DOI:
10.1039/D4MA00129J
(Review Article)
Mater. Adv., 2024, Advance Article
Development of medical masks: performance, properties, and prospects
Received
10th February 2024
, Accepted 7th July 2024
First published on 17th July 2024
Abstract
The resurgence of masks due to the COVID-19 pandemic underscores their essential role as a simple yet crucial innovation. The evolution of masks mirrors the dynamic shifts in societal demands over time. Through a comprehensive examination of textile materials employed in medical contexts, this review highlights the enduring significance of filtering and antibacterial attributes of masks since their inception. As living standards advance, there is an escalating emphasis on the breathability of masks. Furthermore, this paper delves into the prospective significance and potential challenges associated with the development of comfortable, biocompatible, self-cleaning masks in the future. Different technologies, including mask detection systems, and advanced materials have been proposed in the framework of a multidisciplinary context. The multifaceted journey of masks encapsulates their pivotal role in safeguarding public health and underscores the ongoing pursuit of improved performance and user comfort.
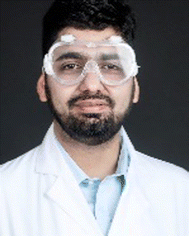 Hafeezullah Memon | Hafeezullah Memon is currently serving as a Distinguished Associate Professor at the Zhejiang Sci-Tech University, Hangzhou, China, after completing his postdoc at the same institute. He has been serving as Materials Scientist for Wuxi Paradise Textiles Mills, China, as well. His academic journey commenced at the Zhejiang Sci-Tech University as a postgraduate, where he earned a M.Sc degree in Textile Science and Engineering before pursuing a PhD degree in Textile Engineering at the Donghua University. |
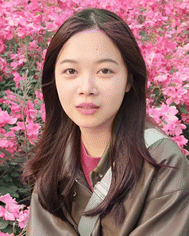 Siyi Liao | Siyi Liao embarked on her academic journey at the Zhejiang Sci-Tech University, Hangzhou, China, in 2021, where she became a part of Dr Hafeezullah Memon's esteemed research team. Her focus is within the team centres on the comprehensive study of fabric materials, delving into crucial areas such as antibacterial textiles, electrospinning, biomedical textiles, and structural colour. Siyi's commitment to exploring these innovative aspects of fabric materials showcases her dedication to contributing to advancements in textile engineering and materials science. |
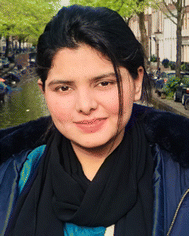 Rabia Maryam | Rabia Maryam is a PhD student in the Department of Physics at the University of Milano-Bicocca (Italy). She obtained her master's degree in physics from the COMSATS University Islamabad (Pakistan). Her scientific research interests are in the fields of nanomaterials, photocatalysis, antibacterial, and energy storage devices. She is doing research focusing on the functionalization of polymers by plasma etching and depositing graphene-based nanomaterials exploring their applications based on their properties. |
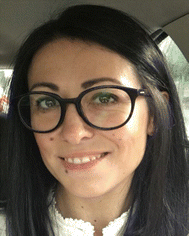 Alessia Patrucco | Prof. Alessia Patrucco is a researcher at the Institute of Intelligent Industrial Technologies and Systems for Advanced Manufacturing (STIIMA) of the Italian National Research Council since 2008. She has been cooperating in national and international research projects in textile and bio-polymer fields. She is a Contract Professor for the course of Textile fibres and Textile Sustainability for different Textile Academies. |
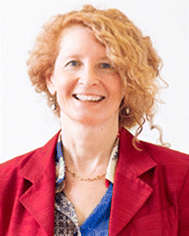 Claudia Riccardi | Prof. Claudia received her PhD degree in Physics at the University of Milano (Italy). Her scientific interests in the field of plasma physics are also oriented towards applications to materials and nanomaterials in sectors such as energy, environment, manufacturing and biomedicine. CR was involved in the development of plasma sources, diagnostics and processing, which expanded the understanding of plasma-material interaction and plasma applications. She is the director of the Plasma Prometeo Center at the University of Milano-Bicocca dedicated to the research and development of plasma applications and to the technological transfer to industries. |
1. Introduction
Textile materials are composed of different kinds of commodities used by humans for different purposes. They are composed of textile fibres usually joined together by surface friction forces. These fibres have different shapes, chemical compositions, and physical and chemical properties, suitable for specific applications.1 The variety of properties depends on both polymer fibre chemistry and supermolecular organization and morphology created during the fibre growth phase (natural fibres) or resulting from fibre chemical spinning (man-made fibres).1 Moreover, the final properties of textile goods depend on the hierarchical structure of the textile network (knitted, woven, non-woven structures, etc.). Fiber-based materials include conventional textiles (apparel) and technical textiles, which found application in different sectors such as building, agriculture, aerospace, medical, etc.
In the case of medical masks, the materials used have changed over the years as it has become clear that specific properties are required to make these devices effective. Firstly, for high filtration efficiency and safety, the chemical composition should result in hydrophobicity (preventing droplets from being absorbed by the fibres), and the physical shape of the fibres and the texture of the textile material should result in a small pore size (the best results are achieved by nonwovens based on micro/nanofibers). Traditionally, masks have been designed as disposable devices to ensure maximum sterility and safety for users. After the COVID pandemic, the importance of eco-design of future masks increased, but we are far from developing washable and reusable devices; for this reason also studies on the durability of antibacterial finishing through washing and sterilization cycles are still unknown and under investigation.
Medical textiles constitute a distinctive category of fabrics intended for medical applications, representing the culmination of interdisciplinary efforts.2 Notably, the research and development trajectory of biomedical textiles often extends beyond that of conventional textiles, such as those employed in domestic and apparel contexts. Consequently, the approval and market introduction of biomedical textile products typically entails more protracted processes.
Certain medical settings necessitate aseptic conditions, underscoring the critical role played by antibacterial and deodorant fibres. Antibacterial fibres encompass those endowed with bactericidal and/or bacteriostatic attributes. These fibres can be classified into two primary categories: one comprises fibres intrinsically embedded with antibacterial components like chitosan, apocynum, hemp, and metallic fibres; the other involves the incorporation of chelates, nanoparticles, metal powders, and antibacterial agents during spinning or surface treatment, as depicted in Fig. 1. Commercially available antibacterial fibres predominantly encompass inorganic variants like silver and zeolites, along with organic counterparts exemplified by the antibacterial acrylic staple fibres Amicor AB and antifungal Amicor AF, developed by the British firm Courtaulds. Additionally, bio-regenerated fibres like chitosan-based fibres find utility in diverse applications encompassing undergarments, bedding, hosiery, nursing attire, and hygiene textiles. The 1980s witnessed the emergence of nonwoven technology and the concurrent advent of novel materials, catalysing the gradual appearance of nonwoven masks within the purview of medical textiles. The exigencies of the SARS outbreak in 2003 notably accelerated the development of nonwoven medical masks, owing to the expedient and cost-effective nature of nonwoven fabrication techniques.
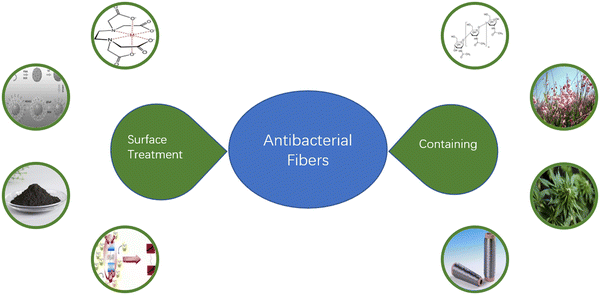 |
| Fig. 1 Different approaches for making antibacterial fibres. | |
The efficacy of antibacterial agents such as chitosan, metallic fibres (copper and silver), copper oxide (CuO), titanium oxide (TiO2), and ZnO in the medical industry has shown that these materials exhibit high levels of antibacterial activity. The bacterial and cell membranes of viruses are very sensitive to oxygen and the production of cations from metallic materials. In the last two decades, scientists have investigated metal oxide nanomaterials widely, demonstrating their adaptivity to various length scales and competitive morphological properties for different applications.3,4 The oxide materials are particularly notable because their synthesis is easy, cost-effective, and suitable for biomedical applications. Carbon-based nanomaterials and their compounds are effective agents in antibacterial activity due to their rare physical and chemical properties and high surface-to-volume ratio. D. Alagarasan et al.5 reported that the CuO NP-coated cotton textile showed excellent antibacterial activity against Escherichia coli (E. coli), Staphylococcus aureus (S. aureus), Bacillus subtilis (B. subtilis), and Pseudomonas fluorescens (P. fluorescens). OB Ahmed et al.6 examined that TiO2 is effective against various bacteria, with a higher concentration (2%) exhibiting stronger antibacterial activity against S. aureus and E. coli compared to lower concentrations (1% and 0.5%) after 18 hours. X. Zhang et al.7 reported that GO-modified ZnO microtube PAA of length 2–5 mm and a diameter of 200 mm was synthesized via the drop-spraying process. This showed excellent antibacterial activity with η > 100% under UV irradiation and η > 98% under sunlight. Furthermore, chitosan, a natural polymer with amino and hydroxyl groups, improves the antibacterial properties of polypropylene due to its biocompatibility. To maintain safety and efficacy over time, antibacterial agents must be stable over the long term. Evaluating the performance of the efficacy of these materials is their durability, especially through repeated laundering. J. Zhao et al.8 reported that graphene oxide (GO) is an excellent antibacterial agent and performed a laundering durability test. They synthesized GO sheets onto cotton fabrics prepared in three ways which possess strong antibacterial activity and good laundering durability. Antibacterial tests demonstrated that GO-containing fabrics can kill 98 percent of bacteria and continue to be inactive over 90 percent of bacteria even after 100 washes.
Moreover, the highly porous and air-permeable nature of nonwoven fibre meshes, coupled with their efficacious filtration capabilities, positioned them as the predominant filter materials for medical masks. This pertinence is further underscored by the reduced occurrence of skin allergies associated with microfiber nonwovens. Traditional woven fabrics are made using a weaving process that consists of forming a fabric by interlacing threads with an ordinate structure. The traditional woven process is applied on all fibres (natural and man-made) of common fineness (higher than 7 microns).
In contrast, the non-woven process consists of the production of a finished product made of synthetic thermoplastic fibre materials, in which the fibres are not woven, and they form a random network. This process makes it possible to produce fibres with a high fineness (small diameter) and a very tight network, both of which result in a low pore size and therefore high filtration. In order to scientifically compare the environmental impact of the two processes, an LCA analysis should be carried out; however, the first method results in a material that is no longer used for mask filtration.
In any case, not only the woven process but also the non-woven process is a physical process as the polymer forming fibre is melted and not dissolved (no chemistry used). For this reason, we assume that the impact of these products is not the process but the material for its poor end of life (synthetic polymers used remain in the environment for years and common masks are currently not recyclable due to different fibres/polymer compositions).
In the backdrop of mounting demand, the evolution from self-priming filter masks to intelligent masks has been a discernible trend. A study introduces the concept of a smart face mask, which incorporates a pivotal element in the form of an air-permeable and biodegradable self-powered breath sensor.9 The addition of smart technologies into medical masks provides significant advancements but it involves several restrictions and obstacles, including high costs, weight, and restrictions of size.10 Energy efficiency is a big challenge in smart wearable masks; thus, energy management is an important aspect of smart wearable devices. The battery life significantly affects user satisfaction, as frequent recharging can be annoying. Also, a rechargeable battery is a major contributor to the mask's weight, and sensor data acquisition may need a larger battery, increasing the mask's overall weight.11 Strong encryption, privacy, and data security are all necessary to protect sensitive health information. Therefore, J. Hyysalo et al.11 suggested that edge caching can improve a user experience by reducing latency, while federated learning and edge training offer solutions for privacy by authorizing the model training without revealing sensitive information. Additionally, it is necessary to establish security measures and privacy controls, possibly through government policies, to avoid the abusive use of smart mask data.12
However, recent medical observations have unveiled concerning aspects linked to mask usage. Instances of mucormycosis, a potentially fatal fungal infection, have exhibited a correlation with an extended usage of oxygen masks and nonwoven medical masks among middle-aged COVID-19 patients undergoing steroid treatments for diabetes mellitus.13 Furthermore, certain variants of N-95 masks have been implicated in cases of contact dermatitis due to the presence of free formaldehyde.14 These developments have underscored the burgeoning requirement for more comfortable mask fabrics, with an emphasis on materials demonstrating heightened biocompatibility. Consequently, the trajectory of mask material development is poised to pivot towards materials that enhance user comfort and biocompatibility, signifying a crucial avenue for future advancements.
2. Masks
Within the realm of clinical diagnosis, treatment, repair, healthcare, protection, and other domains, biomedical textiles manifest in diverse categories. Varied medical textile requirements necessitate the development of biomedical textile materials possessing specific attributes tailored to meet distinct demands. In the context of biomedical textiles, a mask emerges as a fundamental medical protective apparatus employed to filtrate the air entering the nasal passage of individuals, serving to impede the ingress or the release of certain microorganisms, particulates, and other contaminants.
The eruption of SARS-CoV-2, the virus responsible for COVID-19, in late 2019 in Wuhan, China, marked a profound turning point in the use, fabrication, and research. A rigorous scientific evaluation conducted by Chu DK et al. confirmed that masks substantially diminish the risk of infection by SARS-CoV-2, SARS-CoV, and MERS-CoV viruses.15 Notably, N-95 masks exhibited superior efficacy compared to medical masks or cloth masks.16 Furthermore, the research conducted by Mehmet Ferhat Sari et al. indicated that mask usage substantially curtailed the risk of infection, with medical triple-layer masks demonstrating enhanced effectiveness compared to regular cloth masks, thus offering significant protection against the spread of infections, particularly respiratory diseases.17
A mask can be defined as an array of filtration mechanisms underlying mask filter materials, including diffusion deposition, electrostatic attraction deposition, trapping deposition, and inertial deposition.18 The efficacy of these mechanisms is contingent upon particle size. In general, masks may have different purposes: on the one side, a mask may be useful for preventing biological/physical materials from entering the mouth and nose in healthy people (“self-protective” face masks) and on the other side a mask may be designed to prevent the spread of biological contaminants from the mouth and nose in potentially infected people (“protection of others” face masks).1
Medical protective masks adhere to the technical requisites outlined in GB 19083-2010. When subjected to a gas flow rate of 85 L min−1, these masks must meet the following filtration efficiency criteria for non-oily particles: a 1st stage filtration efficiency of ≥95%, a 2nd stage filtration efficiency of ≥99%, and a 3rd stage filtration efficiency of ≥99.97%.19
Surgical masks are aimed to protect patients from the bacterial infection exhaled by the team performing the surgery.20 Surgical masks conform to the specifications delineated in YY 0469-2011 and their efficacy is measured through various criteria: bacterial filtration efficiency (BFE) should surpass 95%. Particle filtration efficiency (PFE) for non-oily particles should be at least 30%. Synthetic blood, sprayed at a pressure of 6.0 kPa (120 mmHg) onto the outer side of the mask, should not penetrate to the inner side.
Disposable medical masks must meet the stipulations outlined in YY/T 0969-2013: bacterial filtration efficiency (BFE) should exceed 95%. Non-sterile masks should adhere to specific microbiological limits (total bacterial colonies ≤100 CFU g−1, the absence of coliform bacteria, picocyanobacterial, Staphylococcus aureus, haemolytic streptococcus, and fungi).19 Technical requisites of masks for non-medical devices for respiratory protective equipment and self-priming filter anti-particulate respirators adhere to GB 2626-2006. Filtration efficiency varies for different filter elements: KN90: filtration efficiency ≥90% for sodium chloride (NaCl) particles. KN95: filtration efficiency ≥95%. KN100: filtration efficiency ≥99.97%.19
3. Materials for masks
3.1. Nonwovens are popular materials for masks
Historically, masks were fashioned from materials like cotton and linen, but today, nonwovens have surged to the forefront as the dominant material for mask production, as presented in Fig. 2. In simple terms, nonwovens diverge from conventional textile methods that involve spinning fibres into yarns and then weaving them into the fabric.
 |
| Fig. 2 Manufacturing of masks from nonwovens. | |
Instead, nonwovens employ specialized techniques to directly fashion fibres into fabric-like textiles. From a technical standpoint, nonwovens consist of sheets, webs, or pads composed of fibres arranged in oriented or random configurations through processes like rubbing, entwining, bonding, or synergy of these methods.20 Dispensing with many stages intrinsic to traditional textile production – such as framing, roving, fine yarn, and winding – nonwovens streamline their process, bypassing spinning, weaving (or knitting), sizing, and weaving, leading to an abbreviated workflow, as presented in Fig. 3. Remarkably efficient, nonwovens boast high production speeds (ranging from 10 to 300 meters per minute) and extensive automation, culminating in the manufacture of fibre-based products. Most non-woven materials are disposable and single use produced with low-cost technologies. During the past several years, different technologies have been implemented in the fabrication of non-woven fabrics, even though spun-bonding and meltblown are the most used techniques.20
 |
| Fig. 3 Steps involved in the formation of nonwovens. | |
In meltblown and spun bonding processes, different thermoplastic polymers such as polypropylene, polystyrene, polyesters, polyurethane, nylon, polyethylene, and polycarbonate are used, although the most popular polymer is polypropylene.20 Certain nonwoven techniques, such as the spun bonding and meltblown methods,21 can seamlessly transition from the chemical fibre spinning stage to fabric formation, even achieving astounding speeds of 600 to 800 meters per minute. This confers upon nonwovens a production capacity, speed, and cost-effectiveness per unit area that conventional textile techniques struggle to match. Nonwovens can be created using various fabrication processes as illustrated in Fig. 4. Structurally, nonwovens predominantly assume three-dimensional configurations formed from individual fibres, contrasting the two-dimensional, interwoven yarn structures typical of traditional textiles.21 This distinction imbues nonwovens with microporous air permeability, exceptional surface coverage, dimensional stability, and gradient filtration capabilities. Comparative analyses of nonwoven and conventional medical cotton fabrics in terms of bacteriological barriers and infection rates following a surgery reveal marked superiority of nonwoven fabrics, a consensus observed across Europe, America, and Japan. The factors underpinning the enhanced efficacy of medical nonwovens involve their diminished pore size, reduced presence of fibrils, resistance to hair shedding, and intrinsic water repellency.
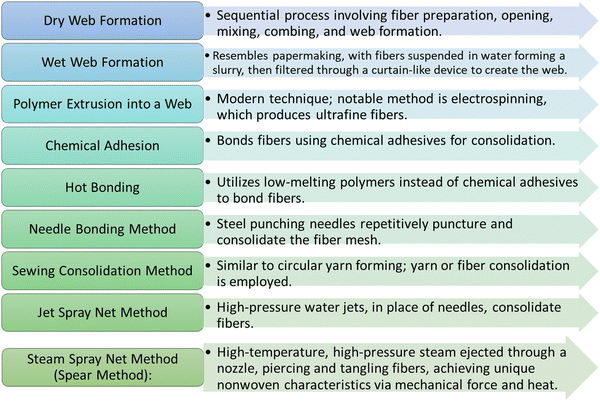 |
| Fig. 4 Nonwoven fabrication processes. | |
These attributes are especially salient during surgical procedures, where nonwovens effectively counteract bacteria in the critical 3–11 μm size range most detrimental to incisions while minimizing bacterial collection facilitated by surface fluff. Notably, water repellency emerges as an essential prerequisite for effective bacteriological barriers, avoiding droplet adsorption, a notion explored in the review by W. Wang et al. of superhydrophobic filterable nonwovens,22 as depicted in Fig. 5. Beyond medical applications, nonwoven fabrics are embraced as convenient, hygienic, and sterilizable options for disposable health products, with suitability for travel and emergency medical scenarios.
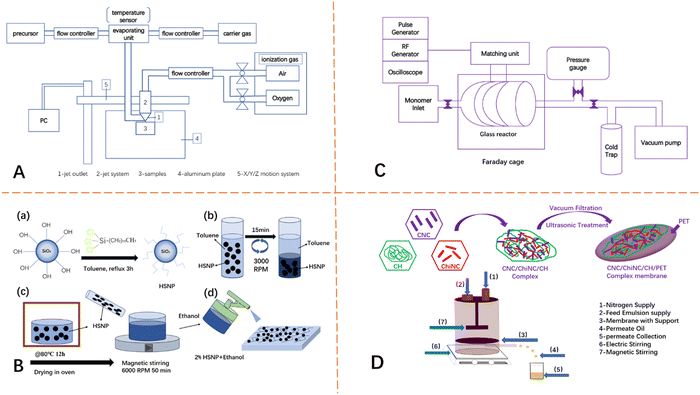 |
| Fig. 5 Preparation diagram of superhydrophobic nonwovens using other methods. (A) An atmospheric pressure plasma jet system schematic illustration. (B) A demonstration of the essential steps in depositing and preparing a superhydrophobic coating. (a) SiO2 nanoparticle derivatization through hydrophobicity. (b) Using centrifugation to separate hydrophobic SiO2 nanoparticles (HSNPs). (c) Using magnetic stirrer to help HSNP drying and dispersion in ethanol. (d) Spraying superhydrophobic coating on textiles. (C) Schematic illustration of pulsed plasma polymerization device. (D) This figure shows a schematic depiction of creating the complex membrane based on polysaccharides and the filtration process.22 (All images redrawn by authors). | |
These materials streamline and modernize hospital operations, optimizing efficiency, and cost-effectiveness by eliminating numerous stages inherent to medical cotton fabric usage. While nonwovens demand robust fibre performance, they exhibit greater adaptability in processing than traditional textiles. Virtually, all natural and synthetic fibres feasible for traditional textiles find applications in nonwoven production, and this versatility extends to include unconventional fibres like textile scraps, recycled fibres, and even metal fibres. By accommodating a diverse array of fibres with distinct attributes, nonwovens seamlessly cater to varied textile requirements, substantiating their central role in contemporary medical textiles.
3.2. Electrostatic spinning: a nanofiber fabrication technique
Electrostatic spinning, a process that involves subjecting a polymer solution to a high-voltage electric field, results in the stretching of nanofibers, as evidenced by various studies.21,23 This technique has demonstrated its significance not only in traditional textile engineering but also in advanced applications such as kidney tissue engineering and oral peroral delivery of biologic therapeutics.24–26 In the context of mask fabrication, the central layer typically consists of a melt-blown nonwoven fabric, specifically designed to filter particulate matter (PM) and bacteria. It is worth noting that the efficacy of particle filtration is compromised when the fibres within this layer are of substantial thickness, consequently hampering the attainment of both high efficiency and low impedance. To address this challenge, alternative materials such as polytetrafluoroethylene membranes27 or polyvinyl butyral (PVB) matrices can be employed, serving as a platform for the production of nanofiber membranes through the electrospinning process. Remarkably, recent investigations have showcased a straightforward approach wherein nontoxic polyvinyl butyral (PVB) serves as the polymeric matrix for electrospinning. The resulting nanofibrous structure incorporates thymol, a natural phenol monoterpene known for its antibacterial properties.28 Notably, Fig. 5 illustrates the schematic diagram of the electrospun thymol/PVB nanofibrous facemask, highlighting the feasibility of this technique in crafting novel antimicrobial protective materials, as shown in Fig. 6. This dynamic process, often associated with textile engineering, is increasingly demonstrating its potential for diverse medical applications, as indicated by the growing trend toward its integration into medical treatments and healthcare solutions, as illustrated in Fig. 7.
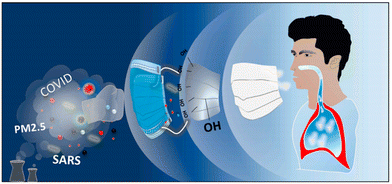 |
| Fig. 6 Schematic of the electrospun thymol/PVB nanofibrous facemask.28 | |
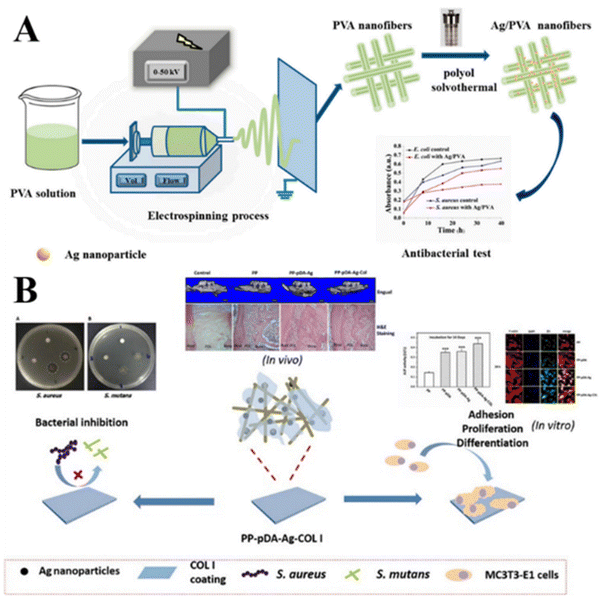 |
| Fig. 7 (A) Schematic illustration of fabrication and the antibacterial test for Ag/PVA composite nanofibers through the electrospinning and solvothermal methods.29 Copyright 2020 by the authors. (B) Triple PLGA/PCL scaffold modification including silver impregnation, collagen coating, and electrospinning significantly improves biocompatibility and antimicrobial and osteogenic properties for orofacial tissue regeneration.30 Copyright 2019 American Chemical Society. | |
3.3. 3D printing: customized medical masks
3D printing technology can be very effective in performing medical devices including medical masks. In ref. 31, the study of the efficacy of 3D printing as a fabrication method concerning conventional technologies is reported for protective equipment against COVID-19. Then, the development of polymers with antimicrobial properties to be used for 3D printing makes possible the fabrication of different critical medical tools. In ref. 32, 3D manufacturing based on Internet of Things technology has been proposed for medical personalized masks. A design process utilizing 3D printing based on individual faces has been proposed, including also temperature sensors, to control some health parameters such as breathing temperature and irregular breathing behaviour and strain sensors to monitor face irritation. A sophisticated data acquisition system permits real-time data control and fabrication.
3.4. Metals and graphene based materials as filtration media
In ref. 33, a complete overview of the mask and respirator (MAR) fabrication techniques focusing on the new material and technologies is presented. It includes different potential solutions as technologies such as laser scanning, 3D printing, and related materials such as nanofibers, graphene-based filters, and metal–organic base frameworks,20 as can be seen in Fig. 8. Here, the inclusion of graphene-based materials can be used as antimicrobial nanomaterial coating, enhancing hydrophobicity. The authors outlined the need to deepen research on new functional materials to enhance health and safety. Recently, scientists34 have been working to enhance the functionality of medical masks including a variety of metal nanoparticles to efficiently kill pathogens.
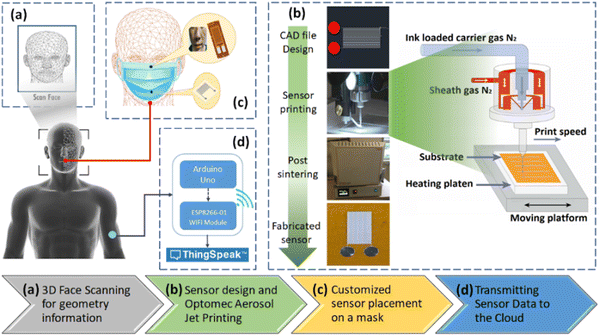 |
| Fig. 8 The process of designing a smart mask system. (a) Obtaining 3D facial scan data and scrutinizing face geometry (b) using AJP technique printing temperature and strain sensors. (c) Schematic of the smart mask. (d) Data processing. | |
Graphene, with renowned antiviral and antimicrobial properties, has enhanced the interest of researchers in probing its use in the diagnosis of COVID-19. In ref. 35, they proposed that the antistatic activity, antibacterial activity, sharp edge, photosensitivity, electrical conductivity, and large surface area of graphene nanomaterials20 provide an optimal base for making a shielding membrane and delivering a lot of benefits to the face mask as shown in Fig. 9.
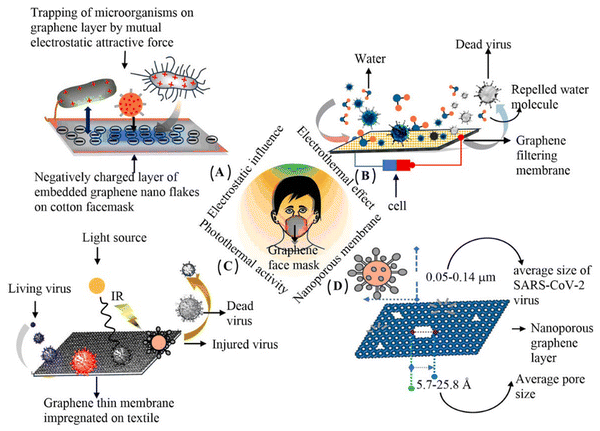 |
| Fig. 9 A schematic diagram of graphene-based face mask filtering efficiency. (A) By electrostatic force of attraction, graphene materials trap microorganisms. (B) The electrothermal properties of graphene inactive harmful viruses. (C) Cloth-mounted graphene can damage the envelope of the protein virus in IR radiation. (D) The average diameter is smaller than that of SARS-CoV-2 virus.35 | |
Using laser-induced graphene (LIG) techniques, graphene layers with superior bacterial efficacy were fabricated.36
The bactericidal effect is due to a combination of photothermal action with the graphene wettability properties.
LIG converts different carbon precursors, the most efficient was obtained by polyimide (PI), where super hydrophobic/hydrophilic graphene layers were obtained, showing high antibacterial properties, as shown in Fig. 10. The device can be used as a LIG hygroelectric generator to detect human exhalation.
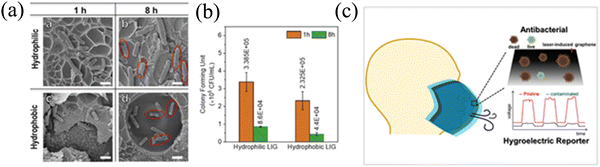 |
| Fig. 10 (a) Antibacterial activity of hydrophilic/hydrophobic LIG substrates: E. coli SEM images on hydrophilic/hydrophobic LIG substrates (2 μm scale). (b) CFU (colony forming unit) evaluated for the two samples of LIG. (c) A schematic of the hydroelectric generator application.36 Copyright © 2020 American Chemical Society. | |
3.5. Plasmas for surface treatments and active disinfection
Cold plasmas are greatly used to functionalize material surfaces for different applications.37 Grafting,38 coating,39 and morphological modifications40 can change wettability,41 enhance anti-bacterial properties, and modulate the permeability42 to favour.
The adhesion of coating and coupling between different thin films could be helpful for mask functionalization and disinfection. Hexamethyldisilane (HMDSO) plasmas have been used on textile surfaces to generate water-repellent masks. By applying plasma-enhanced chemical vapor deposition on cotton fabrics characterized by very small water contact angles (around 0°), fabrics behave hydrophobic with contact angles reaching 120°. The water repellence, maintained after many washing cycles, proved to be adequate for the fabrication of protective masks.43 To enhance the ability of non-woven polypropylene-base fabrics (NWF-PP) to retain active biomolecules, treatment of cold plasma with oxygen was used. The results showed that the plasma treatment alters the chemical properties of the fabric (NWF-PP), favouring the incorporation of chemical functionalities and their stabilization on the surface.44 To treat surfaces composed of polymethyl methacrylate and polycarbonate, low-pressure plasmas of oxygen (O2), carbon tetrafluoride (CF4), and air were used. Applying CF4 plasma treatment, the contact angle was increased, by O2 plasma treatment the surface exploited hydrophilicity, and by air plasma the surface behaved as super hydrophilic.45,46
In ref. 47, the effects of plasma treatment at reduced pressure are discussed. Plasma was efficiently used as a sterilization tool on the three-layer medical mask. Plasma treatment with argon and air also affected the vapour permeability, increasing its hygroscopicity which improves the hygienic properties of the mask preserving the mask hydrophobicity. In ref. 48, researchers proposed a medical mask with an active plasma layer to enhance the filtration efficiency against viruses and bacteria, producing an active mask self-disinfection. The technology uses pulsed atmospheric barrier discharges synchronized with the respiration, to disinfect the air flows in the two flowing directions, as shown in Fig. 11. In ref. 49, the authors present an innovative approach to making filters involving bacterial cellulose (BC) treated with low-pressure argon plasma (LPP-Ar). The attained results with (LPP-Ar) on BC materials exploit excellent antiviral and antimicrobial properties against bacteria. These filters consist of biodegradable three layers and can be used for respiratory personal protective equipment (PPE), such as respirators and medical masks.
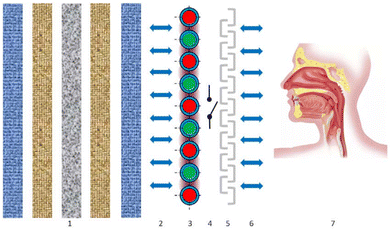 |
| Fig. 11 A scheme of the system. 1 is coupling layers; 2 and 6 show the bidirectional air flows; 3 is electrodes that are used to generate plasma (violet light) filling the space between the layers and the dielectric layer (5); 4 is the switching system that is able to switch on/off the plasma during the expiration/inspiration; 5 is the dielectric material to isolate the body from the electrodes; 7 is mask wearers.48 Copyright © 2020 American Chemical Society. | |
3.6. Face mask recognition systems
During the pandemic period, it was necessary to be equipped with mask-dressing control systems. Mask detection is very important as a control tool also in specific hospital environments that need their use.
The Review Paper50 recommends straightforward techniques using different machine learning tools to detect face masks in images and videos with high accuracy. The suggested recognition systems are able to recognize both stationary and moving faces with or without masks, optimizing parameter values to prevent overfitting.
In ref. 51, deep learning tools with Python to detect the different types and shapes of masks in video streams for following government rules have been proposed. In ref. 52, researchers advocate for the adoption of a specific tool (YOLOV5), a most robust object detection algorithm, to swap the manual inspections and enhance real-world implementation in public spaces. The experiments demonstrated the accurate recognition of face masks and effective monitoring of the individuals.
3.7. Pushing boundaries: innovations in materials for masks
The masks are gone through surface treatments, i.e., functional finishing, to enhance their performance. Besides, two or more nonwovens (or nonwovens and other textiles, plastics, etc.) are combined with different properties through chemical, thermal, or mechanical methods, to integrate the excellent properties of a variety of materials. In addition to nonwovens, various high-tech materials such as nanofilter materials are also constantly emerging. The unique size effect of nanofibers makes nanofiltration materials have higher porosity than melt-blown microfiber nonwoven.53 The integral role of adhesives within nonwovens warrants attention. Adhesives assume a pivotal function in the dry, wet, extrusion, and finishing processes of nonwovens. Classified into synthetic and natural categories, adhesives predominantly rely on water-based formulations, encompassing water-dispersible and water-soluble adhesives. The selection of adhesives significantly influences the ultimate properties of nonwovens, impacting their performance and functionality.
4. Mask structure
In the pursuit of optimal mask functionality, a delicate balance is required between effective particle filtration and user comfort. The front arched air cavity consists of two parts, three layers of textile materials, and a skeleton,21 where each layer of the mask serves a distinct purpose:
Surface layer
This layer, often created using the polypropylene spun-bonding method or hot rolling, endows the mask with moisture-resistant properties.
Core layer
Positioned for efficient filtration and air permeability, the core layer employs melt-blown polypropylene microfibers with an average diameter of less than 4 microns (or even less than 2 microns in tight arch configurations).
Inner layer
Designed for a skin affinity, the inner layer employs white spun-bond materials, enhancing user comfort.
The three-layer design of medical masks addresses this concern, employing varying compositions for each layer, as shown in Fig. 12. Typically crafted from materials like polypropylene (PP) and polyacrylonitrile, the outer layer is made of spun-bond nonwoven PP, featuring larger pores of approximately 100 μm. Meanwhile, the middle layer utilizes the melt-blown nonwoven fabric with smaller pores measuring around 20 μm, as discussed by Ju et al. in 2021.19 The mask's structure is further enhanced using a nose bridge clip, crafted from rust-resistant plastic. This component can be customized to ensure a secure and snug fit, aligning with an individual's unique face shape and nose bridge height. When it comes to securing the mask, options include ear-hook and strap types, with a preference against using latex elastic bands for ear-loop elastic bands, considering sensitivities. Innovative advancements continue to shape mask technology. Addressing concerns surrounding respiratory infection risks and environmental impact, J. Liang et al. introduced an inventive approach. Their research led to the development of a novel mask material incorporating an antibacterial and biodegradable aerogel as an intermediate filter layer, resulting in improved air filtration, antibacterial properties, and environmental responsibility.54
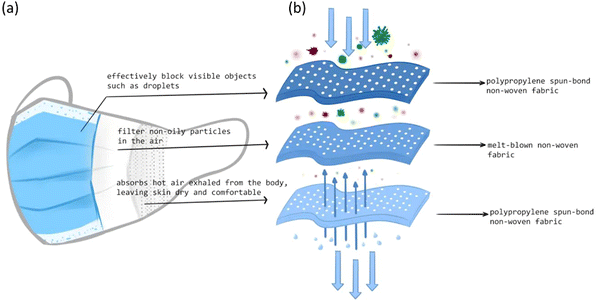 |
| Fig. 12 Three layers in the mask structure. (a) Schematic diagram of the medical protective mask.55 (b) Three-layer nonwoven mask with spun-bond and melt-blown polypropylene sheets.15 | |
5. Mask performance analysis
Masks exhibit diverse performance characteristics that cater to varying medical and health requirements. Notable facets of mask performance encompass filterability, antibacterial attributes, breathability, comfort, fit, and surface moisture resistance, among others. This article primarily delves into four crucial aspects: breathability, filterability, antibacterial properties, and comfort. The qualitative assessment of mask suitability serves as a valuable tool for medical personnel, aiding them in selecting appropriate medical protective masks based on test outcomes. This not only enhances the medical personnel's understanding of protection measures but also diminishes the risk of infection.56
5.1. Air permeability
Across all mask types, an important consideration is the potential impact on breathing resistance and consequent pressure exerted on the cardio-pulmonary system of the wearer.57 Some studies have reported heightened cardio-pulmonary strain upon wearing protective face masks.58,59 Conversely, other investigations have indicated minor effects with negligible repercussions on wearers’ health.60–62 A pivotal parameter influencing air filter performance is the areal density of the membrane. This metric, which denotes the mass-to-surface area ratio of the film, can also be employed as a reference index for fabric filtration efficacy. Researchers argue that the filtration efficiency of nanofiber membranes escalates with an increase in areal density. For instance, Lin Du et al. observed nanofibers forming a porous network structure, augmenting porosity and enabling efficient interception of particulate matter,63 which was otherwise considered as serious burden to the environment and health.64
5.2. Filterability
The size spectrum of viral particles typically ranges between 0.05 and 3 μm, with a smaller end often around 18 to 22 nm. These particles tend to coalesce with droplets and aerosols. Medical masks predominantly rely on the physical structure of microfiber nonwovens to obstruct particles larger than 3 μm in diameter. Conversely, for particles, droplets, and aerosols below 3 μm, electrostatic adsorption plays a pivotal role. In addition to innovating spinning techniques to craft sub-micron and nanofiber nonwovens, medical masks incorporate technologies such as electret, negative ions, and activated carbon to enhance nonwoven filtration efficiency. Notably, Lin Du et al. dissolved polyethylene terephthalate (PET) in fluoroacetic acid (TFA)/dichloromethane (DCM), electrospinning nanofibers to yield nonwovens with a uniform diameter distribution and optimal shape. This novel nanofiber nonwoven boasts a remarkable filtration efficiency of up to 87% for PM2.5, signifying a significant advancement in mask filter materials.63 It is important to note that two principal standards outlined by NF EN 14683:2019 include BFE (filtration capacity of display mask materials) and DP (indicating mask material breathability). Comparative analysis of various mask materials can be performed based on these standards. To quantify these parameters, tests involved the incubation of samples at 37 ± 2 °C for 22 ± 2 hours. A colony counter (Inter-science) scanned the resulting dishes to enumerate colony formation units (CFUs), and the outcomes were presented in CFU and BFE. BFE is calculated using the following relationship: BFE = C − T
C × 100(1), where C is the total CFU counted by 6 plates. The average of the two running sessions, and T counts the total CFU for each test sample of 6 plates. BFE analysis was performed on 5 masks per series, and the BFE level of the masks was determined by the lowest BFE obtained in the 5 test samples.19 Based on these tests, it is possible to compare the filtering capacity and breathability of the mask. A paper65 reports a huge study on the filtration efficiency of common cloth mask fabrics (cotton, silk, chiffon, flannel, and synthetic) for aerosol-containing particulates composed of particles from 10 nm to 10 mm in size. They were able to measure a spectrum of filtration efficiencies varying with the size of the particulate matter, depending also on the fabric materials. From these studies, the suggestion was to create a multilayer system of different coupled fabric materials, to be able to make the highest efficiency over the entire dimensional spectrum. Notably, the fabric combinations including cotton with other fabrics gave the highest efficiency up to 90% for particles >300 nm. The review article66 provides a comprehensive review of the mechanisms of the aerosol across the mask in dependence under different environmental conditions. They studied the filtration mechanisms on various face and respiratory masks and numerically simulated different phenomena. In ref. 67, a specific study on the filtration properties of masks revealed that effective cloth masks typically have specific properties. These include muslin with 100 threads per inch in 3 to 4 layers, single-layer tea towels, or high-quality cotton T-shirts with layers and stitched edges. According to their experiments with flat cloth, linen tea towels, 600-thread per inch cotton in 2 layers and 600 thread per inch cotton with 90 thread flannels, were obtained well. According to this reference, it is important to note that using more layers, 3 to 4 improves filtration but makes breathing harder.
5.3. Antibacterial attributes
A fundamental prerequisite for effective antimicrobial attributes in medical textiles is the conveyance of active agents to target microbial cells through water or leaching. The resilience of the weather-resistant antimicrobial finishing agent, citric acid, has demonstrated its efficacy in diminishing the presence of specific bacteria and fungi on medical cotton textiles. Notably, enhanced antimicrobial outcomes were observed in microwave-dried/cured cotton samples, highlighting the agent's potential.68 The evaluation of antibacterial efficacy in the test fabric was conducted using GB/T 20944.2-2007 standards. For this assessment, Escherichia coli (E. coli) and Staphylococcus aureus (S. aureus) were subjected to measurement. The antibacterial rate (A) was quantified using the formula:
. Here, Ct signifies the count of colonies cultured in cotton fabrics, while Tt represents the colony count in the fabric culture.69 In the realm of inorganic antimicrobial agents, titanium dioxide nanoparticles (TiO2 NCs) have garnered increasing attention, particularly considering their remarkable attributes such as high stability, nontoxicity, and ease of manipulation. Especially, noteworthy is their potential as a promising nano-antibacterial material, especially when subjected to light irradiation,23,70–72 see Fig. 13.
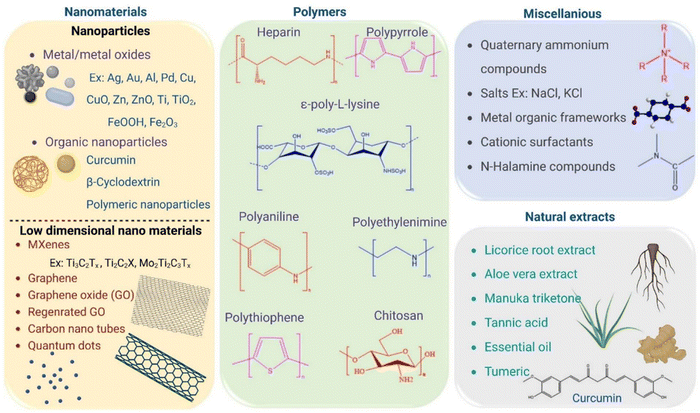 |
| Fig. 13 Different materials that possess antimicrobial properties (created with https://BioRender.com).72 | |
Further exploration of antimicrobial strategies reveals the work of Mazyar Ahrari et al. Their findings demonstrated that the integration of silver nanoparticles into a three-layer mask resulted in remarkable antimicrobial and antiviral properties. However, it is important to note that this enhancement in antimicrobial performance was accompanied by reduced breathability, as indicated by air permeability results.15
5.4. Comfort
Typically, the assessment of mask comfort involves a two-fold approach – scrutinizing the material properties of the masks and gauging the subjective impressions of test subjects. The paramount aspect of mask comfort lies in the sensation of facial skin contact. This comfort can be objectively evaluated through dynamic and elastic friction coefficients.
In the realm of material property analysis, Y. Kasasawa et al.73 conducted a comprehensive study. Their investigation encompassed the examination of compression, tensile, and bending characteristics of face mask (FM) fabrics in a dry state, employing the Kawabata evaluation system (KES). Additionally, the surface attributes of the FM were taken into consideration. The properties of various materials, including cotton and polyester fibre, were meticulously measured under dry conditions.
Upon deriving the dynamic and elastic friction coefficients, it becomes evident that non-slip fabrics exhibit commendable tensile properties, rendering them suitable for the fabrication of comfortable FM materials. Notably, Rayon demonstrated the lowest dynamic friction coefficient, making it the smoothest option, followed by polyester and cotton in the descending order of non-slip properties.
The work of Masayo Suekawa et al.74 showcases a comparison of results from diverse masks investigated by Teesing et al.75 Masayo Suekawa et al.74 pursued a unique approach by creating a thinner nanofiber layer, which was meticulously integrated onto the surface of the habutae fabric at a density of 0.5 g m−2. Their study yielded a compelling conclusion: nanofiber habutae masks exhibited a notably higher level of comfort compared to traditional nonwoven masks.
5.5. Biodegradable materials
The filter layer of conventional single-use face masks (SFMs) primarily relies on non-biodegradable petroleum-based polypropylene melt-blown cloth, functioning through electrostatic adsorption to capture ultrafine particles.
Consequently, the filtration efficiency experiences a gradual decline due to moisture generated by the wearer's exhalation, leading to changes in humidity. This decline in effectiveness, combined with the lack of durability, has contributed to a significant increase in mask consumption and the resulting accumulation of non-degradable waste. Masayo Suekawa and colleagues designed a unique three-dimensional mask configuration, comprising two layers of woven fabrics.74 One of these layers featured an inner nanofiber layer, while the other utilized a standard woven fabric without nanofibers on the exterior. This innovative mask design was subjected to testing involving 95 individuals. Impressively, the participants’ feedback indicated a preference for the comfort of the nanofiber habutae masks over conventional nonwoven masks. It is noteworthy that the silk woven masks did not induce allergic reactions or skin irritation symptoms in wearers, further highlighting their potential benefits.
Fig. 14a illustrates that the floss silk fibres carry different kinds of microbes that enhance the degradation of TRespirator, directing to the degradation of MP. On the other hand, without plants attracting the microbes, the absence of enzymatic contact with the plastic delays the degradation process. However, in the TRespirator, the mixture of leaf fibres and plastic promotes effective degradation in nature. Fig. 14b shows the microbial effect on the TRespirator. The microscopic analysis of TRespirator samples at the start and after two weeks were performed. Fig. 14c demonstrates that the TRespirator with the 95% plant fibre achieves almost total plastic degradation in 30 days.76
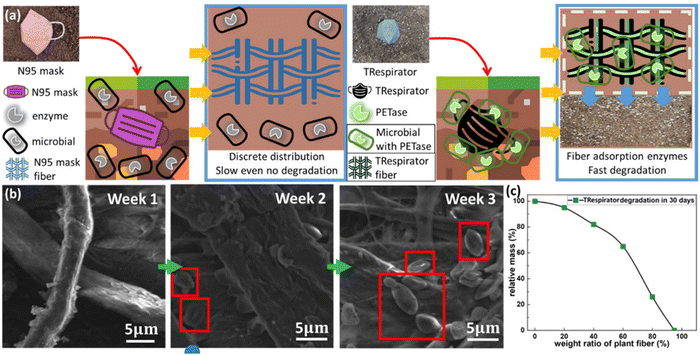 |
| Fig. 14 The degradation mechanism of the plant–fibre–plastic system in the TRespirator. (a) The degradation mechanism of an N95 mask and the TRespirator. (b) SEM images of degrading TRespirator samples from week 1 to week 3. (c) Image about the degradation effect in 30 days with a different weight ratio of plant fibres.76 | |
This predicament underscores the pressing necessity to transition away from petrochemical-derived materials and embrace more environmentally sustainable bio-based alternatives.77 Extensive research has explored and harnessed bioderived materials for the creation of nanofibers,78 including chitosan,79 β-cyclodextrin,71 collagen,80 and soy protein,81 among others. Also, polylactic acid (PLA), a thermoplastic synthetic polymer usually produced starting from plant derivatives (bio-based) in the place of petrol, can be processed into a non-woven material. PLA is not biodegradable in the environment, but it is compostable, which makes it better in terms of end-of-life environmental impact.20 These endeavours hold promise in addressing the environmental impact of disposable masks and advancing toward a more ecologically responsible approach.
5.6. Reuse and recycling: decontamination techniques and waste processing
Currently, the majority of the waste is disposed of using traditional methods such as landfilling and incineration,82 and these methods may lead to environmental pollution for gases resulting from the incineration of microplastics released from landfilling.83
Unlike biomedical wastes, waste masks mainly were disposed of in mixed methods not treated as bio-medical waste and not reused or recycled at all.84 Many studies refer to the big challenges associated with the production and disposal of face masks, highlighting the potential threats posed by disposable and manufacturing techniques. Improper waste management allows viral propagation leading to long-term health concerns. This Review85 assimilates information on the various forms of personal protective equipment currently in use; in addition, the proper waste management strategies for the specialized materials are discussed. In ref.86, 5 techniques used for mask decontamination are reported based on ethylene oxide gas, autoclave processing, plasma treatment at low pressure, peroxides, and peracetic acid. A single cycle of treatment efficiently decontaminated masks without causing structural or functional destruction, according to the five decontamination techniques on a variety of N95 masks contaminated with SARS-CoV-2 or a surrogate virus. Particularly promising methods included autoclaving, vaporized hydrogen peroxide, and dry fogging with peracetic acid. In ref. 87, a study on the prolonged use of medical masks on the skin has been performed, and atmospheric pressure plasmas were used for mask disinfection. They found that nitrogen plasmas were more effective for the mask disinfection for three model bacteria, without decreasing its functionality permitting the mask reusability. As also sketched in Fig. 15, bacteria were inoculated on a Petri plate before entering the88 vacuum plasma chamber. Treatments took a few minutes. Standard microbiological protocols were used to test the presence of microorganisms before and after plasma treatments. In ref. 89, the use of cold atmospheric plasmas (CAPs) to decontaminate FFP3 face masks during the COVID-19 pandemic has been explored aimed at the possibility of mask reusing. It investigates the effectiveness of the CAP in eliminating the bacteria by injecting the test germs into fabric samples. CAP treatment was effective for bacteria and spores. Referring to recycling, masks are not usually a mono-material, since in addition to a non-woven spun layer, elastic yarns (ear loop) and metal nose wires are needed for mask wearability. In addition, elastic yarns are usually composed of elastane filament (polyurethane) covered with another yarn (i.e., polyamide or others). Indeed, mask recycling through a closed-loop process through thermos-mechanical treatment (from waste masks to novel mask products) is currently quite complicated due to the presence of multiple materials; in contrast, open-loop recycling processes can be easily carried out. The possible solution is recycling used sanitary masks reutilizing them as reinforcement in construction materials.90 The three main parts of masks should be separated before recycling.91
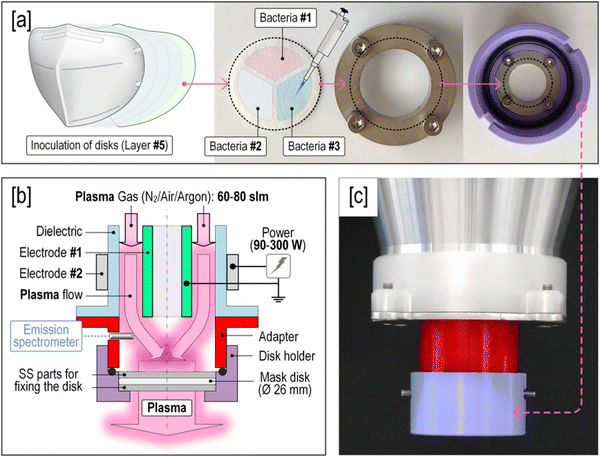 |
| Fig. 15 (a) Extracting the ∅ 26 mm disks from KN95 masks for inoculation with Staphylococcus aureus ATCC29213 and Escherichia coli. ATCC25922. (b) Sketch of atmospheric pressure cold plasma equipment. (c) Plasma apparatus designed for treating the inoculated mask disks.87 | |
6. Conclusions
In conclusion, the diverse requirements of medical protective masks, particularly in terms of special properties imparted (i.e., antibacterial or antiviral effect) and filtration efficiency, the last one achieved through particular chemical spinning techniques (i.e., electrospinning), have led to significant advancements in mask performance. While current mask functionalities have reached a high level of refinement, future research may focus on novel sterilization methodologies for reuse, which ensure the maintenance of the fibre-network geometry in order to avoid a decrease in filtration efficiency after sterilization. In addition, the development of degradable and recyclable materials that offer enhanced comfort and cost-effectiveness while aligning with green concepts through eco-design is a key objective since the end-of-life of masks is a highly concerning issue. Looking back at the evolution of mask development throughout history, it becomes evident that the demand acts as a driving force for innovation, leading to the emergence of novel solutions. Presently, the industry is increasingly discussing functional and smart fibres. Given the stringent demands of medical supplies, these high-performance fibres will likely be a primary area of exploration for scientists. It is noteworthy that many of these fibres are currently petroleum-based, but future trends will emphasize environmental sustainability, with the adoption of bio-based materials gaining prominence as a focal point of innovation as well as biodegradable masks that maintain high filtration efficiency over prolonged use.
Author contributions
All authors wrote the manuscript. H. M. and L. S. Y. conceived the review. C. R., R. M., and A. P. worked mainly on the technological issues. All authors read the manuscript and provided comments.
Data availability
There are no raw data on experiments related to this review paper.
Conflicts of interest
The authors declare no competing financial interest.
Acknowledgements
A part of this research was performed within the MUSA—Multilayered Urban Sustainability Action—project, funded by the European Union—NextGenerationEU, under the National Recovery and Resilience Plan (NRRP) Mission 4, Component 2, Investment Line 1.5: Strengthening of research structures and creation of R&D “innovation ecosystems”, set up of “territorial leaders in R&D”. This work was also funded by the National Plan for NRRP Complementary Investments (PNC, established with the decree-law 6 May 2021, no. 59, converted by law no. 101 of 2021) in the call for the funding of research initiatives for technologies and innovative trajectories in the health and care sectors (Directorial Decree no. 931 of 6 June 2022)—project no. PNC0000003—AdvaNced Technologies for Human-centrEd Medicine (project acronym: ANTHEM). This work reflected only the authors’ views and opinions, neither the Ministry for University and Research nor the European Commission can be considered responsible for them. This work was supported by the Research Fund for International Scientists (RFIS-52150410416), the National Natural Science Foundation of China, and the Research Startup grant of ZSTU (20202294-Y) and (22202327-Y). The authors would also like to acknowledge Dr. Anastasia Anceschi from CNR-STIIMA, Italian National Research Council for her invaluable assistance in creating the graphics.
References
- J. Militky, et al., A review of impact of textile research on protective face masks, Materials, 2021, 14(8), 1937 CrossRef CAS PubMed.
- J. F. Kennedy and C. J. Knill, Biomaterials utilised in medical textiles: An overview, Medical textiles and biomaterials for healthcare, 2006, pp. 3–22 Search PubMed.
- O. Baranov, et al., Recent innovations in the technology and applications of low-dimensional CuO nanostructures for sensing, energy and catalysis, Nanoscale Horiz., 2023, 8(5), 568–602, 10.1039/d2nh00546h.
- R. Maryam, et al., Enhanced photocatalytic and antibacterial activity of NiSe2-TiO2 nanocomposites under visible light, Opt. Mater., 2023, 143, 114183, DOI:10.1016/j.optmat.2023.114183.
- D. Alagarasan, A. Harikrishnan, M. Surendiran, K. Indira, A. S. Khalifa and B. H. Elesawy, Synthesis and characterization of CuO nanoparticles and evaluation of their bactericidal and fungicidal activities in cotton fabrics, Appl. Nanosci., 2021, 11, 1–10, DOI:10.1007/s13204-021-02054-5.
- O. B. Ahmed and T. Alamro, Evaluation of the antibacterial activities of face masks coated with titanium dioxide nanoparticles, Sci. Rep., 2022, 12(1), 18739, DOI:10.1038/s41598-022-23615-w.
- X. G. Zhang, et al., Graphene Oxide Modified Microtubular ZnO Antibacterial Agents for a Photocatalytic Filter in a Facial Mask, ACS Appl. Nano Mater., 2022, 5(11), 16332–16343, DOI:10.1021/acsanm.2c03371.
- J. M. Zhao, et al., Graphene Oxide-Based Antibacterial Cotton Fabrics, Adv. Healthcare Mater., 2013, 2(9), 1259–1266, DOI:10.1002/adhm.201200437.
- K. Zhang, et al., Biodegradable Smart Face Masks for Machine Learning-Assisted Chronic Respiratory Disease Diagnosis, ACS Sens., 2022, 7(10), 3135–3143, DOI:10.1021/acssensors.2c01628.
- M. Chan, D. Estève, J. Y. Fourniols, C. Escriba and E. Campo, Smart wearable systems: Current status and future challenges, Artif. Intell. Med., 2012, 56(3), 137–156, DOI:10.1016/j.artmed.2012.09.003.
- J. Hyysalo, et al., Smart mask - Wearable IoT solution for improved protection and personal health, Internet of Things, 2022, 18, 100511, DOI:10.1016/j.iot.2022.100511.
- T. M. Fagbola, F. I. Fagbola, O. J. Aroba, R. Doshi, K. K. Hiran and S. C. Thakur, Smart Face Masks for COVID-19 Pandemic Management: A Concise Review of Emerging Architectures, Challenges and Future Research Directions, IEEE Sens. J., 2023, 23(2), 877–888, DOI:10.1109/jsen.2022.3225067.
- V. U. S. Rao, G. Arakeri, G. Madikeri, A. Shah, R. S. Oeppen and P. A. Brennan, COVID-19 associated mucormycosis (CAM) in India: a formidable challenge, British J. Oral Maxillofac. Surg., 2021, 59(9), 1095–1098, DOI:10.1016/j.bjoms.2021.06.013.
- F. M. A. Badri, Surgical mask contact dermatitis and epidemiology of contact dermatitis in healthcare workers, Curr. Opin. Allergy Clin. Immunol., 2017, 30(3), 183–188 Search PubMed.
- M. Ahrari, M. Karahan, M. Hussain, Y. Nawab, A. Khan and A. A. J. T. Shirazi, Development of anti-bacterial and anti-viral nonwoven surgical masks for medical applications, Tekstilec, 2022, 65(2), 135–146 CrossRef CAS.
- D. K. Chu, et al., Physical distancing, face masks, and eye protection to prevent person-to-person transmission of SARS-CoV-2 and COVID-19: a systematic review and meta-analysis, Lancet, 2020, 395(10242), 1973–1987, DOI:10.1016/s0140-6736(20)31142-9.
- M. F. Sari, F. Esen and Y. Tasdemir, Investigation of polychlorinated biphenyls (PCBs) residues and retention rates in two different face masks used during the COVID-19 lockdown period, Hyg. Environ. Health Adv., 2022, 4, 100016, DOI:10.1016/j.heha.2022.100016.
- T. Zhou, W. Chengxue and H. Lijun, Clinical application scenarios of various types of masks during epidemic prevention and control, China Med. News, 2020, 002 Search PubMed.
- Q. Armand, et al., Impact of medical face mask wear on bacterial filtration efficiency and breathability, Environ. Technol. Innovation, 2022, 28, 102897 CrossRef.
- J. E. N. Khan, M. Mariatti, V. Vilay and M. Todo, A comprehensive review on facemask manufacturing,
testing, and its environmental impacts, J. Ind. Text., 2022, 52, 15280837221111175 Search PubMed.
- A. Tuñón-Molina, et al., Protective face masks: current status and future trends, ACS Appl. Mater. Interfaces, 2021, 13(48), 56725–56751 CrossRef.
- W. Wang, et al., Fabrication and application of superhydrophobic nonwovens: a review, Mater. Today Chem., 2022, 26, 101227, DOI:10.1016/j.mtchem.2022.101227.
- H. Li, M. Xu, R. Shi, A. Zhang and J. Zhang, Advances in Electrostatic Spinning of Polymer Fibers Functionalized with Metal-Based Nanocrystals and Biomedical Applications, Molecules, 2022, 27(17), 5548 CrossRef CAS PubMed.
- B. Baskapan and A. Callanan, Electrospinning Fabrication Methods to Incorporate Laminin in Polycaprolactone for Kidney Tissue Engineering, Tissue Eng. Regener. Med., 2021, 19(1), 73–82, DOI:10.1007/s13770-021-00398-1.
- H. Frizzell, T. J. Ohlsen and K. A. Woodrow, Protein-loaded emulsion electrospun fibers optimized for bioactivity retention and pH-controlled release for peroral delivery of biologic therapeutics, Int. J. Pharm., 2017, 533(1), 99–110, DOI:10.1016/j.ijpharm.2017.09.043.
- J. Wang and M. Windbergs, Controlled dual drug release by coaxial electrospun fibers – Impact of the core fluid on drug encapsulation and release, Int. J. Pharm., 2019, 556, 363–371, DOI:10.1016/j.ijpharm.2018.12.026.
- Y. Bai, et al., Washable Multilayer Triboelectric Air Filter for Efficient Particulate Matter PM2.5Removal, Adv. Funct. Mater., 2018, 28(15), 1706680, DOI:10.1002/adfm.201706680.
- W.-C. Lu, et al., Antibacterial Activity and Protection Efficiency of Polyvinyl Butyral Nanofibrous Membrane Containing Thymol Prepared through Vertical Electrospinning, Polymers, 2021, 13(7), 1122 CrossRef CAS PubMed.
- Y. Yang, et al., A Facile Method for the Fabrication of Silver Nanoparticles Surface Decorated Polyvinyl Alcohol Electrospun Nanofibers and Controllable Antibacterial Activities, Polymers, 2020, 12(11), 2486 CrossRef CAS PubMed.
- Y. Qian, X. Zhou, F. Zhang, T. G. H. Diekwisch, X. Luan and J. Yang, Triple PLGA/PCL Scaffold Modification Including Silver Impregnation, Collagen Coating, and Electrospinning Significantly Improve Biocompatibility, Antimicrobial, and Osteogenic Properties for Orofacial Tissue Regeneration, ACS Appl. Mater. Interfaces, 2019, 11(41), 37381–37396, DOI:10.1021/acsami.9b07053.
- S. Belhouideg and management, Impact of 3D printed medical equipment on the management of the Covid19 pandemic, Int. J. Health Plann. Manage., 2020, 35(5), 1014–1022 CrossRef PubMed.
- N. Kim, J. L. J. Wei, J. Ying, H. Zhang, S. K. Moon and J. Choi, A customized smart medical mask for healthcare personnel, in 2020 IEEE International Conference on Industrial Engineering and Engineering Management (IEEM), 2020: IEEE, 581–585.
- S. Bhattacharjee, P. Bahl, A. A. Chughtai, D. Heslop and C. R. J. N. S. MacIntyre, Face masks and respirators: Towards sustainable materials and technologies to overcome the shortcomings and challenges, Nano Select, 2022, 3(10), 1355–1381 CrossRef CAS.
- W. Deng, et al., Masks for COVID-19, Adv. Sci., 2022, 9(3), 2102189 CrossRef CAS PubMed.
- S. K. Chaudhary, N. Chaudhary, R. Chaudhary and N. K. J. P. M. S. Chaudhary, Review on benefits, toxicity, challenges, and future of graphene-based face masks in the prevention of COVID-19 pandemic, PeerJ Mater. Sci., 2022, 4, e20 CrossRef.
- L. Huang, et al., Self-reporting and photothermally enhanced rapid bacterial killing on a laser-induced graphene mask, ACS Nano, 2020, 14(9), 12045–12053 CrossRef CAS PubMed.
- L. Russo, S. Zanini, C. Riccardi, F. Nicotra and L. J. M. T. Cipolla, Diazo transfer for azido-functional surfaces, Mater. Today, 2011, 14(4), 164–169 CrossRef CAS.
- S. Zanini, et al., Plasma-induced graft-polymerization of polyethylene glycol acrylate on polypropylene films: Chemical characterization and evaluation of the protein adsorption, J. Colloid Interface Sci., 2010, 341(1), 53–58 CrossRef CAS.
- S. Zanini, L. Zoia, R. Della Pergola, C. J. S. Riccardi and C. Technology, Pulsed plasma-polymerized 2-isopropenyl-2-oxazoline coatings: Chemical characterization and reactivity studies, Surf. Coat. Technol., 2018, 334, 173–181 CrossRef CAS.
- E. Dell’Orto, S. Caldirola, A. Sassella, V. Morandi and C. J. A. S. S. Riccardi, Growth and properties of nanostructured titanium dioxide deposited by supersonic plasma jet deposition, Appl. Surf. Sci., 2017, 425, 407–415 CrossRef.
- C. Piferi, et al., Hydrophilicity and Hydrophobicity Control of Plasma-Treated Surfaces via Fractal Parameters, Adv. Mater. Interfaces, 2021, 8(19), 2100724 CrossRef CAS.
- E. Dell'Orto, A. Vaccaro and C. Riccardi, Morphological and chemical analysis of PP film treated by Dielectric Barrier Discharge, J. Phys.: Conf. Ser., 2014, 550(1), 012032 CrossRef.
- M. Prado, et al., Hexamethyldisiloxane coating by plasma to create a superhydrophobic surface for fabric masks, J. Mater. Res. Technol., 2022, 17, 913–924 CrossRef CAS.
- F. Cicogna, et al., Surface Functionalization of Face Masks with Cold Plasma and Its Effect in Anchoring Polyphenols Extracted from Agri-Food, Molecules, 2022, 27(23), 8632 CrossRef CAS.
- S. G. Gizer, V. R. Bhethanabotla, R. S. Ayyala and N. J. S. Sahiner, Low-pressure plasma treated polycarbonate and polymethyl methacrylate (PMMA) sheets with different surface patterns to change their surface properties, Surf. Interfaces, 2023, 37, 102646 CrossRef CAS.
- H. E. Roman, F. Cesura, R. Maryam, I. Levchenko, K. Alexander and C. Riccardi, The fractal geometry of polymeric materials surfaces: surface area and fractal length scales, Soft Matter, 2024, 20(14), 3082–3096, 10.1039/d3sm01497e.
- A. Azanova, R. Y. Galimzyanova, L. Abutalipova and L. Khisamiyeva, Plasma modification physical and mechanical properties of nonwoven materials of medical masks, J. Phys.: Conf. Ser., 2022, 2379(1), 012005 CrossRef CAS.
- A. Y. Starikovskiy and D. R. Usmanova, Medical mask with plasma sterilizing layer, arXiv, 2020, preprint, arXiv:2004.00807 DOI:10.48550/arXiv.2004.00807.
- A. Żywicka, et al., Argon plasma-modified bacterial cellulose filters for protection against respiratory pathogens, Carbohydr. Polym., 2023, 302, 120322 CrossRef PubMed.
- G. Kaur, et al., Face mask recognition system using CNN model, Neurosci. Inform., 2022, 2(3), 100035 CrossRef.
- S. Sen and K. Sawant, Face mask detection for covid_19 pandemic using pytorch in deep learning, IOP Conf. Ser.: Mater. Sci. Eng., 2021, 1070(1), 012061 Search PubMed.
- G. Yang et al., Face mask recognition system with YOLOV5 based on image recognition, in 2020 IEEE 6th International Conference on Computer and Communications (ICCC), 2020: IEEE, 1398–1404.
- L. Du, et al., High performance anti-smog window screens via electrospun nanofibers, J. Appl. Polym. Sci., 2020, 137(19) DOI:10.1002/app.48657.
- J. Liang, et al., Pump-inject antimicrobial and biodegradable aerogel as mask intermediate filter layer for medical protection of air filtration, Mater. Today Sustainability, 2022, 19, 100211, DOI:10.1016/j.mtsust.2022.100211.
- L. Qianyu and C. Ting, Research status of comfort of medical protective masks, Wool spinning technology, 50, 102–112, 2022 Search PubMed.
- W. Mengmeng Sally, L. Yan, L. Xinyue, T. Suyao and S. Wei, Application of qualitative suitability test for medical protective masks in clinical staff, Chin. J. Infect. Control, 2023, 22, 110–116 Search PubMed.
- R. Seibt, M. Bär, M. A. Rieger and B. Steinhilber, Limitations in evaluating COVID-19 protective face masks using open circuit spirometry systems: respiratory measurement mask introduces bias in breathing pressure and perceived respiratory effort, Physiol. Meas., 2023, 44(1), 015001, DOI:10.1088/1361-6579/aca7ab.
- S. Driver, et al., Effects of wearing a cloth face mask on performance, physiological and perceptual responses during a graded treadmill running exercise test, Br. J. Sports Med., 2022, 56(2), 107–113, DOI:10.1136/bjsports-2020-103758.
- S. Fikenzer, et al., Effects of surgical and FFP2/N95 face masks on cardiopulmonary exercise capacity, Clin. Res. Cardiol., 2020, 109(12), 1522–1530, DOI:10.1007/s00392-020-01704-y.
- C. Georgi, A. Haase-Fielitz, D. Meretz, L. Gäsert and C. Butter, The Impact of Commonly-Worn Face Masks on Physiological Parameters and on Discomfort During Standard Work-Related Physical
Effort, Dtsch. Ärztebl. Int., 2020, 117(40), 674–675, DOI:10.3238/arztebl.2020.0674.
- D. J. Ramos-Campo, S. Pérez-Piñero, J. C. Muñoz-Carrillo, F. J. López-Román, E. García-Sánchez and V. Ávila-Gandía, Acute Effects of Surgical and FFP2 Face Masks on Physiological Responses and Strength Performance in Persons with Sarcopenia, Biology, 2021, 10(3), 213 CrossRef PubMed.
- B. Steinhilber, et al., Effects of Face Masks on Physical Performance and Physiological Response during a Submaximal Bicycle Ergometer Test, Int. J. Environ. Res. Public Health, 2022, 19(3), 1063 CrossRef CAS PubMed.
- L. Du, Y. Zhang, X. Li, J. Wang, M. Chen, X. Zuo, W. Yang, M. Yousefzadeh, S. Ramakrishana and H. Li, High performance anti-smog window screens via electrospun nanofibers, J. Appl. Polym. Sci., 2020, 137(19) DOI:10.1002/app.48657.
- S. Zhang, H. Liu, N. Tang, N. Ali, J. Yu and B. Ding, Highly Efficient, Transparent, and Multifunctional Air Filters Using Self-Assembled 2D Nanoarchitectured Fibrous Networks, ACS Nano, 2019, 13(11), 13501–13512, DOI:10.1021/acsnano.9b07293.
- A. Konda, A. Prakash, G. A. Moss, M. Schmoldt, G. D. Grant and S. Guha, Aerosol filtration efficiency of common fabrics used in respiratory cloth masks, ACS Nano, 2020, 14(5), 6339–6347 CrossRef CAS.
- A. Tcharkhtchi, N. Abbasnezhad, M. Z. Seydani, N. Zirak, S. Farzaneh and M. Shirinbayan, An overview of filtration efficiency through the masks: Mechanisms of the aerosols penetration, Bioactive Mater., 2021, 6(1), 106–122 CrossRef CAS.
- C. M. Clase, et al., Forgotten technology in the COVID-19 pandemic: filtration properties of cloth and cloth masks—a narrative review, Mayo Clin. Proc., 2020, 95(10), 2204–2224 CrossRef CAS.
- A. Budimir, S. Bischof Vukusic and S. Grgac Flincec, Study of antimicrobial properties of cotton medical textiles treated with citric acid and dried/cured by microwaves, Cellulose, 2012, 19(1), 289–296, DOI:10.1007/s10570-011-9614-z.
- Z. Zhao, et al., Multi-functional Calotropis gigantea fabric using self-assembly silk fibroin, chitosan and nano-silver microspheres with oxygen low-temperature plasma treatment, Colloids Surf., B, 2022, 215, 112488, DOI:10.1016/j.colsurfb.2022.112488.
- H. R. Pant, et al., Electrospun nylon-6 spider-net like nanofiber mat containing TiO2 nanoparticles: A multifunctional nanocomposite textile material, J. Hazard. Mater., 2011, 185(1), 124–130, DOI:10.1016/j.jhazmat.2010.09.006.
- T. V. Toniatto, et al., Nanostructured poly (lactic acid) electrospun fiber with high loadings of TiO2 nanoparticles: Insights into bactericidal activity and cell viability, Mater. Sci. Eng., C, 2017, 71, 381–385, DOI:10.1016/j.msec.2016.10.026.
- S. S. Athukoralalage, C. A. Bell, A. C. Gemmell, A. E. Rowan and N. Amiralian, Recent advances and future perspectives in engineering biodegradable face masks, J. Mater. Chem. A, 2023, 11(4), 1575–1592, 10.1039/D2TA08019B.
- Y. Karasawa, M. Uemae, M. Kamijo and IEEE, Investigation of factors for evaluating skin touch comfort sensation of cloth used for cosmetic face masks, International Conference on Biometrics and Kansei Engineering (ICBAKE), Kyoto, JAPAN, 2017, 18–22.
- M. Suekawa, Y. Hashizume, S. Tanoue, H. Uematsu and Y. Yamashita, Infection Prevention Mask Consisting of Nanofiber Filter and Habutae Silk Fabrics, Materials, 2021, 14(23), 7391, DOI:10.3390/ma14237391.
- G. R. Teesing, B. van Straten, P. de Man and T. Horeman-Franse, Is there an adequate alternative to commercially manufactured face masks? A comparison of various materials and forms, J. Hosp. Infect., 2020, 106(2), 246–253, DOI:10.1016/j.jhin.2020.07.024.
- Z. Wang, Y. Xu, R. Liu and X. J. P. Zhu, The 3D-Printing-Accelerated Design for a Biodegradable Respirator from Tree Leaves (TRespirator), Polymers, 2022, 14(9), 1681 CrossRef CAS.
- R. Shen, Y. Guo, S. Wang, A. Tuerxun, J. He and Y. Bian, Biodegradable Electrospun Nanofiber Membranes as Promising Candidates for the Development of Face Masks, Int. J. Environ. Res. Public Health, 2023, 20(2), 1306 CrossRef CAS PubMed.
- Y. Bian, C. Zhang, H. Wang and Q. Cao, Degradable nanofiber for eco-friendly air filtration: Progress and perspectives, Sep. Purif. Technol., 2023, 306, 122642, DOI:10.1016/j.seppur.2022.122642.
- J. Jalvandi, M. White, Y. Gao, Y. B. Truong, R. Padhye and I. L. Kyratzis, Polyvinyl alcohol composite nanofibres containing conjugated levofloxacin-chitosan for controlled drug release, Mater. Sci. Eng., C, 2017, 73, 440–446, DOI:10.1016/j.msec.2016.12.112.
- Y. B. Truong, V. Glattauer, K. L. Briggs, S. Zappe and J. A. M. Ramshaw, Collagen-based layer-by-layer coating on electrospun polymer scaffolds, Biomaterials, 2012, 33(36), 9198–9204, DOI:10.1016/j.biomaterials.2012.09.012.
- S. Sinha-Ray, Y. Zhang, A. L. Yarin, S. C. Davis and B. Pourdeyhimi, Solution Blowing of Soy Protein Fibers, Biomacromolecules, 2011, 12(6), 2357–2363, DOI:10.1021/bm200438v.
- M. Iyer, et al., Environmental survival of SARS-CoV-2–a solid waste perspective, Environ. Res., 2021, 197, 111015 CrossRef CAS PubMed.
- O. O. Fadare and E. D. Okoffo, Covid-19 face masks: A potential source of microplastic fibers in the environment, Sci. Total Environ., 2020, 737, 140279 CrossRef CAS PubMed.
- A. Richter, K. T. W. Ng, H. L. Vu and G. J. W. M. Kabir, Waste disposal characteristics and data variability in a mid-sized Canadian city during COVID-19, Waste Manage., 2021, 122, 49–54 CrossRef CAS.
- M. Ganesapillai, et al., The face behind the Covid-19 mask-A comprehensive review, Environ. Technol. Innovation, 2022, 102837 CrossRef CAS.
- A. Hicks, S. Temizel-Sekeryan, W. Kontar, R. Ghamkhar and M. R. J. R. Morris, Conservation, and Recycling, Personal respiratory protection and resiliency in a pandemic, the evolving disposable versus reusable debate and its effect on waste generation, Conserv. Recycl., 2021, 168, 105262 CrossRef CAS.
- A. Sainz-García, et al., Mask disinfection using atmospheric pressure cold plasma, Int. J. Infect. Dis., 2022, 123, 145–156 CrossRef.
- Y.-W. Cheng, Y.-T. Lin, K.-H. Liu, J.-S. Chen, S.-H. Wang and T.-Y. Liu, In situ and initiator-free atmospheric plasma-induced functionalization of poly (ethylene glycol) methacrylate on nonwoven cosmetic masks for the evaluation of the bacteria inhibitory effect, Colloids Surf., A, 2022, 642, 128719 CrossRef CAS.
- A. Schmidt, C.-Y. T. Tschang, J. Sann, M. H. Thoma and P. M. Sciences, Cold Atmospheric Plasma Decontamination of FFP3 Face Masks and Long-Term Material Effects, IEEE Trans. Radiat. Plasma Med. Sci., 2021, 6(4), 493–502 Search PubMed.
- K. Selvaranjan, S. Navaratnam, P. Rajeev and N. J. E. C. Ravintherakumaran, Environmental challenges induced by extensive use of face masks during COVID-19: A review and potential solutions, Environ. Challenges, 2021, 3, 100039 CrossRef CAS.
- D. Battegazzore, F. Cravero and A. J. P. Frache, Is it possible to mechanical recycle the materials of the disposable filtering masks?, Polymers, 2020, 12(11), 2726 CrossRef CAS PubMed.
|
This journal is © The Royal Society of Chemistry 2024 |