DOI:
10.1039/C9GC00457B
(Paper)
Green Chem., 2019,
21, 2316-2325
A bifunctional dermaseptin–thanatin dipeptide functionalizes the crop surface for sustainable pest management†
Received
5th February 2019
, Accepted 15th March 2019
First published on 1st April 2019
Abstract
To reduce pesticide use while preserving crop productivity, alternative pest and disease control measures are needed. We thought of an alternative way of functionalizing leaves of soybean to fight its most severe disease, Asian soybean rust (Phakopsora pachyrhizi). To do so, we produced bifunctional peptides that adhere to the soybean leaf surface and prevent the germination of P. pachyrhizi spores. In detail, amphiphilic peptides liquid chromatography peak I (LCI), thanatin (THA), tachystatin A2 (TA2), and lactoferricin B (LFB) were all fused to enhanced green fluorescent protein (eGFP). Of these fusion peptides, eGFP–LCI and eGFP–THA bound strongly and in a rainfast manner to the surface of soybean, barley, and corn leaves. eGFP–THA binding to soybean also withstood high temperature, sunlight and biotic degradation for at least 17 days. The dipeptides seem to bind mainly to the surface wax layer of leaves because eGFP–THA and eGFP–LCI did not stick to the wax-depleted cer-j59 mutant of barley or to corn leaves with their surface wax removed. A fusion of the antimicrobial peptide dermaseptin 01 and THA (DS01–THA) inhibits the germination of P. pachyrhizi spores in vitro and reduces Asian soybean rust disease in a rainfast manner. Therefore, this study reveals that bifunctional peptides can be used to functionalize the crop surface for sustainable disease management.
Introduction
Pesticides benefit society in many ways. They kill insects, weeds, microbes, and other potentially harmful organisms. They create jobs and protect millions from malaria and other insect-borne diseases.1 They have a broad spectrum of application but their main utilization is in agriculture where they help to secure crop yield.2 However, some synthetic pesticides can also pose a threat to human health and the environment.3,4 Many of them succumb to wash off by rain5,6 and, thus, need to be applied several times in a growing season. They can hit nontarget species7,8 and drive the evolution of pesticide-insensitive insects, weeds, microbes, and other organisms that are hard to control. Some pesticides are even suspected to cause neurodevelopmental toxicity in humans. For example, chlorpyrifos, a widely used broad-spectrum organophosphate insecticide, at standard use levels, can cause brain anomalies in children exposed prenatally to the insecticide.4 Hence, although safer than ever,9 synthetic pesticides increasingly raise ecological and health concerns.3,4,7 Therefore, alternative pest management products are desired.
Peptide-based adhesion promoters enable the immobilization of peptides or proteins on various materials.10–13 They have a broad range of applications, such as in enzyme catalysis, biosensors, compound delivery, and binding to hydrophobic surfaces such as polypropylene and polystyrene.10–13 The binding strength of an adhesion-promoting peptide to a given hydrophobic surface can be increased by protein engineering, either through directed evolution or KnowVolution campaigns to better understand the molecular interactions that govern surface binding.12,14
Antimicrobial peptides (AMPs) are abundant in nature. They have different modes of action, which include detergent-like activity and pore-forming action through self-assembly.15–17 Despite their differing modes of action, all AMPs destabilize lipid membranes and this confers them antimicrobial activity.15–17 AMPs are natural peptides and usually subject to residue-free degradation. Thus, they do not pollute soil or water. As most pathogens attack plants from the outside, functionalizing the crop surface with AMPs should constitute a possible alternative to conventional disease management with synthetic agrochemicals.
The aerial surface of primary plant tissues is represented by the cuticle, a continuous extracellular membrane atop the stem, leaves, reproductive structures, and fruits.18,19 The cuticle is built up of the crosslinked biopolymer cutin that has incorporated (intracuticular) and superimposed (epicuticular) waxes. The latter constitute complex mixtures of water-repellent lipophilic compounds (such as esters of long-chain fatty acids with long-chain aliphatic and cyclic alcohols, phytosterols, pentacyclic triterpenoids, or epicuticular flavonoids).18,19
Identification of small peptides adhering to epicuticular surface waxes and being capable of equipping with AMPs for functionalizing the plant surface are highly promising for alternative and eco-friendly pest management. Dermaseptins (DSs) are a family of α-helical, amphiphilic AMPs of 28–34 amino acid residues.20–25 They originate from the skin of frogs in the Phyllomedusa genus20,23 and effectively kill various bacteria, protozoa, yeasts, and filamentous fungi.20,22–25 However, they do not seem to harm mammalian cells.20 Therefore, DSs are excellent candidates for novel and nonhazardous AMP-based pesticides.
Soybean (Glycine max) is one of the most important crops on the Earth.26 Its beans provide amino acids, protein, and oil. As a member of the Fabaceae family of plants and their unique symbiosis with nitrogen-fixing bacteria in the Rhizobium genus, soybean also serves as a natural soil fertilizer.
Soybean's major disease is caused by Phakopsora pachyrhizi, a dread fungus that causes Asian soybean rust (SBR).27–29 The disease spreads rapidly and hits plants hard, defoliating fields within a couple of days and reducing the yield by up to 80%.28 SBR thus threatens global food security. The best defense would be a soybean variety that resists SBR. However, no such variety is grown today mainly because of their low yield.27 SBR is rather controlled by synthetic fungicides to which it increasingly evolves insensitivity.27,30 In Brazil, which is second in global soybean production, fungicides are applied at least three times per season currently running up a bill of more than two billion US dollars a year.31
Here, we introduce a novel approach and a promising alternative to conventional pest management with synthetic pesticides. We demonstrate that the amphiphilic peptide thanatin (THA) upon spray application tightly anchors enhanced green fluorescent protein (eGFP)32 to the surface of soybean leaves in a rainfast manner. We also show that the bifunctional peptide DS01–THA inhibits the germination of P. pachyrhizi spores in vitro and reduces Asian soybean rust symptoms on soybean plants.
Results
Binding of bipartite proteins to soybean leaves
We thought of an eco-friendly way of functionalizing soybean plants to defeat Asian soybean rust. First, to identify amphipathic peptides that may serve as adhesion promoters for functionalizing soybean leaves, we selected the natural amphiphilic peptides tachystatin A2 (TA2)33 and liquid chromatography peak I (LCI)34 with known binding to hydrophobic polymers.11,12 TA2 and LCI contain β-sheet folding. We also tested THA35 and lactoferricin B (LFB)36 for leaf-binding capacity because these peptides too have amphiphilic properties and β-sheet folding. Using genetic engineering we fused each of these peptides to the eGFP reporter protein for the easy detection of the fusion protein in later assays. Fusion proteins and eGFP (control) were produced in Escherichia coli cells of which we prepared crude extracts.
Next, leaf discs were punched out of soybean leaves and floated on the E. coli cell extracts. Floating leaf discs on a buffer served as an additional control. After 10 min, leaf discs were thoroughly washed in water and assayed for green fluorescence, which indicates the presence of eGFP or the adequate fusion protein, by microscopy. As shown in Fig. 1A, eGFP–LCI and eGFP–THA, but not eGFP–TA2, eGFP–LFB, or eGFP alone bound to the surface of the soybean leaf discs in a washing-resistant manner. This finding pointed to LCI and THA as suitable anchor peptides for functionalizing the soybean leaf surface.
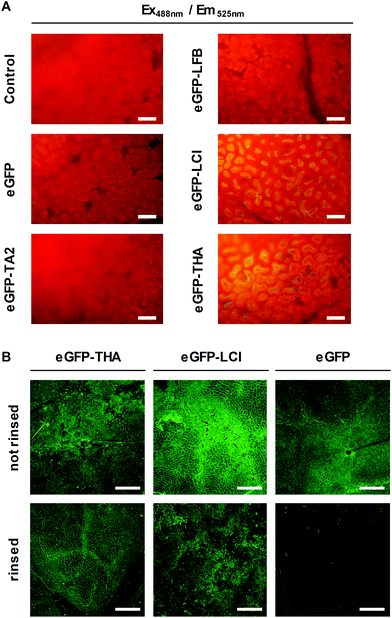 |
| Fig. 1 Identification of amphiphilic peptides that anchor eGFP to the soybean leaf surface. (A) Leaf discs from 3-week-old soybean plants were floated on E. coli lysates containing recombinant eGFP, eGFP–TA2, eGFP–LFB, eGFP–LCI, or eGFP–THA. Floating leaf discs on a buffer (50 mM Tris/HCl, pH 8.0) served as a control. After incubation for 10 min, leaf discs were thoroughly washed in water and green fluorescence detected using fluorescence microscopy (scale bar: 50 μm). (B) Leaves of 3-week-old soybean plants were sprayed with aqueous solutions of purified eGFP–THA, eGFP–LCI, or eGFP (all at 750 nM). After drying off, treated leaf areas were thoroughly rinsed with water and analyzed for the absence or presence of green fluorescence using confocal microscopy. (Scale bar: 250 μm). | |
Next, we purified eGFP–THA, eGFP–LCI, and eGFP from the crude E. coli extracts and sprayed aqueous solutions of these proteins on soybean leaves (Fig. 1B). After drying off, sprayed leaves were exhaustively rinsed with water. This experiment confirmed the water-resistant binding of eGFP–THA and eGFP–LCI, but not eGFP, upon spraying them onto intact soybean leaves (Fig. 1B) (ESI, Fig. S1†). We attribute the binding of the amphiphilic peptides THA and LCI (Fig. 2) mainly to the hydrophobic interaction with lipophilic constituents (e.g. long-chain fatty acids) in the surface wax layer (see the Discussion section).
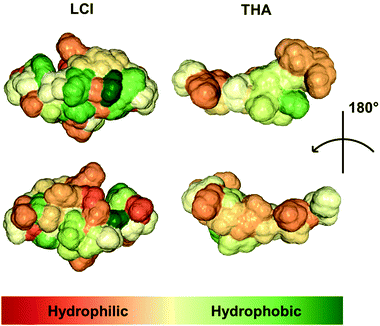 |
| Fig. 2 Surface of the peptides LCI (PDB ID: 2b9k) and THA (PDB ID: 8tfv). The molecular structure of the two peptides was visualized using the NGL Viewer of protein data bank RCSB PDB (http://www.rcsb.org). The color code indicates overall hydrophobicity with hydrophilic parts of the adequate peptide being displayed in red whereas hydrophobic regions are shown in green. | |
Long-term binding of eGFP–THA to leaves of soybean plants under semi-field conditions
To evaluate the persistence of a fusion protein on the crop surface, we sprayed leaflets of the first trifolium of soybean plants with eGFP–THA. After drying off, plants were transferred outside, kept on a university plot, and assayed for the presence of eGFP fluorescence at various times over 17 d. At later time points, leaves showed senescence, which precluded longer investigation. As shown in Fig. 3A, while eGFP fluorescence seemed to decrease with time, clear eGFP presence was still seen at 17 days post application (dpa). During the test period, there was one moderate rainfall event (0.3 L m−2 total precipitation) to which eGFP–THA seems to be largely insensible (Fig. 5A and the below text). Therefore, the observed moderate reduction of eGFP fluorescence (Fig. 3A) over time may be caused by the loss of eGFP from the eGFP–THA dipeptide due to high temperature (Fig. 3B), sunlight (Fig. 3B), microbial degradation, or a combination of these possibilities.
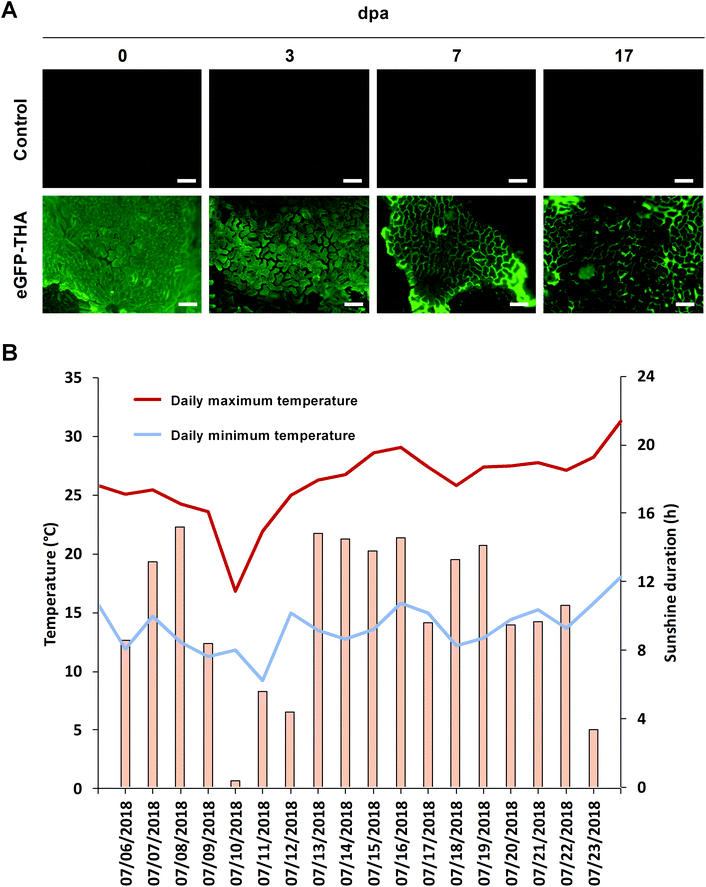 |
| Fig. 3 Long-term binding of eGFP–THA to leaves of soybean plants under semi-field conditions. Leaflets of the first trifolium of soybean plants were sprayed with eGFP–THA (750 nM). After 2 h, plants were transferred outdoors and kept for 17 d. (A) Abundance of eGFP–THA on the leaflets was examined at the day of spray treatment (0 dpa) and after 3, 7, and 17 dpa. (B) During the experiment, meteorological data were retrieved from http://www.wetterkontor.de. During experimentation, there was a rainfall event on 07/10/2018 with 0.3 L m−2 total precipitation. dpa, days post application. (Scale bar: 100 μm). | |
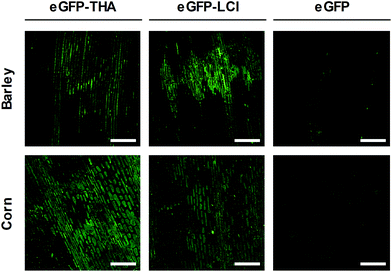 |
| Fig. 4 Binding of eGFP–THA and eGFP–LCI to leaves of barley and corn. Leaves were treated with aqueous solutions (750 nM) of eGFP or eGFP-tagged versions of THA (eGFP–THA) and LCI (eGFP–LCI). After drying off, treated leaves were intensively rinsed with water and analyzed for green fluorescence using confocal microscopy. (Scale bar: 250 μm). | |
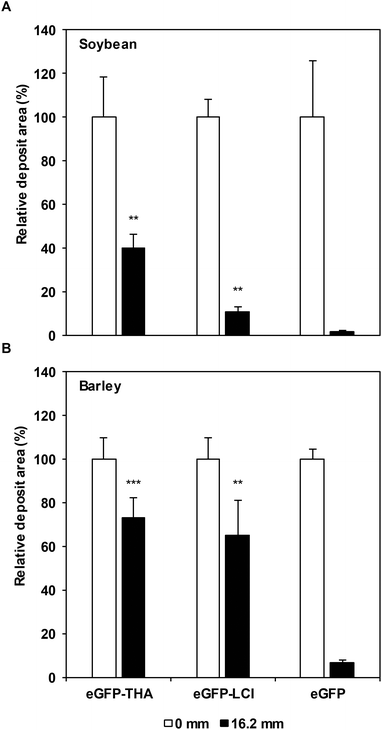 |
| Fig. 5 Binding of eGFP–THA and eGFP–LCI in a field experiment and at natural rainfall. The binding efficacy of eGFP-tagged anchor peptides THA (eGFP–THA, 1 μM) and LCI (eGFP–LCI, 5 μM) and of eGFP (5 μM, control) was evaluated on soybean (A) and barley (B) leaves after four rainfall events (16.2 mm total precipitation). The absence or presence of eGFP–THA, eGFP–LCI, and eGFP was determined by multispectral fluorescence microscopy. Bars represent means ± SEM. Stars indicate significant differences (**p < 0.01; ***p < 0.001) in the paired t-test between plants treated with eGFP–THA, eGFP–LCI, or eGFP alone. In (A) and (B), n ≥ 6. | |
Binding to monocotyledonous crops like barley and corn
Barley (Hordeum vulgare) is one of the first cultivated grains and is used as animal fodder, for brewing beer and some other distilled beverages, and as a component of stews, bread and various health foods.37 Corn (Zea mays) has become a staple food in many parts of the world. However, corn is also used to provide ethanol, animal feed, and other products that make our lives enjoyable, such as sweet corn and popcorn.38
We used eGFP–THA and eGFP–LCI to determine whether the amphiphilic peptides THA and LCI, in addition to the eudicot soybean crop, would anchor eGFP to the leaf surface of monocotyledonous plants and if they would do so in a water-resistant manner. For this purpose, we sprayed leaves of barley and corn plants with the eGFP–THA and eGFP–LCI fusions, as well as with eGFP (control). After drying off, sprayed leaves were intensively rinsed with water. As is obvious in Fig. 4, the eGFP–THA and eGFP–LCI fusion proteins, but not eGFP alone, adhere to the leaves of barley and corn in a water-withstanding manner. Thus, the amphiphilic peptides THA and LCI are suited for functionalizing the surface of the monocot as well as eudicot crops in a rinsing-resistant manner.
Peptide anchoring to crop leaves is rainfast
Our previous results (Fig. 1 and 4) suggested that THA and LCI promote the adhesion of proteins to crop leaves in a rain-resistant manner. To investigate whether their binding indeed is rainfast, we treated leaves of soybean and barley plants that we had grown under field conditions with purified eGFP–THA, eGFP–LCI, and eGFP. After treatment, the plants within three days experienced a light, two moderate, and a heavy rainfall events (16.2 mm precipitation in total). As shown in Fig. 5A, while ∼98% of sprayed eGFP was washed off soybean leaves by the natural rainfall events, ∼11% of eGFP–LCI and ∼40% eGFP–THA remained on the leaves as indicated by the remaining green fluorescence.
For barley, only ∼7% unanchored eGFP, but ∼65% eGFP–LCI and ∼73% eGFP–THA were still found on the leaf surface after the rainfall events (Fig. 5B). Together, these findings revealed that THA adheres more tightly than LCI to the leaves of soybean and barley plants. Because these findings were made under field conditions with natural rainfall events, they disclosed the general suitability of THA and LCI for functionalizing the aerial crop surface for the applied pest management.
Binding to the surface wax layer
Next, we investigated whether the amphiphilic THA and LCI peptides would anchor eGFP to leaves by the interaction with the hydrophobic surface wax layer. To do so, we sprayed the eGFP–THA and eGFP–LCI fusion peptides on leaves of the surface wax-depleted eceriferum mutant cer-j59 of barley39 and on corn leaves with their surface wax removed (Fig. 6). Spraying leaves of the barley wild type and corn plants, both of which have an intact surface wax layer, served as the controls. Upon drying off, sprayed leaves were thoroughly rinsed with water. We did not include soybean in these assays because wax-deficient mutants are not available for this species. In addition, the aerial soybean surface is extremely rich in trichomes, which precludes noninvasive detachment of surface wax from this plant.
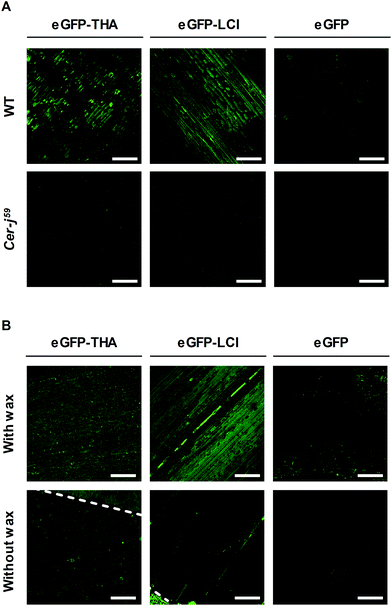 |
| Fig. 6 eGFP–THA and eGFP–LCI bind to the surface wax layer. The binding capacity of eGFP–THA, eGFP–LCI, and eGFP was evaluated with leaves of the surface wax-deficient cer-j59 mutant of barley and with corn leaves with their surface wax layer removed. Leaves of the barley wild type (WT) and corn plants without wax removal served as controls. After spraying aqueous solutions of eGFP, eGFP–THA, or eGFP–LCI (all at 750 nM) and drying off, sprayed leaves were exhaustively rinsed with water. Green fluorescence was detected by confocal microscopy. (A) Barley. (B) Corn. White broken line in (B) indicates the boundary of the surface wax-free and surface wax-containing leaf surface. (Scale bar: 250 μm). | |
As is obvious in Fig. 6, the eGFP–THA and eGFP–LCI fusion proteins, but not eGFP alone, adhered to leaves of barley (Fig. 6A, upper row) and corn (Fig. 6B, upper row) in a rinsing-resistant manner. However, both proteins did not adhere to the barley cer-j59 mutant (Fig. 6A, lower row), or to corn leaves of which the surface wax had been removed (Fig. 6B, lower row). This result revealed that the amphiphilic anchor peptides THA and LCI bind to epicuticular waxes on leaves.
DS01–THA protects soybean from SBR
DS01 is an amphiphilic peptide that can protect soybean plants from P. pachyrhizi infection.40 To investigate whether DS01, THA, or a bifunctional fusion peptide of DS01 and THA would reduce SBR disease, we sprayed soybean leaves with water (control), THA, DS01, or a DS01–THA fusion before we inoculated the leaves with urediospores of P. pachyrhizi. Fig. 7 demonstrates that while DS01 and THA alone did not significantly reduce the infection severity of soybean leaves, the DS01–THA fusion peptide diminished SBR symptoms by almost 30% (Fig. 7A). In the field, this would roughly correspond to a 20% increase in yield.41 Notably, the protection by DS01–THA was fully retained when the treated leaves were extensively rinsed with water before they were inoculated with P. pachyrhizi (Fig. 7B). Moreover, the DS01–THA fusion peptide inhibited the formation of P. pachyrhizi appressoria in vitro (ESI, Fig. S2†). This inhibitory activity of DS01–THA was sensitive to proteinase K (ESI, Fig. S2†) which clarifies that the DS01–THA fusion peptide is the likely cause for the observed decrease in SBR symptoms.
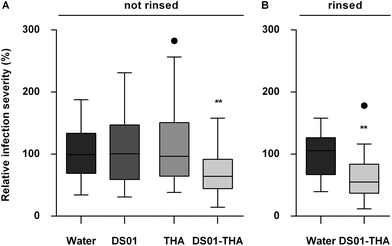 |
| Fig. 7 A DS01–THA fusion peptide protects soybean from SBR in a rinsing-resistant manner. (A) Leaves of soybean plants were treated with water (control), DS01, THA, or DS01–THA (all peptides at 25 μM). After drying off for 2 h, we inoculated treated leaves with P. pachyrhizi spores (1 mg mL−1). (B) Leaflets sprayed with water or 25 μM DS01–THA were thoroughly rinsed with water before inoculation with P. pachyrhizi spores (1 mg mL−1). In (A) and (B) we evaluated the extent of infection at 10 dpi. Mean values of three independent experiments relative to the average infection severity of water-treated control plants (set to 100%) are shown. To adequately present the variance in symptom development across all samples in all the experiments, data are presented as boxplots with the boxes spanning the interquartile range (IQR) and whiskers extending to 1.5*IQR (Tukey boxplot). Potential outliers are represented by solid dots and refer to values that are 1.5*IQR beyond the first or third quartile. Stars indicate significant differences (**p < 0.01) in the paired t-test between plants treated with water or DS01–THA. In (A) and (B), n ≥ 9. | |
Discussion
Because synthetic pesticides may cause ecological and health concerns and since they promote the evolution of pesticide-insensitive pests and pathogens, alternative measures for crop management are needed. We showed that natural amphiphilic peptides THA and LCI could be used to anchor proteins or peptides (e.g. eGFP and DS01) to the leaf surface of soybean (Fig. 1, 3, 5 and 7) (ESI, Fig. S1†), barley (Fig. 4–6), and corn (Fig. 4 and 6). We do not know the chemical component to which THA and LCI bind in surface wax, if there is only one. However, because epicuticular waxes constitute complex mixtures of mainly lipophilic compounds (such as esters of long-chain fatty acids with long-chain aliphatic and cyclic alcohols, phytosterols, pentacyclic triterpenoids, or epicuticular flavonoids)18,19 and because THA and LCI are amphiphilic peptides (Fig. 2), hydrophobic interactions are likely.34,35
The anchor peptide-mediated binding largely resists washing and rain (Fig. 1, 4, and 6) and seems not to impair photosynthetic activity (ESI, Fig. S3†). On soybean plants in the yard, the dipeptide binding to a major part also withstands heat, sunlight, and microbial degradation for at least 17 d (Fig. 3). The observed moderate decrease of eGFP fluorescence on plants under semi-field conditions (Fig. 3A) may simply be caused by the loss of eGFP from the eGFP–THA dipeptide due to high temperature (Fig. 3B), sunlight (Fig. 3B), microbial degradation, or a combination of these possibilities. However, it could also be caused by degradation of the entire eGFP–THA dipeptide on the plant. However, as eGFP fluorescence was still detected after 17 d, we conclude that the anchor peptide-mediated dipeptide binding largely withstands the major environmental challenges over a reasonable time and thus has potential for field applications.
In general, the production of peptides by recombinant microbes can be bulked up to reduce the costs for recombinant peptide production. Standard production for specialty peptides, including bipartite peptides, is about 1140 US $ per kilogram. Because one gram of the fusion peptide is known to cover ∼650 square meters,11,12 coating a surface of 100 square meters with dipeptides costs ∼0.18 US $ further supporting that our here introduced approach has potential for field applications.
The anchor peptide-mediated binding is independent of the type of leaf, as THA and LCI anchored eGFP to primary leaves, and the first and second trifolium of soybean plants (ESI, Fig. S1†). Experiments with the barley eceriferum cer-j59 mutant and surface wax-free corn leaves disclosed that the amphiphilic anchor peptides adhere to the surface wax layer of leaves (Fig. 6), thus enabling noninvasive crop protection.
DS01–THA inhibited the formation of P. pachyrhizi appressoria in vitro (ESI, Fig. S2†) and reduced SBR symptoms on soybean leaves in a rainfast manner (Fig. 7B). THA and DS01 alone were inactive at diminishing SBR on soybean leaves (Fig. 7A) although both these peptides exert activity against P. pachyrhizi in vitro (ESI, Fig. S2†). The surface wax of soybean consists of dense platelets of 1.0–1.2 μm in between wax-free trichomes.42 Clusters of five to ten platelets are perpendicularly aligned to the epidermal cell surface and, as for most members in the Fabaceae family of plants, radially assembled in rosettes.42 The reduction of SBR symptoms by the surface-attached DS01–THA dipeptide (Fig. 7) suggests that DS01–THA protrudes from the wax rosettes and inhibits P. pachyrhizi at the early stages of development, such as spore germination, germ tube development, or appressoria formation, before the fungus penetrates the plant. This hypothesis is supported by the finding that the incubation of P. pachyrhizi urediospores with DS01 before plant inoculation effectively attenuates SBR disease41 and that DS01–THA effectively inhibits P. pachyrhizi development in vitro (ESI, Fig. S2†).
All terrestrial plants to a varying extent possess a cuticle with intracuticular and epicuticular wax or a hydrophobic periderm.18 In addition, amphiphilic anchor peptides seem to adhere to the hydrophobic surface of diverse crops, though the binding occurs with different strengths (Fig. 5). State-of-the-art methods in protein engineering enabled tailoring of LCI's binding strength to the hydrophobic polymers polypropylene and polystyrene.12,14 The same techniques can be used for tailoring the binding intensity of anchor peptides to the surface of essentially any land plant. Our here developed approach thus seems to provide a unique opportunity for the development of a platform technology that enables the functionalization of the aerial plant surface for diverse purposes. We expect that bifunctional peptides or proteins consisting of plant-attaching anchor peptides and pesticidal peptides or proteins can be used to fight essentially any plant pest and disease in a rainfast manner. In fact, a THA–DS01 dipeptide inhibits the development of the anthracnose-causing pathogen Colletotrichum graminicola on corn leaves (ESI, Fig. S4†). Because the technology introduced here utilizes natural AMPs with an unspecific mode of action, there seems to be (i) no threat to human health and the environment, (ii) a reduced chance of developing AMP insensitivity in the pathogen, and (iii) no need for genetic modification of the target crop. In addition, while synthetic pesticides succumb to washing off by rain and dew, bifunctional peptides to a remarkable degree seem to resist these weather events and are thus promising for sustainable next-generation agriculture.
Conclusions
In this work, we in an interdisciplinary approach developed a sustainable means of functionalizing leaves of soybean to fight its most severe disease, Asian soybean rust (Phakopsora pachyrhizi). Using eGFP as a reporter macromolecule, we showed that THA could be used to anchor proteins in a strong and rainfast manner on the surface of soybean, and barley and corn leaves. The anchoring seemed to occur on the surface wax layer of leaves and withstood high temperature, sunlight and biotic degradation for at least 17 days. When THA was used to anchor the antimicrobial peptide DS01 to soybean leaves, Asian soybean rust disease was markedly reduced. We expect that bifunctional peptides or proteins consisting of an adequate plant-attaching anchor peptide (e.g. THA) and antimicrobial or pesticidal peptides, proteins, or cells can be used to fight essentially any plant pest and disease in an eco-friendly and rainfast manner.
Experimental section
Material
Growth of plants in chambers.
Soybean (G. max cv. Williams 82) and corn (Z. mays cv. Golden Bantam) plants were grown in a chamber for 2–4 weeks in 16-h light/24 °C and 8-h dark/21 °C cycles. Barley (H. vulgare cv. Bonus and cv. Bonus cer-j59) was grown under the same conditions except for 18 °C in both the light and dark cycles. P. pachyrhizi is a biotroph pathogen that we continuously maintain on soybean. To do so, 3-week-old plants are inoculated with freshly harvested P. pachyrhizi (isolate BR05) spores (1 mg mL−1 in water containing 0.02% Tween 20) and incubated for 24 h in the dark at saturating humidity. Afterwards, plants are kept in a growth chamber until uredosori form on the abaxial leaf side. DS01 and THA (Biomatik) and bifunctional peptide DS01–THA (Numaferm) were custom synthesized.
Growth of plants under field conditions.
Soybean and barley (cv. Sissy) plants were grown in pots (1 L volume) in a soil/sand/perlite mixture (1.0
:
1.2
:
0.3) under field conditions for 2–4 weeks with regular fertilization and watering. Climate data (global radiation, temperature, relative humidity, rainfall) were recorded. Mean meteorological parameters in June 2017 were 220 W m−2 global radiation, 21 °C, 66% relative humidity and 26 rain events with 10 heavy rainfalls (>5 mm h−1).
eGFP and eGFP-anchor peptide fusions
Generation of constructs.
eGFP-anchor peptide fusions consisted of N-terminal His6-eGFP and a C-terminal anchor peptide (LCI, LFB, TA2, or THA). eGFP and the adequate anchor peptide were linked by a stiff spacer helix of 17 amino acids (AEAAAKEAAAKEAAAKA)43 and a tobacco etch virus (TEV) protease cleavage site of 7 amino acids (ENLYFQG).44 eGFP-anchor dipeptide constructs were generated by phosphorothioate-based ligase-independent gene cloning as described.14 Synthetic genes (DS01, LCI, LFB, TA2, and THA) (ESI, Table S1†) and the pET28a(+)::His6-eGFP backbone were amplified in separate PCR reactions (ESI, Table S2†) using primer pairs F-pET28a/R-pET28a and F-anchor peptide/R-anchor peptide (ESI, Table S3†) with complementary phosphorothioated nucleotides at the 5′ end. eGFP (His6-eGFP-17x helix-TEV) was generated by introducing two stop codons (TAATAA) at the 3′ end of the TEV cleavage site using pET28a(+)::His6-eGFP-17x helix-TEV-TA2 as a template.14 After hybridization, the plasmid was transformed into E. coli BL21-Gold (DE3) cells. Successful cloning of pET28a::His6-eGFP-17x-TEV-anchor peptide coding sequence and eGFP was confirmed by sequencing.
Production of eGFP and eGFP-anchor peptides.
eGFP, eGFP–LFB, eGFP–LCI, eGFP–TA2, and eGFP–THA were produced in E. coli BL21 (DE3) gold cells. Bacterial pre-cultures were grown in 10 mL LB medium (10 g L−1 tryptone, 5 g L−1 yeast extract, 10 g L−1 NaCl, 0.1 mM kanamycin) for 16 h at 37 °C, 250 rpm and 70% rel. humidity on an orbital shaker. 100 mL main cultures were grown in TB medium (12 g L−1 peptone, 24 g L−1 yeast extract, 4 mL L−1 glycerol, 2.31 g L−1 KH2PO4, 12.54 g L−1 K2HPO4, 0.1 mM kanamycin) to an OD600 = 0.6 under the same conditions. Protein synthesis was induced by adding isopropyl β-D-1-thiogalactopyranoside (IPTG) at 0.1 mM (final concentration). Upon IPTG addition, cultivation temperature was reduced to 20 °C. Cells were harvested 40 h after IPTG addition by centrifugation (3200g, 10 min, 4 °C) and stored at −20 °C. The pellet was suspended in 50 mM Tris/HCl buffer (pH 8.0) and cells were disrupted by sonication on ice (3 × 30 s, interval 30 s, 70% amplitude). Soluble proteins were separated by centrifugation (3200g, 15 min, 4 °C). Supernatants were filtered through a 0.45 μm cellulose acetate filter and used for leaf binding assays.
Purification of eGFP and eGFP-anchor peptides.
eGFP and the dipeptides eGFP–LCI and eGFP–THA contained a N-terminal His6 tag (see the above text). They were purified by affinity chromatography on a HiTrap™ HP column (GE Healthcare) using 50 mM Tris/HCl buffer (pH 8.0) and gradual elution with 0.5 M imidazole in 50 mM Tris/HCl buffer (pH 8.0). Purified proteins were dialyzed against deionized water. The protein concentration was determined using a NanoDrop 2000 (Thermo Scientific) and was based on the extinction coefficient and the molecular weight (ESI, Table S4†). Protein purity was checked by SDS-PAGE using a 5% stacking gel and 12% separating gel (ESI, Fig. S5†).
Visualization of the LCI and THA surface.
The molecular structure of LCI and THA was displayed using the NGL Viewer of protein data bank RCSB PDB (http://www.rcsb.org).46 The peptide structure is shown in the surface style. The color code indicates overall hydrophobicity with hydrophilic parts of the adequate peptide being displayed in red whereas hydrophobic regions are shown in green. Display settings were as follows: full structure assembly, model 1, no symmetry, surface style, and color by hydrophobicity, no ligand, high quality, no water, ions, hydrogens, no clashes, and perspective camera. LCI (PDB ID: 2b9k); THA (PDB ID: 8tfv).47
Detection of green fluorescence
eGFP fluorescence was detected using a Leica TCS SP fluorescence microscope with an epifluorescence filter (cube A-513804, 340–380 nm excitation, 425 nm emission). Photos were taken with a digital camera. In experiments with intact leaves, eGFP fluorescence was detected using a Leica TCS SP spectral confocal laser-scanning microscope. eGFP fluorescence was recorded at 488 nm excitation and 505–525 nm emission. A Leica x20 HCX PL FLUOTAR (numerical aperture 0.5) lens was used for micrograph production. A series of optical sections (z-stack) was acquired by scanning multiple sections. Images were processed and analyzed using Leica confocal software. For the display of data in the same picture, confocal images are presented as 2D projections of 3D image stacks along the orthogonal axis.
Analysis of eGFP-anchor peptide binding to the plant surface
eGFP-anchor peptide binding to soybean leaf discs.
Leaf discs (1 cm diameter) of 3-week-old soybean plants were floated on E. coli lysates in microtiter plates containing recombinant eGFP, eGFP–LCI, eGFP–LFB, eGFP–TA2, and eGFP–THA (all at 750 nM). After 10 min, leaf discs were thoroughly washed in water and subjected to eGFP fluorescence detection as described (see the above text).
Binding of eGFP–LCI and eGFP–THA to soybean, barley, and corn leaves.
Purified eGFP–THA and eGFP–LCI fusion peptides (750 nM) were sprayed onto leaves using a commercial diffuser (Carl Roth). Spraying eGFP (750 nM) served as a control. After drying off, the leaves were thoroughly rinsed by spraying with 50 mL deionized water. Green fluorescence was detected using confocal microscopy.
Long-term binding of eGFP–THA to leaves of soybean plants under semi-field conditions.
Leaflets of the first trifolium of three soybean plants were sprayed with eGFP–THA (750 nM). After 2 h on the bench, the plants were transferred to the outside and kept under semi-field conditions for 17 d (July 6–23, 2018) on a plot at RWTH Aachen University's Biology Department (geographic coordinates: longitude 6.047018, latitude 50.778272, altitude 204 meters; global positioning system: 50°46′41.779′′N 6°2′49.265′′E). eGFP–THA abundance on leaflets was examined at the day of treatment (0 dpa) and after 3, 7, and 17 days. Meteorological data during the field experiment were retrieved from http://www.wetterkontor.de.
Fusion peptide binding
Binding in the field and at natural rainfall.
The rainfastness of eGFP–THA and eGFP–LCI was evaluated on the upper side of soybean (16-d-old at the time of treatment) and barley (26-d-old at the time of treatment) leaves. 5–7 droplets of peptide solution (1 μM eGFP–THA, 5 μM eGFP–LCI, and 5 μM eGFP) were applied. After drying off, photos of peptide deposits were taken by nondestructive fluorescence microscopy. Images were recorded using a Nuance Multispectral Fluorescence Imaging System (PerkinElmer), which is integrated in a stereo microscope (Carl Zeiss MicroImaging). Fluorescence images were recorded under standardized conditions in the dark at 25 °C using the full CCD frame. Photos were taken using a 0.8 X Zeiss Neo Lumar lens, 30× magnification with an object field of 17.4 mm2 and a Lumar filter 09FITC at 530 nm (green, eGFP emission) and at 680 nm (red, chlorophyll emission). After taking the photos, plants were returned to the field and exposed to natural rainfall. Three days later, fluorescence photos were taken again. Peptide residues (deposit area) before and after rainfall were quantified using the software ImageJ.
Binding to the surface wax layer.
Leaves of the wild type and cer-j59 mutant of barley (Nødskov Giese, 1976) were sprayed with aqueous solutions of eGFP–THA, eGFP–LCI, or eGFP (all at 750 nM). From leaves of an aliquot of corn plants, the surface wax layer was removed by coating with super glue (Uhu Plus Superfast 45700 two-component epoxy resin glue). Upon drying off, the glue was carefully removed using forceps as described.45 Using microscopy, we ensured that this procedure removes the epicuticular wax layer without apparent harm of epidermal cells. Leaf areas with an intact epicuticular wax layer and areas with the surface wax layer removed were sprayed with eGFP–THA, eGFP–LCI, and eGFP (all at 750 nM). Upon drying off, the sprayed leaf areas were intensively rinsed with water. eGFP fluorescence was detected by confocal microscopy.
Antifungal activity assays
In vitro assay.
Freshly harvested P. pachyrhizi uredospores were suspended in water by intense shaking. For the in vitro assays, spores were used at a density of 1 mg spores per mL. Uredospores were sprayed on glass slides coated with polyethylene foil. Slides were kept at saturated humidity for 18 h. Germination of spores and fungal development were evaluated by counting developing appressoria in the presence of water (control), DS01, THA, or DS01–THA (all at 10 μM).
Evaluation of soybean protection from SBR.
Trifolia were sprayed with water or aqueous solutions of DS01, THA, or DS01–THA (all at 25 μM). After drying off for 2 h, trifolia were inoculated with P. pachyrhizi spores in water (1 mg mL−1). To investigate whether DS01–THA would resist rinsing with water, an aliquot of leaflets sprayed with water or DS01–THA was thoroughly rinsed with water before fungal inoculation. At least 50 mL water were used for rinsing one trifolium. Trifolia were then inoculated with P. pachyrhizi (1 mg spores per mL water) and plants were kept in the dark at saturated humidity. After 24 h, plants were transferred to a growth chamber. Disease severity was evaluated at 10 dpi by measuring the lesion size with a color scanner (Canon CanoScan LiDe 220). Scans were assessed using Assess 2.0 software (APS).
Determination of photosynthetic activity
Soybean leaves were analyzed using an imaging pulse-amplitude modulated (PAM) chlorophyll fluorometer and ImagingWin v2.40b (Heinz Walz GmbH). For each image, four leaf areas were randomly selected. The mean electron transfer rate of the four areas was used for analysis of variance. Measurements were done with plants in a growth chamber (23 °C, 60% relative humidity, photoactive radiation PAR = 308 ± 7 μmol m−2 s−1). Day/night cycles were 12 h/12 h. Leaves were treated with 50 μM DS01–THA dipeptide or 0.3% Maneb (80% in water). Treatment with water served as a control. Five droplets were placed onto ∼2 cm2 surface of 14-d-old leaves. PAM measurement was done at 1 d after treatment.
Author contributions
All authors designed the research; P. S., S. P., M. R., L. A., M. H., and C. L. performed the research; all authors analyzed the data; U. C. and U. S. wrote the paper; and P. S., S. P., F. J., L. S., G. N., and C. L. revised the manuscript.
Conflicts of interest
A patent application covering the presently described work has been filed by RWTH Aachen University.
Acknowledgements
We thank Ulrich Schaffrath for cer-j59, Holger Deising for the C. graminicola strain, Lukas Schreiber for discussion, and Maren Schwinges for composing the table of contents entry. We also appreciate provision of DS01–THA by Numaferm. The scientific activities of the Bioeconomy Science Center were financially supported by the Ministry of Innovation, Science and Research in the NRW Strategy Project BioSC (No. 313/323-400-00213).
References
- M. Enserink, P. J. Hines, S. N. Vignieri, N. S. Wigginton and J. S. Yeston, Science, 2013, 341, 729 CAS.
- E.-C. Oerke, J. Agric. Sci., 2005, 144, 31 CrossRef.
- A. Mascarelli, Science, 2013, 341, 740 CrossRef CAS PubMed.
- V. A. Rauh, F. P. Perera, M. K. Horton, R. M. Whyatt, R. Bansal, X. Hao, J. Liu, D. Boyd Barr, T. A. Slotkin and B. S. Peterson, Proc. Natl. Acad. Sci. U. S. A., 2012, 109, 7871 CrossRef CAS PubMed.
- M. Hunsche, K. Bringe, M. Schmitz-Eiberger and G. Noga, Pest Manage. Sci., 2006, 62, 839 CrossRef CAS PubMed.
- G. H. Willis and L. L. McDowell, Rev. Environ. Contam. Toxicol., 1987, 100, 23 CrossRef CAS.
- P. A. E. Alarcón and S. A. Lambertucci, Science, 2018, 360, 612 Search PubMed.
- B. A. Woodcock, N. J. B. Isaac, J. M. Bullock, D. B. Roy, D. G. Garthwaite, A. Crowe and R. F. Pywell, Nat. Commun., 2016, 7, 12459 CrossRef CAS PubMed.
- C. Lamberth, S. Jeanmart, T. Luksch and A. Plant, Science, 2013, 341, 742 CrossRef PubMed.
- J. M. Bolivar, V. Gascon, C. Marquez-Alvarez, R. M. Blanco and B. Nidetzky, Langmuir, 2017, 33, 5065 CrossRef CAS PubMed.
- K. Rübsam, B. Stomps, A. Böker, F. Jakob and U. Schwaneberg, Polymer, 2017a, 116, 124 Search PubMed.
- K. Rübsam, L. Weber, F. Jakob and U. Schwaneberg, Biotechnol. Bioeng., 2017b, 115, 321 Search PubMed.
- A. B. Sanghvi, K. P. H. Miller, A. M. Belcher and C. E. Schmidt, Nat. Mater., 2005, 4, 496 CrossRef CAS PubMed.
- K. Rübsam, M. D. Davari, F. Jakob and U. Schwaneberg, Polymer, 2018, 10, 423 Search PubMed.
- N. Rangarajan, S. Bakshi and J. C. Weisshaar, Biochemistry, 2013, 52, 6584 CrossRef CAS PubMed.
- A. Tossi, L. Sandri and A. Giangaspero, Biopolymers, 2000, 55, 4 CrossRef CAS PubMed.
- M. Zasloff, Nature, 2002, 415, 389 CrossRef CAS PubMed.
-
H. Bargel, W. Barthlott, K. Koch, L. Schreiber and C. Neinhuis, in The Evolution of Plant Physiology, ed. A. R. Hemsley and I. Poole, Elsevier, London, 2004, p. 171 Search PubMed.
- K. Koch and H.-J. Ensikat, Micron, 2008, 39, 759 CrossRef CAS PubMed.
- G. D. Brand, J. R. Leite, L. P. Silva, S. Albuquerque, M. V. Prates, R. B. Azevedo, V. Carregaro, J. S. Silva, V. C. Sá, R. A. Brandão and C. Bloch Jr., J. Biol. Chem., 2002, 277, 49332 CrossRef CAS PubMed.
- G. D. Brand, J. R. Leite, S. M. de Sá Mandel, D. A. Mesquita, L. P. Silva, M. V. Prates, E. A. Barbosa, F. Vinecky, G. R. Martins, J. H. Galasso, S. A. Kuckelhaus, R. N. Sampaio, J. R. Furtado Jr., A. C. Andrade and C. Bloch Jr., Biochem. Biophys. Res. Commun., 2006, 347, 739 CrossRef CAS PubMed.
- A. J. De Lucca, J. M. Bland, T. J. Jacks, C. Grimm and T. J. Walsh, Med. Mycol., 1998, 36, 291 CrossRef CAS PubMed.
- J. R. Leite, G. D. Brand, L. P. Silva, S. A. Kückelhaus, W. R. Bento, A. L. Araújo, G. R. Martins, A. M. Lazzari and C. Bloch Jr., Comp. Biochem. Physiol., Part A: Mol. Integr. Physiol., 2008, 151, 336 CrossRef PubMed.
- A. Mor, Drug Dev. Res., 2000, 50, 440 CrossRef CAS.
- L. C. Salay, T. M. Nobre, M. C. Colhone, M. E. Zaniquelli, P. Ciancaglini, R. G. Stabeli, J. R. Leite and V. Zucolotto, J. Pept. Sci., 2011, 17, 700 CrossRef CAS PubMed.
-
The Soybean: Botany, Production and Uses, ed. G. Singh, CAB International, Oxfordshire, 2010 Search PubMed.
- C. Langenbach, R. Campe, S. F. Beyer, A. Müller and U. Conrath, Front. Plant Sci., 2016, 7, 797 Search PubMed.
- E. Pennissi, Science, 2010, 327, 804 CrossRef PubMed.
- E. Stokstad, Science, 2004, 306, 1672 CrossRef CAS PubMed.
- C. V. Godoy, C. D. S. Seixas, R. M. Soares, F. C. Marcelino-Guimarães, M. C. Meyer and L. M. Costamilan, Pesqui. Agropecu. Bras., 2016, 51, 407 CrossRef.
- C. V. Godoy, A. F. Bueno and D. L. P. Gazziero, Outlooks Pest Manage., 2015, 26, 113 CrossRef.
- M. Zimmer, Chem. Rev., 2002, 102, 759 CrossRef CAS PubMed.
- T. Osaki, M. Omotezako, R. Nagayama, M. Hirata, S. Iwanaga, J. Kasahara, J. Hattori, I. Ito, H. Sugiyama and S. Kawabata, J. Biol. Chem., 1999, 274, 26172 CrossRef CAS PubMed.
- W. Gong, J. Wang, Z. Chen, B. Xia and G. Lu, Biochem., 2011, 50, 3621 CrossRef CAS PubMed.
- P. Fehlbaum, P. Bulet, S. Chernysh, J. P. Briand, J. P. Roussel, L. Letellier, C. Hetru and J. A. Hoffmann, Proc. Natl. Acad. Sci. U. S. A., 1996, 93, 1221 CrossRef CAS.
- E. M. Jones, A. Smart, G. Bloomberg, L. Burgess and M. R. Millar, J. Appl. Bacteriol., 1994, 77, 208 CrossRef CAS PubMed.
-
Domestication of Plants in the Old World, ed. D. Zohary, M. Hopf and E. Weiss, Oxford University Press, 4th edn, 2012 Search PubMed.
-
L. Campbell Franklin, in The Oxford Encyclopedia of Food and Drink in America, ed. A. F. Smith, Oxford University Press, 2nd edn, 2013, p. 551 Search PubMed.
- B. Nødskov Giese, Hereditas, 1976, 82, 137 CrossRef.
- G. D. Brand, M. T. Q. Magalhães, M. L. P. Tinoco, F. J. L. Aragão, J. Nicoli, S. M. Kelly, A. Cooper and C. Bloch Jr., PLoS One, 2012, 7, e45848 CrossRef CAS PubMed.
- F. Dalla Lana, P. K. ZiegelmannK, A. de H. N. Maia, C. V. Godoy and E. M. Del Ponte, Phytopathol., 2015, 105, 307 CrossRef PubMed.
- O. Hagedorn, I. Fleute-Schlachter, H. G. Mainx, V. Zeisler-Diehl and K. Koch, Beilstein J. Nanotechnol., 2017, 8, 2345 CrossRef CAS PubMed.
- R. Arai, H. Ueda, A. Kitayama, N. Kamiya and T. Nagamune, Protein Eng., 2001, 14, 529 CrossRef CAS PubMed.
- R. B. Kapust, J. Tozser, J. D. Fox, D. E. Anderson, S. Cherry, T. D. Copeland and D. S. Waugh, Protein Eng., 2001, 14, 993 CrossRef CAS PubMed.
- G. Powell, S. P. Maniar, J. A. Pickett and J. Hardie, Entomol. Exp. Appl., 1999, 91, 115 CrossRef.
- A. S. Rose, A. R. Bradley, Y. Valasatava, J. D. Duarte, A. Prlić and P. W. Rose, Bioinformatics, 2018, 34, 3755 CrossRef PubMed.
- N. Mandard, P. Sodano, H. Labbe, J. M. Bonmatin, P. Bulet, C. Hetru, M. Ptak and F. Vovelle, Eur. J. Biochem., 1998, 256, 404 CrossRef CAS PubMed.
Footnotes |
† Electronic supplementary information (ESI) available. See DOI: 10.1039/c9gc00457b |
‡ Present address: Swammerdam Institute for Life Sciences, University of Amsterdam, Amsterdam 1098 XH, The Netherlands. |
§ Present address: COMPO Expert GmbH, 10 Krögerweg, Münster 48155, Germany. |
|
This journal is © The Royal Society of Chemistry 2019 |