DOI:
10.1039/D3AY00844D
(Critical Review)
Anal. Methods, 2023,
15, 4351-4376
New technologies and reagents in lateral flow assay (LFA) designs for enhancing accuracy and sensitivity
Received
24th May 2023
, Accepted 9th August 2023
First published on 9th August 2023
Abstract
Lateral flow assays (LFAs) are a popular method for quick and affordable diagnostic testing because they are easy to use, portable, and user-friendly. However, LFA design has always faced challenges regarding sensitivity, accuracy, and complexity of the operation. By integrating new technologies and reagents, the sensitivity and accuracy of LFAs can be improved while minimizing the complexity and potential for false positives. Surface enhanced Raman spectroscopy (SERS), photoacoustic techniques, fluorescence resonance energy transfer (FRET), and the integration of smartphones and thermal readers can improve LFA accuracy and sensitivity. To ensure reliable and accurate results, careful assay design and validation, appropriate controls, and optimization of assay conditions are necessary. Continued innovation in LFA technology is crucial to improving the reliability and accuracy of rapid diagnostic testing and expanding its applications to various areas, such as food testing, water quality monitoring, and environmental testing.
1. Introduction
The demand for quick tests is growing, encouraging the creation of novel methods for agriculture, human and animal health diagnosis, environmental monitoring, food analysis, military, and forensic science.1 Therefore, creating affordable, reliable, and quick diagnostic testing equipment for use outside laboratories is crucial. The availability of accurate point-of-care (POC) diagnostic tools for just four infective diseases—malaria, bacterial pneumonia, syphilis, and tuberculosis—is thought to be able to avert at least 1.2 million deaths annually worldwide.2–4 The point-of-care (POC) test is commonly known as the lateral flow assay (LFA), alternatively referred to as a lateral flow device (LFD), lateral flow test (LFT), or quick test. This method was first reported in 1956 (ref. 3) and is a straightforward technique designed to identify a target material in a liquid sample without sophisticated or expensive equipment. In the early 1970s, the LFA was first commercially developed as a home-use pregnancy test to analyze human chorionic gonadotropin (hCG) hormone in urine samples.5
The assay is typically formed by sandwiching6 a sample between two material layers, a test strip, and a conjugate pad (Fig. 1). The test strip is typically made of a porous material, such as nitrocellulose or cellulose acetate, that allows the sample to flow through it. The strip contains a test line, where a colored line appears if the analyte is present in the sample. The test line is made of a material that binds specifically to the analyte of interest, such as an antibody or a protein. The conjugate pad includes a second reagent that binds precisely to the target analyte. The conjugate pad is often made of a non-porous substance that prevents the flow of the sample, like cellulose or polyester. Usually, a tracer, such as gold nanoparticles, an enzyme, or a fluorescent dye, is added to the conjugate pad to attach to the analyte and give an indication that can be seen. The sample runs through the test strip after being applied to it, and then it bonds to the test line. The second reagent is subsequently bound to the sample as it passes through the conjugation pad. If the analyte is present in the sample, a colored line will appear on the test strip, indicating a positive result. A control line should appear for both positive and negative results to check the test's validity and verify the device's functioning. The sensitivity and specificity of the assay can be fine-tuned by using different antibodies, proteins, or other reagents, which bind specifically to different regions of the analyte.
 |
| Fig. 1 Schematic showing the essential parts and working principle of an LFA device. (a) The main components of LFA devices are the absorbent pad, conjugate pad, membrane, and sample pad. All these parts are laminated on a card. An example of LFA based on immunosandwich recognition is demonstrated in panels b and c. (b) When the target analyte is available in the sample, nanoparticles build up on both the control and test lines; (c) when the target analyte is absent in the sample, the nanoparticles exclusively accumulate on the control line.7 | |
The lateral flow assay (LFA) design has evolved, with different reagents being used to detect specific analytes. Some of the most common trends in LFA design include the use of antibodies, enzymes, peptides, mRNAs, fluorescence, and quantum dots. Antibodies are proteins that bind to a specific target, such as a virus or disease marker.8 Enzymes are proteins that catalyze chemical reactions and are commonly used in LFAs. For example, the enzyme replaces glucose with gluconic acid and hydrogen peroxide in glucose testing, generating a visible signal.9 Peptides are short chains of amino acids used in LFAs as the test line reagent.10 Messenger RNA (mRNA) is a genetic material that carries genetic information from DNA to the ribosome.11 Fluorescence is the property of some molecules to release light when excited by the specific wavelength of light. Fluorophores are often used as tracers in LFAs12 to generate a visible signal. Quantum dots are tiny semiconductor particles that can be used as tracers in LFAs13 and are often used in LFAs due to their high fluorescence intensity, stability, and versatility. Finally, antibodies, enzymes, mRNA, and peptides are commonly used in LFAs as the test line reagent. They are often labeled with a tracer such as gold nanoparticles, enzymes, or fluorescent dyes to generate a visible signal. These are some common trends in LFA design, but new technologies and reagents are constantly being developed.
This review presents the LFAs' new integration trends using Raman spectroscopy, photoacoustic, fluorescence, smartphones, thermal readers, and other reagents. Integrating these advanced technologies and reagents with lateral flow assays can enhance the accuracy and sensitivity of the results, achieve faster results, accomplish more convenient sample preparation, and lead to cost savings. In addition, integrating these technologies and reagents has enabled the development of rapid POC diagnostics, which can be used in isolated and underdeveloped areas and traditional clinical settings.
2. LFA design and readout systems
2.1 Comparison of different LFA designs
Many POC lateral flow assays are available, each with unique design features. The following table compares the features of five popular POC LFAs based on the limit of detection (LOD) ranges, analytical sensitivity, and detection times. It should be noted that the transformation of units between g mL−1 and molarity (M) can be done by calculating the molar weight of the analytes. The table compares the performance of traditional LFA devices with that of electrochemical, surface enhanced Raman scattering (SERS), fluorescence, photothermal, and magnetic-based LFA devices. An electrochemical lateral flow assay (eLFA), which is mainly a biosensor, introduces an automated signal detection technique for improving analytical implementation in terms of facilitating sensitivity, rapidity, and selectivity.14 Chemically enhanced LFAs (laser-induced fluorescence assays) use new fluorescence-based labels and reagents to improve specificity and accuracy.15 Photothermal LFAs use photothermal laser speckle imaging, thermal contrast photoacoustic imaging, and thermal photonic lock-in imaging.16–19 SERS coupled with PCR (polymerase chain reaction) utilizes amplification of the analyte to detect single base changes in DNA.20 Magnetic lateral flow immunoassays utilize magnetic nanoparticles (MNPs) as the detection label instead of the traditional gold or latex beads to facilitate the enrichment of the analytes. These MNPs can be detected and measured using external devices, enabling the creation of immunochromatographic tests that can produce quantitative results (Table 1).21
Table 1 Comparison of different LFA designs
LFA design |
LOD (M) |
Analytical sensitivity (μg mL−1) |
Time of detection (min) |
Reference |
Traditional |
10−5 to 10−7 |
0.1 |
5–10 |
22
|
Electrochemical |
10−9 to 10−15 |
4.6 × 10−6 |
10–60 |
23
|
SERS |
10−9 to 10−15 |
10−7 |
15–30 |
24
|
Fluorescence |
10−8 to 10−15 |
0.008 |
10–60 |
25
|
Photothermal |
10−7 to 10−13 |
10−5 |
10–25 |
17
|
Magnetic |
10−7 to 10−13 |
1.6 × 10−8 |
10–30 |
26
|
2.2 Comparison of the readout systems
Different readout systems are available for LFAs, including smartphones, upconverting nanoparticles (UCNPs), surface-enhanced Raman spectroscopy (SERS), and photoacoustic and thermal contrast reader systems. Each type of readout system has advantages and disadvantages that must be considered when selecting the appropriate one for a given application. Greater emphasis will be given to those readers best suited to commercialization based on comparing their price, dimensions, operation mode, and reported assay sensitivity, as shown in Table 2.27
Table 2 Comparison of the readout systems
Readout system |
Signal |
Application |
LOD (pg mL−1) |
Price ($) |
Size (mm3) |
Weight |
Ref. |
Smartphone |
Colorimetric and luminescence |
E. coli 0157:H7 HIgG, EVD-IgG, HIgG, PSA, AFB1 |
590 to 2 × 105 |
200–250 |
15 × 8 × 1 to 136 × 69 × 7 |
130–400 |
28–33
|
UCNP reader |
Luminescence |
Triamcinolone acetonide, ST-2, ochratoxin A |
980–5000 |
650–700 |
240 × 94 × 54 to 300 × 300 × 150 |
900–2000 |
34–36
|
SERS reader |
SERS |
hCG |
106 |
3000 |
180 × 110 × 47 |
1000 |
37
|
Photoacoustic reader |
PA |
Glucose |
5.4 × 106 |
1300 |
40 × 20 × 20 |
4000 |
38
|
Thermal contrast reader |
TCA |
hCG |
180 |
500 |
133 × 108 × 73 |
|
39
|
3. New technologies, systems, and reagents used in LFA design
3.1 Surface-enhanced Raman spectroscopy-based LFAs
Gold nanoparticles are commonly used in traditional colorimetric LFA (CLFA) because they are easy to make and can be seen with the naked eye. However, CLFA has low sensitivity compared to other methods, so it is not always suitable for measuring analytes with low concentrations. CLFAs are primarily used for yes/no type binary decision-making applications (presence or absence of the analyte). To measure the analytes' concentration, the test zone's color can be scanned and analyzed with equipment or a smartphone app. However, this procedure has a restricted working range and is highly nonlinear. Therefore, there is a need to find new labels and readout technologies for LFA. SERS tags leverage the plasmon response of nanoparticles used in numerous analytical and imaging applications. They consist of Au or metal nanoparticles with Raman-active molecules and recognizing antibodies. SERS tags are more efficient than fluorescent labels, and their Raman scattering intensity allows for detecting analytes with very low concentrations. Additionally, the narrow spectral bandwidth of Raman spectra is suitable for multiplexing, and studies have shown increased sensitivity and dynamic range in LFA using SERS readout modalities.
SERS-LFA has many advantages over traditional LFA, including a much lower LOD (Table 3) and the ability to detect a wide range of analytes quantitatively and multiplexed. However, the need for costly equipment and long signal processing time restricts its use as a first choice for POC applications. Recent developments in compact SERS readers for LFA strips show promise in solving these issues. Further developments could include creating a lateral flow strip with low fluorescence background and modifying SERS tags to eliminate nonspecific adsorption. The integration of SERS-LFA with automated biochips has the potential to create a compact and highly sensitive detection platform suitable for POC diagnostics.
Table 3 Comparison of LOD of traditional and SERS LFAs
Sample |
LOD of traditional LFA |
LOD of SERS LFA |
Reference |
Troponin I |
5 ng mL−1 |
0.09 ng mL−1 |
40
|
TSH |
1.5 μIU mL−1 |
0.025 μIU mL−1 |
41
|
HIV |
80 pg mL−1 |
8 pg mL−1 |
42
|
Staphylococcal enterotoxin B |
10 ng mL−1 |
0.001 ng mL−1 |
43
|
H1N1 |
67 ng mL−1 |
6.7 ng mL−1 |
44
|
Influenza virus A |
5 × 104 pfu mL−1 |
1.9 × 104 pfu mL−1 |
45
|
Zika
|
10 ng mL−1 |
0.72 ng mL−1 |
46
|
Dengue |
50 ng mL−1 |
7.67 ng mL−1 |
46
|
SERS-based sandwich immunoassay was used to detect rabbit immunoglobin G, significantly saving assay time without losing sensitivity.47 A similar concept had been used for SERS-based LFAs assay to quantitatively test a human immunodeficiency virus type 1 (HIV-1) DNA in the low concentration range. This SERS-based lateral flow assay has a detection limit of 0.24 pg mL−1, which was at least a thousand times more sensitive than colorimetric or fluorescent detection techniques.48 The schematic diagram of the arrangement and the operational principle of the SERS-based lateral flow assay is depicted in Fig. 2.
 |
| Fig. 2 Schematic showing the (a) design and (b) working principle of the SERS-based LFA device. Here, T = test line, and C = control line.48 | |
3.2 Photoacoustic-based LFAs
Compared to traditional lateral flow assay devices, photoacoustic lateral flow assay devices have multiple benefits. Firstly, they are more sensitive and specific since they use a photoacoustic signal generated by laser absorption to detect small amounts of analyte with high precision. Secondly, the ability to detect numerous analytes simultaneously in one sample is feasible with photoacoustic-based LFAs. Thirdly, they have a wider dynamic range, allowing for accurate measurement of high and low analyte concentrations. Lastly, they are convenient and portable, perfect for point-of-care testing (POCT) in distant or resource-constrained environments.
The pioneering work of Zhao et al.17 introduced the concept of a photoacoustic-based lateral flow test, showcasing the quantitative identification of a disease biomarker by leveraging the strong interaction between light and gold nanoparticles. The history of photoacoustic point-of-care testing dates to the 1970s,49 when the first prototype devices were developed. These devices were used to measure blood oxygen saturation in a non-invasive approach. Since then, photoacoustic point-of-care testing has evolved to include a variety of measurements, including glucose, cholesterol, uric acid, and other biomarkers. Compared to colorimetric measurements, photoacoustic analysis improved the detection limit of a commercially available lateral flow test strip by two orders of magnitude.17Fig. 3 shows the typical design of photoacoustic-based LFA and two different arrangements of PA detector setups. The test strip includes nanoparticles that are bound to the target analyte. A photoacoustic sensor detects the sound waves produced when light is illuminated on the test strip and after the nanoparticles have absorbed the light. This creates a signal showing the analyte's presence in the sample. This kind of test has the potential to be extremely sensitive, precise, and quick, making it helpful in several contexts, including remote or underdeveloped places. Its integration with existing diagnostic platforms makes the potential to transition from laboratory research to preclinical and clinical reality possible.50
 |
| Fig. 3 Schematics of two different arrangements of PA detection setups. (a) Illustration of a laser beam producing PA signals on an LFA device. The acoustic signal can be detected using a simple microphone. Schematic of (b) chopper-based and (c) scanner-based PA detection systems.17 | |
3.3 Fluorescence resonance energy transfer (FRET) based LFAs
Compared to traditional LFAs, FRET-based LFAs have several benefits. They offer higher sensitivity and specificity and can be used for multiplex detection and real-time monitoring of analyte binding. They also have a more comprehensive dynamic range and can be easily automated for high-throughput analysis. These advantages are due to the FRET signal, generated only when the target analyte is present, reducing false positives. Further, FRET-based LFA could be used in complex sample matrices, including blood, saliva, serum, and urine, making them useful for various applications.
Wang et al.51 developed a high-performance fluorescence LFA strip (Fig. 4) by using S protein-conjugated dual quantum dot (SiO2@DQD) nanotags for the detection of SARS-CoV-2-specific antigens (IgM/IgG) in clinical samples. The synthesized NPs had excellent luminescence and good stability properties and were monodispersed. The anti-human IgM and IgG antibodies were immobilized on two test lines to simultaneously and accurately identify anti-SARS-CoV-2 IgM and IgG in a single human sample. They achieved this by immobilizing the SARS-CoV-2 spike protein onto the surfaces of SiO2@DQD NPs. The proposed SiO2@DQD-based LFA strip demonstrated the ability to rapidly and precisely detect low concentrations of IgM/IgG (at a dilution of 1
:
107) from just one μL of serum in only 15 minutes. Similar approaches have been utilized to design LFAs for detecting the H5N2 influenza virus52 by changing the antibodies specific to the H5N2 virus.
 |
| Fig. 4 Schematic representation of (a) the synthesis of the dual quantum dot (DQD) shell using SiO2 NPs (SiO2@DQD). (b) Design of LFA strips to detect SARS-CoV-2 antigens using SiO2@DQD.51 | |
In another study, Rong et al.53 developed an integrated fluorescent LFA platform (Fig. 5) for multiplexed detection of four antibodies (HCV, TP, HIV, and HBsAg). The assay was able to detect very low levels of these antibodies, with the lowest detection limit for HCV, TP, HIV, and HBsAg were 0.14 NCU mL−1, 0.62 IU L−1, 0.11 NCU mL−1, and 0.22 IU mL−1, respectively. The test was able to provide results within 20 min.
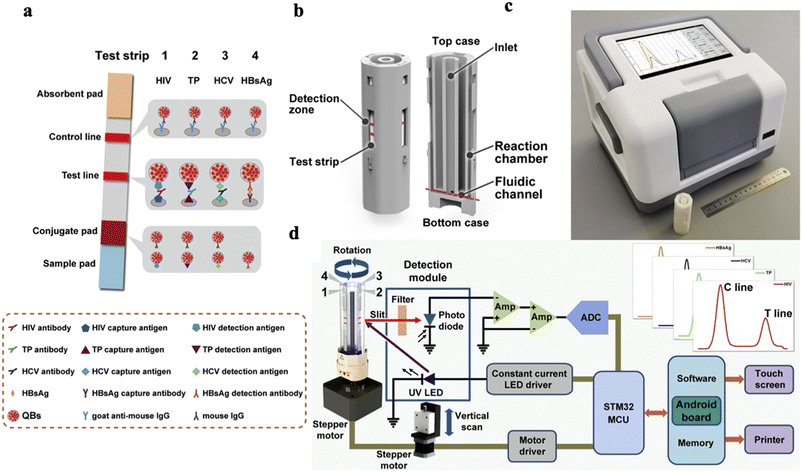 |
| Fig. 5 Fluorescence-based LFA. (a) Schematic of the multiplexed LFA device for infectious disease detection. Optical image of the (b) disposable LFA cartridge, (c) LFA reader, and (d) circuit design of the read-out system.53 | |
3.4 Thermal contrast reader-based LFAs
The light-to-heat conversion of metallic nanoparticles after exposure to a NIR laser is the basis for operating thermal contrast readers.27 This method uses thermal contrast amplification (TCA) to improve the sensitivity of the traditional colorimetric LFAs. It utilizes surface plasmon resonance of gold nanoparticles under laser irradiation.16 Here, the main investigation points are the thermophysical and biological effects of aqueous media heated by laser-activated gold nanoparticles (GNPs).54
The TCA reader is designed to align its laser wavelength precisely with the peak of the GNP plasmon resonance. This alignment results in the generation of heat, which leads to an increase in temperature. The infrared detector within the TCA reader can monitor this temperature increase.56 This heat production is proportional to the laser intensity, wavelength, GNP geometry, and concentration. As a result, we can accurately identify and measure the GNP signal for a known disease biomarker.56 A TCA reader system has three main components according to their functions – IR camera, green laser (as the plasmon resonance of GNP is ∼525 nm), and control. LabVIEW is generally used for position and time control, and specific holders are designed to house the LFAs.16 An example of a TCA-based readout is shown in Fig. 6.
 |
| Fig. 6 Thermal contrast amplification (TCA) principle and system.55 | |
Wang et al.16 (Fig. 7) demonstrated the utilization of the area under the curve (AUC) at the test line location in the LFA as a means to obtain a thermal signal. In this proof of concept, the TCA reader successfully validated a visually negative result of a malaria first response lateral flow assay (LFA) as a true positive. This LFA's thermal signal score was 1.144 °C as opposed to the negative control's average of 0.179 °C (SD = 0.053).
 |
| Fig. 7 The TCA reader utilizes the area under the curve (AUC) at the test line to measure and identify the temperature rise in a lateral flow assay (LFA).16 | |
3.5 Magnetic nanoparticle-based LFAs
Magnetic LFAs combine the speed and ease of LFAs using magnetic nanoparticles (MNPs) as markers. MNPs are particles with a surface that has been functionalized to specifically attach to target analytes. Additionally, these particles possess a magnetic core typically composed of iron oxide. Magnetic readout methods include giant magnetoresistance (GMR),57 tunneling magnetoresistance (TMR), and magnetic particle quantification (MPQ).58 Due to its capacity to recognize the magnetic signal produced by the MNPs, magnetic sensors are frequently utilized in magnetic LFAs. These sensors can quantify or qualitatively describe changes in magnetic fields or magnetic characteristics brought on by the presence of MNPs. In their study, Nguyen et al.59 conducted a comprehensive review encompassing these sensors (Fig. 8). The GMR effect, observed in multilayers with alternating ferromagnetic and nonmagnetic conductive layers, leads to significant changes in electrical resistance based on the orientation of neighboring ferromagnetic layers. When applied, magnetic fields interact with MNPs, resulting in modifications to the measured electric resistance. This enables the quantification of MNPs in GMR-based detection for lateral flow tests. GMR sensors have been demonstrated to detect human chorionic gonadotropin (hCG), interferon gamma (IFN-γ), and cardiac troponin I (cTnI) with greater sensitivity compared to traditional colorimetric detectors.60,61 However, GMR-based LFA has limitations, including the need for precise spacing between the sensing element and MNPs, limited reusability, susceptibility to damage or contamination from toxic chemicals, and low dose–response sensitivity that requires improvement.59 To overcome these limitations, TMR sensors were developed. TMR sensors are more sensitive than GMR sensors and function based on the spin-dependent tunneling phenomenon.62 The MNPs-embedded assay chip is positioned between magnets in TMR-based detection for lateral flow assays, and the magnetic field passing through the chip causes a change in resistance of the nearby TMR sensor. The biomarkers bound to the MNPs can be quantitatively measured by monitoring the subsequent resistance changes. Compared to GMR sensors, TMR sensors offer a better magnetoresistance ratio and less field noise. Lei et al.63 created a prototype TMR sensor to detect hCG at a 25 mIU mL−1 concentration.
 |
| Fig. 8 Enhancing the sensitivity of LFAs using a magnetic field. Schematic representation of LFA using (a) giant magnetoresistance (GMR),57 (b) an example of a GMR-based LFA device and results. (c) Schematic representation of LFA using tunneling magnetoresistance (TMR), (d) representative results from a TMR device. (e) Schematic representation of magnetic particle quantification (MPQ)-based LFA, (f) representative results from an MPQ device.59 | |
MPQ sensors, a type of magnetic-LFA, assess the nonlinear magnetization response of particles when subjected to a magnetic field to quantify them. The concentration of particles can be determined by measuring the induction response they generate in an alternating magnetic field. By combining two AC frequencies (f1 and f2), sensitive and reliable measurements can be achieved at specific combinatorial frequencies.64 This method enables sensitive measurement of analytes based on the relative changes in magnetic susceptibility. For the identification of prostate-specific antigen (PSA), Orlov et al.65 created an immuno-magnetic MPQ-based LFA detector. The results showed a sensitive and linear response. With a linear response spanning a 7-order shift in magnetic particle mass, the LOD observed for 200 nm magnetic beads was 60 zmol. The MPQ sensor can monitor membrane-dwelling particles, which is challenging with traditional optical detection techniques. The MPQ detector has also been used in multiplexed detection for the detection of cardiac biomarkers and the identification of several botulinum neurotoxins.
3.6 Smartphone-based LFAs
Smartphone-based lateral flow assay (SLFA) significantly affects many different types of point-of-care medical diagnostics. LFAs for infectious diseases, cardiac and tumor biomarkers, other hormones, or medication screening may all be readily integrated into this system.66 Gong et al.35 developed up-conversion nanoparticle-based lateral flow assays (UCNP-LFAs) (Fig. 9) to improve the sensitivity. The LFA was integrated into a smartphone to improve its usability.
 |
| Fig. 9 Up-conversion nanoparticle (UCNP)-based LFA. Schematic illustration of UCNP (a) LFA, (b) reader, and (c) smartphone app.35 | |
Jung et al.67 proposed an SLFA system that utilizes the smartphone as a colorimetric and quantitative reader. This procedure enhances the classification of the slight variations brought on by various analyte concentrations. While the naked eye cannot reliably verify the existence of a test band, especially at very low analyte concentration, these smartphone readers can detect the test band using image analysis tools. Smartphones can capture a digital image of the experiment's outcome with time and date stamps, which is useful for building databases and subsequent implementation of machine learning (ML)/artificial intelligence (AI) tools. In one example, You et al.66 utilized a smartphone with a built-in camera to read TSH levels. However, they used the Mie scatter to improve the sensitivity and repeatability of the TSH LFA. They demonstrated a limit of detection (LOD) of 0.3 mIU L−1 using the Mie scattering technology (Fig. 10). This made it possible to identify hyperthyroid conditions, which otherwise need a clinical laboratory. The gadget demonstrated good sensitivity, repeatability, and a testing time of ∼12 min.
 |
| Fig. 10 Smartphone coupled LFA detection system. (a) An exploded view shows the smartphone's arrangement, optical system, and LFA sensor. A collimating lens and optical fiber (light pipe) were used to enhance the collection of signals (scattered light from the NPs at the control and test lines). (b) Design of a disposable lateral flow assay test strip for TSH detection. Anti-TSH antibody was immobilized in the test band, anti-IgG in the control band, and gold nanoparticle-conjugated antibodies in the gold conjugate pad. (c and d) Optical images showing the assembled smartphone and TSH detection system.66 | |
Another study used a smartphone-integrated LFA system for infectious disease detection. Rong et al.68 developed a platform for the point-of-care detection of nonstructural protein 1 from the Zika virus (ZIKV NS1) using fluorescent LFIAs coupled to a smartphone. The study demonstrated the ability of a 3D-printed attachment to integrate external optical and electrical components with a smartphone, resulting in downsizing and cost savings. This method allowed for quantitative detection of ZIKV NS1 at the point of care in less than 20 min., with limits of detection (LODs) of 0.15 ng mL−1 for serum and 0.045 ng mL−1 for buffer. Fig. 11 presents the smartphone-based imaging system used in the ZIKV NS1 study. To generate the fluorescence signal, QD microspheres were used. The excitation wavelength of λ = 365 nm was achieved using a high-power UV LED (5 W). To reduce direct reflection, the incident angle of UV light was set to 60°. An improved signal-to-noise ratio (SNR) was achieved using a combination of a short-pass filter (425 nm) and a bandpass filter (624/40 nm).
 |
| Fig. 11 Overview of the design and use of the smartphone-integrated fluorescent LFA platform. (a) A 3D schematic of the internal organization of the smartphone-based imaging equipment. (b) A picture of the luminous LFIA reader in use. (c) Design of the fluorescent LFA for ZIKV NS1 detection. (d) Photographs of the test strips with (positive) and without (negative) ZIKV NS1.68 | |
Ross et al.69 demonstrated a method using surface plasmon resonance (SPR) for selecting crude anti-hazelnut antibodies for use in a quick ligand binding assay based on comparative association rates, cross-reactivity, and sandwich combination capacities. When using smartphone apps, these LFAs produce semi-quantitative data and a qualitative outcome when read visually. Fig. 12 shows an example of smartphone-based SPR biosensors.69
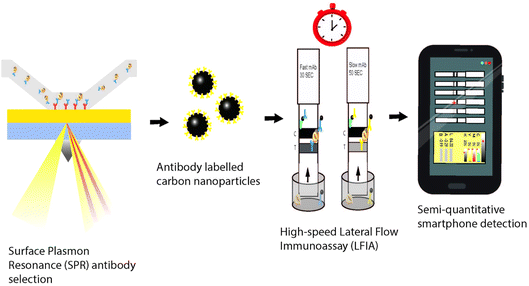 |
| Fig. 12 Schematic overview of a smartphone-based surface plasmon resonance biosensor.69 | |
Exploiting smartphones' capacity for SERS detection is vital since it might significantly broaden the applications of LFA test strips based on SERS.70 Mu et al.71 recently developed a smartphone Raman system, in which the spectrometer is integrated into the back of a smartphone, as shown in Fig. 13, through a slit coupling and rational optical path design. The ease of data sharing is another critical advantage of the smartphone. The collected Raman data can be sent to a computer or cloud over wireless networks for further analysis and processing. The chemical could then be identified using a matching algorithm and the Raman spectrum database. Although the system cost was ∼$10
000, it was still significantly lower than that of a standard laboratory Raman system (which can cost > $50
000).70,71
 |
| Fig. 13 Raman substance detection method for smartphones based on cloud network architecture.70 | |
4. Incorporating new technologies and reagents in LFA design
LFAs require several micrometer pores sizes in the paper, limiting the biomolecule capturing capability and assay sensitivity. Most of the LFAs can only detect one biomarker at a time. Due to the complexity of human disease, overlapping symptoms, and co-infection states, there is rising demand for multiplexed systems that can detect many biomarkers simultaneously. Multiplexing may only be possible with a few biomarkers because of inherent restrictions in designing conventional membrane-based LFAs. The multiplexing capability of microarray-based LFAs necessitates precise flow positioning between upstream and downstream spots.72,73 There are several strategies that researchers are exploring to increase the sensitivity and specificity of LFAs; some of the most common are discussed in this review. One of the ways to improve the specificity is by multiplexing, which allows for concurrent recognition of multiple analytes in a single sample, as shown in Fig. 14. Multiplexing on a single strip has been studied for detecting the dengue virus NS1 protein, dengue/chikungunya virus (IgG/IgM),74 antibodies against HIV-1 and -2, Mycobacterium,75 ebola virus, Zaire strain glycoprotein GP,76 anti-HCV IgG, anti-HIV IgG, anti-HAV IgG and IgM.77 Multiplexing with multiple strips has been studied for E. coli O157:H7, S. paratyphoid A, V. cholerae O139, S. paratyphoid C, S. paratyphoid B, S. typhi, S. enteritidis, S. chlorosis, V. cholerae O1, and V. parahaemolyticus,78 Influenza A and influenza B virus antigen,79Pseudomonas aeruginosa, and Staphylococcus aureus.79 Integration of lateral flow and microarray technologies was studied for identifying the malaria antigen plasmodium falciparum histidine-rich protein 2.80
 |
| Fig. 14 Diagram of a multiplexed lateral flow immunoassay to detect infectious disease biomarkers.81 | |
Optimally designed nanoparticles (size, shape, and materials) can also increase the assay's sensitivity by amplifying the signal generated by binding the analyte to the test line (Fig. 15). The most frequently used labeling agents in LFAs are antibodies combined with nanoparticles like gold nanoparticles, magnetic nanoparticles,82 carbon-based nanoparticles,83 silver nanoparticles,84 phosphor, fluorescent dyes, quantum dots,85 and up-converting86 nanomaterials. Gold nanoparticles are the most popular and widely employed for biosensing due to the visible (red) color they create through localized surface plasmon resonance.87,88 Aptamers have emerged as versatile alternatives to antibodies in bioanalysis applications due to their structural diversity, adaptability, and non-immunogenic nature. Aptamers offer flexibility in various assay formats and can be utilized for the detection of non-immunogenic and toxic targets. For instance, Shim et al.89 created aptamer-based lateral flow assays (LFAs) to detect aflatoxin B1. Cyanine 5-based fluorescence dye was attached to the aptamer to detect aflatoxin B1. By optimizing the assay, they achieved a limit of detection (LOD) of 0.1 ng mL−1. Fu et al.48 developed a highly responsive lateral flow immunoassay method for the specific detection of thrombin in clinical analysis. This method utilized gold nanoparticles (GNPs) and a pair of aptamer probes to enhance sensitivity. However, due to the higher cost associated with GNPs, researchers have explored using common dyes like blue dye as alternative labels for protein detection on LFAs90,91. Mao et al. (ref. 92) proposed latex beads instead of GNPs and a blue dye instead of an expensive fluorescent dye to construct the DNA-based LFAs. The LFA was demonstrated for human plasma and achieved a detection limit of 3.75 fmol L−1. In another study, Lee et al. (ref. 74) employed two-color latex beads to detect acute febrile illnesses (AFIs).
 |
| Fig. 15 (a) The schematic illustrates the overall design of an LFA test kit and its components (represented by colored boxes), which can be enhanced and improved. (b) In the test line, a sandwich complex is formed when the target molecule binds to the antibodies (left), peptides (middle), or aptamers (right) used as capture and detector agents.107 | |
mRNA (messenger RNA), an alternative reagent, has gained significant attention recently, particularly in molecular diagnostics.93 Rapid and precise detection of RNA targets, such as viral RNA or certain gene expressions, is a benefit of mRNA-based LFAs.94 Infectious disease diagnosis or gene expression95 profiling is made possible by capturing and detecting mRNA targets on the test line using complementary probes or primers. Similarly, DNA-based reagent LFAs are used to find specific DNA sequences or genetic variants. DNA targets can be identified and captured on the test line using DNA probes or primers. Applications made possible by this include genetic testing,96 pathogen detection,97 and the discovery of specific genetic variants linked to diseases.98 LFAs may also contain synthetic polymers to enhance assay performance.99 These polymers can improve the sample's flow dynamics,100 the test line's ability to bind more substances,101 or the stability of the assay's constituent parts. The sensitivity and dependability of LFAs can be improved by modifying the composition and characteristics of the synthetic polymers.99 Other reagent enzymes are essential components in many LFAs as they amplify signals102 and allow for visual or quantitative detection of the target analyte. Substrate molecules can be transformed by enzymes into a visible signal, such as a color shift or the production of fluorescence. Horseradish peroxidase and alkaline phosphatase are usual enzymes in LFAs.103 These enzymes can catalyze particular reactions that result in a measurable or visual signal, allowing for the sensitive detection of the target analyte. The choice of reagents in LFAs depends on the assay's specific requirements, including the target analyte's nature, the desired sensitivity, and the detection method employed. Researchers continuously explore and develop novel reagents and strategies to improve the performance and versatility of LFAs for a wide range of applications in diagnostics and biosensing.104–106
Researchers are also exploring the use of signal amplification methods, like enzyme-linked immunosorbent assay (ELISA) or PCR, to increase the sensitivity of LFAs.108 Santiago-Felipe et al. proposed recombinase polymerase amplification (RPA) with ELISA for screening common food safety threats, such as allergens, genetically modified organisms, pathogenic bacteria, and fungi. It was found that the technique had a satisfactory level of sensitivity and reproducibility. This technique eliminates the need for thermocycling and is cost-effective, making it an ideal method for resource-limited settings.109
Some research is being pursued on label-free detection methods. These are typically created on the assessment of differences in the physical or chemical properties of the test line, such as changes in refractive index, surface plasmon resonance or impedance, which can increase the sensitivity of the LFAs. Li et al.110 developed a new method that combines the lateral flow test strip technique with fluorescence immunoassay to detect avian influenza virus (AIV). This method does not require labels but uses a multi-functional nanocomposite to generate signals. This nanocomposite featured a unique combination of magnetic-adhesion-color-nanozyme properties, and it was able to detect the highly pathogenic Escherichia coli O157:H7 with limits of detection of 102 and 10 CFU mL−1 for colorimetric and catalytic quantitative analyses, respectively.111 Tasbasi et al.112 demonstrated LFA for whole-cell applications (Fig. 16) to detect Listeria monocytogenes. Finally, the integration of these technologies has allowed for more sophisticated data analysis, providing better insights into the causes of diseases and conditions.
 |
| Fig. 16 The assay principle is depicted schematically. L. monocytogenes cells move to the conjugation pad region to bind to aptamer gates. Peroxidase immobilized at the test region uses TMB from a nanopore to produce blue precipitates.112 | |
4.1 Sensitivity enhancement strategy
Lateral flow assay devices are popular for medical diagnostics, but their limited sensitivity can cause false-negative results. To improve their performance, sensitivity enhancement strategies have been developed, including modifications to the device design and optimization of assay parameters. The goal is to increase the detection limit and accurately detect low levels of analytes. Two alternative formats of enzyme-linked immunosorbent assays that can be incorporated into LFAs are sandwich LFAs and competitive LFAs. Even though each style has benefits and drawbacks, sandwich LFAs are more frequently employed than competitive LFAs.113 In sandwich-type LFAs, the target analyte is caught in a sandwich-like fashion between two distinct antibodies, often called the capture and detection antibodies. The target analyte in the sample is bound to the capture antibody. The detection (or recognition) antibody then attaches with a separate epitope on the target analyte, creating a “sandwich” complex.8 The detection antibody is tagged with an enzyme or another detectable marker (e.g., fluorescence dye). Thanks to the enzyme-linked detection antibody (or the fluorescent signals), the target analyte can be quantified. By utilizing two distinct antibodies to collect and detect the target analyte, the sandwich format enables the detection of low quantities of the analyte.114 Sandwich LFAs are appropriate for qualitative and quantitative assessments since they can cover a broad range of analyte concentrations. Different capture and detection antibody combinations can simultaneously detect several target analytes.6 Since the detection antibody does not directly compete with the analyte in the sample, potential interfering substances in the sample are less likely to affect the assay performance. A labeled analyte (the tracer) is created in a competitive LFA. The labeled analyte competes with the analyte present in the sample to bind to the immobilized capture antibody. The idea is that less tagged analyte will attach to the capture antibody if more analyte is present in the sample.8 The analyte amount in the sample has an inverse relationship with the signal that is detected. Since the labeled analyte competes with the analyte in the sample for binding to the capture antibody, competitive LFAs often have lesser sensitivity compared to sandwich LFAs.115
Competitive LFAs are better suited for qualitative or semi-quantitative measures rather than accurate quantification because they have a more constrained dynamic range.116 The competitive format necessitates optimizing several variables, such as the labeled analyte concentration, incubation periods, and the capture antibody to labeled analyte ratio.117 The development and interpretation of assays may be more difficult due to this intricacy. Considering the higher sensitivity, more comprehensive dynamic range, and more straightforward assay design, the sandwich LFA design is generally preferred over the competitive LFA design. However, it's worth noting that the preference for the LFA format varies according to the specific requirements of LFAs, including the target analyte, the desired sensitivity, and the available resources. Table 4 shows different approaches to enhance sensitivity and their LOD, and applications.
Table 4 Sensitivity enhancement strategies
Sensitivity enhancement strategy |
LOD without using the strategy |
LOD with using the strategy |
Application |
Reference |
Flow rate decrease |
5 nM |
0.5 nM |
Synthetic Zika virus |
118
|
100 ng mL−1 |
0.01 ng mL−1 |
Mouse IgG |
119
|
Preconcentration |
25 fg mL−1 |
100 pg mL−1 |
Valosin containing protein |
120
|
107 CFU mL−1 |
106 CFU mL−1 |
E. coli
|
121
|
Nanoparticle based signal enhancement |
8.4 × 10−2 pg μL−1 |
2.2 × 10−2 pg μL−1 |
E. coli
|
122
|
2000 pg mL−1 |
20 pg μL−1 |
PSA |
123
|
Alternative signal transduction (SERS/fluorescence/photoacoustic/thermal) |
∼10−14 |
8.2 × 10−19 M |
Biotin |
124
|
10 000 pfu mL−1 |
50 pfu mL−1 |
H1N1 |
125
|
1.1 ng mL−1 |
0.01 ng mL−1 |
Cryptococcal antigen (CrAg) from Cryptococcus |
17
|
4.2 Comparison of multiplexing strategies
Multiplexing is a valuable tool in diagnostic tests, enabling the simultaneous analysis of multiple analytes in a single sample. Multiplexing finds utility in various fields such as food, environment, and safety applications. Additionally, it helps conserve sample volume, time, and cost, which is particularly advantageous as diagnostic samples are often limited in volume.27 However, the widespread use of multiplexing in LFAs is hampered by several issues.126 Cross-reactivity, for instance, is a notable challenge as it can lead to non-specific binding, increased background noise, and the generation of false positive results. This phenomenon has been noted in different infectious diseases, including Flavivirus infections (such as dengue and Zika virus) as well as helminthic infections (like strongyloidiasis and filariasis). Interestingly, these infections often exhibit overlapping symptoms, further complicating the accurate detection and differentiation of specific biomarkers in the multiplexed LFAs.127,128 The quantity and variety of biomarkers that can be included in a single LFA are constrained by this restriction. Physical limitations arise from the inadequacy of multiplexing multiple test lines on a single LFA strip due to conjugate quantity, fluid flow variations, and bypassing multiple lines.129 Converting existing single-biomarker LFAs to multiplexed LFAs brings operational and quality assurance challenges, including patient and control population selection, calibration techniques, and process validation. The interpretation of multiple test results complicates LFAs, undermining their inherent simplicity. The proprietary nature of single-test LFA biomarkers hinders the sharing and teamwork required for developing multiplexed LFAs. Although promising prototypes have been developed in research, the translation of multiplexed LFAs into commercial devices remains limited,130 underscoring the necessity of addressing these issues through more research and development efforts.131
Recent research has seen the emergence of several intriguing reports of multiplexed LFA prototypes, demonstrating the possibility for simultaneous detection of several targets. Yen et al.76 proposed a multiplexed LFA that could simultaneously detect the viruses that cause dengue, yellow fever, and ebola by using multicolored silver nanoparticles (AgNPs) to create different colored test lines. Another cutting-edge design included a disc format with 10 distinct dipsticks,129 each lined with a different biomarker for detecting foodborne pathogens. Up-converting phosphor (UCP) particles were used in this design as the reporter to enable increased sensitivity and sample interference tolerance. The promise of multiplexed LFAs in the realm of immunology was also demonstrated by a multiplexed LFA that was created using UCP technology to detect several cytokines in leprosy. Lee et al. developed a multiplexed test with different viral antigens for diagnosing AIDS, hepatitis C and A (HCV and HAV), and HCV in one test strip.77 By enabling the simultaneous detection of several targets, these extraordinary developments in multiplexed LFAs emphasize the potential for increased diagnostic capabilities, leading to more efficient disease management and control. Table 5 shows the comparison of different multiplexing strategies for LFA devices.
Table 5 Comparison of multiplexing strategies
Strategy |
Transduction method |
Number of targets |
Biomarker detected |
Application |
Sample type |
LOD; enhancement |
Ref. |
Antibodies on multiple test lines |
SiO2@DQD |
2 |
HIgG, HIgM |
SARS and Cov-2 |
Human serum |
104-fold |
51
|
FAM and ROX fluorophores |
13 |
HPV (16, 18, 31, 33, 35, 39, 45, 51, 52, 56, 58, 59, and 68) |
Medical diagnostics, food safety testing, and environmental monitoring |
Human cervical swab |
No |
12
|
AuNPs |
3 |
miRNA-21, miRNA-155, miRNA-210, Giardia, Cryptosporidium, Entamoeba, HIV, HCV, and HAV antibodies |
Cancer, cardiovascular disease, and neurological disorders |
Human serum and stool |
No |
132
|
Microarray |
AuNPs |
4 |
Morphine, amphetamine, methamphetamine, and benzoylecgonine |
Monitoring of drug |
Human urine |
No |
133
|
Multiple strips |
UCP nanoparticles |
10 |
S. Paratyphi A, E. coli O157:H7, S. typhi, S. paratyphi B, S. paratyphi C, S. enteritidis, V. cholerae O1, S. choleraesuis, V. cholerae O139, and V. parahaemolyticus |
Dairy products, marine products, beverages, snacks, and meats |
Food samples |
No |
129
|
Multiple channels |
Catalytic signal amplification of AuNPs |
3 |
Clostridioides difficile toxins A and B and glutamate dehydrogenase (GDH) |
Clostridioides difficile infection |
Human stool |
8-fold |
134
|
Multiple dots |
AuNPs |
7 |
DNA alleles (GYPB*03, GYPB*04, FY*01, FY*02, FY02N.01, JK*01, and JK*02) related to four blood group SNPs |
Blood group detection |
Human whole blood |
No |
135
|
Multiple antibodies on a single test line |
AgNBA@Au, AgMB@Au, and AgR6G@Au SERS nanotags |
3 |
CK-MB, cTnI, and Myo cardiac biomarkers |
Myocardial infarction |
Human serum |
100-fold |
136
|
Red, orange, and green AgNPs |
3 |
ZEBOV glycoprotein, YFV NS1 protein and DENV NS1 protein |
Dengue, yellow fever, and ebola viruses |
Human serum |
No |
76
|
4.3 Application of LFA in various infectious diseases
Lateral flow assays (LFAs) are frequently utilized to diagnose different types of infectious diseases, such as viral, bacterial, and parasitic infections. By detecting a diverse range of biomarkers, such as antibodies, antigens, and nucleic acids, LFAs offer rapid and precise diagnosis of infectious diseases in various clinical settings. Table 6 compares the metrics relevant to LFAs applied to detect contagious diseases.
Table 6 LFA applied to various infectious diseases
Pathogen type |
Pathogen name |
Type of approach/detection element |
Analysis time |
LOD |
Sensitivity |
Type of LFA |
Recognition element/reporter (DNA/RNA/protein/aptamer/small molecule) |
Reference |
Virus |
COVID-19 |
Nucleic acid |
30 min |
1 Ag of the N-gene RNA |
— |
RT-LAMP colorimetric |
RNA |
137
|
36 min |
200 copies per mL |
— |
Colorimetric |
FeS2 nanozyme strips |
138
|
60 min |
200 pM |
— |
Fluorescent |
AIEgen@GO |
139
|
90 min |
2000 copies per mL |
— |
Catalytic hairpin assembly (CHA) reaction |
DNA-hairpin |
140
|
— |
5 Ag per μL |
— |
Electrochemical |
Universal DNA-hairpin (UDH) |
141
|
Antibody |
8 min |
100 fg mL−1 |
— |
SERS |
AuNPs |
24
|
10 min |
IgG 5 ng mL−1 |
93.33% |
Colorimetric |
Selenium nanoparticle-modified SARS-CoV-2 nucleoprotein |
142
|
IgM 20 ng mL−1 |
10 min |
— |
IgG 98.72% |
Fluorescent |
Eu(III) fluorescent microsphere |
143
|
IgM 98.68% |
10 min |
— |
IgG 100% |
Colorimetric |
AuNPs |
144
|
IgM 92% |
15 min |
IgG 0.1 ng mL−1 |
— |
SERS |
Gap enhancement nanotags |
145
|
IgM 1 ng mL−1 |
Antigen |
10 min |
— |
100% |
Fluorescent |
Europium(III) chelate microparticles |
146
|
10 min |
1.6–2.2 ng mL−1 |
— |
Fluorescent |
UCNPs@mSiO2 |
147
|
16 min |
0.1 ng mL−1 |
— |
Chemiluminescent |
CoeFe@hemin-peroxidase nanoenzyme |
148
|
20 min |
10 pg mL−1 |
— |
Fluorescent |
Carbon dot-based silica spheres |
149
|
20 min |
3.03 ng mL−1 |
— |
Colorimetric |
Carboxylic red latex beads |
150
|
HIV |
Nucleic acid |
15 min |
0.76 pM |
— |
Fluorescent |
QDs |
151
|
15 min |
0.24 pg mL−1 |
— |
SERS |
Surface modified AuNP by MGITC |
48
|
15 min |
0.1 nM |
— |
Colorimetric |
Oligonucleotide-linked AuNPs |
152
|
|
20 min |
0.5–13 log10 copies per mL (50 copies of gag RNA) |
— |
Colorimetric |
AuNPs |
153
|
<60 min |
0.3 fm |
— |
CRISPR-cas12a mediated SERS |
SiO2@PEI NPs |
154
|
Antibody |
20 min |
0.11 NCU mL−1 |
— |
Fluorescent |
QBs |
155
|
30 min |
— |
100% |
Proteinticle probe-based colorimetric |
AuNPs |
156
|
20–40 min |
|
96.6% |
Colorimetric |
UCNPs |
157
|
Antigen |
18 s |
0.0064 ng mL−1 |
— |
Amperometric |
AuNP-MWCNTs-AEP |
158
|
15 min |
— |
100% |
Colorimetric |
AuNPs |
159
|
20 min |
8 pg mL−1 |
— |
Colorimetric |
PtNPs |
160
|
40 min |
30 pg mL−1 |
— |
MICT |
AuNPs |
161
|
40 min |
50 pg mL−1 |
90% |
Colorimetric |
Carbon NPs |
162
|
Synthetic Zika virus |
Nucleic acid |
1.5–15 min |
0.5 nM |
— |
Electro-spin coating and colorimetric |
Hydrophobic PCL nanofibers |
118
|
15 min |
1 copy per μL |
— |
RT-LAMP smartphone |
Microfluidic chips |
163
|
Antibody |
— |
1.905 μg mL−1 |
— |
SERS |
Si–AuNPs |
164
|
20 min |
10 pg mL−1 |
— |
Colorimetric |
Silanized carbon dots |
165
|
Antigen |
20 min |
0.045 ng mL−1 |
— |
Fluorescent |
Quantum dot microspheres |
166
|
Influenza A |
Antibody |
10 min |
0.25 HA units |
— |
Colorimetric |
AuNPs |
167
|
20 min |
— |
78.57% |
Colorimetric |
Streptavidin-coated gold colloid |
168
|
30 min |
50 pfu mL−1 |
— |
SERS |
Fe3O4@Ag magnetic tags |
169
|
35 min |
22 pfu mL−1 |
— |
Fluorescent |
MQBs |
170
|
Antigen |
15 min |
50 pfu mL−1 |
— |
Fluorescent |
QDs |
155
|
30 min |
250 ng mL−1 |
— |
Fluorescent |
Cy5 doped silica nanoparticles |
171
|
Influenza B |
Antibody |
20 min |
— |
87.50% |
Colorimetric |
Streptavidin-coated gold colloid |
168
|
30 min |
0.55 μg |
— |
Fluorescent |
Cy5-loaded silica nanoparticles |
172
|
Avian influenza |
Antibody |
10 min |
0.09 ng mL−1 |
— |
Fluorescent |
QDs-AuNPs |
108
|
Influenza |
Enzyme |
15 min |
995 TCID50 per mL |
— |
Bio chemiluminescent |
Luciferin derivatized substrate |
173
|
Dengue |
Nucleic acid |
20 min |
Cut-off value of 1.2, 104 pfu mL−1 |
— |
Colorimetric |
Dextrin-capped AuNPs |
174
|
Antibody |
15 min |
512 pfu |
— |
Colorimetric |
AuNPs |
175
|
— |
150 ng mL−1 |
— |
Colorimetric |
AgNPs |
155
|
Antigen |
10 min |
4.9 ng mL−1 |
— |
Colorimetric |
Gold decorated graphene oxide sheets |
176
|
25 min |
0.1 ng mL−1 (DENV 1) |
— |
Magneto enzyme LFA-colorimetric |
Carboxyl-adembeads |
177
|
0.25 ng mL−1 (DENV 2,3) |
1 ng mL−1 (DENV 4) |
30 min |
7.67 ng mL−1 |
— |
SERS |
Gold nanostars |
178
|
Hepatitis A and C |
Antigen |
30 min |
— |
100% |
Colorimetric |
AuNPs |
77
|
Hepatitis B |
Nucleic acid |
7 min |
7.23 pM |
— |
Colorimetric |
AuNPs |
179
|
Antibody |
10 min |
60 mIU mL−1 |
99.19% |
Colorimetric |
Up-converting phosphor |
180
|
20 min |
2.5–10.0 IU HBV surface antigen per mL |
— |
Fluorescent |
DNA-fluoro-max fluorescent nanoparticles |
181
|
Antigen |
10 min |
0.05 ng mL−1 |
— |
Fluorescent |
ZnSe/CdSe/CdS/Cd xZn1–xS/ZnS QDs |
182
|
15 min |
75 pg mL−1 |
— |
Colorimetric |
Carboxyl modified CdSe/ZnS QDs |
183
|
Bacteria |
Staphylococcus aureus
|
Antibody |
12 min (testing) + 10 min (chromatography) |
104 cells per mL |
— |
Colorimetric |
Vancomycin (Van)-modified SiO2–Au-QD tags |
184
|
100 cells per mL |
— |
Fluorescent |
Foodborne bacteria |
Antibodies |
20 min |
10 CFU/0.6 mg |
— |
TC-UPT-LF |
UCP |
129
|
Clostridioides difficile |
Antibody |
10 min |
0.16 ng mL−1 |
— |
mPAD |
Glutamate dehydrogenase |
185
|
0.09 ng mL−1 |
Toxin A |
0.03 ng mL−1 |
Toxin B |
Vibrio cholerae
|
Antibody |
10 min |
5 ng mL−1 (3σ) |
— |
Colorimetric |
Aptamer coated AuNPs |
186
|
10 ng mL−1 (visual) |
— |
20 min |
0.6 ng mL−1 (3σ) |
— |
1 ng mL−1 (visual) |
— |
Salmonella enteritidis |
Phage-antibody |
30 min |
7.06 CFU mL−1 |
— |
SERS + colorimetric |
DTNB-AuNPs |
187
|
15 min |
4.1 × 106 CFU mL−1 |
— |
Colorimetric |
Phage |
188
|
Salmonella typhimurium
|
Antibody |
10 min |
75 cfu mL−1 |
— |
SERS |
4-MBA-modified AuNPs |
189
|
15 min |
75 cfu mL−1 |
— |
Magnetic |
AuNR@Pt |
190
|
50 cfu mL−1 |
Colorimetric |
35 min |
30 cells per mL |
— |
Ultrasensitive fluorescent |
Mag@QDs-WGA |
191
|
15 min |
50 cells per mL |
— |
Fluorescent |
Si@DQD |
192
|
90 min |
8.6 × 100 CFU mL−1 (pure culture) |
— |
Colorimetric |
Multifold AuNPs |
193
|
4.1 × 102 CFU mL−1 (real sample) |
Cronobacter sakazakii
|
Antibody |
3 h |
103 cfu mL−1 |
— |
Colorimetric |
AuNPs |
194
|
Mycobacterium tuberculosis
|
Nucleic acid |
20 min |
25 fg |
100% |
Colorimetric |
— |
195
|
75 min |
— |
60% |
Colorimetric |
— |
196
|
Streptococcus pneumoniae
|
Nucleic acid |
2–10 min |
25 fg μL−1 |
— |
Colorimetric |
AUDG |
197
|
Antigen |
15 min |
— |
82.8% |
Colorimetric |
Antibody |
198
|
5. Challenges in incorporating new technologies and reagents in LFA design
Incorporating new technologies and reagents into LFA design can be a challenging process. One of the primary challenges is achieving sufficient sensitivity for the target analyte.199 New technologies and reagents may require more complex detection systems than traditional LFAs, which can be costly and challenging to implement.131 Additionally, as LFAs become more complex, they become more prone to errors and can be more expensive to manufacture. Integrating new components into existing LFA platforms requires careful optimization and integration, which can be time-consuming and costly if the components are not compatible.113 Environmental conditions such as humidity, temperature, and light can also impact new components' stability, posing additional challenges.200 Before any new technology or reagent can be approved for use in a diagnostic assay, it must undergo a thorough evaluation for safety and efficacy, which can be lengthy and costly. Despite these challenges, the successful implementation of new technologies and reagents in LFAs can offer significant benefits. Collaboration between scientists, engineers, and regulatory experts is crucial to ensuring that the assay is effective, reliable, and safe for use in clinical settings.
5.1 Complexity of operation
The complexity of the operation is another critical challenge that can arise when incorporating new technologies and reagents into LFA design.199 LFAs may require extensive user training to ensure proper assay performance as they become more complex. This can be difficult in point-of-care settings where trained personnel may not be available. Additionally, new technologies and reagents may require additional assay steps, increasing the complexity and time needed to perform the assay, leading to increased costs and reduced throughput. Interpreting assay results may also become more complex with integrating new technologies and reagents, which can be challenging for non-expert users.201
In some cases, specialized instrumentation may be necessary for implementing new technologies and reagents in LFAs, further adding to the complexity and cost of the assay. Integrating new technologies and reagents into existing LFA platforms requires careful integration and optimization, which requires significant engineering expertise.202 Addressing the complexity of operation requires careful assay design, user-friendly interfaces, and robust quality control measures to ensure reliable and accurate results. Therefore, managing the complexity of the operation is critical for successfully implementing new technologies and reagents in LFA design.
5.2 Potential for false positives
The potential for false positives is a critical challenge that can arise when incorporating new technologies and reagents into LFA design. Some new reagents or technologies may cross-react with other substances in the sample, leading to false positive results.203 This can be particularly problematic in complex samples like blood or urine. In addition, some new reagents may bind non-specifically to LFA components, leading to false positives that can be difficult to detect and correct in the assay design.204 Contamination of LFA components or the sample can also lead to false positives, which can be challenging to prevent in low-resource settings or areas with poor laboratory infrastructure.
Furthermore, some new technologies or reagents may lack the specificity to distinguish between the target analyte and related compounds, leading to false positive results.205 The sample matrix can also impact the potential for false positives, as high levels of interfering substances can increase the likelihood of false positives.206 Addressing the potential for false positives requires careful assay design and validation, including appropriate controls and optimization of assay conditions. Thorough characterization of the target analyte and potential interferents is also necessary to minimize the potential for false positives. Thus, addressing the challenge of potential false positives is expected to improve the accuracy and reliability of LFA design.
6. Conclusion
New technologies and reagents can potentially improve the accuracy and sensitivity of lateral flow assays (LFAs). Surface enhanced Raman spectroscopy (SERS) and photoacoustic techniques can enhance the sensitivity of LFAs, while fluorescence resonance energy transfer (FRET) can enhance specificity. Integrating smartphones and thermal readers can provide quantitative measurements and improve the accuracy of LFAs. However, incorporating these technologies into LFA design can present significant challenges, such as higher cost, complexity of operation, and potential for false positives. Careful assay design and validation, appropriate controls, and optimization of assay conditions are necessary to minimize the potential for false positives.207 LFA development is increasingly driven by consumer needs, creating user-friendly technologies that enable easier testing by untrained individuals208–210 Incorporating artificial intelligence and machine learning can help automate the interpretation of test results, making LFAs more accessible to the general population.211 LFA technology innovation extends beyond healthcare.212 Enhancements in accuracy and sensitivity can increase their adoption in other areas, resulting in safer environments, reliable food testing, and effective forensic investigations. Continued innovation in LFA technology is vital for improving diagnostic tools, expanding their applications, and ultimately enhancing human health and well-being.
Data availability
The data used in the study are available from the corresponding author upon reasonable request.
Author contributions
Conceptualization: M. R. G., M. K. D.; data curation: M. K. D., M. I.; funding acquisition: M. R. G., R. D.; methodology: M. R. G., M. K. D., R. D.; project administration: M. R. G.; resources: M. R. G., R. D.; supervision: M. R. G., R. D.; writing – original draft: M. K. D., M. I.; writing – review & editing: M. R. G., M. K. D., M. I., R. D.
Conflicts of interest
The authors declare no competing financial interests.
Acknowledgements
M. R. G. acknowledges support from the National Science Foundation (NSF CAREER award number: 2045640). R. D. and M. K. D. acknowledge support from the National Institute of General Medical Sciences (NIGMS) of the National Institutes of Health under award number R15GM141653-01.
References
- N. A. Taranova,
et al., ‘Traffic light’ immunochromatographic test based on multicolor quantum dots for the simultaneous detection of several antibiotics in milk, Biosens. Bioelectron., 2015, 63, 255–261 CrossRef CAS PubMed.
- P. K. Drain,
et al., Diagnostic point-of-care tests in resource-limited settings, Lancet Infect. Dis., 2014, 14(3), 239–249 CrossRef PubMed.
- C. M. Plotz and J. M. Singer, The latex fixation test. Application to the serologic diagnosis of rheumatoid arthritis, Am. J. Med., 1956, 21(6), 888–892 CrossRef CAS PubMed.
- P. Yager, G. J. Domingo and J. Gerdes, Point-of-care diagnostics for global health, Annu. Rev. Biomed. Eng., 2008, 10, 107–144 CrossRef CAS PubMed.
- J. L. Vaitukaitis, G. D. Braunstein and G. T. Ross, A radioimmunoassay which specifically measures human chorionic gonadotropin in the presence of human luteinizing hormone, Am. J. Obstet. Gynecol., 1972, 113(6), 751–758 CrossRef CAS PubMed.
- M. Sajid, A.-N. Kawde and M. Daud, Designs, formats and applications of lateral flow assay: a literature review, J. Saudi Chem. Soc., 2015, 19(6), 689–705 CrossRef.
- C. Parolo,
et al., Tutorial: design and fabrication of nanoparticle-based lateral-flow immunoassays, Nat. Protoc., 2020, 15(12), 3788–3816 CrossRef CAS PubMed.
- E. B. Bahadır and M. K. Sezgintürk, Lateral flow assays: principles, designs and labels, TrAC, Trends Anal. Chem., 2016, 82, 286–306 CrossRef.
- K. Neubauerova,
et al., Nanocellulose-based biosensor for colorimetric detection of glucose, Sens. Bio-Sens. Res., 2020, 29, 100368 CrossRef.
- P. K. Kulabhusan,
et al., Lateral flow assay for rapid detection of white spot syndrome virus (WSSV) using a phage-displayed peptide as bio-recognition probe, Appl. Microbiol. Biotechnol., 2017, 101(11), 4459–4469 CrossRef CAS PubMed.
- N. Wang,
et al., Recent advances in the rapid detection of microRNA with lateral flow assays, Biosens. Bioelectron., 2022, 211, 114345 CrossRef CAS PubMed.
- Y. Xu,
et al., Fluorescent probe-based lateral flow assay for multiplex nucleic acid detection, Anal. Chem., 2014, 86(12), 5611–5614 CrossRef CAS PubMed.
- J. Chen,
et al., A facile fluorescence lateral flow biosensor for glutathione detection based on quantum dots-MnO2 nanocomposites, Sens. Actuators, B, 2018, 260, 770–777 CrossRef CAS.
- C. Srisomwat,
et al., Amplification-free DNA sensor for the one-step detection of the hepatitis B virus using an automated paper-based lateral flow electrochemical device, Anal. Chem., 2020, 93(5), 2879–2887 CrossRef PubMed.
- V. Gubala,
et al., Point of care diagnostics: status and future, Anal. Chem., 2012, 84(2), 487–515 CrossRef CAS PubMed.
- Y. Wang,
et al., Thermal contrast amplification reader yielding 8-fold analytical improvement for disease detection with lateral flow assays, Anal. Chem., 2016, 88(23), 11774–11782 CrossRef CAS PubMed.
- Y. Zhao,
et al., Nanoparticle-based photoacoustic analysis for highly sensitive lateral flow assays, Nanoscale, 2016, 8(46), 19204–19210 RSC.
- S. Song,
et al., Highly sensitive paper-based immunoassay using photothermal laser speckle imaging, Biosens. Bioelectron., 2018, 117, 385–391 CrossRef CAS PubMed.
- A. Ojaghi,
et al., High-sensitivity interpretation of lateral flow immunoassays using thermophotonic lock-in imaging, Sens. Actuators, A, 2018, 273, 189–196 CrossRef CAS.
- G. Dastgir,
et al., Surface-enhanced Raman spectroscopy of polymerase chain reaction (PCR) products of Rifampin resistant and susceptible tuberculosis patients, Photodiagn. Photodyn. Ther., 2022, 38, 102758 CrossRef CAS PubMed.
- A. Moyano,
et al., Magnetic lateral flow immunoassays, Diagnostics, 2020, 10(5), 288 CrossRef CAS PubMed.
- X. Huang,
et al., Membrane-based lateral flow immunochromatographic strip with nanoparticles as reporters for detection: a review, Biosens. Bioelectron., 2016, 75, 166–180 CrossRef CAS PubMed.
- D. Hong,
et al., Electrochemiluminescence-incorporated lateral flow immunosensors using Ru (bpy) 32+-labeled gold nanoparticles for the full-range detection of physiological C-reactive protein levels, Anal. Chem., 2021, 93(22), 7925–7932 CrossRef CAS PubMed.
- S. Srivastav,
et al., Rapid and sensitive SERS-based lateral flow test for SARS-CoV2-specific IgM/IgG antibodies, Anal. Chem., 2021, 93(36), 12391–12399 CrossRef CAS PubMed.
- Z. Li,
et al., Rapid and sensitive detection of protein biomarker using a portable fluorescence biosensor based on quantum dots and a lateral flow test strip, Anal. Chem., 2010, 82(16), 7008–7014 CrossRef CAS PubMed.
- S. L. Znoyko,
et al., Ultrasensitive quantitative detection of small molecules with rapid lateral-flow assay based on high-affinity bifunctional ligand and magnetic nanolabels, Anal. Chim. Acta, 2018, 1034, 161–167 CrossRef CAS PubMed.
- A. Sena-Torralba,
et al., Toward Next Generation Lateral Flow Assays: Integration of Nanomaterials, Chem. Rev., 2022, 122(18), 14881–14910 CrossRef CAS PubMed.
- Y. Jung,
et al., Smartphone-based lateral flow imaging system for detection of food-borne bacteria E.coli O157:H7, J. Microbiol. Methods, 2020, 168, 105800 CrossRef CAS PubMed.
- D. Quesada-González,
et al., Signal enhancement on gold nanoparticle-based lateral flow tests using cellulose nanofibers, Biosens. Bioelectron., 2019, 141, 111407 CrossRef PubMed.
- P. Brangel,
et al., A serological point-of-care test for the detection of IgG antibodies against Ebola virus in human survivors, ACS Nano, 2018, 12(1), 63–73 CrossRef CAS PubMed.
- B. S. Miller,
et al., Quantifying biomolecular binding constants using video paper analytical devices, Chem.–Eur. J., 2018, 24(39), 9783–9787 CrossRef CAS PubMed.
- A. N. Danthanarayana,
et al., A multicolor multiplex lateral flow assay for high-sensitivity analyte detection using persistent luminescent nanophosphors, Anal. Methods, 2020, 12(3), 272–280 RSC.
- Z. Liu,
et al., A smartphone-based dual detection mode device integrated with two lateral flow immunoassays for multiplex mycotoxins in cereals, Biosens. Bioelectron., 2020, 158, 112178 CrossRef CAS PubMed.
- S. Zhang,
et al., Upconversion luminescence nanoparticles-based immunochromatographic assay for quantitative detection of triamcinolone acetonide in cosmetics, Spectrochim. Acta, Part A, 2019, 214, 302–308 CrossRef CAS PubMed.
- Y. Gong,
et al., A portable and universal upconversion nanoparticle-based lateral flow assay platform for point-of-care testing, Talanta, 2019, 201, 126–133 CrossRef CAS PubMed.
- B. Jin,
et al., Lateral flow aptamer assay integrated smartphone-based portable device for simultaneous detection of multiple targets using upconversion nanoparticles, Sens. Actuators, B, 2018, 276, 48–56 CrossRef.
- V. Tran,
et al., Rapid, quantitative, and ultrasensitive point-of-care testing: a portable SERS reader for lateral flow assays in clinical chemistry, Angew. Chem., Int. Ed., 2019, 58(2), 442–446 CrossRef CAS PubMed.
- Y.-J. Zhang,
et al., A miniaturized photoacoustic device with laptop readout for point-of-care testing of blood glucose, Talanta, 2020, 209, 120527 CrossRef CAS PubMed.
- Z. Qu,
et al., A plasmonic thermal sensing based portable device for lateral flow assay detection and quantification, Nanoscale Res. Lett., 2020, 15(1), 1–12 CrossRef PubMed.
- T. Bai,
et al., Functionalized Au@ Ag-Au nanoparticles as an optical and SERS dual probe for lateral flow sensing, Anal. Bioanal. Chem., 2018, 410, 2291–2303 CrossRef CAS PubMed.
- S. Choi,
et al., Quantitative analysis of thyroid-stimulating hormone (TSH) using SERS-based lateral flow immunoassay, Sens. Actuators, B, 2017, 240, 358–364 CrossRef CAS.
- Z. Cheng,
et al., Simultaneous detection of dual prostate specific antigens using surface-enhanced Raman scattering-based immunoassay for accurate diagnosis of prostate cancer, ACS Nano, 2017, 11(5), 4926–4933 CrossRef CAS PubMed.
- J. Hwang, S. Lee and J. Choo, Application of a SERS-based lateral flow immunoassay strip for the rapid and sensitive detection of staphylococcal enterotoxin B, Nanoscale, 2016, 8(22), 11418–11425 RSC.
- W. Maneeprakorn,
et al., Surface-enhanced Raman scattering based lateral flow immunochromatographic assay for sensitive influenza detection, RSC Adv., 2016, 6(113), 112079–112085 RSC.
- H. J. Park, S. C. Yang and J. Choo, Early diagnosis of influenza virus A using surface-enhanced Raman scattering-based lateral flow assay, Bull. Korean Chem. Soc., 2016, 37(12), 2019–2024 CrossRef CAS.
- M. Sánchez-Purrà,
et al., Surface-enhanced Raman spectroscopy-based sandwich immunoassays for multiplexed detection of Zika and Dengue viral biomarkers, ACS Infect. Dis., 2017, 3(10), 767–776 CrossRef PubMed.
- G. Wang,
et al., Rotationally induced hydrodynamics: fundamentals and applications to high-speed bioassays, Annu. Rev. Anal. Chem., 2010, 3(1), 387 CrossRef CAS PubMed.
- X. Fu,
et al., A SERS-based lateral flow assay biosensor for highly sensitive detection of HIV-1 DNA, Biosens. Bioelectron., 2016, 78, 530–537 CrossRef CAS PubMed.
- J. F. McClelland and R. N. Kniseley, Photoacoustic spectroscopy with condensed samples, Appl. Opt., 1976, 15(11), 2658–2663 CrossRef CAS PubMed.
- E. I. Galanzha,
et al., In vivo magnetic enrichment and multiplex photoacoustic detection of circulating tumour cells, Nat. Nanotechnol., 2009, 4(12), 855–860 CrossRef CAS PubMed.
- C. Wang,
et al., Development of spike protein-based fluorescence lateral flow assay for the simultaneous detection of SARS-CoV-2 specific IgM and IgG, Analyst, 2021, 146(12), 3908–3917 RSC.
- S. H. Kim,
et al., Specific detection of avian influenza H5N2 whole virus particles on lateral flow strips using a pair of sandwich-type aptamers, Biosens. Bioelectron., 2019, 134, 123–129 CrossRef CAS PubMed.
- Z. Rong,
et al., Integrated fluorescent lateral flow assay platform for point-of-care diagnosis of infectious diseases by using a multichannel test cartridge, Sens. Actuators, B, 2021, 329, 129193 CrossRef CAS.
- Z. Qin and J. C. Bischof, Thermophysical and biological responses of gold nanoparticle laser heating, Chem. Soc. Rev., 2012, 41(3), 1191–1217 RSC.
- Z. Qin,
et al., Significantly improved analytical sensitivity of lateral flow immunoassays by using thermal contrast, Angew. Chem., Int. Ed. Engl., 2012, 51(18), 4358–4361 CrossRef CAS PubMed.
- D. R. Boulware,
et al., Multisite validation of cryptococcal antigen lateral flow assay and quantification by laser thermal contrast, Emerging Infect. Dis., 2014, 20(1), 45–53 CrossRef PubMed.
- C. Marquina,
et al., GMR sensors and magnetic nanoparticles for immuno-chromatographic assays, J. Magn. Magn. Mater., 2012, 324(21), 3495–3498 CrossRef CAS.
- A. V. Orlov,
et al., Multiplex biosensing based on highly sensitive magnetic nanolabel quantification: rapid detection of botulinum neurotoxins A, B, and E in liquids, Anal. Chem., 2016, 88(21), 10419–10426 CrossRef CAS PubMed.
- V.-T. Nguyen,
et al., Recent advances in high-sensitivity detection methods for paper-based lateral-flow assay, Biosens. Bioelectron., 2020, 152, 112015 CrossRef CAS PubMed.
- J. Park, A giant magnetoresistive reader platform for quantitative lateral flow immunoassays, Sens. Actuators, A, 2016, 250, 55–59 CrossRef CAS.
- Y. Ryu,
et al., Increase in the detection sensitivity of a lateral flow assay for a cardiac marker by oriented immobilization of antibody, BioChip J., 2011, 5, 193–198 CrossRef CAS.
- W. Shen,
et al., Detection of DNA labeled with magnetic nanoparticles using MgO-based magnetic tunnel junction sensors, J. Appl. Phys., 2008, 103(7), 07A306 CrossRef.
- H. Lei,
et al., Contactless measurement of magnetic nanoparticles on lateral flow strips using tunneling magnetoresistance (TMR) sensors in differential configuration, Sensors, 2016, 16(12), 2130 CrossRef PubMed.
- M. P. Nikitin,
et al., Ultrasensitive detection enabled by nonlinear magnetization of nanomagnetic labels, Nanoscale, 2018, 10(24), 11642–11650 RSC.
- A. V. Orlov,
et al., Rapid dry-reagent immunomagnetic biosensing platform based on volumetric detection of nanoparticles on 3D structures, Biosens. Bioelectron., 2016, 79, 423–429 CrossRef CAS PubMed.
- D. J. You, T. S. Park and J.-Y. Yoon, Cell-phone-based measurement of TSH using Mie scatter optimized lateral flow assays, Biosens. Bioelectron., 2013, 40(1), 180–185 CrossRef CAS PubMed.
- Y. Jung,
et al., Smartphone-based lateral flow imaging system for detection of food-borne bacteria E. coli O157: H7, J. Microbiol. Methods, 2020, 168, 105800 CrossRef CAS PubMed.
- Z. Rong,
et al., Smartphone-based fluorescent lateral flow immunoassay platform for highly sensitive point-of-care detection of Zika virus nonstructural protein 1, Anal. Chim. Acta, 2019, 1055, 140–147 CrossRef CAS PubMed.
- G. M. Ross,
et al., Rapid antibody selection using surface plasmon resonance for high-speed and sensitive hazelnut lateral flow prototypes, Biosensors, 2018, 8(4), 130 CrossRef CAS PubMed.
- L. Wang,
et al., SERS-based test strips: principles, designs and applications, Biosens. Bioelectron., 2021, 189, 113360 CrossRef CAS PubMed.
- T. Mu,
et al., High-sensitive smartphone-based Raman system based on cloud network architecture, IEEE J. Sel. Top. Quantum Electron., 2018, 25(1), 1–6 Search PubMed.
- K. Mohd Hanafiah,
et al., Development of Multiplexed Infectious Disease Lateral Flow Assays: Challenges and Opportunities, Diagnostics, 2017, 7(3), 51 CrossRef PubMed.
- J. Gantelius,
et al., A lateral flow protein microarray for rapid determination of contagious bovine pleuropneumonia status in bovine serum, J. Microbiol. Methods, 2010, 82(1), 11–18 CrossRef CAS PubMed.
- S. Lee, S. Mehta and D. Erickson, Two-color lateral flow assay for multiplex detection of causative agents behind acute febrile illnesses, Anal. Chem., 2016, 88(17), 8359–8363 CrossRef CAS PubMed.
- P. L. Corstjens,
et al., Rapid assay format for multiplex detection of humoral immune responses to infectious disease pathogens (HIV, HCV, and TB), Ann. N. Y. Acad. Sci., 2007, 1098(1), 437–445 CrossRef CAS PubMed.
- C.-W. Yen,
et al., Multicolored silver nanoparticles for multiplexed disease diagnostics: distinguishing dengue, yellow fever, and Ebola viruses, Lab Chip, 2015, 15(7), 1638–1641 RSC.
- J.-H. Lee,
et al., Multiplex diagnosis of viral infectious diseases (AIDS, hepatitis C, and hepatitis A) based on point of care lateral flow assay using engineered proteinticles, Biosens. Bioelectron., 2015, 69, 213–225 CrossRef CAS PubMed.
- Y. Zhao,
et al., ZnO-nanorods/graphene heterostructure: a direct electron transfer glucose biosensor, Sci. Rep., 2016, 6(1), 1–7 CrossRef PubMed.
- A. C. Cazacu,
et al., Comparison of a new lateral-flow chromatographic membrane immunoassay to viral culture for rapid detection and differentiation of influenza A and B viruses in respiratory specimens, J. Clin. Microbiol., 2004, 42(8), 3661–3664 CrossRef CAS PubMed.
- G. E. Fridley, H. Le and P. Yager, Highly sensitive immunoassay based on controlled rehydration of patterned reagents in a 2-dimensional paper network, Anal. Chem., 2014, 86(13), 6447–6453 CrossRef CAS PubMed.
- H. Kim, D.-R. Chung and M. Kang, A new point-of-care test for the diagnosis of infectious diseases based on multiplex lateral flow immunoassays, Analyst, 2019, 144(8), 2460–2466 RSC.
- G. A. Pietersz,
et al., Therapeutic targeting in nanomedicine: the future lies in recombinant antibodies, Nanomedicine, 2017, 12(15), 1873–1889 CrossRef CAS PubMed.
- P. F. Mens,
et al., Molecular diagnosis of malaria in the field: development of a novel 1-step nucleic acid lateral flow immunoassay for the detection of all 4 human Plasmodium spp. and its evaluation in Mbita, Kenya, Diagn. Microbiol. Infect. Dis., 2008, 61(4), 421–427 CrossRef CAS PubMed.
- H. Li and D. Xu, Silver nanoparticles as labels for applications in bioassays, TrAC, Trends Anal. Chem., 2014, 61, 67–73 CrossRef CAS.
- Y. Wang,
et al., Quantum-dot-based lateral flow immunoassay for the rapid detection of crustacean major allergen tropomyosin, Food Control, 2019, 106, 106714 CrossRef CAS.
- N. Kang,
et al., Facile synthesis of upconversion nanoparticles with high purity using lanthanide oleate compounds, Nanotechnology, 2018, 29(7), 075601 CrossRef PubMed.
- P. Valentini,
et al., Gold-nanoparticle-based colorimetric discrimination of cancer-related point mutations with picomolar sensitivity, ACS Nano, 2013, 7(6), 5530–5538 CrossRef CAS PubMed.
- A. L. Garner,
et al., Development of a high-throughput screen and its use in the discovery of Streptococcus pneumoniae immunoglobulin A1 protease inhibitors, J. Am. Chem. Soc., 2013, 135(27), 10014–10017 CrossRef CAS PubMed.
- W.-B. Shim,
et al., An aptamer-based dipstick assay for the rapid and simple detection of aflatoxin B1, Biosens. Bioelectron., 2014, 62, 288–294 Search PubMed.
- R. Greenwald,
et al., Improved serodetection of Mycobacterium bovis infection in badgers (Meles meles) using multiantigen test formats, Diagn. Microbiol. Infect. Dis., 2003, 46(3), 197–203 CrossRef CAS PubMed.
- S. Zhao,
et al., State of the art: lateral flow assay (LFA) biosensor for on-site rapid detection, Chin. Chem. Lett., 2018, 29(11), 1567–1577 CrossRef CAS.
- X. Mao, W. Wang and T. E. Du, Dry-reagent nucleic acid biosensor based on blue dye doped latex beads and lateral flow strip, Talanta, 2013, 114, 248–253 CrossRef CAS PubMed.
- I. Kohaar,
et al., A urine exosome gene expression panel distinguishes between indolent and aggressive prostate cancers at biopsy, J. Urol., 2021, 205(2), 420–425 CrossRef PubMed.
- T. R. Damase,
et al., The limitless future of RNA therapeutics, Front. Bioeng. Biotechnol., 2021, 161 Search PubMed.
- N. Sayed,
et al., Gene therapy: comprehensive overview and therapeutic applications, Life Sci., 2022, 120375 CrossRef CAS PubMed.
- X. Liu,
et al., Multiple SNPs detection based on lateral flow assay for phenylketonuria diagnostic, Anal. Chem., 2018, 90(5), 3430–3436 CrossRef CAS PubMed.
- W. Wu,
et al., Research advances of DNA aptasensors for foodborne pathogen detection, Crit. Rev. Food Sci. Nutr., 2020, 60(14), 2353–2368 CrossRef CAS PubMed.
- G. H. Lopez,
et al., A D+ blood donor with a novel RHD* D-CE (5-6)-D gene variant exhibits the low-frequency antigen RH23 (DW) characteristic of the partial DVa phenotype, Transfusion, 2016, 56(9), 2322–2330 CrossRef CAS PubMed.
- J. T. Heggestad,
et al., In pursuit of zero 2.0:
recent developments in nonfouling polymer brushes for immunoassays, Adv. Mater., 2020, 32(2), 1903285 CrossRef CAS PubMed.
- A. N. Baker,
et al., The SARS-COV-2 spike protein binds sialic acids and enables rapid detection in a lateral flow point of care diagnostic device, ACS Cent. Sci., 2020, 6(11), 2046–2052 CrossRef CAS PubMed.
- Q. Zhang,
et al., Optical lateral flow test strip biosensors for pesticides: recent advances and future trends, TrAC, Trends Anal. Chem., 2021, 144, 116427 CrossRef CAS.
- H. Ye and X. Xia, Enhancing the sensitivity of colorimetric lateral flow assay (CLFA) through signal amplification techniques, J. Mater. Chem. B, 2018, 6(44), 7102–7111 RSC.
- W. Xie,
et al., A high-resolution colorimetric immunoassay platform realized by coupling enzymatic multicolor generation with smartphone readout, Analyst, 2018, 143(12), 2901–2907 RSC.
- C. Parolo and A. Merkoçi, Paper-based nanobiosensors for diagnostics, Chem. Soc. Rev., 2013, 42(2), 450–457 RSC.
- V. Shirshahi and G. Liu, Enhancing the analytical performance of paper lateral flow assays: from chemistry to engineering, TrAC, Trends Anal. Chem., 2021, 136, 116200 CrossRef.
- H. Geng,
et al., Noble Metal Nanoparticle Biosensors: From Fundamental Studies toward Point-of-Care Diagnostics, Acc. Chem. Res., 2022, 55(5), 593–604 CrossRef CAS PubMed.
- J. H. Soh, H.-M. Chan and J. Y. Ying, Strategies for developing sensitive and specific nanoparticle-based lateral flow assays as point-of-care diagnostic device, Nano Today, 2020, 30, 100831 CrossRef CAS.
- J. Nichols,
et al., Commercially Available Enzyme-Linked Immunosorbent Assay and Polymerase Chain Reaction Tests for Detection of Feline Immunodeficiency Virus Infection, J. Vet. Intern. Med., 2017, 31(1), 55–59 CrossRef CAS PubMed.
- S. Santiago-Felipe,
et al., Recombinase polymerase and enzyme-linked immunosorbent assay as a DNA amplification-detection strategy for food analysis, Anal. Chim. Acta, 2014, 811, 81–87 CrossRef CAS PubMed.
- X. Li,
et al., A fast and sensitive immunoassay of avian influenza virus based on label-free quantum dot probe and lateral flow test strip, Talanta, 2012, 100, 1–6 CrossRef CAS PubMed.
- L. Dou,
et al., ‘Three-To-One’ multi-functional nanocomposite-based lateral flow immunoassay for label-free and dual-readout detection of pathogenic bacteria, Biosens. Bioelectron., 2022, 204, 114093 CrossRef CAS PubMed.
- B. B. Tasbasi,
et al., Label-free lateral flow assay for Listeria monocytogenes by aptamer-gated release of signal molecules, Anal. Biochem., 2019, 587, 113449 CrossRef CAS PubMed.
- J. D. Bishop,
et al., Sensitivity enhancement in lateral flow assays: a systems perspective, Lab Chip, 2019, 19(15), 2486–2499 RSC.
- Y. Wu,
et al., How nanoparticles transform single molecule measurements into quantitative sensors, Adv. Mater., 2020, 32(18), 1904339 CrossRef CAS PubMed.
- P. Schubert-Ullrich,
et al., Commercialized rapid immunoanalytical tests for determination of allergenic food proteins: an overview, Anal. Bioanal. Chem., 2009, 395, 69–81 CrossRef CAS PubMed.
- T. Mahmoudi, M. de la Guardia and B. Baradaran, Lateral flow assays towards point-of-care cancer detection: a review of current progress and future trends, TrAC, Trends Anal. Chem., 2020, 125, 115842 CrossRef CAS.
-
K. L. Cox, V. Devanarayan, A. Kriauciunas, J. Manetta, C. Montrose, S. Sittampalam, Immunoassay Methods, Assay Guidance Manual, 2019, https://www.ncbi.nlm.nih.gov/books/NBK92434/ Search PubMed.
- C. T. Yew,
et al., Electrospin-coating of nitrocellulose membrane enhances sensitivity in nucleic acid-based lateral flow assay, Anal. Chim. Acta, 2018, 1009, 81–88 CrossRef CAS PubMed.
- Y. Tang,
et al., Nanocellulose aerogel inserts for quantitative lateral flow immunoassays, Biosens. Bioelectron., 2021, 192, 113491 CrossRef CAS PubMed.
- W. Ren,
et al., Magnetic Focus Lateral Flow Sensor for Detection of Cervical Cancer Biomarkers, Anal. Chem., 2019, 91(4), 2876–2884 CrossRef CAS PubMed.
- J. F. Bergua,
et al., Lateral flow device for water fecal pollution assessment: from troubleshooting of its microfluidics using bioluminescence to colorimetric monitoring of generic Escherichia coli, Lab Chip, 2021, 21(12), 2417–2426 RSC.
- J. C. Porras,
et al., Comparative Study of Gold and Carbon Nanoparticles in Nucleic Acid Lateral Flow Assay, Nanomaterials, 2021, 11(3), 741 CrossRef CAS PubMed.
- Z. Gao,
et al., Platinum-Decorated Gold Nanoparticles with Dual Functionalities for Ultrasensitive
Colorimetric in Vitro Diagnostics, Nano Lett., 2017, 17(9), 5572–5579 CrossRef CAS PubMed.
- B. S. Miller,
et al., Spin-enhanced nanodiamond biosensing for ultrasensitive diagnostics, Nature, 2020, 587(7835), 588–593 CrossRef CAS PubMed.
- C. Wang,
et al., Magnetic SERS Strip for Sensitive and Simultaneous Detection of Respiratory Viruses, ACS Appl. Mater. Interfaces, 2019, 11(21), 19495–19505 CrossRef CAS PubMed.
- A. A. Ellington,
et al., Antibody-based protein multiplex platforms: technical and operational challenges, Clin. Chem., 2010, 56(2), 186–193 CrossRef CAS PubMed.
- W. Dejnirattisai,
et al., Dengue virus sero-cross-reactivity drives antibody-dependent enhancement of infection with zika virus, Nat. Immunol., 2016, 17(9), 1102–1108 CrossRef CAS PubMed.
- A. Norsyahida,
et al., Laboratory detection of strongyloidiasis: I g G-, I g G 4-and I g E-ELISA s and cross-reactivity with lymphatic filariasis, Parasite Immunol., 2013, 35(5–6), 174–179 CrossRef CAS PubMed.
- Y. Zhao,
et al., Rapid multiplex detection of 10 foodborne pathogens with an up-converting phosphor technology-based 10-channel lateral flow assay, Sci. Rep., 2016, 6(1), 1–8 CrossRef PubMed.
- C. Dincer,
et al., Multiplexed point-of-care testing–xPOCT, Trends Biotechnol., 2017, 35(8), 728–742 CrossRef CAS PubMed.
- K. Mohd Hanafiah,
et al., Development of multiplexed infectious disease lateral flow assays: challenges and opportunities, Diagnostics, 2017, 7(3), 51 CrossRef PubMed.
- W. Zheng,
et al., Lateral flow test for visual detection of multiple MicroRNAs, Sens. Actuators, B, 2018, 264, 320–326 CrossRef CAS PubMed.
- N. A. Taranova,
et al., Integration of lateral flow and microarray technologies for multiplex immunoassay: application to the determination of drugs of abuse, Microchim. Acta, 2013, 180, 1165–1172 CrossRef CAS.
- D. K. Han,
et al., Paper-based multiplex analytical device for simultaneous detection of Clostridioides difficile toxins and glutamate dehydrogenase, Biosens. Bioelectron., 2021, 176, 112894 CrossRef CAS PubMed.
- J. Gomez-Martinez,
et al., Multiplex lateral flow assay for rapid visual blood group genotyping, Anal. Chem., 2018, 90(12), 7502–7509 CrossRef CAS PubMed.
- D. Zhang,
et al., Quantitative detection of multiplex cardiac biomarkers with encoded SERS nanotags on a single T line in lateral flow assay, Sens. Actuators, B, 2018, 277, 502–509 CrossRef CAS.
- Y. Zhou,
et al., Point-of-care COVID-19 diagnostics powered by lateral flow assay, Trends Anal. Chem., 2021, 145, 116452 CrossRef CAS PubMed.
- X. Meng,
et al., Nanozyme-strip for rapid and ultrasensitive nucleic acid detection of SARS-CoV-2, Biosens. Bioelectron., 2022, 217, 114739 CrossRef CAS PubMed.
- Q. Zhang,
et al., An AIEgen/graphene oxide nanocomposite (AIEgen@GO)-based two-stage "turn-on" nucleic acid biosensor for rapid detection of SARS-CoV-2 viral sequence, Aggregate, 2022, e195 Search PubMed.
- M. Zou,
et al., Rapid point-of-care testing for SARS-CoV-2 virus nucleic acid detection by an isothermal and nonenzymatic signal amplification system coupled with a lateral flow immunoassay strip, Sens. Actuators, B, 2021, 342, 129899 CrossRef CAS PubMed.
- L. Kashefi-Kheyrabadi,
et al., Rapid, multiplexed, and nucleic acid amplification-free detection of SARS-CoV-2 RNA using an electrochemical biosensor, Biosens. Bioelectron., 2022, 195, 113649 CrossRef CAS PubMed.
- Z. Wang,
et al., A point-of-care selenium nanoparticle-based test for the combined detection of anti-SARS-CoV-2 IgM and IgG in human serum and blood, Lab Chip, 2020, 20(22), 4255–4261 RSC.
- M. Feng,
et al., Development of a Sensitive Immunochromatographic Method Using Lanthanide Fluorescent Microsphere for Rapid Serodiagnosis of COVID-19, ACS Sens., 2020, 5(8), 2331–2337 CrossRef CAS PubMed.
- M. A. Black,
et al., Analytical performance of lateral flow immunoassay for SARS-CoV-2 exposure screening on venous and capillary blood samples, J. Immunol. Methods, 2021, 489, 112909 CrossRef CAS PubMed.
- S. Chen,
et al., SERS-based lateral flow immunoassay for sensitive and simultaneous detection of anti-SARS-CoV-2 IgM and IgG antibodies by using gap-enhanced Raman nanotags, Sens. Actuators, B, 2021, 348, 130706 CrossRef CAS PubMed.
- C. Zhang,
et al., Foundation and Clinical Evaluation of a New Method for Detecting SARS-CoV-2 Antigen by Fluorescent Microsphere Immunochromatography, Front. Cell. Infect. Microbiol., 2020, 10, 553837 CrossRef CAS PubMed.
- J. Guo,
et al., 5G-enabled ultra-sensitive fluorescence sensor for proactive prognosis of COVID-19, Biosens. Bioelectron., 2021, 181, 113160 CrossRef CAS PubMed.
- D. Liu,
et al., Nanozyme chemiluminescence paper test for rapid and sensitive detection of SARS-CoV-2 antigen, Biosens. Bioelectron., 2020, 173, 112817 CrossRef PubMed.
- L. D. Xu, J. Zhu and S. N. Ding, Immunoassay of SARS-CoV-2 nucleocapsid proteins using novel red emission-enhanced carbon dot-based silica spheres, Analyst, 2021, 146(16), 5055–5060 RSC.
- B. D. Grant,
et al., SARS-CoV-2 Coronavirus Nucleocapsid Antigen-Detecting Half-Strip Lateral Flow Assay Toward the Development of Point of Care Tests Using Commercially Available Reagents, Anal. Chem., 2020, 92(16), 11305–11309 CrossRef CAS PubMed.
- X. Deng,
et al., Applying strand displacement amplification to quantum dots-based fluorescent lateral flow assay strips for HIV-DNA detection, Biosens. Bioelectron., 2018, 105, 211–217 CrossRef PubMed.
- J. Hu,
et al., Oligonucleotide-linked gold nanoparticle aggregates for enhanced sensitivity in lateral flow assays, Lab Chip, 2013, 13(22), 4352–4357 RSC.
- B. A. Rohrman,
et al., A lateral flow assay for quantitative detection of amplified HIV-1 RNA, PLoS One, 2012, 7(9), e45611 CrossRef CAS PubMed.
- Y. Pang,
et al., CRISPR-cas12a mediated SERS lateral flow assay for amplification-free detection of double-stranded DNA and single-base mutation, Chem. Eng. J., 2022, 429, 132109 CrossRef CAS.
- N. Abas,
et al., Natural and synthetic refrigerants, global warming: a review, Renewable Sustainable Energy Rev., 2018, 90, 557–569 CrossRef CAS.
- J. H. Lee,
et al., Multiplex diagnosis of viral infectious diseases (AIDS, hepatitis C, and hepatitis A) based on point of care lateral flow assay using engineered proteinticles, Biosens. Bioelectron., 2015, 69, 213–225 CrossRef CAS PubMed.
- I. Martiskainen,
et al., Double-Antigen Lateral Flow Immunoassay for the Detection of Anti-HIV-1 and -2 Antibodies Using Upconverting Nanoparticle Reporters, Sensors, 2021, 21(2), 330 CrossRef CAS PubMed.
- F. Kheiri,
et al., A novel amperometric immunosensor based on acetone-extracted propolis for the detection of the HIV-1 p24 antigen, Biosens. Bioelectron., 2011, 26(11), 4457–4463 CrossRef CAS PubMed.
- J. H. Kwon,
et al., Performance of point-of-care diagnosis of AIDS: label-free one-step-immunoassay vs. lateral flow assay, Analyst, 2018, 143(4), 936–942 RSC.
- C. N. Loynachan,
et al., Platinum Nanocatalyst Amplification: Redefining the Gold Standard for Lateral Flow Immunoassays with Ultrabroad Dynamic Range, ACS Nano, 2018, 12(1), 279–288 CrossRef CAS PubMed.
- S. Workman,
et al., Rapid detection of HIV-1 p24 antigen using magnetic immuno-chromatography (MICT), J. Virol. Methods, 2009, 160(1–2), 14–21 CrossRef CAS PubMed.
- Z. A. Parpia,
et al., p24 antigen rapid test for diagnosis of acute pediatric HIV infection, J. Acquired Immune Defic. Syndr., 2010, 55(4), 413–419 CrossRef CAS PubMed.
- K. Kaarj, P. Akarapipad and J. Y. Yoon, Simpler, Faster, and Sensitive Zika Virus Assay Using Smartphone Detection of Loop-mediated Isothermal Amplification on Paper Microfluidic Chips, Sci. Rep., 2018, 8(1), 12438 CrossRef PubMed.
- J. Jeon,
et al., Improvement of reproducibility and thermal stability of surface-enhanced Raman scattering-based lateral flow assay strips using silica-encapsulated gold nanoparticles, Sens. Actuators, B, 2020, 321, 128521 CrossRef CAS.
- L. D. Xu,
et al., Luminous silica colloids with carbon dot incorporation for sensitive immunochromatographic assay of Zika virus, Analyst, 2021, 146(2), 706–713 RSC.
- Z. Rong,
et al., Smartphone-based fluorescent lateral flow immunoassay platform for highly sensitive point-of-care detection of Zika virus nonstructural protein 1, Anal. Chim. Acta, 2019, 1055, 140–147 CrossRef CAS PubMed.
- F. Peng,
et al., Development of an immunochromatographic strip for rapid detection of H9 subtype avian influenza viruses, Clin. Vaccine Immunol., 2008, 15(3), 569–574 CrossRef CAS PubMed.
- N. Sun,
et al., Visual signal generation for the detection of influenza viruses by duplex recombinase polymerase amplification with lateral flow dipsticks, Anal. Bioanal. Chem., 2019, 411(16), 3591–3602 CrossRef CAS PubMed.
- C. Wang,
et al., Sonochemical synthesis of highly branched flower-like Fe(3)O(4)@SiO(2)@Ag microcomposites and their application as versatile SERS substrates, Nanoscale, 2016, 8(47), 19816–19828 RSC.
- Z. Bai,
et al., Rapid Enrichment and Ultrasensitive Detection of Influenza A Virus in Human Specimen using Magnetic Quantum Dot Nanobeads Based Test Strips, Sens. Actuators, B, 2020, 325, 128780 CrossRef CAS PubMed.
- S. Bamrungsap,
et al., Rapid and sensitive lateral flow immunoassay for influenza antigen using fluorescently-doped silica nanoparticles, Microchim. Acta, 2014, 181(1), 223–230 CrossRef CAS.
- N. Wiriyachaiporn,
et al., A simple fluorescence-based lateral flow test platform for rapid influenza B virus screening, Anal. Methods, 2021, 13(14), 1687–1694 RSC.
- X. Lin,
et al., A rapid influenza diagnostic test based on detection of viral neuraminidase activity, Sci. Rep., 2022, 12(1), 505 CrossRef CAS PubMed.
- F. M. Yrad, J. M. Castanares and E. C. Alocilja, Visual Detection of Dengue-1 RNA Using Gold Nanoparticle-Based Lateral Flow Biosensor, Diagnostics, 2019, 9(3), 74 CrossRef CAS PubMed.
- T. N. Aynur, Variable refrigerant flow systems: a review, Energy Build., 2010, 42(7), 1106–1112 CrossRef.
- S. Kumar,
et al., Tapered lateral flow immunoassay based point-of-care diagnostic device for ultrasensitive colorimetric detection of dengue NS1, Biomicrofluidics, 2018, 12(3), 034104 CrossRef PubMed.
- T. V. Tran,
et al., Development of a highly sensitive magneto-enzyme lateral flow immunoassay for dengue NS1 detection, PeerJ, 2019, 7, e7779 CrossRef PubMed.
- M. Sanchez-Purra,
et al., Surface-Enhanced Raman Spectroscopy-Based Sandwich Immunoassays for Multiplexed Detection of Zika and Dengue Viral Biomarkers, ACS Infect. Dis., 2017, 3(10), 767–776 CrossRef CAS PubMed.
- C. Srisomwat,
et al., Amplification-free DNA Sensor for the One-Step Detection of the Hepatitis B Virus Using an Automated Paper-Based Lateral Flow Electrochemical Device, Anal. Chem., 2021, 93(5), 2879–2887 CrossRef CAS PubMed.
- L. Li,
et al., Development of up-converting phosphor technology-based lateral-flow assay for rapidly quantitative detection of hepatitis B surface antibody, Diagn. Microbiol. Infect. Dis., 2009, 63(2), 165–172 CrossRef CAS PubMed.
- L. W. Song,
et al., Rapid fluorescent lateral-flow immunoassay for hepatitis B virus genotyping, Anal. Chem., 2015, 87(10), 5173–5180 CrossRef CAS PubMed.
- H. Shen,
et al., Phosphine-free synthesis of high-quality reverse type-I ZnSe/CdSe core with CdS/Cd(x)Zn(1 - x)S/ZnS multishell nanocrystals and their application for detection of human hepatitis B surface antigen, Nanotechnology, 2011, 22(37), 375602 CrossRef PubMed.
- J. Shen,
et al., Immunochromatographic assay for quantitative and sensitive detection of hepatitis B virus surface antigen using highly luminescent quantum dot-beads, Talanta, 2015, 142, 145–149 CrossRef CAS PubMed.
- S. Wang,
et al., Dual-signal lateral flow assay using vancomycin-modified nanotags for rapid and sensitive detection of Staphylococcus aureus, RSC Adv., 2021, 11(22), 13297–13303 RSC.
- D. K. Han,
et al., Paper-based multiplex analytical device for simultaneous detection of Clostridioides difficile toxins and glutamate dehydrogenase, Biosens. Bioelectron., 2021, 176, 112894 CrossRef CAS PubMed.
- E. Frohnmeyer,
et al., Aptamer lateral flow assays for rapid and sensitive detection of cholera toxin, Analyst, 2019, 144(5), 1840–1849 RSC.
- H. Ilhan,
et al., Replacement of antibodies with bacteriophages in lateral flow assay of Salmonella Enteritidis, Biosens. Bioelectron., 2021, 189, 113383 CrossRef CAS PubMed.
- R. Charlermroj,
et al., A rapid colorimetric lateral flow test strip for detection of live Salmonella Enteritidis using whole phage as a specific binder, Front. Microbiol., 2022, 13, 1008817 CrossRef PubMed.
- Z. Wu, Simultaneous Detection of Listeria monocytogenes and Salmonella typhimuriumby a SERS-Based Lateral Flow Immunochromatographic Assay, Food Anal. Methods, 2019, 12(5), 1086–1091 CrossRef.
- Z. Du,
et al., Improving the sensitivity of lateral flow immunoassay for Salmonella typhimurium detection via flow-rate regulation, Food Chem., 2022, 397, 133756 CrossRef CAS PubMed.
- Z. Tu,
et al., Ultrasensitive Fluorescence Lateral Flow Assay for Simultaneous Detection of Pseudomonas aeruginosa and Salmonella typhimurium via Wheat Germ Agglutinin-Functionalized Magnetic Quantum Dot Nanoprobe, Biosensors, 2022, 12(11), 942 CrossRef CAS PubMed.
- S. Zheng,
et al., Sensitive detection of Escherichia coli O157:H7 and Salmonella typhimurium in food samples using two-channel fluorescence lateral flow assay with liquid Si@quantum dot, Food Chem., 2021, 363, 130400 CrossRef CAS PubMed.
- P. Gao,
et al., An Enhanced Lateral Flow Assay Based on Aptamer-Magnetic Separation and Multifold AuNPs for Ultrasensitive Detection of Salmonella Typhimurium in Milk, Foods, 2021, 10(7), 1605 CrossRef CAS PubMed.
- R. Pan,
et al., Gold nanoparticle-based enhanced lateral flow immunoassay for detection of Cronobacter sakazakii in powdered infant formula, J. Dairy Sci., 2018, 101(5), 3835–3843 CrossRef CAS PubMed.
- Q. Ma,
et al., Rapid and visual detection of Mycobacterium tuberculosis complex using recombinase polymerase amplification combined with lateral flow strips, Mol. Cell. Probes, 2017, 36, 43–49 CrossRef CAS PubMed.
- A. R. Pavankumar,
et al., Proficient Detection of Multi-Drug-Resistant Mycobacterium tuberculosis by Padlock Probes and Lateral Flow Nucleic Acid Biosensors, Anal. Chem., 2016, 88(8), 4277–4284 CrossRef CAS PubMed.
- Y. Wang,
et al., Detection of nucleic acids and elimination of carryover contamination by using loop-mediated isothermal
amplification and antarctic thermal sensitive uracil-DNA-glycosylase in a lateral flow biosensor: application to the detection of Streptococcus pneumoniae, Mikrochim. Acta, 2018, 185(4), 212 CrossRef PubMed.
- C. S. Jorgensen,
et al., Evaluation of a new lateral flow test for detection of Streptococcus pneumoniae and Legionella pneumophila urinary antigen, J. Microbiol. Methods, 2015, 116, 33–36 CrossRef PubMed.
- L. Duchesne and K. Lacombe, Innovative technologies for point-of-care testing of viral hepatitis in low-resource and decentralized settings, J. Viral Hepatitis, 2018, 25(2), 108–117 CrossRef CAS PubMed.
- S. Shrivastava, T. Q. Trung and N.-E. Lee, Recent progress, challenges, and prospects of fully integrated mobile and wearable point-of-care testing systems for self-testing, Chem. Soc. Rev., 2020, 49(6), 1812–1866 RSC.
- J. Morrison,
et al., Field-based detection of biological samples for forensic analysis: established techniques, novel tools, and future innovations, Forensic Sci. Int., 2018, 285, 147–160 CrossRef CAS PubMed.
- H. R. Boehringer and B. J. O'Farrell, Lateral flow assays in infectious disease diagnosis, Clin. Chem., 2022, 68(1), 52–58 CrossRef PubMed.
- R. Wijngaard,
et al., Tetracaine from urethral ointment causes false positive amphetamine results by immunoassay, Clin. Toxicol., 2021, 59(6), 500–505 CrossRef CAS PubMed.
- D. R. Hristov,
et al., Designing paper-based immunoassays for biomedical applications, Sensors, 2019, 19(3), 554 CrossRef PubMed.
- F. S. R. R. Teles, Biosensors and rapid diagnostic tests on the frontier between analytical and clinical chemistry for biomolecular diagnosis of dengue disease: a review, Anal. Chim. Acta, 2011, 687(1), 28–42 CrossRef CAS PubMed.
- W. J. Shim, S. H. Hong and S. E. Eo, Identification methods in microplastic analysis: a review, Anal. Methods, 2017, 9(9), 1384–1391 RSC.
- N. Jiang,
et al., Low-cost optical assays for point-of-care diagnosis in resource-limited settings, ACS Sens., 2021, 6(6), 2108–2124 CrossRef CAS PubMed.
- L. Syedmoradi, M. L. Norton and K. Omidfar, Point-of-care cancer diagnostic devices: from academic research to clinical translation, Talanta, 2021, 225, 122002 CrossRef CAS PubMed.
- J. Hu,
et al., Multiple test zones for improved detection performance in lateral flow assays, Sens. Actuators, B, 2017, 243, 484–488 CrossRef CAS.
- Y. Shen,
et al., Recent advances in nanotechnology for simultaneous detection of multiple pathogenic bacteria, Nano Today, 2021, 38, 101121 CrossRef CAS.
- H. Harpaldas,
et al., Point-of-care diagnostics: recent developments in a pandemic age, Lab Chip, 2021, 21(23), 4517–4548 RSC.
- C. Dincer,
et al., Disposable sensors in diagnostics, food, and environmental monitoring, Adv. Mater., 2019, 31(30), 1806739 CrossRef PubMed.
|
This journal is © The Royal Society of Chemistry 2023 |
Click here to see how this site uses Cookies. View our privacy policy here.