The multifaceted effects of DMSO and high hydrostatic pressure on the kinetic constants of hydrolysis reactions catalyzed by α-chymotrypsin†
Received
7th June 2020
, Accepted 6th July 2020
First published on 6th July 2020
Abstract
The use of cosolvents and high hydrostatic pressure (HHP) has been described as an efficient means to modulate the stability of enzymes and their catalytic activity. Cosolvents and pressure can lead to increased reaction rates without affecting the stability of the enzyme. Here, we studied the combined effects of one of the most used organic cosolvents, dimethyl sulfoxide (DMSO), and HHP to reveal their combined effect on the kinetic constants of an α-chymotrypsin-catalyzed peptide hydrolysis reaction. The Michaelis constant and the turnover number of the reaction respond differently to the two variables, and we observed an opposite effect of hydrostatic pressure and the dipolar cosolvent DMSO on the kinetic parameters. The results could be rationalized by determining the volume diagram of the reaction at the different solution conditions. In our case, the use of high hydrostatic pressure in concert with DMSO does not lead to an improvement of the enzymatic activity. However, the advantages of DMSO and HHP to increase the temperature stability of the enzyme and to increase the solubility of more hydrophobic substrates could still be useful.
Introduction
Enzymes are specific proteins that act as the cell's own biological catalysts. They catalyze almost all chemical reactions which are necessary to sustain life processes in cells. To this end, not only the great selectivity and catalytic efficiency of the enzymes, but also mild reaction conditions are required.1 In the fields of biotechnology, pharmaceuticals and the food industry, the interest in such biocatalytic processes has continuously increased in recent years, as they often offer better options than chemically catalyzed pathways.2–5 One of the major drawbacks of the use of enzymes is their insufficient stability under industrial processing conditions, which demand that an enzyme be also robust with long shelf life and high chemical and thermal stability.6 Numerous studies, known as protein engineering, are being conducted to develop new, tailor-made enzymes for technical applications.7 However, the optimum of the process parameters (e.g., temperature, pH) of enzyme-catalyzed reactions is generally within a small range, which can lead to limited stability of the enzymes as well as low reaction rates and yields under industrial process conditions.8
By using the pressure variable, the range of process conditions can be extended.5,7,9–14 Hydrostatic pressures have been shown to be able to stabilize some enzymes at higher temperatures and modulate their conformational dynamics and reaction rates.5,7,9–16 In fact, nature has explored the effect of pressure on enzymatic reactions, as many organisms thrive at high-pressure conditions in the deep sea, where pressures up to 1 kbar (100 MPa) and beyond are encountered.17–19 The enzymes operating in the deep sea have adapted themselves to the pressure in mainly two ways. On the one hand, some enzymes can adapt intrinsically to the high pressure, i.e. by mutations. On the other hand, enzymes may be stabilized by upregulation of particular cellular natural cosolvents, such as trimethylamine-N-oxide.20,21
A further possibility to increase the stability of enzymes and to improve the solubility of substrates, suppress water-dependent side reactions and direct the enantioselectivity of the reaction is the use of cosolvents (‘solvent engineering’), such as dimethyl sulfoxide (DMSO).2,7,22 DMSO is known as the most powerful organic solvent currently available due to the wide variety of dissolving organic substances such as carbohydrates, polymers, peptides and many inorganic salts and gases, with loading levels of up to 50–60 wt%. Fundamental to the solvating ability is the high dielectric constant and the chemical-make-up, i.e. the dipolar character of DMSO. Further, DMSO is miscible with water and does not form azeotropes. Moreover, DMSO has a low environmental toxicity.22 Depending on the concentration, DMSO is able to stabilize proteins, i.e. to enhance the denaturation temperature of proteins, e.g. of lipase and amylase.23,24 Thereby, the hydrophilic properties of DMSO as well as the possibility to enhance the characteristic three-dimensional water structure seem to be of importance.25 But DMSO may also act as a denaturant, e.g. for hen egg white lysozyme.26–28 Such unfolding is possible if the non-polar side chains are exposed to DMSO and the unfolded structure binds more DMSO.29 It is also known that DMSO is an inhibitor, activator or molecular chaperone for some enzymatic reactions and may change the enantioselectivity of the reaction.27,30–39 Eremeev et al. describe a critical concentration beyond which the conformational changes induced in the protein globule by the solvent are irreversible. For α-chymotrypsin, this concentration is 4.2 M DMSO.40
Whereas the effects of DMSO or pressure on enzymatic reactions have been studied in the past, the combined effect of both parameters in concert on enzymatic reactions is essentially unknown. In this work, we studied the effect of high hydrostatic pressure (HHP) and DMSO concentration on the activity of the enzyme α-chymotrypsin (α-CT) using the fluorescent model peptide alanine-alanine-phenylalanine-7-amido-4-methylcoumarin (AAF-AMC) as substrate. The enzyme selectively hydrolyzes the amide bond at the C-terminus of aromatic residues (Scheme 1). Tretyakova et al. have shown that DMSO at concentrations up to 20 vol% is able to stabilize α-chymotrypsin against temperature denaturation.41
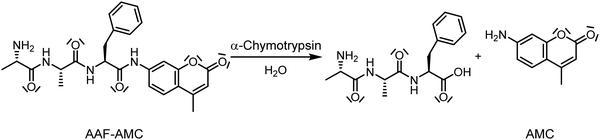 |
| Scheme 1 Representation of the cleavage of the amide bond of the substrate alanine-alanine-phenylalanine-7-amido-4-methylcoumarin (AAF-AMC) by α-chymotrypsin. After the hydrolysis, the product 7-amido-4-methylcoumarin (AMC) is released.42 | |
Materials and methods
Materials
The lyophilized powder of the enzyme α-chymotrypsin (α-CT) from bovine pancreas, the substrate AAF-AMC (alanine-alanine-phenylalanine-7-amido-4-methylcoumarin), the reaction product AMC (7-amido-4-methylcoumarin) and dimethyl sulfoxide (DMSO) were obtained from Sigma-Aldrich and used without further purification. All the solutions were prepared in pressure stable buffer (0.1 M Tris–HCl and CaCl2 10 mM, pH 7.8). Deionized water was used for the buffer and all sample preparations.
Sample preparation
A Tris buffer solution with and without DMSO (0, 5, 10 or 20 vol%) was adjusted at a pH value of 7.8 by titration with hydrochloric acid and filtered using a syringe filter (0.45 μm). A 1 mM stock solution of the product AMC (ε370nm = 7600 M−1 cm−1) was prepared by adding a small aliquot of AMC previously dissolved in pure DMSO to the respective buffer (0, 5, 10 or 20 vol% DMSO).43 Starting from this solution, 2 mL solutions of 1 μM, 2 μM, 3 μM and 4 μM AMC were prepared for the calibration. A 7.5 μM stock solution of the enzyme α-CT (ε280nm = 51000 M−1 cm−1) was prepared by dilution in the respective buffer (0, 5, 10 or 20 vol%).44 From this stock solution, 2 μL were added to each sample to be fluorescence-spectroscopically examined. The substrate AAF-AMC (ε325nm = 16
000 M−1 cm−1) was prepared in DMSO at a concentration of 29.05 mM.43 A certain amount of this stock solution was added to the sample solution depending on the substrate concentration investigated (in the range 20–135 μM). The reaction mixture was filled up with the appropriate buffer to the final volume of 1500 μL. For the circular dichroism (CD) spectroscopic measurements, the concentration of α-CT was set to 100 μM for all water/DMSO mixtures investigated. The exact concentrations of the enzyme, the substrate AAF-AMC and the product AMC were determined by UV spectroscopy (UV-1800 spectrometer from Shimadzu Corporation).
Fluorescence spectroscopy – kinetics
To investigate the catalytic activity of α-CT, fluorescence measurements were carried out by means of a K2 Multifrequency Phase Fluorometer from ISS Inc. (Champaign, IL, USA) at the temperature of 20 °C. The light source in this instrument was a xenon arc lamp. For the intensity measurements, the slit widths for both the excitation and emission monochromator were set to 8 nm. The excitation wavelength was set at 370 nm while the emission wavelength was set at 460 nm. At 460 nm, the release of the reported group AMC can be followed without any interference from the substrate.8 For measurements at atmospheric pressure, the prepared samples were filled into a quartz cuvette with a path length of 1.0 cm. To examine the enzyme kinetics of α-CT under high hydrostatic pressure, the pressure was varied between 1 bar and 2000 bar. To be able to use fluorescence spectroscopy at higher pressures, the HHP cell system from ISS and a special cuvette with a path length of about 0.9 cm were used. After filling, the cuvette was sealed with a polyethylene membrane (Sigma-Aldrich; catalogue no. Z379026) and positioned in the beam path of the high-pressure cell. Afterwards, the pressure was increased by using a manual pump. The fluorescence measurements could only be started after the filled cuvette was correctly positioned in the light path and the high-pressure cell closed. Therefore, the intensities of the first 0.5 to 3 min could not be recorded, which has been taken into account in the data analysis. Since it is not possible to correlate directly the intensity of the released AMC to concentration, a calibration of the instrument was necessary by using standard solutions of AMC. The hydrolysis of AAF-AMC catalyzed by α-CT follows the Michaelis–Menten kinetics, according to the following reaction: |  | (1) |
In this scheme, E is the enzyme α-CT, S is the substrate AAF-AMC, ES is the enzyme–substrate complex and P is the released product AMC.
The initial rate of the enzyme reaction, v0, can be determined by following the formation of the product, v0= d[P]/dt, where [P] is the concentration of the product, which was calculated from the slope of the linear fit of the time dependent fluorescence data at 410 nm.7,14 Based on the definition of the rate determining step, the rate constant of product formation, k2, is also denoted kcat or turnover number. To prevent back reaction, the substrate is used in excess so that the enzyme is saturated. The Michaelis constant, KM, a measure of the affinity of substrate binding to the enzyme, is given by KM = (k−1 + k2)/k1. By the linear regression analysis of the Lineweaver–Burk plot, KM and kcat are obtained:7
|  | (2) |
where
vmax is the maximum rate achievable and [S] is the initial substrate concentration; [E]
0 = [E] + [ES] is the total enzyme concentration.
9 The results reported are the average of at least three independent measurements.
Analysis of the pressure dependence of the enzymatic reaction
Generally, a chemical reaction is defined both by its energy and volume profile. For a one-step reaction, meaning that one transition state is involved only, the reaction volume is given by ΔV = Vp − Vr, where Vp and Vr are the partial molar volumes of the product and the sum of the partial molar volumes of the reactants under standard-state conditions. At constant temperature, ΔV can be obtained from measurement of the pressure dependence of the equilibrium constant of the reaction, K:12,21 |  | (3) |
According to Eyring's transition state theory,45 the rate constant, k, of the reaction may be expressed as
| 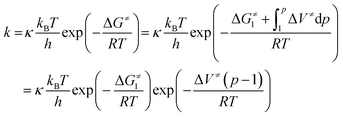 | (4) |
where Δ
G≠ = Δ
H≠ −
TΔ
S≠ is the Gibbs energy of activation, and Δ
H≠ and Δ
S≠ the enthalpy and entropy of activation;
κ is the transmission coefficient across the activated complex (≠), which is assumed here to be temperature and pressure independent. Δ
G≠1 is the corresponding value at atmospheric pressure (1 bar). Δ
V≠ is the volume of activation and represents
stricto sensu the difference between the partial molar volume of the transition state and the initial state. Hence, the magnitude of Δ
V≠ informs on the rate when running the reaction at a given pressure. The determination of Δ
V≠ proceeds from the relation reported above by plotting
k-values against pressure in a relatively narrow pressure range, usually up to 1–2 kbar (neglecting the 1 in the (
p − 1) term):
| 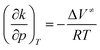 | (5) |
Over a larger pressure range, the pressure dependence of ΔV≠ must be taken into account, which requires an additional compressibility term, (Δβ≠p/(RT)).10 In opposition of a simple chemical reaction, the activation volume, ΔV≠, for an enzymatic reaction is a rather intricate quantity, which may be considered as the sum of several contributions,
; i represents volumetric contributions such as (i) an intrinsic structural term (chemical reaction term) due to changes in intramolecular interactions, invoking bond transformations, steric hindrance and accompanying conformational changes, (ii) a solvational term resulting from the rearrangement of surrounding water (or solvent) molecules and solute–solvent interactions in the active site of the enzyme, e.g. due to electrostriction or release of water via entropy-driven association of hydrophobic residues, and (iii) pressure-induced changes in protein flexibility and conformation (e.g., via selection of a particular conformational substrate).9–11,46,47
Henceforth, exploring the temperature parameter together with the pressure variable, it becomes possible to obtain a complete estimate of the thermodynamic quantities relating to the activated complex, namely, ΔH≠ and ΔS≠ and ΔV≠. Certainly, such kinetic approach is still an approximation for a complex biochemical reaction as the one considered here. Dynamic effects related to pressure-induced changes in transport properties such as diffusion and viscosity might play a role in determining k(p) as well. A more elaborate treatment would also require knowledge of the particular properties of the solvent, such as by Kramer's theory, which takes into account the viscosity in the preexponential factor, and of preferential adsorption or exclusion of cosolvent molecules from the protein. In dilute aqueous solutions in the low kbar range, the effect of pressure on the viscosity is almost negligible, however.46,48
For an enzymatic reaction as given by the Michaelis–Menten formalism (1) and k2 ≪ k−1,10 determination of the pressure dependence of KM and kcat through
|  | (6) |
allows to determine the activation volume, Δ
V≠, and the binding volume, Δ
Vb. In our case, at high substrate concentrations, the activation volume is defined as the difference between the volume in the transition state,
V≠, and the ES complex in the ground state,
VES: Δ
V≠ =
V≠ −
VES.
7 The volume difference between the ES complex in the ground state,
VES, and the reactants,
VE+S, represents the binding volume, Δ
Vb =
VES −
VE+S.
8,49
Circular dichroism spectroscopy
Circular dichroism (CD) spectra of α-CT were recorded to obtain information about the tertiary structure of the enzyme in the different media. Near-UV (260 nm to 320 nm) CD spectra were recorded by means of a Jasco J-715 spectropolarimeter (Jasco Corporation, Tokyo, Japan) at the temperature of 20 °C using a quartz cuvette with a path length of 0.1 cm. The concentration of the enzyme was 100 μM. For each sample, a background blank (buffer without and with DMSO) was subtracted. The spectra recorded are the results of 5 accumulations. The spectra were normalized per mole of residue. Due to the strong absorbance of DMSO in the far-UV region, it was not possible to evaluate secondary structural changes of the enzyme in this spectral region.50
Results and discussion
DMSO effect on the hydrolysis of AAF-AMC at ambient conditions
The impact of the organic solvent DMSO on the hydrolysis of the substrate AAF-AMC catalyzed by α-CT was investigated by means of fluorescence spectroscopy at T = 20 °C. Fig. 1a shows the Lineweaver–Burk plots for the hydrolysis of AAF-AMC in buffer and in the presence of different amounts of DMSO (5, 10 and 20 vol%) at ambient pressure. By fitting the experimental data points to the Lineweaver–Burk equation (eqn (2)), a complete characterization of the kinetic parameters was obtained. The dependence of the kinetic parameters, kcat and KM as well as the catalytic efficiency, kcat/KM, on the DMSO concentration at ambient conditions are summarized in Fig. 2a–c.
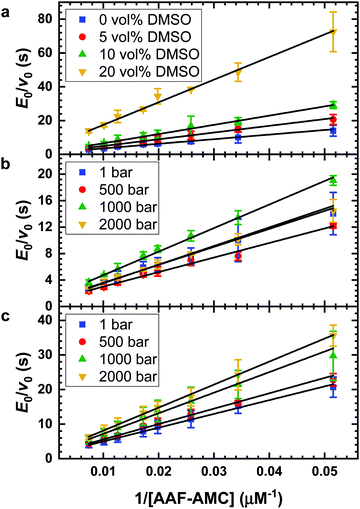 |
| Fig. 1 Lineweaver–Burk plots for the hydrolysis of AAF-AMC catalyzed by the enzyme α-CT. (a) Plots at ambient pressure as function of DMSO vol%: neat buffer (blue); 5 vol% DMSO (red); 10 vol% DMSO (green); 20 vol% DMSO (orange). (b) Plots in neat buffer at different pressures: 1 bar (blue); 500 bar (red); 1000 bar (green); 2000 bar (orange). (c) Plots in buffer containing 5 vol% DMSO at different pressures: 1 bar (blue); 500 bar (red); 1000 bar (green); 2000 bar (orange). | |
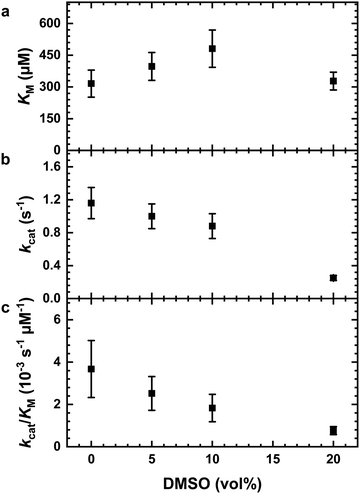 |
| Fig. 2 Effect of DMSO (in vol%) on the kinetic parameters for the hydrolysis of AAF-AMC catalyzed by the enzyme α-CT. (a) Michaelis constant, KM, (b) turnover number, kcat, and (c) catalytic efficiency, kcat/KM. Buffer conditions: 0.1 M Tris, 10 mM CaCl2, pH 7.8; T = 20 °C, p = 1 bar. | |
Fig. 2a shows that increasing DMSO concentration from 0 to 10 vol% leads to a slight increase of the Michaelis constant, KM, indicating a decrease of the affinity of the enzyme for the substrate (for all data, see Table S1, ESI†).8,13,14 At 20 vol% DMSO, a decrease of KM to a value comparable to that obtained in neat buffer was detected. Overall, the presence of DMSO has only a small effect on the Michaelis constant, signifying that the formation of the enzyme–substrate complex (ES) is not largely affected even in the presence of high DMSO concentrations. Fig. 2b depicts the change of the turnover number, kcat, as function of DMSO concentration. Increasing DMSO concentrations lead to a marked decrease of kcat, which passes from 1.16 s−1 in neat buffer to 0.25 s−1 at 20 vol% DMSO, i.e., in DMSO, the release of AMC from the ES complex is retarded.
Several reasons can be invoked to explain the decrease of kcat with increasing DMSO concentration, such as conformational changes or even denaturation of the enzyme or changes in the hydration shell of the enzyme.7,51 To proof if conformational changes of the enzyme occur in the presence of DMSO, near-UV circular dichroism (CD) spectra were recorded, which are reported in Fig. 3. CD spectroscopy is a convenient technique which allows to obtain information on the secondary (far-UV region, 190–260 nm) as well as tertiary (near-UV region, 260–320 nm) structure of proteins.52 Due to the strong absorbance of DMSO in the far-UV region, it is not possible to explore the effect of DMSO on the secondary structure of the enzyme.50 Thus, only spectra in the near-UV region were recorded. As evident from Fig. 3, at all concentrations explored, no effect of the DMSO on the tertiary structure of the protein was observed, indicating that the structural integrity of the enzyme is preserved. Therefore, denaturation of the α-CT even at high DMSO concentrations can be excluded. However, local conformational changes cannot be excluded a priori, which might contribute to the decrease of the turnover number.
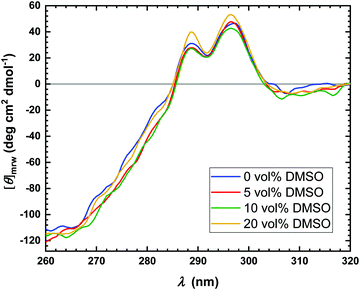 |
| Fig. 3 Near-UV CD spectra of α-CT in neat buffer (blue line) and in the presence of 5 vol% DMSO (red line), 10 vol% DMSO (green line) and 20 vol% DMSO (orange line). The spectra were acquired at 20 °C and at ambient pressure. The spectra were normalized per mole of residue. | |
To carry out the hydrolysis reaction, the presence of water molecules in the enzyme's active site is of key importance.53 It is possible that DMSO can disrupt hydrogen bonds between water molecules and the enzyme, which in turn may also affect the dynamical properties of the enzyme, leading to a decrease of the observed enzymatic activity.54 Moreover, it is known that the organic solvent DMSO preferentially solvates tryptophan residues in the active site of the enzyme.54,55 Thus, a lower turnover number may be explained by a displacement of water molecules from the hydration shell in the active site of the α-CT by DMSO molecules. To highlight the effect of DMSO on the overall catalytic efficiency of the enzyme, Fig. 2c shows kcat/KM values at different DMSO concentration (data in Table S1, ESI†). The catalytic efficiency decreases with increasing DMSO concentration, reaching a decrease of ∼80% of the catalytic efficiency at 20 vol% DMSO.
The combined effects of DMSO and HHP on the hydrolysis of AAF-AMC catalyzed by α-CT
It is known that DMSO can increase the temperature stability of α-CT. An increase of the denaturation temperature, Tm, was observed up to 20 vol% DMSO.56 Thus, a fundamental reason to use DMSO–water mixtures in enzymatic reactions for biotechnological applications is that the reaction can be carried out at higher temperatures to improve the reaction yield. Further, it has been shown that the application of high hydrostatic pressure (HHP) can accelerate enzymatic reactions as well, including hydrolysis reactions catalyzed by α-CT.5,7,8,13,14,49 To reveal the combined effect of both parameters, we explored the effect of HHP on the reaction in the presence of 5 vol% DMSO, the DMSO concentration which exhibited the highest catalytic efficiency. For comparison, the effects of HHP on the hydrolysis reaction at neat buffer conditions were also evaluated. The Lineweaver–Burk plots for the reaction carried out at different pressure conditions (1, 500, 1000 and 2000 bar) in neat buffer and in buffer containing 5 vol% of DMSO are reported in Fig. 1b and c, respectively. The kinetic parameters kcat, KM and kcat/KM are shown in Fig. 4 and Table S2 (ESI†).
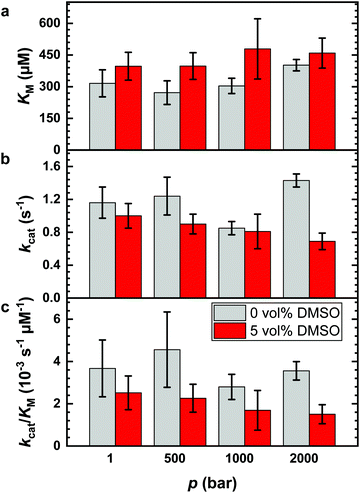 |
| Fig. 4 Pressure dependence of the kinetic parameters for the hydrolysis of the substrate AAF-AMC catalyzed by the enzyme α-CT at 20 °C in neat buffer (grey bars) and in the presence of 5 vol% DMSO (red bars). (a) Michaelis constant, KM, (b) turnover number, kcat, and (c) catalytic efficiency, kcat/KM. | |
Before discussing the effect of HHP on the kinetic parameters, it is important to recall that the structure of the enzyme α-CT is stable in the whole pressure range covered.7,57 As reported in Fig. 4a, increasing pressure has a minor, if any, effect on the Michaelis constant in neat buffer condition. The KM values at 1 bar, 500 bar and 1000 bar are similar within the experimental errors. Only at 2000 bar, KM seems to have slightly increased. In 5 vol% DMSO, the value of the Michaelis constant remains roughly constant throughout the whole pressure range explored. However, the KM values are slightly higher compared to the KM value obtained in neat buffer solution. Hence, the application of pressure imposes only minor effects on the formation of the enzyme–substrate complex for both solution conditions.
Fig. 4b depicts the effect of HHP on the turnover number, kcat, in neat buffer and 5 vol% DMSO. In neat buffer, the application of HHP leads to a slight increase of the turnover number, from 1.16 s−1 at 1 bar to 1.43 s−1 at 2000 bar. Conversely, kcat decreases in 5 vol% DMSO significantly with increasing pressure, from 1.00 s−1 at 1 bar to 0.69 s−1 at 2000 bar. Thus, HHP has an opposite effect on kcat in neat buffer and the DMSO solution. These observations reveal a rather complex behavior of the reaction when carried out both under pressure and in the presence of the cosolvent DMSO. As an acceleration of the reaction upon pressurization is favored by small activation volumes and DMSO affects the activities of both enzyme (e.g. by binding to the active site) and reactants, which may also be pressure dependent (see below), simple additivity of both parameters on kcat is not expected to be seen.
Finally, Fig. 4c shows the catalytic efficiency in neat buffer and in buffer containing 5 vol% DMSO as function of pressure, demonstrating the unfavorable effect of HHP in the DMSO solution as well.
To shed more light on the influence of HHP on kcat and KM in both media, a volumetric analysis was carried out. According to eqn (6), it is possible to determine the activation volume, ΔV≠, and the binding volume, ΔVb, from the pressure dependence of kcat and KM, respectively (Fig. S1 and S2, ESI†). At our concentrations chosen, the activation volume refers to the volume difference between the transition state (ES≠) and the ground state complex (ES). The binding volume refers to the volume difference between the complex ES in the ground state and the reactants in the ground state (E + S). Fig. 5 shows the volume profile for the reaction in the two media.
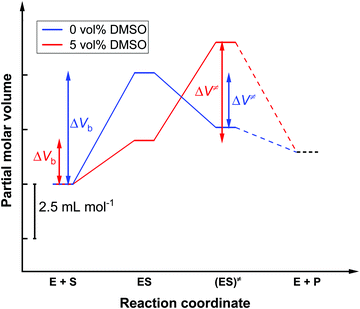 |
| Fig. 5 Schematic representation of the volume profile for the enzymatic reaction in neat buffer (blue line) and in 5 vol% DMSO solution (red line) using as reference the uncomplexed state (E + S). The partial molar volume of the product (E + P) cannot be given and is therefore shown as a dotted line. | |
In the absence of DMSO, the activation volume, ΔV≠, for the reaction is −2.5 ± 0.1 mL mol−1, indicating that the transition state occupies a slightly smaller volume compared to the enzyme–substrate complex in the ground state. The binding volume, ΔVb, amounts to a value of 5.1 ± 1.6 mL mol−1, revealing that the reactants have a smaller volume with respect to the ES complex. In 5 vol% DMSO, the activation volume of the reaction is 4.5 ± 0.2 mL mol−1 and the binding volume is 2.0 ± 0.8 mL mol−1. These values reveal that the transition state ES‡ occupies a larger volume with respect to the ES complex in the ground state in the DMSO solution, and that the reactants have a smaller partial molar volume compared to the ES complex formed.
One reason for a negative activation volume can be a higher density of the hydration shell (electrostrictive effect). The density of the hydration shell increases when charged and polar amino acid residues are exposed to the water solvent during the formation of the transition state.58,59 Furthermore, small conformational changes in the enzyme's active site may be associated with substrate binding. A positive activation volume implicates a retardation of the enzyme reaction, which could be due to a decreased hydration or to packing defects in the active site induced by DMSO binding.7,13 In the two buffer solutions investigated (0 vol% DMSO and 5 vol% DMSO), a positive binding volume ΔVb was found, this is to say, the volume of the reactants must be smaller than the volume of the ES complex. This is typically due to the release of hydration water during binding of the substrate to the binding pocket of the enzyme.60,61 The lower value of the binding volume in 5 vol% DMSO could be explained by a lower degree of hydration of the substrate in the DMSO solution, thereby changing its chemical activity.7,8
Conclusions
In summary, the organic solvent DMSO has a significant influence on the enzymatic activity of α-CT. The Michaelis constant, KM, changes in a non-linear way with the DMSO concentration. KM is lowest, i.e. the affinity of the enzyme α-CT to the substrate AAF-AMC is highest at 0 and 20 vol% DMSO.8,13,14 Cosolvent–substrate interactions may be mainly responsible for the dependence of KM on the cosolvent.7 In addition, weaker hydrophobic interactions between the substrate AAF-AMC and the enzyme could be invoked in the presence of DMSO.7 Due to the excess of substrate, the catalytic efficiency is mainly controlled by the turnover number, kcat, which decreases with increasing DMSO concentration, as does the catalytic efficiency kcat/KM. This can be explained by a displacement of water molecules in the hydration shell and the active site of the α-CT by DMSO molecules.51 In thermodynamic terms, a change in the activity coefficient due to interactions of the enzyme with the substrate, the solvent and the cosolvent may be responsible.7 A significant change of the tertiary structure of the enzyme upon addition of the DMSO up to 20 vol% could be ruled out.
Regarding the effect of high hydrostatic pressure, no significant change in KM, i.e. in the affinity of the enzyme to the substrate AAF-AMC, was observed.8,13,14 Whereas kcat increases at high pressure in neat buffer solution, it decreases in 5 vol% DMSO solution, i.e. the positive effect of HHP in neat buffer is lost in the presence of DMSO. Accordingly, volumetric analysis of the reaction yields an activation volume, ΔV≠, of −2.5 ± 0.1 mL mol−1 in neat buffer and of 4.5 ± 0.2 mL mol−1 in 5 vol% DMSO. For the corresponding binding volume, ΔVb, values of 5.1 ± 1.6 mL mol−1 in neat buffer and 2.0 ± 0.8 mL mol−1 in 5 vol% DMSO have been obtained. The negative ΔV≠ indicates a more compact transition state compared to the ground state of the ES complex, which might be due to an electrostrictive effect and/or a more efficient packing of the activated complex. The positive ΔV≠ observed in 5 vol% DMSO indicates a slight volume increase of the ES‡ complex, most likely due to binding of some DMSO molecules in the active site of α-CT.7,13 The positive binding volumes suggest a release of hydration water when the substrate binds to the active site of the enzyme.8 Please note, that these volume changes observed here are very small, actually a fraction of the volume of one water molecule (18 mL mol−1), only. This also demonstrates the strength of the method used here to determine volume changes with high precision.
To conclude, although there is an opposite effect of hydrostatic pressure and the dipolar solvent DMSO on the enzymatic activity of α-CT, no drastic changes are observed. The use of HHP in concert with DMSO does not lead to an improvement of the enzymatic activity in our case. The advantages of DMSO and HHP to increase the temperature stability of the enzyme and to increase the solubility of more hydrophobic substrates might still be useful, however. To this end, a few %, typically <10%, of DMSO are needed, only.62 Even if many issues remain to be solved, temperature, pressure and non-aqueous cosolvents in combination may be useful to modulate and optimize enzymatic reactions.
Conflicts of interest
There are no conflicts to declare.
Acknowledgements
We are grateful for financial support by the Deutsche Forschungsgemeinschaft (DFG, German Research Foundation) under Germany's Excellence Strategy – EXC 2033 – 390677874 – RESOLV.
References
- J.-X. Tan, H. Lv, F. Wang, F.-Y. Dao, W. Chen and H. Ding, A Survey for Predicting Enzyme Family Classes Using Machine Learning Methods, Curr. Drug Targets, 2019, 20, 540–550 CrossRef CAS PubMed.
-
K. Drauz, H. Gröger and O. May, Enzyme Catalysis in Organic Synthesis, 3 Volume Set, John Wiley & Sons, 2012 Search PubMed.
- R. Wohlgemuth, Biocatalysis—key to sustainable industrial chemistry, Curr. Opin. Biotechnol, 2010, 21, 713–724 CrossRef CAS PubMed.
- R. Singh, M. Kumar, A. Mittal and P. K. Mehta, Microbial enzymes: industrial progress in 21st century, 3 Biotech, 2016, 6, 174 CrossRef PubMed.
- M. Knierbein, A. Wangler, T. Q. Luong, R. Winter, C. Held and G. Sadowski, Combined co-solvent and pressure effect on kinetics of a peptide hydrolysis: an activity-based approach, Phys. Chem. Chem. Phys., 2019, 21, 22224–22229 RSC.
- V. I. Tishkov and V. O. Popov, Catalytic mechanism and application of formate dehydrogenase, Biochemistry, 2004, 69, 1252–1267 CAS.
- T. Q. Luong and R. Winter, Combined pressure and cosolvent effects on enzyme activity – a high-pressure stopped-flow kinetic study on α-chymotrypsin, Phys. Chem. Chem. Phys., 2015, 17, 23273–23278 RSC.
- R. Oliva, S. Banerjee, H. Cinar and R. Winter, Modulation of enzymatic activity by aqueous two-phase systems and pressure – rivalry between kinetic constants, Chem. Commun., 2020, 56, 395–398 RSC.
- M. J. Eisenmenger and J. I. Reyes-De-Corcuera, High pressure enhancement of enzymes: A review, Enzyme Microb. Technol., 2009, 45, 331–347 CrossRef CAS.
-
E. Morild, Advances in Protein Chemistry, Elsevier, 1981, vol. 34, pp. 93–166 Search PubMed.
- V. V. Mozhaev, R. Lange, E. V. Kudryashova and C. Balny, Application of high hydrostatic pressure for increasing activity and stability of enzymes, Biotechnol. Bioeng., 1996, 52, 320–331 CrossRef CAS PubMed.
-
High Pressure Bioscience: Basic Concepts, Applications and Frontiers, ed. K. Akasaka and H. Matsuki, Springer, Netherlands, Dordrecht, 2015, vol. 72 Search PubMed.
- C. Held, T. Stolzke, M. Knierbein, M. W. Jaworek, T. Q. Luong, R. Winter and G. Sadowski, Cosolvent and pressure effects on enzyme-catalysed hydrolysis reactions, Biophys. Chem., 2019, 252, 106209 CrossRef CAS PubMed.
- M. W. Jaworek, V. Schuabb and R. Winter, The effects of glycine, TMAO and osmolyte mixtures on the pressure dependent enzymatic activity of α-chymotrypsin, Phys. Chem. Chem. Phys., 2018, 20, 1347–1354 RSC.
- J. Roche, J. A. Caro, D. R. Norberto, P. Barthe, C. Roumestand, J. L. Schlessman, A. E. Garcia, B. Garcia-Moreno E. and C. A. Royer, Cavities determine the pressure unfolding of proteins, Proc. Natl. Acad. Sci. U. S. A., 2012, 109, 6945–6950 CrossRef CAS PubMed.
- J. L. Silva, A. C. Oliveira, T. C. R. G. Vieira, G. A. P. de Oliveira, M. C. Suarez and D. Foguel, High-Pressure Chemical Biology and Biotechnology, Chem. Rev., 2014, 114, 7239–7267 CrossRef CAS PubMed.
- I. Daniel, P. Oger and R. Winter, Origins of life and biochemistry under high-pressure conditions, Chem. Soc. Rev., 2006, 35, 858 RSC.
- M. W. Jaworek, V. Schuabb and R. Winter, Pressure and cosolvent modulation of the catalytic activity of amyloid fibrils, Chem. Commun., 2018, 54, 5696–5699 RSC.
- F. Meersman, I. Daniel, D. H. Bartlett, R. Winter, R. Hazael and P. F. McMillan, High-Pressure Biochemistry and Biophysics, Rev. Mineral. Geochem., 2013, 75, 607–648 CrossRef CAS.
- M. E. Gerringer, J. C. Drazen and P. H. Yancey, Metabolic enzyme activities of abyssal and hadal fishes: pressure effects and a re-evaluation of
depth-related changes, Deep Sea Res., Part I, 2017, 125, 135–146 CrossRef CAS.
- R. Winter, Interrogating the Structural Dynamics and Energetics of Biomolecular Systems with Pressure Modulation, Annu. Rev. Biophys., 2019, 48, 441–463 CrossRef CAS PubMed.
- K. V. Balakin, Y. A. Ivanenkov, A. V. Skorenko, Y. V. Nikolsky, N. P. Savchuk and A. A. Ivashchenko, In Silico Estimation of DMSO Solubility of Organic Compounds for Bioscreening, J. Biomol. Screening, 2004, 9, 22–31 CrossRef CAS PubMed.
- A. N. Rajeshwara and V. Prakash, Structural stability of lipase from wheat germ, Int. J. Pept. Protein Res., 1994, 44, 435–440 CrossRef CAS PubMed.
- S. Rajendran, C. Radha and V. Prakash, Mechanism of solvent-induced thermal stabilization of α-amylase from Bacillus amyloliquefaciens, Int. J. Pept. Protein Res., 1995, 45, 122–128 CrossRef CAS PubMed.
- A. Torreggiani, M. Di Foggia, I. Manco, A. De Maio, S. A. Markarian and S. Bonora, Effect of sulfoxides on the thermal denaturation of hen lysozyme: a calorimetric and Raman study, J. Mol. Struct., 2008, 891, 115–122 CrossRef CAS.
- K. Hamaguchi, Structure of Muramidase (Lysozyme), J. Biochem., 1964, 56, 441–449 CrossRef CAS PubMed.
- M. Kotik, S. E. Radford and C. M. Dobson, Comparison of the Refolding Hen Lysozyme from Dimethyl Sulfoxide and Guanidinium Chloride, Biochemistry, 1995, 34, 1714–1724 CrossRef CAS PubMed.
- I. K. Voets, W. A. Cruz, C. Moitzi, P. Lindner, E. P. G. Arêas and P. Schurtenberger, DMSO-Induced Denaturation of Hen Egg White Lysozyme, J. Phys. Chem. B, 2010, 114, 11875–11883 CrossRef CAS PubMed.
- T. Arakawa, Y. Kita and S. N. Timasheff, Protein precipitation and denaturation by dimethyl sulfoxide, Biophys. Chem., 2007, 131, 62–70 CrossRef CAS PubMed.
- R. L. Perlman and J. Wolff, Dimethyl Sulfoxide: An Inhibitor of Liver Alcohol Dehydrogenase, Science, 1968, 160, 317–319 CrossRef CAS PubMed.
- M. Banasik and K. Ueda, Dual Inhibitory Effects of Dimethyl Sulfoxide on Poly(ADP-Ribose) Synthetase, J. Enzyme Inhib., 1999, 14, 239–250 CrossRef CAS PubMed.
- Ö. Almarsson and A. M. Klibanov, Remarkable activation of enzymes in nonaqueous media by denaturing organic cosolvents, Biotechnol. Bioeng., 1996, 49, 87–92 CrossRef PubMed.
- H.-J. Zhang, X.-R. Sheng, X.-M. Pan and J.-M. Zhou, Activation of Adenylate Kinase by Denaturants Is Due to the Increasing Conformational Flexibility at Its Active Sites, Biochem. Biophys. Res. Commun., 1997, 238, 382–386 CrossRef CAS PubMed.
- A. Steinschneider and K. Druck, Ribonuclease properties and activity in the presence of dimethylsulfoxide, Biochim. Biophys. Acta, Nucleic Acids Protein Synth., 1972, 287, 77–89 CrossRef CAS.
- Z.-W. Yu and P. J. Quinn, Dimethyl sulphoxide: A review of its applications in cell biology, Biosci. Rep., 1994, 14, 259–281 CrossRef CAS PubMed.
- J. E. Lovelock and M. W. H. Bishop, Prevention of Freezing Damage to Living Cells by Dimethyl Sulphoxide, Nature, 1959, 183, 1394–1395 CrossRef CAS PubMed.
- W.-B. Ou, Y.-D. Park and H.-M. Zhou, Effect of osmolytes as folding aids on creatine kinase refolding pathway, Int. J. Biochem. Cell Biol., 2002, 34, 136–147 CrossRef CAS PubMed.
- E. Jaspard, Role of Protein–Solvent Interactions in Refolding: Effects of Cosolvent Additives on the Renaturation of Porcine Pancreatic Elastase at Various pHs, Arch. Biochem. Biophys., 2000, 375, 220–228 CrossRef CAS PubMed.
- K. Watanabe and S. Ueji, Dimethyl sulfoxide as a co-solvent dramatically enhances the enantioselectivity in lipase-catalysed resolutions of 2-phenoxypropionic acyl derivatives, J. Chem. Soc., Perkin Trans. 1, 2001, 1386–1390 RSC.
- N. L. Eremeev, Interaction of a-Chymotrypsin with Dimethyl Sulfoxide: A Change of Substrate Could “Change” the Interaction Mechanism, Russ. J. Bioorg. Chem., 2003, 29, 434–440 CrossRef CAS PubMed.
- T. Tretyakova, M. Shushanyan, T. Partskhaladze, M. Makharadze, R. van Eldik and D. E. Khoshtariya, Simplicity within the complexity: Bilateral impact of DMSO on the functional and unfolding patterns of α-chymotrypsin, Biophys. Chem., 2013, 175–176, 17–27 CrossRef CAS PubMed.
- A. Das, A. Parta and R. K. Mitra, Modulation of anionic reverse micellar interface with non-ionic surfactants can regulate enzyme activity within the micellar waterpool, Colloid Polym. Sci., 2016, 294, 715–726 CrossRef CAS.
- J. K. A. Kamal, T. Xia, S. K. Pal, L. Zhao and A. H. Zewail, Enzyme functionality and solvation of Subtilisin Carlsberg: from hours to femtoseconds, Chem. Phys. Lett., 2004, 387, 209–215 CrossRef CAS.
- A. Patra, N. Samanta, D. K. Das and R. K. Mitra, Enhanced Catalytic Activity of α-Chymotrypsin in Cationic Surfactant Solutions: The Component Specificity Revisited, J. Phys. Chem. B, 2017, 121, 1457–1465 CrossRef CAS PubMed.
-
M. J. Pilling and P. W. Seakins, Reaction kinetics, Oxford University Press, Oxford, New York, 1995 Search PubMed.
-
G. Jenner, in High Pressure Molecular Science, ed. R. Winter and J. Jonas, Springer, Netherlands, 1999 Search PubMed.
- P. K. Verma, S. Rakshit, R. K. Mitra and S. K. Pal, Role of hydration on the functionality of a proteolytic enzyme α-chymotrypsin under crowded environment, Biochimie, 2011, 93, 1424–1433 CrossRef CAS PubMed.
-
H. Weingärtner, E. U. Franck, G. Wiegand, N. Dahmen, G. Schwedt, F. H. Frimmel and B. C. Gordalla, Ullmann's Encyclopedia of Industrial Chemistry, Wiley-VCH Verlag GmbH & Co. KGaA, Weinheim, Germany, 2011, p. a28_001.pub2 Search PubMed.
- C. Czeslik, T. Q. Luong and R. Winter, Enzymatic activity under pressure, MRS Bull., 2017, 42, 738–742 CrossRef.
- A. J. Hynes and P. H. Wine, The atmospheric chemistry of dimethylsulfoxide (DMSO) kinetics and mechanism of the OH + DMSO reaction, J. Atmos. Chem., 1996, 24, 23–37 CrossRef CAS.
- V. A. Sirotkin and A. A. Kuchierskaya, α-Chymotrypsin in water-acetone and water-dimethyl sulfoxide mixtures: Effect of preferential solvation and hydration: SIROTKIN and KUCHIERSKAYA, Proteins: Struct., Funct., Bioinf., 2017, 85, 1808–1819 CrossRef CAS PubMed.
- S. M. Kelly, T. J. Jess and N. C. Price, How to study proteins by circular dichroism, Biochim. Biophys. Acta, Proteins Proteomics, 2005, 1751, 119–139 CrossRef CAS PubMed.
-
D. L. Purich, Enzyme kinetics: catalysis & control; a reference of theory and best-pratice methods, Elsevier/AP, Amsterdam, 1st edn, 2010 Search PubMed.
- F. Moyano, E. Setien, J. J. Silber and N. M. Correa, Enzymatic Hydrolysis of N-Benzoyl-L-Tyrosine p-Nitroanilide by α-Chymotrypsin in DMSO-Water/AOT/n-Heptane Reverse Micelles. A Unique Interfacial Effect on the Enzymatic Activity, Langmuir, 2013, 29, 8245–8254 CrossRef CAS PubMed.
- D. R. Canchi and A. E. García, Cosolvent Effects on Protein Stability, Annu. Rev. Phys. Chem., 2013, 64, 273–293 CrossRef CAS PubMed.
- T. Tretyakova, M. Shushanyan, T. Partskhaladze, M. Makharadze, R. Eldik and D. E. Khoshtariya, Simplicity within the complexity: Bilateral impact of DMSO on the functional and unfolding patterns of α-chymotrypsin, Biophys. Chem., 2013, 175–176, 17–27 CrossRef CAS PubMed.
-
Z. Sun and R. Winter, Advances in High Pressure Bioscience and Biotechnology II, Berlin, 2002, pp. 117–120 Search PubMed.
- C. Held, T. Stolzke, M. Knierbein, M. W. Jaworek, T. Q. Luong, R. Winter and G. Sadowski, Cosolvent and pressure effects on enzyme-catalysed hydrolysis reactions, Biophys. Chem., 2019, 252, 106209 CrossRef CAS PubMed.
- R. Van Eldik, T. Asano and W. J. Le Noble, Activation and reaction volumes in solution. 2, Chem. Rev., 1989, 89, 549–688 CrossRef CAS.
- I. Son, Y. L. Shek, D. N. Dubins and T. V. Chalikian, Volumetric Characterization of Tri-N-acetylglucosamine Binding to Lysozyme, Biochemistry, 2012, 51, 5784–5790 CrossRef CAS PubMed.
- R. Oliva, S. Banerjee, H. Cinar, C. Ehrt and R. Winter, Alteration of Protein Binding Affinities by Aqueous Two-Phase Systems Revealed by Pressure Perturbation, Sci. Rep., 2020, 10, 8074 CrossRef CAS PubMed.
- J. Wallerstein and M. Akke, Minute Additions of DMSO Affect Protein Dynamics Measurements by NMR Relaxation Experiments through Significant Changes in Solvent Viscosity, ChemPhysChem, 2019, 20, 326–332 CrossRef CAS PubMed.
Footnote |
† Electronic supplementary information (ESI) available. See DOI: 10.1039/d0cp03062g |
|
This journal is © the Owner Societies 2020 |