DOI:
10.1039/D2RA06813C
(Paper)
RSC Adv., 2023,
13, 914-925
Silver-catalyzed direct selanylation of indoles: synthesis and mechanistic insights†
Received
28th October 2022
, Accepted 17th December 2022
First published on 4th January 2023
Abstract
Herein we describe the Ag(I)-catalyzed direct selanylation of indoles with diorganoyl diselenides. The reaction gave 3-selanylindoles with high regioselectivity and also allowed direct access to 2-selanylindoles when the C3 position of the indole ring was blocked via a process similar to Plancher rearrangement. Experimental analyses and density functional theory calculations were carried out in order to picture the reaction mechanism. Among the pathways considered (via concerted metalation–deprotonation, Ag(III), radical, and electrophilic aromatic substitution), our findings support a classic electrophilic aromatic substitution via Lewis adducts between Ag(I) and diorganoyl diselenides. The results also afforded new insights into the interactions between Ag(I) and diorganoyl diselenides.
Introduction
Coinage metal (copper, silver, and gold) salts are among the major players in modern catalysis.1 Although silver catalysts have long been believed to have low catalytic efficiency in comparison with other coinage metals, the rapid development of silver chemistry revealed several valuable synthetic transformations over the last two decades.2 In addition, silver has been applied as homogeneous or heterogeneous mediators or catalysts in important industrial applications.2e,3 In particular, Ag(I) salts are employed as σ-Lewis acids due to the availability of their empty f orbitals and relativistic contraction of the electron cloud14,1a,b,2a and as a π-Lewis acid as a result of the d10 electronic configuration which allows the back-donation of electron density to the antibonding π* orbitals.1a,b,2a,4 These features offer numerous opportunities in organic synthesis. For instance, Ag(I) salts have been used in cycloadditions,5 alkynylations,2a,6 cycloisomerizations,7 hydrofunctionalizations,8 halogenations,9 azidations,10 C–H functionalizations,2c,11 and chalcogenylations12 and also as additives in transition metal-catalyzed reactions.13
Selenium-containing compounds are of great importance since they show numerous biological activities14 and also play an important role in organic synthesis12a,15 and materials science.16 For instance, selenium or sulfur-functionalized indoles display promising therapeutic properties,17 and some of them are already commercially-available drugs (Fig. 1).18 In this scenario, the development of efficient and selective methods for the C–Se bond formation have become of paramount importance, which encourages studies on the direct conversion of an inert C–H bond to a C–Se bond that can eliminate pre-functionalized starting materials, resulting in more step- and atom-economical synthesis.12a,19
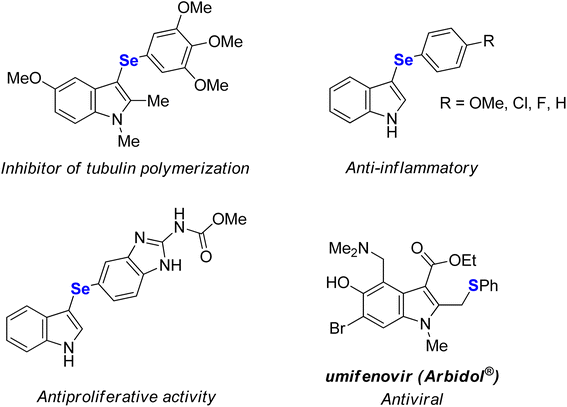 |
| Fig. 1 Biologically relevant selenium or sulfur-functionalized indoles. | |
Generally, the direct selanylation of indole derivatives under transition metal-catalyzed20 or transition metal-free conditions21 relies on the nucleophilicity of the C3 position of this electron-rich heteroarene, and structurally diverse 3-selanylindoles were prepared using these methodologies. Despite the advantages of the transition-metal free methods, the use of stoichiometric amounts of catalysts or the synthesis of starting materials through multistep transformations limits their applications. With this in mind, we report the Ag(I)-catalyzed regioselective C3 selanylation of indoles without the addition of any external additive or ligand in a single step. The mechanism of this reaction was also further investigated by means of density functional theory (DFT) calculations.
Results and discussion
As shown in Table 1, 1-methylindole (1a) and diphenyl diselenide (2a) were employed to optimize the reaction conditions (Table 1). The first experiment was developed with 5.0 mol% Ag2SO4 in DMSO (dimethyl sulfoxide) as the solvent at 100 °C for 24 h, which furnished a 23% yield of the desired product 3a (Table 1, entry 1). Under the same conditions of time and temperature, when the amount of Ag2SO4 was increased to 10 mol% and 20 mol% the product 3a was obtained in 42% and 79% yields respectively (Table 1, entries 2 and 3). Additionally, the use of 30 mol% Ag2SO4 gave the expected product in 87% yield (Table 1, entry 4). Considering the good yields of the desired product obtained with 20 mol% Ag2SO4, the influence of the reaction time was evaluated under this condition. It was observed that a shorter reaction time slightly increased the yield of 3a to 85% (Table 1, entry 5); however, an even shorter time provided the product in a 65% yield (Table 1, entry 6). Next, the reaction temperature was also evaluated (Table 1, entries 7 and 8), and when the experiments were performed at lower temperatures, the yields of 3a were lower than that observed at 100 °C (Table 1, entry 5). On the other hand, when the temperature was 110 °C, the reaction yield was 83% (Table 1, entry 9). In addition, when the reaction was carried out under inert atmosphere, the yield was 56%, which suggests that O2 could be involved in the reaction pathway (Table 1, entry 10). The screening of the catalyst revealed that other sources of Ag(I) were not effective even when the mol% amount of Ag(I) ions in the system were matched (Table 1, entries 11–15) to the reaction with Ag2SO4 (Table 1, entry 5). Finally, among the solvents examined (Table 1, entries 16–23), DMSO was still the most effective (Table 1, entry 5).
Table 1 Optimization of the reaction conditions
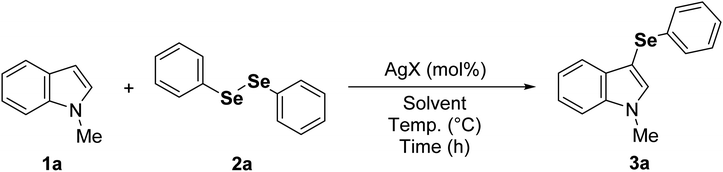
|
Entrya |
Catalyst (mol%) |
Solvent |
Time (h) |
Temp. (°C) |
Yieldb,d (%) |
Reaction conditions: 1a (0.50 mmol), 2a (0.25 mmol), AgX (mol%) and dry solvent (1.0 mL) under air atmosphere. Isolated yields. Under argon atmosphere. In all reactions, we have not identified any side products. |
1 |
Ag2SO4 (5) |
DMSO (1.0) |
24 |
100 |
23 |
2 |
Ag2SO4 (10) |
DMSO (1.0) |
24 |
100 |
42 |
3 |
Ag2SO4 (20) |
DMSO (1.0) |
24 |
100 |
79 |
4 |
Ag2SO4 (30) |
DMSO (1.0) |
24 |
100 |
87 |
5 |
Ag2SO4 (20) |
DMSO (1.0) |
18 |
100 |
85 |
6 |
Ag2SO4 (20) |
DMSO (1.0) |
12 |
100 |
65 |
7 |
Ag2SO4 (20) |
DMSO (1.0) |
18 |
80 |
66 |
8 |
Ag2SO4 (20) |
DMSO (1.0) |
18 |
60 |
58 |
9 |
Ag2SO4 (20) |
DMSO (1.0) |
18 |
110 |
83 |
10c |
Ag2SO4 (20) |
DMSO (1.0) |
18 |
100 |
56 |
11 |
AgNO3 (20) |
DMSO (1.0) |
18 |
100 |
32 |
12 |
AgNO3 (40) |
DMSO (1.0) |
18 |
100 |
60 |
13 |
AgBF4 (40) |
DMSO (1.0) |
18 |
100 |
29 |
14 |
AgCl (40) |
DMSO (1.0) |
18 |
100 |
25 |
15 |
AgOAc (40) |
DMSO (1.0) |
18 |
100 |
36 |
16 |
Ag2SO4 (20) |
DMF (1.0) |
18 |
100 |
37 |
17 |
Ag2SO4 (20) |
NMP (1.0) |
18 |
100 |
45 |
18 |
Ag2SO4 (20) |
1,4-Dioxane (1.0) |
18 |
100 |
11 |
19 |
Ag2SO4 (20) |
Isopropanol (1.0) |
18 |
100 |
9 |
20 |
Ag2SO4 (20) |
Water |
18 |
100 |
33 |
21 |
Ag2SO4 (20) |
THF |
18 |
100 |
32 |
22 |
Ag2SO4 (20) |
DCE |
18 |
100 |
2 |
23 |
Ag2SO4 (20) |
Toluene |
18 |
100 |
20 |
To further examine the efficiency of this reaction, the substrate scope was evaluated (Table 2) under the best reaction parameters (Table 1, entry 5). The results demonstrated that the indole 1a was selanylated at the C3 position with moderate to good yields employing diaryl diselenides with either electron-donating or electron-withdrawing groups (3a–e). In general, higher yields were observed with diaryl diselenides bearing electron-donating groups (3b and 3c), and longer times did not improve the yields of reactions employing diaryl diselenides with electron-withdrawing groups (3d and 3e). Moreover, sterically-hindered diaryl diselenides were tolerated; however, only moderate reaction yields were obtained (3f and 3g). The reaction also worked with dialkyl diselenides, but the compound 3h was obtained in only 38% yield. On the other hand, the reaction of 1-benzylindole or indole afforded good yields of 3i and 3j, respectively. In these cases, longer times (24 h) slightly increased the reaction yields. Surprisingly, the protocol allows the direct access to 2-selanylindoles (3l) when the C3 position of the indole ring was blocked (escatol), probably via a process similar to Plancher rearrangement.20b,22 In addition, the electron-richer 1,2-dimethylindole provided a good yield of 3k despite its more sterically-hindered C3 position, and a low yield of 3m was obtained when diphenyl disulfide was employed instead of diphenyl diselenide (2a). Finally, the method was not suitable for diaryl ditellurides, and the product 3n was not detected under standard conditions.
Table 2 Substrate scope for silver-catalyzed direct selanylation of indolesa,b
Reaction conditions: 1 (0.50 mmol), 2 (0.25 mmol), Ag2SO4 (20 mol%) and dry DMSO (1.0 mL) under air atmosphere. Isolated yields. 24 h. |
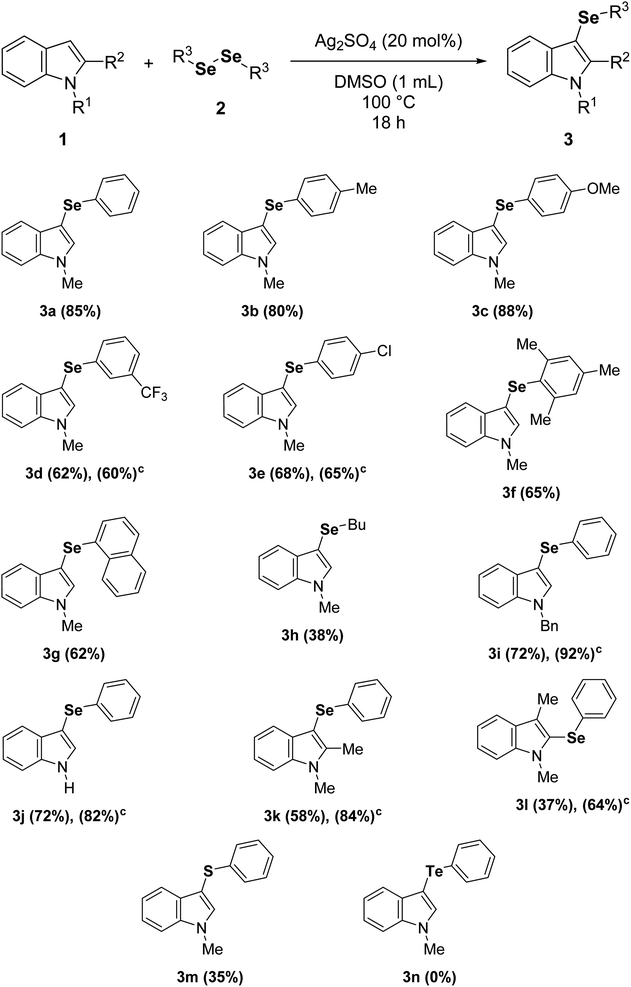 |
To obtain more insights into the mechanism of the current reaction, radical scavenging experiments were conducted by adding 1.0 equivalent of 2,6-di-tert-butyl-4-methylphenol (BHT) or 2,2,6,6-tetramethyl-1-piperidinyloxy (TEMPO). The reaction with BHT proceeded smoothly to afford the product 3a without affecting the yield (Scheme 1a), and the addition of TEMPO reduced the yield but did not completely suppress the reaction, which suggests that a radical pathway would not be dominant. The radical mechanism was further ruled out by an experiment employing 1.0 equivalent of diphenyl diselenide (2a) and TEMPO, which afforded a 70% yield of 3a (Scheme 1a). In order to evaluate if Lewis adducts between Ag(I) and diorganoyl diselenides (1) are involved in the mechanism, we conducted an experiment using anions with a high affinity to Ag(I) that can compete with diorganoyl diselenides (1) (Scheme 1b). In this way, the reaction yield of 3a was only 11% with KBr as an additive (1.0 equivalent) under optimized conditions, which indicates that these Lewis adducts could be involved. The yield of 3a was 35% when the reaction was developed under inert atmosphere (Scheme 1c), which suggests an important role for O2 from atmospheric air in this protocol. In addition, when the reaction was carried out in another polar aprotic solvent (N,N-dimethylformamide, DMF) under O2 atmosphere, the yield of 3a was increased to 45% (Scheme 1d) when compared to the reaction in DMF under air atmosphere (Table 1, entry 16; Scheme 1d). Regarding the role of O2 from atmospheric air (Scheme 1d), given the reaction stoichiometry of 1 (0.50 mmol) and 2 (0.25 mmol), it seems that O2 can restore the diorganoyl diselenides (1) by oxidation of the Ag(I)-organoselenolate.23 It is well known that DMSO can also regenerate diorganoyl diselenides (1) by oxidation of organoselenolate anions;24 however, an experiment using DMF and 5.0 equivalents of DMSO under inert atmosphere gave only 28% of 3a, further supporting the role of O2 in this reaction (Scheme 1d). Given the data obtained from the control experiments (Scheme 1), plus the reaction regioselectivity at C3 position of indoles (1), we believe that the favorable interaction between the soft Lewis acid Ag(I) and the soft Lewis base selenium on the diorganoyl diselenides (2) is crucial in reducing the diselenide bond order, then accelerating its nucleophilic cleavage by the indole derivatives (1) via electrophilic aromatic substitution.23e,25
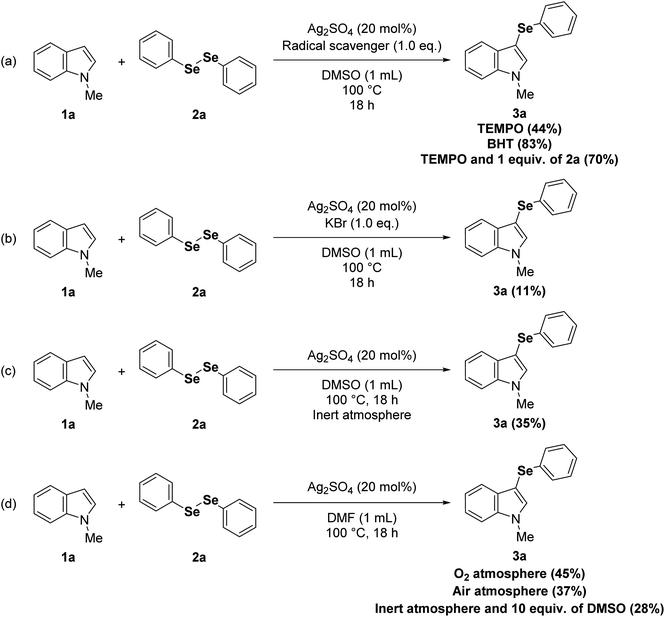 |
| Scheme 1 Control experiments for mechanistic studies. | |
In order to further shed light on the reaction mechanism, calculations at the M06-2X/BS level of theory (BS: LANL2DZ for Ag atom and 6-311++G(d,p) for other atoms) were performed (computational details are provided in the Experimental section). Considering that a radical pathway does not have support based on the experimental results (Scheme 1), additional plausible mechanistic proposals were investigated considering the reaction between 1a and 2a in the presence of Ag2SO4. In a recent work on the silver-catalyzed synthesis of diaryl selenides using arylboronic acids,26 authors proposed that the reaction mechanism involves the formation of [RSe–Ag(III)–SeR]+ as a key intermediate, leading to the final product R–Se–Ar after additional steps. We checked this hypothesis for the formation of 3a; however, all of the attempts to optimize [PhSe–Ag(III)–SePh]+ as a minimum failed, leading to 2a coordinated with Ag(I). For that reason, we turned our attention to two other mechanisms: (i) concerted metalation–deprotonation (CMD) and (ii) electrophilic aromatic substitution. For mechanism (i), the transition state structure (TSS1(i)) involved in a CMD process was located as presented in Fig. 2, consistent with the formation of a new Ag(I)–C bond concerted with the deprotonation of 1a by SO42−. For such event, an energy barrier of 28.7 kcal mol−1 was found. As mentioned, all attempts to optimize Ag(III)–phenylselenolate produced from an oxidative addition of 2a to Ag(I) as a minimum failed; however, we found that the reaction proceeds to the rate determining step forming 3a via nucleophilic attack of the C3 atom of Ag(I)–indole intermediate (INT(i)) to 2a with a barrier of 35.0 kcal mol−1 in an overall endergonic process. The CMD mechanism is commonly observed for higher valency transition metals (e.g., Pd(II), Rh(III), Ru(II)), therefore the barrier likely relies on low valence nature of Ag(I).
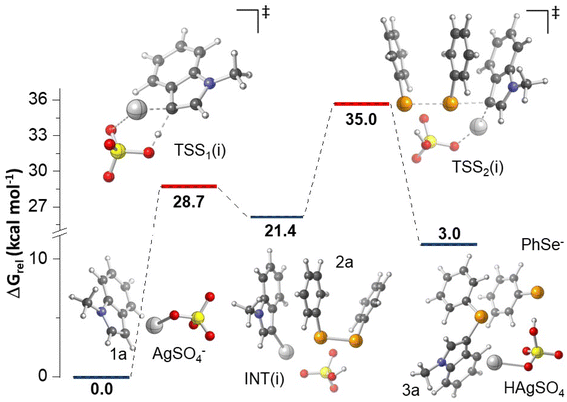 |
| Fig. 2 Potential energy surface (PES) of the CMD mechanism (i) proposed for the formation of 3a obtained using M062X functional combined with LANL2DZ (for Ag atom) and 6-311++G(d,p) (for additional atoms). Atom color: S-yellow, O-red, Se-orange, N-blue, C-gray, and H-white. | |
Regarding mechanism (ii), taking into account the solvated ions in DMSO (Ag(I) and SO42−), a TSS consistent with the nucleophilic attack of indole C3 to 2a forming a new Se–C bond was located (TSS1(ii)). As shown in Fig. 3, an initial complex formed by 1a and 2a coordinated with Ag(I) was confirmed. A rate determining energy barrier of 25.3 kcal mol−1 was observed, emphasizing the key role of the catalyst supporting the phenylselenolate departure. On the basis of bond distances, results indicate that the first step of the reaction occurs asynchronously; in the TSS1(ii), the Se–C bond formation is 87% completed, whereas the Se–Se bond is only 35% cleaved. Although we managed to locate the TSS involved in the deprotonation of the tetrahedral intermediate (INT(ii)) by the sulfate ion (TSS2(ii)), calculations suggest that the formation of 3a, along with the regeneration of the catalyst, occurs in a barrierless fashion, as TSS2(ii) features a lower energy (17.9 kcal mol−1) than the preceding stationary point (19.5 kcal mol−1), as depicted in Fig. 3. We have also investigated the deprotonation of INT(ii) promoted by Ag(I)–phenylselenolate formed in the first step. In this case, however, the results indicated a much higher energy barrier of 30.9 kcal mol−1, confirming that the reaction pathway involves sulfate ions acting as a base (details are provided in ESI†). Despite the well-known higher basicity of free phenylselenolate, it seems that the soft Lewis acid Ag(I) considerably reduces its basicity, therefore the deprotonation with free sulfate ion has a lower energy barrier. Although additional proposals could be envisioned, our calculations suggest that the classic electrophilic aromatic substitution mechanism for silver-catalyzed selanylation of indoles is predicted to be energetically feasible, considering the experimental conditions evaluated herein.
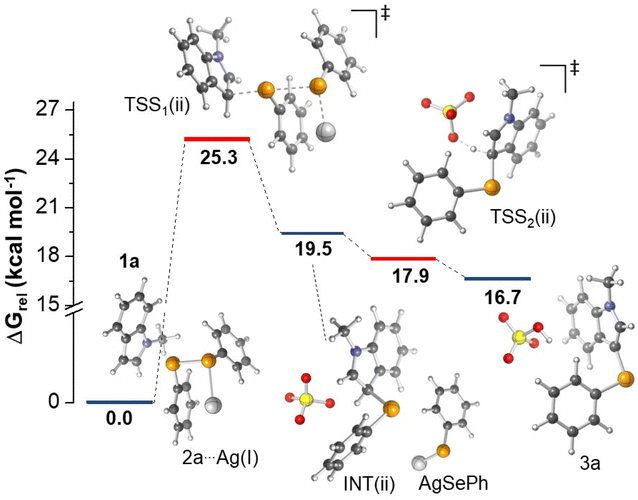 |
| Fig. 3 Potential energy surface (PES) of the electrophilic substitution mechanism (ii) proposed for the formation of 3a obtained using M062X functional combined with LANL2DZ (for Ag atom) and 6-311++G(d,p) (for additional atoms). | |
In view of the experimental data and theoretical calculations, we found that the energetically plausible mechanism follows a classic electrophilic aromatic substitution, where the interaction between the soft Lewis acid Ag(I) and the soft Lewis base selenium on the diorganoyl diselenides (2) is crucial to support the organoylselenolate departure (Fig. 3). Also, we obtained experimental data agreeing with O2 from air atmosphere restoring the diorganoyl diselenides (1) by oxidation of the Ag(I)–organoselenolates.23 Scheme 2 outlines the full catalytic cycle exemplified by 1a and 2a, which starts with the formation of a Lewis adduct between Ag(I) and diphenyl diselenide (2a) (Fig. 3, 2a⋯Ag(I)). Then, the nucleophilic attack of the C3 position of 1-methylindole (1a) to the Se–Se bond via TSS1(ii) releases the Wheland intermediate INT(ii) and Ag(I)–phenylselenolate. Finally, the aromaticity is restored via a barrierless deprotonation with a sulfate anion to afford 3a.
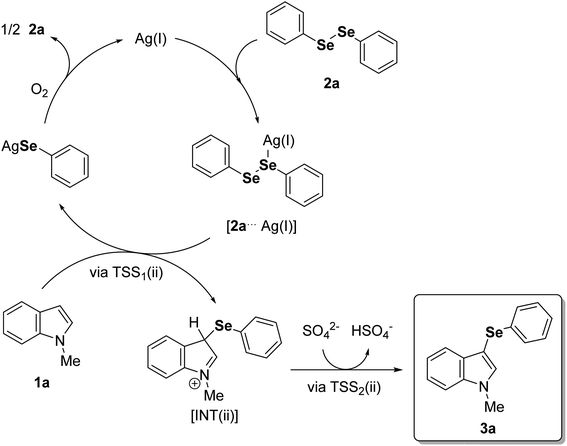 |
| Scheme 2 Full catalytic cycle. | |
Conclusions
In summary, we developed the Ag(I)-catalyzed direct selanylation of indoles with diorganoyl diselenides. The reaction gave 3-selanylindoles with high regioselectivity and also allowed the direct access to 2-selanylindoles when the C3 position of the indole ring was blocked via a process similar to Plancher rearrangement. DFT calculations failed to optimize Ag(III)–organoylselenolates to a minimum, ruling out these intermediates. Experimental and theoretical data supported an energetically plausible mechanism in which Lewis adducts between Ag(I) and diorganoyl diselenides follow an electrophilic aromatic substitution with subsequent barrierless deprotonation by a sulfate anion to afford 3-selanylindoles.
Conflicts of interest
There are no conflicts to declare.
Acknowledgements
We gratefully acknowledge the Coordenação de Aperfeiçoamento de Pessoal de Nível Superior do Brasil (CAPES) – Finance Code 001 and the Federal University of Paraná (UFPR). D. S. R. thanks Fernando da Silveira Rampon from the Content Plus Digital Agency (https://agenciamaisconteudo.com/en/) for the graphical abstract art.
References
-
(a) C.-J. Li and X. Bi, Silver Catalysis in Organic Synthesis, Wiley, 1st edn, 2019, vol. 2 CrossRef;
(b) M. Hermata, Silver in Organic Chemistry, Wiley, 1st edn, 2011 Search PubMed;
(c) A. M. Echavarren, N. Jiao and V. Gevorgyan, Coinage metals in organic synthesis, Chem. Soc. Rev., 2016, 45, 4445 RSC;
(d) A. J. Jordan, G. Lalic and J. P. Sadighi, Coinage metal hydrides: synthesis, characterization, and reactivity, Chem. Rev., 2016, 116, 8318 CrossRef CAS PubMed;
(e) V. K.-Y. Lo, A. O.-Y. Chan and C.-M. Che, Gold and silver catalysis: from organic transformation to bioconjugation, Org. Biomol. Chem., 2015, 13, 6667 RSC;
(f) M. M. Díaz-Requejo and P. J. Pérez, Coinage metal catalyzed C-H bond functionalization of hydrocarbons, Chem. Rev., 2008, 108, 3379 CrossRef PubMed;
(g) B. H. Lipshutz and Y. Yamamoto, Introduction: coinage metals in organic synthesis, Chem. Rev., 2008, 108, 2793 CrossRef CAS PubMed.
-
(a) G. Fang and X. Bi, Silver-catalysed reactions of alkynes: recent advances, Chem. Soc. Rev., 2015, 44, 8124 RSC;
(b) H. Pelissier, Enantioselective silver-catalyzed transformations, Chem. Rev., 2016, 116, 14868 CrossRef PubMed;
(c) S. Radhika, C. M. A. Abdulla, T. Aneeja and G. Anilkumar, Silver-catalysed C-H bond activation: a recent review, New J. Chem., 2021, 45, 15718 RSC;
(d) K. Sekine and T. Yamada, Sylver-catalyzed carboxylation, Chem. Soc. Rev., 2016, 45, 4524 RSC;
(e) M. Naodovic and H. Yamamoto, Asymmetric silver-catalyzed reactions, Chem. Rev., 2008, 108, 3132 CrossRef CAS PubMed;
(f) N. Kaur, N. Ahlawat, P. Bhardwaj, Y. Verma, P. Grewal and N. K. Jangid, Ag-mediated synthesis of six-membered N-heterocycles, Synth. Commun., 2020, 50, 753 CrossRef CAS;
(g) M. Neetha, T. Aneeja, C. M. A. Afsina and G. Anilkumar, An Overview of Ag-catalyzed Synthesis of Six-membered Heterocycles, ChemCatChem, 2020, 12, 5330 CrossRef CAS;
(h) P. Li, W. Wu and H. Jiang, Recent Advances in Silver-Catalyzed Transformations of Electronically Unbiased Alkenes and Alkynes, ChemCatChem, 2020, 12, 5034 CrossRef;
(i) M. Álvarez-Corral, M. Muñoz-Dorado and I. Rodríguez-García, Silver-mediated synthesis of heterocycles, Chem. Rev., 2008, 108, 3174 CrossRef PubMed;
(j) P. Sivaguru, S. Cao, K. R. Babu and X. Bi, Silver-catalyzed activation of terminal alkynes for synthesizing nitrogen-containing molecules, Acc. Chem. Res., 2020, 53, 662 CrossRef CAS PubMed.
-
(a) C. Wen, A. Yin and W.-L. Dai, Recent advances in silver-based heterogeneous catalysts for green chemistry process, Appl. Catal., B, 2014, 160, 730 CrossRef;
(b) G. J. Millar and M. Collins, Industrial production of formaldehyde using polycrystalline silver catalyst, Ind. Eng. Chem. Res., 2017, 56, 9247 CrossRef CAS.
-
(a) D. J. Gorin and F. D. Toste, Relativistic effects in homogeneous gold catalysis, Nature, 2007, 446, 395 CrossRef CAS PubMed;
(b) P. Pyykkö, Theoretical chemistry of gold, Angew. Chem., Int. Ed., 2004, 43, 4412 CrossRef PubMed;
(c) P. S. Bagus, Y. S. Lee and K. S. Pitzer, Effects of relativity and of the lanthanide contraction on the atoms from hafnium to bismuth, Chem. Phys. Lett., 1975, 33, 408 CrossRef CAS.
- Selected examples:
(a) F. Jia and B. Zhang, Mechanistic insight into the silver-catalyzed cycloaddition synthesis of 1,4-disubstituted-1,2,3-triazoles: the key of the role of silver, New J. Chem., 2019, 43, 8634 RSC;
(b) S. Wang, L.-J. Yang, J.-L. Zeng, Y. Zheng and J.-A. Ma, Silver-catalyzed [3+2] cycloaddition of isocyanides with diazo compounds: new regioselective access to 1,4-disubstituted-1,2,3-triazoles, Org. Chem. Front., 2015, 2, 1468 RSC;
(c) J. McNulty, K. Keskar and R. Vemula, The First Well-Defined Silver (I)-Complex-Catalyzed Cycloaddition of Azides onto Terminal Alkynes at Room Temperature, Chem.–Eur. J., 2011, 17, 14727 CrossRef CAS PubMed;
(d) I. Nakamura, T. Nemoto, Y. Yamamoto and A. Meijere, Thermally induced and silver-salt-catalyzed [2+2] cycloadditions of imines to (alkoxymethylene)cyclopropanes, Angew. Chem., Int. Ed., 2006, 45, 5176 CrossRef CAS PubMed;
(e) S. Zhu, R. Liang, H. Jiang and W. Wu, An Efficient Route to Polysubstituted Tetrahydronaphtols: Silver-Catalyzed [4+2] Cyclization of 2-Alkylbenzaldehydes and Alkene, Angew. Chem., Int. Ed., 2012, 51, 10861 CrossRef CAS PubMed;
(f) M. Gao, H. Chen, R. Bai, B. Cheng and A. Lei, Synthesis of pyrroles by click reaction: silver-catalyzed cycloaddition of terminal alkynes with isocyanides, Angew. Chem., Int. Ed., 2013, 52, 6958 CrossRef CAS PubMed.
- Selected examples:
(a) M. Meng, G. Wang, L. Yang, K. Cheng and C. Qi, Silver-Catalyzed Double Decarboxylative Radical Alkynylation/Annulation of Arylpropiolic Acid with α-keto Acids: Access to Ynones and Flavones under Mild Conditions, Adv. Synth. Catal., 2018, 360, 1218 CrossRef CAS;
(b) X. Liu, Z. Wang, X. Cheng and C. Li, Silver-Catalyzed Decarboxylative Alkynylation of Aliphatic Carboxylic Acids in Aqueous Solution, J. Am. Chem. Soc., 2012, 134, 14330 CrossRef CAS PubMed;
(c) F. Lazreg, M. Lesieur, A. J. Samson and C. S. J. Cazin, Light-Stable Silver N-Heterocyclic Carbene Catalysts for the Alkynylation ok Ketones in Air, ChemCatChem, 2016, 8, 209 CrossRef CAS;
(d) U. Halbes-Letinois, J.-M. Weibel and P. Pale, The organic chemistry of silver acetylides, Chem. Soc. Rev., 2007, 36, 759 RSC.
- Selected examples:
(a) M. Abreu, Y. Tang, E. Brachet, M. Selkti, V. Michelet and P. Belmont, Silver-catalyzed tandem cycloisomerization/hydroarylation reactions and mechanistic investigations for an efficient access to 1,2-dihydroisoquinolines, Org. Biomol. Chem., 2021, 19, 1037 RSC;
(b) X. Bantreil, A. Bourderioux, P. Mateo, C. E. Hagerman, M. Selkti, E. Brachet and P. Belmont, Phosphine-triggered selectivity switch in silver-catalyzed o-alkynylbenzohydroxamic acid cycloisomerizations, Org. Lett., 2016, 18, 4814 CrossRef CAS PubMed;
(c) P. Belmont and E. Parker, Silver and gold catalysis for cycloisomerization reactions, Eur. J. Org. Chem., 2009, 2009, 6075 CrossRef;
(d) J. A. Marshall and G. S. Bartley, Observations regarding the Ag(I)-catalyzed conversion of allenones to furans, J. Org. Chem., 1994, 59, 7169 CrossRef CAS;
(e) D. S. Ermolat’ev, V. P. Mehta and E. V. V. Eycken, Ag+-mediated synthesis of substituted furo[2,3-b]pyrazines, Synlett, 2007, 20, 3117 Search PubMed.
- Selected examples:
(a) M. Li, W. Wu and H. Jiang, Recent Advances in Silver-Catalyzed Transformations of Electronically Unbiased Alkenes and Alkynes, ChemCatChem, 2020, 12, 5034 CrossRef CAS;
(b) A. Luna, F. Herrera, S. Higuera, A. Murillo, I. Fernandéz and P. Almendros, AgNO3-SiO2: convinient AgNPs source for the sustainable hydrofunctionalization of alllenyl-indoles using heterogeneous catalysis, J. Catal., 2020, 389, 432 CrossRef CAS;
(c) X. Hong, F. Ma, D. Zha and H. Li, Silver-Catalyzed Stereoselective trans Addition of 4-Hydrocoumarins to Haloalkynes and Late-Stage Nitration, Asian J. Org. Chem., 2018, 7, 2552 CrossRef CAS;
(d) Z. Chen, J. Li, H. Jiang, S. Zhu, Y. Li and C. Qi, Silver-catalyzed difunctionalization of terminal alkynes: highly regio- and stereoselective synthesis of (Z)-β-haloenol acetates, Org. Lett., 2010, 12, 3262 CrossRef CAS PubMed;
(e) G. Jiang, C. Zhu, J. Li, W. Wu and H. Jiang, Silver-Catalyzed Regio- and Stereoselective Thiocyanation of Haloalkynes: Access to (Z)-Vinyl Thiocyanates, Adv. Synth. Catal., 2017, 359, 1208 CrossRef CAS;
(f) J. Zhang, L.-G. Meng, P. Li and L. Wang, The sequential reactions of tetrazoles with bromoalkynes for the synthesis of (Z)-N-(2-bromo-1-vinyl)-N-arylcyanamides and 2-arylindoles, RSC Adv., 2013, 3, 6807 RSC.
- Selected examples:
(a) R. Al-Zoubi and D. G. Hall, Mild silver(I)-mediated regioselective iodination and bromination of arylboronic acids, Org. Lett., 2010, 12, 2480 CrossRef CAS PubMed;
(b) T. Furuya and T. Ritter, Fluorination of boronic acids mediated by silver(I) triflate, Org. Lett., 2009, 11, 2860 CrossRef CAS PubMed;
(c) X. Tan, T. Song, Z. Wang, H. Chen, L. Cui and C. Li, Silver-catalyzed decarboxylative bromination of aliphatic carboxylic acids, Org. Lett., 2017, 19, 1634 CrossRef CAS PubMed;
(d) W. Wu, S. Yi, W. Huang, D. Luo and H. Jiang, Ag-catalyzed oxidative cyclization reaction of 1,6-enynes and sodium sulfinate: access to sulfonylated benzofurans, Org. Lett., 2017, 19, 2825 CrossRef CAS PubMed;
(e) Z. Wang, Comprehensive Organic Name Reactions and Reagents, Wiley, 1st edn, 2010, vol. 3 CrossRef.
- Selected examples:
(a) C. Liu, X. Wang, Z. Li, L. Cui and C. Li, Silver-catalyzed decarboxylative radical azidation of aliphatic carboxylic acids in aqueous solution, J. Am. Chem. Soc., 2015, 137, 9820 CrossRef CAS PubMed;
(b) Z. Liu, J. Liu, L. Zhang, P. Liao, J. Song and X. Bi, Silver(I)-catalyzed hydroazidation of ethynyl carbinols: synthesis of 2-azidoallyl alcohols, Angew. Chem., Int. Ed., 2014, 53, 5305 CrossRef CAS PubMed;
(c) Y. Ning, N. Wu, H. Yu, P. Liao, X. Li and X. Bi, Silver-catalyzed tandem hydroazidation/alkyne-azide cycloaddition of dyines with TMS-N3: an easy access to 1,5-fused 1,2,3-triazole frameworks, Org. Lett., 2015, 17, 2198 CrossRef CAS PubMed;
(d) G. R. Kumar, Y. K. Kumar, R. Kant and M. S. Reddy, Synthesis of benzofuranyl and indolyl methyl azides by tandem silver-catalyzed cyclization and azidation, Org. Biomol. Chem., 2016, 14, 4077 RSC;
(e) Y. Zhu, X. Li, X. Wang, X. Huang, T. Shen, Y. Zhang, X. Sun, M. Zou, S. Song and N. Jiao, Silver-catalyzed decarboxylative azidation of aliphatic carboxylic acids, Org. Lett., 2015, 17, 4702 CrossRef CAS PubMed.
- F. Liu, Z. Zhang, H.-Y. Diao and Z.-J. Shi, Silver in C(sp2)-H Functionalization, ChemCatChem, 2021, 13, 1475 CrossRef CAS.
-
(a) D. S. Rampon, E. Q. Luz, D. B. Lima, R. A. Balaguez, P. H. Schneider and D. Alves, Transition metal catalysed direct selenylation of arenes and heteroarenes, Dalton Trans., 2019, 48, 9851 RSC;
(b) D. S. Rampon, D. Seckler, E. Q. Luz, D. B. Paixão, A. Larroza, P. H. Schneider and D. Alves, Transition metal catalysed direct sulfanylation of unreactive C-H bonds: an overview of the last two decades, Org. Biomol. Chem., 2022, 20, 6072 RSC;
(c) M. C. D. F. Xavier, B. Goldani, R. F. Schumacher, G. Perin, P. H. Schneider and D. Alves, Silver-catalyzed direct selenylation of terminal alkynes through C-H bond functionalization, Mol. Catal., 2017, 427, 73 CrossRef CAS;
(d) A. M. Barcellos, M. Sacramento, G. P. Costa, G. Perin, E. J. Lenardão and D. Alves, Organoboron compounds as versatile reagents in the transition metal-catalyzed C-S, C-Se and C-Te bond formation, Coord. Chem. Rev., 2021, 442, 214012 CrossRef CAS.
-
(a) M. D. Lotz, N. M. Camasso, A. J. Canty and M. S. Sanford, Role of silver salts in palladium-catalyzed arene and heteroarene C-H functionalization reaction, Organometallics, 2017, 36, 165 CrossRef CAS;
(b) K. L. Bay, Y.-F. Yang and K. N. Houk, Multiple roles of silver salts in palladium-catalyzed C-H activations, J. Organomet. Chem., 2018, 864, 19 CrossRef CAS;
(c) T. Bhattacharya, S. Dutta and D. Maiti, Deciphering the Role of Silver in Palladium-Catalyzed C-H Functionalizations, ACS Catal., 2021, 11, 9702 CrossRef CAS;
(d) N. Kaplaneris, L. Ackermann and M. J. Johansson, Late-stage C-H functionalization offers new opportunities in drug discovery, Nat. Rev. Chem., 2021, 5, 522 CrossRef;
(e) R. L. Carvalho, G. G. Dias, C. L. M. Pereira, P. Ghosh, D. Maiti and E. N. Silva, A Catalysis Guide Focusing on C-H Activation Processes, J. Braz. Chem. Soc., 2021, 32, 917 CAS.
-
(a) H. J. Reich and R. J. Hondal, Why nature chose selenium, ACS Chem. Biol., 2016, 11, 821 CrossRef CAS PubMed;
(b) C. W. Nogueira, G. Zeni and J. B. T. Rocha, Organoselenium and organotellurium compounds: toxicology and pharmacology, Chem. Rev., 2004, 104, 6255 CrossRef CAS PubMed;
(c) D. Manna, G. Roy and G. Mugesh, Antithyroid drugs and their analogues: synthesis, structure, and mechanism of action, Acc. Chem. Res., 2013, 46, 2706 CrossRef CAS PubMed;
(d) V. K. Jain, An Overview of Organoselenium Chemistry: From Fundamentals to Synthesis in Organoselenium Compounds in Biology and Medicine: Synthesis, Biological and Therapeutic Treatments, Royal Society of Chemistry, 1st edn, 2017 RSC;
(e) E. J. Lenardão, C. Santi and L. Sancineto, New Frontiers in Organoselenium compounds, Springer, Cham, 1st edn, 2018 CrossRef;
(f) V. K. Jain and K. I. Priyadarsini, Organoselenium Compounds in Biology and Medicine: Synthesis, Biological and Therapeutic Treatments, Royal Society of Chemistry, 1st edn, 2018 Search PubMed;
(g) C. W. Nogueira, N. V. Barbosa and J. B. T. Rocha, Toxicology and pharmacology of synthetic organoselenium compounds: an update, Arch. Toxicol., 2021, 95, 1179 CrossRef CAS PubMed;
(h) M. Liu, X. Zhang, S. Chu, Y. Ge, T. Huang, Y. Liu and L. Yu, Selenization of cotton products with NaHSe endowing the antibacterial activities, Chin. Chem. Lett., 2022, 33, 205 CrossRef CAS;
(i) H. Cao, R. Ma, S. Chu, J. Xi, L. Yu and R. Guo, Synergistic effect of T80/B30 vesicles and T80/PN320 mixed micelles with Se/C on nasal mucosal immunity, Chin. Chem. Lett., 2021, 32, 2761 CrossRef CAS;
(j) L. Xian, Q. Li, T. Li and L. Yu, Methylselenized glucose: An efficient organoselenium fertilizer enhancing the selenium content in wheat grains, Chin. Chem. Lett., 2022 DOI:10.1016/j.cclet.2022.107878;
(k) X. Xiao, Z. Shao and L. Yu, A perspective of the engineering applications of carbon-based selenium-containing materials, Chin. Chem. Lett., 2021, 32, 2933 CrossRef CAS;
(l) H. Cao, Y. Yang, X. Chen, J. Liu, C. Chen, S. Yuan and L. Yu, Synthesis of selenium-doped carbon from glucose: An efficient antibacterial material against Xcc, Chin. Chem. Lett., 2020, 31, 1887 CrossRef CAS.
-
(a) G. Perin, E. J. Lenardão, R. G. Jacob and R. B. Panatieri, Synthesis of vinyl selenides, Chem. Rev., 2009, 109, 1277 CrossRef CAS PubMed;
(b) M. Godoi, M. W. Paixão and A. L. Braga, Chiral organoselenium-transition-metal catalysts in asymmetric transformation, Dalton Trans., 2011, 40, 11347 RSC;
(c) D. M. Freudendahl, S. Santoro, S. A. Shahzad, C. Santi and T. Wirth, Green chemistry with selenium reagents: development of efficient catalytic reactions, Angew. Chem., Int. Ed., 2009, 48, 8409 CrossRef CAS PubMed;
(d) S. Santoro, J. B. Azeredo, V. Nascimento, L. Sancineto, A. L. Braga and C. Santi, The green side of the moon: ecofriendly aspects of organoselenium chemistry, RSC Adv., 2014, 4, 31521 RSC;
(e) T. Guo, Z. Li, L. Bi, L. Fan and P. Zhang, Recent advances in organic synthesis applying elemental selenium, Tetrahedron, 2022, 112, 132752 CrossRef CAS;
(f) M. S. Silva, D. Alves, D. Hartwig, R. G. Jacob, G. Perin and E. J. Lenardão, Selenium-NMR Spectroscopy in Organic Synthesis: From Structural Characterization Towards New Investigations, Asian J. Org. Chem., 2021, 10, 91 CrossRef CAS;
(g) F. V. Singh and T. Wirth, Selenium and Tellurium Electrophiles in Organic Synthesis, Phys. Sci. Rev., 2019, 4, 20170131 Search PubMed.
-
(a) S. M. Lee, H. R. Lee, G. Dutta, J. Lee, J. H. Oh and G. Yang, Furan-flanked diketopyrrolopyrrole-based chalcogenophene copolymers with siloxane hybrid side chains for organic field-effect transistors, Polym. Chem., 2019, 10, 2854 RSC;
(b) C.-C. Liu, S.-W. Mao and M.-Y. Kuo, Cyanated pentaceno[2,3-c]chalcogenophenes for potential application in air-stable ambipolar organic thin-film transistors, J. Phys. Chem. C, 2010, 114, 22316 CrossRef CAS;
(c) Z. Yi, S. Wang and L. Liu, Design of high-mobility diketopyrrolopyrrole-based π-conjugated copolymers for organic thin-film transistors, Adv. Mater., 2015, 27, 3589 CrossRef CAS PubMed;
(d) K. Takimiya, Y. Kunugi, Y. Konda, H. Ebata, Y. Toyoshima and T. Otsubo, 2,7-Diphenyl[1]benzoselenopheno[3,2-b][1]benzoselenophene as a stable organic semiconductor for a high performance field-effect transistor, J. Am. Chem. Soc., 2006, 128, 3044 CrossRef CAS PubMed;
(e) T. Yamamoto and K. Takimiya, Facile synthesis of highly π-extended heteroarenes, dinaphto[2,3-b: 2’, 3’-f]chalcogenophenes[3,2-b]chalcogenophenes, and their application to field-effect, J. Am. Chem. Soc., 2007, 129, 2224 CrossRef CAS PubMed;
(f) Y. An, J. Oh, S. Chen, B. Lee, S. M. Lee, D. Han and C. Yang, Effects of incorporating different chalcogenophene comonomers into random acceptor terpolymers on the morphology and performance of all-polymer solar cells, Polym. Chem., 2018, 9, 593 RSC;
(g) S. Yamaguchi, C. Xu and T. Okamoto, Ladder π-conjugated materials with main group elements, Pure Appl. Chem., 2006, 78, 721 CAS;
(h) P. Arsenyan, A. Petrenko, K. Leitonas, D. Volyniuk, J. Simokaitiene, T. Klinavičius, E. Skuodis, J.-H. Lee and J. V. Gražulevičius, Synthesis and performance in OLEDs of selenium-containing phosphorescent emitters with red emission color deeper than the corresponding NTSC standard, Inorg. Chem., 2019, 58, 10174 CrossRef CAS PubMed;
(i) C. Wang, H. Dong, W. Hu, Y. Liu and D. Zhu, Semiconducting π-conjugated systems in field-effect transistors: a material odyssey of organic electronics, Chem. Rev., 2012, 112, 2208 CrossRef CAS PubMed;
(j) B. Kim, H. R. Yeom, M. H. Yun, J. Y. Kim and C. Yang, A selenophene analogue of PCDTBT: selective fine-tuning of LUMO to lower of the bandgap for efficient polymer solar cells, Macromolecules, 2012, 45, 8658 CrossRef CAS;
(k) S. Haid, A. Mishra, C. Uhrich, M. Pfeiffer and P. Bäuerle, Dicyanovinylene-substituted selenophene-thiophene co-oligomers for small-molecule organic solar cells, Chem. Mater., 2011, 23, 4435 CrossRef CAS;
(l) A. J. Kronemeijer, E. Gili, M. Shahid, J. Rivnay, A. Salleo, M. Heeney and H. Sirringhaus, A selenophene-based low-bandgap donor-acceptor polymer leading to fast ambipolar logic, Adv. Mater., 2012, 24, 1558 CrossRef CAS PubMed;
(m) K. Yamada, T. Okamoto, K. Kudoh, A. Wakamiya and S. Yamaguchi, Single-crystal field-effect transistors of benzoannulated fused oligothiphenes and oligoselenophenes, Appl. Phys. Lett., 2007, 90, 72102 CrossRef;
(n) D. S. Rampon, F. S. Rodembusch, J. M. F. M. Schneider, I. H. Bechtold, P. F. B. Gonçalves, A. A. Merlo and P. H. Schneider, Novel selenoesters fluorescent liquid crystalline exhibiting a rich phase polymorfism, J. Mater. Chem., 2010, 20, 715 RSC.
-
(a) S. Zhang, B. An, J. Li, J. Hu, L. Huang, X. Li and A. S. C. Chan, Synthesis and evaluation of selenium-containing indole chalcone and diarylketone derivatives as tubulin polymerization inhibition agents, Org. Biomol. Chem., 2017, 15, 7404 RSC;
(b) P. T. Birmann, F. S. S. Sousa, D. H. Oliveira, M. Domingues, B. M. Vieira, E. J. Lenardão and L. Savegnago, 3-(4-Chlorophenylselanyl)-1-methyl-1H-indole, a new selenium compound elicits an antinociceptive and anti-inflammatory effects in mice, Eur. J. Pharmacol., 2018, 827, 71 CrossRef CAS PubMed;
(c) A. M. Casaril, M. T. Ignasiak, C. Y. Chuang, B. Vieira, N. B. Padilha, L. Carroll, E. J. Lenardão, L. Savegnago and M. J. Davies, Selenium-containing indolyl compounds: kinetics of reactions with inflammation-associated oxidants and protective effect against oxidation of extracellular matrix proteins, Free Radical Biol. Med., 2017, 113, 395 CrossRef CAS PubMed;
(d) Z. Wen, J. Xu, Z. Wang, H. Qi, Q. Xu, Z. Bai, Q. Zhang, K. Bao, Y. Wu and W. Zhang, 3-(3,4,5-Trimethoxyphenylselenyl)-1H-indoles and their selenoxides as combretastatin A-4 analogs: microwave-assisted synthesis and biological evaluation, Eur. J. Med. Chem., 2015, 90, 184 CrossRef CAS PubMed;
(e) Q. Guan, C. Han, D. Zuo, M. Zhai, Z. Li, Q. Zhang, Y. Zhai, X. Jiang, K. Bao, Y. Wu and W. Zhang, Synthesis and evaluation of benzimidazole carbamates bearing indole moieties for antiproliferative and antitubulin activities, Eur. J. Med. Chem., 2014, 87, 306 CrossRef CAS PubMed;
(f) G. La Regina, R. Bai, W. M. Rensen, E. Di Cesare, A. Coluccia, F. Piscitelli, V. Famiglini, A. Reggio, M. Nalli, S. Pelliccia, E. Pozzo, B. Costa, I. Granata, A. Porta, B. Maresca, A. Soriani, M. L. Iannitto, A. Santoni, J. Li, M. M. Cona, F. Chen, Y. Ni, A. Brancale, G. Dondio, S. Vultaggio, M. Varasi, C. Mercurio, C. Martini, E. Hamel, P. Lavia, E. Novellino and R. Silvestri, Toward highly potent cancer agents by modulating the C-2 group of the arylthioindole class of tubulin polymerization inhibitors, J. Med. Chem., 2013, 56, 123 CrossRef CAS PubMed;
(g) M. Nuth, H. Guan, N. Zhukovskaya, Y. L. Saw and R. P. Ricciardi, Design of potent poxvirus inhibitor of the heterodimeric processivity factor required for viral replication, J. Med. Chem., 2013, 56, 3235 CrossRef CAS PubMed.
-
(a) D. Huang, H. Yu, T. Wang, H. Yang, R. Yao and Z. Liang, Efficacy and safety of umifenovir for coronavirus disease 2019 (COVID19): a systematic and meta-analysis, J. Med. Virol., 2020, 93, 481 CrossRef PubMed;
(b) J. Haviernik, M. Stefánik, M. Fojtíková, S. Kali, N. Tordo, I. Rudolf, Z. Hubalék, L. Eyer and D. Ruzek, Arbidol (Umifenovir): a broad-spectrum antiviral drug that inhibits medically impotant arthropod-borne flaviviruses, Viruses, 2018, 10, 184 CrossRef PubMed;
(c) A. M. Rapoport, M. E. Bigal, M. Volcy, F. D. Sheftell, M. Fellepa and S. J. Tepper, Naratriptan in the preventive treatment of refractory chronic migraine: a review of 27 cases, Headache, 2003, 43, 482 CrossRef PubMed;
(d) B. Casolla, L. Lionetto, S. Candela, L. D'Alonzo, A. Negro, M. Simmaco and P. Martelletti, Treatment of perimenstural migraine with triptans: un update, Curr. Pain Headache Rep., 2012, 16, 445 CrossRef PubMed;
(e) N. T. Mathew, Naratriptan: a review, Expert Opin. Invest. Drugs, 2005, 8, 687 CrossRef PubMed.
-
(a) W. Ma, N. Kaplaneris, X. Fang, L. Gu, R. Mei and L. Ackermann, Chelation-assisted transition metal-catalysed C-H chalcogenylations, Org. Chem. Front., 2020, 7, 1022 RSC;
(b) M. Iwasaki and Y. Nishihara, Palladium-catalysed direct thiolation and selenation of aryl C-H bonds assisted by directing groups, Dalton Trans., 2016, 45, 15278 RSC;
(c) J. Rafique, D. S. Rampon, J. B. Azeredo, F. L. Coelho, P. H. Schneider and A. L. Braga, Light-Mediated Seleno-Functionalization of Organic Molecules: Recent Advances, Chem. Rec., 2021, 21, 2739 CrossRef CAS PubMed;
(d) G. M. Martins, A. G. Meirinho, N. Ahmed, A. L. Braga and S. R. Mendes, Recent advances in electrochemical chalcogen (S/Se)-functionalization of organic molecules, ChemElectroChem, 2019, 6, 5928 CrossRef CAS.
-
(a) Y. Cao, J. Liu, F. Liu, L. Jiang and W. Yi, Copper-catalyzed direct and odorless selenylation with a sodium selenite-based reagent, Org. Chem. Front., 2019, 6, 825 RSC;
(b) E. Q. Luz, D. Seckler, J. S. Araújo, L. Angst, D. B. Lima, E. A. M. Rios, R. R. Ribeiro and D. S. Rampon, Fe(III)-Catalyzed direct C3 chalcogenylation of indoles: the effect of iodide ions, Tetrahedron, 2019, 75, 1258 CrossRef CAS;
(c) B. M. Vieira, S. Thurow, M. da Costa, A. M. Casaril, M. Domingues, R. F. Schumacher, G. Perin, D. Alves, L. Savegnano and E. J. Lenardão, Ultrasound-assisted synthesis and antioxidant activity of 3-selanyl-1H-indole and 3-selanylimidazo[1,2-a]pyridine derivatives, Asian J. Org. Chem., 2017, 6, 1635 CrossRef CAS;
(d) L. Dongping, G. Wu, H. Yang, M. Liu, W. Gao, X. Huang, J. Chen and H. Wu, Copper-catalyzed three-component reaction for regioselective aryl- and heteroarylselenation of indoles using selenium powder, J. Org. Chem., 2016, 81, 4485 CrossRef PubMed;
(e) T. Guo, Z. Dong, P. Zhang, W. Xing and L. Li, Direct selenation of imidazoheterocycles and indoles with selenium powder in a copper-catalyzed three-component one-pot system, Tetrahedron Lett., 2018, 59, 2554 CrossRef CAS;
(f) B. M. Vieira, S. Thurow, J. S. Brito, G. Perin, D. Alves, R. G. Jacob, C. Santi and E. J. Lenardão, Sonochemistry: an efficient alternative to the synthesis of 3-selanylindoles using CuI as catalyst, Ultrason. Sonochem., 2015, 27, 192 CrossRef CAS PubMed;
(g) N. Mukherjee, D. Kundu and B. C. Ranu, Copper-Silver Dual Catalyzed Decyanative C-Se Cross-Coupling, Adv. Synth. Catal., 2017, 359, 329 CrossRef CAS;
(h) S. Guan, Y. Chen, H. Wu and R. Xu, Iron-catalyzed tandem reaction of C-Se bond coupling/selenosulfonation of indoles with benzeneselenols, RSC Adv., 2020, 10, 27058 RSC.
-
(a) I. D. Lemir, W. D. Castro-Godoy, A. A. Heredia, L. C. Schmidt and J. E. Arguello, Metal- and photocatalyst- free synthesis of 3-selenylindoles and asymmetric diarylselenides promoted by visible light, RSC Adv., 2019, 9, 22685 RSC;
(b) N. L. Ferreira, J. B. Azeredo, B. L. Fiorentin and A. L. Braga, Synthesis of 3-Selenylindoles under Eco-friendly Conditions, Eur. J. Org. Chem., 2015, 2015, 5070 CrossRef CAS;
(c) X. Zhang, C. Wang, H. Jiang and L. Sun, Convenient synthesis of selenyl-indoles via iodide ion-catalyzed electrochemical C-H selenation, Chem. Commun., 2018, 54, 8781 RSC;
(d) W. Zhang, S. Li, X. Tang, J. Tang, C. Pan and Y. Guipeng, Phenothiazine core promoted charge transfer in conjugated microporous polymers for photocatalytic Ugi-type reaction and aerobic selenation of indoles, Appl. Catal., B, 2020, 272, 118982 CrossRef CAS;
(e) R. Jamal, S. Sumbal, M. S. Franco, L. Bettanin, A. R. Schneider, L. T. Silva and A. L. Braga, Direct, Metal-free C(sp2) Chalcogenation of Indoles and Imidazopyridines with Dichalcogenides Catalysed by KIO3, Chem.–Eur. J., 2018, 24, 4173 CrossRef PubMed;
(f) H. Li, J. Wang, X. Wang and J. Yan, NaBr mediated regioselective synthesis of 3-selanylindoles, Phosphorus, Sulfur Silicon Relat. Elem., 2018, 193, 394 CrossRef CAS;
(g) V. Rathore and S. Kumar, Visible-light-induced metal and reagent-free oxidative coupling of sp2 C-H bond with organodichalcogenides: synthesis of 3-organochalcogenyl indoles, Green Chem., 2019, 21, 2670 RSC;
(h) F. L. Coelho, E. S. Gil, P. F. B. Gonçalves, L. F. Campo and P. H. Schneider, Intramolecular Hydroamination of Selenoalkynes to 2-Selenylindoles in the Absence of Catalyst, Eur. J. Chem., 2019, 25, 8157 CrossRef CAS PubMed;
(i) X. Zhang, C. Wang, H. Jiang and L. Sun, A low-cost electrochemical thio- and selenocyanation strategy for electron-rich arenes under catalyst- and oxidant-free condition, RSC Adv., 2018, 8, 22042 RSC;
(j) J. B. Azevedo, M. Godoi, G. M. Martins, C. C. Silveira and A. L. Braga, A solvent- and metal-free synthesis of 3-chalcogenyl-indoles employing DMSO/I2 as an eco-friendly catalytic oxidation system, J. Org. Chem., 2014, 79, 4125 CrossRef PubMed.
- Plancher's Rearrangement:
(a) Z. Wang, Comprehensive Organic Name Reactions and Reagents, 1st edn, Wiley, vol. 3, 2010 CrossRef;
(b) M. Mari, S. Lucarini, F. Bartoccini, G. Piersanti and G. Spadoni, Synthesis of 2-substituted tryptophans via a C3- to C2-alkyl migration, Beilstein J. Org. Chem., 2014, 10, 1991 CrossRef PubMed;
(c) A. H. Jackson and P. Smith, Electrophilic substitution in indoles–III: rearrangement of 3,3-dialkylindolenines, Tetrahedron, 1968, 24, 2227 CrossRef CAS;
(d) A. H. Jackson, B. Naidoo and P. Smith, Electrophilic substitution in indoles – IV: The cyclization of indolylbutanol to tetrahydrocarbazole, Tetrahedron, 1968, 24, 6119 CrossRef CAS;
(e) K. M. Biswas and A. H. Jackson, Electrophilic substitution in indoles-V: Indolenine as intermediates in the benzylation of 3-substituted indoles, Tetrahedron, 1969, 25, 227 CrossRef CAS;
(f) K. G. Liu, A. J. Robichaud, J. R. Lo, J. F. Mattes and Y. Cai, Rearrangement of 3,3-disubstituted indolenine and synthesis of 2,3-substituted indoles, Org. Lett., 2006, 8, 5769 CrossRef CAS PubMed;
(g) P. Hamel and P. Preville, Regioselective synthesis of mixed indole 2,3-bis(sulfides). A study of the mechanism of the second sulfenylation of Indoles, J. Org. Chem., 1996, 61, 1573 CrossRef CAS PubMed.
-
(a) M. Hirano, M. Monobe, S. Yakabe and T. Morimoto, Hydrotalcite clay-catalyzed air oxidation of thiols, J. Chem. Res., Synop., 1999, 6, 374 Search PubMed;
(b) J. Xan, E. A. Wilson, L. D. Roberts and N. H. Norton, The absorption of oxygen by mercaptans in alkaline solution, J. Am. Chem. Soc., 1941, 63, 1139 CrossRef CAS;
(c) C. F. Cullis, J. D. Hopton and D. L. Trimm, Oxidation of thiols in gas-liquid systems. Reaction in the presence of added metal catalysts, J. Appl. Chem., 1968, 18, 335 CrossRef CAS;
(d) P. C. B. Page, R. D. Wilkes and D. Reynolds, Comprehensive Organic Functional Group Transformations, vol. 2, Pergamon Oxford, 1995 Search PubMed;
(e) D. B. Lima, P. H. V. Santos, P. Fiori, G. Badshah, E. Q. Luz, D. Seckler and D. S. Rampon, Base-Promoted Direct Chalcogenylation of 2-Naphtols, ChemistrySelect, 2019, 13, 13558 CrossRef;
(f) Y. Ni, H. Zuo, Y. Li, Y. Wu and F. Zhong, Cooper-catalyzed regioselective intramolecular electrophilic sulfenoamination via Lewis acid activation of disulfides under aerobic conditions, Org. Lett., 2018, 20, 4350 CrossRef CAS PubMed.
-
(a) C. N. Yiannios and J. V. Karabinos, Oxidation of thiols by dimethyl sulfoxide, J. Org. Chem., 1963, 11, 3246 CrossRef;
(b) J.-G. Sun, W.-Z. Weng, P. Li and B. Zhang, Dimethyl sulfoxide as a mild oxidant in S-P(O) bond construction: simple and metal-free approaches to phosphinothioates, Green Chem., 2017, 19, 1128 RSC;
(c) E. Q. Luz, G. L. Silvério, D. Seckler, D. B. Lima, F. S. Santana, R. V. Barbosa, C. R. M. D'Oca and D. S. Rampon, One-Pot Synthesis of 3-Halo-2-organochalcogenylbenzo[b]chalcogenophenes from 1-(2,2-Dibromovinyl)-2-organochalcogenylbenzenes, Adv. Synth. Catal., 2021, 363, 2610 CrossRef CAS;
(d) M. Iwasaki, Y. Tsuchiya, K. Nakajima and Y. Nishihara, Chelate-assisted direct selenation of aryl C-H bonds with diselenides catalyzed by palladium, Org. Lett., 2014, 16, 4920 CrossRef CAS PubMed;
(e) K. S. Santos, E. M. A. Sandagorda, R. Cargnelutti, T. Barcellos, R. G. Jacob, D. Alves and R. F. Schumacher, Copper-Catalyzed Selective Synthesis of 5-Selanyl-imidazo[2,1-b]thiazoles, ChemistrySelect, 2017, 2, 10793 CrossRef CAS;
(f) S. F. Fonseca, N. B. Padilha, S. Thurow, J. A. Roehrs, L. Svegnago, M. N. Souza, M. G. Fronza, T. Collares, J. Buss, F. K. Seixas, D. Alves and E. J. Lenardão, Ultrasound-promoted copper-catalyzed synthesis of bis-arylselanyl chrysin derivatives with boosted antioxidant and anticancer activities, Ultrason. Sonochem., 2017, 39, 827 CrossRef CAS PubMed;
(g) M. Iwasaki, M. Iyanaga, Y. Tsuchiya, Y. Nishimura, W. Li, Z. Li and Y. Nishihara, Palladium-Catalyzed Direct Thiolation of Aryl C-H Bonds with Disulfides, Chem.–Eur. J., 2014, 20, 2459 CrossRef CAS PubMed;
(h) W. E. Fristad and J. R. Peterson, Oxidative coupling of arylthiols to diaryl disulfides, Synth. Commun., 1985, 15, 1 CrossRef CAS;
(i) T. J. Wallace, Reactions of thiols with sulfoxides. Scope of the reaction and synthetic applications, J. Am. Chem. Soc., 1964, 86, 2018 CrossRef CAS;
(j) T. Zhang, G. Deng, H. Li, B. Liu, Q. Tan and B. Xu, Cyclization of 2-Biphenylthiols to Dibenzothiophenes under PdCl2/DMSO Catalysis, Org. Lett., 2018, 20, 5439 CrossRef CAS PubMed;
(k) C. Zhang, J. McClure and J. Chou, Silver-catalyzed direct thiolation of quinones by activation of aryl disulfides to synthesize quinonyl aryl thioethers, J. Org. Chem., 2015, 80, 4919 CrossRef CAS PubMed;
(l) A. Gupta, A. Rahaman and S. Bhadra, Direct α-chalcogenation of aliphatic carboxylic acid equivalents, Org. Lett., 2019, 21, 6164 CrossRef CAS PubMed;
(m) J.-M. Li, Y. Yu, J. Weng and G. Lu, Nickel-catalyzed direct C-H bond sulfenylation of acylhydrazines, Org. Biomol. Chem., 2018, 16, 6047 RSC.
-
(a) P. Gogoi, S. R. Gogoi, M. Kalita and P. Barman, Silver ion mediated in situ synthesis of mixed diaryl sulfides from diaryl disulfides, Synlett, 2013, 24, 873 CrossRef CAS;
(b) A. Thupyai, C. Pimpasri and S. Yotphan, DABCO-catalyzed silver-promoted direct thiolation of pyrazolones with diaryl disulfides, Org. Biomol. Chem., 2018, 16, 424 RSC;
(c) M. Bortoli, L. P. Wolters, L. Orian and F. M. Bickelhaupt, Addition-elimination or nucleophilic substitution? Understanding the energy profiles for the reaction of chalcogenolates with dichalcogenides, J. Chem. Theory Comput., 2016, 12, 2752 CrossRef CAS PubMed;
(d) G. S. Heverly-Coulson, R. J. Boyd, O. Mó and M. Yanez, Revealing Unexpected Mechanisms for Nucleophilic Attack on S-S and Se-Se Bridges, Chem.–Eur. J., 2013, 19, 3629 CrossRef CAS PubMed.
- B. Goldani, V. G. Ricordi, N. Seus, E. J. Lenardão, R. F. Schumacher and D. Alves, Silver-catalyzed synthesis of diaryl selenides by reaction of diaryl diselenides with aryl boronic acids, J. Org. Chem., 2016, 81, 11472 CrossRef CAS PubMed.
|
This journal is © The Royal Society of Chemistry 2023 |