DOI:
10.1039/D3QI00432E
(Research Article)
Inorg. Chem. Front., 2023,
10, 4147-4156
A wheel-shaped gallium-sulfide molecular ring with enhanced photocatalytic activity via indium alloying†
Received
8th March 2023
, Accepted 27th May 2023
First published on 28th May 2023
Abstract
Wheel-shaped molecular rings are one of the most striking types of molecular aggregates due to their naturally aesthetically appealing architecture, giant size and interesting physical properties and potential applications. Herein, unlike common supertetrahedral cluster-based main-group metal chalcogenides, we report an unprecedented wheel-shaped double-decker {Ga24S40} molecular ring (WSC-1) with an ∼1.6 nm external diameter, which is the first main-group metal sulfide nanoring and the largest ring in the family of inorganic metal sulfide molecular rings. Furthermore, indium was successfully introduced to obtain the first bimetallic main-group metal chalcogenide molecular ring (WSC-1-In), exhibiting enhanced photocatalytic dye degradation. This work is of great significance to expand the family of metal chalcogenide molecular rings and demonstrated the great potential of metal chalcogenide molecular rings in the application of photocatalysis.
Introduction
Over the last thirty years, cluster-based crystalline metal chalcogenides (CCMCs),1,2 combining the porosity and semiconducting features with diverse structures and precisely adjustable compositions, have attracted considerable attention in photo/electro-catalysis,3–8 ion exchange,9–11 nonlinear optics12,13etc. Structurally, as the most important basic building unit in CCMCs, metal chalcogenide clusters with different sizes, shapes, compositions and diversiform assembly modes played a vital role in determining the structure and function of CCMCs.1,2 Hitherto, the dominant metal chalcogenide clusters assembled in the superlattices of CCMCs are tetrahedrally shaped Tn (n indicates the number of metal layers),14–16 penta-(Pn),17–19 capped-(Cn),20–22 super-(Tp,q)23–27 and TOn28,29 supertetrahedral clusters due to the inherent tetrahedral coordination mode of metal ions (M) in CCMCs and an inflexible Q–M–Q angle in a tetrahedral MQ4 subunit (Q = S/Se/Te).1,2 Since metal chalcogenide clusters fundamentally promote the development of CCMCs, it is desirable to synthesize novel non-supertetrahedral clusters with diverse structures and versatile functions to enrich CCMCs with more applications in different fields.
So far, great unremitting efforts have been devoted to the development of non-supertetrahedral CCMCs, including copper-rich open-framework chalcogenides (COCs),30–34 sphere shaped nano-balls,35 molecular rings36–39 and defective atypical supertetrahedral cluster-based chalcogenide frameworks.40–43 Among these non-supertetrahedral CCMCs, molecular rings deserve attention and research, but limited reports can be found.36–39 Molecular rings constructed by metal atoms and bridged by pure inorganic O and S/Se anions or O/S/Se atoms of the organic ligands,44–53 such as cyclic high-nuclear transition-metal compounds,54,55 have been reported much and have aroused great attention due to their naturally aesthetically appealing architecture and interesting physical properties and potential applications.52,54,55 So far, only Dai et al. reported two kinds of molecular rings based on In–Te/Se clusters which are wheel-shaped In18Te30/Se30 and triangular In33Se60 ring clusters decorated by organic amines37–39 and Dehnen et al. recently reported a square shaped molecular ring constructed by 4 T2 Ge–Se clusters.56 The slow development of the molecular ring in CCMCs is probably due to the intrinsic tetrahedral coordination mode and inflexible Q–M–Q angle of the MQ4 subunit (M = In/Ga/Sn/Ge, Q = S/Se/Te),57,58 and compared with In–Te/Se and Ge–Se molecular rings, creating sulfide based nanorings is much more challenging due to the lower structural flexibility reflected by the relatively rigid S–M–S angle.37,39
Herein, we report an unprecedented wheel-shaped Ga–S molecular ring—[Ga24S40(CPA)8][(H+-CPA)8(CPA)8] (denoted as WSC-1, CPA = cyclopentylamine). Decorated by 8 CPA molecules, WSC-1 consists of 24 Ga and 40 S atoms, forming a regular double-decker ring structure. Noteworthily, although gallium based molecular wheels built by Ga atoms and bridged organic OMe and OAc ligands have been reported,47–49 a gallium-sulfide molecular ring has been obtained for the first time and it represents the first example of main-group metal sulfide based nanorings. Besides, WSC-1 is the largest circular ring cluster in pure metal chalcogenides to date. To adjust the band structure of WSC-1 for better photocatalytic activity, indium was successfully introduced in a sulfide-based molecular ring for the first time (denoted as WSC-1-In) and this first heteronuclear main-group metal chalcogenide molecular ring exhibited enhanced photodegradation of methylene blue (MB) and comparable photocatalytic hydrogen evolution activity.
Experimental
Chemicals
Gallium oxide powder (Ga2O3, 99.8%), indium powder (In, 99.99%), sulfur powder (S, 99.9%), cyclopentylamine (CPA, AR, liquid), formamide (AR, liquid), ethanol (AR, liquid), tetraammine platinum(II) nitrate (Pt(NH3)4(NO3)2), and deionized water were all used as supplied without further purification.
Synthesis of WSC-1
Gallium oxide powder (187 mg, 1.00 mmol) and sulfur powder (128 mg, 4.00 mmol) were mixed with cyclopentylamine (4.00 mL) in a 23 mL Teflon-lined stainless steel autoclave and stirred for half an hour. The vessel was then sealed and heated at 190 °C for 7 days and then taken out of the oven. The autoclave was then cooled to room temperature and yellow prism-like crystals (yield: 99.922 mg, 24% based on Ga) were obtained. The raw products were washed three times with ethanol and then dried in air, and the compounds were stable under ambient conditions. Elemental analysis of C, N, and H, found (wt%): C, 28.79; N, 6.80; H, 5.25; calc. (wt%): C, 28.78; N, 6.71; H, 5.47.
Synthesis of In-alloyed WSC-1 (WSC-1-In)
Gallium oxide powder (Ga2O3, 112 mg, 0.60 mmol), indium oxide (In2O3, 128 mg, 0.46 mmol) and sulfur powder (128 mg, 4.0 mmol) were all mixed with cyclopentylamine (4 mL) in a 23 mL Teflon-lined stainless steel autoclave and stirred for half an hour. The vessel was then sealed and heated at 190 °C for 7 days and then taken out of the oven. Then the autoclave was cooled to room temperature and colorless transparent prism-like crystals (yield: 50.446 mg, 13.3% based on Ga) were obtained. The raw products were washed with ethanol three times and then dried in air, and the compounds were stable under ambient conditions. Elemental analysis of C, N, and H, found (wt%): C, 26.87; N, 6.34; H, 5.37; calc. (wt%): C, 26.85; N, 6.26; H, 5.11.
Methylene blue photodegradation experiments
The photocatalytic activities of the as-synthesized samples and commercial TiO2 were evaluated from the degradation reaction of Methylene Blue (MB) dye in a glass bottle with a water-cooling system with a 350 W xenon arc lamp as the radiation source. Firstly, 5 mg of each sample was immersed in 20 mL of MB aqueous solution (3.5 × 10−5 M) and stirred for 30 min and then kept still for 10 h in the dark to achieve the adsorption–desorption equilibrium before irradiation. Then, the dispersion was illuminated and stirred under a 350 W xenon arc lamp at ambient temperature. During irradiation, 2 mL solution was sucked up from the reaction reactor at every specific interval and centrifuged at 8000 rpm for 3 minutes. The resulting supernatant was analyzed on a UV-Vis spectrophotometer (UV2450, Shimadzu). The degradation efficiency was reported as Ct/C0, where Ct is each irradiated time interval and C0 is the initial equilibrium concentration of MB according to the main peak of absorption. After the reaction, the mixture was filtered and the powder was collected for the powder X-ray diffraction (PXRD) test.
Results and discussion
Crystal structure
Pale-yellow prismatic crystals of WSC-1 were synthesized via the solvothermal reaction of Ga2O3 and S powder in the mixed solvents of CPA and deionized water at 190 °C for 7 days (Fig. S1a†). Single-crystal X-ray diffraction (SCXRD) analysis revealed that WSC-1 crystallizes in a tetragonal system with the space group of I4 (Table S1†) and represents a unique wheel-shaped molecular ring (Fig. 1), while the charge balancing counter ions and solvent molecules could not be completely located due to significant disorder. Notably, CPA is essential for the crystallization of WSC-1 since no crystals were obtained when replaced by other cycloamines such as DACH (1,2-diaminocyclohexane), the one used in In–Te/Se molecular rings.37,39 According to the charge balance rule, C/H/N elemental analysis (EA) and energy-dispersive spectroscopy (EDS) measurements (Fig. S2†), the empirical formula of WSC-1 was determined to be [Ga24S40(C5H11N)16(C5H12N)8] (cationic C5H12N stands for protonated CPA). WSC-1 was also characterized by thermogravimetric analysis (TGA) and Fourier transform infrared (FTIR) spectroscopy (Fig. S3a and S4†) and the experimental powder X-ray diffraction (PXRD) pattern of the as-synthesized WSC-1 confirmed its phase purity (Fig. S5a†).
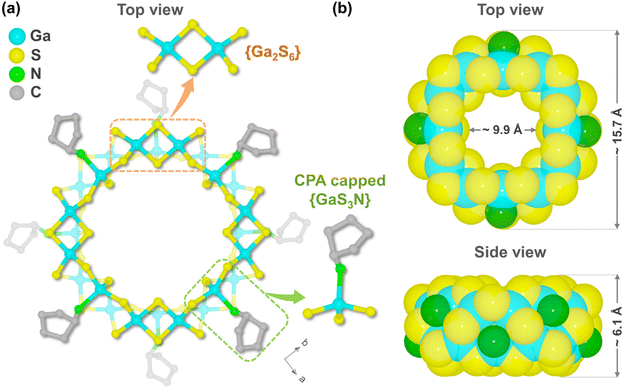 |
| Fig. 1 View of the structure of the WSC-1 molecular ring in (a) ball-stick and (b) space-filling modes. All H and some C atoms are omitted for clarity. | |
As shown in Fig. 1a, WSC-1 comprises a wheel-shaped anion [Ga24S40(CPA)8]8−, where eight CPA molecules are mono-capped with eight Ga atoms and are located alternately above and below the outer side of the wheel. In this ring, all 24 Ga3+ sites are tetrahedrally coordinated while there are two types of coordination modes of S2− (μ2-S2− and μ3-S2−). Structurally, [Ga24S40(CPA)8]8− is constructed by eight {Ga2S6} units alternately interlinked with eight CPA mono-coordinated {GaS3N} tetrahedra through sharing the adjacent vertex μ2/μ3-S2− (Fig. 1a and S6†). Each {Ga2S6} building unit is 5-connected with three {GaS3N} tetrahedra and two other {Ga2S6} units while each {GaS3N} unit is capped by three {Ga2S6} units and one CPA molecule (Fig. S7†).
On the other hand, WSC-1 can also be viewed as a double-decker ring with a ‘two-stranded’ topology with respect to Ga atoms and the two [Ga12] folded quasi-ideal squares (coordinated CPA is omitted) are mirror symmetric by twisting ∼45°, linking together through 8 μ2-S2− and 8 μ3-S2− (Fig. 2a). In each [Ga12] unit, 3 Ga3+ ions and 4 adjacent bridged μ2-S2− are alternately arranged approximately in a 1D polymeric chain of ca. 10.98 Å and such 4 chains connected end-to-end by sharing an S2− ion to form a folded quasi-ideal square (Fig. 2b). This structure is analogous to the reported In–Te nanoring. Besides, the WSC-1 ring shows a pseudo-C4v symmetry, but it is an accurate C4 point group when considering the eight decorated CPA molecules. It is reported that the small molecules or ions located at the center of the ring can act as important templates in the formation of ring structures and we also found peaks of residual electron density at the center of the ring from the Fourier electron density map, which are believed to act as key templates to shape this wheel-like molecular ring.37,39 However, these peaks cannot be well resolved due to serious disorder, and hence removed by SQUEEZE in SCXRD data refinement.
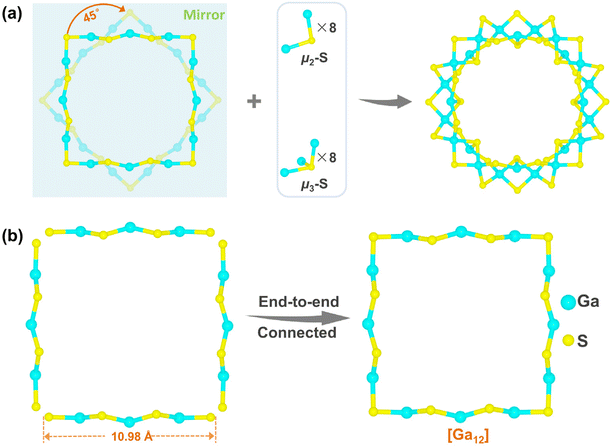 |
| Fig. 2 (a) Mirror symmetric two [Ga12] folded quasi-ideal square (coordinated CPA is omitted) linked together through 8 μ2-S2− and 8 μ3-S2− by twisting ∼45° to form the wheel-shaped molecular ring and (b) a [Ga12] unit constructed by 4 1D polymeric chains of 3 Ga3+ ions and 4 adjacent bridged μ2-S2−. | |
The bond length of Ga–S and bond angles of S–Ga–S of WSC-1 are in the range of 2.203(4)–2.378(5) Å and 94.72(15)–115.27(16)°, respectively (Tables S2 and S3†), while for Ga1 and Ga4 in the asymmetric unit (Fig. S8†), the Ga–N distances are 2.053(12) and 1.98(4) Å, and the bond angles of N–Ga–S range from 102.3(3) to 110.7(4)°. All the above values are consistent with those in literature reports.59–61 Provided by space-filling models (Fig. 1b), the average central hole and external diameter and thickness of the molecular ring are ca. 9.92 Å, 15.72 Å and 6.06 Å (estimated from the distance of two opposite S atoms without considering van der Waals radii), respectively, demonstrating that WSC-1 is the largest standard molecular ring ever found in pure metal chalcogenides (Table S4†).
As shown in Fig. 3, the anion rings of WSC-1 stacked parallel to each other and packed together to form a staggered pseudo-supramolecular nanotubular architecture along the c direction, where the distance between two adjacent rings is ∼6.6 Å in the c direction, while the adjacent nanotubes are ∼13.2 Å apart in the a or b direction and separated by ∼4.7 Å along the [110] direction. Moreover, by connecting the barycenter of the adjacent cluster, the arrangement mode of rings is a squashed BCU (body centered cubic)-type superlattice (Fig. S9†).
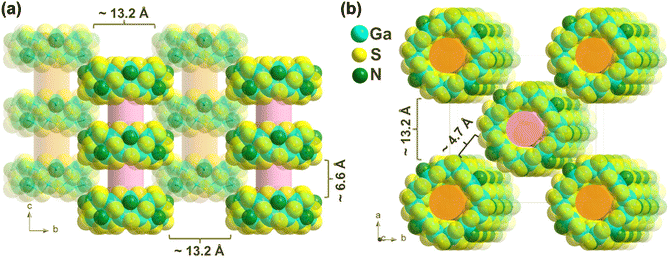 |
| Fig. 3 Packing diagrams of WSC-1 molecular rings viewed along (a) the a axis and (b) c axis showing different alternations of adjacent pseudo-supramolecular nanotubes (emphasized with disparate colors). H and C atoms are omitted for clarity. | |
The solvent-accessible void space of WSC-1 is only 56.0% as calculated using PLATON software, which is not outstanding in discrete CCMCs (Table S5†), indicating that it is insoluble in common solvents such as water due to strong cation–anion electrostatic interactions and van der Waals forces between clusters and protonated organic amines. This is like other known closely packed discrete CCMCs and different from the thermodynamic unstable CCMCs constructed by loosely packed discrete clusters.6,62–66
Indium alloying, optoelectronic properties and photocatalytic activities
As alloying is an effective strategy to modulate the bandgap sizes and optical- and electronic-related properties of CCMCs for better photocatalytic activity,19,34,65 indium alloyed WSC-1 (denoted as WSC-1-In) was successfully prepared (Fig. S1b†). As the introduced In atoms could theoretically appear at every tetrahedral metal site of the cluster since In and Ga are in the same main group with equivalent valence states and the SCXRD data match the statistical result, it is difficult to find defined Ga or In positions for every metal site in the cluster which could be occupied in a mixed way by Ga and In.19,34 Nonetheless, an In/Ga ratio of 0.491 (ca. 7.9 per 24 metal atoms in one molecular ring) could be determined for WSC-1-In crystals by inductively coupled plasma atomic emission spectrometry (ICP-AES) analysis, which is close to the energy-dispersive spectroscopy (EDS) results (Fig. S10†). The corresponding elemental mapping (Fig. S11†) pictures demonstrated that indium is evenly distributed in WSC-1 crystals. Besides, the PXRD pattern of WSC-1-In matches well with that of WSC-1 (Fig. S5b†), indicating that WSC-1-In is isostructural. In addition, both WSC-1 and WSC-1-In were stable in open air for at least 6 months (Fig. S12†).
Solid-state UV-vis diffuse reflectance (DRS) spectroscopy and Mott–Schottky (MS) measurements were firstly performed to determine the band structures of the title compounds. As depicted in Fig. 4a, from the extrapolation of linear regions of Tauc plots, the band gaps of WSC-1 and WSC-1-In are ca. 3.79 eV and 3.83 eV, respectively, consistent with their different colors (Fig. S1†). Beside the optical absorption capability, the positive slopes of MS plots in Fig. 4b and Fig. S13† indicate that both WSC-1 and WSC-1-In are n-type semiconductors.6,67,68 From the MS plots, the flat-band potential (EF) of WSC-1 and WSC-1-In is found to be −0.8 V and −1.01 (vs. NHE, pH = 7), respectively, where the conduction band (CB) potential (ECB) can be estimated to be −0.9 V and −1.11 V via the equation ECB = EF − 0.1 for an n-type semiconductor.6,8,67,68 In combination with the measured band gaps from DRS, the calculated valence band potentials of WSC-1 and WSC-1-In are 2.89 V and 2.72 V (vs. NHE, pH = 7), respectively. Meanwhile, as low recombination rates of photo-induced electron–hole pairs are conducive to photocatalysis, photoelectric response and electrochemical impedance spectroscopy (EIS) measurements were, therefore, performed to explore the effect of In-alloying on the separation efficiency of charge carries.6,8,65 As shown in Fig. 4c, both WSC-1 and WSC-1-In exhibited rapid responses upon illumination and good reproducibility during the light on–off mode, and the photocurrent density of WSC-1-In (∼0.96 μA cm−2) is ca. 2-fold larger than that of WSC-1 (∼0.47 μA cm−2). Besides, WSC-1-In displayed a smaller arc radius than WSC-1 in the Nyquist plots (Fig. 4d). The above results indicate the suppressed charge recombination and superior conductivity of WSC-1-In compared to those of WSC-1, consistent with previous research studies that In-alloying could facilitate electron transport in CCMCs.19,34
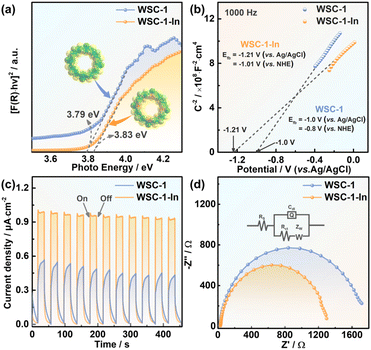 |
| Fig. 4 (a) Tauc plots of WSC-1 and WSC-1-In derived from solid-state UV-Vis diffuse reflectance spectra; (b) Mott–Schottky plots of WSC-1 and WSC-1-In measured at a frequency of 1000 Hz; (c) photocurrent response curves of WSC-1 and WSC-1-In under a 0.4 V bias potential; and (d) Nyquist plots of impedance measured from WSC-1 and WSC-1-In (inset is the fitted equivalent circuit model). | |
Given their different band structures and optoelectronic features, we further investigated their performance in dye photodegradation and photocatalytic H2 evolution. As shown in Fig. S14a,† the blank experiment shows that the characteristic absorption peak of MB remains almost constant for 13 min without any catalysts, suggesting negligible photo-induced self-decomposition. Conversely, the absorbance of MB rapidly decreases over illumination time when WSC-1, WSC-1-In and commercial TiO2 are used as catalysts (Fig. 5a, b and Fig. S14b†). As displayed in Fig. 5c, WSC-1-In exhibits the best photocatalytic performance among all catalysts as 98.1% of MB was decomposed within 5 min, whereas only 58.2% MB and 33.8% MB were degraded within the same time for WSC-1 and commercial TiO2. Assuming that the dye degradation is a pseudo-first-order reaction,10,34,69–72 the apparent rate constant (Ka) was calculated to be 0.8144 min−1 for WSC-1-In, which is ca. 4 times faster than that of WSC-1 (Ka = 0.2000 min−1) (Fig. 5d).
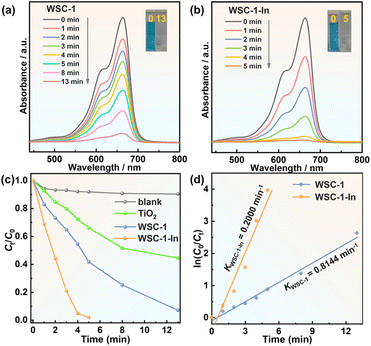 |
| Fig. 5 UV-Vis absorption spectra of MB versus time with (a) WSC-1 and (b) WSC-1-In as catalysts under full spectrum solar light irradiation; (c) photodegradation efficiency curves of different catalysts with the blank as the control; and (d) linear plots of ln(C0/Ct) over time for the photocatalytic degradation of MB. | |
As the indirect dye photosensitization degradation or self-sensitized degradation usually occurred during the degradation process,73,74 it should be excluded in this experiment. According to the literature,75 the redox potential of MB is +0.05 V (vs. NHE, pH = 7), which is much more positive than the CB potential of WSC-1-In (−1.11 eV vs. NHE, pH = 7). As a result, the photo-induced electrons originating from MB molecules cannot transfer to the CB of WSC-1-In; hence it could be inferred that there would be no dye-sensitive effect during the degradation process. Consequently, such superior photocatalytic activity should be related to the suitable band structure and high charge carrier separation efficiency of WSC-1-In as demonstrated above.6,8,65,69,71
The correlative degradation mechanism proposed for the photocatalytic degradation of MB is based on the known band structure as illustrated in Fig. 6. WSC-1-In is taken as an example, as its CB potential is negative compared to E°(O2/˙O−2) (−0.28 V vs. NHE, pH = 7); when irradiated with solar light, the electrons underwent excitation from the valence band (VB) to the CB. According to literature reports,8,70,71 the excited photoelectrons are captured by the chemically adsorbed O2 molecules on the surface to yield the superoxide radical ˙O−2, which further participates in the degradation of dye molecules. On the other hand, its VB potential is positive compared to E°(˙OH/H2O) (+2.27 V vs. NHE, pH = 7), and the remaining photogenerated holes oxidize H2O molecules to yield reactive ˙OH species. These radicals are reactive species contributing to the degradation of MB to H2O and CO2 as the final products. Although the band gap of WSC-1-In is slightly larger than that of WSC-1 (Fig. 4a), its CB is more negative, which is more favourable for the formation of ˙O−2 with high oxidative ability. Thus, the photocatalytic activity of In-alloyed WSC-1-In is significantly higher than that of WSC-1. As photogenerated electrons worked with holes to promote photocatalytic oxidation during the photocatalytic process, the photodegadation efficiency of WSC-1-In and WSC-1 is greatly enhanced, making them among the most active cluster-based metal chalcogenides for photocatalytic dye degradation (Table S6†). Noticeably, the title compounds are stable and retain their original crystallinity after photodegradation (Fig. S15†), yet the crystallinity of WSC-1 and WSC-1-In was destroyed after two runs of photodegradation (results not shown in this study), probably because of the quite common photocorrosion in sulfide based photocatalysts.76 Moreover, the H2 generation rate of WSC-1-In (∼22.82 μmol h−1 g−1) is ca. 3.4 faster than that of WSC-1 (6.71 μmol h−1 g−1) (Fig. S16†), which is consistent with the dye photodegradation results.
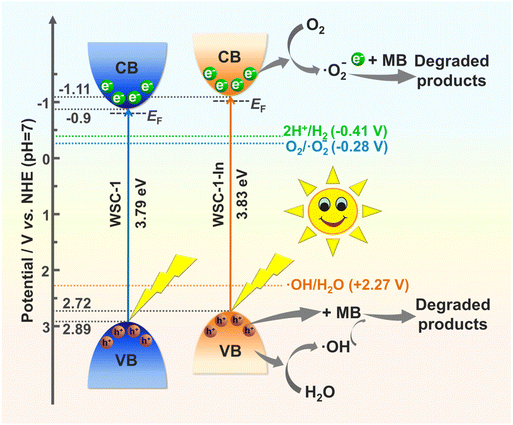 |
| Fig. 6 Schematic illustration of the energy band diagrams of WSC-1 and WSC-1-In and the possible electron and hole transfer mechanism upon solar light illumination. | |
Conclusions
In summary, different from the usual supertetrahedral clusters, a unique wheel-shaped double-decker inorganic gallium-sulfide molecular ring (WSC-1) with an external diameter of ∼1.6 nm is reported here, representing the first case of a main-group metal sulfide nanoring and the largest standard ring in pure metal chalcogenides thus far. The introduction of indium to WSC-1 makes the heteronuclear molecular ring (WSC-1-In) exhibit highly efficient photocatalytic dye degradation toward MB and comparable photocatalytic H2 evolution related to a modulated suitable band structure and enhanced photogenerated carrier separation efficiency. This work revealed the great potential of a new generation of large-sized inorganic metal chalcogenide molecular rings with tunable properties in the application of photocatalysis.
Author contributions
Tao Wu: funding acquisition, project administration, resources, and supervision. Bin Han: data curation, formal analysis, investigation, methodology, and validation. Jia-Xin Liu: investigation. Jiaxu Zhang: formal analysis. Chaozhuang Xue: formal analysis. Xiang Wang: conceptualization, data curation, formal analysis, investigation, methodology, writing – reviewing and editing, and supervision. Dong-Sheng Li: funding acquisition.
Conflicts of interest
There are no conflicts to declare.
Acknowledgements
We acknowledge the National Natural Science Foundation of China (92261205 and 22071165) and the 111 Project (D20015).
References
- J. Zhang, X. Bu, P. Feng and T. Wu, Metal Chalcogenide Supertetrahedral Clusters: Synthetic Control over Assembly, Dispersibility, and Their Functional Applications, Acc. Chem. Res., 2020, 53, 2261–2272 CrossRef CAS PubMed
.
- J. Zhang, P. Feng, X. Bu and T. Wu, Atomically Precise Metal Chalcogenide Supertetrahedral Clusters: Frameworks to Molecules, and Structure to Function, Natl. Sci. Rev., 2021, 9, nwab076 CrossRef PubMed
.
- W. Wang, X. Wang, D. Hu, H. Yang, C. Xue, Z. Lin and T. Wu, An Unusual Metal Chalcogenide Zeolitic Framework Built from the Extended Spiro-5 Units with Supertetrahedral Clusters as Nodes, Inorg. Chem., 2018, 57, 921–925 CrossRef CAS PubMed
.
- J. Lv, J. Zhang, C. Xue, D. Hu, X. Wang, D.-S. Li and T. Wu, Two Penta-Supertetrahedral Cluster-Based Chalcogenide Open Frameworks: Effect of the Cluster Spatial Connectivity on the Electron-Transport Efficiency, Inorg. Chem., 2019, 58, 3582–3585 CrossRef CAS PubMed
.
- J. Zhang, X. Wang, J. Lv, D.-S. Li and T. Wu, A Multivalent Mixed-Metal Strategy for Single-Cu+-Ion-Bridged Cluster-Based Chalcogenide Open Frameworks for Sensitive Nonenzymatic Detection of Glucose, Chem. Commun., 2019, 55, 6357–6360 RSC
.
- M. Hao, Q. Hu, Y. Zhang, M. Luo, Y. Wang, B. Hu, J. Li and X. Huang, Soluble Supertetrahedral Chalcogenido T4 Clusters: High Stability and Enhanced Hydrogen Evolution Activities, Inorg. Chem., 2019, 58, 5126–5133 CrossRef CAS PubMed
.
- D. Hu, X. Wang, X. Chen, Y. Wang, A. N. Hong, J. Zhong, X. Bu, P. Feng and T. Wu, S-Doped Ni(OH)2 Nano-Electrocatalyst Confined in Semiconductor Zeolite with Enhanced Oxygen Evolution Activity, J. Mater. Chem. A, 2020, 8, 11255–11260 RSC
.
- Y. Wang, Z. Zhu, Z. Sun, Q. Hu, J. Li, J. Jiang and X. Huang, Discrete Supertetrahedral T5 Selenide Clusters and Their Se/S Solid Solutions: Ionic-Liquid-Assisted Precursor Route Syntheses and Photocatalytic Properties, Chem. – Eur. J., 2020, 26, 1624–1632 CrossRef CAS PubMed
.
- H. Yang, M. Luo, L. Luo, H. Wang, D. Hu, J. Lin, X. Wang, Y. Wang, S. Wang, X. Bu, P. Feng and T. Wu, Highly Selective and Rapid Uptake of Radionuclide Cesium Based on Robust Zeolitic Chalcogenide Via Stepwise Ion-Exchange Strategy, Chem. Mater., 2016, 28, 8774–8780 CrossRef CAS
.
- X. Chen, X. Bu, Q. Lin, C. Mao, Q.-G. Zhai, Y. Wang and P. Feng, Selective Ion Exchange and Photocatalysis by Zeolite-Like Semiconducting Chalcogenide, Chem. – Eur. J., 2017, 23, 11913–11919 CrossRef CAS PubMed
.
- M.-L. Feng, D. Sarma, Y.-J. Gao, X.-H. Qi, W.-A. Li, X.-Y. Huang and M. G. Kanatzidis, Efficient Removal of [UO2]2+, Cs+, and Sr2+ Ions by Radiation-Resistant Gallium Thioantimonates, J. Am. Chem. Soc., 2018, 140, 11133–11140 CrossRef CAS PubMed
.
- N. W. Rosemann, J. P. Eußner, A. Beyer, S. W. Koch, K. Volz, S. Dehnen and S. Chatterjee, A Highly Efficient Directional Molecular White-Light Emitter Driven by a Continuous-Wave Laser Diode, Science, 2016, 352, 1301–1304 CrossRef CAS PubMed
.
- B.-W. Liu, X.-M. Jiang, H.-Y. Zeng and G.-C. Guo, [ABa2Cl][Ga4S8] (A = Rb, Cs): Wide-Spectrum Nonlinear Optical Materials Obtained by Polycation-Substitution-Induced Nonlinear Optical (NlO)-Functional Motif Ordering, J. Am. Chem. Soc., 2020, 142, 10641–10645 CrossRef CAS PubMed
.
- G. Férey, Supertetrahedra in Sulfides: Matter against Mathematical Series?, Angew. Chem., Int. Ed., 2003, 42, 2576–2579 CrossRef PubMed
.
- X. Xu, W. Wang, D. Liu, D. Hu, T. Wu, X. Bu and P. Feng, Pushing up the Size Limit of Metal Chalcogenide Supertetrahedral Nanocluster, J. Am. Chem. Soc., 2018, 140, 888–891 CrossRef CAS PubMed
.
- D.-D. Yang, W. Li, W.-W. Xiong, J.-R. Li and X.-Y. Huang, Ionothermal Synthesis of Discrete Supertetrahedral Tn (n = 4, 5) Clusters with Tunable Components, Band Gaps, and Fluorescence Properties, Dalton Trans., 2018, 47, 5977–5984 RSC
.
- C. Zimmermann, M. Melullis and S. Dehnen, Reactivity of Chalcogenostannate Salts: Unusual Synthesis and Structure of a Compound Containing Ternary Cluster Anions [Co4(M4-Se)(SnSe4)4]10−, Angew. Chem., Int. Ed., 2002, 41, 4269–4272 CrossRef CAS PubMed
.
- S. Dehnen and M. K. Brandmayer, Reactivity of Chalcogenostannate Compounds: Syntheses, Crystal Structures, and Electronic Properties of Novel Compounds Containing Discrete Ternary Anions [MII4(M4-Se)(SnSe4)4]10− (MII = Zn, Mn), J. Am. Chem. Soc., 2003, 125, 6618–6619 CrossRef CAS PubMed
.
- J. Zhang, C. Qin, Y. Zhong, X. Wang, W. Wang, D. Hu, X. Liu, C. Xue, R. Zhou, L. Shen, Y. Song, D. Xu, Z. Lin, J. Guo, H. Su, D.-S. Li and T. Wu, Atomically Precise Metal-Chalcogenide Semiconductor Molecular Nanoclusters with High Dispersibility: Designed Synthesis and Intracluster Photocarrier Dynamics, Nano Res., 2020, 2, 2828–2836 CrossRef
.
- Y. Liu, Q. Lin, Q. Zhang, X. Bu and P. Feng, Visible-Light-Driven, Tunable, Photoelectrochemical Performance of a Series of Metal-Chelate, Dye-Organized, Crystalline, CdS Nanoclusters, Chem. – Eur. J., 2014, 20, 8297–8301 CrossRef CAS PubMed
.
- C. Xu, N. Hedin, H.-T. Shi and Q.-F. Zhang, A Semiconducting Microporous Framework of Cd6Ag4(Sph)16 Clusters Interlinked Using Rigid and Conjugated Bipyridines, Chem. Commun., 2014, 50, 3710–3712 RSC
.
- F. Kato and K. R. Kittilstved, Site-Specific Doping of Mn2+ in a CdS-Based Molecular Cluster, Chem. Mater., 2018, 30, 4720–4727 CrossRef CAS
.
- X. Han, Z. Wang, D. Liu, J. Xu, Y. Liu and C. Wang, Co-Assembly of a Three-Dimensional Open Framework Sulfide with a Novel Linkage between an Oxygen-Encapsulated T3 Cluster and a Supertetrahedral T2 Cluster, Chem. Commun., 2014, 50, 796–798 RSC
.
- Q. Lin, X. Bu and P. Feng, An Infinite Square Lattice of Super-Supertetrahedral T6-Like Tin Oxyselenide Clusters, Chem. Commun., 2014, 50, 4044–4046 RSC
.
- X. Han, J. Xu, Z. Wang, D. Liu and C. Wang, A Hybrid Linkage Mode between T2,2 and T3 Selenide Clusters, Chem. Commun., 2015, 51, 3919–3922 RSC
.
- W. Wang, H. Yang, C. Xue, M. Luo, J. Lin, D. Hu, X. Wang, Z. Lin and T. Wu, The First Observation on Dual Self-Closed and Extended Assembly Modes in Supertetrahedral T3 Cluster Based Open-Framework Chalcogenide, Cryst. Growth Des., 2017, 17, 2936–2940 CrossRef CAS
.
- J. Lv, W. Wang, L. Zhang, C. Xue, D. Hu and T. Wu, Assembly of Oxygen-Stuffed Supertetrahedral T3-Snos Clusters into Open Frameworks with Single Sn2+ Ion as Linker, Cryst. Growth Des., 2018, 18, 4834–4837 CrossRef CAS
.
- T. Wu, F. Zuo, L. Wang, X. Bu, S.-T. Zheng, R. Ma and P. Feng, A Large Indium Sulfide Supertetrahedral Cluster Built from Integration of Zns-Like Tetrahedral Shell with Nacl-Like Octahedral Core, J. Am. Chem. Soc., 2011, 133, 15886–15889 CrossRef CAS PubMed
.
- W. Wang, X. Wang, J. Zhang, H. Yang, M. Luo, C. Xue, Z. Lin and T. Wu, Three-Dimensional Superlattices Based on Unusual Chalcogenide Supertetrahedral in–Sn–S Nanoclusters, Inorg.
Chem., 2019, 58, 31–34 CrossRef CAS PubMed
.
- X. Zhang, Q. Wang, Z. Ma, J. He, Z. Wang, C. Zheng, J. Lin and F. Huang, Synthesis, Structure, Multiband Optical, and Electrical Conductive Properties of a 3d Open Cubic Framework Based on [Cu8Sn6S24]Z− Clusters, Inorg. Chem., 2015, 54, 5301–5308 CrossRef CAS PubMed
.
- H. Yang, L. Wang, D. Hu, J. Lin, L. Luo, H. Wang and T. Wu, A Novel Copper-Rich Open-Framework Chalcogenide Constructed from Octahedral Cu4Se6 and Icosahedral Cu8Se13 Nanoclusters, Chem. Commun., 2016, 52, 4140–4143 RSC
.
- M. Luo, D. Hu, H. Yang, D. Li and T. Wu, Pcu-Type Copper-Rich Open-Framework Chalcogenides: Pushing up the Length Limit of the Connection Mode and the First Mixed-Metal [Cu7GeSe13] Cluster, Inorg. Chem. Front., 2017, 4, 387–392 RSC
.
- R.-C. Zhang, J.-C. Zhang, Z. Cao, J.-J. Wang, S.-S. Liang, H.-J. Cong, H.-J. Wang, D.-J. Zhang and Y.-L. An, Unusual Flexibility of Microporous Sulfides During Ion Exchange, Inorg. Chem., 2018, 57, 13128–13136 CrossRef CAS PubMed
.
- B. Han, J. Wang, Y. Liu, X. Wang, C. Xue, J. Lv, Z. Wu, R. Zhou, D. Xu, D.-S. Li and T. Wu, Two Copper-Rich Open-Framework Chalcogenides Built from Unusual [Cu5(SnxM1−x)Se10] Clusters and [(SnxM1−x)2Se6] Dimeric Linkers (M = In and Ga), Inorg. Chem., 2020, 59, 7919–7923 CrossRef CAS PubMed
.
- Y. Lin, W. Massa and S. Dehnen, “Zeoball” [Sn36Ge24Se132]24−: A Molecular Anion with Zeolite-Related Composition and Spherical Shape, J. Am. Chem. Soc., 2012, 134, 4497–4500 CrossRef CAS PubMed
.
- B. Seidlhofer, J. Djamil, C. Näther and W. Bensch, From Zero- to Three-Dimensional Thioantimonates: [Ni(Aepa)2]3Sb6S12 (Aepa = C5H15N3 = N-(Aminoethyl)-1,3-Propandiamine), Containing the Unique [Sb6S12]6− Cyclic Anion, [Ni(Aepa)2]6(Sb3S6)2(SO4)3·2H2O, with Isolated [Sb3S6]3− Anions and [Ni(Aepa)2]Sb4S7, Characterized by a Three-Dimensional Network Structure, Cryst. Growth Des., 2011, 11, 5554–5560 CrossRef CAS
.
- Y.-H. Wang, W. Luo, J.-B. Jiang, G.-Q. Bian, Q.-Y. Zhu and J. Dai, A Wheel-Shaped Indium–Telluride Nanocluster [In18Te30(Dach)6]6−: Its Formation and Structure, Inorg. Chem., 2012, 51, 1219–1221 CrossRef CAS PubMed
.
- X. Zhang, Y.-Y. Pu, L.-S. You, G.-Q. Bian, Q.-Y. Zhu and J. Dai, Wheel-Shaped Indium Telluride Nanoclusters Co-Crystallized with Metal-Phenanthroline Complexes, Polyhedron, 2013, 52, 645–649 CrossRef CAS
.
- Y.-H. Wang, J. Wu, X.-W. Zhao, L.-W. Qian, Q.-Y. Zhu and J. Dai, A Nano-Scale Triangular Ring Cluster of Indium–Selenide: The Structure and Templating Effect, Chem. Commun., 2015, 51, 10668–10671 RSC
.
- C. Wang, X. Bu, N. Zheng and P. Feng, A 3D Open–Framework Indium Telluride and Its Selenide and Sulfide Analogues, Angew. Chem., Int. Ed., 2002, 41, 1959–1961 CrossRef CAS PubMed
.
- N. Ding, D.-Y. Chung and M. G. Kanatzidis, K6Cd4Sn3Se13: A Polar Open-Framework Compound Based on the Partially Destroyed Supertetrahedral [Cd4Sn4Se17]10− Cluster, Chem. Commun., 2004, 10, 1170–1171 RSC
.
- S. J. Ewing and P. Vaqueiro, Structural Complexity in Indium Selenides Prepared Using Bicyclic Amines as Structure-Directing Agents, Dalton Trans., 2015, 44, 1592–1600 RSC
.
- C. Xue, L. Zhang, X. Wang, X. Wang, J. Zhang and T. Wu, Highly Open Chalcogenide Frameworks Built from Unusual Defective Supertetrahedral
Clusters, Dalton Trans., 2019, 48, 10799–10803 RSC
.
- M. G. Sorolla, X. Wang, T. Makarenko and A. J. Jacobson, A Large Spin, Magnetically Anisotropic, Octanuclear Vanadium(III) Wheel, Chem. Commun., 2019, 55, 342–344 RSC
.
- J. F. You, G. C. Papaefthymiou and R. H. Holm, [Beta-Na2Fe18S30]8− and [Na9Fe20Se38]9−: High-Nuclearity Clusters by Mono- or Bicyclization of Unidimensional Polymeric Fragments and the Existence of Isomeric Monocyclic Clusters, J. Am. Chem. Soc., 1992, 114, 2697–2710 CrossRef CAS
.
- J. F. You, B. S. Snyder and R. H. Holm, [Na2Fe18S30]8−. A High-Nuclearity Cyclic Cluster Generated Solely by Iron-Sulfur Bridge Bonding, J. Am. Chem. Soc., 1988, 110, 6589–6591 CrossRef CAS
.
- P. King, T. C. Stamatatos, K. A. Abboud and G. Christou, Reversible Size Modification of Iron and Gallium Molecular Wheels: A Ga10 “Gallic Wheel” and Large Ga18 and Fe18 Wheels, Angew. Chem., Int. Ed., 2006, 45, 7379–7383 CrossRef CAS PubMed
.
- E. C. Sañudo, C. A. Muryn, M. A. Helliwell, G. A. Timco, W. Wernsdorfer and R. E. P. Winpenny, Al, Ga and in Heterometallic Wheels and Their by-Products, Chem. Commun., 2007, 801–803 RSC
.
- T. C. Stamatatos, S. Mukherjee, K. A. Abboud and G. Christou, The Largest Single-Strand Molecular Wheel: Ga20 from a Targeted, Diolate-Induced Size Modification of the Ga10′Gallic Wheel’, Chem. Commun., 2009, 62–64 RSC
.
- D. Shi, X. Yang, H. Chen, D. Jiang, J. Liu, Y. Ma, D. Schipper and R. A. Jones, Large Ln42 Coordination Nanorings: Nir Luminescence Sensing of Metal Ions and Nitro Explosives, Chem. Commun., 2019, 55, 13116–13119 RSC
.
- L. Geng, C.-H. Liu, S.-T. Wang, W.-H. Fang and J. Zhang, Designable Aluminum Molecular Rings: Ring Expansion and Ligand Functionalization, Angew. Chem., Int. Ed., 2020, 59, 16735 CrossRef CAS PubMed
.
- Q. Xu, B. Xu, H. Kong, P. He, J. Wang, T. Kannan, P. Ma, J. Wang and J. Niu, Synthesis and Characterization of a Crown-Shaped 36-Molybdate Cluster and Application in Catalyzing Knoevenagel Condensation, Inorg. Chem., 2020, 59, 10665–10672 CrossRef CAS PubMed
.
- R. C. Maji, P. P. Das, A. Bhandari, S. Mishra, M. Maji, K. B. Ghiassi, M. M. Olmstead and A. K. Patra, Mixed Valence Copper–Sulfur Clusters of Highest Nuclearity: A Cu8 Wheel and a Cu16 Nanoball, Chem. Commun., 2017, 53, 3334–3337 RSC
.
- X.-Y. Zheng, Y.-H. Jiang, G.-L. Zhuang, D.-P. Liu, H.-G. Liao, X.-J. Kong, L.-S. Long and L.-S. Zheng, A Gigantic Molecular Wheel of {Gd140}: A New Member of the Molecular Wheel Family, J. Am. Chem. Soc., 2017, 139, 18178–18181 CrossRef CAS PubMed
.
- J. Lin, N. Li, S. Yang, M. Jia, J. Liu, X.-M. Li, L. An, Q. Tian, L.-Z. Dong and Y.-Q. Lan, Self-Assembly of Giant Mo240 Hollow Opening Dodecahedra, J. Am. Chem. Soc., 2020, 142, 13982–13988 CrossRef CAS PubMed
.
- Z. Wu, I. Nußbruch, S. Nier and S. Dehnen, Ionothermal Access to Defined Oligomers of Supertetrahedral Selenido Germanate Clusters, JACS Au, 2022, 2, 204–213 CrossRef CAS PubMed
.
- X. Bu, N. Zheng and P. Feng, Tetrahedral Chalcogenide Clusters and Open Frameworks, Chem. – Eur. J., 2004, 10, 3356–3362 CrossRef CAS PubMed
.
- P. Feng, X. Bu and N. Zheng, The Interface Chemistry between Chalcogenide Clusters and Open Framework Chalcogenides, Acc. Chem. Res., 2005, 38, 293–303 CrossRef CAS PubMed
.
- S. D. Nogai and H. Schmidbaur, Preparation and Structure of Soluble Complexes of the Ternary Compounds Gasbr and Gasebr, Dalton Trans., 2003, 2488–2495 RSC
.
- P. Vaqueiro, M. L. Romero, B. C. Rowan and B. S. Richards, Arrays of Chiral Nanotubes and a Layered Coordination Polymer Containing Gallium–Sulfide Supertetrahedral Clusters, Chem. – Eur. J., 2010, 16, 4462–4465 CrossRef CAS PubMed
.
- W.-W. Xiong, J.-R. Li, B. Hu, B. Tan, R.-F. Li and X.-Y. Huang, Largest Discrete Supertetrahedral Clusters Synthesized in Ionic Liquids, Chem. Sci., 2012, 3, 1200–1204 RSC
.
- Z.-Q. Li, C.-J. Mo, Y. Guo, N.-N. Xu, Q.-Y. Zhu and J. Dai, Discrete Supertetrahedral Cuins Nanoclusters and Their Application in Fabrication of Cluster-Sensitized Tio2 Photoelectrodes, J. Mater. Chem. A, 2017, 5, 8519–8525 RSC
.
- Y. Zhang, X. Wang, D. Hu, C. Xue, W. Wang, H. Yang, D. Li and T. Wu, Monodisperse Ultrasmall Manganese-Doped Multimetallic Oxysulfide Nanoparticles as Highly Efficient Oxygen Reduction Electrocatalyst, ACS Appl. Mater. Interfaces, 2018, 10, 13413–13424 CrossRef CAS PubMed
.
- D. Liu, X. Fan, X. Wang, D. Hu, C. Xue, Y. Liu, Y. Wang, X. Zhu, J. Guo, H. Lin, Y. Li, J. Zhong, D. Li, X. Bu, P. Feng and T. Wu, Cooperativity by Multi-Metals Confined in Supertetrahedral Sulfide Nanoclusters to Enhance Electrocatalytic Hydrogen Evolution, Chem. Mater., 2019, 31, 553–559 CrossRef CAS
.
- C. Xue, L. Zhang, X. Wang, D. Hu, X.-L. Wang, J. Zhang, R. Zhou, D.-S. Li, H. Su and T. Wu, Enhanced Water Dispersibility of Discrete Chalcogenide Nanoclusters with a Sodalite-Net Loose-Packing Pattern in a Crystal Lattice, Inorg. Chem., 2020, 59, 15587–15594 CrossRef CAS PubMed
.
- M.-B. Luo, S.-L. Huang, H.-D. Lai, J. Zhang and Q. Lin, Tin-Oxychalcogenide Supertetrahedral Clusters Maintained in a Mtn Zeolite-Analog Arrangement by Coulombic Interactions, Chem. Commun., 2020, 56, 8388–8391 RSC
.
- J. Lin, Y. Dong, Q. Zhang, D. Hu, N. Li, L. Wang, Y. Liu and T. Wu, Interrupted Chalcogenide-Based Zeolite-Analogue Semiconductor: Atomically Precise Doping for Tunable Electro-/Photoelectrochemical Properties, Angew. Chem., Int. Ed., 2015, 54, 5103–5107 CrossRef CAS PubMed
.
- S.-L. Huang, L. He, E.-X. Chen, H.-D. Lai, J. Zhang and Q. Lin, A Wide Ph-Range Stable Crystalline Framework Based on the Largest Tin-Oxysulfide Cluster [Sn20O10S34], Chem. Commun., 2019, 55, 11083–11086 RSC
.
- L. Nie and Q. Zhang, Recent Progress in Crystalline Metal Chalcogenides as Efficient Photocatalysts for Organic Pollutant Degradation, Inorg. Chem. Front., 2017, 4, 1953–1962 RSC
.
- H. Pei and L. Wang, An Open Framework Chalcogenide Supertetrahedral Cluster as Visible-Light Driven Photocatalysts for Selective Degradation, Cryst. Growth Des., 2019, 19, 5716–5719 CrossRef CAS
.
- H. Pei, L. Wang and M.-H. Zeng, Selectively Photocatalytic Activity of an Open-Framework Chalcogenide Built from Corner-Sharing T4 Supertetrahedral Clusters, Inorg. Chem., 2019, 58, 12011–12016 CrossRef CAS PubMed
.
- C.-Y. Yue, Y.-D. Yue, H.-X. Sun, D.-Y. Li, N. Lin, X.-M. Wang, Y.-X. Jin, Y.-H. Dong, Z.-H. Jing and X.-W. Lei, Transition Metal Complex Dye-Sensitized 3d Iodoplumbates: Syntheses, Structures and Photoelectric Properties, Chem. Commun., 2019, 55, 6874–6877 RSC
.
- H. Wang, Y. Wu, M. Feng, W. Tu, T. Xiao, T. Xiong, H. Ang, X. Yuan and J. W. Chew, Visible-Light-Driven Removal of Tetracycline Antibiotics and Reclamation of Hydrogen Energy from Natural Water Matrices and Wastewater by Polymeric Carbon Nitride Foam, Water Res., 2018, 144, 215–225 CrossRef CAS PubMed
.
- H. Wang, X. Yuan, Y. Wu, G. Zeng, X. Chen, L. Leng and H. Li, Synthesis and Applications of Novel Graphitic Carbon Nitride/Metal-Organic Frameworks Mesoporous Photocatalyst for Dyes Removal, Appl. Catal., B, 2015, 174–175, 445–454 CrossRef CAS
.
- Y. Yan, M. Zhang, K. Gong, L. Su, Z. Guo and L. Mao, Adsorption of Methylene Blue Dye onto Carbon Nanotubes: A Route to an Electrochemically Functional Nanostructure and Its Layer-by-Layer Assembled Nanocomposite, Chem. Mater., 2005, 17, 3457–3463 CrossRef CAS
.
- Z. Wu, X.-L. Wang, X. Wang, X. Xu, D.-S. Li and T. Wu, 0D/2D Heterostructure Constructed by Ultra-Small Chalcogenide-Cluster Aggregated Quaternary Sulfides and g-C3N4 for Enhanced Photocatalytic H2 Evolution, Chem. Eng. J., 2021, 426, 131216 CrossRef CAS
.
Footnote |
† Electronic supplementary information (ESI) available: Experimental details and additional structural presentations: SCXRD data, EDS, PXRD, TGA, FTIR, additional structural figures, Mott–Schottky plots, UV–vis absorption spectra and additional photocatalytic results (PDF). CCDC 2012952 (WSC-1). For ESI and crystallographic data in CIF or other electronic format see DOI: https://doi.org/10.1039/d3qi00432e |
|
This journal is © the Partner Organisations 2023 |