DOI:
10.1039/D1QO01088C
(Research Article)
Org. Chem. Front., 2021,
8, 5793-5803
Taming the reactivity of alkyl azides by intramolecular hydrogen bonding: site-selective conjugation of unhindered diazides†
Received
25th July 2021
, Accepted 11th August 2021
First published on 14th August 2021
Abstract
Organic azides are still in the center of click chemistry connecting two molecules. However, taming the conjugation selectivity of azides is difficult without the help of bulky groups. We report herein the unique reactivities of α-azido secondary acetamides (α-AzSAs) as minimal and unhindered azide structures. The NH–azide interaction in the α-AzSAs, supposed by DFT calculations, allowed selective conjugation in the presence of other azido moieties, even without steric hindrance. With Staudinger–Bertozzi ligation, α-AzSAs underwent conjugation prior to the other primary alkyl azides. On the other hand, in propargyl cation-mediated triazole synthesis, other alkyl azides, including tertiary alkyl azides, underwent the conjugation faster than α-AzSAs. We also demonstrated site-selective integration of the functional components onto the diazide modular hubs.
Introduction
In a broad range of scientific areas, including chemical biology and polymer synthesis,1,2 click chemistry3 represented by organic azides4 has received much attention, and it involves conjugation of two molecules concisely. Beyond this established one-on-one conjugation,5 a multi-click modular hub strategy can integrate multiple compounds onto one scaffold molecule (Fig. 1).6 Owing to the high reactivity with sufficient stability and small steric influence, multi-azides, compounds possessing multiple azido groups, have sparked interest in click scaffolds of multicomponent integration. In addition, multi-azides are easily accessible multi-click substrates, for example, by late-stage global azidation and polymerization of monoazides.7,8 For these reasons, multi-azides could serve as so-called functionalized element-block materials9 such as cross-linking, energetic, and Janus-type polymers in polymer chemistry,10,11 chemical probes, and pharmaceuticals in chemical biology and life sciences.12,13 However, although global azide-click conjugation of the same components has been well-documented, site-specific conjugation remains limited in multicomponent integration.14,15 In particular, similar reactivities among alkyl azides lead to difficulty in site-specificity.
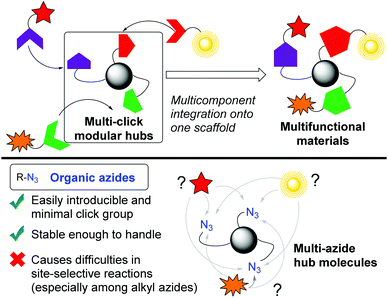 |
| Fig. 1 The multi-click modular hub strategy toward multifunctional materials, and issues of multi-azides as modular hubs. | |
For discrimination of each azido position in multi-azides, suitable molecular structures have been studied (Fig. 2). Along with the different characters between alkyl and aryl (alkenyl) azides,14,16 steric influence,17,18 metal coordination,19,20 and electron-poor aryl groups21 are often utilized along with a recently developed azide-protecting strategy.22 However, discrimination of the azides mostly relies on the bulky substituents such as aromatic rings and tert-alkyl groups, and these could negatively impact the physiochemical properties and dynamics of the materials.23,24 Thus, a new azide-discrimination strategy which does not require the help of bulky substituents should be investigated.
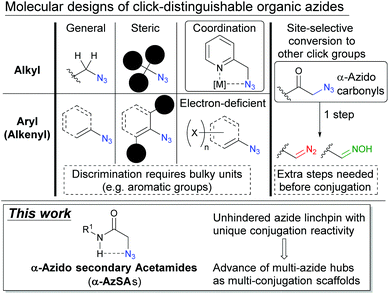 |
| Fig. 2 Molecular designs of distinguishable organic azides toward multicomponent integration. | |
Focusing on multi-azide chemistry, we recently reported the site-selective conversion of azido groups at carbonyl α-positions to diazo or oxime click groups with the retention of other azide moieties and one-pot multi-component conjugation onto the triple-click scaffold converted from the tris(alkyl azide) compound.15,25 Although our methods allow distinguishing multiple alkyl azido groups, the extra conversion step is undesired for conjugation. Inspired by metal-coordination19 and the α-azido carbonyl strategy,15 we envisioned that the intramolecular azide–NH interaction in α-azido secondary acetamides (henceforth simply “α-AzSAs”)26,27 could lead to unique reactivity without bulky substituents. Herein, we report α-AzSAs as minimal and unhindered azido units, which allow selective conjugation in the presence of other organic azides. We also showcase the site-selective integration of the functional components onto the diazide modular hubs.
Results and discussion
In general, unlike those of alkyl azides, electrophilic addition reactions of aryl (alkenyl) azides are favored because of the stabilized triazene intermediates (Fig. 3).28 In contrast, nucleophilic reactions with aryl (alkenyl) azides are suppressed due to the low nucleophilicity caused by the delocalization. We hypothesized that intramolecular hydrogen bonding29 in α-AzSAs could change the reactivity of alkyl azides. In other words, by the hydrogen interaction, α-AzSAs could be supposed to promote electrophilic reactions,30,31 but suppress nucleophilic reactions. Although α-AzSAs, also described as secondary amides of azidoglycine, are general in click chemistry, their specificity has not been mentioned to the best of our knowledge. To evaluate the characteristics of α-AzSAs on the selective reaction in the presence of other alkyl azides, we chose the Staudinger–Bertozzi ligation reaction for the electrophilic reaction of azides.32 For the nucleophilic reaction, we have developed propargyl cation-mediated rapid triazole synthesis through the nucleophilic addition of alkyl azides followed by cyclization.33 Thus, we chose this method.
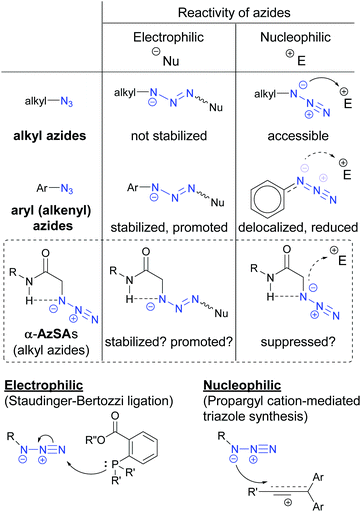 |
| Fig. 3 General reactivity of organic azides and working hypothesis on α-AzSAs. | |
Prior to the synthesis, we began our study using DFT calculations to prove our hypothesis shown in Fig. 3 (Table 1, see also the ESI†).34,35 From the obtained stable conformations, the direction of the C–N3 bonds of the ketone, ester, and secondary amide of α-azido carbonyl compounds 1b–d is in the s-trans conformation. In contrast, tertiary amide 4 is an eclipsed conformation for its steric repulsion between azido and N-methyl groups. Alongside these s-trans conformations, we found that the charge density on the N1 atom of the azido group in α-AzSA 1e increased compared to those of other compounds, especially among the amides. In the case of its conformers (1e′ and 1e′′), the charge distribution value on the N1 atom of non-s-trans1e′ is much decreased, whereas s-trans1e′′ retains a similar value. These suggest an interaction between the N1 atom in the azide group and the N-hydrogen atom in the amide group.26 Propanamide of α-AzSA 1f shows a similar stable conformation with an increased value on N1. Because a positive interaction with the dipolar azido group is unlikely, the NH–N1 interaction would be observed by the dipolar repulsion-induced stable s-trans conformation of the α-AzSA structure. Indeed, α-difluoroazidoacetamide 1g, which is known to be isolable,36 is s-trans between carbonyl and fluoride groups. Neither 1h with azidoalkyl side chains nor β-AzSA 1i shows any NH–N1 interaction. Unlike amides, sulfonamide 1j does not show specific interactions due to the loss of planarity.37 These results suggest the interaction between NH and the azide group, which influences the electronic situation of the azido group, and prompted us to use α-AzSAs as uniquely clickable azides.
Table 1 Calculated stable conformations of organic azides and charge distribution on their azido groupsa
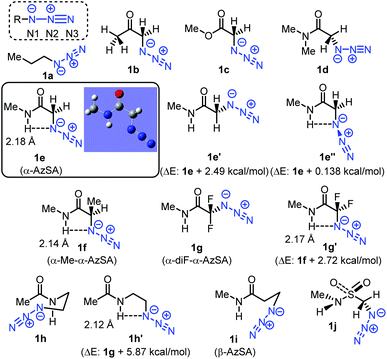
|
Entry |
Compounds/conformations |
Mulliken charge distribution (a.u.) |
N1 |
N2 |
N3 |
The DFT calculations performed with the Gaussian09 suite of programs using the dispersion-corrected B3LYP-D3 density functional with the 6-311G** basis set.
|
1 |
1a
|
−0.284 |
+0.253 |
−0.167 |
2 |
1b
|
−0.310 |
+0.258 |
−0.151 |
3 |
1c
|
−0.268 |
+0.259 |
−0.154 |
4 |
1d
|
−0.295 |
+0.270 |
−0.140 |
5 |
1e
|
−0.333 |
+0.256 |
−0.145 |
6 |
1e′
|
−0.275 |
+0.260 |
−0.145 |
7 |
1e′′
|
−0.312 |
+0.265 |
−0.144 |
8 |
1f
|
−0.329 |
+0.266 |
−0.147 |
9 |
1g
|
−0.292 |
+0.285 |
−0.093 |
10 |
1g′
|
−0.351 |
+0.274 |
−0.086 |
11 |
1h
|
−0.261 |
+0.255 |
−0.168 |
12 |
1h′
|
−0.319 |
+0.253 |
−0.148 |
13 |
1i
|
−0.272 |
+0.251 |
−0.184 |
14 |
1j
|
−0.261 |
+0.270 |
−0.129 |
We turned to a feasibility study by performing both electrophilic and nucleophilic reactions of various azides under competition with a general alkyl azide. First, we examined the Staudinger–Bertozzi ligation reaction with 2a as an electrophilic reaction (Scheme 1).32 Because the addition of phosphines to the organic azides is a reversible step, stabilization of phosphazide intermediates can improve the reaction progress. In the case of aryl azides of this reaction, stabilization of phosphazide from the aryl azides by hydrogen bonding with NH of the amide has been demonstrated.38 With α-AzSAs of alkyl azides, as expected, ligation products 4b–e from α-AzSAs 3b–e were obtained almost predominantly (nearly >20
:
1 ratio) in excellent yields, even under competition with 3-phenylpropyl azide 3a. α-AzSA 3f of the secondary alkyl azide only showed moderate selectivity due to the steric influence at the stage of aza-ylide formation in the Staudinger reaction.30,31 The low selectivity of 3g with a β-azido group and 3h with an azidoalkyl side chain39 revealed the importance of azide positioning. Although the values are variable, the downfield chemical shifts of the N–H in 1H NMR26e,34,35,40 compared to those without the azido group would also suggest the hydrogen bonding of α-AzSAs. Despite the same α-azidocarbonyl structures, the tertiary amide, ester, and ketone 3i–k gave 4i–k with only moderate selectivity. Benzyl azide 3l did not show specific selectivity.
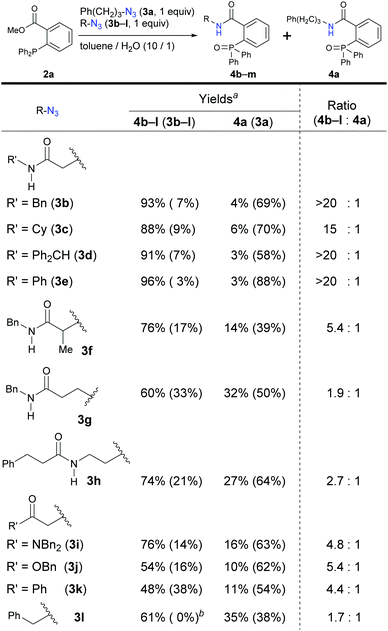 |
| Scheme 1 Competitive Staudinger–Bertozzi ligations (0.1 mmol scale). a Yield determined by 1H NMR. b Not observed due to the volatility. | |
Because aryl azides have been known to exhibit strong reactivity in the Staudinger reaction or ligation, we also examined the competitive reactions with α-AzSA 3b and aryl azides 3m and 3n (Scheme 2). Interestingly, α-AzSA 3b also produced the corresponding compounds with excellent selectivity rather than aryl azide 3m. α-AzSA 3b also underwent the Staudinger ligation prior to the sterically hindered but uniquely reactive aryl azide 3n.18 In addition, this selectivity was also observed in traceless Staudinger ligation.41
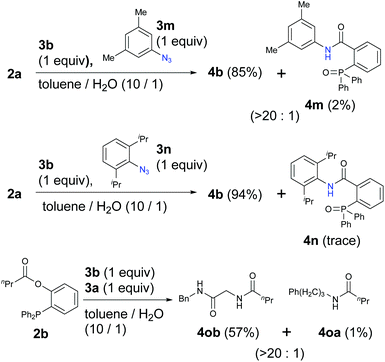 |
| Scheme 2 Competitive Staudinger–Bertozzi ligation with aryl azides and application to the traceless reaction (0.1 mmol scale; yield determined by 1H NMR). | |
Encouraged by the positive reactivity of the electrophilic behaviors, we moved to evaluate the nucleophilic characteristics of α-AzSAs by our developed propargyl cation-mediated triazole synthesis shown in Fig. 3 (Scheme 3).33 With propargyl alcohol 5 and alkyl azide 3a, we examined the competitive reaction followed by aqueous quenching for the introduction of the hydroxy group. As expected, the reactivities of N-benzyl and N-cyclohexyl α-AzSAs 3b and 3c were very low compared to that of 3a, and most of the starting α-AzSAs were recovered. On the other hand, 3a was converted to triazole 6a in excellent yields. The observed excellent selectivity (1
:
>20 ratio) was inverse to that of Staudinger–Bertozzi ligation (Schemes 1 and 2). 3d with a bulky side chain showed moderate selectivity, but the selectivity was improved in toluene. Unexpectedly, N-phenyl α-AzSA 3e did not show selectivity in dichloromethane, and the reaction suppression by toluene solvent was not satisfactory. Secondary alkyl α-AzSA 3f also exhibited good selectivity (1
:
17), whereas β-AzSA 3g or N-azidoalkyl amide 3h did not. The selectivities of the tertiary amide, ester, ketone, and benzyl azides 3i–l were moderate or not observed. This reaction strongly depends on the nucleophilicity of azido groups. Thus, general aryl azides did not afford the products because of the lack of nucleophilicity by the delocalization (Fig. 3).33a For this reason, we did not test aryl azides in this reaction.
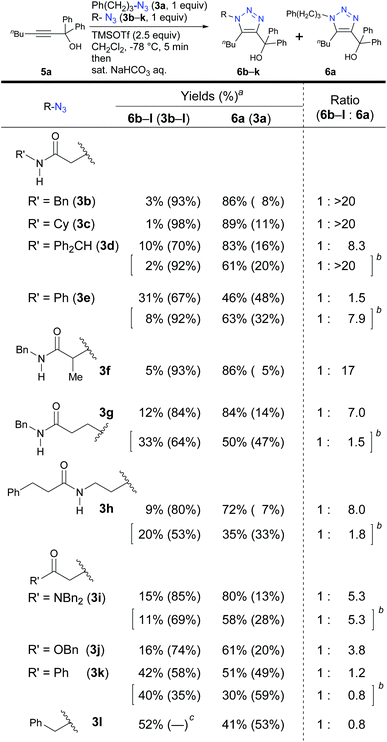 |
| Scheme 3 Competitive propargyl cation-mediated triazole formation reactions with propargyl alcohol 5a (0.1 mmol scale). a Yield determined by 1H NMR. b Reaction in toluene. c Not determined due to the volatility. | |
The specificity of α-AzSAs was also demonstrated with bulky tertiary alkyl azide 3o (Scheme 4). While the reaction with 3a and 3o gave less-hindered 6a from 3a as a major product, bulky 6o from 3o was obtained as a major product under competition with primary alkyl α-AzSA 3b. These results indicate that the α-AzSA skeleton is a primary alkyl azide that can exhibit high selectivity by both promoting electrophilic reactions and inhibiting nucleophilic reactions.
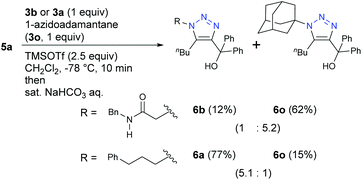 |
| Scheme 4 Competitive propargyl cation-mediated triazole formation reactions with azidoadamantane of bulky tert-alkyl azide (0.1 mmol scale; isolated yields). | |
However, unlike the tested stepwise reactions, strain-promoted azide–alkyne cyclization (SPAAC) of pericyclic reaction42 with 7 showed no selectivity (Scheme 5). This result indicates that the azido groups in α-AzSAs retain the same 1,3-dipolar reactivity as general alkyl azides. Indeed, the reaction with 3a and a bulky 3n gave 8n in a similar ratio to the reported values.18a,b On the other hand, very recently, Raines and co-workers reported the SPAAC with the novel aza-dibenzocyclooctyne.26e Although competitive reactions were not examined, the reaction rate constants showed the fast SPAAC reaction of α-AzSA 3b compared to other alkyl azides. The inter- and intramolecular hydrogen bonding of 3b with the alkyne are also suggested in the transition state. Thus, the azide-hydrogen-bonding-assisted selective conjugation approach should also work in the pericyclic reaction by developing the molecular design of azidophiles.
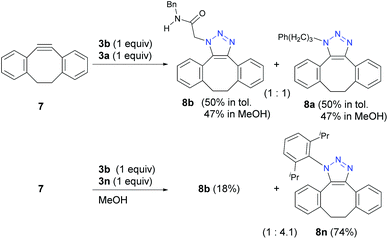 |
| Scheme 5 Strain-promoted azide-alkyne cycloaddition (SPAAC) reactions (0.1 mmol scale; yield determined by 1H NMR except for 8n (isolated yield)). | |
Having identified the unique reactivities of α-AzSAs, we examined the site-selective conjugation of diazides containing an α-AzSA structure (Scheme 6). For a diazide of aryl and α-AzSA 9a, Staudinger–Bertozzi ligation occurred at the α-AzSA moiety selectively. With a 2,6-dichloro azido benzene unit forming stable aza-ylides,21e the one-pot double Staudinger reaction also successfully gave 10ac. Next, bis(alkylazido) compounds, which face difficulty in undergoing site-selective conjugation, were investigated. α-AzSA-selective ligation of 9b was accomplished in 70% yield with 11% of overreacted 10bb. On the other hand, alkyl azide-selective triazole synthesis was achieved to give 10bc without the overreaction byproduct, although the azide close to tert-amide was also unreactive. With 9c consisting of primary and tertiary alkyl azides, SPAAC42 occurred only at the α-AzSA moiety owing to the steric hindrance. Nevertheless, by our method,33 we could reverse this selectivity to obtain 10cb of the bulky azide-reacted triazole in 43% yield with the recovered 9c in 47% yield. Longer reaction time led to decomposition of 9c and 10cc by the generation of the tertiary carbocation. Although not perfect, we demonstrated a way to the prior use of the sterically hindered azide even in the presence of unmasked and unhindered azides. In all cases, one-on-one adducts at the opposite azide positions were not observed.
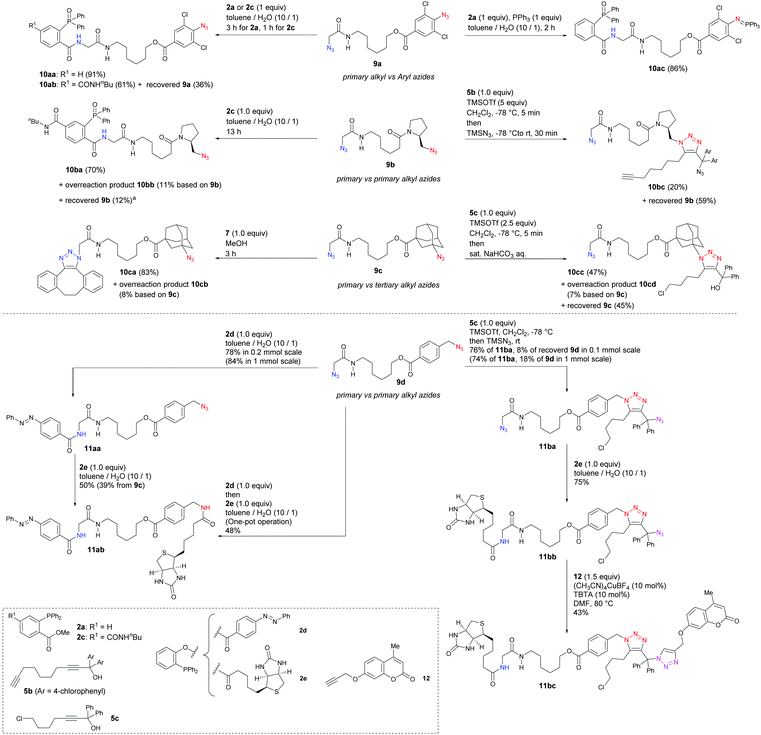 |
| Scheme 6 Site-selective use of azido groups in α-AzSA-containing diazides. Isolated yield except for recovered 9b (1H NMR yield) in the reaction from 9b to 10ba due to the difficulty of purification. TBTA: tris[(1-benzyl-1H-1,2,3-triazol-4-yl)methyl]amine. | |
Finally, we sought to showcase the site-selective conjugation of functional groups onto the bis(primary alkyl azide) compound 9d (Scheme 6). The traceless Staudinger ligation41 achieved the prior use of the α-AzSA moiety to attach the fluorescent azobenzene moiety to give 11aa followed by the conjugation at the benzylic position with biotin 2e. The conjugation from 9d to 11ab was also successful in one pot. In contrast, selective conjugation at the benzylic azide was demonstrated by three-component coupling with chloroalkyl propargyl alcohol 5c followed by azidation33a,b to give diazide 11ba. The first steps of each selective conjugation reaction were also successful on large scale (1 mmol). To the less-hindered α-AzSA moiety in 11ba, 2e was attached selectively. Lastly, CuAAC of the highly hindered triarylmethyl azide16b,43,44 in 11bb with the propargyl ether of the fluorescent unit 12 was accomplished to afford 11bc.
Conclusions
In summary, we reported the unique reactivities of the α-AzSA structure as a minimal and unhindered azido unit. The amide–NH–azide interaction in the α-AzSA, supposed by DFT calculations, allowed selective conjugation in the presence of other organic azides. With Staudinger–Bertozzi ligation, α-AzSAs could conjugate prior to the other primary alkyl azides. On the other hand, in the case of propargyl cation-mediated triazole synthesis we have developed, α-AzSAs remained inert, and other alkyl azides, including even tertiary alkyl azides, underwent the conjugation. We also demonstrated site-selective integration of the functional components onto the diazide modular hubs. The unique characteristics of α-AzSAs44 would open a new methodology of discriminative azide click reaction free from bulky substituents. We also believe that this work could help develop multifunctional chemical probes and polymer materials. Further research based on this strategy is currently underway in our group.
Author contributions
KM and SO performed the synthetic experiments and collected the analytical data. HT conceptualized this project, performed the computational study, checked the collected analytical data and performed supervision. TT (molecular design for chemical biology), TM (synthesis), and KK (photochemical analysis) contributed to the discussion on this project, especially from the viewpoint of each research area. The first draft was written by HT, KM, and SO, and all authors contributed to the review.
Conflicts of interest
There are no conflicts to declare.
Acknowledgements
This work was supported in part by Iketani Science and Technology Foundation (0321124-A), Firstbank of Toyama Scholarship Foundation Research Grant, and JSPS grant KAKENHI (C, JP18K05460). We acknowledge Assoc. Prof. Miho Hatanaka of Keio University for her helpful discussion on our DFT calculations and Assoc. Prof. Suguru Yoshida of Tokyo University of Science for his fruitful advice on click reactions. We also thank Ms Yoshiko Nishikawa and Ms Mieko Yamagaki (MS measurements) of NAIST.
Notes and references
-
(a) N. J. Agard, J. M. Baskin, J. A. Prescher, A. Lo and C. R. Bertozzi, A Comparative Study of Bioorthogonal Reactions with Azides, ACS Chem. Biol., 2006, 1, 644–648 CrossRef CAS;
(b) E. Haldón, M. C. Nicasio and P. J. Pérez, Copper-catalysed Azide–alkyne Cycloadditions (CuAAC): An Update, Org. Biomol. Chem., 2015, 13, 9528–9550 RSC;
(c) L. Zhu, C. J. Brassard, X. Zhang, P. M. Guha and R. J. Clark, On the Mechanism of Copper(I)-Catalyzed Azide–Alkyne Cycloaddition, Chem. Rec., 2016, 16, 1501–1517 CrossRef CAS PubMed;
(d) E. Kim and H. Koo, Biomedical Applications of Copper-free Click Chemistry: in vitro, in vivo, and ex vivo, Chem. Sci., 2019, 10, 7835–7851 RSC.
-
(a) A. Qin, J. W. Y. Lamb and B. Z. Tang, Click Polymerization, Chem. Soc. Rev., 2010, 39, 2522–2544 RSC;
(b) Y. Shi, X. Cao and H. Gao, The Use of Azide–alkyne Click Chemistry in Recent Syntheses and Applications of Polytriazole-based Nanostructured Polymers, Nanoscale, 2016, 8, 4864–4881 RSC;
(c) D. Huang, Y. Liu, A. Qin and B. Z. Tang, Recent Advances in Alkyne-based Click Polymerizations, Polym. Chem., 2018, 9, 2853–2867 RSC;
(d)
D. Huang, Y. Liu, A. Qin and B. Z. Tang, in Click Polymerization, ed. A. Qin, B. Z. Tang, The Royal Society of Chemistry, Croydon, 2018 Search PubMed;
(e) M. Schock and S. Bräse, Reactive & Efficient: Organic Azides as Cross-Linkers in Material Sciences, Molecules, 2020, 25, 1009 CrossRef CAS PubMed.
-
(a)
Click Chemistry for Biotechnology and Materials Science, ed. J. Lahann, John Wiley & Sons, West Sussex, 2009 Search PubMed;
(b) H. C. Kolb, M. G. Finn and K. B. Sharpless, Click Chemistry: Diverse Chemical Function from a Few Good Reactions, Angew. Chem., Int. Ed., 2001, 40, 2004–2021 CrossRef CAS;
(c) C. W. Tornøe, C. Christensen and M. Meldal, Cu-Catalyzed Azide-Alkyne Cycloaddition, Chem. Rev., 2008, 108, 2952–3015 CrossRef PubMed;
(d) M. F. Debets, C. W. J. van der Doelen, F. P. J. T. Rutjes and F. L. van Delft, Azide: A Unique Dipole for Metal-Free Bioorthogonal Ligations, ChemBioChem, 2010, 11, 1168–1184 CrossRef CAS;
(e) E. M. Sletten and C. R. Bertozzi, From Mechanism to Mouse: A Tale of Two Bioorthogonal Reactions, Acc. Chem. Res., 2011, 44, 666–676 CrossRef CAS PubMed;
(f) D. M. Patterson, L. A. Nazarova and J. A. Prescher, Finding the Right (Bioorthogonal) Chemistry, ACS Chem. Biol., 2014, 9, 592–605 CrossRef CAS PubMed;
(g) A. Lossouarn, P.-Y. Renard and C. Sabot, Tailored Bioorthogonal and Bioconjugate Chemistry: A Source of Inspiration for Developing Kinetic Target-Guided Synthesis Strategies, Bioconjugate Chem., 2021, 32, 63–72 CrossRef CAS PubMed.
-
(a)
Organic Azides: Synthesis and Applications, ed. S. Bräse and K. Banert, John Wiley & Sons, West Sussex, 2010 Search PubMed. For selected reviews published after this perspective book, see:
(b) H. Tanimoto and K. Kakiuchi, Recent Applications and Developments of Organic Azides in Total Synthesis of Natural Products, Nat. Prod. Commun., 2013, 8, 1021–1034 CrossRef CAS;
(c) D. Intrieri, P. Zardi, A. Caselli and E. Gallo, Organic Azides: “Energetic Reagents” for the Intermolecular Amination of C–H Bonds, Chem. Commun., 2014, 50, 11440–11453 RSC;
(d) X.-R. Song, Y.-F. Qiu, X.-Y. Liu and Y.-M. Liang, Recent Advances in the Tandem Reaction of Azides with Alkynes or Alkynols, Org. Biomol. Chem., 2016, 14, 11317–11331 RSC;
(e) K. Wu, Y. Liang and N. Jiao, Azidation in the Difunctionalization of Olefins, Molecules, 2016, 21, 352 CrossRef PubMed;
(f) G. Huang and G. Yan, Recent Advances in Reactions of Azides, Adv. Synth. Catal., 2017, 359, 1600–1619 CrossRef;
(g) R. Sala, C. Loro, F. Foschi and G. Broggini, Transition Metal Catalyzed Azidation Reactions, Catalysts, 2020, 10, 1173 CrossRef CAS.
-
(a) P. Lundberg, C. J. Hawker, A. Hult and M. Malkoch, Click Assisted One–Pot Multi–Step Reactions in Polymer Science: Accelerated Synthetic Protocols, Macromol. Rapid Commun., 2008, 29, 998–1015 CrossRef CAS;
(b) G. Viault, S. Dautrey, N. Maindron, J. Hardouin, P.-Y. Renard and A. Romieu, The First “Ready-to-use” Benzene-based Heterotrifunctional Cross-linker for Multiple Bioconjugation, Org. Biomol. Chem., 2013, 11, 2693–2705 RSC;
(c) B. Thomas, M. Fiore, G. C. Daskhan, N. Spinellia and O. Renaudet, A Multi-ligation Strategy for the Synthesis of Heterofunctionalized Glycosylated Scaffolds, Chem. Commun., 2015, 51, 5436–5439 RSC;
(d) G. C. Daskhan, C. Pifferi and O. Renaudet, Synthesis of a New Series of Sialylated Homo- and Heterovalent Glycoclusters by using Orthogonal Ligations, ChemistryOpen, 2016, 5, 477–484 CrossRef CAS PubMed;
(e) A.-C. Knall, M. Hollauf, R. Saf and C. Slugovc, A trifunctional linker suitable for conducting three orthogonal click chemistries in one pot, Org. Biomol. Chem., 2016, 14, 10576–10580 RSC;
(f) M.-L. Winz, E. C. Linder, J. Becker and A. Jäschke, Site-specific One-pot Triple Click Labeling for DNA and RNA, Chem. Commun., 2018, 54, 11781–11784 RSC;
(g) Z. Li, S. Kosuri, H. Foster, J. Cohen, C. Jumeaux, M. M. Stevens, R. Chapman and A. J. Gormley, A Dual Wavelength Polymerization and Bioconjugation Strategy for High Throughput Synthesis of Multivalent Ligands, J. Am. Chem. Soc., 2019, 141, 19823–19830 CrossRef CAS PubMed;
(h) Y. Wang, J. Weng, J. Lin, D. Ye and Y. Zhang, NIR Scaffold Bearing Three Handles for Biocompatible Sequential Click Installation of Multiple Functional Arms, J. Am. Chem. Soc., 2020, 142, 2787–2794 CrossRef CAS PubMed.
- For recently published reviews on this strategy, see:
(a) S. Yoshida, Sequential Conjugation Methods Based on Triazole Formations and Related Reactions Using Azides, Org. Biomol. Chem., 2020, 18, 1550–1562 RSC;
(b) D. Sato, Z. Wu, H. Fujita, J. S. Lindsey, D. Sato, Z. Wu, H. Fujita and J. S. Lindsey, Design, Synthesis, and Utility of Defined Molecular Scaffolds, Organics, 2021, 2, 161–273 CrossRef.
- For recent review on preparation methods of aliphatic azides, see: P. Sivaguru, Y. Ning and X. Bi, New Strategies for the Synthesis of Aliphatic Azides, Chem. Rev., 2021, 121, 4253–4307 CrossRef CAS PubMed.
-
(a) P. Biallas, J. Heider and S. F. Kirsch, Functional Polyamides with gem-Diazido Units: Synthesis and Diversification, Polym. Chem., 2019, 10, 60–64 RSC;
(b) S. K. Boopathi, N. Hadjichristidis, Y. Gnanou and X. Feng, Direct Access to Poly(glycidyl Azide) and its Copolymers Through Anionic (Co-)polymerization of Glycidyl Azide, Nat. Commun., 2019, 10, 293 CrossRef PubMed. See also the references cited therein.
-
(a) Y. Chujo and K. Tanaka, New Polymeric Materials Based on Element-Blocks, Bull. Chem. Soc. Jpn., 2015, 88, 633–643 CrossRef CAS;
(b) M. Gon, K. Tanaka and Y. Chujo, Recent progress in the development of advanced element-block materials, Polym. J., 2018, 50, 109–126 CrossRef CAS;
(c)
New Polymeric Materials Based on Element-Blocks, ed. Y. Chujo, Springer, Singapore, 2019 Search PubMed;
(d) M. Gon, S. Ito, K. Tanaka and Y. Chujo, Design Strategies and Recent Results for Near-Infrared-Emissive Materials Based on Element-Block π-Conjugated Polymers, Bull. Chem. Soc. Jpn. DOI:10.1246/bcsj.20210235.
- For reviews on multi-azides as hub molecules for multi-functional materials, see:
(a) D. Fournier, R. Hoogenboom and U. S. Schubert, Clicking Polymers: A Straightforward Approach to Novel Macromolecular Architectures, Chem. Soc. Rev., 2007, 36, 1369–1380 RSC;
(b) W. H. Binder and R. Sachsenhofer, ‘Click’ Chemistry in Polymer and Material Science: An Update, Macromol. Rapid Commun., 2008, 29, 952–981 CrossRef CAS;
(c) K. Li, D. Fong, E. Meichsner and A. Adronov, A Survey of Strain-Promoted Azide–Alkyne Cycloaddition in Polymer Chemistry, Chem. – Eur. J., 2021, 27, 5057–5073 CrossRef CAS PubMed. For recent research on multi-azides as energetic materials or as material precursors, see:
(d) Y. Wang, Y. Liu, T. Lu, F. Gao and B. Zhao, Synthesis and Properties of 3-Azido-2,2-bis(azidomethyl)propyl 2-Azidoacetate: A Potential Azido Ester Plasticizer, ChemPlusChem, 2019, 84, 107–111 CAS;
(e) M. Claßen, S. B. Heimsch and T. M. Klapötke, Synthesis and Characterization of New Azido Esters Derived from Malonic Acid, Propellants, Explos., Pyrotech., 2019, 44, 1515–1520 CrossRef;
(f) T. Ikeda and Y. Matsushita, Tetrahedral Tetra-Cationic Ionic Liquids, Chem. Lett., 2020, 49, 14–16 CrossRef CAS;
(g) M. Oba, H. Takazaki, N. Kawabe, M. Doi, Y. Demizu, M. Kurihara, H. Kawakubo, M. Nagano, H. Suemune and M. Tanaka, Helical Peptide-Foldamers Having a Chiral Five-Membered Ring Amino Acid with Two Azido Functional Groups, J. Org. Chem., 2014, 79, 9125–9140 CrossRef CAS PubMed.
- A.-M. Caminade, R. Laurent, B. Delavaux-Nicota and J. P. Majoral, “Janus” Dendrimers: Syntheses and Properties, New J. Chem., 2012, 36, 217–226 RSC.
- R. Miyajima, K. Sakai, Y. Otani, T. Wadatsu, Y. Sakata, Y. Nishikawa, M. Tanaka, Y. Yamashita, M. Hayashi, K. Kondo and T. Hayashi, Novel Tetrafunctional Probes Identify Target Receptors and Binding Sites of Small-Molecule Drugs from Living Systems, ACS Chem. Biol., 2020, 15, 2364–2373 CrossRef CAS PubMed.
-
(a) H. Wang and D. J. Mooney, Metabolic Glycan Labelling for Cancer-Targeted Therapy, Nat. Chem., 2020, 12, 1102–1114 CrossRef CAS PubMed;
(b) A. I. Ponomarenko, V. A. Brylev, K. A. Sapozhnikova, A. V. Ustinova, I. A. Prokhorenko, T. S. Zatsepin and V. A. Korshun, Tetrahedral DNA Conjugates From Pentaerythritol-based Polyazides, Tetrahedron, 2016, 72, 2386–2391 CrossRef CAS;
(c) H. Yang and F. Seela, “Bis–Click” Ligation of DNA: Template–Controlled Assembly, Circularisation and Functionalisation with Bifunctional and Trifunctional Azides, Chem. – Eur. J., 2017, 23, 3375–3385 CrossRef CAS PubMed;
(d) R. V. Thaner, I. Eryazici, O. K. Farha, C. A. Mirkina and S. T. Nguyen, Facile one-step solid-phase synthesis of multitopic organic–DNA hybrids via “click” chemistry, Chem. Sci., 2014, 5, 1091–1096 RSC.
-
(a) T. Hosoya, T. Hiramatsu, T. Ikemoto, M. Nakanishi, H. Aoyama, A. Hosoya, T. Iwata, K. Maruyama, M. Endo and M. Suzuki, Novel Bifunctional Probe for Radioisotope-Free Photoaffinity Labeling: Compact Structure Comprised of Photospecific Ligand Ligation and Detectable Tag Anchoring Units, Org. Biomol. Chem., 2004, 2, 637–641 RSC;
(b) S. Yoshida, K. Kanno, I. Kii, Y. Misawa, M. Hagiwara and T. Hosoya, Convergent Synthesis of Trifunctional Molecules by Three Sequential Azido-type-selective Cycloadditions, Chem. Commun., 2018, 54, 3705–3708 RSC;
(c) M. Belkheira, D. El Abed, J.-M. Pons and C. Bressy, Chemoselective Organoclick–Click Sequence, Synthesis, 2018, 4254–4262 CAS;
(d) S. Yoshida, Y. Sakata, Y. Misawa, T. Morita, T. Kuribara, H. Ito, Y. Koike, I. Kii and T. Hosoya, Assembly of four modules onto a tetraazide platform by consecutive 1,2,3-triazole formations, Chem. Commun., 2021, 57, 899–902 RSC. For recent review, see ref. 6.
-
(a) T. Yokoi, H. Tanimoto, T. Ueda, T. Morimoto and K. Kakiuchi, Site-Selective Conversion of Azido Groups at Carbonyl α-Positions to Diazo Groups in Diazido and Triazido Compounds, J. Org. Chem., 2018, 83, 12103–12121 CrossRef CAS;
(b) T. Yokoi, T. Ueda, H. Tanimoto, T. Morimoto and K. Kakiuchi, Site-Selective Conversion of Azido Groups at Carbonyl α-Positions into Oxime Groups Leading Triazide to Triple Click Conjugation Scaffold, Chem. Commun., 2019, 55, 1891–1894 RSC.
- Recently, 2-azidoacrylamides have been developed as compact platforms for efficient modular synthesis through selective click reaction. See.
(a) S. Ariyasu, H. Hayashi, B. Xing and S. Chiba, Site-Specific Dual Functionalization of Cysteine Residue in Peptides and Proteins with 2-Azidoacrylates, Bioconjugate Chem., 2017, 28, 897–902 CrossRef CAS PubMed;
(b) H. Takemura, S. Goto, T. Hosoya and S. Yoshida, 2-Azidoacrylamides as compact platforms for efficient modular synthesis, Chem. Commun., 2020, 56, 15541–15544 RSC.
-
(a) N. Münster, P. Nikodemiak and U. Koert, Chemoselective Layer-by-Layer Approach Utilizing Click Reactions with Ethynylcyclooctynes and Diazides, Org. Lett., 2016, 18, 4296–4299 CrossRef PubMed;
(b) V. Udumula, H. S. Nazari, S. R. Burt, M. N. Alfindee and D. J. Michaelis, Chemo- and Site-Selective Alkyl and Aryl Azide Reductions with Heterogeneous Nanoparticle Catalysts, ACS Catal., 2016, 6, 4423–4427 CrossRef CAS;
(c) D. Svatunek, N. Houszka, T. Hamlin, M. Bickelhaupt and H. Mikula, Chemoselectivity of Tertiary Azides in Strain-promoted Alkyne-Azide Cycloadditions, Chem. – Eur. J., 2019, 25, 754–758 CrossRef CAS PubMed.
- For interesting reaction acceleration by a steric effect, see:
(a) S. Yoshida, A. Shiraishi, K. Kanno, T. Matsushita, K. Johmoto, H. Uekusa and T. Hosoya, Enhanced Clickability of Doubly Sterically-hindered Aryl Azides, Sci. Rep., 2011, 1, 82 CrossRef PubMed;
(b) S. Yoshida, J. Tanaka, Y. Nishiyama, Y. Hazama, T. Matsushita and T. Hosoya, Further Enhancement of the Clickability of Doubly Sterically-hindered Aryl Azides by para-Amino Substitution, Chem. Commun., 2018, 54, 13499–13502 RSC;
(c) S. Yoshida, S. Goto, Y. Nishiyama, Y. Hazama, M. Kondo, T. Matsushita and T. Hosoya, Effect of Resonance on the Clickability of Alkenyl Azides in the Strain-promoted Cycloaddition with Dibenzo-fused Cyclooctynes, Chem. Lett., 2019, 48, 1038–1041 CrossRef CAS.
-
(a) C. Uttamapinant, A. Tangpeerachaikul, S. Grecian, S. Clarke, U. Singh, P. Slade, K. R. Gee and A. Y. Ting, Fast, Cell-Compatible Click Chemistry with Copper-Chelating Azides for Biomolecular Labeling, Angew. Chem., Int. Ed., 2012, 51, 5852–5856 CrossRef CAS;
(b) Z. Yuan, G.-C. Kuang, R. J. Clark and L. Zhu, Chemoselective Sequential “Click” Ligation Using Unsymmetrical Bisazides, Org. Lett., 2012, 14, 2590–2593 CrossRef CAS;
(c) S. A. Ingale and F. Seela, Stepwise Click Functionalization of DNA through a Bifunctional Azide with a Chelating and a Nonchelating Azido Group, J. Org. Chem., 2013, 78, 3394–3399 CrossRef CAS PubMed;
(d) V. Bevilacqua, M. King, M. Chaumontet, M. Nothisen, S. Gabillet, D. Buisson, C. Puente, A. Wagner and F. Taran, Copper-Chelating Azides for Efficient Click Conjugation Reactions in Complex Media, Angew. Chem., Int. Ed., 2014, 53, 5872–5876 CrossRef CAS;
(e) Y. Su, L. Li, H. Wang, X. Wang and Z. Zhang, All-in-one Azides: Empowered Click Reaction for in vivo Labeling and Imaging of Biomolecules, Chem. Commun., 2016, 52, 2185–2188 RSC;
(f) A. Sallustrau, S. Bregant, C. Chollet, D. Audisio and F. Taran, Scalable and Practical Synthesis of Clickable Cu-Chelating Azides, Chem. Commun., 2017, 53, 7890–7893 RSC.
- For enantioselective discrimination of organic azides, see:
(a) J. Meng, V. V. Fokin and M. G. Finn, Kinetic Resolution by Copper-catalyzed Azide-alkyne Cycloaddition, Tetrahedron Lett., 2005, 46, 4543–4546 CrossRef CAS;
(b) E.-C. Liu and J. J. Topczewski, Enantioselective Copper Catalyzed Alkyne–Azide Cycloaddition by Dynamic Kinetic Resolution, J. Am. Chem. Soc., 2019, 141, 5135–5138 CrossRef CAS;
(c) J. R. Alexander, A. A. Ott, E.-C. Liu and J. J. Topczewski, Kinetic Resolution of Cyclic Secondary Azides, Using an Enantioselective Copper-Catalyzed Azide–Alkyne Cycloaddition, Org. Lett., 2019, 21, 4355–4358 CrossRef CAS PubMed;
(d) W. D. G. Brittain, A. G. Dalling, Z. Sun, C. S. Le Duff, L. Male, B. R. Buckley and J. S. Fossey, Coetaneous Catalytic Kinetic Resolution of Alkynes and Azides Through Asymmetric Triazole Formation, Sci. Rep., 2019, 9, 15086 CrossRef PubMed;
(e) C. Wang, R.-Y. Zhu, K. Liao, F. Zhou and J. Zhou, Enantioselective Cu(I)-Catalyzed Cycloaddition of Prochiral Diazides with Terminal or 1-Iodoalkynes, Org. Lett., 2020, 22, 1270–1274 CrossRef CAS PubMed;
(f) E.-C. Liu and J. J. Topczewski, Enantioselective Nickel-Catalyzed Alkyne–Azide Cycloaddition by Dynamic Kinetic Resolution, J. Am. Chem. Soc., 2021, 143, 5308–5313 CrossRef CAS.
-
(a) J. Dommerholt, O. van Rooijen, A. Borrmann, C. F. Guerra, F. M. Bickelhaupt and F. L. van Delft, Highly Accelerated Inverse Electron-demand Cycloaddition of Electron-deficient Azides with Aliphatic Cyclooctynes, Nat. Commun., 2014, 5, 5378 CrossRef CAS PubMed;
(b) S. Xie, S. A. Lopez, O. Ramström, M. Yan and K. N. Houk, 1,3-Dipolar Cycloaddition Reactivities of Perfluorinated Aryl Azides with Enamines and Strained Dipolarophiles, J. Am. Chem. Soc., 2015, 137, 2958–2966 CrossRef CAS PubMed;
(c) S. V. Chapyshev, A. V. Chernyak and I. K. Yakushchenko, Chemoselective Staudinger-Phosphite Reaction on the Azido Groups of 2,4,6-Triazido-3,5-dibromopyridine, J. Heterocycl. Chem., 2016, 53, 970–974 CrossRef CAS;
(d) M. Sundhoro, S. Jeon, J. Park, O. Ramström and M. Yan, Perfluoroaryl Azide Staudinger Reaction: A Fast and Bioorthogonal Reaction, Angew. Chem., Int. Ed., 2017, 56, 12117–12121 CrossRef CAS;
(e) T. Meguro, N. Terashima, H. Ito, Y. Koike, I. Kii, S. Yoshida and T. Hosoya, Staudinger Reaction Using 2,6-Dichlorophenyl Azide Derivatives for Robust Aza-ylide Formation Applicable to Bioconjugation in Living Cells, Chem. Commun., 2018, 54, 7904–7907 RSC;
(f) N. Terashima, Y. Sakata, T. Meguro, T. Hosoya and S. Yoshida, Triazole Formation of Phosphinyl Alkynes with Azides Through Transient Protection of Phosphine by Copper, Chem. Commun., 2020, 56, 14003–14006 RSC;
(g) L. Cheng, X. Kang, D. Wang, Y. Gao, L. Yi and Z. Xi, The One-pot Nonhydrolysis Staudinger Reaction and Staudinger or SPAAC Ligation, Org. Biomol. Chem., 2019, 17, 5675–5679 RSC;
(h) X. Kang, X. Cai, L. Yi and Z. Xi, Multifluorinated Aryl Azides for Development of Improved H2S Probes, and Fast SPAAC and Staudinger Reactions, Chem. – Asian J., 2020, 15, 1420–1429 CrossRef CAS PubMed.
-
(a) T. Meguro, S. Yoshida, K. Igawa, K. Tomooka and T. Hosoya, Transient Protection of Organic Azides from Click Reactions with Alkynes by Phosphazide Formation, Org. Lett., 2018, 20, 4126–4130 CrossRef CAS;
(b) T. Aimi, T. Meguro, A. Kobayashi, T. Hosoya and S. Yoshida, Nucleophilic transformations of azido-containing carbonyl compounds via protection of azido group, Chem. Commun., 2021, 57, 6062–6065 RSC.
-
(a) W. D. Lambert, Y. Fang, S. Mahapatra, Z. Huang, C. W. am Ende and J. M. Fox, Installation of Minimal Tetrazines through Silver-Mediated Liebeskind–Srogl Coupling with Arylboronic Acids, J. Am. Chem. Soc., 2019, 141, 17068–17074 CrossRef CAS PubMed;
(b) S. D. Schnell, L. V. Hoff, A. Panchagnula, M. H. H. Wurzenberger, T. M. Klapötke, S. Sieber, A. Linden and K. Gademann, 3-Bromotetrazine: Labelling of Macromolecules via Monosubstituted Bifunctional s-Tetrazines, Chem. Sci., 2020, 11, 3042–3047 RSC;
(c) E. Ros, M. Bellido, X. Verdaguer, L. R. de Pouplana and A. Riera, Synthesis and Application of 3–Bromo-1,2,4,5-Tetrazine for Protein Labeling to Trigger Click-to-Release Biorthogonal Reactions, Bioconjugate Chem., 2020, 31, 933–938 CrossRef CAS.
- Y. Tian and Q. Lin, Fitness Factors for Bioorthogonal Chemical Probes, ACS Chem. Biol., 2019, 14, 2489–2496 CrossRef CAS PubMed.
- Although no example of selective reaction has been shown, direct conversion of alkyl azides to diazo compounds has been reported before our works (ref. 15). See:
(a) G. B. Feigelson, On the conversion of azides to diazocompounds, Tetrahedron Lett., 1998, 39, 1129–1130 CrossRef CAS;
(b) E. L. Myers and R. T. Raines, A Phosphine-Mediated Conversion of Azides into Diazo Compounds, Angew. Chem., Int. Ed., 2009, 48, 2359–2363 CrossRef CAS PubMed;
(c) H.-H. Chou and R. T. Raines, Conversion of Azides into Diazo Compounds in Water, J. Am. Chem. Soc., 2013, 135, 14936–14939 CrossRef CAS PubMed.
-
(a) M. Kumasaki, K. Kinbara, Y. Wada, M. Arai and M. Tamura, Azidoacetamide, a Neutral Small Organic Azide, Acta Crystallogr., Sect. E: Struct. Rep. Online, 2001, 57, o6–o8 CrossRef CAS;
(b) J. M. Dyke, G. Levita, A. Morris, J. S. Ogden, A. A. Dias, M. Algarra, J. P. Santos, M. L. Costa, P. Rodrigues and M. T. Barros, Study of the Thermal Decomposition of 2-Azidoacetamide by Ultraviolet Photoelectron Spectroscopy and Matrix-Isolation Infrared Spectroscopy: Identification of the Imine Intermediate H2NCOCHNH, J. Phys. Chem. A, 2004, 108, 5299–5307 CrossRef CAS;
(c) S. Cecioni, J.-P. Praly, S. E. Matthews, M. Wimmerová, A. Imberty and S. Vidal, Rational Design and Synthesis of Optimized Glycoclusters for Multivalent Lectin–Carbohydrate Interactions: Influence of the Linker Arm, Chem. – Eur. J., 2012, 18, 6250–6263 CrossRef CAS PubMed;
(d) N. Collins, R. Connon, G. Sánchez-Sanz and P. Evans, Isomerisation of Vinyl Sulfones for the Stereoselective Synthesis of Vinyl Azides, Eur. J. Org. Chem., 2020, 6228–6235 CrossRef CAS. Very recently, Raines and co-workers also proposed the presence of intramolecular hydrogen bonding in α-AzSAs, which caused a significant difference in the reaction rates of SPAAC reactions with their newly developed cycloalkynes. See.
(e) J. M. Dones, N. S. Abularrage, N. Khanal, B. Gold and R. T. Raines, Acceleration of 1,3-Dipolar Cycloadditions by Integration of Strain and Electronic Tuning, J. Am. Chem. Soc., 2021, 143, 9489–9497 CrossRef CAS PubMed.
- For acidity of N-H in amides, see.
(a) F. G. Bordwell, J. A. Harrelson and T. Y. Lynch, Homolytic Bond Dissociation Energies for the Cleavage of α-N-H Bonds in Carboxamides, Sulfonamides, and Their Derivatives. The Question of Synergism in Nitrogen-Centered Radicals, J. Org. Chem., 1990, 55, 3337–3341 CrossRef CAS;
(b) F. G. Bordwell and G. Z. Ji, Effects of Structural Changes on Acidities and Homolytic Bond Dissociation Energies of the Hydrogen-nitrogen Bonds in Amidines, Carboxamides, and Thiocarboxamides, J. Am. Chem. Soc., 1991, 113, 8398–8401 CrossRef CAS.
- S. Xie, M. Sundhoro, K. N. Houk and M. Yan, Electrophilic Azides for Materials Synthesis and Chemical Biology, Acc. Chem. Res., 2020, 53, 937–948 CrossRef CAS.
-
(a)
T.-A. Okamura, H. Yamamoto and N. Ueyama, Conformational Switching Between Acids and their Anions by Hydrogen Bonding, in Hydrogen Bonding and Transfer in the Excited State, ed. K.-L. Han, G.-J. Zhao, John Wiley & Sons, West Sussex, 2011 Search PubMed. For synthetic application using intramolecular bonding with N–H of amides, see:
(b) I. A. O'Neil, N. D. Miller, J. Peake, J. V. Barkley, C. N. R. Low and B. Kalindjian, The Novel Use of Proline Derived Amine Oxides in Controlling Amide Conformation, Synlett, 1993, 515–517 CrossRef;
(c) X. H. Liu, L. L. Lin and X. M. Feng, Chiral N,N′-Dioxides: New Ligands and Organocatalysts for Catalytic Asymmetric Reactions, Acc. Chem. Res., 2011, 44, 574–587 CrossRef CAS PubMed.
-
(a) W. Q. Tian and Y. A. Wang, Mechanisms of Staudinger Reactions within Density Functional Theory, J. Org. Chem., 2004, 69, 4299–4308 CrossRef CAS PubMed;
(b) W. Q. Tian and Y. A. Wang, Dynamics of the Staudinger Reaction, J. Chem. Theory Comput., 2005, 1, 353–362 CrossRef CAS PubMed;
(c) G. C. Fortman, B. Captain and C. D. Hoff, Thermodynamic Investigations of the Staudinger Reaction of Trialkylphosphines with 1-Adamantyl Azide and the Isolation of an Unusual s-cis Phosphazide, Inorg. Chem., 2009, 48, 1808–1810 CrossRef CAS;
(d) M. W. P. Bebbington and D. Bourissou, Stabilised phosphazides, Coord. Chem. Rev., 2009, 253, 1248–1261 CrossRef CAS.
-
(a) F. L. Lin, H. M. Hoyt, H. van Halbeek, R. G. Bergman and C. R. Bertozzi, Mechanistic Investigation of the Staudinger Ligation, J. Am. Chem. Soc., 2005, 127, 2686–2695 CrossRef CAS PubMed;
(b) M. B. Soellner, B. L. Nilsson and R. T. Raines, Reaction Mechanism and Kinetics of the Traceless Staudinger Ligation, J. Am. Chem. Soc., 2006, 128, 8820–8828 CrossRef CAS PubMed.
-
(a) E. Saxon and C. R. Bertozzi, Cell Surface Engineering by a Modified Staudinger Reaction, Science, 2000, 287, 2007–2010 CrossRef CAS PubMed;
(b) E. Saxon, S. J. Luchansky, H. C. Hang, C. Yu, S. C. Lee and C. R. Bertozzi, Investigating Cellular Metabolism of Synthetic Azidosugars with the Staudinger Ligation, J. Am. Chem. Soc., 2002, 124, 14893–14902 CrossRef CAS PubMed. For recent reviews, see:
(c) Z.-P. A. Wang, C.-L. Tiana and J.-S. Zheng, The Recent Developments and Applications of the Traceless-Staudinger Reaction in Chemical Biology Study, RSC Adv., 2015, 5, 107192–107199 RSC;
(d) S. Liu and K. J. Edgar, Staudinger Reactions for Selective Functionalization of Polysaccharides: A Review, Biomacromolecules, 2015, 16, 2556–2571 CrossRef CAS PubMed;
(e) C. Bednarek, I. Wehl, N. Jung, U. Schepers and S. Bräse, The Staudinger Ligation, Chem. Rev., 2020, 120, 4301–4354 CrossRef CAS PubMed;
(f) T. K. Heiss, R. S. Dorn and J. A. Prescher, Bioorthogonal Reactions of Triarylphosphines and Related Analogues, Chem. Rev., 2021, 121, 6802–6849 CrossRef CAS PubMed.
-
(a) H. Zhang, H. Tanimoto, T. Morimoto, Y. Nishiyama and K. Kakiuchi, Regioselective Rapid Synthesis of Fully Substituted 1,2,3-Triazoles Mediated by Propargyl Cations, Org. Lett., 2013, 15, 5222–5225 CrossRef CAS;
(b) H. Zhang, H. Tanimoto, T. Morimoto, Y. Nishiyama and K. Kakiuchi, Acid-mediated Synthesis of Fully Substituted 1,2,3-Triazoles: Multicomponent Coupling Reactions, Mechanistic Study, Synthesis of Serine Hydrolase Inhibitor and its Derivatives, Tetrahedron, 2014, 70, 9828–9835 CrossRef CAS;
(c) Y. Zhang, J. Li, M. Wang, H. Zhang, H. Tanimoto, T. Morimoto and K. Kakiuchi, Synthesis of Fused 1,2,3-Triazoles through Carbocation-Mediated Intramolecular [3 + 2] Cycloaddition of Azido-propargyl Alcohols, Heterocycles, 2017, 94, 1775–1782 CrossRef CAS;
(d) Y. Zhang, M. Wang, J. Li, Q. Di, Z. Tian, B. Chen, H. Zhang, H. Tanimoto, T. Morimoto and K. Kakiuchi, Acid Promoted Metal Free Synthesis of Triazole-Fused Heterocycles via Intramolecular [3 + 2] Cycloaddition, Heterocycles, 2018, 96, 943–953 CrossRef CAS.
- Since the wavenumbers of the azido groups in IR absorption hardly change, IR spectra are not suitable to evaluate NH-N3 interactions. See: E. Lieber, C. N. R. Rao, T. S. Chao and C. W. W. Hoffman, Infrared Spectra of Organic Azides, Anal. Chem., 1957, 29, 916–918 CrossRef CAS.
- In Wang's works, the reactivity against Staudinger reduction was estimated from the chemical shifts of NMR. However, in our case, the tendency against the reaction was not clear. For precedented examples, see.
(a) P. T. Nyffeler, C.-H. Liang, K. M. Koeller and C.-H. Wong, The Chemistry of Amine-Azide Interconversion: Catalytic Diazotransfer and Regioselective Azide Reduction, J. Am. Chem. Soc., 2002, 124, 10773–10778 CrossRef CAS PubMed. For the application of chemical shift indication, see:
(b) J. Li, H.-N. Chen, H. Chang, J. Wang and C.-W. T. Chang, Tuning the Regioselectivity of the Staudinger Reaction for the Facile Synthesis of Kanamycin and Neomycin Class Antibiotics with N-1 Modification, Org. Lett., 2005, 7, 3061–3064 CrossRef CAS;
(c) J. Li, F.-I. Chiang, H.-N. Chen and C.-W. T. Chang, Investigation of the Regioselectivity for the Staudinger Reaction and Its Application for the Synthesis of Aminoglycosides with N-1 Modification, J. Org. Chem., 2007, 72, 4055–4066 CrossRef CAS;
(d) Y. Berkov-Zrihen, I. M. Herzog, M. Feldman and M. Fridman, Site-Selective Displacement of Tobramycin Hydroxyls for Preparation of Antimicrobial Cationic Amphiphiles, Org. Lett., 2013, 15, 6144–6147 CrossRef CAS.
- For example, see.
(a) O. Bakhanovich and P. Beier, Synthesis, Stability and Reactivity of α–Fluorinated Azidoalkanes, Chem. – Eur. J., 2020, 26, 773–782 CrossRef CAS;
(b) M. Mamone, R. S. B. Gonçalves, F. Blanchard, G. Bernadat, S. Ongeri, T. Milcent and B. Crousse, N-Difluoromethyl-triazole, as a constrained scaffold in peptidomimetics, Chem. Commun., 2017, 53, 5024–5027 RSC;
(c) J. Engel-Andreasen, I. Wellhöfer, K. Wich and C. A. Olsen, Backbone-Fluorinated 1,2,3-Triazole-Containing Dipeptide Surrogates, J. Org. Chem., 2017, 82, 11613–11619 CrossRef CAS PubMed.
- O. P. Blahun, A. B. Rozhenko, E. Rusanov, S. Zhersh, A. A. Tolmachev, D. M. Volochnyuk and O. O. Grygorenko, Twisting and Turning the Sulfonamide Bond: A Synthetic, Quantum Chemical, and Crystallographic Study, J. Org. Chem., 2020, 85, 5288–5299 CrossRef CAS PubMed.
- M. D. Velasco, P. Molina, P. M. Fresneda and M. A. Sanz, Isolation, Reactivity and Intramolecular Trapping of Phosphazide Intermediates in the Staudinger Reaction of Tertiary Phosphines with Azides, Tetrahedron, 2000, 56, 4079–4084 CrossRef CAS.
- M. Formica, D. Rozsar, G. Su, A. J. M. Farley and D. J. Dixon, Bifunctional Iminophosphorane Superbase Catalysis: Applications in Organic Synthesis, Acc. Chem. Res., 2020, 53, 2235–2247 CrossRef CAS PubMed.
-
(a) See the ESI.†;
(b) L. Yao, A. Grishaev, G. Cornilescu and A. Bax, The Impact of Hydrogen Bonding on Amide 1H Chemical Shift Anisotropy Studied by Cross-Correlated Relaxation and Liquid Crystal NMR Spectroscopy, J. Am. Chem. Soc., 2010, 132, 10866–10875 CrossRef CAS PubMed.
-
(a) B. L. Nilsson, L. L. Kiessling and R. T. Raines, Staudinger Ligation: A Peptide from a Thioester and Azide, Org. Lett., 2000, 2, 1939–1941 CrossRef CAS PubMed;
(b) E. Saxon, J. I. Armstrong and C. R. Bertozzi, A “Traceless” Staudinger Ligation for the Chemoselective Synthesis of Amide Bonds, Org. Lett., 2000, 2, 2141–2143 CrossRef CAS PubMed;
(c) P. B. Kapadnis, E. Hall, M. Ramstedt, W. R. J. D. Galloway, M. Welch and D. R. Spring, Towards quorum-quenching catalytic antibodies, Chem. Commun., 2009, 538–540 RSC;
(d) H. Itoh, K. Miura, K. Kamiya, T. Yamashita and M. Inoue, Solid-Phase Total Synthesis of Yaku'amide B Enabled by Traceless Staudinger Ligation, Angew. Chem., Int. Ed., 2020, 59, 4564–4571 CrossRef CAS PubMed;
(e) C. Kitoun, M. Fonvielle, N. Sakkas, M. Lefresne, F. Djago, Q. B. Remaury, P. Poinot, M. Arthur, M. Etheve-Quelquejeu and L. Iannazzo, Phosphine-Mediated Bioconjugation of the 3′-End of RNA, Org. Lett., 2020, 22, 8034–8038 CrossRef CAS PubMed.
-
(a) J. Dommerholt, F. P. J. T. Rutjes and F. L. van Delft, Strain-Promoted 1,3-Dipolar Cycloaddition of Cycloalkynes and Organic Azides, Top. Curr. Chem., 2016, 374, 16 CrossRef PubMed;
(b) E. G. Chupakhin and M. Y. Krasavin, Achievements in the synthesis of cyclooctynes for ring strain-promoted [3 + 2] azide-alkyne cycloaddition, Chem. Heterocycl. Compd., 2018, 54, 483–501 CrossRef CAS.
-
(a) V. S. Sadu, H. N. Roy, P. Arigala, I.-T. Hwang and K.-I. Lee, Entry to Highly Hindered Chiral β-Amino Triazoles Bearing a gem-Diaryl Group by Azide-alkyne Click Chemistry, Bull. Korean Chem. Soc., 2014, 35, 1605–1612 CrossRef CAS;
(b) V. S. Sadu, S. Sadu, S. Kim, I.-T. Hwang, K.-J. Kong and K.-I. Lee, Influence of steric demand on ruthenium-catalyzed cycloaddition of sterically hindered azides, RSC Adv., 2017, 7, 3229–3232 RSC.
- Although only one competitive reaction example was examined, Sun and Wang's group reported NHC-CuAAC with a silylated alkyne, affording a triazole product from α-AzSA selectively. See: Y. Xia, L.-Y. Chen, S. Lv, Z. Sun and B. Wang, Microwave-Assisted or Cu–NHC-Catalyzed Cycloaddition of Azido-Disubstituted Alkynes: Bifurcation of Reaction Pathways, J. Org. Chem., 2014, 79, 9818–9825 CrossRef CAS.
Footnote |
† Electronic supplementary information (ESI) available: Experimental procedures, analytical data (1H, 31P, and 13C NMR, IR, and mass spectroscopy, melting points, optical rotation, and Rf values), and computational results of the compounds. See DOI: 10.1039/d1qo01088c |
|
This journal is © the Partner Organisations 2021 |
Click here to see how this site uses Cookies. View our privacy policy here.