Effects of carbon diversion to primary sludge production on thermal hydrolysis pretreatment-enhanced anaerobic digestion†
Received
21st September 2023
, Accepted 16th January 2024
First published on 17th January 2024
Abstract
Redirecting wastewater organic carbon to anaerobic digesters through primary sludge (PS) production from aeration tanks has been pursued as a viable means for achieving energy neutrality in water resource recovery facilities (WRRFs). A comprehensive evaluation of the approach was investigated in this study by taking into account the solid reduction, energy recovery and savings, sludge dewaterability, and recalcitrant dissolved organic nitrogen (rDON) formation when thermal hydrolysis pretreatment (THP) was used. Experimental results revealed that increasing the PS-to-waste activated sludge (WAS) ratio from 0
:
1 to 1
:
1 and 3
:
1 through chemically enhanced primary treatment (CEPT) led to residual solids increase of 9% and 16%, aeration energy savings of 35% and 60%, and energy recovery increase of 67% and 120%, respectively. Very importantly, this study reported for the first time that blending PS and WAS led to excessive rDON formation during THP. Fortunately, high Al3+ doses used during CEPT precipitated most of the rDON along with orthophosphate removal, which also led to sludge dewaterability improvement. Thereby, a major drawback of carbon diversion is the extra biosolid production plus increased chemical use in comparison with the scenario without diversion. Since the primary responsibility of WRRFs is discharge water quality control and solid reduction, it was concluded that carbon diversion may not fit all WRRFs and should be considered on a case-by-case basis with an overall evaluation of its gains.
Water impact
It is the intention of this study to inform the wastewater industry of not only the benefits but also the hidden pitfalls of carbon diversion to primary sludge production with first-hand experimental data. The findings from this study would be interesting to researchers and engineers who are considering the adoption of carbon diversion technologies in water resource recovery facilities.
|
1. Introduction
Public wastewater treatment consumes 3–4% energy production annually and contributes 1% of the greenhouse gas emission in the U.S.1,2 Meanwhile, the chemical energy contained in wastewater was estimated to be 9.3 times the energy it takes to treat it.3 Therefore, harvesting wastewater energy has been proposed as a viable means for achieving energy neutral wastewater treatment. Redirecting wastewater organic carbon as much as possible to anaerobic digesters (ADs) through primary sludge (PS) production in lieu of aeration tanks has been pursued as a pioneer approach for energy recovery.4,5 This is opposite to the design of many water resource recovery facilities (WRRFs) built during the first half of the 1900s when the primary focus was on water quality rather than energy management.6 As a consequence, many of these WRRFs are not even equipped with primary clarifiers and/or ADs. Minimizing total biosolid production through carbon conversion to CO2 in aeration tanks has been a common design, making aeration a major contributor to energy consumption and greenhouse gas emission in WRRFs.5 That being said, redirecting influent organic carbon to PS not only holds promise to increase energy recovery in ADs but also decreases electrical energy requirements for aeration, and in turn expands the WRRF capacity by reducing the organic loading upstream of the secondary process.7
Various primary treatment technologies have been implemented for PS production. These include chemically or biologically enhanced sedimentation or mechanical screening and filtration. Among these technologies, chemically enhanced primary treatment (CEPT) is the most popular as it achieves the greatest total suspended solids capture efficiency (60–90%),6 is easy and economical to implement, and removes phosphorus and dissolved heavy metal as well as many other micropollutants.8
Every coin has two sides, and PS production is not an exception. Although well-established jar test methods have been developed to select the coagulant and dosage for a desired amount of PS production,5 popular coagulants such as alum (Al2(SO4)3) are known to reduce the sludge biodegradability through their binding of organics. This is especially true at the high dose used for high PS production.5 Although PS possesses much better biodegradability than waste activated sludge (WAS), alum dosed into PS may play down the overall biodegradability of the blended sludge.9 Moreover, diverting influent organics into PS is bound to increase the solid loading of ADs. In recent years, full-scale thermal hydrolysis pretreatment (THP) has been broadly applied to improve sludge digestibility and augment the capacity of ADs by increasing anaerobic digestion rates.10 The chemical bonds between coagulants and organics are also expected to be broken by THP.9 While addressing these PS production-caused limitations, THP also brings side effects, one of which is the formation of recalcitrant dissolved organic nitrogen (rDON).11 It has been recognized that rDON formed during THP is mainly from the Maillard reaction between the carbonyl groups of reducing sugar and the amino groups of proteins.11,12 This is because the typical THP conditions, e.g., 165 °C for 30 min, overlap with that of the Maillard reaction. More importantly, PS and WAS are mainly composed of carbohydrates and proteins, which are the precursors of carbonyl groups and amino groups, respectively. Therefore, blending PS with WAS as commonly practiced in WRRFs provides an ideal recipe for the Maillard reaction and in turn rDON production in THP.13 rDON is responsible for total nitrogen (TN) increase in the discharge, biological nitrogen removal inhibition, and UV transmission blockage.14,15
Therefore, the objective of this research is to experimentally examine the pros and cons of carbon diversion to PS in terms of overall energy recovery, solid reduction, and sludge dewaterability as well as the removal of dissolved compounds such as rDON. These important aspects were compared in parallel in three scenarios, i.e., (i) WAS only without PS production; (ii) PS production to 1
:
1 dry mass ratio with WAS; and (iii) PS production to 3
:
1 dry mass ratio with WAS. Additional control experiments were also added to assist in the understanding of the mechanisms behind. THP was adopted in all three scenarios to reflect the state of the art of the sewage sludge anaerobic digestion enhancement. The coagulant dose-dependent mass balance between PS and WAS production was also taken into consideration. Thereby, the pros and cons of each scenario were analyzed. It is the intention of this study to inform the wastewater industry of not only the benefits but also the hidden pitfalls of carbon diversion with first-hand experimental data. The findings from this study would be interesting to researchers and engineers who are considering the adoption of carbon diversion technologies in WRRFs.
2. Materials and methods
2.1. Sludge sampling and blending
Raw PS collected from a local WRRF was dewatered using a pilot dewatering unit (ES-051, PWTECH, Rosedale, MD). The coagulant dosing was performed during this pilot PS dewatering by mixing alum solution (50% purity) with raw PS (2% TS). To adjust to the exact Al3+ doses targeted in this study, extra coagulant solution was also added into the dewatered PS prior to the THP. The final Al3+ contents measured in PS and WAS fed into THP are summarized in Table 1.
Table 1 Summary of PS-to-WAS blending ratios and Al3+ doses used in each experimental group
Role |
Group |
Dry mass ratio |
Individual Al3+ content (%) |
Total Al3+ content (%) |
PS |
WAS |
PS |
WAS |
Scenario 1 |
WAS only |
0 |
1 |
N/A |
1.7 |
1.7 |
Scenario 2 |
PS : WAS = 1 : 1 with low Al3+ dose |
1 |
1 |
2.2 |
1.7 |
1.95 |
Scenario 3 |
PS : WAS = 3 : 1 with high Al3+ dose |
3 |
1 |
3.23 |
1.7 |
2.85 |
Blank |
PS : WAS = 1 : 1 with no Al3+ dose |
1 |
1 |
1.28 |
1.7 |
1.49 |
Control |
PS : WAS = 1 : 1 with high Al3+ dose |
1 |
1 |
4 |
1.7 |
2.85 |
Control |
PS only |
1 |
0 |
1.28 |
N/A |
1.28 |
After a 90-day AD startup, alum was dosed as a coagulant into the PS. Referring to results in Fig. S1a–d,† as soon as the alum was dosed, the digester performance quickly crashed with a dramatic pH drop, volatile fatty acid (VFA) accumulation, soluble chemical oxygen demand (sCOD) increase, and biogas production cease. A closer look into the biogas composition revealed that its H2S content had reached 35
000 ppm (Fig. S1e†), indicating that the sulfate ions in the alum have been reduced to H2S in the ADs and in turn crashed the digester performance. It should be realized that the sulfate ions are usually dissolved in and discharged with mainstream water and do not end up in the side stream system as observed in Fig. S1.† To rectify this artifact, from day 200 and onward, aluminum chloride (AlCl3) (30% purity, USALCO. LLC, Baltimore, MD) was dosed as a coagulant to avoid H2S formation and inhibition (Fig. S1†).
Dewatered WAS with total solids (TS) of around 20% was collected from another local WRRF. The PS and WAS cakes came with 1.28% and 1.7% (g Al3+ per g TS) background Al3+ contents. Thereby, a group of WAS-only samples with 1.7% background Al3+ content was used to represent scenario 1 when no PS was produced in WRRFs (Table 1). In order to predict the other two scenarios, a BioWin model (Envirosim Associates Ltd, Hamilton, Canada) was run in concert with jar tests to estimate the alum-dependent PS and WAS production through mass balance. Briefly, jar tests predicted the correlation between alum doses and PS production. The BioWin model predicted the WAS reduction as a result of PS production. Integration of the two predicted results showed that dosing external AlCl3 into PS to 2.2% and 3.23% Al3+ contents including background levels is required to produce PS with dry mass ratios to WAS of 1
:
1 and 3
:
1, respectively (Table 1). Thereby, we named these two spikes as ‘low’ and ‘high’ Al3+ doses, respectively. Collectively, they gave total Al3+ contents of 1.95% and 2.85% in sludges blended with PS-to-WAS dry mass ratios of 1
:
1 and 3
:
1, respectively (Table 1). These two groups represent the low and high PS production scenarios for the two different extents of carbon diversion, namely scenarios 2 and 3 in Table 1. Besides the three scenarios, a group of blanks without Al3+ dosing and a group of controls with high Al3+ dosing were added in Table 1 to assist in understanding the effect of Al3+ dosing when PS-to-WAS dry mass ratios were fixed at 1
:
1. Meanwhile, two high Al3+ dose groups with PS-to-WAS dry mass ratios of 1
:
1 and 3
:
1 can also be parallelly compared to help understand the effect of the blending ratios (Table 1). In the end, a PS-only group was also added in concert with the WAS-only group as a control as shown in Table 1 to help check the effect of sludge blending.
2.2. Pilot THP–AD system setup
A flow chart of the THP–AD system operation procedures is shown in Fig. 1. In short, dewatered PS and WAS cakes were blended and diluted with deionized (DI) water from 20% TS to 16% TS prior to being fed into a pilot scale THP facility (CAMBI™, Norway) heated with in situ generated steam as shown in Fig. 2a. This THP facility was operated at 165 °C and 6 bar for 30 min. Afterwards, the treated sludge was ejected into a flash tank where it was cooled to room temperature. The steam addition into the THP lowered the sludge TS to 12.5% in the THP effluent which was further diluted with DI water to 9% prior to being fed into mesophilic ADs. Six units of mechanically mixed ADs, each with 5 L working volume and 10 L headspace as shown in Fig. 2b, were operated in parallel with the temperature maintained at 36.5 ± 0.3 °C. These ADs were inoculated with fresh effluent sludge from a full-scale THP-fed mesophilic AD. The 9% TS sludge was fed into each AD on a daily basis. The SRTs of these six digesters were controlled at 15 days.
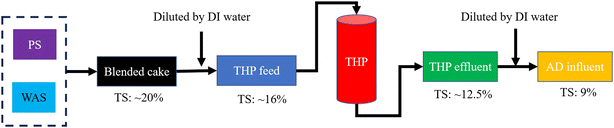 |
| Fig. 1 THP–AD system operation flow chart. | |
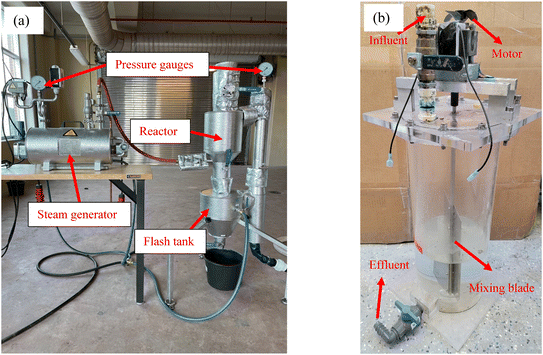 |
| Fig. 2 (a) Pilot-scale THP setup; (b) mechanically mixed AD setup. | |
2.3. Chemical analysis
The concentrations of chemical oxygen demand (COD), TN, total Kjeldahl nitrogen (TKN), total ammonia nitrogen (TAN), and orthophosphate (OP) were measured using Hach test kits of TNT 822, TNT 880, TNT 832, and TNT 845, respectively (Hach, Loveland, CO). Samples for soluble substance measurement including sCOD, soluble TKN (sTKN), TAN, and OP were filtered through 0.45 μm nylon filters (Aura Industries, New York, NY). Meanwhile, pH was measured with a benchtop pH meter (AE150, Fisher Scientific, Hampton, NH). TS and volatile solids (VS) were measured according to standard methods.16 In addition, the biogas production rate was monitored in real time using calibrated tipping-bucket meters (Archae Press, Nashville, USA) coupled with automatic data loggers (MicroDAQ, Contoocook, NH). Moreover, the CH4 content in biogas was measured using a gas chromatograph equipped with a thermal conductivity detector (GC-2014, Shimadzu, Columbia, MD). The dewaterability of the AD effluent was evaluated by capillary suction time (CST) measured with a capillary suction timer (294-50, Ofite, TX, USA).
2.4. rDON quantification
As defined in our previous studies,12 rDON can be regarded as dissolved organic nitrogen (DON) that can survive aerobic treatment commonly used in the secondary wastewater treatment process. Therefore, centrate was collected from the AD digestate and then exposed to fresh activated sludge for aerobic degradation until all ammonia had been fully nitrified and the DON level did not decrease anymore, which was regarded as the level of rDON. To obtain the centrate, 300 ml of the AD effluent sample was mixed with 50 ml of polymer composed of 40% active acrylamide (4110B, Univar Solutions, Downers Grove, IL) in a commercial blender. Then, the mixture was pre-dewatered using a lab centrifuge at 5000g for 20 min, after which the centrate was collected for rDON quantification. Because the TAN concentration in the centrate was significantly greater than that of DON, the background TAN level has to be reduced to achieve an accurate DON reading because DON is calculated as the difference between sTKN and TAN. To this end, an air stripping method was used to remove TAN prior to the aerobic treatment. Briefly, the pH of the centrate was adjusted to 11 with 0.1 N NaOH. Then, an air flow was introduced into the centrate at a rate of 2.7 L min−1 for air stripping (Fig. 3a). The TAN concentration was monitored daily until its level was below 200 mg L−1. After that, fresh activated sludge collected from the aeration basin at a local WRRF was collected and thickened using a lab centrifuge at 2000g for 10 min. 10 g of the thickened activated sludge was inoculated as the seed into 200 ml centrate that has TAN stripped, pH adjusted to 7.5, and concentration diluted two times to avoid inhibition based on previous experimental experience. Then, an air flow at a rate of 2.7 L min−1 was introduced into the mixed liquor through an aeration stone to provide dissolved oxygen and mixing (Fig. 3b). During this aerobic treatment, the pH of the mixed liquor was monitored and lowered to 7.5 on a daily basis by adding 0.1 N H2SO4. Meanwhile, the TAN, NO3−, and DON levels were monitored until all TAN was oxidized to NO3−, and DON reached a constant minimum level. A seed-only group with centrate replaced by DI water was used as a control to deduct the DON contribution from the seed sludge. All experimental groups were duplicated to check data reproducibility.
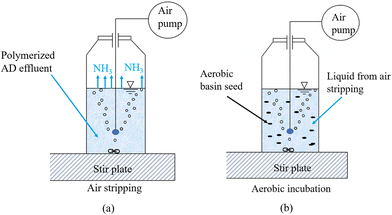 |
| Fig. 3 rDON test experimental design: (a) air stripping to remove TAN; (b) aerobic treatment to remove biodegradable DON. | |
2.5. Data analysis
A t-test was performed to assess whether statistical difference can be found in the daily biogas production rates as a means to determine whether steady states have been reached. At steady state during days 280 and 295 in Fig. S1c,† sample was collected from each AD effluent twice a week and analyzed in duplicate groups. The average values of all steady-state data were presented with mean ± standard errors in all figures and tables. The effect of steam dilution on concentration changes before and after THP was taken into calculation by using sludge inorganic matter as an internal standard, i.e., the inorganic matter concentration change before and after THP can be used to calculate a dilution factor for their conservative nature.
For solid mass flow analysis in the scenarios with and without primary settling, the following assumptions were made: (1) influent TS contains 80% VS; (2) aerobic growth yield is 0.5 g g−1,32 which means half of the VS removed in the aeration tank was turned into cell biomass through anabolism, and the other half (ΔVS) was oxidized by aeration to carbon dioxide through catabolism; (3) PS
:
WAS production ratios of 0
:
1, 1
:
1, and 3
:
1 on a dry mass basis were assumed according to the three scenarios specified in Table 1; (4) the percent of aeration electricity reduction is proportional to the percent of VS reduction in the aeration tank influent; (5) the VSR values obtained from the aforementioned AD study were adopted for each carbon diversion scenario.
3. Results
3.1. Effects of blending ratios and Al3+ dosing on THP performance
The pre- and post-THP total COD (tCOD), sCOD, TKN, TAN, and DON concentrations were measured to understand the effects of THP on sludge solubilization. As shown in Table 2, tCOD remained almost the same before and after THP for all groups. Similarly, TKN consisting of both protein and ammonia nitrogen remained unchanged during THP. PS exhibited higher tCOD but lower TKN than WAS because PS is known to contain higher carbon and lower nitrogen than WAS.17 Significant amounts of sCOD and soluble nitrogen including TAN and DON were released during THP as a result of high temperature and pressure for all groups. Consistently, more TAN and DON were released from WAS than from PS after THP as a result of hydrolysis during THP.10 Consequently, the sludges with higher PS-to-WAS blending ratios tended to produce lower TKN, TAN, and DON concentrations for the lower nitrogen content in PS. Very importantly, higher Al3+ doses led to lower sCOD, TAN, and DON levels in the THP effluent probably due to precipitation (Table 2).
Table 2 The pre- and post-THP sludge characteristics
Groups |
tCOD (mg L−1) |
sCOD (mg L−1) |
TKN (mg L−1) |
TAN (mg L−1) |
DON (mg L−1) |
Pre |
Post |
Pre |
Post |
Pre |
Post |
Pre |
Post |
Pre |
Post |
PS only |
157 060 ± 9861 |
151 100 ± 6555 |
147 ± 22 |
18 285 ± 399 |
4324 ± 179 |
4478 ± 221 |
7 ± 1 |
308 ± 14 |
35 ± 2 |
1006 ± 21 |
WAS only |
140 481 ± 5321 |
136 500 ± 4214 |
142 ± 4 |
28 774 ± 694 |
7529 ± 165 |
7248 ± 197 |
15 ± 0.2 |
649 ± 17 |
46 ± 7 |
1863 ± 101 |
PS : WAS = 1 : 1 with no Al3+ |
166 757 ± 8689 |
154 000 ± 9500 |
173 ± 16 |
24 304 ± 260 |
6535 ± 198 |
6220 ± 52 |
11 ± 0.5 |
427 ± 21 |
34 ± 6 |
1550 ± 35 |
PS : WAS = 1 : 1 with low Al3+ |
154 364 ± 6416 |
149 200 ± 1565 |
58 ± 14 |
24 750 ± 525 |
6445 ± 114 |
6730 ± 64 |
9 ± 0.3 |
383 ± 6 |
34 ± 6 |
1610 ± 30 |
PS : WAS = 1 : 1 with high Al3+ |
160 560 ± 5121 |
151 600 ± 5698 |
70 ± 10 |
15 237 ± 588 |
5335 ± 105 |
5074 ± 142 |
14 ± 0.1 |
318 ± 25 |
30 ± 5 |
1170 ± 117 |
PS : WAS = 3 : 1 with high Al3+ |
154 364 ± 6932 |
149 200 ± 5748 |
52 ± 6 |
12 800 ± 125 |
5290 ± 102 |
4645 ± 111 |
12 ± 0.2 |
396 ± 44 |
32 ± 4 |
1092 ± 35 |
3.2. Effects of blending ratios and Al3+ dosing on volatile solid reduction and CH4 yield
Volatile solid reduction (VSR) and CH4 yield are two key parameters indicating the THP–AD performance as they represent the solid reduction and energy production efficiencies, respectively. As can be seen in Fig. 4a, PS exhibited superior VSR and CH4 yield to WAS, which is expected. For the sludge blended with PS and WAS at a dry mass ratio of 1
:
1, the groups without Al3+ dosing and with low Al3+ dosing exhibited similar VSR and CH4 yield, indicating that a low Al3+ dose, as shown in Table 1, did not affect anaerobic digestion (Fig. 4a). Hence, it can be concluded that the Al3+ dose required to produce PS with a 1
:
1 dry mass ratio to WAS does not affect the AD performance. In contrast, increasing the Al3+ dose from low to high dramatically decreased VSR and CH4 yield from 45% to 32% and from 0.26 to 0.17 L g−1 VS fed, respectively (Fig. 4a). Interestingly, increasing the PS-to-WAS blending ratio from 1
:
1 to 3
:
1 mitigated this negative effect of high Al3+ dosing (Fig. 4a).
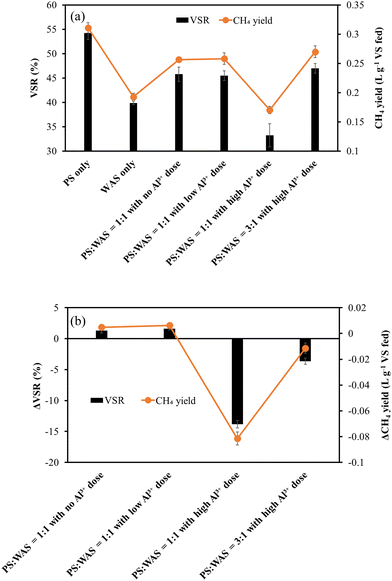 |
| Fig. 4 (a) VSR and CH4 yield of all experimental groups; (b) ΔVSR and ΔCH4 yield of four blended groups. | |
To evaluate the sludge blending effect, results from PS-only and WAS-only groups were used as baselines to calculate the VSR and CH4 yield values of blended sludge without blending and Al3+ dosing effects. Then, the difference between the baselines and actual experimental values are calculated as ΔVSR and ΔCH4 yield, which reflect the effects of blending and Al3+ dosing and are plotted in Fig. 4b. As can be seen, high Al3+ doses did negatively impact both ΔVSR and ΔCH4 yield (Fig. 4b). At a 1
:
1 blending ratio, compared to the neglectable values of the ΔVSR and ΔCH4 yield in the two groups with and without a low Al3+ dose, a high Al3+ dose can decrease the ΔVSR and ΔCH4 yield to −13% and −0.07 L g−1 VS fed, respectively (Fig. 4b). By increasing this blending ratio to 3
:
1, ΔVSR and ΔCH4 losses can be mitigated to −7% and −0.02 L g−1 VS fed, respectively. This is probably because Al3+ had less effect on PS than on WAS. As a matter of fact, the chemical binding between Al3+ and proteins rich in WAS has been recognized as a primary contributor to the adverse effects of Al3+ on sludge digestibility.9
3.3. Effects of blending ratios and Al3+ dosing on sludge dewaterability and OP levels
Dewaterability is an important digestate characteristic because it determines the final cake dryness for economical sludge disposal. As shown in Fig. 5a, the CST of the AD effluent tended to decrease with the increase of Al3+ doses, indicating that Al3+ dosing improved the blended sludge dewaterability. As a matter of fact, the improvement of digestate dewaterability as a result of Al3+-based coagulant addition has been broadly reported.9,18,19 The enhancement of the dewaterability was explained by the interaction between Al3+ and extracellular polymeric substances (EPSs), i.e., the structure of water holding EPSs can be compressed as a result of surface charge neutralization and adsorption by Al3+ ions.9,10 Moreover, Al3+-based coagulants also act as skeleton builders during sludge dewatering, i.e., the precipitates formed as a result of the binding can create a rigid structure around sludge flocs, transmitting the mechanical dewatering stress through the filter cake.9 In addition, since PS usually possesses a better dewaterability than WAS,20 it is reasonable to see a higher PS blending ratio resulting in better sludge dewaterability (Fig. 5a).
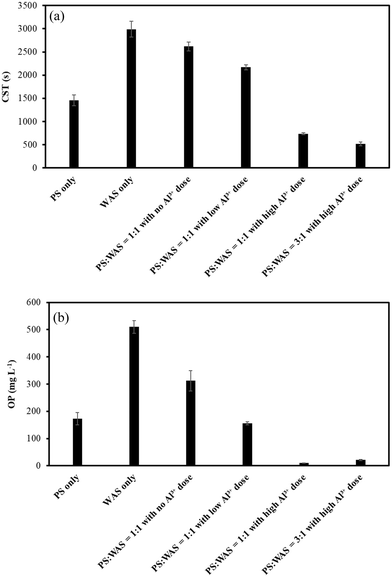 |
| Fig. 5 Steady-state (a) CST and (b) OP of all experimental groups. | |
OP also decreased with the increase in Al3+ addition in the blended groups (Fig. 5b). This can be explained by the precipitation between OP and Al3+.21 A previous study reported that OP removal by inorganic coagulant addition improved sludge dewaterability.21 An explanation was that the precipitates formed by the binding between Al3+ and OP can create a rigid permeable lattice structure that maintains high sludge structural strength and in turn impedes compression. Thus, the porous structure of the precipitate may serve as a draining medium for dewatering.9,21 Moreover, the improved sludge dewaterability as a result of OP removal can also be explained by the competition between OP and negatively charged sludge particles for cationic polymers.21 The reduced OP concentration is bound to leave more binding sites available on the cationic polymers for neutralizing and flocculating sludge particles.21 Thus, the negligible OP levels in the two blended groups with high Al3+ addition indicate the improvement of sludge dewaterability in this study (Fig. 5b). It should be noted that OP concentration in WAS is almost three times that in PS (Fig. 5b), probably due to the unintended biological phosphorus removal.
3.4. Effects of blending ratios and Al3+ dosing on TAN and DON production
The remarkably higher nitrogen content in WAS than in PS led to the same differences in TAN and DON production (Fig. 6a and b). Compared to the baseline average of PS-only and WAS-only groups, blending the two in a 1
:
1 ratio with no or low Al3+ dose resulted in an average TAN level but a much greater than average DON level (Fig. 6a and b). The higher-than-average DON levels in blended sludges have to do with the Maillard reaction between the reducing sugar hydrolyzed from PS and the amino acids hydrolyzed from WAS. This is because PS rich in carbohydrates and WAS rich in proteins provided precursors of reducing sugar and amino acids required for the Maillard reaction at 165 °C heating in the THP.11,12 It is interesting to observe that high Al3+ doses substantially reduced both TAN and DON levels regardless of the blending ratios used (Fig. 6a and b), which indicates that high Al3+ must have resulted in less available protein for the TAN and DON production. The precipitation of DON by Al3+ as indicated in a previous study may further explain the even lower DON levels under the high Al3+ doses.11 Mechanisms such as chelation and coagulation by metallic ions were believed to be involved in the DON precipitation.26,27
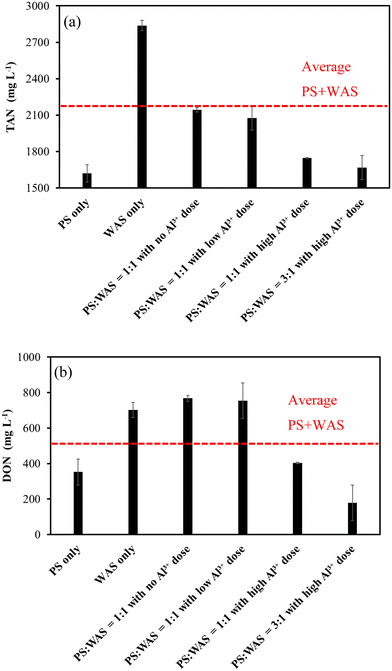 |
| Fig. 6 (a) TAN and (b) DON concentrations of all experimental groups at steady state. | |
3.5. Effects of blending ratios and Al3+ dosing on rDON formation
The centrates from four groups of digestates were subjected to rDON tests to understand the effects of sludge blending and Al3+ addition on rDON formation during THP. As can be seen in Fig. 7a, after 5 days of air stripping, the TAN concentrations of all four centrates became lower than 200 mg L−1. After that, all centrates were diluted two times to prevent nitrification from rDON inhibition. With 14 days of aeration, all remaining TAN gradually decreased to an undetectable level as a result of nitrification (Fig. 7b). This can also be confirmed by the corresponding NO3−-N profiles in Fig. 7c. Meanwhile, a gradual decrease in DON was also observed because the biodegradable fraction of DON was metabolized into TAN and then nitrate (Fig. 7d). For the WAS-only group and the blended group without Al3+ addition, their centrate TAN levels stopped dropping during days 7 and 11. A close inspection revealed that activated sludge has stuck to and accumulated on the inter wall of these glass jars above the water surface as a result of aeration, which must have compromised sludge activity in the mixed liquor. This issue was corrected by spiking 10 g thickened activated sludge in each bottle on the 12th day, and thereafter all glass jars were manually shaken on a daily basis to keep all biomass in the mixed liquid. As shown in Fig. 7b and c, as soon as the new inoculum was added, both groups' TAN levels quickly dropped to undetectable levels, and correspondingly nitrate concentrations dramatically increased. In contrast, the control group produced a negligible amount of TAN and DON during the 14 days of aerobic treatment.
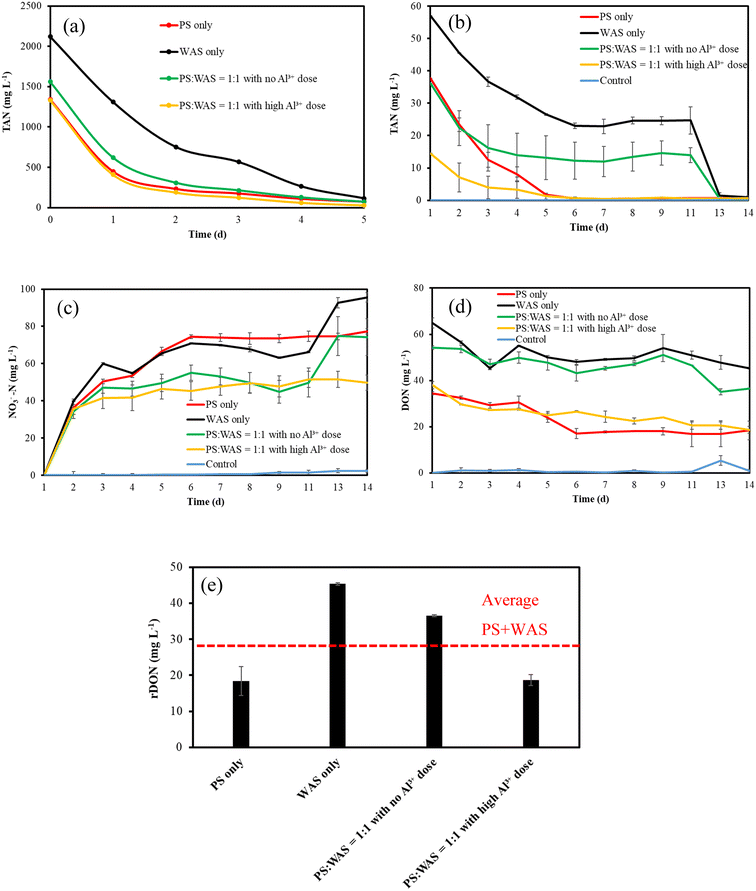 |
| Fig. 7 (a) TAN profiles in four experimental groups over five days of air stripping; (b) TAN, (c) NO3-N, and (d) DON concentration profiles for 14 days of aerobic treatment; and (e) final rDON in four AD centrates after aerobic treatment. | |
The final rDON concentrations in centrates from four groups are summarized in Fig. 7e. As can be seen, the rDON in the blended group without Al3+ addition was 15% higher than that of the average of the two individual PS-only and WAS-only groups. rDON is a product from the Maillard reaction between carbohydrates rich in PS hydrolysate and amino acids rich in WAS hydrolysate. It is confirmed from Fig. 7e that PS and WAS blending can substantially increase the rDON formation in THP. Hence, separating PS from WAS in the course of THP could be taken advantage of as an effective means to control rDON. Furthermore, with high Al3+ addition, the rDON concentration in the blended mixture was 50% lower than that without Al3+ addition (Fig. 7e), indicating rDON precipitation by Al3+. Therefore, Al3+ addition could be taken advantage of as another effective means to control rDON.
4. Discussion
4.1. Pros and cons of CEPT-enabled carbon diversion
To redirect wastewater organic carbon to ADs via PS production through the most popular CEPT method, coagulants such as alum need to be employed. Results in Fig. 4 exhibited that only high Al3+ doses may bring negative impacts on the sludge digestibility. It is usually believed that this adverse effect is from Al3+ binding with WAS protein but not the toxicity of Al3+.22,23 It is known that PS usually contains less protein but more carbohydrates than WAS.24 Therefore, blending more PS as shown in Fig. 4 was able to mitigate the problem to a great extent by reducing the protein content in the blended sludge so as to minimize the unavailability of protein for bacterial degradation. Although THP might have temporarily broken down the binding between Al3+ and proteins, their binding might just resume in the subsequent ADs. Moreover, adding Al3+ also improved sludge dewaterability as indicated by a much lower CST value of the digestate (Fig. 5a). OP precipitation by Al3+ is presumed to be the reason behind this. This is because these precipitates are known to form skeleton builders in aid of water drainage through the sludge cake.9 In addition, precipitation also reduced the competition of OP with negatively charged sludge particles for cationic polymers.21
Although THP is commonly used for enhancing sludge digestibility, blending PS with WAS has been shown in this study to lead to excessive rDON production, which is not the case when WAS only or PS only was pretreated (Fig. 7). This is because PS rich in carbohydrates and WAS rich in proteins provided precursors of reducing sugar and amino acids required for the Maillard reaction in THP.11,12 Fortunately, a high Al3+ dose was able to precipitate rDON and attenuated its concentration from 38 to 19 mg L−1. As a matter of fact, adding metallic ions such as Al3+ has been proposed as a strategy to control rDON in previous studies.11,25 Various mechanisms including chelation and coagulation were involved in the interaction of rDON with metallic ions as reported in previous studies.26,27 For example, the ketone or hydroxyl groups in a primary type of rDON, i.e., melanoidins, can act as a donor group and participate in the chelation with metals.26 In addition, the chelation of metal ions with amino and carboxyl groups in amino acids or the hydroxyl groups in sugar can also decrease the rate of the Maillard reaction.28 Moreover, high molecular melanoidins in colloidal range with the negative charge can be removed through coagulation by inorganic coagulants such as Al3+.29
4.2. Implication on carbon diversion adoption
PS production through the cost-effective CEPT is a crucial step towards the implementation of carbon diversion. First-hand experimental data obtained from this study revealed that there is a trade-off between PS production and energy recovery, i.e., the more carbon is diverted into PS, the higher the dose of coagulant is required, which in turn makes the mixture of PS and WAS less biodegradable (Fig. 4). It is actually broadly accepted that Al3+ addition can adversely affect the blended sludge anaerobic digestibility.9,23,30,31 Although the implementation of THP may improve the sludge biodegradability, rDON formation due to blending PS with WAS is another side effect that has not been previously taken into the consideration of carbon diversion. Many WRRFs such as those in Chesapeake Bay watershed, coastal areas of North Carolina, mid-Colorado, and Okanagan Lake area of British Columbia, Canada, have a stringent TN requirement, e.g., <3 mg L−1.12 Therefore, for WRRFs considering to adopt the carbon diversion strategy, a comprehensive evaluation of the overall gains is important. This is because energy neutrality is not the primary responsibility of WRRFs. Instead, their discharge quality and solid reduction efficiency are more important considerations. Hence, whether to adopt or to what extent to adopt carbon diversion depends on the priorities of WRRFs and thus should be decided on a case-by-case basis. To assist decision making, a comparison of the aforementioned three scenarios using data obtained from this study is presented in Fig. 8. A 0.5 g g−1 aerobic growth yield is assumed for WAS production in the aeration tank.32 In addition, 80% VS content in TS is also assumed. Based on the mass balance, Fig. 8a shows that without any carbon diversion, namely WAS only, 55% TS reduction can be achieved with 50% wastewater organics oxidized in the aeration tank and another 19% organics turned into CH4 in ADs (Fig. 8a). In comparison, diverting carbon into PS produced with a dry mass-to-WAS ratio equal to 1
:
1 achieved 51% TS reduction with 33% wastewater organics oxidized in the aeration tank and another 31% organics turned into CH4 in ADs (Fig. 8b). In the case of high PS production with a dry mass-to-WAS ratio equal to 3
:
1, only 48% TS reduction can be achieved with 20% wastewater organics oxidized in the aeration tank and another 41% organics turned into CH4 in ADs (Fig. 8c). A pattern that can be seen is that as the PS-to-WAS blending ratio increased from 0
:
1 to 1
:
1 and 3
:
1, the final residual TS increased to 9% and 16%, respectively. The aeration electricity can be potentially saved to 35% and 60%, respectively. The CH4 energy recovery can be increased to 67% and 120%, respectively. Apparently, the extents of energy savings and recovery are both substantially greater than that of the residual solid increase. A previous study reported that the cost of the biosolid treatment can be as high as 40–50% of the total wastewater treatment operational costs in the U.S.33 Therefore, a comprehensive technoeconomic analysis is needed for decision making down the road to take into account the increased biosolid disposal cost, the PS clarifier installation capital, CEPT operational cost, and secondary capacity upgrading savings as well as energy bill savings. Very importantly, the cost of activated carbon for rDON removal should be also taken into consideration if the room for accommodating the effluent permissible TN level is limited. Moreover, the dose of supplemental carbon in the denitrification process is also expected to increase as a result of carbon diversion to the solid stream because less influent carbon is available for denitrification. This negative effect from carbon diversion can potentially be mitigated by another new technology being developed and applied, namely partial denitrification anammox (PDNA).34 This is because anammox uses ammonia but not carbon as a reducer to reduce nitrate into nitrogen gas. To our knowledge, PDNA is the only anammox-based technology applicable in the mainstream wastewater treatment in temperate zones.35 At the same time, the energy cost for aeration can also be saved because only a portion of influent ammonia needs to be oxidized into nitrate.34 Hence, innovative technologies such as PDNA may work in synergy with carbon diversion to achieve sustainable wastewater treatment when it comes to infrastructure upgrading.
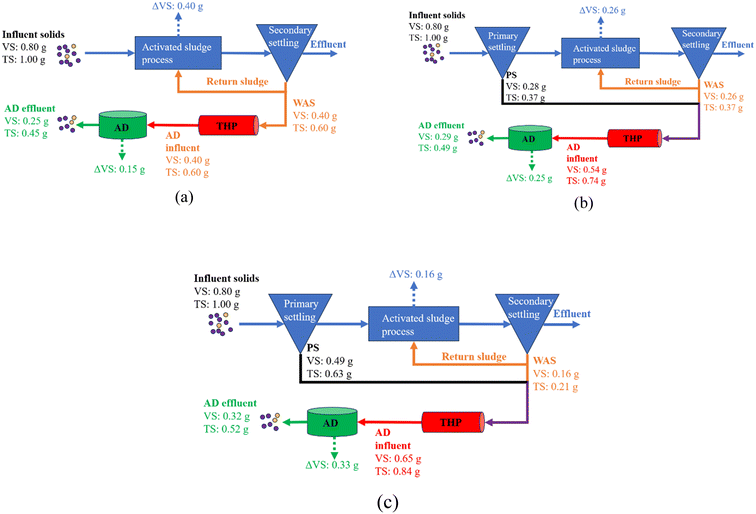 |
| Fig. 8 Solid mass flow through the WRRF (a) without primary settling, (b) with primary settling and a PS-to-WAS ratio of 1 : 1, and (c) with primary settling and a PS-to-WAS ratio of 3 : 1. | |
5. Conclusions
The following concluding remarks can be drawn from this study:
1. Increasing the PS production and blending can on one hand bring greater extents of energy recovery and savings but on the other hand lead to extra residual solids production.
2. Although coagulant addition through CEPT can deteriorate the sludge digestibility, increasing the PS
:
WAS blending ratio can mitigate the problem.
3. When THP is used as a pretreatment method, blending PS with WAS can result in the formation of excessive rDON which meanwhile can be removed by Al3+ dosing.
4. High Al3+ doses used for PS production can also improve sludge dewaterability by removing OP.
Conflicts of interest
There are no conflicts to declare.
Acknowledgements
This study was supported by the United States Department of Agriculture National Institute of Food and Agriculture (Award No. 2023-79000-38973) and the National Science Foundation (Award ID 2001568 and 2234562).
References
-
EPA, Energy efficiency in water and wastewater facilities, A Guide to Developing and, 2013.
-
EPA, Inventory of US Greenhouse gas emissions and sinks: 1990–2020, US Environmental Protection Agency, EPA, 2022, 430-R-422-003.
- I. Shizas and D. M. Bagley, Experimental determination of energy content of unknown organics in municipal wastewater streams, J. Energy Eng., 2004, 130, 45–53 CrossRef.
- A. S. Nizami, K. Shahzad, M. Rehan, O. K. M. Ouda, M. Z. Khan, I. M. I. Ismail, T. Almeelbi, J. M. Basahi and A. Demirbas, Developing waste biorefinery in Makkah: A way forward to convert urban waste into renewable energy, Appl. Energy, 2017, 186, 189–196 CrossRef CAS.
- H. Guven, R. K. Dereli, H. Ozgun, M. E. Ersahin and I. Ozturk, Towards sustainable and energy efficient municipal wastewater treatment by up-concentration of organics, Prog. Energy Combust. Sci., 2019, 70, 145–168 CrossRef.
- H. E. Schmidt Jr and S. Dhulashia, Carbon diversion and its role in energy efficiency, Florida Water Resources Journal, 2020, 54–58 Search PubMed.
- W. A. Shewa, T. Dong, W. Mu, K. Murray and M. Dagnew, The impact of chemically enhanced primary treatment on the downstream liquid and solid train processes, Water Environ. Res., 2020, 92, 359–368 CrossRef CAS PubMed.
- W. A. Shewa and M. Dagnew, Revisiting Chemically Enhanced Primary Treatment of Wastewater: A Review, Sustainability, 2020, 12, 5928 CrossRef CAS.
- H. Luo, Y. Sun, M. Taylor, C. Nguyen, M. Strawn, T. Broderick and Z. W. Wang, Impacts of aluminum-and iron-based coagulants on municipal sludge anaerobic digestibility, dewaterability, and odor emission, Water Environ. Res., 2022, 94, e1684 CrossRef CAS PubMed.
- W. Barber, Thermal hydrolysis for sewage treatment: a critical review, Water Res., 2016, 104, 53–71 CrossRef CAS PubMed.
- D. Zhang, Y. Feng, H. Huang, W. Khunjar and Z.-W. Wang, Recalcitrant dissolved organic nitrogen formation in thermal hydrolysis pretreatment of municipal sludge, Environ. Int., 2020, 138, 105629 CrossRef CAS PubMed.
- D. Zhang, Z. An, M. Strawn, T. Broderick, W. Khunjar and Z.-W. Wang, Understanding the formation of recalcitrant dissolved organic nitrogen as a result of thermal hydrolysis and anaerobic digestion of municipal sludge, Environ. Sci.: Water Res. Technol., 2021, 7, 335–345 RSC.
- J. E. Hodge, B. E. Fisher and F. D. Mills, Compounds of browned flavor derived from sugar-amine reactions, Cereal Sci. Today, 1972, 17, 34–40 Search PubMed.
- J. Dwyer, D. Starrenbury, S. Tait, K. Barr, D. J. Batstone and P. Lant, Decreasing activated sludge thermal hydrolysis temperature reduces product colour, without decreasing degradability, Water Res., 2008, 42, 4699–4709 CrossRef CAS PubMed.
- J. M. Abelleira-Pereira, S. I. Perez-Elvira, J. Sanchez-Oneto, R. de la Cruz, J. R. Portela and E. Nebot, Enhancement of methane production in mesophilic anaerobic digestion of secondary sewage sludge by advanced thermal hydrolysis pretreatment, Water Res., 2015, 71, 330–340 CrossRef CAS PubMed.
-
APHA, Standard methods for the examination of water and wastewater, American Public Health Association, Washington DC, 22nd edn, 2012 Search PubMed.
- N. Pinto, A. Carvalho, J. Pacheco and E. Duarte, Study of different ratios of primary and waste activated sludges to enhance the methane yield, Water Environ. J., 2016, 30, 203–210 CrossRef CAS.
- W. Zhang, P. Xiao, Y. Liu, S. Xu, F. Xiao, D. Wang and C. W. Chow, Understanding the impact of chemical conditioning with inorganic polymer flocculants on soluble extracellular polymeric substances in relation to the sludge dewaterability, Sep. Purif. Technol., 2014, 132, 430–437 CrossRef CAS.
- S. Jing, Y. Lin, Y. Lin, C. Hsu, C. Huang and D. Lee, Evaluation of effective conditioners for enhancing sludge dewatering and subsequent detachment from filter cloth, J. Environ. Sci. Health, Part A: Toxic/Hazard. Subst. Environ. Eng., 1999, 34, 1517–1531 CrossRef.
- M. J. Higgins, S. Beightol, U. Mandahar, R. Suzuki, S. Xiao, H.-W. Lu, T. Le, J. Mah, B. Pathak, H. DeClippeleir, J. T. Novak, A. Al-Omari and S. N. Murthy, Pretreatment of a primary and secondary sludge blend at different thermal hydrolysis temperatures: Impacts on anaerobic digestion, dewatering and filtrate characteristics, Water Res., 2017, 122, 557–569 CrossRef CAS PubMed.
- D. Zhang, B. Angelotti, E. Schlosser, J. T. Novak and Z. W. Wang, Using cerium chloride to control soluble orthophosphate concentration and improve the dewaterability of sludge: Part I. Mechanistic understanding, Water Environ. Res., 2019, 320–330 Search PubMed.
- J.-O. Kim and J. Chung, Inhibitory effects of inorganic and organic coagulants on acidogenic fermentation, KSCE J. Civ. Eng., 2015, 19, 572–577 CrossRef.
- J. M. Gossett, P. L. McCarty, J. C. Wilson and D. S. Evans, Anaerobic digestion of sludge from chemical treatment, J. - Water Pollut. Control Fed., 1978, 533–542 CAS.
-
U. Tezel, M. Tandukar and S. G. Pavlostathis, Anaerobic biotreatment of municipal sewage sludge, in Book Comprehensive Biotechnology, 2011, pp. 447–461 Search PubMed.
- T. Gomyo and M. Horikoshi, On the interaction of melanoidin with metallic ions, Agric. Biol. Chem., 1976, 40, 33–40 CAS.
- J. A. Rufián-Henares and S. P. de la Cueva, Antimicrobial Activity of Coffee Melanoidins A Study of Their Metal-Chelating Properties, J. Agric. Food Chem., 2009, 57, 432–438 CrossRef PubMed.
- H.-Y. Wang, H. Qian and W.-R. Yao, Melanoidins produced by the Maillard reaction: Structure and biological activity, Food Chem., 2011, 128, 573–584 CrossRef CAS.
- D. T. Ramonaitytė, M. Keršienė, A. Adams, K. A. Tehrani and N. De Kimpe, The interaction of metal ions with Maillard reaction products in a lactose–glycine model system, Food Res. Int., 2009, 42, 331–336 CrossRef.
- M. M. Arimi, Y. Zhang, G. Götz and S.-U. Geißen, Treatment of melanoidin wastewater by anaerobic digestion and coagulation, Environ. Technol., 2015, 36, 2410–2418 CrossRef CAS PubMed.
-
J. T. Novak and C. Park, Effect of aluminum and iron on odors, digestion efficiency, and dewatering properties, Water Environment Research Foundation, 2010 Search PubMed.
- T. Abbott and C. Eskicioglu, Effects of metal salt addition on odor and process stability during the anaerobic digestion of municipal waste sludge, Waste Manage., 2015, 46, 449–458 CrossRef CAS PubMed.
- J. Wan, J. Gu, Q. Zhao and Y. Liu, COD capture: a feasible option towards energy self-sufficient domestic wastewater treatment, Sci. Rep., 2016, 6, 25054 CrossRef CAS PubMed.
-
I. S. Turovskiy and P. Mathai, Wastewater sludge processing, John Wiley & Sons, 2006 Search PubMed.
- Q.-G. You, J.-H. Wang, G.-X. Qi, Y.-M. Zhou, Z.-W. Guo, Y. Shen and X. Gao, Anammox and partial denitrification coupling: a review, RSC Adv., 2020, 10, 12554–12572 RSC.
- Q. Liu, Y. Peng, Y. Zhao, Q. Zhao, X. Li, Q. Zhang, J. Sui, C. Wang and J. Li, Excellent anammox performance driven by stable partial denitrification when encountering seasonal decreasing temperature, Bioresour. Technol., 2022, 364, 128041 CrossRef CAS PubMed.
|
This journal is © The Royal Society of Chemistry 2024 |
Click here to see how this site uses Cookies. View our privacy policy here.