DOI:
10.1039/D0QI00779J
(Review Article)
Inorg. Chem. Front., 2020,
7, 3258-3281
Thermo-responsive light-emitting metal complexes and related materials
Received
2nd July 2020
, Accepted 26th July 2020
First published on 28th July 2020
Abstract
Thermo-responsive light-emitting materials have wide potential applications in temperature sensing, bioimaging, optoelectronic devices and information security, due to their intriguing luminescence property changes in response to temperature. Among them, light-emitting metal complexes and related coordination materials are appealing in possessing enormous structural diversity and excellent luminescence properties. The general thermo-responsive types and the underlying mechanism of these materials and recent progress in the development of thermo-responsive metal complexes and related materials are surveyed in this critical review, including small molecular metal complexes with different electronic configurations (d6, d8, d10, among others), metal clusters, coordination polymers, metal–organic frameworks (MOFs), and multicomponent coordination polymers and assemblies. A particular focus is given on how to build systems showing ratiometric lumunescence changes in response to temperature, including molecular dyads containing two chromophores, heterodimetallic clusters, mixed-metal MOFs, and energy donor–acceptor assemblies. In addition, the applications of these materials in temperature sensing, bioimaging, and information security are briefly discussed. A perspective is given in the Conclusion section to reflect potential guidelines for future research in this field.
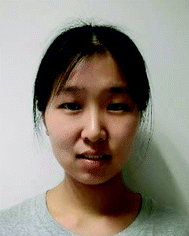 Rui Li | Rui Li received her B.S. degree from Central China Normal University in 2015. She is currently a Ph.D. candidate at the Institute of Chemistry, Chinese Academy of Sciences, under the supervision of Prof. Yu-Wu Zhong. Her research interests focus on emissive metal complexes and their assembled structures. |
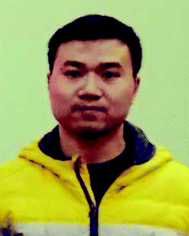 Fa-Feng Xu | Fa-Feng Xu obtained his B.S. degree from Jilin University in 2014 and Ph.D. from the Institute of Chemistry, Chinese Academy of Sciences (ICCAS) in 2020 under the supervision of Prof. Jiannian Yao and Prof. Yong Sheng Zhao. Currently, he works as a postdoctoral fellow with Prof. Yu-Wu Zhong at ICCAS. His research interests focus on the fabrication and application of organic luminescent materials and micro/nano lasers. |
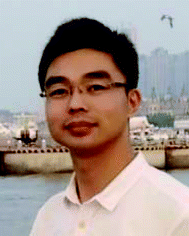 Zhong-Liang Gong | Zhong-Liang Gong received his Ph.D. degree from the Institute of Chemistry, Chinese Academy of Sciences under the supervision of Prof. Yu-Wu Zhong in 2015. After that, he worked as an assistant professor in the same research group and was promoted as an associate professor in 2020. His current interests mainly focus on the synthesis and optoelectronic applications of luminescent transition metal complexes. |
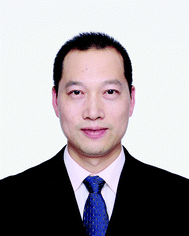 Yu-Wu Zhong | Yu-Wu Zhong obtained his Ph.D. in 2004 from the Shanghai Institute of Organic Chemistry, Chinese Academy of Sciences. After that, he worked as a postdoctoral associate at the University of Tokyo (2004–2006) and Cornell University (2006–2009), respectively. He joined the Institute of Chemistry, Chinese Academy of Sciences, to start his independent career in 2009. He received the China Electrochemistry Young Scientist Award in 2015, the China Photochemistry Young Scientist Award, the National Science Fund for Distinguished Young Scholars, and the Chinese Chemical Society-Evonik Chemical Innovation Award for Distinguished Scientist in 2019. His current research interests focus on the synthesis, assembly, and applications of photo- and electro-functional molecular materials. |
1. Introduction
Temperature is one of the most fundamental physical parameters in scientific research and industrial production. The variation of temperature may lead to distinct changes of the chemical and physical properties of natural systems and synthetic materials.1–4 In particular, thermo-responsive functional materials with specific luminescence changes have attracted wide attention due to their potential applications in temperature sensing,5–12 biological imaging,13–20 optoelectronic devices,21–25 and information processing.26–28 To date, tremendous progress has been made in the development of thermo-responsive light-emitting materials, including various organic dyes and polymers,29–37 metal complexes,38–44 metal clusters and metal–organic frameworks (MOFs),45–49 quantum dots (QDs),50,51 and nanomaterials.52–55
Among the abovementioned materials, metal complexes and related coordination materials have several outstanding advantages.56–59 Metal complexes are known to possess various metal–ligand coordination modes, such as linear, planar, tetrahedral, and octahedral configurations. They are characterized by rich radiative excited states, including the intraligand (IL) or ligand-centered (LC) transition, the metal-to-ligand or ligand-to-metal charge-transfer (MLCT or LMCT) transition, the ligand-to-ligand charge-transfer (LLCT) transition, the metal–metal-to-ligand charge-transfer (MMLCT) transition, and the cluster/metal-centered (MC) transition. Due to the strong spin–orbit coupling effect, metal complexes often display triplet luminescence, namely phosphorescence, with long excited-state lifetimes (τ) and good to excellent quantum yields (ϕ). However, the involvement of ligand-related singlet fluorescence is also possible, leading to the co-presence or overlap of multiple radiative transitions. These features allow us to modulate the chemical compositions and luminescence properties of metal complexes in a wide emission spectral coverage from the ultraviolet (UV) to near-infrared (NIR) region that could respond to temperature changes in a wide temperature range.
Several reviews focusing on thermo-responsive MOFs and lanthanide or Cu-based clusters have been published recently;2–4,60 however temperature-responsive metal complexes in a broader scope have not been reviewed. We present here a systematic summary and discussion on thermo-responsive metal complexes including small molecular metal complexes, metal–ligand coordination clusters and polymers, and related multicomponent materials. Considering that a great number of related complexes and materials have been reported in this field, only some representative and latest examples are in particular selected for discussions. In this sense, the article is structured mainly in four aspects: (1) the outline of general thermo-responsive types and underlying mechanism; (2) thermo-responsive small molecular metal complexes, including those with d6, d8, d10 and other electronic configurations and lanthanide complexes; (3) thermo-responsive metal clusters, coordination polymers (CPs), and MOFs; (4) multi-component CPs and assemblies. These discussions are followed by some guided perspectives for future research in the Conclusion section.
2. General thermo-responsive types and underlying mechanism
The thermal responsiveness of materials could be caused by a number of different factors. Firstly, the non-radiative rate constant knr increases with increasing temperatures, obeying the Arrhenius equation (2.1),where ΔE represents the energy gap between the level of the lowest excited state and the overlap point to non-radiative transition states, and k is the Boltzmann constant.1,3 For a simple molecular system, the emission intensity (I) is dependent on temperature, considering that I is proportional to ϕ and ϕ is in inverse proportion to knr according to eqn (2.2),where kr is the radiative rate constant. In this sense, all emissive materials are intrinsically thermo-responsive.
In addition to the thermal-activated non-radiative decay, many other factors may be activated or influenced by temperature, including the effective molecular conformation or configuration transformation of flexible systems,61,62 the degree of inter- or intramolecular π–π stacking and metal–metal interactions existing in aggregates or clusters of Rh(I), Pt(II), Cu(I), Ag(I), and Au(I) complexes,39,63–65 the dynamic association or dissociation process of metal and lanthanide complexes,66,67 phase transition,68–71 the degree of inter- or intramolecular energy transfer of donor/acceptor systems,72,73 as well as the thermal equilibrium of different excited states.74,75 These mechanisms will be discussed in more detail in specific examples in the following chapters.
In general, four types of readouts are employed to characterize the thermo-responsiveness of materials, including the change of emission intensity, maximum emission wavelength (λemi), emission lifetime, and emission polarization (or anisotropy).1,3 The sensitivity of a particular process is defined as the slope of the readout signal change as a function of temperature, usually expressed as percent change per Kelvin (% K−1), which is an important indicator for evaluating temperature-sensitive materials. The intensity-based thermo-responsiveness is the simplest process. As was discussed above, the thermal-activated non-radiative decay could lead to the variation of emission intensity. However, the emission intensity changes could also be caused by many other factors, such as the dynamic association/dissociation process or phase transition.66,71 The shift of λemi is another general readout during the studies of thermo-responsive materials, which could be a result of dynamic thermal-equilibrium of different excited states or the change of the energy/electron transfer efficiency.76,77 The shift of λemi often leads to the emission color changes of materials, allowing us to conveniently monitor the thermo-responsive process. Compared to the emission intensity, the emission lifetime is less dependent on conditions such as photo-bleaching, concentration of materials, and energy fluctuation of the excitation source. It presents another important readout to monitor the thermo-responsiveness. Lifetime-based thermo-responsible materials have potential applications in time-resolved biological imaging and cellular temperature monitoring.78,79 The last readout is emission polarization or anisotropy, which generally decreases with increasing temperature as a result of the temperature-dependent Brownian motion of individual molecules.80 For more detailed discussions on the thermo-responsive mechanism and readouts, the authors are directed to a previous review article.1
3. Thermo-responsive small molecular metal complexes
Small molecular discrete metal complexes possess good thermo and photostability and rich luminescence in a broad spectral range. Their thermo-responsive behaviour accompanied by luminescence property changes has received much interest. In this section, some representative examples with different electronic configurations (d6, d8, d10, and others and lanthanide complexes) are discussed.
3.1 d6 metal complexes
Luminescent metal complexes with a d6 electronic configuration mainly include Re(I), Ru(II), Os(II), Rh(III), and Ir(III) complexes, which exhibit remarkable luminescence properties and are extensively used in OLEDs and chemical sensing.81–84 The applications of emissive Ru(II) complexes in temperature sensing are well established.85,86 For instance, Orellana and coworkers show that the luminescence lifetimes of a series of Ru(II) polyazaheteroaromatic complexes 1a–1f decrease in accordance with the Arrhenius equation (Fig. 1).85 These complexes are further embedded into poly(ethyl cyanoacrylate) as the responsive media to sense temperature. By taking fully advantage of the low permeability to O2, excellent optical quality, and high temperature-sensitive luminescence intensity and lifetimes, the doped film of 1c demonstrated a sensing resolution of 0.05 °C with a linear response to temperature in the range of 0–40 °C based on the lifetime readout. These features make it potentially applicable for temperature monitoring in bioreactor and biological tissues.
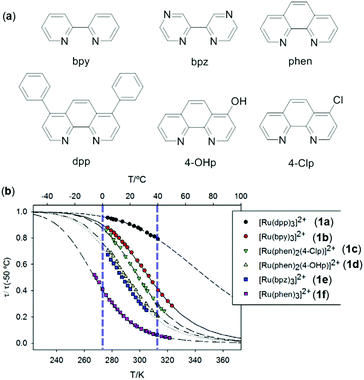 |
| Fig. 1 (a) Bidentate ligands to make ruthenium complexes 1a–1f. (b) Luminescence lifetimes (normalized at 50 °C) as a function of temperature of 1a–1f (counter anions are Cl−) in deoxygenated propylene carbonate solution. Reprinted with permission from ref. 85. License MDPI, Basel, Switzerland. | |
Ir(III) complexes are another important thermochromic luminescent d6 metal complexes.87–89 For example, Zhao, Huang and coworkers have reported a series of thermo-responsive Ru(II) and Ir(III) complexes functionalized with methyl pyridinium chromophores.89 Shown in Fig. 2 are the results of a representative Ir(III) complex 2. This complex is only weakly emissive (Φ < 0.1%) in degassed CH3CN, caused by oxidative quenching of the deficient methyl pyridinium unit via intramolecular electron transfer. However, when the methyl pyridinium units are reduced, the luminescence of 2 can be strongly enhanced in situ. In particular, the reduction of 2 by KI is remarkably temperature-dependent. At 60 °C or below, no reduction occurs. When the temperature is increased from 60 to 75 °C, the reduction occurs smoothly accompanied by a significant yellow-emission enhancement. On the basis of the synergy between KI and temperature, a novel information protection device has been fabricated with complex 2.
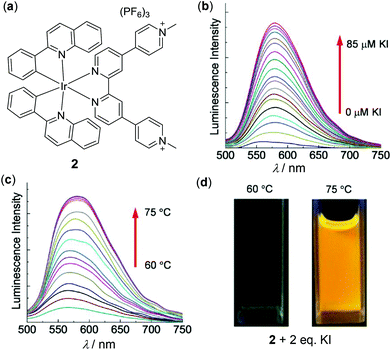 |
| Fig. 2 (a) Molecular structure of Ir(III) complex 2. (b) Luminescence spectra change of 2 in degassed CH3CN (1.0 × 10−5 M) at 75 °C with an increasing amount of KI from 0 to 10 equiv. (c) Temperature-dependent luminescence spectral change of 2 with 2 equiv. of KI upon heating from 60 to 75 °C. (d) Photographs of the solution of 2 at 60 or 75 °C in the presence of 2 equiv. of KI. Reprinted with permission from ref. 89. Copyright 2016 WILEY-VCH. | |
The above example demonstrates the thermo-responsive behaviour by controlling the electron transfer process. It is also possible to utilize the intramolecular energy transfer process to realize thermo-responsive properties. For instance, the Ir(III)-naphthalimide dyad 3 displays dual emissions at 507 and 606 nm at 77 K in cryogenic Me-THF, originating from the 3LC emission of the naphthalimide unit and the 3MLLCT (metal/ligand-to-ligand charge-transfer) emission of the Ir(III) component, respectively.90 In contrast, complex 3 only shows a single broad and unstructured 3MLLCT emission at 593 nm (τ = 8.8 ms) at rt due to the efficient energy transfer process from the naphthalimide unit to the Ir(III) component.
3.2 d8 metal complexes
Metal complexes with a d8 electronic configuration, e.g. Rh(I), Pd(II) and Pt(II) complexes, are another type of important luminescent material.56 In particular, widespread attention has been paid to square planar Pt(II) complexes as thermo-responsive materials in recent years.91–98 These complexes are prone to form intermolecular metal–metal or π–π interactions in aggregate states, which is highly dependent on external conditions such as concentration and temperature. For instance, Wang and coworkers reported the tetradentate Pt(II) complex 4, which displayed distinguished temperature-responsive luminescence (Fig. 4a and b).91 Only one structured monomeric blue emission at 477 nm was observed for complex 4 in degassed Me-THF (1 × 10−3 M) at temperatures below 130 K. However, as the temperature increased from 130 to 345 K, a new unstructured excimer emission at 650 nm appeared, accompanied by a dramatic emission color change from sky-blue to red. This luminescent thermochromism is fully reversible and reproducible. The authors deduced that the red emission was due to π-stacked excimers as elevated temperatures tended to increase the kinetic energy of the molecules and the probability of forming excimers. Similarly, the formation of the 3MMLCT transition via inter- or intramolecular Pt–Pt interactions is also strongly dependent on temperature. In this context, Lodeiro and coworkers prepared luminescent polymers by doping the bis(pyrazolate) Pt(II) complex 5 into PMMA, which showed interesting multistep thermochromic luminescence (Fig. 4c and d).92 With an increase of temperature from 21 to 137.3 °C, three-step emission changes were clearly observed. Within the temperature range between 21 and 70 °C, the original greenish emission gradually decreased due to the aggregation-caused quenching (ACQ) effect. An orange emission in the lower-energy region appeared at 70–75 °C, attributed to the formation of the 3MMLCT excited state via intermolecular Pt–Pt interactions. When the temperature was further increased from 77 to 137 °C, the 3MMLCT emission was progressively quenched, caused by the thermally-activated non-radiative transition process. This Pt(II) complex provides a potential candidate for the preparation of multistep thermochromic materials.
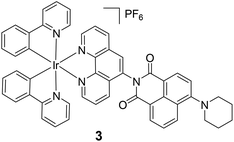 |
| Fig. 3 Thermo-responsive Ir(III) complex 3 on the basis of the controlled energy transfer mechanism. Reprinted with permission from ref. 90. Copyright 2016 Wiley-VCH. | |
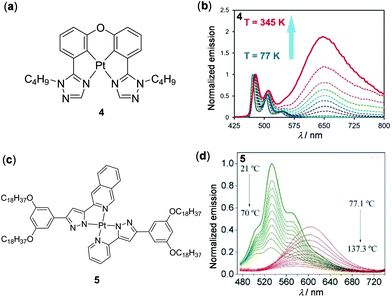 |
| Fig. 4 (a) Molecular structure of Pt(II) complex 4. (b) Normalized temperature-dependent emission spectra of 4 in degassed Me-THF (1 × 10−3 M, λex = 397 nm). Reprinted with permission from ref. 91. Copyright 2017 Wiley-VCH. (c) Molecular structure of Pt(II) complex 5. (d) Normalized temperature-dependent emission spectral changes of the PMMA film doped with 5 upon heating from 21 to 137.3 °C. Reprinted with permission from ref. 92. Copyright 2020 Elsevier Ltd. | |
The above two examples demonstrate the thermoresponsive behaviour of Pt complexes as a result of the changes of intermolecular interactions. As an example of thermo-responsive Pt(II) complexes by manipulating the intramolecular Pt–Pt interactions, Yam and coworkers showed that a series of oligo(ethynylpyridine)-bridged diplatinum metallofoldamers, represented by complex 6 in Fig. 5, displayed reversible emission color changes between green and red as a result of the solvent or temperature-controlled helix–coil configuration transformation.93
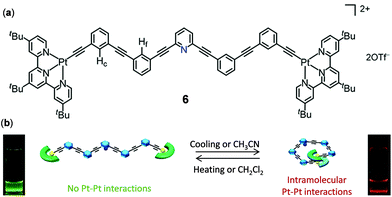 |
| Fig. 5 (a) Molecular structure of Pt(II) complex 6. (b) Schematic diagram showing the reversible helix–coil molecular configuration transformation regulated by solvents and temperature. Reprinted with permission from ref. 93. Copyright 2019 American Chemical Society. | |
As has been discussed above in the case of Ir(III)-naphthalimide complex 3 (Fig. 3), thermal-equilibrium of different excited states in molecules containing two or more than two chromophores is an effective strategy to induce thermo-responsive properties. Using a similar strategy, Zhao and coworkers prepared the platinum bis(acetylide) complex 7 with the incorporations of two naphthalimide units on the acetylide ligand (Fig. 6).96 Two closely-lying triplet excited states, i.e.3MLCT and the naphthalimide-localized emissive triplet state (3LE), co-exist in this complex, the population ratio of which could be finely tuned by solvent polarity and temperature. For instance, upon heating from 298 to 358 K, the 3LE emission of 7 at 605 nm diminished whereas the 3MLCT emission at 565 nm was intensified in toluene. However, when measured in benzonitrile, the 3MLCT emission of 7 at 536 nm was only slightly increased upon heating to 363 K; however the 3LE emission at 620 nm was dramatically decreased. This work demonstrates the importance of thermo-responsive behaviour in understanding the intrinsic photophysical properties of light-emitting materials containing multiple chromophores.
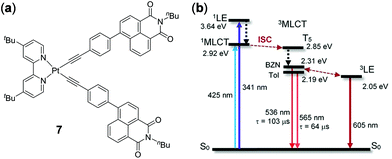 |
| Fig. 6 (a) Molecular structure of Pt(II) complex 7. (b) Simplified Jablonski diagram showing the energy levels of 7. BZN = benzonitrile. Tol = toluene. Reprinted with permission from ref. 96. Copyright 2019 American Chemical Society. | |
As an example of thermo-responsive Pt(II) complexes with more complex molecular structures, Zhong, Stang, and coworkers examined two fluorescent hexagonal organoplatinum metallacycles 8a and 8b with appended anthracene chromophores (Fig. 7).97 Upon increasing the temperature from −20 to 60 °C, the emission of 8a and 8b at 520 nm in THF was gradually decreased with a sensitivity of −0.66% and −0.76% per °C, ascribed to the accelerated molecular rotation of the appended anthracene units. In addition, complex 8a displayed two-step temperature-dependent emission spectral changes in DMF. Upon heating from −20 to 30 °C, the emission at 530 nm was gradually decreased. Upon further heating from 30 to 70 °C, this emission band shifted to 470 nm with a diminished intensity. Concurrently, a distinct emission color change from green to cyan was clearly visualized. However, the emission shift in the second step is irreversible, which is caused by the decomposition of the metallacycle by DMF.
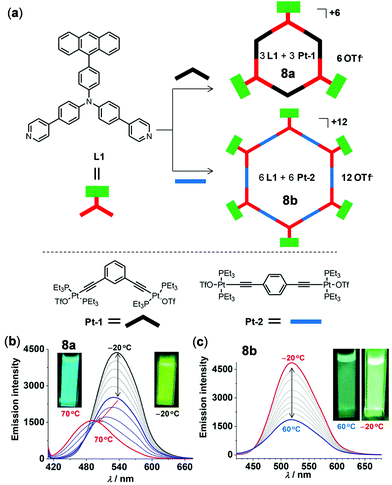 |
| Fig. 7 (a) Synthetic routes and schematic diagram of metallacycles 8a and 8b. (b and c) Temperature-dependent emission spectral changes of (b) 8a in DMF and (c) 8b in THF (λex = 400 nm, 2 × 10−5 M). The insets show the emission colors at lower and higher temperature, respectively. Reprinted with permission from ref. 97. Copyright 2018 American Chemical Society. | |
We discussed in this section mainly on the thermo-responsive small molecular complexes. However, it should be pointed out that it is also possible to achieve thermo-responsiveness by incorporating metal complexes into thermosensitive polymeric materials. As an example in this context, Huang, Sun, and coworkers prepared a luminescent polymer 9 by introducing an AIE-active (AIE = aggregation induced emission) Pt(II) component into the thermosensitive poly N-isopropylacrylamide backbone through copolymerization (Fig. 8).98 Upon increasing the temperature from 28 to 39 °C in the physiological range, the yellow emission of 9 in PBS buffer (pH = 7.4) exhibited a significant enhancement due to the rigidified microenvironment of the Pt component induced by the aggregation of polymer chains at elevated temperatures. This thermo-sensitive luminescent polymer material was subsequently applied in intracellular imaging for monitoring the temperature distribution, taking advantage of its high stability and biocompatibility, and the good reversibility of the thermochromic process.
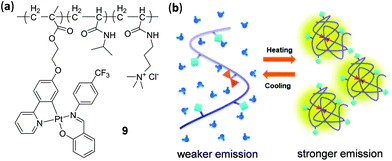 |
| Fig. 8 (a) Polymer 9 with an appended Pt(II) complex. (b) Schematic illustration showing the thermo-responsive mechanism. Reprinted with permission from ref. 98. Copyright 2019 The Royal Society of Chemistry. | |
3.3 d10 metal complexes
In addition to d6 and d8 metal complexes, luminescent d10 metal complexes, including Cu(I), Ag(I), Au(I), Zn(II), and Cd(II) complexes, have also been demonstrated to display thermo-responsive properties, which will be discussed in this section. As an example of thermo-responsive Cu(I) complexes, Wolf and coworkers reported the thermochromic solid-state emission of the dipyridyl sulfoxide Cu(I) complex 10 (Fig. 9).99 The crystalline sample (solid or film) of 10 displayed orange emission at rt. Upon cooling to −190 °C, the orange emission turned to yellow with an emission spectral shift of around 100 nm. The yellow-orange thermochromic luminescence of 10 is fully reversible between −190 and 27 °C, which is ascribed to the excited state coordination geometry change of 10 triggered by temperature (a more flattened state at rt versus a pseudo-tetrahedral geometry at −190 °C).
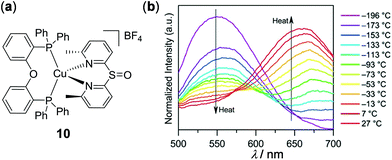 |
| Fig. 9 (a) Molecular structure of Cu(I) complex 10. (b) Normalized temperature-dependent emission spectral changes of 10 from −196 to 27 °C as thin films by drop-casting on quartz from MeOH solution (λex = 380 nm). Reprinted with permission from ref. 99. Copyright 2018 American Chemical Society. | |
Shown in Fig. 10 is a thermochromic square-planar Ag(I) complex 11 reported by Artem'ev and coworkers. At 298 K, complex 11 exhibited pronounced solid-state yellow emission (λemi = 585 nm), assigned to the 3MLCT phosphorescence with an admixture of 1MLCT fluorescence. Upon cooling to 77 K, the maximum emission shifted to 620 nm with a concomitant 35% enhancement of intensity and emission color change from yellow to orange-red. This phenomenon was attributed to a small alternation of the ground and excited states of 11, resulting in a narrowing of the energy gap between the T1 and S0 states upon cooling.77
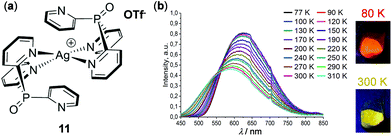 |
| Fig. 10 (a) Molecular structure of Ag(I) complex 11. (b) Normalized temperature-dependent emission spectral changes in the range of 77–310 K. The pictures on the right show the solid emission colors of the samples at 80 and 300 K, respectively. Reprinted with permission from ref. 77. Copyright 2019 the Partner Organisations. | |
In addition to mononuclear metal complexes, d10 complexes with multiple metal centers have been reported regarding their thermosensitive properties. Strelnik and coworkers prepared a binuclear Au(I) complex 12 bridged by a flexible bidentate cyclic phosphine ligand (Fig. 11).100 This complex exhibited very weak greenish emission in solution at rt. Upon cooling to 180 K, a slight blue shift of the emission spectrum with a significant intensity enhancement was observed, mainly attributed to the configuration rigidification of 12 at low temperatures. Upon heating back to 298 K, the initial emission can be completely restored, demonstrating the excellent thermo-reversibility of the complex. Besides, a series of cyclic trinuclear Cu(I), Ag(I) and Au(I) complexes 13a–13c have been prepared by Giménez and coworkers, which exhibited excellent thermochromic luminescence switching (Fig. 12).101 The gold and copper complexes 13a and 13c are highly emissive with Φ > 90% in doped PMMA films (5 wt%) at rt. Upon cooling from 298 to 77 K, the initial red emissions of 13a and 13c, originated from centered excimeric (3MM) states via intermolecular aurophilic interactions, were gradually transformed into blue and yellow emission, respectively. In contrast, the PMMA film of the silver complex 13b was not luminescent at rt, but it showed a blue luminescence turn-on upon cooling, demonstrating the important influence of the metal in influencing the photophysical properties.
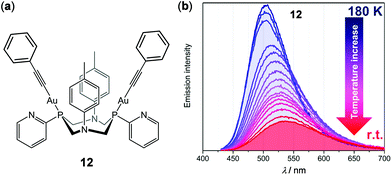 |
| Fig. 11 (a) Molecular structure of Au(I) complex 12. (b) Temperature-dependent emission spectral changes of 12 in the range of 180–298 K (λex = 340 nm). Reprinted with permission from ref. 100. Copyright 2019 American Chemical Society. | |
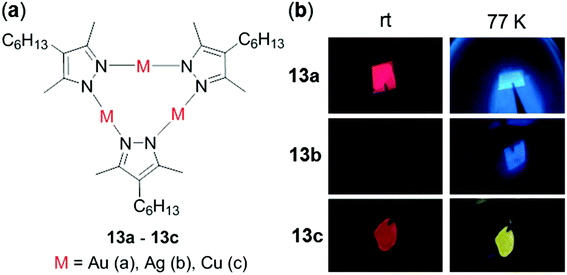 |
| Fig. 12 (a) Molecular structures of complexes 13a–13c. (b) Video snapshots of the luminescence color switching of PMMA films (5 wt%) excited at 254 nm from rt to 77 K for 13a–13c. Reprinted with permission from ref. 101. Copyright 2018 American Chemical Society. | |
In addition to the above examples, earth-abundant Zn(II) and Cd(II) complexes are other d10 complexes that have been reported to show thermo-responsive luminescence behaviours. For instance, Zhao, Huang, and coworkers have fabricated chameleon-like thermochromic luminescent materials by doping complex Zn-BPPA (14) into polyethylene glycol (PEG) matrices (Fig. 13).102 Complex 14 was prepared from the bipyridine ligand BPPA and it showed completely different emission spectra with respect to BPPA. When 0.3 wt% of 14 was doped into PEG8000, the yellow emission of the obtained material turned blue, accompanied by a distinct intensity enhancement, upon increasing the temperature from 25 to 65 °C. This response is highly reversible (20 cycles of heating and cooling being tested) and it functions as a fluorescent thermometer with good sensitivity. The emission spectral changes are caused by the dynamic metal–ligand coordination and dissociation of the BPPA ligand and Zn(II) ions. Interestingly, when the doping ratio of 14 was increased to 1.0 wt%, the yellow emission of the obtained material exhibited a red shift, as a result of the excited state conformation changes. In addition, by choosing PEG matrices with different molecular weights, the thermochromic transition temperature of these materials could be well tuned (Fig. 13d), making them potentially useful to anti-counterfeiting and security printing.
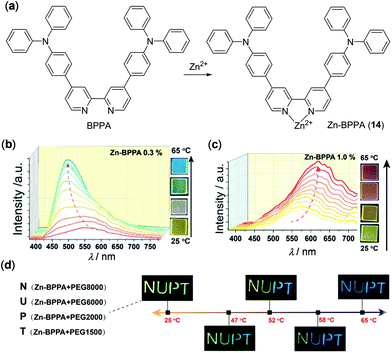 |
| Fig. 13 (a) Chemical structures of BPPA and Zn(II) complex 14. (b and c) Temperature-dependent emission spectral and color change of PEG8000 containing (b) 0.3 wt% and (c) 1.0 wt% of 14 within the temperature range of 25–65 °C. (d) Photographs displaying thermochromic luminescent NUPT patterns at different temperatures. The letters “N, U, P, and T” were written on a glass substrate using 14/PEG8000, 14/PEG6000, 14/PEG2000 and 14/PEG1500 composites containing 0.3, 0.3, 0.2, and 0.2 wt% of 14, respectively. Reprinted with permission from ref. 102. Copyright 2020 WILEY-VCH. | |
Fig. 14 shows an example of thermo-responsive Cd(II) complex 15, Cd(Sal)4(PyNH2)2 (Sal = 2-formylphenolato, PyNH2 = 4-aminopyridine), reported by Miao, Nie, and coworkers.103 The crystalline 15 showed white emission at rt. Upon grinding, it was transformed into a green-emissive solid. This amorphous solid displayed thermo-sensitive luminescent changes from green at rt to yellow upon heating to 180 °C. These multistep emission changes of 15 were mainly attributed to the effective phase transformation in the solid state rather than the change of the molecular structure. Remarkably, the plot of color temperature of emission versus the absolute temperature recorded from 323 to 453 K indicated a good linear relationship with a maximum relative sensitivity (Sr) of 0.95% K−1 at 453 K. This demonstrated that complex 15 can be used as an accurate and sensitive solid luminescent thermometer.
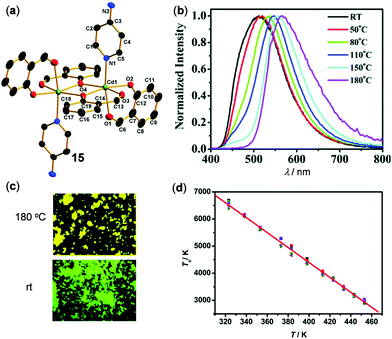 |
| Fig. 14 (a) Single crystal structure of Cd(II) complex 15. (b) Temperature-dependent emission spectral changes of amorphous 15 in the range of 25–180 °C. (c) Photographs showing the emission color change of 15 at rt vs. 180 °C. (d) The linear relationship (R2 = 0.997) of color temperature (Tc) versus heating temperature (T). Reprinted with permission from ref. 103. Copyright 2019 The Royal Society of Chemistry. | |
3.4 Metal complexes with other electronic configurations
In addition to d6, d8, and d10 complexes discussed above and the lanthanide complexes discussed below, luminescent metal complexes with other electronic configurations have been less developed. This is partially limited by the poor emitting properties of complexes with other electronic configurations. However, recent research endeavours have been able to access emissive complexes with d3 and d5 complexes by ligand design and some of them display interesting thermochromic properties. For example, d3 Cr(III) complex 16 with two N,N′-dimethyl-N,N′-dipyridin-2-yl-pyridin-2,6-diamine ligands has been prepared by Heinze and coworkers, which features strong dual emissions at 775 and 739 nm with a Φ of 11%, originated from the metal-centered 2E → 4A2 and 2T1 → 4A2 transition, respectively (Fig. 15).104 This complex can be used as a ratiometric luminescent thermometer in the dispersion or aggregate state within a wide temperature range of 210–373 K. In the case of the dispersed sample in H2O, upon heating from 278 to 358 K, a gradual decrease of the lower-energy emission of 775 nm and a concomitant increase of the higher-energy emission of 739 nm were observed. The Boltzmann fitting plot of the logarithm intensity ratio of the dual emissions, ln(I738/I775), versus the inverse temperature T−1 exhibited a good linear relationship (Fig. 15b).
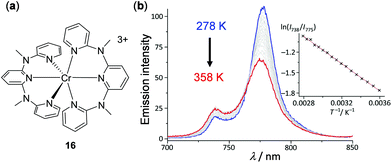 |
| Fig. 15 (a) Molecular structure of Cr(III) complex 16 with BF4− counter anions. (b) Temperature-dependent emission spectra of 16 in air-saturated H2O from 278 to 358 K. Inset: The related Boltzmann fitting plots of ln(I738/I775) vs. T−1. The emission changes are reversible. Reprinted with permission from ref. 104. Copyright 2017 Wiley-VCH. | |
Recently, the research on the development of the earth-abundant d5 Mn(II) complexes and coordination polymers has received increasing attention. A series of organic–inorganic hybrid Mn(II) complexes with three bidentate phosphine oxide ligands, represented by complex 17 in Fig. 16, have been prepared by Artem'ev and coworkers.105a Complex 17 possesses two different coordination models of Mn(II), i.e. the octahedral coordination of the [MnO6]2+ component and the tetrahedral geometry of the [MnBr4]2− counteranion. Upon photoexcitation at 440 nm, it exhibited distinguished dual emissions at 620 and 520 nm with a high total quantum yield of 61% and a lifetime of 12–15 ms. These two emissions are assigned to different metal-centered transitions in octahedral [MnO6]2+ and tetrahedral [MnBr4]2−, respectively. As displayed in Fig. 16b, it showed distinct thermochromic luminescence induced by the dynamic thermal equilibrium of two metal-centered transitions. In addition to discrete complexes, thermochromic Mn(II) clusters or coordination polymers have been known based on single-crystal to single-crystal transformations.105b
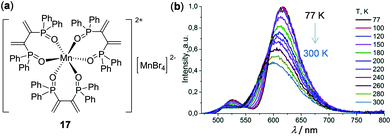 |
| Fig. 16 (a) Molecular structure of Mn(II) complex 17. (b) Temperature-dependent solid-state emission spectra of 17 under excitation at 440 nm in the range of 77–300 K. Reprinted with permission from ref. 105a. Copyright 2018 The Royal Society of Chemistry. | |
3.5 Lanthanide complexes
Lanthanide complexes are important luminescent materials that have been widely applied in various optoelectronic fields. The narrow 4f–4f emission bands and long emission lifetimes of lanthanide complexes are advantageous for temperature sensing and imaging.4 The temperature-sensing ability of a green luminescent Tb(III) complex associated with a back energy transfer from the excited state of Tb(III) 5D4 to the triplet ligand-centered state has been known since 2004.106 Using a similar strategy, a luminescent film based on a mesogenic Tb(III)-diketonate complex 18 has been recently fabricated by Lapaev and coworkers, which exhibited highly-sensitive and reversible temperature-dependent luminescence (Fig. 17).107 Upon cooling from 284 to 143 K, the characteristic multiple emissions corresponding to the 5D4 → 7FJ (J = 6–3) transitions of the ligand-to-metal energy transfer Tb(III) complex were dramatically enhanced, with a mean relative temperature sensitivity of −1.0% K−1. Furthermore, the average luminescence decay time of this film also showed a distinct temperature dependence, with an absolute and relative mean temperature sensitivity of −3.3 μs K−1 and −0.9% K−1, respectively. This demonstrates that complex 18 is a potential thermometer on the basis of both emission intensity and lifetime readouts.
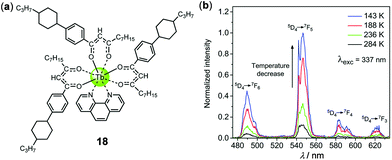 |
| Fig. 17 (a) Molecular structure of Tb(III) complex 18. (b) Temperature-dependent time-delayed emission spectra of a film with a time delay of 2.5 μs. Reprinted with permission from ref. 107. Copyright 2018 The Royal Society of Chemistry. | |
Tb(III) complexes are typically green emissive. In contrast, the constructions of red or near-infrared (NIR) emissive thermometers are advantageous in bioapplications due to the high transparency of red and NIR lights in biological tissues. In this context, NIR luminescent Yb(III) complexes with distinct thermosensitive properties have been demonstrated.108 In addition, highly red-emissive Eu(III) complexes have been reported as luminescent molecular thermometers by a few groups.108–110 Hasegawa and coworkers recently have prepared the mononuclear Eu(III) complex 19 with bright LMCT red emissions (Fig. 18a and b).109 Due to its steric coordination structure and dipole–dipole interactions, complex 19 displayed high thermal stability. Even at a temperature as high as 500 K, bright emission can still be observed. Moreover, complex 19 showed highly temperature-dependent emission lifetimes in a broad temperature range of 300–500 K with a relative sensitivity of −0.62% K−1, making it a good candidate for temperature-sensitive paints in aerospace applications. Recently, one type of stable nanothermometer based on the thermo-responsive Eu(III) complex 20 has been prepared by Tunik and coworkers (Fig. 18c and d).111 Complex 20 in toluene displayed a highly reversible temperature-dependent luminescence and excited-state lifetime in the physiological temperature range of 30–50 °C. The nanothermometer was fabricated by encapsulation of 20 into the assembled nanoparticle copolymer of butyl and methyl methacrylates, which showed mono-exponential lifetime decay with a relative sensitivity of 0.84% K−1 and a temperature resolution of 0.26 K in the presence of human serum albumin (HAS). This work provides a good nanothermometer candidate for biological applications. Beyond these results, a vitrified film of an anisometric Eu(II) β-diketone complex has been recently reported as a reusable luminescent temperature probe with excellent sensitivity in the range of 270–370 K.110
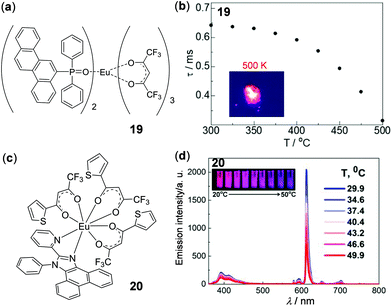 |
| Fig. 18 (a) Molecular structure of Eu(III) complex 19. (b) Plot of emission lifetime of 19versus temperature monitored from 300 to 500 K. Inset: Photograph showing solid bright emission of 19 at 500 K. Reprinted with permission from ref. 109. Copyright 2020 American Chemical Society. (c) Molecular structure of Eu(III) complex 20. (d) Temperature-dependent emission spectra of 20 in toluene. Inset: Emission color changes upon heating from 20 to 50 °C. Reprinted with permission from ref. 111. Copyright 2020 American Chemical Society. | |
In addition to monometallic complexes, dinuclear lanthanide complexes have been explored for temperature sensing.112 In particular, heterodinuclear complexes are appealing for ratiometric temperature sensing by controlling the intramolecular energy transfer process. For this purpose, Hasegawa and coworkers prepared a seven-coordinated Eu(III)/Tb(III) hetero-dimetallic complex 21 bridged by a ditopic phosphine oxide ligand (Fig. 19).113 This complex showed an unexpected red-to-green luminescence change upon increasing the temperature from 100 to 400 K. This phenomenon was attributed to the presence of a LMCT (from EuIII to the tetramethylheptanedionate ligand) state. The complex functioned as a ratiometric thermometer with a relative thermal sensitivity and temperature uncertainty of 2.2% K−1 and 0.01 K, respectively.
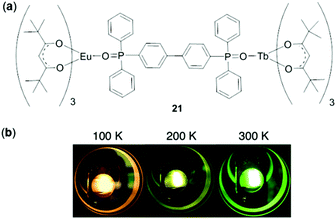 |
| Fig. 19 (a) Molecular structure of the Eu/Tb hetero-dimetallic complex 21. (b) Photographs showing emission colors of 21 at 100, 200, and 300 K, respectively. Reprinted with permission from ref. 113. Copyright 2018 Wiley-VCH. | |
4. Thermo-responsive metal clusters, coordination polymers, and MOFs
The research on luminescent organic–inorganic hybrid metal clusters and MOFs has been rapidly growing in the past few decades, and many of them display interesting thermo-responsive luminescence. These materials are mostly examined in the solid state. In comparison, the small molecular metal complexes discussed in the previous section are investigated in both the solution and solid states. By judicious choice of metal centers and organic ligands of various geometries and functionalities, CPs and MOFs provide an appealing platform for the development of thermo-responsive solid materials, which have been the topics of a few reviews.3,11 We highlight herein some representative works reported in recent years.
4.1 Organic–inorganic hybrid clusters
Copper(I) halide clusters are particularly attractive materials owing to their large variety of photophysical properties associated with an extraordinary structural diversity.114,115 On the basis of a low-energy triplet cluster-centered (3CC) or halide to copper charge transfer (3XMCT) transition at rt and a high-energy triplet halide-to-ligand charge transfer (3XLCT) or 3MLCT transition at low temperatures, a series of thermochromic luminescent materials have been developed in recent years.3 Two examples in this context are shown in Fig. 20. Perruchas and coworkers prepared the cubane Cu4I4 cluster 22, which exhibited temperature-dependent dual emissions (Fig. 20a and b).116 At higher temperatures above 110 °C, the low-energy 3CC emission at 520 nm was almost completely quenched and the high-energy emission at 400 nm caused by the intrinsic emission of the cyanobiphenyl unit was dominant. As the temperature gradually decreased to −150 °C, the 3CC emission progressively enhanced, accompanied by the emission color change from deep blue to cyan. Shown in Fig. 20c and d is an octahedral Cu4I4 cluster (23) recently reported by Kolesnikov and coworkers.117 It showed dual emissions which were both considered to be of 3(X + M)LCT character. Interestingly, with an increase of the temperature from −175 to 125 °C, both emissions at 505 and 621 nm were thermally quenched albeit with different degrees of quenching. Using 23 as a sensitive thermometer, a relative thermal sensitivity of 0.12% K−1 at 24 °C was determined, which is comparable to the best well-known ratiometric luminescent thermometers.
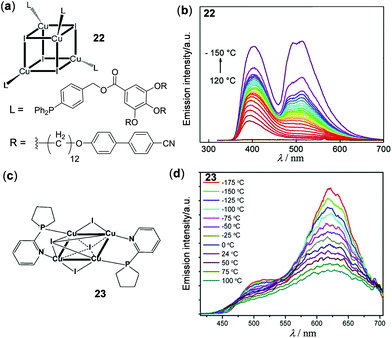 |
| Fig. 20 (a) Chemical structure of the cubane-like Cu4–I4 cluster 22. (b) Temperature-dependent emission spectra of 22 within the temperature range between −150 and 120 °C (λex = 280 nm). Reprinted with permission from ref. 116. Copyright 2016 American Chemical Society. (c) Chemical structure of the octahedral Cu4–I4 cluster 23. (d) Temperature-dependent emission spectra of 23 recorded from −175 to 100 °C. Reprinted with permission from ref. 117. Copyright 2019 American Chemical Society. | |
In addition to Cu4I4 clusters, other copper(I) halide clusters have been reported to show interesting thermo-sensitive properties. For instance, a supramolecular Cu2I2(NH3)2-sandwiched Cu3(pyrazolate)3 adduct 24 stabilized by multiple intermolecular Cu3⋯I, Cu⋯Cu, and N⋯H interactions was reported by Zhan, Li, and coworkers as a temperature sensor (Fig. 21a and b).118 Upon cooling from 340 to 50 K, the 3CC emission of the solid Cu8I2 cluster at 590 nm was linearly enhanced with a relative sensitivity of −0.685% K−1. At around the same time, an unusual [I@Cu8(HMTZ)(MTZ)7] (HMTZ = 1-{2-(N,N-dimethylamino)ethyl}-5-mercapto-1H-tetrazole) Cu(I) cluster 25 was reported to show reversible temperature-dependent dual emissions (Fig. 21c and d).119 At rt, the powder of 25 exhibited a red emission band at 724 nm (τ = 268 ns, Φ = 3.7%) with a weak shoulder green emission band at 512 nm (τ = 32.3 ns). Upon cooling from 300 to 80 K, the green emission of 25 was enhanced much faster than the red one, causing the emission color to change from red to orange, yellow, and green, successively. As a promising ratiometric thermometer, the emission intensity ratio between 512 and 724 nm was found to display a linear relationship in the range of 80–150 K with a relative sensitivity of 8.98% K−1 and a power function relation in the range of 150–250 K with a relative sensitivity of 8.32% K−1.
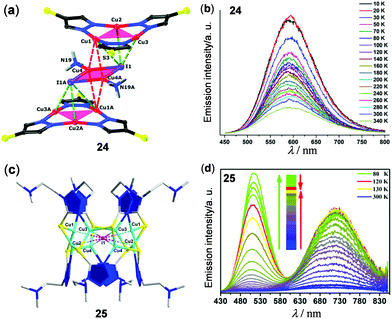 |
| Fig. 21 (a) Crystal structure of the Cu3–Cu2–Cu3 cluster 24. (b) Temperature-dependent solid-state luminescence spectra of 24 monitored from 10 K to 340 K (λex = 345 nm). Reprinted with permission from ref. 118. Copyright 2018 The Royal Society of Chemistry. (c) Perspective view of the I@Cu8 cluster 25 viewed along the a axis. (d) Normalized temperature-dependent emission spectra of 25 in the solid state within the temperature range of 80–300 K. Reprinted with permission from ref. 119. Copyright 2017 American Chemical Society. | |
Luminescent Ag(I) and Au(I) clusters have also received attention as solid thermo-responsive materials. For example, a Ag–S hybrid supertetrahedral cluster 26 with a discrete zero-dimensional V3,4-type structure has been constructed by Sun and coworkers (Fig. 22).76 This material showed highly-sensitive temperature-dependent luminescence. The solid of 26 is only weakly emissive at rt. Upon cooling to 80 K, it displayed brightly yellow 3LMCT emission at around 570 nm. The maximum emission peak at 180 K is slightly blue-shifted with respect to that at rt, possibly induced by the restricted peripheral pyridyl/phenyl rotation upon cooling. Additionally, the good linear relationship of maximum emission intensity versus temperature in the range of 80–180 K made 26 a good thermometer candidate in the low-temperature region.
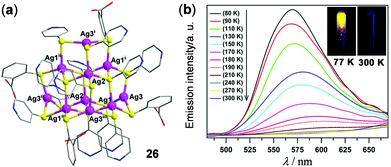 |
| Fig. 22 (a) Crystal structure of the Ag–S hybrid cluster 26. (b) Temperature-dependent luminescence spectra of 26 in the solid state within the temperature range of 90–300 K. Inset: Photographs showing the emission color of 26 at 77 and 300 K under 365 nm irradiation. Reprinted with permission from ref. 76. Copyright 2017 Wiley-VCH. | |
The above clusters are used as solid materials to sense temperature. In contrast, ultra bright luminescent Au nanoclusters (NCs) are in particular useful in various biomedical applications owing to their small size and good photostability and biocompatibility.120,121 In a recent example, Sarkar and coworkers reported the 6-aza-2-thiothymine/L-arginine stabilized Au NCs 27 with an average diameter of 1.5 nm for temperature-sensitive applications (Fig. 23).122 Upon heating from 15 to 55 °C in water, the green emission at 525 nm of 27 (Φ = 30%, τavg = 46 ns) was linearly decreased without a detectable change of the emission maxima. Based on this phenomenon, an average sensitivity of −2.1% per °C in the physiological temperature range of 15–55 °C was calculated. Moreover, by employing the fluorescence lifetime imaging microscopy (FLIM) technique, the intracellular temperature changes of MG-63 cells with the internalized NCs 27 can be in situ monitored by the emission lifetime and intensity changes with high sensitivity and resolution, demonstrating the great potential of Au NCs as bio-nanothermometers (Fig. 23c).
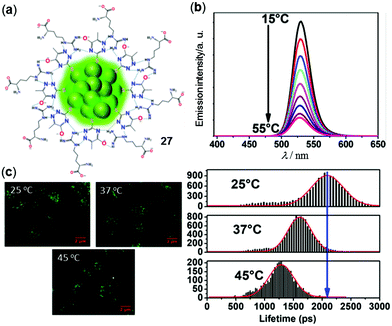 |
| Fig. 23 (a) Chemical structural mode of Au NCs 27. (b) Temperature-dependent emission spectra of 27 in water in the range of 15–55 °C (λex = 375 nm). (c) Left: FLIM images of MG-63 cells with the internalized NCs 27 at 25, 37, and 45 °C. Right: Lifetime distribution histogram of intracellular NCs showing the changes in the lifetime and emission intensity at different temperatures. Reprinted with permission from ref. 122. Copyright 2019 American Chemical Society. | |
Considering the rich luminescence properties of lanthanide complexes, metal clusters with lanthanide ions have been prepared to show thermo-responsive behaviour. Lu and coworkers prepared Eu(III)5–Ti(IV)4 and Eu(III)8–Ti(IV)10 clusters and found them to have temperature-sensing abilities from 180 to 240 K of 0.31% and 0.74%, respectively.123 The temperature dependent emission response of a Eu(III)2–Na2 cluster was reported by Bruno and cowokers.124 In addition, Wu and coworkers prepared the edge-defective cubane-like hetero-dimetallic Zn2Ln2 clusters 28a and 28b (Fig. 24).125 By incorporation of different lanthanides, either ligand-based yellow-green emission (28a, Ln = Gd) or lanthanide-based red emission (28b, Ln = Eu) was selectively observed upon decreasing the temperature.
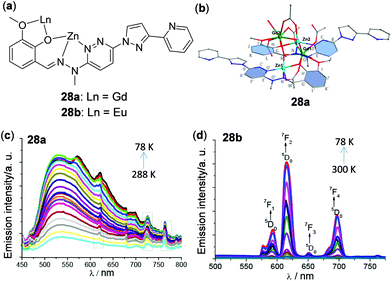 |
| Fig. 24 (a) Structure segment of the Zn2Ln2 clusters 28a and 28b. (b) Cation core structure of 28a. (c and d) Temperature-dependent emission spectra of (c) 28a (78–288 K) and (d) 28b (78–300 K) with excitation at 350 nm. The three sharp peaks marked with a gray rectangle in panel (c) are the emission spectra of a background light source. Reprinted with permission from ref. 125. Copyright 2017 American Chemical Society. | |
Recently, Calvez and coworkers reported a series of hexalanthanide clusters with m-halogenobenzoic acid ligands as solid molecular thermometric probes.126 Shown in Fig. 25 is the structure of a hexa-Dy cluster 29, [Dy6(3-bb)14] (3-bb = m-bromo-benzoic acid). By tuning the metal ion compositions, a series of thermochromic homo and heterodimetallic clusters have been obtained. For instance, upon cooling from 300 to 80 K, the Eu(III)-centered red emission of the [Tb4Eu2(3-bb)14] cluster 30 was gradually diminished, while the green emission originated from the Tb(III)-centered excited state became dominant (Fig. 25b). These temperature-dependent emissions were mainly induced by the tunable ligand-involved intermetallic energy transfer.
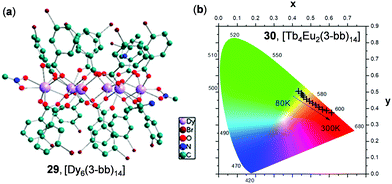 |
| Fig. 25 (a) The core chemical structure of the Dy6 cluster 29 (3-bb = 3-bromobenzonate). (b) Temperature-dependent CIE chromaticity coordinates of 30, an isostructural Tb4Eu2 cluster, in the range of 80–300 K (λex = 298 nm). Reprinted with permission from ref. 126. Copyright 2019 American Chemical Society. | |
4.2 Coordination polymers and frameworks
Luminescent CPs and frameworks have been widely investigated recently. Many of them display solid-state luminescent thermochromism. Among them, copper CPs are the focus of many research groups. One-dimensional (1D) coordination polymer 31 based on double Cu2I2 chains has been reported by the simple reaction of CuI and methyl 2-amino-isonicotinate by Amo-Ochoa and coworkers (Fig. 26a and b)127 At rt, 31 is barely emissive. Upon cooling to 80 K, it exhibits bright yellow emission. The large emission enhancement was ascribed to an increase of structural rigidity. Thin film composite 31@PVDF (4 wt%, PVDF is polyvinylidene difluoride) was subsequently fabricated, which displayed similar reversible thermochromic luminescence. Xie and coworkers prepared the Cu(I) cluster-based 1D polymer 32 from the reaction of cis- and trans-1,2-bis(4-(pyridin-2-yl)phenyl)ethane (cis- and trans-bpype) ligand with the {Cu4I4(PPh3)4} cluster (Fig. 26c–e).128 Polymer 32 showed reversible thermochromic dual luminescence at 516 and 640 nm. Upon cooling from 300 to 80 K, the original green emission at 516 nm, assigned to the ligand-dominated transition, was moderately enhanced. Concurrently, a new red emission band at 640 nm appeared with high intensity, causing a visual emission color change from green to red. The lower-energy red emission of 32 might be attributed to complicated charge transfer processes among Cu(I) metal sites. This study opens a new prospect for the fabrication of thermochromic luminescent CPs based on higher-nuclearity Cu(I) clusters.
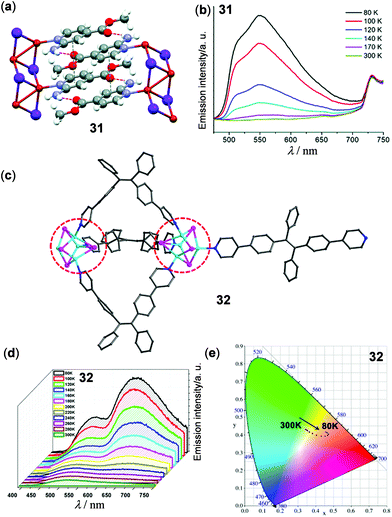 |
| Fig. 26 (a) Crystal structure segment of the 1D Cu(I) polymer 31. (b) Temperature-dependent solid-state emission spectra of 31 in the range of 80–300 K. Reprinted with permission from ref. 127. Copyright 2018 The Royal Society of Chemistry. (c) Asymmetric unit of the 1D Cu(I) polymer 32. (d) Temperature-dependent solid-state emission spectra and (e) CIE chromaticity diagram of 32 in the range of 80–300 K (λex = 380 nm). Reprinted with permission from ref. 128. Copyright 2017 American Chemical Society. | |
In contrast to the rich structural diversity and rich photophysics of Cu(I)-halide multinuclear systems and polymers, luminescent Cu(I)-thiolate materials are less well-known. Demessence and coworkers have fabricated the two-dimensional (2D) Cu–S polymer [Cu(p-SPhCO2Me)]n (33, Fig. 27).129 This 2D sheet exhibited a simple Cu3S3 hexagonal tilling and half-chair conformation without direct cuprophilic interactions. Remarkably, 33 displayed intrinsic triplet emission and reversible thermochromic behavior. At a high temperature of 500 K, the material is barely emissive. Upon cooling to 93 K, two intense green and red emission bands progressively appeared at 560 and 740 nm. The maximum relative sensitivity (Sr) reached 1.37% K−1 at 353 K, suggesting the great potential of 33 as a ratiometric high-temperature thermometer.
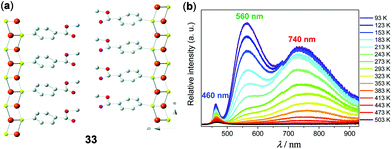 |
| Fig. 27 (a) Structure representation of the 2D Cu–S polymer 33 viewed from the c axis. Orange, Cu; yellow, S; red, O; grey, C. Hydrogen atoms are omitted for clarity. (b) Temperature-dependent solid-state emission spectra of 33 between 93 and 503 K (λex = 380 nm). Reprinted with permission from ref. 129. Copyright 2016 The Royal Society of Chemistry. | |
In addition to 1D and 2D CPs, Cu cluster-based three-dimensional (3D) MOFs with temperature-responsive luminescent properties have been investigated. For instance, the copper halide-based 3D MOF 34, [Cu6I6Br2C16H32N4], has been reported by Xin and coworkers (Fig. 28).65 MOF 34 is composed of a 2-fold interpenetrated pillared-layered framework structure with two different coordination modes of the rhombohedral Cu2I2 dimers with a trans conformation. Depending on the excitation wavelength, MOF 34 displayed different thermochromic luminescence. Under excitation at 290 nm, the two emissions at 500 and 620 nm were both significantly enhanced as the temperature decreased from 300 K down to 6 K (Fig. 28c). In contrast, under the excitation at 350 nm, a distinct shift of the maxima emission peak from 530 to 602 nm was observed (Fig. 28d). The authors deduced that this difference was caused by the different magnitudes of structural changes of two Cu2I2 dimers.
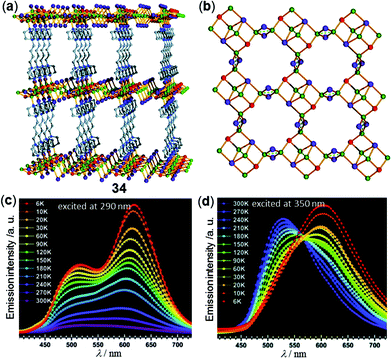 |
| Fig. 28 (a) Independent 3D framework pillared-layered structure and (b) copper-halide single layer structure of Cu-MOF 34. (c and d) Temperature-dependent solid-state emission spectral changes of 34 in the range of 6–300 K excited at (c) 290 nm or (d) 350 nm. Reprinted with permission from ref. 65. Copyright 2018 The Royal Society of Chemistry. | |
Silver-chalcogenolate cluster-based MOFs are a new family of coordination luminescent materials, whose stability is enhanced by the extended network structures. In 2016, Sun and coworkers have reported the [Ag2(pz)(bdc)·H2O]n (pz = pyrazine, and H2bdc = benzene-1,3-dicarboxylic acid) MOF to show two-step thermo-responsive luminescence mainly induced by the single-crystal to single-crystal phase transition.130 Two years later, Zang and coworkers reported the luminescent hydrogen-bonded pillared-layered silver MOF, [Ag10(SBut)6(CF3COO)2(PhPO3H)2(bpy)2]n (35) to display thermochromic properties (Fig. 29).131 MOF 35 showed typical nonradiative decay at 518 nm with increasing temperature (Fig. 29c). Interestingly, the solvent-included MOF 35·CHCl3 displayed multistep emission spectral (534 → 522 → 579 nm) and color (green to yellow) changes upon heating from 83 to 213 K (Fig. 29d), suggesting the potential of MOF materials in modulating the thermochromic properties by host–guest chemistry.132
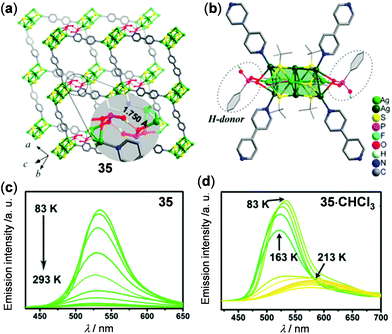 |
| Fig. 29 (a) Two-layer stack and (b) perspective view of the coordination environment of Ag-MOF 35. (c and d) Temperature-dependent solid-state emission spectral change of (c) 35 and (d) 35·CHCl3. Reprinted with permission from ref. 131. Copyright 2018 American Chemical Society. | |
In addition to Cu and Ag materials, luminescent Zn(II) polymers and MOFs have been shown to possess interesting thermochromic properties. A recent report by Tom and coworkers presented the 2D layered Zn(II) polymer 37, which was prepared from Zn(NO3)2 with the 2,3-butanedionebisisonicotinylhydrazone ligand 36 (Fig. 30).133 Polymer 37 is characterized by a 4-connected unimodal 2D layered framework and reversible temperature-dependent luminescence. Upon heating from 30 to 100 °C, the intense LMCT yellow emission at 550 nm of 37 shifted progressively to 600 nm with a partial decrease of the emission intensity. This phenomenon was induced by the proposition of fast dehydration/hydration processes without alternation of the crystal structure of 37. Polymer 37 could be used as a wavelength- and intensity-based thermometer with a maximum relative sensitivity of 0.02% at 45 °C and 0.04% at 70 °C, respectively. As a demonstration in document security application, the patterned security feature of “2019” encrypted with thermochromic 37 could be readily visualized in red emission colors upon heating to 80 °C (Fig. 30c).
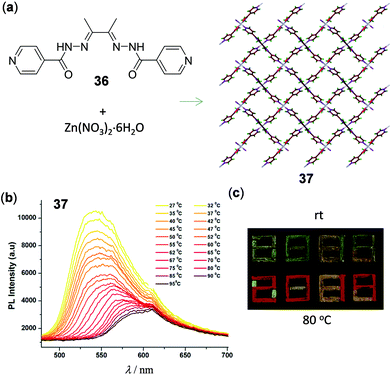 |
| Fig. 30 (a) Schematic representation of the synthesis of 2D Zn(II) polymer 37. (b) Temperature-dependent solid-state emission spectra of 37 in the range of 27–95 °C. (c) Photographs under UV irradiation of 37 at ambient temperature and 80 °C, respectively. Reprinted with permission from ref. 133. Copyright 2020 The Royal Society of Chemistry. | |
As an example of 3D thermo-responsive luminescent Zn(II) MOFs, a mixed-ligand MOF ZnATZ-BTB (ATZ = 5-amino-1-H-tetrazolate, BTB = 1,3,5-tris(4-carboxyphenyl)-benzene) has been constructed by Wu and coworkers to function as a ratiometric thermometer at cryogenic temperatures.134 In addition, based on the dual emissive 5-(2-(5-fluoro-2-hydroxyphenyl)-4,5-bis(4-fluorophenyl)-1H-imidazol-1-yl)isophthalic acid ligand 38 (H2hpi2cf) with the characteristic excited state intramolecular proton transfer (ESIPT) properties, a robust microporous (<3 Å) Zn(II) MOF 39, Zn(hpi2cf)(DMF)(H2O), with temperature-dependent luminescence was fabricated by Su, Pan, and coworkers (Fig. 31).135 Upon heating or cooling, green and blue emissions of 39 were reversibly switched, in which the ESIPT process triggered by the interconversion of hydrated and dehydrated crystalline phases was proposed to play a significant role. Subsequently, ZnO-supported hybrid films based on 39 were prepared, which exhibited potential applications in the detection of trace water (<0.05%, v/v) and thermal imaging (Fig. 31c). Apart from these results, Yan and coworkers recently constructed a Zn5-cluster based MOF with 5-bromoisophthalic acid and 2-methylimidazole mixed ligands to show temperature-responsive dual fluorescence and room temperature phosphorescence.136 Li and coworkers have fabricated a series of dye–MOF composites by employing the biocompatible Zn(II) MOF, Zn3(benzene-1,3,5-tricarboxyl)2(adenine)(H2O) as a host and different thermo-sensitive organic dyes as guests.137 By making full use of the energy transfer between the frameworks and incubated dyes, highly sensitive and reversible ratiometric thermometers were realized under physiological cellular conditions.
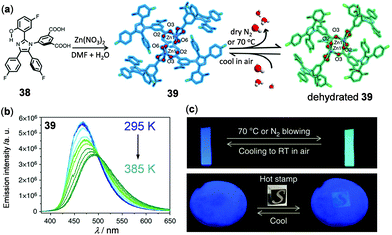 |
| Fig. 31 (a) Schematic diagram showing the synthetic route and structural transformation of Zn MOF 39. (b) Temperature-dependent emission spectral changes of 39 microcrystals. (c) Photographs showing the thermochromic applications of the ZnO-supporting hybrid film of 39. Up: Blue and green emission color switching upon heating/N2 blowing and cooling. Below: Thermal imaging using a heated stone seal of 100 °C on the paper film. Reprinted with permission from ref. 135. Copyright 2017 Nature. | |
Similar to the small molecular lanthanide complexes, lanthanide CPs and MOFs are characterized by strong and narrow solid-state emissions with long lifetimes. The applications of lanthanide CPs and MOFs in temperature sensing have been well-established.138–140 In a classical example, Zhou and coworkers reported the isostructural lanthanide MOF (Me2NH2)3[Eu3(FDC)4(NO3)4]·4H2O (H2FDC = 9-fluorenone-2,7-dicarboxylic acid) to display ratiometric temperature sensing over a wide temperature range (12–320 K) with a high maximum relative sensitivity of 2.3% K−1 at 140 K.141 In addition, a single phosphonate-based MOF 40, [Tb(H5btp)]·2H2O [H8btp = (1,1′-biphenyl)-3,3′,5,5′-tetrayltetrakis(phosphonic acid)], was disclosed by Rocha and coworkers (Fig. 32).142 MOF 40 contained an uncommon slightly distorted {TbO6} octahedron and lozenge-shaped tubular channels through intermolecular π–π interactions (d = 3.856 Å) of adjacent biphenyl ligands. Upon heating from 299 to 319 K, the ligand-centered emission at 316–386 nm, the ligand-associated excimer emission at 390–476 nm, and the 5D4 → 7F5 emission of Tb(III) at 490–560 nm all decreased linearly as a function of temperature, with the maximum relative errors of 1% at 313 K, 1.3% at 299 K, and 0.4% at 301 K, respectively.
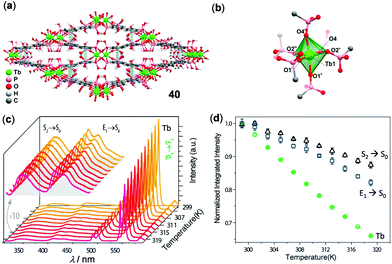 |
| Fig. 32 (a) Crystal packing network and (b) Tb(III) octahedral coordination environment of Tb MOF 40. (c) Temperature-dependent solid-state emission spectral change of 40 in the range of 299–319 K (λex = 300 nm). (d) Temperature dependence of integrated intensities of different emissions. Reprinted with permission from ref. 142. Copyright 2017 American Chemical Society. | |
In addition to homometallic CPs or MOFs, a number of lanthanide coordination materials with two different metal ions have been reported for the purpose of ratiometric temperature sensing. In a classical example, Hasegawa reported the color-changeable Tb(III)/Eu(III) mixed 1D CPs, bridged by a ditopic phosphine oxide ligand, to display highly sensitive ratiometric temperature sensing over a wide range (200–500 K).143 Recently, phenanthroline 1D CPs grafted with Eu(III) and Tb(III) trifluoactylacetone (tfac) complexes have been reported as a noncontact temperature sensor in the range of 260–460 K.144 Based on the energy transfer mechanism, Zaręba and coworkers reported the Eu/Tb-co-doped 2D CPs 41 with good three-photon absorption capability, which could be used as a NIR-to-VIS ratiometric luminescent thermometer under the excitation of NIR light (Fig. 33).145 Polymer 41 exhibited a 2D, three-connected coordination network with a honeycomb-like layer. More interestingly, under the three-photon excitation at 800 nm, the emission of 41 showed a similar temperature-dependency of emission in the range of 299–373 K with respect to that under one-photon excitation at 337 nm. Upon heating from 299 K to 373 K, the green emission of the 5D4 → 7F5 transition of Tb(III) dropped abruptly while the red emission of the 5D0 → 7F2 excited state of Eu(III) increased slightly, in accompany with an obvious solid emission color change from green to red. The relative sensitivity under the three-photon excitation at 800 nm was calculated to be 2.91% K−1, demonstrating the possibility of NIR light-excited optical thermosensing.
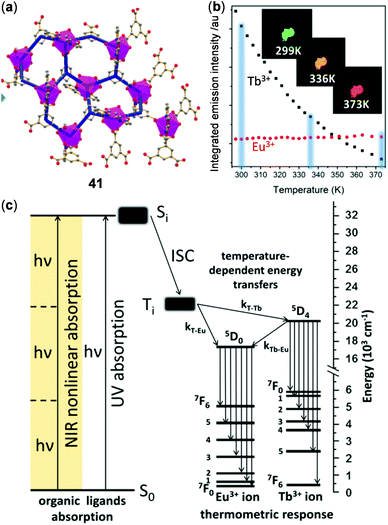 |
| Fig. 33 (a) Honeycomb-like Eu/Tb mixed 2D coordination polymer 41. (b) Plots of the integrated intensity of 5D0 → 7F2 of Eu(III) (red dots) and 5D4 → 7F5 of Tb(III) (black dots) f–f emission as a function of temperature (excited at 337 nm). Insets: Photographs showing the emission color of 41 at 299, 336, and 373 K, respectively. (c) Energy level diagram highlighting the use of three-photon excitation for effecting the thermometric luminescent response. Reprinted with permission from ref. 145. Copyright 2019 American Chemical Society. | |
In the case of thermo-sensitive Tb(III)/Eu(III) mixed 3D MOFs, the first report was given by Qian and coworkers.73 Stimulated by this study, a great number of mixed MOFs have been fabricated for the purpose of temperature sensing.11 Two of the recently reported examples are highlighted below. Zhao, Qian, and coworkers reported the Eu/Tb mixed MOF 42, Eu0.05Tb0.95FTPTC (H4FTPTC = 2′-fluoro-[1,1′:4′,1′′-terphenyl]-3,3′′,5,5′′-tetracarboxylic acid), which featured a 3D network and distorted tricapped trigonal prism coordination of Ln atoms (Fig. 34a and b).146 As a result of the thermal-driven energy transfer from Tb(III) to Eu(III), 42 showed a reversible linear response to temperature with high relative sensitivity (9.11% K−1 at 125 K) in the cryogenic range of 25–125 K. In addition, Su and coworkers synthesized the Eu(III)/Tb(III) mixed MOF 43, [(CH3)2NH2]Eu0.036Tb0.964BPTC (BPTC is the deprotonated form of biphenyl-3,3′,5,5′-tetracarboxylic acid), which showed highly-sensitive temperature-dependent ratiometric and colormetric luminescence (Fig. 34c and d).72 Upon heating from 167 to 377 K, the emission intensity of the Tb(III) 5D4 → 7F5 transition of 43 decreased by about 89%, while the emission intensity of the Eu(III) 5D0 → 7F2 transition showed a 4-fold enhancement. Concurrently, the solid emission color of 43 gradually changed from green to yellow and red. The plot of the Tb/Eu emission intensity ratio versus temperature in the 220–310 K range displayed a good linear relationship with a high maximum relative sensitivity of 9.42% K−1 at 310 K.
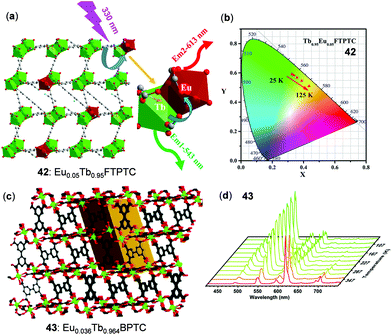 |
| Fig. 34 (a) Schematic representation of the resonance energy transfer mechanism of MOF 42. (b) Temperature-dependent CIE chromaticity coordinates of 42 within the range of 25–125 K (λex = 350 nm). Reprinted with permission from ref. 146. Copyright 2018 American Chemical Society. (c) Structure of Ln-MOF 43. (d) Temperature-dependent solid-state emission spectra of 43 between 77 and 377 K (λex = 322 nm). Reprinted with permission from ref. 72. Copyright 2019 The Royal Society of Chemistry. | |
5. Multi-component coordination copolymers and assemblies
Donor–acceptor energy transfer is long known to be highly dependent on temperature. By manipulating the efficiency of energy transfer in materials containing two or more than two chromophores, distinct ratiometric luminescence changes are expected. This has been discussed in bridged dyads, hetero-dimetallic clusters, and mixed-metal MOFs in the previous sections (Fig. 19, 25 and 34).113,116,146 Using a similar strategy, thermo-responsive luminescent assemblies have been developed with tuneable excited states. However, controlled energy transfer is not the only reason for the thermo-responsiveness of assembled or composite materials. Other factors such as reversible coordination/dissociation and changes in the microenvironment polarity have been utilized to fabricate thermo-responsive assemblies.
In order to utilize effective energy transfer between luminescent metal complexes and organic chromophores, Wolfbeis and coworkers presented a nanothermometer by the co-assembly of the thermo-stable blue-emissive polyfluorene 44 and the thermo-sensitive red-emissive Eu(III) complex 45 (Fig. 35).147 In the obtained nanocomposite 46, efficient fluorescence resonant energy transfer (FRET) from the light-harvesting polyfluorene antenna to the doped Eu(III) complex occurred. Under the two-photon excitation at 720 nm at rt, dual emissions in the blue and red region were observed. Upon heating from 270 to 320 K, both emissions decreased gradually. However, relative to the blue emission of polyfluorenes, the red Eu(III) emission dropped much faster. The fitting plot of the Eu and polyfluorene emission ratio versus temperature showed a good linear relationship with a relative sensitivity of 1.6% K−1, suggesting its potential applications as ratiometric nanothermometers. In addition to 46, FRET nanoparticles with the combination of other donors and acceptors are known.148
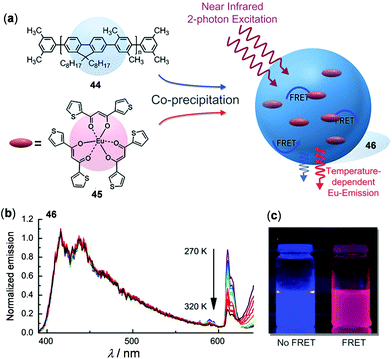 |
| Fig. 35 (a) Molecular structures of 44 and 45 and schematic illustration showing the mechanism of the FRET-based nano-thermometer of the binary-assembled composite 46. (b) Normalized temperature-dependent emission spectra of 46 with two-photon excitation (λex = 720 nm). (c) Photographs exhibiting the emission color change of the nanoparticles before (left) and after precipitation (right) in water under UV irradiation. Reprinted with permission from ref. 147. Copyright 2016 WILEY-VCH. | |
Recently, Zhong, Yao, and coworkers have fabricated a class of highly ordered crystalline phosphorescent nanotubes from the co-assembly of two neutral Ir(III) complexes, 47 and 48, acting as the effective antenna chromophore and energy acceptor, respectively (Fig. 36).149 Due to the excellent light-harvesting and energy transfer process, the phosphorescence color of these nanotubes was finely tuned from green to red by varying temperature. Upon cooling from 298 to 77 K, the red emission of these nanotubes containing 0.06% of acceptor was gradually turned green, which was caused by the inhibited energy transfer at low temperatures.
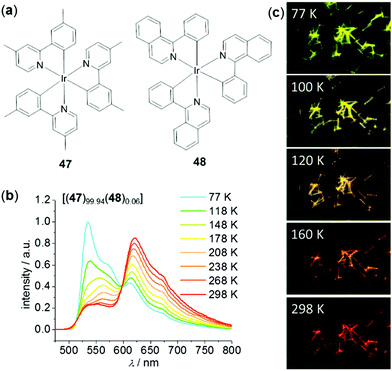 |
| Fig. 36 (a) Molecular structures of donor 47 and acceptor 48. (b) Temperature-dependent emission spectra of nanotube 47 doped with 0.06% of 48 (λex = 375 nm). (c) Fluorescence microscopy images displaying the temperature-dependent emission color changes of the doped nanotubes. Reprinted with permission from ref. 149. Copyright 2018 Wiley-VCH. | |
As an example of thermo-responsive assembled or composite materials that are not based on the energy transfer mechanism, Li and coworkers reported a ratiometric luminescent thermometer through the combination of the Eu(III) complex 50 and the Zn(II) complex 51 prepared from the same tridentate ligand 49 (Fig. 37).150 The composite material 52 was obtained from two individual complexes in a 1
:
19 molar ratio. Upon cooling from 500 to 80 K, the red Eu(III) emission of 52 increased dramatically, whereas the blue emission associated with the Zn(II) component showed a little change. Based on this, a good linear relationship between two emission intensities was observed from 80 to 410 K with a temperature sensitivity of 0.896% K−1. The excellent thermo-responsiveness of 52 was considered to be mainly attributed to the dynamic coordination (at low temperatures) and dissociation (at higher temperatures) of the Eu(III) component. However, temperature had little effect on the Zn(II) component and it simply behaved as an internal reference for the ratiometric sensing. No intermetallic energy transfer between Eu(III) and Zn(II) was involved in this system.
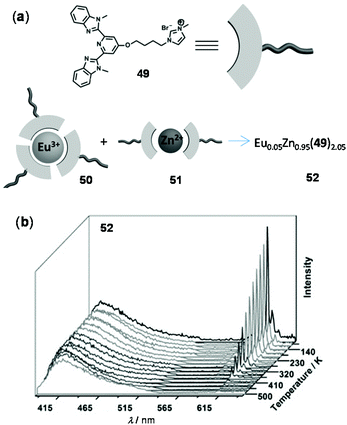 |
| Fig. 37 (a) Molecular structures of 49–52. (b) Temperature-dependent emission spectra of 52 recorded from 80 to 500 K. Reprinted with permission from ref. 150. Copyright 2015 Wiley-VCH. | |
The combination of two or more chromophores through copolymerization is another appealing strategy to construct ratiometric luminescent thermo-responsive materials. Zhao, Huang, and coworkers prepared copolymer 53 by introducing two different Ir(III) complexes into the thermosensitive poly N-isopropylacrylamide backbone for the in vitro and in vivo temperature sensing and imaging (Fig. 38).151 Upon increasing temperature, this polymer underwent a coil–globule transition, leading to a decrease in microenvironment polarity surrounding the Ir(III) complexes and hence enhanced the emissions of both Ir(III) complexes. However, owing to the different sensitivity to the surrounding environment, the green/red emission intensity ratio was linearly correlated with temperature. Thus, polymer 53 has been successfully used for temperature sensing and imaging of HeLa cells (Fig. 38c) and zebrafish. Recently, the same research group reported another copolymer 54 containing a green emissive Ir(III) component and a red emissive Eu(III) component (Fig. 38d).79 In addition to the application as a ratiometric temperature probe in the physiological temperature range, polymer 54 also exhibited a high temperature-dependence of excited state lifetimes, making it applicable for temperature-dependent ratiometric luminescence imaging and phosphorescent lifetime imaging in biological systems.
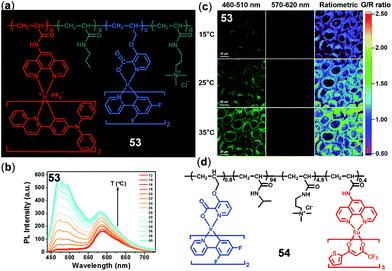 |
| Fig. 38 (a) Chemical structure of copolymer 53 (a/b/c/d = 0.11/100/0.89/2.32). (b) Temperature-dependent emission spectra of 53 (0.01 w/v%) in PBS buffer (12–40 °C). (c) Confocal laser scanning microscopy images of HeLa cells labelled with 53 at 15 °C (top), 25 °C (middle), and 35 °C (bottom). Reprinted with permission from ref. 151. Copyright 2016 WILEY-VCH. (d) Chemical structure of copolymer 54. Reprinted with permission from ref. 79. Copyright 2018 American Chemical Society. | |
6. Conclusion
In summary, this review provides the fundamentals and design strategies for the development of thermo-responsive metal–ligand coordination materials. The thermo-responsiveness is commonly read out by the signal changes in the emission intensity, maximum emission wavelength, or excited-state lifetime, with more than one parameter changes being involved in many systems. This temperature-responsiveness could be caused by the thermal-activated non-radiative transition, the thermal equilibrium of different excited states, molecular structural or conformation changes, the changes in molecular stacking, controlled inter-/intramolecular energy transfer, changes in the microenvironment polarity, etc. Ratiometric luminescence response is particularly appealing for highly-sensitive temperature sensing and imaging, which can be realized on molecular dyads, mixed-metal clusters, MOFs, host–guest systems, and binary polymers and assemblies.
Among the materials surveyed in this review, small molecular metal complexes with well-determined molecular structures are particularly useful for studies in the solution state. In addition, they could be used as building blocks to prepare thermo-responsive polymers and assemblies with tailored sizes for biomedical applications. In comparison, metal clusters and MOFs are particularly used as solid-state thermo probes owing to their high thermal stability. They could also be good candidates for applications under harsh conditions, such as cryogenic conditions or a high-temperature environment. In addition to these materials, another type of thermo-responsive luminescent material is thermally activated delayed fluorescence (TADF) compounds, which have received intense interest but not discussed in this review. We are aware that the topic of metal complex-based TADF materials, in particular Cu(I), Ag(I), Au(I) complexes, has been recently reviewed by Yersin, Li, and coworkers.152 Although these complexes have been mainly investigated as OLED materials in the current stage, the exploitation of the thermo-responsive nature of these materials is expected to receive growing attention in the future.
Regarding the future research in this field, attention could also be paid to the preparation of non-precious earth-abundant metal complexes with distinct thermo-responsive properties, e.g. Cr, Mn, W complexes, and the incorporation of these complexes into more sophisticated systems. In addition, the thermo input and luminescence readout could be combined with other stimulus and readout means, such as optical, magnetic, electrical, and chemical signals to develop multifunctional intelligent systems. During the practical applications of thermo-responsive coordination materials, the material stability and robustness, that could ensure high reversibility and long-term cyclability, must be considered.
Conflicts of interest
The authors declare no conflicts of interest.
Acknowledgements
The authors acknowledge the funding support from the National Science Fund for Distinguished Young Scholars (No. 21925112), the National Natural Science Foundation of China (grants 21601194 and 21872154) and the Beijing Natural Science Foundation (grant 2191003).
Notes and references
- X.-d. Wang, O. S. Wolfbeis and R. J. Meier, Luminescent probes and sensors for temperature, Chem. Soc. Rev., 2013, 42, 7834 RSC.
- Y. Cui, F. Zhu, B. Chen and G. Qian, Metal–organic frameworks for luminescence thermometry, Chem. Commun., 2015, 51, 7420 RSC.
- B. Li, H.-T. Fan, S.-Q. Zang, H.-Y. Li and L.-Y. Wang, Metal-containing crystalline luminescent thermochromic materials, Coord. Chem. Rev., 2018, 377, 307 CrossRef CAS.
- Y. Hasegawa and Y. Kitagawa, Thermo-sensitive luminescence of lanthanide complexes, clusters, coordination polymers and metal–organic frameworks with organic photosensitizers, J. Mater. Chem. C, 2019, 7, 7494 RSC.
- Y. Cheng, Y. Gao, H. Lin, F. Huang and Y. Wang, Strategy design for ratiometric luminescence thermometry: circumventing the limitation of thermally coupled levels, J. Mater. Chem. C, 2018, 6, 7462 RSC.
- E. J. McLaurin, L. R. Bradshaw and D. R. Gamelin, Dual-Emitting Nanoscale Temperature Sensors, Chem. Mater., 2013, 25, 1283 CrossRef CAS.
- P. Mahata, S. K. Mondal, D. K. Singha and P. Majee, Luminescent rare-earth-based MOFs as optical sensors, Dalton Trans., 2017, 46, 301 RSC.
- D. Zhao, Y. Cui, Y. Yang and G. Qian, Sensing-functional luminescent metal–organic frameworks, CrystEngComm, 2016, 18, 3746 RSC.
- C. D. S. Brites, P. P. Lima, N. J. O. Silva, A. Millá, V. S. Amaral, F. Palacio and L. D. Carlos, Lanthanide-based luminescent molecular thermometers, New J. Chem., 2011, 35, 1177 RSC.
- F.-Y. Yi, D. Chen, M.-K. Wu, L. Han and H.-L. Jiang, Chemical Sensors Based on Metal–Organic Frameworks, ChemPlusChem, 2016, 81, 675 CrossRef CAS PubMed.
- L. Chen, D. Liu, J. Peng, Q. Du and H. He, Ratiometric fluorescence sensing of metal-organic frameworks: Tactics and perspectives, Coord. Chem. Rev., 2020, 404, 213113 CrossRef CAS.
- X. Zhou, H. Wang, S. Jiang, G. Xiang, X. Tang, X. Luo, L. Li and X. Zhou, Multifunctional Luminescent Material Eu(III) and Tb(III) Complexes with Pyridine-3,5-Dicarboxylic Acid Linker: Crystal Structures, Tunable Emission, Energy Transfer, and Temperature Sensing, Inorg. Chem., 2019, 58, 3780 CrossRef CAS PubMed.
- P. M. Gschwend, D. Niedbalka, L. R. H. Gerken, I. K. Herrmann and S. E. Pratsinis, Simultaneous Nanothermometry and Deep-Tissue Imaging, Adv. Sci., 2020, 7, 2000370 CrossRef PubMed.
- T. Bai and N. Gu, Micro/Nanoscale Thermometry for Cellular Thermal Sensing, Small, 2016, 12, 4590 CrossRef CAS.
- M. Nakano and T. Nagai, Thermometers for monitoring cellular temperature, J. Photochem. Photobiol., C, 2017, 30, 2 CrossRef CAS.
- X. Hu, Y. Li, T. Liu, G. Zhang and S. Liu, Intracellular Cascade FRET for Temperature Imaging of Living Cells with Polymeric Ratiometric Fluorescent Thermometers, ACS Appl. Mater. Interfaces, 2015, 7, 15551 CrossRef CAS.
- J. Dong, Y. Pan, H. Wang, K. Yang, L. Liu, Z. Qiao, Y. D. Yuan, S. B. Peh, J. Zhang, L. Shi, H. Liang, Y. Han, X. Li, J. Jiang, B. Liu and D. Zhao, Self-Assembly of Highly Stable Zirconium(IV) Coordination Cages with Aggregation Induced Emission Molecular Rotors for Live-Cell Imaging, Angew. Chem., Int. Ed., 2020, 59, 10151 CrossRef CAS.
- J. Dong, Z. Qiao, Y. Pan, S. B. Peh, Y. D. Yuan, Y. Wang, L. Zhai, H. Yuan, Y. Cheng, H. Liang, B. Liu and D. Zhao, Encapsulation and Protection of Ultrathin Two-Dimensional Porous Organic Nanosheets within Biocompatible Metal–Organic Frameworks for Live-Cell Imaging, Chem. Mater., 2019, 31, 4897 CrossRef CAS.
- B. d. Rosal, E. Ximendes, U. Rocha and D. Jaque, In Vivo Luminescence Nanothermometry: from Materials to Applications, Adv. Opt. Mater., 2017, 5, 1600508 CrossRef.
- S. Xu, Y. Yu, Y. Gao, Y. Zhang, X. Li, J. Zhang, Y. Wang and B. Chen, Mesoporous silica coating NaYF4:Yb,Er@NaYF4 upconversion nanoparticles loaded with ruthenium(II) complex nanoparticles: Fluorometric sensing and cellular imaging of temperature by upconversion and of oxygen by downconversion, Microchim. Acta, 2018, 185, 454 CrossRef PubMed.
- P. Wang, H. Wang, Y. Fang, H. Li, J. He, Y. Ji, Y. Li, Q. Xu, J. Zheng and J. Lu, Thermoresponsive Memory Behavior in Metallosupramolecular Polymer-Based Ternary Memory Devices, ACS Appl. Mater. Interfaces, 2017, 9, 32930 CrossRef CAS.
- Y. Ye, W. Zhang, Z. Zhao, J. Wang, C. Liu, Z. Deng, X. Zhao and J. Han, Highly Luminescent Cesium Lead Halide Perovskite Nanocrystals Stabilized in Glasses for Light-Emitting Applications, Adv. Opt. Mater., 2019, 7, 1801663 CrossRef.
- A. Seeboth, R. Ruhmann and O. Mühling, Thermotropic and Thermochromic Polymer Based Materials for Adaptive Solar Control, Materials, 2010, 3, 5143 CrossRef CAS PubMed.
- M. Li, Z. Yang, Y. Ren, J. Ruan, J. Qiu and Z. Song, Reversible Modulated Upconversion Luminescence of MoO3:Yb3+,Er3+ Thermochromic Phosphor for Switching Devices, Inorg. Chem., 2019, 58, 6950 CrossRef CAS.
- J. Li, X. Liu, P. Cui, J. Li, T. Ye, X. Wang, C. Zhang and Y. S. Zhao, Lead-free thermochromic perovskites with tunable transition temperatures for smart window applications, Sci. China: Chem., 2019, 62, 1257 CrossRef CAS.
- S. T. Zimmermann, D. W. R. Balkenende, A. Lavrenova, C. Weder and J. Brugger, Nanopatterning of a Stimuli-Responsive Fluorescent Supramolecular Polymer by Thermal Scanning Probe Lithography, ACS Appl. Mater. Interfaces, 2017, 9, 41454 CrossRef CAS PubMed.
- S. Srinivasan, P. A. Babu, S. Mahesh and A. Ajayaghosh, Reversible Self-Assembly of Entrapped Fluorescent Gelators in Polymerized Styrene Gel Matrix: Erasable Thermal Imaging via Recreation of Supramolecular Architectures, J. Am. Chem. Soc., 2009, 131, 15122 CrossRef CAS PubMed.
- B. Yoon, H. Shin, E.-M. Kang, D. W. Cho, K. Shin, H. Chung, C. W. Lee and J.-M. Kim, Inkjet-Compatible Single-Component Polydiacetylene Precursors for Thermochromic Paper Sensors, ACS Appl. Mater. Interfaces, 2013, 5, 4527 CrossRef CAS PubMed.
- A. Seeboth, D. Lötzsch, R. Ruhmann and O. Muehling, Thermochromic Polymers—Function by Design, Chem. Rev., 2014, 114, 3037 CrossRef CAS PubMed.
- M. M. Ogle, A. D. S. McWilliams, B. Jiang and A. A. Martí, Latest Trends in Temperature Sensing by Molecular Probes, ChemPhotoChem, 2020, 4, 255 CrossRef CAS.
- S. Liang, Y. Wang, X. Wu, M. Chen, L. Mu, G. She and W. Shi, An ultrasensitive ratiometric fluorescent thermometer based on frustrated static excimers in the physiological temperature range, Chem. Commun., 2019, 55, 3509 RSC.
- A. Lavrenova, D. W. R. Balkenende, Y. Sagara, S. Schrettl, Y. C. Simon and C. Weder, Mechano- and Thermoresponsive Photoluminescent Supramolecular Polymer, J. Am. Chem. Soc., 2017, 139, 4302 CrossRef CAS PubMed.
- J.-F. Chen, X. Yin, B. Wang, K. Zhang, G. Meng, S. Zhang, Y. Shi, N. Wang, S. Wang and P. Chen, Planar Chiral Organoboranes with Thermoresponsive Emission and Circularly Polarized Luminescence: Integration of Pillar[5]arenes with Boron Chemistry, Angew. Chem., 2020, 59, 11267 CrossRef CAS PubMed.
- J. Zhang, A. Li, H. Zou, J. Peng, J. Guo, W. Wu, H. Zhang, J. Zhang, X. Gu, W. Xu, S. Xu, S. H. Liu, A. Qin, J. W. Y. Lama and B. Z. Tang, A “simple” donor–acceptor AIEgen with multi-stimuli responsive behaviour, Mater. Horiz., 2020, 7, 135 RSC.
- J.-H. Kim, Y. Jung, D. Lee and W.-D. Jang, Thermoresponsive Polymer and Fluorescent Dye Hybrids for Tunable Multicolor Emission, Adv. Mater., 2016, 28, 3499 CrossRef CAS PubMed.
- S. Mukherjee and P. Thilagar, Stimuli and shape responsive ‘boron-containing’ luminescent organic materials, J. Mater. Chem. C, 2016, 4, 2647 RSC.
- T. Jiang, X. Wang, J. Wang, G. Hu and X. Ma, Humidity- and Temperature-Tunable Multicolor Luminescence of Cucurbit[8]uril-Based Supramolecular Assembly, ACS Appl. Mater. Interfaces, 2019, 11, 14399 CrossRef CAS PubMed.
- Y. Li, Z. Li, Y. Hou, Y.-N. Fan and C.-Y. Su, Photoluminescent Phosphinine Cu(I) Halide Complexes: Temperature Dependence of the Photophysical Properties and Applications as a Molecular Thermometer, Inorg. Chem., 2018, 57, 13235 CrossRef CAS PubMed.
- Y. Ai, Y. Li, H. L.-K. Fu, A. K.-W. Chan and V. W.-W. Yam, Aggregation and Tunable Color Emission Behaviors of l -Glutamine-Derived Platinum(II) Bipyridine Complexes by Hydrogen-Bonding, π–π Stacking and Metal–Metal Interactions, Chem. – Eur. J., 2019, 25, 5251 CrossRef CAS PubMed.
- Z. Zhang, Z. Zhao, L. Wu, S. Lu, S. Ling, G. Li, L. Xu, L. Ma, Y. Hou, X. Wang, X. Li, G. He, K. Wang, B. Zou and M. Zhang, Emissive Platinum(II) Cages with Reverse Fluorescence Resonance Energy Transfer for Multiple Sensing, J. Am. Chem. Soc., 2020, 142, 2592 CrossRef CAS PubMed.
- M. Yamamoto, Y. Kitagawa, T. Nakanishi, K. Fushimi and Y. Hasegawa, Ligand-Assisted Back Energy Transfer in Luminescent TbIII Complexes for Thermosensing Properties, Chem. – Eur. J., 2018, 24, 17719 CrossRef CAS PubMed.
- K. Ohno, Y. Kusano, S. Kaizaki, A. Nagasawa and T. Fujihara, Chromism of Tartrate-Bridged Clamshell-like Platinum(II) Complex: Intramolecular Pt–Pt Interaction-Induced Luminescence Vapochromism and Intermolecular Interactions-Triggered Thermochromism, Inorg. Chem., 2018, 57, 14159 CrossRef CAS PubMed.
- K. Chen and V. J. Catalano, Luminescent Thermochromism in a Gold(I)–Copper(I) Phosphine–Pyridine Complex, Eur. J. Inorg. Chem., 2015, 5254 CAS.
- A. Kobayashi and M. Kato, Stimuli-responsive Luminescent Copper(I) Complexes for Intelligent Emissive Devices, Chem. Lett., 2017, 46, 154 CrossRef CAS.
- W. P. Lustig, S. Mukherjee, N. D. Rudd, A. V. Desai, J. Li and S. K. Ghosh, Metal–organic frameworks: functional luminescent and photonic materials for sensing applications, Chem. Soc. Rev., 2017, 46, 3242 RSC.
- J. Rocha, C. D. S. Brites and L. D. Carlos, Lanthanide Organic Framework Luminescent Thermometers, Chem. – Eur. J., 2016, 22, 14782 CrossRef CAS PubMed.
- L. Qiu, C. Yu, X. Wang, Y. Xie, A. M. Kirillov, W. Huang, J. Li, P. Gao, T. Wu, X. Gu, Q. Nie and D. Wu, Tuning the Solid-State White Light Emission of Postsynthetic Lanthanide-Encapsulated Double-Layer MOFs for Three-Color Luminescent
Thermometry Applications, Inorg. Chem., 2019, 58, 4524 CrossRef CAS PubMed.
- C. Li, K. Wang, J. Li and Q. Zhang, Recent Progress in Stimulus-Responsive Two-Dimensional Metal–Organic Frameworks, ACS Mater. Lett., 2020, 2, 779 CrossRef CAS.
- M.-E. Sun, Y. Li, X.-Y. Dong and S.-Q. Zang, Thermoinduced structural-transformation and thermochromic luminescence in organic manganese chloride crystals, Chem. Sci., 2019, 10, 3836 RSC.
- G. Wang, Z. Li, X. Luo, R. Yue, Y. Shen and N. Ma, DNA-templated nanoparticle complexes for photothermal imaging and labeling of cancer cells, Nanoscale, 2018, 10, 16508 RSC.
- Y. S. Borghei and M. Hosseini, An approach toward miRNA detection via different thermo-responsive aggregation/disaggregation of CdTe quantum dots, RSC Adv., 2018, 8, 30148 RSC.
- C. Bradac, S. F. Lim, H.-C. Chang and I. Aharonovich, Optical Nanoscale Thermometry: From Fundamental Mechanisms to Emerging Practical Applications, Adv. Opt. Mater., 2020, 8, 202000183 CrossRef.
- C. Wang, L. Ling, Y. Yao and Q. Song, One-step synthesis of fluorescent smart thermo-responsive copper clusters: A potential nanothermometer in living cells, Nano Res., 2015, 8, 1975 CrossRef CAS.
- J. Ye, X. Dong, H. Jiang and X. Wang, An intracellular temperature nanoprobe based on biosynthesized fluorescent copper nanoclusters, J. Mater. Chem. B, 2017, 5, 691 RSC.
- E. Hemmer, M. Quintanilla, F. Légaré and F. Vetrone, Temperature-Induced Energy Transfer in Dye-Conjugated Upconverting Nanoparticles: A New Candidate for Nanothermometry, Chem. Mater., 2015, 27, 235 CrossRef CAS.
- V. W.-W. Yam, V. K.-M. Au and S. Y.-L. Leung, Light-Emitting Self-Assembled Materials Based on d8 and d10 Transition Metal Complexes, Chem. Rev., 2015, 115, 7589 CrossRef CAS.
- Y. Han, Z. Gao, C. Wang, R. Zhong and F. Wang, Recent progress on supramolecular assembly of organoplatinum(II) complexes into long-range ordered nanostructures, Coord. Chem. Rev., 2020, 414, 213300 CrossRef CAS.
- O. S. Wenger, Photoactive Complexes with Earth-Abundant Metals, J. Am. Chem. Soc., 2018, 140, 13522 CrossRef CAS PubMed.
- K. Y. Zhang, S. Liu, Q. Zhao and W. Huang, Stimuli–responsive metallopolymers, Coord. Chem. Rev., 2016, 319, 180 CrossRef CAS.
- C. D. S. Brites, S. Balabhadra and L. D. Carlos, Lanthanide-Based Thermometers: At the Cutting-Edge of Luminescence Thermometry, Adv. Opt. Mater., 2019, 7, 1801239 CrossRef.
- C. Yuan, S. Saito, C. Camacho, S. Irle, I. Hisaki and S. Yamaguchi, A π-Conjugated System with Flexibility and Rigidity That Shows Environment-Dependent RGB Luminescence, J. Am. Chem. Soc., 2013, 135, 8842 CrossRef CAS PubMed.
- Y. Cao, J. K. Nagle, M. O. Wolf and B. O. Patrick, Tunable Luminescence of Bithiophene-Based Flexible Lewis Pairs, J. Am. Chem. Soc., 2015, 137, 4888 CrossRef CAS PubMed.
- A. K.-W. Chan, D. Wu, K. M.-C. Wong and V. W.-W. Yam, Rhodium(I) Complexes of Tridentate N-Donor Ligands and Their Supramolecular Assembly Studies, Inorg. Chem., 2016, 55, 3685 CrossRef CAS PubMed.
- L. Liu, X. Wang, F. Hussain, C. Zeng, B. Wang, Z. Li, I. Kozin and S. Wang, Multiresponsive Tetradentate Phosphorescent Metal Complexes as Highly Sensitive and Robust Luminescent Oxygen Sensors: Pd(II) Versus Pt(II) and 1,2,3-Triazolyl Versus 1,2,4-Triazolyl, ACS Appl. Mater. Interfaces, 2019, 11, 12666 CrossRef CAS PubMed.
- B. Xin, J. Sang, Y. Gao, G. Li, Z. Shi and S. Feng, A pillared-layered copper(I) halide-based metal–organic framework exhibiting dual emission, and piezochromic and thermochromic properties with a large temperature-dependent emission red-shift, RSC Adv., 2018, 8, 1973 RSC.
- Q. Ma, M. Zhang, X. Xu, K. Meng, C. Yao, Y. Zhao, J. Sun, Y. Du and D. Yang, Multiresponsive Supramolecular Luminescent Hydrogels Based on a Nucleoside/Lanthanide Complex, ACS Appl. Mater. Interfaces, 2019, 11, 47404 CrossRef CAS PubMed.
- P. Chen, Q. Li, S. Grindy and N. Holten-Andersen, White-Light-Emitting Lanthanide Metallogels with Tunable Luminescence and Reversible Stimuli-Responsive Properties, J. Am. Chem. Soc., 2015, 137, 11590 CrossRef CAS PubMed.
- G. A. Filonenko, D. Sun, M. Weber, C. Müller and E. A. Pidko, Multicolor Organometallic Mechanophores for Polymer Imaging Driven by Exciplex Level Interactions, J. Am. Chem. Soc., 2019, 141, 9687 CrossRef CAS PubMed.
- J. Pitchaimani, S. Karthikeyan, N. Lakshminarasimhan, S. P. Anthony, D. Moon and V. Madhu, Reversible Thermochromism of Nickel(II) Complexes and Single-Crystal-to-Single-Crystal Transformation, ACS Omega, 2019, 4, 13756 CrossRef CAS.
- A. Y.-Y. Tam, K. M.-C. Wong and V. W.-W. Yam, Unusual Luminescence Enhancement of Metallogels of Alkynylplatinum(II) 2,6-Bis(N-alkylbenzimidazol-2′-yl)pyridine Complexes upon a Gel-to-Sol Phase Transition at Elevated Temperatures, J. Am. Chem. Soc., 2009, 131, 6253 CrossRef CAS PubMed.
- M.-S. Jiang, Y.-H. Tao, Y.-W. Wang, C. Lu, D. J. Young, J.-P. Lang and Z.-G. Ren, Reversible Solid-State Phase Transitions between Au–P Complexes Accompanied by Switchable Fluorescence, Inorg. Chem., 2020, 59, 3072 CrossRef CAS PubMed.
- Y. Pan, H.-Q. Su, E.-L. Zhou, H.-Z. Yin, K.-Z. Shao and Z.-M. Su, A stable mixed lanthanide metal–organic framework for highly sensitive thermometry, Dalton Trans., 2019, 48, 3723 RSC.
- Y. Cui, H. Xu, Y. Yue, Z. Guo, J. Yu, Z. Chen, J. Gao, Y. Yang, G. Qian and B. Chen, A Luminescent Mixed-Lanthanide Metal–Organic Framework Thermometer, J. Am. Chem. Soc., 2012, 134, 3979 CrossRef CAS PubMed.
- K. Yang, S.-L. Li, F.-Q. Zhang and X.-M. Zhang, Simultaneous Luminescent Thermochromism, Vapochromism, Solvatochromism, and Mechanochromism in a C3-Symmetric Cubane [Cu4I4P4] Cluster without Cu–Cu Interaction, Inorg. Chem., 2016, 55, 7323 CrossRef CAS PubMed.
- Q. Benito, A. Fargues, A. Garcia, S. Maron, T. Gacoin, J.-P. Boilot, S. Perruchas and F. Camerel, Photoactive Hybrid Gelators Based on a Luminescent Inorganic [Cu4I4] Cluster Core, Chem. – Eur. J., 2013, 19, 15831 CrossRef CAS PubMed.
- G.-G. Luo, H.-F. Su, A. Xiao, Z. Wang, Y. Zhao, Q.-Y. Wu, J.-H. Wu, D. Sun and L.-S. Zheng, Silver–Sulfur Hybrid Supertetrahedral Clusters: The Hitherto Missing Members in the Metal–Chalcogenide Tetrahedral Clusters, Chem. – Eur. J., 2017, 23, 14420 CrossRef CAS PubMed.
- A. V. Artem'ev, M. R. Ryzhikov, A. S. Berezin, I. E. Kolesnikov, D. G. Samsonenko and I. Y. Bagryanska, Photoluminescence of Ag(I) complexes with a square-planar coordination geometry: the first observation, Inorg. Chem. Front., 2019, 6, 2855 RSC.
- K. Okabe, N. Inada, C. Gota, Y. Harada, T. Funatsu and S. Uchiyama, Intracellular temperature mapping with a fluorescent polymeric thermometer and fluorescence lifetime imaging microscopy, Nat. Commun., 2012, 3, 705 CrossRef PubMed.
- H. Zhang, J. Jiang, P. Gao, T. Yang, K. Y. Zhang, Z. Chen, S. Liu, W. Huang and Q. Zhao, Dual-Emissive Phosphorescent Polymer Probe for Accurate Temperature Sensing in Living Cells and Zebrafish Using Ratiometric and Phosphorescence Lifetime Imaging Microscopy, ACS Appl. Mater. Interfaces, 2018, 10, 17542 CrossRef CAS PubMed.
- J. S. Donner, S. A. Thompson, M. P. Kreuzer, G. Baffou and R. Quidant, Mapping Intracellular Temperature Using Green Fluorescent Protein, Nano Lett., 2012, 12, 2107 CrossRef CAS PubMed.
- C. Fan and C. Yang, Yellow/orange emissive heavy-metal complexes as phosphors in monochromatic and white organic light-emitting devices, Chem. Soc. Rev., 2014, 43, 6439 RSC.
- C.-W. Lu, Y. Wang and Y. Chi, Metal Complexes with Azolate-Functionalized Multidentate Ligands: Tactical Designs and Optoelectronic Applications, Chem. – Eur. J., 2016, 22, 17892 CrossRef CAS PubMed.
- S. Guo, M. Han, R. Chen, Y. Zhuang, L. Zou, S. Liu, W. Huang and Q. Zhao, Mitochondria-localized iridium(III) complexes with anthraquinone groups as effective photosensitizers for photodynamic therapy under hypoxia, Sci. China: Chem., 2019, 62, 1639 CrossRef CAS.
- F. Wei, S.-L. Lai, S. Zhao, M. Ng, M.-Y. Chan, V. W.-W. Yam and K. M.-C. Wong, Ligand Mediated Luminescence Enhancement in Cyclometalated Rhodium(III) Complexes and Their Applications in Efficient Organic Light-Emitting Devices, J. Am. Chem. Soc., 2019, 141, 12863 CrossRef CAS PubMed.
- N. Bustamante, G. Ielasi, M. Bedoya and G. Orellana, Optimization of Temperature Sensing with Polymer-Embedded Luminescent Ru(II) Complexes, Polymers, 2018, 10, 234 CrossRef PubMed.
- M. Mirenda, V. Levi, M. L. Bossi, L. Bruno, A. V. Bordoni, A. E. Regazzoni and A. Wolosiuk, Temperature response of luminescent tris(bipyridine)ruthenium(II)-doped silica nanoparticles, J. Colloid Interface Sci., 2013, 392, 96 CrossRef CAS PubMed.
- F. Sguerra, R. Marion, G. H. V. Bertrand, R. Coulon, É. Sauvageot and R. Daniellou, Thermo- and radioluminescent polystyrene based plastic scintillators doped with phosphorescent iridium(III) complexes, J. Mater. Chem. C, 2014, 2, 6125 RSC.
- L. H. Fischer, M. I. J. Stich, O. S. Wolfbeis, N. Tian, E. Holder and M. Schäferling, Red- and Green-Emitting Iridium(III) Complexes for a Dual Barometric and Temperature-Sensitive Paint, Chem. – Eur. J., 2009, 15, 10857 CrossRef CAS PubMed.
- K. Y. Zhang, X. Chen, G. Sun, T. Zhang, S. Liu, Q. Zhao and W. Huang, Utilization of Electrochromically Luminescent Transition-Metal Complexes for Erasable Information Recording and Temperature-Related Information Protection, Adv. Mater., 2016, 28, 7137 CrossRef CAS PubMed.
- J. E. Yarnell, C. E. McCusker, A. J. Leeds, J. M. Breaux and F. N. Castellano, Exposing the Excited-State Equilibrium in an IrIII Bichromophore: A Combined Time Resolved Spectroscopy and Computational Study, Eur. J. Inorg. Chem., 2016, 1808 CrossRef CAS.
- L. Liu, X. Wang, N. Wang, T. Peng and S. Wang, Bright, Multi-responsive, Sky-Blue Platinum(II) Phosphors Based on a Tetradentate Chelating Framework, Angew. Chem., Int. Ed., 2017, 56, 9160 CrossRef CAS PubMed.
- S. C. Cuerva, J. A. Campo, M. Cano, M. Caño-García, J. M. Otón and C. Lodeiro, Aggregation-induced emission enhancement (AIEE)-active Pt(II) metallomesogens as dyes sensitive to Hg2+ and dopant agents to develop stimuli-responsive luminescent polymer materials, Dyes Pigm., 2020, 175, 108098 CrossRef.
- M. H.-Y. Chan, S. Y.-L. Leung and V. W.-W. Yam, Rational Design of Multi-Stimuli-Responsive Scaffolds: Synthesis of Luminescent Oligo(ethynylpyridine)-Containing Alkynylplatinum(II) Polypyridine Foldamers Stabilized by Pt⋯Pt Interactions, J. Am. Chem. Soc., 2019, 141, 12312 CrossRef CAS PubMed.
- Z. Chen, J.-H. Tang, W. Chen, Y. Xu, H. Wang, Z. Zhang, H. Sepehrpour, G.-J. Cheng, X. Li, P. Wang, Y. Sun and P. J. Stang, Temperature- and Mechanical-Force-Responsive Self-Assembled Rhomboidal Metallacycle, Organometallics, 2019, 38, 4244 CrossRef CAS.
- K.-C. Chang, J.-L. Lin, Y.-T. Shen, C.-Y. Hung, C.-Y. Chen and S.-S. Sun, Synthesis and Photophysical Properties of Self-Assembled Metallogels of Platinum(II) Acetylide Complexes with Elaborate Long-Chain Pyridine-2,6-Dicarboxamides, Chem. – Eur. J., 2012, 18, 1312 CAS.
- F. Zhong, J. Zhao, M. Hayvali, A. Elmali and A. Karatay, Effect of Molecular Conformation Restriction on the Photophysical Properties of N^N Platinum(II) Bis(ethynylnaphthalimide) Complexes Showing Close-Lying 3MLCT and 3LE Excited States, Inorg. Chem., 2019, 58, 1850 CAS.
- J.-H. Tang, Y. Sun, Z.-L. Gong, Z.-Y. Li, Z. Zhou, H. Wang, X. Li, M. L. Saha, Y.-W. Zhong and P. J. Stang, Temperature-Responsive Fluorescent Organoplatinum(II) Metallacycles, J. Am. Chem. Soc., 2018, 140, 7723 CrossRef CAS PubMed.
- S. Lin, H. Pan, L. Li, R. Liao, S. Yu, Q. Zhao, H. Sun and W. Huang, AIPE-active platinum(II) complexes with tunable photophysical properties and their application in constructing thermosensitive probes used for intracellular temperature imaging, J. Mater. Chem. C, 2019, 7, 7893 RSC.
- C. M. Brown, V. Carta and M. O. Wolf, Thermochromic Solid-State Emission of Dipyridyl Sulfoxide Cu(I) Complexes, Chem. Mater., 2018, 30, 5786 CrossRef CAS.
- I. D. Strelnik, V. V. Sizov, V. V. Gurzhiy, A. S. Melnikov, I. E. Kolesnikov, E. I. Musina, A. A. Karasik and E. V. Grachova, Binuclear Gold(I) Phosphine Alkynyl Complexes Templated on a Flexible Cyclic Phosphine Ligand: Synthesis and Some Features of Solid-State Luminescence, Inorg. Chem., 2020, 59, 244 CrossRef CAS.
- J. Cored, O. Crespo, J. L. Serrano, A. Elduque and Ra. Giménez, Decisive Influence of the Metal in Multifunctional Gold, Silver, and Copper Metallacycles: High Quantum Yield Phosphorescence, Color Switching, and Liquid Crystalline Behavior, Inorg. Chem., 2018, 57, 12632 CrossRef CAS PubMed.
- Y. Ma, Y. Dong, S. Liu, P. She, J. Lu, S. Liu, W. Huang and Q. Zhao, Chameleon-Like Thermochromic Luminescent Materials with Controllable Response Behaviors for Multilevel Security Printing, Adv. Opt. Mater., 2020, 8, 1901687 CrossRef CAS.
- J. Miao, Y. Nie, Y. Li, C. Qin, Y. Ren, C. Xu, M. Yan, K. Liu and G. Liu, Single-component solid state white-light emission and photoluminescence color tuning of a Cd(II) complex and its application as a luminescence thermometer, J. Mater. Chem. C, 2019, 7, 13454 RSC.
- S. Otto, N. Scholz, T. Behnke, U. Resch-Genger and K. Heinze, Thermo-Chromium: A Contactless Optical Molecular Thermometer, Chem. – Eur. J., 2017, 23, 12131 CrossRef CAS PubMed.
-
(a) A. S. Berezin, D. G. Samsonenko, V. K. Brel and A. V. Artem'ev, “Two-in-one” organic–inorganic hybrid MnII complexes exhibiting dual-emissive phosphorescence, Dalton Trans., 2018, 47, 7306 RSC;
(b) Y. Wu, X. Zhang, Y.-Q. Zhang, M. Yang and Z.-N. Chen, Achievement of ligand-field induced thermochromic luminescence via two-step single-crystal to single-crystal transformations, Chem. Commun., 2018, 54, 13961 RSC.
- S. Katagiri, Y. Hasegawa, Y. Wada and S. Yanagida, Thermo-sensitive Luminescence Based on the Back Energy Transfer in Terbium(III) Complexes, Chem. Lett., 2004, 33, 1438 CrossRef CAS.
- D. V. Lapaev, V. G. Nikiforov, V. S. Lobkov, A. A. Knyazev and Y. G. Galyametdinov, A photostable vitrified film based on a terbium(III) β-diketonate complex as a sensing element for reusable luminescent thermometers, J. Mater. Chem. C, 2018, 6, 9475 RSC.
- M. Tang, Y. Huang, Y. Wang and L. Fu, An ytterbium complex with unique luminescence properties: detecting the temperature based on a luminescence spectrum without the interference of oxygen, Dalton Trans., 2015, 44, 7449 CAS.
- Y. Kitagawa, M. Kumagai, T. Nakanishi, K. Fushimi and Y. Hasegawa, The Role of π–f Orbital Interactions in Eu(III) Complexes for an Effective Molecular Luminescent Thermometer, Inorg. Chem., 2020, 59, 5865 CrossRef CAS PubMed.
- D. V. Lapaev, V. G. Nikiforov, V. S. Lobkov, A. A. Knyazev, R. M. Ziyatdinova and Y. G. Galyametdinov, A vitrified film of an anisometric europium(III) β-diketonate complex with a low melting point as a reusable luminescent temperature probe with excellent sensitivity in the range of 270–370 K, J. Mater. Chem. C, 2020, 8, 6273 RSC.
- J. R. Shakirova, N. N. Shevchenko, V. A. Baigildin, P. S. Chelushkin, A. F. Khlebnikov, O. A. Tomashenko, A. I. Solomatina, G. L. Starova and S. P. Tunik, Eu-Based Phosphorescence Lifetime Polymer Nanothermometer: A Nanoemulsion Polymerization Approach to Eliminate Quenching of Eu Emission in Aqueous Media, ACS Appl. Polym. Mater., 2020, 2(2), 537 CrossRef.
- S. Swavey, J. A. Krause, D. Collins, D. D'Cunha and A. Fratini, X-ray structure and temperature dependent luminescent properties of two bimetallic europium complexes, Polyhedron, 2008, 27, 1061 CrossRef CAS.
- K. Yanagisawa, Y. Kitagawa, T. Nakanishi, T. Seki, K. Fushimi, H. Ito and Y. Hasegawa, A Luminescent Dinuclear EuIII/TbIII Complex with LMCT Band as a Single-Molecular Thermosensor, Chem. – Eur. J., 2018, 24, 1956 CAS.
- R. Peng, M. Li and D. Li, Copper(I) halides: A versatile family in coordination chemistry and crystal engineering, Coord. Chem. Rev., 2010, 254, 1 CrossRef CAS.
- M. Wallesch, D. Volz, D. M. Zink, U. Schepers, M. Nieger, T. Baumann and S. Brase, Bright Coppertunities: Multinuclear CuI Complexes with N–P Ligands and Their Applications, Chem. – Eur. J., 2014, 20, 6578 CrossRef CAS PubMed.
- B. Huitorel, Q. Benito, A. Fargues, A. Garcia, T. Gacoin, J.-P. Boilot, S. Perruchas and F. Camerel, Mechanochromic Luminescence and Liquid Crystallinity of Molecular Copper Clusters, Chem. Mater., 2016, 28, 8190 CrossRef CAS.
- A. V. Shamsieva, I. E. Kolesnikov, I. D. Strelnik, T. P. Gerasimova, A. A. Kalinichev, S. A. Katsyuba, E. I. Musina, E. Lähderanta, A. A. Karasik and O. G. Sinyashin, Fresh Look on the Nature of Dual-Band Emission of Octahedral Copper-Iodide Clusters—Promising Ratiometric Luminescent Thermometers, J. Phys. Chem. C, 2019, 123, 25863 CrossRef CAS.
- S.-Z. Zhan, X. Jiang, J. Zheng, X.-D. Huang, G.-H. Chen and D. Li, A luminescent supramolecular Cu2I2(NH3)2-sandwiched Cu3(pyrazolate)3 adduct as a temperature sensor, Dalton Trans., 2018, 47, 3679 RSC.
- C.-Y. Yue, F.-L. Liu, W.-T. Deng, J. Tao and M.-C. Hong, Iodide-Centered Cuprous Octatomic Ring: A Luminescent Molecular Thermometer Exhibiting Dual-Emission Character, Cryst. Growth Des., 2018, 18, 22 CrossRef CAS.
- L. Shang, F. Stockmar, N. Azadfar and G. U. Nienhaus, Intracellular Thermometry by Using Fluorescent Gold Nanoclusters, Angew. Chem., Int. Ed., 2013, 52, 11154 CrossRef CAS PubMed.
- Y.-T. Wu, C. Shanmugam, W.-B. Tseng, M.-M. Hiseh and W.-L. Tseng, A gold nanocluster-based fluorescent probe for simultaneous pH and temperature sensing and its application to cellular imaging and logic gates, Nanoscale, 2016, 8, 11210 RSC.
- S. Kundu, D. Mukherjee, T. K. Maiti and N. Sarkar, Highly Luminescent Thermoresponsive Green Emitting Gold Nanoclusters for Intracellular Nanothermometry and Cellular Imaging: A Dual Function Optical Probe, ACS Appl. Bio Mater., 2019, 2, 2078 CrossRef CAS.
- D.-F. Lu, Z.-F. Hong, J. Xie, X.-J. Kong, L.-S. Long and L.-S. Zheng, High-Nuclearity Lanthanide–Titanium Oxo Clusters as Luminescent Molecular Thermometers
with High Quantum Yields, Inorg. Chem., 2017, 56, 12186 CrossRef CAS PubMed.
- S. M. Bruno, D. Ananias, F. A. A. Paz, M. Pillinger, A. A. Valente, L. D. Carlos and I. S. Gonçalves, Crystal structure and temperature-dependent luminescence of a heterotetranuclear sodium–europium(III) β-diketonate complex, Dalton Trans., 2015, 44, 488 RSC.
- W. Huang, M. Zhang, S. Huang and D. Wu, Tunable Luminescent Heterometallic Zn2Ln2 Edge-Defective Molecular Cubane with Stimuli-Responsive Properties, Inorg. Chem., 2017, 56, 6768 CrossRef CAS PubMed.
- H. Yao, G. Calvez, C. Daiguebonne, K. Bernot, Y. Suffren and O. Guillou, Hetero-hexalanthanide Complexes: A New Synthetic Strategy for Molecular Thermometric Probes, Inorg. Chem., 2019, 58, 16180 CrossRef CAS PubMed.
- J. Conesa-Egea, N. Nogal, J. I. Martínez, V. Fernández-Moreira, U. R. Rodríguez-Mendoza, J. González-Platas, C. J. Gómez-García, S. Delgado, F. Zamora and P. Amo-Ochoa, Smart composite films of nanometric thickness based on copper–iodine coordination polymers. Toward sensors, Chem. Sci., 2018, 9, 8000 RSC.
- S.-S. Zhao, L. Wang, Y. Liu, L. Chen and Z. Xie, Stereochemically Dependent Synthesis of Two Cu(I) Cluster-Based Coordination Polymers with Thermochromic Luminescence, Inorg. Chem., 2017, 56, 13975 CrossRef CAS PubMed.
- O. Veselska, D. Podbevšek, G. Ledoux, A. Fateeva and A. Demessence, Intrinsic triple-emitting 2D copper thiolate coordination polymer as a ratiometric thermometer working over 400 K range, Chem. Commun., 2017, 53, 12225 RSC.
- Z.-H. Yan, X.-Y. Li, L.-W. Liu, S.-Q. Yu, X.-P. Wang and D. Sun, Single-Crystal to Single-Crystal Phase Transition and Segmented Thermochromic Luminescence in a Dynamic 3D Interpenetrated AgI Coordination Network, Inorg. Chem., 2016, 55, 1096 CrossRef CAS PubMed.
- X.-Y. Dong, H.-L. Huang, J.-Y. Wang, H.-Y. Li and S.-Q. Zang, A Flexible Fluorescent SCC-MOF for Switchable Molecule Identification and Temperature Display, Chem. Mater., 2018, 30, 2160 CrossRef CAS.
- Y. Wang, B. Wang, H. Shi, C. Zhang, C. Tao and J. Li, Carbon nanodots in ZIF-8: synthesis, tunable luminescence and temperature sensing, Inorg. Chem. Front., 2018, 5, 2739 RSC.
- L. Tom and M. R. P. Kurup, A reversible thermo-responsive 2D Zn(II) coordination polymer as a potential self-referenced luminescent thermometer, J. Mater. Chem. C, 2020, 8, 2525 RSC.
- H. Zhang, C. Lin, T. Sheng, S. Hu, C. Zhuo, R. Fu, Y. Wen, H. Li, S. Su and X. Wu, A Luminescent Metal–Organic Framework Thermometer with Intrinsic Dual Emission from Organic Lumophores, Chem. – Eur. J., 2016, 22, 4460 CrossRef CAS PubMed.
- L. Chen, J.-W. Ye, H.-P. Wang, M. Pan, S.-Y. Yin, Z.-W. Wei, L.-Y. Zhang, K. Wu, Y.-N. Fan and C.-Y. Su, Ultrafast water sensing and thermal imaging by a metal-organic framework with switchable luminescence, Nat. Commun., 2017, 8, 15985 CrossRef CAS PubMed.
- D. Li, X. Yang and D. Yan, Cluster-Based Metal–Organic Frameworks: Modulated Singlet–Triplet Excited States and Temperature-Responsive Phosphorescent Switch, ACS Appl. Mater. Interfaces, 2018, 10, 34377 CrossRef CAS PubMed.
- H. Cai, W. Lu, C. Yang, M. Zhang, M. Li, C.-M. Che and D. Li, Tandem Förster Resonance Energy Transfer Induced Luminescent Ratiometric Thermometry in Dye-Encapsulated Biological Metal–Organic Frameworks, Adv. Opt. Mater., 2019, 7, 1801149 CrossRef.
- H. Zhang, L. Zhou, J. Wei, Z. Li, P. Lin and S. Du, Highly luminescent and thermostable lanthanide-carboxylate framework materials with helical configurations, J. Mater. Chem., 2012, 22, 21210 RSC.
- A. Balamurugan, M. L. P. Reddy and M. Jayakannan, π-Conjugated polymer–Eu3+ complexes: versatile luminescent molecular probes for temperature sensing, J. Mater. Chem. A, 2013, 1, 2256 RSC.
- T. Xia, Y. Cui, Y. Yang and G. Qian, A luminescent ratiometric thermometer based on thermally coupled levels of a Dy-MOF, J. Mater. Chem. C, 2017, 5, 5044 RSC.
- L. Li, Y. Zhu, X. Zhou, C. D. S. Brites, D. Ananias, Z. Lin, F. A. A. Paz, J. Rocha, W. Huang and L. D. Carlos, Visible-Light Excited Luminescent Thermometer Based on Single Lanthanide Organic Frameworks, Adv. Funct. Mater., 2016, 26, 8677 CrossRef CAS.
- D. Ananias, A. D. G. Firmino, R. F. Mendes, F. A. A. Paz, M. Nolasco, L. D. Carlos and J. Rocha, Excimer Formation in a Terbium Metal–Organic Framework Assists Luminescence Thermometry, Chem. Mater., 2017, 29, 9547 CrossRef CAS.
- K. Miyata, Y. Konno, T. Nakanishi, A. Kobayashi, M. Kato, K. Fushimi and Y. Hasegawa, Chameleon Luminophore for Sensing Temperatures: Control of Metal-to-Metal and Energy Back Transfer in Lanthanide Coordination Polymers, Angew. Chem., Int. Ed., 2013, 52, 6413 CrossRef CAS PubMed.
- F. V. Bussche, A. M. Kaczmarek, J. Schmidt, C. V. Stevens and P. Van Der Voort, Lanthanide grafted phenanthroline-polymer for physiological temperature range sensing, J. Mater. Chem. C, 2019, 7, 10972 RSC.
- J. K. Zaręba, M. Nyk, J. Janczak and M. Samoć, Three-Photon Absorption of Coordination Polymer Transforms UV-to-VIS Thermometry into NIR-to-VIS Thermometry, ACS Appl. Mater. Interfaces, 2019, 11, 10435 CrossRef PubMed.
- D. Zhao, D. Yue, L. Zhang, K. Jiang and G. Qian, Cryogenic Luminescent Tb/Eu-MOF Thermometer Based on a Fluorine-Modified Tetracarboxylate Ligand, Inorg. Chem., 2018, 57, 12596 CrossRef CAS PubMed.
- X.-D. Wang, R. J. Meier, M. Schäferling, S. Bange, J. M. Lupton, M. Sperber, J. Wegener, V. Ondrus, U. Beifuss, U. Henne, C. Klein and O. S. Wolfbeis, Two-Photon Excitation Temperature Nanosensors Based on a Conjugated Fluorescent Polymer Doped with a Europium Probe, Adv. Opt. Mater., 2016, 4, 1854 CrossRef CAS.
- S. Li, X.-F. Jiang and Q.-H. Xu, Polyfluorene based conjugated polymer nanoparticles for two-photon live cell imaging, Sci. China: Chem., 2018, 61, 88 CrossRef CAS.
- M.-J. Sun, Y.-W. Zhong and J. Yao, Thermal-Responsive Phosphorescent Nanoamplifiers Assembled from Two Metallophosphors, Angew. Chem., Int. Ed., 2018, 57, 7820 CrossRef CAS PubMed.
- Z. Li, Z. Hou, D. Ha and H. Li, A Ratiometric Luminescent Thermometer Co-doped with Lanthanide and Transition Metals, Chem. – Asian J., 2015, 10, 2720 CrossRef CAS PubMed.
- Z. Chen, K. Y. Zhang, X. Tong, Y. Liu, C. Hu, S. Liu, Q. Yu, Q. Zhao and W. Huang, Phosphorescent Polymeric Thermometers for In Vitro and In Vivo Temperature Sensing with Minimized Background Interference, Adv. Funct. Mater., 2016, 26, 4386 CrossRef CAS.
-
(a) H. Yersin, R. Czerwieniec, M. Z. Shafikov and A. F. Suleymanova, TADF Material Design: Photophysical Background and Case Studies Focusing on CuI and AgI complexes, ChemPhysChem, 2017, 18, 3508 CrossRef CAS;
(b) G. Li, Z.-Q. Zhu, Q. Chen and J. Li, Metal complex based on delayed fluorescence materials, Org. Electron., 2019, 69, 135 CrossRef CAS.
Footnote |
† These authors contributed equally to this work. |
|
This journal is © the Partner Organisations 2020 |
Click here to see how this site uses Cookies. View our privacy policy here.