DOI:
10.1039/D3QI00983A
(Research Article)
Inorg. Chem. Front., 2023,
10, 7212-7221
Surface functionalization of discrete metal-chalcogenide supertetrahedral clusters and the photocatalytic application†
Received
26th May 2023
, Accepted 15th October 2023
First published on 19th October 2023
Abstract
Atomically precise metal-chalcogenide supertetrahedral clusters (MCSCs) are supposed to be more attractive for functionalization than conventional metal-sulfide quantum dots owing to their potential ability to establish precise structure–composition–property relationships. However, the accurate surface functionalization of such cluster-based species remains difficult. In this paper, we present a facile method for synthesizing discrete MCSCs decorated with different functional groups via a one-step solvothermal reaction, which was demonstrated to have better solvent dispersibility compared with ligand-free ones. In addition, the composites were also prepared by combining ligand-free clusters (or ligand-partially protected or amino-modified ones) with two-dimensional MXene nanosheets. The composites derived from amino-modified clusters exhibited optimal performance of photocatalytic hydrogen evolution. Furthermore, the hydrogen bonding interactions between modified amino groups and MXene nanosheets were verified by 1H-NMR spectroscopy. This work provides a facile approach for the surface functionalization of MCSCs, and facilitates the expansion of the functionality of atomically precise nano-species.
Introduction
Discrete metal-chalcogenide supertetrahedral clusters (MCSCs), known as ultra-small “quantum dots”, represent regularly fragmented units of zinc blende type materials.1–6 Since their structures can be accurately identified at the atomic level, they have been widely studied in the fields of fluorescence,7–13 photocatalysis,14–20 and electrocatalysis21–25 for establishing an accurate structure–composition–property relationship. However, such clusters usually exhibit poor dispersibility and conductivity during practical applications, and the weak binding forces between clusters and conductive substrates limit their further development.26–32 Therefore, rational modification of clusters for improving the interactions between clusters and conductive substrates would be helpful for their applications in catalysis.
Owing to the advancement of organic template-guided synthesis, most of the discrete Tn and Pn clusters (two main types of MCSC structures, where n represents the number of metal layers in the basic cluster units, Scheme S1†) reported in recent years are predominantly ligand-free types.29 The high-degree negative charges of these clusters are usually balanced by protonated organic amines (i.e., counterions), which results in poor dispersibility and instability. Alternatively, a group of Cn clusters (fully coated with organic ligands, Scheme S1†) has superior dispersibility. However, their monomeric composition and poor structural tunability make them a suboptimal choice for subsequent functional applications. Therefore, by combining the advantages of the above two types of structures, a series of ligand-partially protected Tn and Pn clusters has been developed (Scheme S2†). The few ligands at the cluster surface help to improve their contact with the solution molecules and reduce their negative charge density, thereby improving structural stability and dispersibility. This feature is well-suited for functional expansion, and makes them a promising choice.
Since MCSCs generally exhibit poor electrical conductivity, combining them with a suitable conductive substrate is desirable for promoting the separation of the photogenerated electrons and holes, which may effectively enhance photocatalytic performance. In recent decades, MXenes, a layered transition metal carbide/carbonitride/nitride family, have attracted attention in many scientific fields, such as energy storage and conversion,33 sensing,34 and photocatalysis.35–38 In the domain of photocatalytic hydrogen evolution (PHE), Ti3C2Tx is one of the most studied MXenes for the following reasons: (1) the favorable hydrophilic ability supports its chemical reactions within aqueous solutions;39 (2) the excellent electrical conductivity promotes the separation and transfer of the photoinduced charge carriers from semiconductors;40 and (3) the appropriate work function allows it to form a Schottky heterojunction with most semiconductors. Therefore, the ultra-thin Ti3C2Tx substrate is regarded as a suitable and promising substrate, in which abundant surface O/F sites can also serve as potential hydrogen bonding receptors for anchoring discrete MCSCs.
This work aims to improve the interactions between a cluster and a conductive substrate by manipulating the cluster surface environment, and eventually, improve the performance of photocatalytic hydrogen evolution (PHE). On the basis of the synthetic route of Pn-type clusters that are partially protected by ligands,32 we changed the types of diphenyl disulfides to modify the cluster surface with different functional groups (Scheme 1). By taking advantage of the large number of O/F sites on the surface of MXene, which can easily form hydrogen bonds with amino-modified clusters, the PHE performance of amino-modified MCSCs (and ligand-free clusters) combined with MXene nanosheets was investigated.
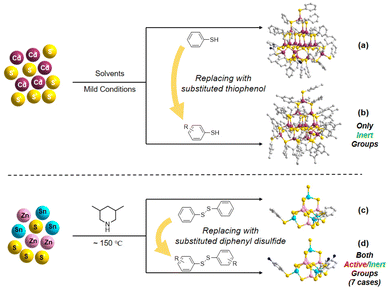 |
| Scheme 1 Schematics of the surface functionalization of discrete MCSCs: (a and b) using the modified thiophenol and (c and d) using modified diphenyl disulfide. | |
Experimental
Materials
All chemicals were purchased from commercial sources and used without further purification. Deionized water was used in all synthetic procedures.
Synthetic procedures
Synthesis of ISC-24, 10(3,5-DMPH+)·[(Zn4Sn4S17)10−]·4H2O.
The synthetic procedure for ISC-24 followed our previous work,32 except that the manganese source was replaced by a zinc source. A mixture of zinc acetate tetrahydrate (Zn(OAc)2·4H2O, 120 mg), tin dichloride (SnCl2, 60 mg), sulfur powder (S, 120 mg), 3,5-dimethylpiperidine (3,5-DMP, 3.0 mL), and deionized water (H2O, 1.0 mL) was added to a 25 mL Teflon-lined stainless steel autoclave and stirred for 30 min, then was heated to 125 °C for 7 days. After cooling down to room temperature, transparent colorless block crystals were obtained with a yield of 19.4% based on zinc acetate dihydrate.
Synthesis of ISC-25, 7(3,5-DMPH+)·[Zn4Sn4S14(SPh)37−]·H2O.
The synthetic procedure for ISC-25 followed our previous work,32 except that the manganese source was replaced by a zinc source. Zinc acetate tetrahydrate (Zn(OAc)2·4H2O, 120 mg), tin dichloride (SnCl2, 60 mg), sulfur powder (S, 120 mg), diphenyl disulfide (Ph2S2, 160 mg), 3,5-dimethylpiperidine (3,5-DMP, 3.0 mL), and deionized water (H2O, 1.0 mL) were mixed in a 25 mL Teflon-lined stainless steel autoclave and stirred for 30 min, then heated to 125 °C for 14 days. After cooling down to room temperature, transparent colorless rhombohedral crystals were obtained with a yield of 16.8% based on zinc acetate dihydrate.
Synthesis of ISC-25-4′-NH2, 7(3,5-DMPH+)·{[Zn4Sn4S14(SPhNH2)3]7−}·H2O.
A mixture of zinc acetate tetrahydrate (Zn(OAc)2·4H2O, 120 mg), tin dichloride (SnCl2, 60 mg), sulfur powder (S, 120 mg), di-(4,4′-amino)-diphenyl disulfide (S2(NH2Ph)2, 160 mg), and deionized water (H2O, 1.0 mL) was added to a 25 mL Teflon-lined stainless steel autoclave and stirred for 30 min, then heated to 125 °C for 14 days. After cooling down to room temperature, oblique octahedral or hexagonal transparent crystals were obtained with a yield of 12.6% based on zinc acetate dihydrate.
Synthesis of other ISC-25 and ISC-26 series samples with different decorated functional groups.
Similar to the synthetic processes described above, the ligand of Ph2S2 was replaced by substituted Ph2S2 (e.g. (ClPh)2S2, the categories are summarized in Table S2†) in the same molar ratio with the other components unchanged.
Preparation of Ti3C2Tx nanosheets.
Ti3C2Tx nanosheets (here T represents the oxygen terminus generated by HF etching) were synthesized according to the reported method (Schemes S2 and S3†).41 Ti3AlC2 powders (>98 wt% purity; particle size <75 μm, i.e., 200 mesh) were immersed in HF (10 wt%) solution at 300 K by stirring for 24 h. Then the resulting suspension was washed several times with deionized water and centrifuged to remove the remaining impurities and HF until the pH value of the solution reached 5–6. The wet sediment was washed twice with ethanol and transferred to a wide-necked jar to air-dry for 3–4 days. The finally obtained sample was named Ti3C2Tx. The multilayered Ti3C2Tx was then stirred with dimethyl sulfoxide (DMSO) at 310 K for 24 h. The colloidal suspension was centrifuged to obtain the intercalated powders. The powders were then washed several times with deionized water to remove the residual DMSO. Next, the residue was dispersed in deionized water in a weight ratio of Ti3C2Tx to water of 1
:
100. Afterward, ultrasonication treatment was carried out for 4 h to exfoliate the Ti3C2Tx powder into 2-D sheets under argon. The suspension was centrifuged at 3500 rpm for 10 min to remove the unexfoliated MXenes. Finally, the Ti3C2Tx nanosheets were obtained by filtration and dried in a vacuum for further experiments.
Preparation of P1-CNPs (CNPs: cluster-derived nanoparticles).
P1-CNPs were prepared in the mixed solution of formamide and water (1
:
1 in volume). The corresponding crystals of 10 mg of ISC-24, ISC-25, and ISC-25-4′-NH2 were ground into powder and dispersed in 10 mL of 1
:
1 formamide/water. After 30 min of ultrasonication treatment, the formamide/water solution of P1-CNPs with a concentration of 1 mg mL−1 was prepared by mechanical stirring for 4 h.
Preparation of CMn samples.
CMn samples were prepared by mixing 1 mg mL−1 of the corresponding P1-CNP formamide aqueous solution with the one containing the ultra-thin Ti3C2Tx nanosheets according to the mass percentage (CM0 is P1-CNPs without Ti3C2Tx nanosheets).
Results and discussion
Synthetic procedure and crystal structure description
The title compounds constructed from supertetrahedral P1-ZnSnS clusters were obtained by a facile one-step solvothermal reaction (see the Experimental section and the ESI† for details). To directly realize the functionalization of the partially protected P1-ZnSnS, various diphenyl disulfides decorated with different functional groups were selected, and the synthetic routine and molecular structure of the relevant compounds are shown in Fig. 1.
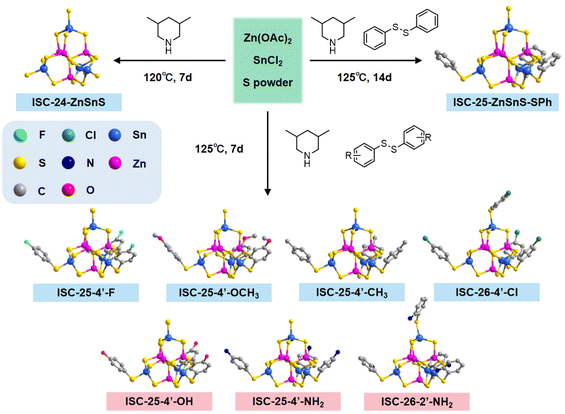 |
| Fig. 1 Synthetic processes and molecular structures of ISC-24, ISC-25, and ISC-25&26 with different functional groups. The products with functional groups have been distinguished by different colored backgrounds in comment boxes (light blue: inert functional groups, e.g., –CH3, –OCH3, –Cl, –F; pink: active functional groups: e.g., –OH, –NH2). | |
The obtained clusters mentioned in this work are all composed of the [Zn4Sn4S17]10− core, so the functionalized samples are represented in a simplified form (e.g., ISC-25-ZnSnS-SPh is simplified to ISC-25, and the functionalized ISC-25 are represented as the site of the substituent on their benzene ligand, e.g., ISC-25-4′-NH2). The derivatives of ISC-26 are also showcased in a similar pattern. The structural difference between ISC-25 and ISC-26 is the number of protecting ligands (three-corner protected in ISC-25 and four-corner protected in ISC-26, Fig. 1).
Interestingly, ISC-26-2′-NH2 was obtained using 2,2′-dinitrodiphenyl disulfide, instead of 2,2′-diaminodiphenyl disulfide. This may be attributed to excess sulfur powder in the reaction system, which reduces the nitro group to the amino group. A similar situation also occurs in ISC-25-4′-NH2, while ISC-25-4′-NH2 can also be obtained by directly using 4,4-diaminodiphenyl disulfide. This process is also shown in detail in Table S2.†
A series of functionalized crystalline ISC-25 and ISC-26 samples was obtained in the transparent rhombohedral form, but ISC-26-4′-Cl appeared in the form of a transparent decagonal octahedron. Single crystal X-ray structural analysis revealed that the ISC-24 sample crystallized in P
3n (No. 218), all ISC-25 series samples and ISC-26-2′-NH2 crystallized in the R
space group (No. 148), and the ISC-26-4′-Cl sample crystallized in the C2/c space group (No. 15). These functionalized clusters are basically isomorphic to the structures previously reported.32 Combined with the results of C, H, and N elemental analysis of ISC-24, ISC-25, and ISC-25-4′-NH2 (Table S3†) and their SCXRD data (Table S1†), the formulas of the above three compounds were determined as follows: ISC-24: 10(3,5-DMPH+)·[(Zn4Sn4S17)10−]·H2O; ISC-25: 7(3,5-DMPH+)·{[Zn4Sn4S14(SPh)3]7−}·H2O and ISC-25-4′-NH2: 7(3,5-DMPH+)·{[Zn4Sn4S14(SPhNH2)3]7−}·H2O. Compared to the P1 cluster reported previously, the cluster reported here retained the pristine atomic arrangement of the divalent and tetravalent metal ions, i.e., each P1-ZnSnS contained one (MII4S)6+ and four (MIVS4)4− units to meet Pauling's electrostatic valence rule.
Morphology of Ti3C2Tx nanosheets and dispersed P1-CNPs
Layered Ti3C2Tx nanosheets were prepared by exfoliating bulk Ti3AlC2 powders in HF aqueous solution. The corresponding structure information for Ti3AlC2 and the preparation process are shown in Schemes S3 and S4.† After immersing them in HF solution for 24 h, the Ti3AlC2 powders (200 mesh) were successfully expanded and converted to the layered Ti3C2Tx structure (Fig. 2a). The emergence of layers was caused by the fact that the Al layers in Ti3AlC2 nanoparticles were etched away, and the interlayer space was increased by released H2. After intercalation by dimethyl sulfoxide (DMSO), the layered structures were further delaminated into 2D Ti3C2Tx nanosheets by ultrasonication (Fig. 2b). The AFM image in Fig. 2c shows that the thickness of Ti3C2Tx nanosheets is about 4 nm. In addition, PXRD characterization was performed on the Ti3AlC2 MAX phase and Ti3C2Tx nanosheets to distinguish the outcome of Al etching. As shown in Fig. 2d, there are a number of characteristic peaks at 2θ = 9.95°, 19.50°, 34.38°, 37.49°, 39.21°, 42.13°, 48.72°, and 56.66°, which correspond to the (002), (004), (101), (103), (104), (105), (107), and (109) planes of the Ti3AlC2 MAX phase. This result is in agreement with the one in the published literature.42,43 The degree of the (002) plane in PXRD is generally used to calculate the interlayer spacing of the layered material by applying Bragg's law, and the d-spacing of the Ti3AlC2 MAX phase is calculated to be approximately 5.0 Å. Ti3C2Tx MXene nanosheets show a (002) peak at 2θ = 7.42°, referring to a d-spacing of 13.6 Å (Fig. 2d). It is clearly demonstrated that the Al layer in the Ti3AlC2 MAX phase has been well etched, which allows water molecules to further exfoliate the Al layer for preparing single-layered Ti3C2Tx MXene nanosheets.45
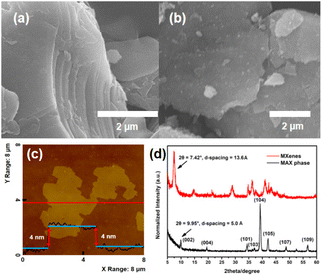 |
| Fig. 2 SEM images of layered (a) and exfoliated Ti3C2Tx nanosheets (b) obtained by HF treatment of Ti3AlC2 powder; (c) AFM images of dispersed single-layered Ti3C2Tx nanosheets; and (d) PXRD results of Ti3AlC2 powder and exfoliated Ti3C2Tx nanosheets. | |
The nanoparticles generated from the cluster dispersion are denoted as P1-CNPs (CNPs: cluster-derived nanoparticles) in this work. Three examples of clusters were selected for comparison to test their dispersibility: ISC-24 without ligand protection, ISC-25 with a partially protected ligand, and ISC-25-4′-NH2 with a partially protected ligand modified with amino (–NH2) groups. The corresponding P1-CNP solutions were prepared by adding the ground crystals of the above three compounds to the formamide/water solution (1
:
1), followed by stirring for 4 h and sonicating for 1 h. The particle sizes of P1-CNPs formed from ISC-24 were found to be 25–55 nm (Fig. 3a, mean size ∼40 nm), which far exceeded the size of individual clusters (∼1.6 nm). This suggests that the dispersed particles existed in the aggregated form of multi-clusters. In contrast, the mean particle sizes of P1-CNPs formed by ligand-partially protected ISC-25 and amino-modified ISC-25-4′-NH2 were found to be 4–7 nm and 1–4 nm, respectively (Fig. 3b and c). The latter two were significantly more accessible to solution. This can be explained by the low negative charge of the clusters and the improved solubility.
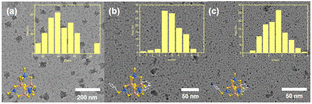 |
| Fig. 3 TEM images of P1-CNPs formed by (a) ISC-24; (b) ISC-25, and (c) ISC-25-4′-NH2, and the insets show the size distribution and the molecular structures of the corresponding clusters. | |
In addition, no effective lattice striations were observed in the HRTEM images of the P1-CNPs formed by the dispersed clusters. This suggests that P1-CNPs have adopted an amorphous state, which is probably due to the small size of the individual clusters (∼1.6 nm).
Fabrication and morphology of P1-CNPs/Ti3C2Tx nanocomposites (CMn)
The nanocomposites consisting of P1-CNPs and exfoliated Ti3C2Tx are denoted as CMn (n indicates the weight percentage of the added Ti3C2Tx, n = 0 indicates that no Ti3C2Tx was added). The fabrication process for the composite is shown in Scheme S4.† The results of PXRD characterization of CM1 (composed of ISC-25-4′-NH2 derived CM0 and Ti3C2Tx) are shown in Fig. S11.†
Due to the low loading amount and low crystallinity, only the diffraction peaks of MXene nanosheets were observed. In order to characterize the morphology and structure of CMn, we performed HRTEM tests on the corresponding samples. Firstly, the morphology of Ti3C2Tx was characterized, and clear lattice stripes were observed as shown in Fig. 4a and b. However, for CM1, the lattice stripes were not detected in the HRTEM images (Fig. 4c and d). This was also confirmed by the selected area electron diffraction (SAED) characterization. As shown in Fig. S3a,† single-crystal diffraction patterns of Ti3C2Tx were observed when selected electron diffraction was performed on the regions shown in Fig. 4a and b, while the CM1 composites exhibited a polycrystalline form (Fig. S3b†), consistent with the states shown in Fig. 4c and d. In addition, we also performed HAADF-STEM characterization on CM1 prepared with ISC-25-4′-NH2 as the precursor, and performed EDS mapping tests (Fig. 4e–j). The constituents of Zn, Sn, S, and N in the clusters were uniformly distributed on the Ti3C2Tx nanosheets, demonstrating the successful preparation of the composites.
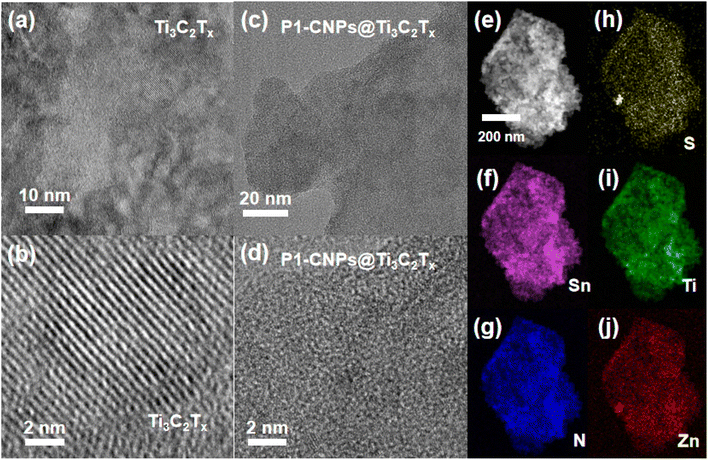 |
| Fig. 4 HRTEM images of MXene nanosheets (a and b) and ISC-25-4′-NH2 derived CM1 (c and d). HAADF-STEM image of P1-CNPs/Ti3C2Tx nanocomposites (e), and EDS elemental mapping images of (f) Sn, (g) N, (h) S, (i) Ti, and (j) Zn, respectively. | |
PHE performance of CMn
The photocatalytic performance of the above composites was tested by conducting PHE reactions under full-spectrum irradiation using the Na2S/Na2SO3 reagent as the sacrificial reagent. Firstly, ISC-25-4′-NH2 derived CM0 was selected for the PHE reaction, and the H2 production rate was calculated to be 17.51 μmol g−1 h−1 according to the data shown in Fig. 5a. This poor PHE performance could be attributed to the low separation efficiency of the photogenerated electron–hole pairs of CM0. Enhanced photocatalytic activities were obtained for the ISC-25-4′-NH2 derived samples containing a certain percentage of Ti3C2Tx (1%, 2.5%, 5%, and 10%, i.e., CM1, CM2.5, CM5, and CM10, respectively). The PHE rate from CM1 was calculated to be 158.32 μmol g−1 h−1 (Fig. 5a and b). This result indicates that the Ti3C2Tx nanosheets play an important role in separating the photogenerated electron–hole pairs for P1-CNPs. The PHE activity of CM2.5, CM5 and CM10 decreased to 113.37, 121.07, and 89.43 μmol g−1 h−1, respectively. These results were caused by the aggregation of Ti3C2Tx and P1-CNPs, which was verified by its TEM images (Fig. S4†). Under such situation, the Ti3C2Tx nanosheets partially mask the active sites of P1-CNPs, thereby leading to a reduced photocatalytic efficiency.
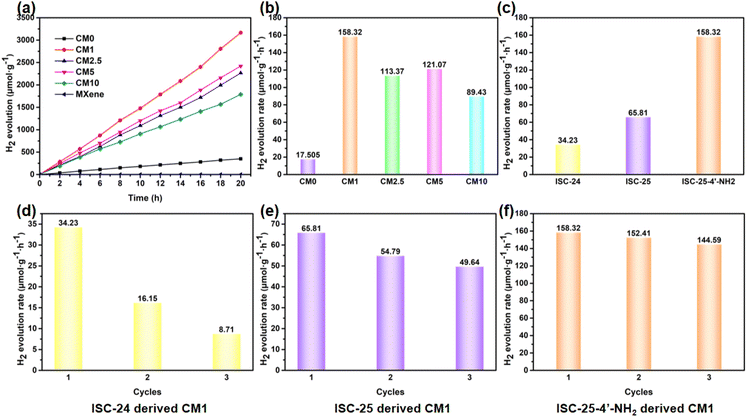 |
| Fig. 5 Investigation of photocatalytic properties of CMn. (a and b) Comparison of PHE performance of CM0, CM1, CM2.5, CM5, and CM10 nanocomposites under irradiation using ISC-25-4′-NH2 derived CMn. (c) Comparison of PHE performance of CM1 composite materials using ISC-24, ISC-25, and ISC-25-4′-NH2 derived CMn. (d–f) Test results of multiple cycles on CM1 composite materials using ISC-24, ISC-25, and ISC-25-4′-NH2 derived CMn. | |
The photocatalytic stability of ISC-24, ISC-25, and ISC-25-4′-NH2 derived CM1 composites was also investigated (Fig. 5d–f). The activity of the ISC-24 derived CM1 decreased by 52.9% for the second round of photocatalysis, and further decreased by 25.4% to 8.71 μmol g−1 h−1 in the third round of testing (Fig. 5d). This can be attributed to the poor stability of the ISC-24 derived sample. For the CM1 composites prepared with ISC-25 and ISC-25-4′-NH2 as precursors, the photocatalytic activity of the second and third rounds decreased by 83.2%, 75.4% and 96.3%, 91.3%, respectively, compared to the first round. The results show that the composites containing P1-CNPs with the amino-modified clusters have good stability.
1H-NMR test for P1-CNPs and CMn
Considering that the CM1 derived from ISC-25-4′-NH2 has good PHE performance and high stability, we assume that the amino groups in the modified P1 cluster play an important role. Therefore, the 1H-NMR test was conducted to verify the above conjecture (Fig. 6). The formation of hydrogen bonds between the amino groups and the oxygen terminus of Ti3C2Tx nanosheets caused the chemical shift values of hydrogen atoms to be shifted towards the lower field due to this effect. Similar situations have been reported in the case of an S⋯H–N hydrogen bond.44 In addition, protonated 3,5-dimethylpiperidine, which acts as counterion during cluster dispersion, also interacts electrostatically with the oxygen terminus on the Ti3C2Tx nanosheets to form hydrogen bonds, as evidenced by the result of the Hd signal moving from 7.1 ppm to 8.0 ppm in Fig. 6. This interaction also provides a theoretical basis for the enhanced photocatalytic performance of ISC-24 and ISC-25 based CM1 (Fig. 5c).
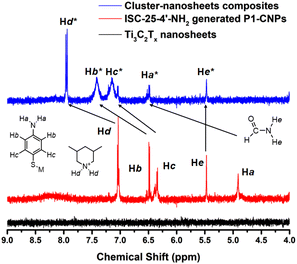 |
| Fig. 6
1H-NMR spectra of Ti3C2Tx nanosheets, P1-CNPs formed by ISC-25-4′-NH2, and their composite materials with a chemical shift value of 4.0–9.0 ppm (see Fig. S5–S7† for original data). | |
Optical and electrochemical properties of CMn
To further understand the enhanced photocatalytic performance of CMn composites compared to P1-CNPs, a series of optical and electrochemical properties were characterized. Firstly, UV–vis absorption spectra were obtained to characterize the light absorption capability of the samples. As shown in Fig. 7a, the light absorption of CM1 was significantly increased compared to CM0 in the whole range of 260–460 nm due to the dispersed Ti3C2Tx nanosheets, and similar phenomena were observed for CM2.5, CM5 and CM10. Subsequently, steady-state photoluminescence (PL) spectra were recorded for the ISC-25-4′-NH2 derived CM0 and CM1, as shown in Fig. 7b. The emission intensity of CM1 was significantly weakened compared to that of CM0, suggesting that the electron–hole recombination in CM1 composites is well-suppressed because of the effective charge transfer between P1-CNPs and Ti3C2Tx.
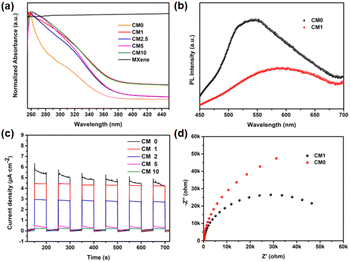 |
| Fig. 7 (a) UV–vis absorption spectra of MXene nanosheets and CMn; (b) PL spectra of ISC-25-4′-NH2 derived P1-CNPs and CM1 (Ex = 380 nm); (c and d) photoelectric response curves (c) and electrochemical impedance spectra (d) of ISC-25-4′-NH2 derived CMn. | |
In addition, the photoelectric response and electrochemical impedance spectra (EIS) were recorded to detect the separation of photogenerated electron–hole pairs and the interfacial charge transfer. As shown in Fig. 7c, CM0 showed the highest transient photocurrent densities, but were less stable compared to the prepared CMn decorated electrodes. According to this situation, CM0 should have a higher PHE efficiency. However, considering the feature of sharp reduction of the photocurrent (Fig. 7c), we also compared the EIS curves of CM0 and CM1, as shown in Fig. 7d. The fitted EIS spectra of CM1 showed a smaller semicircle and a lower interfacial resistance, revealing the more efficient electron–hole pair separation and transfer efficiency of the CM1 heterostructure. We therefore attribute the low photocatalytic efficiency of CM0 to poor stability and weak conductivity. CM1 exhibited the highest photocurrent density in the CMn series, corresponding to its highest PHE performance and stability, while CM2.5, CM5, and CM10 exhibited lower photocurrent densities due to the formation of a sandwich-like structure, as shown in Fig. S4,† where the multilayered Ti3C2Tx nanosheets may cover the active sites of P1-CNPs.
X-ray photoelectron spectroscopy (XPS) tests were also carried out on ISC-25-4′-NH2 derived P1-CNPs, Ti3C2Tx nanosheets, and CM1 composites to further verify the formation of composites between the two substances, and the results are shown in Fig. S8–S10.† The Zn, Sn and S signals of ISC-25-4′-NH2 are consistent with those of P1-CNPs, and the high-resolution XPS spectra of Ti 2p showed four deconvoluted peaks (Fig. S10†) with binding energies of 464.8 eV and 459.0 eV corresponding to Ti–O 2p1/2 and Ti–O 2p3/2 and 461.2 eV and 455.3 eV corresponding to Ti–C 2p1/2 and Ti–C 2p3/2, respectively. This result is in agreement with the HRTEM ones described above.
Photocatalytic mechanism and the band structure
To further understand the enhanced photocatalytic performance of the CMn series composites compared to CM0, we conducted a series of characterization studies. The Mott–Schottky analysis was first performed to evaluate the flat-band potential and Fermi levels of CM0 and CM1 prepared from ISC-25-4′-NH2.45–47 As shown in Fig. 8a, the flat-band potentials of CM0 and CM1 were calculated to be −0.87 V and −0.26 V (versus NHE, pH = 7), which were directly treated as Fermi levels since the surface Fermi levels are very close to the flat-band potential.48,49 To further determine the valence band (VB) and Eg
50 of CM0 and CM1, XPS valence band spectroscopy (VB-XPS) and solid-state UV–vis diffuse reflectance spectroscopy were also carried out. From the VB-XPS results (Fig. 8c and d), the VB positions of CM0 and CM1 were determined to be 1.44 eV and 0.96 eV relative to the Fermi level,51 which indicates that the VB positions of CM0 and CM1 are 0.57 V and 0.70 V (versus NHE, pH = 7), respectively. The band gaps of CM0 and CM1 were found to be 3.27 eV and 3.26 eV, respectively, according to Fig. 8b. The corresponding conduction bands (CB) edges of CM0 and CM1 were calculated to be −2.70 V and −2.56 V (versus NHE, pH = 7), respectively, by combining the CB = Eg − VB equation.52
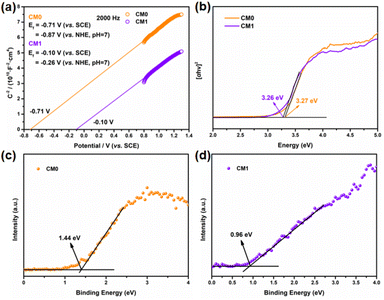 |
| Fig. 8 (a) Mott–Schottky plots of ISC-25-4′-NH2 derived P1-CNPs (i.e.CM0) and CM1 composites; (b) solid-state UV–vis diffuse reflectance spectra of CM0 and CM1; (c and d) valence band XPS spectra of CM0 (c) and CM1 (d). | |
Based on the above analysis, we proposed a photocatalytic mechanism for illustrating the higher PHE performance of the CMn series composites, as shown in Fig. 9. After P1-CNPs combined with the Ti3C2Tx nanosheets to form a binary complex, the Fermi level of CM1 shifted upwards relative to CM0. The Mott–Schottky curve in Fig. 8a shows that P1-CNPs are n-type semiconductors and their flat-band potential is much more negative than that of the Ti3C2Tx nanosheets, so the close contact between the two components in CM1 results in the transfer of electrons from the P1-CNPs to the Ti3C2Tx nanosheets. This is evidenced by the flat-band potential correction (−0.10 V vs. NHE) in CM1 compared to CM0, suggesting that the Fermi level of P1-CNPs in CM1 decreases upon their binding to Ti3C2Tx. A similar situation was reported in the studies of Jakob53 and Ran.46 Furthermore, the immobilized positive charges in the P1-CNPs are near the P1-CNPs/Ti3C2Tx interface, where a space charge layer (or internal electric field) is formed and the CB and VB in the P1-CNPs are bent “upwards”, thus forming a Schottky junction between them. Under full-spectrum solar irradiation, electrons are excited from the VB of the P1-CNPs to the CB, and due to the reduced thickness of the space charge layer in the P1-CNP particles, photogenerated electrons in the CB can still migrate through the “upward” bent CB to the Fermi level of Ti3C2Tx, leaving photogenerated holes in the VB of P1-CNPs. The previously mentioned formation of Schottky junctions can act as electron traps for trapping photogenerated electrons without blocking the transfer of electrons from P1-CNPs to Ti3C2Tx.54–56 After the transfer of electrons to the Ti3C2Tx nanosheets through the above pathway, the excellent electrical conductivity of Ti3C2Tx allows electrons to quickly shuttle to the surface. As a result, based on the excellent HER capabilities of Ti3C2Tx, the CMn series composites achieved more efficient PHE performance compared to pure P1-CNPs.
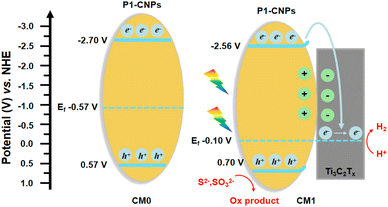 |
| Fig. 9 Proposed schematic diagram of the photocatalytic mechanism for CM0 and CM1 under light irradiation. | |
Conclusions
In summary, we proposed a strategy for disulfide bond cleavage to prepare discrete MCSCs with multiple active/inactive modified functional groups. Furthermore, three models with different structures were tested for their dispersibility, binding strength with MXene nanosheets, and photocatalytic hydrogen generation performance. The results showed that the amino-modified clusters had better performance than ligand-free type ones. This work provides insights for the subsequent design and synthesis of other types of discrete MCSCs with active functional groups, and is expected to have long-term implications for the functional expansion of metal chalcogenide cluster-based nanomaterials.
Author contributions
Jin Wu: Investigation, writing – original draft, and writing – review & editing. Qiang Fu and Zixin Wu: TEM image testing. Peipei Sun: data curation. Xing Zhu and Ying Wang: HRTEM and SAED testing. Ning Chen: funding acquisition. Tao Wu: Writing – review & editing, supervision, project administration, and funding acquisition.
Conflicts of interest
The authors declare no competing financial interests.
Acknowledgements
The authors acknowledge financial support from the National Natural Science Foundation of China (No. 22071165 and 21875150), and the 111 Project (D20015).
References
- J. Zhang, X. Bu, P. Feng and T. Wu, Metal chalcogenide supertetrahedral clusters: Synthetic control over assembly, dispersibility, and their functional applications, Acc. Chem. Res., 2020, 53, 2261–2272 CrossRef CAS PubMed.
- J. Zhang, P. Feng, X. Bu and T. Wu, Atomically precise metal chalcogenide supertetrahedral clusters: Frameworks to molecules, and structure to function, Natl. Sci. Rev., 2022, 9, nwab076 CrossRef CAS PubMed.
- S. Santner, J. Heine and S. Dehnen, Synthesis of crystalline chalcogenides in ionic liquids, Angew. Chem., Int. Ed., 2016, 55, 876–893 CrossRef CAS PubMed.
- B. Peters, N. Lichtenberger, E. Dornsiepen and S. Dehnen, Current advances in tin cluster chemistry, Chem. Sci., 2020, 11, 16–26 RSC.
- P. Feng, X. Bu and N. Zheng, The interface chemistry between chalcogenide clusters and open framework chalcogenides, Acc. Chem. Res., 2005, 38, 293–303 CrossRef CAS PubMed.
- Y. Peng, Q. Hu, Y. Liu, J. Li and X. Huang, Discrete supertetrahedral Tn chalcogenido clusters synthesized in ionic liquids: Crystal structures and photocatalytic activity, ChemPlusChem, 2020, 85, 2487–2498 CrossRef CAS PubMed.
- Z. Wang, Y. Liu, J. Zhang, X. Wang, Z. Wu, J. Wu, N. Chen, D.-S. Li and T. Wu, Unveiling the impurity-modulated photoluminescence from Mn2+-containing metal chalcogenide semiconductors via Fe2+ doping, J. Mater. Chem. C, 2021, 9, 13680–13686 RSC.
- Y. Liu, J. Zhang, B. Han, X. Wang, Z. Wang, C. Xue, G. Bian, D. Hu, R. Zhou, D. S. Li, Z. Wang, Z. Ouyang, M. Li and T. Wu, New insights into Mn–Mn coupling interaction-directed photoluminescence quenching mechanism in Mn2+-doped semiconductors, J. Am. Chem. Soc., 2020, 142, 6649–6660 CrossRef CAS PubMed.
- T. Wu, Q. Zhang, Y. Hou, L. Wang, C. Mao, S. T. Zheng, X. Bu and P. Feng, Monocopper doping in Cd–In–S supertetrahedral nanocluster via two-step strategy and enhanced photoelectric response, J. Am. Chem. Soc., 2013, 135, 10250–10253 CrossRef CAS PubMed.
- J. Lin, Q. Zhang, L. Wang, X. Liu, W. Yan, T. Wu, X. Bu and P. Feng, Atomically precise doping of monomanganese ion into coreless supertetrahedral chalcogenide nanocluster inducing unusual red shift in Mn2+ emission, J. Am. Chem. Soc., 2014, 136, 4769–4779 CrossRef CAS PubMed.
- J. Lin, D.-D. Hu, Q. Zhang, D.-S. Li, T. Wu, X. Bu and P. Feng, Improving photoluminescence emission efficiency of nanocluster based materials by in-situ doping synthetic strategy, J. Phys. Chem. C, 2016, 120, 29390–29396 CrossRef CAS.
- F. Wang, J. Lin, T. Zhao, D. Hu, T. Wu and Y. Liu, Intrinsic “vacancy point defect” induced electrochemiluminescence from coreless supertetrahedral chalcogenide nanocluster, J. Am. Chem. Soc., 2016, 138, 7718–7724 CrossRef CAS PubMed.
- Q. Zhang, J. Lin, Y.-T. Yang, Z.-Z. Qin, D. Li, S. Wang, Y. Liu, X. Zou, Y.-B. Wu and T. Wu, Exploring Mn2+-location-dependent red emission from (Mn/Zn)–Ga–Sn–S supertetrahedral nanoclusters with relatively precise dopant positions, J. Mater. Chem. C, 2016, 4, 10435–10444 RSC.
- N. Zheng, X. Bu, H. Vu and P. Feng, Open-framework chalcogenides as visible-light photocatalysts for hydrogen generation from water, Angew. Chem., Int. Ed., 2005, 44, 5299–5303 CrossRef CAS PubMed.
- D. Liu, Y. Liu, P. Huang, C. Zhu, Z. Kang, J. Shu, M. Chen, X. Zhu, J. Guo, L. Zhuge, X. Bu, P. Feng and T. Wu, Highly tunable heterojunctions from multimetallic sulfide nanoparticles and silver nanowires, Angew. Chem., Int. Ed., 2018, 57, 5374–5378 CrossRef CAS PubMed.
- R. Hu, X. L. Wang, J. Zhang, D. Hu, J. Wu, R. Zhou, L. Li, M. Li, D. S. Li and T. Wu, Multi-metal nanocluster assisted Cu–Ga–Sn tri-doping for enhanced photoelectrochemical water splitting of BiVO4 film, Adv. Mater. Interfaces, 2020, 7, 2000016 CrossRef CAS.
- Z. Wu, X.-L. Wang, X. Wang, X. Xu, D.-S. Li and T. Wu, 0D/2D heterostructure constructed by ultra-small chalcogenide-cluster aggregated quaternary sulfides and g-C3N4 for enhanced photocatalytic H2 evolution, Chem. Eng. J., 2021, 426, 131216 CrossRef CAS.
- W. Yang, X.-L. Wang, N. Kong, C. Liu, P. Sun, Z. Wang, Y. Ding, H. Lin, D. Li and T. Wu, Minimized external electric field on asymmetric monolayer maximizes charge separation for photocatalysis, Appl. Catal., B, 2021, 295, 120266 CrossRef CAS.
- W. Yang, X. Wang, Z. Wang, P. Sun, J. Tang, J. Li, D.-S. Li and T. Wu, Interstitially O-doped CdxZn1−xS solid solution derived from chalcogenide molecular clusters for photocatalytic hydrogen evolution, Inorg. Chem. Front., 2022, 9, 3771–3778 RSC.
- C. Xue, J. Zhang, X. Wang, M. Gu, Y. Zhu, D. S. Li, J. Guo, Y. Liu and T. Wu, Light-triggered evolution of molecular clusters toward sub-nanoscale heterojunctions with high interface density, Chem. Commun., 2019, 55, 8146–8149 RSC.
- Y. Zhang, X. Wang, D. Hu, C. Xue, W. Wang, H. Yang, D. Li and T. Wu, Monodisperse ultrasmall manganese-doped multimetallic oxysulfide nanoparticles as highly efficient oxygen reduction electrocatalyst, ACS Appl. Mater. Interfaces, 2018, 10, 13413–13424 CrossRef CAS PubMed.
- D. L. Liu, X. Fan, X. Wang, D. D. Hu, C. Z. Xue, Y. Liu, Y. Wang, X. Zhu, J. Guo, H. P. Lin, Y. Y. Li, J. Zhong, D. S. Li, X. H. Bu, P. Y. Feng and T. Wu, Cooperativity by multi-metals confined in supertetrahedral sulfide nanoclusters to enhance electrocatalytic hydrogen evolution, Chem. Mater., 2019, 31, 553–559 CrossRef CAS.
- X. Wang, X.-L. Wang, J. Lv, Z. Wu, J. Zhang, D. Hu, C. Xue, D. Li, X. Zhu and T. Wu, 0D/1D heterostructure for efficient electrocatalytic CO2-to-C1 conversion by ultra-small cluster-based multi-metallic sulfide nanoparticles and MWCNTs, Chem. Eng. J., 2021, 422, 130045 CrossRef CAS.
- X.-L. Wang, Z. Wu, X. Wang, C. Xue, C. Liu, J. Zhang, R. Zhou, D.-S. Li and T. Wu, Bifunctional electrocatalysts derived from cluster-based ternary sulfides for oxygen electrode reactions, Electrochim. Acta, 2021, 376, 138048 CrossRef CAS.
- X. L. Wang, C. Xue, N. Kong, Z. Wu, J. Zhang, X. Wang, R. Zhou, H. Lin, Y. Li, D. S. Li and T. Wu, Molecular modulation of a molybdenum-selenium cluster by sulfur substitution to enhance the hydrogen evolution reaction, Inorg. Chem., 2019, 58, 12415–12421 CrossRef CAS PubMed.
- J. Zhang, C. Qin, Y. Zhong, X. Wang, W. Wang, D. Hu, X. Liu, C. Xue, R. Zhou, L. Shen, Y. Song, D. Xu, Z. Lin, J. Guo, H. Su, D.-S. Li and T. Wu, Atomically precise metal-chalcogenide semiconductor molecular nanoclusters with high dispersibility: Designed synthesis and intracluster photocarrier dynamics, Nano Res., 2020, 13, 2828–2836 CrossRef CAS.
- B. Peters, S. Santner, C. Donsbach, P. Vopel, B. Smarsly and S. Dehnen, Ionic liquid cations as methylation agent for extremely weak chalcogenido metalate nucleophiles, Chem. Sci., 2019, 10, 5211–5217 RSC.
- B. Peters, G. Stuhrmann, F. Mack, F. Weigend and S. Dehnen, Highly soluble supertetrahedra upon selective partial butylation of chalcogenido metalate clusters in ionic liquids, Angew. Chem., Int. Ed., 2021, 60, 17622–17628 CrossRef CAS PubMed.
- T. Wu, X. Bu, P. Liao, L. Wang, S. T. Zheng, R. Ma and P. Feng, Superbase route to supertetrahedral chalcogenide clusters, J. Am. Chem. Soc., 2012, 134, 3619–3622 CrossRef CAS PubMed.
- Y. Wang, Z. Zhu, Z. Sun, Q. Hu, J. Li, J. Jiang and X. Huang, Discrete supertetrahedral T5 selenide clusters and their Se/S solid solutions: Ionic-liquid-assisted precursor route syntheses and photocatalytic properties, Chem. – Eur. J., 2020, 26, 1624–1632 CrossRef CAS PubMed.
- N.-N. Shen, B. Hu, C.-C. Cheng, G.-D. Zou, Q.-Q. Hu, C.-F. Du, J.-R. Li and X.-Y. Huang, Discrete supertetrahedral T3 InQ clusters (Q = S, S/Se, Se, Se/Te): Ionothermal syntheses and tunable optical and photodegradation properties, Cryst. Growth Des., 2018, 18, 962–968 CrossRef CAS.
- J. Wu, P. Sun, X. Wang, N. Chen and T. Wu, Ligand-partially-protected metal-chalcogenide supertetrahedral clusters, Dalton Trans., 2022, 51, 18257–18263 RSC.
- F. Shahzad, M. Alhabeb, C. Hatter, B. Anasori, S. M. Hong, C. M. Koo and Y. Gogotsi, Electromagnetic interference shielding with 2D transition metal carbides (MXenes), Science, 2016, 353, 1137–1140 CrossRef CAS PubMed.
- Y. Gao, C. Yan, H. Huang, T. Yang, G. Tian, D. Xiong, N. Chen, X. Chu, S. Zhong, W. Deng, Y. Fang and W. Yang, Microchannel-confined MXene based flexible piezoresistive multifunctional micro-force sensor, Adv. Funct. Mater., 2020, 30, 1909603 CrossRef CAS.
- C. Peng, P. Wei, X. Li, Y. Liu, Y. Cao, H. Wang, H. Yu, F. Peng, L. Zhang, B. Zhang and K. Lv, High efficiency photocatalytic hydrogen production over ternary Cu/TiO2@Ti3C2Tx enabled by low-work-function 2D titanium carbide, Nano Energy, 2018, 53, 97–107 CrossRef CAS.
- G. Zuo, Y. Wang, W. L. Teo, A. Xie, Y. Guo, Y. Dai, W. Zhou, D. Jana, Q. Xian, W. Dong and Y. Zhao, Ultrathin ZnIn2S4 nanosheets anchored on Ti3C2Tx MXene for photocatalytic H2 evolution, Angew. Chem., Int. Ed., 2020, 59, 11287–11292 CrossRef CAS PubMed.
- X. Xie, N. Zhang, Z.-R. Tang, M. Anpo and Y.-J. Xu, Ti3C2Tx MXene as a Janus cocatalyst for concurrent promoted photoactivity and inhibited photocorrosion, Appl. Catal., B, 2018, 237, 43–49 CrossRef CAS.
- Z. Wu, C. Li, Z. Li, K. Feng, M. Cai, D. Zhang, S. Wang, M. Chu, C. Zhang, J. Shen, Z. Huang, Y. Xiao, G. A. Ozin, X. Zhang and L. He, Niobium and titanium carbides (MXenes) as superior photothermal supports for CO2 Photocatalysis, ACS Nano, 2021, 15, 5696–5705 CrossRef CAS PubMed.
- M. Naguib, O. Mashtalir, J. Carle, V. Presser, J. Lu, L. Hultman, Y. Gogotsi and M. W. Barsoum, Two-dimensional transition metal carbides, ACS Nano, 2012, 6, 1322–1331 CrossRef CAS PubMed.
- H. Wang, Y. Wu, X. Yuan, G. Zeng, J. Zhou, X. Wang and J. W. Chew, Clay-inspired MXene-based electrochemical devices and photo-electrocatalyst: State-of-the-art progresses and challenges, Adv. Mater., 2018, 30, e1704561 CrossRef PubMed.
- Y. Ying, Y. Liu, X. Wang, Y. Mao, W. Cao, P. Hu and X. Peng, Two-dimensional titanium carbide for efficiently reductive removal of highly toxic chromium(VI) from water, ACS Appl. Mater. Interfaces, 2015, 7, 1795–1803 CrossRef CAS PubMed.
- X. Sheng, Y. Zhao, L. Zhang and X. Lu, Properties of two-dimensional Ti3C2 MXene/thermoplastic polyurethane nanocomposites with effective reinforcement via melt blending, Compos. Sci. Technol., 2019, 181, 107710 CrossRef CAS.
- P. Lin, J. Xie, Y. He, X. Lu, W. Li, J. Fang, S. Yan, L. Zhang, X. Sheng and Y. Chen, MXene aerogel-based phase change materials toward solar energy conversion, Sol. Energy Mater. Sol. Cells, 2020, 206, 110229 CrossRef CAS.
- J. Wu, B. Jin, X. Wang, Y. Ding, X.-L. Wang, D. Tang, X. Li, J. Shu, D.-S. Li, Q. Lin, Y.-B. Wu and T. Wu, Breakdown of valence shell electron pair repulsion theory in an H-bond-stabilized linear sp-hybridized sulfur, CCS Chem., 2021, 3, 2584–2590 CrossRef CAS.
- J. Ran, W. Guo, H. Wang, B. Zhu, J. Yu and S. Z. Qiao, Metal-free 2D/2D phosphorene/g-C3N4 van der Waals heterojunction for highly enhanced visible-light photocatalytic H2 production, Adv. Mater., 2018, 30, e1800128 CrossRef PubMed.
- J. Ran, G. Gao, F. T. Li, T. Y. Ma, A. Du and S. Z. Qiao, Ti3C2 MXene co-catalyst on metal sulfide photo-absorbers for enhanced visible-light photocatalytic hydrogen production, Nat. Commun., 2017, 8, 13907 CrossRef CAS PubMed.
- Z. Li, W. Huang, J. Liu, K. Lv and Q. Li, Embedding CdS@Au into Ultrathin Ti3−xC2Ty to build dual Schottky barriers for photocatalytic H2 production, ACS Catal., 2021, 11, 8510–8520 CrossRef CAS.
- L. Kavan, M. Gratzel, S. E. Gilbert, C. Klemenz and H. J. Scheel, Electrochemical and photoelectrochemical investigation of single-crystal anatase, J. Am. Chem. Soc., 1996, 118, 6716–6723 CrossRef CAS.
- P. Xia, S. Cao, B. Zhu, M. Liu, M. Shi, J. Yu and Y. Zhang, Designing a 0D/2D S-scheme heterojunction over polymeric carbon nitride for visible-light photocatalytic inactivation of bacteria, Angew. Chem., Int. Ed., 2020, 59, 5218–5225 CrossRef CAS PubMed.
- Z. Lin, J. Xiao, L. Li, P. Liu, C. Wang and G. Yang, Nanodiamond-embedded p-type copper(I) oxide nanocrystals for broad-spectrum photocatalytic hydrogen evolution, Adv. Energy Mater., 2016, 6, 1501865 CrossRef.
- Z. Hu, G. Liu, X. Chen, Z. Shen and J. C. Yu, Enhancing charge separation in metallic photocatalysts: A case study of the conducting molybdenum dioxide, Adv. Funct. Mater., 2016, 26, 4445–4455 CrossRef CAS.
- F.-Y. Tian, D. Hou, F. Tang, M. Deng, X.-q. Qiao, Q. Zhang, T. Wu and D.-S. Li, Novel Zn0.8Cd0.2S@g-C3N4 core–shell heterojunctions with a twin structure for enhanced visible-light-driven photocatalytic hydrogen generation, J. Mater. Chem. A, 2018, 6, 17086–17094 RSC.
- M. Jakob, H. Levanon and P. V. Kamat, Charge distribution between UV-irradiated TiO2 and gold nanoparticles: Determination of shift in the Fermi level, Nano Lett., 2003, 3, 353–358 CrossRef CAS.
- H. Yan, J. Yang, G. Ma, G. Wu, X. Zong, Z. Lei, J. Shi and C. Li, Visible-light-driven hydrogen production with extremely high quantum efficiency on Pt-PdS/CdS photocatalyst, J. Catal., 2009, 266, 165–168 CrossRef CAS.
- D. Y. Leung, X. Fu, C. Wang, M. Ni, M. K. Leung, X. Wang and X. Fu, Hydrogen production over titania-based photocatalysts, ChemSusChem, 2010, 3, 681–694 CrossRef CAS PubMed.
- J. Yang, D. Wang, H. Han and C. Li, Roles of cocatalysts in photocatalysis and photoelectrocatalysis, Acc. Chem. Res., 2013, 46, 1900–1909 CrossRef CAS PubMed.
Footnote |
† Electronic supplementary information (ESI) available: Supplementary tables and figures, PXRD patterns, EDS and 1H-NMR analysis. CCDC 2254140–2254148 for ISC-24, ISC-25, ISC-25-4′-NH2, ISC-25-4′-CH3, ISC-25-4′-OH, ISC-25-4′-F, ISC-25-4′-OCH3, ISC-26-2′-NH2 and ISC-26-4′-Cl. For ESI and crystallographic data in CIF or other electronic format see DOI: https://doi.org/10.1039/d3qi00983a |
|
This journal is © the Partner Organisations 2023 |