Single-molecule nanoscale drug carriers with quantitative supramolecular loading†
Received
29th July 2019
, Accepted 21st August 2019
First published on 28th August 2019
Abstract
While there are examples of successful nanomedicine technologies, challenges remain in engineering systems with controlled and defined characteristics such as size and drug loading. Dendrimers are a class of synthetic macromolecules which offer routes to precise nanoscale objects. Here, highly efficient and orthogonal methods for dendrimer synthesis are combined with the covalent attachment of a tunable number of cucurbit[7]uril macrocycle carriers. This synthetic macrocycle affords uniquely high-affinity binding to certain guests, lending modularity to the prepared dendrimer in terms of its drug cargo. To verify this concept, the chemotherapeutic doxorubicin was conjugated to a high-affinity guest for CB[7]. When combined with CB[7]-modified dendrimers, quantitative drug loading was realized, with one drug bound per CB[7] on the dendrimer. The guest-modified drug was attached via a pH-labile linker to facilitate release at sites of disease or upon cell internalization. This approach offers a new combination of discrete and efficient synthetic methodology coupled with modular and high-affinity supramolecular motifs to engineer platforms with exceptional precision for use in drug delivery.
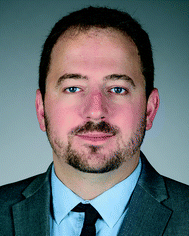 Matthew J. Webber | Matthew Webber is an Assistant Professor at the University of Notre Dame, with a primary appointment in the Department of Chemical & Biomolecular Engineering. He received his PhD in Biomedical Engineering from Northwestern University under the direction of Prof. Samuel Stupp and was an NIH postdoctoral fellow at MIT under the direction of Profs. Robert Langer and Daniel Anderson. His lab is interested in engineering new materials and therapeutics using principles from supramolecular chemistry and peptide self-assembly. |
Design, System, Application
The efficient radial growth of dendrimers using high-yielding and orthogonal reactions results in spherical nanoscale objects with a fixed number of addressable chemical handles on their periphery. Leveraging synthetic precedent for monofunctional cucurbit[7]uril macrocycles, these are then attached to the periphery of defined dendrimers to enable high-affinity supramolecular recognition of guests for drug loading on the dendrimer. By combining the precision of synthetic dendrimers with the predictability of CB[7] supramolecular macrocycles, this approach seeks a platform of highly defined carriers which can be easily modified for an assortment of uses in different drug delivery applications.
|
Introduction
The field of nanomedicine has emerged in part due to the many benefits of nanoscale drug carriers, including improved drug solubility or stability, reduced clearance rate, and increased percent of drug reaching a desired site with limited off-site exposure.1–3 Yet, challenges remain in the design of traditional nanoparticle drug carriers. Typical methods to generate nanoparticles use emulsification or precipitation,4 processes that produce inherent dispersity in size of the carrier. This is juxtaposed with findings that biodistribution, tissue penetration, and function are highly correlated with nanoparticle size,5–9 and a reality that variation in size may complicate regulatory approval.10 Nanoparticle drug loading is typically achieved by preferential partition of hydrophobic drugs into liposomes, micelles, or hydrophobic polymers. This leads to inherent variation in the amount of drug within each particle, yet addressing this variation is not often considered as a design parameter in nanoparticle use.11 Furthermore, the payload is often limited to hydrophobic drugs, and the encapsulation of hydrophilic drugs for delivery remains a challenge. Drug release is typically governed by diffusion and/or particle erosion,12 and it can be difficult to control or tune the rate of drug availability. As such, poorly defined drug loading and inherent leakage arising from partition-driven encapsulation present several additional design challenges in creating effective nanomedicine. The design of modular nanoparticle platforms offering improved geometric precision and enhanced control of drug loading and release may therefore help to accelerate the clinical realization of nanoscale drug delivery.
Dendrimers are highly branched macromolecules; robust synthetic precedent makes it feasible for these to form uniformly-sized and molecular weight-defined nanoscale materials.13–16 A typical dendrimer is synthesized divergently, growing radially from a core through alternating and step-wise addition of monomeric building blocks to realize a globular macromolecule.17 Convergent methodologies have also been demonstrated.18 A dendrimer is defined by its generation, signifying the cycles of monomer addition and radial growth.19 Most synthetic strategies affording dendritic architectures rely on each of these additions occurring to near-completion, and methodology has advanced for a number of dendrimer chemistries that are relatively defect-free after several generations.20 Dendrimers have been widely explored for their use as drug carriers,19,21 such as through covalent attachment of drugs via labile linkages,22 preferential drug partitioning within hydrophobic domains, or electrostatic association.23 There are inherent benefits to using dendrimers for therapeutic applications, including their well-defined size distribution, batch-to-batch reproducibility, and a suite of chemical tools which enable varied sizes, tunable branch densities, and controlled surface-presentation of charged or reactive groups.15 The branched structure of dendrimers typically affords longer circulation half-life compared to linear polymers of the same molecular weight or hydrodynamic size due to a reduced ability of dendrimers to reptate through glomerular fenestrations.19 The precise control over size furthermore provides control over pharmacokinetics and biodistribution.24
There are many benefits that arise from integrating supramolecular motifs into biomaterials and drug delivery vehicles.25,26 The present work explores the integration of supramolecular macrocycles as modular drug carriers on the periphery of efficiently synthesized dendrimers, coupling the molecularly defined and nanoscale features of dendrimers with enhanced modularity and greater assurance in drug loading facilitated by host–guest interactions (Fig. 1). The macrocycle we have chosen for this work is cucurbit[7]uril (CB[7]), a synthetic cavitand prepared from 7 repeats of the glycoluril monomer,27 and which binds with high affinity to an array of guest molecules.28 Though not as commonly used in pharmaceutical practice as cyclodextrins, CB[7] offers binding (Keq) that may be as much as 8–10 orders of magnitude higher for the same guest than possible with cyclodextrin, which does not typically bind in excess of 105 M−1 to most guests.29,30 CB[7] participates in some of the highest affinity motifs of any host–ligand interaction, with equilibrium binding constants as high as 1015 M−1 for certain guests in buffer.31 If CB[7]–guest interactions are approximated as being roughly diffusion-governed (kon ∼ 108 M−1 s−1),32 a model interaction of 1012 M−1 is thus quite stable and long-lived (koff ∼ 10−4 s−1). While dendrimers and related nanoscale materials have been previously modified with cyclodextrin macrocycles for drug delivery,33 the limited affinity and rapid exchange dynamics offered by this macrocycle in binding to its guest would be thought to contribute to drug leakage upon competition with native compounds in the body (e.g., cholesterol) and dilution in serum or biological fluids. By instead appending high-affinity guests for CB[7], which are far in excess of anything encountered in the body, to a drug or payload of interest its stable interaction with CB[7] is assured and the approach becomes more generalized and modular. We recently reported on a new use for high-affinity CB[7]–guest interactions to drive guest-modified prodrugs to desired sites in the body.34 CB[7] has molecular weight of 1163 Da and diameter of 1.6 nm, meaning it may be attached to materials or devices with minimal footprint. It also has excellent water solubility to remain accessible for loading in biological contexts, and has shown limited toxicity in pre-clinical models (LD50 > 250 mg kg−1 in mice).35 Though limited in clinical use, early work has suggested promise for cucurbit[n]urils as excipients in different pharmaceutical dosage forms.35,36
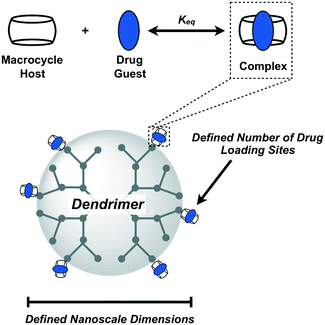 |
| Fig. 1 Vision for integrating supramolecular macrocycles, which participate in dynamic equilibrium-governed binding to guests characterized by tunable affinity (Keq), onto dendritic macromolecules for nanoscale drug carriers with more well-defined size and drug loading. | |
Results and discussion
Dendrimer carrier synthesis and characterization
Dendrimer chemistry has evolved in recent years with the demonstration of divergent synthetic routes using highly efficient and orthogonal “click” or comparable reactions to facilitate dendritic structures which are relatively defect-free even after several generations of growth.37,38 We were thus inspired to implement a synthetic approach which consisted of alternating epoxy–amine and thiol–yne reactions,39–42 a scheme that resulted in odd-numbered generations being terminated with an alkyne and even-numbered generations being terminated with an amine (Fig. 2a). Hexamethylenediamine was chosen as the core for this divergent synthesis because its characteristic 1H-NMR signal (δ = 1.2–1.7 ppm) does not overlap with signals from the dendrons (δ = 2.5–4.5 ppm) and therefore would enable tracking of dendrimer growth with each generation. From this diamine core, we first reacted with glycidyl propargyl ether, resulting in a G1(-yne)4 dendrimer; each primary amine can react with two epoxides. Terminal alkynes were then reacted by thiol–yne addition with cysteamine hydrochloride using 365 nm light along with a DMPA photo-initiator, resulting in the G2(-NH2)8 dendrimer. This process using highly efficient and orthogonal reactions proceeded iteratively to access a fifth generation G5(-yne)64 dendrimer with 64 terminal alkynes. Using a combination of gel permeation chromatography (GPC) and 1H-NMR, successive growth of the dendrimer was verified at each step according to expectations. By GPC, the dendrimers showed a narrow molecular weight distribution (PDI: G1-1.08; G3-1.09; G5-1.20) and increased in size as predicted with each generation (Fig. 2b). Using 1H-NMR normalized to protons in the core, protons assigned to components of the dendron also increased with generation (Fig. 2c and d). Full details for dendrimer synthesis are available in the ESI.†
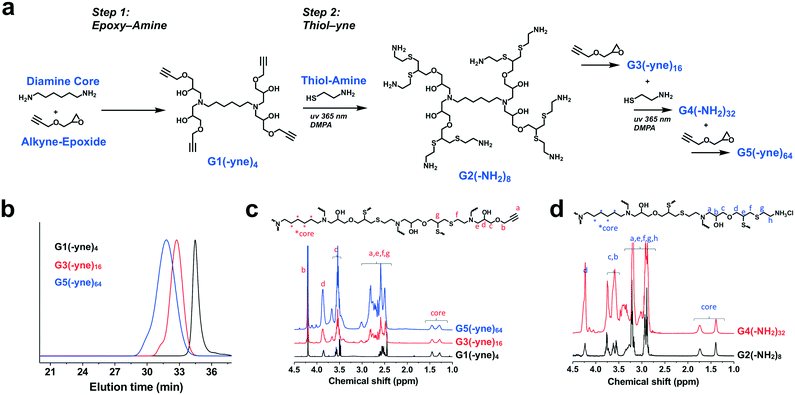 |
| Fig. 2 Dendrimer synthesis. (a) Orthogonal epoxy–amine and thiol–yne reactions were performed to access an eventual fifth generation product (G5(-yne)64) containing 64 terminal alkynes. (b) GPC confirmed growth of the dendrimer core with each iteration. (c) 1H-NMR of the first, third, and fifth generation alkyne-terminated dendrimers, normalized to the protons in the core in CDCl3. (d) 1H-NMR of the second and fourth generation dendrimers, normalized to the protons in the core, in D2O. | |
In spite of the many benefits to using CB[7] as a carrier of small molecules, this macrocycle has proven difficult to modify with functional handles for inclusion on materials. At least three routes to functionalized CB[7] have been reported.43–46 While the most commonly used routes achieve functionalized CB[7] by oxidation to different hydroxy-modified species, the method by Isaacs exclusively obtains a monofunctional CB[7] product through a retrosynthetic route from an acyclic hexamer cyclized using a bis-cyclic ether monomer.43 This method results in an alkyl-halide modified CB[7] which can be converted to an azide (CB[7]-N3). Using copper-catalyzed “click” chemistry methods,47 CB[7]-N3 was reacted with the G5(-yne)64 dendrimer at a ratio calculated to ensure an average of 8 CB[7] macrocycles per dendrimer (Fig. 3a). After allowing this reaction to proceed to completion, azide-modified polyethylene glycol (mPEG-N3, 2000 Da) was added to the reaction mixture to “click” to remaining alkyne sites.
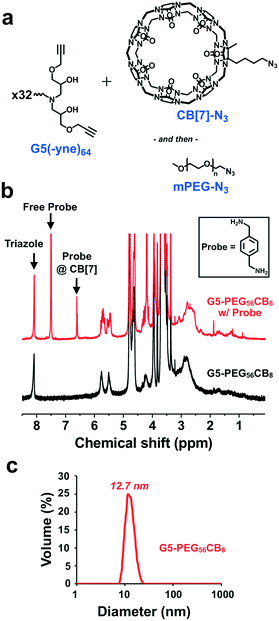 |
| Fig. 3 Attachment of CB[7] to G5(-yne)64 dendrimer. (a) Alkyne-terminated dendrimer was clicked first to CB[7]-N3 and subsequently to PEG-N3. (b) 1H-NMR in D2O was performed on the G5-PEG56CB8 dendrimer with and without the addition of p-xylylenediamine guest (probe) to verify the presence and average amount of CB[7] on the dendrimer. (c) Dynamic light scattering of G5-PEG56CB8 dendrimer. | |
Following reaction and purification, FT-IR confirmed the absence of the characteristic azide stretch (2160–2120 cm−1) and thus the product only contained reacted CB[7] and PEG chains (Fig. S1†). Paired with data from FT-IR, 1H-NMR performed on this product confirmed attachment of CB[7] via a triazole linkage, leveraging the known shift in protons of the p-xylylenediamine guest (probe) upon binding to CB[7] in order to enhance visualization of the macrocycle (Fig. 3b). Specifically, the 1
:
1 complex of this probe with CB[7] enables the exact content of CB[7] in the dendrimer to be measured from the integrated aromatic signals of the threaded (δ = 6.56 ppm) and free probe (δ = 7.46 ppm) in the spectra (details available in ESI†). Through this method, it was determined that each dendrimer contained an average of 8 CB[7] macrocycles, with the balance of the available sites being modified with PEG. This aligned with our intended extent of modification in the “click” reaction and also confirmed that the portal of CB[7] was accessible for binding to guest molecules following conjugation. This dendrimer is hereafter referred to as G5-PEG56CB8.
The ratio of CB[7]-N3 to G5(-yne)64 dendrimer in the “click” reaction was also varied to target either 4 or 12 CB[7] groups per dendrimer (Fig. S2†). Again, 1H-NMR quantification aided by the same probe as before was used and successfully verified the desired CB[7] modification which was consistent with the ratio at which CB[7] was added to the “click” reaction. These two dendrimers are referred to moving forward by comparable nomenclature as before (G5-PEG60CB4 and G5-PEG54CB12).
Using dynamic light scattering (DLS), the diameter of each dendrimer was measured (Fig. 3b and S3†). Accordingly, G5-PEG56CB8 was found to have a diameter of 12.7 nm. This was larger than the diameter measured for G5-PEG60CB4 (7.9 nm) but smaller than the diameter for G5-PEG54CB12 (110.8 nm). Each CB[7] has a relatively rigid diameter of ∼1.6 nm, and the addition of the ∼1200 Da CB[7] macrocycles to a G5(-yne)64 dendrimer core of approximately 12
000 Da would be expected to increase the effective particle diameter. It is also suspected that CB[7] would be more visible in light scattering than elongated and typically well-hydrated PEG chains, even though the PEG chains used here were of somewhat larger molecular weight than CB[7]. As such, the increase in diameter seen for G5-PEG56CB8 relative to G5-PEG60CB4 is reasonable. However, the very large diameter apparent in the sample of G5-PEG54CB12 is suggestive of particle aggregation which may arise from insufficient PEG shielding leading to association of patchy surfaces of particles having a higher density of CB[7]. Such aggregation is not desirable for a nanoscale drug carrier. Accordingly, in an effort to maximize the number of CB[7] carriers appended to each dendrimer without inducing aggregation, G5-PEG56CB8 was used throughout the remainder of the studies reported here.
Prodrug synthesis and characterization
One benefit of attaching CB[7] to a nanoparticle or material intended for use in drug delivery is the inherent modularity it affords in subsequently loading a variety of drugs. For example, a drug could be modified, perhaps using a labile linker common in the creation of attenuated prodrug molecules and drug carriers,48 for attachment to a guest that binds with high affinity (e.g., 1012 M−1) to CB[7]. Then, the CB[7]-modified drug carrier could be used in a “mix-and-match” fashion to deliver any desired drug which offered a handle for guest attachment. By this approach, a doxorubicin prodrug (Dox-Ad) was synthesized by conjugating the drug to a high-affinity adamantyl amine guest using a short acyl hydrazone linker (Fig. 4a). Full synthetic details for the creation of this prodrug as well as 1H-NMR spectra of intermediates and the final product are found in the ESI† (Fig. S4 and S5). For assurance in the synthesis and assignment of spectra of this prodrug species, 13C-NMR and 1H–1H-COSY NMR were also performed (Fig. S6 and S7†) along with electrospray ionization mass spectrometry. As such, the prodrug synthesis was verified and NMR could be fully assigned (Fig. 4b).
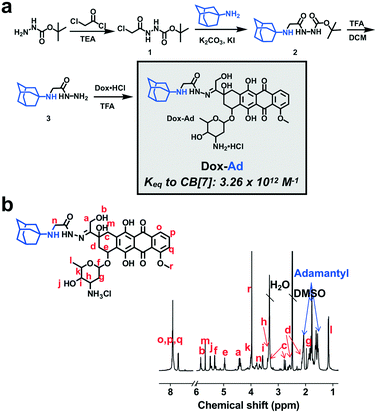 |
| Fig. 4 Design of Dox-Ad prodrug for binding to CB[7]. (a) Synthetic scheme to arrive at a doxorubicin variant modified with an adamantane guest via a labile hydrazone linker. Also listed is its binding affinity for CB[7] which was determined through competition 1H-NMR. (b) 1H-NMR in DMSO-d6 was performed to verify the synthesis and purity of this new prodrug. | |
The adamantyl amine guest in this design was intended to enable binding to CB[7] with affinity on the order of ∼1012 M−1. Our assumption for such binding was based on extensive literature evidence for binding of adamantane guests with adjacent protonating amines,49 as well as our own experience characterizing guest molecules of this type.47 In order to verify that Dox-Ad bound CB[7] with its expected affinity, competition 1H-NMR was performed for this molecule (Fig. S8†) against a known high-affinity guest, trimethylammoniumethyl ferrocene iodide.49 This method has been previously reported to enable the determination of binding constants in high-affinity regimes which are typically in excess of the limits of detection for more common calorimetric binding studies.31 By averaging three independent competition experiments, a binding constant of (3.26 ± 1.07) × 1012 M−1 was calculated for this new Dox-Ad prodrug. As such, this prodrug binds with extraordinarily high affinity to CB[7], which should promote complex stability and thereby overcome competition and extreme dilution in the body. Using similar affinity from another synthetic guest, we previously showed that binding on this same order of magnitude was so strong that it could facilitate stable recognition of separately administered components in a living animal.34 Loading of the G5-PEG56CB8 dendrimer with Dox-Ad at stoichiometric proportion did not impact the size of the nanoparticles, as measured by DLS (Fig. S3†).
Drug loading and controlled release
The inclusion of CB[7] in the described dendrimer platform was intended to enable modularity in the loading and delivery of guest-modified drugs. With the Dox-Ad prodrug exhibiting high-affinity binding in excess of 1012 M−1, this model drug payload was thus explored for loading onto the G5-PEG56CB8 dendrimer (Fig. 5a). When one equivalent of Dox-Ad was loaded relative to the number of CB[7] carriers on the dendrimer, the drug co-eluted with the dendrimer at the beginning of the HPLC chromatogram. However, when two equivalents were added relative to the number of CB[7] carriers on the dendrimer, half of the Dox-Ad co-eluted with the dendrimer while half eluted at the time expected for elution the unbound Dox-Ad compound. These studies further confirm 1
:
1 stoichiometry in bidding of Dox-Ad by CB[7]. This finding is particularly exciting, as it illustrates precise and quantitative drug loading onto G5-PEG56CB8 dendrimers which is directly determined by the number of CB[7] carriers installed. This general approach offers an opportunity to fully define drug loading on a nanoscale carrier and would be thought to more generally extend to related efforts by lending predictable and quantitative loading to other drug delivery materials or devices.
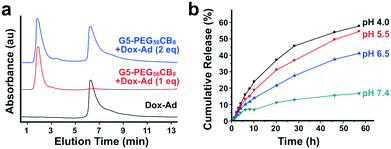 |
| Fig. 5 Dox-Ad loading and release. (a) HPLC absorbance traces measured at 260 nm for G5-PEG56CB8 dendrimer loaded with 2 equivalents of Dox-Ad per CB[7] (top) or 1 equivalent (middle), compared to the elution of the free Dox-Ad prodrug (bottom). (b) Quantified free doxorubicin release from G5-PEG56CB8 dendrimer as a function of varied solution pH. | |
Hydrazones of the type used in designing the model Dox-Ad payload are labile linkers which are known to hydrolyze at reduced pH, comparable to levels encountered in the peritumoral space or during endosomal processing following cellular internalization.50,51 Hydrazone linkers also become protonated at reduced pH,52 and while the impact of such protonation on CB[7]–guest binding was not explored here its position would not be expected to dramatically alter affinity. In order to verify pH-responsive release of doxorubicin, G5-PEG56CB8 dendrimers were loaded with Dox-Ad and exposed to buffers ranging in pH from 4.0 to 7.4 (Fig. 5b). When dialyzing against these buffers and sampling the bulk solution to quantify drug concentration by fluorescence, the expected pH-dependent trends in release were observed. This trend is in line with our previous observations for other doxorubicin conjugates linked to guests using hydrazone linkers.34 In addition, these data for differential release as a function of pH support that the host–guest interaction which keeps the drug bound to the dendrimer is stable and has very slow dynamics. When combined with our previous results showing the stability and long-lived nature for CB[7]–guest interactions of 1012 M−1,34 these data support release which occurs almost exclusively due to linker rupture rather than release of intact prodrug, validating the need to include the hydrazone linker in the design of Dox-Ad. The CB[7]–adamantyl amine motif has koff which can be estimated to be on the order of ∼10−4–10−5 s−1 based on estimates for a diffusion-governed interaction. As such, the use of this modular approach which assures quantitative and stable drug loading also necessitates the use of a labile linker between the drug and guest to account for the very slow inherent release possible from host–guest exchange.
In vitro functional studies
With quantitative drug loading and pH-responsive release verified for Dox-Ad in combination with the G5-PEG56CB8 dendrimer, in vitro function was next evaluated in a standard human breast cancer cell line (MDA-MB-231). When assessing potency of this chemotherapeutic (Fig. 6a), the G5-PEG56CB8 dendrimer loaded with Dox-Ad was roughly one order of magnitude less potent (IC50 = 1.07 μM) compared to delivery of the free drug (IC50 = 0.11 μM). We have previously observed a similar reduction in potency for hydrazone-linked guest–doxorubicin prodrugs compared to the parent drug,34 and as such the finding of reduced potency here was not surprising. In order to further assess the delivery function of this CB[7]-linked nanocarrier platform, fluorescence microscopy was performed (Fig. 6b), staining for endosomes/lysosomes (Lysotracker® Green) and cell nuclei (DAPI, blue), while also relying on the inherent fluorescence of the doxorubicin payload (red). This imaging revealed almost complete overlap of free doxorubicin with the cell nucleus, which is the site of action for this DNA-intercalating chemotherapy. By comparison, significantly more doxorubicin signal was found to overlap with the punctate staining of endocytotic vesicles when Dox-Ad was delivered with the G5-PEG56CB8 dendrimer, suggesting that the majority of the drug remains in endosomal processing and associated with the nanocarrier. Accordingly, limited drug trafficking to the nucleus likely accounts for the reduced potency of Dox-Ad delivered with our dendrimer nanocarriers in these studies, yet some drug still becomes available to function in inducing apoptosis of these cancer cells. The stability of the CB[7]–guest complex in physiological conditions thus would necessitate even more rapidly hydrolyzing linkers in order to achieve comparable drug function to the unmodified doxorubicin, but such a design would also sacrifice stability of the linker for systemic applications in circulation, leading to undesirable drug leakage. Ongoing work is evaluating the utility of integrating targeting motifs onto the surface of these nanocarriers so as to enable enhanced accumulation in cells or tissues of interest once introduced in the body, leveraging improvements in tissue localization from targeting to overcome the reduced potency observed here. In addition, we are exploring other labile linker chemistries for more rapid and potent drug action upon cell internalization.
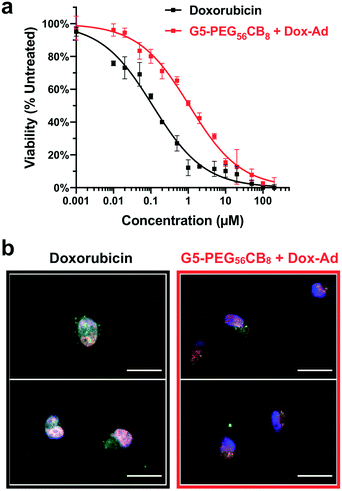 |
| Fig. 6
In vitro evaluation of drug delivery. (a) Dose–response for the delivery of Dox-Ad by G5-PEG56CB8 dendrimer compared to doxorubicin alone in MDA-MB-231 cells (n = 3/point). Data normalized to untreated cells and fit to a dose–response curve (R2 > 0.99). (b) Fluorescence microscopy of MDA-MB-231 cells treated with doxorubicin (left) or Dox-Ad delivered using the G5-PEG56CB8 dendrimer (right), showing lysosomes (green), doxorubicin (red), and cell nuclei (blue). All scale bars are 25 μm. | |
Conclusions
In spite of progress in the field of nanomedicine, controlling the size and drug loading of nanoscale drug delivery vehicles remains a challenge. Here, we have coupled two highly efficient chemical modalities by combining high-yielding orthogonal dendrimer chemistry with high-affinity supramolecular recognition from the attachment of CB[7] macrocycles. This platform resulted in defined nanoscale dendrimer architectures with a tunable number of appended CB[7] macrocycle carriers. In demonstrating a possible use of this modular nanocarrier, doxorubicin was conjugated to a high-affinity guest for CB[7], enabling quantitative drug loading with release controlled using a pH-responsive linker. These CB[7]-modified dendrimers were able to deliver active drug to cancer cells in vitro. This molecularly engineered approach addresses common problems encountered in nanoparticle design by combining two disparate fields of chemistry which each offer predictable outcomes. Furthermore, this is a generalizable platform approach with “mix-and-match” possibilities. CB[7] carriers could be affixed to a variety of other materials or devices for drug delivery, and provided a drug of interest can be modified with a high-affinity guest for this macrocycle, predictable and quantitative drug loading can be achieved. As the field of molecular engineering continues to seek improved definition in molecular/material form leading to transcendent function, this platform offers a new design opportunity.
Experimental section
Detailed experimental methods and complete molecular characterization are available in the ESI.† In brief, dendrimers were synthesized by alternating generational growth from orthogonal epoxy–amine and thiol–yne conjugation (Fig. 2a). Dendrimers were characterized throughout the synthesis using a combination of 1H-NMR and gel permeation chromatography (GPC) to confirm expected radial growth with each synthetic step. CB[7]-N3 (Fig. 3a) was synthesized according to literature,43 and attached to terminal alkynes on the dendrimer by copper-catalyzed “click” chemistry. Remaining alkynes were modified by “click” chemistry with 2000 Da PEG-N3. Click conjugation was verified by FT-IR as well as 1H-NMR with the aid of a guest probe which binds in the portal of CB[7] to enable NMR visualization of the macrocycle. To create a model payload, doxorubicin was modified with an adamantyl–amine guest using an acyl hydrazone linker (Fig. 4a). Successful synthesis was verified by 1H-NMR, 13C-NMR, and 1H–1H-COSY NMR. Its affinity for CB[7] was confirmed by competition 1H-NMR, according to previously published methodology for determining Keq in high-affinity motifs.31 Quantitative and stoichiometric drug loading was verified using HPLC, and pH-dependent hydrolysis of the hydrazone linker was verified by dialysis against different pH buffers and measuring free drug by fluorescence. Functional studies were performed in vitro in MDA-MB-231 cells to assess viability upon dendrimer-mediated drug delivery, as well as cell internalization using fluorescence microscopy with the aid of a dye (Lysotracker® Green) which labels lysosomes.
Conflicts of interest
There are no conflicts to declare.
Acknowledgements
M. J. W. acknowledges funding support from the Harper Cancer Research Institute American Cancer Society Institutional Research Grant (IRG-14-195-01) and the University of Notre Dame “Advancing our Vision” initiative.
Notes and references
- S. M. Moghimi, A. C. Hunter and J. C. Murray, Nanomedicine: current status and future prospects, FASEB J., 2005, 19(3), 311–330 CrossRef CAS.
- V. Wagner, A. Dullaart, A. K. Bock and A. Zweck, The emerging nanomedicine landscape, Nat. Biotechnol., 2006, 24(10), 1211–1217 CrossRef CAS PubMed.
- B. Y. Kim, J. T. Rutka and W. C. Chan, Nanomedicine, N. Engl. J. Med., 2010, 363(25), 2434–2443 CrossRef CAS PubMed.
- S. Galindo-Rodriguez, E. Allemann, H. Fessi and E. Doelker, Physicochemical parameters associated with nanoparticle formation in the salting-out, emulsification-diffusion, and nanoprecipitation methods, Pharm. Res., 2004, 21(8), 1428–1439 CrossRef CAS.
- B. D. Chithrani, A. A. Ghazani and W. C. Chan, Determining the size and shape dependence of gold nanoparticle uptake into mammalian cells, Nano Lett., 2006, 6(4), 662–668 CrossRef CAS.
- W. Jiang, B. Y. S. Kim, J. T. Rutka and W. C. W. Chan, Nanoparticle-mediated cellular response is size-dependent, Nat. Nanotechnol., 2008, 3(3), 145–150 CrossRef CAS PubMed.
- M. Lundqvist, J. Stigler, G. Elia, I. Lynch, T. Cedervall and K. A. Dawson, Nanoparticle size and surface properties determine the protein corona with possible implications for biological impacts, Proc. Natl. Acad. Sci. U. S. A., 2008, 105(38), 14265–14270 CrossRef CAS.
- A. Stano, C. Nembrini, M. A. Swartz, J. A. Hubbell and E. Simeoni, Nanoparticle size influences the magnitude and quality of mucosal immune responses after intranasal immunization, Vaccine, 2012, 30(52), 7541–7546 CrossRef CAS.
- L. Tang, X. Yang, Q. Yin, K. Cai, H. Wang, I. Chaudhury, C. Yao, Q. Zhou, M. Kwon, J. A. Hartman, I. T. Dobrucki, L. W. Dobrucki, L. B. Borst, S. Lezmi, W. G. Helferich, A. L. Ferguson, T. M. Fan and J. Cheng, Investigating the optimal size of anticancer nanomedicine, Proc. Natl. Acad. Sci. U. S. A., 2014, 111(43), 15344–15349 CrossRef CAS.
- S. E. McNeil, Nanoparticle therapeutics: a personal perspective, Wiley Interdiscip. Rev.: Nanomed. Nanobiotechnol., 2009, 1(3), 264–271 CAS.
- K. S. Chu, A. N. Schorzman, M. C. Finniss, C. J. Bowerman, L. Peng, J. C. Luft, A. J. Madden, A. Z. Wang, W. C. Zamboni and J. M. DeSimone, Nanoparticle drug loading as a design parameter to improve docetaxel pharmacokinetics and efficacy, Biomaterials, 2013, 34(33), 8424–8429 CrossRef CAS.
- N. Kamaly, B. Yameen, J. Wu and O. C. Farokhzad, Degradable Controlled-Release Polymers and Polymeric Nanoparticles: Mechanisms of Controlling Drug Release, Chem. Rev., 2016, 116(4), 2602–2663 CrossRef CAS.
- M. J. Liu and J. M. J. Frechet, Designing dendrimers for drug delivery, Pharm. Sci. Technol. Today, 1999, 2(10), 393–401 CrossRef CAS.
- E. R. Gillies and J. M. J. Frechet, Dendrimers and dendritic polymers in drug delivery, Drug Discovery Today, 2005, 10(1), 35–43 CrossRef CAS.
- P. Kesharwani, K. Jain and N. K. Jain, Dendrimer as nanocarrier for drug delivery, Prog. Polym. Sci., 2014, 39(2), 268–307 CrossRef CAS.
- I. N. Kurniasih, J. Keilitz and R. Haag, Dendritic nanocarriers based on hyperbranched polymers, Chem. Soc. Rev., 2015, 44(12), 4145–4164 RSC.
- E. Abbasi, S. F. Aval, A. Akbarzadeh, M. Milani, H. T. Nasrabadi, S. W. Joo, Y. Hanifehpour, K. Nejati-Koshki and R. Pashaei-Asl, Dendrimers: synthesis, applications, and properties, Nanoscale Res. Lett., 2014, 9, 247 CrossRef.
- C. J. Hawker and J. M. J. Frechet, Preparation of Polymers with Controlled Molecular Architecture - a New Convergent Approach to Dendritic Macromolecules, J. Am. Chem. Soc., 1990, 112(21), 7638–7647 CrossRef CAS.
- C. C. Lee, J. A. MacKay, J. M. J. Frechet and F. C. Szoka, Designing dendrimers for biological applications, Nat. Biotechnol., 2005, 23(12), 1517–1526 CrossRef CAS.
- K. L. Killops, L. M. Campos and C. J. Hawker, Robust, efficient, and orthogonal synthesis of dendrimers via thiol-ene "Click" chemistry, J. Am. Chem. Soc., 2008, 130(15), 5062–5064 CrossRef CAS.
- J. B. Wolinsky and M. W. Grinstaff, Therapeutic and diagnostic applications of dendrimers for cancer treatment, Adv. Drug Delivery Rev., 2008, 60(9), 1037–1055 CrossRef CAS.
- M. A. Mintzer and M. W. Grinstaff, Biomedical applications of dendrimers: a tutorial, Chem. Soc. Rev., 2011, 40(1), 173–190 RSC.
- A. D'Emanuele and D. Attwood, Dendrimer-drug interactions, Adv. Drug Delivery Rev., 2005, 57(15), 2147–2162 CrossRef.
- L. M. Kaminskas, B. J. Boyd and C. J. H. Porter, Dendrimer pharmacokinetics: the effect of size, structure and surface characteristics on ADME properties, Nanomedicine, 2011, 6(6), 1063–1084 CrossRef CAS.
- M. J. Webber, E. A. Appel, E. W. Meijer and R. Langer, Supramolecular biomaterials, Nat. Mater., 2016, 15(1), 13–26 CrossRef CAS.
- M. J. Webber and R. Langer, Drug delivery by supramolecular design, Chem. Soc. Rev., 2017, 46(21), 6600–6620 RSC.
- J. Kim, I. S. Jung, S. Y. Kim, E. Lee, J. K. Kang, S. Sakamoto, K. Yamaguchi and K. Kim, New cucurbituril homologues: Syntheses, isolation, characterization, and X-ray crystal structures of cucurbit[n]uril (n=5, 7, and 8), J. Am. Chem. Soc., 2000, 122(3), 540–541 CrossRef CAS.
- S. J. Barrow, S. Kasera, M. J. Rowland, J. del Barrio and O. A. Scherman, Cucurbituril-Based Molecular Recognition, Chem. Rev., 2015, 115(22), 12320–12406 CrossRef CAS.
- W. S. Jeon, K. Moon, S. H. Park, H. Chun, Y. H. Ko, J. Y. Lee, E. S. Lee, S. Samal, N. Selvapalam, M. V. Rekharsky, V. Sindelar, D. Sobransingh, Y. Inoue, A. E. Kaifer and K. Kim, Complexation of ferrocene derivatives by the cucurbit[7]uril host: A comparative study of the cucurbituril and cyclodextrin host families, J. Am. Chem. Soc., 2005, 127(37), 12984–12989 CrossRef CAS.
- J. Mohanty, A. C. Bhasikuttan, W. M. Nau and H. Pal, Host-guest complexation of neutral red with macrocyclic host molecules: Contrasting pK(a) shifts and binding affinities for cucurbit[7]uril and beta-cyclodextrin, J. Phys. Chem. B, 2006, 110(10), 5132–5138 CrossRef CAS.
- L. Cao, M. Sekutor, P. Y. Zavalij, K. Mlinaric-Majerski, R. Glaser and L. Isaacs, Cucurbit[7]urilguest pair with an attomolar dissociation constant, Angew. Chem., Int. Ed., 2014, 53(4), 988–993 CrossRef CAS.
- H. Tang, D. Fuentealba, Y. H. Ko, N. Selvapalam, K. Kim and C. Bohne, Guest binding dynamics with cucurbit[7]uril in the presence of cations, J. Am. Chem. Soc., 2011, 133(50), 20623–20633 CrossRef CAS PubMed.
- J. Zhang and P. X. Ma, Cyclodextrin-based supramolecular systems for drug delivery: recent progress and future perspective, Adv. Drug Delivery Rev., 2013, 65(9), 1215–1233 CrossRef CAS PubMed.
- L. Zou, A. S. Braegelman and M. J. Webber, Spatially Defined Drug Targeting by in Situ Host-Guest Chemistry in a Living Animal, ACS Cent. Sci., 2019, 5(6), 1035–1043 CrossRef CAS.
- S. Walker, R. Oun, F. J. McInnes and N. J. Wheate, The Potential of Cucurbit[n]urils in Drug Delivery, Isr. J. Chem., 2011, 51(5–6), 616–624 CrossRef CAS.
- N. J. Wheate and C. Limantoro, Cucurbit[n]urils as excipients in pharmaceutical dosage forms, Supramol. Chem., 2016, 28(9–10), 849–856 CrossRef CAS.
- P. Antoni, M. J. Robb, L. Campos, M. Montanez, A. Hult, E. Malmstrom, M. Malkoch and C. J. Hawker, Pushing the Limits for Thiol-Ene and CuAAC Reactions: Synthesis of a 6th Generation Dendrimer in a Single Day, Macromolecules, 2010, 43(16), 6625–6631 CrossRef CAS.
- M. V. Walter and M. Malkoch, Simplifying the synthesis of dendrimers: accelerated approaches, Chem. Soc. Rev., 2012, 41(13), 4593–4609 RSC.
- D. Konkolewicz, A. Gray-Weale and S. Perrier, Hyperbranched Polymers by Thiol-Yne Chemistry: From Small Molecules to Functional Polymers, J. Am. Chem. Soc., 2009, 131(50), 18075–18077 CrossRef CAS PubMed.
- T. Kang, R. J. Amir, A. Khan, K. Ohshimizu, J. N. Hunt, K. Sivanandan, M. I. Montanez, M. Malkoch, M. Ueda and C. J. Hawker, Facile access to internally functionalized dendrimers through efficient and orthogonal click reactions, Chem. Commun., 2010, 46(9), 1556–1558 RSC.
- R. J. Amir, L. Albertazzi, J. Willis, A. Khan, T. Kang and C. J. Hawker, Multifunctional trackable dendritic scaffolds and delivery agents, Angew. Chem., Int. Ed., 2011, 50(15), 3425–3429 CrossRef CAS PubMed.
- Y. Shen, Y. N. Ma and Z. B. Li, Facile Synthesis of Dendrimers Combining aza-Michael Addition with Thiol-yne Click Chemistry, J. Polym. Sci., Part A: Polym. Chem., 2013, 51(3), 708–715 CrossRef CAS.
- B. Vinciguerra, L. P. Cao, J. R. Cannon, P. Y. Zavalij, C. Fenselau and L. Isaacs, Synthesis and Self-Assembly Processes of Monofunctionalized Cucurbit[7]uril, J. Am. Chem. Soc., 2012, 134(31), 13133–13140 CrossRef CAS PubMed.
- Y. Ahn, Y. Jang, N. Selvapalam, G. Yun and K. Kim, Supramolecular velcro for reversible underwater adhesion, Angew. Chem., Int. Ed., 2013, 52(11), 3140–3144 CrossRef CAS PubMed.
- L. Cao, G. Hettiarachchi, V. Briken and L. Isaacs, Cucurbit[7]uril containers for targeted delivery of oxaliplatin to cancer cells, Angew. Chem., Int. Ed., 2013, 52(46), 12033–12037 CrossRef CAS PubMed.
- M. M. Ayhan, H. Karoui, M. Hardy, A. Rockenbauer, L. Charles, R. Rosas, K. Udachin, P. Tordo, D. Bardelang and O. Ouari, Comprehensive Synthesis of Monohydroxy-Cucurbit[n]urils (n = 5, 6, 7, 8): High Purity and High Conversions, J. Am. Chem. Soc., 2015, 137(32), 10238–10245 CrossRef CAS.
- L. Zou, A. S. Braegelman and M. J. Webber, Dynamic Supramolecular Hydrogels Spanning an Unprecedented Range of Host-Guest Affinity, ACS Appl. Mater. Interfaces, 2019, 11(6), 5695–5700 CrossRef CAS PubMed.
- J. Khandare and T. Minko, Polymer-drug conjugates: Progress in polymeric prodrugs, Prog. Polym. Sci., 2006, 31(4), 359–397 CrossRef CAS.
- S. M. Liu, C. Ruspic, P. Mukhopadhyay, S. Chakrabarti, P. Y. Zavalij and L. Isaacs, The cucurbit[n]uril family: Prime components for self-sorting systems, J. Am. Chem. Soc., 2005, 127(45), 15959–15967 CrossRef CAS.
- K. Ulbrich and V. Subr, Polymeric anticancer drugs with pH-controlled activation, Adv. Drug Delivery Rev., 2004, 56(7), 1023–1050 CrossRef CAS.
- F. Kratz, DOXO-EMCH (INNO-206): the first albumin-binding prodrug of doxorubicin to enter clinical trials, Expert Opin. Invest. Drugs, 2007, 16(6), 855–866 CrossRef CAS.
- J. Kalia and R. T. Raines, Hydrolytic stability of hydrazones and oximes, Angew. Chem., Int. Ed., 2008, 47(39), 7523–7526 CrossRef CAS.
Footnote |
† Electronic supplementary information (ESI) available: Supplemental data and experimental methods. See DOI: 10.1039/c9me00088g |
|
This journal is © The Royal Society of Chemistry 2020 |
Click here to see how this site uses Cookies. View our privacy policy here.