DOI:
10.1039/C5BM00383K
(Review Article)
Biomater. Sci., 2016,
4, 219-229
Strategies for transporting nanoparticles across the blood–brain barrier
Received
15th September 2015
, Accepted 16th November 2015
First published on 8th December 2015
Abstract
The existence of blood–brain barrier (BBB) hampers the effective treatment of central nervous system (CNS) diseases. Almost all macromolecular drugs and more than 98% of small molecule drugs cannot pass the BBB. Therefore, the BBB remains a big challenge for delivery of therapeutics to the central nervous system. With the structural and mechanistic elucidation of the BBB under both physiological and pathological conditions, it is now possible to design delivery systems that could cross the BBB effectively. Because of their advantageous properties, nanoparticles have been widely deployed for brain-targeted delivery. This review paper presents the current understanding of the BBB under physiological and pathological conditions, and summarizes strategies and systems for BBB crossing with a focus on nanoparticle-based drug delivery systems. In summary, with wider applications and broader prospection the treatment of brain targeted therapy, nano-medicines have proved to be more potent, more specific and less toxic than traditional drug therapy.
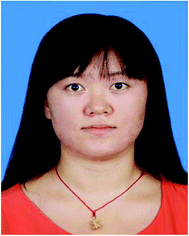 Tian-Tian Zhang | Tiantian Zhang is now a Ph.D. candidate in the School of Light Industry and Food Science at South China University of Technology. Her research mainly focuses on nutrition and functional food factors, as follows: (a) the functional evaluation of natural food or active ingredients and their molecular mechanisms; (b) chemical biology research of functional factors in vivo; (c) the molecular basis of functional food development by nanotechnology. |
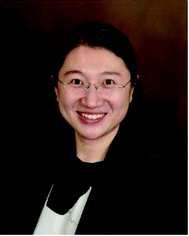 Wen Li | Wen Li, Proteomics Laboratory Scientist from IHRC, Inc. She graduated as Ph.D. of molecular genetics and biochemistry from Georgia State University. Her research mainly focuses on two areas: (1) multidrug resistance in cancer: identifying the structure and function of the ABC drug transporter involved in drug efflux; (2) identifying cell surface antigen targets of microorganisms using genomics, transcriptomics and proteomics analyses. |
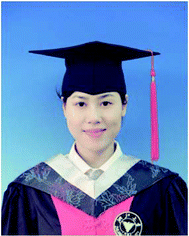 Guanmin Meng | Dr Guanmin Meng completed her Ph.D. in Biochemistry and Molecular Biology at Zhejiang University in December 2011. From 2012 to date, she is a medical laboratory scientist in the Department of Clinical Laboratory in Tongde Hospital of Zhejiang Province. Currently, she is also a visiting researcher in the Department of Biochemistry and Signal Transduction Research Group at the University of Alberta, Canada. Her research mainly focuses on the key molecules involved in the progression of tumours, spread (metastasis) and the development of resistance to chemotherapy and radiotherapy, and to discover and develop specific herbal extracts or combination formulae. |
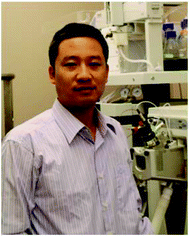 Pei Wang | Dr Pei Wang completed his Ph.D. in pharmaceutical analysis at Shenyang Pharmaceutical University in June 2015. He is currently a postdoctoral associate at the Center for Excellence in Post-Harvest Technologies (CEPHT) at North Carolina Agricultural and TechnicalState University, North Carolina Research Campus. His research mainly focuses on studying the bioavailability, biotransformation, and pharmacokinetics of drug candidates in animals and humans. |
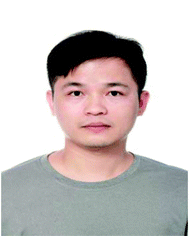 Wenzhen Liao | Wenzhen Liao obtained his Ph.D. degree in Food Science at South China University of Technology in June 2015. His present interest mainly focuses on these areas: (1) molecular design, synthesis, modification and biological activity evaluation of bio-nanomaterials. (2) Purification, structure characterization and bioactivity evaluation (immunoregulatory activity, cytotoxicity, anti-oxidant, anti-hemolysis and anti-inflammatory, etc.) of active compounds in food. |
1. Introduction
Central nervous system (CNS) diseases, such as Alzheimer's disease, Parkinson's disease, brain cancers, and strokes, have become more and more common and are the second largest category of life-threatening diseases.1,2 However, the success rate of CNS drug development is very low.3 Discovery of new drugs treating CNS disease is restricted by many factors, and the most important one is the difficulty for drugs to pass through the blood–brain barrier (BBB).4,5 Studies show that the ability of the drug to travel through the BBB highly depends on the size of the molecules, lipid solubility, hydrophilicity, degree of dissociation, and other properties.6,7 Almost all macromolecular drugs, including polypeptides, recombinant proteins, monoclonal antibodies, and drugs based on RNA interference technologies, as well as more than 98% of small molecule drugs, cannot pass through the BBB, which has seriously hampered the success of clinical therapy for CNS diseases.8 Therefore, along with having suitable bioactivity, metabolic and low toxicity properties, CNS drugs must also be able to overcome the BBB and achieve sufficient exposure in the CNS, which are the keys to the success of CNS drug research and development. The BBB is a bottleneck in the medication of CNS diseases, and the development of effective drug delivery systems has received substantial attention.
Many nanocarriers, including polymeric nanoparticles, inorganic nanoparticles, liposomes, nanofibers, and micelles have been engineered to deliver therapeutic and diagnostic agents.9–13 Nanoparticles have a series of advantages, such as relatively high drug loading, stability in body fluids and storage conditions, controlled drug release and targeting effect, and easily industrialized production. They have become the hot spot of brain targeted research.14,15 Nanoparticles can carry drugs through the BBB and act in the CNS, especially after surface modification. They can avoid phagocytosis by the reticuloendothelial system to make drugs available through the BBB and significantly improve the concentration of drugs in the brain, which has become an important component of not only basic research, but also applied research on drug delivery systems.16
Nanoparticles are solid colloidal particles which are composed of a polymer or lipid. Particle size ranges from 10–1000 nm (usually 50–300 nm).17 Drugs can be embedded either in a matrix or deposited on the surface, and the whole particle can be targeted to specific parts of the body in a controllable manner.18,19 Nanoparticles used as drug carriers should have the following properties: (1) non-toxic, biodegradable and biocompatible; (2) particle diameter <100 nm; (3) stability in the blood and no aggregation reaction; (4) the particles must not be taken up by the mononuclear phagocytic system (no conditioning effect) and must possess a long blood circulation time; (5) targeted delivery of drugs through the BBB into the brain (via receptor mediated endocytosis of brain capillary endothelial cells); (6) suitable for carrying small molecules, peptides, proteins or nucleotides; (7) minimal drug changes (chemical degradation/structural changes, protein denaturation) induced by the nanoparticle excipient; (8) controllable drug release; (9) economic and efficient production process.
Many kinds of nanoparticles have been developed so far, nanoparticles that carry the CNS drugs into the brain include lipid based nanoparticles, polymer based nanoparticles, nanoemulsions, dendrimers etc.20,21 Lipid-based nanoparticles, such as cationic liposomes, solid lipid nanoparticles and nanostructured lipid carriers, have been extensively studied for drug delivery to the brain.22 One application is the PEGylated liposomes, encapsulated with FK506 (Tacrolimus), were used to treat cerebral ischemia reperfusion injury. Research showed that FK506 encapsulated liposomes not only prevent cells from hydrogen peroxide induced toxicity, but also significantly suppressed neutrophil invasions and apoptotic cell death, at a much lower dosage than regular drug administration.23 The ATP-binding cassette (ABC) transporters, such as P-glycoprotein, multidrug resistance related proteins, play an essential role for the active transport of drugs through BBB. Liposome carried drugs were able to reduce the efflux of drugs out of the BBB by ABC drug transporters, thereby, increasing the cellular uptake of drugs.24–26 Polymeric nanoparticles are another extensively used drug delivery system targeting CNS. One of the latest studies described the effect of modification of the surface of polymeric nanoparticles on drug delivery. In order to improve the ability of nanoparticle to cross the BBB, the surface of polymeric nanoparticles were modified (named PLA-HPG-Ad), which showed significantly higher brain uptake and more controlled release of drugs.27 Nanoemulsions are another novel drug delivery system formed by emulsion oil and water. The average size of the nanoemulsion ranges from 100 to 500 nm. Saquinavir mesylate (SQVM) is a poorly BBB permeable anti-HIV drug. By incorporating SQVM into the nanoemulsion nanoparticle, the bioavailability increases drug permeation rate significantly. Higher drug distribution in the brain after intranasal administration was observed than plain drug suspensions.28
2. BBB in physiological and pathological conditions
The brain is the center of the central nervous system of the body, and the blood–brain barrier has evolved as a protection system. The BBB is a physiological barrier between blood and brain tissue; it plays an important role in maintaining the stability of the CNS. It is a dynamic regulation interface between the capillaries in the brain, spinal cord and the nerve tissue. Under normal physiological conditions, only gas molecules and small lipid soluble molecules (with a molecular weight less than 400–600) are allowed through the barrier. In addition to passive protection, it can also selectively pump harmful or excess substances out of the brain to maintain the stability of the brain's internal environment, so that the CNS can function effectively.29,30 These features of the BBB allow it to play an essential role in the protection of the brain; however, they also create difficulties in developing drugs that can pass through the BBB.
Anatomically, the BBB is a layer of continuous endothelial cell membrane which covers 99% of the cerebral capillary surface. It is composed of three parts: the brain micro vessel endothelial cells (BMVEC) and tight junctions in the innermost layer, the matrix and peripheral cells in the middle layer, and the astrocytes and extracellular matrix in the outermost layer.31,32 The main structure of the BBB are endothelial cells, and CNS drugs must pass through endothelial cells to enter the brain cells. Besides the physical barrier formed by endothelial cells and astrocytes, the BBB also includes a biochemical barrier composed of a variety of enzymes and transporters.33 Tight junctions overlapping BMVECs (physical), high resistance between BMVECs (electrochemical), and the highly efficient efflux system on the cell membrane (physiological) constitute the physiological bases of BBB (Fig. 1).33 The permeation mechanisms of the BBB include passive diffusion, active influx, and efflux (Fig. 2). Passive diffusion allows the free movement of small molecules across the membrane.34 Under normal circumstances, the effective pore size of the brain capillary endothelial cells is 1.4–1.8 nm, and small molecules with less than 1.8 nm in diameter can be moved by passive diffusion through the BBB. Active influx is the transport method of inverse concentration difference and reverse electrochemical gradient, which requires the coupling of energy and carrier proteins.35,36 The efflux system mainly excretes toxic metabolites and heterologous substances to maintain the normal physiological function of the brain.37–40 When the brain develops a neurological disorder, either due to inflammation or pathogen invasion, the properties of the BBB undergo significant changes. Under pathological conditions, all cells, including the blood vessel endothelium in the central nervous system, can induce the generation of arachidonic acid, nitric oxide, cytokine, adhesion molecules, and other vasoactive substances, thereby affecting the structure and function of the BBB. Harmful particles will enter the brain, and brain damage will be further aggravated. The BBB permeability to sucrose, horseradish peroxidase, or plasma protein increases after cerebral ischemia.41,42 The BBB is opened in two phases after cerebral ischemia reperfusion. A sensitive quantitative fluorescence method was used to explore the time course and regional pattern of BBB opening after transient middle cerebral artery occlusion (MCAo) and the results indicated that an initial, acute disruption of the BBB occurs between 3 and 5 h following MCAo, and that a later, more widespread increase in regional BBB permeability is present at 48 h, suggesting that cerebral ischemia reperfusion caused BBB breakdown and was accompanied by the further expansion of cerebral infarct size.43 A large amount of oxygen free radicals can be generated after cerebral ischemia reperfusion, which does further damage to membrane structure and function. Hypoxia is also linked to changes in BBB permeability, and loss of BBB integrity is characteristic of many pathological brain diseases, including stroke. The brain microvascular endothelial cells produce a large number of free radicals in the hypoxic stimulation, which destroys the tight junctions of endothelial cells; astrocytes eliminate free radicals to reduce the damage caused by hypoxia.44 With the extension of hypoxia time; however, astrocyte function will also be affected, which reduces the protective effects on the BBB. Cevik et al. indicated that the hyperbaric oxygen (HBO) administration to intact rats increased BBB permeability to both Evans blue dye and horseradish peroxidase, while hyperbaric air (HBA) increased only horseradish peroxidase extravasation in these animals, suggesting that HBA also impairs the BBB integrity in intact rats as well as HBO.45 Ginsenoside Rg1 may protect the structure of the BBB by protecting BMVECs and reducing the expression and activity of matrix metalloproteinases under pathological conditions.46 In the cerebral ischemia recovery period, the neuronal regeneration and recovery of neurological function are critical. It is difficult, however, for drugs with functions of promoting neuronal regeneration and recovery of neurological effects to play a role in the brain due to the BBB specificity.47,48 Therefore, in the early stage of cerebral ischemia, inhibition of BBB opening can reduce brain damage caused by ischemia. Conversely, in the cerebral ischemia recovery period, the timely BBB opening can be utilized to administer effective drugs into the brain and improve neuronal regeneration and recovery of neurological function after ischemia.49,50
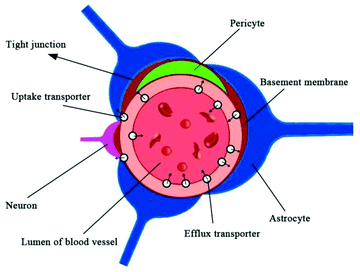 |
| Fig. 1 Schematic representation of the blood–brain barrier (BBB). | |
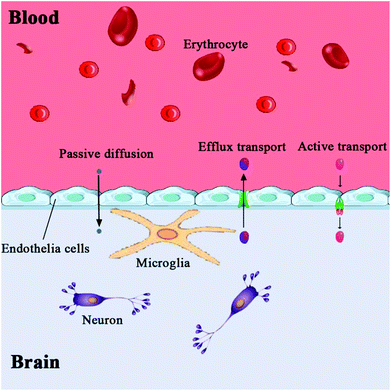 |
| Fig. 2 Permeation mechanism of the blood–brain barrier (BBB). | |
3. Strategies for nanoparticles to cross the BBB
Many drug delivery methods have been identified using a large number of experiments with animal models and clinical studies. A large variation of drugs has been successfully transported into the brain across the BBB using nanoparticles.
3.1. Bypass BBB via intranasal delivery
Intranasal delivery has the positive characteristics of rapid absorption, rapid onset, non-destructive, non-invasive, and ease of use, which has become one of the most popular methods in drug preparation and delivery.51,52 Drugs can be delivered to the cerebral spinal fluid or the brain through the olfactory mucosa, along the connective tissue around the olfactory nerve bundle or axons of olfactory neurons, thus bypassing the BBB to play a therapeutic role in the CNS (Fig. 3).53 A variety of peptides, proteins and small molecule drugs can be delivered into the brain via intranasal delivery.4 Fonseca et al. proposed amphiphilic methacrylic copolymer-functionalized poly(epsilon-caprolactone) nanocapsules as a muco-adhesive system to deliver olanzapine through intranasal administration.54 Results showed that these nanocapsules could interact with mucin and nasal mucosa, and increase the retention of olanzapine (about 40%) on the nasal mucosa after continuous washing. The result shows that the nanocapsules enhanced the amount of olanzapine in the rats’ brains (1.5-fold higher compared to the drug solution). In addition, nanoencapsulated olanzapine did not affect the nasal mucosa integrity after repeated doses. These data demonstrated that the designed nanocapsule is a promising muco-adhesive system for nose-to-brain drug delivery.54
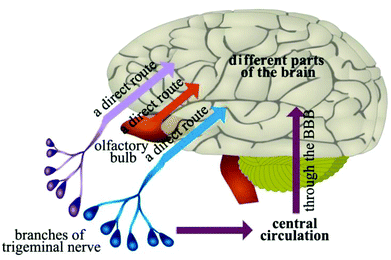 |
| Fig. 3 Schematic of the pathways of drug delivery to the brain from the nasal cavity. | |
Intranasal delivery is limited; however, by drug dose, physical and chemical properties. The surface area of the olfactory region of the nasal epithelium is about 50% in rodent, and only 5% in human. Therefore, intranasal delivery is not expected to achieve therapeutic drug levels in most brain regions. Kozlovskaya et al. analyzed the available quantitative data on intranasal delivery to reveal the efficiency of brain drug delivery and targeting by different types of nasally-administered delivery systems.55 Results indicated that efficiency of intranasal delivery differs dramatically between the studies, and does not correlate with the drug's physicochemical properties. Combined with the intranasal administration of drug solution, particle- and gel-based delivery systems offer limited advantages for brain drug delivery. Nevertheless, incorporation of specialized reagents (e.g., absorption enhancers, muco-adhesive compounds, targeting residues) can increase the efficiency of drug delivery to the brain via the nasal route.55
Drug delivery via the nasal route to the brain, as an alternative route for the vaccine administration, has been studied extensively;4,56–58 however, quantitative analysis of the efficiency of drug delivery by nasal route varies significantly between studies.53,59,60 Deeper understanding of the mechanism of intranasal delivery and more reliable quantification methodologies are required for qualification and standardization of the drug delivery system.59,61
3.2. Physical methods (invasive) for drug transport across the BBB
Blood–brain barrier disruption (BBBD) is one of the most prevalent methods for enhancing the delivery of drugs to the brain and allows drugs to flow directly from the circulatory system into the brain.62 Some barrier disruption methods include osmotic disruption, ultrasound disruption, and magnetic disruption.
Mannitol can be used to open the blood–brain barrier temporarily, however, its effectiveness varies based on the protocol of mannitol injection.63 The factors that influence the BBB opening are mannitol concentration, administration speed, time and retention time after injection.64 The main mechanism of BBB opening induced by mannitol is: the vascular endothelial cells are dehydrated, causing shrink and disruption of the tight junctions. The permeability of the blood–brain barrier is increased thereafter, which causes irreversible CNS damage.65 Materials such as mannitol, Arabia sugar, urea, fructose, milk amide and glycerol can induce a high osmotic pressure, opening the BBB. However, such alteration of the internal environment of the brain allows other high-molecular weight substances to cross the BBB, penetrate into the brain and induce neuropathological changes such as myelin disintegration.66 Opening the BBB will allow some toxic and harmful substances to enter the brain during a short period of time, which may affect the normal functions of CNS.67 If the concentration of hypertonic solution is too high, it will damage the brain.68
Convection-enhanced delivery (CED) has been considered as a promising technique for bypassing the BBB to deliver therapeutic agents.69 It relies on the method of positive pressure and continuous infusion solution over hours to days to maintain a constant pressure gradient and promote interstitial fluid convection, as a complement to osmotic effects. It enhances the diffusion of small molecules, macromolecules, macromolecular proteins, and other drugs, making it a good drug delivery system to cross the BBB.70 It has been observed that the combination of nanoliposomal irinotecan and radiation shows greater survival benefit than the irinotecan or radiation alone, or radiation with vascularly administered irinotecan.71 Animal studies and clinical trials using this technique revealed that it may require further optimization before it can be safely used in humans. Although the distribution volume in the target tissue can be controlled, the geometrical spread into a desired target region is highly variable from one experiment to another.72
In recent years, ultrasound-based techniques have also been shown to reversibly open the blood–brain barrier.73 The ultrasound-based drug delivery system depends on the capability of systemically-administered and acoustically-activated microbubbles to noninvasively, transiently and reversibly permeabilize vascular endothelium and cellular membranes, thus allowing drug molecules to cross the barrier and to be delivered to the brain parenchyma.74 Alkins et al. investigated the use of focused ultrasound with injectable microbubbles, to noninvasively and focally augment the uptake of B-10-enriched L-4-boronophenylalanine-fructose (BPA-f).75 A 9L gliosarcomatumor model was used in Fisher 344 rats, and the barriers were disrupted with pulsed ultrasound using a 558 kHz transducer and Definity microbubbles. Results showed that ultrasound increases the accumulation of B-10 in the main tumor and infiltrating cells, this focused ultrasound with the microbubbles drug delivery system may offer improvements in boron neutron capture therapy and the treatment of glioblastoma.75 Aryal et al. also found that the focused ultrasound technique could enhance the delivery of liposomal doxorubicin, which has a pronounced therapeutic effect in a rat glioma model.76
Magnetic gradients can increase transport rates of nanoparticle ferrofluids. When the magnetic nanoparticles are exposed to different magnetic fields, the nanoparticles will generate heat due to magnetic hysteresis loss and become a locally strong heat source in the alternating magnetic field (Fig. 4).77 Shinkai et al. utilized Fe3O4 nanoparticles as a new type of heating medium to study the subcutaneous hyperthermia effect of glioma tissues in F344 rats. Complete tumor regression was observed in about 90% of the rats.78 In pilot animal studies, Weinberg et al. had shown that magnetic gradients could dramatically increase the transport of magnetic nanoparticles across the cribiform plate into the brain olfactory bulb.79 Additional studies are underway to exploit the toxicity of magnetite (Fe3O4) and maghemite (γ-Fe2O3) particles.80–83 However, because of insufficient local accumulation and retention induced by their inability to traverse biological barriers, their therapeutic use in treating CNS pathologies in vivo is limited. Magnetic targeting in combination with focused ultrasound (FUS) can synergistically deliver therapeutic magnetic nanoparticles across the BBB to enter the brain both passively and actively. The technology can be used in normal or pathologically affected brains, and significantly increases the deposition of drug-coated magnetic nanoparticles in brains with intact or compromised blood–brain barriers.84–86 Lammers et al. found that BBB permeation can be mediated and monitored by poly(butyl cyanoacrylate)-based microbubbles (MB), which carry ultrasmall superparamagnetic iron oxide (USPIO) nanoparticles within their shell.85 When USPIO-MB was exposed to transcranial ultrasound pulses, the result was induction of vessel permeability by acoustic forces. At the same time, USPIO was released from the MB shell and extravasated across the permeabilized BBB to reach the brain tissue (Fig. 5). A key feature in this case is the double-targeting scenario. USPIO-MB is first magnetically guided to the non-target side by an external magnetic field and then transcranial ultrasound pulse exposure is used to locally disrupt the targeted BBB. The use of an external magnetic field combined with osmotic disruption can also enhance the permeability of iron oxide nanoparticles (IONPs) across a BBB model in vitro. The flux of IONPs across the bEnd3 cell monolayers was increased from 28% (only osmotic disruption) to 44% when a magnetic field was present.87
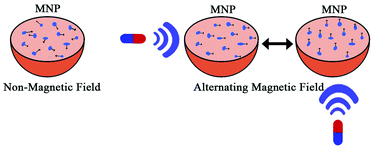 |
| Fig. 4 Schematic of magnetic nanoparticle (MNP) for thermotherapy. | |
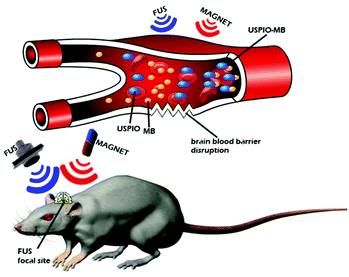 |
| Fig. 5 Schematic diagram of the experimental setup to demonstrate the concept of disrupting the blood–brain barrier (BBB). | |
3.3. Drug transport across the BBB via cell penetrating peptides (CPPs)
Cell penetrating peptides (CPPs) is a general designation for some short peptides with cell penetrating potential, they are generally less than 30 amino acids, composed predominantly of the basic amino acids.88 CPPs can not only carry small molecules into the cell, but can also deliver macromolecules (100 times higher than their own molecular weight), such as protein, plasmid, siRNA, nanoparticles, nucleic acid, liposome, etc.89 In addition, CPPs have the advantages of high biological safety and low cytotoxicity. Beyond acting as the carrier of drug molecules, some CPPs also have biological functions. These features of CPPs make them effective carriers for drug delivery, and provide a new and powerful tool for biological treatment.90–93 Owing to the differences in sequence, hydrophobicity, and polarity, CPPs can be divided into three categories: cationic peptides, antimicrobial sequences, and chimeric peptides.88,94
The transmembrane mechanism of CPPs is still not very clear. CPPs were considered a direct penetrating process without endocytosis or energy dependence in the initial research (1988–2003),95 however, these experimental results have been challenged recently. Further research conclusively showed that the main mechanism of CPP internalization is endocytosis.88,95 The endocytosis pathway mainly includes clathrin-mediated endocytosis, caveolin-mediated endocytosis, and macropinocytosis.96,97 Macropinocytosis is the main pathway of cellular internalization and can occur in all cells to varying degrees (Fig. 6).88 When the nanoparticles are connected to the CPPs, their transfer efficiency can be greatly improved. Lu et al. revealed that the CPP modified poly(lactic-co-glycolic acid) (PLGA) nanoparticles may deliver insulin into the brain through the nasal route, indicating a total brain delivery efficiency of 6%.98 Functionalization of magnetic nanoparticles with cell-penetrating peptides can promote efficient translocation of cargo into the cell. Chaudhary et al. demonstrated that penetration increased particle uptake and proved more cell specific, and clathrin-mediated endocytosis appeared to be responsible for the uptake.99
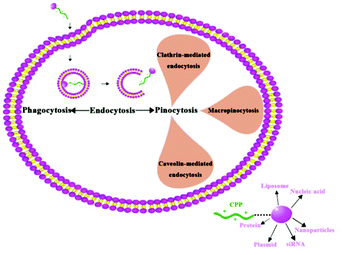 |
| Fig. 6 The mechanism for cell-penetrating peptide (CPP) internalization. | |
3.3. Bypass BBB via passive targeting
Targeted drug delivery is a selective way for drugs to reach a specific physiological site (organs, tissues or cells) and play a therapeutic role. Selective administration can enhance the drug activity in the target sites and reduce its toxic and side effects in the non-target sites to improve the drug therapeutic index. Drug targeting generally has two methods: active targeting and passive targeting. Active targeting is the use of biological specificity (such as antigen–antibody binding or ligand–receptor binding) to achieve targeted delivery of drugs, and the active targeting carrier has antibody, transferrin, etc. Passive targeting refers to the favorable accumulation of drugs in the diseased tissue because of distinct physiological conditions. For instance, liposomes, nanoparticles, and nanoemulsions can be selectively enriched in the liver and spleen by the reticuloendothelial system and they can also accumulate in tumor and inflammatory tissues via enhanced permeability and retention (EPR) effects after intravenous injection.100,101
The targeted drug delivery system has been heavily developed recently, especially for cancer drugs for brain tumors, including neuroglioma (astrocytoma, oligodendroglioma and ependymoma) and meningioma located outside the BBB, and metastatic lesions (mainly from lung cancer, breast cancer, malignant melanoma, renal carcinoma, colon cancer and other cancers). One of the most promising areas of nanoparticle-mediated brain delivery is the treatment of brain tumors, using the enhanced permeability and retention (EPR) effect. This effect occurs in many types of tumors that are characterized by defective hypervasculature and an incomplete lymphatic drainage system.100,102,103 The size of a nanoparticle is a fundamental characteristic that determines the passive targeting of drugs and biodistribution within brain tumors. Nanoparticles within a size range of 10–100 nm and having a hydrophilic surface can escape from phagocytosis of the reticuloendothelial system in vivo. They are able to circulate in the blood vessels for a longer time, thus increasing their chance of reaching the tumor tissues. The unique characteristics of tumor tissues also enable nanoparticles to be targeted selectively. The rapid proliferation of tumor cells requires oxygen and nutrients supplied by existing and new blood vessels, while the disordered growth of tumor cells creates a highly irregular generation of tumor angiogenesis. This leads to an increasing gap between tumor vascular endothelial cells and lymphatic vessels, called the EPR effect. The rapidly growing tumor cells show a high metabolic state and need far more oxygen and nutrients than the tumor itself can provide. Therefore, tumor cells produce additional energy by glycolysis, which can cause acidification around tumor cells. One of the important research directions of nano drug delivery systems is to develop nanoparticle loaded drugs based on the acid–base imbalance of the micro environment. Under normal neutral pH values, nanospheres are stable, but when they enter the acidic environment of the tumor tissue, the microspheres will accelerate the disintegration, and thus quickly release its internal loaded drugs to achieve a high drug concentration in the target location. Ruan et al. developed a shrinkable nanocarrier, G-AuNPs-DOX-PEG. In this platform, DOX and PEG chains were tethered to the surface of gold nanoparticles via Au–S bonds. The drug loaded gold nanoparticles were then coated with gelatin nanoparticles.104 The release of DOX from G-AuNPs-DOX-PEG occurred in a pH- and time-dependent manner. At pH 5.0, the release of DOX was more rapid than at a high pH value, with the release rate of DOX from G-AuNPs-DOX-PEG about 90.9%.
3.4. Receptor mediated endocytosis
Receptor mediated endocytosis is one of the mechanisms by which endogenous macromolecules travel across the BBB. Some macromolecular polypeptides (such as insulin and transferrin) in the blood cross the BBB mainly by the receptor mediated transport system. Endogenous substances such as ligands combine with specific receptors expressed on the BBB cavity surface to form corpuscles through endocytosis, then release the ligand by exocytosis and traverse the BBB to enter the brain tissue and play a biological role. Therefore, various corresponding ligands of receptors are designed and used as a carrier to deliver drugs into the brain via the receptor mediated transport pathway.
The transferrin receptor (TfR) is highly expressed in the brain capillaries; transferrin can help transport iron, its natural ligand, into the brain by the TfR mediated transport pathway. TfR is saturated for the endogenous TF, meaning that the TF containing drug molecules compete with natural ligands to combine with TfR. Peptidomimetic monoclonal antibody OX26 (to the transferrin receptor) was designed and interacts with the TfRepitopeat, a non-natural TF binding site, which avoids the competition of targeting TF loaded drugs with natural ligands, thus it can carry different drug molecules through the BBB into the brain. Intravenous OX26-BDNF (brain-derived neurotrophic factor) causes a 65–70% reduction in stroke volume in rats with a middle cerebral artery occlusion.105 Loperamide-loaded HSA (human serum albumin) nanoparticles with covalently bound transferrin or the OX26 or R17217 antibodies induced significant anti-nociceptive effects in the tail-flick test in ICR (CD-1) mice after intravenous injection, demonstrating that transferrin or these antibodies covalently coupled to HSA nanoparticles are able to transport loperamide and possibly other drugs across the BBB.106
Like TfR, the insulin receptor expressed on the surface of capillary endothelial cells in the brain transports insulin via a receptor mediated transport system. The half-life of insulin in the blood is only 10 min, though, and disturbance of the natural insulin balance can cause hypoglycemia. To address this, mouse monoclonal antibody 83-14 was designed.107–109 Carmustine (BCNU)-loaded solid lipid nanoparticles (SLNs) were grafted with 83-14 monoclonal antibody (MAb) (83-14 MAb/BCNU-SLNs) and applied to the brain-targeting delivery system.108 83-14 MAb/BCNU-SLNs are a promising antitumor drug delivery system for transporting BCNU to the brain.
Low density lipoprotein receptor-related protein 1 (LRP1) and LRP2 ligands are also used as carriers for drug delivery. The low density lipoprotein receptor family is highly expressed in BBB and may mediate a series of substances with the structure of corresponding ligands to stride across the BBB through endocytosis, such as lactoferrin, melanotransferrin, receptor associated protein, etc.110–113 At the same time, low density lipoproteins also exhibit high expression in glioma cells.114 Low density lipoprotein is also used as a target for a drug delivery system, which can kill tumor cells at the same time as the drug crosses BBB. Angiopep-2, a specific ligand of LRP1, can mediate the system to penetrate the BBB and target glioma cells.115 Angiopep-2 (ANG) was further conjugated onto the surface of the PF127-modified water-dispersible poly(acrylic acid)-bound iron oxide nanoparticles (ANG-PF127-PAAIO) for brain targeting.116 Results revealed that the ANG-PF127-PAAIO showed negligible cell cytotoxicity, better cellular uptake, and higher T2-weighted image enhancement than the PF127-PAAIO in U87 cells. In addition, ANG-PF127-PAAIO showed better permeability to bypass the BBB because of dual-targeting ability, recognition of the low density lipoprotein receptor-related protein and the clathrin-mediated receptor on the U87 surface.116 Scavenger receptor type-B1 (SR-B1) and opioid receptor could also improve delivery efficiency.117,118
3.5. Combinational strategies
To improve the targeting efficiency and cell internalization, combinational strategies are used in the nanoparticle systems. Two targeting ligands, angiopep-2 and cell penetrating peptide (CPP), were functionalized onto nanoparticles for tumor targeting delivery.92 Results showed that either CPP modification orangiopep-2 modification could enhance the U-87 MG cell uptake in vitro. More importantly, the constructed dual targeting nanoparticles (AnACNPs) showed higher uptake level than the single ligand modified nanoparticles. In vivo, AnACNPs showed more enhanced tumor targeting efficiency. The distribution of AnACNPs in the tumor was higher than the other particles tested.
4. Challenges and future directions
With the rapid development of nanotechnology, medical biological nanomaterials have made unprecedented progress. Nano drug carriers have shown great potential in the treatment of CNS diseases. So far, the U. S. Food and Drug Administration (FDA) has approved the listing of a variety of nano products, such as Emend, Rapamune, Abraxane, and Megace ES. More nano products are in the clinical evaluation stage, and nano controlled release drug delivery systems present a trend of accelerated development. The study of nanoparticle brain targeting drug delivery is still in the experimental stage, and its clinical research is less reported. Some possible roadblocks to this research include: (i) the understanding of the BBB material transport regulation mechanism is not sufficient; (ii) in vitro models and mathematical models to predict or evaluate drugs through the BBB still need to be improved; (iii) the preparation technology of nanoparticles is not mature, especially the industrial production and quality control; (iv) the storage stability of nanoparticle preparation, the safety when injected, and the effect of nanoparticles on the CNS after permeating the BBB need to be further studied; (v) the effect of nanoparticle properties (such as particle size, surface properties, drug and polymer materials, etc.) on brain targeting is not very clear and their toxic and side effects have been controversial. Future research goals should include: (i) to study the mechanism and influencing factors of nanoparticles on the brain and drug release in the brain; (ii) to strengthen the research on nanoparticle preparation technology and focus on solving the problems of low encapsulation efficiency, organic solvent and surfactant residues, and process stability; (iii) to try more types of drugs, more varieties of materials, and more diverse preparation processes. With the emergence of new materials, methods and technologies, the brain targeting nanoparticles will receive more and more attention. Nanoparticles will have a broader prospect in the treatment of brain targeted therapy, and become a powerful weapon to conquer brain diseases.
References
- M. D. Sweeney, A. P. Sagare and B. V. Zlokovic, J. Cereb. Blood Flow Metab., 2015, 35, 1055–1068 CrossRef CAS PubMed.
- B. Newland, H. Newland, C. Werner, A. Rosser and W. Wang, Prog. Polym. Sci., 2015, 44, 79–112 CrossRef CAS.
- A. Vilella, B. Ruozi, D. Belletti, F. Pederzoli, M. Galliani, V. Semeghini, F. Forni, M. Zoli, M. A. Vandelli and G. Tosi, Pharmaceutics, 2015, 7, 74–89 CrossRef PubMed.
- H. Gao, Z. Pang and X. Jiang, Pharm. Res., 2013, 30, 2485–2498 CrossRef CAS PubMed.
- U. H. Weidle, J. Niewoethner and G. Tiefenthaler, Cancer Genomics Proteomics, 2015, 12, 167–177 CAS.
- F. E. O'Brien, T. G. Dinan, B. T. Griffin and J. F. Cryan, Br. J. Pharmacol., 2012, 165, 289–312 CrossRef PubMed.
- M. Rajadhyaksha, T. Boyden, J. Liras, A. El-Kattan and J. Brodfuehrer, Curr. Drug Discovery Technol., 2011, 8, 87–101 CrossRef CAS.
- R. Gabathuler, Neurobiol. Dis., 2010, 37, 48–57 CrossRef CAS PubMed.
- Y. Wen, W. Liu, C. Bagia, S. Zhang, M. Bai, J. M. Janjic, N. Giannoukakis, E. S. Gawalt and W. S. Meng, Acta Biomater., 2014, 10, 4759–4767 CrossRef CAS PubMed.
- Y. Zheng, Y. Wen, A. M. George, A. M. Steinbach, B. E. Phillips, N. Giannoukakis, E. S. Gawalt and W. S. Meng, Biomaterials, 2011, 32, 249–257 CrossRef CAS PubMed.
- C. Engman, Y. Wen, W. S. Meng, R. Bottino, M. Trucco and N. Giannoukakis, Clin. Immunol., 2015, 160, 103–123 CrossRef CAS PubMed.
- Y. Wen and J. H. Collier, Curr. Opin. Immunol., 2015, 35, 73–79 CrossRef CAS PubMed.
- Y. Wen, H. R. Kolonich, K. M. Kruszewski, N. Giannoukakis, E. S. Gawalt and W. S. Meng, Mol. Pharm., 2013, 10, 1035–1044 CrossRef CAS PubMed.
- L. Gastaldi, L. Battaglia, E. Peira, D. Chirio, E. Muntoni, I. Solazzi, M. Gallarate and F. Dosio, Eur. J. Pharm. Biopharm., 2014, 87, 433–444 CrossRef CAS PubMed.
- E. Lueshen, I. Venugopal, T. Soni, A. Alaraj and A. Linninger, J. Biomed. Nanotechnol., 2015, 11, 253–261 CrossRef CAS PubMed.
- C. Fornaguera, A. Dols-Perez, G. Caldero, M. J. Garcia-Celma, J. Camarasa and C. Solans, J. Controlled Release, 2015, 211, 134–143 CrossRef CAS PubMed.
- J. Lalani, S. Patil, A. Kolate, R. Lalani and A. Misra, AAPS PharmSciTech, 2015, 16, 413–427 CrossRef CAS PubMed.
- G. C. Mello Santos, M. Tiago, S. S. Maria-Engler and T. d. J. Andreoli Pinto, Int. J. Polym. Mater. Polym. Biomater., 2015, 64, 695–707 CrossRef.
- T. Brunella, T. Giovanni, B. Barbara, D. Diego, M. Alessandro, D. M. Eleonora, U. Lorena, R. Barbara, F. Flavio, E. Carla, V. M. Angela and S. G. Maria, J. Nanosci. Nanotechnol., 2015, 15, 2657–2666 CrossRef.
- M. Masserini, ISRN Biochem., 2013, 2013, 238428 Search PubMed.
- J. Kreuter, Adv. Drug Delivery Rev., 2001, 47, 65–81 CrossRef CAS PubMed.
- A. Puri, K. Loomis, B. Smith, J.-H. Lee, A. Yavlovich, E. Heldman and R. Blumenthal, Crit. Rev. Ther. Drug Carrier Syst., 2009, 26, 523–580 CrossRef CAS PubMed.
- T. Ishii, T. Asai, D. Oyama, Y. Agato, N. Yasuda, T. Fukuta, K. Shimizu, T. Minamino and N. Oku, FASEB J., 2013, 27, 1362–1370 CrossRef CAS PubMed.
- V. K. Venishetty, R. Komuravelli, M. Kuncha, R. Sistla and P. V. Diwan, Nanomedicine, 2013, 9, 111–121 CrossRef CAS PubMed.
- A. Trapani, N. Denora, G. Iacobellis, J. Sitterberg, U. Bakowsky and T. Kissel, AAPS PharmSciTech, 2011, 12, 1302–1311 CrossRef CAS PubMed.
- P. Pradhan, W. Li and P. Kaur, J. Mol. Biol., 2009, 385, 831–842 CrossRef CAS PubMed.
- J. K. Saucier-Sawyer, Y. Deng, Y.-E. Seo, C. J. Cheng, J. Zhang, E. Quijano and W. M. Saltzman, J. Drug Targeting, 2015, 23, 736–749 CrossRef CAS PubMed.
- H. S. Mahajan, M. S. Mahajan, P. P. Nerkar and A. Agrawal, Drug Delivery, 2014, 21, 148–154 CrossRef CAS PubMed.
- M. E. Meredith, T. S. Salameh and W. A. Banks, AAPS J., 2015, 17, 780–787 CrossRef CAS PubMed.
- K. K. Pulicherla and M. K. Verma, AAPS PharmSciTech, 2015, 16, 223–233 CrossRef CAS PubMed.
- P. A. Stewart, Cell. Mol. Neurobiol., 2000, 20, 149–163 CrossRef CAS PubMed.
- N. J. Abbott, Cell. Mol. Neurobiol., 2005, 25, 5–23 CrossRef PubMed.
- I. Cooper, D. Last, D. Guez, S. Sharabi, S. E. Goldman, I. Lubitz, D. Daniels, S. Salomon, G. Tamar, T. Tamir, R. Mardor, M. Fridkin, Y. Shechter and Y. Mardor, J. Cereb. Blood Flow Metab., 2015, 35, 967–976 CrossRef CAS PubMed.
- M. Gynther, A. Petsalo, S. H. Hansen, L. Bunch and D. S. Pickering, Neurochem. Res., 2015, 40, 542–549 CrossRef CAS PubMed.
- S. R. Hwang and K. Kim, Arch. Pharmacal Res., 2014, 37, 24–30 CrossRef CAS PubMed.
- N. L. Klyachko, M. J. Haney, Y. Zhao, D. S. Manickam, V. Mahajan, P. Suresh, S. D. Hingtgen, R. L. Mosley, H. E. Gendelman, A. V. Kabanov and E. V. Batrakova, Nanomedicine, 2014, 9, 1403–1422 CrossRef CAS PubMed.
- P. Gupta, T. Garg, M. Tanmay and S. Arora, Crit. Rev. Ther. Drug Carrier Syst., 2015, 32, 247–275 CrossRef PubMed.
- N. H. On and D. W. Miller, Curr. Pharm. Des., 2014, 20, 1499–1509 CrossRef CAS PubMed.
- W. Li, M. Sharma and P. Kaur, J. Biol. Chem., 2014, 289, 12633–12646 CrossRef CAS PubMed.
- W. Li, D. K. Rao and P. Kaur, J. Biol. Chem., 2013, 288, 11854–11864 CrossRef CAS PubMed.
- W. Dietrich, O. Alonso and R. Busto, Stroke, 1993, 24, 111–116 CrossRef CAS PubMed.
- S. Hatashita and J. T. Hoff, Stroke, 1990, 21, 582–588 CrossRef CAS PubMed.
- L. Belayev, R. Busto, W. Zhao and M. D. Ginsberg, Brain Res., 1996, 739, 88–96 CrossRef CAS PubMed.
- N. Schmid-Brunclik, C. Buergi-Taboada, X. Antoniou, M. Gassmann and O. O. Ogunshola, Am. J. Physiol.: Regul., Integr. Comp. Physiol., 2008, 295, R864–R873 CrossRef CAS PubMed.
- N. G. Cevik, N. Orhan, C. U. Yilmaz, N. Arican, B. Ahishali, M. Kucuk, M. Kaya and A. S. Toklu, Brain Res., 2013, 1531, 113–121 CrossRef CAS PubMed.
- R. Wang, G.-J. Wang, X.-L. Wu, F. Zhou and Y.-N. Li, Chin. J. Nat. Med., 2013, 11, 30–37 CrossRef CAS.
- T. Hagg and M. Oudega, J. Neurotrauma, 2006, 23, 264–280 Search PubMed.
- M. A. Ajmone-Cat, E. Cacci and L. Minghetti, Curr. Pharm. Des., 2008, 14, 1435–1442 CrossRef CAS PubMed.
- D. A. Greenberg and K. L. Jin, Nature, 2005, 438, 954–959 CrossRef CAS PubMed.
- Z. Kokaia, G. Martino, M. Schwartz and O. Lindvall, Nat. Neurosci., 2012, 15, 1078–1087 CrossRef CAS PubMed.
- A. O'Donnell, A. Moollan, S. Baneham, M. Ozgul, R. M. Pabari, D. Cox, B. P. Kirby and Z. Ramtoola, J. Pharm. Pharmacol., 2015, 67, 525–536 CrossRef PubMed.
- T. B. Devkar, A. R. Tekade and K. R. Khandelwal, Colloids Surf., B, 2014, 122, 143–150 CrossRef CAS PubMed.
- C. V. Pardeshi and V. S. Belgamwar, Expert Opin. Drug Delivery, 2013, 10, 957–972 CrossRef CAS PubMed.
- F. N. Fonseca, A. H. Betti, F. C. Carvalho, M. P. D. Gremiao, F. A. Dimer, S. S. Guterres, M. L. Tebaldi, S. M. K. Rates and A. R. Pohlmann, J. Biomed. Nanotechnol., 2015, 11, 1472–1481 CrossRef CAS PubMed.
- L. Kozlovskaya, M. Abou-Kaoud and D. Stepensky, J. Controlled Release, 2014, 189, 133–140 CrossRef CAS PubMed.
- N. M. Veldhorst-Janssen, A. A. Fiddelers, P. H. van der Kuy, C. Neef and M. A. Marcus, Clin. Ther., 2009, 31, 2954–2987 CrossRef CAS PubMed.
- S. Md, G. Mustafa, S. Baboota and J. Ali, Drug Dev. Ind. Pharm., 2015, 1–13, DOI:10.3109/03639045.2015.1052081.
- A. Serralheiro, G. Alves, A. Fortuna and A. Falcao, Int. J. Pharmacol., 2015, 490, 39–46 CrossRef CAS PubMed.
- S. V. Dhuria, L. R. Hanson and W. H. Frey 2nd, J. Pharm. Sci., 2010, 99, 1654–1673 CAS.
- L. Kozlovskaya and D. Stepensky, J. Controlled Release, 2013, 171, 17–23 CrossRef CAS PubMed.
- S. Gizurarson, Curr. Drug Delivery, 2012, 9, 566–582 CrossRef CAS.
- J. A. Martin, A. S. Maris, M. Ehtesham and R. J. Singer, J. Visualized Exp., 2012, e50019, DOI:10.3791/50019.
- C. P. Foley, D. G. Rubin, A. Santillan, D. Sondhi, J. P. Dyke, Y. P. Gobin, R. G. Crystal and D. J. Ballon, J. Controlled Release, 2014, 196, 71–78 CrossRef CAS PubMed.
- J. A. Boockvar, A. J. Tsiouris, C. P. Hofstetter, I. Kovanlikaya, S. Fralin, K. Kesavabhotla, S. M. Seedial, S. C. Pannullo, T. H. Schwartz, P. Stieg, R. D. Zimmerman, J. Knopman, R. J. Scheff, P. Christos, S. Vallabhajosula and H. A. Riina, J. Neurosurg., 2011, 114, 624–632 CrossRef CAS PubMed.
- D. Fortin, C. Gendron, M. Boudrias and M.-P. Garant, Cancer, 2007, 109, 751–760 CrossRef CAS PubMed.
- P. Kozler, V. Riljak and J. Pokorny, Physiol. Res., 2013, 62, S75–S80 Search PubMed.
- P. Kozler and J. Pokorny, Neuroendocrinol. Lett., 2012, 33, 782–786 CAS.
- P. Kozler, V. Riljak, K. Jandova and J. Pokorny, Physiol. Res., 2014, 63, S529–S534 Search PubMed.
- H. Ngoc Trinh, C. Passirani, E. Allard-Vannier, L. Lemaire, J. Roux, E. Garcion, A. Vessieres and J.-P. Benoit, Int. J. Pharm., 2012, 423, 55–62 CrossRef PubMed.
- C. P. Foley, N. Nishimura, K. B. Neeves, C. B. Schaffer and W. L. Olbricht, Ann. Biomed. Eng., 2012, 40, 292–303 CrossRef PubMed.
- P.-Y. Chen, T. Ozawa, D. C. Drummond, A. Kalra, J. B. Fitzgerald, D. B. Kirpotin, K.-C. Wei, N. Butowski, M. D. Prados, M. S. Berger, J. R. Forsayeth, K. Bankiewicz and C. D. James, Neuro-Oncology, 2013, 15, 189–197 CrossRef CAS PubMed.
- E. Lueshen, M. LaRiviere, B. Yamini and A. Linninger, Comput. Chem. Eng., 2014, 71, 672–676 CrossRef CAS.
- S. Joshi, R. Singh-Moon, M. Wang, D. B. Chaudhuri, J. A. Ellis, J. N. Bruce, I. J. Bigio and R. M. Straubinger, J. Neuro-Oncol., 2014, 120, 489–497 CrossRef CAS PubMed.
- E.-J. Park, Y.-Z. Zhang, N. Vykhodtseva and N. McDannold, J. Controlled Release, 2012, 163, 277–284 CrossRef CAS PubMed.
- R. D. Alkins, P. M. Brodersen, R. N. S. Sodhi and K. Hynynen, Neuro-Oncology, 2013, 15, 1225–1235 CrossRef CAS PubMed.
- M. Aryal, N. Vykhodtseva, Y.-Z. Zhang, J. Park and N. McDannold, J. Controlled Release, 2013, 169, 103–111 CrossRef CAS PubMed.
-
S. N. Tabatabaei, S. Duchemin, H. Girouard and S. Martel and IEEE, 2012 IEEE International Conference on Robotics and Automation (ICRA), 2012, pp. 727–732.
- M. Shinkai, M. Yanase, M. Suzuki, H. Honda, T. Wakabayashi, J. Yoshida and T. Kobayashi, J. Magn. Magn. Mater., 1999, 194, 176–184 CrossRef CAS.
-
I. N. Weinberg, M. G. Urdaneta, P. Y. Stepanov, D. Beylin, A. Nacev, A. Sarwar, B. Shapiro, O. C. Rodriguez, C. Albanese, R. Probst and S. T. Fricke, in 2012 IEEE Nuclear Science Symposium and Medical Imaging Conference Record, ed. B. Yu, 2012, pp. 3732–3734 Search PubMed.
- H. Gupta, P. Paul, N. Kumar, S. Baxi and D. P. Das, J. Colloid Interface Sci., 2014, 430, 221–228 CrossRef CAS PubMed.
- Y. Kim, S.
D. Kong, L.-H. Chen, T. R. Pisanic, II, S. Jin and V. I. Shubayev, Nanomedicine: Nanotechnol., Biol., Med., 2013, 9, 1057–1066 CrossRef CAS PubMed.
- L. B. Thomsen, T. Linemann, K. M. Pondman, J. Lichota, K. S. Kim, R. J. Pieters, G. M. Visser and T. Moos, ACS Chem. Neurosci., 2013, 4, 1352–1360 CrossRef CAS PubMed.
- H. Piraux, J. Hai, T. Gaudisson, S. Ammar, F. Gazeau, J. M. E. H. Chahine and M. Hemadi, J. Appl. Phys., 2015, 117 Search PubMed.
- R. J. Diaz, P. Z. McVeigh, M. A. O'Reilly, K. Burrell, M. Bebenek, C. Smith, A. B. Etame, G. Zadeh, K. Hynynen, B. C. Wilson and J. T. Rutka, Nanomedicine: Nanotechnol., Biol., Med., 2014, 10, 1075–1087 CrossRef CAS PubMed.
- T. Lammers, P. Koczera, S. Fokong, F. Gremse, J. Ehling, M. Vogt, A. Pich, G. Storm, M. van Zandvoort and F. Kiessling, Adv. Funct. Mater., 2015, 25, 36–43 CrossRef CAS PubMed.
- H. Hsin-Yang, L. Hao-Li, H. Po-Hung, C. Chih-Sheng, T. Chih-Hung, C. Huei-Shang, C. San-Yuan and C. You-Yin, Adv. Mater., 2015, 27, 655–661 CrossRef PubMed.
- Z. Sun, M. Worden, Y. Wroczynskyj, V. Yathindranath, J. van Lierop, T. Hegmann and D. W. Miller, Int. J. Nanomed., 2014, 9, 3013–3026 CrossRef PubMed.
- C. Bechara and S. Sagan, FEBS Lett., 2013, 587, 1693–1702 CrossRef CAS PubMed.
- E. A. Eugenin, J. E. Clements, M. C. Zink and J. W. Berman, J. Neurosci., 2011, 31, 9456–9465 CrossRef CAS PubMed.
- C. Zheng, C. Ma, E. Bai, K. Yang and R. Xu, Int. J. Clin. Exp. Med., 2015, 8, 1658–1668 CAS.
- H. Liu, W. Zhang, L. Ma, L. Fan, F. Gao, J. Ni and R. Wang, Int. J. Pharm., 2014, 476, 1–8 CrossRef CAS PubMed.
- L. Mei, Q. Zhang, Y. Yang, Q. He and H. Gao, Int. J. Pharm., 2014, 474, 95–102 CrossRef CAS PubMed.
- G. Sharma, A. Modgil, T. Zhong, C. Sun and J. Singh, Pharm. Res., 2014, 31, 1194–1209 CrossRef CAS PubMed.
- F. Milletti, Drug Discovery Today, 2012, 17, 850–860 CrossRef CAS PubMed.
- F. Madani, S. Lindberg, U. Langel, S. Futaki and A. Graslund, J. Biophys., 2011, 2011, 414720–414729 Search PubMed.
- S. D. Conner and S. L. Schmid, Nature, 2003, 422, 37–44 CrossRef CAS PubMed.
- M. Rizzuti, M. Nizzardo, C. Zanetta, A. Ramirez and S. Corti, Drug Discovery Today, 2015, 20, 76–85 CrossRef CAS PubMed.
- Y. Lu, W. Huiyuan, J. Yifan, L. Jinhua, W. Zhao, Y. Yongxin, H. Shengwu and H. Yongzhuo, Macromol. Res., 2013, 21, 435–441 CrossRef.
- S. Chaudhary, C. A. Smith, P. Del Pino, J. M. de la Fuente, M. Mullin, A. Hursthouse, D. Stirling and C. C. Berry, Pharmaceuticals, 2013, 6, 204–222 CrossRef CAS PubMed.
- Y. Wen and W. S. Meng, J. Pharm. Innov., 2014, 9, 158–173 CrossRef PubMed.
- A. Balducci, Y. Wen, Y. Zhang, B. M. Helfer, T. K. Hitchens, W. S. Meng, A. K. Wesa and J. M. Janjic, OncoImmunology, 2013, 2, e23034 CrossRef PubMed.
- H. Maeda, Bioconjugate Chem., 2010, 21, 797–802 CrossRef CAS PubMed.
- J. Kreuter, Adv. Drug Delivery Rev., 2014, 71, 2–14 CrossRef CAS PubMed.
- S. Ruan, X. Cao, X. Cun, G. Hu, Y. Zhou, Y. Zhang, L. Lu, Q. He and H. Gao, Biomaterials, 2015, 60, 100–110 CrossRef CAS PubMed.
- Y. Zhang and W. M. Pardridge, Brain Res., 2006, 1111, 227–229 CrossRef CAS PubMed.
- K. Ulbrich, T. Hekmatara, E. Herbert and J. Kreuter, Eur. J. Pharm. Biopharm., 2009, 71, 251–256 CrossRef CAS PubMed.
- Y.-C. Kuo and H.-F. Ko, Biomaterials, 2013, 34, 4818–4830 CrossRef CAS PubMed.
- Y.-C. Kuo and C.-Y. Shih-Huang, J. Drug Targeting, 2013, 21, 730–738 CrossRef CAS PubMed.
- M. J. Coloma, H. J. Lee, A. Kurihara, E. M. Landaw, R. J. Boado, S. L. Morrison and W. M. Pardridge, Pharm. Res., 2000, 17, 266–274 CrossRef CAS.
- R. D. Bell, A. P. Sagare, A. E. Friedman, G. S. Bedi, D. M. Holtzman, R. Deane and B. V. Zlokovic, J. Cereb. Blood Flow Metab., 2007, 27, 909–918 CAS.
- L. Y. Lim, P. Y. Koh, S. Somani, M. Al Robaian, R. Karim, Y. L. Yean, J. Mitchell, R. J. Tate, R. Edrada-Ebel, D. R. Blatchford, M. Mullin and C. Dufes, Nanomedicine: Nanotechnol., Biol., Med., 2015, 11, 1445–1454 CrossRef CAS PubMed.
- M. Nounou, C. Adkins, T. R. Terrell, A. Mohamed, T. Vitalis, R. Gabathuler and P. R. Lockman, Cancer Res., 2014, 74 Search PubMed.
- J. M. Prasad, M. Migliorini, R. Galisteo and D. K. Strickland, J. Biol. Chem., 2015, 290, 17262–17268 CrossRef CAS PubMed.
- L. Maletinska, E. A. Blakely, K. A. Bjornstad, D. F. Deen, L. J. Knoff and T. M. Forte, Cancer Res., 2000, 60, 2300–2303 CAS.
- S. Ruan, M. Yuan, L. Zhang, G. Hu, J. Chen, X. Cun, Q. Zhang, Y. Yang, Q. He and H. Gao, Biomaterials, 2015, 37, 425–435 CrossRef CAS PubMed.
- C. Guo-Jing, S. Ying-Zhen, H. Chin, L. Yu-Lun, H. Shih-Jer, K. Jyun-Han, K. Yung-Chih and W. Li-Fang, J. Mater. Chem. B, 2014, 2, 5666–5675 RSC.
- M. M. Farid, R. M. Hathout, M. Fawzy and K. Abou-Aisha, Colloids Surf., B, 2014, 123, 930–937 CrossRef CAS PubMed.
- S. Gelperina, O. Maksimenko, A. Khalansky, L. Vanchugova, E. Shipulo, K. Abbasova, R. Berdiev, S. Wohlfart, N. Chepurnova and J. Kreuter, Eur. J. Pharm. Biopharm., 2010, 74, 157–163 CrossRef CAS PubMed.
|
This journal is © The Royal Society of Chemistry 2016 |
Click here to see how this site uses Cookies. View our privacy policy here.