DOI:
10.1039/D2BM02059A
(Review Article)
Biomater. Sci., 2023,
11, 3034-3050
Engineering optical tools for remotely controlled brain stimulation and regeneration
Received
14th December 2022
, Accepted 28th February 2023
First published on 18th March 2023
Abstract
Neurological disorders are one of the world's leading medical and societal challenges due to the lack of efficacy of the first line treatment. Although pharmacological and non-pharmacological interventions have been employed with the aim of regulating neuronal activity and survival, they have failed to avoid symptom relapse and disease progression in the vast majority of patients. In the last 5 years, advanced drug delivery systems delivering bioactive molecules and neuromodulation strategies have been developed to promote tissue regeneration and remodel neuronal circuitry. However, both approaches still have limited spatial and temporal precision over the desired target regions. While external stimuli such as electromagnetic fields and ultrasound have been employed in the clinic for non-invasive neuromodulation, they do not have the capability of offering single-cell spatial resolution as light stimulation. Herein, we review the latest progress in this area of study and discuss the prospects of using light-responsive nanomaterials to achieve on-demand delivery of drugs and neuromodulation, with the aim of achieving brain stimulation and regeneration.
1. Introduction
Neurological disorders are one of the world's leading medical and societal challenges, affecting approximately 2.6 billion people worldwide.1 Diseases such as stroke, epilepsy, dementia, Alzheimer's disease (AD), and Parkinson's disease (PD) are characterised by cognitive decline and/or motor impairment, as a result of accelerated neurodegeneration compared to physiological ageing in selective brain regions and cell types.2 The first line treatment includes the administration of medicines targeting ion channels or modulating neurotransmitter levels to regulate neuronal activity, as well as anti-inflammatory drugs and growth factors conferring neuroprotection.3,4 However, these treatments have failed to avoid symptom relapse in the vast majority of patients, due to progressive neuroinflammation and tissue damage which ultimately result in poor quality of life and eventually death.
Stem cells have been considered a promising solution to restore brain function by regenerating damaged tissues. They are capable of proliferating and differentiating into multiple cell types including neurons, astrocytes, and oligodendrocytes.5 Although stem cells derived from foetal (e.g. umbilical cord, placenta) and adult tissues (e.g. bone marrow, adipose tissue) have been employed in patients suffering from stroke or PD,6–10 cell transplantation has shown limited clinical efficacy. This can be attributed to the low engraftment efficiency due to a pro-inflammatory tissue microenvironment, hampering cell survival and homing at the implanted site. An alternative strategy consists of targeting mature cells in the brain to become pluripotent stem cells through the overexpression of transcription factors.11 However, prolonged expression of these factors may also induce tumorigenesis, thus requiring delivery strategies that ensure a transient and safe action. In order to surmount these caveats, a more recent avenue of research consists of stimulating the proliferation of endogenous neural stem cells (NSCs) and endothelial progenitor cells (EPCs) which further mediate repair mechanisms in the brain (e.g. via neurogenesis, synaptogenesis, and angiogenesis).12–14 However, NSCs are located in niche reservoirs deep in the adult brain, such as the subventricular zone (SVZ) adjacent to the lateral ventricles, or the dentate gyrus (DG) in the hippocampus.15 Moreover, these regions lose neuronal plasticity with ageing, thus becoming strongly susceptible to neurodegeneration particularly in pathological processes such as AD.16–18 Therefore, pharmacological approaches stimulating neurogenesis in these target regions could potentially restore brain function in neurodegenerative diseases and age-related impairments.
Given the lack of therapeutic approaches promoting brain regeneration in the clinic, advanced drug delivery systems based on synthetic nanoparticle (NP)-based formulations have been developed for the delivery of bioactive molecules (e.g. retinoic acid, microRNAs, transcription factors, etc.), in order to modulate the proliferation and differentiation of brain endogenous stem cells (Fig. 1).5,12–14 Despite the promising results in preclinical models, some of these formulations tend to diffuse across the brain even after surgical delivery to the site of interest by intracerebral administration, which may lead to drug diffusion to distant locations.19 Thus, formulations developed with the capacity to release the drugs under tight spatial control, potentially able to modulate specific cell populations of interest, are in high demand. In addition, in case the formulations are administered by systemic administration to promote their clinical application, the blood–brain barrier (BBB) also poses a significant obstacle for targeted delivery to the brain parenchyma. Several strategies have been developed to target the BBB,20 including surface functionalisation of NPs with biomolecules (e.g. peptides derived from lactoferrin, transferrin, or low-density lipoprotein receptors) which promote transcytosis through endothelial cells. Nevertheless, this strategy has shown limited efficacy. More recently, NPs stimulated by external stimuli (e.g. ultrasounds, magnetic fields, light) have been employed as transducers with the aim of destabilizing the BBB, in order to increase its permeability which allows for drug accumulation in the specific regions of the brain.21–23
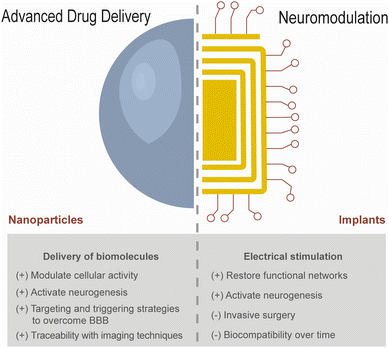 |
| Fig. 1 Applications of nanomaterials for brain regeneration and stimulation. Nanomaterials have been investigated as advanced drug delivery carriers and incorporated into biointerfaces for neuromodulation. The advantages and limitations of these strategies are outlined with respect to their application in the targeted delivery of biomolecules and neural circuitry remodelling, in order to promote brain regeneration. | |
Restoring brain function involves not only tissue regeneration through the modulation of proliferation and differentiation mechanisms of brain endogenous stem cells, but also brain stimulation involving the activation of these cells. Neuromodulation has been employed for more than 30 years with the aim of restructuring functional networks in order to circumvent neurological impairment (Fig. 1).24 This was originally achieved by surgical implantation of devices delivering electrical pulses in order to activate voltage-sensitive ion channels in neurons, which in turn generate electrochemical gradients and induce the release of neurotransmitters. Electrical stimulation can also manipulate the transmembrane resting potential, which may be linked to cell proliferation and differentiation.25 Interestingly, PD patients subjected to deep brain stimulation (DBS) targeting the subthalamic nucleus exhibited signs of neurogenesis in the neighbouring SVZ.26 This could be ascribed to the activation of functional neurons which constitute new connections with other cells surrounding the damaged tissue.27 These findings suggest that the electrical stimulation of NSCs can be a promising strategy to complement pharmacological interventions aiming to regenerate damaged brain tissue. However, there is limited clinical evidence because DBS is restricted to patients with advanced disease progression when pharmacological treatments have failed.28 The majority of 160
000 patients undergoing DBS worldwide have experienced significant and frequent adverse effects related to the highly invasive surgical procedure to implant a device in the brain, to which a foreign body response is developed by the host.29–32 In addition to biocompatibility issues affecting long-term performance, inadequate device programming and poor spatial targeting may demand multiple surgeries to adjust the position or to replace the device or any of its components.24 Electrical stimulation warrants a greater specificity over specific brain locations, in order to avoid overstimulating undesired areas bearing a wide diversity of cells, ranging from neurons to endothelial, glial, and immune cells.28
In this review, we discuss the use of external stimuli to address the aforementioned limitations of drug delivery and electrical stimulation by targeting specific brain regions. While ultrasound and electromagnetic fields have been employed in the clinic for non-invasive transcranial neuromodulation, we propose the application of light owing to its spatiotemporal resolution, targeting precise regions down to single cells (Fig. 2).33,34 Although light has been employed in diverse applications in biomedicine,35 there are some considerations regarding its application in the brain. Biological tissue contains a variety of molecules capable of absorbing light and significantly attenuating its penetration depth. This results in the application of high irradiance (i.e. radiation power) which is usually accompanied by heat generation that may be deleterious for cell survival. Because the brain is highly sensitive to temperature variations, heat generation must be limited; whilst an increase of 1 °C can induce functional alterations, exposure to 42 °C for 5 min may be sufficient to irreversibly inactivate neurons.36 These limitations have motivated the development of nanomaterials capable of transducing optical signals in order to improve the safety and efficacy of light. Herein, we will cover the recent progress related to stimuli-responsive drug delivery systems and how the use of light compares with other external stimuli. In addition, we will discuss the critical parameters regarding the use of minimally invasive approaches for targeted neuromodulation, including optogenetics and non-genetic alternatives applying nanoformulations in medical devices capable of converting light to electricity with unprecedented precision.
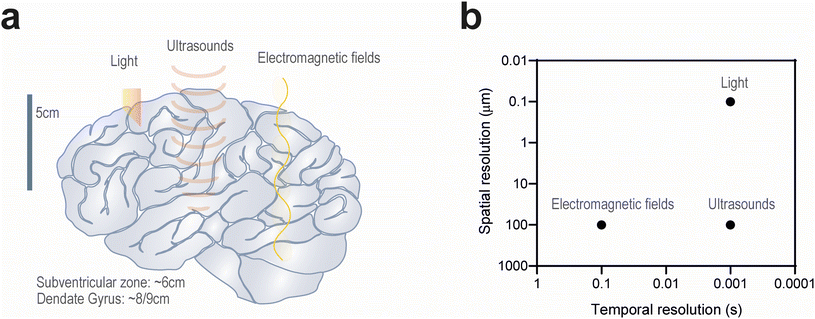 |
| Fig. 2 Comparison of external stimuli employed in neuromodulation and targeted drug delivery. (a) Schematic representation of the brain highlighting the penetration depth of transcranial stimulation modalities. Regions such as the SVZ and dentate gyrus are attractive targets to promote neurogenesis. (b) Light enables 1000× more precise spatial resolution compared to other external stimuli such as ultrasounds and electromagnetic fields. In addition, it can improve temporal resolution by 100-fold compared to clinically used electrical stimulation. | |
2. Using external stimuli to control brain stimulation and regeneration
Recent technological advances have aimed to reduce the invasiveness of neuromodulation by improving device biocompatibility and refining actuation on the brain using external triggers.37 While non-invasive transcranial electrical stimulation has been explored in the clinic since the 1980s, other external stimuli such as magnetic fields and ultrasounds have also been employed to elicit neurotransmitter release from neurons.38,39 A common mechanism shared by these stimuli consists of heat generation which activates transmembrane ion channels. These stimuli can also be exploited to trigger cargo release from advanced drug delivery systems. In both cases, light is particularly versatile owing to its temporal precision in inducing faster cellular responses. The following subsections describe the application of these external stimuli in neuromodulation and drug delivery to the brain.
2.1. Electromagnetic fields
Stimulation of the brain by electromagnetic fields has been described in several studies.38,40–42 Transcranial electrical stimulation modulates voltages across the cell membrane by adjusting the pulse amplitude, frequency and the duration of alternating or direct current.38 Although this modality has been applied in the clinic for the activation of the cortex in order to restore memory, cognitive and motor functions, there is limited evidence of its efficacy.43 This could be attributed to electric current dissipation through the skull, which can be compensated by applying high voltages in the patient's scalp albeit at the expense of potential discomfort.44 The use of cathodes or anodes to modulate neuronal activity can be replaced by high- (>5 Hz) or low-frequency (<1 Hz) magnetic fields, respectively.40–42 Transcranial magnetic stimulation circumvents the need for electrodes contacting the surface of the skin using an insulated coil over the scalp, which generates fast-oscillating magnetic fields with a greater penetration depth into the brain.45 Perpendicular to the magnetic field, the generated electric field modulates neuronal activity with greater depth and resolution by manipulating the coil shape and magnetic field strength. A variety of protocols varying in stimulation time and frequency have been investigated in the clinic,46,47 with encouraging results in the management of some psychiatric conditions.48 One of these protocols involves the repeated application of intermittent pulses known as theta burst stimulation, which can promote the recovery of neuronal activity in neurodegenerative diseases by attenuating inflammation and oxidative stress.49 However, magnetic stimulation struggles to precisely target the deep regions of the brain such as the hippocampus. Temporal interference between multiple high-frequency electromagnetic fields (∼1 kHz) slightly differing in frequency has dramatically improved the spatial resolution of magnetic fields, albeit without matching the resolution of conventional DBS.50,51 Selective targeting and control over the depth of stimulation may be achieved by modulating the amplitude of the applied electromagnetic field.52
Electromagnetic fields can also be used for the activation of drug release systems in the brain. For example, iron oxide NPs can be activated by alternating magnetic fields (20–40 MHz) to generate heat which can be exploited for targeted drug delivery to the brain using temperature-sensitive liposomes,51 and to accelerate proton release from polymeric scaffolds undergoing hydrolytic degradation, thus eliciting Ca2+ influx.53 Heat generation from magnetic NPs can also activate heat-sensitive ion channels (e.g. TRPV1) which may be promising in the stimulation of peripheral nerves and organs such as the adrenal gland.54–56 These approaches can be synergistically combined for multiplexed control over cell signalling using two different magnetic NP populations sensitive to different frequencies and amplitudes.57 Nevertheless, further demonstration of the long-term safety and efficacy of these stimulation strategies is warranted, especially considering the poorer temporal resolution of magnetic fields (Fig. 2). Given that magnetic NPs can readily generate heat under high-frequency magnetic fields, reaching local temperatures above 42 °C, it is vital to demonstrate that these thermal effects are localised and reversible. In order to minimise undesired thermal effects, a recent study has enabled the potential use of clinically relevant, low-frequency magnetic fields (5 Hz) to stimulate mechanically sensitive channels (e.g. Piezo2, TRPV4) through the activation of oscillating magnetite nanodiscs.58
2.2. Ultrasounds
Ultrasounds are an emerging alternative for brain stimulation. Ultrasounds can target neurons in the deep regions of the brain, owing to the use of focused transducers narrowing their resolution down to 2–4 mm.59 Spatial precision can be adjusted by the sonication frequency which is limited between 200 and 700 kHz.59 Whilst higher frequencies (>1 MHz) could further improve their resolution, such acoustic waves could experience significant attenuation across the skull and propagation to undesired regions. A recent study suggested that the pulse repetition frequency could also be modulated to tailor the application of ultrasounds in either excitatory or inhibitory neurons.60 Another aspect to consider is the intensity of the applied ultrasounds. Neuromodulation is limited to low-intensity ultrasounds (<200 W cm−2) in order to expand synaptic junctions without excessive heat generation, which may otherwise inhibit synaptic activity by enhancing potassium and suppressing sodium ion channels, especially during prolonged exposures.59
Hyperthermia induced by high-intensity ultrasounds can be employed to transiently open the BBB and to trigger drug release from NPs targeting the brain. Low-intensity ultrasounds can induce similar effects through the administration of microbubbles, which render the BBB permeable to drug delivery carriers. Focused ultrasounds were necessary for the transport of polymeric NPs delivering gene therapy to the brain, encoding neurotrophic factors which promoted the replenishment of dopaminergic neurons in a rat model of PD.61 This strategy may also be applied for targeted neuromodulation owing to the translocation of viral vectors at the desired site, transfecting specific neurons with receptors for chemo- and optogenetics.62,63 Alternatively, neurons can be manipulated to express heat- or mechanosensitive ion channels, in order to enhance their sensitivity to ultrasounds.64 These strategies are required to overcome the noise caused by auditory neurons which are strongly affected by ultrasounds directed to the brain cortex, and to ensure that the application of ultrasounds is not also restricted to peripheral organs.65,66
The limitations imposed by skull density and unwanted BBB opening have motivated the development of alternative approaches for targeted and safe ultrasound application. Engineered microbubbles tethered to drug-loaded liposomes can accumulate at the site of application of ultrasound using only 1% of the acoustic intensity (pressure amplitude = 75 kPa, corresponding to ∼0.18 W cm−2) used for conventional focused ultrasound stimulation. Aggregated drug carriers can therefore burst through cavitation forces and release their cargo to the target site using 10× more power (pressure amplitude = 188 kPa, corresponding to ∼1.14 W cm−2), with minimal temperature fluctuation and without opening the BBB.67 Another strategy to mitigate thermal and mechanical tissue damage involves the administration of piezoelectric nanomaterials that convert the applied ultrasounds to an electrical stimulus. Boron nitride and barium titanate (BaTiO3) rank among the main candidates for piezoelectric stimulation owing to their biocompatibility, and can be synthesised in a variety of shapes resulting in unique physicochemical properties.68 These NPs maintained their capability of electrically stimulating SH-SY5Y neuroblastoma cells, either in suspension or being immobilised in piezoelectric films made of poly(vinylidene fluoride-trifluoroethylene) (PVDF-TrFE).69–71 Carbon-coated BaTiO3 NPs were recently demonstrated to stimulate dopaminergic neurons in a zebrafish model of PD.72 Despite these promising results, further investigation on the control over the ultrasound dose delivered in vivo, as well as on the long-term in vivo biocompatibility and stability of these NPs, irrespective of their immobilisation in medical devices, is warranted to support their clinical translation.
2.3. Light
Light can be used for brain stimulation and brain regeneration, in both cases, with or without the use of light-sensitive biomaterials (this section will be further expanded in section 3). Although transcranial activation constitutes an attractive alternative to DBS owing to its minimal invasiveness, the aforementioned methods currently employed in the clinic lack temporal resolution and anatomical specificity to target small brain structures (e.g. subthalamic nucleus, SVZ) with spatiotemporal resolution, without affecting the physiological activity of neighbouring cells.73 The application of near infrared (NIR) radiation (λ = 650–1000 nm) is attractive owing to its reduced absorption by biological fluids, which results in a high penetration rate compared to other wavelengths, with 3–5% of the applied energy reaching the cortical surface of the brain (Fig. 2).74,75 Moreover, NIR radiation (λ = 800–1000 nm) has been associated with beneficial effects on cellular activity by enhancing mitochondrial activity and increasing blood flow and oxygenation.76 As a result, NIR radiation can upregulate the expression of the growth factor BDNF in the DG and SVZ, promoting neurogenesis after tissue injury.77–79 In addition to promoting tissue regeneration, infrared light (λ = 2–6 μm) has been observed to activate temperature-gated Ca2+ channels in neurons and trigger action potentials after membrane depolarisation.39,80 This could be ascribed to the molecular vibrations of water molecules induced by light absorption, which generate heat capable of eliciting mechanical changes in the phospholipid cell membrane (Fig. 3).81 The spatial precision of membrane depolarization can be further refined by the gene delivery of heat-sensitive ion channels (e.g. TRPV1) which can then be targeted by polymeric nanoparticles exhibiting excellent photothermal properties, enabling heat generation with NIR activation at low power densities and thereby preserving tissue health.82,83 However, such depolarisation is only transient as longer irradiation exposures can instead induce membrane hyperpolarisation and suppress synaptic activity.84 Nevertheless, transcranial NIR stimulation has been investigated in several clinical trials to treat neurological disorders including depression, anxiety, and AD.76 However, the largest clinical trial exploring the therapeutic potential of NIR radiation (λ = 808 nm) in patients undergoing acute ischemic stroke failed to demonstrate measurable neuroprotective effects.85–87 This could be attributed to the poor clinical translation of the delivered radiation dose, considering that the human scalp and skull can absorb 5–10× more radiation than their rodent counterparts.75 Further investigation is therefore warranted to translate the application of NIR radiation from small animals to humans.
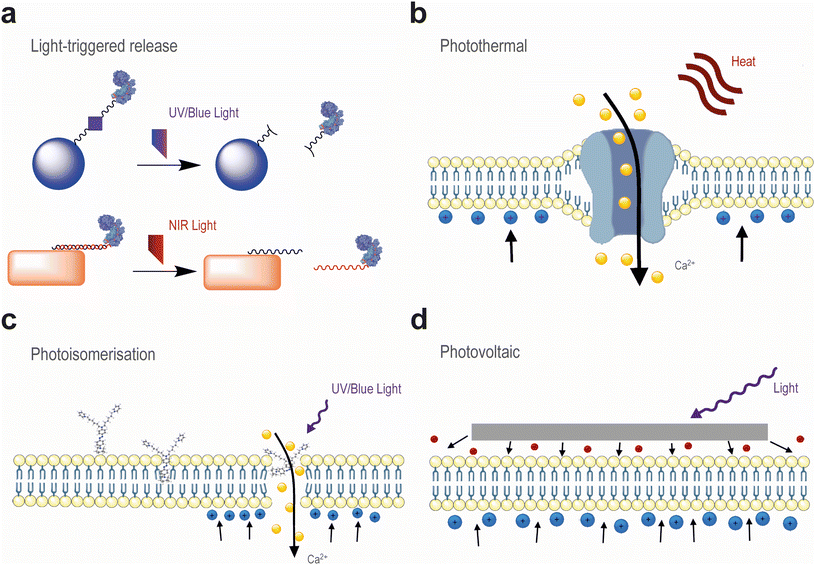 |
| Fig. 3 Applications of light for targeted drug delivery and neuromodulation. (a) Conjugation of NPs with biomolecules through light- or heat-sensitive linkers can improve the specificity over their release. (b) Heat generation near cell membranes may increase their permeability and activate heat-sensitive channels (e.g. TRPVs), which mediate Ca2+ influx and the migration of cations toward the cell membrane, triggering membrane depolarisation. (c) Similar effects can be achieved by the artificial introduction of azobenzene-based molecules which intercalate the cell membrane and mechanically open it via photoisomerisation upon exposure to UV/blue light. (d) Membrane depolarisation can also be achieved by the application of electrodes, which may generate electrons upon visible light activation. These electrons are injected to the neighbouring cell membranes. | |
3. Optical tools to modulate brain activity and regeneration
In order to overcome the limited penetration of light, sources such as fibre optics and light-emitting diodes have been implanted in pre-clinical models.88 However, this invasive procedure may be detrimental to the brain due to localised heat dissipating from the light emission point, which enhances the glial response to these implants and compromises their long-term performance. Transcranial light activation can surmount these issues by enabling a minimally invasive procedure, which would nonetheless require the implantation of transducers capable of increasing the sensitivity to the external light trigger. In this section, we cover how these transducers can enable the application of tissue-penetrating NIR radiation in drug delivery and neuromodulation.
3.1. Light-triggered drug delivery
As mentioned earlier, NIR radiation and infrared radiation usually induce photothermal effects which can modulate cell membrane permeability. Similarly to ultrasound activation, heat generation can be exploited to transiently open the BBB and enable drug delivery to the brain. With this aim, gold NPs are highly versatile candidates owing to their localised surface plasmon resonance (SPR), enabling heat generation in response to a specific wavelength of light, which can be readily tuned by adjusting the NP size and shape.89–91 The selectivity of gold NPs has been further improved by functionalisation with biomolecules (e.g. antibodies, peptides) targeting specific receptors in endothelial cells, which have enhanced in vivo NP transport to the brain parenchyma of mice without long-lasting modifications to the brain vasculature.21,92 Iron oxide NPs have also been recently reported to generate heat upon exposure to red light, thus facilitating the cellular uptake of polymeric nanofibre complexes for in vitro delivery of gene editing components.93
In addition to modulating the permeability of the BBB, light-generated heat can also trigger on-demand release of proteins and non-coding RNA (Fig. 3).94,95 For this purpose, gold NPs can be functionalised with oligonucleotides whose complementary sequences are bound to therapeutic cargoes for hybridisation. These sequences can be designed with different melting temperatures, thus requiring different laser powers for on-demand release. Another approach consists of labelling liposomes with gold NPs for enhanced heat generation, in order to mechanically destabilise the ordered lipid structure by reaching its phase transition temperature.96,97 However, heat generation lacks control over the targeted location, thus potentially causing cellular damage to the undesired regions of the brain.
The spatiotemporal resolution of light can be exploited to precisely destabilise lipid membranes using small molecules. For instance, photosensitising dyes can be incorporated into the lipid bilayer of liposomes and generate reactive oxygen species (ROS) upon irradiation.98 These ROS react with the hydrophobic chains of unsaturated lipids, inducing lipid peroxidation which renders them more hydrophilic. As a result, the liposome integrity is compromised and its cargo is released on-demand. However, this strategy generally requires the use of ultraviolet (UV) light which has also been employed for the activation of photocleavable moieties (Fig. 3).34,99,100 In addition to manipulating oxidative processes, lipid membranes can be mechanically destabilised via the photoisomerisation of azobenzene-based compounds, which can be readily reversed by applying different wavelengths to the brain.101–105 Photoswitchable molecules targeting cannabinoid and neurotransmitter receptors, as well as calcium channels, were demonstrated to enable influx and stimulate the intracellular release of Ca2+ upon UV light exposure.101–104 Rather than docking in protein-based receptors, a recent strategy consisted of designing an azobenzene-based compound bearing a hydrophobic azepane group and two alkyl chains terminated with ionisable moieties, which allow the molecule to spontaneously intercalate the cell membrane by alignment with the phospholipid head groups (Fig. 3).105,106 This compound exhibited a remarkable affinity toward lipid rafts, where it could mechanically alter the cell membrane thickness by photoisomerisation and induce membrane voltage changes resembling action potentials typically elicited by ion channels.106
However, the modulation of biological tissues with UV/blue light is not readily amenable for their application in deep regions, because of its limited penetration depth.99 Lanthanide-doped upconversion nanoparticles (UCNPs) can address this limitation by locally emitting light after absorbing multiple photons in the tissue-penetrating NIR region.107 UCNPs have demonstrated their capability for the on-demand release of gene editing components, and can precisely modulate cell differentiation processes in the brain.34,100,108 These processes can be further modulated with the synergistic activation of signalling pathways by ROS generation.109
3.2. Optogenetics
Optogenetics has been explored for the last 20 years for the study of physiological processes in neurons by genetically manipulating them to become responsive to light.110–112 In addition to fundamental neuroscience research, the development of therapeutic applications of optogenetics is underway.113,114 A recent study demonstrated the clinical potential of optogenetics by partially restoring vision in a patient suffering from a rare disease leading to retinal photoreceptor degeneration.115 This therapy consisted of the combination of opsin gene delivery to the retina with the use of goggles converting ambient to monochromatic red light. This approach can be further expanded to modulate specific electrical circuits mediated by neurons, which could not only elucidate the functional effects of brain diseases but also provide deeper insight into the potential mechanisms explaining ineffective pharmacological therapies. For instance, the synaptic activity of neurons populating different regions of the cortex can be readily monitored and modulated, in order to attenuate neurological impairment caused by extensive tissue damage.114 Both the cortex and the cerebellum constitute attractive targets due to their widespread neuronal projections to the thalamus and spinal cord, with strong implications in multiple motor and sensory regions.114,116,117 Another interesting target is the striatum, where optogenetic stimulation of glutamatergic neurons was demonstrated to promote neurogenesis through their projections to the adjacent SVZ, thus activating proliferative NSCs.118 These results suggest that targeted neuromodulation could promote tissue regeneration.
Several strategies have been developed to improve the control over opsin expression, and thus refine the use of light to regulate neuronal activity.119 One approach consists of developing genetic constructs for conditional expression of opsins using inducible, cell-specific promoters that are sensitive to the activity of Cre recombinase.120,121 Selective opsin expression could be further controlled using light as an external trigger for the on-demand release of Cre recombinase.34,122 Another approach consists of the use of UCNPs. UCNPs have enabled transcranial neuromodulation using NIR radiation by emitting blue or green light to activate channelrhodopsins and halorhodopsins, respectively.123,124 Importantly, the lack of glial responses suggests that these nanocrystals could be employed for minimally invasive chronic neuromodulation.124 Furthermore, the optical tuneability of UCNPs by selecting different lanthanides enables the selective activation of opsins at different wavelengths,125 thus avoiding the need for multiple implanted light sources in the brain. A recent study has shown that engineering UCNPs with 3 different activators (Nd, Yb, Er) enabled specific NIR activation using 3 different wavelengths (808, 980, and 1532 nm, respectively), resulting in 3 different colours (green, blue, and red, respectively).126 This nanoformulation was employed to specifically stimulate 3 different opsins for the targeted optogenetic activation of 3 different neuronal populations in the mouse brain (Fig. 4). These results pave the way for precise modulation of complex neuronal circuits using a single transducer.
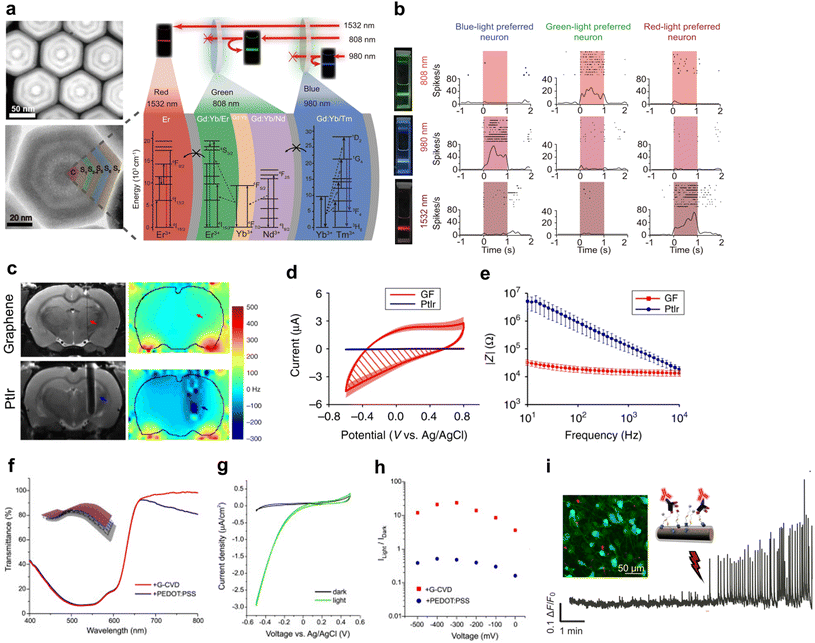 |
| Fig. 4 Optical tools for neuromodulation. (a) Representative high-resolution TEM image of UCNPs doped with different layers of lanthanides, conferring specific emission of light at the desired wavelengths. (b) Representative photographs illustrating that the NIR laser excitation of UCNPs at λ = 808, 980, and 1532 nm resulted in the emission of green, blue, and red light, respectively. Specific light emission was employed to stimulate neuronal populations sensitive to each colour, resulting in an increased neuronal firing rate under light exposure. Images of (a) and (b) are adapted from ref. 126 with permission from Springer Nature Customer Service Centre GmbH: Springer Nature, Nature Communications, under a Creative Commons license (CC BY 4.0). Copyright© 2021. (c) Representative T2-weighted images (grayscale) and B0 distortion maps (colourscale) of the coronal sections of rat brains illustrating the minimal interference of graphene-based electrodes in MRI (highlighted with red arrows), compared to clinically relevant platinum–iridium (PtIr) electrodes (highlighted with blue arrows). (d) Cyclic voltammetry curves showing that both graphene-based (GF) and PtIr electrodes did not exhibit current peaks indicative of redox processes, suggesting that these materials mediate capacitive charge conduction. (e) Electrochemical impedance spectroscopy was used to demonstrate the high electrical conductivity of graphene-based electrodes (GF) that maintained lower impedance values than PtIr over a wide range of frequencies. Images of (c), (d), and (e) are adapted from ref. 138 with permission from Springer Nature Customer Service Centre GmbH: Springer Nature, Nature Communications, under a Creative Commons license (CC BY 4.0). Copyright© 2020. (f) Graphene revealed superior optical transparency than the transparent polymer PEDOT:PSS, particularly in the NIR region, which demonstrated its compatibility with optically active P3HT. (g) Representative cyclic voltammetry curves showing a pronounced increase in the current density on graphene-based electrodes. (h) This increase was more pronounced for graphene-based electrodes compared to PEDOT:PSS, indicating a stronger photovoltaic effect. Images of (f), (g), and (h) are reprinted from ref. 162 with permission from Elsevier, under a Creative Commons license (CC BY-NC-ND 4.0). Copyright© 2020. (i) SiNWs can be functionalised with antibodies for targeted neuromodulation. The representative confocal image illustrates the uptake of functionalised SiNWs (red) by neuronal cells explanted from the mouse spinal cord mediating calcium transport (green). Light activation resulted in an increased fluorescence signal, suggesting increased synaptic activity. Adapted from ref. 169 with permission from AAAS, under a Creative Commons license (CC BY-NC 4.0). Copyright© 2022. | |
3.3. Optoelectronic neuromodulation
Despite these promising advances in optogenetics, safety concerns related to the potential integration of transgenes in target neurons for synaptic activation or inhibition have hindered clinical translation. Non-genetic approaches using minimally invasive devices are therefore attractive to modulate neuronal activity using light. Inspired by the recent development of artificial retinas,127 conversion of light to an electric signal could provide a safer alternative for localised neuromodulation with unprecedented resolution. Therefore, optoelectronic devices warrant the design of biointerfaces comprised of compatible materials presenting the following characteristics: (i) high transparency, (ii) high surface area, (iii) low electrochemical impedance, and (iv) high flexibility.
Medical devices for electrical stimulation have been developed using metallic electrodes, such as platinum, gold, and iridium.128,129 These metals are characterised by their excellent electrical conductivity, albeit with significant differences in how charge transfer is mediated in the physiological milieu. Electrolytes will dissociate into anions and cations which will adsorb onto the electrode surface depending on its charge. Ideally, electricity is generated from this charge redistribution at the electrode–electrolyte interface, which is known as capacitive charge conduction.128 However, most metals are not inert and gain or lose electrons upon charge injection, thus mediating reduction and oxidation reactions with electrolytes. Although faradaic charge conduction allows for a greater amount of generated electric current than capacitive charge conduction, repeated redox reactions may be irreversibly corrosive and compromise the electrode's long-term performance and biocompatibility.128 Hence, pseudocapacitive materials such as platinum and iridium alloys have become the gold standard in medical devices because they combine both faradaic and capacitive processes, thereby increasing the amount of injected current without inducing significant redox reactions.128 However, the lack of optical transparency from metals hinders their application in optogenetics. Moreover, most metals interfere with clinically used neuroimaging techniques such as MRI,24 thus impeding the objective optimisation of stimulation parameters (Fig. 4).130
Transparent materials capable of capacitive charge conduction are therefore desired for biointerfaces. Although indium tin oxide (ITO) has been widely applied as a transparent component in the fabrication of electronic devices, its implementation in medical devices faces numerous drawbacks. First, ITO's brittleness restricts the active area of recording electrodes for their safe integration in the brain.131 Mechanical mismatch and electrode micromotion could result in severe complications in the patient due to the formation of glial scars interfacing the electrode's surface,132 which also hamper the amount of electric current flowing during stimulation and recording.133 In addition, ITO significantly absorbs light in both high (UV/blue) and low (NIR/infrared) regions of the visible spectrum. Flexible polymers such as poly(3,4-ethylenedioxythiophene) (PEDOT) have thus been employed in neural interfaces.134 When stabilised by poly(styrenesulfonate) (PSS), PEDOT can be readily processed in aqueous dispersions, which enables affordable device production by inkjet printing.135 However, the pseudocapacitive charge conduction of PEDOT:PSS films may result in the oxidation of PEDOT when operating at a high power,128 causing their delamination. Electrode degradation can be minimised by coating with nanomaterials,136 which were demonstrated to enhance charge injection and minimise impedance, thus allowing for device miniaturisation and lengthening its longevity.128,129 Carbon nanomaterials such as carbon nanotubes (CNTs) and graphene have emerged in recent years owing to their greater stability in the physiological environment whilst maintaining high electrical conductivity and low impedance.137–139 These materials have been employed in the development of transparent devices combining optogenetics with electrical recording with high fidelity,139–141 owing to the capacitive charge conduction resulting from the ejection of π electrons in sp2 hybridised carbon atoms (Fig. 4).142,143 In particular, graphene reunites interesting qualities such as improved biocompatibility compared to CNTs and enhanced neuronal activity,144–146 and its protective action over the corrosion and electromagnetic susceptibility of metal electrodes.137 However, graphene electrodes were shown to suffer from light-induced artefacts during optogenetic stimulation.141 These artefacts were proportional to the light exposure time and power, and resulted from the photovoltaic generation of electric current, which is known as the Becquerel effect (Fig. 3). This phenomenon was exploited by Savchenko et al., who developed a non-genetic platform for capacitive optoelectronic stimulation of cardiomyocytes using visible light.147 Nevertheless, it is unclear how these substrates would respond to tissue-penetrating NIR radiation towards their in vivo application. Although graphene's semimetal behaviour confers it with high sensitivity to light, significant photothermal responses restrict the applied light intensity and exposure time to GBM electrodes.148 Light-induced heat in graphene is caused by local collisions between electrons, resulting in energy dissipation in the form of phonons.149 This issue can be alleviated by chemical doping of graphene or by introducing an electric current to the electrode, in order to modulate the Fermi energy.150 Alternatively, efficient electron cooling after light exposure can be ensured by minimising the presence of defects in graphene's crystal structure, which are responsible for heat-generating acoustic phonon emission.151
An attractive strategy consists of incorporating these conductive nanomaterials with photoactive compounds efficiently absorbing light, such as poly-3-hexylthiophene (P3HT),127 carbon nitride,152 and other organic dye molecules,153–155 as well as semiconducting quantum dots and nanorods.156,157 These compounds often combine photovoltaic and photothermal effects. P3HT can be conjugated with oxygen-containing materials such as ITO or PEDOT:PSS which act as electron acceptors allowing for effective charge dissociation at the interface of the illuminated site (Fig. 4).127,158–161 GBMs were demonstrated to elicit greater charge separation than PEDOT:PSS, thus attracting cations from the electrolyte and subsequently inducing membrane depolarisation of primary hippocampal neurons.162 Nevertheless, such depolarisation is only transient as longer irradiation exposures can instead induce membrane hyperpolarisation and suppress synaptic activity.84 This could be attributed to the dominance of the non-radiative transitions of excited electrons back to the ground state after light exposure,158 which generates heat that could alter the activity of Na+ and K+ channels, as mentioned earlier. Another possibility could reside in coupling GBMs with UCNPs, which has dramatically enhanced their electrical response to NIR light for highly sensitive photodetectors.163–165 These composites could be explored for optical stimulation of cells using NIR radiation.166
In spite of the growing interest in transparent materials, the application of silicon in optoelectronics has also been investigated owing to its extensive implementation in the market. Among a variety of developed nanoarchitectures, silicon nanowires (SiNWs) have been recently shown to stimulate cells upon light exposure (Fig. 4).167–169 Photothermal and photoelectrochemical generation of electrical current was catalysed by atomic gold used to produce SiNWs,167 and enhanced by doping crystalline silicon to constitute p-i-n nanostructures, which mediate faradaic charge conduction to their physiological surroundings.168 However, this may require high radiation power to compensate silicon's poor light absorption,169 or the development of larger and thicker structures which are not suitable for long-term implantation in the body due to the increased likelihood of gliosis due to mechanical mismatch. The combination with other materials with excellent optical absorption (e.g. perovskites) could improve optoelectronic conversion and enable device miniaturisation.170
4. Conclusion and outlook
In this review, we have covered the recent progress in the development of pharmacological and non-pharmacological interventions with application in brain stimulation and regeneration. Due to their limited targeting specificity to the brain, stimuli-responsive formulations have been developed for on-demand release to a desired target region. The application of millisecond light pulses by two-photon microscopy has further improved the spatiotemporal resolution of light to trigger drug release and stimulate individual cells.33,34 However, they require high-power femtosecond lasers which lack efficiency and are thus not conducive to clinical application.35 This has led to the development of alternative strategies. One avenue of research consists of improving the laser efficiency by developing holographic techniques in combination with temporal focusing, in order to increase the activation volume without compromising its resolution.171 Another approach consists of employing microlenses to focus incident light on target cells without affecting the neighbouring neurons.172 A recent study showed that transparent polystyrene microspheres used as microlenses enabled the reduction of the applied laser power by 75%, thus dramatically decreasing the likelihood of photothermal damage in an in vivo optogenetic model.
Another aspect that warrants further development consists of the design of targeted nanocarriers to deliver medicines to the brain, in order to refine light activation with cellular tropism. One example capitalising on this strategy could be the modulation of immune cells to adopt an anti-inflammatory phenotype, thus supporting regenerative pathways mediated by endogenous stem cells.173 Thus far, synthetic NPs have failed to precisely target one cell type.174 Considering this limitation, extracellular vesicles (EVs) are naturally derived nanocarriers which have been explored as a cell-free option to promote tissue regeneration via paracrine signalling.175,176 Stem cell-derived EVs can supply trophic factors that promote angiogenesis and modulate the activity of glial cells, thus reducing oxidative stress and cell death.177 Furthermore, these EVs were demonstrated to support synaptic plasticity and improved neuronal function in aged mice.178 However, their clinical translation is hampered by technical challenges related to the variability in their production and purification.179 Nevertheless, EVs can serve as a model for the identification of targeting moieties directing their tropism to specific brain cells.180 Labelled with gold NPs to track their biodistribution by computerized tomography, EVs exhibited different biodistributions depending on the brain pathology.181 High-throughput screening methodologies can therefore be employed for rapid identification of bioactive molecules from EVs.176 In order to identify which candidates may be suitable for targeting the BBB, relevant in vitro models need to consider the impact of blood flow on the distribution of nanocarriers, as well as the structural impact of ageing on the BBB.182
Regarding the application of light in neuromodulation, we compared herein several mechanisms mediating wireless stimulation. The application of electromagnetic fields, ultrasound, or light depends on the location of the region of interest; all three can target the brain cortex, despite the limited penetration of light. Nevertheless, this could be sufficient to reach deeper regions such as the basal ganglia through cortical projections, which could ultimately target striatal regions linked to pathologies such as PD.183 Compared to the other external stimuli, light can achieve greater anatomical specificity and precisely stimulate cells using equipment that is less expensive and more miniaturised, which democratises access for a routine and continuous use. This technology is also compatible with closed-loop operation184 and has been recently implemented in clinically approved DBS devices. Moreover, this approach surmounts the need for implanted batteries in current medical devices, thus improving patients’ comfort and mobility. Further investigation to optimise stimulation parameters for each neurological disorder will determine which modality is the most suited. Nevertheless, in cases requiring continuous or targeted modulation of deep regions in the brain, surgical implantation of DBS devices is required.
Recent advances in materials science and engineering have improved the management of neurological disorders that currently lack effective pharmacological therapies, with the development of medical devices for brain stimulation. However, the risk of bleeding and infection and the foreign body response to the implanted device typically result in multiple surgeries which limit the clinical implementation of DBS. Currently, one third of clinical trials employing DBS are led by medical researchers aiming to minimise associated adverse effects.185 Implantable devices are expected to undergo significant changes in their design, as conventionally used metals like gold or platinum will be replaced by softer and/or flexible materials for minimally invasive procedures.133 The incorporation of carbon-based nanomaterials in soft viscoelastic hydrogels was demonstrated to seamlessly integrate in the brain, heart and muscle to both stimulate and record electrophysiological activity.186 Devices incorporated into injectable meshes or bioresorbable substrates have also been investigated to further reduce invasiveness and increase their biocompatibility.187,188 Another important aspect to consider in these implanted devices is their in vivo traceability. Recent medical devices have been designed to be compatible with neuroimaging tools (e.g. MRI, CT, and X-ray) and electrophysiological recording for intraoperative guidance. Future devices are expected to be more complex and combine electrophysiological recordings with biochemical information through the detection of neurotransmitters (e.g. dopamine), in order to fully characterise the synaptic circuitry.189
The development of devices capable of optoelectronic stimulation could pave the way for novel neuromodulation strategies. For instance, photocapacitive injection of electric current could be complemented with the heat-triggered release of small molecules, nucleic acids or proteins, conferring long-term control over cell behaviour in specific neural circuits with precise temporal resolution.190 In order to achieve this, UCNPs could exploit the greater penetration of NIR radiation to actuate in the deeper regions of the brain and combine optical stimulation with controlled drug release. Following their application in ITO films to promote neuronal differentiation,191 these NPs could also be attached to silicon- or graphene-based nanomaterials which rank among the most promising candidates for optoelectronic neuromodulation. Nevertheless, slow responses especially when the light is turned off may distort physiological action potentials, and thus require novel strategies to minimise the pure thermal effects of these stimulation modalities. Further investigation of device architecture and operation is also warranted to ensure long-term safety and improved control over stimulation. This includes studying the effects of prolonged exposure to the various components of these devices along their life cycle. In addition, optimisation of device fabrication processes should contribute to its clinical translation by incorporating cost-effective procedures such as inkjet printing.192 Finally, these devices should ideally become multifunctional, not only providing targeted stimulation but also recording their physiological surroundings for improved neuromodulation.133
Conflicts of interest
There are no conflicts to declare.
Acknowledgements
This work was supported by the Twinning (RESETageing, ref: 952266) project through the European Union's Horizon 2020 research and innovation programme, projects “NeuroAtlantic” (Ref: EAPA_791/2018) and “2IQBioNeuro” (Ref: 0624_2IQBIONEURO_6_E), which are co-funded by Program Interreg Atlantic Space through QREN-COMPETE, by the “Prova de conceito” project with the acronym Bio-Med (Ref: 181228) funded by the COMPETE 2020 and PRR Project “Agenda/Alianças mobilizadoras para a reindustrialização” (Ref: C630926586-00465198) funded by EC funds. AFR gratefully acknowledges the funding from the EU Horizon 2020 programme under grant agreement no. 101003413. The authors would like to thank Bárbara Pinho for assistance with figure preparation.
References
- V. L. Feigin, E. Nichols, T. Alam, M. S. Bannick, E. Beghi, N. Blake, W. J. Culpepper, E. R. Dorsey, A. Elbaz, R. G. Ellenbogen, J. L. Fisher, C. Fitzmaurice, G. Giussani, L. Glennie, S. L. James, C. O. Johnson, N. J. Kassebaum, G. Logroscino, B. Marin, W. C. Mountjoy-Venning, M. Nguyen, R. Ofori-Asenso, A. P. Patel, M. Piccininni, G. A. Roth, T. J. Steiner, L. J. Stovner, C. E. I. Szoeke, A. Theadom, S. E. Vollset, M. T. Wallin, C. Wright, J. R. Zunt, N. Abbasi, F. Abd-Allah, A. Abdelalim, I. Abdollahpour, V. Aboyans, H. N. Abraha, D. Acharya, A. A. Adamu, O. M. Adebayo, A. M. Adeoye, J. C. Adsuar, M. Afarideh, S. Agrawal, A. Ahmadi, M. B. Ahmed, A. N. Aichour, I. Aichour, M. T. E. Aichour, R. O. Akinyemi, N. Akseer, A. Al-Eyadhy, R. Al-Shahi Salman, F. Alahdab, K. A. Alene, S. M. Aljunid, K. Altirkawi, N. Alvis-Guzman, N. H. Anber, C. A. T. Antonio, J. Arabloo, O. Aremu, J. Ärnlöv, H. Asayesh, R. J. Asghar, H. T. Atalay, A. Awasthi, B. P. Ayala Quintanilla, T. B. Ayuk, A. Badawi, M. Banach, J. A. M. Banoub, M. A. Barboza, S. L. Barker-Collo, T. W. Bärnighausen, B. T. Baune, N. Bedi, M. Behzadifar, M. Behzadifar, Y. Béjot, B. B. Bekele, A. B. Belachew, D. A. Bennett, I. M. Bensenor, A. Berhane, M. Beuran, K. Bhattacharyya, Z. A. Bhutta, B. Biadgo, A. Bijani, N. Bililign, M. S. Bin Sayeed, C. K. Blazes, C. Brayne, Z. A. Butt, I. R. Campos-Nonato, C. Cantu-Brito, M. Car, R. Cárdenas, J. J. Carrero, F. Carvalho, C. A. Castañeda-Orjuela, F. Castro, F. Catalá-López, E. Cerin, Y. Chaiah, J. C. Chang, I. Chatziralli, P. P. C. Chiang, H. Christensen, D. J. Christopher, C. Cooper, P. A. Cortesi, V. M. Costa, M. H. Criqui, C. S. Crowe, A. A. M. Damasceno, A. Daryani, V. De la Cruz-Góngora, F. P. De la Hoz, D. De Leo, G. T. Demoz, K. Deribe, S. D. Dharmaratne, D. Diaz, M. T. Dinberu, S. Djalalinia, D. T. Doku, M. Dubey, E. Dubljanin, E. E. Duken, D. Edvardsson, Z. El-Khatib, M. Endres, A. Y. Endries, S. Eskandarieh, A. Esteghamati, S. Esteghamati, F. Farhadi, A. Faro, F. Farzadfar, M. H. Farzaei, B. Fatima, S. M. Fereshtehnejad, E. Fernandes, G. T. Feyissa, I. Filip, F. Fischer, T. Fukumoto, M. Ganji, F. G. Gankpe, M. A. Garcia-Gordillo, A. K. Gebre, T. G. Gebremichael, B. K. Gelaw, J. M. Geleijnse, D. Geremew, K. E. Gezae, M. Ghasemi-Kasman, M. Y. Gidey, P. S. Gill, T. K. Gill, E. T. Girma, E. V. Gnedovskaya, A. C. Goulart, A. Grada, G. Grosso, Y. Guo, R. Gupta, R. Gupta, J. A. Haagsma, T. B. Hagos, A. Haj-Mirzaian, A. Haj-Mirzaian, R. R. Hamadeh, S. Hamidi, G. J. Hankey, Y. Hao, J. M. Haro, H. Hassankhani, H. Y. Hassen, R. Havmoeller, S. I. Hay, M. I. Hegazy, B. Heidari, A. Henok, F. Heydarpour, C. L. Hoang, M. K. Hole, E. Homaie Rad, S. M. Hosseini, G. Hu, E. U. Igumbor, O. S. Ilesanmi, S. S. N. Irvani, S. M. S. Islam, M. Jakovljevic, M. Javanbakht, R. P. Jha, Y. B. Jobanputra, J. B. Jonas, J. J. Jozwiak, M. Jürisson, A. Kahsay, R. Kalani, Y. Kalkonde, T. A. Kamil, T. Kanchan, M. Karami, A. Karch, N. Karimi, A. Kasaeian, T. D. Kassa, Z. Y. Kassa, A. Kaul, A. T. Kefale, P. N. Keiyoro, Y. S. Khader, M. A. Khafaie, I. A. Khalil, E. A. Khan, Y. H. Khang, H. Khazaie, A. A. Kiadaliri, D. N. Kiirithio, A. S. Kim, D. Kim, Y. E. Kim, Y. J. Kim, A. Kisa, Y. Kokubo, A. Koyanagi, R. V. Krishnamurthi, B. Kuate Defo, B. Kucuk Bicer, M. Kumar, B. Lacey, A. Lafranconi, V. C. Lansingh, A. Latifi, C. T. Leshargie, S. Li, Y. Liao, S. Linn, W. D. Lo, J. C. F. Lopez, S. Lorkowski, P. A. Lotufo, R. M. Lucas, R. Lunevicius, M. T. Mackay, N. B. Mahotra, M. Majdan, R. Majdzadeh, A. Majeed, R. Malekzadeh, D. C. Malta, N. Manafi, M. A. Mansournia, L. G. Mantovani, W. März, T. P. Mashamba-Thompson, B. B. Massenburg, K. K. V. Mate, C. McAlinden, J. J. McGrath, V. Mehta, T. Meier, H. G. Meles, A. Melese, P. T. N. Memiah, Z. A. Memish, W. Mendoza, D. T. Mengistu, G. Mengistu, A. Meretoja, T. J. Meretoja, T. Mestrovic, B. Miazgowski, T. Miazgowski, T. R. Miller, G. K. Mini, E. M. Mirrakhimov, B. Moazen, B. Mohajer, N. Mohammad Gholi Mezerji, M. Mohammadi, M. Mohammadi-Khanaposhtani, R. Mohammadibakhsh, M. Mohammadnia-Afrouzi, S. Mohammed, F. Mohebi, A. H. Mokdad, L. Monasta, S. Mondello, Y. Moodley, M. Moosazadeh, G. Moradi, M. Moradi-Lakeh, M. Moradinazar, P. Moraga, I. Moreno Velásquez, S. D. Morrison, S. M. Mousavi, O. S. Muhammed, W. Muruet, K. I. Musa, G. Mustafa, M. Naderi, G. Nagel, A. Naheed, G. Naik, F. Najafi, V. Nangia, I. Negoi, R. I. Negoi, C. R. J. Newton, J. W. Ngunjiri, C. T. Nguyen, L. H. Nguyen, D. N. A. Ningrum, Y. L. Nirayo, M. R. Nixon, B. Norrving, J. J. Noubiap, M. Nourollahpour Shiadeh, P. S. Nyasulu, O. S. Ogah, I. H. Oh, A. T. Olagunju, T. O. Olagunju, P. R. Olivares, O. E. Onwujekwe, E. Oren, M. O. Owolabi, M. Padukudru Anand, A. H. Pakpour, W. H. Pan, S. Panda-Jonas, J. D. Pandian, S. K. Patel, D. M. Pereira, M. Petzold, J. D. Pillay, M. A. Piradov, G. V. Polanczyk, S. Polinder, M. J. Postma, R. Poulton, H. Poustchi, S. Prakash, V. Prakash, M. Qorbani, A. Radfar, A. Rafay, A. Rafiei, F. Rahim, V. Rahimi-Movaghar, M. Rahman, M. H. U. Rahman, M. A. Rahman, F. Rajati, U. Ram, A. Ranta, D. L. Rawaf, S. Rawaf, N. Reinig, C. Reis, A. M. N. Renzaho, S. Resnikoff, S. Rezaeian, M. S. Rezai, C. M. Rios González, N. L. S. Roberts, L. Roever, L. Ronfani, E. M. Roro, G. Roshandel, A. Rostami, P. Sabbagh, R. L. Sacco, P. S. Sachdev, B. Saddik, H. Safari, R. Safari-Faramani, S. Safi, S. Safiri, R. Sagar, R. Sahathevan, A. Sahebkar, M. A. Sahraian, P. Salamati, S. Salehi Zahabi, Y. Salimi, A. M. Samy, J. Sanabria, I. S. Santos, M. M. Santric Milicevic, N. Sarrafzadegan, B. Sartorius, S. Sarvi, B. Sathian, M. Satpathy, A. R. Sawant, M. Sawhney, I. J. C. Schneider, B. Schöttker, D. C. Schwebel, S. Seedat, S. G. Sepanlou, H. Shabaninejad, A. Shafieesabet, M. A. Shaikh, R. A. Shakir, M. Shams-Beyranvand, M. Shamsizadeh, M. Sharif, M. Sharif-Alhoseini, J. She, A. Sheikh, K. N. Sheth, M. Shigematsu, R. Shiri, R. Shirkoohi, I. Shiue, S. Siabani, T. J. Siddiqi, I. D. Sigfusdottir, R. Sigurvinsdottir, D. H. Silberberg, J. P. Silva, D. G. A. Silveira, J. A. Singh, D. N. Sinha, E. Skiadaresi, M. Smith, B. H. Sobaih, S. Sobhani, M. Soofi, I. N. Soyiri, L. A. Sposato, D. J. Stein, M. B. Stein, M. A. Stokes, M. B. Sufiyan, B. L. Sykes, P. N. Sylaja, R. Tabarés-Seisdedos, B. J. Te Ao, A. Tehrani-Banihashemi, M. H. Temsah, O. Temsah, J. S. Thakur, A. G. Thrift, R. Topor-Madry, M. Tortajada-Girbés, M. R. Tovani-Palone, B. X. Tran, K. B. Tran, T. C. Truelsen, A. G. Tsadik, L. Tudor Car, K. N. Ukwaja, I. Ullah, M. S. Usman, O.
A. Uthman, P. R. Valdez, T. J. Vasankari, R. Vasanthan, Y. Veisani, N. Venketasubramanian, F. S. Violante, V. Vlassov, K. Vosoughi, G. T. Vu, I. S. Vujcic, F. S. Wagnew, Y. Waheed, Y. P. Wang, E. Weiderpass, J. Weiss, H. A. Whiteford, T. Wijeratne, A. S. Winkler, C. S. Wiysonge, C. D. A. Wolfe, G. Xu, A. Yadollahpour, T. Yamada, Y. Yano, M. Yaseri, H. Yatsuya, E. M. Yimer, P. Yip, E. Yisma, N. Yonemoto, M. Yousefifard, C. Yu, Z. Zaidi, S. Bin Zaman, M. Zamani, H. Zandian, Z. Zare, Y. Zhang, S. Zodpey, M. Naghavi, C. J. L. Murray and T. Vos, Lancet Neurol., 2019, 18, 459–480 CrossRef PubMed.
- D. C. Park and P. Reuter-Lorenz, Annu. Rev. Psychol., 2009, 60, 173–196 CrossRef PubMed.
- M. Manford, J. Neurol., 2017, 264, 1811–1824 CrossRef PubMed.
- G. J. Hankey, Lancet, 2017, 389, 641–654 CrossRef PubMed.
- S. Pinho, M. H. Macedo, C. Rebelo, B. Sarmento and L. Ferreira, Drug Discovery Today, 2018, 23, 1071–1078 CrossRef CAS PubMed.
- C. R. Freed, P. E. Greene, R. E. Breeze, W.-Y. Tsai, W. DuMouchel, R. Kao, S. Dillon, H. Winfield, S. Culver, J. Q. Trojanowski, D. Eidelberg and S. Fahn, N. Engl. J. Med., 2001, 344, 710–719 CrossRef CAS PubMed.
- D. Kondziolka, G. K. Steinberg, L. Wechsler, C. C. Meltzer, E. Elder, J. Gebel, S. DeCesare, T. Jovin, R. Zafonte, J. Lebowitz, J. C. Flickinger, D. Tong, M. P. Marks, C. Jamieson, D. Luu, T. Bell-Stephens and J. Teraoka, J. Neurosurg., 2005, 103, 38–45 CrossRef PubMed.
- R. Mays and R. Deans, Transfusion, 2016, 56, 6S–8S CrossRef PubMed.
- L.-Y. Qiao, F.-J. Huang, M. Zhao, J.-H. Xie, J. Shi, J. Wang, X.-Z. Lin, H. Zuo, Y.-L. Wang and T.-C. Geng, Cell Transplant., 2014, 23, S65–S72 Search PubMed.
- K. W. Muir, D. Bulters, M. Willmot, N. Sprigg, A. Dixit, N. Ward, P. Tyrrell, A. Majid, L. Dunn, P. Bath, J. Howell, P. Stroemer, K. Pollock and J. Sinden, J. Neurol., Neurosurg. Psychiatry, 2020, 91, 396–401 CrossRef PubMed.
- W. Tai, X. M. Xu and C. L. Zhang, Front. Cell. Neurosci., 2020, 14, 107 CrossRef CAS PubMed.
- S. Aday, J. Zoldan, M. Besnier, L. Carreto, J. Saif, R. Fernandes, T. Santos, L. Bernardino, R. Langer, C. Emanueli and L. Ferreira, Nat. Commun., 2017, 8, 747 CrossRef PubMed.
- M. Machado-Pereira, T. Santos, L. Ferreira, L. Bernardino and R. Ferreira, Neurosci. Lett., 2018, 673, 116–121 CrossRef CAS PubMed.
- T. Santos, R. Ferreira, J. Maia, F. Agasse, S. Xapelli, L. Cortes, J. Bragancia, J. O. Malva, L. Ferreira and L. Bernardino, ACS Nano, 2012, 6, 10463–10474 CrossRef CAS PubMed.
- P. Navarro Negredo, R. W. Yeo and A. Brunet, Cell Stem Cell, 2020, 27, 202–223 CrossRef CAS PubMed.
- E. P. Moreno-Jiménez, M. Flor-García, J. Terreros-Roncal, A. Rábano, F. Cafini, N. Pallas-Bazarra, J. Ávila and M. Llorens-Martín, Nat. Med., 2019, 25, 554–560 CrossRef PubMed.
- X. Feng, J. Guo, H. C. Sigmon, R. P. Sloan, A. M. Brickman, F. A. Provenzano and S. A. Small, PLoS One, 2020, 15, e0234255 CrossRef CAS PubMed.
- M. Boldrini, C. A. Fulmore, A. N. Tartt, L. R. Simeon, I. Pavlova, V. Poposka, G. B. Rosoklija, A. Stankov, V. Arango, A. J. Dwork, R. Hen and J. J. Mann, Cell Stem Cell, 2018, 22, 589–599 CrossRef CAS PubMed.
- D. J. Wolak and R. G. Thorne, Mol. Pharm., 2013, 10, 1492–1504 CrossRef CAS PubMed.
- C. Saraiva, C. Praça, R. Ferreira, T. Santos, L. Ferreira and L. Bernardino, J. Controlled Release, 2016, 235, 34–47 CrossRef CAS PubMed.
- C. Praça, A. Rai, T. Santos, A. C. Cristovão, S. L. Pinho, R. Cecchelli, M. P. Dehouck, L. Bernardino and L. S. Ferreira, J. Controlled Release, 2018, 284, 57–72 CrossRef PubMed.
- Y. Yan, Y. Chen, Z. Liu, F. Cai, W. Niu, L. Song, H. Liang, Z. Su, B. Yu and F. Yan, Int. J. Nanomed., 2021, 16, 7433–7447 CrossRef CAS PubMed.
- J. Chen, M. Yuan, C. A. Madison, S. Eitan and Y. Wang, J. Controlled Release, 2022, 345, 557–571 CrossRef CAS PubMed.
- J. K. Krauss, N. Lipsman, T. Aziz, A. Boutet, P. Brown, J. W. Chang, B. Davidson, W. M. Grill, M. I. Hariz, A. Horn, M. Schulder, A. Mammis, P. A. Tass, J. Volkmann and A. M. Lozano, Nat. Rev. Neurol., 2021, 17, 75–87 CrossRef PubMed.
- M. Levin, G. Pezzulo and J. M. Finkelstein, Annu. Rev. Biomed. Eng., 2017, 19, 353–387 CrossRef CAS PubMed.
- V. Vedam-Mai, B. Gardner, M. S. Okun, F. A. Siebzehnrubl, M. Kam, P. Aponso, D. A. Steindler, A. T. Yachnis, D. Neal, B. U. Oliver, S. J. Rath, R. L. M. Faull, B. A. Reynolds and M. A. Curtis, PLoS One, 2014, 9, e88770 CrossRef PubMed.
- B. J. Hilton, E. Anenberg, T. C. Harrison, J. D. Boyd, T. H. Murphy and W. Tetzlaff, J. Neurosci., 2016, 36, 4080–4092 CrossRef PubMed.
- M. Jakobs, A. Fomenko, A. M. Lozano and K. L. Kiening, EMBO Mol. Med., 2019, 11, e9575 Search PubMed.
- C. Buhmann, T. Huckhagel, K. Engel, A. Gulberti, U. Hidding, M. Poetter-Nerger, I. Goerendt, P. Ludewig, H. Braass, C. U. Choe, K. Krajewski, C. Oehlwein, K. Mittmann, A. K. Engel, C. Gerloff, M. Westphal, J. A. Köppen, C. K. E. Moll and W. Hamel, PLoS One, 2017, 12, e0178984 CrossRef PubMed.
- A. P. Burdick, H. H. Fernandez, M. S. Okun, Y. Y. Chi, C. Jacobson and K. D. Foote, Neurosurg. Focus, 2010, 29, E4 Search PubMed.
- M. I. Hariz, S. Rehncrona, N. P. Quinn, J. D. Speelman and C. Wensing, Mov. Disord., 2008, 23, 416–421 CrossRef PubMed.
- M. S. Okun, N. Engl. J. Med., 2012, 367, 1529–1538 CrossRef CAS PubMed.
- O. A. Shemesh, D. Tanese, V. Zampini, C. Linghu, K. Piatkevich, E. Ronzitti, E. Papagiakoumou, E. S. Boyden and V. Emiliani, Nat. Neurosci., 2017, 20, 1796–1806 CrossRef CAS PubMed.
- C. Rebelo, T. Reis, J. Guedes, C. Saraiva, A. F. Rodrigues, S. Simões, L. Bernardino, J. Peça, S. L. C. Pinho and L. Ferreira, Nat. Commun., 2022, 13, 4135 CrossRef CAS PubMed.
- S. H. Yun and S. J. J. Kwok, Nat. Biomed. Eng., 2017, 1, 0008 CrossRef CAS PubMed.
- H. Wang, B. Wang, K. P. Normoyle, K. Jackson, K. Spitler, M. Sharrock, C. M. Miller, C. Best, D. Llano and R. Du, Front. Neurosci., 2014, 8, 307 Search PubMed.
- S. M. Won, E. Song, J. T. Reeder and J. A. Rogers, Cell, 2020, 181, 115–135 CrossRef CAS PubMed.
- D. M. Thompson, A. N. Koppes, J. G. Hardy and C. E. Schmidt, Annu. Rev. Biomed. Eng., 2014, 16, 397–430 CrossRef CAS PubMed.
- M. Shapiro, K. Homma and S. Villarreal, Nat. Commun., 2012, 3, 736 CrossRef PubMed.
- M. A. Nitsche, M. S. Nitsche, C. C. Klein, F. Tergau, J. C. Rothwell and W. Paulus, Clin. Neurophysiol., 2003, 114, 600–604 CrossRef PubMed.
- A. Pascual-Leone, J. Valls-Solé, E. M. Wassermann and M. Hallett, Brain, 1994, 117, 847–858 CrossRef PubMed.
- W. Muellbacher, U. Ziemann, B. Boroojerdi and M. Hallett, Clin. Neurophysiol., 2000, 111, 1002–1007 CrossRef CAS PubMed.
- H. L. Filmer, P. E. Dux and J. B. Mattingley, Trends Neurosci., 2014, 37, 742–753 CrossRef CAS PubMed.
- P. M. Rossini, D. Burke, R. Chen, L. G. Cohen, Z. Daskalakis, R. Di Iorio, V. Di Lazzaro, F. Ferreri, P. B. Fitzgerald, M. S. George, M. Hallett, J. P. Lefaucheur, B. Langguth, H. Matsumoto, C. Miniussi, M. A. Nitsche, A. Pascual-Leone, W. Paulus, S. Rossi, J. C. Rothwell, H. R. Siebner, Y. Ugawa, V. Walsh and U. Ziemann, Clin. Neurophysiol., 2015, 126, 1071–1107 CrossRef CAS PubMed.
- R. Polanía, M. A. Nitsche and C. C. Ruff, Nat. Neurosci., 2018, 21, 174–187 CrossRef PubMed.
- Y. Z. Huang, M. J. Edwards, E. Rounis, K. P. Bhatia and J. C. Rothwell, Neuron, 2005, 45, 201–206 CrossRef CAS PubMed.
- L. Cárdenas-Morales, D. A. Nowak, T. Kammer, R. C. Wolf and C. Schönfeldt-Lecuona, Brain Topogr., 2010, 22, 294–306 CrossRef PubMed.
- Z.-D. Deng, B. Luber, N. L. Balderston, M. Velez Afanador, M. M. Noh, J. Thomas, W. C. Altekruse, S. L. Exley, S. Awasthi and S. H. Lisanby, Annu. Rev. Pharmacol. Toxicol., 2020, 60, 591–614 CrossRef CAS PubMed.
- A. Stekic, M. Zeljkovic, M. Z. Kontic, K. Mihajlovic, M. Adzic, I. Stevanovic, M. Ninkovic, I. Grkovic, T. V. Ilic, N. Nedeljkovic and M. Dragic, Front. Aging Neurosci., 2022, 14, 889983 CrossRef CAS PubMed.
- N. Grossman, D. Bono, N. Dedic, S. B. Kodandaramaiah, A. Rudenko, H. J. Suk, A. M. Cassara, E. Neufeld, N. Kuster, L. H. Tsai, A. Pascual-Leone and E. S. Boyden, Cell, 2017, 169, 1029–1041 CrossRef CAS PubMed.
- S. Rao, R. Chen, A. A. LaRocca, M. G. Christiansen, A. W. Senko, C. H. Shi, P. H. Chiang, G. Varnavides, J. Xue, Y. Zhou, S. Park, R. Ding, J. Moon, G. Feng and P. Anikeeva, Nat. Nanotechnol., 2019, 14, 967–973 CrossRef CAS PubMed.
- Z. Esmaeilpour, G. Kronberg, D. Reato, L. C. Parra and M. Bikson, Brain Stimul., 2021, 14, 55–65 CrossRef PubMed.
- J. Park, A. Tabet, J. Moon, P. H. Chiang, F. Koehler, A. Sahasrabudhe and P. Anikeeva, Nano Lett., 2020, 20, 6535–6541 CrossRef CAS PubMed.
- R. Chen, G. Romero, M. G. Christiansen, A. Mohr and P. Anikeeva, Science, 2015, 347, 1477–1480 CrossRef CAS PubMed.
- H. Huang, S. Delikanli, H. Zeng, D. M. Ferkey and A. Pralle, Nat. Nanotechnol., 2010, 5, 602–606 CrossRef CAS PubMed.
- D. Rosenfeld, A. W. Senko, J. Moon, I. Yick, G. Varnavides, D. Gregureć, F. Koehler, P. H. Chiang, M. G. Christiansen, L. Y. Maeng, A. S. Widge and P. Anikeeva, Sci. Adv., 2020, 6, eaaz3734 CrossRef CAS PubMed.
- J. Moon, M. G. Christiansen, S. Rao, C. Marcus, D. C. Bono, D. Rosenfeld, D. Gregurec, G. Varnavides, P. H. Chiang, S. Park and P. Anikeeva, Adv. Funct. Mater., 2020, 30, 2000577 CrossRef CAS PubMed.
- D. Gregurec, A. W. Senko, A. Chuvilin, P. D. Reddy, A. Sankararaman, D. Rosenfeld, P. H. Chiang, F. Garcia, I. Tafel, G. Varnavides, E. Ciocan and P. Anikeeva, ACS Nano, 2020, 14, 8036–8045 CrossRef CAS PubMed.
- A. Fomenko, C. Neudorfer, R. F. Dallapiazza, S. K. Kalia and A. M. Lozano, Brain Stimul., 2018, 11, 1209–1217 CrossRef PubMed.
- K. Yu, X. Niu, E. Krook-Magnuson and B. He, Nat. Commun., 2021, 12, 2519 CrossRef CAS PubMed.
- B. P. Mead, N. Kim, G. W. Miller, D. Hodges, P. Mastorakos, A. L. Klibanov, J. W. Mandell, J. Hirsh, J. S. Suk, J. Hanes and R. J. Price, Nano Lett., 2017, 17, 3533–3542 CrossRef CAS PubMed.
- J. O. Szablowski, A. Lee-Gosselin, B. Lue, D. Malounda and M. G. Shapiro, Nat. Biomed. Eng., 2018, 2, 475–484 CrossRef CAS PubMed.
- A. N. Pouliopoulos, M. F. Murillo, R. L. Noel, A. J. Batts, R. Ji, N. Kwon, H. Yu, C.-K. Tong, J. N. Gelinas, D. K. Araghy, S. A. Hussaini and E. E. Konofagou, Brain Stimul., 2022, 15, 927–941 CrossRef PubMed.
- Y. Yang, C. P. Pacia, D. Ye, L. Zhu, H. Baek, Y. Yue, J. Yuan, M. J. Miller, J. Cui, J. P. Culver, M. R. Bruchas and H. Chen, Brain Stimul., 2021, 14, 790–800 CrossRef PubMed.
- V. Cotero, Y. Fan, T. Tsaava, A. M. Kressel, I. Hancu, P. Fitzgerald, K. Wallace, S. Kaanumalle, J. Graf, W. Rigby, T. J. Kao, J. Roberts, C. Bhushan, S. Joel, T. R. Coleman, S. Zanos, K. J. Tracey, J. Ashe, S. S. Chavan and C. Puleo, Nat. Commun., 2019, 10, 952 CrossRef CAS PubMed.
- T. Sato, M. G. Shapiro and D. Y. Tsao, Neuron, 2018, 98, 1031–1041 CrossRef CAS PubMed.
- M. S. Ozdas, A. S. Shah, P. M. Johnson, N. Patel, M. Marks, T. B. Yasar, U. Stalder, L. Bigler, W. von der Behrens, S. R. Sirsi and M. F. Yanik, Nat. Commun., 2020, 11, 4929 CrossRef CAS PubMed.
- A. Marino, G. G. Genchi, V. Mattoli and G. Ciofani, Nano Today, 2017, 14, 9–12 CrossRef CAS.
- G. Ciofani, S. Danti, D. D'Alessandro, L. Ricotti, S. Moscato, G. Bertoni, A. Falqui, S. Berrettini, M. Petrini, V. Mattoli and A. Menciassi, ACS Nano, 2010, 4, 6267–6277 CrossRef CAS PubMed.
- A. Marino, S. Arai, Y. Hou, E. Sinibaldi, M. Pellegrino, Y. T. Chang, B. Mazzolai, V. Mattoli, M. Suzuki and G. Ciofani, ACS Nano, 2015, 9, 7678–7689 CrossRef CAS PubMed.
- G. G. Genchi, L. Ceseracciu, A. Marino, M. Labardi, S. Marras, F. Pignatelli, L. Bruschini, V. Mattoli and G. Ciofani, Adv. Healthcare Mater., 2016, 5, 1808–1820 CrossRef CAS PubMed.
- D. Zhao, P. J. Feng, J. H. Liu, M. Dong, X. Q. Shen, Y. X. Chen and Q. D. Shen, Adv. Mater., 2020, 32, 2003800 CrossRef CAS PubMed.
- D. J. Lee, C. S. Lozano, R. F. Dallapiazza and A. M. Lozano, J. Neurosurg., 2019, 131, 333–342 CAS.
- C. E. Tedford, S. Delapp, S. Jacques and J. J. Anders, Lasers Surg. Med., 2015, 47, 312–322 CrossRef PubMed.
- P. A. Lapchak, P. D. Boitano, P. V. Butte, D. J. Fisher, T. Hölscher, E. J. Ley, M. Nuño, A. H. Voie and P. S. Rajput, PLoS One, 2015, 10, e0127580 CrossRef PubMed.
- F. Salehpour, J. Mahmoudi, F. Kamari, S. Sadigh-Eteghad, S. H. Rasta and M. R. Hamblin, Mol. Neurobiol., 2018, 55, 6601–6636 CrossRef CAS PubMed.
- A. Oron, U. Oron, J. Chen, A. Eilam, C. Zhang, M. Sadeh, Y. Lampl, J. Streeter, L. Detaboada and M. Chopp, Stroke, 2006, 37, 2620–2624 CrossRef PubMed.
- F. Reinhart, N. El Massri, F. Darlot, N. Torres, D. M. Johnstone, C. Chabrol, T. Costecalde, J. Stone, J. Mitrofanis, A. L. Benabid and C. Moro, Neurosci. Res., 2015, 92, 86–90 CrossRef PubMed.
- L. Yang, D. Tucker, Y. Dong, C. Wu, Y. Lu, Y. Li, J. Zhang, T. C. Y. Liu and Q. Zhang, Exp. Neurol., 2018, 299, 86–96 CrossRef CAS PubMed.
- J. Zhang, Y. He, S. Liang, X. Liao, T. Li, Z. Qiao, C. Chang, H. Jia and X. Chen, Nat. Commun., 2021, 12, 2730 CrossRef CAS PubMed.
- M. Plaksin, E. Shapira, E. Kimmel and S. Shoham, Phys. Rev. X, 2018, 8, 011043 CAS.
- X. Wu, Y. Jiang, N. J. Rommelfanger, F. Yang, Q. Zhou, R. Yin, J. Liu, S. Cai, W. Ren, A. Shin, K. S. Ong, K. Pu and G. Hong, Nat. Biomed. Eng., 2022, 6, 754–770 CrossRef CAS PubMed.
- W. H. Chen, T. Onoe and M. Kamimura, Nanoscale, 2022, 14, 2210–2220 RSC.
- P. Feyen, E. Colombo, D. Endeman, M. Nova, L. Laudato, N. Martino, M. R. Antognazza, G. Lanzani, F. Benfenati and D. Ghezzi, Sci. Rep., 2016, 6, 22718 CrossRef CAS PubMed.
- Y. Lampl, J. A. Zivin, M. Fisher, R. Lew, L. Welin, B. Dahlof, P. Borenstein, B. Andersson, J. Perez, C. Caparo, S. Ilic and U. Oron, Stroke, 2007, 38, 1843–1849 CrossRef PubMed.
- J. A. Zivin, G. W. Albers, N. Bornstein, T. Chippendale, B. Dahlof, T. Devlin, M. Fisher, W. Hacke, W. Holt, S. Ilic, S. Kasner, R. Lew, M. Nash, J. Perez, M. Rymer, P. Schellinger, D. Schneider, S. Schwab, R. Veltkamp, M. Walker and J. Streeter, Stroke, 2009, 40, 1359–1364 CrossRef PubMed.
- W. Hacke, P. D. Schellinger, G. W. Albers, N. M. Bornstein, B. L. Dahlof, R. Fulton, S. E. Kasner, A. Shuaib, S. P. Richieri, S. G. Dilly, J. Zivin, K. R. Lees, J. A. Zivin, J. Broderick, A. Ivanova, K. Johnston, B. Norrving, G. Albars, A. Alexandrov, D. Brown, P. Capone, D. Chiu, W. Clark, J. Cochran, C. Deredyn, T. Devlin, W. Hickling, G. Howell, D. Huang, S. Hussain, S. Mallenbaum, M. Moonis, M. Nash, M. Rymer, R. Taylor, M. Tremwel, B. Buck, J. Perez, C. Gerloff, B. Greiwing, M. Grond, G. Hamman, T. Haarmeiter, S. Jander, M. Köhrmann, M. Ritter, P. Schallinger, D. Schneider, J. Sobesky, T. Steiner, H. Steinmetz, R. Veltkamp, C. Weimar, F. Gruber, B. Andersson, L. Welin, D. Leys, T. Tatlisumak, A. Luft, P. Lyrer, P. Michel, C. Molina and T. Segura, Stroke, 2014, 45, 3187–3193 CrossRef PubMed.
- S. B. Gonçalves, J. F. Ribeiro, A. F. Silva, R. M. Costa and J. H. Correia, J. Neural Eng., 2017, 14, 041001 CrossRef PubMed.
- J. L. Carvalho-de-Souza, J. S. Treger, B. Dang, S. B. H. Kent, D. R. Pepperberg and F. Bezanilla, Neuron, 2015, 86, 207–217 CrossRef CAS PubMed.
- H. Kang, W. Hong, Y. An, S. Yoo, H. J. Kwon and Y. Nam, ACS Nano, 2020, 14, 11406–11419 CrossRef CAS PubMed.
- R. Damnjanovic, P. Bazard, R. D. Frisina and V. R. Bhethanabotla, ACS Nano, 2020, 14, 10917–10928 CrossRef CAS PubMed.
- X. Li, V. Vemireddy, Q. Cai, H. Xiong, P. Kang, X. Li, M. Giannotta, H. N. Hayenga, E. Pan, S. R. Sirsi, C. Mateo, D. Kleinfeld, C. Greene, M. Campbell, E. Dejana, R. Bachoo and Z. Qin, Nano Lett., 2021, 21, 9805–9815 CrossRef CAS PubMed.
- R. Xiong, D. Hua, J. Van Hoeck, D. Berdecka, L. Léger, S. De Munter, J. C. Fraire, L. Raes, A. Harizaj, F. Sauvage, G. Goetgeluk, M. Pille, J. Aalders, J. Belza, T. Van Acker, E. Bolea-Fernandez, T. Si, F. Vanhaecke, W. H. De Vos, B. Vandekerckhove, J. van Hengel, K. Raemdonck, C. Huang, S. C. De Smedt and K. Braeckmans, Nat. Nanotechnol., 2021, 16, 1281–1291 CrossRef CAS PubMed.
- M. M. Lino, S. Simões, A. Vilaça, H. Antunes, A. Zonari and L. Ferreira, ACS Nano, 2018, 12, 5207–5220 CrossRef CAS PubMed.
- M. M. Lino, S. Simões, S. Pinho and L. Ferreira, Nanoscale, 2017, 9, 18668–18680 RSC.
- H. Xiong, X. Li, P. Kang, J. Perish, F. Neuhaus, J. E. Ploski, S. Kroener, M. O. Ogunyankin, J. E. Shin, J. A. Zasadzinski, H. Wang, P. A. Slesinger, A. Zumbuehl and Z. Qin, Angew. Chem., Int. Ed., 2020, 59, 8608–8615 CrossRef CAS PubMed.
- Z. Al-Ahmady, N. Lozano, K. C. Mei, W. T. Al-Jamal and K. Kostarelos, Int. J. Pharm., 2016, 514, 133–141 CrossRef CAS PubMed.
- A. Y. Rwei, J. J. Lee, C. Zhan, Q. Liu, M. T. Ok, S. A. Shankarappa, R. Langer and D. S. Kohane, Proc. Natl. Acad. Sci. U. S. A., 2015, 112, 15719–15724 CrossRef CAS PubMed.
- M. M. Lino and L. Ferreira, Drug Discovery Today, 2018, 23, 1062–1070 CrossRef CAS PubMed.
- Y. Pan, J. Yang, X. Luan, X. Liu, X. Li, J. Yang, T. Huang, L. Sun, Y. Wang, Y. Lin and Y. Song, Sci. Adv., 2019, 5, eaav7199 CrossRef CAS PubMed.
- J. Morstein, G. Romano, B. E. Hetzler, A. Plante, C. Haake, J. Levitz and D. Trauner, Angew. Chem., Int. Ed., 2022, 61, e202117094 CrossRef CAS PubMed.
- C. Matera, P. Calvé, V. Casadó-Anguera, R. Sortino, A. M. J. Gomila, E. Moreno, T. Gener, C. Delgado-Sallent, P. Nebot, D. Costazza, S. Conde-Berriozabal, M. Masana, J. Hernando, V. Casadó, M. V. Puig and P. Gorostiza, Int. J. Mol. Sci., 2022, 23, 10114 CrossRef CAS PubMed.
- T. Fehrentz, F. M. E. Huber, N. Hartrampf, T. Bruegmann, J. A. Frank, N. H. F. Fine, D. Malan, J. G. Danzl, D. B. Tikhonov, M. Sumser, P. Sasse, D. J. Hodson, B. S. Zhorov, N. Klöcker and D. Trauner, Nat. Chem. Biol., 2018, 14, 764–767 CrossRef CAS PubMed.
- R. C. Sarott, A. E. G. Viray, P. Pfaff, A. Sadybekov, G. Rajic, V. Katritch, E. M. Carreira and J. A. Frank, J. Am. Chem. Soc., 2021, 143, 736–743 CrossRef CAS PubMed.
- M. L. DiFrancesco, F. Lodola, E. Colombo, L. Maragliano, M. Bramini, G. M. Paternò, P. Baldelli, M. D. Serra, L. Lunelli, M. Marchioretto, G. Grasselli, S. Cimò, L. Colella, D. Fazzi, F. Ortica, V. Vurro, C. G. Eleftheriou, D. Shmal, J. F. Maya-Vetencourt, C. Bertarelli, G. Lanzani and F. Benfenati, Nat. Nanotechnol., 2020, 15, 296–306 CrossRef CAS PubMed.
- G. M. Paternò, E. Colombo, V. Vurro, F. Lodola, S. Cimò, V. Sesti, E. Molotokaite, M. Bramini, L. Ganzer, D. Fazzi, C. D'Andrea, F. Benfenati, C. Bertarelli and G. Lanzani, Adv. Sci., 2020, 7, 1903241 CrossRef PubMed.
- F. Auzel, Chem. Rev., 2004, 104, 139–174 CrossRef CAS PubMed.
- H. Kang, K. Zhang, Q. Pan, S. Lin, D. S. H. Wong, J. Li, W. Y. W. Lee, B. Yang, F. Han, G. Li, B. Li and L. Bian, Adv. Funct. Mater., 2018, 28, 1802642 CrossRef.
- T. Santos, R. Ferreira, E. Quartin, C. Boto, C. Saraiva, J. Bragança, J. Peça, C. Rodrigues, L. Ferreira and L. Bernardino, Acta Biomater., 2017, 59, 293–302 CrossRef CAS PubMed.
- E. S. Boyden, F. Zhang, E. Bamberg, G. Nagel and K. Deisseroth, Nat. Neurosci., 2005, 8, 1263–1268 CrossRef CAS PubMed.
- B. R. Arenkiel, J. Peca, I. G. Davison, C. Feliciano, K. Deisseroth, G. J. J. Augustine, M. D. Ehlers and G. Feng, Neuron, 2007, 54, 205–218 CrossRef CAS PubMed.
- J. A. Steinbeck, S. J. Choi, A. Mrejeru, Y. Ganat, K. Deisseroth, D. Sulzer, E. V. Mosharov and L. Studer, Nat. Biotechnol., 2015, 33, 204–209 CrossRef CAS PubMed.
- M. Y. Cheng, E. H. Wang, W. J. Woodson, S. Wang, G. Sun, A. G. Lee, A. Arac, L. E. Fenno, K. Deisseroth and G. K. Steinberg, Proc. Natl. Acad. Sci. U. S. A., 2014, 111, 12913–12918 CrossRef CAS PubMed.
- K. A. Tennant, S. L. Taylor, E. R. White and C. E. Brown, Nat. Commun., 2017, 8, 15879 CrossRef CAS PubMed.
- J. A. Sahel, E. Boulanger-Scemama, C. Pagot, A. Arleo, F. Galluppi, J. N. Martel, S. D. Esposti, A. Delaux, J. B. de Saint Aubert, C. de Montleau, E. Gutman, I. Audo, J. Duebel, S. Picaud, D. Dalkara, L. Blouin, M. Taiel and B. Roska, Nat. Med., 2021, 27, 1223–1229 CrossRef CAS PubMed.
- A. M. Shah, S. Ishizaka, M. Y. Cheng, E. H. Wang, A. R. Bautista, S. Levy, D. Smerin, G. Sun and G. K. Steinberg, Sci. Rep., 2017, 7, 46612 CrossRef CAS PubMed.
- A. S. Wahl, U. Büchler, A. Brändli, B. Brattoli, S. Musall, H. Kasper, B. V. Ineichen, F. Helmchen, B. Ommer and M. E. Schwab, Nat. Commun., 2017, 8, 1187 CrossRef CAS PubMed.
- M. Song, S. P. Yu, O. Mohamad, W. Cao, Z. Z. Wei, X. Gu, M. Q. Jiang and L. Wei, Neurobiol. Dis., 2017, 98, 9–24 CrossRef CAS PubMed.
- B. Chen, M. Cui, Y. Wang, P. Shi, H. Wang and F. Wang, Adv. Drug Delivery Rev., 2022, 188, 114457 CrossRef CAS PubMed.
- L. Madisen, T. Mao, H. Koch, J. M. Zhuo, A. Berenyi, S. Fujisawa, Y. W. A. Hsu, A. J. Garcia, X. Gu, S. Zanella, J. Kidney, H. Gu, Y. Mao, B. M. Hooks, E. S. Boyden, G. Buzsáki, J. M. Ramirez, A. R. Jones, K. Svoboda, X. Han, E. E. Turner and H. Zeng, Nat. Neurosci., 2012, 15, 793–802 CrossRef CAS PubMed.
- H. Taniguchi, M. He, P. Wu, S. Kim, R. Paik, K. Sugino, D. Kvitsani, Y. Fu, J. Lu, Y. Lin, G. Miyoshi, Y. Shima, G. Fishell, S. B. Nelson and Z. J. Huang, Neuron, 2011, 71, 995–1013 CrossRef CAS PubMed.
- W. Zhang, A. W. Lohman, Y. Zhuravlova, X. Lu, M. D. Wiens, H. Hoi, S. Yaganoglu, M. A. Mohr, E. N. Kitova, J. S. Klassen, P. Pantazis, R. J. Thompson and R. E. Campbell, Nat. Methods, 2017, 14, 391–394 CrossRef CAS PubMed.
- X. Lin, X. Chen, W. Zhang, T. Sun, P. Fang, Q. Liao, X. Chen, J. He, M. Liu, F. Wang and P. Shi, Nano Lett., 2018, 18, 948–956 CrossRef CAS PubMed.
- S. Chen, A. Z. Weitemier, X. Zeng, L. He, X. Wang, Y. Tao, A. J. Y. Huang, Y. Hashimotodani, M. Kano, H. Iwasaki, L. K. Parajuli, S. Okabe, D. B. Loong Teh, A. H. All, I. Tsutsui-Kimura, K. F. Tanaka, X. Liu and T. J. McHugh, Science, 2018, 359, 679–684 CrossRef CAS PubMed.
- X. Lin, Y. Wang, X. Chen, R. Yang, Z. Wang, J. Feng, H. Wang, K. W. C. Lai, J. He, F. Wang and P. Shi, Adv. Healthcare Mater., 2017, 6, 1700446 CrossRef PubMed.
- X. Liu, H. Chen, Y. Wang, Y. Si, H. Zhang, X. Li, Z. Zhang, B. Yan, S. Jiang, F. Wang, S. Weng, W. Xu, D. Zhao, J. Zhang and F. Zhang, Nat. Commun., 2021, 12, 5662 CrossRef CAS PubMed.
- D. Ghezzi, M. R. Antognazza, R. MacCarone, S. Bellani, E. Lanzarini, N. Martino, M. Mete, G. Pertile, S. Bisti, G. Lanzani and F. Benfenati, Nat. Photonics, 2013, 7, 400–406 CrossRef CAS PubMed.
- S. F. Cogan, Annu. Rev. Biomed. Eng., 2008, 10, 275–309 CrossRef CAS PubMed.
- S. M. Won, E. Song, J. Zhao, J. Li, J. Rivnay and J. A. Rogers, Adv. Mater., 2018, 30, 1800534 CrossRef PubMed.
- A. Boutet, R. Madhavan, G. J. B. Elias, S. E. Joel, R. Gramer, M. Ranjan, V. Paramanandam, D. Xu, J. Germann, A. Loh, S. K. Kalia, M. Hodaie, B. Li, S. Prasad, A. Coblentz, R. P. Munhoz, J. Ashe, W. Kucharczyk, A. Fasano and A. M. Lozano, Nat. Commun., 2021, 12, 3043 CrossRef CAS PubMed.
- P. Ledochowitsch, A. Yazdan-Shahmorad, K. E. Bouchard, C. Diaz-Botia, T. L. Hanson, J. W. He, B. A. Seybold, E. Olivero, E. A. K. Phillips, T. J. Blanche, C. E. Schreiner, A. Hasenstaub, E. F. Chang, P. N. Sabes and M. M. Maharbiz, J. Neurosci. Methods, 2015, 256, 220–231 CrossRef CAS PubMed.
- S. P. Lacour, G. Courtine and J. Guck, Nat. Rev. Mater., 2016, 1, 16063 CrossRef CAS.
- J. A. Frank, M. J. Antonini and P. Anikeeva, Nat. Biotechnol., 2019, 37, 1013–1023 CrossRef CAS PubMed.
- M. Wang, G. Mi, D. Shi, N. Bassous, D. Hickey and T. J. Webster, Adv. Funct. Mater., 2018, 28, 1700905 CrossRef.
- H. Yuk, B. Lu, S. Lin, K. Qu, J. Xu, J. Luo and X. Zhao, Nat. Commun., 2020, 11, 1604 CrossRef CAS PubMed.
- A. T. Young, N. Cornwell and M. A. Daniele, Adv. Funct. Mater., 2018, 28, 1700239 CrossRef PubMed.
- S. Zhao, X. Liu, Z. Xu, H. Ren, B. Deng, M. Tang, L. Lu, X. Fu, H. Peng, Z. Liu and X. Duan, Nano Lett., 2016, 16, 7731–7738 CrossRef CAS PubMed.
- S. Zhao, G. Li, C. Tong, W. Chen, P. Wang, J. Dai, X. Fu, Z. Xu, X. Liu, L. Lu, Z. Liang and X. Duan, Nat. Commun., 2020, 11, 1788 CrossRef CAS PubMed.
- E. W. Keefer, B. R. Botterman, M. I. Romero, A. F. Rossi and G. W. Gross, Nat. Nanotechnol., 2008, 3, 434–439 CrossRef CAS PubMed.
- J. Zhang, X. Liu, W. Xu, W. Luo, M. Li, F. Chu, L. Xu, A. Cao, J. Guan, S. Tang and X. Duan, Nano Lett., 2018, 18, 2903–2911 CrossRef CAS PubMed.
- D. W. Park, A. A. Schendel, S. Mikael, S. K. Brodnick, T. J. Richner, J. P. Ness, M. R. Hayat, F. Atry, S. T. Frye, R. Pashaie, S. Thongpang, Z. Ma and J. C. Williams, Nat. Commun., 2014, 5, 5258 CrossRef CAS PubMed.
- K. Kostarelos, M. Vincent, C. Hebert and J. A. Garrido, Adv. Mater., 2017, 29, 1700909 CrossRef PubMed.
- E. Masvidal-Codina, X. Illa, M. Dasilva, A. B. Calia, T. Dragojević, E. E. Vidal-Rosas, E. Prats-Alfonso, J. Martínez-Aguilar, J. M. De la Cruz, R. Garcia-Cortadella, P. Godignon, G. Rius, A. Camassa, E. Del Corro, J. Bousquet, C. Hébert, T. Durduran, R. Villa, M. V. Sanchez-Vives, J. A. Garrido and A. Guimerà-Brunet, Nat. Mater., 2019, 18, 280–288 CrossRef CAS PubMed.
- A. Fabbro, D. Scaini, V. León, E. Vázquez, G. Cellot, G. Privitera, L. Lombardi, F. Torrisi, F. Tomarchio, F. Bonaccorso, S. Bosi, A. C. Ferrari, L. Ballerini and M. Prato, ACS Nano, 2016, 10, 615–623 CrossRef CAS PubMed.
- N. P. Pampaloni, M. Lottner, M. Giugliano, A. Matruglio, F. D'Amico, M. Prato, J. A. Garrido, L. Ballerini and D. Scaini, Nat. Nanotechnol., 2018, 13, 755–764 CrossRef CAS PubMed.
- A. F. Rodrigues, L. Newman, D. A. Jasim, I. A. Vacchi, C. Ménard-Moyon, L. E. Crica, A. Bianco, K. Kostarelos and C. Bussy, Arch. Toxicol., 2018, 92, 3359–3379 CrossRef CAS PubMed.
- A. Savchenko, V. Cherkas, C. Liu, G. B. Braun, A. Kleschevnikov, Y. I. Miller and E. Molokanova, Sci. Adv., 2018, 4, eaat0351 CrossRef PubMed.
- K. Yang, L. Feng, X. Shi and Z. Liu, Chem. Soc. Rev., 2013, 42, 530–547 RSC.
- K. J. Tielrooij, L. Piatkowski, M. Massicotte, A. Woessner, Q. Ma, Y. Lee, K. S. Myhro, C. N. Lau, P. Jarillo-Herrero, N. F. Van Hulst and F. H. L. Koppens, Nat. Nanotechnol., 2015, 10, 437–443 CrossRef CAS PubMed.
- H. B. Balch, A. F. McGuire, J. Horng, H. Z. Tsai, K. K. Qi, Y. S. Duh, P. R. Forrester, M. F. Crommie, B. Cui and F. Wang, Nano Lett., 2021, 21, 4944–4949 CrossRef CAS PubMed.
- E. A. A. Pogna, X. Jia, A. Principi, A. Block, L. Banszerus, J. Zhang, X. Liu, T. Sohier, S. Forti, K. Soundarapandian, B. Terrés, J. D. Mehew, C. Trovatello, C. Coletti, F. H. L. Koppens, M. Bonn, H. I. Wang, N. Van Hulst, M. J. Verstraete, H. Peng, Z. Liu, C. Stampfer, G. Cerullo and K. J. Tielrooij, ACS Nano, 2021, 15, 11285–11295 CrossRef CAS PubMed.
- Z. Zhang, R. Xu, Z. Wang, M. Dong, B. Cui and M. Chen, ACS Appl. Mater. Interfaces, 2017, 9, 34736–34743 CrossRef CAS PubMed.
- D. Rand, M. Jakešová, G. Lubin, I. Vėbraitė, M. David-Pur, V. Đerek, T. Cramer, N. S. Sariciftci, Y. Hanein and E. D. Głowacki, Adv. Mater., 2018, 30, 1707292 CrossRef PubMed.
- O. S. Abdullaeva, M. Schulz, F. Balzer, J. Parisi, A. Lützen, K. Dedek and M. Schiek, Langmuir, 2016, 32, 8533–8542 CrossRef CAS PubMed.
- M. Jakešová, M. Silverå Ejneby, V. Đerek, T. Schmidt, M. Gryszel, J. Brask, R. Schindl, D. T. Simon, M. Berggren, F. Elinder and E. D. Głowacki, Sci. Adv., 2019, 5, eaav5265 CrossRef PubMed.
- T. C. Pappas, W. M. S. Wickramanyake, E. Jan, M. Motamedi, M. Brodwick and N. A. Kotov, Nano Lett., 2007, 7, 513–519 CrossRef CAS PubMed.
- L. Bareket, N. Waiskopf, D. Rand, G. Lubin, M. David-Pur, J. Ben-Dov, S. Roy, C. Eleftheriou, E. Sernagor, O. Cheshnovsky, U. Banin and Y. Hanein, Nano Lett., 2014, 14, 6685–6692 CrossRef CAS PubMed.
- N. Martino, P. Feyen, M. Porro, C. Bossio, E. Zucchetti, D. Ghezzi, F. Benfenati, G. Lanzani and M. R. Antognazza, Sci. Rep., 2015, 5, 8911 CrossRef CAS PubMed.
- J. F. Maya-Vetencourt, D. Ghezzi, M. R. Antognazza, E. Colombo, M. Mete, P. Feyen, A. Desii, A. Buschiazzo, M. Di Paolo, S. Di Marco, F. Ticconi, L. Emionite, D. Shmal, C. Marini, I. Donelli, G. Freddi, R. MacCarone, S. Bisti, G. Sambuceti, G. Pertile, G. Lanzani and F. Benfenati, Nat. Mater., 2017, 16, 681–689 CrossRef CAS PubMed.
- M. R. Antognazza, M. Di Paolo, D. Ghezzi, M. Mete, S. Di Marco, J. F. Maya-Vetencourt, R. Maccarone, A. Desii, F. Di Fonzo, M. Bramini, A. Russo, L. Laudato, I. Donelli, M. Cilli, G. Freddi, G. Pertile, G. Lanzani, S. Bisti and F. Benfenati, Adv. Healthcare Mater., 2016, 5, 2271–2282 CrossRef CAS PubMed.
- J. F. Maya-Vetencourt, G. Manfredi, M. Mete, E. Colombo, M. Bramini, S. Di Marco, D. Shmal, G. Mantero, M. Dipalo, A. Rocchi, M. L. DiFrancesco, E. D. Papaleo, A. Russo, J. Barsotti, C. Eleftheriou, F. Di Maria, V. Cossu, F. Piazza, L. Emionite, F. Ticconi, C. Marini, G. Sambuceti, G. Pertile, G. Lanzani and F. Benfenati, Nat. Nanotechnol., 2020, 15, 698–708 CrossRef CAS PubMed.
- M. L. DiFrancesco, E. Colombo, E. D. Papaleo, G. Manfredi, G. Lanzani and F. Benfenati, Carbon, 2020, 162, 308–317 CrossRef CAS.
- M. K. Thakur, A. Gupta, M. Y. Fakhri, R. S. Chen, C. T. Wu, K. H. Lin and S. Chattopadhyay, Nanoscale, 2019, 11, 9716–9725 RSC.
- M. Kataria, K. Yadav, G. Haider, Y. M. Liao, Y. R. Liou, S. Y. Cai, H. I. Lin, Y. H. Chen, C. R. Paul Inbaraj, K. P. Bera, H. M. Lee, Y. T. Chen, W. H. Wang and Y. F. Chen, ACS Photonics, 2018, 5, 2336–2347 CrossRef CAS.
- M. Kataria, K. Yadav, S. Y. Cai, Y. M. Liao, H. I. Lin, T. L. Shen, Y. H. Chen, Y. T. Chen, W. H. Wang and Y. F. Chen, ACS Nano, 2018, 12, 9596–9607 CrossRef CAS PubMed.
- A. F. Rodrigues, A. P. M. Tavares, S. Simões, R. P. F. F. Silva, T. Sobrino, B. R. Figueiredo, G. Sales and L. Ferreira, Nanoscale, 2023, 15, 687–706 RSC.
- R. Parameswaran, J. L. Carvalho-De-Souza, Y. Jiang, M. J. Burke, J. F. Zimmerman, K. Koehler, A. W. Phillips, J. Yi, E. J. Adams, F. Bezanilla and B. Tian, Nat. Nanotechnol., 2018, 13, 260–266 CrossRef CAS PubMed.
- Y. Jiang, X. Li, B. Liu, J. Yi, Y. Fang, F. Shi, X. Gao, E. Sudzilovsky, R. Parameswaran, K. Koehler, V. Nair, J. Yue, K. H. Guo, Y. Fang, H. M. Tsai, G. Freyermuth, R. C. S. Wong, C. M. Kao, C. T. Chen, A. W. Nicholls, X. Wu, G. M. G. Shepherd and B. Tian, Nat. Biomed. Eng., 2018, 2, 508–521 CrossRef CAS PubMed.
- A. Thalhammer, M. Fontanini, J. Shi, D. Scaini, L. Recupero, A. Evtushenko, Y. Fu, S. Pavagada, A. Bistrovic-Popov, L. Fruk, B. Tian and L. Ballerini, Sci. Adv., 2022, 8, eabp9257 CrossRef CAS PubMed.
- L. Yin, W. Huang, R. Xiao, W. Peng, Y. Zhu, Y. Zhang, X. Pi and D. Yang, Nano Lett., 2020, 20, 3378–3387 CrossRef CAS PubMed.
- N. C. Pégard, A. R. Mardinly, I. A. Oldenburg, S. Sridharan, L. Waller and H. Adesnik, Nat. Commun., 2017, 8, 1228 CrossRef PubMed.
- J. Guo, Y. Wu, Z. Gong, X. Chen, F. Cao, S. Kala, Z. Qiu, X. Zhao, J. jiang Chen, D. He, T. Chen, R. Zeng, J. Zhu, K. F. Wong, S. Murugappan, T. Zhu, Q. Xian, X. Hou, Y. C. Ruan, B. Li, Y. C. Li, Y. Zhang and L. Sun, Adv. Sci., 2022, 9, 2104140 CrossRef CAS PubMed.
- Y. Ziv, H. Avidan, S. Pluchino, G. Martino and M. Schwartz, Proc. Natl. Acad. Sci. U. S. A., 2006, 103, 13174–13179 CrossRef CAS PubMed.
- F. Zhang, Y. A. Lin, S. Kannan and R. M. Kannan, J. Controlled Release, 2016, 240, 212–226 CrossRef CAS PubMed.
- Z. G. Zhang, B. Buller and M. Chopp, Nat. Rev. Neurol., 2019, 15, 193–203 CrossRef PubMed.
- H. Henriques-Antunes, R. M. S. Cardoso, A. Zonari, J. Correia, E. C. Leal, A. Jiménez-Balsa, M. M. Lino, A. Barradas, I. Kostic, C. Gomes, J. M. Karp, E. Carvalho and L. Ferreira, ACS Nano, 2019, 13, 8694–8707 CrossRef CAS PubMed.
- M. C. S. Boshuizen and G. K. Steinberg, Stroke, 2018, 49, 1563–1570 CrossRef PubMed.
- J. M. Castellano, K. I. Mosher, R. J. Abbey, A. A. McBride, M. L. James, D. Berdnik, J. C. Shen, B. Zou, X. S. Xie, M. Tingle, I. V. Hinkson, M. S. Angst and T. Wyss-Coray, Nature, 2017, 544, 488–492 CrossRef CAS PubMed.
- M. M. Lino, S. Simões, F. Tomatis, I. Albino, A. Barrera, D. Vivien, T. Sobrino and L. Ferreira, J. Controlled Release, 2021, 338, 472–485 CrossRef CAS PubMed.
- M. Esteves, R. Abreu, H. Fernandes, C. Serra-Almeida, P. A. T. Martins, M. Barão, A. C. Cristóvão, C. Saraiva, R. Ferreira, L. Ferreira and L. Bernardino, Mol. Ther., 2022, 30, 3176–3192 CrossRef CAS PubMed.
- N. Perets, O. Betzer, R. Shapira, S. Brenstein, A. Angel, T. Sadan, U. Ashery, R. Popovtzer and D. Offen, Nano Lett., 2019, 19, 3422–3431 CrossRef CAS PubMed.
- L. Estronca, V. Francisco, P. Pitrez, I. Honório, L. Carvalho, H. Vazão, J. Blersch, A. Rai, X. Nissan, U. Simon, M. Grãos, L. Saúde and L. Ferreira, Nanoscale Horiz., 2021, 6, 245–259 RSC.
- K. Ashkan, P. Rogers, H. Bergman and I. Ughratdar, Nat. Rev. Neurol., 2017, 13, 548–554 CrossRef PubMed.
- S. S. Srinivasan, B. E. Maimon, M. Diaz, H. Song and H. M. Herr, Nat. Commun., 2018, 9, 5303 CrossRef CAS PubMed.
- I. E. Harmsen, G. J. B. Elias, M. E. Beyn, A. Boutet, A. Pancholi, J. Germann, A. Mansouri, C. S. Lozano and A. M. Lozano, Brain Stimul., 2020, 13, 378–385 CrossRef PubMed.
- C. M. Tringides, N. Vachicouras, I. de Lázaro, H. Wang, A. Trouillet, B. R. Seo, A. Elosegui-Artola, F. Fallegger, Y. Shin, C. Casiraghi, K. Kostarelos, S. P. Lacour and D. J. Mooney, Nat. Nanotechnol., 2021, 16, 1019–1029 CrossRef CAS PubMed.
- G. Hong and C. M. Lieber, Nat. Rev. Neurosci., 2019, 20, 330–345 CrossRef CAS PubMed.
- X. Yu, W. Shou, B. K. Mahajan, X. Huang and H. Pan, Adv. Mater., 2018, 30, 1707624 CrossRef PubMed.
- J. B. Price, A. E. Rusheen, A. S. Barath, J. M. Rojas Cabrera, H. Shin, S. Y. Chang, C. J. Kimble, K. E. Bennet, C. D. Blaha, K. H. Lee and Y. Oh, Neurosurg. Focus, 2020, 49, E6 Search PubMed.
- R. Qazi, A. M. Gomez, D. C. Castro, Z. Zou, J. Y. Sim, Y. Xiong, J. Abdo, C. Y. Kim, A. Anderson, F. Lohner, S. H. Byun, B. Chul Lee, K. I. Jang, J. Xiao, M. R. Bruchas and J. W. Jeong, Nat. Biomed. Eng., 2019, 3, 655–669 CrossRef PubMed.
- H. Sun, D. Yu, Y. Guan, Z. Du, J. Ren and X. Qu, Chem. Commun., 2019, 55, 9833–9836 RSC.
- A. Kamyshny and S. Magdassi, Chem. Soc. Rev., 2019, 48, 1712–1740 RSC.
|
This journal is © The Royal Society of Chemistry 2023 |
Click here to see how this site uses Cookies. View our privacy policy here.