DOI:
10.1039/D4EN00488D
(Critical Review)
Environ. Sci.: Nano, 2024,
11, 4421-4448
Understanding the role of biomolecular coronas in human exposure to nanomaterials
Received
30th May 2024
, Accepted 17th August 2024
First published on 9th September 2024
Abstract
Nanomaterials (NMs) are increasingly used in medical treatments, electronics, and food additives. However, nanosafety—the possible adverse effects of NMs on human health—is an area of active research. This review provides an overview of the influence of biomolecular coronas on NM transformation following various exposure routes. We discuss potential exposure pathways, including inhalation and ingestion, describing the physiology of exposure routes and emphasising the relevance of coronas in these environments. Additionally, we review other routes to NM exposure, such as synovial fluid, blood (translocation and injection), dermal and ocular exposure, as well as the dose and medium impact on NM interactions. We emphasize the need for an in-depth characterisation of coronas in different biological media, highlighting the need and opportunity to study lung and gastric fluids to understand NM behaviour and potential toxicity. Future research aims to predict better in vivo outcomes and address the complexities of NM interactions with biological systems.
Environmental significance
This critical review reports on the environmental implications that the human route of exposure have on nanomaterials (NMs) and highlights the important role of biomolecular coronas in shaping NM behaviour. As NPs are increasingly applied in many fields, including medicine, construction, and food, the concerns about their nanosafety are increasing. In this work, we review biomolecular coronas in diverse biological fluids, taking into account the exposure routes to NPs in order to introduce more detail. Recognizing inhalation and ingestion as primary exposure routes, we emphasize the need for an in-depth characterisation of coronas in different biological environments to achieve a thorough understanding of NM toxicity. This work contributes substantively to the field of environmental science by remarking on the interplay between nanoscale materials' behaviour and the role of biomolecular coronas to understand the impact on human health.
|
Introduction
During the last few decades, engineered nanoparticles (NPs), i.e., materials smaller than 100 nm in at least one dimension, have received considerable attention because of their unique properties, such as their chemical reactivity, electrical conductivity, fluorescence, and magnetism.1–5 Owing to the technological advancements in the last years, they have rapidly been incorporated in a wide range of products to improve the properties of the final products. The medical sector is the fastest growing NP market6 where NPs have found application in both the diagnosis and therapy of several diseases.7 Electronics is a big market, and technology is evolving at high speed owing to the properties of metals at the nanoscale.8 Meanwhile, painting, coating, and construction industries have recently been driven by the introduction of nanomaterials (NMs) into existing products. This results in more hydrophobic or antimicrobial surfaces9 or more durable and stronger concrete due to the addition of nanosilica and carbon nanofibers.10 The global NM market size was valued at $11B in 2022 and is expected to grow by ∼15% annually in the next decade, eventually surpassing the $50B market size.6,11,12
Because of their increasing use, human exposure to NMs has become a matter of concern as workers or end users could be indirectly exposed to them with unpredictable outcomes. Moreover, exposure to humans occurs following NM release into the environment during the life cycle of products.13 During the last few years, safe and sustainable by design (SSbD) strategies have been developed to modify products and processes involving advanced NMs to reduce their potential for release or hazard potency without changing their technological properties.14,15 In the same direction, a wide range of multicomponent NMs have been recently developed. These advanced hybrid materials are formed by two or more functional components such as organic molecules conjugated by molecular bonds to NPs or by a NM modified by hard or soft coatings.16,17
Unintentional NM exposure generally occurs via inhalation (during the manufacturing process or release), by ingestion, or even by dermal or ocular exposure, while intramuscular or intravenous injection is more common for intentional exposure to bio–nano materials with therapeutic effect (Fig. 1).18 Moreover, the mechanisms associated with the bio-physicochemical interactions of NMs in humans are complex. In particular, the initial interactions between NMs and biological fluids are difficult to accurately model.19 When dispersed in aqueous solutions containing electrolytes, NMs first interact with the ions present in the media, forming a thick solvation sheath that gives their hydrodynamic appearance. Likewise, in biological media, they are quickly and spontaneously covered by biomolecules, forming what is called biomolecular corona. While this paradigm initially attracted attention from the nanotoxicity context, it is now widely studied in the field of nanomedicine and environmental science.20 Additionally, initial corona studies mostly focused on identifying the protein component. However, recent studies have revealed that the corona is also made of other metabolites,21,22 such as lipids23 and glycans,24,25 and the concept is often referred to as the biomolecular corona.19,26–28 The interaction between biomolecules and NMs is governed by many factors, including particle size, shape, surface chemistry, hydrophobicity and surface charge.29–31 Additionally, affinity-based interactions of proteins towards the NM surface and protein-to-protein interactions are responsible for changes in the corona composition over time.32 Accordingly, two stages define the formation mechanism of the biomolecular corona on a NP. Firstly, engineered NPs enter the biological medium and come into contact with biomolecules, which adsorb onto the NPs' surface within seconds, forming the initial corona.33 Secondly, competition between proteins takes place, evolving the corona composition over time, until a stationary or slowly-evolving state is reached.33 The biomolecular corona is usually divided into hard and soft corona, depending on factors such as the binding strength and rate of exchange of biomolecules from the surface of the NP. The hard corona (HC) is typically made of proteins directly adsorbed onto the NP's surface, which therefore exhibit long/constant exchange time with the surrounding molecules in the medium. On the contrary, soft corona (SC) proteins are known to be loosely attached to the NP surface, or most likely attached to an already formed hard corona via weak protein–protein interactions. Between them, the intermediate (or displacement) corona (IC) has been recently described as the transition layer made of biomolecules with moderate binding affinities and exchange rate with the NP surface compared to the HC and SC.34 Consequently, the proteins of the HC are arranged in the inner layer of the corona, whereas the SC proteins are distributed in the upper layer of the corona. This makes them more dynamic and easily exchangeable over time with other biomolecules in the surrounding medium (Fig. 2).35,36 This is the paradigm for inorganic NMs, which typically form robust coronas due to higher surface energy and charge density.37 Recent studies have reported that the biomolecular corona is also formed in organic NMs and exosomes,38–40 which in turn affects the biological fate. It is expected that the corona will have a different half-life and exchange on organic and inorganic NPs, mainly due to the difference in binding interactions. In fact, the presence of hydrophobic or hydrophilic groups dictates the type and amount of proteins that bind. Hydrophobic surfaces tend to bind more proteins, as has been observed in the case of both organic and inorganic NMs.41 Moreover, the addition of surface functional groups, ligands or linkers – especially those providing steric hindrance or specific chemical functionalities – can tailor protein binding, usually reducing the amount of biomolecules adsorbed and influencing the overall composition and behavior of the corona.42–44 Charge also plays a crucial role, with positively charged NPs generally attracting more proteins due to strong electrostatic interactions.41 Therefore, more comparative research is needed to take all these factors into consideration and closely study the potential differences in the formation of the biomolecular corona between inorganic and organic NMs.
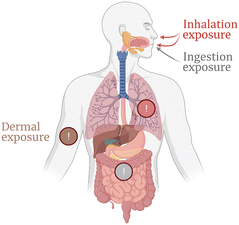 |
| Fig. 1 Common routes of exposure to NMs. Schematic view of the human body with pathways of exposure to NMs, and the organs through which NMs can translocate to the bloodstream. | |
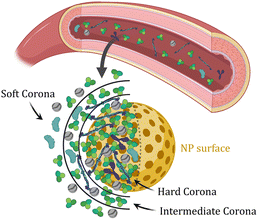 |
| Fig. 2 Biomolecular corona structure on top of NPs. Representative picture of corona formation onto a mesoporous silica NP when exposed to biological fluids such as blood plasma. Different proteins may adsorb to the surface of the NP, depending on several factors such as NP surface chemistry and buffer pH. | |
The interaction between NMs and biomolecules can alter their properties and thus influence protein function, but also the NMs' fate in vitro and in vivo. For instance, binding proteins to NPs may induce structural changes,45 potentially exposing new epitopes. Epitope mapping has been a valuable approach to investigating these newly exposed epitopes.46,47 Research in the field has provided insights into the protein structural alterations caused by the adsorption to NPs,48,49 such as the NP-induced unfolding of fibrinogen promoting Mac-1 receptor activation and inflammation.50 In some occasions, this protein structural change has been reported to be as big as losing its enzymatic activity, disturbing biological processes, and even accelerating pathogenic events.51 On the other hand, the adsorption of proteins onto the NP surface can alter the colloidal characteristics of NPs, including their aggregation characteristics and/or hydrodynamic diameter, which may impact the cellular response to the NPs exposure, leading to accumulation, toxicity, and clearance.52
The colloidal characteristics of NMs are significantly influenced by the type of biological fluid, its composition, and the concentrations of biomolecules.53 The formation of a biomolecular corona on the surface of NPs plays a critical role in modulating their interactions with biological systems.35 In fact, this corona can attenuate the surface energy of the NPs, potentially reducing their toxicity. For example, studies have shown that pristine NPs may disrupt cellular membranes and induce necrosis, whereas NPs coated with a biomolecular corona do not exhibit this effect.54,55 Additionally, the corona can delay the dissolution of NMs, further mitigating their toxic impact.55,56 Therefore, the risk assessment of such NPs must evaluate their colloidal properties in various biological fluids by exploring different routes of exposure to NMs. Common routes of exposure include inhalation (lungs) or ingestion (gut), and each could potentially lead to different biodistribution and translocation into different organs, resulting in different outcomes.57 For instance, inhaled NMs can be deposited deep into the lungs where they could induce oxidative stress and promote inflammation,58,59 or travel into the bloodstream and accumulate at inflamed vascular sites.60 A potential link between diesel exhaust NMs and cardiovascular damage has been recently reported, based on the induction of oxidative stress via the NRF-2 pathway.61,62 Moreover, particles suspended in the air are also capable of adsorbing some compounds. One example is bacterial lipopolysaccharide (LPS), a strong pro-inflammatory agent commonly found as an environmental contaminant in some parts of the body like the gut. It was observed that the co-incubation of LPS with TiO2 NPs significantly increases its pro-inflammatory impact on mouse macrophages, suggesting that LPS's activity is amplified when forming part of a NP bio-corona.63 The concept of an ecological corona or eco-corona arises in this context to understand the nanoparticle interactions within biological and environmental systems.64 In contrast to the biomolecular corona, the eco-corona forms in environmental settings, like water, soil, or air, and is composed of natural organic matter, extracellular polymeric substances, and humic substances. This pre-formed eco-corona also influences the nanoparticles' behaviour, bioavailability, and potential effects on human health and ecosystems.65 For instance, proteins released by Daphnia magna create an eco-corona around polystyrene NPs, which causes heightened uptake of the NPs and increases toxicity.66 Recent studies have emphasised the significance of eco-corona formation in determining the fate and ecotoxicity of NMs in aquatic environments.67,68 Understanding the eco-corona is therefore important to assess the environmental and health risks associated with NP exposure, as well as the associated molecular mechanisms.69 Other examples contributing to this “exposome”70 include the release of NMs from medically implanted devices such as the leaching of NMs from hip implants or dental composites, which may have a long-term toxic impact on patients.71 Thus, for a proper assessment of the engineered NP hazard, it is necessary to focus on all body systems that are potential targets for NMs.
In this context, this review summarizes the latest results on the specific mechanisms of NP interaction with different biological systems. The biological identity of the NMs depends on their surrounding environment. Therefore, the main routes of human exposure are thoroughly explained throughout the text. Moreover, the subsequent implications of embedding different NMs in these environments are reviewed in detail, highlighting the impact of the exposure route on the biomolecular corona, as well as the recent advancements and challenges in nanosafety.
NM exposure through inhalation
Physiology of the exposure route
The respiratory system, which is the main route of unintentional exposure to NMs, encompasses various parts, including the nasal cavity, pharynx, larynx, trachea, bronchi, bronchioles, and alveoli.72 As shown in Fig. 3, the conduction airways of the lung (bronchus and bronchiole) and the epithelium tissues are protected by a viscous layer of mucus (mainly composed of glycoproteins), which makes them a relatively efficient barrier where particles and microbes are retained by the mucus and transported back to the oral cavity by the cilia located on the lung epithelial cells. The alveoli are responsible for the exchange of oxygen and carbon dioxide in the blood, and they have a simple squamous epithelium tissue with large surface area and intense air–blood contact compared to conduction airways.73 In fact, studies that utilised airway casting – a method for creating detailed 3D models of lung structures – reported an approximate alveolar surface area of 8900 m2, which is significantly higher than the 1.78 m2 reported for the nasopharyngeal regions.74 This makes the alveoli more exposed to airborne pathogens and pollutants, a common target of particles and one of the most permeable barriers to blood circulation. For NMs to come into contact with epithelium tissues, they need to either penetrate the mucus barrier or move down in the direction of the inhaled air to the alveolar region, and encounter the last thin liquid amphipathic film composed of surfactants.75 The alveolar epithelium is structurally simple to ensure a high surface area for oxygen/carbon dioxide exchange into/from the circulatory system. The surface tension at the air/liquid interface is controlled to prevent the collapse of the alveoli, and it is maintained by the presence of biomolecules, such as surfactants, proteins, and phospholipids. The lung lining fluid also exerts functions in protection against pathogens' translocation into the bloodstream.76
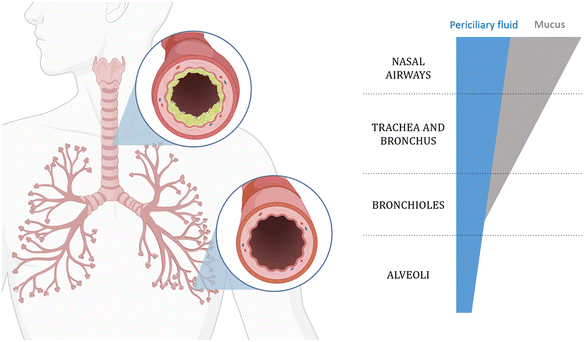 |
| Fig. 3 Different parts of the respiratory system and their fluid composition. As represented, the composition of the different components changes along the respiratory airways. On the right, a schematic of the component changes occurring in the LLF that are present in different regions of the respiratory tract, from the nasal airways to the alveoli. In the upper airways, the LLF is composed of two layers: a periciliary watery sol layer covered by a viscous mucous layer, which decreases its presence until disappearing in the bronchoalveolar region. | |
Lung fluids have been extensively simulated to reproduce the characteristics of biological lung fluids. A wide range of artificial respiratory tract lining fluids (RTLFs), also known as lung lining fluids (LLFs), have been developed to emulate the biological fluid that overlays the underlying epithelial cells.77 The lungs comprise different cell types, such as epithelial, endothelial, and inflammatory, all having an impact on the composition of the LLFs. The components they secrete lead to significantly varying compositions along all of the respiratory airways. In the upper airways, it is particularly enriched in mucus, which is made of lipids, glycoproteins, and salts, and is the primary barrier for the clearance of bacteria. However, it was also shown to be effective in the retention of inhaled NMs.78 Moving from the nasal cavity to the inner parts of the lung, the diameter of the airways decreases and the mucus layer thickness decreases, as shown in Fig. 3. When the alveolar region is reached, no mucus is present anymore and it is replaced by surfactants secreted from type II pneumocytes, which lower the surface tension and prevent alveolar collapse.78
However, the complexity and heterogeneity along the respiratory tract of the lung present a major challenge. To obtain lung fluids, the usual procedure is to perform a bronchoalveolar lavage (BAL), first used to treat several airway diseases by pumping out the LLFs. This medical procedure involves the introduction of saline fluid into the lung airways, followed by the collection of this same fluid, along with any biomolecules present in the airways using suction.79 This practice, despite being invasive, continues to be a common clinical diagnosis tool that is able to monitor diverse airway disorders. However, its main drawback is the loss of the regional composition of the different parts of the lung while pumping out the lung fluids, as well as the alteration of the salt concentration, as most BAL procedures consist of the previous introduction of large volume aliquots of saline solution.80,81 These drawbacks lead to the complexity of characterising the biological fluid, as there is still not enough data and techniques able to track how this change in the concentration and composition of substances occurs along the respiratory system.82
Several studies have been focused on understanding the NM behaviour after inhalation, and the development of simulant LLF has become largely studied. The addition of various components to mimic the LLF holds critical significance in physiological contexts. Salts play a pivotal role by ensuring proper protein folding, crucial for maintaining biological functions. In this context, buffers, such as phosphate-buffered saline (PBS), are essential to keep the correct pH and buffering capacity, creating a physiologically stable environment for protein stability and cellular processes.83 Proteins in the LLF have a dual role, as they not only contribute to the protective layer but also participate in immune responses, safeguarding against pathogens.84,85 Additionally, lipids emerge as fundamental constituents, particularly as pulmonary surfactants, where they are vital for lowering surface tension, preventing alveolar collapse at the air–liquid interface, and enabling efficient gas exchange.86 In regards to simulating the composition of the LLF, two of the most established protocols were developed decades ago: Gamble's and Hatch's solutions. Gamble's solution was first developed with compositions similar to the extracellular fluid in the skeletal muscle.87 The basic components are cations (magnesium, sodium, calcium, and potassium) and anions (proteins, bicarbonate, sulphate, organic acids, chloride, and phosphate), as well as some non-electrolytes such as glucose, reaching a pH of approximately 7.4.88 Lately, the original Gamble's solution has been modified to add proteins, amino acids and phospholipids constituting lung surfactants, such as dipalmitoylphosphatidylcholine (DPPC).89–91 The other widely established method to simulate the LLF is Hatch's solution. In this protocol, the composition of the mucous layer of the respiratory tract is considered, and the simulated lung fluid contains a high number of proteins, enzymes, lung surfactants, and complex organic molecules.92 Recently, Kumar et al. have also included lipids when building a biocompatible synthetic lung fluid based on human LLF composition.93
Although these simulated fluids do not have the protein complexity of their biological counterpart, as long as the main BAL corona biomolecules are present in the LLF corona, the approach will be appropriate to evaluate and study the impact of lung fluids on the corona composition of inhaled NPs and their possible translocation in blood.94 Another approach to recreate the LLF complexity is using the secreted media from an in vitro 3D cell culture of human lung epithelial cells as a representative fluid.95 After the particles have been inhaled into the lungs, the resulting corona is mainly formed by phospholipids and proteins and is fundamental to reproducing the biological fluid impact on the NM.96 It has been recently reported to allow the particles to be phagocytised and expelled from the lung, but might also be used as a target for anticancer drugs to increase their antiproliferative effect.97,98
Bio–nano interactions
Inhalation of NMs via the respiratory system represents an important route of human exposure. In addition to the major risk of exposure in occupational settings, anthropogenic atmospheric pollution coming from vehicle exhausts, industrial emissions, ore extraction, and energy production has widened the risk exposure to the greater population.99 In some cases, exposure to NMs can be intentional for medical purposes, such as with colloidal silver sprays, which have been recently used to treat conditions like chronic rhinosinusitis.100 Depending on the NM characteristics, the particles can become deposited into the different structures of the human lungs if they are not cleared from the upper airways. This can potentially lead to chronic inflammation, epithelial injury, and further pulmonary diseases, especially fibrosis.101 The particle deposition in the lung may occur through five different mechanisms: sedimentation (gravity), inertial impaction, interception (particle–surface contact), electrostatic deposition, and diffusion.102 Many factors can affect the deposition of NMs, including properties such as size, surface charge or hydrophilicity, aggregation state after the interaction with the LLF, concentration, and surface reactivity, as well as physiological characteristics of the respiratory system, such as air volume, breathing frequency, and flow rate (Fig. 4).103–105 Soliman et al. investigated the effects of exposing iron oxide NPs (IONPs) to SmallAir-HF™ cultures and MucilAir-HF™ cultures. These cultures represent models of two different anatomical sites: large bronchial airways for MucilAir-HF™ and small airways for SmallAir-HF™. During the incubation time, particle aggregation was observed. This is most likely because they became trapped in the viscous mucus.95
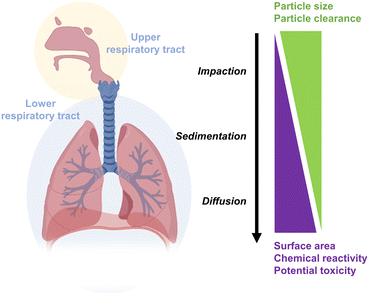 |
| Fig. 4 The interaction of particles with the human respiratory tract. At the left, a scheme of the respiratory system with the upper and lower respiratory tract. At the right, the different interactions between the particles and the respiratory tract, depending on the particle characteristics. | |
Several studies also assessed the effects of inhaled NPs' properties in vitro (using either diffusion chambers or more advanced devices in static or dynamic exposure) and in vivo (through whole-body exposure, nose/head-only exposure, or lung-only exposure).106 For instance, it was reported that the neutral small particles can penetrate the mucus layer better than charged large particles, which will most likely be trapped and removed by the cilia.107,108 The in vitro effects of silica NPs (SiO2 NPs) with different sizes (10, 150, and 500 nm at concentrations of 50, 100, and 100 μg mL−1, respectively) were investigated in human lung submucosal cells (Calu-3). Results showed that 10 nm SiO2 NPs induced ROS production, lipid peroxidation, and loss of viability, but no toxic effect was detected in the case of 150 and 500 nm. They also observed limited bioavailability of both fisetin and catalase to prevent toxicity as a result of biomolecular corona formation.109 Another factor that can enhance the particles' toxicity is the adsorption of surfactants on the NPs' surface, as reported by Kasper et al. This in vitro study investigated the effect of different surface charges of amorphous SiO2 NPs (unmodified, negatively or positively charged modified) on lung toxicity in the presence and absence of lung surfactants – i.e., the phospholipid fraction from bovine lung extracts, which is used for the treatment of respiratory distress syndrome (RDS) in neonates.110 The alveolar epithelial cell line A549 was used in both mono- and co-culture with the microvascular cell line ISO-HAS-1. As a result, high toxicity was detected in the presence of surfactants with unmodified or positively charged NPs. The increased cytotoxicity is believed to result from the formation of the reactive silanol groups (Si–O–H) in the presence of surfactants. In other words, the biomolecular corona formed in surfactant-containing media becomes less biocompatible, with its composition varying based on the surface properties of the NPs.
Investigation of the lipid and protein corona composition after exposure to pulmonary surfactants from porcine lung lavage was reported by Raesch et al.23 The study was performed for three different NP coatings: phosphatidylcholine coating (lipid-NP), PEG5000 coating (PEG-NP), and poly(lactic-co-glycolic acid) coating (PLGA-NP). It was found that the amount of lipids adsorbed was dependent on the NPs' surface properties. In particular, the highest amount of lipids was detected for lipid-NPs, while the smallest amount was detected for PEG-NPs. However, the total lipid composition did not show any relevant differences in both coronas, in contrast to the changes observed in the protein composition. These results indicate that the corona might be of great importance for further interactions with the lung cells, especially in the alveolar region, since some proteins induce agglomeration of NPs, which in turn favours phagocytosis by macrophages and/or protein-mediated binding for particle internalisation. Gasser et al. also used porcine lung lavage to understand how the presence of pulmonary surfactant influences the adsorption of biomolecules to multi-walled carbon nanotubes (MWCNTs) and MWCNTs functionalised with positively (–NH2) and negatively (–COOH) charged side groups.111 They showed that surfactant lipids exhibit nonspecific binding to different functionalized MWCNTs. This behavior contrasts with the selective and characteristic binding patterns observed in the blood plasma proteins during corona formation. In later research, they used the same particles to demonstrate that the pulmonary surfactant coating of the nanotubes decreases their oxidative and pro-inflammatory potential, highlighting the relevance of the corona. Moreover, this surfactant coating not only affects how NMs interact with lung cells, but also affects their biodistribution and toxicity in secondary target organs.112In vivo studies also support the latter findings. For instance, it was reported that large NPs (>5 μm) can be trapped in the upper airway, while small NPs of 1–5 μm can enter the lower airway and reach the alveoli, within the deepest region of the lungs.106,113,114 Surface activity/treatment of titanium dioxide (TiO2) NPs by alumina or amorphous silica showed mild toxicity in vivo (lung inhalation) compared to reference NPs (without surface treatment).115 Similar results were obtained in a case of whole-body inhalation by TiO2 NPs in terms of their moderate toxicity.116 Regardless of the exposure mechanism, the toxicity of inhaled particles could be attributed to the formation of a NP corona in LLF and their accumulation/retention in the lungs for a long time. Reasons for the prolonged retention include deep penetration into the mucus and a slow clearance mechanism, prolonging interaction with the lung cells. However, the retention time could differ depending on the particle size, as reported by Kreyling et al.117 It was found that gold NPs (AuNPs) with a core diameter of either 5, 18, 80, or 200 nm had longer retention times than the NPs with core sizes of 1.4 and 2.8 nm. This short retention time for the two smallest particles is because they can cross the air–blood barrier more easily. Other studies have indicated that the translocation of NPs from the lungs to extrapulmonary compartments is also governed by their size.118,119 For instance, organic and inorganic NPs with a hydrodynamic size of ≥34 nm demonstrated rapid translocation from the lungs to the mediastinal lymph node, regardless of their chemical composition, shape, or conformational flexibility. In contrast, for NPs smaller than this threshold (34 nm), surface chemistry becomes a critical factor in facilitating their rapid translocation from the lungs to regional lymph nodes. This is probably due to the formation of different coronas.119 In the case of colloidal silver nasal sprays, despite having no reported adverse health effects on humans,120 recent studies have pointed out that the administration of silver nasal spray leads to NP accumulation in rat brain tissues.121
From a toxicological point of view, both positively and negatively charged small NPs (<34 nm) have the potential to translocate into the bloodstream and thereby have a direct impact on cells and molecules in the circulatory system, as well as reaching other organs.119 To our knowledge, no study has been reported to evaluate the specific effect of lung mucus on the physico-chemical properties of NPs following exposure to it, which is definitely of interest. Additionally, inhaled NPs have the potential to be adsorbed through the olfactory epithelium, transported alongside the olfactory bulb, and reach the brain. For instance, following exposure to Al2O3 NPs at a concentration of 0.5 mg m−3 for 28 consecutive days, levels were found to be significantly increased in the cortex of mice.122 A study by Oberdörster et al. reported the direct transfer of radiolabelled nanoparticulate carbon from the nose of rats into the brain.118 Following transportation, NP deposition and interaction with brain tissue can take place.
The interaction between NMs and proteins might affect their physiological functions through conformational changes, as well as through either inhibiting or enhancing enzymatic activities. Shim and colleagues analysed the interactions between NPs and proteins from brain homogenates —a mixture created by thoroughly blending brain tissue. They later identified the adsorbed biomolecular corona from this solution. Two different sizes (20 and 100 nm) of SiO2 NPs with two different surface charges (positive and negative) were used and incubated with brain homogenate collected from adult rats. The mixture was incubated for 1 h at 37 °C. As a result, it was found that a greater number of proteins (>140) were bound onto the positively charged 20 nm SiO2 NPs than onto their negative counterparts in brain homogenate, while almost the same number of proteins were adsorbed to the 100 nm NPs regardless of their surface charge. This could be attributed to a larger surface area/volume ratio of the 20 nm NPs compared to the 100 nm ones, which allowed for greater numbers of proteins to bind onto the surface. In addition, it was found that different proteins involved in different physiological pathways, including the acetyl-CoA metabolic process, endocytosis, protein folding, glycolysis, energy-coupled proton transport, protein polymerisation, regulation of neurotransmitters, blood coagulation, and the acute inflammatory response, were attached to the NPs' surface.123 This indicates that the biomolecular corona may play a key role in the NMs' interactions with cells for endocytosis and their involvement in tissue, leading to the overall toxicity or beneficial effects to an organism. Furthermore, a proportion of inhaled NMs can be mobilised up the trachea via the mucociliary escalator and thus reach the gastrointestinal tract (GIT), which will be discussed in the following section.
Despite the lung has been considered for long a sterile organ, it was found that the trachea and the bronchial tree can contain more than 2000 organisms per cm2 surface (calculated as the number of bacterial/fungal/viral genomes).124 The alveolar region is characterised by a thin layer of squamous epithelial cells, Alveolar Type I Epithelial Cells, that are covered by a thin layer of alveolar surfactant, which is secreted by alveolar type II cells and contains free fatty acids, phospholipoproteins, phosphatidylcholine-containing lipids and surfactant proteins. It has been shown that free fatty acids have antibacterial activity against Gram-positive bacteria, while Surfactant Proteins A and D exert bacteriostatic action against Gram-negative bacteria, increasing the permeability of the microbial membrane.125,126 The lung microbiota is complex, and varies in composition between healthy and diseased conditions. Its functions include immune-modulation, support to nutrient uptake, and protection against other airborne pathogens.127
Most nanotoxicological studies have focused on the potential for NMs to induce respiratory inflammation. However, little is known about the direct effects that inhaled NMs have on the lung microbiota, and how this can affect the entire body.128 Inflammatory processes, such as those potentially induced by inhaled NMs, can lead to increased alveolar permeability, which in turn can increase the local nutrient levels, alter the growth conditions, and favour bacterial adhesion. Once in the lung, NMs can modulate the body's immune response, leading to the recruitment of additional immune cells to the site of inflammation. This can lead to disruption of the microbiota homeostasis. Alternatively, NMs can directly affect the resident bacterial population, i.e., by releasing bactericidal ions (e.g., Ag or Zn ions), inducing oxidative stress (e.g., nano TiO2), or the bound NMs can induce rupture of the bacterial cell. The resulting imbalance in the microbial population could potentially lead to the proliferation of other, potentially pathological strains.
NM exposure through ingestion
Physiology of the exposure route
Ingestion is an important route of exposure for NMs since they are intentionally present as food additives,129 contained in pharmaceutical and cosmetic products that can be accidentally ingested,130 and unintentionally present in food from materials that are in contact with it.131 Moreover, NMs ingestion might be accidental, or it can follow inhalation, since NMs can reach the gastrointestinal tract via the mucociliary escalator.132 The mucociliary escalator is a key defence mechanism in the respiratory system based on the coordinated movement of cilia from cells in the respiratory tract to push mucus that traps particles and pathogens up toward the throat. This mucus can then be swallowed or expelled, helping to protect the lungs from infections and clear inhaled particles.133 Despite its relevance, the number of studies assessing the hazard of ingested NMs is still relatively low if compared to inhaled NMs. One of the reasons can be found in the poor availability of in vitro models able to simulate the complexity of the gastrointestinal apparatus. This apparatus, whose main functions are motility, secretion, and absorption, is composed of accessory organs and the alimentary canal or oral-gastro-intestinal tract (OGIT).132,134 The accessory organs are salivary glands, liver, gallbladder, and pancreas, which produce the secretions that comprise digestive fluids.135 The alimentary canal consists of the mouth, oesophagus, stomach, and intestine. It is responsible for the motility and digestion of food, as well as nutrient absorption. This is possible owing to the mucosal barrier, having a surface area and length of approximately 200 m2 and 5 m in adult humans, respectively.135 All areas of the OGIT are mechanically protected by a layer of mucus of variable thickness and composition that is produced by specialised OGI epithelial cells (i.e., goblet cells).132,136 This mucus layer represents the first barrier through which ingested substances, including NMs, must diffuse and pass before coming into contact with the OGI epithelial cells. To add more complexity, a heterogeneous community of microorganisms colonise the OGI tract. The gut microbiota is formed by more than 1014 symbiotic microbial species, and it is known to play a major role in human health and disease.137
The mouth and stomach are responsible for the breakdown and mixing of food, and the initial partial digestion of lipids, starches, and proteins.138,139 Meanwhile, the intestine is the main organ responsible for the final digestion and absorption of nutrients and water. Water reabsorption occurs in the large intestine (i.e., colon, rectum), while the other nutrients are absorbed in the small intestine (i.e., duodenum, jejunum, and ileum) after being digested by pancreatic enzymes.139 To ensure efficient absorption of nutrients, the intestinal wall of this anatomical portion presents particular structures, such as folds, villi, and microvilli, which increase the available area for absorption.139 The units making up the villi structures are specialised cells that are divided into 5 different types: enterocytes, which is responsible for absorption, goblet cells that produce mucus, enteroendocrine cells that are responsible for the hormone production, Paneth cells that maintain intestinal homeostasis in different ways, and microfold cells (M-cells) that phagocytise large foreign particles and present antigens to the immune system.140 After the mucous layer, epithelial cells represent the second physical barrier of the intestine. Epithelial cells are connected by an intercellular junctional system represented by tight junctions (TJs), adherent junctions (AJs), and desmosomes.140 They regulate the absorption of nutrients, and prevent the passage of bacteria and other particles throughout the epithelial barrier.141 Altogether, epithelial cells maintain the integrity of the epithelium by forming strong adhesive bonds.140,142 AJs are located below TJs and contribute to their assembly, while TJs are the most apical and adhesive junctions that strongly seal the intercellular space. Several proteins and biomolecules are involved in their formation: transmembrane proteins, such as claudins and occludin; peripheral membrane proteins like zonula occludens (ZO) proteins, specifically ZO-1 and ZO-2; and junctional adhesion molecules (JAMs),140,142 in which ZO-1 acts as a scaffold for the others proteins.143,144 TJs and AJs are directly connected to the cytoskeleton, and can be regulated via actin and myosin.140,142 Owing to TJs thickness (less than 0.4 nm), just water and solutes can pass through the paracellular route145 in the case of an intact barrier.146–150
To avoid the diffusion of pathogens and other dangerous particles or molecules, the epithelial barrier is linked to a competent lymphoid tissue called the gut-associated lymphoid tissue (GALT). GALT includes Payer's patches, mesenteric lymph nodes, and competent immune cells present in both epithelium and lamina propria.151 The main components are dendritic cells (DCs) that transport the antigens to mesenteric lymph nodes152,153 and stimulate T-lymphocytes,154,155 macrophages that present antigens to T-cells156,157 and contribute to maintaining the immune tolerance,158,159 B-lymphocytes that produce antibodies and cytokines160 and activate T-cells,161 and T-lymphocytes that are the effectors of the immune response.151 This specialised tissue is indirectly in communication with the intestinal lumen via M-cells, which can phagocytise luminal antigens (including bacteria, viruses, fungi, toxins, prions), and transport them to the GALT components by transcytosis.162–164 The microbiota also plays a role in gut immune function, contributing to the maintenance of immune tolerance and promotion of intestinal homeostasis by producing molecules such as short-chain fatty acids.165,166
Simulated digestion systems are essential tools for studying the exposure to NMs through ingestion. At the moment, there are no validated models for NMs. This is mainly due to the difficulty in mimicking the different variables of the OGIT. Initial studies, such as those described in Minekus et al.,168 settled the groundwork for creating these artificial fluids, focusing on the non-fasted state to better mimic the physiological conditions during food ingestion. These efforts led to the development of the INFOGEST protocol, a standardised and comprehensive approach widely adopted for simulating gastrointestinal conditions.169 Published in 2019, the INFOGEST protocol represents a significant advancement by offering a more accurate representation of digestive processes. It incorporates various physiological parameters, such as enzyme activities and pH levels typically observed postprandially, and fixes the ratio between the ingested amount (bolus), simulated saliva fluid, simulated gastric fluid, and simulated intestinal fluid, ensuring consistency and reproducibility across studies. Other protocols, like those used by Sohal et al.170 and Antonello et al.,171 focus on the fasted state, which is crucial as well for different types of digestion studies. These protocols address specific research needs and complement the INFOGEST guidelines by providing alternative conditions under which the biomolecular corona of NMs might exhibit different physicochemical and biomolecular characteristics.
Bio–nano interactions
Upon reaching the gut and being internalised by cells, NMs can have various fates, i.e., i) secretion back into surroundings; ii) accumulation in the cells; iii) degradation in the cell; and iv) gain access to the blood and other organs.134 Therefore, ingested NMs may induce both local and systemic effects. The barrier crossing can occur via a transcellular route mediated by M-cells or epithelial cells.172–176 An example can be found for polystyrene NPs that were found to be able to cross an in vitro epithelial barrier model (Caco-2 cells) cultured both on porous inserts and on an organ-on-chip device.177 Alternatively, barrier crossing may occur via a paracellular route if the barrier's integrity is perturbed. For example, some NPs have been reported to interact with cytoskeleton proteins, leading to the disruption of TJs.149,178 It is thus clear that the set-up of in vitro models able to reproduce the complexity of the gut and to monitor the different possible effects of NMs is not straightforward. During the transit in the OGIT, NPs experience conditions that can dramatically modify their bio-identity, such as a pH ranging from 2 to 8, strong ionic strength, interaction with mucus, and several proteins (including enzymes). Consequently, dissolution, enzymatic degradation, aggregation, and surface modifications, comprising corona formation, may occur depending upon the chemical nature of the NMs, thus affecting the absorption and biological response, including the uptake rate, uptake pathways, and toxicity (Fig. 5).170,179,180
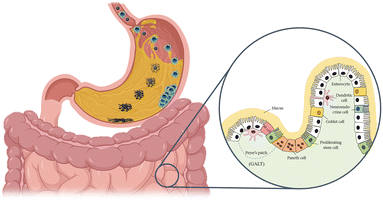 |
| Fig. 5 NM transformation through the oral-gastro-intestinal tract. Ingested NMs might undergo dissolution/degradation, aggregation/agglomeration, and surface modification following formation of a biomolecular corona. These transformations show great potential in modifying their bioactivity toward the gut epithelium. In the inset, a scheme of the complex structure of the intestinal barrier is shown. Adapted from Kong et al.167 | |
The dissolution of NMs after the exposure to biological fluids is a widely studied process. For inorganic NMs, the solubility and dissolution rate determine their bio-durability (in turn, affecting their bio-persistence)132,170 in the biological systems.181 On the other hand, the solubility and dissolution rates may increase the hazard of NMs when the released species are toxic. Dissolution is less important for organic NMs. In this case, enzymatic degradation is the most relevant process affecting their bio-persistence. For example, the enzyme-mediated partial degradation of lipid NMs was shown by Antonello et al.171 Interestingly, because of the extreme conditions of the OGIT fluids, NMs might be formed following precipitation processes. For example, following the exposure of an intestinal model to AgNO3, NMs were detected in the cell fraction.182,183 Similarly, hydroxyapatite NMs dissolved in simulated gastric fluid were found to form novel NMs in simulated intestinal fluid.171 Particle agglomeration and aggregation are highly probable in the OGIT. This is due to the high ionic strength of the fluids and the presence of the glycoprotein mucin. This process is known to modify the bioactivity of NMs. Particle size can actually modulate the uptake of NMs and their ability to cross biological barriers, including the intestinal one. For example, a study reported by Jani et al.184 showed much more absorption in the colon mucosa for 50 nm polystyrene NPs compared to 100 nm ones, while 300 nm NPs were not absorbed. Additionally, 100 nm particles remained within the submucosa (deeper connective tissue) of OGIT, while 50 nm NPs entered the bloodstream and accumulated in the liver and spleen. This is because small particles can more easily diffuse through the pores of the mucus network, and gain more access to the systemic circulation.
The NMs dissolution and agglomeration/aggregation were reported in a previous study by Sohal et al. In this study, a series of inorganic particles (TiO2, Fe2O3, ZnO, and SiO2) with different size ranges were selected from commercial food products, and analysed for their dissolution and agglomeration using an in vitro system simulating the OGIT (saliva, gastric, and intestinal).170 Upon incubation in simulated saliva fluid, SiO2 and ZnO were shown to have a lower biodurability and persistence in comparison to TiO2 and Fe2O3. No significant changes in agglomerate size were observed for TiO2, ZnO, and SiO2 in simulated saliva fluid. However, a large agglomeration was detected in the case of Fe2O3 when evaluated by DLS and TRPS (tuneable resistive pulse sensing). Dissolution can be predicted to be more relevant in gastric fluid for metals and metal oxides due to the strong acidic pH. As expected, SiO2 particles were reported to only partially dissolve in simulated gastric fluid, whereas ZnO particles were dissolved completely after 2 h. On the contrary, TiO2 and Fe2O3 particles showed large agglomeration, but no significant ion release.170 Rapid aggregation in simulated gastric fluid was reported also for silver NPs (AgNPs) in other studies.185,186 Dissolution and agglomeration/aggregation were reported to occur also in intestinal fluid. In this case, the basic pH condition promoted the dissolution of SiO2, while no significant dissolution was reported for TiO2 or Fe2O3.170
Being a surface-driven process, dissolution could be affected by the presence of a biomolecular corona. For example, this was shown by Ngamchuea et al. for AgNPs, when they reported that coating AgNPs with organics/proteins reduced the access of dissolved oxygen to the NP surface, thus minimising the release of Ag+ ions.187 A similar protective effect was reported by other authors on AgNPs170 or hydroxyapatite NPs.171 The latter material was completely dissolved in simulated gastric fluid deprived of the proteins, but only partially when proteins were present. The protective effect of the biomolecular corona is expected to be dependent on the surface chemistry of NMs, which in turn determines the nature of the protein–surface interaction. For example, Abdelkhaliq et al. reported that coating AgNPs with lipoic acid or with citrate affects the cellular uptake. This is likely due to the formation of a different corona because of the different surface chemistry.188 Another study in simulated gastric fluid using AgNPs of 20 nm and 110 nm with two different coatings – citrate and polyvinylpyrrolidone (PVP) – indicated a significant increase in diameter, especially in the presence of pepsin, suggesting aggregation. Despite this, there was minimal impact on pepsin's proteolytic function and no detectable changes in its secondary structure.186 Extensive work has been done with TiO2 NPs due to their use as a food additive (E171), despite their use in some countries having been recently banned.189 For instance, the interactions of TiO2 NPs with casein, a major milk protein, were characterised in one study. The formation of a protein corona around the TiO2 NPs led to the dissociation of casein micelles and the creation of NP–protein complexes, which extensively aggregated. This aggregation hindered gastric digestion by reducing the accessibility of pepsin to its substrate, influencing the bioavailability and toxicity of the NPs.190 Another article examined the biomolecular corona on food-grade TiO2 NPs in different food models. After digestion, a biocorona rich in lipids was observed, with its composition varying significantly with the fat content of the diet. This lipid-rich corona appeared to reduce oxidative stress and toxicological impacts, suggesting a protective role.191
The effect of the biomolecular corona on the colloidal properties of NMs is only one of the possible mechanisms potentially leading to a modification of the NMs bio-identity. In fact, the biomolecular corona can contain molecules that have a biological activity. In a study by Antonello et al.,171 different kinds of NPs (lipid-surfactant NPs, carbon NPs, surface-modified Fe3O4 NPs, and hydroxyapatite NPs) were studied for their effect on an intestinal barrier in vitro model after treatment with a simulated digestion system. The formation of a bio-corona, which contains proteases, was demonstrated for Fe3O4 NPs. This was related to the ability of the NPs to upregulate the tight junction genes in the intestinal barrier, and to increase the expression of both pro- and anti-inflammatory cytokines (IL-1β, TNF-α, IL-22, IL-10). The INFOGEST protocol has also been used to study NMs, such as magnetite NPs, after gastric and duodenal digestion phases.192 Using sucrose gradient ultracentrifugation, they isolated and characterised the size and protein composition of the NP–corona complexes, and conducted translocation studies on Caco-2 cell monolayers in a serum-free environment. The findings revealed that the NP corona differed in size, surface charge, and protein composition between the gastric and duodenal phases. The digestive protein corona enhanced NP cellular uptake, inducing morphological changes in the Caco-2 cell monolayer.192 Brouwer et al. also used the INFOGEST protocol to explore how gastrointestinal digestion affects the protein corona on polystyrene micro- and nanoplastics, and their subsequent uptake by human THP-1-derived macrophages.193 Researchers found that digestion creates a unique protein corona, which can persist in serum-containing cell culture medium. This digestion-induced protein corona significantly increases the uptake of uncharged plastics smaller than 500 nm, but does not affect larger or charged micro- and nanoplastics.193 Other examples of molecules in the bio-corona that can modulate the biological effect of NPs exist. For example, transferrin194 and bile acids195–197 were found to promote transcytosis of NPs since they are able to interact with specific receptors present on the apical side of the enterocytes. The transformations described above are material-dependent. In a study performed on food-grade TiO2, aggregation and formation of a hard corona were observed during simulated digestion. Nevertheless, these processes have little effect on the acute toxicity and genotoxicity of TiO2 toward epithelial HCT116 cells, while a slight decrease of the surface reactivity and of the ability to induce oxidative stress was observed.198 Furthermore, the composition of the biomolecular corona can be dramatically affected by food. For instance, an increase of the uptake of poly(acrylic acid)-coated AgNPs by Caco-2 intestinal cells was observed when digested in the presence of food.199,200
Finally, an increasing number of studies provided evidence of the effects that follow the interaction of NMs with the intestinal microbiota. NMs may induce modification of the microbial physiological community.201–203 At the same time, molecules derived from the microbiota may modulate the biological effect of NMs. For example, Pseudomonas aeruginosa exotoxin A was found to enhance the transcytosis of NPs.204 It is important to note that data obtained by in vitro studies concerning ingestion are in general poorly comparable. This is due to the lack of standardised methodologies and validated models for both OGI fluids and the intestinal epithelium.205 On the other hand, the large variability of the composition of the fluids due to physio/pathological conditions and food makes the definition of standards particularly challenging.
NM exposure in the blood
Physiology of the exposure route
Blood is a specialised connective tissue responsible for transporting different components to different organs, playing a vital role in the physiological regulation and defence of the body. The most abundant element is red blood cells or erythrocytes, whose primary function is to transport oxygen from the lungs to the different tissues, and to carry carbon dioxide back to the lungs for exhalation. Red blood cells achieve this vital role through the protein haemoglobin, which binds to both oxygen and carbon dioxide.206 They have a distinctive biconcave shape, an evolutionary approach – which is reminiscent of the one in nanoscience – to acquire larger surface areas, enabling them to exchange gases more efficiently.207 White blood cells or leukocytes are also an important part of blood, being essential for immune responses and maintaining body health.208 There are several types of white blood cells, each with specific roles in the immune system. The main types include neutrophils, lymphocytes, monocytes, eosinophils, and basophils.209 However, to understand the bio-identity of NMs in blood, the most important item is plasma. Blood plasma, the liquid component of blood, constitutes about 55% of its volume and is primarily constituted by water (92% of its volume), which acts as a solvent to transport proteins, electrolytes, nutrients, gases, and wastes throughout the body.210 It supports vital functions such as maintaining homeostasis, regulating body temperature, and ensuring pH balance. Plasma proteins like albumin, globulins, and fibrinogen are essential in maintaining osmotic pressure, facilitating immune responses, and enabling blood clotting.211 Abnormalities in these protein levels can indicate various diseases, such as nutritional deficiencies, liver disease, immune disorders, and clotting dysfunctions.212 Thus, plasma plays an integral role not only in transporting substances but also in diagnosing and managing various health conditions, making it a central element in cardiovascular health and overall body maintenance.
Bio–nano interactions
As stated in previous sections, the translocation of NMs from different entry routes could end up in the bloodstream.213 In addition, NMs can access the bloodstream either by direct injection,214 or rarely, through the hair follicles of the skin.215 When NMs come into contact with the bloodstream, they can interact with associated cells and biomolecules, and trigger a variety of potentially adverse effects. In this context, the potential toxic effects of NMs exposure in blood and the influence of the corona have been highlighted.
For example, a study reported by Kim et al. showed that SiO2 NPs caused hemolysis, deformation, and aggregation of red blood cells (erythrocytes).216 Negatively charged NPs can also induce hemolysis of erythrocytes because of the interactions with organic cations of their membrane.217 NMs can also interact with platelets, leading to activation of the coagulation cascade, the formation of blood clots, and the partial or total occlusion of blood vessels by thrombi, as reported in the case of SiO2 NPs, AuNPs, AgNPs, CdTe, and CdSe quantum dots, among others.218–221 Therefore, hemocompatibility extends beyond hemolysis to encompass factors like erythrocyte aggregation, deformation, and vascular occlusion.222 While hemolysis serves as an indicator, the formation of thrombi due to NPs aggregation poses significant risks, potentially leading to vascular blockages.223 However, not all intravenous nanoformulations result in health risks. Some products that are already on the market, like nanoparticle-based intravenous injections indicated for the treatment of iron deficiency, seem to improve traditional products.224 In this case, the particles are multicomponent NMs consisting of an iron core surrounded by a carbohydrate shell, emulating the structure of serum ferritin.225 The shell formulation varies, depending on the product and the iron content, with concentrations ranging from 12.5 to 100 mg ml−1.225 To assess the NM safety, the physicochemical properties that modulate NP aggregation in blood have been recently studied.226 The interaction of NPs with blood biomolecules results in the aforementioned corona, guided by the NPs' physico-chemical properties. For instance, smaller NPs adsorb relatively more proteins compared to larger NPs due to having a larger surface area-to-volume ratio in smaller NPs.227 Additionally, hydrophobic NPs adsorb more than twice the amount of proteins of hydrophilic NPs (12 vs. 26 μg of proteins per mg of NP, respectively).228 Other studies showed that hydrophobic NPs have a higher binding affinity for apolipoproteins, while hydrophilic NPs favoured the adsorption of fibrinogen, IgG, and albumin.27,229 Researchers also found that hydroxylation can reduce the adsorption of certain proteins like HSA and IgE, which typically promote immune clearance, while maintaining the adsorption of ApoE, which prolongs circulation in the bloodstream.230 Charged NPs can cause more protein denaturation than neutral NPs.231 Studies on polystyrene NPs demonstrated that positively charged NPs have higher binding affinity to proteins with an isoelectric point of less than 5.5 (e.g., albumin), while negatively charged NPs prefer proteins with higher values (e.g., IgG).232 Another study on various organic and inorganic NPs has demonstrated that their exposure to human serum albumin results in diverse corona formation and composition. These differences manifest in the hydrodynamic size of the NPs.119 Porous particles decreased the amount of adsorbed proteins due to the size-exclusion effect.227 In turn, coating NPs with polyethylene glycol (PEG), zwitterionic compounds or polysaccharides can also minimise protein adsorption.233,234 However, when targeting ligands are attached to the NP surface, serum proteins interfere with their binding to target cells. Conjugating targeting ligands to an equilibrated corona is an alternative to overcome the issue and enhance their binding.235
On the other hand, the biomolecular corona can impact the NMs' physico-chemical properties and highly influence their circulation time, as well as their deposition in tissue. Binding proteins known as opsonins, such as immunoglobulins and complement proteins, facilitate the recognition and uptake of NMs by phagocytic cells, thereby reducing their circulation half-life.236,237 In contrast, desopsonins, such as albumin and apolipoproteins, promote prolonged NM circulation times and enhance the potential for targeted delivery to specific tissues.238–240 For instance, a study reported by Wang et al. outlined that blood proteins can mask the surface charge of cationic NPs, and thereby inhibit cell death caused by positively charged NPs.241 The masking effects of several types of blood proteins, specifically, bovine fibrinogen (BFG), BSA, transferrin (Tf), and γ-globulin (Ig), can be extended to reduce NPs' toxicity compared to naked/pristine ones, as reported for poly(3-hydroxybutyrate-co-3-hy-droxyhexanoate) NPs and SWCNTs.54,242 The superparamagnetic properties of magnetic NPs were reduced upon interaction with serum proteins because of corona formation, which prevented the core spins from aligning along the field direction.243 The hydrodynamic size of AuNPs was increased by approximately 50 nm upon incubation with blood plasma proteins for 30 min.244 Such events can cause changes in the NPs' cellular internalisation and co-localization based on the new identity of the NPs. For instance, SiO2 NPs under serum conditions exhibited stronger accumulation at lysosomes. Meanwhile, under serum-free conditions, they showed a higher degree of attachment to the cell membrane and greater internalisation (both lysosomes and cytosols).245 In addition, translocated NMs from different entry routes may be subjected to a partial displacement of the original corona by new molecules from the new environment. Other biomolecules that are not replaced would serve as a corona ‘memory’ of the NMs' previous exposure environment.246 This could be evolved to map the transport pathways utilised by NMs, and eventually explore the potential hazards from their exposure.
During the last few years, a wide range of the population has been voluntarily exposed to NPs in their blood.247 The worldwide administration of vaccines during the COVID-19 pandemic led to a novel formulation, where the vaccines were based on encapsulated mRNA in lipid NPs.248,249 Since then, the research on NP-based vaccines via intramuscular or intravenous injection has become a critical aspect of immunisation strategies,250 but also for cancer treatment.251,252 For example, Siemer et al. developed cisplatin-loaded, polysarcosine-based polymeric NPs that bypass the LRRC8A-mediated resistance mechanism through endocytic delivery, effectively eradicating cisplatin-resistant cells.253 In this context, injection routes will need to be addressed for future human trials. Intramuscular injection, commonly performed in the deltoid muscle, offers advantages such as slower absorption and prolonged immune response.254 On the other hand, intravenous injection represents a more direct route into the bloodstream, but can lead to rapid immune activation with severe effects on health, such as myopericarditis.255 These recent advancements in vaccine delivery technology, however, still lack comprehensive characterisation of the NP stability and the potential biomolecular corona formation on top of the nanosized vaccines.
NM exposure in synovial fluid
Physiology of the exposure route
The synovial fluid is a viscous non-Newtonian fluid found in the cavities of synovial joints.256,257 It is mainly characterised by its critical role in facilitating proper joint function by reducing friction between the articular cartilages during movement. Synovial fluid is primarily an ultrafiltrate of plasma containing hyaluronic acid, which gives viscous and elastic properties, and lubricin (or proteoglycan 4), crucial for lubrication to protect cartilage surfaces.258,259 Additionally, it includes serum proteins such as albumin and globulins, cytokines, and growth factors that regulate synovial cell functions and respond to inflammation and healing,260 as well as phospholipids and proteoglycans that contribute to the biochemical environment.261 Beyond lubrication, synovial fluid serves as a transport medium for nutrients and metabolic wastes to support the avascular cartilage, and contains phagocytic cells for removing debris and microorganisms, therefore contributing to immune protection.260 Alterations in the composition or quantity of synovial fluid can lead to joint diseases such as osteoarthritis or rheumatoid arthritis, where the fluid often becomes inflamed, losing its protective properties and leading to pain and reduced mobility.262,263 Treatment for joint diseases such as rheumatoid arthritis focuses on reducing inflammation, managing pain, and maintaining joint function. Medications like non-steroidal anti-inflammatory drugs (NSAIDs), corticosteroids, and disease-modifying antirheumatic drugs (DMARDs) are commonly used.264 In severe cases, joint replacement surgery may be necessary. Here, implants designed to mimic the movement of natural joints are used to replace damaged areas, and can significantly improve quality of life by restoring mobility and reducing pain. However, they can end up degrading over time due to several reasons, from the body's immune response to the implant to mechanical wear and tear (Fig. 6). To avoid this, much research has been carried out in recent years to find biocompatible265 and biodegradable266 implants.
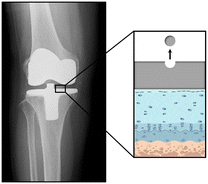 |
| Fig. 6 Scheme of NM release in synovial fluid from knee orthopaedic devices. Representative picture of how NMs are discharged into the synovial fluid from the surface of orthopaedic devices implanted within the knee joint. Adapted from Sato et al.267 with permission from Elsevier, copyright 2024. | |
Bio–nano interactions
NM presence in joints has become a matter of concern in orthopaedic devices, where particles generated at the contact surface of the implant bearing are the primary cause of aseptic loosening. This is often a limiting factor for patient response to an implant, particularly when metallic residues of the implants have the potential to translocate into the circulatory system. The viscosity and complex biological composition of the synovial fluid could influence the NMs' properties that are released by articular prosthesis since it is the first substance with which they come into contact prior to their eventual translocation to the bloodstream.246 For instance, Brown and co-authors have reported the effect of synovial fluid on the colloidal properties of two groups of PLGA nanocarriers (with cationic and anionic functional groups) and cartilage retention ex vivo. PLGA is one of the most effective biodegradable polymeric NM, and widely used as a carrier for drug delivery systems.268,269 As a result, it was stated that the synovial fluid induced aggregation of cationic PLGA NPs after incubation at 37 °C for 30 minutes, probably because of the NPs' interaction with the predominate hyaluronic acid.270 This was confirmed by an increase in the hydrodynamic diameter, as well as a reverse change in the zeta potential. However, no aggregation was detected in the case of anionic PLGA NPs, i.e., no change in the hydrodynamic diameter and zeta potential. Additionally, cationic PLGA NPs showed higher cartilage retention compared to anionic NPs, which clearly suggests that synovial fluid is a modulator of the NPs' fate in the joint. The effect of synovial fluid on the NMs' properties can be extended to include the corrosion induction of the implantable materials that may end up with adverse human health effects.71 For instance, in a study conducted on selected patients who had previously undergone hip resurfacing arthroplasty using an alloy of chromium–cobalt metal-on-metal (MoM), it was reported that the release of oxidised cobalt ions (Co+2) from the metal debris that wears away from the MoM hips caused inflammation to the surrounding tissues, accumulation in white blood cells, mitochondrial damage, and abnormal nuclear morphology of macrophages. In addition, the patient's blood levels exhibited extremely high Cr and Co contents, which confirmed the release of NMs from the joint replacement to the bloodstream.71,271 This raises a serious question about the mechanism of in vivo dissolution of Co/Cr/MoM because, on a bulk scale, this MoM alloy is extremely stable/corrosion resistant. A recent study reported by Simoes et al. found a much higher release of MoM ions relative to Co and Cr in the presence of bovine serum albumin (BSA), as confirmed by inductively coupled plasma mass spectrometry (ICP-MS).272 In the same context, the adsorption of synovial proteins can affect the MoM hips' properties, inhibit their function, and thus increase ion dissolution. Accordingly, the MoM hips were revised after 3.5 years due to squeaking, clicking, and painful hip movements, which opens another question about how materials are tested in terms of safety assessment before they are used as implantable materials. To address these issues, plasma coating of hip joints (ball, socket, and shaft) with Si3N4 has been studied as a safe-by-design solution to offer potential lifelong in vivo use without revision.273 These new joints were subjected to extensive wear testing by robot flexing under load, encased in simulated synovial fluid (BSA, Lubricin, and PBS). The NPs generated were substantially reduced in concentration compared to MoM joints, confirming higher lubricity. Toxicity was assessed by oxidative stress, cytokine release, TNF-α cytotoxicity, and DNA strand break assays. All 4 toxicity assays yielded non-significant outcomes in contrast to MoM joints. As a result of these findings, two CEN, one ISO, and one ASTM standards have been secured based on the joint testing method and the toxicology assays to ensure the safety-by-design of hip joints.274
Dermal and ocular exposure to NM
Despite the permeability of the involved organs being much lower, dermal and ocular exposures to NMs have also been studied in recent years. For instance, the in vitro absorption of TiO2 and ZnO in cosmetic formulations through porcine skin found that these particles do not penetrate the outermost layer of the epidermis. Total recoveries of zinc and titanium were close to the amount applied, indicating no significant absorption into the skin. Therefore, their use in topical products such as sunscreens is not considered a health risk due to the absence of internal exposure.275 However, studies have demonstrated that particles smaller than 10 nm could passively penetrate the skin through the stratum corneum lipid matrix and hair follicle orifices, reaching deeper skin layers and hair follicles, which can lead to potentially adverse effects.276 Additionally, researchers highlighted that damaged skin allows for greater penetration of NPs.277 To evaluate this rather unlikely scenario,278in vitro and in vivo studies have been applied to skin cells. Monteiro-Riviere et al. found that AgNPs complexed with different serum proteins significantly influence their uptake by human epidermal keratinocytes (HEK), with proteins generally suppressing citrate-coated AgNP uptake, while having minimal effect on silica-coated AgNPs.279 The presence of a biomolecular corona actively influences NM behaviour, as researchers have also shown in a study on ZnO NPs, where particle size and protein corona formation affected toxicity and cellular responses in HEK cell lines.280
Research on ocular environmental exposure to NMs is less well characterised. Research studies have indicated that metallic NMs can cause eye irritation and inflammation through direct contact, with chronic exposure leading to elevated toxicity.281,282 For example, the exposure of rabbit eyes to TiO2 NP induced ocular surface damage.283 In contrast, when investigating the in vivo safety of oral and ocular topical applications of 100
nm SiO2 NPs in rats, blood biochemical parameters and ophthalmic examinations revealed no abnormal findings in any of the animals after 12 weeks.284 However, organic NPs like liposomes are becoming a safer alternative for ocular drug delivery nanosystems.285 Furthermore, researchers showed that coating cationic liposome–DNA complexes with artificial biomolecular coronas made of fibronectin (FBN) and Val-Gly-Asp (VGA) tripeptide significantly enhanced their uptake by corneal epithelial cells, which was being reduced by mucin.286
Dose and media impact on NM interactions
Understanding the impact of NM exposure on cells is essential to guarantee their safe use. Apart from the size, which does not seem to drastically alter the corona composition,287 surface chemistry is also an essential factor to foresee potential NM toxicity.288,289 For example, in the case of oxide particles (OxPs) such as SiO2 NPs, hydroxyls (OH) exposed at the surface can lead to cell toxicity.290 However, the quantity of NPs and the biological fluid in which they are dispersed are two critical variables, which may also affect NP–cell interactions.
When assessing the nanosafety profile of NMs, the presence or absence of proteins in cell culture medium was shown to affect the NMs' toxicity. This indicated that the cell culture medium has a role in “feeding” the cells, and can indirectly affect viability by protecting them. Early studies by Hu et al.291 with graphene oxide NPs and Lesniak et al. with silica NPs245 showed that the protein corona mitigates NP toxicity by lowering their surface energy and interaction. NMs exposed to serum-free media and without corona lower their binding energy by strongly interacting with cellular membranes, causing rupture and necrosis.292 While a serum-free environment does not represent a realistic exposure scenario, a high dose of NPs in a cell culture media with low protein might lead to an incomplete coverage of the NPs by the corona, and a possible toxic response caused solely by this phenomenon. Recent studies indicate that the corona can be visualised by Cryo-TEM, representing a realistic picture of the NP in situ corona, which could provide useful information of the dosimetry.293,294
The nature of the cell culture medium greatly impacts how NMs behave and interact with cells. Citrate-capped AuNPs exposed to Dulbecco modified Eagle's medium (DMEM) and Roswell Park Memorial Institute medium (RPMI) with fetal bovine serum showed significant differences in biomolecular corona composition and cell uptake. Testing 15 nm AuNPs on HeLa and U937 cell lines, the NPs showed higher internalisation and cytotoxicity when incubated with RPMI, compared to DMEM.295 Furthermore, studies have shown that matching biological media with the cell type favours the uptake of NPs, as if cells preferred to uptake proteins from the same species.296 It is also crucial to take into account how the amount of NPs has an impact on the biomolecular corona formation. Changing the dosage of NPs can alter the ratio of the NP surface area to accessible proteins, which in turn, can alter the corona structure.297 This dose-dependent effect can profoundly impact the interaction of NPs with biological systems, including cellular absorption, signalling pathways, and possible toxicological reactions (Fig. 7).
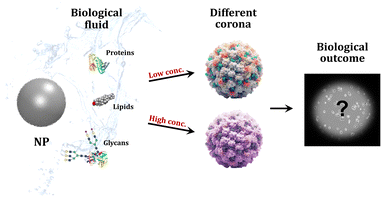 |
| Fig. 7 Differences in the fluid/NP ratio change the corona with possible biological impacts. When particles are exposed to biological fluids, the concentration of their biomolecules and the NP dose can produce different corona compositions, which at the same time may alter the biological outcome. | |
Overall, the corona is derived from biomolecules embedded in biological fluid, which may undergo post-translational modifications (PTMs), such as phosphorylation, ubiquitination, acetylation, and glycosylation.298 These PTMs can have a direct effect on the biomolecular conformation, and possibly on the binding to the NPs' surface.299 Proteins in the blood are highly glycosylated, a common PTM where glycans – structures of chained and branched monosaccharides – are covalently linked to proteins during their synthesis.300 While glycans were believed to be inert biomolecules, it is now well established that they affect protein folding and stability, controlling a wide range of biological processes as well. Glycan abundance in proteins raises the question of whether they could be an active component of the biomolecular corona. Circulating plasma proteins are highly abundant in the N-glycan form, and depending on the protein, the glycan structures and abundance change.301
By interacting with glycan-binding proteins (or lectins), glycans can trigger specific biological pathways.301 Glycans are recognised by lectins, which are ubiquitously expressed receptors in nature and involved in cell adhesion and immune response.302 For example, endothelial cells express E-selectin, an adhesion lectin that recruits leukocytes.303 In contrast, macrophages express lectins such as the mannose receptor to recognise microbial ligands and promote innate immunity.304 In particular, glycan structures that carry a terminal neuraminic acid (also known as sialic acid) can interact with sialic acid immunoglobulin-like lectin (SIGLEC) receptors and modulate the recognition of the “self”, attenuating inflammation and promoting phagocytosis evasion.305–308 Additionally, loss of sialic acid glycan can lead to the exposure of the galactose residue,309 which is recognised by asialoglycoprotein receptors, such as the Ashwell-Morell receptor in hepatocytes,310,311 leading to protein uptake and turnover.309
There are noticeable differences in glycans and monosaccharides between species. For instance, Lin et al. found significant divergences in glycosylation among human, bovine, and recombinant fetuin.312 Differences are also relevant with sialic acids, where humans lack the CMP-N-acetylneuraminic acid hydroxylase enzyme that generates the N-glycolylneuraminic acid (Neu5Gc), common in mammals (such as bovines), starting from the acetylated form N-acetylneuraminic acid (Neu5Ac).313,314 Studies have shown that the different nature of the monosaccharide (Neu5Ac or Neu5Gc) affects the binding of the glycan with the specific SIGLEC receptor, leading to different modulation of the immunological system.315–317
Since blood plasma is highly glycosylated,301 the biomolecular corona is expected to carry glycans at the bio–nano interface. Wan et al. have first shown that loss of glycosylation in the biomolecular corona leads to higher cellular uptake in M1 and M2 macrophages.318 Moreover, Clemente et al. identified the glycan structures as part of the AuNPs' biomolecular corona, confirming that they are biologically accessible and available for binding towards specific lectins.24 These findings open the doors to new potential applications of NMs, exploiting the biomolecular corona and its glycosylation. Trinh et al. demonstrated that NP's exposure to biological fluids derived from patients with lung cancer carries glycan features specific to the chronic disease,25 giving a new angle to the existing paradigm of the personalised corona previously described.319,320 Because the same NPs can carry different glycosylation structures as reflected in the diverse terminal monosaccharide residues, this may suggest that the glycan components of the biomolecular corona can influence the NP's biological interactions when binding to lectins in organisms (Fig. 8).321
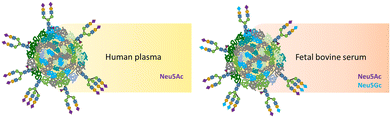 |
| Fig. 8 The origin of biological fluid impacts corona composition. Matching the biological fluid with the testing model can affect the outcomes of the experiments, especially considering the glycan component of the biomolecular corona. For instance, glycans in the human serum contain only the terminal monosaccharide sialic acid in the form of Neu5Ac, while other mammalian species, such as bovine, contain multiple sialic acids, including the non-human form Neu5Gc. | |
Recent studies have also studied whether protein's glycation, a non-enzymatic PTM where the presence of an excess of monosaccharides leads to a covalent linkage to biomolecules (typically occurring under disease conditions such as diabetes), affects the protein corona formation and toxicity of AgNPs. Interestingly, protein glycation resulted in subtle changes in the protein corona secondary structure and corona formation, and both glycated and non-glycated proteins resulted in a decrease in the NPs' dissolution-mediated toxicity.322
Summary and perspective
In this manuscript, we reviewed the human exposure to NMs and their impact on health. Central to this narrative is the pivotal role played by the biomolecular corona – the dynamic layer of biomolecules that cover NMs upon contact with biological fluids. Common routes of exposure include inhalation (lungs) and ingestion (gut), with the potential to cause a distinct biodistribution and organ translocation, as well as varying levels of toxicity. We have outlined the significance of the biomolecular corona not only for toxicity studies, but also as a key factor to consider in terms of NM colloidal stability and material transformation.
Inhalation is a main route of exposure for humans, potentially leading to both local and systemic adverse health effects, as particles suspended in the air can potentially enter the body through the lungs. The ability of NMs to reach deep lung tissues and possible translocation to the blood raises concerns not only about respiratory health, but also about systemic toxicity. This is an important reason for further research to evaluate the NM interaction with lung fluids. On the other hand, the ingestion exposure pathway presents a different set of considerations. Ingested NMs have the potential to interact with the gastrointestinal system with the possibility of potential absorption into the bloodstream. Although more research is being done nowadays to understand NM interactions with the digestive system and its organs, there is a need for a comprehensive understanding of NM degradability and changes in the corona composition along the digestive pathway. It is also important to evaluate the direct exposure of NMs to the bloodstream, a scenario that can occur intentionally for medical purposes, or unintentionally due to accidental entry or after translocation from other exposure routes. The immediate distribution of NPs throughout the body, facilitated by direct contact with the bloodstream, carries both therapeutic promise and potential toxicity concerns. NMs can be also released from orthopaedic implants into synovial fluid, raising concerns about potential health risks and the stability of the materials used. The current research focus is to create safer and biocompatible implants to avoid wear and corrosion, especially in cases involving metallic joint replacements. Lastly, dermal and ocular exposures represent other routes of exposure to diverse NMs, although translocation is less likely as the permeability of the involved organs is much lower.
Understanding the biological role and possible cell toxicity of NMs requires a complex understanding of the interaction between NMs and biological media, accounting for the surface chemistry, size, dosage, and the biological fluid environment. For instance, aligning the in vitro model's species with the relevant biological fluid ensures a more accurate representation of real-world conditions. Overall, the biological fluids of different exposure routes can impact the biochemical and physicochemical properties of NMs. Therefore, they can be studied for monitoring potential hazards upon exposure. Recent advancements in understanding the biomolecular corona in fluids other than blood have been made in recent years. The differences in the corona composition when embedded in different biological fluids might be a potential tool for biomarker discovery. Equally important is an in-depth characterisation of the NMs' biomolecular corona. In this sense, it is still difficult to link the biomolecular corona to adverse or beneficial (nanomedicine) effects. Using a standardised framework to ensure consistency and comparability would help to minimise artefacts and common errors,323 such as biomolecular carryover in the background, potential aggregation induced by separation processes, and unrealistic exposure conditions. For example, there are no specific OECD guidelines dedicated to the evaluation of the biomolecular corona of NMs. However, it is firstly necessary to establish a strong link between the biomolecular corona to biological processes that can be linked to regulatory relevant endpoints. For this to happen, it would be pivotal that standardized methods for the qualitative and quantitative characterisation of the corona are developed and endorsed by international regulatory bodies, such as ISO. Furthermore, it would be beneficial to know the details on the NM characteristics, the dosage (hence the surface area), and the properties of the biological fluid being used, in order to facilitate proper testing and research with them. Emphasis is placed on the need for further research on NMs' interactions with lung and gastric fluids, as these environments are most relevant to possible human exposure. More data and tools are needed to model the biomolecular corona formation for a better prediction of the in vivo data. We believe that through targeted corona characterisation, a more accurate assessment of NM behaviour, and potential toxicity, this goal will be achieved.
Data availability
No primary research results, software or code has been included and no new data were generated or analysed as part of this review.
Author contributions
M. P. M., I. F., T. S., T. W., M. D., G. A., A. M.-S. and M. G. S. critically assessed the literature and discussed the content of the review. M. G. S. and A. M.-S. drafted the review. All co-authors contributed to the review and its revision.
Conflicts of interest
There are no conflicts to declare.
Acknowledgements
This work was supported by the European Union's Research and Innovation Programme Horizon 2020 under grant agreements no. 952924 (SUNSHINE), no. 760928 (BIORIMA) and Marie Skłodowska-Curie no. 814236 (Nanocarb), as well as Horizon Europe Research and Innovation Programme under grant agreement no. 101092901 (POTENTIAL). M. G. S. and M. P. M. also acknowledge Science Foundation in Ireland (SFI) under research grant no. 12/RC/2278_P2, as well as the H2020-MSCA-IF grant no. 894656. T. W. gratefully acknowledges grant agreement no. GA-310477 (LifeLongJoints) funded under the European Union’s Seventh Framework Programme. Fig. 1–6 were created with https://www.biorender.com/.
References
-
Nanoscience and Nanotechnologies: Opportunities and Uncertainties: Summary and Recomendations, Royal Society of Chemistry, 2004 Search PubMed.
- G. M. Whitesides, Nanoscience, nanotechnology, and chemistry, Small, 2005, 1, 172–179 CrossRef CAS PubMed.
- F. Caruso, M. Spasova, V. Salgueiriño-Maceira and L. Liz-Marzán, Multilayer assemblies of silica-encapsulated gold nanoparticles on decomposable colloid templates, Adv. Mater., 2001, 13, 1090–1094 CrossRef CAS.
- D. Khang, J. Carpenter, Y. W. Chun, R. Pareta and T. J. Webster, Nanotechnology for regenerative medicine, Biomed. Microdevices, 2010, 12, 575–587 CrossRef CAS.
- Z. Wang, J. Ruan and D. Cui, Advances and prospect of nanotechnology in stem cells, Nanoscale Res. Lett., 2009, 4, 593–605 CrossRef CAS PubMed.
-
G. V. Research, Nanomaterials Market Size, Share & Trends Analysis Report By Material (Gold, Silver, Iron, Copper), By Application (Aerospace, Automotive, Medical), By Region, And Segment Forecasts, 2023–2030, 2023 Search PubMed.
- J. Lan, Overview of application of nanomaterials in medical domain, Contrast Media Mol. Imaging, 2022, 2022, 3507383 CrossRef PubMed.
- P. Pandey, Role of nanotechnology in electronics: A review of recent developments and patents, Recent Pat. Nanotechnol., 2022, 16, 45–66 CrossRef.
- Q. Zhu, M. H. Chua, P. J. Ong, J. J. C. Lee, K. L. O. Chin, S. Wang, D. Kai, R. Ji, J. Kong and Z. Dong, Recent advances in nanotechnology-based functional coatings for the built environment, Mater. Today Adv., 2022, 15, 100270 CrossRef CAS.
- M. Tabish, M. M. Zaheer and A. Baqi, Effect of nano-silica on mechanical, microstructural and durability properties of cement-based materials: A review, J. Build. Eng., 2023, 65, 105676 CrossRef.
-
N. Kaitwade, Analysis of the Nanomaterials Market by Carbon-Based, Metal-Dased, Dendrimer-Based, and Composite Segments, Future Market Insights, 2023 Search PubMed.
-
P. Research, Nanomaterials Market - Global Industry Analysis, Size, Share, Growth, Trends, Regional Outlook, and Forecast 2023–2032, 2023 Search PubMed.
- B. Nowack, J. F. Ranville, S. Diamond, J. A. Gallego-Urrea, C. Metcalfe, J. Rose, N. Horne, A. A. Koelmans and S. J. Klaine, Potential scenarios for nanomaterial release and subsequent alteration in the environment, Environ. Toxicol. Chem., 2012, 31, 50–59 CrossRef CAS PubMed.
- I. Furxhi, A. Costa, S. Vázquez-Campos, C. Fito-López, D. Hristozov, J. A. T. Ramos, S. Resch, M. Cioffi, S. Friedrichs and C. Rocca, Status, implications and challenges of European safe and sustainable by design paradigms applicable to nanomaterials and advanced materials, RSC Sustainability, 2023, 1, 234–250 RSC.
- D. Hristozov, A. Zabeo, L. G. Soeteman-Hernández, L. Pizzol and S. Stoycheva, Safe-and-sustainable-by-design chemicals and advanced materials: a paradigm shift towards prevention-based risk governance is needed, RSC Sustainability, 2023, 1, 838–846 RSC.
- U. Banin, Y. Ben-Shahar and K. Vinokurov, Hybrid semiconductor–metal nanoparticles: from architecture to function, Chem. Mater., 2014, 26, 97–110 CrossRef CAS.
- N. B. Saleh, N. Aich, J. Plazas-Tuttle, J. R. Lead and G. V. Lowry, Research strategy to determine when novel nanohybrids pose unique environmental risks, Environ. Sci.: Nano, 2015, 2, 11–18 RSC.
- R. Gupta and H. Xie, Nanoparticles in daily life: applications, toxicity and regulations, J. Environ. Pathol., Toxicol. Oncol., 2018, 37, 209–230 CrossRef.
- A. E. Nel, L. Mädler, D. Velegol, T. Xia, E. M. Hoek, P. Somasundaran, F. Klaessig, V. Castranova and M. Thompson, Understanding biophysicochemical interactions at the nano–bio interface, Nat. Mater., 2009, 8, 543–557 CrossRef CAS PubMed.
- B. Önal Acet, D. Gül, R. H. Stauber, M. Odabaşı and Ö. Acet, A Review for Uncovering the “Protein-Nanoparticle Alliance”: Implications of the Protein Corona for Biomedical Applications, Nanomaterials, 2024, 14, 823 CrossRef.
- A. J. Chetwynd and I. Lynch, The rise of the nanomaterial metabolite corona, and emergence of the complete corona, Environ. Sci.: Nano, 2020, 7, 1041–1060 RSC.
- M. Tavakol, A. Montazeri, R. Naghdabadi, M. J. Hajipour, S. Zanganeh, G. Caracciolo and M. Mahmoudi, Disease-related metabolites affect protein–nanoparticle interactions, Nanoscale, 2018, 10, 7108–7115 RSC.
- S. S. Raesch, S. Tenzer, W. Storck, A. Rurainski, D. Selzer, C. A. Ruge, J. Perez-Gil, U. F. Schaefer and C.-M. Lehr, Proteomic and lipidomic analysis of nanoparticle corona upon contact with lung surfactant reveals differences in protein, but not lipid composition, ACS Nano, 2015, 9, 11872–11885 CrossRef CAS.
- E. Clemente, M. Martinez-Moro, D. N. Trinh, M. G. Soliman, D. I. Spencer, R. A. Gardner, M. Kotsias, A. S. Iglesias, S. Moya and M. P. Monopoli, Probing the glycans accessibility in the nanoparticle biomolecular corona, J. Colloid Interface Sci., 2022, 613, 563–574 CrossRef CAS.
- D. N. Trinh, R. A. Gardner, A. N. Franciosi, C. McCarthy, M. P. Keane, M. G. Soliman, J. S. O'Donnell, P. Meleady, D. I. Spencer and M. P. Monopoli, Nanoparticle biomolecular corona-based enrichment of plasma glycoproteins for N-glycan profiling and application in biomarker discovery, ACS Nano, 2022, 16, 5463–5475 CrossRef CAS PubMed.
- C. Buzea, I. I. Pacheco and K. Robbie, Nanomaterials and nanoparticles: sources and toxicity, Biointerphases, 2007, 2, MR17–MR71 CrossRef.
- T. Cedervall, I. Lynch, S. Lindman, T. Berggård, E. Thulin, H. Nilsson, K. A. Dawson and S. Linse, Understanding the nanoparticle–protein corona using methods to quantify exchange rates and affinities of proteins for nanoparticles, Proc. Natl. Acad. Sci. U. S. A., 2007, 104, 2050–2055 CrossRef CAS.
- C. D. Walkey and W. C. Chan, Understanding and controlling the interaction of nanomaterials with proteins in a physiological environment, Chem. Soc. Rev., 2012, 41, 2780–2799 RSC.
- R. Bilardo, F. Traldi, A. Vdovchenko and M. Resmini, Influence of surface chemistry and morphology of nanoparticles on protein corona formation, Wiley Interdiscip. Rev.: Nanomed. Nanobiotechnol., 2022, 14, e1788 CAS.
- I. Fenoglio, B. Fubini, E. M. Ghibaudi and F. Turci, Multiple aspects of the interaction of biomacromolecules with inorganic surfaces, Adv. Drug Delivery Rev., 2011, 63, 1186–1209 CrossRef CAS PubMed.
- O. Vilanova, J. J. Mittag, P. M. Kelly, S. Milani, K. A. Dawson, J. O. Rädler and G. Franzese, Understanding the Kinetics of Protein–Nanoparticle Corona Formation, ACS Nano, 2016, 10, 10842–10850 CrossRef CAS.
- M. P. Monopoli, D. Walczyk, A. Campbell, G. Elia, I. Lynch, F. Baldelli Bombelli and K. A. Dawson, Physical− chemical aspects of protein corona: relevance to in vitro and in vivo biological impacts of nanoparticles, J. Am. Chem. Soc., 2011, 133, 2525–2534 CrossRef CAS.
- P. Vilaseca, K. A. Dawson and G. Franzese, Understanding and modulating the competitive surface-adsorption of proteins through coarse-grained molecular dynamics simulations, Soft Matter, 2013, 9, 6978–6985 RSC.
- K. A. Dawson and Y. Yan, Current understanding of biological identity at the nanoscale and future prospects, Nat. Nanotechnol., 2021, 16, 229–242 CrossRef CAS.
- M. P. Monopoli, C. Aberg, A. Salvati and K. A. Dawson, Biomolecular coronas provide the biological identity of nanosized materials, Nano-Enabled Med. Appl., 2020, 205–229 Search PubMed.
- S. Milani, F. Baldelli Bombelli, A. S. Pitek, K. A. Dawson and J. Radler, Reversible versus irreversible binding of transferrin to polystyrene nanoparticles: soft and hard corona, ACS Nano, 2012, 6, 2532–2541 CrossRef CAS.
- E. Casals and V. F. Puntes, Inorganic nanoparticle biomolecular corona: formation, evolution and biological impact, Nanomedicine, 2012, 7, 1917–1930 CrossRef CAS PubMed.
- E. I. Buzas, Opportunities and challenges in studying the extracellular vesicle corona, Nat. Cell Biol., 2022, 24, 1322–1325 CrossRef CAS.
- O. K. Kari, J. Ndika, P. Parkkila, A. Louna, T. Lajunen, A. Puustinen, T. Viitala, H. Alenius and A. Urtti, In situ analysis of liposome hard and soft protein corona structure and composition in a single label-free workflow, Nanoscale, 2020, 12, 1728–1741 RSC.
- A. Salvati, The biomolecular corona of nanomedicines: effects on nanomedicine outcomes and emerging opportunities, Curr. Opin. Biotechnol., 2024, 87, 103101 CrossRef CAS PubMed.
- R. Bilardo, F. Traldi, A. Vdovchenko and M. Resmini, Influence of surface chemistry and morphology of nanoparticles on protein corona formation, Wiley Interdiscip. Rev.: Nanomed. Nanobiotechnol., 2022, 14, e1788 CAS.
- I. Vaskan, V. Dimitreva, M. Petoukhov, E. Shtykova, N. Bovin, A. Tuzikov, M. Tretyak, V. Oleinikov and A. Zalygin, Effect of ligand and shell densities on the surface structure of core–shell nanoparticles self-assembled from function–spacer–lipid constructs, Biomater. Sci., 2024, 12, 798–806 RSC.
- E. Padín-González, P. Lancaster, M. Bottini, P. Gasco, L. Tran, B. Fadeel, T. Wilkins and M. P. Monopoli, Understanding the role and impact of poly (ethylene glycol)(PEG) on nanoparticle formulation: Implications for COVID-19 vaccines, Front. Bioeng. Biotechnol., 2022, 10, 882363 CrossRef PubMed.
- M. Barz, W. J. Parak and R. Zentel, Concepts and Approaches to Reduce or Avoid Protein Corona Formation on Nanoparticles: Challenges and Opportunities, Adv. Sci., 2024, 2402935 CrossRef PubMed.
- J. S. Gebauer, M. Malissek, S. Simon, S. K. Knauer, M. Maskos, R. H. Stauber, W. Peukert and L. Treuel, Impact of the nanoparticle–protein corona on colloidal stability and protein structure, Langmuir, 2012, 28, 9673–9679 CrossRef CAS PubMed.
- S. Lara, F. Alnasser, E. Polo, D. Garry, M. C. Lo Giudice, D. R. Hristov, L. Rocks, A. Salvati, Y. Yan and K. A. Dawson, Identification of receptor binding to the biomolecular corona of nanoparticles, ACS Nano, 2017, 11, 1884–1893 CrossRef CAS PubMed.
- P. M. Kelly, C. Åberg, E. Polo, A. O'connell, J. Cookman, J. Fallon, Ž. Krpetić and K. A. Dawson, Mapping protein binding sites on the biomolecular corona of nanoparticles, Nat. Nanotechnol., 2015, 10, 472–479 CrossRef CAS PubMed.
- A. Marucco, F. Turci, L. O'Neill, H. J. Byrne, B. Fubini and I. Fenoglio, Hydroxyl density affects the interaction of fibrinogen with silica nanoparticles at physiological concentration, J. Colloid Interface Sci., 2014, 419, 86–94 CrossRef CAS PubMed.
- A. Marucco, E. Gazzano, D. Ghigo, E. Enrico and I. Fenoglio, Fibrinogen enhances the inflammatory response of alveolar macrophages to TiO2, SiO2 and carbon nanomaterials, Nanotoxicology, 2016, 10, 1–9 CAS.
- Z. J. Deng, M. Liang, M. Monteiro, I. Toth and R. F. Minchin, Nanoparticle-induced unfolding of fibrinogen promotes Mac-1 receptor activation and inflammation, Nat. Nanotechnol., 2011, 6, 39–44 CrossRef CAS.
- B. Wang, E. H. Pilkington, Y. Sun, T. P. Davis, P. C. Ke and F. Ding, Modulating protein amyloid aggregation with nanomaterials, Environ. Sci.: Nano, 2017, 4, 1772–1783 RSC.
- D. Walczyk, F. B. Bombelli, M. P. Monopoli, I. Lynch and K. A. Dawson, What the cell “sees” in bionanoscience, J. Am. Chem. Soc., 2010, 132, 5761–5768 CrossRef CAS PubMed.
- A. Marucco, E. Aldieri, R. Leinardi, E. Bergamaschi, C. Riganti and I. Fenoglio, Applicability and limitations in the characterization of poly-dispersed engineered nanomaterials in cell media by dynamic light scattering (DLS), Materials, 2019, 12, 3833 CrossRef CAS.
- C. Ge, J. Du, L. Zhao, L. Wang, Y. Liu, D. Li, Y. Yang, R. Zhou, Y. Zhao and Z. Chai, Binding of blood proteins to carbon nanotubes reduces cytotoxicity, Proc. Natl. Acad. Sci. U. S. A., 2011, 108, 16968–16973 CrossRef CAS PubMed.
- S. Behzadi, V. Serpooshan, W. Tao, M. A. Hamaly, M. Y. Alkawareek, E. C. Dreaden, D. Brown, A. M. Alkilany, O. C. Farokhzad and M. Mahmoudi, Cellular uptake of nanoparticles: journey inside the cell, Chem. Soc. Rev., 2017, 46, 4218–4244 RSC.
- N. Mei, J. Hedberg, I. Odnevall Wallinder and E. Blomberg, Influence of biocorona formation on the transformation and dissolution of cobalt nanoparticles under physiological conditions, ACS Omega, 2019, 4, 21778–21791 CrossRef CAS PubMed.
- A. Seaton, L. Tran, R. Aitken and K. Donaldson, Nanoparticles, human health hazard and regulation, J. R. Soc., Interface, 2010, 7, S119–S129 CrossRef CAS.
- K. Donaldson, L. Tran, L. A. Jimenez, R. Duffin, D. E. Newby, N. Mills, W. MacNee and V. Stone, Combustion-derived nanoparticles: a review of their toxicology following inhalation exposure, Part. Fibre Toxicol., 2005, 2, 1–14 CrossRef.
- M. R. Miller, C. A. Shaw and J. P. Langrish, From particles to patients: oxidative stress and the cardiovascular effects of air pollution, Future Cardiol., 2012, 8, 577–602 CrossRef CAS.
- M. R. Miller, J. B. Raftis, J. P. Langrish, S. G. McLean, P. Samutrtai, S. P. Connell, S. Wilson, A. T. Vesey, P. H. B. Fokkens, A. J. F. Boere, P. Krystek, C. J. Campbell, P. W. F. Hadoke, K. Donaldson, F. R. Cassee, D. E. Newby, R. Duffin and N. L. Mills, Inhaled nanoparticles accumulate at sites of vascular disease, ACS Nano, 2017, 11, 4542–4552 CrossRef CAS PubMed.
- I. Fizeşan, A. Chary, S. Cambier, E. Moschini, T. Serchi, I. Nelissen, B. Kiss, A. Pop, F. Loghin and A. C. Gutleb, Responsiveness assessment of a 3D tetra-culture alveolar model exposed to diesel exhaust particulate matter, Toxicol. In Vitro, 2018, 53, 67–79 CrossRef.
- S. G. Klein, S. Cambier, J. Hennen, S. Legay, T. Serchi, I. Nelissen, A. Chary, E. Moschini, A. Krein and B. Blömeke, Endothelial responses of the alveolar barrier in vitro in a dose-controlled exposure to diesel exhaust particulate matter, Part. Fibre Toxicol., 2017, 14, 1–17 CrossRef.
- M. G. Bianchi, M. Allegri, M. Chiu, A. L. Costa, M. Blosi, S. Ortelli, O. Bussolati and E. Bergamaschi, Lipopolysaccharide adsorbed to the bio-Corona of TiO2 nanoparticles powerfully activates selected pro-inflammatory transduction pathways, Front. Immunol., 2017, 8, 866 CrossRef.
-
I. Lynch, K. A. Dawson, J. R. Lead and E. Valsami-Jones, in Frontiers of nanoscience, Elsevier, 2014, vol. 7, pp. 127–156 Search PubMed.
- K. E. Wheeler, A. J. Chetwynd, K. M. Fahy, B. S. Hong, J. A. Tochihuitl, L. A. Foster and I. Lynch, Environmental dimensions of the protein corona, Nat. Nanotechnol., 2021, 16, 617–629 CrossRef CAS PubMed.
- F. Nasser and I. Lynch, Secreted protein eco-corona mediates uptake and impacts of polystyrene nanoparticles on Daphnia magna, J. Proteomics, 2016, 137, 45–51 CrossRef CAS.
- L. Natarajan, S. Omer, N. Jetly, M. A. Jenifer, N. Chandrasekaran, G. Suraishkumar and A. Mukherjee, Eco-corona formation lessens the toxic effects of polystyrene nanoplastics towards marine microalgae Chlorella sp, Environ. Res., 2020, 188, 109842 CrossRef CAS.
- S. Liu, M. Junaid, H. Liao, X. Liu, Y. Wu and J. Wang, Eco-corona formation and associated ecotoxicological impacts of nanoplastics in the environment, Sci. Total Environ., 2022, 836, 155703 CrossRef CAS PubMed.
- S. Liu, X. Zhang, K. Zeng, C. He, Y. Huang, G. Xin and X. Huang, Insights into eco-corona formation and its role in the biological effects of nanomaterials from a molecular mechanisms perspective, Sci. Total Environ., 2023, 858, 159867 CrossRef CAS.
- C. P. Wild, Complementing the genome with an “exposome”: the outstanding challenge of environmental exposure measurement in molecular epidemiology, Cancer Epidemiol., Biomarkers Prev., 2005, 14, 1847–1850 CrossRef CAS.
- A. E. Goode, J. M. Perkins, A. Sandison, C. Karunakaran, H. Cheng, D. Wall, J. A. Skinner, A. J. Hart, A. E. Porter and D. W. McComb, Chemical speciation of nanoparticles surrounding metal-on-metal hips, Chem. Commun., 2012, 48, 8335–8337 RSC.
-
E. R. Weibel, A. F. Cournand and D. W. Richards, Morphometry of the human lung, Springer, 1963 Search PubMed.
- L. Knudsen and M. Ochs, The micromechanics of lung alveoli: structure and function of surfactant and tissue components, Histochem. Cell Biol., 2018, 150, 661–676 CrossRef CAS PubMed.
-
G. Hatch, Comparative biochemistry of airway lining fluid, Treatise on Pulmonary Toxicology, 1992, vol. 1, pp. 617–632 Search PubMed.
- C. A. Ruge, U. F. Schaefer, J. Herrmann, J. Kirch, O. Canadas, M. Echaide, J. Perez-Gil, C. Casals, R. Müller and C.-M. Lehr, The interplay of lung surfactant proteins and lipids assimilates the macrophage clearance of nanoparticles, PLoS One, 2012, 7, e40775 CrossRef CAS PubMed.
- E. Parra, A. Alcaraz, A. Cruz, V. M. Aguilella and J. Pérez-Gil, Hydrophobic pulmonary surfactant proteins SP-B and SP-C induce pore formation in planar lipid membranes: evidence for proteolipid pores, Biophys. J., 2013, 104, 146–155 CrossRef CAS.
- H. Ren, Y. Yu and T. An, Bioaccessibilities of metal (loid) s and organic contaminants in particulates measured in simulated human lung fluids: A critical review, Environ. Pollut., 2020, 265, 115070 CrossRef CAS.
-
E. M. Bicer, Compositional characterisation of human respiratory tractlining fluids for the design of disease specific simulants, PhD Thesis, King's College London, 2015 Search PubMed.
-
B. Palen and R. Helmers, Bronchoalveolar lavage, Flexible Bronchoscopy, 2012, pp. 132–146 Search PubMed.
- R. P. Baughman, Technical aspects of bronchoalveolar lavage: recommendations for a standard procedure, Seminars in Respiratory and Critical Care Medicine, 2007, 28, 475–485 CrossRef PubMed.
- R. Bastola, P. M. Young and S. C. Das, Simulation of respiratory tract lining fluid for in vitro dissolution study, Expert Opin. Drug Delivery, 2021, 18, 1091–1100 CrossRef CAS.
- E. Innes, H. H. Yiu, P. McLean, W. Brown and M. Boyles, Simulated biological fluids–a systematic review of their biological relevance and use in relation to inhalation toxicology of particles and fibres, Crit. Rev. Toxicol., 2021, 51, 217–248 CrossRef CAS.
- K. Corin and J. U. Bowie, How bilayer properties influence membrane protein folding, Protein Sci., 2020, 29, 2348–2362 CrossRef CAS PubMed.
- J. E. Horne, D. J. Brockwell and S. E. Radford, Role of the lipid bilayer in outer membrane protein folding in Gram-negative bacteria, J. Biol. Chem., 2020, 295, 10340–10367 CrossRef CAS.
- D. Li, X. Wang, Y. Liao, S. Wang, J. Shan and J. Ji, Insights Gained Into the Treatment of COVID19 by Pulmonary Surfactant and Its Components, Front. Immunol., 2022, 13, 842453 CrossRef CAS PubMed.
-
A. B. Fisher, in Comparative biology of the normal lung, Elsevier, 2015, pp. 423–466 Search PubMed.
-
J. L. Gamble, Extracellular Fluid. A Lecture Syllabus, Department of Pediatrics, Harvard Medical School, 1942 Search PubMed.
- O. R. Moss, Simulants of lung interstitial fluid, Health Phys., 1979, 36, 447–448 CAS.
- N. Boisa, N. Elom, J. R. Dean, M. E. Deary, G. Bird and J. A. Entwistle, Development and application of an inhalation bioaccessibility method (IBM) for lead in the PM10 size fraction of soil, Environ. Int., 2014, 70, 132–142 CrossRef CAS PubMed.
- G. Kanapilly, O. Raabe, C. Goh and R. Chimenti, Measurement of in vitro dissolution of aerosol particles for comparison to in vivo dissolution in the lower respiratory tract after inhalation, Health Phys., 1973, 24, 497–507 CrossRef CAS.
- N. A. Dennis, M. H. Blauer and J. E. Kent, Dissolution fractions and half-times of single source yellowcake in simulated lung fluids, Health Phys., 1982, 42, 469–477 CrossRef CAS.
- B. Berlinger, D. G. Ellingsen, M. Náray, G. Záray and Y. Thomassen, A study of the bio-accessibility of welding fumes, J. Environ. Monit., 2008, 10, 1448–1453 RSC.
- A. Kumar, W. Terakosolphan, M. Hassoun, K.-K. Vandera, A. Novicky, R. Harvey, P. G. Royall, E. M. Bicer, J. Eriksson and K. Edwards, A biocompatible synthetic lung fluid based on human respiratory tract lining fluid composition, Pharm. Res., 2017, 34, 2454–2465 CrossRef CAS.
- W. Yang, J. I. Peters and R. O. Williams III, Inhaled nanoparticles—a current review, Int. J. Pharm., 2008, 356, 239–247 CrossRef CAS.
- M. G. Soliman, D. N. Trinh, C. Ravagli, P. Meleady, M. Henry, D. Movia, S. Doumett, L. Cappiello, A. Prina-Mello, G. Baldi and M. P. Monopoli, Development of a fast and simple method for the isolation of superparamagnetic iron oxide nanoparticles protein corona from protein-rich matrices, J. Colloid Interface Sci., 2024, 659, 503–519 CrossRef CAS.
- N. V. Konduru, R. M. Molina, A. Swami, F. Damiani, G. Pyrgiotakis, P. Lin, P. Andreozzi, T. C. Donaghey, P. Demokritou and S. Krol, Protein corona: implications for nanoparticle interactions with pulmonary cells, Part. Fibre Toxicol., 2017, 14, 1–12 CrossRef.
- T. Praphawatvet, J. I. Peters and R. O. Williams III, Inhaled nanoparticles–An updated review, Int. J. Pharm., 2020, 587, 119671 CrossRef CAS.
- S. Shahabi, L. Treccani, R. Dringen and K. Rezwan, Utilizing the protein corona around silica nanoparticles for dual drug loading and release, Nanoscale, 2015, 7, 16251–16265 RSC.
- S. Sonwani, S. Madaan, J. Arora, S. Suryanarayan, D. Rangra, N. Mongia, T. Vats and P. Saxena, Inhalation exposure to atmospheric nanoparticles and its associated impacts on human
health: a review, Front. Sustain. Cities., 2021, 3, 690444 CrossRef.
- A. Taylor, J. Fuzi, A. Sideris, C. Banks and T. Havas, Non-steroid, non-antibiotic anti-biofilm therapy for the treatment of chronic rhinosinusitis: a systematic review, J. Laryngol. Otol., 2021, 135, 196–205 CrossRef CAS.
- A. K. Madl, L. E. Plummer, C. Carosino and K. E. Pinkerton, Nanoparticles, lung injury, and the role of oxidant stress, Annu. Rev. Physiol., 2014, 76, 447–465 CrossRef CAS.
- P. Andujar, S. Lanone, P. Brochard and J. Boczkowski, Respiratory effects of manufactured nanoparticles, Rev. Mal. Respir., 2011, 28, e66–e75 CrossRef CAS.
- J. Löndahl, W. Möller, J. H. Pagels, W. G. Kreyling, E. Swietlicki and O. Schmid, Measurement techniques for respiratory tract deposition of airborne nanoparticles: a critical review, J. Aerosol Med. Pulm. Drug Delivery, 2014, 27, 229–254 CrossRef PubMed.
- H. Qiao, W. Liu, H. Gu, D. Wang and Y. Wang, The transport and deposition of nanoparticles in respiratory system by inhalation, J. Nanomater., 2015, 2015, 2 Search PubMed.
- S. Bakand, A. Hayes and F. Dechsakulthorn, Nanoparticles: a review of particle toxicology following inhalation exposure, Inhalation Toxicol., 2012, 24, 125–135 CrossRef CAS.
- E. Fröhlich and S. Salar-Behzadi, Toxicological assessment of inhaled nanoparticles: role of in vivo, ex vivo, in vitro, and in silico studies, Int. J. Mol. Sci., 2014, 15, 4795–4822 CrossRef.
- R. A. Cone, Barrier properties of mucus, Adv. Drug Delivery Rev., 2009, 61, 75–85 CrossRef CAS PubMed.
- B. S. Schuster, J. S. Suk, G. F. Woodworth and J. Hanes, Nanoparticle diffusion in respiratory mucus from humans without lung disease, Biomaterials, 2013, 34, 3439–3446 CrossRef CAS PubMed.
- J. McCarthy, I. Inkielewicz-Stepniak, J. J. Corbalan and M. W. Radomski, Mechanisms of toxicity of amorphous silica nanoparticles on human lung submucosal cells in vitro: protective effects of fisetin, Chem. Res. Toxicol., 2012, 25, 2227–2235 Search PubMed.
- J. Y. Kasper, L. Feiden, M. I. Hermanns, C. Bantz, M. Maskos, R. E. Unger and C. J. Kirkpatrick, Pulmonary surfactant augments cytotoxicity of silica nanoparticles: Studies on an in vitro air–blood barrier model, Beilstein J. Nanotechnol., 2015, 6, 517–528 Search PubMed.
- M. Gasser, B. Rothen-Rutishauser, H. F. Krug, P. Gehr, M. Nelle, B. Yan and P. Wick, The adsorption of biomolecules to multi-walled carbon nanotubes is influenced by both pulmonary surfactant lipids and surface chemistry, J. Nanobiotechnol., 2010, 8, 1–9 CrossRef PubMed.
- M. Gasser, P. Wick, M. J. Clift, F. Blank, L. Diener, B. Yan, P. Gehr, H. F. Krug and B. Rothen-Rutishauser, Pulmonary surfactant coating of multi-walled carbon nanotubes (MWCNTs) influences their oxidative and pro-inflammatory potential in vitro, Part. Fibre Toxicol., 2012, 9, 1–13 CrossRef.
- J. Heyder, J. Gebhart, G. Rudolf, C. F. Schiller and W. Stahlhofen, Deposition of particles in the human respiratory tract in the size range 0.005–15 μm, J. Aerosol Sci., 1986, 17, 811–825 CrossRef.
- J. C. Sung, B. L. Pulliam and D. A. Edwards, Nanoparticles for drug delivery to the lungs, Trends Biotechnol., 2007, 25, 563–570 CrossRef CAS.
- A. A. Shvedova, E. R. Kisin, R. Mercer, A. R. Murray, V. J. Johnson, A. I. Potapovich, Y. Y. Tyurina, O. Gorelik, S. Arepalli and D. Schwegler-Berry, Unusual inflammatory and fibrogenic pulmonary responses to single-walled carbon nanotubes in mice, Am. J. Physiol., 2005, 289, L698–L708 CAS.
- V. H. Grassian, P. T. O'Shaughnessy, A. Adamcakova-Dodd, J. M. Pettibone and P. S. Thorne, Inhalation exposure study of titanium dioxide nanoparticles with a primary particle size of 2 to 5 nm, Environ. Health Perspect., 2007, 115, 397–402 CrossRef CAS.
- W. G. Kreyling, S. Hirn, W. Moller, C. Schleh, A. Wenk, G. l. Celik, J. Lipka, M. Schäffler, N. Haberl and B. D. Johnston, Air–blood barrier translocation of tracheally instilled gold nanoparticles inversely depends on particle size, ACS Nano, 2014, 8, 222–233 CrossRef CAS PubMed.
- W. G. Kreyling, M. Semmler-Behnke, J. Seitz, W. Scymczak, A. Wenk, P. Mayer, S. Takenaka and G. Oberdörster, Size dependence of the translocation of inhaled iridium and carbon nanoparticle aggregates from the lung of rats to the blood and secondary target organs, Inhalation Toxicol., 2009, 21, 55–60 CrossRef CAS.
- H. S. Choi, Y. Ashitate, J. H. Lee, S. H. Kim, A. Matsui, N. Insin, M. G. Bawendi, M. Semmler-Behnke, J. V. Frangioni and A. Tsuda, Rapid translocation of nanoparticles from the lung airspaces to the body, Nat. Biotechnol., 2010, 28, 1300–1303 CrossRef CAS PubMed.
- J. R. Scott, R. Krishnan, B. W. Rotenberg and L. J. Sowerby, The effectiveness of topical colloidal silver in recalcitrant chronic rhinosinusitis: a randomized crossover control trial, J. Otolaryngol Head Neck Surg., 2017, 46, 64 CrossRef.
- N. Liu, Y. Li, L. Liu, X. Liu, Y. Yin, G. Qu, J. Shi, M. Song, B. He and L. Hu, Administration of silver nasal spray leads to nanoparticle accumulation in rat brain tissues, Environ. Sci. Technol., 2021, 56, 403–413 CrossRef.
- Y.-S. Kim, Y.-H. Chung, D.-S. Seo, H.-S. Choi and C.-H. Lim, Twenty-eight-day repeated inhalation toxicity study of aluminum oxide nanoparticles in male Sprague-Dawley rats, Toxicol. Res., 2018, 34, 343–354 CrossRef CAS.
- K. H. Shim, J. Hulme, E. H. Maeng, M.-K. Kim and S. S. A. An, Analysis of SiO2 nanoparticles binding proteins in rat blood and brain homogenate, Int. J. Nanomed., 2014, 9, 207–215 Search PubMed.
- M. Hilty, C. Burke, H. Pedro, P. Cardenas, A. Bush, C. Bossley, J. Davies, A. Ervine, L. Poulter and L. Pachter, Disordered microbial communities in asthmatic airways, PLoS One, 2010, 5, e8578 CrossRef PubMed.
- H. Wu, A. Kuzmenko, S. Wan, L. Schaffer, A. Weiss, J. H. Fisher, K. S. Kim and F. X. McCormack, Surfactant proteins A and D inhibit the growth of Gram-negative bacteria by increasing membrane permeability, J. Clin. Invest., 2003, 111, 1589–1602 CrossRef CAS PubMed.
- G. Huffnagle, R. Dickson and N. Lukacs, The respiratory tract microbiome and lung inflammation: a two-way street, Mucosal Immunol., 2017, 10, 299–306 CrossRef CAS PubMed.
- K. Yagi, G. B. Huffnagle, N. W. Lukacs and N. Asai, The lung microbiome during health and disease, Int. J. Mol. Sci., 2021, 22, 10872 CrossRef CAS.
- T. Y. Poh, N. A. B. M. Ali, M. Mac Aogáin, M. H. Kathawala, M. I. Setyawati, K. W. Ng and S. H. Chotirmall, Inhaled nanomaterials and the respiratory microbiome: clinical, immunological and toxicological perspectives, Part. Fibre Toxicol., 2018, 15, 1–16 CrossRef PubMed.
- M. Moradi, R. Razavi, A. K. Omer, A. Farhangfar and D. J. McClements, Interactions between nanoparticle-based food additives and other food ingredients: A review of current knowledge, Trends Food Sci. Technol., 2022, 120, 75–87 CrossRef CAS.
- E. Fröhlich and E. Roblegg, Models for oral uptake of nanoparticles in consumer products, Toxicology, 2012, 291, 10–17 CrossRef.
- Y. Echegoyen and C. Nerín, Nanoparticle release from nano-silver antimicrobial food containers, Food Chem. Toxicol., 2013, 62, 16–22 CrossRef CAS PubMed.
- I. L. Bergin and F. A. Witzmann, Nanoparticle toxicity by the gastrointestinal route: evidence and knowledge gaps, Int. J. Biomed. Nanosci. Nanotechnol., 2013, 3, 163–210 CrossRef CAS PubMed.
- T. A. Volsko, Airway clearance therapy: finding the evidence, Respir. Care, 2013, 58, 1669–1678 CrossRef PubMed.
- S. Bellmann, D. Carlander, A. Fasano, D. Momcilovic, J. A. Scimeca, W. J. Waldman, L. Gombau, L. Tsytsikova, R. Canady and D. I. Pereira, Mammalian gastrointestinal tract parameters modulating the integrity, surface properties, and absorption of food-relevant nanomaterials, Wiley Interdiscip. Rev.: Nanomed. Nanobiotechnol., 2015, 7, 609–622 CAS.
-
B. Greenwood-Van Meerveld, A. C. Johnson and D. Grundy, Gastrointestinal physiology and function, Gastrointestinal Pharmacology, in Handbook of Experimental Pharmacology, ed. B. Greenwood-Van Meerveld, 2017, vol. 239, pp. 1–16 Search PubMed.
- M. E. Johansson, J. M. H. Larsson and G. C. Hansson, The two mucus layers of colon are organized by the MUC2 mucin, whereas the outer layer is a legislator of host–microbial interactions, Proc. Natl. Acad. Sci. U. S. A., 2011, 108, 4659–4665 CrossRef CAS PubMed.
- J. C. Clemente, L. K. Ursell, L. W. Parfrey and R. Knight, The impact of the gut microbiota on human health: an integrative view, Cell, 2012, 148, 1258–1270 CrossRef CAS PubMed.
-
W. F. Ganong, Review of medical physiology, Dynamics of blood lymph flow, 1995, vol. 30, pp. 525–541 Search PubMed.
- T. Hoyle, The digestive system: linking theory and practice, Br. J. Nurs., 1997, 6, 1285–1291 CrossRef CAS PubMed.
- K. R. Groschwitz and S. P. Hogan, Intestinal barrier function: molecular regulation and disease pathogenesis, J. Allergy Clin. Immunol., 2009, 124, 3–20 CrossRef CAS PubMed.
- J. M. Anderson and C. M. Van Itallie, Physiology and function of the tight junction, Cold Spring Harbor Perspect. Biol., 2009, 1, a002584 Search PubMed.
- J. R. Turner, Intestinal mucosal barrier function in health and disease, Nat. Rev. Immunol., 2009, 9, 799–809 CrossRef CAS PubMed.
- B. R. Stevenson, J. D. Siliciano, M. S. Mooseker and D. A. Goodenough, Identification of ZO-1: a high molecular weight polypeptide associated with the tight junction (zonula occludens) in a variety of epithelia, J. Cell Biol., 1986, 103, 755–766 CrossRef CAS PubMed.
- S. Tsukita, M. Furuse and M. Itoh, Multifunctional strands in tight junctions, Nat. Rev. Mol. Cell Biol., 2001, 2, 285–293 CrossRef CAS PubMed.
- K. Dokladny, M. N. Zuhl and P. L. Moseley, Molecular Adaptations to Exercise, Heat Acclimation, and Thermotolerance: Intestinal epithelial barrier function and tight junction proteins with heat and exercise, J. Appl. Physiol., 2016, 120, 692 CrossRef CAS PubMed.
- A. Fasano, B. Baudry, D. W. Pumplin, S. S. Wasserman, B. D. Tall, J. M. Ketley and J. Kaper, Vibrio cholerae produces a second enterotoxin, which affects intestinal tight junctions, Proc. Natl. Acad. Sci. U. S. A., 1991, 88, 5242–5246 CrossRef CAS PubMed.
- D. E. Shifflett, D. R. Clayburgh, A. Koutsouris, J. R. Turner and G. A. Hecht, Enteropathogenic E. coli disrupts tight junction barrier function and structure in vivo, Lab. Invest., 2005, 85, 1308–1324 CrossRef CAS PubMed.
- D. J. Philpott, D. M. McKay, W. Mak, M. H. Perdue and P. M. Sherman, Signal transduction pathways involved in enterohemorrhagic Escherichia coli-induced alterations in T84 epithelial permeability, Infect. Immun., 1998, 66, 1680–1687 CrossRef CAS PubMed.
- R. Cornu, C. Chrétien, Y. Pellequer, H. Martin and A. Béduneau, Small silica nanoparticles transiently modulate the intestinal permeability by actin cytoskeleton disruption in both Caco-2 and Caco-2/HT29-MTX models, Arch. Toxicol., 2020, 94, 1191–1202 CrossRef CAS PubMed.
- P. Pedata, G. Ricci, L. Malorni, A. Venezia, M. Cammarota, M. G. Volpe, N. Iannaccone, V. Guida, C. Schiraldi and M. Romano, In vitro intestinal epithelium responses to titanium dioxide nanoparticles, Food Res. Int., 2019, 119, 634–642 CrossRef CAS PubMed.
- W. W. Agace, T-cell recruitment to the intestinal mucosa, Trends Immunol., 2008, 29, 514–522 CrossRef CAS PubMed.
- A. J. Macpherson and T. Uhr, Induction of protective IgA by intestinal dendritic cells carrying commensal bacteria, Science, 2004, 303, 1662–1665 CrossRef CAS PubMed.
- S. Voedisch, C. Koenecke, S. David, H. Herbrand, R. Förster, M. Rhen and O. Pabst, Mesenteric lymph nodes confine dendritic cell-mediated dissemination of Salmonella enterica serovar Typhimurium and limit systemic disease in mice, Infect. Immun., 2009, 77, 3170–3180 CrossRef CAS PubMed.
- R. M. Steinman, Decisions about dendritic cells: past, present, and future, Annu. Rev. Immunol., 2012, 30, 1–22 CrossRef CAS PubMed.
- R. M. Steinman, Dendritic cells: understanding immunogenicity, Eur. J. Immunol., 2007, 37, S53–S60 CrossRef CAS PubMed.
- U. Hadis, B. Wahl, O. Schulz, M. Hardtke-Wolenski, A. Schippers, N. Wagner, W. Müller, T. Sparwasser, R. Förster and O. Pabst, Intestinal tolerance requires gut homing and expansion of FoxP3+ regulatory T cells in the lamina propria, Immunity, 2011, 34, 237–246 CrossRef CAS PubMed.
- A. M. Platt and A. M. Mowat, Mucosal macrophages and the regulation of immune responses in the intestine, Immunol. Lett., 2008, 119, 22–31 CrossRef CAS PubMed.
- T. L. Denning, Y.-c. Wang, S. R. Patel, I. R. Williams and B. Pulendran, Lamina propria macrophages and dendritic cells differentially induce regulatory and interleukin 17–producing T cell responses, Nat. Immunol., 2007, 8, 1086–1094 CrossRef CAS PubMed.
- A. M. Platt, C. C. Bain, Y. Bordon, D. P. Sester and A. M. Mowat, An independent subset of TLR expressing CCR2-dependent macrophages promotes colonic inflammation, J. Immunol., 2010, 184, 6843–6854 CrossRef CAS PubMed.
- A. Cerutti, The regulation of IgA class switching, Nat. Rev. Immunol., 2008, 8, 421–434 CrossRef CAS PubMed.
- F. D. Batista and N. E. Harwood, The who, how and where of antigen presentation to B cells, Nat. Rev. Immunol., 2009, 9, 15–27 CrossRef CAS PubMed.
- M. R. Neutra, N. J. Mantis and J.-P. Kraehenbuhl, Collaboration of epithelial cells with organized mucosal lymphoid tissues, Nat. Immunol., 2001, 2, 1004–1009 CrossRef CAS PubMed.
- M. R. Neutra, E. Pringault and J.-P. Kraehenbuhl, Antigen sampling across epithelial barriers and induction of mucosal immune responses, Annu. Rev. Immunol., 1996, 14, 275–300 CrossRef CAS PubMed.
- R. L. Owen, Uptake and transport of intestinal macromolecules and microorganisms by M cells in Peyer's patches—a personal and historical perspective, Semin. Immunol., 1999, 11(3), 157–163 CrossRef CAS PubMed.
- E. Patterson, J. F. Cryan, G. F. Fitzgerald, R. P. Ross, T. G. Dinan and C. Stanton, Gut microbiota, the pharmabiotics they produce and host health, Proc. Nutr. Soc., 2014, 73, 477–489 CrossRef CAS PubMed.
- K. V. Sandhu, E. Sherwin, H. Schellekens, C. Stanton, T. G. Dinan and J. F. Cryan, Feeding the microbiota-gut-brain axis: diet, microbiome, and neuropsychiatry, Transl. Res., 2017, 179, 223–244 CrossRef CAS PubMed.
- S. Kong, Y. H. Zhang and W. Zhang, Regulation of intestinal epithelial cells properties and functions by amino acids, BioMed Res. Int., 2018, 2018(1), 2819154 Search PubMed.
- M. Minekus, M. Alminger, P. Alvito, S. Ballance, T. Bohn, C. Bourlieu, F. Carrière, R. Boutrou, M. Corredig and D. Dupont, A standardised static in vitro digestion method suitable for food–an international consensus, Food Funct., 2014, 5, 1113–1124 RSC.
- A. Brodkorb, L. Egger, M. Alminger, P. Alvito, R. Assunção, S. Ballance, T. Bohn, C. Bourlieu-Lacanal, R. Boutrou and F. Carrière, INFOGEST static in vitro simulation of gastrointestinal food digestion, Nat. Protoc., 2019, 14, 991–1014 CrossRef CAS PubMed.
- I. S. Sohal, Y. K. Cho, K. S. O'Fallon, P. Gaines, P. Demokritou and D. Bello, Dissolution behavior and biodurability of ingested engineered nanomaterials in the gastrointestinal environment, ACS Nano, 2018, 12, 8115–8128 CrossRef CAS PubMed.
- G. Antonello, A. Marucco, E. Gazzano, P. Kainourgios, C. Ravagli, A. Gonzalez-Paredes, S. Sprio, E. Padín-González, M. G. Soliman and D. Beal, Changes of physico-chemical properties of nano-biomaterials by digestion fluids affect the physiological properties of epithelial intestinal cells and barrier models, Part. Fibre Toxicol., 2022, 19, 49 CrossRef CAS PubMed.
- H. Bouwmeester, J. Poortman, R. J. Peters, E. Wijma, E. Kramer, S. Makama, K. Puspitaninganindita, H. J. Marvin, A. Peijnenburg and P. J. Hendriksen, Characterization of translocation of silver nanoparticles and effects on whole-genome gene expression using an in vitro intestinal epithelium coculture model, ACS Nano, 2011, 5, 4091–4103 CrossRef CAS PubMed.
- E. Brun, F. Barreau, G. Veronesi, B. Fayard, S. Sorieul, C. Chanéac, C. Carapito, T. Rabilloud, A. Mabondzo and N. Herlin-Boime, Titanium dioxide nanoparticle impact and translocation through ex vivo, in vivo and in vitro gut epithelia, Part. Fibre Toxicol., 2014, 11, 1–16 CrossRef PubMed.
- A. des Rieux, V. Fievez, I. Théate, J. Mast, V. Préat and Y.-J. Schneider, An improved in vitro model of human intestinal follicle-associated epithelium to study nanoparticle transport by M cells, Eur. J. Pharm. Sci., 2007, 30, 380–391 CrossRef CAS PubMed.
- A. des Rieux, E. G. Ragnarsson, E. Gullberg, V. Préat, Y.-J. Schneider and P. Artursson, Transport of nanoparticles across an in vitro model of the human intestinal follicle associated epithelium, Eur. J. Pharm. Sci., 2005, 25, 455–465 CrossRef CAS PubMed.
- E. Gullberg, Å. V. Keita, Y. S. Sa'ad, M. Andersson, K. D. Caldwell, J. D. Söderholm and P. Artursson, Identification of cell adhesion molecules in the human follicle-associated epithelium that improve nanoparticle uptake into the Peyer's patches, J. Pharmacol. Exp. Ther., 2006, 319, 632–639 CrossRef CAS PubMed.
- L. C. Delon, M. Faria, Z. Jia, S. Johnston, R. Gibson, C. A. Prestidge and B. Thierry, Capturing and Quantifying Particle Transcytosis with Microphysiological Intestine-on-Chip Models, Small Methods, 2023, 7, 2200989 CrossRef CAS PubMed.
- R. J. Snyder, S. Hussain, A. B. Rice and S. Garantziotis, Multiwalled carbon nanotubes induce altered morphology and loss of barrier function in human bronchial epithelium at noncytotoxic doses, Int. J. Nanomed., 2014, 4093–4105 CrossRef CAS PubMed.
- L. C. Abbott and A. D. Maynard, Exposure assessment approaches for engineered nanomaterials, Risk Anal., 2010, 30, 1634–1644 CrossRef PubMed.
- G. Oberdörster, A. Maynard, K. Donaldson, V. Castranova, J. Fitzpatrick, K. Ausman, J. Carter, B. Karn, W. Kreyling and D. Lai, Principles for characterizing the potential human health effects from exposure to nanomaterials: elements of a screening strategy, Part. Fibre Toxicol., 2005, 2, 1–35 CrossRef PubMed.
- D. Bernstein, V. Castranova, K. Donaldson, B. Fubini, J. Hadley, T. Hesterberg, A. Kane, D. Lai, E. E. McConnell and H. Muhle, Testing of fibrous particles: short-term assays and strategies: report of an ILSI Risk Science Institute Working Group, Inhalation Toxicol., 2005, 17, 497–537 CrossRef CAS PubMed.
- K. Loeschner, N. Hadrup, K. Qvortrup, A. Larsen, X. Gao, U. Vogel, A. Mortensen, H. R. Lam and E. H. Larsen, Distribution of silver in rats following 28 days of repeated oral exposure to silver nanoparticles or silver acetate, Part. Fibre Toxicol., 2011, 8, 1–14 CrossRef PubMed.
- B. E. Wildt, A. Celedon, E. I. Maurer, B. J. Casey, A. M. Nagy, S. M. Hussain and P. L. Goering, Intracellular accumulation and dissolution of silver nanoparticles in L-929 fibroblast cells using live cell time-lapse microscopy, Nanotoxicology, 2016, 10, 710–719 CrossRef CAS PubMed.
- P. Jani, G. W. Halbert, J. Langridge and A. T. Florence, Nanoparticle uptake by the rat gastrointestinal mucosa: quantitation and particle size dependency, J. Pharm. Pharmacol., 1990, 42, 821–826 CrossRef CAS PubMed.
- J. L. Axson, D. I. Stark, A. L. Bondy, S. S. Capracotta, A. D. Maynard, M. A. Philbert, I. L. Bergin and A. P. Ault, Rapid kinetics of size and pH-dependent dissolution and aggregation of silver nanoparticles in simulated gastric fluid, J. Phys. Chem. C, 2015, 119, 20632–20641 CrossRef CAS PubMed.
- A. P. Ault, D. I. Stark, J. L. Axson, J. N. Keeney, A. D. Maynard, I. L. Bergin and M. A. Philbert, Protein corona-induced modification of silver nanoparticle aggregation in simulated gastric fluid, Environ. Sci.: Nano, 2016, 3, 1510–1520 RSC.
- K. Ngamchuea, C. Batchelor-McAuley and R. G. Compton, The fate of silver nanoparticles in authentic human saliva, Nanotoxicology, 2018, 12, 305–311 CrossRef CAS PubMed.
- A. Abdelkhaliq, M. van der Zande, A. K. Undas, R. J. Peters and H. Bouwmeester, Impact of in vitro digestion on gastrointestinal fate and uptake of silver nanoparticles with different surface modifications, Nanotoxicology, 2020, 14, 111–126 CrossRef CAS PubMed.
- E. P. o. F. A. a. F. (FAF), M. Younes, G. Aquilina, L. Castle, K. H. Engel, P. Fowler, M. J. Frutos Fernandez, P. Fürst, U. Gundert-Remy and R. Gürtler, Safety assessment of titanium dioxide (E171) as a food additive, EFSA J., 2021, 19, e06585 Search PubMed.
- X. Cao, Y. Han, F. Li, Z. Li, D. J. McClements, L. He, E. A. Decker, B. Xing and H. Xiao, Impact of protein-nanoparticle interactions on gastrointestinal fate of ingested nanoparticles: Not just simple protein corona effects, NanoImpact, 2019, 13, 37–43 CrossRef.
- R. Coreas, X. Cao, G. M. Deloid, P. Demokritou and W. Zhong, Lipid and protein corona of food-grade TiO2 nanoparticles in simulated gastrointestinal digestion, NanoImpact, 2020, 20, 100272 CrossRef PubMed.
- D. Di Silvio, N. Rigby, B. Bajka, A. Mackie and F. B. Bombelli, Effect of protein corona magnetite nanoparticles derived from bread in vitro digestion on Caco-2 cells morphology and uptake, Int. J. Biochem. Cell Biol., 2016, 75, 212–222 CrossRef CAS PubMed.
- H. Brouwer, M. Porbahaie, S. Boeren, M. Busch and H. Bouwmeester, The in vitro gastrointestinal digestion-associated protein corona of polystyrene nano-and microplastics increases their uptake by human THP-1-derived macrophages, Part. Fibre Toxicol., 2024, 21, 4 CrossRef CAS PubMed.
- J. M. Yong, J. Mantaj, Y. Cheng and D. Vllasaliu, Delivery of nanoparticles across the intestinal epithelium via the transferrin transport pathway, Pharmaceutics, 2019, 11, 298 CrossRef CAS PubMed.
- X. Bao, K. Qian and P. Yao, Oral delivery of exenatide-loaded
hybrid zein nanoparticles for stable blood glucose control and β-cell repair of type 2 diabetes mice, J. Nanobiotechnol., 2020, 18, 1–15 CrossRef PubMed.
- W. Fan, D. Xia, Q. Zhu, X. Li, S. He, C. Zhu, S. Guo, L. Hovgaard, M. Yang and Y. Gan, Functional nanoparticles exploit the bile acid pathway to overcome multiple barriers of the intestinal epithelium for oral insulin delivery, Biomaterials, 2018, 151, 13–23 CrossRef CAS PubMed.
- S. Wu, W. Bin, B. Tu, X. Li, W. Wang, S. Liao and C. Sun, A delivery system for oral administration of proteins/peptides through bile acid transport channels, J. Pharm. Sci., 2019, 108, 2143–2152 CrossRef CAS PubMed.
- A. Marucco, M. Prono, D. Beal, E. Alasonati, P. Fisicaro, E. Bergamaschi, M. Carriere and I. Fenoglio, Biotransformation of food-grade and nanometric TiO2 in the oral–gastro–intestinal tract: driving forces and effect on the toxicity toward intestinal epithelial cells, Nanomaterials, 2020, 10, 2132 CrossRef CAS PubMed.
- D. Lichtenstein, J. Ebmeyer, P. Knappe, S. Juling, L. Böhmert, S. Selve, B. Niemann, A. Braeuning, A. F. Thünemann and A. Lampen, Impact of food components during in vitro digestion of silver nanoparticles on cellular uptake and cytotoxicity in intestinal cells, Biol. Chem., 2015, 396, 1255–1264 CAS.
- A. P. Walczak, R. Fokkink, R. Peters, P. Tromp, Z. E. Herrera Rivera, I. M. Rietjens, P. J. Hendriksen and H. Bouwmeester, Behaviour of silver nanoparticles and silver ions in an in vitro human gastrointestinal digestion model, Nanotoxicology, 2013, 7, 1198–1210 CrossRef CAS PubMed.
- F. Bietto, R. Scardaci, M. Brovia, I. Kokalari, F. Barbero, I. Fenoglio and E. Pessione, Food-grade titanium dioxide can affect microbiota physiology, adhesion capability, and interbacterial interactions: A study on L. rhamnosus and E. faecium, Food Chem. Toxicol., 2023, 176, 113760 CrossRef CAS PubMed.
- B. Lamas, N. Martins Breyner and E. Houdeau, Impacts of foodborne inorganic nanoparticles on the gut microbiota-immune axis: potential consequences for host health, Part. Fibre Toxicol., 2020, 17, 1–22 CrossRef PubMed.
- T. Stalder, T. Zaiter, W. El Basset, R. Cornu, H. Martin, M. Diab and A. Béduneau, Interaction and toxicity of ingested nanoparticles on the intestinal barrier, Toxicology, 2022, 153353 CrossRef CAS PubMed.
- R. Li, F. Laurent, A. Taverner, J. Mackay, P. A. De Bank and R. J. Mrsny, Intestinal transcytosis of a protein cargo and nanoparticles mediated by a non-toxic form of pseudomonas aeruginosa exotoxin A, Pharmaceutics, 2021, 13, 1171 CrossRef CAS PubMed.
- S. Lama, O. Merlin-Zhang and C. Yang, In vitro and in vivo models for evaluating the oral toxicity of nanomedicines, Nanomaterials, 2020, 10, 2177 CrossRef CAS PubMed.
-
L. Sherwood, R. T. Kell and C. Ward, Human physiology: from cells to systems, Cengage Learning, Belmont, CA: Brooks/Cole, 2004 Search PubMed.
- K. Namdee, M. Carrasco-Teja, M. Fish, P. Charoenphol and O. Eniola-Adefeso, Effect of variation in hemorheology between human and animal blood on the binding efficacy of vascular-targeted carriers, Sci. Rep., 2015, 5, 11631 CrossRef CAS PubMed.
-
V. Hoffbrand and D. P. Steensma, Hoffbrand's essential haematology, John Wiley & Sons, 2019 Search PubMed.
-
C. Janeway, P. Travers, M. Walport and M. Shlomchik, Immunobiology: the immune system in health and disease, Garland Pub, New York, 2001 Search PubMed.
-
J. Mathew, P. Sankar and M. Varacallo, Physiology, Blood Plasma, StatPearls Publishing, Treasure Island (FL), 2023 Search PubMed.
- N. L. Anderson and N. G. Anderson, The human plasma proteome: history, character, and diagnostic prospects, Mol. Cell. Proteomics, 2002, 1, 845–867 CrossRef CAS PubMed.
-
C. Porth, Essentials of pathophysiology: concepts of altered health states, Lippincott Williams & Wilkins, 2011 Search PubMed.
- S. Gaillet and J.-M. Rouanet, Silver nanoparticles: their potential toxic effects after oral exposure and underlying mechanisms–a review, Food Chem. Toxicol., 2015, 77, 58–63 CrossRef CAS PubMed.
- J. W. Nichols and Y. H. Bae, Odyssey of a cancer nanoparticle: from injection site to site of action, Nano Today, 2012, 7, 606–618 CrossRef CAS PubMed.
- M. Schneider, F. Stracke, S. Hansen and U. F. Schaefer, Nanoparticles and their interactions with the dermal barrier, Derm.-Endocrinol., 2009, 1, 197–206 CrossRef CAS PubMed.
- J. Kim, Y.-J. Heo and S. Shin, Haemocompatibility evaluation of silica nanomaterials using hemorheological measurements, Clin. Hemorheol. Microcirc., 2016, 62, 99–107 CAS.
- L. Q. Chen, L. Fang, J. Ling, C. Z. Ding, B. Kang and C. Z. Huang, Nanotoxicity of silver nanoparticles to red blood cells: size dependent adsorption, uptake, and hemolytic activity, Chem. Res. Toxicol., 2015, 28, 501–509 Search PubMed.
- A. Aseychev, O. Azizova, E. Beckman, L. Dudnik and V. Sergienko, Effect of gold nanoparticles coated with plasma components on ADP-induced platelet aggregation, Bull. Exp. Biol. Med., 2013, 155, 685–688 CrossRef CAS PubMed.
- R. Dunpall, A. A. Nejo, V. Pullabhotla, S. Rajasekhar, A. R. Opoku, N. Revaprasadu and A. Shonhai, An in vitro assessment of the interaction of cadmium selenide quantum dots with DNA, iron, and blood platelets, IUBMB Life, 2012, 64, 995–1002 CrossRef CAS PubMed.
- J. Laloy, V. Minet, L. Alpan, F. Mullier, S. Beken, O. Toussaint, S. Lucas and J.-M. Dogné, Impact of silver nanoparticles on haemolysis, platelet function and coagulation, Nanobiomedicine, 2014, 1, 4 CrossRef PubMed.
- S. P. Samuel, M. J. Santos-Martinez, C. Medina, N. Jain, M. W. Radomski, A. Prina-Mello and Y. Volkov, CdTe quantum dots induce activation of human platelets: implications for nanoparticle hemocompatibility, Int. J. Nanomed., 2015, 2723–2734 CAS.
- V. Govindarajan, V. Rakesh, J. Reifman and A. Y. Mitrophanov, Computational study of thrombus formation and clotting factor effects under venous flow conditions, Biophys. J., 2016, 110, 1869–1885 CrossRef CAS PubMed.
- K. M. de la Harpe, P. P. Kondiah, Y. E. Choonara, T. Marimuthu, L. C. du Toit and V. Pillay, The hemocompatibility of nanoparticles: a review of cell–nanoparticle interactions and hemostasis, Cells, 2019, 8, 1209 CrossRef CAS PubMed.
- A. B. Pai, Complexity of intravenous iron nanoparticle formulations: implications for bioequivalence evaluation, Ann. N. Y. Acad. Sci., 2017, 1407, 17–25 CrossRef CAS PubMed.
- A. M. Mehedinti, C. Capusa, I. Andreiana and G. Mircescu, Intravenous iron-carbohydrate nanoparticles and their similars. What do we choose?, Maedica, 2022, 17, 436 Search PubMed.
- L. Soddu, D. N. Trinh, E. Dunne, D. Kenny, G. Bernardini, I. Kokalari, A. Marucco, M. P. Monopoli and I. Fenoglio, Identification of physicochemical properties that modulate nanoparticle aggregation in blood, Beilstein J. Nanotechnol., 2020, 11, 550–567 CrossRef CAS PubMed.
- A. M. Clemments, P. Botella and C. C. Landry, Protein adsorption from biofluids on silica nanoparticles: corona analysis as a function of particle diameter and porosity, ACS Appl. Mater. Interfaces, 2015, 7, 21682–21689 CrossRef CAS PubMed.
- Q. Yu, L. Zhao, C. Guo, B. Yan and G. Su, Regulating protein corona formation and dynamic protein exchange by controlling nanoparticle hydrophobicity, Front. Bioeng. Biotechnol., 2020, 8, 210 CrossRef PubMed.
- A. Gessner, R. Waicz, A. Lieske, B.-R. Paulke, K. Mäder and R. Müller, Nanoparticles with decreasing surface hydrophobicities: influence on plasma protein adsorption, Int. J. Pharm., 2000, 196, 245–249 CrossRef CAS PubMed.
- X. Lu, P. Xu, H.-M. Ding, Y.-S. Yu, D. Huo and Y.-Q. Ma, Tailoring the component of protein corona via simple chemistry, Nat. Commun., 2019, 10, 4520 CrossRef PubMed.
- I. Lynch, A. Salvati and K. A. Dawson, What does the cell see?, Nat. Nanotechnol., 2009, 4, 546–547 CrossRef CAS PubMed.
- A. Gessner, A. Lieske, B. R. Paulke and R. H. Müller, Influence of surface charge density on protein adsorption on polymeric nanoparticles: analysis by two-dimensional electrophoresis, Eur. J. Pharm. Biopharm., 2002, 54, 165–170 CrossRef CAS PubMed.
- C. D. Walkey, J. B. Olsen, H. Guo, A. Emili and W. C. Chan, Nanoparticle size and surface chemistry determine serum protein adsorption and macrophage uptake, J. Am. Chem. Soc., 2012, 134, 2139–2147 CrossRef CAS PubMed.
- M. Yahyaei, F. Mehrnejad, H. Naderi-Manesh and A. H. Rezayan, Protein adsorption onto polysaccharides: Comparison of chitosan and chitin polymers, Carbohydr. Polym., 2018, 191, 191–197 CrossRef CAS PubMed.
- B. Stordy, Y. Zhang, Z. Sepahi, M. H. Khatami, P. M. Kim and W. C. Chan, Conjugating ligands to an equilibrated nanoparticle protein corona enables cell targeting in serum, Chem. Mater., 2022, 34, 6868–6882 CrossRef CAS.
- M. Cao, R. Cai, L. Zhao, M. Guo, L. Wang, Y. Wang, L. Zhang, X. Wang, H. Yao and C. Xie, Molybdenum derived from nanomaterials incorporates into molybdenum enzymes and affects their activities in vivo, Nat. Nanotechnol., 2021, 16, 708–716 CrossRef CAS PubMed.
- Y. Li, S. Jacques, H. Gaikwad, G. Wang, N. K. Banda, V. M. Holers, R. I. Scheinman, S. Tomlinson, S. M. Moghimi and D. Simberg, Inhibition of acute complement responses towards bolus-injected nanoparticles using targeted short-circulating regulatory proteins, Nat. Nanotechnol., 2024, 19, 246–254 CrossRef CAS PubMed.
- A. Åkesson, M. Cárdenas, G. Elia, M. P. Monopoli and K. A. Dawson, The protein corona of dendrimers: PAMAM binds and activates complement proteins in human plasma in a generation dependent manner, RSC Adv., 2012, 2, 11245–11248 RSC.
- E. Papini, R. Tavano and F. Mancin, Opsonins and dysopsonins of nanoparticles: facts, concepts, and methodological guidelines, Front. Immunol., 2020, 11, 567365 CrossRef CAS PubMed.
- V. P. Vu, G. B. Gifford, F. Chen, H. Benasutti, G. Wang, E. V. Groman, R. Scheinman, L. Saba, S. M. Moghimi and D. Simberg, Immunoglobulin deposition on biomolecule corona determines complement opsonization efficiency of preclinical and clinical nanoparticles, Nat. Nanotechnol., 2019, 14, 260–268 CrossRef CAS PubMed.
- F. Wang, L. Yu, M. P. Monopoli, P. Sandin, E. Mahon, A. Salvati and K. A. Dawson, The biomolecular corona is retained during nanoparticle uptake and protects the cells from the damage induced by cationic nanoparticles until degraded in the lysosomes, Nanomedicine, 2013, 9, 1159–1168 CrossRef CAS PubMed.
- Q. Peng, S. Zhang, Q. Yang, T. Zhang, X.-Q. Wei, L. Jiang, C.-L. Zhang, Q.-M. Chen, Z.-R. Zhang and Y.-F. Lin, Preformed albumin corona, a protective coating for nanoparticles based drug delivery system, Biomaterials, 2013, 34, 8521–8530 CrossRef CAS PubMed.
- H. Amiri, L. Bordonali, A. Lascialfari, S. Wan, M. P. Monopoli, I. Lynch, S. Laurent and M. Mahmoudi, Protein corona affects the relaxivity and MRI contrast efficiency of magnetic nanoparticles, Nanoscale, 2013, 5, 8656–8665 RSC.
- M. A. Dobrovolskaia, A. K. Patri, J. Zheng, J. D. Clogston, N. Ayub, P. Aggarwal, B. W. Neun, J. B. Hall and S. E. McNeil, Interaction of colloidal gold nanoparticles with human blood: effects on particle size and analysis of plasma protein binding profiles, Nanomedicine, 2009, 5, 106–117 CrossRef CAS PubMed.
- A. Lesniak, F. Fenaroli, M. P. Monopoli, C. Åberg, K. A. Dawson and A. Salvati, Effects of the presence or absence of a protein corona on silica nanoparticle uptake and impact on cells, ACS Nano, 2012, 6, 5845–5857 CrossRef CAS PubMed.
- O. Vilanova, J. J. Mittag, P. M. Kelly, S. Milani, K. A. Dawson, J. O. Rädler and G. Franzese, Understanding the kinetics of protein–nanoparticle corona formation, ACS Nano, 2016, 10, 10842–10850 CrossRef CAS PubMed.
-
H. Ritchie, E. Mathieu, L. Rodés-Guirao, C. Appel, C. Giattino, E. Ortiz-Ospina, J. Hasell, B. Macdonald, D. Beltekian and M. Roser, Coronavirus pandemic (COVID-19)) Search PubMed.
- Y.-D. Li, W.-Y. Chi, J.-H. Su, L. Ferrall, C.-F. Hung and T.-C. Wu, Coronavirus vaccine development: from SARS and MERS to COVID-19, J. Biomed. Sci., 2020, 27, 1–23 CrossRef CAS PubMed.
- L. Schoenmaker, D. Witzigmann, J. A. Kulkarni, R. Verbeke, G. Kersten, W. Jiskoot and D. J. Crommelin, mRNA-lipid nanoparticle COVID-19 vaccines: Structure and stability, Int. J. Pharm., 2021, 601, 120586 CrossRef CAS PubMed.
- M. I. M. Razif, N. Nizar, N. H. Z. Abidin, S. N. M. Ali, W. N. N. W. Zarimi, J. Khotib, D. Susanti, M. T. M. Jailani and M. Taher, Emergence of mRNA vaccines in the management of cancer, Expert Rev. Vaccines, 2023, 22, 629–642 CrossRef PubMed.
- H. You, M. K. Jones, C. A. Gordon, A. E. Arganda, P. Cai, H. Al-Wassiti, C. W. Pouton and D. P. McManus, The mRNA Vaccine Technology Era and the Future Control of Parasitic Infections, Clin. Microbiol. Rev., 2023, 36, e00241-21 CrossRef PubMed.
- A. Saorin, G. Saorin, F. Duzagac, P. Parisse, N. Cao, G. Corona, E. Cavarzerani and F. Rizzolio, Microfluidic production of amiodarone loaded nanoparticles and application in drug repositioning in ovarian cancer, Sci. Rep., 2024, 14, 6280 CrossRef CAS PubMed.
- S. Siemer, T. A. Bauer, P. Scholz, C. Breder, F. Fenaroli, G. Harms, D. Dietrich, J. Dietrich, C. Rosenauer, M. Barz, S. Becker, S. Strieth, C. Reinhardt, T. Fauth, J. Hagemann and R. H. Stauber, Targeting Cancer Chemotherapy Resistance by Precision Medicine-Driven Nanoparticle-Formulated Cisplatin, ACS Nano, 2021, 15, 18541–18556 CrossRef CAS PubMed.
- H. Petousis-Harris, Vaccine injection technique and reactogenicity—evidence for practice, Vaccine, 2008, 26, 6299–6304 CrossRef PubMed.
- C. Li, Y. Chen, Y. Zhao, D. C. Lung, Z. Ye, W. Song, F.-F. Liu, J.-P. Cai, W.-M. Wong and C. C.-Y. Yip, Intravenous injection of coronavirus disease 2019 (COVID-19) mRNA vaccine can induce acute myopericarditis in mouse model, Clin. Infect. Dis., 2022, 74, 1933–1950 CrossRef CAS PubMed.
- K. Maqbool, A. Siddiqui, H. Mehboob and Q. Jamil, Study of non-Newtonian synovial fluid flow by a recursive approach, Phys. Fluids, 2022, 34, 111903 CrossRef CAS.
- H. Fam, J. Bryant and M. Kontopoulou, Rheological properties of synovial fluids, Biorheology, 2007, 44, 59–74 CAS.
- S. R. Brannan and D. A. Jerrard, Synovial fluid analysis, J. Emerg. Med., 2006, 30, 331–339 CrossRef PubMed.
- E. Pascual and V. Jovaní, Synovial fluid analysis, Best Pract. Res., Clin. Rheumatol., 2005, 19, 371–386 CrossRef PubMed.
- D. H. Sohn, J. Sokolove, O. Sharpe, J. C. Erhart, P. E. Chandra, L. J. Lahey, T. M. Lindstrom, I. Hwang, K. A. Boyer and T. P. Andriacchi, Plasma proteins present in osteoarthritic synovial fluid can stimulate cytokine production via Toll-like receptor 4, Arthritis Res. Ther., 2012, 14, 1–13 CrossRef.
- D. Furmann, D. Nečas, D. Rebenda, P. Čípek, M. Vrbka, I. Křupka and M. Hartl, The effect of synovial fluid composition, speed and load on frictional behaviour of articular cartilage, Materials, 2020, 13, 1334 CrossRef CAS PubMed.
- M. K. Kosinska, T. E. Ludwig, G. Liebisch, R. Zhang, H.-C. Siebert, J. Wilhelm, U. Kaesser, R. B. Dettmeyer, H. Klein and B. Ishaque, Articular joint lubricants during osteoarthritis and rheumatoid arthritis display altered levels and molecular species, PLoS One, 2015, 10, e0125192 CrossRef PubMed.
- J.-H. Lee, J. H. Jung, J. Kim, W.-K. Baek, J. Rhee, T.-H. Kim, S.-H. Kim, K. P. Kim, C.-N. Son and J.-S. Kim, Proteomic analysis of human synovial fluid reveals potential diagnostic biomarkers for ankylosing spondylitis, Clin. Proteomics, 2020, 17, 1–11 CrossRef PubMed.
- J. Bullock, S. A. Rizvi, A. M. Saleh, S. S. Ahmed, D. P. Do, R. A. Ansari and J. Ahmed, Rheumatoid arthritis: a brief overview of the treatment, Med. Princ. Pract., 2019, 27, 501–507 CrossRef PubMed.
- A. Bandyopadhyay, I. Mitra, S. B. Goodman, M. Kumar and S. Bose, Improving biocompatibility for next generation of metallic implants, Prog. Mater. Sci., 2023, 133, 101053 CrossRef CAS PubMed.
- V. Tsakiris, C. Tardei and F. M. Clicinschi, Biodegradable Mg alloys for orthopedic implants–A review, J. Magnesium Alloys, 2021, 9, 1884–1905 CrossRef CAS.
- R. Sato, S. Nakano, T. Takasago, M. Nakamura, M. Kashima, T. Chikawa, D. Hamada, T. Goto and K. Sairyo, Chondrogenesis in the synovial tissue is associated with the onset of pseudogout after total knee arthroplasty, Arthroplast. Today, 2016, 2, 101–104 CrossRef PubMed.
- F. S. Tabatabaei Mirakabad, K. Nejati-Koshki, A. Akbarzadeh, M. R. Yamchi, M. Milani, N. Zarghami, V. Zeighamian, A. Rahimzadeh, S. Alimohammadi and Y. Hanifehpour, PLGA-based nanoparticles as cancer drug delivery systems, Asian Pac. J. Cancer Prev., 2014, 15, 517–535 CrossRef PubMed.
- X. Guo, X. Zuo, Z. Zhou, Y. Gu, H. Zheng, X. Wang, G. Wang, C. Xu and F. Wang, PLGA-based micro/nanoparticles: an overview of their applications in respiratory diseases, Int. J. Mol. Sci., 2023, 24, 4333 CrossRef CAS PubMed.
- S. Brown, J. Pistiner, I. M. Adjei and B. Sharma, Nanoparticle properties for delivery to cartilage: the implications of disease state, synovial fluid, and off-target uptake, Mol. Pharmaceutics, 2017, 16, 469–479 CrossRef PubMed.
- M. A. Koronfel, A. E. Goode, J. N. Weker, S. E. Tay, C. A. Stitt, T. A. Simoes, J. F. W. Mosselmans, P. Quinn, R. Brydson and A. Hart, Understanding the reactivity of CoCrMo-implant wear particles, npj Mater. Degrad., 2018, 2, 8 CrossRef.
- T. A. Simoes, M. G. Bryant, A. P. Brown, S. J. Milne, M. Ryan, A. Neville and R. Brydson, Evidence for the dissolution of molybdenum during tribocorrosion of CoCrMo hip implants in the presence of serum protein, Acta Biomater., 2016, 45, 410–418 CrossRef CAS PubMed.
- S. Lal, E. A. Caseley, R. M. Hall and J. L. Tipper, Biological impact of silicon nitride for orthopaedic applications: role of particle size, surface composition and donor variation, Sci. Rep., 2018, 8, 9109 CrossRef PubMed.
- E. Giubilato, V. Cazzagon, M. J. Amorim, M. Blosi, J. Bouillard, H. Bouwmeester, A. L. Costa, B. Fadeel, T. F. Fernandes and C. Fito, Risk management framework for nano-biomaterials used in medical devices and advanced therapy medicinal products, Materials, 2020, 13, 4532 CrossRef CAS PubMed.
- A. Gamer, E. Leibold and B. Van Ravenzwaay, The in vitro absorption of microfine zinc oxide and titanium dioxide through porcine skin, Toxicol. In Vitro, 2006, 20, 301–307 CrossRef CAS PubMed.
- B. Baroli, M. G. Ennas, F. Loffredo, M. Isola, R. Pinna and M. A. López-Quintela, Penetration of metallic nanoparticles in human full-thickness skin, J. Invest. Dermatol., 2007, 127, 1701–1712 CrossRef CAS PubMed.
- F. F. Larese, F. D'Agostin, M. Crosera, G. Adami, N. Renzi, M. Bovenzi and G. Maina, Human skin penetration of silver nanoparticles through intact and damaged skin, Toxicology, 2009, 255, 33–37 CrossRef CAS PubMed.
- B. Baroli, Penetration of nanoparticles and nanomaterials in the skin: fiction or reality?, J. Pharm. Sci., 2010, 99, 21–50 CrossRef CAS PubMed.
- N. A. Monteiro-Riviere, M. E. Samberg, S. J. Oldenburg and J. E. Riviere, Protein binding modulates the cellular uptake of silver nanoparticles into human cells: implications for in vitro to in vivo extrapolations?, Toxicol. Lett., 2013, 220, 286–293 CrossRef CAS PubMed.
- M. Mitjans, L. Marics, M. Bilbao, A. S. Maddaleno, J. J. Piñero and M. P. Vinardell, Size matters? A comprehensive in vitro study of the impact of particle size on the toxicity of ZnO, Nanomaterials, 2023, 13, 1800 CrossRef CAS PubMed.
- K. M. Cosert, S. Kim, I. Jalilian, M. Chang, B. L. Gates, K. E. Pinkerton, L. S. Van Winkle, V. K. Raghunathan, B. C. Leonard and S. M. Thomasy, Metallic engineered nanomaterials and ocular toxicity: a current perspective, Pharmaceutics, 2022, 14, 981 CrossRef CAS PubMed.
- C. Yang, J. Yang, A. Lu, J. Gong, Y. Yang, X. Lin, M. Li and H. Xu, Nanoparticles in ocular applications and their potential toxicity, Front. Mol. Biosci., 2022, 9, 931759 CrossRef CAS PubMed.
- Y. Eom, J. S. Song, D. Y. Lee, M.-K. Kim, B.-R. Kang, J.-H. Heo, H. K. Lee and H. M. Kim, Effect of titanium dioxide nanoparticle exposure on the ocular surface: an animal study, Ocul. Surf., 2016, 14, 224–232 CrossRef PubMed.
- M. Kim, J.-H. Park, H. Jeong, J. Hong, W. S. Choi, B.-H. Lee and C. Y. Park, An evaluation of the in vivo safety of nonporous silica nanoparticles: Ocular topical administration versus oral administration, Sci. Rep., 2017, 7, 8238 CrossRef PubMed.
- A. Meza-Rios, J. Navarro-Partida, J. Armendariz-Borunda and A. Santos, Therapies based on nanoparticles for eye drug delivery, Ophthalmol. Ther., 2020, 9, 1–14 CrossRef PubMed.
- C. Astarita, S. Palchetti, M. Massaro-Giordano, M. Di Domenico, F. Petrillo, S. Boffo, G. Caracciolo and A. Giordano, Artificial protein coronas enable controlled interaction with corneal epithelial cells: new opportunities for ocular drug delivery, Pharmaceutics, 2021, 13, 867 CrossRef CAS PubMed.
- L. Marichal, G. Klein, J. Armengaud, Y. Boulard, S. Chédin, J. Labarre, S. Pin, J.-P. Renault and J.-C. Aude, Protein corona composition of silica nanoparticles in complex media: Nanoparticle size does not matter, Nanomaterials, 2020, 10, 240 CrossRef CAS PubMed.
- B. Hellack, C. Nickel, C. Albrecht, T. A. Kuhlbusch, S. Boland, A. Baeza-Squiban, W. Wohlleben and R. P. Schins, Analytical methods to assess the oxidative potential of nanoparticles: a review, Environ. Sci.: Nano, 2017, 4, 1920–1934 RSC.
- Z. Zhang, J. Ren, W. Dai, H. Zhang, X. Wang, B. He and Q. Zhang, Fast and Dynamic Mapping of the Protein Corona on Nanoparticle Surfaces by Photocatalytic Proximity Labeling, Adv. Mater., 2023, 35, 2206636 CrossRef CAS PubMed.
- C. Pavan, R. Santalucia, G. Escolano-Casado, P. Ugliengo, L. Mino and F. Turci, Physico-Chemical Approaches to Investigate Surface Hydroxyls as Determinants of Molecular Initiating Events in Oxide Particle Toxicity, Int. J. Mol. Sci., 2023, 24, 11482 CrossRef CAS PubMed.
- W. Hu, C. Peng, M. Lv, X. Li, Y. Zhang, N. Chen, C. Fan and Q. Huang, Protein corona-mediated mitigation of cytotoxicity of graphene oxide, ACS Nano, 2011, 5, 3693–3700 CrossRef CAS PubMed.
- A. Saorin, A. Martinez-Serra, G. J. Paparoni-Bruzual, M. Crozzolin, V. Lombardi, M. Back, P. Riello, M. P. Monopoli and F. Rizzolio, Understanding the impact of silica nanoparticles in cancer cells through physicochemical and biomolecular characterizations, Mater. Adv., 2024, 5, 5106–5117 RSC.
- L. S. Franqui, M. A. De Farias, R. V. Portugal, C. A. Costa, R. R. Domingues, A. G. Souza Filho, V. R. Coluci, A. F. Leme and D. S. T. Martinez, Interaction of graphene oxide with cell culture medium: Evaluating the fetal bovine serum protein corona formation towards in vitro nanotoxicity assessment and nanobiointeractions, Mater. Sci. Eng., C, 2019, 100, 363–377 CrossRef CAS PubMed.
- S. Sheibani, K. Basu, A. Farnudi, A. Ashkarran, M. Ichikawa, J. F. Presley, K. H. Bui, M. R. Ejtehadi, H. Vali and M. Mahmoudi, Nanoscale characterization of the biomolecular corona by cryo-electron microscopy, cryo-electron tomography, and image simulation, Nat. Commun., 2021, 12, 573 CrossRef CAS PubMed.
- G. Maiorano, S. Sabella, B. Sorce, V. Brunetti, M. A. Malvindi, R. Cingolani and P. P. Pompa, Effects of cell culture media on the dynamic formation of protein− nanoparticle complexes and influence on the cellular response, ACS Nano, 2010, 4, 7481–7491 CrossRef CAS PubMed.
- Y. Hayashi, T. Miclaus, C. Scavenius, K. Kwiatkowska, A. Sobota, P. Engelmann, J. J. Scott-Fordsmand, J. J. Enghild and D. S. Sutherland, Species differences take shape at nanoparticles: protein corona made of the native repertoire assists cellular interaction, Environ. Sci. Technol., 2013, 47, 14367–14375 CrossRef CAS PubMed.
- D. N. Trinh, M. Radlinskaite, J. Cheeseman, G. Kuhnle, H. M. Osborn, P. Meleady, D. I. Spencer and M. P. Monopoli, Biomolecular corona stability in association with plasma cholesterol level, Nanomaterials, 2022, 12, 2661 CrossRef CAS PubMed.
- Y.-H. Yang, R. Wen, N. Yang, T.-N. Zhang and C.-F. Liu, Roles of protein post-translational modifications in glucose and lipid metabolism: mechanisms and perspectives, Mol. Med., 2023, 29, 93 CAS.
- H. Černocká, L. Římánková and V. Ostatná, Fetuin and asialofetuin at charged surfaces: Influence of sialic acid presence, J. Electroanal. Chem., 2021, 902, 115801 CrossRef.
-
M. E. Taylor and K. Drickamer, Introduction to glycobiology, Oxford University Press, USA, 2011 Search PubMed.
- F. Clerc, K. R. Reiding, B. C. Jansen, G. S. Kammeijer, A. Bondt and M. Wuhrer, Human plasma protein N-glycosylation, Glycoconjugate J., 2016, 33, 309–343 CrossRef CAS PubMed.
- S. S. Pinho, I. Alves, J. Gaifem and G. A. Rabinovich, Immune regulatory networks coordinated by glycans and glycan-binding proteins in autoimmunity and infection, Cell. Mol. Immunol., 2023, 20, 1101–1113 CrossRef CAS PubMed.
- L. Nimrichter, M. M. Burdick, K. Aoki, W. Laroy, M. A. Fierro, S. A. Hudson, C. E. Von Seggern, R. J. Cotter, B. S. Bochner and M. Tiemeyer, E-selectin receptors on human leukocytes, Blood, 2008, 112, 3744–3752 CrossRef CAS PubMed.
- M. Paurević, M. Šrajer Gajdošik and R. Ribić, Mannose Ligands for Mannose Receptor Targeting, Int. J. Mol. Sci., 2024, 25, 1370 CrossRef PubMed.
- O. Blixt, B. E. Collins, I. M. van den Nieuwenhof, P. R. Crocker and J. C. Paulson, Sialoside specificity of the siglec family assessed using novel multivalent probes: identification of potent inhibitors of myelin-associated glycoprotein, J. Biol. Chem., 2003, 278, 31007–31019 CrossRef CAS PubMed.
- H. Tateno, P. R. Crocker and J. C. Paulson, Mouse Siglec-F and human Siglec-8 are functionally convergent paralogs that are selectively expressed on eosinophils and recognize 6′-sulfo-sialyl Lewis X as a preferred glycan ligand, Glycobiology, 2005, 15, 1125–1135 CrossRef CAS PubMed.
- P. R. Crocker, J. C. Paulson and A. Varki, Siglecs and their roles in the immune system, Nat. Rev. Immunol., 2007, 7, 255–266 CrossRef CAS PubMed.
- B. S. Bochner, R. A. Alvarez, P. Mehta, N. V. Bovin, O. Blixt, J. R. White and R. L. Schnaar, Glycan array screening reveals a candidate ligand for Siglec-8, J. Biol. Chem., 2005, 280, 4307–4312 CrossRef CAS PubMed.
- W. H. Yang, P. V. Aziz, D. M. Heithoff, M. J. Mahan, J. W. Smith and J. D. Marth, An intrinsic mechanism of secreted protein aging and turnover, Proc. Natl. Acad. Sci. U. S. A., 2015, 112, 13657–13662 CrossRef CAS PubMed.
- W. H. Yang, P. V. Aziz, D. M. Heithoff, M. J. Mahan, J. W. Smith and J. D. Marth, An intrinsic mechanism of secreted protein aging and turnover, Proc. Natl. Acad. Sci. U. S. A., 2015, 112, 13657–13662 CrossRef CAS PubMed.
- K. G. Rice and Y. C. Lee, Modification of triantennary glycopeptide into probes for the asialoglycoprotein receptor of hepatocytes, J. Biol. Chem., 1990, 265, 18423–18428 CrossRef CAS PubMed.
- Y.-H. Lin, V. Franc and A. J. Heck, Similar albeit not the same: in-depth analysis of proteoforms of human serum, bovine serum, and recombinant human fetuin, J. Proteome Res., 2018, 17, 2861–2869 CrossRef CAS PubMed.
- U. Galili, Anti-G al: an abundant human natural antibody of multiple pathogeneses and clinical benefits, Immunology, 2013, 140, 1–11 CrossRef CAS PubMed.
- M. O. Altman and P. Gagneux, Absence of Neu5Gc and presence of anti-Neu5Gc antibodies in humans—an evolutionary perspective, Front. Immunol., 2019, 10, 789 CrossRef CAS PubMed.
- S. Wang, C. Chen, M. Guan, D. Liu, X.-F. Wan and L. Li, Terminal epitope-dependent branch preference of Siglecs toward N-glycans, Front. Mol. Biosci., 2021, 8, 645999 CrossRef CAS PubMed.
- T. Angata, Possible influences of endogenous and exogenous ligands on the evolution of human Siglecs, Front. Immunol., 2018, 9, 2885 CrossRef CAS PubMed.
- V. Padler-Karavani, N. Hurtado-Ziola, Y.-C. Chang, J. L. Sonnenburg, A. Ronaghy, H. Yu, A. Verhagen, V. Nizet, X. Chen and N. Varki, Rapid evolution of binding specificities and expression patterns of inhibitory CD33-related Siglecs in primates, FASEB J., 2014, 28, 1280 CrossRef CAS PubMed.
- S. Wan, P. M. Kelly, E. Mahon, H. Stockmann, P. M. Rudd, F. Caruso, K. A. Dawson, Y. Yan and M. P. Monopoli, The “sweet” side of the protein corona: effects of glycosylation on nanoparticle–cell interactions, ACS Nano, 2015, 9, 2157–2166 CrossRef CAS PubMed.
- G. Caracciolo, O. C. Farokhzad and M. Mahmoudi, Biological identity of nanoparticles in vivo: clinical implications of the protein corona, Trends Biotechnol., 2017, 35, 257–264 CrossRef CAS PubMed.
- E. Quagliarini, D. Pozzi, F. Cardarelli and G. Caracciolo, The influence of protein corona on Graphene Oxide: implications for biomedical theranostics, J. Nanobiotechnol., 2023, 21, 267 CrossRef CAS PubMed.
- M. M. Neves, S.-J. Richards, A. N. Baker, M. Walker, P. G. Georgiou and M. I. Gibson, Discrimination between protein glycoforms using lectin-functionalised gold nanoparticles as signal enhancers, Nanoscale Horiz., 2023, 8, 377–382 RSC.
- H.-Y. Park, C. Chung, M. K. Eiken, K. V. Baumgartner, K. M. Fahy, K. Q. Leung, E. Bouzos, P. Asuri, K. E. Wheeler and K. R. Riley, Silver nanoparticle interactions with glycated and non-glycated human serum albumin mediate toxicity, Front. Toxicol., 2023, 5, 1081753 CrossRef PubMed.
- M. Faria, M. Björnmalm, K. J. Thurecht, S. J. Kent, R. G. Parton, M. Kavallaris, A. P. Johnston, J. J. Gooding, S. R. Corrie and B. J. Boyd, Minimum information reporting in bio–nano experimental literature, Nat. Nanotechnol., 2018, 13, 777–785 CrossRef CAS PubMed.
Footnote |
† Contributed equally as the first author. |
|
This journal is © The Royal Society of Chemistry 2024 |
Click here to see how this site uses Cookies. View our privacy policy here.