DOI:
10.1039/D3NR03527A
(Minireview)
Nanoscale, 2023,
15, 17313-17325
The nano-revolution in the diagnosis and treatment of endometriosis
Received
19th July 2023
, Accepted 8th October 2023
First published on 19th October 2023
Abstract
Endometriosis is a painful gynecological disease with a high prevalence, affecting millions of women worldwide. Innovative, non-invasive treatments, and new patient follow-up strategies are needed to deal with the harmful social and economic effects. In this scenario, considering the recent, very promising results already reported in the literature, a commitment to new research in the field of nanomedicine is urgently needed. Study findings clearly show the potential of this approach in both the diagnostic and therapeutic phases of endometriosis. Here, we offer a brief review of the recent exciting and effective applications of nanomedicine in both the diagnosis and therapy of endometriosis. Special emphasis will be placed on the emerging theranostic application of nanoproducts, and the combination of phototherapy and nanotechnology as new therapeutic modalities for endometriosis. The review will also provide interested readers with a guide to the selection process and parameters to consider when designing research into this type of approach.
1. Introduction
Nanotechnology is the area of science and engineering in which nanoscale phenomena occur, and is gaining considerable worldwide attention in the field of medicine.1,2 A large number of reports suggest that nanoparticles (NPs) have the potential to enhance conventional therapeutic modalities (e.g., chemotherapy) and imaging modalities (e.g., MRI) in order to detect (e.g., photoacoustic imaging) and treat (e.g., photothermal therapy [PTT] and magnetic hyperthermia) various diseases.3,4 NPs are promising vehicles for the delivery of drugs and imaging agents to disease sites because they can be conjugated to drugs, antibodies, or other chemical compounds.5,6 In particular, several studies have shown how NPs, with their low toxicity, high stability, and the possibility of conjugation with several biomolecules, could be used to deliver anti-inflammatory, antioxidant, anti-angiogenetic, or immunomodulating molecules at specific targets.7–9 NPs are mainly designed to enhance stability, delivery efficiency, and targeted release length for molecules with limited bioavailability.10–12 They are used to optimize the biodistribution of drugs to diseased organs, tissues, or cells to improve and target drug delivery. NPs can release drugs in response to a laser triggering photothermal effects, radiofrequency waves, and ultrasounds; for all these reasons, they have been investigated in combination with radiation physics for on-demand drug delivery.13–15 With the rapid development of nanotechnology, several multifunctional nanoscale agents have been successfully synthesized, especially in the field of cancer therapies.16–18 However, many researchers/experts agree that manipulating things at the nanometer level could be successful in tissue engineering and vaccine development and could pave the way for the development of diagnostic/therapeutic approaches and monitoring of other human diseases, which will revolutionize health care in the coming years.19 A growing body of work clearly demonstrates that the “nanoscale revolution” has also laid its foundations in a complex disease, namely endometriosis. The endometriosis is an estrogen-dependent chronic gynecological disorder in which the endometrium tissue that normally lines the uterus forms lesions outside of the uterus and is one of the most disabling conditions for women of reproductive age, for which there are no side-effect-free therapies and for which early diagnosis is indispensable to limit the physical and psychological health damage associated with the progression of the disease.20–22 Several reports have already shown that NPs could help treat endometriosis in animal models by localizing and removing painful and dangerous lesions in the ovaries, fallopian tubes and pelvis without the need for invasive surgery.3,23 This is possible by exploiting the tendency of NPs to have a higher accumulation in tissue characterized by higher vascularization, such as the endometriotic tissue by the so-called enhanced permeability and retention (EPR) effect, or promoting the accumulation of NPs in endometriotic lesions by modifying their surface with targeting ligands that bind overexpressed receptors in endometriotic cells (the so-called active targeting).24 Although nanomedicine for endometriosis is a budding field, this review intends to highlight the diagnostic and therapeutic importance of the application of NPs in endometriosis, and at the same time provide insights of interest to researchers aiming for new diagnostic/therapeutic approaches to address other diseases of the reproductive system, and finally represent a great hope for physicians and patients to address the challenges of endometriosis more effectively.
After a brief description of the disease, we provide an update on recent advances in nanomedicine in the diagnosis and treatment of this condition, concluding with a review of published work, showing how the combination of photothermal therapy with nanotechnology can achieve breakthroughs in the treatment of endometriosis. With regards to the latter, we focused on the selection of the laser device to be used and of the light treatment parameters, on which the outcomes of photothermal experiments depend.
2. Endometriosis – an overview
Endometriosis typically affects women and girls of reproductive age, with a prevalence ranging from 6% to 10%,25 although it may sometimes be diagnosed also in menopause.26 Endometriosis is responsible for pain symptoms and infertility, which can severely impact the quality of life of affected patients.27,28 Endometriotic implants may be found in different sites. They are found most frequently on the pelvic peritoneum, ovaries, uterosacral ligaments, bowel, and rectovaginal septum. More rarely, they can be found in extra pelvic localizations, such as the diaphragm, umbilicus, pericardium, and pleura. The exact pathogenesis of endometriosis is still unknown, but several leading theories include retrograde menstruation, altered immunity, coelomic metaplasia, and metastatic cell spread.29 Nevertheless, a unifying theory regarding the origin of endometriosis still remains elusive; in fact, the heterogeneity and differences among the three main classes of endometriosis presentation may suggest different multiple pathogenetic pathways.
There is increasing evidence to support the concept of endometriosis as a pelvic inflammatory condition. In women with endometriosis, the peritoneal fluid is remarkable for an increased number of activated macrophages and important differences in the cytokine/chemokine profile. The peritoneal microenvironment is notably rich in prostaglandins, and these mediators likely play a central role in disease pathophysiology, as well as clinical sequelae of pain and infertility.30 In addition, hormonal alterations may influence the ability of endometrial cells to proliferate, establishing and maintaining implants. Long-appreciated clinically, the concept of endometriosis as an estrogen-dependent disorder is well supported by molecular evidence. A striking finding in endometriotic tissue relative to eutopic endometrium is the increased expression of the aromatase enzyme and decreased expression of 17β-hydroxysteroid dehydrogenase (17β-HSD) type 2. The sum consequence of this differential expression profile is a marked increase in the locally bioavailable estradiol concentration. Estradiol stimulates the production of prostaglandin E2, which further stimulates aromatase activity.31
There are several methods to diagnose endometriosis, such as laparoscopy, transvaginal ultrasound (TVUS), and magnetic resonance imaging (MRI).32 Due to the heterogeneity of location and appearance of endometriotic lesions, these diagnostic tests are inadequate, and often lead to a delay in diagnosis, or even misdiagnosis.33 In addition, available treatments, such as pharmacological and surgical approaches, help relieve the symptoms but cannot lead to a permanent cure.34 Pain can be treated by excising peritoneal implants, deep nodules, and ovarian cysts, or inducing lesion suppression by abolishing ovulation and menstruation through hormonal manipulation with progestins, oral contraceptives and gonadotropin-releasing hormone agonists. Medical therapy is symptomatic, not cytoreductive, while surgery is associated with high recurrence rates. Although lesion eradication is considered a fertility-enhancing procedure, the benefit on reproductive performance is moderate. Assisted reproductive technologies represent a valid alternative. Surgical excision of endometriosis significantly ameliorates pain symptoms;35 however, it may be associated with complications. Moreover, recurrence rates of pain symptoms after surgery are such as to require attention.36 In addition, in the case of ovarian endometriomas, there is concern about the risk of damage to the ovarian reserve.37 Faced with the paucity of treatment approaches, research has been focusing on nanotechnologies. Although their application in endometriosis is still to be considered innovative, the available reports suggest that NPs-based strategies can provide new tools for treating this disease.
3. Applications of nanotechnology in endometriosis
As stated in the Introduction section, in the field of endometriosis nanomedicine, the range of new diagnostic and therapeutic approaches is incredibly exciting.3 As recently reviewed by Yuxue et al. nanomaterial-based strategies have a great impact in the treatment of endometriosis, such as nanomaterials alone, nanomaterial-based drug therapy, gene therapy, photothermal therapy, immunotherapy, and magnetic hyperthermia.23 In general, what clearly emerges from the literature, and which the interested reader will find out in the following subsections, similarly to cancer field, are the nanotechnological approaches developed targeting specific features of endometriosis, while exploiting the enormous diagnostic, therapeutic and theranostic potential of certain types of NPs. Endometriosis and cancer share many pathophysiological features (e.g., angiogenesis, reactive oxygen species (ROS) production, etc.), and some fundamental principles of cancer nanomedicine can be or have been adapted for the development of novel nanoparticle-based strategies for the treatment and imaging of endometriosis.3 As with cancer nanotechnology, NPs differing in chemical composition (organic, inorganic and carbon-based) have been used for the diagnosis and therapy of endometriosis, by surface modification with specific targeting molecules and with/without drug loading (Table 1). Here, we set out to offer a summary of the enormous potential of nanotechnology as a diagnostic, therapeutic and theranostic approach in endometriosis by reporting some compelling results described in the literature. As regards therapy, given the great promise of PTT-nanomaterials, we highlight the results from PTT-nanomaterial combinatorial therapeutic approaches. To this end, we also discuss some of the characteristics of lasers to be considered during the experimental planning phase.
Table 1 Summary NP-based approaches for endometriosis diagnosis and therapy
Type of NP |
Cargo molecules |
Target |
Model/concentration NP |
Route of administration |
Biological effect |
Application |
Ref. |
Abbreviations: USPIOs, ultrasmall super magnetic iron oxide nanoparticles; MRI, magnetic resonance imaging; Fe3O4 NPs, magnetic iron oxide nanoparticles; HA, hyaluronic acid; CD, cluster of differentiation; AuNRs, gold nanorods; PA, photoacoustic imaging; MWCN, multi-walled carbon nanotubes; Ab, antibody; CA, carbohydrate antigen; AuNPs, gold nanoparticle; HP, haptoglobin; nanoceria, cerium oxide nanoparticles; OS, oxidant stress; ROS, reactive oxygen species; PLGA, poly(lactic-co-glycolic acid); EGCG, epigallocatechin gallate; Dox, doxycycline; MMP, metalloproteinase; ESCs, primary endometrial stromal cells; CESCs, ESCs obtained from patient without endometriosis; EuESCs, ESCs from eutopic endometriotic lesions; EctESCs, ESCs obtained from ectopic endometrium of patients with endometriosis; CTLA4, cytotoxic T-lymphocyte antigen 4; CCR5, C–C chemokine receptor type 5; TGF-β, transforming growth factor-B; PCL, poly ε-Caprolactone; CaNPs, calcium carbonate nanoparticles; LDE, lipid nanoparticle; LDL, low-density lipoprotein receptor; PEI, polyethyleneimine; SA, stearic acid; albumin-NP, albumin nanoparticles; GOx, glucose oxidase; HAuNS, hollow gold nanospheres; EphB4, Ephrin type-B receptor 4; TNF-α Tumor necrosis factor alpha; PTT, photothermal therapy; SiNC, dye silicon naphthalocyanine; AgNPs, silver nanoparticles; MMAE, monomethyl auristatin E; TNC-C, tenascin C domain C; Fn-EDB, fibronectin extra domain-B; 12Z cells, human immortalized endometriotic epithelial; HESC, human immortalized endometrial stromal HESC; MN, iron oxide magnetic; KDR, kinase insert domain receptor; VEGFR-2, vascular endothelial growth factor receptor 2; \: not reported. |
USPIOs |
— |
Macrophages |
Rats |
Intravenously delivered via the tail vein |
— |
MRI |
38
|
Fe3O4 NPs |
HA |
CD44 |
Rat 10 mg per kg |
Intravenously delivered via the tail vein |
— |
MRI |
51
|
AuNRs |
— |
Endometriotic lesions |
Mice 0.06 mg Au mL−1 |
Injected into peritoneal cavity |
\ |
PA imaging |
63
|
MWCN |
Ab Anti-CA19-9 |
CA19.9 |
Women blood |
— |
— |
Immunosensor |
77
|
AuNPs |
Ab Anti-CA125 |
CA125 |
Women blood |
— |
— |
Lmmunosensor |
81
|
AuNPs |
Ab anti-HP |
HP |
Serum samples |
— |
— |
Lmmunosensor |
83
|
Nanoceria |
— |
OS marker; angiogenesis marker |
Mice 0.5 mg/kg |
Injection into the peritoneal cavity of the abdominal wall |
Decreasing ROS, angiogenesis; endometrial glands and microvessels density |
Therapy |
88
|
PLGA |
EGCG and DOx |
OS marker; angiogenesis marker |
Mice |
Intravenously delivered via the tail vein |
Decreasing OS, angiogenesis, and MMP activity |
Therapy |
91
|
PLGA |
Copaiba oleoresin |
— |
ESCs, CESCs, EuESCs and EctESCs 0.3 mg per ml |
Cell medium |
Morphology alterations, necrosis, apoptosis |
Therapy |
96
|
PLGA |
Anti-CTLA-4 |
CTL4 |
Mice |
intravenously delivered via the tail vein |
cell inhibition, proliferation, invasion |
Therapy |
99
|
PLGA |
Anti-CCR5 |
CCR5 |
Mice |
Intravenously delivered via the tail vein |
Reduction of macrophages, IL-10, TGF-β |
Therapy |
100
|
PCL |
Curcumin |
\ |
Mice |
Implanted nanosystem in the peritoneum |
Reduction of endometrial glands, stroma, inflammatory cells, MMP-activity |
Therapy |
101
|
CaNPs |
BML-111 |
\ |
Mice |
Intravenously injected |
Enhanced efferocytosis, reduced inflammation |
Therapy |
102
|
LDE |
— |
LDL receptor |
Women |
Injected intravenously |
Cell internalization |
Therapy |
104
|
PEI–SA |
HA/DNA and siRNA |
CD44/beclin-1 gene |
Mice |
Intravenously injected |
Regulation of autophagic activity |
Therapy |
106
|
Albumin-NPs |
GOx |
Neutrophils (CD11b+ cells) |
Mice |
Intraperitoneally injected |
Reduction of neutrophils |
Therapy |
108
|
HAuNS |
TNYL peptides |
EphB4 |
Mice 1.25 mg per ml |
Intravenously injected |
Inhibition of lesion growth, decreased levels of TNF-α and estradiol |
PTT |
128
|
PCL |
SiNc |
Vasculature of endometriotic grafts |
Mice |
Via lateral tail-vein injection |
Apoptosis of endometriotIc lesions |
Imaging and PTT |
129
|
AgNPs |
PL1/PL1-MMAE |
TNC-C and Fn-EDB |
12Z and HESC cells 0.3 nM |
Cell medium |
Guided surgery, imaging, viability suppressor |
Imaging and therapy |
56
|
MN |
KDR |
VEGFR-2 |
Mice |
Intravenous injected with 200 μL of KDR-MN |
MRI application, cell death |
Imaging and therapy |
107
|
3.1 Molecular imaging and immunosensors: the promise of nanotechnology for endometriosis diagnosis
The diagnosis of endometriosis can be a lengthy procedure and it is complicated by the various mechanisms involved in the etiologies.32 Several pre-clinical studies have explored the application of NPs for endometriosis diagnosis, with exciting up-front results (Table 1). Lee et al. presented a new diagnostic tool for the evaluation of experimentally-induced endometriosis.38 They considered the role of ultrasmall superparamagnetic iron oxide (USPIO). USPIOs (size <50 nm) belong to the SPIO family, which have been approved by the US Food and Drug Administration (FDA) and European Commission as MRI contrast agent.39 USPIOs possess a magnetic core composed of magnetite, maghemite or other insoluble ferrites which makes them ideal candidates for MRI.40,41 MRI usually involves imaging in which the tissue contrast is weighted according to the T1 and T2 relaxation properties of the tissue.42 In brief, T1-weighted images optimally show normal soft tissue anatomy and adipose tissue, while T2-weighted images optimally show fluid and pathological conditions.43 USPIOs are considered T2 contrast agents and can therefore be used instead of gadolinium-based as they are less toxic and can increase magnetic susceptibility.44 Taking advantages of low cost, biosafety and flexibility of surface modifications,40,45–47 in the present work, USPIO were applied as contrast agent in MRI detection for the evaluation of ectopic uterine tissue (EUT). Since they are internalized by macrophages48,49 that are abundant in the endometriotic lesions and peritoneum, it is could be a new diagnostic approach.38,50 A few years later, Zhang et al. synthesized NPs modified with hyaluronic acid (HA) and magnetic iron oxide nanoparticles (Fe3O4 NPs).51 In recent years, Fe3O4 NPs have gained increasing attention in various biomedical applications due to their magnetic properties.52,53 In the proposed work, HA-Fe3O4 NPs was used as negative contrast agents for MRI in tumor cells over-expressing CD44 receptors. Since this receptor is over-expressed also in endometriotic cells,54,55 they successfully applied this nanosystem also in in vivo imaging of endometriotic lesions in rats. Moreover, Simòn-Gracia et al. designed a nanovector based on silver NPs (AgNPs) functionalized with synthetic PL1 peptide (Fig. 1A).56 Among the promising nanomaterials, AgNPs belong to inorganic materials and are increasingly used in biomedical field. They possess several properties, including optical, electrical, thermal, and high electrical conductivity.57–59 AgNPs can be functionalized with ligand and homing peptides, but also labelled with a fluorophore that makes them brighter by increasing their fluorescence intensity.57,58,60,61 Thanks to their low cytotoxicity, easy preparation, high stability, biocompatibility, and functionalization, in the work proposed by Simòn-Gracìa, AgNPs were used as tracker in cells and tissues by optical imaging. The AgNPs were synthesized according to the citrate method of Lee and Meisel62 and functionalized with PL1, that is well internalized by endometriotic immortalized cell lines. It is possible to apply the nanosystem both in diagnostics (e.g., guided surgery, imaging) as well as in the therapeutic field with the conjugation of a drug.56 Marquardt et al. showed an imaging gold-fluorescein isothiocyanate (FITC) with photoacoustic (PA)-coupled NPs that can be used in deep endometriosis.63 Gold nanoparticles (AuNPs) are one of the most widely investigated inorganic nanomaterials. The high surface area, unique size-dependent properties and precise tunability of NPs make them ideal candidate for multifunctional platform in biomedical application.64–67 AuNPs application depend strongly upon their size and shape, and they may be controlled trough their synthesis methods.68,69 Moreover, they exhibit a strong and tunable optical absorption resulting from the surface plasmon resonance (SPR) effect.70 The SPR effect occurs when the free charges on the surface of AuNPs oscillate with the electromagnetic field, leading to an optical absorption that is several orders of magnitude higher than that of organic dyes.64,71,72 In the present work, gold nanorods were coated with silica shells via the silica sol–gel process and attached them with a distal fluorescein tag (FITC) using simple silane chemistry.73 Transmission electron microscopy (TEM) showed the distinctive rod structure of gold particles with a width of 12–14 nm and a length of 50–55 nm.63 The major advantage was the increased signal penetration due to the fact that ultrasonic waves can spread further than normal light; they are emitted by thermoelastic expansion under near-infrared (NIR) light illumination (680–980 nm).74–76
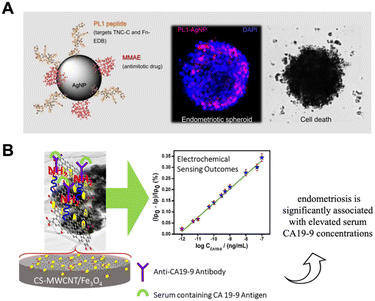 |
| Fig. 1 Use in diagnosis of endometriosis. (A) Design of AgNPs with PL1 peptide that can be combined with MMAE. Confocal images show the internalization of PL1-AgNPs into Z12 cell spheroids. SEM images demonstrate the therapeutic capacity of the nanovector. Adapted with the permission of ref. 56. (B) Schematic representation of CS-MWCNT/Fe3O4 bio-nanocomposite based on electrochemical immunosensor for detecting CA19.9 antigen in endometriosis diagnostic applications.77 Reprinted with permission from Elsevier (License number 5582481039205). | |
In a similar way, Ding et al. reported a (photoacoustic) PA imaging microscopy method for in vivo endometriosis mice models.78 In the context of diagnosis, several diagnostic techniques are available for the preparation of NPs-based immunosensors. They are highly sensitive, and can be used for direct, non-invasive measurement of protein levels in the clinical sample, in comparison to ELISA and fluorescence tests.79 They are receiving increasing attention because of their many advantages, such as cost-effectiveness, high sensitivity, and rapid results. Kalyani et al.77 reported the first bio-nanocomposite immunosensor that showed high sensitivity for the detection of CA19-9 (tumor antigen 19-9),80 a potential biomarker for endometriosis diagnosis (Fig. 1B).77 The film of nanocomposite, composed of chitosan (CS), a linear polysaccharide polymer, multi-walled carbon nanotubes (MWCNT), and Magnetite (Fe3O4), was fabricated dropping the solution of CS-MWCNT-Fe3O4 on a glassy carbon electrode (GCE) surface.77 In detail, CS has been shown to interact with MWCNTs to form stable dispersions; Fe3O4 has superparamagnetic property, biocompatibility, good electrocatalytic activity, and low toxicity and used as an attention-grabbing material for the immobilization of biomolecules. The CS capping MWCNT-Fe3O4 composite on the modified GCE enhances the stability of the electrode surface and effective immobilization of the antibody.77 In this study, a monoclonal anti-CA19-9 antibody was immobilized on the composite modified electrode for label-free, selective, and sensitive capture of CA19-9 antigen. Antibodies are commonly used in immunosensors for antigen detection. Therefore, the proper immobilization of antibodies on the transducer surface is very important and is possible using micro/nanostructured composites. The sensing performance of the immunosensor was tested by detecting serum samples from endometriosis patients and validated using the enzyme-linked immunosorbent assay (ELISA). The results showed that this CA19-9 detection system showed high sensitivity and rapid detection of the CA19-9 biomarker, as well as being very accurate and precise, cost-effective and easier to perform than conventional techniques such as ELISA.77 In a similar way, another group81 proposed an immunosensor to detect CA125: a carbohydrate antigen irregularly produced in severe endometriosis.82 This immunosensor is based on AuNPs and reduced graphene oxide (rGO) obtained through electrochemical deposition, an approach that facilitated the in situ reduction of GO and Au3+. Interestingly, the fabricated immunosensor demonstrated, showed better results for the detection of CA 125 in endometriosis patient blood samples compared to the standard ELISA method (the calculated maximum Relative Standard Deviation (RSD) from the fabricated immunosensor was 6.6% while the maximum RSD value obtained from the standard ELISA kit was 7.3%).81 The immunosensor proposed for the evaluation of glycoprotein haptoglobin (HP) is also based on Au/rGO hybrid film.83 In cases of endometriosis, the HP gene and HP protein have abnormal levels in liver cells, and they are unusually glycosylated.84 The anti-HP antibody was immobilized on the Au/rGO GCE via ethyl-N′-(3-dimethyl aminopropyl) carbodiimide hydrochloride–N–hydroxysuccinimide (EDC–NHS) coupling with the help of 1-mecaptoundecanoic acid (11-MUA).83 Although negligible variation in the detection of HP in human serum plasma was detected compared to the standard ELISA method, the result clearly showed that the proposed detection platform has high sensitivity, selectivity, reproducibility, stability and reusability and could be used for the robust determination of the endometriotic HP biomarker in clinical laboratories.83
3.2 Emerging therapeutic and theranostic nanotechnology approaches in endometriosis
Although endometriosis is a complex disease and the underlying mechanisms still elusive, there are distinctive features that can be harnessed for the development of selective and targeted therapeutic strategies, including NPs.85 For example, it has been shown that there is a positive association between oxidative stress (OS) and endometriosis, just as excessive endometrial angiogenesis is believed to be a critical mechanism in the pathogenesis of this disease.86,87 Chaudhury et al. obtained successful outcomes in the mitigation of endometriosis using regenerative cerium oxide NPs (nanoceria).88 Nanoceria, characterized by the co-existence of fully oxidized Ce4+ and fully reduced Ce3+, has attracted much attention due to its ability to act as a scanner of free radicals (especially superoxide radical and hydrogen peroxide) in biological systems. The authors showed that injection of nanoceria (a single dose of 0.5 mg per kg body weight) into the peritoneal cavity of the abdominal wall significantly mitigated the endometrial lesions induced in mice model by decreasing oxidative stress lower OS parameter such as ROS, lipid peroxidation (LPO) and total antioxidant capacity (TAC) and inhibiting angiogenesis lower expression of vascular endothelial growth factor (VEGF) and adrenomedullin (ADM) as compared to a known antioxidant (N-acetyl cysteine; NAC- a dose of 250 mg per kg body weight thrice a week for 15 days)88 This model also showed a promising reduction in endometriosis-related negative effects on oocyte quality, which is a critical factor for pregnancy success.88 Poly(lactic-co-glycolic acid) (PLGA) is one of the most effective biodegradable polymeric NPs. It has been approved by both the US Food and Drug Administration (FDA) and the European Medicines Agency (EMA) for use in drug delivery systems due to its controlled and sustained release properties, low toxicity, and biocompatibility with tissues and cells.89 The Extracellular matrix degradation play also an important role the pathogenesis of endometriosis.90 Singh et al. developed a dual drug-loaded PLGA NPs that combines the anti-angiogenic and antioxidant properties of epigallocatechin gallate (EGCG) and the matrix metalloproteinase inhibitory activity of antibiotic doxycycline (Dox)91 in order to improve and reduce the limitations when they are used in their native forms. The novelty of this nanosystem is given by the delivery of two different agents (Dox and EGCG) in a single vehicle: the dual agent-NPs was more efficient at reducing levels of metalloproteinase (MMPs) and angiogenic factors in mice with endometriosis than single drug-loaded NPs. Another further confirmation of efficacy by Dox-EGCG NP compared to the Dox NPs and EGCG NPS-treated groups and drugs alone was the significant reductions in endometrial gland and micro vessel density. Moreover, treatment with Dox-EGCG NPs in mice with endometriosis, seemed to be favorable in terms of the number and quality of oocytes.91 A few years later, De Almeida Borges et al. developed a PLGA NPs loaded with copaiba oleoresin (COPA), obtained from Copaifera landgroffii.92,93 COPA is chemically defined as a solution of diterpene acids in an essential oil. The diterpene (the β-caryophyllene) in COPA possess anti-inflammatory and antitumorigenic activity94 and it is able to cause regression of endometrial implants in a rat model of endometriosis without affecting fertility.95 The authors hypothesized that the administration of COPA in lamellar silicate nanocomposites could increase the potential of its therapeutic effects by enhancing the pharmacological efficacy of COPA and reducing its distribution in other tissues. From the in vitro investigation, resulted that COPA was released from the nanocomposite in a delayed manner, a finding associated with reduced viability and proliferation of endometriosis cell cultures than untreated controls.96 PLGA NPs were also studied as a delivery method for certain antibodies, such as the anti-CTLA-4 antibody (the main suppressive immune checkpoint)97 and the anti-CCR5 antibody (C–C chemokine receptor type 5).98 Anti-CTLA-4/PLGA NPs were reported to constantly release anti-CTLA-4 antibodies providing a greater inhibition of ectopic endometrial cell proliferation and invasion in a mouse model than anti-CTLA-4 alone.99 Anti-CCR5/PLGA bioconjugate showed a significant reduction in macrophage, IL-10, and TGF-β levels, with an associated reduction in the proliferation and invasion capacity of ectopic endometrial cells compared to anti-CCR5 alone.100 Furthermore, many studies have investigated the potential role and molecular mechanisms of curcumin in endometriosis by suppressing angiogenesis, oxidative stress, inflammation, and controlling cell proliferation, apoptosis, invasion, and attachment. For example, Boroumand et al. designed a curcumin-loaded nanofiber to target endometriosis in the peritoneum of mice. The authors investigated nanofibers of poly ε-caprolactone (PCL) and polyethylene glycol (PEG) polymers loaded with curcumin as an implantable anti-endometriosis scaffold capable of continuously releasing curcumin onto endometriotic implants. PCL is a biodegradable, biocompatible polymer that makes it a more reasonable choice for an implanted scaffold. The release of curcumin from this nanosystem was approximately of 23% during 30 min, 35% at 24 h, and 50% at 30 days compared to the curcumin alone; in addition, curcumin was more stable in the nanosystem than alone.101 Similarly, a group of researchers proposed an acid-sensitive calcium carbonate NP (CaNP) with BML-111, which is an anti-inflammatory agent.102 Among different inorganic materials, calcium carbonate NPs offer many advantages, including bioavailability, low cost, safety, biocompatibility, pH sensitivity and slow biodegradability. They can be combined with drugs to achieve various treatments, including chemical therapy, gene therapy, phototherapy and immunotherapy.102,103
This nanosystem promoted apoptosis of endometriotic stromal cells, increased the uptake of apoptotic cells by macrophages (a process called efferocytosis), and reduced inflammation by decreasing cytokine secretion.11 Another preliminary nanotechnological approach consisted of an artificial lipid emulsion (LDE) with LDL-receptor-like characteristics. Bedin et al. showed preliminary results in which they found a high uptake of labelled LDE by the topical endometrium, which can be used as a nanosystem.104 Another mechanism of endometriosis that can be studied regard the autophagy process, in which Beclin-1 play an essential positive regulatory role.105 Zhao et al. devised a hyaluronic acid (HA) (PEI-SA/DNA) as a tool for Beclin-1 gene delivery and consequent Beclin-1 upregulation within endometrial lesions. (Fig. 2A). In details, (PEI-SA/DNA) HA can reach CD44, which is over-expressed in the lesion tissue and internalized by endometrial cells.55 It resulted in an enhanced autophagy and inhibition of endometriotic pathogenesis and progression. From these preliminary data, the nanosystem appears to be sufficient to promote autophagy induction and, to some extent, mediate therapeutic efficacy in vivo.106
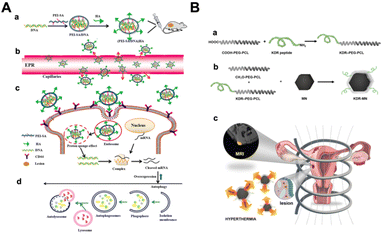 |
| Fig. 2 Therapeutic application in endometriosis. (A) Schematic representation of autophagy process mediated by HA with Beclin-1 nanovector (PEI-SA/DNA): (a) after PEI-SA/DNA construction, (b) it is internalized through trough passive targeting (EPR effect), (c–d) and then binds the CD44 receptor. Finally, it enters the endometriotic cells. Adapted with the permission of ref. 106. (B) Schematic illustration of KDR-MN used as MRI contrast agents: (a and b) NPs were first prepared using PEG-PCL, KDR peptide and MN. (c) KDR-MN accumulation allowed MRI to detect the endometriotic lesion.107 Reprinted with permission from Reprinted with permission from John Wiley and Sons (License number 5582490980873). | |
A recent article described a new therapeutic approach using neutrophils as a target.108 Here, Zhu et al. designed a glucose oxidase-loaded bovine serum albumin NP (BSA-GOx-NP) that is internalized by neutrophils in vivo. Albumin nanoparticles can be synthesized using various approaches109 and can be exploited for active and passive targeting110 and in controlled drug delivery. Moreover, they have been approved by FDA for clinical use.108 The enzymatic activity and cytotoxicity of GOx were preserved, inducing eutopic lesion apoptosis with undetectable side effects. The proposed nanosystem can be a promising tool in the inflammatory endometriosis microenvironment.108
The combination of diagnostics and therapy (the so-called theranostic) is also possible in the context of nanomedicine. For example, Simòn-Gracia et al.56 tested the efficacy of AgNPs functionalized with synthetic PL1111 both in endometriosis diagnostics (see above) and therapy. PL1 is able to recognize tenascin C domain (TNC-C) and fibronectin extra domain-B (Fn-EDB) on endometriotic cells and can be internalized (Fig. 1A). The study showed the ability of AgNPs loaded with the antimitotic drug monomethyl auristatin E (MMAE) to suppress the viability of endometriotic cells cultured in 2D, and as spheroids in a peptide-dependent manner.56 Moreover, Park et al. devised iron oxide magnetic NPs (MN) coupled with a kinase insert domain receptor (KDR) able to accumulate in endometriotic lesions targeting vascular endothelial growth factor receptor 2 (VEGFR-2) (Fig. 2B).107 In the study on KDR-MN, exposure to an alternating magnetic field (AMF) can result in it being heated to over 50 °C, causing cell death. In addition, the nanovector showed promising use as MRI contrast agents (before AFM) in endometriosis diagnosis.107
Taken together, all these examples make it clear that the increasing success of these therapies is due to their ability to induce cell death through key cellular mechanisms such as the induction of ROS generation mediated by NPs.
3.3 Combining nanotechnology with phototherapy
Recent efforts in nanomedicine have promoted the rapid development of light-induced theranostics based on versatile nano-agents with rich, light-induced functions, including near-infrared (NIR) to visible light conversion, photodynamic therapy (PDT), and photothermal therapy (PTT).112 Here we discuss recent advances in the application of phototherapy in endometriosis (Table 1). In PTT, NIR light is converted into heat to induce local hyperthermia, thus exploiting the susceptibility of cells to denature their proteins and induce cell death (Fig. 3A).113,114 NPs-based phototherapy involves the systemic or local administration of a nanosystem, followed by NIR laser irradiation (700 nm to 1350 nm). The ideal nanosystem should possess high absorption efficiency, photothermal conversion efficiency, low toxicity, and a good targeting ability.115 Many NPs can be used as photothermal agents such as metal NPs, carbon nanomaterials, black phosphorus-based nanomaterials, and metal sulphide.116 It is now understood that to improve photothermal performance, photothermal nanomaterials can be developed to be composed of a single or more components and can involve more than one photothermal conversion mechanism.116,117 Among all, AuNPs have been the most widely used nanomaterials in PTT in recent decades. Due to special interactions with light, the free electrons of gold nanostructures undergo a collective, coherent oscillation process known as localized surface plasmon resonance.118–120 The AuNPs can be designed and produced through different techniques, including chemical synthesis (i.e. Turkevich121 and the Brust122 methods, Seed-Mediated Growth123 and Digestive Ripening)124 and biological synthesis using biomass a plethora of organisms, ranging from bacteria to plants, algae, and fungi.65 Considering AuNPs, in order to improve their photothermal effect, it was found useful to modify their organization as clusters or shells or similarly, combining them with different materials (e.g., silica and graphene).125 Moreover, a group of researchers show that the photothermal conversion efficiency of the flower-like-nanocopper sulphide was elevated by 50% in comparison to ordinary hexagonal sulphide NPs,126 demonstrating the importance of NPs’ morphology. Within the different strategies explored to enhance NPs-mediated PTT, the encapsulation of NIR responsive small molecules have shown to display promising results.127 To further improve PTT and reduce side effects, nanomaterials must be functionalized to achieve site-specific PTT with protein denaturation, membrane disruption and irreparable damage leading to cell death.120
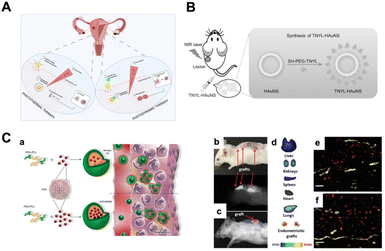 |
| Fig. 3 Phototherapy in endometriosis. (A) Photothermal (PPT) and photodynamic (PDT) therapy. Briefly, when NPs are internalized and exposed to laser, in PTT, the increase in temperature leads to cell death, while in PDT, the PS favors the production of reactive oxygen species (ROS) that impact cell metabolism. Created with BioRender.com. (B) Set-up of PTT mediated by TNYL-HAuNS nanovector applied in a mouse model. Reprinted with permission from John Wiley and Sons (License number 5582490179408)128 (C) (a) Schematic illustration of “always on” and “activable” SiNc-NPs. The difference is represented by the greater numbers of SiNc NPs in “activable” NPs in comparison to “always on” NPs in the hydrophobic core. This results in a generation of fluorescence only after internalization into endometriotic cells. (b, c, d, e and f) Illustration of endometriotic grafts 24 h after injection of “activable” SiNc-NP: (b and c) images of mouse bearing endometriotic grafts, and corresponding NIR fluorescence images; (d) NIR fluorescence images of resected tissue; (e and f) fluorescence microscopy images of endometriotic graft section. Red: NIR fluorescence generated by SiNc-NP. Yellow: blood vessels (anti-CD31 antibody). Scale bars are 50 μm.129 Reprinted with permission from Copyright John Wiley and Sons (License number 5582490434492). | |
Considering the pathophysiological similarities between cancer and endometriosis, NP-based PTT could represent the ideal therapeutic strategy for endometriosis. However, the application of PTT combined with NPs in endometriosis is not as well explored as in cancer. To date, there are few articles in the literature dealing with this topic in endometriosis. In 2017, Guo et al. developed targeted EphB4 hollow gold nanospheres (HAuNS) for endometriosis therapy based on photothermia (Fig. 3B).128 One of the mechanisms involved in both endometriosis and cancer is neovascularization, which is associated with overexpression of Eph receptors. To achieve the targeting effect, HAuNS was conjugated with TNYL peptides capable of binding (Ephrin type-B receptor 4) EphB4 receptors. In in vivo experiments under NIR light irradiation, TNYL-HAuNS significantly inhibited lesion growth by photothermal ablation without significant damage to normal tissue. However, congestive patients cannot be treated with this method, as EphB4 is also highly expressed in the uterus.128 Moreover, Moses et al. designed a nanoplatform based on the dye silicon naphthalocyanine (SiNc) and polymeric NPs, which can be used for real-time near-infrared fluorescence imaging and photothermal therapy (Fig. 3C).129 SiNc NPs are ideal candidate for PTT because they have shown strong absorption of NIR light and are able to generate both high NIR fluorescence and heat upon exposure to NIR light of relatively low power (>0.3 W cm−2).130–132 As these NPs have poor solubility and tend to aggregate in aqueous environments, the researchers applied a solvent evaporation approach to encapsulate SiNc molecules within the hydrophobic core of [methoxy poly(ethylene glycol)-b-poly(ε-caprolactone)] (PEG-PCL) polymer NPs. The aim of this work was to achieve high contrast during fluorescence imaging of endometriotic lesions: “always-on” fluorescent SiNc-NPs were remodeled to produce “activable” SiNc-NPs by increasing the amount of SiNc molecules from 0.5 to 6% SiNc per 1 mg PEG-PCL. In this way, the NPs were only activated once inside the endometriosis tissue. Both the in vitro and in vivo experiments demonstrated the validity of the system compared to SiNc alone.129 Photodynamic therapy (PDT) involves light energy combining with a photosensitizing agent (PS) (Fig. 3A). PDT uses light and oxygen to stimulate the production of reactive oxygen species (ROS) by PSs, which leads to disorders in cell metabolism.132 The photodynamic effect can be used both therapeutically and as a fluorescence-guided surgical procedure133 (see above).63 PDT combined with NPs is widely used in tumors,134 but also as an antibacterial,135 and can find applications in endometriosis treatment. A few years ago, a PS alone derived from 5-aminolevulinic called protoporphyrin IX was used in endometriosis.136 The absence of a vehicle to deliver the PS led to a non-specified accumulation in nearby cells. In this case, if the authors had used a nanosystem delivery approach, there probably would have been a more targeted effect.3
The studies described above confirmed that NPs-based PTT can be useful in endometriosis and suggest that other NPs previously used in the field of oncology may be repurposed for PTT treatment of endometriosis. However, the technique has limitations due to its inadequate tissue penetration and the intensity of light required to activate the photosensitizers that are currently available.
3.4 Selecting laser parameters and the delivery approach
As a state-of-the-art approach, several light sources can be used for PDT and PTT studies.137 The main parameters affecting the selection of the ideal light source are: emission wavelength, NPs absorption bandwidth, the required optical power, and the position of the surface to be irradiated.138,139
The correct emission wavelength must be chosen so as to maximize the interaction with the NPs used for the treatment, generally trying to match the NPs absorption peak.132,140 Nevertheless, it is important to bear in mind that the light penetration in biological tissue is very low in the visible range (especially on the green/blue side of the visible spectrum), while it is significantly larger in the 800–1200 nm region (Fig. 4A).141,142 Optical absorption and scattering are the two physical effects that limit light penetration at the different wavelengths, and they are both strongly dependent on the tissue composition; therefore, the 800–1200 nm range must only be considered to be a general reference. In this wavelength range, the absorption of water is much higher than that observed for blue light, but the main limitations are generally produced by blood (that strongly absorbs blue or green light) and by scattering. Several laser sources are usually available at this wavelength range, ranging from semiconductor lasers at 808 or 980 nm to solid-state lasers at 1064 nm (Nd:YAG laser) or to fiber lasers emitting around 1070 nm (Yb-doped fiber laser).143–145 Another key aspect to be considered is the absorption bandwidth of the NPs to be used.146 While working with a narrowband laser source (with an emission band narrower than 0.1 nm), it may be useful to carry out specific studies and to fully address the impact of wavelength selection. In practical applications where the NPs exhibit large absorption peaks (tens of nm), it may be convenient to use LED light sources instead of lasers. While LED sources have a larger emission band, and their light is not coherent and cannot be as tightly focused as laser light, they may offer significant advantages in terms of reduced cost, higher efficiency in the electric-to-optical power conversion, and greater uniformity.
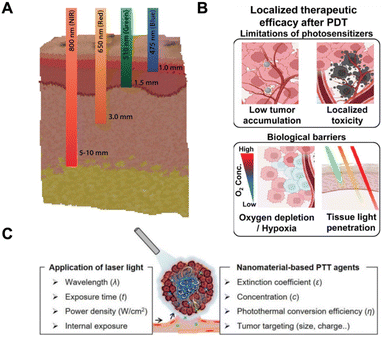 |
| Fig. 4 Laser parameters for PTT and PDT applications: (A) schematic illustration of different light penetration in biological tissue depending on the laser emission wavelength; adapted with the permission of ref. 142. (B) Photodynamic nanomedicines enable highly localized PDT and can better overcome barriers.151 Reprinted with permission from Elsevier (license number: 5582490789244) (C) schematic laser parameters to be used in PTT application. Adapted with the permission of ref. 152. | |
The required optical power is a fundamental aspect of their use and a key element for the light source.147–149 A preliminary evaluation of the required power can be obtained simply by multiplying the required light intensity (in mW cm−2) and the area of the surface to be treated (Fig. 4B and C). But it is important to consider that, in general, only a fraction of the emitted power can be confined with high homogeneity on the target surface, and thus it is useful to multiply the obtained power for a factor of between 2 and 5 to estimate the optical power required at light source output.147–149 Another aspect that must be considered is the location of the surface to be irradiated, as this impacts on the power parameter. In fact, in the case of both PDT and PTT, the presence of targeted illumination produces a localized change within the tissue (i.e., production of ROS/temperature increase) that will necessarily spread to the surrounding areas according to specific mechanisms of molecule diffusion/heat transfer.
Therefore, the maximum increase in ROS-concentration/temperature also critically depends on the environment around the treated area and on its level of “isolation” from surrounding tissues. As an example, considering the in vivo experiments carried out on subcutaneous lesions, it is to be expected that a higher intensity (roughly by a factor of 2 or 3) will be required to produce the same effects in deep tissues.150,151 Finally, the position and the extension of the area to be treated can require specific tools to correctly guide light to the target. In the case of externally visible lesions, free-space light propagation (at least in the final section of the path) is probably the simplest alternative, while appropriate arrangements of optical fibers and catheters (with balloons if needed to extend the surface to be treated) could be the ideal choice when deep tissue treatments are necessary. Considering that the light-treatment of endometriotic lesions would require surgery (or laparoscopy), an interesting possibility is that offered by the development of bioresorbable optical fibers and implants, which could bring light to the tissues even for a few days following surgery, without any need for the implant to be removed.
4. Concluding remarks
Although nanomedicine for endometriosis is still in its infancy, it offers great hope to physicians and patients of a more effective management of the challenges of this disease. At the same time, it is of interest to researchers who are aiming to develop new diagnostic and therapeutic approaches to tackle other reproductive system diseases. Nanomedicine is one of the fastest-growing fields in translational medicine, and nanotechnological innovations may have implications not only in endometriosis research, but also in the study of other reproductive diseases,85e.g., uterine fibroids, ovarian cancer, or microbial infections.135 Nanomedicine could also be useful in the development of new treatment procedures, and in the identification of biological markers that could be applied in clinical practice to stratify the risk of disease recurrence or persistence, with a significant impact on disease management and decision-making approaches for personalized treatment and patient follow-up.153,154 Due to similarities between cancer and endometriosis, some fundamental principles of cancer nanomedicine can be adapted to develop novel NPs-based strategies.3 In general, data available in the literature demonstrate that these nanosystems can be optimized and functionalized in order to be applied in endometriosis. Summarizing the nano-approaches discussed in this review (Table 1), mainly inorganic NPs were applied in the diagnostic field, whereas organic NPs in the therapeutic area. Several studies functionalized nanomaterials to better achieve the target, but in same works the NPs alone were used to exploit their intrinsic characteristics. Many of the proposed literature evaluate the effectiveness of nanocarriers in animal models, such as rats and mice, and a few portion (10%) on 2D in vitro cell models.
However, in order to further facilitate their clinical application, there are still several issues and challenges that need to be overcome. Firstly, some of the trials involving nanomaterials are still at an early stage,80 and most of these materials are not yet commercially available.63,89 Secondly, a few NPs show potential toxicity, according to their size or shape and concentration.88,89,91 Thirdly, some studies only described the absence of adverse effects on endometriotic tissue,88 but nothing is known about the biodistribution of NPs in other organs. Finally, results from studies on endometriosis using rat or mouse models may not be transferable to humans. Based on the 3R principles (refine, replace, and reduce), there are ethical and scientific reasons to support alternative non-animal-based models. In fact, animal models cannot always reproduce all the features of some diseases, and a non-animal-based model could better reproduce the human microenvironment.155,156 A challenging 3D in vitro model has been proposed by Simon Garcia56 and others.157 A 3D model recreates the highly inflammatory microenvironment of endometriosis better than monolayer models since it includes different cell types and stimuli, allowing us to explore the cell complex while helping to reduce the number of animals used. In summary, more studies are needed to clarify the potential role of nanotechnology in diagnostic, therapeutic, and theranostic applications in endometriosis.
Conflicts of interest
The authors declare no conflict of interests.
Acknowledgements
LV, BG and SF would like to acknowledge the grant from the Italian Ministry of Health for the project titled “AntiCD44-gold nanoparticles for endometriosis photothermal therapy” (code ENDO-2021-12371975; BANDO RICERCA ENDOMETRIOSI). The authors acknowledge the grant of the Italian Ministry of University and Research (MUR) to the Department of Molecular Medicine of the University of Pavia under the initiative “Dipartimenti di Eccellenza (2023-2027)”. The authors would also thank Anne Freckleton (UNIPV) for the English revision.
References
- G. R. Rudramurthy and M. K. Swamy, J. Biol. Inorg. Chem., 2018, 23, 1185–1204 CrossRef CAS PubMed.
- A. Haleem, M. Javaid, R. P. Singh, S. Rab and R. Suman, Glob. Health J., 2023, 7, 70–77 CrossRef.
- A. S. Moses, A. A. Demessie, O. Taratula, T. Korzun, O. D. Slayden and O. Taratula, Small, 2021, 17, 2004975 CrossRef CAS PubMed.
- J. K. Patra, G. Das, L. F. Fraceto, E. V. R. Campos, M. D. P. Rodriguez-Torres, L. S. Acosta-Torres, L. A. Diaz-Torres, R. Grillo, M. K. Swamy, S. Sharma, S. Habtemariam and H.-S. Shin, J. Nanobiotechnol., 2018, 16, 71 CrossRef PubMed.
- A. P. Singh, A. Biswas, A. Shukla and P. Maiti, Signal Transduction Targeted Ther., 2019, 4, 33 CrossRef PubMed.
- A. C. Marques, P. J. Costa, S. Velho and M. H. Amaral, J. Controlled Release, 2020, 320, 180–200 CrossRef CAS PubMed.
- V. R. Yadav, S. Suresh, K. Devi and S. Yadav, J. Pharm. Pharmacol., 2010, 61, 311–321 CrossRef PubMed.
- S. L. Taheri, M. Rezazadeh, F. Hassanzadeh, V. Akbari, A. Dehghani, A. Talebi and S. A. Mostafavi, Int. J. Biol. Macromol., 2022, 220, 1605–1618 CrossRef CAS PubMed.
- L. Qiao, H. Yang, S. Gao, L. Li, X. Fu and Q. Wei, J. Mater. Chem. B, 2022, 10, 1908–1922 RSC.
- T. Kim, J. E. Lemaster, F. Chen, J. Li and J. V. Jokerst, ACS Nano, 2017, 11, 9022–9032 CrossRef CAS PubMed.
- Q. Sun, Y. Lei, H. Zhang, X. Ding, M. Yang, T. Zhang, J. Chen, Z. Huang, L. Wang, J. Lan, Q. Huang and Q. Chen, Colloids Surf., B, 2022, 220, 112893 CrossRef CAS PubMed.
- D. V. Talapin and E. V. Shevchenko, Chem. Rev., 2016, 116, 10343–10345 CrossRef CAS PubMed.
- I. Rezić, Polymers, 2022, 14, 4961 CrossRef PubMed.
- Y. B. Zheng, B. Kiraly, P. S. Weiss and T. J. Huang, Nanomedicine, 2012, 7, 751–770 CrossRef CAS PubMed.
- S. Vimalraj, T. Ashokkumar and S. Saravanan, Biomed. Pharmacother., 2018, 105, 440–448 CrossRef CAS PubMed.
- S. Mosleh-Shirazi, M. Abbasi, Mr Moaddeli, A. Vaez, M. Shafiee, S. R. Kasaee, A. M. Amani and S. Hatam, Nanotheranostics, 2022, 6, 400–423 CrossRef PubMed.
- W. Wu, Y. Pu and J. Shi, J. Nanobiotechnol., 2022, 20, 4 CrossRef CAS PubMed.
- D. Hanahan, Cancer Discovery, 2022, 12, 31–46 CrossRef CAS PubMed.
- C. Fornaguera and M. García-Celma, J. Pers. Med., 2017, 7, 12 CrossRef PubMed.
- H. S. Taylor, A. M. Kotlyar and V. A. Flores, Lancet, 2021, 397, 839–852 CrossRef CAS PubMed.
- M. Kvaskoff, Y. Mahamat-Saleh, L. V. Farland, N. Shigesi, K. L. Terry, H. R. Harris, H. Roman, C. M. Becker, S. As-Sanie, K. T. Zondervan, A. W. Horne and S. A. Missmer, Hum. Reprod. Update, 2021, 27, 393–420 CrossRef CAS PubMed.
- P. T. K. Saunders and A. W. Horne, Cell, 2021, 184, 2807–2824 CrossRef CAS PubMed.
- J. Yuxue, S. Ran, F. Minghui and S. Minjia, Front. Bioeng. Biotechnol., 2023, 11, 1184155 CrossRef PubMed.
- R. O. Burney and L. C. Giudice, Fertil. Steril., 2012, 98, 511–519 CrossRef CAS PubMed.
- S. Ferrero, E. Arena, A. Morando and V. Remorgida, Int. J. Gynecol. Obstet., 2010, 110, 203–207 CrossRef PubMed.
- M. Morotti, V. Remorgida, P. L. Venturini and S. Ferrero, Arch. Gynecol. Obstet., 2012, 286, 1571–1575 CrossRef PubMed.
- V. L. La Rosa, P. De Franciscis, F. Barra, A. Schiattarella, A. Tropea, J. Tesarik, M. Shah, I. Kahramanoglu, T. Marques Cerentini, M. Ponta and S. Ferrero, Minerva Med., 2020, 111(1), 79–89 Search PubMed.
- V. L. La Rosa, P. De Franciscis, F. Barra, A. Schiattarella, P. Török, M. Shah, E. Karaman, T. Marques Cerentini, F. Di Guardo, G. Gullo, M. Ponta and S. Ferrero, Minerva Med., 2020, 111, 79–89 Search PubMed.
- P. Vercellini, P. Viganò, E. Somigliana and L. Fedele, Nat. Rev. Endocrinol., 2014, 10, 261–275 CrossRef CAS PubMed.
- Z. Liu, Front. Biosci., 2016, 21, 941–948 CrossRef PubMed.
- F. Barra, A. Romano, G. Grandi, F. Facchinetti and S. Ferrero, Expert Opin. Invest. Drugs, 2019, 28, 501–504 CrossRef CAS PubMed.
- L. Kiesel and M. Sourouni, Climacteric, 2019, 22, 296–302 CrossRef CAS PubMed.
- P. R. Koninckx, R. Fernandes, A. Ussia, L. Schindler, A. Wattiez, S. Al-Suwaidi, B. Amro, B. Al-Maamari, Z. Hakim and M. Tahlak, Front. Endocrinol., 2021, 12, 745548 CrossRef PubMed.
- C. B. Sieberg, C. E. Lunde and D. Borsook, Neurosci. Biobehav. Rev., 2020, 108, 866–876 CrossRef PubMed.
-
J. M. Duffy, K. Arambage, F. J. Correa, D. Olive, C. Farquhar, R. Garry, D. H. Barlow and T. Z. Jacobson, in Cochrane Database of Systematic Reviews, ed. The Cochrane Collaboration, John Wiley & Sons, Ltd, Chichester, UK, 2014, p. CD011031. pub2 Search PubMed.
- K. Shakiba, J. F. Bena, K. M. McGill, J. Minger and T. Falcone, Obstet. Gynecol., 2008, 111, 1285–1292 CrossRef PubMed.
- U. L. R. Maggiori, J. K. Gupta and S. Ferrero, Eur. J. Obstet. Gynecol. Reprod. Biol., 2017, 209, 81–85 CrossRef PubMed.
- H. J. Lee, H. J. Lee, J. M. Lee, Y. Chang and S. T. Woo, Magn. Reson. Imaging, 2012, 30, 860–868 CrossRef PubMed.
- J. M. Johnson, A. S. R. Mohamed, Y. Ding, J. Wang, S. Y. Lai, C. D. Fuller, R. Shah, R. T. Butler and R. S. Weber, Clin. Case Rep., 2021, 9, 123–127 CrossRef PubMed.
- M. Gkagkanasiou, A. Ploussi, M. Gazouli and E. P. Efstathopoulos, J. Neuroimaging, 2016, 26, 161–168 CrossRef PubMed.
- M. Czarniecki, F. Pesapane, B. J. Wood, P. L. Choyke and B. Turkbey, Transl. Androl. Urol., 2018, 7, S453–S461 CrossRef PubMed.
- J. F. Schenck, Med. Phys., 1996, 23, 815–850 CrossRef CAS PubMed.
- T. Y. Tang, A. J. Patterson, S. R. Miller, M. J. Graves, S. P. S. Howarth, J. M. U-King-Im, Z. Y. Li, U. Sadat, V. E. Young, S. R. Walsh, J. R. Boyle, M. E. Gaunt and J. H. Gillard, Neuroradiology, 2009, 51, 457–465 CrossRef CAS PubMed.
- R. C. H. Stijns, B. W. J. Philips, I. D. Nagtegaal, F. Polat, J. H. W. De Wilt, C. A. P. Wauters, P. Zamecnik, J. J. Fütterer and T. W. J. Scheenen, Eur. J. Radiol., 2021, 138, 109636 CrossRef PubMed.
- M. Di Marco, C. Sadun, M. Port, I. Guilbert, P. Couvreur and C. Dubernet, Int. J. Nanomed., 2007, 2, 609–622 CAS.
- J. Saleh, Radiol. Imaging Cancer, 2022, 4, e229020 CrossRef PubMed.
- X. Ma, S. Wang, L. Hu, S. Feng, Z. Wu, S. Liu, S. Duan, Z. Chen, C. Zhou and X. Zhao, Contrast Media Mol. Imaging, 2019, 2019, 1–9 Search PubMed.
- R. Weissleder, P. F. Hahn, D. D. Stark, G. Elizondo, S. Saini, L. E. Todd, J. Wittenberg and J. T. Ferrucci, Radiology, 1988, 169, 399–403 CrossRef CAS PubMed.
- D. D. Stark, R. Weissleder, G. Elizondo, P. F. Hahn, S. Saini, L. E. Todd, J. Wittenberg and J. T. Ferrucci, Radiology, 1988, 168, 297–301 CrossRef CAS PubMed.
- G. Bierry, F. Jehl, N. Boehm, P. Robert, G. Prévost, J.-L. Dietemann, H. Desal and S. Kremer, Radiology, 2008, 248, 114–123 CrossRef PubMed.
- H. Zhang, J. Li, W. Sun, Y. Hu, G. Zhang, M. Shen and X. Shi, PLoS One, 2014, 9, e94718 CrossRef PubMed.
- E. Kozenkova, K. Levada, M. V. Efremova, A. Omelyanchik, Y. A. Nalench, A. S. Garanina, S. Pshenichnikov, D. G. Zhukov, O. Lunov, M. Lunova, I. Kozenkov, C. Innocenti, M. Albino, M. A. Abakumov, C. Sangregorio and V. Rodionova, Nanomaterials, 2020, 10, 1646 CrossRef CAS PubMed.
- H. Li, S. Yang, D. Hui and R. Hong, Nanotechnol. Rev., 2020, 9, 1265–1283 CrossRef CAS.
- Z. Lu and Y. Gao, Ann. Med., 2021, 53, 1377–1389 CrossRef CAS PubMed.
- J. S. Griffith, Y.-G. Liu, R. R. Tekmal, P. A. Binkley, A. E. C. Holden and R. S. Schenken, Fertil. Steril., 2010, 93, 1745–1749 CrossRef CAS PubMed.
- L. Simón-Gracia, K. Kiisholts, V. Petrikaitė, A. Tobi, M. Saare, P. Lingasamy, M. Peters, A. Salumets and T. Teesalu, Nanomaterials, 2021, 11, 3257 CrossRef PubMed.
- X.-F. Zhang, Z.-G. Liu, W. Shen and S. Gurunathan, Int. J. Mater. Sci., 2016, 17, 1534 Search PubMed.
- A.-M. A. Willmore, L. Simón-Gracia, K. Toome, P. Paiste, V. R. Kotamraju, T. Mölder, K. N. Sugahara, E. Ruoslahti, G. B. Braun and T. Teesalu, Nanoscale, 2016, 8, 9096–9101 RSC.
- H. D. Beyene, A. A. Werkneh, H. K. Bezabh and T. G. Ambaye, Sustainable Mater. Technol., 2017, 13, 18–23 CrossRef CAS.
- A.-C. Burduşel, O. Gherasim, A. M. Grumezescu, L. Mogoantă, A. Ficai and E. Andronescu, Nanomaterials, 2018, 8, 681 CrossRef PubMed.
-
M. Dhayalan, P. Karikalan, M. R. S. Umar and N. Srinivasan, in Silver Micro-Nanoparticles - Properties, Synthesis, Characterization, and Applications, ed. S. Kumar, P. Kumar and C. Shakher Pathak, IntechOpen, 2021 Search PubMed.
- P. C. Lee and D. Meisel, J. Phys. Chem., 1982, 86, 3391–3395 CrossRef CAS.
- R. M. Marquardt, M. Nafiujjaman, T. H. Kim, S.-J. Chung, K. Hadrick, T. Kim and J.-W. Jeong, Reprod. Sci., 2022, 29, 2947–2959 CrossRef CAS PubMed.
- W. Li and X. Chen, Nanomedicine, 2015, 10, 299–320 CrossRef CAS PubMed.
- S. J. Amina and B. Guop, Int. J. Nanosci., 2020, 15, 9823–9857 CAS.
- P. Zhao, N. Li and D. Astruc, Coord. Chem. Rev., 2013, 257, 638–665 CrossRef CAS.
- J. Zhang, L. Mou and X. Jiang, Chem. Sci., 2020, 11, 923–936 RSC.
- S. A. Bansal, V. Kumar, J. Karimi, A. P. Singh and S. Kumar, Nanoscale Adv., 2020, 2, 3764–3787 RSC.
- H. Moustaoui, J. Saber, I. Djeddi, Q. Liu, A. T. Diallo, J. Spadavecchia, M. L. De La Chapelle and N. Djaker, J. Phys. Chem. C, 2019, 123, 17548–17554 CrossRef CAS.
- W. Yang, H. Liang, S. Ma, D. Wang and J. Huang, Sustainable Mater. Technol., 2019, 22, e00109 CrossRef CAS.
- Y. Chen and X. Feng, Int. J. Pharm., 2022, 625, 122122 CrossRef CAS PubMed.
- P. Ghosh, G. Han, M. De, C. Kim and V. Rotello, Adv. Drug Delivery Rev., 2008, 60, 1307–1315 CrossRef CAS PubMed.
- J. V. Jokerst, M. Thangaraj, P. J. Kempen, R. Sinclair and S. S. Gambhir, ACS Nano, 2012, 6, 5920–5930 CrossRef CAS PubMed.
- S. Zackrisson, S. M. W. Y. Van De Ven and S. S. Gambhir, Cancer Res., 2014, 74, 979–1004 CrossRef CAS PubMed.
- L. V. Wang and S. Hu, Science, 2012, 335, 1458–1462 CrossRef CAS PubMed.
- L. V. Wang, Nat. Photonics, 2009, 3, 503–509 CrossRef CAS PubMed.
- T. Kalyani, A. Sangili, A. Nanda, S. Prakash, A. Kaushik and S. K. Jana, Bioelectrochemistry, 2021, 139, 107740 CrossRef CAS PubMed.
- Y. Ding, M. Zhang, J. Lang, J. Leng, Q. Ren, J. Yang and C. Li, J. Biophotonics, 2015, 8, 94–101 CrossRef PubMed.
- N. Ma, T. Zhang, T. Yan, X. Kuang, H. Wang, D. Wu and Q. Wei, Biosens. Bioelectron., 2019, 143, 111608 CrossRef CAS PubMed.
- A. Lertkhachonsuk, S. Buranawongtrakoon, N. Lekskul, N. Rermluk, W. Wee–Stekly and C. Charakorn, J. Obstet. Gynaecol. Res., 2020, 46, 2287–2291 CrossRef CAS PubMed.
- A. Sangili, T. Kalyani, S.-M. Chen, A. Nanda and S. K. Jana, ACS Appl. Bio Mater., 2020, 3, 7620–7630 CrossRef CAS PubMed.
- M. Zhang, S. Cheng, Y. Jin, Y. Zhao and Y. Wang, Biochim. Biophys. Acta, Rev. Cancer, 2021, 1875, 188503 CrossRef CAS PubMed.
- T. Kalyani, A. Sangili, H. Kotal, A. Kaushik, K. Chaudhury and S. K. Jana, Biosens. Bioelectron.: X, 2023, 14, 100353 CAS.
- S. Mary, M. J. Kulkarni, D. Malakar, S. R. Joshi, S. S. Mehendale and A. P. Giri, J. Proteome Res., 2017, 16, 1050–1060 CrossRef CAS PubMed.
- R. Shandilya, N. Pathak, N. K. Lohiya, R. S. Sharma and P. K. Mishra, Clin. Exp. Reprod. Med., 2020, 47, 245–262 CrossRef PubMed.
- A. S. Melo, J. C. Rosa-e-Silva, A. C. J. D. S. Rosa-e-Silva, O. B. Poli-Neto, R. A. Ferriani and C. S. Vieira, Fertil. Steril., 2010, 93, 2433–2436 CrossRef CAS PubMed.
- D. Healy, Hum. Reprod. Update, 1998, 4, 736–740 CrossRef CAS PubMed.
- K. Chaudhury, K. N. Babu, A. K. Singh, S. Das, A. Kumar and S. Seal, Nanomedicine, 2013, 9, 439–448 CrossRef CAS PubMed.
- M. C. Operti, A. Bernhardt, S. Grimm, A. Engel, C. G. Figdor and O. Tagit, Int. J. Pharm., 2021, 605, 120807 CrossRef CAS PubMed.
- T. Padežnik, A. Oleksy, A. Cokan, I. Takač and M. Sobočan, Int. J. Mater. Sci., 2023, 24, 5463 Search PubMed.
- A. K. Singh, B. Chakravarty and K. Chaudhury, J. Biomed. Nanotechnol., 2015, 11, 789–804 CrossRef CAS PubMed.
- V. R. D. A. Borges, J. H. Da Silva, S. S. Barbosa, L. E. Nasciutti, L. M. Cabral and V. P. De Sousa, Mater. Sci. Eng., C, 2016, 64, 310–317 CrossRef CAS PubMed.
- J. H. Da Silva, V. R. D. A. Borges, L. D. C. B. Pereira, R. Ferrari, R. M. De Mattos, E. G. D. O. Barros, C. Y. Palmero, P. D. Fernandes, P. R. De Carvalho, V. P. De Sousa, L. M. Cabral and L. E. Nasciutti, J. Pharm. Pharmacol., 2015, 67, 1744–1755 CrossRef PubMed.
- Y.-T. Tung, M.-T. Chua, S.-Y. Wang and S.-T. Chang, Bioresour. Technol., 2008, 99, 3908–3913 CrossRef CAS PubMed.
- M. A. Abbas, M. O. Taha, M. A. Zihlif and A. M. Disi, Eur. J. Pharmacol., 2013, 702, 12–19 CrossRef CAS PubMed.
- V. R. D. A. Borges, M. R. Tavares, J. H. Da Silva, L. Tajber, F. Boylan, A. F. Ribeiro, L. E. Nasciutti, L. M. Cabral and V. P. De Sousa, Pharm. Dev. Technol., 2018, 23, 343–350 CrossRef CAS PubMed.
- N. Ohkura, Y. Kitagawa and S. Sakaguchi, Immunity, 2013, 38, 414–423 CrossRef CAS PubMed.
- M. Oppermann, Cell. Signalling, 2004, 16, 1201–1210 CrossRef CAS PubMed.
- Q. Liu, P. Ma, L. Liu, G. Ma, J. Ma, X. Liu, Y. Liu, W. Lin and Y. Zhu, Eur. J. Pharm. Sci., 2017, 96, 542–550 CrossRef CAS PubMed.
- Y. Y. Zhang and P. Li, Zhonghua Fuchanke Zazhi, 2019, 54, 680–686 CAS.
- S. Boroumand, S. Hosseini, Z. Pashandi, R. Faridi-Majidi and M. Salehi, J. Mater. Sci.: Mater. Med., 2020, 31, 8 CrossRef CAS PubMed.
- S. M. Dizaj, M. Barzegar-Jalali, M. H. Zarrintan, K. Adibkia and F. Lotfipour, Expert Opin. Drug Delivery, 2015, 12, 1649–1660 CrossRef PubMed.
- P. Zhao, Y. Tian, J. You, X. Hu and Y. Liu, Bioengineering, 2022, 9, 691 CrossRef CAS PubMed.
- A. Bedin, R. C. Maranhão, E. R. Tavares, P. O. Carvalho, E. C. Baracat and S. Podgaec, Clinics, 2019, 74, e989 CrossRef PubMed.
- R. He, X. Liu, J. Zhang, Z. Wang, W. Wang, L. Fu, Y. Fan, S. Sun, Y. Cao, L. Zhan and L. Shui, Front. Pharmacol., 2020, 11, 1281 CrossRef CAS PubMed.
- M. Zhao, M. Zhang, Q. Yu, W. Fei, T. Li, L. Zhu, Y. Yao, C. Zheng and X. Zhang, Front. Bioeng. Biotechnol., 2022, 10, 918368 CrossRef PubMed.
- Y. Park, A. A. Demessie, A. Luo, O. R. Taratula, A. S. Moses, P. Do, L. Campos, Y. Jahangiri, C. R. Wyatt, H. A. Albarqi, K. Farsad, O. D. Slayden and O. Taratula, Small, 2022, 18, 2107808 CrossRef CAS PubMed.
- S. Zhu, J. Zhang, N. Xue, X. Zhu, F. Li, Q. Dai, X. Qing, D. Chen, X. Liu, Z. Wei and Y. Cao, J. Nanobiotechnol., 2023, 21, 81 CrossRef CAS PubMed.
- A. Parodi, J. Miao, S. Soond, M. Rudzińska and A. Zamyatnin, Biomolecules, 2019, 9, 218 CrossRef PubMed.
- H. Maeda, J. Wu, T. Sawa, Y. Matsumura and K. Hori, J. Controlled Release, 2000, 65, 271–284 CrossRef CAS PubMed.
- P. Lingasamy, K. Põšnograjeva, S. Kopanchuk, A. Tobi, A. Rinken, I. J. General, E. K. Asciutto and T. Teesalu, Pharmaceutics, 2021, 13, 1998 CrossRef CAS PubMed.
- N. Fernandes, C. F. Rodrigues, A. F. Moreira and I. J. Correia, Biomater. Sci., 2020, 8, 2990–3020 RSC.
- H. S. Jung, P. Verwilst, A. Sharma, J. Shin, J. L. Sessler and J. S. Kim, Chem. Soc. Rev., 2018, 47, 2280–2297 RSC.
- C. Ling, X. Wang and Y. Shen, Int. J. Nanosci., 2021, 16, 493–513 Search PubMed.
- X. Dai, X. Li, Y. Liu and F. Yan, Mater. Des., 2022, 217, 110656 CrossRef CAS.
- Z. Yang, Z. Sun, Y. Ren, X. Chen, W. Zhang, X. Zhu, Z. Mao, J. Shen and S. Nie, Mol. Med. Rep., 2019, 20, 5–15 CAS.
- X. Cui, Q. Ruan, X. Zhuo, X. Xia, J. Hu, R. Fu, Y. Li, J. Wang and H. Xu, Chem. Rev., 2023, 123, 6891–6952 CrossRef CAS PubMed.
- X. Huang and M. A. El-Sayed, J. Adv. Res., 2010, 1, 13–28 CrossRef.
- Z. Abed, J. Beik, S. Laurent, N. Eslahi, T. Khani, E. S. Davani, H. Ghaznavi and A. Shakeri-Zadeh, J. Cancer Res. Clin. Oncol., 2019, 145, 1213–1219 CrossRef CAS PubMed.
- M. M. Movahedi, A. Mehdizadeh, F. Koosha, N. Eslahi, V. P. Mahabadi, H. Ghaznavi and A. Shakeri-Zadeh, Photodiagn. Photodyn. Ther, 2018, 24, 324–331 CrossRef CAS PubMed.
- A. Pal, K. Esumi and T. Pal, J. Colloid Interface Sci., 2005, 288, 396–401 CrossRef CAS PubMed.
- M. Brust, M. Walker, D. Bethell, D. J. Schiffrin and R. Whyman, J. Chem. Soc., Chem. Commun., 1994, 801–802 RSC.
- J. G. Hinman, A. J. Stork, J. A. Varnell, A. A. Gewirth and C. J. Murphy, Faraday Discuss., 2016, 191, 9–33 RSC.
- S. Kundu, L. Peng and H. Liang, Inorg. Chem., 2008, 47, 6344–6352 CrossRef CAS PubMed.
- A. S. C. Gonçalves, C. F. Rodrigues, A. F. Moreira and I. J. Correia, Acta Biomater., 2020, 116, 105–137 CrossRef PubMed.
- Q. Tian, M. Tang, Y. Sun, R. Zou, Z. Chen, M. Zhu, S. Yang, J. Wang, J. Wang and J. Hu, Adv. Mater., 2011, 23, 3542–3547 CrossRef CAS PubMed.
- Z. Jiang, T. Li, H. Cheng, F. Zhang, X. Yang, S. Wang, J. Zhou and Y. Ding, Asian J. Pharm. Sci., 2021, 16, 738–761 CrossRef PubMed.
- X. Guo, W. Li, J. Zhou, W. Hou, X. Wen, H. Zhang, F. Kong, L. Luo, Q. Li, Y. Du and J. You, Small, 2017, 13, 1603270 CrossRef PubMed.
- A. S. Moses, O. R. Taratula, H. Lee, F. Luo, T. Grenz, T. Korzun, A. S. Lorenz, F. Y. Sabei, S. Bracha, A. W. G. Alani, O. D. Slayden and O. Taratula, Small, 2020, 16, 1906936 CrossRef CAS PubMed.
- O. Taratula, C. Schumann, T. Duong, K. L. Taylor and O. Taratula, Nanoscale, 2015, 7, 3888–3902 RSC.
- O. Taratula, B. S. Doddapaneni, C. Schumann, X. Li, S. Bracha, M. Milovancev, A. W. G. Alani and O. Taratula, Chem. Mater., 2015, 27, 6155–6165 CrossRef CAS.
- X. Min, F. Yi, X.-L. Han, M. Li, Q. Gao, X. Liang, Z. Chen, Y. Sun and Y. Liu, Chem. Eng. J., 2022, 432, 134327 CrossRef CAS.
- J. Karges, Angew. Chem., Int. Ed., 2022, 61, e202112236 CrossRef CAS PubMed.
- S. S. Lucky, K. C. Soo and Y. Zhang, Chem. Rev., 2015, 115, 1990–2042 CrossRef CAS PubMed.
-
N. Bloise, P. Minzioni, M. Imbriani and L. Visai, in Photomedicine - Advances in Clinical Practice, ed. Y. Tanaka, InTech, 2017 Search PubMed.
- M. Wołuń-Cholewa, K. Szymanowski, E. Nowak-Markwitz and W. Warchoł, Photodiagn. Photodyn. Ther., 2011, 8, 58–63 CrossRef PubMed.
- M. Overchuk, R. A. Weersink, B. C. Wilson and G. Zheng, ACS Nano, 2023, 17, 7979–8003 CrossRef CAS PubMed.
- S. Liu, X. Pan and H. Liu, Angew. Chem., 2020, 132, 5943–5953 CrossRef.
- S. C. Freitas, J. H. Belo, A. Granja, M. Canhota, A. S. Silva, S. Reis, H. Crespo, J. P. Araújo and C. T. Sousa, Adv. Mater. Interfaces, 2023, 10, 2202214 CrossRef CAS.
- P. Singh, S. Pandit, V. R. S. S. Mokkapati, A. Garg, V. Ravikumar and I. Mijakovic, Int. J. Mater. Sci., 2018, 19, 1979 Search PubMed.
- Z. Zhou, J. Song, L. Nie and X. Chen, Chem. Soc. Rev., 2016, 45, 6597–6626 RSC.
- N. Teraphongphom, C. S. Kong, J. M. Warram and E. L. Rosenthal, Laryngoscope Investig. Otolaryngol., 2017, 2, 447–452 CrossRef PubMed.
- X. Ding, C. H. Liow, M. Zhang, R. Huang, C. Li, H. Shen, M. Liu, Y. Zou, N. Gao, Z. Zhang, Y. Li, Q. Wang, S. Li and J. Jiang, J. Am. Chem. Soc., 2014, 136, 15684–15693 CrossRef CAS PubMed.
- A. Sun, H. Guo, Q. Gan, L. Yang, Q. Liu and L. Xi, Opt. Express, 2020, 28, 9002 CrossRef PubMed.
- Z.-H. Hsieh, C.-H. Fan, Y.-J. Ho, M.-L. Li and C.-K. Yeh, Sci. Rep., 2020, 10, 17406 CrossRef CAS PubMed.
- M. Yorulmaz, S. Nizzero, A. Hoggard, L.-Y. Wang, Y.-Y. Cai, M.-N. Su, W.-S. Chang and S. Link, Nano Lett., 2015, 15, 3041–3047 CrossRef CAS PubMed.
- S. Pascal, S. David, C. Andraud and O. Maury, Chem. Soc. Rev., 2021, 50, 6613–6658 RSC.
- N. Liaros, E. Koudoumas and S. Couris, Appl. Phys. Lett., 2014, 104, 191112 CrossRef.
- M. G. Silly, L. Porrès, O. Mongin, P.-A. Chollet and M. Blanchard-Desce, Chem. Phys. Lett., 2003, 379, 74–80 CrossRef CAS.
- B. Ouyang, F. Liu, S. Ruan, Y. Liu, H. Guo, Z. Cai, X. Yu, Z. Pang and S. Shen, ACS Appl. Mater. Interfaces, 2019, 11, 38555–38567 CrossRef CAS PubMed.
- J. Choi, I.-C. Sun, H. S. Hwang, H. Y. Yoon and K. Kim, Adv. Drug Delivery Rev., 2022, 186, 114344 CrossRef CAS PubMed.
- H. S. Han and K. Y. Choi, Biomedicines, 2021, 9, 305 CrossRef CAS PubMed.
- G. F. Combes, A.-M. Vučković, M. P. Bakulić, R. Antoine, V. Bonačić-Koutecky and K. Trajković, Cancers, 2021, 13, 4206 CrossRef CAS PubMed.
- M. A. Alghamdi, A. N. Fallica, N. Virzì, P. Kesharwani, V. Pittalà and K. Greish, J. Pers. Med., 2022, 12, 673 CrossRef PubMed.
- X. Huang, A. K. Nussler, M. K. Reumann, P. Augat, M. M. Menger, A. Ghallab, J. G. Hengstler, T. Histing and S. Ehnert, Bioengineering, 2022, 9, 337 CrossRef PubMed.
- A. Bassi, ALTEX, 2020, 37, 493–495 CrossRef PubMed.
- J. R. H. Wendel, X. Wang, L. J. Smith and S. M. Hawkins, Biomedicines, 2020, 8, 525 CrossRef CAS PubMed.
|
This journal is © The Royal Society of Chemistry 2023 |