of nanostructured architectures. Scientific community has spent millions of rupees for developing the first and foremost nanomaterial-based anti-HIV drug, which is readily soluble in water, abides by the appropriate procedure of administration, and is primarily applicable to newly born babies. Currently, a diverse range of nanostructured materials, viz., inorganic, polymeric nanomaterials, liposomes, and micelles act as promising contenders for adjuvating HIV vaccines.213
4.3.1. Inorganic nanomaterials.
Inorganic nanomaterials are a class of nanostructured materials that primarily comprises of metallic elements, gold, silver, titanium, and silicon and their compounds including carbon nanomaterials, and calcium. These are widely explored owing to the therapeutic applications, bioimaging, and molecular tagging of biomarkers. Amongst these, appropriately functionalized gold, silver, and platinum noble metals exhibit potential activity in the targeted drug delivery for HIV inflammation treatment.214 The inhibition of HIV-induced contamination could be improved by the alteration of nanoparticle size, its physical properties along with the conjugate density on NPs surface. AgNPs can readily bind with the gp120 protein and stops the entry of the virus, thereby inhibiting CD4-assisted viral fusion and finally interferes with the HIV life cycle in its post-invasion phase. Likewise, surface-functionalized gold nanoparticles (AuNPs) provide an absolute nanostructured platform as a vaccine neo-adjuvant delivery vehicle for AIDS treatment.215,216 Furthermore, the conjugation of AuNPs with TAK-779 and SDC-1721 promotes improved antiHIV property in comparison to the aqueous AuNP solution. Improving the multivalency of the AuNPs by suitable engineering would efficaciously enhance the interaction of AuNPs with the targeted receptors.217
4.3.2. Polymeric nanomaterials.
Polymeric nanoparticles have acquired immense attention and are potential contenders as vaccine adjuvants owing to their properties including easy synthesis, cost-effective nature, biocompatibility, biodegradability, water solubility, nontoxicity, and most significantly, the protection of antigen from rapid degradation. These are generated via the suitable approach for targeted antiretroviral (ARV) drug-based delivery. Different types of polymers are explored for constructing antiHIV drugs that includes chitosan, poly(lactic acid) (PLA), poly(lactic-co-glycolic acid) (PLGA), and poly(methyl) methacrylate.218 Polymer-based NPs as drug delivery vehicles are endowed with several advantages that includes controlled in vivo drug release by the adjustment of solubility, pH, zeta potential, effective crossing of the blood-brain barrier, and reticuloendothelial system, which promotes improved absorption by gastrointestinal mucosa, thereby promoting novel drug-administrating routes with reduced cytotoxicity and improved effectivity.219
Chitosan is extensively investigated for the controlled release and transportation of drugs across the biological barriers220 on account of its intense immunostimulatory characteristics and reduced immunogenicity.221 Furthermore, chitosan can also be utilized for enhancing the penetration of active compounds by promoting the bioavailability of trans-mucosa of HIV enveloped glycoprotein vaccine, CN54gp140. This polymeric nanomaterial encourages the delivery of mucosal vaccine and enhances the definite antigen IgG and IgA-induced antibody responses.222
Likewise, PLGA is also clinically exploited owing to its appreciable biocompatible and biodegradable nature. In this regard, Zhou and coworkers synthesized microspheres comprising of PLGA that entrapped HIV vaccine DNA as well as polyethyleneimine complexes. The inclusion of PLGA-derived microspheres enhanced the DNA delivery into APC protein and subsequently unconfined the complexes of PEI/DNA for over a couple of weeks (in vitro). Most importantly, embedding the vaccine within the microspheres protected the drug from degrading and substantially increased the responses of serum antibody and potent CTL.223
4.3.3. Liposomes.
The surface of these phospholipid-containing spherical vesicles are readily functionalized with various molecules and lipids to promote the uptake capacity of phagocytic cells. Liposomes, upon getting into a living organism, embeds itself within the mononuclear phagocytic cells (macrophages), such that the liposomes become useful anti-HIV drug transporter toward the targeted cell.224 Consequently, liposomes improve the efficacy of the anti-HIV medicine by reducing its side effects. Literature reports that around 20 years ago, the primary attempts made for delivering HIV antigens involved in the utilization of liposomes.225 Philips and coworkers investigated the effect of liposome components on the immune system response assisted by the subcutaneous injections (s.c.) route. It was speculated that liposomes comprising of dipalmitoylphosphatidylcholine (DPPC)/dimyristoylphosphatidylglycerol (DMPG) prompted substantially higher HIV gp120 specific levels of IgG over alum.226 It is familiar that conventional vaccines may plausibly induce cytotoxic T-cell responses, which is necessitated for protecting against HIV-induced intracellular infection. In this consideration, Korsholm and coworkers reported a cationic adjuvant formulation (CAF) 09 that comprised dimethyldioctadecylammonium (DDA)-liposomes stabilized by monomycoloyl glycerol (MMG)-1 in association with the Toll-like receptor 3 (TLR3) ligand. Interestingly, CAF09-HIV Gag p24 tempted a higher number of antigen-specific CD8 + T cells in comparison to aluminium-containing adjuvants. As a step ahead, the intraperitoneal injection (i.p.) administration process route was proven to be better in the comparison of the subcutaneous injections (s.c.) administration process route in case of CD8 + T-cell response priming.227
4.3.4. Dendrimers.
Dendritic cells with an appreciable number of surface-active groups and central cavity acts as promising adjuvants of chemical drugs, peptides along with HIV inhibiting genes. Likewise, during gene therapy, dendrimers play the role of viruses for transferring the interfering genes to the targeted cells for suppressing the HIV replication. Aline and coworkers loaded dendritic cells (DCs) with HIV p24 protein on to the surface of surfactant-free anionic (D,L-lactic acid, PLA) NPs. The dendrimers efficaciously swallowed the p24 embedded NPs and prompted the enhancement of the surface expression of MHC-I, MHC-II, CD40, CD80, and CD86 and secretion of IL-12 (p70) and IL-4. Furthermore, dendrimer-pulsed p-24 NPs instigated high serum, mucosal antibodies, and also produced intense Th1/Th2-type and extensive CTL responses.228 Polyvalent phosphorous-comprising catanionic dendritic cells engineered with galactosyl ceramide derived analogues induce the substantial inclination to the V3 loop of HIV-1 viral envelope protein gp120, which hinders the viral fusion with the plasma membrane and thereby inhibits its entry.229 Thus, dendritic architectures can also be measured to have strong influence toward discerning HIV exclusion. The first dendrimer-functionalized drug, namely, VivaGel® (tropical microbicides), has been presented to the US FDA for investigating the novelty of the antiHIV drug. The drug comprises of dendritic architecture in the nanoscale dimension, which adheres to the HIV virus and prevents it from infecting the body cells.
4.3.5. Niosomes.
Niosomes are referred to as nonionic surfactant vesicles, which are synthesized by the self-assembly of hydrous synthetic nonionic surfactant monomers and are able to encapsulate a wide range of drugs. These expand the bioavailability of ineffectively absorbed oral drugs and improve the penetrating power of drugs via skin. Florence and Bailie in the year 1989 reported niosomes to be an alternative to liposomes and are considered to exhibit reduced toxicity over liposomes.230 Herein, the solubilized drugs exist within the hydrophilic compartment amidst the bilayer, while the insoluble portion is entrapped within the dual layered matrix. In 2001, Gopinath and coworkers reported the intravenous delivery of antiHIV drugs encapsulated in niosomes.231 Herein, it has been observed that prolonged levels of azidothymidine AZT within the serum of rabbit soon after niosomal AZT treatment occur owing to a mutual effect of steady in vivo release and avoidance of extravascular distribution. Fig. 11 schematically describes the role of niosomes in targeted anti-HIV drugs. Zidan et al. in 2011 unveiled a novel paediatric anti-HIV tenofovir niosomes assisted by high-pressure homogenizer. The performance of these high pressure homogenized niosomes were influenced by innumerable factors including vesicular size, electrical characteristics, data of drug encapsulation, as well as its targeted delivery.
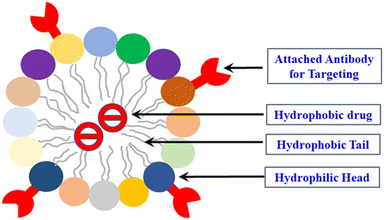 |
| Fig. 11 Schematic outline of niosomes and its role as antiHIV drug. | |
4.3.5. Membrane-camouflaged nanoparticles.
In the recent past, there has been a plethora of reports wherein a great deal of attention is devoted toward the exploitation of Inorganic Nanoparticles (INPs) in therapeutic applications. However, there are certain lacunas related to conventional INPs that includes its speedy elimination by the immune system, lower accumulation in the targeted sites, and acute lethality to the organism.232 To overcome the distinctive confinements of INPs, researchers have taken inspiration from nature by exploiting cell membrane camouflaged INPs by embedding varying cell membranes onto the surface of INPs. The nanotechnology of cell membrane-camouflaging associates the strength of cell membrane with INPs and triggers the integration of targeting ability, biocompatibility of nanocarriers. M. Pereira-Silva et al., unleased a promising cell-membrane–coated NPs which is a potential contender for developing novel therapeutic and vaccination strategies toward the management of COVID-19. The unprecedented approach described herein restricts the entry of SARS-CoV-2 and prevents its replication in the host cells.233 In another work, Zhang et al., engineered white blood cell-derived cell membrane vesicles that were coated onto the surface of magnetic nanoclusters toward the formation of artificial antigen-presenting cells and uses magnetic control for achieving targeted delivery. Furthermore, this strategy delivers promising application in the domain of adoptive T-cell-mediated cancer immunotherapy and provides insights toward targeting the inflammation of and corresponding imaging therapeutics.234 In a nutshell, the rational engineering of cell membrane engineering using physical engineering technique, followed by chemical modification and biological functionalization approach endows this biological cell membrane with abundant functionalities, thereby making it a potential weapon toward chronic diseases-related therapeutics.
Table 1 gives a detailed description of the composition, size, and applications of different loaded nanodrugs.
Table 1 The composition, size, loaded drug, applications, etc., of different nanodrugs
S. no. |
Name |
Composition |
Size (nm) |
Loaded drug |
Therapeutic applications |
Ref. |
1. |
AuNPs |
Gold |
5–400 |
Sulfonate mercaptoethanesulfonate (MES) and undecanesulfonic acid (MUS) |
COVID-19 treatment |
163
|
2. |
AgNPs |
Silver |
10 |
Polyvinylpyrrolidone |
Anti-SARS-CoV-2 activity |
164 and 165
|
3. |
ZnONPs |
Zinc oxide |
16–20 |
PEG (polyethylene glycol) |
Influenza H1N1 virus |
168 and 169
|
4. |
CNTs |
Carbon |
— |
Doxorubicin, paclitaxel |
Different cancer |
235
|
5. |
IONPs |
Fe3O4 |
150 |
Doxorubicin |
Cervical cancer |
235
|
6. |
Liposome-PEG |
Polyethylene glycol |
100 |
Doxorubicin |
Metastatic breast cancer, metastatic ovarian cancer |
236
|
7. |
Polyamidoamine dendrimer |
Polyamidoamine |
— |
Cisplatin and doxorubicin |
Different cancer |
237
|
8. |
Zeolitic imidazolate framework-8 NPs |
Zeolite and Imidazole |
100 |
Dihydroartemisinin |
Liver cancer |
238
|
9. |
Polymeric NG (PNIPAAm) |
Poly(N-isopropylacrylamide) |
— |
Lopinavir |
HIV |
239
|
10. |
Polytyrosine nanoparticles (PTNs) |
Poly(ethylene glycol)-b-poly(L-tyrosine) |
97–181 |
Doxorubicin (DOX) |
Human colorectal cancer treatment |
240
|
5. Conclusion and future perspectives
To date, several drugs have been widely explored for the treatment of life-threatening ailments including COVID-19, cancer, and HIV. Nevertheless, the shortcomings of traditional dosage systems have provided an added impetus and tremendous opportunities to researchers for the engineered development of novel drug delivery vehicles. In this consequence, over the last few years, nanotechnology has opened a new dimension for improved drug delivery by overcoming the transport barriers, intrinsic exclusion, and metabolism-induced problems related to the conventional drugs delivery system. NP-functionalized drug releasing vehicles offer enhanced pharmacokinetics, biocompatibility, pharmacodynamic properties, and stability, which have contributed to the improved prevention, diagnosis, and treatment of these diseases. Most significantly, nanotechnology promotes the targeted delivery of drugs in the infected organs with nominal toxicity or side-effects owing to its acute specificity. Nanostructured architectures including liposomes, dendrimers, inorganic nanoparticles, polymeric micelles, quantum dots, and niosomes provide an absolute platform for scaling up the efficacious therapeutic application, downgrading of drug resistance profiles, and thereafter providing an unprecedented formulation toward catering a wide range of biological applications. Although nanotechnology is a promising candidate for therapeutic applications, however, at times, it induces the general toxicity of organs, reduced stability in biological conditions, and mass scale production, which may plausibly hinder its medicinal applications in the long run. Therefore, further advancement is necessitated in the domain of nanotechnology-mediated advanced drug delivery system by combining newer treatment approaches such as gene therapy and immunotherapy with the existing nanotechnology to maximize the efficiency of targeted drug distribution while minimizing its bottlenecks. The improvement of interactions amidst the physicochemical properties of nanomaterials would provide an inexpensive, safer, and more effectual multifunctional therapeutic derivatives or adjuvants for preliminary diagnosis and longstanding therapeutic actions.
Conflicts of interest
There are no conflicts to declare.
Acknowledgements
Financial support from DST SERB-CRG sponsored project (vide Ref. No. CRG/2022/001679 & Project No. GAP-240712) is hereby acknowledged.
Notes and references
- V. M. O. Cardoso, B. J. Moreira, E. J. Comparetti, I. Sampaio, L. M. B. Ferreira, P. M. P. Lins and V. Zucolotto, Front. Nanotechnol., 2020, 2, 588915 CrossRef.
- A. Sánchez, S. P. Mejía and J. Orozco, Molecules, 2020, 25(16), 3760 CrossRef PubMed.
- M. J. Mitchell, M. M. Billingsley and R. M. Haley, Nat. Rev. Drug Discovery, 2021, 20, 101–124 CrossRef CAS PubMed.
- K. Riehemann, S. W. Schneider, T. A. Luger, B. Godin, M. Ferrari and H. Fuchs, Angew. Chem., Int. Ed., 2009, 48(5), 872–897 CrossRef CAS PubMed.
- D. Chenthamara, S. Subramaniam, S. G. Ramakrishnan, S. Krishnaswamy, M. M. Essa, F. H. Lin and M. W. Qoronfleh, Biomater. Res., 2019, 20, 1–29 Search PubMed.
- B. Wilson and K. M. Geetha, Vaccine, 2022, 40(29), 3931–3941 CrossRef CAS PubMed.
- W. M. Grady, Biochem. Soc. Trans., 2005, 33, 684–688 CrossRef CAS PubMed.
- D. A. Groneberg, K. F. Rabe and A. Fischer, Eur. J. Pharmacol., 2006, 533, 182–194 CrossRef CAS PubMed.
- A. A. Yetisgin, S. Cetinel, M. Zuvin, A. Kosar and O. Kutlu, Molecules, 2020, 25(9), 2193 CrossRef CAS PubMed.
- A. P. Singh, A. Biswas, A. Shukla and P. Mait, Signal Transduction Targeted Ther., 2019, 4, 33 CrossRef PubMed.
- G. Yang, Y. Liu, J. Chen, J. Ding and X. Chen, Acc. Mater. Res., 2022, 3, 1232–1247 CrossRef CAS.
- M. Abd Elkodous, S. O. Olojede, M. Morsi and G. S. ElSayyad, RSC Adv., 2021, 11, 26463–26480 RSC.
- S. Su and P. M. Kang, Pharmaceutics, 2020, 12, 837 CrossRef CAS PubMed.
- A. Biswas, T. Ghosh, P. K. Gavel and A. K. Das, ACS Appl. Bio Mater., 2020, 3(2), 1052–1060 CrossRef CAS PubMed.
- T. Ghosh, A. Biswas, P. K. Gavel and A. Kumar Das, Langmuir, 2020, 36, 1574–1584 CrossRef CAS PubMed.
- A. K. Das and P. K. Gavel, Soft Matter, 2020, 16, 10065–10095 RSC.
- S. Adepu and S. Ramakrishna, Molecules, 2021, 26(19), 5905 CrossRef CAS PubMed.
- L. O. Ouali, M. Noppe, X. Langlois, B. Willems, P. T. Riele, P. Timmerman, M. E. Brewster, A. Arien and V. Preat, J. Controlled Release, 2005, 102(3), 657–668 CrossRef PubMed.
- J. E. Kipp, Int. J. Pharm., 2004, 284(1–2), 109–122 CrossRef CAS PubMed.
- J. Panyam and V. Labhasetwar, Mol. Pharmaceutics, 2004, 1(1), 77–84 CrossRef CAS PubMed.
- C. Fonseca, S. Simoes and R. Gaspar, J. Controlled Release, 2002, 83(2), 273–286 CrossRef CAS PubMed.
- J. M. Koziara, T. R. Whisman, M. T. Tseng and R. J. Mumper, J. Controlled Release, 2006, 112(3), 312–319 CrossRef CAS PubMed.
- H. S. Yoo, K. H. Lee, J. E. Oh and T. G. Park, J. Controlled Release, 2000, 68(3), 419–431 CrossRef CAS PubMed.
- D. Bhadra, S. Bhadra, S. Jain and N. K. Jain, Int. J. Pharm., 2003, 257(1–2), 111–124 CrossRef CAS PubMed.
- J. M. Koziara, P. R. Lockman, D. D. Allen and R. J. Mumper, J. Controlled Release, 2004, 99(2), 259–269 CrossRef CAS PubMed.
- P. Ma and R. J. Mumper, J. Nanomed. Nanotechnol., 2013, 4(2), 1000164 Search PubMed.
- R. Singh and J. W. Lillard, Exp. Mol. Pathol., 2009, 86, 215–223 CrossRef CAS PubMed.
- C. E. M. Huertas, H. Fessi and A. Elaissari, Int. J. Pharm., 2010, 385, 113–142 CrossRef PubMed.
- G. Deutsc, N. C. Phillips and C. M. Tsoukas, Phase-I study of liposomal- azidothymidine (AZT) in advanced HIV disease, Int. Conf. AIDS, 1993, 9, 474 Search PubMed (abstract no. PO-B26 – 2032).
- C. Weiss, M. Carriere, L. Fusco, I. Capua, J. A. R. Nava and M. Pasquali, ACS Nano, 2020, 14, 6383–6406 CrossRef CAS PubMed.
- G. Chauhan, M. J. Madou, S. Kalra, V. Chopra, D. Ghosh and S. O. M. Chapa, ACS Nano, 2020, 14, 7760–7782 CrossRef CAS PubMed.
- D. Lombardo, M. A. Kiselev and M. T. Caccamo, J. Nanomat., 2019, 3702518, DOI:10.1155/2019/3702518.
- C. L. Ventola, Pharmacol. Ther., 2017, 42(12), 742–755 Search PubMed.
- S. Tran, P. J. De Giovanni, B. Piel and P. Rai, Clin. Trans. Med., 2017, 6(1), 44 Search PubMed.
- W. N. Souery and C. J. Bishop, Acta Biomater., 2018, 67, 1–20 CrossRef CAS PubMed.
-
J. Siepmann, R. A. Siegel and M. J. Rathbone, Fundamentals and Applications of Controlled Release Drug Delivery, CRS & Springer, New York, NY, USA, 2012, pp. 255–288 Search PubMed.
- C. Shi, Y. He, M. Ding, Y. Wang and J. Zhong, Trends Food Sci. Technol., 2019, 87, 3–13 CrossRef CAS.
- C. Shi, Y. He, M. Ding, Y. Wang and J. Zhong, Trends Food Sci. Technol., 2019, 87, 14–25 CrossRef CAS.
- In Physics of Amphiphiles: Micelles, Vesicles and Microemulsions, ed. V. Degiorgio and M. Corti, North-Holland, Amsterdam, 1985 Search PubMed.
- F. Masood, Mater. Sci. Eng., C, 2016, 60, 569–578 CrossRef CAS PubMed.
- Y. Y. Won, A. K. Brannan, H. T. Davis and F. S. Bates, J. Phys. Chem. B, 2002, 106(13), 3354–3364 CrossRef CAS.
- A. S. Mikhail and C. Allen, J. Controlled Release, 2009, 138(3), 214–223 CrossRef CAS PubMed.
- M. Elsabahy and K. L. Wooley, Chem. Soc. Rev., 2012, 41(7), 2545–2561 RSC.
- W. Wang, J. Ding, C. Xiao, Z. Tang, D. Li, J. Chen, X. Zhuang and X. Chen, Biomacromolecules, 2011, 12, 2466–2474 CrossRef CAS PubMed.
- F. Danhier, E. Ansorena, J. M. Silva, R. Coco, A. Le Breton and V. Preat, J. Controlled Release, 2012, 161, 505–522 CrossRef CAS PubMed.
- D. Wei, R. Qiao, J. Dao, J. Su, C. Jiang, X. Wang, M. Gao and J. Zhong, Small, 2018, 14(22), e1800063 CrossRef PubMed.
- A. S. Mikhail and C. Allen, J. Controlled Release, 2009, 138(3), 214–223 CrossRef CAS PubMed.
- J. Yin, Y. Chen, Z. H. Zhang and X. Han, Polymers, 2016, 8(7), 268 CrossRef PubMed.
- E. L. Siegler, Y. J. Kim and P. Wang, J. Cell. Immunother., 2016, 2(2), 69–78 CrossRef.
- T. M. Allen and P. R. Cullis, Science, 2004, 303(5665), 1818–1822 CrossRef CAS PubMed.
- X. Ma and Y. Zhao, Chem. Rev., 2015, 115(15), 7794–7839 CrossRef CAS PubMed.
- D. Lombardo, M. A. Kiselev, S. Magazù and P. Calandra, Adv. Condens. Matter Phys., 2015, 151683 Search PubMed.
- D. Landesman-Milo, M. Goldsmith, B. S. Leviatan, B. Witenberg, E. Brown, S. Leibovitch, S. Azriel, S. Tabak, V. Morad and D. Peer, Cancer Lett., 2013, 334, 221–227 CrossRef CAS PubMed.
- T. M. Allen and P. R. Cullis, Adv. Drug Delivery Rev., 2013, 65, 36–48 CrossRef CAS PubMed.
- M. L. Immordino, F. Dosio and L. Cattel, Int. J. Nanomed., 2006, 1(3), 297–315 CrossRef CAS PubMed.
- P. Yingchoncharoen, D. S. Kalinowski and D. R. Richardson, Pharmacol. Rev., 2016, 68, 701–787 CrossRef CAS PubMed.
-
E. Sackmann, in Handbook of Biological Physics 1, ed. R. Lipowsky and E. Sackmann, Elsevier, Amsterdam, 1995, vol. 1, pp. 213–303 Search PubMed.
-
J. Katsaras and T. Gutberlet, Lipid Bilayers. Structure and Interactions, Springer-Verlag, Berlin Heidelberg, 2000 Search PubMed.
- A. Khosa, S. Reddi and R. N. Saha, Biomed. Pharmacother., 2018, 103, 598–613 CrossRef CAS PubMed.
- D. Lombardo, P. Calandra, S. Magazù, U. Wanderlingh, D. Barreca, L. Pasqua and M. A. Kiselev, Colloids Surf., B, 2018, 170, 609–616 CrossRef CAS PubMed.
- D. Lombardo, P. Calandra, D. Barreca, S. Magazù and M. A. Kiselev, Nanomaterials, 2016, 6(7), 125 CrossRef PubMed.
- S. Pogodin, M. Werner, J. U. Sommer and V. A. Baulin, ACS Nano, 2012, 6(12), 10555–10561 CrossRef CAS PubMed.
-
N. Dan, Structure and kinetics of synthetic, lipid-based nucleic acid carriers: Lipoplexes, Lipid Nanocarriers for Drug Targeting, Elsevier, 2018, pp. 529–562 Search PubMed.
- H. C. Villanueva, I. M. Carlin, G. L. Berestein and A. C. Reyes, J. Buon., 2015, 20(6), 1471–1479 Search PubMed.
- Y. Fan, C. Chen, Y. Huang, F. Zhang and G. Lin, Colloids Surf., B, 2017, 151, 19–25 CrossRef CAS PubMed.
- M. Riaz, M. Riaz, X. Zhang, C. Lin, K. H. Wong, X. Chen, G. Zhang, A. Lu and Z. Yang, Int. J. Mol. Sci., 2018, 19(1), 195 CrossRef PubMed.
- M. S. Muthu and S. S. Feng, Expert Opin. Drug Delivery, 2013, 10(2), 151–155 CrossRef CAS PubMed.
- D. A. Tomalia, A. M. Naylor and W. A. Goddard, Angew. Chem., Int. Ed. Engl., 1990, 29(2), 138–175 CrossRef.
- R. Duncan and L. Izzo, Adv. Drug Delivery Rev., 2005, 57(15), 2215–2237 CrossRef CAS PubMed.
- P. Kesharwani, V. Gajbhiye and N. K. Jain, Biomaterials, 2012, 33, 7138–7150 CrossRef CAS PubMed.
- H. Yang and W. J. Kao, J. Biomater. Sci., Polym. Ed., 2006, 17(1–2), 3–19 CrossRef CAS PubMed.
- R. M. Kannan, E. Nance, S. Kannan and D. A. Tomalia, J. Intensive Med., 2014, 276(6), 579–617 CAS.
- D. Astruc, E. Boisselier and C. Ornelas, Chem. Rev., 2010, 110(4), 1857–1959 CrossRef CAS PubMed.
- M. Ballauffand and C. N. Likos, Angew. Chem., Int. Ed., 2004, 43(23), 2998–3020 CrossRef PubMed.
- A. Ramzi, R. Scherrenberg, J. Brackman, J. Joosten and K. Mortensen, Macromolecules, 1998, 31(5), 621–1626 CrossRef.
- J. J. Giner-Casares, M. Henriksen-Lacey, M. Coronado-Puchau and L. M. Liz-Marzán, Mater. Today, 2016, 19(1), 19–28 CrossRef CAS.
- M. Swierczewska, S. Lee and X. Chen, Mol. Imaging, 2011, 10(1), 3–16 CrossRef CAS PubMed.
- A. Baeza, D. Ruiz-Molina and M. Vallet-Regi, Expert Opin. Drug Delivery, 2017, 14, 783–796 CrossRef CAS PubMed.
- J. Conde, J. T. Dias, V. Grazú, M. Moros, P. V. Baptista and J. M. de la Fuente, Front. Chem., 2014, 2, 48 Search PubMed.
- A. Eatemadi, H. Daraee, H. Karimkhanloo, M. Kouhi, N. Zarghami, A. Akbarzadeh, M. Abasi, Y. Hanifehpour and S. W. Joo, Nanoscale Res. Lett., 2014, 9(1), 393 CrossRef PubMed.
- K. H. Son, J. H. Hong and J. W. Lee, Int. J. Nanomed., 2016, 11, 5163–5185 CrossRef CAS PubMed.
- Y. Li Zhao and J. Fraser Stoddart, Acc. Chem. Res., 2009, 42(8), 1161–1171 CrossRef PubMed.
- Z. Chen, A. Zhang, X. Wang, J. Zhu, Y. Fan, H. Yu and Z. Yang, J. Nanomater., 2017, 3418932 Search PubMed.
- C. L. Lay, J. Liu and Y. Liu, Expert Rev. Med. Devices, 2011, 8(5), 561–566 CrossRef CAS PubMed.
- J. Li, S. Q. Yap, C. F. Chin, Q. Tian, S. L. Yoong, G. Pastorin and W. H. Ang, Chem. Sci., 2012, 3(6), 2083–2087 RSC.
- P. Ghosh, G. Han, M. De, C. Kim and V. Rotello, Adv. Drug Delivery Rev., 2008, 60(11), 1307–1315 CrossRef CAS PubMed.
- A. A. Yetisgin, S. Cetinel, M. Zuvin, A. Kosar and O. Kutlu, Molecules, 2020, 25, 2193 CrossRef CAS PubMed.
- R. M. Reilly, J. Nucl. Med., 2007, 48, 1039–1042 CrossRef CAS PubMed.
- C. Fan, W. Gao, Z. Chen, H. Fan, M. Li, F. Deng and Z. Chen, Int. J. Pharm., 2011, 404, 180–190 CrossRef CAS PubMed.
- G. Andocs, H. Renner, L. Balogh, L. Fonyad, C. Jakab and A. Szasz, Strahlenther. Onkol., 2009, 185, 120–126 CrossRef PubMed.
- B. Pandey, N. Shetake, M. S. Balla and A. Kumar, J. Radiat. Cancer Res., 2016, 7, 13 CrossRef.
- F. Y. Kong, J. W. Zhang, R. F. Li, Z. X. Wang, W. J. Wang and W. Wang, Molecules, 2017, 22(9), 1445 CrossRef PubMed.
- E. B. Voura, J. K. Jaiswal, H. Mattoussi and S. M. Simon, Nat. Med., 2004, 10(9), 993–998 CrossRef CAS PubMed.
- T. J. Daou, L. Li, P. Reiss, V. Josserand and I. Texier, Langmuir, 2009, 25(5), 3040–3044 CrossRef CAS PubMed.
- C. E. Probst, P. Zrazhevskiy, V. Bagalkot and X. Gao, Adv. Drug Delivery Rev., 2013, 65(5), 703–718 CrossRef CAS PubMed.
- C. Matea, T. Mocan and F. Tabaran, Int. J. Nanomed., 2017, 12, 5421–5431 CrossRef CAS PubMed.
- D. Vasudevan, R. R. Gaddam, A. Trinchi and I. Cole, J. Alloys Compd., 2015, 636, 395–404 CrossRef CAS.
- J. Yao, P. Li, L. Li and M. Yang, Acta Biomater., 2018, 74, 36–55 CrossRef CAS PubMed.
- F. Chen, G. Hableel, E. R. Zhao and J. V. Jokerst, J. Colloid Interface Sci., 2018, 521, 261–279 CrossRef CAS PubMed.
- H. Lu, J. Wang, T. Wang, J. Zhong, Y. Bao and H. Hao, J. Nanomater., 2016, 5762431 Search PubMed.
- F. Dilnawaz, Curr. Med. Chem., 2019, 26(31), 5745–5763 CrossRef CAS PubMed.
- D. J. Bharali, I. Klejbor, E. K. Stachowiak, P. Dutta, I. Roy, N. Kaur, E. J. Bergey, P. N. Prasad and M. K. Stachowiak, Proc. Natl. Acad. Sci. U. S. A., 2005, 102(32), 11539–11544 CrossRef CAS PubMed.
- F. Dilnawaz, Curr. Med. Chem., 2019, 26(31), 5745–5763 CrossRef CAS PubMed.
- L. Pasqua, A. Leggio, D. Sisci, S. Andò and C. Morelli, Mini-Rev. Med. Chem., 2016, 16(9), 743–753 CrossRef CAS PubMed.
- S. Mura, J. Nicolas and P. Couvreur, Nat. Mater., 2013, 12, 991–1003 CrossRef CAS PubMed.
- E. Bagheri, L. Ansari, K. Abnous, S. M. Taghdisi, F. Charbgoo, M. Ramezani and M. Alibolandi, J. Controlled Release, 2018, 277, 57–76 CrossRef CAS PubMed.
- Z. Li, N. Song and Y. W. Yang, Matter., 2019, 1, 345–368 CrossRef.
- N. Song, X. Y. Lou, L. Ma, H. Gao and Y. W. Yang, Theranostics, 2019, 9, 3075–3093 CrossRef CAS PubMed.
- D. Wei, Y. Yu, X. Zhang, Y. Wang, H. Chen, Y. Zhao, F. Wang, G. Rong, W. Wang, X. Kang, J. Cai, Z. Wang, J. Y. Yin, M. Hanif, Y. Sun, G. Zha, L. Li, G. Nie and H. Xiao, ACS Nano, 2020, 14, 16984–16996 CrossRef CAS PubMed.
- Z. H. Zhou, S. Y. Liang, T. C. Zhao, X. Z. Chen, X. K. Cao, M. Qi, Y. Y. Huang, W. T. Ju, M. Yang, D. W. Zhu, Y. C. Pang and L. P. Zhong, J. Nanobiotechnol., 2021, 19, 157 CrossRef CAS PubMed.
- L. Jin, S. Shen, Y. Huang, D. Li and X. Yang, Biomaterials, 2021, 268, 120582 CrossRef CAS PubMed.
- R. R. Adhikary, P. More and R. Banerjee, Nanoscale, 2015, 7, 7520–7534 RSC.
- M. E. Davis, Z. G. Chen and D. M. Shin, Nat. Rev. Drug Discovery, 2008, 7, 771–782 CrossRef CAS PubMed.
- M. Rai, S. Bonde, A. Yadav, A. Bhowmik, S. Rathod, P. Ingle and A. Gade, Viruses, 2021, 13(17), 1224 CrossRef CAS PubMed.
- C. Menni, A. M. Valdes, M. B. Freidin, C. H. Sudre, L. H. Nguyen, D. A. Drew, S. Ganesh, T. Varsavsky, M. J. Cardoso and J. S. E.-S. Moustafa, Nat. Med., 2020, 26, 1037–1040 CrossRef CAS PubMed.
- D. Lembo, M. Donalisio, A. Civra, M. Argenziano and R. Cavalli, Expert Opin. Drug Delivery, 2018, 15, 93–114 CrossRef CAS PubMed.
- V. S. Sivasankarapillai, A. M. Pillai, A. Rahdar, A. P. Sobha, S. S. Das, A. C. Mitropoulos, M. H. Mokarrar and G. Z. Kyzas, Nanomaterials, 2020, 10, 852 CrossRef CAS PubMed.
- E. V. Campos, A. E. Pereira, J. L. de Oliveira, L. B. Carvalho, M. G. Casagrande, R. de Lima and L. F. Fraceto, J. Nanobiotechnol., 2020, 18, 1–23 CrossRef PubMed.
- R. Nakashima, M. Kawamoto, S. Miyazaki, R. Onishi, K. Furusaki and M. Osaki, J. Vet. Med. Sci., 2017, 79, 939–942 CrossRef CAS PubMed.
- A. Sakudo, R. Yamashiro, M. Haritani, K. Furusaki, R. Onishi and T. Onodera, Int. J. Nanomed., 2020, 15, 1387–1395 CrossRef CAS PubMed.
- C. Weiss, M. Carriere, L. Fusco, I. Capua, J. A. Regla-Nava and M. Pasquali, ACS Nano, 2020, 14, 6383–6406 CrossRef CAS PubMed.
- F. Murphy, A. Tchetchik and I. Furxhi, Nanomaterials, 2020, 10, 999 CrossRef CAS PubMed.
- J. M. Boyce, Antimicrob. Resist. Infect. Control., 2016, 5, 1–10 CrossRef PubMed.
-
M. S. Joshi, 2020, Researchers Come Eco-Friendly and Non-Toxic Handwash. Available online at: https://www.sakaltimes.com/pune/researchers-come-ecofriendly-and-non-toxic-handwash-50456 Search PubMed.
- M. W. Wang, M. Y. Zhou, G. H. Ji, L. Ye, Y. R. Cheng, Z. H. Feng and J. Chen, Eur. Rev. Med. Pharmacol. Sci., 2020, 24, 3397–3399 Search PubMed.
- Y. M. Bar-On, A. Flamholz, R. Phillips and R. Milo, eLife, 2020, 9, e57309 CrossRef PubMed.
- Y. Si, Z. Zhang, W. Wu, Q. Fu, K. Huang and N. Nitin, Sci. Adv., 2018, 4, eaar5931 CrossRef PubMed.
- Y. Imai, K. Kuba, S. Rao, Y. Huan, F. Guo, B. Guan, P. Yang, R. Sarao, T. Wada and H. Leong-Poi, Nature, 2005, 436, 112–116 CrossRef CAS PubMed.
- D. Aydemir, F. Gecili, N. Özdemir and N. Nuray Ulusu, J. Biosci. Bioeng., 2020, 129, 679–686 CrossRef CAS PubMed.
- R. Kumar, S. Nagpal, S. Kaushik and S. Mendiratta, VirusDisease, 2020, 31, 1–9 CrossRef PubMed.
- Y. Gong, J. Hu, J. R. Choi, M. You, Y. Zheng, B. Xu, T. Wen and F. Xu, Int. J. Nanomed., 2017, 12, 4455–4466 CrossRef CAS PubMed.
- B. Udugama, P. Kadhiresan, H. N. Kozlowski, A. Malekjahani, M. Osborne, V. Y. Li, H. Chen, S. Mubareka, J. B. Gubbay and W. C. Chan, ACS Nano, 2020, 14, 3822–3835 CrossRef CAS PubMed.
- E. Tuaillon, K. Bolloré, A. Pisoni, S. Debiesse, C. Renault, S. Marie, S. Groc, C. Niels, N. Pansu and A. M. Dupuy, J. Infect., 2020, 81, e39–e45 CrossRef CAS PubMed.
- C. Huang, Y. Wang, X. Li, I. Ren, J. Zhao and Y. Hu, Lancet, 2020, 395, 497–506 CrossRef CAS PubMed.
- L. C. Brazaca, J. R. Moreto, A. Martín, F. Tehrani, J. Wang and V. Zucolotto, ACS Nano, 2019, 13, 13325–13332 CrossRef CAS PubMed.
- K. M. Koczula and A. Gallotta, Biochem., 2016, 60, 111–120 Search PubMed.
- C. Sheridan, Nat. Biotechnol., 2020, 38, 515–518 CrossRef CAS PubMed.
- T. N. N. Dau, V. H. Vu, T. T. Cao, V. C. Nguyen, C. T. Ly and D. L. Tran, Sens. Actuators, B, 2019, 283, 52–60 CrossRef CAS.
- V. T. Tran, J. Kim, L. T. Tufa, S. Oh, J. Kwon and J. Lee, Anal. Chem., 2018, 90, 225–239 CrossRef CAS PubMed.
- M. T. Glynn, D. J. Kinahan and J. Ducrée, Lab Chip, 2014, 14, 2844–2851 RSC.
- S. Somvanshi, B. P. Kharat, S. T. Saraf, S. Somwanshi, S. Shejul and K. Jadhav, Mater. Res. Innovations, 2020, 25, 169–174 CrossRef.
- H. Zhu, Z. Fohlerová, J. Pekárek, E. Basova and P. NeuZil, Biosens. Bioelectron., 2020, 153, 112041 CrossRef CAS PubMed.
- D. Peer, J. M. Karp, S. Jong, O. C. Farokhzad, R. Margalit and R. Langer, Nat. Nanotechnol., 2007, 2, 751–760 CrossRef CAS PubMed.
- H. Ashiba, Y. Sugiyama, X. Wang, H. Shirato, K. H. Moriguchi, K. Taniguchi, Y. Ohki and M. Fujimaki, Biosens. Bioelectron., 2017, 93, 260–266 CrossRef CAS PubMed.
- C. Roh and S. K. Jo, J. Chem. Technol. Biotechnol., 2011, 86, 1475–1479 CrossRef CAS PubMed.
- S. L. Liu, Z. G. Wang, H. Y. Xie, A. A. Liu, D. C. Lamb and D. W. Pang, Chem. Rev., 2020, 120, 1936–1979 CrossRef CAS PubMed.
- V. Georgakilas, J. A. Perman, J. Tucek and R. Zboril, Chem. Rev., 2015, 115, 4744–4822 CrossRef CAS PubMed.
- R. L. Pinals, F. Ledesma, D. Yang, N. Navarro, S. Jeong, J. E. Pak, L. Kuo, Y. C. Chuang, Y. W. Cheng and H. Y. Sun, Nano Lett., 2021, 21, 2272–2280 CrossRef CAS PubMed.
- G. Seo, G. Lee, M. J. Kim, S. H. Baek, M. Choi and K. M. Ku, ACS Nano, 2020, 14, 5135–5142 CrossRef CAS PubMed.
- D. Jiang, D. Ni, Z. T. Rosenkrans, P. Huang, X. Yan and W. Cai, Chem. Soc. Rev., 2019, 48, 3683–3704 RSC.
- Q. Wang, H. Wei, Z. Zhang, E. Wang and S. Dong, TrAC, Trends Anal. Chem., 2018, 105, 218–224 CrossRef CAS.
- H. Wei and E. Wang, Chem. Soc. Rev., 2013, 42, 6060–6093 RSC.
- D. Liu, C. Ju, C. Han, R. Shi, X. Chen, D. Duan, J. Yan and X. Yan, Biosens. Bioelectron., 2021, 173, 112817 CrossRef CAS PubMed.
- B. Flühmann, I. Ntai, G. Borchard, S. Simoens and S. Mühlebach, Eur. J. Pharm. Sci., 2019, 128, 73–80 CrossRef PubMed.
- R. M. Mainardes and C. Diedrich, Ther. Delivery, 2020, 11, 411–414 CrossRef CAS PubMed.
- R. G. Kerry, S. Malik, Y. T. Redda, S. Sahoo, J. K. Patra and S. Majhi, Nanomedicine, 2019, 18, 196–220 CrossRef CAS PubMed.
-
Z. Zhao, H. Cui, W. Song, X. Ru, W. Zhou and X. Yu, 2020, preprint DOI:10.1101/2020.02.22.961268.
- T. E. GartnerIII and A. Jayaraman, Macromolecules, 2019, 52, 755–786 CrossRef.
-
S. E. McNeil, Unique benefits of nanotechnology to drug delivery and diagnostics, in Characterization of Nanoparticles Intended for Drug Delivery, Methods in Molecular Biology, Springer, New York, 2011, vol. 697, pp. 3–8 Search PubMed.
- R. A. Petros and J. M. DeSimone, Nat. Rev. Drug Discovery, 2010, 9, 615–627 CrossRef CAS PubMed.
- S. Galdiero, A. Falanga, M. Vitiello, M. Cantisani, V. Marra and M. Galdiero, Molecules, 2011, 16, 8894–8918 CrossRef CAS PubMed.
- X. Yang, M. Yang, B. Pang, M. Vara and Y. Xia, Chem. Rev., 2015, 115, 10410–10488 CrossRef CAS PubMed.
- V. Cagno, P. Andreozzi, M. D’Alicarnasso, P. J. Silva, M. Mueller and M. Galloux, Nat. Mater., 2018, 17, 195–203 CrossRef CAS PubMed.
- S. S. Jeremiah, K. Miyakawa, T. Morita, Y. Yamaoka and A. Ryo, Biochem. Biophys. Res. Commun., 2020, 533, 195–200 CrossRef CAS PubMed.
- G. Behbudi, Effect of Silver Nanoparticles Disinfectant on COVID-19, Adv. Appl. NanoBioTechnol., 2021, 2, 63–67 CAS.
- K. Williams, J. Milner, M. D. Boudreau, K. Gokulan, C. E. Cerniglia and S. Khare, Nanotoxicology, 2015, 9, 279–289 CrossRef CAS PubMed.
- Y. Abo-zeid and G. R. Williams, Wiley Interdiscip. Rev.: Nanomed. Nanobiotechnol., 2020, 12, e1592 Search PubMed.
- H. Ghaffari, A. Tavakoli, A. Moradi, A. Tabarraei, F. Bokharaei-Salim, M. Zahmatkeshan, M. Farahmand, D. Javanmard, S. J. Kiani and M. Esghaei, J. Biomed. Sci., 2019, 26, 70 CrossRef PubMed.
- G. I. Fouad, Bull. Natl. Res. Cent., 2021, 45, 1–22 CrossRef.
- S. Alidori, R. L. Bowman, D. Yarilin, Y. Romin, A. Barlas and J. J. Mulvey, Nat. Commun., 2016, 7, 12343 CrossRef CAS PubMed.
- P. Sanpui, X. Zheng, J. C. Loeb, J. H. Bisesi Jr, I. A. Khan and A. R. M. N. Afrooz, Fibre Toxicol., 2014, 11, 66 CrossRef PubMed.
- A. Z. Stein, G. Wang and M. A. Fierke, Adv. Mater., 2009, 21, 265 CrossRef CAS.
- Y. N. Chen, Y. H. Hsueh, C. T. Hsieh, D. Y. Tzou and P. L. Chang, Int. J. Environ. Res., Public Health, 2016, 13, 430 CrossRef PubMed.
- A. Loczechin, K. Séron, A. Barras, E. Giovanelli, S. Belouzard, Y. T. Chen, N. Metzler-Nolte, R. Boukherroub, J. Dubuisson and S. Szunerits, Appl. Mater. Interfaces, 2019, 11, 42964–42974 CrossRef CAS PubMed.
- M. Nasrollahzadeh, M. Sajjadi, G. J. Soufi, S. Iravani and R. S. Varma, Nanomaterials, 2020, 10, 1072 CrossRef CAS PubMed.
- D. Ting, N. Dong, L. Fang, J. Lu, J. Bi, S. Xiao and H. Han, ACS Appl. Nano Mater., 2018, 1, 5451–5459 CrossRef.
- M. Chidambaram, R. Manavalan and K. Kathiresan, J. Pharm. Pharm. Sci., 2011, 14, 67–77 Search PubMed.
- A. A. Stavrovskaya, Biochemistry, 2000, 65, 95–106 CAS.
- B. Mishra, B. B. Patel and S. Tiwari, Nanomedicine, 2010, 6(1), 9–24 CrossRef CAS PubMed.
- F. Danhier, O. Feron and V. Preat, J. Controlled Release, 2010, 148, 135–146 CrossRef CAS PubMed.
- H. Fu, Y. Hu, S. Shi, S. Ren, W. Liu and S. Su, Analyst, 2018, 143, 1705–1712 RSC.
- C. Zylberberg and S. Matosevic, Drug Delivery, 2016, 23, 3319–3329 CrossRef CAS PubMed.
- Y. Malam, M. Loizidou and A. M. Seifalian, Trends Pharmacol. Sci., 2009, 30, 592–599 CrossRef CAS PubMed.
- M. L. Immordino, F. Dosio and L. Cattel, Int. J. Nanomed., 2006, 1, 297–315 CrossRef CAS PubMed.
- J. L. Berger, A. Smith, K. K. Zorn, P. Sukumvanich, A. B. Olawaiye and J. Kelley, OncoTargets Ther., 2014, 7, 1409–1413 CAS.
- H. Chou, H. Lin and J. M. Liu, OncoTargets Ther., 2015, 8, 1719–1720 Search PubMed.
- H. Yari, G. Nkepang and V. Awasthi, Materials, 2019, 12, 756 CrossRef CAS PubMed.
- X. Tang, A. Li, C. Xie, Y. Zhang, X. Liu and Y. Xie, Nanoscale Res. Lett., 2020, 15, 63 CrossRef CAS PubMed.
- J. Meng, F. Guo, H. Xu, W. Liang, C. Wang and X. D. Yang, Sci. Rep., 2016, 6, 22390 CrossRef CAS PubMed.
- G. Han, P. Ghosh and V. M. Rotello, Nanomedicine, 2007, 2, 113–123 CrossRef CAS PubMed.
- J. Cheng, Y. J. Gu, S. H. Cheng and W. T. Wong, J. Biomed. Nanotechnol., 2013, 9, 1362–1369 CrossRef CAS PubMed.
- Y. Jiang, S. Huo, J. Hardie, X. J. Liang and V. M. Rotello, Expert Opin. Drug Delivery, 2016, 13, 547–559 CrossRef CAS PubMed.
- R. S. Riley and E. S. Day, Wiley Interdiscip. Rev.: Nanomed. Nanobiotechnol., 2017, 9, e1449 Search PubMed.
- A. Bianco, K. Kostarelos and M. Prato, Curr. Opin. Chem. Biol., 2005, 9, 674–679 CrossRef CAS PubMed.
- N. W. Kam, M. O’Connell, J. A. Wisdom and H. Dai, Proc. Natl. Acad. Sci. U. S. A., 2005, 102, 11600–11605 CrossRef CAS PubMed.
- A. Burlaka, S. Lukin, S. Prylutska, O. Remeniak, Y. Prylutskyy and M. Shuba, Exp. Oncol., 2010, 32, 48–50 CAS.
- X. Yu, D. Gao, L. Gao, J. Lai, C. Zhang and Y. Zhao, ACS Nano, 2017, 11, 10147–10158 CrossRef CAS PubMed.
- K. R. Karnati and Y. Wang, Phys. Chem. Chem. Phys., 2018, 20, 9389–9400 RSC.
- S. Svenson and D. A. Tomalia, Adv. Drug Delivery Rev., 2005, 57, 2106–2129 CrossRef CAS PubMed.
- L. Palmerston Mendes, J. Pan and V. P. Torchilin, Molecules, 2017, 22(9), 1401 CrossRef PubMed.
- A. P. Sherje, M. Jadhav, B. R. Dravyakar and D. Kadam, Int. J. Pharm., 2018, 548, 707–720 CrossRef CAS PubMed.
- R. Roy and M. G. Baek, J. Biotechnol., 2002, 90, 291–309 CAS.
- P. Couvreur, Crit. Rev. Ther. Drug Carrier Syst., 1988, 5, 1–20 CAS.
- E. Blanco, E. A. Bey, C. Khemtong, S. G. Yang, J. Setti-Guthi and H. Chen, Cancer Res., 2010, 70, 3896–3904 CrossRef CAS PubMed.
- M. E. Davis, Z. G. Chen and D. M. Shin, Nat. Rev. Drug Discovery, 2008, 7, 771–782 CrossRef CAS PubMed.
- G. Sharma, S. Anabousi, C. Ehrhardt and M. N. Ravi Kumar, J. Drug Targeting, 2006, 14, 301–310 CrossRef CAS PubMed.
- A. Shiohara, A. Hoshino, K. Hanaki, K. Suzuki and K. Yamamoto, Microbiol. Immunol., 2004, 48, 669–675 CrossRef CAS PubMed.
- Q. M. Xiang, L. W. Wang, J. P. Yuan, J. M. Chen, F. Yang and Y. Li, Exp. Mol. Pathol., 2015, 99, 133–138 CrossRef CAS PubMed.
- I. L. Medintz, H. T. Uyeda, E. R. Goldman and H. Mattoussi, Nat. Mater., 2005, 4, 435–446 CrossRef CAS PubMed.
- S. Y. Sung, Y. L. Su, W. Cheng, P. F. Hu, C. S. Chiang and W. T. Chen, Nano Lett., 2019, 19, 69–81 CrossRef CAS PubMed.
- C. Chen, J. Peng, H. S. Xia, G. F. Yang, Q. S. Wu and L. D. Chen, Biomaterials, 2009, 30, 2912–2918 CrossRef CAS PubMed.
- T. W. Baba, V. Liska, R. Hofmann-Lehmann, J. Vlasak, W. Xu, S. Ayehunie, L. A. Cavacini, M. R. Posner, H. Katinger, G. Stiegler, B. J. Bernacky, T. A. Rizvi, R. Schmidt, L. R. Hill, M. E. Keeling, Y. Lu, J. E. Wright, T. C. Chou and R. M. Ruprecht, Nat. Med., 2000, 6, 200–206 CrossRef CAS PubMed.
- Y. Liu and C. Chen, Adv. Drug Delivery Rev., 2016, 103, 76–89 CrossRef CAS PubMed.
- M. Rai, A. P. Ingle, S. Birla, A. Yadav and C. A. Santos, Cri. Rev. Microbiol., 2015, 1–24 Search PubMed.
- L. Xu, Y. Liu, Z. Chen, W. Li, L. Wang, X. Wu, Y. Ji, Y. Zhao, L. Ma, Y. Shao and C. Chen, Nano Lett., 2012, 12, 2003–2012 CrossRef CAS PubMed.
- U. Gupta and N. K. Jain, Adv. Drug Delivery Rev., 2010, 62, 478–490 CrossRef CAS PubMed.
- L. Dykman and N. Khlebtsov, Chem. Soc. Rev., 2012, 41, 2256–2282 RSC.
- S. D. Mahajan, R. Aalinkeel, W. C. Law, J. L. Reynolds, B. B. Nair, D. E. Sykes, K. T. Yong, I. Roy, P. N. Prasad and S. A. Schwartz, Int. J. Nanomed., 2012, 7, 5301–5314 CrossRef CAS PubMed.
- A. Bolhassani, S. Javanzad, T. Saleh, M. Hashemi, M. R. Aghasadeghi and S. M. Sadat, Hum. Vacc. Immunother., 2014, 10, 321–332 CrossRef CAS PubMed.
- Y. J. Qiao, Z. J. Zhai, L. M. Chen and H. Liu, Sci. Bull., 2015, 60, 1193–1202 CrossRef CAS.
- S. Boyapalle, S. Mohapatra and S. Mohapatra, J. Global Infect. Dis., 2012, 4, 62–68 CrossRef PubMed.
- K. Klein, J. F. Mann, P. Rogers and R. J. Shattock, J. Controlled Release, 2014, 183, 43–50 CrossRef CAS PubMed.
- X. Zhou, B. Liu, X. Yu, X. Zha, X. Zhang, X. Wang, Y. Jin, Y. Wu, Y. Chen, Y. Shan, Y. Chen, J. Liu, W. Kong and J. Shen, Eur. J. Pharm. Biopharm., 2008, 68, 589–595 CrossRef CAS PubMed.
-
I. Sailaja, M. K. Baghel and I. Shaker, Nanotechnology Based Drug Delivery for HIV-AIDS Treatment, 2021 DOI:10.5772/intechopen.97736.
- T. Bui, T. Dykers, S. L. Hu, C. R. Faltynek and R. J. Ho, J. Acquired Immune Defic. Syndr., 1994, 7, 799–806 CAS.
- N. C. Phillips, L. Gagne, N. Ivanoff and G. Riveau, Vaccine, 1996, 14, 898–904 CrossRef CAS PubMed.
- K. S. Korsholm, J. Hansen, K. Karlsen, J. Filskov, M. Mikkelsen, T. Lindenstrom, S. T. Schmidt, P. Andersen and D. Christensen, Vaccine, 2014, 32, 3927–3935 CrossRef CAS PubMed.
- F. Aline, D. Brand, J. Pierre, P. Roingeard, M. Severine, B. Verrier and I. Dimier-Poisson, Vaccine, 2009, 27, 5284–5291 CrossRef CAS PubMed.
- C. S. Dezzutti, V. N. James, A. Ramos, S. T. Sullivan, A. Siddig, T. J. Bush and C. E. Hart, Antimicrob. Agents Chemother., 2004, 48(10), 3834–3844 CrossRef CAS PubMed.
- L. Kumar, S. Verma, D. N. Prasad, A. Bhardwaj, B. Vaidya and A. K. Jain, Artif. Cells, Nanomed., Biotechnol., 2014, 1–16, DOI:10.3109/21691401.2014.883400 , ISSN: 2169-1401 print/2169-141X.
- D. Gopinath, D. Ravi, R. Karwa, B. R. Rao, A. Shashank and D. Rambhau, Arzneimittelforschung, 2001, 51(11), 924–930 CAS.
- W. Song, P. Jia, T. Zhang, K. Dou, L. Liu, Y. Ren, F. Liu, J. Xue, M. S. Hasanin, H. Qi and Q. Zhou, J. Nanobiotechnol., 2022, 20, 289 CrossRef CAS PubMed.
- M.
P. Silva, G. Chauhan, M. D. Shin, C. Hoskins, M. J. Madou, S. O. M. Chapa, N. F. Steinmetz, F. Veiga and A. C. P. Santos, Expert Opin. Drug Delivery, 2021, 1–20 Search PubMed.
- Q. Zhang, W. Wei, P. Wang, L. Zuo, F. Li, J. Xu, X. Xi, X. Gao, G. Ma and H. Xie, ACS Nano, 2017, 11, 10724–10732 CrossRef CAS PubMed.
- F. H. Chen, L. M. Zhang, Q. T. Chen, Y. Zhang and Z. J. Zhang, Chem. Commun., 2010, 46, 8633–8635 RSC.
- Y. Dang and J. Guan, Smart Mater. Med., 2020, 1, 10–19 CrossRef PubMed.
- X. L. Guo, X. X. Kang, Y. Q. Wang, X. J. Zhang, C. J. Li, Y. Liu and L. B. Du, Acta Biomater., 2019, 84, 367–377 CrossRef CAS PubMed.
- Y. Li, Y. Song, W. Zhang, J. Xu, J. Hou, X. Feng and W. Zhu, J. Mater. Chem. B, 2020, 8, 7382–7389 RSC.
- A. R. Town, J. Taylor, K. Dawson, E. Niezabitowska, N. M. Elbaz and A. Corker, J. Mater. Chem. B, 2019, 7, 373–383 RSC.
- X. Gu, M. Qiu, H. Sun, J. Zhang, L. Cheng, C. Deng and Z. Zhong, Biomater. Sci., 2018, 6, 1526–1534 RSC.
|
This journal is © The Royal Society of Chemistry 2023 |