DOI:
10.1039/C8AY02526F
(Paper)
Anal. Methods, 2019,
11, 642-647
A highly selective and instantaneously responsive Schiff base fluorescent sensor for the “turn-off” detection of iron(III), iron(II), and copper(II) ions†
Received
20th November 2018
, Accepted 11th December 2018
First published on 11th December 2018
Abstract
A new and tri-responsive fluorescent Schiff base probe (DBAB) has been designed and developed for the recognition of iron(III) ions (Fe3+), iron(II) ions (Fe2+) and copper(II) ions (Cu2+) simultaneously. This sensor displays a favorable selectivity for Fe3+, Fe2+ and Cu2+ ions over a range of other common metal cations in DMF solution, leading to prominent fluorescence on–off. The detection limits were 2.17 × 10−6 M for Fe3+, 2.06 × 10−6 M for Fe2+ and 2.48 × 10−6 M for Cu2+ respectively. This tri-responsive fluorescent probe for Fe3+, Fe2+ and Cu2+ was distinguished by further reduction of the ascorbic acid. The recognition mechanism of DBAB toward Fe3+, Fe2+ and Cu2+ has been investigated in detail by Job plot measurements and 1H NMR.
1. Introduction
In recent years, the design and synthesis of smart organic molecules used as chemosensors with high selectivity, sensitivity, and instantaneous responsiveness toward metal cations has received considerable attention.1 Sensing multiple analytes with a single chemosensor is a challenging task.2 It involves eliciting different responses from the molecule in the presence of different analytes.
Among biological metal ions, iron ions and copper ions both play crucial roles in many biological and environmental processes.3 Fe3+ deficiency and overloading induce serious disorders such as Huntington’s disease.4 Cu2+ plays a critical role as a catalytic cofactor in a variety of metallo-enzymes, including tyrosinase, superoxide dismutase and cytochrome oxidase. Excessive Cu2+ ions will also cause imbalance in cellular processes, resulting in neurodegenerative diseases.5 At present, many methods are employed to determine Fe3+, Fe2+ and Cu2+ including inductively coupled plasma mass spectrometry (ICP-MS),6 fluorescence,7 atomic absorption,8 colorimetry,9 flow injection and electrochemical methods.10
Currently, compared with these methods, the fluorescent sensor strategy is proven to be the most convenient and popular approach for metal ion detection due to its superiority over other methods, such as high sensitivity, remarkable selectivity, easy operation, and rapid response. In the past decade, diverse fluorescent chemosensors for transition metal ions have been reported, including Hg2+,11 Cu2+,12 Zn2+,13 Al3+,14 Fe3+,15 Cd2+,16 and Cr3+.17 However, fluorescent chemosensors which can recognize two or more transition metal ions simultaneously and instantaneously remain scarce. Therefore, developing simple sensors which can detect more than one ion in environmental and biological samples has gained increasing attention due to their high efficiency, low-cost, and operational simplicity.18
Herein we introduce a fluorescent Schiff base ((E)-2-((4-(diethylamino)benzylidene)amino)benzoic acid, DBAB) that exhibits remarkable optical responses when interacting with a metal ion. This sensor displays a favorable selectivity for Fe3+, Fe2+ and Cu2+ ions over a range of other common metal cations in DMF solution, leading to prominent fluorescence on–off. The “turn-off” signals between DBAB and Fe3+/Fe2+/Cu2+ can be distinguished by ascorbic acid (AA) via the reduction ability difference between Fe3+/AA, Fe2+/AA and Cu2+/AA (Scheme 1). In order to investigate the fluorescence quenching mechanism of DBAB toward Fe3+, Fe2+ and Cu2+, Job plot measurements and 1H NMR were applied to the structure of the chelate of DBAB and Fe3+/Fe2+/Cu2+. The DBAB Schiff base molecule shows great potential to serve as a Fe3+, Fe2+ and Cu2+ sensor.
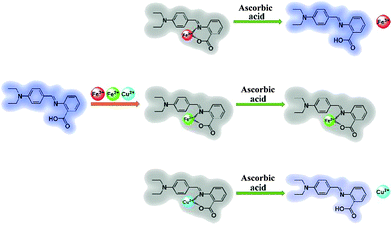 |
| Scheme 1 DBAB detection pathway for Fe3+, Fe2+ and Cu2+. | |
2. Results and discussion
2.1 The synthesis of DBAB
The fluorescent probe DBAB was facilely synthesized via 4-(diethylamino)benzaldehyde with 2-aminobenzoic acid in an ethanol and acetic acid mixed solution at 60 °C as shown in Scheme 2, and its structure was then confirmed via1H NMR, 13C NMR, and MS measurements (Fig. S1–S3†).
 |
| Scheme 2 Synthesis of DBAB. | |
2.2 Detection of metal ions
The selectivity of DBAB toward various metal ions, such as Fe3+, Fe2+, Cu2+, Cd2+, Cr3+, Li2+, Mn2+, Na+, K+, Mg2+, Zn2+, Ba2+, Ca2+, and Al3+, was examined in DMF solvent. As observed by the naked eye, it revealed a very distinctive selectivity towards Fe3+, Fe2+ and Cu2+ ions. When the probe was treated with a series of individual metal ions in DMF, Cu2+ showed an obvious colour change from colorless to yellow, Fe3+ and Fe2+ showed an obvious colour change from colorless to light brown. In contrast, the other metal ions did not show any significant colour change as observed by the naked eye (Fig. 1a). Correspondingly, Fig. 1b shows the photograph of fluorescence change from purple to colorless under UV-light in 1.7 μM DBAB solution with different metal ions. Then, to study the fluorescence properties of DBAB towards metal ions, the emission changes were measured in DMF at room temperature by using only 1.7 uM DBAB with Fe3+, Fe2+, Cu2+ and other metal ions (including Cd2+, Cr3+, Li2+, Mn2+, Na+, K+, Mg2+, Zn2+, Ba2+, Ca2+, and Al3+). As shown in Fig. 1c, 1.7 μM DBAB solution showed a strong purple emission signal (λem = 393 nm) at an excitation wavelength of 341 nm, and the addition of 353 equiv. of Cd2+, Cr3+, Li2+, Mn2+, Na+, K+, Mg2+, Zn2+, Ba2+, Ca2+, and Al3+, and Ce3+ resulted in nearly negligible effects on fluorescence emission. However, when equivalent Fe3+, Fe2+ and Cu2+ were added to the solution of DBAB, the fluorescence intensity showed remarkable quenching (∼98% of Fe3+, Fe2+ and ∼92% of Cu2+) due to the chelation-enhanced fluorescence quenching (CHEQ) effect of paramagnetic Fe3+, Fe2+ and Cu2+.19
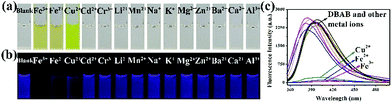 |
| Fig. 1 (a) Colour changes and (b) fluorescence changes under UV light irradiation observed after the addition of metal ions (353 equiv.) to DBAB (1.7 μM) solution in DMF. (c) Fluorescence spectra of DBAB (1.7 μM) in the presence of different metal ions (353 equiv.) in DMF. | |
To estimate the specificity of DBAB detection for Fe3+/Fe2+/Cu2+, competitive experiments were carried out in the presence of Fe3+, Fe2+, and Cu2+ (353 equiv.) mixed with other competitive metal ions (353 equiv.). Competitive ions were initially added to the detection solution, then Fe3+, Fe2+, and Cu2+ ions were added 10 min later. Changes in fluorescence intensity at 393 nm before and after the addition of Fe3+, Fe2+, and Cu2+ are displayed in Fig. 2. The results showed that other competitive metal ions had no obvious effect on the fluorescence of DBAB besides Fe3+, Fe2+, and Cu2+, which reveals that DBAB is a tri-responsive and highly effective fluorescent probe for the detection of Fe3+, Fe2+, and Cu2+ ions over other common metal ions.
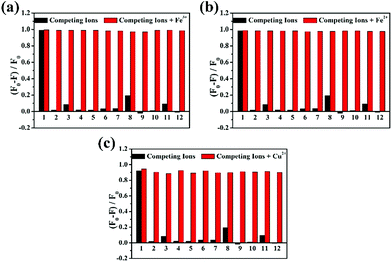 |
| Fig. 2 Selectivity of DBAB (1.7 μM) toward (a) Fe3+, (b) Fe2+ and (c) Cu2+ (353 equiv.) and other competing metal ions (353 equiv.). (1), Fe3+ (a)/Fe2+ (b)/Cu2+ (c); (2), Cd2+; (3), Cr3+; (4), Li2+; (5), Mn2+; (6), Na+; (7), K+; (8), Mg2+; (9), Zn2+; (10), Ba2+; (11), Ca2+; (12), Al3+. | |
Then, the time dependent fluorescence intensity of DBAB was measured in the presence of Fe3+, Fe2+, and Cu2+ ions, respectively (Fig. 3). After addition of Fe3+/Fe2+/Cu2+ (353 equiv.), the fluorescence intensity of the DBAB solution at 393 nm quenched instantaneously in 2 seconds owing to the formation of a DBAB complex with Fe3+/Fe2+/Cu2+ metal ions, which indicates that the DBAB Schiff base can be used as an instantaneous fluorescence probe for the detection of Fe3+/Fe2+/Cu2+. This fluorescence quenching phenomenon may be attributed to strong CHEQ of DBAB–Fe3+/Fe2+/Cu2+. The short response time demonstrates that DBAB possesses good responsiveness to Fe3+, Fe2+, and Cu2+, which can be further used for wide practical applications.
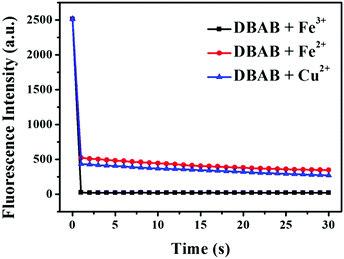 |
| Fig. 3 The response time of DBAB–Fe3+, DBAB–Fe2+ and DBAB–Cu2+. | |
To quantify the quenching effect between DBAB and Fe3+, Fe2+, and Cu2+, the sensing capability of DBAB towards Fe3+, Fe2+, and Cu2+ was further investigated in detail by fluorescence titration analysis. As shown in Fig. 4, as Fe3+, Fe2+, and Cu2+ ions were gradually added to the DBAB solution, the fluorescence intensity (at 393 nm) of the chemosensor gradually decreased and reached a plateau after addition of 1912 equiv. of Fe3+, 5882 equiv. of Fe2+ and 5294 equiv. of Cu2+. There was good linear correlation between the metal ion concentrations and fluorescence intensity in the range 0–0.03 mM (Fig. 4a, d and f), which demonstrated that Fe3+, Fe2+, and Cu2+ could be quantitatively detected by DBAB. The limits of detection (LOD) were calculated as 2.17 × 10−6 M for Fe3+, 2.06 × 10−6 M for Fe2+ and 2.48 × 10−6 M for Cu2+ using the equation LOD = 3 × Sb/S (eqn (S1)†),20 which are far lower than the maximum contaminant levels (MCL) for iron (5.4 M) and copper (20 M) in drinking water based on the EPA guidelines.
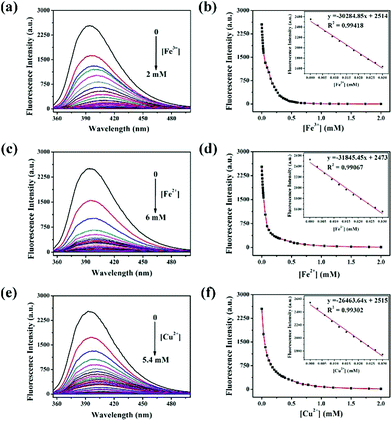 |
| Fig. 4 Fluorescence emission spectra of 1.7 μM DBAB in the presence of different concentrations of (a) Fe3+, (c) Fe2+ and (e) Cu2+ in DMF, respectively (λex = 341 nm). The plot of fluorescence intensity of DBAB (1.7 μM) at 393 nm versus concentration of (b) Fe3+, (d) Fe2+ and (f) Cu2+ in DMF, respectively, the inset shows the linear range of the curve. | |
2.3 Distinguishing Fe3+, Fe2+ and Cu2+ by ascorbic acid reduction
In order to distinguish Fe3+, Fe2+ and Cu2+, we used ascorbic acid (AA) as a reducing agent. It is well known that AA is liable to be oxidized in air.21 Fe2+ ions were easily obtained via the oxidation–reduction reaction between AA and Fe3+ ions, while the reaction of Fe2+ and Cu2+ with ascorbic acid did not show such a phenomenon. With the addition of the reducing agent AA, Fe3+, Fe2+ and Cu2+ can be well distinguished (Fig. 5). In the experiment, an excess amount of AA (1470 equiv.) was introduced into the testing solution (1.7 μM DBAB and 353 equiv. of Fe3+, Fe2+, and Cu2+); for DBAB–Fe3+, the quenched fluorescence increased again gradually, and then reached the maximum fluorescence recovery after 90 min (Fig. 5a and 4e). Contrarily, for DBAB–Fe2+, AA had little effect (Fig. 5b). These different phenomena can be attributed to the different reducing abilities of AA toward Fe3+ and Fe2+. For DBAB–Cu2+, the quenched fluorescence increased but only can reach half of the maximum fluorescence recovery value (Fig. 5c). Color changes were also observed under UV light upon the addition of AA, showing that the purple fluorescence remained in the solution containing Fe3+, while the solution containing Fe2+ remained colorless (Fig. 5f). These results solidify that DBAB can further distinguish Fe3+, Fe2+ and Cu2+ effectively by the reduction method of AA.
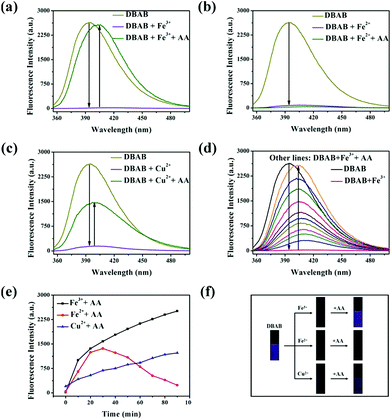 |
| Fig. 5 (a) Fluorescence intensity changes of the probe DBAB (1.7 μM) in DMF solution after the addition of 353 equiv. of Fe3+ and an excess amount of AA (1470 equiv.). (b) and (c) Under the same conditions, fluorescence intensity changes of the probe DBAB solution after the addition of 353 equiv. of Fe2+ and Cu2+ with 1470 equiv. of AA. (d) Fluorescence intensity changes of the probe DBAB (1.7 μM) in DMF solution after the addition of 353 equiv. of Fe3+ and different amounts of AA. (e) Time dependence of fluorescence intensity of DBAB containing Fe3+, Fe3+ and Cu2+ after the addition of 1470 equiv. of AA. (f) Colour changes under UV light irradiation observed for DBAB solution containing Fe3+ (353 equiv.), Fe2+ (353 equiv.) and Cu2+ (353 equiv.) with the addition of 1470 equiv. of AA after 90 min. | |
2.4 Mechanism of DBAB sensing of Fe3+, Fe2+ and Cu2+
In order to determine the binding stoichiometry between DBAB and responding metal ions (Fe3+, Fe2+, and Cu2+), Job's method for fluorescence titration was carried out (Fig. 6). The inflection point was fixed at 0.5, indicating a 1
:
1 ratio for the DBAB and Fe3+/Fe2+/Cu2+ complex. Based on the Job's analysis method, the association constants (Ka) were calculated to be 3.82 × 105 M−1 for DBAB–Fe3+, 3.61 × 105 M−1 for DBAB–Fe2+, and 3.93 × 105 M−1 for DBAB–Cu2+ from nonlinear curve fitting of the fluorescence titration data (Fig. 4b, d and f), according to the Benesi–Hildebrand equation (eqn (S2)†).
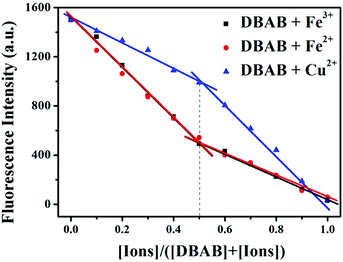 |
| Fig. 6 Job plot obtained for DBAB and Fe3+, Fe2+ and Cu2+ ions. The total concentration of DBAB and cations was fixed at 20 μM. The fluorescence emission was measured at 393 nm. | |
2.5
1H NMR titration
In order to validate the proposed recognition mechanism, 1H NMR titration was performed by adding incremental amounts of Fe3+, Fe2+ and Cu2+ ions (in D2O) to a solution of DBAB in DMSO-d6. Upon addition of Fe3+, Fe2+ and Cu2+ ions, the proton signals became broad due to the paramagnetic nature of the complexed Fe3+, Fe2+ and Cu2+ ions.
As shown in Fig. 7, the –O
C–OH proton signal of DBAB at 8.67 ppm faded away, the –CH
N– proton signal at 8.04 ppm shifted downfield to 9.63 ppm. At the same time proton signals at 7.75 ppm, 7.34 ppm, and 6.87 ppm shifted upfield to 7.66 ppm, 7.21 ppm, and 6.74 ppm, respectively. These spectra exhibit the binding nature with Fe3+ ions in a 1
:
1 stoichiometric ratio, they also indicate that the oxygen in the hydroxyl moiety and the nitrogen in the imine moiety participate in complexation with Fe3+ ions. The proton signal shifts of DBAB/Fe2+ and DBAB/Cu2+ complexes are the same as those of DBAB/Fe2+ (Fig. 7b and c).
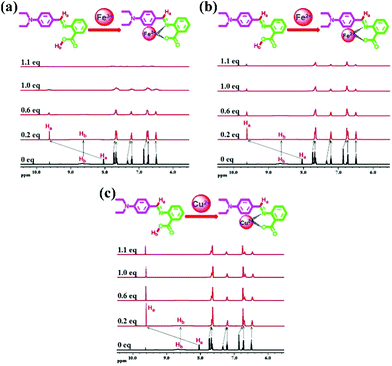 |
| Fig. 7
1H NMR spectra of DBAB in DMSO-d6 upon titration with 0.2 equiv., 0.6 equiv., 1.0 equiv., and 1.1 equiv. of (a) Fe3+, (b) Fe2+ and (c) Cu2+ ions. | |
3. Experimental
3.1 Reagents and chemicals
All chemicals for the synthesis of DBAB were ordered from Sigma-Aldrich and used as received. The chlorinated salts (Fe3+, Fe2+, Cu2+, Cd2+, Cr3+, Li2+, Mn2+, Na+, K+, Mg2+, Zn2+, Ba2+, Ca2+, and Al3+) were purchased from Sinopharm chemical reagent company (Shanghai, China) and used without further purification. Dimethyl formamide (DMF) was purchased from Sigma-Aldrich (chromatographically pure) and used without further purification. All aqueous solutions were prepared with pure water from a Millipore Auto pure WR600A system (Millipore Ltd. USA).
UV-vis spectra were recorded on a Shimadzu UV-2500 spectrophotometer using a 10 mm path length quartz cuvette. 1H and 13C Nuclear Magnetic Resonance (NMR) spectra were recorded on a Varian 600 MHz NMR spectrometer using DMSO-d6. 1H NMR titrations were carried out by dissolving DBAB in DMSO-d6 and the metal salts Fe3+, Fe2+, and Cu2+ in D2O, respectively, at room temperature and the chemical shifts are reported in values (ppm) relative to TMS. Electrospray ionization mass spectra (ESI) were recorded on a Waters mass spectrometer using a mixed solvent of HPLC methanol and triple distilled water.
3.2 Synthesis and characterization of a fluorescent Schiff base ((E)-2-((4-(diethylamino)benzylidene)amino)benzoic acid, DBAB)
4-(Diethylamino)benzaldehyde (2.00 g, 11.28 mmol) was dissolved in dehydrated ethanol (130 mL) in a 250 mL round-bottom flask. Then 2-aminobenzoic acid (1.55 g, 17.06 mmol) was dissolved in a small amount of N,N-dimethylformamide (DMF). The DMF solution and two drops of acetic acid were added to the flask. The mixture was stirred at 50 °C for 5 h. It was then cooled to room temperature before being filtered. The solid product was washed several times using ethanol and hexane. No further purification was needed. Yield: 53%. 1H NMR (600 MHz, DMSO-d6), δ (ppm): δ 8.64 (s, 1H), 8.04–8.02 (d, 1H), 7.72–7.61 (m, 5H), 7.32–7.30 (t, 1H), 7.21–7.18 (t, 1H), 6.82–6.81 (d, 2H), 6.72–6.71 (d, 1H), 6.49–6.46 (t, 1H), 3.45–3.41 (q, 4H), 1.12–1.10 (t, 6H). ESI-MS: m/z: 297.15 ([M + H]+ calcd 297.15).
3.3 Chromogenic detection of iron and copper ions
3.3.1 Fluorescence spectroscopic measurement.
DBAB was dissolved in DMF for a stock solution of 1.7 mM and diluted to 1.7 μM in DMF for absorbance and fluorescence analysis. The chlorinated salts (Fe3+, Fe2+, Cu2+, Cd2+, Cr3+, Li2+, Mn2+, Na+, K+, Mg2+, Zn2+, Ba2+, Ca2+, and Al3+) were prepared in deionized water at 60 mM. Test solutions were prepared by placing 2 mL of DBAB solution and 0.02 mL of different chlorinated salt solutions in a test tube, respectively. The resulting solution was mixed well. Fluorescence spectra were recorded at room temperature on a Hitachi spectrophotometer F-7000 at an excitation wavelength of 341 nm, slit 2.5/2.5 nm.
3.3.2 Job plot measurements.
DBAB was dissolved in DMF–deionized water solution (1
:
1, v/v) for a stock solution of 1.7 mM. The chlorinated salts (Fe3+, Fe2+ and Cu2+) were prepared in DMF–deionized water solution (1
:
1, v/v) of 6 mM.
For Fe3+, 0.142 mL of the DBAB solution were added to 11.40 mL of DMF–deionized water solution (1
:
1, v/v) to obtain a concentration of 20 μM. 0.04 mL of the Fe3+ solution were added to 11.96 mL of DMF–deionized water solution (1
:
1, v/v) to obtain a concentration of 20 μM. 2.0, 1.8, 1.6, 1.4, 1.2, 1.0, 0.8, 0.6, 0.4, 0.2 and 0 mL of the DBAB solution were taken and transferred to vials. 0, 0.2, 0.4, 0.6, 0.8, 1.0, 1.2, 1.4, 1.6, 1.8 and 2.0 mL of the Fe3+ solution were added to each DBAB solution separately. After shaking the vials for a few seconds, the fluorescence spectra were recorded at room temperature at an excitation wavelength of 341 nm, slit 2.5/2.5 nm. The job plots of Fe2+ and Cu2+ were measured in the same way.
3.3.3 Competitive experiments.
DBAB was dissolved in DMF for a stock solution of 1.7 mM and diluted to 1.7 μM in DMF for absorbance and fluorescence analysis. The chlorinated salts (Fe3+, Fe2+, Cu2+, Cd2+, Cr3+, Li2+, Mn2+, Na+, K+, Mg2+, Zn2+, Ba2+, Ca2+, and Al3+) were prepared in deionized water at 60 mM. 0.02 mL of the different chlorinated salt solutions (Fe3+, Cd2+, Cr3+, Li2+, Mn2+, Na+, K+, Mg2+, Zn2+, Ba2+, Ca2+, and Al3+) were added to 2 mL of DBAB solution, respectively. Then 0.02 mL of the Fe3+ solution were taken and added to the solutions prepared above, separately. The resulting solution was mixed well and the fluorescence spectra were recorded at room temperature at an excitation wavelength of 341 nm, slit 2.5/2.5 nm. For Fe2+, 0.02 mL of the different chlorinated salt solutions (Fe2+, Cd2+, Cr3+, Li2+, Mn2+, Na+, K+, Mg2+, Zn2+, Ba2+, Ca2+, and Al3+) were added to 2 mL of DBAB solution, respectively. Then 0.02 mL of the Fe2+ solution were taken and added to the solutions prepared above, separately. The resulting solution was mixed well and the fluorescence spectra were recorded at room temperature at an excitation wavelength of 341 nm, slit 2.5/2.5 nm. For Cu2+, 0.02 mL of the different chlorinated salt solutions (Cu2+, Cd2+, Cr3+, Li2+, Mn2+, Na+, K+, Mg2+, Zn2+, Ba2+, Ca2+, and Al3+) were added to 2 mL of DBAB solution, respectively. Then 0.02 mL of the Cu2+ solution were taken and added to the solutions prepared above, separately. The resulting solution was mixed well and the fluorescence spectra were recorded at room temperature at an excitation wavelength of 341 nm, slit 2.5/2.5 nm.
3.3.4 Distinguishing Fe3+, Fe2+ and Cu2+ by ascorbic acid reduction.
In the experiment, an excess amount of ascorbic acid (1470 equiv.) was introduced into the testing solution (1.7 μM DBAB and 353 equiv. of Fe3+, Fe2+, and Cu2+) which caused the quenched fluorescence to increase again gradually, and the fluorescence reached the maximum fluorescence recovery and half of the maximum, respectively, after 90 min. Contrarily, ascorbic acid had little effect on DBAB/Fe2+ solution. These different phenomena can be ascribed to the different reducing abilities of ascorbic acid toward Fe3+, Fe2+ and Cu2+.
3.3.5 Comparative 1H NMR analysis of DBAB–Fe3+/DBAB–Fe2+/DBAB–Cu2+.
To gain insight into the structure and complexation of Fe3+, Fe2+, and Cu2+ with DBAB, 1H NMR spectroscopy was performed in DMSO-d6. 0.2, 0.6, 1.0, and 1.1 equiv. of Fe3+, Fe2+, and Cu2+ in D2O solution were added to DBAB DMSO-d6 solution. The 1H NMR spectra were recorded by scanning 24 times.
4. Conclusions
In summary, a new approach using DBAB as a fluorescence quenching probe has been proposed to determine Fe3+, Fe2+ and Cu2+. This tri-responsive fluorescent probe for Fe3+, Fe2+ and Cu2+ can be distinguished by further reduction with ascorbic acid. The detection limits were 2.17 × 10−6 M for Fe3+, 2.06 × 10−6 M for Fe2+ and 2.48 × 10−6 M for Cu2+, respectively.
Conflicts of interest
There are no conflicts to declare.
Acknowledgements
This work was supported by the National Natural Science Foundation of China (No. 20474005), the General Program of Science and Technology Development Project of Beijing Municipal Education Commission (No. KM201310028007), and Scientific Research Starting Foundation for the Returned Overseas Beijing Scholars (Grant No. 009125403700).
Notes and references
-
(a) P. Aisen, M. Wessling-Resnick and E. A. Leibold, Curr. Opin. Chem. Biol., 1999, 3, 200 CrossRef CAS PubMed;
(b) D. Touati, Arch. Biochem. Biophys., 2000, 373, 1 CrossRef CAS PubMed;
(c) L. Guo, T. Tang, L. Hu, M. Yang and X. Chen, Sens. Actuators, B, 2017, 241, 773 CrossRef CAS;
(d) A. D. Bacon and M. C. Zerner, Theor. Chim. Acta, 1979, 53, 21 CrossRef CAS;
(e) J. A. A. Ho, H. C. Chang and W. T. Su, Anal. Chem., 2012, 84, 3246 CrossRef PubMed.
-
(a) B. D. Wang, J. Hai, Z. C. Liu, Q. Wang, Z. Y. Yang and S. H. Sun, Angew. Chem., Int. Ed., 2010, 49, 4576 CrossRef CAS PubMed;
(b) S. Denic and M. M. Agarwal, Nutrition, 2007, 23, 603 CrossRef CAS PubMed;
(c) Y. Shiono, H. Hayashi, S. Wakusawa and M. Yano, Med. Electron Microsc., 2001, 34, 54 CrossRef CAS PubMed;
(d) D. R. Brown and H. Kozlowski, Dalton Trans., 2004, 13, 1907 RSC.
-
(a) S. R. Bhupanapadu Sunkesula, X. Luo, D. Das, A. Singh and N. Singh, Mol. Brain, 2010, 3, 1 CrossRef PubMed;
(b) Y. Zheng, J. Orbulescu, X. Ji, F. M. Andreopoulos, S. M. Pham and R. M. Leblanc, J. Am. Chem. Soc., 2003, 125, 2680 CrossRef CAS PubMed;
(c) A. Torrado, G. K. Walkup and B. Imperiali, J. Am. Chem. Soc., 1998, 120, 609 CrossRef CAS.
-
(a) N. Singh, R. C. Mulrooney, N. Kaur and J. F. Callan, Chem. Commun., 2008, 40, 4900 RSC;
(b) X. B. Zhang, J. Peng, C. L. He, G. L. Shen and R. Q. Yu, Anal. Chim. Acta, 2006, 567, 189 CrossRef CAS.
-
(a) P. G. Georgopoulos, A. Roy, M. J. Yonone-Lioy, R. E. Opiekun and P. J. Lioy, J. Toxicol. Environ. Health, Part B, 2001, 4, 341 CrossRef CAS PubMed;
(b) D. J. Waggoner, T. B. Bartnikas and J. D. Gitlin, Neurobiol. Dis., 1999, 6, 221 CrossRef CAS PubMed;
(c) K. J. Barnham, C. L. Masters and A. I. Bush, Drug Discovery Today, 2004, 3, 205 CAS;
(d) D. G. Peters, J. R. Connor and M. D. Meadowcroft, Neurobiol. Dis., 2015, 81, 49 CrossRef CAS PubMed;
(e) H. S. Jung, P. S. Kwon, J. W. Lee, J. I. Kim, C. S. Hong and J. W. Kim,
et al.
, J. Am. Chem. Soc., 2009, 131, 2008 CrossRef CAS PubMed.
- K. Tayade, S. K. Sahoo, B. Bondhopadhyay, V. K. Bhardwaj, N. Singh and A. Basu,
et al.
, Biosens. Bioelectron., 2014, 61, 429 CrossRef CAS PubMed.
-
(a) X. H. Qian and Z. C. Xu, Chem. Soc. Rev., 2015, 44, 4487 RSC;
(b) X. Gao, C. Ding, A. Zhu and Y. Tian, Anal. Chem., 2014, 86, 7071 CrossRef CAS PubMed.
- Q. J. Ma, X. B. Zhang, X. H. Zhao, Z. Jin, G. J. Mao and G. L. Shen,
et al.
, Anal. Chim. Acta, 2010, 663, 85 CrossRef CAS PubMed.
-
(a) D. R. Brown and H. Kozlowski, Dalton Trans., 2004, 13, 1907 RSC;
(b) N. Wanichacheva, K. Setthakarn, N. Prapawattanapol, O. Hanmeng, V. S. Lee and K. Grudpan, J. Lumin., 2012, 132, 35 CrossRef CAS.
- F. Y. Yan, D. L. Cao, N. Yang, M. Wang, L. F. Dai and C. Y. Li,
et al.
, Spectrochim. Acta, Part A, 2013, 106, 19 CrossRef CAS PubMed.
- Y. Xiang, A. J. Tong, P. Y. Jin and Y. Ju, Org. Lett., 2006, 8, 2863 CrossRef CAS PubMed.
- L. Guo, T. Tang, L. Hu, M. Yang and X. Chen, Sens. Actuators, B, 2017, 241, 773 CrossRef CAS.
- Y. M. Zhou, J. L. Zhang, H. Zhou, Q. Y. Zhang, T. S. Ma and J. Y. Niu, J. Lumin., 2012, 132, 1837 CrossRef CAS.
- F. Ge, H. Ye, J. Z. Luo, S. Wang, Y. J. Sun and B. X. Zhao,
et al.
, Sens. Actuators, B, 2013, 181, 215 CrossRef CAS.
- H. Sasaki, K. Hanaoka, Y. Urano, T. Terai and T. Nagano, Bioorg. Med. Chem., 2011, 19, 1072 CrossRef CAS PubMed.
- H. Liu, Y. Dong, B. Zhang, F. Liu, C. Tan and Y. Tan,
et al.
, Sens. Actuators, B, 2016, 234, 616 CrossRef CAS.
-
(a) B. D. Wang, J. Hai, Z. C. Liu, Q. Wang, Z. Y. Yang and S. H. Sun, Angew. Chem., Int. Ed., 2010, 49, 4576 CrossRef CAS PubMed;
(b) X. H. Tian, X. F. Guo, L. H. Jia, R. Yang, G. Z. Cao and C. Y. Liu, Sens. Actuators, B, 2015, 221, 923 CrossRef CAS.
-
(a) Y. Li, C. Liao, S. Huang, H. Xu, B. Zheng and J. Du,
et al.
, RSC Adv., 2016, 6, 25420 RSC;
(b) J. Mao, L. Wang, W. Dou, X. L. Tang, Y. Yan and W. S. Liu, Org. Lett., 2007, 9, 4567 CrossRef CAS PubMed;
(c) S. R. Liu and S. P. Wu, Sens. Actuators, B, 2012, 171, 1110 CrossRef.
-
(a) Y. S. Lipatov, L. F. Kosyanchuk, N. V. Kozak, N. V. Yarovaya and G. Y. Menzheres, Polym. Sci., Ser. A, 2006, 48, 388 CrossRef;
(b) J. P. Holland, P. J. Barnard, S. R. Bayly, H. M. Betts, G. C. Churchill and J. R. Dilworth,
et al.
, Eur. J. Inorg. Chem., 2008, 12, 1985 CrossRef.
- Y. W. Choi, G. J. Park, Y. J. Na, H. Y. Jo, S. A. Lee and G. R. You,
et al.
, Sens. Actuators, B, 2014, 194, 343 CrossRef CAS.
- J. L. Zhang, Y. M. Zhou, W. P. Hu, L. Zhang, Q. Huang and T. S. Ma, Sens. Actuators, B, 2013, 183, 290 CrossRef CAS.
Footnote |
† Electronic supplementary information (ESI) available. See DOI: 10.1039/c8ay02526f |
|
This journal is © The Royal Society of Chemistry 2019 |