Passive air sampling for semi-volatile organic chemicals
Received
28th April 2020
, Accepted 20th July 2020
First published on 21st August 2020
Abstract
During passive air sampling, the amount of a chemical taken up in a sorbent from the air without the help of a pump is quantified and converted into an air concentration. In an equilibrium sampler, this conversion requires a thermodynamic parameter, the equilibrium sorption coefficient between gas-phase and sorbent. In a kinetic sampler, a time-averaged air concentration is obtained using a sampling rate, which is a kinetic parameter. Design requirements for kinetic and equilibrium sampling conflict with each other. The volatility of semi-volatile organic compounds (SVOCs) varies over five orders of magnitude, which implies that passive air samplers are inevitably kinetic samplers for less volatile SVOCs and equilibrium samplers for more volatile SVOCs. Therefore, most currently used passive sampler designs for SVOCs are a compromise that requires the consideration of both a thermodynamic and a kinetic parameter. Their quantitative interpretation depends on assumptions that are rarely fulfilled, and on input parameters, that are often only known with high uncertainty. Kinetic passive air sampling for SVOCs is also challenging because their typically very low atmospheric concentrations necessitate relatively high sampling rates that can only be achieved without the use of diffusive barriers. This in turn renders sampling rates dependent on wind conditions and therefore highly variable. Despite the overall high uncertainty arising from these challenges, passive air samplers for SVOCs have valuable roles to play in recording (i) spatial concentration variability at scales ranging from a few centimeters to tens of thousands of kilometers, (ii) long-term trends, (iii) air contamination in remote and inaccessible locations and (iv) indoor inhalation exposure. Going forward, thermal desorption of sorbents may lower the detection limits for some SVOCs to an extent that the use of diffusive barriers in the kinetic sampling of SVOCs becomes feasible, which is a prerequisite to decreasing the uncertainty of sampling rates. If the thermally stable sorbent additionally has a high sorptive capacity, it may be possible to design true kinetic samplers for most SVOCs. In the meantime, the passive air sampling community would benefit from being more transparent by rigorously quantifying and explicitly reporting uncertainty.
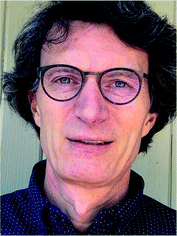 Frank Wania | Frank Wania studied Environmental Science at the University Bayreuth in Germany and received his Doctorate in Chemical Engineering and Applied Chemistry from the University of Toronto in 1995. After two years as a scientist at the Norwegian Institute for Air Research, and three years as an independent researcher, he joined the University of Toronto Scarborough in 1999, where he is currently a professor of environmental chemistry. A co-developer of the XAD-PAS, the FTS and a PAS for gaseous elemental mercury, he has been calibrating, characterising, evaluating and applying passive air sampling techniques for the past two decades. |
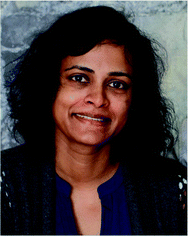 Chubashini Shunthirasingham | Chubashini Shunthirasingham is a chemist in the Air Quality Research Division at Environment and Climate Change Canada. She obtained her BSc in Chemistry and Biology as well as her MSc and PhD in Environmental Chemistry from the University of Toronto. Her PhD work focused on the measurement of pesticides in the physical environment of developing countries using XAD-resin based passive air samplers and on the measurement of Henry's law constants using the inert gas stripping method. Her research interests are atmospheric chemistry, fate and transport of SVOCs and method development and validation for emerging organic pollutants using gas chromatography mass spectrometry. |
Environmental significance
Over the past 25 years, numerous passive air samplers (PASs) for SVOCs have been introduced, characterised, and tested. More recently, the applications of PASs, initially focused on recording the variability in atmospheric contamination on a wide range of spatial scales, has expanded to include measuring indoor inhalation exposure to SVOCs, probing the exchange of SVOCs between the atmosphere and soil and water, mapping the toxicity of airborne contamination, and monitoring interannual trends in SVOC air concentrations. The simplicity of passive air sampling is deceptive and many users of PASs do not fully appreciate their strength and limitations and may have misconceptions as to their applicability and reliability. This comprehensive and critical review assembles and curates the current knowledge on this topic in order to equip anyone to use PAS appropriately and with confidence and to guide further development of PASs in a direction that will overcome their largest shortcomings.
|
A. Introduction
A.1 Definition of passive air sampling and semi-volatile organic compounds
Passive air sampling involves the diffusive uptake of a chemical vapour in a sorbent over time. The amount of vapour being sorbed during a sampler's deployment is determined by both kinetic and thermodynamic factors and can be interpreted in terms of volumetric air concentrations based on a quantitative understanding of these factors. In contrast to active air samplers that rely on a pump to pass a volume of air through or past a sorbent, passive air samplers (PASs) have the advantage of low price, simple operation, and independence of power sources. On the other hand, they typically allow only for coarse temporal resolution. PASs have been developed for a variety of gaseous chemicals, including volatile organic chemicals (VOCs) such as benzene, toluene, ethylbenzene, and xylene, classical air pollutants such as sulfur dioxide, various nitrogen oxides, hydrochloric acid, ozone and ammonia, as well as mercury.1–5
A distinct set of PASs has been developed for semi-volatile organic chemicals (SVOCs). The term SVOCs comprises organic molecules that can occur to a significant extent in both the gas-phase and condensed phases, which corresponds to the vapour pressure range of approx. 10−1 to 10−6 Pa. The group of SVOCs comprises a large number of commercially produced substances, including industrial chemicals, pesticides, and additives to consumer products. Prominent examples are the polychlorinated biphenyls (PCBs), polybrominated diphenyl ethers (PBDEs), and organochlorine pesticides (OCPs). Combustion products such as the polycyclic aromatic hydrocarbons (PAHs) also count among the SVOCs. Many SVOCs are a concern for human and environmental health. The atmosphere often plays an important role in the dispersal of SVOCs and in facilitating organism exposure to SVOCs. PASs for SVOCs thus address a need for information on the concentrations of SVOCs in indoor and outdoor air.
A.2 Motivation for, and scope of, review
Over the past 20 years, there has been explosive growth in the development, characterization, testing and application of passive air sampling techniques of SVOCs. A literature search suggests that more than 40 papers are published in this area every year, garnering ca. 1500 citations annually (Fig. 1).
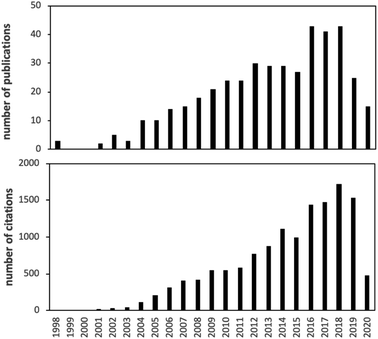 |
| Fig. 1 Number of publications and citations on the topic of passive air sampling for semivolatile organic compounds, based on an analysis using Web of Science using the search string TOPIC: (“passive air sampl*”) and TOPIC: (POPs or SVOCs or PCBs or PAHs or semivolatile or semi-volatile) on April 11, 2020. | |
There have been a number of reviews on the passive sampling of atmospheric SVOCs, but they are either somewhat out-of-date and/or have a wider scope by focusing on passive (air) sampling in general.6–11 In other cases, the scope is limited to only one type of PAS.12,13 Recently, Salim and Górecki14 thoroughly reviewed the theory and modelling of passive sampling, which complements our current effort well, as we do not delve as thoroughly into this aspect in this review.
While the operation of PASs for SVOCs can be deceptively simple, the kinetic and thermodynamic factors controlling the uptake of SVOCs in PASs can be surprisingly complex and are not always fully understood, even by the community using them. Reasons include the diversity of sampler designs, the large and diverse group of SVOCs being sampled, and the wide range of environments in which PASs are finding use. Here we aim to summarise comprehensively the current state-of-knowledge on passive sampling of SVOCs and to provide guidance on the use of PASs for SVOCs to existing and prospective users.
Section B provides an introduction into the basic principles of passive air sampling and in doing so identifies the two central characteristics of a PAS. These are the PAS's uptake capacity at equilibrium, i.e. the maximum amount of a target chemical that the sorbent will take up given a certain concentration in the gas-phase, and the PAS's inherent sampling rate, i.e. the maximum rate at which a target chemical is taken up in the sorbent. Section C then highlights the specific challenges faced when developing a PAS for SVOCs, namely the balancing of conflicting needs (i) for high vs. tightly controlled sampling rates and (ii) of SVOCs of variable volatility. Section D introduces, contrasts and critically assesses the approximately 50 PAS designs for SVOCs that have been presented in the peer-reviewed literature over the past 25 years. Section E discusses the empirical and theoretical means by which the uptake capacity of different PAS sorbents has been determined. Section F presents information on how the kinetics of uptake in PASs is approached experimentally and theoretically. Section G reviews the various ways in which PASs for SVOCs have been applied. Finally, Section H will conclude with an overall assessment of the state-of-the-art in passive air sampling of SVOCs and provide an outlook for future activities in this area. Section I provides a glossary for acronyms, abbreviations and variables used in this review.
Not covered in this review are substances other than SVOCs, namely VOCs and inorganic gases or passive samplers for media other than air. We also do not consider biological matrices, such as plants (moss, needles, tree bark, wood, etc.), that are often used in a similar way as PASs for SVOCs.15 Furthermore, we do not count organic “films” forming on glass surfaces among the passive air samplers in this review.16–19
B. Principles of passive air sampling
B.1 The equation describing diffusive uptake in a PAS
The amount of a target analyte in the passive sampling sorbent, mS in mol, changes over time, t in days, as a chemical is taken up from the atmosphere at a rate proportional to the concentration in the gas-phase, CG in mol m−3, and is lost from the sorbent at a rate proportional to the concentration in the sorbent, CS in mol m−3 (or mol m−2 if it is an adsorbent):20 | 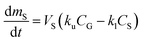 | (1) |
where ku and kl are rate constants for uptake and loss in per day and VS is the volume of the PAS sorbent in m3. If chemical uptake and loss in a PAS is controlled by the air-side resistance, i.e. transport within the sorbent is not rate-limiting, the rate constants for uptake can be derived by applying Fick's first law to the molecular diffusion of the target analyte through a stagnant air boundary layer surrounding the sorbent of thickness Δz in m:20 |  | (2) |
where D is the molecular diffusivity of the target analyte in the gas-phase D in m2 per day and A is the geometric surface area of the PAS sorbent in m2. The rate constant for loss is additionally proportional to the reciprocal of the equilibrium sorption coefficients between the gas-phase and the PAS sorbent KSG in units of m3 gas per m3 absorbent (or m3 gas per m2 adsorbent): |  | (3) |
The amount of analyte in the PAS sorbent, ΔmS in mol, taken up during its deployment time, Δt in days, then becomes:20
|  | (4) |
In
eqn (4), the ratio
CS/
KSG designates the gas-phase concentration in equilibrium with the concentration in the PAS sorbent. The term (
CG −
CS/
KSG) is the concentration difference that provides the driving force for the diffusive transport from bulk air to PAS sorbent. The term
DA/Δ
z designates how fast this diffusive transport can take place and is often called a sampling rate SR in m
3 per day. The ratio
D/Δ
z is sometimes termed a diffusive mass transfer coefficient in units of m per day. A PAS's foremost characteristics are thus described in one kinetic parameter (SR) and one thermodynamic parameter (
KSG) describing the rate of uptake and the maximum uptake capacity of the PAS, respectively.
B.2 The uptake curve: kinetic vs. equilibrium sampling
A plot of the change in the amount of a chemical sorbed to a PAS with increasing length of deployment is called an uptake curve. A generic example of such a curve is shown in Fig. 2. In this curve, the sorbed amount is divided by CG in order to eliminate the variability caused by changing analyte concentrations in the atmosphere. The ratio mS/CG, which has units of m3, is sometimes referred to as the equivalent sampling volume. The uptake curve goes through three distinct phases. The so-called linear uptake phase, when the rate of uptake is kinetically controlled, a transitional curvi-linear uptake phase, and the equilibrium phase when the gas-phase and the PAS sorbent have reached a state of chemical equilibrium and therefore the sorbed amount is thermodynamically controlled. The initial slope of the uptake curve in Fig. 2 corresponds to the sampling rate SR, whereas the maximum amount taken up depends on the uptake capacity, which is the product of the equilibrium sorption coefficient between sorbent and gas-phase KSG and the size of the passive sampling sorbent (VS or AS).
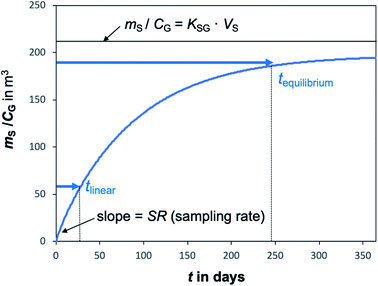 |
| Fig. 2 Generic illustration of the uptake of an SVOC in a passive air sampler, showing the increase in the sorbed amount mS divided by the atmospheric concentration CG as a function of deployment time t. The time period of linear uptake tlinear and the time to equilibrium tequilibrium are important characteristics of a passive sampler. The displayed curve was calculated with PAS-SIM21 for a compound with log KSG of 6 in a PUF-PAS and an SR of 3.2 m3 per day. A tequilibrium of 246 days is based on CS/KSG > 0.90CG and a tlinear of 24 days is based on CS/KSG < 0.25CG. See Section F.5 for more information on the PAS-SIM model. | |
B.2.1 Kinetically controlled uptake.
Initially, the sorbent is clean, i.e. CS is very small. If the sorbent has a high uptake capacity, KSG is large. Then, CS/KSG ≪ CG and (CG − CS/KSG) ∼ CG and eqn (4) simplifies to: | 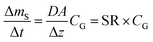 | (5) |
The amount taken up in the sampler ΔmS is then simply the product of the sampling rate SR, the gas-phase concentration CG and the deployment length Δt.
B.2.2 Thermodynamically controlled uptake.
Once equilibrium is established between sorbent and gas-phase, CS/KSG = CG and (CG − CS/KSG) = 0. If that is the case, eqn (4) yields Δm/Δt = 0, i.e. there is no more net uptake of chemical on the PAS sorbent. The amount of chemical in the sorbent then is:if the sorbent is an absorbent, i.e. CS = Δm/VS, orif the sorbent is an adsorbent, i.e. CS = Δm/AS. It is important to stress that AS here refers to the total surface area of the adsorbent and not the geometric surface area of the sorbent or of the container holding the sorbent, i.e. one should not confuse A with AS. The terms KSGVS and KSGAS are expressions of the maximum uptake capacity of a PAS, both with units of m3 of air. The use of an air volume to describe the capacity of a sorbent for a chemical can be understood if we imagine this to be the volume of air that contains the same amount of a chemical at equilibrium as the sorbent within the PAS.
After CS/KSG is no longer negligibly small relative to CG and equilibrium is not yet reached, uptake in the PAS is in the curvi-linear transition region when both kinetic and thermodynamic factors govern the rate of uptake.
Two characteristic times can be used to describe a particular sampler–analyte combination, namely the length of linear uptake (tlinear) and the time to equilibrium (tequilibrium). The precise value of these times depends on the acceptable deviations from linearity and equilibrium. For example, uptake may be considered linear as long as CS/KSG is smaller than 25% of CG and equilibrium may be considered reached when CS/KSG exceeds 90% of CG (Fig. 2 and 3).
There are two types of PASs. A kinetic sampler seeks to remain in the kinetically controlled uptake phase during the entire length of deployment, i.e. tlinear is the maximum deployment length of a kinetic sampler. A kinetic sampler yields a gas-phase concentration averaged over the time of deployment by using a rearrangement of eqn (5):
|  | (7) |
An equilibrium sampler, on the other hand, seeks to reach equilibrium, whereupon the gas-phase concentration at the time of retrieval is obtained using a version of eqn (6):
|  | (8A) |
|  | (8B) |
The tequilibrium is the minimum deployment length of an equilibrium sampler.
Samplers that are neither kinetic nor equilibrium samplers, need to derive CG from eqn (4), which is challenging as CG and KSG generally vary with time (see Section F.3).
B.3 Design considerations for an equilibrium sampler
Because the establishment of equilibrium is essential to the functioning of an equilibrium PAS, optimizing the design of such a PAS involves minimizing the time to equilibrium. There are two means to shorten tequilibrium, namely maximizing the sampling rate SR and minimizing the uptake capacity KSGVS (or KSGAS) (Fig. 3).
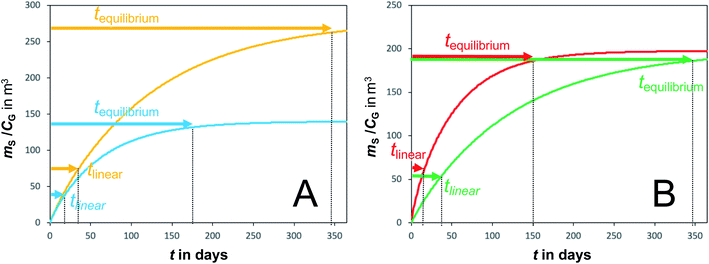 |
| Fig. 3 The length of the linear uptake phase, tlinear, and the time to equilibrium, tequilibrium, depend both on kinetic and thermodynamic factors. Uptake curves for two PASs with equal sampling rate (SR of 3.2 m3 per day), but divergent uptake capacity (log KSG of 5.85 (blue) vs. 6.15 (yellow)) (A) and two PASs with equal uptake capacity (log KSG of 6), yet different uptake kinetics (SR of 2.0 (green) vs. 7.6 m3 per day (red)) (B). tlinear and tequilibrium increase with increasing uptake capacity (17 to 34 days, 175 to 347 days) and decreasing uptake rate (13 to 36 days, 150 to 345 days). The displayed curves were calculated with PAS-SIM21 using the parameterization for the PUF-PAS. See Section F.5 for more information on the PAS-SIM model. | |
A large SR can be achieved by a large A and a small Δz, whereas a small sorption coefficient KSG and a small size of the sorbent VS (or AS) contribute to a small uptake capacity. A small Δz is achieved by not sheltering the sorbent from wind or other air turbulence. If an absorbent is used, it should have a large A and a small VS, i.e. one should seek to maximise its surface area to volume ratio, e.g. by using very thin sheets or films of the absorbent. If an adsorbent is used, it should have a large geometric surface area A, but a small total surface area available for adsorption AS.
There are, however, important limitations to how small the uptake capacity of an equilibrium sampler could be. The first is that the sorbent needs to take up an amount of the target analyte that is sufficient for reliable quantification. If the KSGVS is too small, the amount mS may be below the limit of detection (LOD) of the chosen quantification technique. The second is that the target chemical may be lost too easily by volatilization from a PAS with too small an uptake capacity, because then the chemicals can be lost during retrieval, transport, storage and the processing of the sorbent prior to analysis.
The accuracy of the CG derived from an equilibrium sampler depends on the accuracy of the knowledge of the uptake capacity, in particular the KSG. The sampling rate SR does not need to be known, except that it should be known to be large. The strong dependence of KSG on temperature is one of the challenges of using equilibrium samplers for ambient applications, because the uptake capacity of the PAS is changing substantively with changes in ambient temperature. It is necessary to know the temperature dependence of KSG quantitatively and the temperature of equilibration in order to obtain reliable CG values from an equilibrium PAS.
Temperatures and SVOC air concentrations in the atmosphere are highly variable on a number of time scales. As a consequence, the amount of an SVOC on a sampler that is in equilibrium with the atmosphere is changing rapidly. In other words, chemical equilibrium is a constantly moving target, e.g. being different in a cold night than during a warmer day. To illustrate this effect, Fig. 4 shows simulated uptake curves in a PAS under the hypothetical assumption that air concentrations alternate from high to low every other week. It demonstrates that the more volatile chemicals (low log
KSG), which are suited for equilibrium sampling because of their short tequilibrium, are the ones that experience the most pronounced fluctuations in the amount sequestered in the PAS. For such chemicals, the amount sorbed in the PAS at any moment in time is reflective of the conditions immediately prior to a sampler's retrieval, but also influenced to some extent by the exposure history. This suggests that equilibrium sampling for SVOCs is likely only suitable for situations when both T and CG are reasonably constant, which may apply for certain compounds in indoor environments.
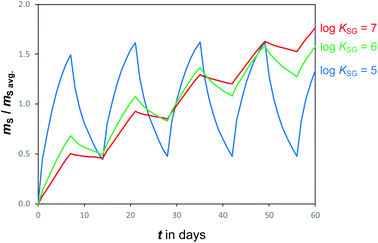 |
| Fig. 4 Illustrative simulations of uptake curves of three hypothetical chemicals in response to fluctuating air concentrations CG. Fluctuations in the amount of a chemical sequestered in a PAS increase with increasing volatility, i.e. decreasing log KSG. | |
B.4 Design considerations for a kinetic sampler
Because the condition CS/KSG ≪ CG is essential to the functioning of a kinetic sampler, optimizing the design of a kinetic PAS involves choosing a sorbent with a very high sorptive capacity, i.e. a large KSG. The actual size of the KSG does not need to be known, as long as it is known to be large enough to assure negligible loss of target analyte from the sorbent during deployment.
The accuracy of the CG derived from a kinetic sampler depends on the accuracy of the knowledge of SR, which is DA/Δz. The molecular diffusion coefficient D of the target analytes in the gas-phase is typically well established or can be easily estimated through relationships between D and molecular size. Also, the dependence of D on atmospheric pressure and temperature can be estimated with good accuracy. The diffusive area A can generally also be derived easily from the geometric dimensions of the sampler.
The most challenging aspect of characterizing the SR of a kinetic sampler is therefore the quantification of the diffusive distance Δz, because Δz depends on the thickness of the stagnant boundary layer surrounding the PAS sorbent and this thickness is (i) difficult to estimate theoretically and (ii) is strongly influenced by atmospheric turbulence, i.e. is susceptible to being dependent on wind speed. As will be discussed next, there are strategies that seek to reduce this dependence of Δz on wind speed, but they tend to lower the SR. This needs to be balanced with the need to sample an amount of chemical during the deployment period that is sufficient for reliable quantification.
B.4.1 Strategies to reduce the wind speed dependence of the sampling rate.
The SR of PASs is dependent on wind speed, which can introduce considerable uncertainty in the derived air concentrations. Mechanistically, we can conceptualise this wind speed dependence as the effect of atmospheric turbulence on the thickness of the stagnant boundary layer surrounding the sampler sorbent (panel A in Fig. 5). Under low wind conditions, this layer is thicker and therefore the diffusive pathlength that the analyte has to travel to reach the sorbent is longer than under high wind conditions. As shown above, in PASs, where the SR is controlled by the air-side resistance, the SR is inversely proportional to the diffusive pathlength Δz. In the design of PASs, two strategies are employed to reduce the wind speed dependence of SR.
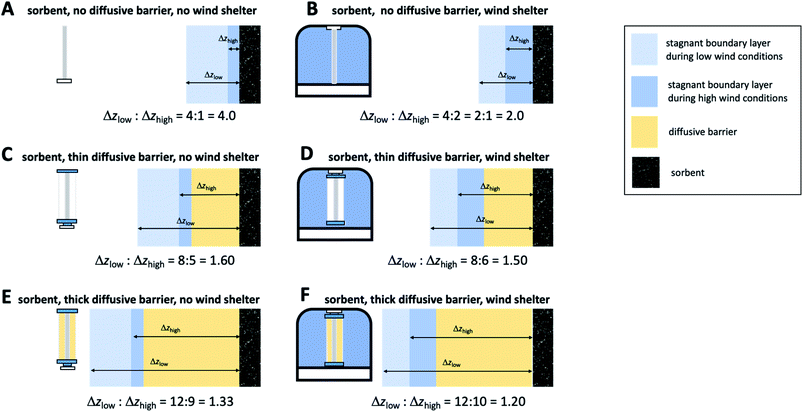 |
| Fig. 5 Illustration of the effect of different sampler configurations on the diffusion distance of an analyte from the ambient atmosphere to the passive sampling sorbent under high (Δzhigh) and low wind conditions (Δzlow). In particular, the effect of a wind shelter (A vs. B, C vs. D, E vs. F) and the effect of a thin or a thick diffusive barrier (A and B vs. C and D vs., E and F) is shown. The ratio Δzlow : Δzhigh indicates the extent to which the SR is influenced by wind speed. | |
The first strategy is wind sheltering that is accomplished by placing the sorbent in some sort of housing that prevents the wind from directly blowing onto the sorbent (panel B in Fig. 5). At low wind conditions this shelter will have little effect on the diffusive pathlength, but at high wind speeds the calming effect of the shelter will result in a thicker stagnant layer, a longer diffusive distance and thus a lower SR. More importantly, the difference in the diffusive pathlengths under different wind conditions will be reduced, or – in other words – the wind speed dependence of the SR will be smaller.
The second strategy involves the addition of a diffusive barrier that forces the analyte to diffuse a longer distance to reach the sorbent (panel C in Fig. 5). The stagnant boundary layer now is no longer adjacent to the sorbent, but next to the diffusive barrier. The thickness of that boundary layer continues to be variable with wind speed, but the relative difference in the total diffusion pathlength, which is the sum of the thickness of the stagnant layer and the effective diffusion distance through the barrier, become much less variable between high and low wind speed conditions. Again, the effect is a reduced wind speed dependence of the SR. Obviously, the thicker the diffusive barrier, the smaller the difference in the relative diffusion pathlengths and therefore the smaller the wind speed dependence of the SR (compare panels C and E in Fig. 5).
It is of course possible to deploy both strategies at the same time, i.e. place a sampler with a diffusive barrier within a wind shelter. However, depending on the thickness of the boundary layer relative to the effective diffusion distance within the barrier, the further reduction in the wind speed dependence afforded by a wind shelter may be marginal and is generally much smaller than the effect of sheltering a sampler without a diffusive barrier (compare panels C and D and E and F in Fig. 5).
Fig. 5 seeks to illustrate the effect of these two strategies by depicting the diffusion distance of an analyte from the bulk atmosphere to the PAS sorbent both at high (Δzhigh) and low wind speed (Δzlow) conditions. The quotient Δzlow/Δzhigh expresses by how much the diffusion distance and therefore the SR is dependent on wind. The numbers for Δz assigned to different PAS configurations in Fig. 5 are hypothetical, but plausible. The schematic of different sampler configuration is based on a sampler design using a sorbent in cylindrical form, a radial diffusion barrier (such as the Radiello), and a jar as a wind shelter, and is inspired by two passive samplers for gaseous mercury22,23 (panels D and E, respectively).
Reducing the wind speed dependence of the SR of a PAS comes at a price, namely the reduction of the SR. This is less pronounced in the wind shelter strategy, as it mostly reduces the SR at high wind speeds, but barely affects the SR under low wind conditions. It is more notable with the application of diffusive barriers, as they increase the diffusion distance under any wind speed regime. Also, the thicker the barrier and therefore the more effective the reduction in wind speed dependence, the lower the SR becomes. Therefore, eliminating the effect of wind speed always needs to be balanced with the desire to sample sufficient amounts of an analyte for reliable quantification.
C. The major challenges of passive air sampling for SVOCs
There are a number of reasons why passive air sampling for SVOCs is uniquely challenging.
C.1 Opposing demands for high vs. tightly controlled uptake rates
Concentrations of individual SVOCs in the atmosphere tend to be very low, often in the range of 10−12 g m−3 (i.e. picograms per m3) or even less. Even though the detection limits of modern analytical methods for SVOCs are extremely low, during active air sampling for SVOCs, sampling volumes of several hundred m3 or even several thousand m3 are commonly used to allow for reliable detection and quantification, especially in remote regions with background concentrations. Such high sampling volumes are not attainable with classical PASs with typical SRs on the order of 0.1 m3 per day or less. In most cases, SRs on the order of 1 m3 per day or higher are required to collect sufficient chemical for quantification during reasonable deployment periods (months).
Kinetic PASs for substances other than SVOCs, whether batch, tube or radial diffusion samplers, have largely relied on diffusive barriers to standardise diffusion distances, and thus control SRs (see Section B.4). PASs for SVOCs, on the other hand, tend not to include diffusion barriers in their design. The main reason is that the uptake rates of PAS designs that incorporate diffusion barriers are too low to be compatible with the very low trace concentrations of SVOCs and the detection limits that can be achieved. Another reason is that the diffusive barrier itself may act as sorbents for SVOCs and thus interfere with sampling.
A fundamental challenge of a PAS for SVOCs is therefore to find the appropriate balance between an SR that is sufficiently high to sample chemical amounts that can be reliably quantified, yet is also reasonably stable and well defined to allow for a quantitative interpretation of the sampled amounts.
Whether a PAS should have a high, but variable SR or a low, tightly controlled SR depends to some extent on the magnitude and the spatial variability of the air concentrations of the target chemical. A compound with low, but spatially highly variable air concentrations is better sampled with a PAS that samples a lot of air (has a high SR), even if the volume sampled is quite uncertain. This makes sure that the sampled amounts are above LOD, yet it is still possible to compare PAS-derived concentrations at different locations. On the other hand, a compound with spatially fairly uniform air concentrations demands a PAS with a well-controlled SR, because only then can differences in the sampled amounts be interpreted as differences in volumetric air concentrations.
The concentrations of most SVOCs in the atmosphere are low and declining. Also, concentration differences of most SVOCs in the atmosphere are quite large, often ranging over many orders of magnitude. As a result, most PASs for SVOCs were designed to have high SRs, even if it meant that the SRs are quite variable and therefore uncertain. For example, the widely used PAS based on polyurethane foam (PUF) disks has an SR around 4 m3 per day (range: 0.5 to 15 m3 per day),24,25 whereas the PAS using XAD-resin as a sorbent has an SR around 0.5 to 1 m3 per day (range 0.4 to 5.5 m3 per day).26–28
On the other hand, some SVOCs, such as hexachlorobenzene (HCB) and hexchlorobutadiene (HCBD), have very long atmospheric residence times and therefore fairly uniform concentrations in the atmosphere.29–31 Unless a sampling site is in the immediate vicinity of a strong source, concentrations in the global atmosphere may only vary within less than an order of magnitude. A PAS would need a tightly controlled SR to distinguish such small concentration differences with confidence. While a PAS for SVOCs with a sufficiently precise SR may not presently exist, an existing PAS for gaseous elemental mercury22 demonstrates that it is feasible in principle for a PAS to elucidate small concentration differences. Its SR is low relative to those of commonly used PAS for SVOCs (0.135 m3 per day), but this is of little concern as the analytical technique for quantification is powerful enough to detect the analyte even after sampling over as little as a week at global background concentrations. One of the main reasons for this is that the entire sampled amount is analyzed (by combustion of the entire sorbent present in the PAS). In the typical PAS for SVOCs, the sorbent is solvent extracted and only a small amount of the sampled compound is injected onto the chromatographic column. Interestingly, SVOCs such as HCB and HCBD, also tend to have relatively high ambient gas-phase concentrations, precisely because of their long atmospheric residence time. If an analytical method were to be used that delivers all of the sorbed amount onto the analytical column (e.g. thermal desorption), even PASs with diffusive barrier may be able to take up amounts of HCB and HCBD above the LOD.
C.2 Large diversity of SVOCs vs. limited applicability range of PASs in terms of volatility
The term SVOCs comprises a wide variety of compounds whose volatility spans several orders of magnitude. For example, the vapour pressure of a 3-ring PAH is approx. 4 orders of magnitude higher than that of a 5-ring PAH.32 PCB congeners of different degree of chlorination cover a similar volatility range.33 Volatility is, of course, also strongly governed by the temperature of deployment. Because the uptake capacity of a sampler is governed to a large extent by compound volatility, this means that a sampler will have widely divergent uptake capacities for different SVOCs and even the uptake capacity for the same compound will be different at different deployment temperatures. One consequence is that the characteristic times, tlinear and tequilibrium, can range over several orders of magnitude for different SVOCs and for the same SVOC at different ambient temperatures.
For example, for the PUF-PAS, the simulation model PAS-SIM21 estimates tlinear to decrease from ∼8 months to ∼8 days, when the log
KPUF–G is decreased from 7 to 5.5. KPUF–G changes by approximately 1.5 log units between a two-ring and a three-ring PAHs or for one compound if temperature is changed by 30 K. It is obvious that (i) equilibrium sampling for less volatile SVOCs is not feasible because of excessively long times to equilibrium, and (ii) it would be difficult to find a deployment period suitable for a range of SVOCs with divergent volatility, because even within the group of relatively volatile SVOCs, tequilibrium will range over orders of magnitude.
However, also kinetic passive sampling is only applicable to substances within a certain range of volatility. A chemical that is too volatile will approach equilibrium too quickly and therefore have a very short tlinear and thus maximum deployment time. A chemical that is too involatile will be sorbed to particles in the atmosphere and therefore not be available for uptake in the PAS in gaseous form (Section F.6 will discuss the uptake of particle-sorbed SVOCs in PAS.). The range of applicability is dependent on the sampler design (uptake capacity and kinetics) and also environmental conditions (e.g. atmospheric particle concentrations), but spans approx. 4 orders of magnitude in volatility. Consequently, it will be impossible to design a kinetic PAS and to use a common set of deployment characteristics (e.g. deployment length) that is applicable to all SVOCs all the time. Unless one is willing to deploy a range of PASs tailored to different volatility ranges, the ambition therefore should be to design a sampler that is applicable to as many SVOCs as possible and under as many different environmental conditions as possible.
D. Description of different types of passive air samplers for SVOCs
A number of different PASs have been proposed for SVOC sampling (Tables 1, 2, 4, and 5). There have been principally two commonly employed strategies for the development of PASs for SVOCs. One involves the use of materials originally developed for water passive sampling, the other the use of sorbents that had found common use in active air sampling for SVOCs. One attractive feature of adopting a passive water sampler design for passive air sampling is the possibility of sampling air and water with the same device and the prospect of recording the air–water equilibrium status. However, caution is necessary to appreciate the different requirements for air and water sampling; for example, while a water sampler can be expected to be exposed to a relatively small range of temperatures during a deployment period, PASs may be deployed at sites with hugely different ambient temperatures that can change rapidly on the time scale of deployment. Also, because the diffusivity of SVOCs in water is so much slower than in the gas-phase and the thickness of boundary layers in air and water varies considerably, different factors may be controlling uptake from water than from air. Most of the PASs that are adopting sorbents from water sampling rely on non-porous materials such as polymeric sheets and films, whereas PASs using sorbents adopted from active sampling tend to rely on porous materials such as polymer foam and resins.
D.1 Samplers involving sorbents enclosed within low-density polyethylene
D.1.1 Semipermeable membrane devices (SPMDs).
The first PAS for SVOCs that adopted a passive sampler for water relied on semipermeable membrane devices (SPMDs), which consist of thin-walled low-density polyethylene (LDPE) lay-flat tubing containing a thin film of the neutral synthetic lipid triolein.34 Most of the early studies using SPMDs as PASs were interested in sampling both air and water.35–37 Ockenden et al. calibrated SPMDs as PASs for PCBs38,39 and then applied them to repeatedly record a latitudinal gradient in atmospheric contamination in Western Europe.40–45 Söderström et al.46,47 were other early adopters and explored the wind speed dependence of uptake in SPMD-based PASs47,48 and were the first to use SPMDs for indoor deployments.49
Cranor et al.50 used a laboratory set-up to determine both the uptake kinetics and the uptake capacity of SPMDs for 48 compounds including several PAHs, PBDEs, OCPs, and current-use pesticides (CUPs). Cicenaite et al.51 also used laboratory experiments to determine these parameters for three compounds (naphthalene, 2-chlorophenol, 1,4-dichlorobenzene) as a function of temperature in the range from −16 to +40 °C.
D.1.2 PASs with other sorbents enclosed in PE membranes.
Several modifications of SPMDs have been proposed for air sampling, which substituted triolein with other sorbents (Table 1). The rationales for this substitution included a simplified preparation and clean-up procedure in samplers not containing triolein52,53 and the high cost of triolein.54 Also, SPMDs are a patented product which can impede its widespread adoption. In principle, a different sorbent may also increase the uptake capacity of a PAS relative to an SPMD.
Table 1 Passive air samplers for SVOCs involving sorbents enclosed within low-density polyethylene (LDPE)
Name |
Passive sampling material |
Housing |
Ref. |
Semipermeable membrane devices (SPMDs) |
Thin-walled LDPE lay flat tubing containing a thin film of synthetic lipid triolein (1,2,3-tri[cis-9-octadecenoyl]glycerol) |
White wooden box with louvred sides (Stevenson screen) |
34
|
Poly(dimethylsiloxane) (PDMS) |
Thin-walled LDPE lay flat tubing containing either PDMS-coated stir bar (VS = 24 ml, A = 2.8 cm2) or silicone tubing (VS = 250 ml, A = 12 cm2) |
Stainless steel box (20 cm diameter, 20 cm high), open at the bottom with perforated sides |
52
|
Poly(dimethylsiloxane) (PDMS) |
LDPE cylinder containing either PDMS-coated stir bar (A = 1.67 cm2) or spiral silicone rod (A = 5 cm2) |
Cylindrical stainless-steel shelter with an opening at the bottom |
55
|
Membrane-enclosed copolymer (MECOP) samplers |
Thin-walled LDPE lay flat tubing containing crystalline ethylvinyl-benzene–divinyl-benzene copolymer |
Perforated steel box |
53
|
Versatile, easy and rapid atmospheric monitor (VERAM) |
Various sorbent materials enclosed in thin-walled LDPE lay flat tubing (a mixture of florisil and activated carbon recommended for VOCs sampling) |
Unknown |
54
|
Wennrich et al.52 and Paschke and Popp55 proposed to substitute triolein with polydimethyl siloxane (PDMS or “silicone”) as a sorbent in different configurations (silicone tubing, silicone spiral rods, PDMS-coated stir-bar), either enclosed in a PE membrane similar to an SPMD52 or in a cylindrical PE tube.55 The fairly low SRs of these PAS designs (on the order of 0.01 m3 per day) are at least partly overcome by an analytical technique that relies on thermal desorption of the PDMS. This not only delivers the entire sampled amount onto a gas chromatographic column, but the lack of sample extraction procedures may allow for lower blank levels and thus detection limits. Unfortunately, very large differences in uptake rates observed between compounds, between indoor and outdoor deployments, and between different sampler configurations remained unexplained52,55 and no follow-up studies based on these approaches appear to exist.
Choi et al.53 enclosed a crystalline ethylvinylbenzene–divinylbenzene copolymer in a PE membrane, calling it membrane-enclosed copolymer (MECOP) samplers. No SRs had been determined, so rates for SPMDs were applied after adjusting for the much smaller surface area of the PE membrane in the MECOP relative to an SPMD. Esteve-Turrillas et al.54 experimented with a number of different sorbents in SPMD-type lay flat PE tubing, and recommended a mixture of florisil and activated carbon.
D.1.3 Limitations of samplers involving sorbents enclosed within LDPE.
The justification for enclosing a sorbent within a PE membrane is the desire to control the diffusion distance through the use of a permeable barrier. As discussed in Section B.4 above, a tightly controlled diffusion distance is a key requirement for a PAS that is not susceptible to large variations in the SR as a result of different wind exposure. A problem with this approach is that a PE membrane itself is a sorbent for SVOCs. Furthermore, capacity and permeability of PE are functions of temperature.41,56,57 Collectively, this leads to highly complex uptake characteristics of PASs using PE-enclosed sorbents that make a reliable, quantitative interpretation of results highly challenging. Some studies essentially concluded that an incomplete understanding of the effect of temperature on the uptake characteristics of those types of samplers precluded a quantitative interpretation.58,59 Whereas SPMDs continue to be used occasionally as PASs,61–65 the PAS designs in Table 1 can no longer be regarded as representing the state-of-the-art in passive air sampling for SVOCs.
D.2 Samplers based on non-porous sorbent materials
The samplers described in Section D.1 consist of two sorbing phases, namely the enclosed sorbent and the polyethylene. This appears redundant, because even with two sorbents present, these PASs often do not have sufficient uptake capacity for more volatile SVOCs to remain in the linear uptake phase during typical lengths of deployment (e.g.ref. 41). Also, the presence of two sorbents makes the understanding and quantification of uptake kinetics and uptake capacity unnecessarily complicated. Accordingly, most PASs rely on a single material able to act as a sorbent. In particular, a fairly large number of PASs relies on a non-porous sorbent material, such as a non-porous polymer or a solid lipid as a sorbent (Table 2).
Table 2 Passive air samplers for SVOCs based on non-porous sorbent materials
Name |
Passive sampling material |
Housing |
Ref. |
Solid phase micro-extraction (SPME) |
SPME fibre made from PDMS |
Needle housing |
104
|
“Synthetic leaf” |
Fibreglass cloth (250 mm × 500 mm) coated with 0.3, 1.5 or 3 g of tristearin (2,3-di(octadecanoyloxy)propyl octadecanoate) |
Stainless-steel frames (0.5 m × 0.5 m) in open-sided container |
120
|
Polymer-coated glass (POG) |
Hollow glass cylinder (68 mm o.d., 64 mm i.d. and 70 mm tall) coated inside and outside surfaces with thin film (0.5 μm thickness) of ethylene vinyl acetate |
Double bowls made of stainless steel |
92
|
Low-density polyethylene (LDPE) films |
Low-density polyethylene film (30 cm by 30 cm) |
Galvanised iron chambers with an open bottom and louvers on all sides |
67
|
Artificial leaf (AL)-PAS |
Paraffin oil on cellulose paper |
Petri dish |
121
|
Ethylene vinyl acetate (EVA) |
Glass fibre filter (GFF) or thick aluminum foil coated with ethylene vinyl acetate |
None |
98
|
PDMS-based PAS |
Silicone disk of 0.5 mm thickness in a 15 cm Petri dish or a baking paper sheet (40 cm × 60 cm) coated on both sides with a thin film (0.001 mm) of silicone (0.5 g Si per m2) |
None |
114
|
PDMS sheet |
PDMS sheets of 0.1 cm thickness (11 cm long × 9 cm wide × 0.1 cm thick with total surface area 202 cm2) |
None |
111
|
PDMS brooch |
PDMS strip (9 cm long × 5.5 cm wide × 0.1 cm thick, surface area was 50 cm2) |
Personal sampler (aluminum plate to prevent contact with clothing) |
113
|
PDMS-coated stir bar |
Stir bar, 2 cm long coated with 1 mm thick PDMS film |
Double stainless-steel domes |
110
|
D.2.1 Samplers based on low-density polyethylene (LDPE).
Müller et al.66 realised that the sorbent enclosed in the PE of the SPMDs may be redundant, as the PE itself can act as a sorbent. Bartkow et al.67 showed that simple PE sheets of different size and thickness suspended in air can constitute a PAS. In particular, they showed that the simultaneous deployment of PE sheets of different thickness can be used to determine whether a chemical is in the linear uptake phase or approaches equilibrium. The amount of substances with kinetically controlled uptake is independent of the volume of the PE sheet VS and related to its surface area A, whereas the amount of substances achieving equilibrium is proportional to VS.67 Bartkow et al.68 demonstrated the viability of using depuration compounds (DCs) spiked into the PE prior to deployment to account for differences in wind exposure of PASs based on PE-sheets (see also Section F.4 below). PE-based PASs have since been used extensively by US-based researchers.
Somewhat surprisingly, there appears little attempt to standardise the deployment of PE-based PAS. A variety of different sizes of PE sheets, strips, and lay-flat tubing appear to be in use, but dimensions and sheet thickness are not always provided. Furthermore, different housings are being used to shelter the PE sorbent. Some use a metal box with louvred sides and an open bottom,67 whereas others use a metal cage that is not further described, but appears to be long and upright.69–71 Lohmann et al.72–75 either use the double bowl shelter of Shoeib and Harner24 or a version where the bottom bowl is eliminated. It is also often not clear how the PE sheets are being placed in these shelters and photographs suggest that little attention is paid to the precise arrangement (see e.g. photograph in ESI in ref. 76). Overall, this suggests that even during outdoor deployments little effort is made to limit or standardise the wind exposure of the PE and a lot of trust is therefore placed in the capability of DCs to correct for variable wind exposure (Section F.4).
Because PE-based samplers are commonly employed in water passive sampling, PE-based PASs offer, similar to SPMDs, the possibility of using the same sampler material in air and water and therefore the possibility to derive the equilibrium status between them.77–90 This is further discussed in Section G.8.1.
D.2.2 Samplers based on ethylene vinyl acetate (EVA).
A thin film of ethylene vinyl acetate (EVA) coated on glass was introduced as a means to sample SVOCs from the headspace of a sample vial.91 Large surface area to volume ratios of the EVA film were meant to assure quick equilibration with the gas-phase. Harner et al.92 proposed to use EVA coated onto glass and placed in a wind-shelter as PAS. Farrar et al.93 determined the uptake and loss kinetics of these so-called POlymer-coated Glass samplers (POGs) and used them to measure concentration variability along a vertical gradient in the urban boundary layer94 and within a European-scale network.95 POGs have also been used for sampling in water.96 Uptake in EVA-films was observed to be somewhat faster than in PE sheets, but equilibration times were longer because the uptake capacity of EVA was larger than that of PE.97 Instead of glass as a base onto which the EVA is applied, it is also possible to use glass fibre filters (GFFs) or aluminum foil.98 While explicitly designed to equilibrate fast (within a number of days of deployment), for less volatile chemicals the time to equilibrium can be quite long. As a result, the interpretation in terms of volumetric air concentrations has to be different for different SVOCs.
D.2.3 Samplers based on polydimethyl siloxane (PDMS).
A possible drawback of PE-based samplers is the relatively low diffusivity of SVOCs in PE,99,100 which may compromise the commonly made assumption that resistance to uptake in a sampler material is dominated by diffusion through the air boundary layer. Other polymers, in particular PDMSs, tend to allow for faster diffusion and thus pose lower resistance than PE99,100 and one can therefore be more confident that uptake is controlled by the air-side resistance. In Section D.1.1, we already learnt that PDMS had been proposed as the main sorbent in a PAS for SVOC,52,55 but the enclosure of that PDMS in PE compromises the advantage of the higher permeability of the PDMS. Just like SPMDs and LDPE, PDMS is now routinely used for passive water sampling101–103 and thus could be used for sampling in multiple environmental media.
One of the first kinetic PASs based on PDMS was a solid phase micro-extraction (SPME) fibre which is retracted into its needle housing. Changing the distance by which the fibre is retracted within the needle housing results in a change in the diffusion distance and therefore in the SR.104 While the SRs are exceptionally small, around 10−5 m3 per day, all of the chemical sorbed to the PDMS is delivered by thermal desorption onto the analytical column without any sample preparation steps. If a chemical is too volatile, it will be lost from the fibre prior to desorption; if it is too involatile, it will sorb to the inside surface of the needle housing.105 Despite the low SR, the tlinear is quite short (less than a day), because the uptake capacity is also small (the PDMS volume VS is small). In its non-retracted mode, an exposed SPME fibre could also function as an equilibrium passive air sampler.106 This approach was applied to the measurements of pesticide concentration in a greenhouse.107
The amount of target chemical sorbed to the small volume of PDMS in an SPME fibre may often be too low for reliable quantification. PDMS-coated stir-bars have a higher sorptive capacity because of a larger sorbent volume VS, yet can still be analyzed with thermal desorption. Stir bar sorptive extraction (SBSE) combined with thermal desorption gas chromatography-mass spectrometry (GC-MS) has been proposed as a passive air sampling technique and applied to record the concentration variability of an insecticide108 and PAHs109 in indoor environments. Matsiko et al. showed that the technique is suitable for measuring organophosphate flame retardants (OPFRs) at ambient atmospheric concentrations and reported initial SRs on the order of 2 to 7 m3 per day.110 These SRs appear abnormally high, when considering that other PDMS-based samplers with much larger surface area than a 2 cm-long stir bar have much lower SRs (see next paragraph111–114). When SBSE is used to extract an analyte from a liquid, the stir bar can be spun magnetically to lower the boundary layer thickness and thus speed up the uptake kinetics. During its use in air sampling, the stir bar is not actually spun, i.e. the fact that the PDMS is coated on a magnetic stir bar is incidental. Unfortunately, it also means that uptake in the stir bar from the air will be dependent on atmospheric turbulence.
In 2016, two research groups111,114 independently proposed samplers made from PDMS for sampling of SVOCs from indoor air. Okeme et al. cut rectangular PDMS sheets of 0.1 cm thickness (A = 202 cm2) and suspended them in air.111 Two 50 day calibrations for this sampler have been reported. The first calibration involving 5 phthalates, 3 brominated monoaromatics and 9 PBDE congeners yielded an average SR of 1.7 m3 per day,111 whereas a second using more or less the same set of compounds (3 PBDEs less, two OPFRs more) gave an average value of 6.1 m3 per day.112 Going forward Okeme et al. recommend the average of all of these values, which translates to a surface area-normalised SR of 1.5 ± 1.1 m3 per day per dm2.112
Vorkamp et al. suggested either a silicone disk of 0.5 mm thickness in a 15 cm Petri dish (A = 177 cm2) or a baking sheet coated on both sides with a thin film (0.001 mm) of silicone (A = 4800 cm2).114 The latter was specifically designed with an extremely high surface area to volume ratio (A/VS), thus aiming for a low tequilibrium. Experiments confirmed that time to equilibrium decreased with A/VS ratio and the baking sheets reached equilibrium within two weeks, even for PCB congeners with an intermediate degree of chlorination.114 SRs increased with surface area, but less than might be expected – while surface area A for the sheet was 27 times larger than for the disk, the SRs for PCB-101 were only higher by an order of magnitude (0.2 m3 per day vs. 1.8 m3 per day), even though the sheet may be expected to have a smaller air side resistance.
Okeme et al. presented another PDMS-based PAS design to be worn as a personal sampler. A 0.1 cm thick sheet of PDMS (50 cm2) is attached to an aluminum plate that serves to prevent contact of the sorbent with clothing when worn as a “brooch”.113 Being calibrated for a number of phthalates against pumped personal samplers, they estimated an SR of around 0.4 m3 per day or 0.86 ± 0.29 m3 per day per dm2, when normalised to surface area. The variability in the SR between individuals and between different SVOCs was large.
Table 3 compares the SRs for the various PDMS-based PASs that have been proposed in the last few years for indoor deployments. The table lists the ranges of the reported SRs for different SVOCs. Unfortunately, the variability in the SRs between different SVOCs for the same sampler and even between different calibration experiments for the same sampler is large. For example, the highest SR for a chemical in a PAS is often a factor of 2 to 4 times higher than the lowest SR, although the theory of PAS uptake predicts that SR for different chemicals should be similar when the resistance to uptake lies on the air side. Even two very similar calibrations for the same sampler yielded SRs that deviate by more than a factor of 3.111,112 More disconcerting is that surface area-normalised SRs of different PDMS samplers deviate by orders of magnitude. They range from a low of less than 0.1 m3 per day per dm2 in the baking sheet114 to more than 100 m3 per day per dm2 for the PDMS-coated stir bar.110
Table 3 Sampling rate, surface area and area-normalised sampling rate for various PDMS-based PAS
Sampler |
SR in m3 per day |
Exposed surface area in cm2 |
Area-normalised SR in m3 per day per dm2 |
Ref. |
Assuming a diameter and length of the stir bar of 0.8 cm and 2 cm, respectively.
|
Suspended sheet |
0.9 to 4.1 |
202 |
0.45 to 2.0 |
111
|
Suspended sheet |
3.5 to 10 |
202 |
1.7 to 5 |
112
|
Suspended XAD-coated sheet |
1.9 to 4.3 |
102 |
1.9 to 4.2 |
112
|
Sheet attached to aluminum plate |
0.2 to 0.66 |
50 |
0.4 to 1.32 |
113
|
Disk in Petri dish |
0.2 to 0.3 |
177 |
0.11 to 0.17 |
114
|
Coated baking sheet |
1.3 to 3 |
4800 |
0.03 to 0.06 |
114
|
Coated stir bar |
1.8 to 6.7 |
5a |
40 to 140 |
110
|
The SR is dependent on the extent of air turbulence and therefore the thickness of the stagnant air layer surrounding the PDMS. Exposure to air turbulence could differ between different deployments, different calibration studies and for the various sampler designs, especially because none of the designs discussed here relies on a housing to reduce these effects (see Section B.4) and because indoor environments can be quite inhomogeneous in terms of the air turbulence they experience. However, taken collectively, it suggests that the experimental evidence presented so far is difficult to reconcile with the theoretical expectations on how PDMS-based samplers should behave, in particular with respect to an SR that is proportional to surface area A and largely invariant for different substances of similar molecular size. Interestingly, the inconsistency in SRs mirrors somewhat the experience with PDMS-based samplers that include a diffusive barrier52,55 that were discussed in Section D.1.2.
D.2.4 Samplers based on lipid-coated fibres.
SPMDs had been conceived as mimicking an aquatic organism consisting of a lipid reservoir and a membrane that controls uptake from the aqueous phase.115 In an analogous way, others have sought to design PASs that are inspired by plant foliage. Leaves and needles have been used for monitoring air concentrations of SVOCs based on diffusive uptake in the waxy plant cuticle116 and the idea of a “synthetic or artificial leaf” is to standardise this sampling medium and eliminate variations between species and individual plants117,118 or even within the same plant over time.119 The approach essentially seeks to represent the plant cuticle with a layer of wax having a large surface area. Proposed designs include stearin-coated fibreglass sheet120 and cellulose paper coated with paraffin oil and placed in a Petri dish.121 Müller et al. determined both stearin-gas-phase partition coefficients (KSG) for PAHs and the mass transfer coefficients controlling uptake in and loss from the synthetic leaf.120 Field evaluations gave mixed results with agreement within a factor of 2 in one location and poorer agreement in another. Stracquadanio et al. exposed their artificial leaf for only a few hours during which PAHs were expected to have achieved equilibrium between paraffin oil and gas-phase.121
D.2.5 Advantages and limitations of samplers based on non-porous sorbent materials.
One advantage of the PASs that rely on a sheet or film of a non-porous polymer, such as PE, PDMS and EVA, is the possibility to custom-design PASs with specific uptake capacities and SRs through the modification of the polymer's surface area-to-volume ratio A/VS. Even if the experimental evidence so far does not support a direct proportionality between surface area A and SR (Table 3), as one should expect if the uptake is limited by the air-side boundary layer, it should eventually be feasible to design a PAS with the desired tlinear and tequilibrium for a particular target compound. In particular, it should be possible to design a PAS that will reach equilibrium for most SVOCs in a reasonable amount of time. The PDMS-coated baking sheet114 had some of the highest achievable A/VS and therefore is the closest of any design to a true equilibrium PAS for SVOCs. Unfortunately, the sheet has to be quite large for quantifiable amounts to accumulate in the PDMS film, which may make it too obtrusive for some indoor applications.
When comparing the relative merits of PE, PDMS and EVA, we can observe that PE has the lowest KSG among the three polymers used in these types of PASs.97,122 However, it is quite simple to increase a sampler's uptake capacity by increasing its VS, e.g. by using thicker sheets. While PDMS and PE are non-polar polymers, EVA also has polar functional groups, which may increase the KSG for polar target analytes. The KSG of PDMS is very well studied and good predictive techniques are available (see Section E.2 below), while the experimental data set for EVA and PE is much thinner and, in the case of PE, relies largely on values derived indirectly from partitioning data between PE and water.
PDMS, and likely also EVA, have the advantage of higher permeability relative to PE,100,123 which in principle should facilitate validity of the assumption of air-side controlled kinetics, which is important when using commonly applied equations for the interpretation of results in the curvi-linear uptake region. On the other hand, PE appears to be a more robust polymer material, which can withstand the rigors of field deployment better than EVA and possibly also PDMS. Interestingly, PDMS-based PASs have mostly been proposed for indoor use, where the demands on the robustness of a polymer are reduced.
The main limitation of PASs using non-porous polymeric sorbents is their relatively low uptake capacity. When an application of these PASs targets multiple analytes that vary in terms of their KSG, some target analytes will be in the linear uptake phase, while others will be in the equilibrium or in the transitional phase. They are therefore neither kinetic nor equilibrium samplers. Deciding whether kinetic or thermodynamic factors or both dominate is not straightforward, as it depends on the complex interplay between chemical properties (KSG, diffusivity in air and polymer), meteorological factors (temperature, wind), sampler characteristics (thickness of polymer) and deployment parameters (deployment length). Quantitative knowledge of how these factors interact is often lacking. For example, in his critical review of LDPE as a passive sampling material, Lohmann admits that “no data were found on the temperature effect” on the partitioning of SVOCs between LDPE and the gas-phase, and that the strong temperature dependence of the diffusivity of compounds in LDPE “has not been addressed in greater detail”.124
Nevertheless, various authors have proposed fitting methods for deriving air concentrations from measurements of SVOCs in the curvi-linear uptake phase of PASs and these are now routinely applied. One should, however, be conscious of the fact that if information required in the quantitative interpretation of relatively low capacity PASs is lacking, incomplete or highly uncertain, this quantitative interpretation will suffer from very considerable uncertainties (see Section F.3 below).
Generally, it appears that PASs based on non-porous polymers have no standardised wind shelter or housing (except possibly the original POGs92). In many cases, no housings are used at all. This is somewhat surprising, since in all of those PASs, it is assumed that the air-side resistance is rate-limiting SVOC uptake and therefore the SRs can be expected to be strongly dependent on the air turbulence surrounding the polymer. While this may be acceptable for true equilibrium samplers or for indoor deployments in places with limited turbulence, it makes the SR of such samplers in outdoor field deployments highly variable and wind exposure the main source of uncertainty. One attempt to reduce these uncertainties is the use of DCs. This is discussed in greater detail in Section F.4 below. It is noteworthy that many authors propose non-porous polymer PASs mostly for use indoors,111–114 where wind exposure is generally limited and where temperatures and concentrations of many SVOCs tend to be reasonably constant, avoiding the challenges described in Section C.
D.3 Samplers based on porous sorbent materials
D.3.1 Samplers based on polyurethane foam (PUF).
D.3.1.1 The PUF-PAS by Shoeib and Harner.
Polyurethane foam (PUF) has been used as a sorbent for SVOCs in active air sampling for a long time.125 Shoeib and Harner24 first introduced this sorbent to PASs for SVOCs, by placing a foam disk in the space between two metal bowls (Fig. 6A). This sampler design has become the most popular PAS for SVOCs because PUF is inexpensive, easy to handle, and has a reasonably high capacity. While the SR for this sampler is often reported to be around 3 to 4 m3 per day, the variability in SRs between different studies and for different compounds in the same study is quite high. Reported SRs range from less than 1 to 30 m3 per day.126,127 Melymuk et al. have discussed at length the factors that contribute to this variability.25 Even under indoor deployment conditions, the variability in SR of the PUF-PAS for different SVOCs and in different calibration studies is large; for example, a range 0.41–11 m3 per day has been reported for single-sheltered PUF-PAS.112 Section F.2 below addresses the issue of SR variability in detail.
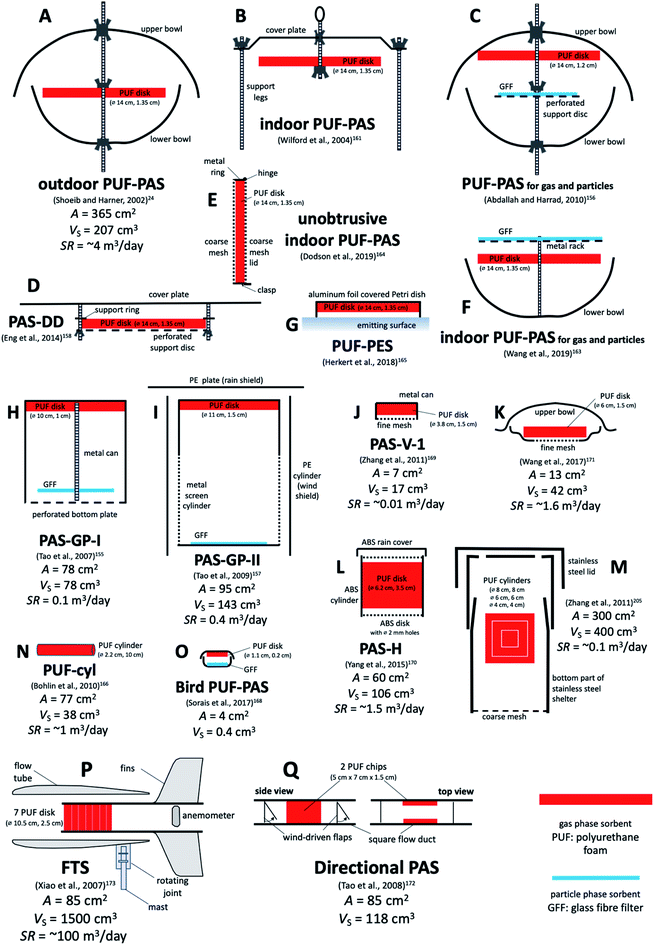 |
| Fig. 6 Various PASs based on polyurethane foam (PUF) as sorbent. Designs (A) to (F) are based on a PUF disk of the same size. Designs (B), (E) and (F) are for indoor deployments. Design (C), (F), (H) and (I) are aiming to sample gaseous and particle-bound SVOCs separately with PUF and GFF. Design (G) measures emissions from a surface. Designs (J), (K) and (L) are used to measure small scale elevation gradients above soil. Design (M) was used to probe the existence of a sampler side resistance to uptake in PUF. Designs (N) and (O) are miniaturised samplers to be worn by people or birds. Designs (P) and (Q) rely on the wind to blow through or past the PUF. A is the PUF surface area exposed to the air and VS is the PUF volume. Sampling rates SR range from 0.01 m3 per day (design (J)) to 100 m3 per day (design (P)). See Table 4 for more detail. | |
One factor contributing to this variability is wind. Laboratory experiments128 and field studies129,130 have shown that the SR of the PUF-PAS is quite strongly dependent on wind speed. This is because the double bowl is not entirely effective in preventing winds from impacting on the PUF disk. Chaemfa et al. measured the wind speed within a double bowl housing exposed to different wind speeds in a wind tunnel.131 The wind speed above the PUF disk was roughly proportional to the outside wind speed and less than a factor of three smaller. The wind speed below the PUF disk was somewhat lower and increased linearly with outside wind speed at outside wind speeds less than 4 m s−1.131 This is also consistent with computational fluid dynamics simulations of housings involving either one or two bowls,132 which indicate the dependence of the SR on wind speed and wind angle of incidence.133 DCs can be used to account for differences in wind exposure between deployments (see also Section F.4 below).130 Because of the double bowl shelter's inefficiency in blocking wind from impacting on the sorbent, PASs using this design tend to also accumulate particle-bound substances (see Section F.6).
Differences in SR can also be expected between indoor and outdoor deployments. Lower SRs indoors than outdoors are explained by the lower exposure to atmospheric turbulence.134 Small differences in the dimensions of the double bowl shelter do not contribute to statistically significant differences in the SR.135
D.3.1.2 The sorbent impregnated PUF disks (SIPs) samplers.
Because the PUF has too limited an uptake capacity to sample relatively volatile SVOCs kinetically (see Section E.1.3), Shoeib et al. coated polyurethane foam with ground-up XAD-4 (divinylbenzene–styrene copolymer) powder to obtain so-called sorbent impregnated PUF (SIP).136 Direct side-by-side comparisons showed that this indeed increased the uptake capacity of the sampler and increased tlinear.137,138 Use of SIPs since has focused on neutral perfluoroalkyl substances139–146 and organosiloxanes,147–149 because these groups of SVOCs comprise some relatively volatile substances. However, SIPs are also being used for SVOCs that had previously been sampled with PUF-PASs150–152 as well as organophosphate esters138 and ionic perfluoroalkyl substances.153
The idea of adding XAD to a sorbent to increase its uptake capacity has since been used also for filter paper154 and PDMS sheets.112 XAD-coated and uncoated PDMS sheets were found to have similar area-normalised SRs, but the SR of the XAD-coated sheet showed less variability for different compounds (RSD reduced almost in half from 31 to 17%).112 The calibration experiment was too short to confirm that the XAD-coated sheet had a longer tlinear.112
The rationale for increasing the sampling capacity of one sorbent by combining it with a high capacity sorbent is not always apparent, considering that one simply could use a sampler based on a high capacity sorbent in the first place. Drawbacks of using combined sorbents include (1) the need for additional preparation steps that require time and introduce the possibility of sorbent contamination, (2) the challenge of reproducing, and maintaining during deployment and handling, the exact composition of the mixed sorbents and therefore the uptake capacity of a PAS, and (3) the likelihood of introducing additional complexity to the uptake kinetics, for example with respect to a sampler side resistance.
D.3.1.3 Other PASs based on PUF.
The design by Shoeib and Harner24 is not the only PAS that is using PUF as a sorbent (Table 4). In particular, several attempts have been made to complement the PUF with a GFF meant to collect particle-bound SVOCs. Tao et al. presented a design, called PAS-GP-I, involving a cylindrical metal can, that has a PUF disk at the closed top and a GFF suspended close to the perforated bottom (Fig. 6H).155 Abdallah and Harrad adopted essentially the same idea, except that they placed the PUF at the top and the GFF in the middle of the double bowl housing of the original PUF-PAS (Fig. 6C).156 During indoor deployments, the PAS-GP-I had very low SR of around 0.1 and 0.01 m3 per day for gaseous and particle-bound PAHs, respectively,155 whereas the modified PUF-PAS had a SR of around 1 and 0.5 m3 per day for gaseous and particulate brominated flame retardants, respectively.156 In 2009, Tao et al. introduced a modified design, called PAS-GP-II, in which the can holding PUF and GFF was made of an open mesh, which in turn was placed in a cylindrical wind shelter made of PE (Fig. 6I).157 The motivation for the re-design was the need to increase the SR, if the sampler is to be useful in locations where SVOCs are not greatly elevated. Indeed, the SRs of PAS-GP-II were determined to be 0.4 and 0.6 m3 per day for gaseous and particulate PAHs, respectively.
Table 4 Passive air samplers for SVOCs based on polyurethane foam described in the literature. The letters in brackets behind the name refer to Fig. 6
Name |
Passive sampling material |
Housing |
Ref. |
PUF-PAS (A) |
PUF disk (14 cm diameter, 1.35 cm thick, A: 365 cm2, VS: 207 cm3, density 0.0213 g cm−3, 4.40 g mass, 0.567 cm effective thickness) |
Stainless steel double bowls (diameter: top 30 cm, bottom 24 cm) or single cover plate (indoors) |
24 and 161
|
Modified PUF-PAS (C) |
PUF disk (14 cm diameter, 1.2 cm thick, A: 360.6 cm2, density 0.02 g cm−3) and GFF (12.5 cm diameter) |
Stainless steel double bowls (top 23 cm, 2 L, bottom: 18 cm, 1 L) |
156
|
PAS-GP-I (H) |
PUF disk (10 cm diameter × 1 cm thick, density 0.024 g cm−3) and GFF (8 cm diameter) |
Stainless steel can (10 cm diameter × 10 cm high) with perforated bottom |
155
|
PAS-GP-II (I) |
PUF disk (11 cm diameter × 1.5 cm thick, density 0.024 g cm−3) and GFF (11 cm diameter) |
Mesh cylinder (11 cm diameter, 23 cm high) inside a PE cylinder |
157
|
Directional PAS (Q) |
2 PUF chips (5 × 7 × 1 cm3 each, 0.024 g cm−3) |
Flow duct (20 cm long, 5 cm high, 5 cm wide) with 2 one-way valves |
172
|
PAS-V-I (J) |
PUF disk (3.8 cm diameter, 1.5 cm thick, density 0.024 g cm−3) |
Stainless steel cylinder (3.8 cm diameter, 2 cm high) with mesh-covered bottom (400 mesh, 3 cm diameter) |
169
|
PAS for vertical gradients (K) |
PUF disk (6 cm diameter, 1.5 cm thick, density 0.024 g cm−3) |
Top bowl, bottom ring and fine mesh (4 cm diameter) |
171
|
PAS-DD (D) |
PUF disk (14 cm diameter, 1.35 cm thick, A: 365 cm2 36% covered) |
Circular flat metal plate placed 2 cm above the disk |
158
|
PAS-H (L) |
PUF disk (3.5 cm thick, 6.2 mm diameter, density 0.024 g cm−3, A: 128 cm2, VS: 106 cm3) |
ABS cylinder (5.5 cm high, 6.2 cm inner diameter), 18 2 mm holes in top and bottom, rain shield |
170
|
Miniature bird-borne PAS (O) |
PUF (0.2 cm-thick, 0.023 g cm−3 density, A: 4 cm2) and GFF (0.045 cm thick, 0.19 g cm−3, A: 3.45 cm2) |
3D-printed elliptical double bowl |
168
|
Alternatively: PDMS (0.1 cm thick, 1.14 g cm−3 density, A: 4 cm2) |
Mini-PUF or PUF-cyl (N) |
PUF cylinder (10 cm long, diameter 2.2 cm, A: 77 cm2, density 0.030 g cm−3) |
Protective cover net mesh size 1.0 mm, placed on helmet or lapel |
166
|
Indoor PUF-PAS (E) |
PUF disk (14 cm diameter, 1.35 cm thick) |
Aluminum rigid mesh holder big enough to just hold the PUF disk |
164
|
Indoor PUF-PAS (F) |
PUF disk (14 cm diameter, 1.35 cm thick) and GFF |
Stack of GFF (top), PUF disk (middle) and bowl (bottom) |
163
|
PUF-PES (G) |
PUF disk (14 cm diameter, 1.35 cm thick) |
Inverted glass Petri dish, covered with aluminium |
165
|
Flow-through sampler (P) |
Seven P10z PUF disk (10.5 cm diameter, 2.54 cm thickness and large porosity, 10 pores per inch) |
Horizontally oriented, aerodynamically shaped, stainless steel flow tube |
173
|
Eng et al. presented a PUF-based PAS specifically designed to quantify the dry deposition of SVOCs.158 A PUF disk of the same dimensions as is used in the regular PUF-PAS is placed 2 cm beneath a circular flat cover plate, which serves as a sun- and rain shelter (Fig. 6D). The more open shelter design of this so-called passive dry deposition (PAS-DD) sampler allows for more efficient sampling of particles, particularly large particles. However, this open design also results in less wind sheltering than in the double bowl housing, which should make the SR of the PAS-DD even more dependent on variations in wind speed than those of the PUF-PAS. The PAS-DD has been used in combination with the PUF-PAS to determine dry deposition fluxes and velocities of PAHs in two Nepalese cities159 and to study the dry deposition of pesticides.160
A number of modified versions of the original PUF-PAS24 have been presented specifically for use indoors. In order to speed up uptake indoors, Shoeib et al.161,162 proposed to eliminate the double bowl housing for indoor deployments and only use a plate to prevent gravitational settling of coarse particles (Fig. 6B). Eliminating the bottom bowl, as done by Harrad and Abdallah,256 amounts to essentially the same thing. The SR of an unsheltered sampler indoors was either ∼2.5 m3 per day161,162 or 1.5 m3 per day.256 In order to separate particle-bound and gaseous SVOCs, Wang et al. placed a GFF for sampling of particles slightly above the standard PUF disk, which itself was placed at the top of a stainless-steel bowl (Fig. 6F).163 This resembles the set-up by Abdallah and Harrad,156 but by not having a cover at the top, the filter will also be sampling larger particles subject to gravitational settling.
Dodson et al. designed a small aluminium housing for a PUF-disk of the size used in the PUF-PAS, specifically for indoor deployments (Fig. 6E).164 The motivation was to take advantage of a well-characterised PAS sorbent, but have a minimally intrusive and easy-to-set-up housing that still allows for efficient airflow past the PUF and prevents gravitational particle deposition. Side-by-side sampling with an active sampler allowed for the estimation of SRs and to confirm that the PAS derived air concentrations of gas-phase SVOCs were accurately ranked when judged by the results of the active sampler.164
Herkert et al. placed a PUF-disk in an inverted Petri dish to record the release of SVOC vapours from indoor surfaces and called the device a passive emission sampler (PES) (Fig. 6G).165 Bohlin et al. used a small PUF cylinder (10 cm long, 2.2 cm diameter) in a small mesh net, attached to a helmet or to a person's clothing, as a personal sampler for SVOCs (Fig. 6N).166 This sampler design could also be used for stationary sampling indoors. SRs for that sampler based on comparisons with concentrations obtained with personal active sampling have been reported.166,167
Sorais et al.168 presented a miniaturised version of the sampler of Abdallah and Harrad156 that could be attached to free-ranging birds, i.e. a personal sampler for birds. It combined a small PUF disk and GFF in an elliptical double polyamide bowl manufactured with a 3D printer (Fig. 6O).
Three PUF-based PASs have been presented that sought to determine vertical concentration gradients above the ground.169–171 In all three cases, a PUF disk was placed in a cylindrical housing, made either of stainless steel169,171 or acrylonitrile butadiene styrene (ABS)170 that was just big enough to accommodate the disk. The housing either had an opening at the bottom covered with a stainless-steel screen mesh (Fig. 6J),169 or had eighteen 2 mm holes drilled into both the top and the bottom of the housing, whereby the top was protected by a rain shield (Fig. 6L).170 SRs, in both cases obtained by calibration against another PUF-based PAS, were 0.01 to 0.03 m3 per day,169 and ∼1.5 m3 per day,170 respectively. In the design by Wang et al. the top of the PUF disk is covered by a small inverted bowl, which allows for a larger area to be exposed to air and therefore an increased SR (∼1.5 m3 per day) (Fig. 6K).171
Whereas most PASs seek to prevent the wind from impacting on the sorbent, two PUF-based samplers were explicitly designed to take advantage of the wind in the sampling process. The “directional” PAS seeks to sample only when the wind blows from a particular direction.172 The air is guided to pass by two small PUF chips within a small wind duct aligned with the desired wind direction. Hinged polypropylene flaps act as one-way valves that shut down the airflow when there is no wind from that direction (Fig. 6Q). Calibrated and field-tested for PAHs,172 this sampler does not seem to have found use since.
Xiao et al. designed a horizontal cylindrical flow tube that turns into the wind with the help of ball bearings and wind baffles.173–175 The wind is blowing the air through a series of PUF disks placed in the flow tube and is then recorded with a battery-operated anemometer at the outflow of the tube (Fig. 6P). The PUF used in this sampler (P10z) is more porous than that used in other PUF-based PAS. The motivation for this design was to achieve SRs that greatly exceed those of other PAS. The sampling principle of this so-called “Flow-Through Sampler” (FTS) resembles more that of a pumped active sampler, because it does not rely on the diffusion of the target analytes to the sorbent. The sampled air volume is derived from a calibration involving the measured wind speed. The FTS is particularly useful when trying to measure very low air concentrations in locations where reliable power is difficult to obtain. Examples are the Arctic,176–178 sub-Arctic,179 the Tibetan plateau,176,180 and Antarctica.181 However, the sampler has also been used closer to sources, for example to measure pesticide spray drift.182
Esen introduced a housing for a PUF-based PAS that seeks to minimise the influence of wind.183 A side-by-side comparison with the double-bowl PUF-PAS indicated lower SRs.184
D.3.2 Activated carbon fibre felt sampler.
Another approach to increase the limited uptake capacity of polyurethane foam is to retain the double bowl housing of the PUF-PAS, but use a different sorbent (Table 5). Oono et al. experimented with activated carbon fibre felt to sample fluorotelomer alcohols from the atmosphere (Fig. 7D).185 During a month-long deployment, uptake remained linear and an equivalent air volume of 300 m3 during that period suggest a high SR of ∼10 m3 per day.185 Despite such promising results, no follow-up studies have appeared to be undertaken with this sorbent.
Table 5 Passive air samplers for SVOCs based on granular sorbent material described in the literature. The letters in brackets behind the name refer to Fig. 7
Name |
Passive sampling material |
Housing |
Ref. |
XAD-PAS (A) |
Stainless steel mesh cylinder (10 cm or 20 cm long, 2 cm diameter) filled with styrene–divinylbenzene copolymer |
Inverted cylindrical steel cans |
26
|
High wind version of the XAD-PAS (B) |
Same as the XAD-PAS |
Inverted cylindrical steel cans with wind-buffering baffles at the bottom |
196
|
Activated carbon felt (ACF PAS) (D) |
Activated carbon felt |
Double bowls |
185
|
Polyurethane foam disks impregnated with ground XAD resin (SIP-disk PAS) (C) |
PUF disk (14 cm diameter, 1.35 cm thick) impregnated with styrene-divinylbenzene copolymer powder (0.4 g of XAD-4 resin) |
Double bowls (upper 30 cm diameter and lower 24 cm diameter) |
136
|
Axial PAS (E) |
GFF (2.5 cm diameter) coated with 2.3 mg of Tenax TA |
Puck holder with spacer ring and mesh cover, placed under plastic rain shelter |
200
|
Tenax TA PAS (H) |
Stainless steel mesh cartridge filled with Tenax TA |
Custom made PVC shelter |
201
|
XAD-pocket (F) |
Stainless steel mesh pocket (9 cm long × 5.5 cm wide × 0.2 cm thick; A = 105 cm2; volume = 9.9 cm3) filled with 7 g of XAD-4 (AS = 750 m2 g−1) |
None |
111
|
XAD-PDMS (G) |
PDMS sheet coated with XAD-4 resin |
None |
112
|
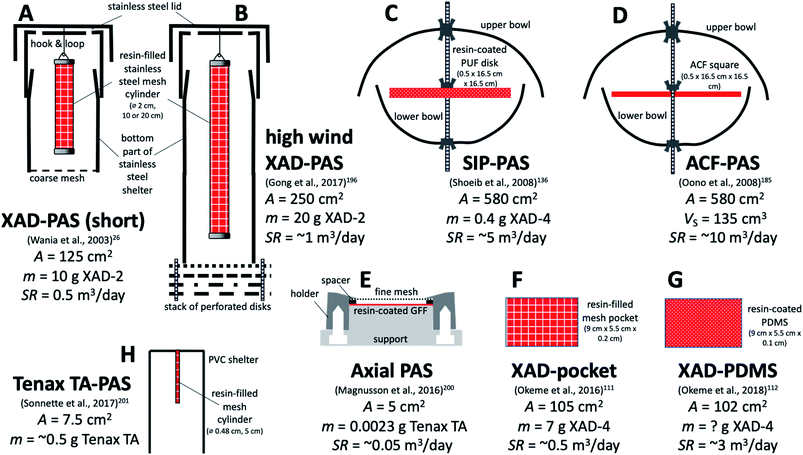 |
| Fig. 7 Various PASs based on porous sorbents other than PUF. Designs (A), (B), and (F) are based on XAD-resin, design (D) on activate carbon felt, and design (H) on Tenax TA. Other designs use resin to enhance the sorptive capacity of other sorbent materials, namely PUF (C), GFF (E) and PDMS (G). A is the sorbent surface area exposed to the air and m is the resin mass. Sampling rates SR range from ∼0.05 m3 per day (design (E)) to 10 m3 per day (design (D)). See Table 5 for more detail. | |
D.3.3 Samplers based on XAD-resin.
The SIP-PAS described in Section D.3.1.2 sought to increase the uptake capacity of the PUF-PAS by impregnating it with XAD-resin (Fig. 7C). Like PUF, XAD, which is a divinylbenzene–styrene copolymer, also has a long history of use as a sorbent in the air sampling of organic compounds.186,187 XAD has a higher uptake capacity for SVOCs than PUF188,189 and also the stability of sorbed compounds during storage of XAD is higher than on PUF.188 In contrast to the non-porous polymers and polyurethane, XAD is an adsorbent and its large uptake capacity is a result of a very high specific surface area (XAD-2: 350 m2 g−1, XAD-4: 750 m2 g−1). Because it is a powder, a container is required that holds the XAD-resin but does not obstruct the diffusion of the target analytes to the sorbent.
Wania et al. introduced a PAS which consists of a XAD-2 resin-filled stainless-steel mesh cylinder, which is suspended in a cylindrical stainless-steel housing (Fig. 7A).26 This sampler has been extensively characterised in terms of the SR of different SVOCs in different climates21,27,28,190 and with respect to the influence of wind on the SR.191 Experiments with different sampler configurations confirmed that the uptake rate is proportional to the surface area of the mesh cylinder192 and both a short (10 cm) and long (20 cm) version of the sampler have been used. The deployment of multiple mesh cylinders in the same housing does not affect the SR, i.e. there is no evidence of a so-called starvation effect.193 Because very high winds have been shown to increase the SR,194,195 Gong et al. introduced a modified housing with wind baffles that reduce the wind speed dependence of the XAD-PAS's SR (Fig. 7B).196
Because of its high uptake capacity (see also section E below), the XAD-PAS is probably the only PAS for SVOCs that can be regarded as a true kinetic sampler. Even for more volatile SVOCs such as the volatile methyl siloxanes (VMS) and the fluorotelomer alcohols (FTOHs), linear uptake was observed for periods as long as three months28 and one year.197 The high uptake capacity also explains, why the XAD-based PAS frequently finds use in extremely remote environments, where limited site accessibility may demand very long deployment periods. Examples are the Tibetan plateau, mountains and polar field camps (for details see Section G.6).
The XAD-PAS is suited for outdoor deployments, whereas its housing may be too bulky for indoor deployments and in fact not necessary. XAD-filled stainless steel mesh bags111 suspended in air have therefore been explored as potential high capacity indoor-PAS (Fig. 7F). Okeme et al. used XAD-4, because of a higher specific surface area (750 m2 g−1).111 Because the XAD-filled mesh bag had a lower SR than a PDMS-based PAS explored at the same time (see Section D.2.3), the authors seemed to favour the PDMS-PAS.111 Later, they also explored the possibility of enhancing the uptake capacity of PDMS by adding XAD-4 powder (Fig. 7G).112
D.3.4 Samplers based on TENAX.
Building on the passive particle sampler by Wagner and Leith,198 Arnoldsson et al. presented an axial diffusion sampler with a GFF (2.5 cm diameter), either bare or coated with 2.3 mg of Tenax TA, as the sorbent (Fig. 7E).199 For analysis, the GFF is folded and placed into a tube, from which the target compounds are thermally desorbed and transferred to a GC-MS. The working hypothesis is that the PAS outfitted with an uncoated GFF takes up particle-sorbed compounds, whereas the Tenax-coated GFF additionally takes up gas-phase substances. By having the GFF facing either up or down, the PAS is expected to distinguish particle-bound substances settling gravitationally or by other means.200 SRs for PAHs and oxygenated PAHs in this sampler have been determined in an exposure chamber receiving diesel exhaust199 and in a field study above an urban roadway.200 While the variability in those SRs was large during the first test,199 the field study yielded more consistent SRs on the order of 0.05 to 0.1 m3 per day.200 The small SR relative to other PASs for SVOCs is mostly due to the small size of the sampler and is compensated for by the quantification technique that delivers the entirety of the sequestered amount onto the gas chromatographic column.200
Sonnette et al. used a Radiello stainless steel mesh cartridge filled with Tenax TA as a PAS sorbent.201 During sampling, they placed the cartridge into a PVC shelter but did not use a Radiello diffusive body to control the diffusion distance (Fig. 7H). They also used thermal desorption onto GC/MS for quantification. While some initial indoor and outdoor deployments are described, no attempts to characterise the samplers have been reported.
D.3.5 Extraction of porous sorbent materials.
Porous sampling sorbents, such as PUF and XAD-resin are typically solvent-extracted using either a Soxhlet-apparatus or pressurised liquid extraction.202 Alternatively, microwave- or ultrasound-assisted techniques have been proposed for extracting organic trace compounds from PUF with the aim to reduce solvent volumes and extraction time. A recently presented micro-scale version of an ultrasound-assisted extraction technique was judged simpler, faster, cheaper and more environmentally friendly than other methods.203
D.3.6 Advantages and limitations of samplers based on porous sorbent materials.
The PASs based on PUF share with the non-porous polymer samplers described in Section D.2, a quite limited uptake capacity and therefore cannot be considered linear samplers for more volatile SVOCs (e.g. lighter PCB congeners during warm deployments204). The same issues related to the uncertainty of the quantitative interpretation (Section D.2.5) apply therefore also to the PUF-PAS. This is discussed in more depth in Section F.3 below.
The kinetic samplers (XAD-PAS, SIP-PAS), on the other hand, have the advantage that no knowledge of KSG is required, and that long deployment times or sampling of relatively volatile SVOCs are possible without worrying about approaching equilibrium. They are also the only samplers that give air concentrations that are truly the average over the entire length of deployment. This is not the case when sampling in the curvi-linear uptake range while the air concentrations vary with time.
A disadvantage of the PASs with porous sorbents is that the assumption of air-side resistance is not valid. This has been shown for both the XAD-PAS and the PUF-PAS, by demonstrating a concentration gradient within the sorbent205 and through a critical evaluation of reported KPUF–G values.206 This also implies that the equations in Section B for interpreting the uptake of SVOCs in the sorbent cannot be applied uncritically. Instead, more complex uptake models are required for a quantitative interpretation of SRs (see Section F.5 below).21,207
Despite the use of wind-sheltering housings, the SRs of PASs using the double bowl design (PUF-PAS, SIP-PAS, carbon felt fibre-PAS and others) are quite substantially impacted by wind, especially, but not only, at high wind speeds.128 The wind effect on the shelter used for the XAD-PAS is much smaller,26,191 especially in the version by Gong et al.196 In the balancing of the conflicting needs for a large vs. a tightly controlled SR (Section C.1), the double bowl design samplers lean towards higher, yet more variable SRs and the XAD-PAS leans towards lower, but less wind-dependent SRs.
Among the strategies to account for the effect of wind on SR is the use of DCs. It is important to realise that the use of DCs requires the assumption of air-side resistance to be valid (see Section F.4 below). DCs therefore have not been used in the XAD-PAS. Even though there is also evidence that this assumption is violated in the PUF-PAS,205 DCs are routinely employed in the PUF-PAS.127,208,209
By normalizing the SR to the sequestered amount of a compound whose concentration can be assumed to be uniform in space,194 it is possible to account for differences in the wind exposure of the XAD-PAS. HCB can often be used if it can be assured that there are no HCB sources in the vicinity of a sampling site.195,210
XAD-resin is quite expensive and it can be difficult to handle, especially when electrostatic effects occur. Coating XAD-resin onto PUF disks when making SIPs in particular, can be laborious and difficult and can easily lead to sorbent contamination. On the other hand, high capacity samplers do not require DCs, which is a considerable expense associated when seeking to quantitatively interpret results of PASs with limited uptake capacity in the curvi-linear uptake regions.
While a surprisingly large number of PASs for SVOCs have been introduced, they are for the most part described in a stand-alone paper with no follow-up studies seeking to characterise, test or apply the sampler. Among the PASs discussed in section D, only the PUF-PAS and XAD-PAS, and to a smaller extent also the SIP-PAS and PE-based samplers have been thoroughly characterised and are used most extensively. Therefore, the remainder of the review will largely focus on those PAS designs.
E. The capacity of different PAS sorbents for SVOCs
As discussed in Section B above, knowledge of the KSG at the temperature of deployment is essential for using equilibrium PASs as well as for interpreting measurements in the curvi-linear part of a PAS's uptake curve. Even if a sampler is operated in the kinetic range, knowledge of the KSG is useful to estimate tlinear.
We defined KSG in Section B.1 as the equilibrium sorption coefficients between the gas-phase and the PAS sorbent, which is the ratio of the concentration of a chemical in the sorbent CS (in units of mol m−3 sorbent or mol m−2 adsorbent) and in the gas-phase CG (in units of mol m−3 gas) if the chemical has reached equilibrium between the two phases:
|  | (9) |
K
SG thus has units of m3 gas per m3 absorbent (or m3 gas per m2 adsorbent). As KSG is an equilibrium constant, we can use a van't Hoff type equation to relate it to temperature:
| 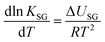 | (10) |
where
T is absolute temperature in K,
R is the ideal gas constant, and Δ
USG is the internal energy of transfer between sorbent and gas-phase in J mol
−1. Note that we use the internal energy Δ
USG instead of the enthalpy of phase transfer Δ
HSG, because in the PAS community the abundance of chemical in the gas-phase is typically expressed in volumetric air concentrations and not in partial pressure. If
KSG is expressed in units of mol per m
3 sorbent per Pa or mol per m
2 adsorbent per Pa, the use of Δ
HSG would be appropriate. The two are easily derived from each other:
If ΔUSG is assumed to be a constant, the integration of eqn (10) yields:
| 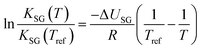 | (12) |
With eqn (12) it is possible to calculate KSG at a temperature T, if ΔUSG and KSG at a reference temperature are known.
This section reviews both the experimental and theoretical studies that have been conducted to determine KSG and ΔUSG for different types of sorbents used in passive air sampling. When referring to the KSG and ΔUSG for a specific sorbent, we substitute S with the sorbent acronym. For example, KPUF–G and ΔUPUF–G refer to the sorption coefficients and internal energy of phase transfer between PUF and the gas phase, respectively.
E.1 Experimentally determined uptake capacities of PAS
E.1.1 Studies reporting on KPE–G.
While there appear to have been no experiments yet that sought to measure the equilibrium sorption coefficient between LDPE and the gas-phase (KPE–G) and its temperature dependence under controlled conditions,124 such data have been repeatedly obtained from field data. In early work, Bartkow et al.67 and Kennedy et al.97 derived such sorption coefficients for PAHs from field data. Khairy and Lohmann73–75 derived KPE–G for PAHs, OCPs, PCBs and PBDEs from deployments in Alexandria, Egypt, and Providence, RI, USA, using concentrations measured in the atmosphere and concentrations in the LDPE-PAS, mathematically adjusted for equilibrium. However, partitioning data derived from field data inevitably apply to the temperature conditions during deployment and need to be adjusted. It should be possible to derive values of KPE–G through a thermodynamic triangle with water–PE equilibrium partition coefficients KPE–W and Henry's law constants KG–W, because uptake in PE is a bulk phase sorption process and is not influenced by the presence of water.124 Measured values of KPE–W are much more common, due to the wide-spread use of PE in passive water sampling.
E.1.2 Studies reporting on KPDMS–G.
Equilibrium sorption coefficients between PDMS and the gas-phase KPDMS–G have been measured for a fairly large number of organic compounds, because of the use of PDMS in SPME fibres. Sprunger et al. have compiled KPDMS–G values measured for 64 solutes at room temperature by a number of research groups.211 Gilbert et al. showed that polymer–polymer partition coefficients can be used to derive KSG values, e.g. by combining KPE–G with KPDMS–PE partition coefficients in a thermodynamic triangle to calculate KPDMS–G values for PCB congeners.122 Recently, Okeme et al.212 and Tromp et al.213 have greatly expanded the set of measured KPDMS–G values, either from isothermal retention volumes on a DB-1 column that has PDMS as a stationary phase212 or by using a calibration involving an exposure vessel with controlled temperature and wind speed.213
E.1.3 Studies reporting on KPUF–G.
Shoeib and Harner derived KPUF–G for several lighter PCB congeners by assuming that they had reached equilibrium with laboratory air after extended exposure, and solving eqn (6A) for KSG and using measured concentrations in air and PUF.24 Zhao et al. reported KPUF–G for eight aromatic VOCs at 21 °C.214 Kamprad and Goss determined KPUF–G for 103 relatively volatile organic compounds with diverse functionalities by measuring the chromatographic retention volume of analytes on a column filled with PUF.215 Bidleman et al. determined KPUF–G by equilibrating PUF with ambient air at different temperatures in the field for HCB, α-hexachlorocyclohexane (α-HCH) and two brominated anisoles.216 More recently, Harner and co-workers have used dedicated laboratory experiments using a generator column approach to determine KPUF–G as a function of temperature for parent PAHs and alkylated PAHs,217–219 pentachlorobenzene (PeCBz), HCB, α- and γ-HCH,219 four OPFRs, six novel brominated flame retardants (BFRs), HCBD and pentachloroanisole (PCA).220 Tromp et al.213 measured KPUF–G for 98 SVOCs at 20.7 °C during a 160 day-long calibration study in an exposure chamber.
We should note that for a porous sorbent such as PUF, the KPUF–G with units of m3 gas per m3 absorbent, as it is reported in many of the studies mentioned here,213,217–220 can be confusing as the volume of the absorbent could refer to solid polyurethane or to foam. To avoid misunderstandings, it would be preferable to report the KPUF–G with units of m3 gas per g absorbent215 or to include information on whether the volume of the foam or the polymer was used.214 No work has been reported that explored whether KPUF–G varies with the type or the density of the PUF, or whether variables such as relative humidity have an influence on the sorptive capacity of PUF.
Comparing different prediction methods for KPUF–G, Okeme et al.206 noted that some experimental KPUF–G are likely erroneous, because the compounds did not achieve equilibrium between all of the exposed PUF and the gas-phase.
E.1.4 Studies reporting on KXAD–G.
Pankow et al. measured and compiled gas chromatographic retention volumes of a whole range of VOCs, including aliphatics, chlorinated aliphatics, monoaromatic (BTEX, halogenated benzenes, phenols), ketones, amines, alcohols, and carboxylic acids at 20 °C using XAD as a stationary phase.221 For a subset of these substances, they also reported retention volumes at temperatures between 40 °C and 90 °C. These retention volumes allow for the calculation of equilibrium sorption coefficients between XAD and the gas-phase KXAD–G. Shen et al. used a headspace technique to directly measure the sorption of 1,2-dichlorobenzene, 1,2,4,5-tetrachlorobenzene and HCB onto XAD-2 resin,222 whereas Hayward et al. applied inverse GC involving a column filled with XAD-resin to determine KXAD–G for a selection of 52 diverse organic compounds as a function of temperature.223 A limitation of these studies is that they often focus on VOCs and not on the SVOCs that are typically sampled with the XAD-PAS. This is because the KXAD–G for SVOCs are typically too large to be amenable to reliable measurements. Sometimes, it is possible to infer minimum values of KXAD–G from a field calibration experiment, even if equilibrium was not reached during the longest deployment. Krogseth et al., for example estimated a lower limit of 6.5 for the log
KXAD–G of a number of cyclical and linear VMS.28
E.2 Prediction techniques for the uptake capacity of different PAS sorbents
E.2.1 Single-parameter linear free energy relationships.
Because measurements of KSG are generally only available for a few substances and at a limited number of temperatures, there has been interest in deriving techniques for predicting KSG. Most often they take the form of single parameter linear free energy relationships that seek to linearly regress log
KSG with the logarithm of either liquid-state vapour pressure or the octanol–air equilibrium partition coefficient (KOA) of a compound. For example, Pankow et al. regressed the retention volumes on XAD resin against vapour pressure.221 Shoeib and Harner regressed the measured log
KPUF–G for eight PCB congeners against log
KOA,24 and Bartkow et al. regressed the KPE–G of several PAHs against log
KOA.67,97 There are many more equations of this type reported in the literature (e.g. see compilation for KPUF–G in Okeme et al.206). However, it is now well established that such relationships tend to be only valid for groups of closely related substances that interact with the sorbent with the same type of intermolecular interactions.224 As such, their use might be defensible for a group of non-polar organic compounds, but they clearly cannot be expected to provide reliable estimates for substances that engage in polar interactions.220
E.2.2 Poly-parameter linear free energy relationships.
More widely applicable are so-called poly-parameter linear free energy relationships (ppLFER), which are multi-variate linear regressions of measured sorption constants against a series of solute descriptors that quantify a compound's ability to undergo various types of intermolecular interactions such as hydrogen bonding.225 Kamprad and Goss,215 Sprunger et al.211 and Hayward et al.223 used their respective data sets to derive such relationships for KPUF–G, KPDMS–G and KXAD–G, respectively. Whereas Hayward et al. presented such equations for KXAD–G at different temperatures,223 Kamprad and Goss derived a separate equation for the enthalpy of PUF–gas-phase transfer ΔHPUF–G.215 Uber et al. presented ppLFERs for KPE–W,226 including one for LDPE based on the experimental data by Choi et al.227 If combined with a ppLFER for air–water partitioning, this should allow for the derivation of a predictive equation for KPE–G. Finally, there are such relationships for solute uptake in other sorbents from the gas-phase. These sorbents have found or could conceivably find use in PAS, such as Chromosorb, Tenax and Porapak.228
While experimental solute descriptors for frequently measured SVOCs, such as the PCBs and PAHs are readily available, there has in recent years also been a concerted effort to determine experimental solute descriptors for other environmentally relevant SVOCs.229–231 Even if no solute descriptors are available, they can now be predicted quite well for many SVOCs using the quantitative structure property relationships implemented in the UFZ LSER website.232 The ppLFER-based prediction of KPUF–G, KPDMS–G, KXAD–G and KPE–G values is now feasible for a wide range of substances.206
E.2.3 Predictions based on quantum-chemical calculations and statistical thermodynamics.
Whereas the single- and poly-parameter linear free energy relationships require experimental data for calibration, prediction techniques based on quantum-chemical calculations and statistical thermodynamics can predict equilibrium sorption properties directly from molecular structure. In particular, Goss demonstrated that the commercial software COSMOtherm can be used to predict partitioning into various polymer matrices by treating the polymer as a “liquid of monomer repeat units that can move independently from each other.”233 The method was successful in predicting the KPDMS–G at 25 °C and the KPUF–G at 15 °C and 95 °C.233 Loschen and Klamt later improved the approach “by incorporation of polymer-specific entropic contributions due to free volume effects”.234 PDMS can be represented as liquid oligomer (hexamethyldisiloxane) when using COSMOtherm to predict KPDMS–G,212 but this approach greatly overpredicts the measured KPDMS–G, with an average difference in excess of 2 orders of magnitude. Smaller overpredictions, generally less than an order of magnitude, were achieved when predicting KPUF–G for various compounds at different temperature, representing polyurethane as “a simple oligomeric 1:1 condensed pair of 2,4-toluene-diisocyanide and glycerol with ethyl and acetate end-caps”. Parnis et al.217,218 used COSMOtherm to predict KPUF–G and its temperature dependence for substituted PAHs. Chen et al. noted that COSMOtherm was able to predict the KXAD–G at different temperatures with a root mean square error of 0.35,235 when compared with the experimental values by Hayward et al.223
E.3 Comparison of uptake capacity of different PASs for HCB
The overall uptake capacity of a PAS is not only dependent on the sorption coefficient between the PAS sorbent and the gas-phase, but also on the amount of the sorbent in the PAS, namely by the products KSGVS and KSGAS for PASs based on absorbents and adsorbents, respectively. In order to compare the uptake capacity of different commonly used PAS, we estimated their KSGVS or KSGAS values for HCB at approximately 20 °C (Table 6). Not only is HCB a relatively volatile SVOC, for which tlinear could be quite short, but experimental KSG values exist for most sorbents relevant for PAS.
Table 6 Estimation of the total uptake capacity of different PASs for hexachlorobenzene at 20 °C
Name |
K
SG
|
Mass of sorbent |
A
S or VS |
Uptake capacity |
Adsorption coefficient has units of length (m is metre).
|
PDMS-PAS111 |
K
PDMS–G = 105.9 (ref. 212) |
|
∼10−5 m3 |
8 m3 |
LDPE-PAS67 |
K
PE–G = 106.8 (ref. 74) |
|
∼10−6 m3 |
6 m3 |
PUF-PAS24 |
K
PUF–G = 107.1 (ref. 213, 216 and 219) |
4.4 g of PUF (10−6 m3 g−1) |
4.4 × 10−6 m3 |
55 m3 |
SIP-PAS136 |
K
XAD–G = ∼30 m (ref. 222)a |
0.435 g of XAD-4 (750 m2 g−1) |
315 m2 |
9000 m3 |
XAD-PAS26 |
K
XAD–G = ∼30 m (ref. 222)a |
Long: 20 g of XAD-2 |
7000 m2 |
200 000 m3 |
Short: 10 g of XAD-2 (350 m2 g−1) |
3500 m2 |
100 000 m3 |
The calculation confirms that in the SIP, the addition of 10% by mass of XAD-4 resin results in a more than 100-fold increase in the overall sorption capacity of the PUF disk for HCB. Overall, this calculation reveals that the sorption capacity of different PAS for one compound can vary by five orders of magnitude. This difference would be even larger if the SPME-PAS104 were to be included in the comparison, as its VS is tiny.
The estimation suggests that the XAD-PAS has a theoretical uptake capacity that is four orders of magnitudes higher than that of most other PASs for SVOCs. The comparison is somewhat misleading, as not all of the sorbent in a PAS necessarily participates in the uptake. For example, most of the XAD in the XAD-PAS is not accessible to the compounds from the atmosphere because of a notable transport resistance within the passive sampling medium.205,207 However, this is partly also true for the other sorbents.205,206 Nevertheless, the comparison illustrates why the XAD-PAS is the closest to a kinetic PAS for SVOCs. It also explains why the XAD-PAS generally does not require the consideration of curvi-linear uptake, even for more volatile SVOCs, and is more suited for long deployment periods than other PAS.
F. The sampling rates of different types of PASs
F.1 Empirical determinations of sampling rates
F.1.1 Deriving a sampling rate from a calibration experiment.
Rearranging eqn (5) we obtain: |  | (13) |
which implies that we can derive the SR of chemical in a PAS by dividing the amount of the chemical ΔmS taken up during the time period Δt by the product of the gas-phase concentration CG and this time period. It thus requires CG during the time of PAS deployment to be determined by another method, most commonly an active sampling method. This empirically derived SRe only corresponds to the inherent SR, if the PAS is operating in the linear uptake period. Quite frequently, SRes are indeed determined with eqn (13) and a single PAS deployment.210,236,237 More commonly, however, SRs of SVOCs in PAS are determined from the slope of an uptake curve.26–28 Assembling such a curve requires the deployment of multiple samplers at the same time and their staggered retrieval after different lengths of time Δt. Being based on multiple PASs deployed for variable lengths of time, such calibrations tend to give more reliable SRs and allow for a recording of the uptake curve, which in turn can provide information on the approach to equilibrium.
In order to linearise an uptake curve during a calibration that experiences variations in CG, one either plots the amount found in the PASs, mS, against the product of CG and Δt (see Fig. 8) or, equivalently plots mS/CG, i.e. the “equivalent sampling volume”, against the time of deployment. In either case, the slope of the resulting curve corresponds to the SR.
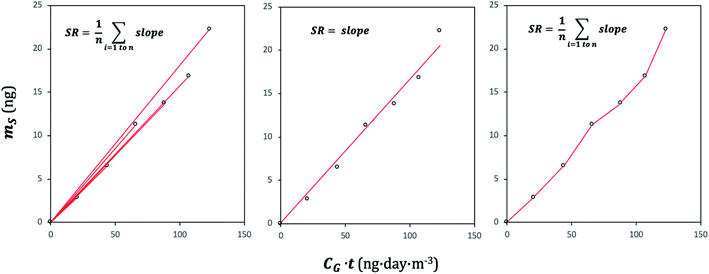 |
| Fig. 8 Illustration of the three different methods of deriving a sampling rate SR from a calibration experiment involving n PASs deployed at the same time and retrieved after variable deployment periods (n = 6 in the displayed example) as discussed in ref. 190. In each case, the amount taken up in a PAS mS is plotted against the product of the length of deployment t and the gas-phase concentration during the time of deployment CG. The SR is derived either (i) as the average of the SRs calculated for each of the n samplers, (ii) from a linear regression involving all n samplers, or (iii) from the increase in mS between subsequently retrieved samplers. Only the last method allows for SR to be time-variant. | |
Different methods can be applied to derive a SR from such a plot (Fig. 8).190 Each PAS can be treated as a single calibration experiment yielding an empirical SRe according to eqn (13). The overall SRe is then the average of the SRe obtained for each PAS. Alternatively, one performs a least square regression on the linearised uptake curve and derives the SRe from its slope. Finally, it is also possible to derive an SRe for each time period between subsequent PAS retrievals, by using the increase in the mS in PASs from one retrieval to the next. The latter approach is advisable if the SR can be expected to vary during the deployment periods, e.g. if different deployment periods experienced different temperatures or wind speeds. Generally, a linear regression gives the most reliable SRe. Longer deployments tend to have a stronger impact on the slope of the regression than short deployments, which is often desirable as the larger mS in PASs deployed for a longer length of time can often be quantified more confidently.
For PASs that also take up particles, CG in eqn (13) can be replaced with the concentration of both gaseous and particle-bound SVOCs or of only the particle-bound substance, and a calibration then will yield SRs for the total SVOCs in the atmosphere or for the particle-bound substance. See Section F.6 for in-depth discussion of particle uptake in PAS.
F.1.2 Outdoor calibration experiments for PAS.
Tables are used to summarise outdoor calibrations studies that have been conducted for the PUF-PAS (Table 7), XAD-PAS (Table 8), LDPE-PAS (Table 9), SIP-PAS (Table 10) and SPMD (Table 11). The PUF-PAS has been calibrated for more different SVOCs than any other PAS. This includes OCPs, CUPs, PCBs, parent PAHs, alkylated PAHs, PBDEs, polychlorinated dibenzo-p-dioxins and -furans (PCDD/Fs), polychlorinated naphthalenes (PCNs), polycyclic musk compounds, BFRs and a number of perfluorinated alkyl substances (PFAS) (Table 7). The XAD-PAS has been calibrated for OCPs, CUPs, PCBs, PAHs, neutral PFAS and VMS (Table 8). The number of analytes for which empirical SRs have been determined in other PASs is much smaller (Tables 9–11).
Table 7 Outdoor studies calibrating the PUF-PAS for semi-volatile organic compounds
Sampler |
Site/type/climate |
Period |
SVOCs |
Active sampling |
Passive sampling |
Ref. |
PUF-PAS |
Regina, Saskatchewan Canada, rural, temperate |
Jun. to Jul. and Jul. to Aug. 2003 |
Dicamba, γ-HCH, 2,4-D, triallate, bromoxynil, MCPA |
Eight 168 hour Hivol samples, borosilicate microfibre filter and PUF-XAD-PUF sandwich |
8 samples (duplicates at 10 m and 30 m during two periods of 28 days) |
241
|
PUF-PAS |
Lancaster, UK, semi-rural temperate |
Apr. to Jun. 2006 |
HCB, HCHs, DDTs, chlordanes, PCBs |
Eight 168 hour HiVol samples using GFF/PUF-PUF |
23 samples (1, 2, 3, 4, 6, 8 weeks) (triplicates during first six weeks, 8 samples at week 8) |
135
|
PBDE |
242
|
PUF-PAS (low & high density) |
|
Oct. to Dec. 2007 |
PCBs, PBDEs |
Thirteen 168 hour samples using GFF/PUF-PUF |
8 triplicated samples (0.5, 1, 2, 3, 4, 6, 8, 12 weeks) |
243
|
PUF-PAS |
Košetice, Czech Republic, sub-urban, temperate |
Oct. 2003 to Jan. 2007 (3 year period) |
PeCB, HCB, HCHs, DDTs, PCBs, PAHs |
Hundred-sixty-eight 24 hour HiVol samples using GFF/PUF-PUF |
42 samples (consecutive periods of 28 days each) |
129
|
PUF-PAS |
Barcelona, Spain, 3 urban/industrial sites, 1 semi-rural site, Mediterranean |
Mar. to Jun. 2005 |
PCDD/Fs, PCBs, PCNs |
HiVol samples using quartz fibre filter/PUF |
4 samples, 3 months |
236
|
PUF-PAS |
Iowa, United States, semi-urban, temperate |
Aug. 2006 to Oct. 2006 |
PCBs |
Three 8 hour HiVol samples using GFF/XAD-2 |
3 quadruplicated samples (21, 28, 46 days) |
209
|
PUF-PAS |
Singapore, urban/industrial, tropical |
Apr. to Jun. 2008 |
PAHs, OCPs |
Twelve 48 hour HiVol samples using quartz fibre filter/PUF |
6 duplicated samples (10, 21, 32, 43, 60, 68 days) |
238
|
PUF-PAS |
Toronto, Ontario Canada, semi-urban, temperate |
Feb. to Jun., 2008 |
PAHs, PCBs, PBDEs, musks (ADBI, ATII, HHCB, AHTN) |
Five 600 hour LoVol samples using GFF/PUF-PUF |
10 samples (0, 25, 50, 75, 90, 100, 125 days), 3 duplicates |
25
|
PUF-PAS |
Toronto, Ontario Canada, semi-urban, temperate |
Mar. to Oct. 2010 |
PFAS (PFBS, PFOS, PFDS) |
Thirty-eight 24 hour HiVol samples using GFF/PUF-XAD-2-PUF |
13 samples (7, 21, 28, 42, 56, 84, 112, 140, 168, 197 days), 3 duplicates |
137
|
VMS not detected |
149
|
PUF-PAS modified |
Bursa, Turkey, urban, Mediterranean |
Jun. 2008 to Jun. 2009 |
PCBs, OCPs |
Thirty 24 hour samples using GFF/PUF-PUF |
3 samples (10 days each) |
183
|
PUF-PAS |
Yakima Valley, Washington USA, rural/agricultural, temperate |
Mar. to Apr. and Jun. to Aug. 2011 |
Chlorpyrifos, azinphos-methyl, chlorpyrifos-oxon, azinphos methyl-oxon |
Five 24 hour active air samples using PUF |
30 days |
244
|
PUF-PAS |
Brno, Czech Republic, urban-background, temperate |
Sep. 2010 |
PCBs, PeCB, HCB, DDTs, PBDEs, PAHs, PCDD/Fs, BFRs (ATE, TBECH, TBCO, p-TBX, PBEB, PBT, DPTE, HBB, EHTBB, BTBPE, DBDPE) |
Twelve 168 hour LoVol samples using GFF/PUF-PUF |
12 triplicated samples (1, 2, 3, 4, 5, 6, 7, 8, 9, 10, 11, 12 weeks) |
245
|
PUF-PAS |
Suwon, and Ansan, Gyeonggi-do, South Korea, urban and industrial areas, temperate |
Feb. 2010 to Feb. 2011 |
PCDD/Fs, PCBs |
Suwon: six 168 hour HiVol samples using GFF/PUF-PUF (28%) |
Suwon: 8 samples; (63, 37, 73, 79, 56, 62 days) |
246
|
Anasan: twenty four 168 hours HiVol samples using GFF/PUF-PUF |
Anasan: 3 samples (51, 56, 66 days) |
PUF-PAS |
3 locations in the Alberta Oilsands region, boreal, industrial |
Dec. 2010 to Jan 2011 |
PAHs, alkylated PAHs, parent and alkylated dibenzo-thiophenes |
21 HiVol samples |
1 sample of 60 days at 3 sites |
247
|
PUF-PAS |
Bursa, Turkey |
Feb. 2013 to Feb. 2014 |
PAHs |
Active sampling (2 days), unknown number of samples |
4 seasonal calibrations, each with 3 to 5 samples (10, 20, 30, 40, 60 days), replication of 10 day samples |
248
|
PCBs |
249
|
PUF-PAS |
Toronto, Ontario, suburban, temperate |
Mar. to Oct. 2010 |
OPFRs |
Twenty-one 24 hour HiVol samples using GFF/PUF-XAD |
7, 21, 28, 42, 56, 84, 112, 140, 168, 197 days, duplication of 3 samples |
250
|
PUF-PAS |
Guangzhou, China, urban, subtropical |
Jul. to Oct. 2016 |
Levoglucosan, galactosan, mannosan |
Sixteen active air samples |
5 triplicated samples (16, 30, 50, 73, 92 days) |
251
|
PUF-PAS modified |
Dalian, China, urban, temperate |
Sep. 2016 to May 2017 |
SCCPs |
Sixteen active air samples |
6 duplicated samples (1, 2, 3, 4, 6, 8 months) |
252
|
Table 8 Outdoor studies calibrating the XAD-PAS for semi-volatile organic compounds
Sampler |
Site/type/climate |
Period |
SVOCs |
Active sampling |
Passive sampling |
Ref. |
XAD-PAS (20 cm sampler) |
Point Petre, Ontario, Canada, background, temperate |
May 2000 to May 2001 |
HCB, α-HCH |
Data taken from ongoing active monitoring |
7 duplicated samples (1, 2, 4, 6, 8, 10, 12 months) |
26
|
Burnt Island, Ontario, Canada, background, temperate |
Jun. 2000 to Jun. 2001 |
HCB, α-HCH, γ-HCH, dieldrin, trans-nonachlor |
Alert, Nunavut, Canada background, polar |
Aug. 2000 to Aug. 2001 |
HCB, α-HCH, γ-HCH, endosulfan I |
XAD-PAS (20 cm sampler) |
San Antonio de Belen, Costa Rica, suburban, tropics |
Oct. 2005 to Oct. 2006 |
HCB, chlorothalonil, chlorpyrifos, dieldrin, pendimethalin, endosulfans, chlordanes |
Nine 24 hour HiVol samples using GFF/PUF-XAD-2-PUF |
5 duplicate samples (4, 6, 8, 10 12 months) |
27
|
8:2 FTOH, 10:2 FTOH, MeFOSA, EtFOSA, MeFOSE |
197
|
XAD-PAS (10 cm sampler) |
Maun, Botswana, semi-urban, semi-arid |
Passive: May 2006 to May 2007, active: Jul. 2006 to Aug. 2007 |
HCHs, HCB, chlorothalonil, chlordanes, endosulfan I, endosulfan II, endosulfan sulfate |
Twenty-seven 24 hour HiVol samples using GFF/PUF-XAD-2-PUF |
1 duplicated sample (1 year) |
210
|
XAD-PAS (10 cm sampler) |
Egbert, Ontario Canada, rural, temperate |
Mar. 2006 to Feb. 2007 |
PAHs, PCBs |
Twenty-one 336 hour LoVol samples using PUF-XAD-2-PUF |
5 duplicate samples (56, 122, 185, 241, 365 days) |
21
|
PFAS (8:2 FTOH, 10:2 FTOH, MeFOSA, EtFOSA, MeFOSE) |
197
|
Chlorthalonil, endosulfan I, endosulfan II, endosulfan sulfate, dacthal, disulfoton, atrazine, alachlor, metolachlor, HCB, HCHs, chlordanes |
190
|
XAD-PAS (10 cm sampler) |
Toronto, Ontario Canada, suburban, temperate |
Mar. to Jun. 2012 |
VMS (D4, D5, MDM, MD2M, MD3M) |
Forty-two 57 hour LoVol samples using SPE (PE cartridge with 120 mg of ENV + resin) |
8 duplicate samples (7, 14, 21, 28, 42, 60, 77, 98 days) |
28
|
XAD-PAS (XAD-4 resin) |
1 urban site and 1 rural site in Minnesota, USA, temperate |
Jun. 2013 to Jun. 2015 |
45 PAHs |
72 hour HiVol samples once every 12 days |
Consecutive 3 months samples |
253
|
Table 9 Outdoor studies calibrating the LDPE-PAS for semi-volatile organic compounds
Sampler |
Site/type/climate |
Period |
SVOCs |
Active sampling |
Passive sampling |
Ref. |
LDPE (film thickness 200 to 100 um) |
Brisbane, Queensland, Australia, semi-urban, tropics |
Apr. 2002 to Jun. 2003 |
PAHs |
One 720 hour, one 1440 hour and one 2160 hour HiVol sample using GFF/two XAD-2 cartridges |
3 duplicated samples (30, 50, 90 days) |
67
|
LDPE sampler |
Providence, Rhode Island USA, semi-urban, temperate |
Nov. to Dec., 2012 |
HCHs, HCB, heptachlors, chlordanes, endosulfans, DDTs, PCBs, PBDEs |
Six 72 and 96 hour samples using GFF/PUF-PUF |
6 duplicated samples (3, 7, 10, 14, 17, 21 days) |
75
|
Table 10 Outdoor studies calibrating the SIP-PAS for semi-volatile organic compounds
Sampler |
Site/type/climate |
Period |
SVOCs |
Active sampling |
Passive sampling |
Ref. |
SIP-PAS |
Lancaster, UK, semi-rural, temperate |
May 2009 to Apr. 2010 |
PCBs, PBDEs |
Twenty-five 336 hour HiVol samples using GFF/PUF-PUF |
22 and more (duplicate or triplicate); 4, 7, 14, 21, 28, 35, 42, 112, 182, 266, 350 days |
150
|
SIP-PAS |
Toronto, Ontario, Canada, semi-urban, temperate |
Mar. to Oct. 2010 |
PFAS (neutral & ionic) |
Thirty-eight 24 hour HiVol samples using GFF/PUF-XAD-2-PUF |
13 samples (7, 21, 28, 42, 56, 84, 112, 140, 168, 197 days), 3 duplicates |
137
|
VMS (D3, D4, D5, D6, MDM, MD2M, MD3M) |
149
|
SIP-PAS |
Toronto, Ontario, Canada, suburban, temperate |
Mar. to Oct. 2010 |
OPFRs |
Twenty-one 24 hour HiVol samples using GFF/PUF-XAD |
7, 21, 28, 42, 56, 84, 112, 140, 168, 197 days, duplication of 3 samples |
250
|
Table 11 Outdoor studies calibrating SPMD-PAS for semi-volatile organic compounds
Sampler |
Site/type/climate |
Period |
SVOCs |
Active sampling |
Passive sampling |
Ref. |
SPMD |
Lancaster, UK, semi-urban, temperate |
Summer, 1995 |
PCBs |
Thirteen 168 hour HiVol samples using GFF/PUF-PUF |
Six duplicated samples (15, 29, 45, 59, 71, 88 days) |
38
|
Winter, 1995/1996 |
Twelve 168 hour HiVol samples using GFF/PUF-PUF |
Five duplicated samples (14, 26, 39, 61, 84 days) |
SPMD |
Lancaster region, UK, urban/industrial/coastal, temperate |
Passive: Nov. to Dec. 1999 |
PCDD/Fs |
Fifteen 72 to 96 hours HiVol samples using GFF/PUF |
Two samples (42 to 45 days) |
39
|
Active: Jan. to Feb. 1998 |
SPMD |
Brisbane, Queensland, Australia, urban, tropics |
Apr. 2002 |
PAHs |
One 32 day HiVol samples using GFF/XAD cartridge |
One quadruplicated sample (32 days) |
254
|
SPMD |
Guangzhou, Guangdong, China, urban, subtropics |
Apr. 2001 to Mar. 2002 |
PAHs |
Fifty-two 24-hour HiVol samples using GFF/PUF-PUF |
Four consecutive 3 month periods |
255
|
SPMD |
Weissfluhjoch, Switzerland |
May 2005 to Nov. 2006 |
HCHs, chlordanes, endosulfan, dieldrin |
Three 4 months LoVol samples using XAD cartridge |
Three consecutive sampling periods (190, 210, 400 days) |
60
|
Zugspitze, Germany |
Sonnblick, Austria, background, alpine |
Air concentrations during the calibrations were determined either with sporadic high volume (HiVol) or more continuous low volume (LoVol) samplers. The fraction of time that is covered by the active sampling ranges from a few percent to 100%.
Despite a large number of studies, the PUF-PAS has been calibrated mostly in temperate climates, with one calibration study in tropical Singapore.238 The outdoor calibration studies for the XAD-PAS also extend to locations in tropical,27,197 subtropical210 and polar26 locations. The longest deployment period in most of the PUF-PAS calibrations was three months. Reflecting the higher uptake capacity of the XAD-PAS and therefore longer tlinear, most of the calibrations involving the XAD-PAS lasted one year, except for the one for VMS, which lasted three months.28
F.1.3 Indoor calibration experiments for PASs.
Table 12 summarises the indoor calibrations studies that have been conducted for PUF-PAS. The PUF-PAS has been calibrated for PCBs, PAHs, PBDEs and a number of PFAS. Indoor calibrations have also been performed for the uptake of PCBs in the XAD-PAS191,192,205 and neutral PFASs239 and phthalates and PBDEs240 in the SIP-PAS.
Table 12 Indoor studies calibrating the PUF-PAS for semi-volatile organic compounds
Sampler |
Site |
SVOCs |
Active sampling |
Passive sampling |
Ref. |
PUF- PAS |
8 offices and laboratories, Ontario, Canada |
PBDEs |
LoVol samples using PUF-PUF |
17–20 days |
161
|
MeFOSE, EtFOSE, EtFOSA, MeFOSEA |
162
|
PUF-PAS |
Office, Birmingham, United Kingdom |
PCBs |
Five 240 hours LoVol samples using GFF/PUF-PUF |
Eight samples (10, 20, 30, 40, 50 days), 2 replicates |
134
|
PUF-PAS |
2 laboratories, Iowa, United States |
PCBs |
Three 8 hours HiVol samples using GFF/XAD-2 |
Three quadruplicated samples (21, 28, 46 days) |
209
|
PUF-PAS (with GFF for particles) |
Office, United Kingdom |
TBBP-A, α-HBCD, β-HBCD, γ-HBCD, PBDEs |
Five 240 hours LoVol samples (triplicate) using GFF/PUF-PUF |
Eight samples (10, 20, 30, 40, 50 days), 2 replicates |
156 and 256
|
PUF-PAS (low protection chamber) |
Alloy factory (4 sampling points), Sweden |
PAHs |
Two 168 hour LoVol samples using GFF/PUF-PUF at each sampling point |
4 triplicated samples, 2 weeks |
257
|
PUF-PAS |
2 homes in each of Seoul, Ansan/Shiheung, Daegu, Korea |
PFAS (1MeFOSE, EtFOSE) |
One 480 hours LoVol sample using PUF-XAD-PUF at each site |
7 samples (20 days) |
239
|
PUF-PAS |
5 living room, Korea |
8:2 FTOH, 10:2 FTOH, EtFOSA, MeFOSE, EtFOSE, MeFOSEA |
504 hour LoVol samples using GFF/PUF-XAD-PUF sandwich (unknown sample number) |
21 days (unknown sample number) |
258
|
PUF-PAS (plug in cylindrical housing) |
University office, Toronto, Canada |
PCBs |
Six 720 hour LoVol samples using PUF-XAD-PUF |
6 duplicated samples (0.5, 1, 2, 4, 6, 12 weeks) |
205
|
PUF-PAS |
Lecture room, Brno, Czech Republic |
PCBs, OCPs, PBDEs, novel BFRs, PAHs, PCDD/Fs |
Twelve consecutive LoVol, QFF and PUF-PUF |
12 triplicated samples (1 to 12 weeks) |
259
|
PUF-PAS |
2 university rooms, Toronto, Canada |
Pentabromobenzene, Pentabromotoluene, Phthalates, PBDEs |
Continuous LoVol samples, collected at weekly intervals |
1st exp.: 0, 7, 14, 21, 28, 35 days, 2nd exp.: 7, 28, 49 days, duplicate |
240
|
PUF-PAS (open bottom) |
Computer laboratory, Toronto, Canada |
Phthalates, PBDEs, OPFRs |
Continuous LoVol samples collected at 10 day intervals |
0, 10, 20, 30, 40, 50 days, triplicate |
112
|
F.2 Explanations of the variability in empirically determined sampling rates
The calibrations studies detailed in Sections F.1.2 and F.1.3 revealed that SRe vary considerably between chemicals and between sampling sites and even between different deployment periods at the same site. This has been well documented for the SRe values of PCBs, PBDEs and PAHs in the PUF-PAS.25 Herkert et al.126 also compiled the ranges of the SRes reported for PCBs in the PUF-PAS, noting large differences within and between studies. For a long time, the prevailing assumption in the SVOC-PAS community was that uptake in PASs is air-side controlled, i.e. the diffusion through air to the sampling sorbent is the rate-limiting step to the uptake of chemical in the sampler. If this were true, we would expect only minor differences in the SR between different chemicals at the same place and during the same deployment, as long as the sampler is operating in the linear uptake phase. The reason is that the effective thickness of the air boundary layer surrounding the PAS sorbent (and therefore the diffusion path length) is the same for all chemicals and differences in the SR would only arise from differences in the diffusion coefficients. Diffusivities in air are related to molecular size, but the dependence is relatively weak and the range of molecular size of SVOCs is limited, so we would expect only slightly faster SRs for smaller SVOCs than larger ones. Similarly, diffusivity in air is dependent on temperature and we might expect slightly faster SRs at higher deployment temperatures than during deployments at low temperatures.
Larger differences in SRs between different sites are easier to reconcile with air-side controlled uptake, as the boundary layer thickness can be expected to vary strongly with air turbulence, and windy sites should have higher SRs for all chemicals than those experiencing less turbulence. This has indeed been observed, both in the field126,129,194,195 and during controlled experiments in the laboratory.26,48,128,191 This is also the reason for the generally much lower SRs observed during indoor compared to outdoor deployments. Other factors also affect the diffusion distance in air, namely the sampler configuration192 and the presence and design of a housing,193 and thereby contribute to variability in SR between different samplers and sampler configurations.
There are a number of reasons, why SRes can differ (i) between different chemicals during the same deployment and (ii) for the same chemical between deployments at different temperature. One reason is that air-side resistance may not be controlling (solely) the diffusive exchange between the ambient atmospheric gas-phase and the PAS sorbent. Zhang et al.205 have demonstrated the existence of a sampler-side resistance in a number of uptake experiments, which revealed a strong gradient of SVOC concentrations within PUF disks and XAD-resin filled cylinders. If the sampler side resistance is rate-controlling, the uptake rate is expected to be proportional to the KSG of the compound being sampled,20i.e. less volatile compounds would have a higher SR than more volatile compounds and the same compound would be taken up faster at low temperatures than at high temperatures. Higher SRes for less volatile PCBs have for example been observed for SPMDs.38,135,209
However, the situation can be even more complex when the kinetics of the sorption is being taken into account.207 Model simulations indicated that both an increase and decrease in the SR with KSG and with temperature can occur. The SR of chemicals that sorb strongly (high KSG) and slowly is predicted to increase with molecular size and temperature, whereas the SR of chemicals that sorb quickly and less strongly (low KSG) show the opposite behaviour.207
Finally, there are reasons why empirically determined SRe can be compound and temperature-dependent, even if the assumption of air-side control is valid:
(1) Because of large differences in sampling capacity for different SVOCs, more volatile chemicals will enter the curvi-linear region of uptake earlier and therefore have lower apparent SRs than less volatile SVOCs, which maintain strictly linear uptake for a longer period of time, i.e. SRe < SR. For the same reason, we might anticipate lower SRes at higher temperatures than at lower temperatures, because the latter increases sampler capacity and lengthens the linear uptake period.
(2) If an SVOC is partially particle-bound and the SR for particles is lower than for vapours, we would expect lower empirical SRes both for the less volatile SVOCs and at lower temperatures.
The trend in (2) is opposite to the effect in (1), but applies to SVOCs at the lower volatility spectrum, whereas the effect in (1) applies to the more volatile SVOCs.
(3) Finally, if a chemical sorbed to the PAS sorbent is undergoing degradation, it could appear as if the SRe is reduced. Evidence for this has been observed for selected pesticide (chlorpyrifos, trifluralin, pendimethalin) in XAD-PASs deployed for long time periods.27,190 There is indeed evidence that transformation of chlorpyrifos into its oxon-analog can occur when air is pulled through XAD-2 resin.260 When exposing parent PAHs and alkylated PAHs spiked onto PUF-disks to ozone levels equivalent to two months of deployment in a flow tube, many compounds showed significant reactive loss, especially at low relative humidity.261 When comparing the amounts of SVOCs sequestered in four PUF-PASs deployed for four consecutive weekly periods with those in a PUF-PAS deployed simultaneously for one full month, much higher levels of several PAHs, PBDEs and other BFRs in the PASs deployed for shorter periods were taken as an indication that those substances were degraded during the longer sampling period.262 This implies that SRes for such degradable compounds would depend on the length of the deployments used during a calibration study.
F.3 Accounting for deviations from non-linear uptake
For the PASs with relatively small uptake capacity for the more volatile SVOCs (e.g. those based on PE and PUF), some researchers try to account for the non-linearity of uptake. If CG is assumed to be constant, the integrated form of eqn (1) is:20 | 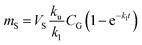 | (14) |
In the specific case, that the air boundary layer is controlling the diffusive exchange between atmospheric gas-phase and PAS sorbent:
| 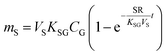 | (15) |
This equation essentially describes the entire uptake curve in a PAS. Harner and colleagues139 routinely use a version of this equation to estimate the effective sampling volume Vair of chemicals in the PUF-PAS and SIP-PAS in the curvi-linear uptake phase:
| 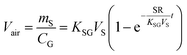 | (16) |
Importantly, this equation for estimating Vair is implemented in the widely used “Template for calculating PUF and SIP disk sample air volumes”263 and also in the model by Herkert et al.,126 which is discussed in detail in Section F.5 below. Lohmann and colleagues take the same approach with the PE-based PAS, when calculating the % of equilibrium that is achieved after a deployment time t using (e.g.ref. 87):
|  | (17) |
While the use of eqn (14) through (17) may be considered the state-of-the-art for calculating the effective sampling volume for situations where linear uptake for a compound during deployment cannot be assumed, it is important to be aware of the underlying assumptions. These equations are based on the assumption that the air-side is controlling the kinetics of uptake. While this is likely the case for some passive sampling material, especially those with high permeability, this has already been shown to not always be the case for the PUF-PAS.205
When applying these equations, one also makes the assumption that CG stays constant during the deployment period of the PAS. If sampling is conducted during the curvi-linear uptake phase, it means that the rate of uptake is gradually decreasing during the sampling period, or in other words, air concentrations at the beginning of the sampling period have more impact on the amount of chemical accumulated in the sorbent than those at the end of the sampling period. This implies that the results will only give an unbiased account of CG if CG is constant. If CG is increasing during deployment, the PAS will underestimate CG and vice versa. Or, stated differently, a PAS does not provide a true time-averaged concentration, if it operates in the curvi-linear uptake region.
We illustrate the magnitude of these effects with PAS-SIM21 calculations of a 90 day deployment of a PUF-PAS for a hypothetical SVOC with a log
KPUF–G of 5.5, i.e. a compound whose uptake is curvi-linear within the deployment period. Three simulations have the same conditions including the same time-averaged concentration CG during the 90 days. The only difference is that CG is either constant, increases from 0 on day 1 to 2 times the average CG on day 90, or decreases from twice the average CG on day 1 to 0 on day 90. The uptake curves are very different (Fig. 9) and importantly the amount taken up after 90 days diverges substantially, which can be expressed by effective sampling volumes Vair of 60 m3 (constant CG), 89 m3 (increasing CG) or 31 m3 (decreasing CG). Eqn (16) yields a Vair of 65 m3. This implies that the assumption of no sampler side resistance implicit in eqn (16) in this case only leads to a minor error (5 m3), largely because of the relatively high volatility of a compound with a log
KPUF-G of 5.5. On the other hand, the assumption of constant CG, also implicit in eqn (16), gives a large error (29 m3).
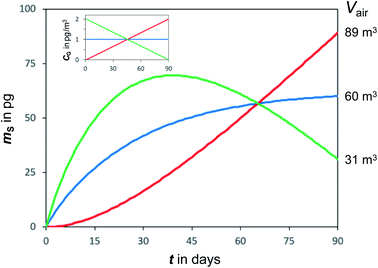 |
| Fig. 9 Simulated uptake curve for a compound with log KPUF–G of 5.5 in a PUF-PAS during 90 days of constant (blue), increasing (red) and decreasing (green) air concentrations. | |
Increasing or decreasing trends of CG during a PAS deployment period are common, considering the pronounced seasonal variability of the air concentrations of many SVOCs, which is due either to seasonal source strength (e.g. pesticides elevated during application season,264 PAHs elevated during heating season265), temperature effects266 or oxidation by photooxidants.267 This issue is not restricted to the PUF-PAS, but applies to all PAS operating in the curvi-linear uptake phase. While the precise size of the error depends on the particular combination of KSGVS, deployment time and temporal concentration trend, and will often be smaller than in the illustrative example of Fig. 9, it is reasonable to assume that in many instances applying eqn (16) and (17) will incur very substantial errors.
It is further important to be aware that these equations require quantitative knowledge of both the PAS's uptake capacity KSGVS and inherent SR, which can be challenging to obtain as both are strongly dependent on meteorological conditions that also can be variable during the time of deployment:
– The SVOC's equilibrium partitioning coefficient KSG between sampler sorbent and the gas-phase is a strong function of the temperature of deployment Td. It is customary to correct KSG to the average temperature of deployment using the van't Hoff equation and the internal energy of phase transfer between sampling sorbent and the gas-phase ΔUSG. If ΔUSG is unknown, the enthalpy of vaporization ΔHvap is often used to approximate ΔUSG (e.g.ref. 87). The relationship between KSG and T is not a linear one, so the use of an average Td is problematic if T is increasing or decreasing during the deployment period (see also ref. 204). A further complication arises if the temperature of the PAS sorbent deviates from ambient temperature, because of radiative effects associated with the sampler housing.193,268,269
– The inherent sampling rate SR (m3 per day), i.e. the SR during the linear uptake phase, is dependent on wind speed (because of the effect on the thickness of the boundary layer) and also somewhat on temperature (because of its effect on the diffusivity). Because SR is most often deployment-specific, it is usually determined from the loss of depuration compounds spiked onto the sampler prior to deployment, which implies that a further set of assumptions needs to be met (see Section F.4 below).
In summary, it is important to keep in mind that even if the assumptions underlying the use of eqn (14) through (17) were correct, the interpretation of PAS results within the curvi-linear region incurs very significant uncertainties. As such, it is generally preferable to use a sampler that remains in the linear uptake region. The XAD-PAS has not been used in the curvi-linear uptake region, because it is generally acknowledged that the sampler-side resistance is not negligible and the assumptions underlying the use of depuration compounds are violated.
F.4 The use of depuration compounds in PAS
As was discussed in Section C.1, PASs for SVOC tend not to have a diffusive barrier in order to allow for SRs that are sufficiently high for reliable quantification. One of the consequences is that the SR of the PASs tends to be quite dependent on wind exposure, especially if the sampling sorbent is not protected by a wind shelter or if the shelter is ineffective in preventing the wind from impacting the sorbent. Adopting an approach pioneered within the passive water sampling community, some PAS users spike the passive sampling sorbent with compounds prior to exposure and derive information on the kinetics of uptake from the extent of loss of those spiked compounds during the deployment. These compounds are referred to as either depuration compounds (DCs) or performance reference compounds. Because it is necessary to distinguish the spiked compounds from those taken up in the PAS sorbent during exposure, DCs are often isotopically labelled SVOCs. Alternatively, compounds are selected that are known to not be present in the sampled atmosphere. The idea underlying the use of DCs is that wind exposure affects the kinetics of loss of DCs to the same extent as it affects the kinetics of uptake of the target compounds.
Müller et al.120 were the first to investigate the rate of loss of compounds spiked onto the sorbent of a PAS, specifically the stearin-coated fibre cloth. A log–log linear relationship between the rate of loss and a compound's KSG was interpreted as indicating an air-side control on the rate of mass transfer between atmospheric gas-phase and the sorbent. Ockenden et al.41 and Booij and van Drooge37 introduced DCs to the use of SPMDs as PAS, arguing that they can be used to account for differences in exposure conditions. Söderström and Bergqvist48 confirmed that the extent of loss of deuterated PAHs and 13C-labelled PCBs from SPMDs was related to their exposure to different wind speeds in a wind tunnel experiment. Farrar et al.93 observed that the loss of different PCB congeners spiked into EVA-coated glass-PASs (POGS) increased with compound volatility, wind speed, temperature and deployment time. Bartkow et al.68 and Moeckel et al.130 showed that this was also the case with LDPE-PASs and PUF-PASs. PUF-PASs and PE-based PASs are frequently used with DCs, largely owing to the short tlinear for many SVOCs in these sorbents.
The concentration of DCs in the ambient gas-phase CG is zero. Therefore, eqn (1) simplifies to:
|  | (18) |
In integrated form:
Therefore, from the amount of the DC in the PAS at the beginning of deployment mS0 and at the time of retrieval mS, the loss rate constant kl can be derived:
|  | (20) |
Under air-side controlled kinetics, eqn (3) relates kl with the inherent SR:20
| 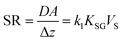 | (21) |
Therefore, the SR for the conditions of the deployment can be calculated from the empirical kl of a DC using:
| 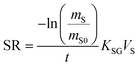 | (22) |
This implies that the KSG of the DC at the temperature of deployment needs to be known. SRs estimated with eqn (22) can be seriously flawed if the actual temperature in the sampler housing deviates from ambient temperature and the latter is used to estimate KSG.268
A special case occurs if target chemical and depuration compound are the same, except that the latter is isotopically labelled. In this case, the KSG in eqn (16) and (22) applies to the same compound under the same temperature conditions and is therefore identical. Accordingly, if we substitute the right side of eqn (22) for SR, eqn (16) simplifies to:
| 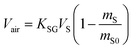 | (23) |
The ratio mS/mS0 should be in the range from approximately 0.2 to 0.8, if the estimated SR should not have too high an uncertainty.48 This implies that the DC should not be lost too much (more than 80%) or too little (less than 20%). Because the extent of loss of a DC depends on KSG, Td, wind speed and deployment length, it requires considerable prior knowledge of the deployment conditions to choose the right DC. In practice, multiple DCs with variable KSG are used, as this increases the likelihood, that at least one of them is lost to the appropriate extent. In the case of low temperature deployments, DC would have to be considerably more volatile than the target compounds to achieve losses of 20% during deployment. In fact, typically applied DCs spiked onto PUF-PASs deployed at low temperatures often do not experience sufficient loss.126 In the case of passive water sampling, the information contained in DCs being lost less than 20% and more than 80% should not be ignored, which likely also applies to passive air sampling.270
It is important to keep in mind that the assumption of air-side resistance-controlled uptake is underlying the use of DCs. Even if this assumption may not be valid, DCs can often give a semiquantitative measure of the wind exposure that a PAS sorbent experienced during a particular deployment and make some allowance for how this affects the SR. However, it would not be appropriate to use DCs in PAS, where the sampler-side resistance is clearly contributing to the exchange kinetics (XAD-PAS, SIP-PAS, PUF-PAS under some conditions). In any case, most SVOCs would not be lost appreciably from the sorbent of the XAD-PAS during normal deployments, because of its very high uptake capacity (Section E.3).
Disadvantages of the use of DCs are the considerable cost of isotopically labelled compounds and restrictions on their use during indoor deployments, i.e. it may not be possible to get approval to deliberately release potentially toxic compounds into indoor living environments. They can also not be used, when the PAS extract is to be used for toxicological characterisation with in vitro bioassays (see Section G.5.5) (e.g.ref. 271). Furthermore, the often very considerable uncertainty in the KSG of the DCs propagates directly to the estimated SR.
F.5 Theoretical estimation of sampling rates
There have been several efforts to describe the uptake of SVOCs in PASs using numerical simulation models.21,126,190,204,207,272 Whereas the models by Zhang and Wania207 and Armitage et al.21 could be applied to any sampler with a porous sorbent and have been parameterised for the XAD-PAS and the PUF-PAS, the model by Petrich et al.272 and Herkert et al.126,204 is specific to the PUF-PAS, because it relies on a sampler-specific empirical fitting constant. These efforts have a variety of motivations, including confirming a comprehensive understanding of the processes and factors controlling the uptake in a PAS,207 aiding the interpretation of calibration studies and monitoring data (e.g. by estimating whether the PAS is operating in the linear or the curvi-linear uptake phase), and informing the design of PAS campaigns (e.g. in terms of deciding on the appropriate deployment length or the selection of DCs that will experience the desired extent of loss).21,126,190,204
These models can also be used for estimating the uptake of a particular target compound during a particular deployment, e.g. by using data on variable temperatures, external wind speeds and ambient air concentrations.21,204 If the model aims to simulate the mass transfer kinetics between atmospheric gas-phase and PAS sorbent, they can be used to predict deployment-specific, inherent SR during the linear uptake phase.21,272 If the model additionally allows for calculation of uptake in the curvi-linear and equilibrium part of the uptake curve, it can be used to calculate what is either called an apparent SR21,190 or an effective sampling volume.126,204 The effective sampling volume is simply the product of the apparent SR and the deployment time.
The models vary widely in terms of the processes being considered. The first such model by Zhang and Wania was the most comprehensive in terms of treating the uptake process as a sequential diffusion through a stagnant air-side boundary layer and a porous sampling sorbent followed by the reversible sorption onto the sorbent.207 The PAS-SIM model21 does not explicitly consider the kinetics of the sorption process, because numerical values for the parameterization of this process for SVOCs on PUF or XAD generally do not exist. The model by Petrich et al.272 and Herkert et al.204 furthermore does not include a description of transport within the sorbent (specifically the pores of the PUF), i.e. disregards the possibility of the importance of a sampler side resistance and uses as a starting point that the “uptake of PCBs on a PUF-PAS can be modelled as a function of the air-side mass transfer coefficient”. This is somewhat questionable, considering both empirical205 and theoretical evidence207 to the contrary.
The models have also a number of things in common. They require input of temporally resolved wind speed and temperature data during a PAS's deployment period and calculate time-variant KSG for the target compound from these temperature data using the van't Hoff equation. Armitage et al.21 directly estimate KSG at a reference temperature and the internal energy of phase transfer between sorbent and the gas-phase ΔUSG using ppLFERs215,223 (see Section E.2.2). Herkert et al.204 estimate the KOA and ΔUOA for the PCBs first and then use the empirical single parameter linear free energy relationships between KPUF–G and KOA by Shoeib and Harner24 (see Section E.2.1) assuming that ΔUOA equals ΔUPUF–G. For the PCBs, these two approaches give reasonably consistent results.204
Zhang and Wania207 and Armitage et al.21 estimate the mass transfer coefficient through the air-side boundary layer using molecular diffusivity D (estimated using the semi-empirical Fuller–Schettler–Giddings equation) and the thickness of that layer Δz. Armitage et al.21 propose a Δz of 1.5 cm for stagnant air conditions as it provides SRs for the XAD-PAS consistent with observations.191 They then apply an empirical function to scale the SR of the XAD-PAS with wind speed based on measurements.191 This function shows a stronger dependence on wind speed below 1 m s−1 than above, which is also consistent with studies on other PASs using an effectively wind-sheltered sorbent.273–276 A similar approach is used to parameterise the PAS-SIM model21 for the PUF-PAS relying on the relationship between SR and windspeed by Thomas et al.132
Petrich et al.272 and Herkert et al.,126,204 on the other hand, estimate the mass transfer coefficient through the air-side boundary layer using an equation for laminar flow along a flat plate, which includes molecular diffusivity, the kinetic viscosity of air, the length of mass transfer (taken to be the diameter of the PUF disk), and the wind speed inside the PAS chamber. The latter is obtained from ambient wind speed using an empirical linear relationship determined in wind tunnel experiments.128 This approach further requires a fitted dimensionless constant, the so-called advective mass transfer coefficient γ, presumably because the flow along the PUF disk is not really laminar. Different values of γ, all based on fitting empirical data of the loss of DCs, have been presented.126,204,272 The γ value depends on the precise formulation of the laminar flow equation, the data set of DC loss rates being used (in particular the range of wind speeds encountered during deployment) as well as the source of the meteorological data being used (e.g. measurements at the nearest airport or meteorological station or data estimated from a global weather reanalysis).126
In summary, both approaches ultimately rely to some extent on fitting to parameterise the mass transfer through the boundary layer. PAS-SIM uses empirical data to fit the boundary layer thickness under stagnant conditions,21 whereas Herkert et al. use the observed loss of DCs to fit γ.126
Herkert et al. argue that models in combination with readily available meteorological data can be used to derive SR when DC-derived SRs are not available or are implausible.126 Mapping model-estimated SRs on a global scale suggests that wind speed is the decisive factor responsible for variable SRs, with higher values over the oceans and lower values within forests. This is consistent with the discussion in Section C.1. They also claim to be able to use their model to estimate effective air sampling volumes Vair for compounds in the curvi-linear uptake regime.126 Incidentally, the PAS-SIM model could be similarly combined with meteorological data to estimate apparent SRs or Vair for specific deployments, while also taking the sampler side resistance into account. It is important to note, however, that such estimations of deployment-specific Vair will inevitably require the assumption of constant air concentrations during deployment, the implications of which were discussed in Section F.3 and illustrated in Fig. 9.
Different approaches have been chosen to evaluate a model's ability to predict the uptake behaviour of SVOCs in a PAS. PAS-SIM has been evaluated by comparing theoretically determined uptake curves with those obtained from a year-long calibration study of the XAD-PAS for PCBs and PAHs21 and pesticides.190 Herkert et al.204 compared concentrations of PCBs obtained using model-derived SRs for the PUF-PAS with those obtained by HiVol sampling in three different studies.
For completeness sake, we also note that there have been attempts to predict SRs using quantitative structure property relationships. Van Mourik et al. linearly regressed empirically determined SR for the XAD-PAS against molecular mass in order to estimate SRs for chlorinated paraffins.277 Empirical SRs in SPMDs were regressed against quantum-chemical descriptors by Zhu et al.278,279 However, these are statistical relationships not based on a mechanistic understanding of the sampling process.
F.6 Sampling of SVOCs in the particle phase
As was mentioned in Section F.2, sorption of SVOCs to the atmospheric particle phase contributes to the variability in empirically determined SRs. Whether a PAS is taking up particle-bound SVOCs or not appears to be quite strongly dependent on the sampler housing. For example, there is no evidence of the uptake of particle-bound SVOCs in the XAD-PAS; substances that are predominantly particle-bound in the atmosphere, such as PAHs with five or more fused rings or more highly brominated PBDEs, tend to have levels below the limit of detection in XAD-PASs.280 On the other hand, the PUF-PAS is much more likely to take up particles, because the double bowl housing does not block wind very effectively. Chaemfa et al. used microscopy to study the entrapment of particles within the PUF disks deployed in the double bowl housings.131 The PUF disk mostly sampled the fine particle fraction (<1 μm) and the size distribution of particles in the PUF was found to diverge strongly from that obtained with an aerosol spectrometer. On the other hand, Markovic et al. reported that particle numbers and size distributions measured with a particle spectrometer in empty double bowls were similar to those measured outside, suggesting that the housing does not discriminate between different particles.281
An extensive evaluation of the PUF-PAS for different particle-associated SVOCs suggested a poorer performance than for gaseous compounds, which was attributed to “unpredictable accumulation behaviour of particles”.245 SRs were reported to be much lower and more variable for particle-bound substances than for gas-phase compounds,25,129,245 which contrasts with other studies that have reported similar SR for particle-bound and gaseous polycyclic aromatic compounds.247,257 Because of the propensity to sample particles, the PUF-PAS has even been used as a sampler for atmospheric trace metals.282,283 However, SR for different metals differed widely, presumably because of their different size distributions and the different SR of particles of different size.
Overall, the evidence as to the capability of the PUF-PAS to sample particle-bound substances reliably and reproducibly is still inconclusive.284 The largest study evaluating the performance of the PUF-PAS for compounds in the particle phase concluded that in all assessment endpoints (detection, precision, fingerprinting performance, and SR consistency and magnitude) the performance was poorer than for gas-phase compounds.245
Instead of looking at the uptake of particle-bound substances by the sorbent meant for gaseous compounds, others have sought to add a GFF to a PAS to explicitly sample particle-bound substances.155–157,168,199 This is discussed in more detail in Section D.3.1.3. Yet others have proposed PASs solely dedicated to particle-bound substances158 or to use different sampler orientation and sorbents to distinguish total atmospheric load from gaseous contributions.200
G. Applications of PASs for SVOCs
G.1 PAS networks
One of the most common uses of PASs is the recording of the spatial variability in time-averaged air concentrations of SVOCs. This can take the form of networks of samplers. These networks vary widely in scale.
G.1.1 Local and urban PAS network studies.
Among the PAS network studies with the smallest scale are those that seek to characterise the influence of a known or suspected point source on local atmospheric SVOC concentrations (Table 13). The use of PAS networks for mapping concentration variability close to sources makes intuitive sense, because it is in those areas that substantial heterogeneity of air concentrations can be expected to occur. The facilities that have been studied this way include chemical plants, steel plants, power plants, petrochemical extraction areas, waste incinerators, sewage treatment plants, and landfills. The target chemicals are often focused on the SVOCs that are expected to be emitted by the studied facility, for example combustion-related SVOCs such as PCDD/Fs, PCBs, PCNs from municipal waste incinerators,236,285,286 volatile PFAS, VMS and musk compounds from waste water treatment plants144,287,288 or parent PAHs and alkyl-PAHs in the oilsands region of Alberta, Canada247 and in the vicinity of natural gas extraction wells.69
Table 13 Studies using PASs to measure the spatial variability of SVOCs around major suspected emission sources
Sampler |
Location, number of sites |
Time frame |
SVOCs |
Ref. |
SPMD |
13 sites around DEZA chemical plant, Valasske Mezirici, Czech Republic |
Sep. 1998, 4 weeks |
PAHs |
36
|
PUF-PAS |
9 sites around chemical factory, Neratovice, 11 sites around coal tar and mixed tar oils processing plant, Valasske Mezirici, Czech Republic |
Jan. to Jul. 2004, six 28 day periods |
PCBs, OCPs, PAHs |
289
|
PUF-PAS |
4 sites around municipal solid waste incinerator and power plant, Barcelona, Spain |
Mar. to Jun. 2005, 3 months |
PCDD/Fs, PCBs, PCNs |
236
|
PUF-PAS |
8 sites around a municipal solid waste incinerator, Tarragona, Spain |
Mar. to Jun. and Sep. to Dec. 2010 and 2011, 3 months |
PCDD/Fs, PCBs, PCNs |
285
|
PUF-PAS |
8 sites around a petrochemical and a chemical complex, Tarragona, Spain |
Nov. 2014 to Jan. 2015, one 2 month period |
PAHs |
290
|
Membrane-enclosed copolymer sampler |
6 sites around steel complex, Pohang, Korea |
Aug. to Sep. 2005, 37 days |
PAHs |
53
|
PUF-PAS |
15 sites around an iron and steel making plant, Pohang, Korea |
Sep. 2006 to Jul. 2007, four 3 month periods |
PCBs |
291
|
SIP-PAS |
12 sites around a municipal wastewater treatment plant and 4 sites close to two municipal solid waste landfill sites, Ontario Canada |
Jul. to Sep. 2009, 63 days |
PFAS |
144
|
Jun. to Aug. 2009, 55 days |
SIP-PAS |
1 site at eight wastewater treatment plants and 1 reference site each |
Aug. to Nov 2013, Jan. to Mar. 2014, two 2 month periods |
PFAS, VMS, UV-filters |
287
|
Synthetic musks |
288
|
PUF-PAS |
15 to 17 sites around oilsands region, Fort McMurray, Alberta, Canada |
Nov. 2010 to Jan. 2016, thirtyone, 2 month periods |
PAHs, alkylated PAHs, dibenzothiophenes |
247, 292 and 293
|
PUF-PAS |
5 sites in oilsands region, Fort McMurray, Alberta, Canada |
Oct. to Nov. 2015, 2 month period |
PAHs, alkylated, nitrated and oxygenated PAHs |
294
|
PAS-DD |
PUF-PAS |
5 sites around a secondary copper and aluminum metallurgical facilities, China |
Apr. to Oct. 2011, two months |
PCDD/Fs, dlPCBs, PCNs |
295
|
PUF-PAS |
13 sites around a municipal solid waste incinerator, China |
May to Aug. 2012, Oct. 2012 to Jan. 2013, two 3 month periods |
PCDD/Fs, PCBs |
286
|
PE-PAS |
23 sites close to natural gas extraction wells, Carroll County, Ohio, USA |
Feb. 2014, one 3 to 4 week period |
PAHs |
69
|
PUF-PAS |
23 sites near ship-breaking activities, Chittagong, Bangladesh |
Feb. 2013, one 1 week sample (7 to 9 days) |
PAHs, PCBs, DDTs, HCB, SCCPs |
296
|
PUF-PAS |
7 sites in an e-waste dismantling region, Taizhou, China |
Sep. 2009 to Aug. 2010, four 3 month periods |
Dechlorane plus |
297
|
PUF-PAS |
5 sites around hazardous waste landfill, Sabiñánigo, Aragon, Spain |
Summer 2014 to autumn 2016, twenty-three consecutive 1 month periods |
HCHs |
298
|
PUF-PAS |
Municipal solid waste landfill, Novi Sad, Serbia |
Jun. and Oct. 2012, Jan. and Apr. 2013, four 1 month periods |
PAHs, PCBs, OCPs |
299
|
PUF-PAS |
5 sites within a chemical manufacturing plant, China |
Sep. to Oct. 2016, one 1 month period |
SCCPs |
300
|
PUF-PAS |
12 sites around a coal-fired power plant on south-west coast of India |
2014/2015, four seasonal samples |
PAHs |
301
|
XAD-PAS |
18 or 20 sites in different waste handling facilities, Norway |
2013/2014, 2 to 3 month periods |
PBDEs, PCBs |
302 and 303
|
PUF-PAS |
16 sites in urban area and vehicular waste processing areas, Northern Vietnam |
Jan. to Mar. 2013 and Sep. to Nov. 2015, two 2 month periods |
>1000 micro-pollutants, incl. PCBs, PBDEs, PAHs, BFRs methylated PAHs |
304 and 305
|
With more than 30 studies, networks of PASs across urban areas are very common (Table 14). This is not surprising as urban areas with their multitude of potential sources and large gradients in land cover and human activities are likely to experience large heterogeneity in SVOC air concentrations. A diverse set of urban areas around the world has been studied, including major metropolitan areas in the USA, such as Philadelphia306 and Chicago,307,308 a number of Chinese cities, including Beijing,309 Shanghai,310 Tianjin,311–313 and Harbin,314 as well as major cities in developing countries such as Bangkok,46 Manila,315 Alexandria,73,74 Karachi and Lahore.316 However, some PAS networks have also been used to measure air concentration variability in less well-known, mid-size cities, such as Manizales, Colombia,317,318 Ulsan, South Korea319,320 and Aliaga, Turkey.321 PUF-PASs are most commonly used, but SPMDs, XAD-PASs and PE-PASs have also been employed.
Table 14 Studies using PASs to measure the spatial variability of SVOCs in the urban atmosphere
Sampler |
Location, no. of sites |
Time frame |
SVOCs |
Ref. |
PUF-PAS |
20 sites in Brno, Czech Republic |
Oct. to Nov. 2004, 28 days |
PCBs, OCPs, PAHs |
322
|
SPMD |
2 sites in Genoa, Italy, industrial area |
April 2007 to May 2008, nine 1 month periods (28 to 36 days) |
PAHs |
59
|
PUF-PAS |
7 sites in Liberec, Czech Republic |
Dec. 2005 to Dec. 2006, thirteen 28 day periods |
PAHs |
323
|
PUF-PAS |
23 sites in Zurich, Switzerland |
Spring 2011 and spring 2013, two periods |
PCBs |
324
|
XAD-PAS |
3 sites in Strasbourg, France |
Every spring between 2013 and 2016, eleven 2 week periods |
PAHs, PCBs, OCPs, CUPs |
325
|
PUF-PAS |
6 sites in Ottawa, Ontario, Canada |
Dec. 2002 to ar. 2003, 3 months |
PFAS |
162
|
PUF-PAS |
32 sites in Philadelphia/Camden, USA |
Apr. to Jul. 2005, 96 to 98 days |
PCBs |
306
|
PUF-PAS |
5 sites in Fairbanks, Alaska, USA |
Dec. 2008 to Mar. 2009, 4 months |
PAHs |
326
|
XAD-PAS |
26 sites in Toronto, Ontario, Canada |
Jul. to Oct. 2012, 65 to 92 days |
VMS |
28
|
PUF-PAS |
21 sites in Cleveland, Ohio and 17 sites in Chicago, Illinois, USA |
Aug. to Sep. 2008, 20 to 22 days (Cleveland) and 13 to 47 days (Chicago) |
PCBs |
307
|
PUF-PAS |
13 sites in Chicago, Illinois, USA |
Jan. 2012 to Jan. 2014, 180 samples of 43 ± 11 days. |
PAHs, BFRs, OPFR, OCPs |
308
|
PUF-PAS |
8 sites in Toronto, Ontario, Canada |
Aug. 2016 to Aug. 2017, six 2 month periods |
PAHs, alk-PAHs, nitro-PAHs, Oxy-PAHs, dibenzothiophene (DBT), alk-DBTs |
327
|
PUF-PAS |
6 sites in Concepcion, Chile |
Jan. to Mar. 2007, 2 months |
PCBs, PAHs, OCPs |
328
|
PUF-PAS |
6 sites in Temuco, Chile |
Apr. 2008 to Apr. 2009, three 4 month periods |
PAHs |
329
|
PUF-PAS |
5 sites in Santiago, 6 sites in Concepcion, 6 sites in Temuco, Chile |
Apr. 2008–Aug. 2009, four 4 month periods |
PCBs, OCPs |
330
|
PUF-PAS |
4 sites in Santiago de Cali, Colombia |
May to Sep. 2011, one 4 month period |
PCBs, OCPs, PAHs |
331
|
PUF-PAS |
4 sites in Manizales and 1 site in Bogota, Colombia |
June 2012 to Nov. 2014, total of 27 periods (90 to 120 days) |
PCDD/Fs, dlPCBs |
317,318
|
PUF-PAS |
11 sites in Mendoza, Argentina |
Dec. 2010 to Apr. 2011, ∼90 days |
PCBs, HCB DDTs, PBDEs, |
332
|
SPMD |
6 sites in Bangkok, Thailand |
Mar. to Apr. 2000, 3 weeks |
PAHs |
46
|
PUF-PAS |
6 sites in and around Manila, Philippines (3 urban, 3 rural) |
May to Dec. 2005, four periods of 42 to 56 days |
PAHs |
315
|
PUF-PAS |
3 sites in Manipur, India |
Jan. to Dec. 2009, one month for a year |
OCPs |
333
|
PAHs |
334
|
PUF-PAS |
5 sites in Kolkota, 1 site in Sundarban, India |
Dec. to Mar. 2014, one 3 month period |
PBDEs, OCPs, PCBs |
335
|
XAD-PAS |
6 sites in Karachi and 4 sites in Lahore, Pakistan |
Jan. to Oct. 2011, 10 months |
OCPs, PCBs |
316
|
PUF-PAS |
6 sites in Kathmandu, 4 sites in Pokhara, 3 sites in Hetauda in Nepal |
Kathmandu and Pokhara: Aug. 2014 to Aug. 2015, Hetauda: Nov. 2015 to Aug. 2016, six 2 month periods |
PAHs |
336
|
PCBs, OCPs |
337
|
PUF-PAS |
6 sites in Shanghai, China |
Aug. 2006 to Jun. 2007, three 3 month periods |
PAHs |
310
|
PUF-PAS |
6 sites in Tianjin, China |
Jul. 2006 to Jun. 2008, one 8 months & five 3 month periods |
OCPs, PCBs |
311
|
PUF-PAS |
8 sites in Harbin, China |
Feb, 2007 to Jan. 2008, four 3 month periods |
PAHs |
314
|
PUF-PAS |
11 sites in Tianjin, China |
Jun. 2008 and Jan. 2009, two 1 month periods |
PCBs, PCDD/Fs |
312
|
PUF-PAS |
13 sites in Beijing, China |
Feb. 2011 to Mar. 2012, four seasonal periods |
PCDD/Fs, dlPCBs, PBDEs |
309
|
PUF-PAS |
28 sites in Nanjing, China |
Jan. to Oct. 2015, four 2 to 3 month periods |
PAHs |
338
|
PUF-PAS |
7 sites in Ningbo, China |
Nov. 2014 to Oct 2015, twelve 1 month periods |
PAHs |
339
|
PUF-PAS |
8 sites in Ezhou, Wuhan, Hubei, China |
Jun. to Aug. 2015, one period |
PAHs |
340
|
PUF-PAS |
20 sites in Dalian, Liaoning, China |
Jul. to Sep. 2017, one 40 day period |
PAHs |
341
|
PUF-PAS |
33 sites in Tianjin, China |
Jul. to Sep. 2016, Nov. 2016 to Jan. 2017, two 90 day periods |
OPEs |
313
|
PUF-PAS |
20 sites in Ulsan, South Korea |
Jan. to Feb 2011, 49 days |
PAHs |
319
|
PUF-PAS |
20 sites in Ulsan, South Korea |
Mar. to May 2013, 84 days |
PAHs, Cl- PAHs, Br-PAHs |
320
|
PUF-PAS |
36 sites in Seoul, South Korea |
Aug. to Sep. 2006, Jan. to Feb. 2007, two 1 month periods |
PAHs |
342
|
PUF-PAS |
7 sites in Tangshan, China |
Jul. to Oct. 2010, 3 months |
OCPs |
343
|
PUF-PAS |
22 sites in Istanbul, Turkey |
Sep. to Dec. 2014, four 1 month periods |
PAHs, PCBs |
344
|
PUF-PAS |
40 sites in Aliaga, Turkey |
Jul. 2009 to May 2010, four 2 month periods |
PAHs, PCBs |
321
|
PUF-PAS |
8 sites in Bursa, Turkey |
Feb. to Dec. 2014, five 2 month periods |
PCBs |
345
|
XAD-PAS |
15 sites in Minneapolis, Minnesota, USA |
2013–2015, several 3 month periods |
PAHs |
253
|
PE-PAS |
11 sites in Alexandria, Egypt |
Jul. 2010 to Jan. 2011, 21 days |
PAHs alkyl-PAHs, thiophenes |
73
|
OCPs |
74
|
G.1.2 Regional PAS network studies.
Studies involving networks of PASs in a region are also very common and have been conducted around the world (Table 15). A region can be an administrative unit, such as a Chinese province251,346–348 or an Italian region349–351 or it could be a country's coastal area352–355 or an entire or partial watershed.356–359 These types of studies have almost exclusively relied upon the PUF-PAS, with a few exceptions. PAHs are most frequently measured, but OCPs and PCBs are also commonly targeted. Occasionally, more unusual target substances have been studied, such as short-chain chlorinated paraffins (SCCPs) in the Yangtze River Delta360 or three monosaccharides in the province of Guangdong.251 While many studies only sampled for one or two periods, some seek to observe temporal patterns by sampling during multiple seasons. The number of sampling sites varies widely and ranges from as little as three to more than 30.361–364
Table 15 Studies using PASs to measure the spatial variability of SVOCs in a region
Sampler |
Location, no. of sites |
Time frame |
SVOCs |
Ref. |
Europe
|
PUF-PAS |
6 sites in Northern Estonia |
Mar. to Aug. 2006, four 4 week periods |
PCBs, OCPs |
353 and 354
|
PUF-PAS |
19 sites in Tuscany, Italy |
Apr. to Jul. 2008, 60 to 160 days |
PAHs, OCPs, PBDEs, PCBs |
349
|
PUF-PAS |
10 sites in Tuscany, Italy |
Apr. 2008 to Jul. 2009, four 3 to 5 month periods |
PCBs, OCPs |
351
|
PUF-PAS |
4 sites in Puglia, Italy |
Jan. 2009 to Feb. 2010, four periods of 2 to 5 months |
PCBs, PAHs |
350
|
PUF-PAS |
8 sites in Sicily, Italy |
Jul. to Dec. 2007, two 2 to 3 month periods |
PCBs, OCPs, PBDEs |
365
|
PUF-PAS |
7 sites in Molise, Italy |
Apr. to Jul. 2011, one 3 month period |
PAHs |
366
|
PUF-PAS |
6 sites around the Mar Menor lagoon, Cartagena, Spain |
2010, four 1 month periods in each season |
PAHs, CUPs |
237
|
PUF-PAS |
3 sites around Bothnian Bay, Sweden |
Jul. 2011 to Jan. 2013, five 3 to 5 month periods |
OCPs, CUPs, brominated anisoles |
367
|
PUF-PAS |
150 sites in Naples, Campania, Italy |
Jul. 2015 to Apr. 2017, three-four 3 month periods |
OCPs, PCBs, PAHs |
368
|
PUF-PAS |
4 sites in Sabiñánigo, Aragón, Spain |
Feb. 2016 to Nov. 2018, 29 consecutive 1 month periods |
HCHs, PeCB, HCB |
369
|
![[thin space (1/6-em)]](https://www.rsc.org/images/entities/char_2009.gif) |
Africa
|
PUF-PAS |
3 sites in Northern Algeria |
Jul. 2008 to Nov. 2009, 28 to 56 days |
PCBs, CUPs, PCDD/Fs |
370
|
PUF-PAS |
3 sites along coastal zone of Ghana |
Jan. to Dec. 2008, 4 weeks and 12 weeks |
OCPs |
355
|
PUF-PAS |
4 sites in Gauteng Province, South Africa |
May 2016 to Jan. 2017, two 100 day periods |
PBDEs, novel BFRs |
371
|
![[thin space (1/6-em)]](https://www.rsc.org/images/entities/char_2009.gif) |
Americas
|
PUF-PAS |
4 sites in Southern Mexico |
2002 to 2004, 31 to 116 days |
OCPs, PCBs |
372
|
PUF-PAS |
23 sites in the Yakima valley, Washington, USA |
Mar. to Apr. and Jun. to Aug. 2011, 5 to 30 days |
CUPs |
244
|
PUF-PAS |
8 sites in Bahia Blanca, Buenos Aires province, Argentina |
2006–2007, 105 to 155 days, 191 to 230 days |
PCBs, OCPs |
373
|
PUF-PAS |
6 sites in Córdoba, Argentina |
Nov. to Dec. 2014, one period (44 to 50 days) |
PAHs, PCBs, OCPs, PBDEs |
374
|
XAD-PAS |
10 sites in Quequén Grande basin, Buenos Aires province, Argentina |
May 2013 to Sep. 2014, three 4 month periods |
OCPs, CUPs, PCBs |
357
|
XAD-PAS |
9 sites around Bahia Blanca, Buenos Aires province, Argentina |
Jan. to Dec. 2015, four seasonal samples |
PAHs |
375
|
![[thin space (1/6-em)]](https://www.rsc.org/images/entities/char_2009.gif) |
Asia (other than China)
|
PUF-PAS |
10 sites in Punjab, Pakistan |
Jan. to Mar. 2011, one 8 week period |
OCPs |
376
|
PUF-PAS |
6 sites in the Indus Basin, Pakistan |
Sep. to Oct. 2013, 56 days |
OCPs |
356
|
PUF-PAS |
6 sites in the Indus Basin, Pakistan |
Oct. to Dec. 2012, 8 weeks |
PCBs |
377
|
PUF-PAS |
32 sites in Azad Jammu and Kashmir region, Pakistan |
Nov. 2016 to Jan. 2017, 8 weeks |
OCP, PCBs |
364
|
PUF-PAS |
Unknown number of sites in Punjab, Pakistan |
Unknown number of consecutive 56 day periods |
PAHs |
378
|
PUF-PAS |
32 sites in Tamil Nadu, India |
Apr. 2009 to Jan. 2010, three seasonal periods |
PAHs |
361
|
PUF-PAS |
9 sites within the Ganga River basin, India |
Apr. to Sept. 2013 (3 to 4 months) and Feb. to May 2014 (1 to 2 months) |
PAHs |
358
|
PUF-PAS |
7 sites at lower reaches of Ganga River, India |
Mar. to Apr. 2015, one 40 day period |
OCPs |
359
|
PUF-PAS |
19 sites along the south coast of South Korea |
Jan. to July 2006, two 3 month periods |
Coplanar PCBs, PCNs, PBDEs |
352
|
PUF-PAS |
10 sites in Gyeonggi Province, South Korea |
Mar. 2011 to Feb. 2013, 80 to 100 days |
PCBs, PCDD/Fs |
379
|
PUF-PAS |
20 sites in Ulsan, South Korea |
Feb. 2011 to Feb. 2012, four 3 month periods |
PAHs |
380
|
PUF-PAS |
41 sites in Kutahya, Turkey |
Jan. to Mar. and Jun. to Aug. 2014, two 2 month periods |
PAHs, PCBs |
363
|
PUF-PAS |
4 sites in Kumluca, Antalya, Turkey |
May to Nov. 2013, 11 periods (1 to 3 months) at one site, two 3 month samples at three sites |
OCPs, PCBs |
381
|
PUF-PAS |
23 sites in Dilovasi district, Kocaeli, Turkey |
Feb. 2015 to Feb. 2016, twelve 1 month periods |
PAHs, PCBs |
382
|
![[thin space (1/6-em)]](https://www.rsc.org/images/entities/char_2009.gif) |
China
|
PUF-PAS |
40 sites in Hebei province |
2007 to 2008, 46 to 123 days |
PAHs |
346
|
PUF-PAS |
7 sites in Hubei province |
Apr. 2012 to Mar. 2013, four seasonal samples |
OCPs |
347
|
PUF-PAS |
14 sites in Gansu province |
Nov. 2010 to Mar. 2011, Jun. to Sep. 2011 |
PAHs |
348
|
PUF-PAS |
10 sites along the Lanzhou valley, Gansu province |
2013, two periods of 107 and 100 days |
PAHs |
383
|
PUF-PAS |
31 sites in Yangtze River Delta, Jiangsu province |
Jul. 2011 to Jun. 2012, four seasonal periods |
PAHs |
362
|
two seasonal periods |
SCCPs |
360
|
PUF-PAS |
11 sites within Guangdong province |
Jan. to Apr. and Jul. to Sep. 2015, two 2 month periods |
Levoglucosan, mannosan, galactosan |
251
|
PUF-PAS |
18 sites along the Lanzhou valley, Gansu province |
Winter 2014, summer 2015, two 12 week periods |
Nitrated PAHs |
384
|
PAD-DD |
G.1.3 National PAS network studies.
A number of studies involved networks of sampling sites across a country (Table 16). The scale of these studies varies obviously with the size of the country, ranging from as little as five sampling sites in Luxembourg385,386 to more than 90 sites within China.387,388 A number of these studies were conducted in countries with no prior measurements of SVOCs in the atmosphere and the PAS network served as an initial reconnaissance of atmospheric SVOC contamination. Examples are Kuwait,389 Costa Rica,280,390,391 Botswana,210 Ghana392,393 and Azerbaijan.394 Some studies focus on a particular type of sampling environment, e.g. on cities239,395 or national parks,396 whereas most national scale studies include a mix of remote, rural and urban sampling locations. Whereas most of these studies analyzed classical SVOCs, such as PCBs and OCPs, some had a focus on chemicals, for which the national database of atmospheric measurements may have been inadequate. Examples are studies on specific CUPs in Canada,397,398 on dechlorane plus in China399 and SCCPs and MCCPs in Australia.277 These studies typically relied on PUF-PASs and XAD-PASs, with deployment periods ranging from 1 month to 1 year.
Table 16 Studies relying on PAS network for SVOCs on a national scale
Sampler |
Location, no. of sites |
Time frame |
SVOCs |
Ref. |
PUF-PAS |
6 sites in Chile |
Dec. 2002 to Feb. 2003, 2 months |
OCPs, PCBs |
400
|
XAD-PAS |
20 sites in Chile |
Feb. 2006 to Jul. 2007, 1 year |
OCPs, CUPs, PAHs, PCBs |
195
|
PUF-PAS |
14 sites in Kuwait |
Feb. to Apr. 2004, 6 weeks |
PBDEs and PAHs |
389
|
XAD-PAS |
23 sites in Costa Rica |
Feb. 2004 to Feb. 2005, 1 year |
OCPs |
390
|
PAHs |
280
|
CUPs |
391
|
XAD-PAS |
7 sites in Costa Rica |
Oct. 2005 to Oct. 2006, 1 year |
OCPs, CUPs |
401
|
PFAS |
197
|
PUF-PAS |
11 sites in Mexico |
2005–2006, up to four consecutive 3 to 4 month periods |
OCPs |
402
|
PUF-PAS |
8 sites in Canada (PEI, QC, ON, SK, BC) |
2004 to 2005, 1 month |
Dacthal |
397
|
PUF-PAS |
10 sites in Central and Northern Canada (SK, AB, NV, NWT) |
May to Aug. 2005, May to Aug. 2007, 78 to 106 days |
Herbicides |
398
|
PUF-PAS |
97 sites in China |
Jul. to Oct. 2005, 3 months |
PCBs |
387
|
Dechlorane plus |
399
|
PUF-PAS |
40 sites in China |
Feb. to Mar., Apr. to May, Jul. to Aug., Sep. to Nov. 2005, 8 weeks |
PAHs |
403
|
OCPs |
404
|
PUF-PAS |
90 sites in Northern China |
Jun. to Oct. 2011 |
PBDEs, TBE, DBDPE |
388
|
PCNs |
405
|
Bromomono-aromatics |
406
|
PAHs, nitro-PAHs |
407
|
PUF-PAS |
18 sites in coastal India |
Jul. to Sep. 26, 2006, 6 weeks |
PCBs, PBDEs |
408
|
PUF-PAS |
68 sites in 7 Indian cities |
Dec. 2006 to Mar. 2007, 28 days |
OCPs |
395
|
20 sites in 3 Indian cities, 10 sites in Pakistan |
Winter 2006 (India) |
SCCPs, MCCPs |
409
|
Winter 2011 (Pakistan) |
|
PCNs |
410
|
PBDEs |
411
|
PUF-PAS |
9 sites in Indian agricultural areas |
2006–2007, four 3 month periods |
PCBs, OCPs |
412
|
PUF-PAS |
8 sites in Australia (WA, QLD, SA) |
Jan. to Feb. and Jul. to Aug. 2007, 40 to 50 days |
PAHs |
268
|
PCBs |
413
|
XAD-PAS |
15 sites in Australia |
Jan. 2012 to Mar. 2013, 1 year (325 to 414 days) |
OCPs, PCBs |
414
|
XAD-PAS |
15 sites in Australia |
Jan. 2016 to Jun. 2017, 1 year (302 to 408 days) |
SCCPs, MCCPs |
277
|
XAD-PAS |
15 sites in Botswana |
May 2006 to May 2007, 1 year |
OCPs |
210
|
PFAS |
197
|
XAD-PAS |
36 sites in 19 USA National parks |
Summer 2005-summer 2006, 1 year |
OCPs, CUPs, PAHs |
396
|
PUF-PAS |
13 locations in Azerbaijan |
Nov. to Dec. 2008, 4 weeks |
PCBs, OCPs |
394
|
SIP-PAS PUF-PAS |
10 cities in Korea |
Apr. to Jul. 2009, 90 days |
PFAS |
239
|
PUF-PAS |
55 sites in Japan |
Mar. to May, Aug. to Oct., Nov. 2008 to Jan. 2009, 8 weeks |
PCBs, PCNs |
415
|
PUF-PAS |
13 sites in Ghana + 2 e-waste sites |
May to Jul. 2010, 56 days |
PCNs |
392
|
OCPs |
393
|
PCBs |
416
|
XAD-PAS |
5 sites in Luxembourg |
Jun. to Oct. 2008, 6 to 87 days |
Pesticides |
385
|
PAHs |
386
|
PUF-PAS |
15 sites in Vietnam |
Jun. to Aug. 2012, Dec. 2012 to Feb. 2013, two 6 week periods |
PCBs, OCPs |
417
|
PUF-PAS |
34 sites in 4 Nepalese cities (Kathmandu, Pokhara, Birgunj, Biratnagar) |
Aug. 2014 to Oct. 2014, one 8 week period |
OCPs, PCBs |
418
|
PUF-PAS |
32 sites in 16 provinces in Turkey |
May 2014 to Apr. 2015, four 3 month periods |
OCPs, PCBs, PBDEs |
419
|
PUF-PAS |
62 sites in China |
2016–2017, three periods of 7 to 8 weeks |
Unintentionally produced PCBs |
420
|
G.1.4 International regional network studies.
Table 17 compiles information on studies with PAS sampling sites across an international region. Most of these have been done in parts of Europe, but there are also two in Western Africa421,422 and a number in East Asia.423,424 One network, relying on SPMDs, has been measuring SVOC concentrations at 10 to 12 sites along a transect from Southern UK to Northern Norway from 1994 to 2008, with an unusually long deployment period of 2 years.40,42–45 Most of the other studies of this type used PUF-PASs and sampling periods ranging from 1 to 3 months. High capacity sorbents, i.e. XAD-PAS and SIP-PAS, find use when a year-long deployment is used.150,423 The studies using PE-PASs around the Laurentian Great Lakes also could be classified as international regional studies, but they are discussed separately in Section G.8.1, because they include paired air and water passive samplers.
Table 17 Studies relying on PAS network on a regional scale involving multiple countries
Sampler |
Location, no. of sites |
Time frame |
SVOCs |
Ref. |
PUF-PAS |
15 sites in the Laurentian Great Lakes region (USA, Canada) |
Jul. 2002 and Jun. 2003, four 3 month periods |
PCBs, PBDEs, OCPs |
425
|
PCNs |
426
|
Chiral pesticides |
427
|
XAD-PAS |
8 sites in Northeast Asia (South Korea, Mongolia, China) |
2007 to 2008, 1 year |
PCBs, OCPs, dechlorane plus |
423
|
SPMD |
10 sites from South of the UK to the north of Norway |
Summer 1994 to summer 1996, 2 years |
PCBs, α-HCH and γ-HCH |
40
|
SPMD |
12 sites from South of the UK to the north of Norway |
Summer 1998 to summer 2000, 2 years |
PCBs, HCB |
42
|
SPMD |
11 sites from South of the UK to the north of Norway |
Summer 2000 to summer 2002, 2 years |
PCBs, PBDEs, OCPs |
43
|
SPMD |
11 sites from South of the UK to the north of Norway |
Summer 2002 to summer 2004, 2 years |
PCBs, OCPs PBDEs, PAHs |
44
|
SPMD |
11 sites from South of the UK to the north of Norway |
Summer 2004 to summer 2008, two 2 year periods |
PCBs, PBDEs |
45
|
SIP-PAS |
10 sites from South of the UK to the north of Norway |
Jul. 2008 to Jun. 2009, 1 year |
PCBs, PBDEs, HCB |
150
|
SPMD |
40 sites in 5 European countries (Austria, Czech Republic, Poland, Slovakia, Sweden) |
Sep. to Dec. 1999 and Jun. to Aug. 2000, 21 days |
PAHs, nitro-PAHs |
47
|
PUF-PAS |
23 sites in 8 Northern European countries (Ireland, UK, Denmark, Norway, Sweden, Russia, Finland, Estonia) |
Aug. to Oct. 2004, 56 to 82 days |
PCBs, PAHs, PBDEs, HCB, p,p′-DDT, p,p′-DDE |
428
|
PUF-PAS |
11 sites around the Aegean Sea (Greece, Turkey) |
Jul. to Aug. 2012, one 1 month period |
OCPs, PCBs, PBDEs, PAHs |
103
|
PUF-PAS |
5 sites Mexico City, Mexico, Gothenburg, Sweden and Lancaster, UK |
Mar. to Apr. 2006, 42 to 50 days |
OCPs, PCBs, PBDEs |
429
|
PUF-PAS |
15 sites in 4 West African Countries (Ghana, Gambia, Sierra Leone, Ivory Coast) |
May to Jul. 2008, 90 days |
PCBs |
421
|
PUF-PAS |
19 sites in 4 West African countries (Togo, Benin, Nigeria, and Cameroon) |
2012, one 8 week period in rainy season, two 8 week periods in Cameroon |
OCPs |
422
|
PUF-PAS |
155 sites in 22 Central and Eastern Europe countries |
2006–2008; 6 periods of 28 day |
PAHs, OCPs, PCBs |
430
|
PUF-PAS |
45 sites in 3 East Asian countries (China, South Korea and Japan) |
Mar. to May, 2008, Aug. to Oct. 2008, 51 to 90 days |
PBDEs |
424
|
PUF-PAS |
6 sites in 4 East Asian countries (The Philippines, Japan, Malaysia, Vietnam) |
2011–2012, two ∼2 month periods (46 to 98 days) |
PCBs |
431
|
G.1.5 Continental PAS network studies.
A few international studies were sufficiently large in scope to categorise them as continental in scale (Table 18). An early network of XAD-PASs ranged from the High Canadian Arctic to Central America and from the Pacific to the Atlantic coast of North America.29,432,434 A number of studies were Pan-European in scale95,434–436 or covered large parts of East Asia.143,437–439 Two studies reported data on African networks440,441 and a regional Global Atmospheric Passive Sampling (GAPS) effort31,442,443 focussed on the group of Latin American and Caribbean countries. While an Australian national study277,414 could also be classified as continental, we have listed those studies in Table 16 in Section G.1.3 above.
Table 18 Studies involving continental scale PAS networks for SVOCs
Sampler |
Location, no. of sites |
Time frame |
SVOCs |
Ref. |
XAD-PAS |
40 sites in North and Central America (Canada, USA, Mexico, Belize, Costa Rica) |
May to Jul. 2000 to May to Jul. 2001, 1 year |
OCPs |
29
|
α-HCH, γ-HCH |
432
|
PCBs, PBDEs |
433
|
PUF-PAS |
71 sites in 22 European countries |
Jun. to Jul. 2002, 6 weeks |
PCBs, PBDEs, OCPs |
434
|
PAHs, PCNs |
435
|
POG |
38 sites in 19 European countries |
Jun. 2002, 1 week |
PCNs, PCBs, PAHs |
95
|
PUF-PAS |
86 sites in 34 European countries |
Summer 2006, 3 months |
PCBs, OCPs, PAHs |
436
|
PUF-PAS |
77 sites in East Asia (China, South Korea, Singapore) |
Sep. to Nov. 16, 2004, 56 days |
PCBs, OCPs, PBDEs |
437
|
SIP-PAS |
46 sites in East Asia (China, Taiwan, Japan, India) |
Mar. to Aug. 2009, 100 days |
PFAS |
143
|
PUF-PAS |
106 sites in East Asia (Japan, China, Korea, Taiwan) |
Mar. to May 2008, 8 weeks |
PCBs, PCNs |
438
|
PUF-PAS |
176 sites in Asia (China, India, Japan, Vietnam, South Korea) |
Sep. 2012vAug. 2013, 4 seasonal periods |
PAHs, alkylated PAHs |
439
|
PUF-PAS |
26 sites in 15 African countries |
Jan. to Jun. 2008, 28 days to 3 months |
PAHs, PCDD/Fs, PCBs, OCPs |
440
|
PUF-PAS |
20 sites in 12 African countries (166 samples) |
2010–2018, 2 to 3 month periods |
CUPs |
441
|
PUF-PAS |
7 sites in Latin America |
2014, two to four 3 month periods |
OPFRs |
442
|
PUF-PAS, SIP-PAS |
9 sites in Latin America |
2014/2015, up to eight 3 month periods, SIP for one period |
HCBD, PCA, dicofol, OCPs, PCBs |
31
|
BFRs, OFRs in PUFs, cVMS, PFAS in SIPs |
443
|
G.1.6 Global PAS network studies.
Table 19 lists the PAS network studies that were truly global in scale. Most of these were conducted as part of the Global Atmospheric Passive Sampling (GAPS) project, which is relying on PUF-PASs,127,208,444–447 XAD-PASs197,448,449 and SIP-PASs.139,147,151–153 Another global scale study with a focus on Africa, Latin America, the Caribbean, and the Pacific Islands also relied on the PUF-PAS.450 In some cases, first long-term time trends could be derived from samples taken in different years.153,197,448
Table 19 Studies involving global scale PAS networks for SVOCs
Sampler |
No. of sites |
Time frame |
SVOCs |
Ref. |
PUF-PAS |
7 sites |
2002, 2 to 7 months |
OCPs |
444
|
PUF-PAS |
41 sites in 28 countries |
Dec. 2004 to Mar. 2005, ∼3 months (70 to 232 days) |
OCPs, PCBs, PBDEs |
208
|
PCNs |
445
|
PUF-PAS |
41 sites in 36 countries |
Dec. 2004 to Dec. 2005, 4 consecutive 3 month periods |
OCPs, PCBs, PBDEs |
127
|
SIP-PAS and PUF-PAS |
20 sites |
Mar. 2009 to Oct. 2009, 86 to 130 days |
PFAS, PCBs |
139
|
VMS |
147
|
OCPs, PBDEs |
151
|
CUPs |
152
|
XAD-PAS |
36 to 46 sites |
2005 to 2008, 4 consecutive 1 year periods |
OCPs, CUPs |
448
|
XAD-PAS |
34 to 35 sites |
2005 to 2006, two 1 year periods |
PAHs |
449
|
PUF-PAS |
31 sites in 11 countries |
3 to 6 months |
PCBs, PCDD/Fs, DDT-compounds |
450
|
XAD-PAS |
17 to 46 sites |
2006 to 2011, six consecutive 1 year periods |
PFAS |
197
|
PUF-PAS |
40 sites |
2005 to 2006, four consecutive seasonal periods |
BFRs |
446
|
PUF-PAS |
48 sites |
2014, several consecutive ∼3 month periods |
PBDEs, HBCD, BFRs, chlorinated FRs, OPEs |
447
|
SIP-PAS |
21 sites |
Apr. to Jul. 2015, one 3 month period, 2013, four consecutive ∼3 month periods |
PFAS, VMS |
153
|
G.2 PAS transects
Another common application of PASs involves a transect of sampling sites along which a change in air concentration may be expected. Again, the scale of such transects varies widely. At the smallest scale, PASs have been used to quantify the impact of roadways and other traffic arteries on the concentrations of SVOCs.451,452 Some of the local scale studies in Section G.1.1 seeking to quantify the influence of specific point sources (Table 13) have relied on linear transects. Quite frequently, the air concentration variability along urban to rural transitions (Table 20) has been studied, with most of these studies sampling North of Toronto, likely because this is an area of such clear transition in population density and industrial and agricultural activity.453–458 Harrad et al. sampled a transect across the Birmingham region.459,460 There is obviously some overlap between transect studies sampling along an urban-rural gradient and the urban network studies in Section G.1.1, if those networks include urban, suburban and rural sites (Table 14).
Table 20 Studies deploying PASs along urban to rural transects
Sampler |
Location, no. of sites |
Time frame |
SVOCs |
Ref. |
PUF-PAS SPMD |
7 sites North of Toronto, Canada |
Jul. to Oct. 2000, 120 days |
PCBs, OCPs |
453
|
PUF-PAS |
7 sites North of Toronto, Canada |
Jun. 2000 to Jul. 2001, three 4 month periods |
PAHs, PCBs, OCPs |
454
|
PBDEs |
456
|
PUF-PAS |
7 sites North of Toronto, Canada |
Apr. to May 2002, 1 month |
PCBs, PBDEs |
455
|
PUF-PAS |
8 sites North of Toronto, Canada |
May to Oct. 2003, seven consecutive 1 month periods |
CUPs |
457
|
PUF-PAS |
10 sites across Birmingham, UK |
Aug. 2003 to Oct. 2004; eleven 1 month periods |
PBDEs |
459
|
PCBs |
460
|
PUF-PAS |
19 sites North, East and West of Toronto, Canada |
Oct. 2007 to Oct. 2008, four 3 month periods |
PCBs, PBDEs, PAHs |
458
|
At the largest scale, PAS transects explore air concentration variability with latitude. These studies have already been mentioned in the context of network studies in Section G.1. Most notable among those are the transects in Northern Europe,40,42–45,150 along the length of Chile,195,400 and across North America.29,432,433 The northern part of the latter transect from Southern Canada to the High Arctic is particularly useful because there are few, if any, emission sources that would confound the recorded air concentration gradient. This transect thus constitutes a gradient of source proximity or remoteness449,461 and lends itself to the derivation of empirical travel distances.29
G.3 PAS vertical gradients
Another frequent theme of PAS studies for SVOCs is their use in the determinations of vertical concentration gradients. Again, these studies have a range of spatial scales.
G.3.1 Small scale gradients above soil.
A number of research groups have sought to quantify small scale (<2 m) vertical gradients of SVOCs above soil (Table 21). The rationale is to deduce the direction and extent of diffusive air–soil exchange. This is a particularly challenging application of PASs, because the concentration differences on such a small scale may be too small to be confidently established, considering the limited precision of most PASs. This is particularly so, because wind speeds decrease close to the ground, and therefore the SR of a PAS will also decrease. It thus needs to be confirmed that sequestered amounts that increase with PAS deployment height are not due to a gradient in the uptake kinetics, but truly reflect a depositional flux of the SVOC. In most cases, this will require the use of DCs to quantify the extent to which wind exposure of the PASs varies with height.
Table 21 Studies using PASs to measure air concentration gradients of SVOCs above soil
Sampler |
Location/time frame |
Sampling height |
SVOCs |
Ref. |
SPMD |
1 contaminated site in landfill, Bitterfeld, Germany |
20 and 150 cm |
HCHs, HCB, DDTs |
462
|
PUF disks in stainless steel can with open mesh-covered bottom (3 cm diameter opening) |
1 contaminated and 2 uncontaminated sites, Beijing, China, one 2 month period |
0.5, 2, 3, 4, 5, 8, 12, 15, 170, 20, 25, 30, 40, 45 cm, 6 per height |
PAHs |
169
|
Regular PUF-PAS |
1 rice paddy field, Guangzhou, China, two ∼6 week periods |
Above (120 cm) and below (40 cm) the rice crop canopy, 6 per height |
PAHs, OCPs |
463
|
PUF disks in ABS can with bottom that has holes |
Sealed PVC chamber within greenhouse over soil spread on the ground, six 10 day periods |
5–8, 15–18, 30–33 cm, no replicates |
HCHs, DDTs |
170
|
PUF disk in stainless steel housing with open mesh-covered bottom (4 cm diameter opening) |
Pasture in Tibet, two 2 month periods |
2, 3, 5, 8, 10, 15, 20, 50, 80, 120, 200 cm, 3 per height pooled |
OCPs |
171
|
PE strip, 5 per metal box with open bottom over soil, 5 per box in upright metal chimney in air |
3 historically contaminated sites (Anniston, Alabama Wyckoff, Washington, Mosie, Oregon) in USA, one 14 day period |
4 replicates right above soil and 3 replicates at ca. 150 cm above it |
PAHs, PCBs |
70
|
PE strips placed beneath a metal plate |
1 urban green space, Shanghai, China, seven 2 to 8 week periods |
5, 10, 15, 20, 25, 30, 35, 40, 45, 50, 60, 70, 80, 90, 100, 150, 200 cm |
PAHs |
76
|
This application of PASs has spawned a whole range of custom-designed PAS, presumably because the most commonly used PASs are too bulky and their sampling height too poorly constrained for finely resolved measurements. In particular, the PUF-based samplers used for fine-scale vertical gradients have small housings that envelop the foam disk and are only open at the bottom. As a result, they have lower SRs than the regular PUF-PAS.169–171
Zhang et al. observed levels that decline with height at contaminated site and levels that increase with height at two other sites, but those gradients were only apparent very close to the soil surface (<3 cm).169 They were interpreted as indicating volatilisation and deposition, respectively. No attempt was made to account for the possibility that the SR is reduced so close the ground surface, even though a photograph of one of the sampling sites shows a vegetation cover. Wang et al. used a slightly modified version of that sampler with double the surface area to record concentration gradients above a Tibetan pasture, but did use DCs to correct for differences in the SR with height.171 Strangely, while SRs varied considerably (ranging from 1 to 3 m3 per day), they showed no apparent relationship with height. α-HCH and o,p′-DDT showed lower levels close to the ground during both summer and winter, indicative of deposition.
Since the largest concentration difference can be expected to occur between the bulk atmosphere and the air right next to the soil surface, Donald and Anderson proposed to only sample at those two heights using PE strips placed in custom-made metal boxes.70 DCs are used to correct for variable atmospheric turbulence in the boxes, which was much higher in air than in soil air samplers. Above two contaminated soils, air concentrations at ground were found higher than at height, indicative of a potential for evaporation. At one site, the gradient was reversed, indicating that PAHs and PCBs are likely to deposit. Air–soil exchange fluxes were estimated using molecular diffusion across a stagnant boundary layer presumed to be 1 mm thick. More recently, this approach has been used to measure potential emissions of chemicals from artificial turf.71
Liu et al. used PE strips covered by a metal plate to sample PAHs in air at numerous heights above the ground.76 SRs derived from DCs were strongly dependent on height, ranging from 2 to 3 m3 per day at the lowest sampling heights to 20 m3 per day and higher for PASs deployed more than 50 cm above ground. Concentration gradients indicated deposition of lighter PAHs and volatilisation of heavier PAHs. Relatively large variations in the reported air concentrations at greater heights, where the air should to be reasonaly well-mixed, indicate a fairly high uncertainty of the recorded concentrations.
Wang et al. used the PUF-PAS to measure concentrations of OCPs and PAHs above and below the canopy of a rice crop, using DCs to allow for different SRs.463 Following expectations, SRs were notably lower below the canopy than above it. Both lower and higher concentrations were observed below the canopy when compared to those measured above.
The modified Bowen ratio method for flux estimation, which relies on vertical concentration gradients, can become problematic when the time resolution of the concentration measurements is too coarse.464 In particular, if during the sampling period, the air–soil exchange flux changes direction, the concentration gradient cannot be interpreted in terms of a flux.464 In the case of SVOCs, it is quite likely that air–soil exchange fluxes experience day and night differences. PAS sampling periods are almost always longer than a day. If, however, it can be assumed that the direction of net flux does not change during the sampling period, the performance of the modified Bowen ratio method is not strongly affected by the length of sampler deployment.464 The condition to measure meaningful vertical concentration differences with PASs is therefore most likely fulfilled when sampling above a highly contaminated soil, because (i) a wind speed/SR gradient would only counter and not create a decrease in the sequestered amounts with PAS deployment height, i.e. a measured contamination gradient is less likely to be a sampling artefact, and (ii) SVOC evaporation is likely to occur continuously, i.e. it is less likely that the net flux direction changes during a deployment. None of the studies reporting SVOC concentration gradients above soil measured with PASs seems to be aware of the limitations of deriving fluxes from long term average concentrations.
G.3.2 Gradients on towers.
At a somewhat larger scale, five studies have used towers to make vertical SVOC concentration measurements with PASs94,130,135,465,466 (Table 22). The issue of wind speed gradients possibly resulting in an SR gradient (as discussed in Section G.3.1) applies here as well. For example, Chaemfa et al. attributed lower sequestered amounts of PCBs at the lowest sampling height to differences in wind speed with height.135 Moeckel et al. quantified the gradient in SR with the help of DCs and noted a four-fold increase of the SR of the PUF-PAS between ground level and 100 m (2 to 8 m3 per day).130 Similarly, Li et al. noted an increase in SR from around 7 m3 per day at 15 m to 11 to 12 m3 per day at 320 m.466 Using height-specific SRs to derive air concentrations largely eliminated a gradient of increasing sequestered amounts of PCBs and OCPs with elevation in a Swedish forest.130 On a tower in Beijing, levels were still slightly higher closer to the ground, suggestive of urban ground sources.466 Both of these studies suggest that DC-corrected SRs are imperative when trying to measure vertical gradients with the PUF-PAS, whose SR becomes highly susceptible to wind at the wind speeds encountered at height.130,466
Table 22 Studies involving PASs measuring vertical gradients of SVOC concentrations on a tower
Sampler |
Location, sampling heights |
Time frame |
SVOCs |
Ref. |
POG |
CN Tower, Toronto, Canada |
Oct. 2001, three 1 week periods |
PAHs, PCBs, OCPs |
94
|
6 heights (30, 90, 150, 210, 270, 360 m) |
PUF-PAS |
CN Tower, Toronto, Canada |
May to Sep. 2005, five 1 month periods |
OCPs |
465
|
5 heights (30, 90, 150, 210, 270 m) |
PUF-PAS |
Meteorological tower Hazelrigg field station, Lancaster, UK |
Apr. to Jun. 2006, one 54 day period |
PCBs, OCPs |
135
|
5 heights (3, 5, 10, 20, 30 m) |
PUF-PAS, SPMD |
Norunda Common, Sweden |
May to Jul. 2007, one 40 day period |
PCBs, OCPs |
130
|
8–9 heights (2.5, 9, 14, 19, 25, 44, 68, 73, 96 m) |
PUF-PAS |
Meteorological tower, Bejing, China |
Dec. 2006 to Jan., May to Jul., Jul. to Aug. 2007, three 2 month periods |
PCBs, OCPs |
466
|
9 heights (15, 47, 80, 120, 160, 200, 240, 280, 320 m) |
G.3.3 Vertical gradients in mountains.
At an even larger vertical scale, PASs have been used repeatedly to study air concentration gradients along mountain slopes (Table 23). Often, those studies could also be classified as transects as defined in Section G.2 above. Studies have been conducted on mountains in Europe,467,468 Western Canada,452,469–471 South American mountains,195,472–475 the Southern and Eastern slope of the Himalayas,65,194,476–481 as well as on the island of Hawaii.482 The largest elevational range exceeds 5000 m on the Southern slope of the Himalayas.476,479
Table 23 Studies using PASs to record gradients in SVOC air concentrations along mountain slopes
PAS |
Location, no. of sites |
Time frame |
SVOCs |
Ref. |
SPMD |
3 sites (1600, 2240, 2600 m) in the Pyrenees, Catalonia, Spain |
Mar. 2002 to Jun. 2003, 85, 161 and 210 days |
HCB, PCBs |
467
|
PUF-PAS |
12 or 13 sites at four elevations (700, 1010, 1420, 1790 m) on Mont Mars, Alps, Italy |
Sep. to Oct. and Oct. to Nov. 2003, 52 and 33 days |
PCBs, HCB, p,p′-DDE, p,p′-DDT |
468
|
XAD-PAS |
8 sites (570–1951 m) on Mount Revelstoke, BC, Canada |
Aug. 2003 to Aug. 2004, one 1 year period |
OCPs |
469
|
6 sites (1109–2561 m) in Yoho National Park, BC, Canada |
PAHs |
452
|
8 sites (1402–2902 m) on Observation Peak, Banff National Park, Alberta, Canada |
XAD-PAS |
5 sites (800–2740 m) in Canadian Rocky and Purcell Mtns., British Columbia, Canada |
Mar. to Aug. 2004, one 5 month period |
PFAS, HCHs, endosulfan |
470
|
PUF-PAS |
4 sites (1820, 2600, 4650, 5200 m) on east side of the Andes, Bolivia |
Feb. 2005 to Jan. 2006, four 3 month periods |
OCPs, PCBs |
472
|
XAD-PAS |
9 sites (1242–4485 m) on Balang Mtn., Wolong Nature Reserve, Sichuan, China |
2005 to 2008, five 6 month periods |
OCPs, PCBs |
194
|
PUF-PAS |
4 sites (400–2200 m) in Serra dos Orgaos National Park, Brazil |
Jun. to Aug. 2007 and Dec. 2007 to Mar. 2008, two 90 day periods |
PCBs, PBDEs |
473
|
|
4 sites (600–1800 m) in Sao Joaquim National Park, Brazil |
OCPs |
474
|
XAD-PAS |
6 sites (135–5100 m) on the Southern slope of the Himalayas in Nepal |
May to Nov. 2012 |
OCPs, PCBs |
476
|
PAHs |
477
|
PUF-PAS |
3 sites (3800, 4200, 4400 m) on S-slope, 2 sites (4200, 4400 m) on N-slope of Sygera Mtn., Tibet, China |
Jan. 2008 to Jan. 2012, sixteen 3 month periods |
OCPs, PCBs |
478
|
XAD-PAS |
18 sites (1983–4553 m) on Shergyla Mtn., Tibet, China |
Jul. 2010 to May 2011, two periods of 4 and 7 months |
OCPs, PCBs, PBDEs, HBCD |
65
|
XAD-PAS |
6 sites (48–4405 m) in Northern Chile |
Feb. 2006 to Jul. 2007, one 1 year period |
OCPs, CUPs, |
195
|
7 sites (10–1874 m) in Central Chile |
PAHs, PCBs |
5 sites (50–760 m) in Southern Chile |
XAD-PAS |
3 sites (500, 1310, 2052 m) in Swiss Alps, Switzerland |
Jul. 2006 to Oct. 2006, one 4 month period |
OCPs, PCBs |
483
|
XAD-PAS |
9 sites (0 to 3400 m) on Hawaii Big Island |
May to Sep. 2011 |
OCPs, PAHs, PBDEs |
193 and 482
|
XAD-PAS |
18 or 20 sites (1983–4553 m) on Shergyla Mtn., Tibet, China |
Jul. 2012 to Jul. 2015, one 1 year period |
SCCPs, MCCPs |
480 and 481
|
LDPE-PAS |
4 sites (700 to 2400 m) on Serra do Mar and 4 sites on (990 to 1700 m) on Serra Geral, Brazil |
May to Jun. 2012, one 30 to 40 day period |
PAHs |
484
|
XAD-PAS |
4 sites (60–600 m) on Mt. Anderson, BC, Canada |
Aug. 2007 to Aug. 2008, 1 year period |
PAHs, PCBs |
471
|
4 sites (350–950 m) on Grouse Mtn. |
4 sites (90–700 m) on Four Brothers Mtn. |
3 sites (400–800 m) on Blue Grouse Mtn. |
1 site on Chilkoot Trail (435 m) |
XAD-PAS |
8 to 9 sites (100–5200 m) on the S-slope of the Himalayas in Nepal |
May 2012 to Nov. 2014, five half year periods |
OCPs, PCBs |
479
|
The issue of SR variability with elevation can also arise along mountain transects, where the wind speed may vary between sites at different elevation. The majority of these studies used the XAD-PAS, but the PUF-PAS and SPMDs have also found use. Because DCs cannot be used with the XAD-PAS, other ways to correct for variable SRs may be required. Sites where a PAS is exposed to very high wind can often be identified when all target chemicals have elevated sequestered amounts.194 The deployment time-normalised sequestered amount of a chemical that can be assumed to have uniform air concentrations along the elevation gradient has been used to adjust SRs. The amounts of HCB sequestered by a XAD-PAS can often serve this purpose, as long as there are no major sources of HCB in the vicinity of the transect.194,195,482
A common theme among those studies is the investigation of mountain cold-trapping, especially when combined with measurements of concentrations in soils (e.g.ref. 391, see also Section G.8.2). Remarkably, air concentrations of endosulfan are often found to increase with elevation,65,195,470 consistent with its high potential for mountain cold trapping.195,391 Some studies compare forested sites with sites in clearings to explore the influence of vegetation.65,468,471,478 Others compare the levels from sampling sites on different sides of a mountain.65,471 In many cases, source proximity is found to govern the air concentration variability along such transects.471 For example, proximity to traffic arteries is the main variable controlling PAHs concentrations in Canadian mountain air452 and on Hawaii, the highest concentrations of many OCPs occur in the vicinity of major towns.482
G.4 Spatial variability in indoor air concentrations and inhalation exposure
Air concentrations vary of course also in different indoor environments, depending mostly on the presence and strength of indoor sources, but also on characteristics of the indoor environment, such as room ventilation rate or the availability of sorbing compartments. Interest in air concentration variability in indoor environments is driven by the desire to characterise human inhalation exposure to SVOCs, considering that most humans spend the majority of their time indoors. While for most SVOCs, exposure routes other than inhalation are considered dominant and health-relevant, inhalation exposure can be an important contributor to total exposure, e.g. if concentration levels are very high.485 Occupational environments are particularly susceptible to having high indoor concentrations of SVOCs.
PASs have been used in several studies seeking to measure air concentrations of SVOCs indoors (Table 24). While access to electricity is usually not difficult in indoor environments and the use of active air sampling techniques is often feasible,486 PAS-based approaches tend to be less noisy and obtrusive and allow for cost-effective sampling in a larger number of locations,487e.g. in various locations within a room108 or within a building,488 or, more typically, in a number of different buildings.145,161,489 A common theme is also the use of PASs to compare levels in different types of indoor environments, such as offices and residences,489 or between the indoors and outdoors.146,161,490 The ultimate goal is often the assessment of personal inhalation exposure for large, epidemiological health studies.491,492 In this context, there is also interest in portable, personal PASs that measures concentrations of SVOCs in the breathing zone of an individual.166,167
Table 24 Studies using PASs to study SVOC air concentrations indoors
PAS |
Location, no. of sites |
Time frame |
SVOCs |
Ref. |
SPMDs |
10 homes and outdoor sites in Makwanpur region, Nepal |
One 11 day period |
PAHs |
493
|
PUF-PAS w/o double bowl |
74 homes and 7 outdoor locations in Ottawa, Ontario, Canada |
One 3 week period (outdoor 3 months) |
PBDEs |
161
|
59 homes for FOSE/FOSA |
MeFOSE, EtFOSE, EtFOSA, MeFOSEA |
162
|
52 homes for FTOHs |
10:2 and 8:2 FTOH |
136
|
SPMDs |
15 homes in Hagfors, Sweden |
One 2 week period |
PAHs, PCBs |
49
|
PUF-PAS |
31 homes, 33 offices, 25 cars, 3 public indoor spaces in Birmingham, UK |
One 28 day period |
PCBs, PBDEs |
489
|
PUF-PAS w/o DB |
35 homes and 11 outdoor locations in Mexico City, Mexico, Gothenburg, Sweden and Lancaster, UK |
One 6 to 7 week period |
PAHs, OCPs, PCBs, PBDEs |
429
|
SPMDs |
52 homes situated along the border between Arizona and Mexico (four locations in each home, pooled) |
One 30 day period |
PAHs, PCBs, OCPs, some non-target |
488
|
SIP-PAS |
59 homes and 6 outdoor locations in Vancouver, Canada |
One 4 week period (outdoor 3 months) |
Neutral and ionic PFAS |
145
|
SIP-PAS |
6 homes, 2 public indoor spaces and 6 outdoor sites in 3 major cities |
One 20 day period (indoor) one 90 day period (outdoor) |
Neutral PFAS |
239
|
PUF-PAS |
17 indoor and 18 outdoor sites in 10 cities in South Korea |
SIP-PAS |
20 homes, 12 offices, and 10 outdoor locations in Birmingham, UK |
One 1 month period, 12 monthly periods at 8 sites |
Neutral and ionic PFAS |
146
|
PUF-PAS |
20 homes, laboratories and offices in Toronto, Canada |
One 27 to 38 day period |
PCBs, PBDEs |
498
|
Synthetic musk |
288
|
Triolein coated vial |
2 car interiors, 4 occupational indoor, 12 outdoor sites in Malaysia |
One 37 day period |
PAHs |
499
|
PUF-PAS (single bowl in North America) |
63 homes in Bloomington, Indiana, United States, Toronto, Canada, Brno, Czech Republic, 1 or 2 rooms per home, 23 homes in Toronto, Canada, 20 homes in Brno, Czech Republic |
One 28 day period |
PBDEs, BFRs |
500
|
13 OPFRs |
501
|
OCPs, PCBs |
502
|
PUF-PAS |
Inside and outside of 6 schools in East Chicago, Indiana and Columbus Junction, Iowa, USA |
Multiple periods of 48 days average (22 to 114 days) |
PCBs, hydroxylated PCBs |
492
|
PUF-PAS |
Indoors and outdoors at schools and homes (n = 293), Greater Chicago Metropolitan Area, USA |
Quarterly (homes), bi-quarterly (schools), 45 days |
PCBs |
491
|
PUF-PAS |
20 homes, offices, 10 outdoor in suburban and urban in Shanghai, China |
Two 56 day period |
PBDEs |
503
|
PUF-PAS |
15 indoor samples (university, hospital, school) and 2 outdoor samples (playing area at university) in Ouargla city, Algeria |
One 61 day period |
PAHs, phthalate |
504
|
PUF-PAS |
34 homes in Pokhara, Birgunj, Biratnagar and Kathmandu, Nepal |
One 2 month period |
PBDEs, HFRs |
505
|
PUF-PAS |
20 homes (20 living rooms and 13 bedrooms) and 20 offices in Hangzhou, China |
One 90 day period |
PBDEs |
506
|
PUF-PAS |
Three offices (low-use, medium-use and high-use) in Beijing, China |
Three consecutive 28 day periods |
BFRs |
507
|
PUF/GFF-PAS |
SIP-PAS |
57 homes in Kuopio, Finland |
One 21 day period |
PFAS |
508
|
PUF-PAS |
16 sites in offices and residential buildings in Beijing, China |
Two 60 day periods |
SCCPs, MCCPs |
509
|
PUF-PAS |
16 residences in Iowa City, Iowa |
One 6 week period |
PCBs |
165
|
PUF-PAS and PDMS |
51 homes in Ottawa and Toronto, Canada |
One 3 week period |
Phthalates, PBDEs, novel BFRs, OPFRs |
112
|
LDPE-PAS |
33 apartments, 33 car interiors, 60 offices, 30 outdoor locations in Alexandria, Egypt |
One 2 month period |
OPFRs |
494
|
SIP-PAS |
3 buildings in Uppsala, Sweden |
One 140 day period |
FTOHs, cVMS, BFRs, OPFRs |
495
|
LDPE-PAS |
6 urban sites with matched indoor and outdoor samples in Eugene, Oregon |
Seven 24 hour samples for 1 week |
63 PAHs |
490
|
PUF-PAS modified |
14 residential houses and 14 offices in Brisbane and Canberra, Australia |
One 2 to 3 month period |
PAHs, PCBs, OCPs, CUPs |
163
|
PUF-PAS |
25 homes in Harbin, China |
One 30 day period |
PAHs, phthalates, PBDEs, OPEs |
510
|
PUF-PAS |
8 residential buildings and 3 outdoor locations in Bursa, Turkey |
Two 44 to 45 day periods |
PCBs |
511
|
PDMS |
63–71 apartments in Toronto, Canada |
Two 1 week periods |
PBDEs, PAHs, BFRs, phthalates |
496
|
PUF-PAS (PUF-cyl) |
Two Swedish vessels (Icebreaker and cruise ship) |
Two 6 to 7 day periods |
PAHs, alkylated PAHs |
512
|
PUF-PAS |
11 biological, 15 chemical and 14 physical laboratories, 3 offices |
One 21 day period |
Phthalates |
513
|
A wide variety of PASs have been used indoors, including SPMDs,24,49,488,493 PE-based PASs,490,494 SIP-PASs,145,146,495 the original PUF-PAS24 or modification of it with different types of housing.156,163,164 Most recently, also a number of PASs based on PDMS have been introduced112,114 and used.496 While some studies compared different PASs with each other,113 others compared PAS-derived indoor air concentrations with concentrations in other media that may serve for exposure assessment, such as surface wipes, window films or house dust.497
G.5 Aspects related to using PASs for probing spatial variability
G.5.1 Reporting amounts sorbed per time period vs. volumetric concentrations.
As shown in eqn (5), an SR is required to convert the amount of an SVOC quantified in the sorbent of a PAS operating as a kinetic sampler into a volumetric air concentration. As discussed in Sections F.2 and F.3, SRs often have considerable uncertainty. If the main objective of a study is the recording of spatial patterns, as may be surmised for most of the studies listed in Sections G.1 to G.4 above, the conversion of sequestered amounts into volumetric air concentrations may not always be necessary. The key question then is whether the spatial concentration differences that a study seeks to resolve are larger than the uncertainty in the SR. If that is the case, the uncertainty in the SR is less of a concern and it may be sufficient to report the concentration patterns in units of mass sequestered per unit of time (e.g. per day or per the nominal deployment period such as one month or one year). Especially, if a generic SR is to be applied i.e. not one that is specific to a particular deployment, it may in fact be more transparent to not report volumetric air concentrations: the time-normalised sequestered amount has less uncertainty than the calculated concentration, because the latter inherits the uncertainty of the SR. Several early PAS studies had reported spatial results in amount per sampler or amount per sampler per time,29,280,390,391,432,433,448,469 but only recently has there been a re-emergence of support for not necessarily reporting volumetric air concentrations.514–517 This approach is clearly advisable when using a PAS whose SR under a given set of circumstances is not well established or even unknown. A good example is the study attaching a PAS to a gull, where it was entirely possible to compare the exposure of different birds using time-normalised sequestered amounts.518
The issue of SR uncertainty becomes paramount when small concentration differences are to be discerned. This issue is further compounded if the concentration differences occur along a spatial gradient where factors with an influence on the SR (most notably wind speed, but possibly also temperature) may be expected to vary systematically. Examples are:
– Comparing indoor and outdoor deployments:490,492 indoor environments tend to experience much lower air turbulence and therefore lower SRs, which could lead to the underestimation of indoor concentrations when compared to outdoor deployment.
– Elevational transects of any scale (see Section G.3) can be expected to experience gradients of wind speed, with wind speeds increasing with height. This is particularly problematic when trying to observe air concentration gradients that increase with elevation.
– Comparing deployments under a forest canopy to deployments in unsheltered locations.65 The SR for the PUF-PAS is predicted to be much lower in forested regions and much higher over the ocean.126
When concentration differences between sites with different wind exposure are small, it is necessary to derive deployment-specific SRs, e.g. through the use of DCs, or choose a sampler design with an SR that is not, or at least less, dependent on wind speed. For example, the application of DC-derived, elevation-specific SRs changed the concentration gradient with height along a tower.130
It is important to realise that during the interpretation of spatial PAS data, one is not limited to comparing concentrations or time-normalised sequestered amounts, but it is possible to investigate compositional changes. If wind speed influences SRs, it does so for all target chemicals and not just for one. Compositional patterns thus are not, or at least less, affected by spatial variability in wind. Cluster analysis is particularly useful when interpreting compositional changes across a PAS network.477,519,520
Of course, there are often objectives of PAS studies that go beyond deciphering of spatial patterns and these may require the calculation of volumetric air concentrations. Examples are: (i) data obtained from different sampling methodologies are to be compared,521 (ii) PAS data are to be combined with data from adjoining environmental media such as water or soil in order to derive information on air–surface exchange, which is discussed in detail in Section G.8 below, or (iii) PAS-derived data on inhalation exposure are to be used in risk assessment, which is discussed in Sections G.4 and G.5.5.
G.5.2 Sampling site representativeness and scale.
The number of sites in PAS networks varies widely from less than a handful to more than 100. No matter how large the number of sites within a network may be, the issue of representativeness of sites is important. This is particularly relevant for large scale networks, where a site is often meant to represent a large area. In some of the continental or global networks listed in Sections G.1.5 and G.1.6, an entire country may often be represented by a single sampling site. Depending on the nature of that site, completely different results could be obtained. For example, within GAPS, the French site often shows the highest concentrations, merely by virtue of being located within Paris.127,447
Gawor et al.197 analyzed PASs from a global and two national networks for PFAS and used it to assess to what extent one site from the global GAPS network in each of Botswana and Costa Rica represents the national average as obtained from national networks in those two countries. In both cases, the levels of PFAS measured at the GAPS site were lower than the levels measured across the country, suggesting that these sites are representative of the more remote parts of Botswana and Costa Rica.
One potential application of a PAS network is in fact to assist in the selection of a representative sampling site or in the determination of the number of sites that is required to appropriately represent a particular area. For example, the purpose of a PAS network across Chicago was to establish how representative an active air sampling site is of the city as a whole.308 A network of PASs across the Czech Republic was used to address the issue of representativeness more comprehensively by not only comparing absolute concentration levels of different SVOCs but also time trends obtained from those sites.516 Cluster analysis based on levels and trends revealed that the fourteen sites belonged to one of three types, whereby sites belonging to a cluster share characteristics related to remoteness, landscape, population, and pollution sources and therefore also their pollution profile. An analysis like this could assist in optimizing the elimination of sampling sites from a network, by finding the number and location of sites that yield the most information with the least number of sites.516
G.5.3 Mapping and interpolation.
Related to this issue of site representativeness is the question of whether it is justified to interpolate the levels measured by PASs at different sampling sites, e.g. by geo-statistical methods, such as kriging. This is likely only appropriate if it can be assured that there are no major sources of an SVOC between any pair of sampling sites, e.g. in a small-scale network with one or a few clearly dominant emission sources (e.g. for some of the studies summarised in Table 13). A few studies have attempted to create urban concentration maps through interpolation (Fig. 10A).342,360 The results are not always very convincing,301,340 mostly because relatively large concentration differences can occur over small distances and the number of sampling sites is generally insufficient. When seeking to map urban air pollution, a minimum number of sampling sites of 0.29 multiplied with the urban surface to be monitored in km2 has been recommended, based on work with VOCs and NO2.522 None of the urban PAS networks for SVOCs (Table 14) comes close to fulfilling this criterion. A more defensible way of displaying the spatial variability of PAS networks is to use a map that visualises the variability in the measured levels, but does not seek to interpolate values in areas where no sampling was done (see Fig. 10B for a good example).
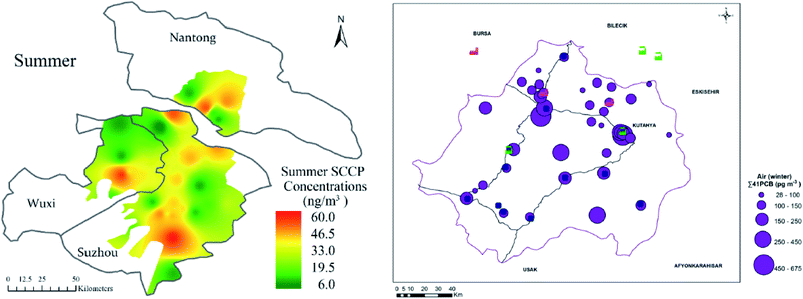 |
| Fig. 10 Two examples of how spatial variability in air concentrations as determined by PAS networks has been displayed, either using interpolation360 (left) or not363 (right). Reproduced from ref. 360 and 363 with permission from Elsevier, Copyright 2017 and 2020. | |
A more sophisticated strategy for urban pollution mapping is land use regression analysis, where air pollution monitoring data are combined with stochastic models that rely on predictor variables that are typically obtained through geographic information systems.523 While quite common for classical air pollutants (such as VOCs, PM2.5 and NO2), land use regression has, to the best of our knowledge, only been applied once to PAS data for SVOCs, namely to identify potential sources of PCBs, PBDEs, PAHs, and polycyclic musk compounds in Toronto.524 A large fraction of the observed variability in PCB air concentrations could be related to the distribution of PCBs in use/storage/building sealants, whereas that of the musk compounds was strongly related to population density.524
While not quite a land use regression, several PAS studies have sought to regress PAS-derived concentration data for PAHs with spatially resolved data on population density. PAHs concentrations measured at different sites of the global GAPS network have been regressed against four different means to quantitatively express the proximity of those sites to people.449 The remoteness index, which is derived from a global atmospheric transport model, explained as much as 60% of the variability in the concentration of fluoranthene. Despite the simplicity of this variable, the number of people living within 20 km of a sampling site explained even more of the variability in the concentrations of the sum of PAHs measured around the lower Great Lakes.82 Concentrations of SCCPs measured across Australia also correlated well with a number of variables, including population density, number of roads and site classification (i.e. remote, rural and urban).277
G.5.4 PAS networks and spatial modelling.
There have been several studies that used the spatial information from PAS networks in modelling studies. Sometimes this involves the comparison of the measured spatial distribution with one obtained from models. Fine-scale emission estimates and a dynamic atmospheric model have been used to simulate the distribution of acenaphthene in the atmosphere of Nanjing, China,338 and of DDTs, HCB, PCBs and PBDEs in Mendoza, Argentina.332 Empirical travel distances could be estimated from the air concentration variability across a latitudinal transect in North America to evaluate the characteristics travel distances of OCPs calculated with models designed to assess a chemical's long-range transport potential.29 At other times, models are used to describe the observed spatial variability. For example, a radial dilution model was applied to fit the SVOC concentration variability across Toronto and to probe the relative importance of sources within and outside of the city's central business district.458
G.5.5 PAS networks and human exposure and toxicity.
One of the motivations for interpolating PAS measurements of air pollution levels is their potential use for human exposure assessment and therefore use in epidemiological research. However, not for all air pollutants can a stationary network of PASs allow for a reliable prediction of personal exposure levels.525 For many SVOCs, air concentrations generally are less pertinent for human exposure and health effects, as inhalation is rarely a dominant pathway for exposure. The PAHs are an exception. Accordingly, numerous PAS studies have sought to estimate inhalation exposure and the health risk associated with exposure to airborne PAHs.336,348,361–363,439,526 This is typically done by summing the products of the concentration of carcinogenic PAHs with their relative potency factors in order to obtain an equivalent concentration of benzo[a]pyrene, which in turn can be converted into a chronic daily inhalation intake rate. Sometimes, a value for the cancer risk due to inhalation is derived as well.
Even though inhalation is generally not believed to represent a large fraction of the total intake of compounds with dioxin-like toxicity, some studies similarly calculate a toxic equivalence concentration (TEQ) using the product of the concentrations of the toxic PCDD/Fs, PCBs, and PCNs congeners and their toxic equivalent factors (TEFs), which in turn is used to calculate the TEQ intake by inhalation.309,312,345,382,416,527 In other studies, the inhalation exposure and risk are estimated for individual substances285,299 rather than for equivalent concentrations. Some studies performed probabilistic exposure-risk modelling.363,382 Again, often a cancer risk is estimated for dioxin-like substances. In other studies, the estimated inhalation exposure is compared with reference doses to estimate risk.304
In the above studies, the health risk assessment is based on the SVOC air concentrations measured with the PAS. Another approach to probing the spatial variability in inhalation toxicity is to perform in vitro bioassays using the extracts and dialysates obtained from PASs (Table 25). In the nine studies we found in the literature, either SPMDs, PUF-PASs or PE-PASs were deployed mostly in areas with elevated SVOC levels, namely urban location271,322,413,528–530 or sites impacted by industrial activity.327,531,532 The bioassays explored genotoxicity,322,327,413,528,529,531 dioxin-like toxicity modulated via the aryl hydrocarbon receptor (AhR),271,413,530,532 estrogenicity,530,532 and cytotoxicity.327
Table 25 Studies that use assays to probe the biological activity of extracts obtained through passive air sampling
Sampler |
Location, no. of sites |
Bioassays |
Ref. |
SPMDs |
5 sites in Caserta, Italy, One 3 week period |
Microtox (inhibition of bioluminescence of V. fischeri) |
528
|
Mutatox (genotoic reversion of dark mutants of V. fischeri) |
PUF-PAS |
20 sites across Brno, Czech Republic, one 28 day period in Oct./Nov. 2004 |
Bacterial genotoxicity test using E. coli sulA::lacZ |
322
|
SPMDs |
4 sites in Vilnius, Lithuania, four 8 week periods in different seasons in 2002–2003 |
Chromosome aberrations and sister chromatid exchanges (SCEs) in human blood lymphocytes in vitro |
529
|
SMART: somatic mutations and recombination in Drosophila melanogaster wing cells in vivo |
SPMDs |
6 sites in an occupational setting of a coke plant, incl. an office as a control, one 24 day period in winter |
Comet assay (in vitro DNA-damaging activity in human cells) |
531
|
Mutagenicity (Ames test TA98 strain, w/o S9) |
PUF-PAS |
6 offices and inside and outside of a suburban home in Brisbane, Queensland, Australia, one 40 to 50 day period in Apr. to Aug. 2007 |
Estrogenic (E-SCREEN-MCF7- BOS) |
530
|
AhR (CAFLUX-H4G1.1c2) |
PUF-PAS |
4 urban, 3 rural, and 1 background sites across Australia, two 40 to 50 days periods in Jan. to Feb. 2007 and Jul. to Aug. 2007 |
Indirect acting genotoxicity (umuC assay) |
413
|
AhR |
PUF-PAS |
1 background and 1 urban/industrial site in each of Lithuania, Slovakia, Romania, and Serbia, one 5 month period in Mar. to Aug. 2006 |
AhR (anti-)estrogenicity, (anti-)androgenicity |
532
|
PUF-PAS |
15 sites across the Athabasca Oil sands region, one 60 day period in Apr. to May 2014 |
Salmonella mutation |
327
|
Mammalian lactate dehydrogenase cytotoxicity |
PE-PAS |
9 sites in the Cleveland, Ohio area, one 60 day period in Jul. to Sep. 2013 |
AhR |
271
|
The PAS extracts were in most cases also subjected to chemical analysis, most frequently for PAHs. In some cases, more extensive chemical characterisation was undertaken, e.g. in addition to PAHs, extracts have been analysed for PCBs and OCPs,322 alkylated PAHs and benzothiophenes,327 and alkylated PAHs and OPE.271 By subjecting the extracts to H2SO4 treatment prior to the bioassay, it is possible to investigate whether recalcitrant or degradable compounds in the extracts are responsible for the detected biological activity.413,530 Eventually, the biological activity of samples deployed in different locations or during different seasons413 is compared. Studies typically also investigate whether biological activity and SVOC concentrations in samples correlate.
PASs have also been used for the assessment of exposure of birds to SVOCs. Stationary PUF-PAS deployed in Hamilton harbour were used to assess the exposure of double-crested cormorant colonies to PAHs,533 whereas a miniaturised sampler strapped to the back of gulls was used to observe that PASs attached to birds foraging in or around landfills accumulated higher levels of PBDEs, but not of three other BFRs.518
While the applications described in Sections G.1 to G.5 in some way take advantage of the ability of PASs to efficiently provide spatial information on SVOCs in the atmosphere, not all PAS studies are motivated by the need for characterisation of spatial variability. Some of the other application areas of PASs are discussed in Sections G.6 to G.8.
G.6 Extreme remote region monitoring (polar regions, Tibet, mountains)
Because they do not require power, PASs have found use in the monitoring of SVOCs in very remote or otherwise difficult to access locations. Examples are high mountain areas, and polar research stations and field camps. One challenge for sampling in such locales is often that access is very difficult and expensive and it may be only possible to visit sampling sites once a year. Long term deployments require a PAS with long tlinear, i.e. a high uptake capacity and the capability to work at extremely low temperatures. DCs often do not work well at low temperatures, because of insufficient loss rates,126 yet these remote sites often experience very high winds and are therefore in particular need of deployment-specific SRs. Its high uptake capacity explains why the XAD-PAS is frequently used in such situations.520,534–540 It is probably not by coincidence that PE, PUF and SIP-PASs are only used during the short and milder summer seasons.90,541,542
Another challenge for passive sampling in remote areas are the often very low SVOC concentrations, which, when combined with relatively low SRs, can result in sequestered amounts below detection limits. The FTS can achieve sampling volumes in the 1000s of m3 and is therefore used when regular site access is possible (e.g. in research stations that are operating year-round), but electricity supply is difficult. Even if a research station has electric power, it is often desirable to sample at a site sufficiently removed from station buildings and human activities (and therefore electricity) to avoid the possibility of contamination. The FTS has been used on the Tibetan plateau and in Arctic, sub-Arctic and Antarctic research stations.176–181
Table 26 summarises studies that have used PASs in extremely remote environments. A vertical mountain transect is a particular type of study in remote regions. These studies are discussed in Section G.3.3 above and summarised in Table 23. Some of the studies in extremely remote regions have been going on for several years and allow for the observation of time trends,181,539 which is further discussed in Section G.7 below.
Table 26 Studies using PASs in extremely remote environments
Sampler |
Location, no. of sites |
Time frame |
SVOCs |
Ref. |
SPMD |
3 sites (Weissfluhjoch, Switzerland, Zugspitze, Germany, Sonnblick, Austria) |
May to Nov. 2005, Dec. 2005 to May 2006, May 2005 to May 2006, Three periods |
OCPs |
60
|
XAD-PAS |
3 sites at each of Ny-Ålesund and King George Island, Antarctica |
Aug. 2005 to Aug. 2006 (Ny-Ålesund), Dec. 2004 to Dec. 2005 (King George) |
PCBs, OCPs |
534
|
XAD-PAS |
3 sites at each of King George Island, Ny-Ålesund, and Chuuk, South Pacific |
Dec. 2004 to Dec. 2007 (Ny-Ålesund), Aug. 2005 to Jun. 2007 (King George), Feb. 2006 to Oct. 2009 (Chuuk), three 1 year periods |
PCBs, OCPs |
535
|
XAD-PAS |
1 site at Cape Vera, Devon Island, Nunavut, Canada |
2006 to 2008, two 1 year periods |
HCB, p,p′-DDE, α-HCH, heptachlor epoxide, PCBs |
537
|
XAD-PAS |
16 sites on the Tibetan Plateau, China |
Jul. 2007 to Jun. 2008, 1 year period |
PCBs, OCPs, PBDEs |
536
|
13 sites |
|
PAHs |
543
|
16 sites |
2007 to 2012, five 1 year periods |
PCBs, OCPs |
520
|
SIP-PAS |
16 sites on the Tibetan Plateau, China |
May to Jul. 2011, May to Jul. 2013, two 3 month periods |
VMS, PFAS |
544
|
FTS |
1 site at Nam Co Lake, Tibet, China |
Oct. 2006 to Feb. 2008, fifteen 1 month periods |
PCBs, PAHs, OCP |
180
|
PBDEs, TBPH, EHTeBB, BTBPE |
176
|
FTS |
1 site at Alert, Nunavut, Canada |
Oct. 2007 to Dec. 2008, eleven consecutive one-month periods |
OCPs, PBDEs, TBPH, EHTBB, BTBPE |
177
|
FTS |
1 site at Little Fox Lake, Yukon, Canada |
Aug 2011 to Dec. 2014, 42 consecutive one-month periods |
PBDEs, non-BDE FRs, OCPs |
179
|
FTS |
2 sites at Toolok field station, Alaska, USA |
Jul. to Aug. 2013, three consecutive 18 day periods |
PBDEs |
178
|
FTS |
1 site at Casey station, Antarctica |
Dec. 2009 to Nov. 2014, thirty-three consecutive samples (4 to 6 weeks, some 3 months) |
PCBs, OCPs |
181
|
PUF-PAS |
5 sites on King George Island, Antarctica |
Dec. 2009 to Feb. 2010, 2 months |
PCBs, PBDEs |
541
|
XAD-PAS |
4 sites on King George Island, Antarctica |
2009–2010, 1 year |
PCBs |
538
|
PUF-PAS |
2 sites in National Parks Itatiaia (2470 m) and Serra dos Orgaos (2200 m), Brazil |
Sep. 2013 to Oct. 2015, eight ∼3 month periods |
OCPs, CUPs |
475
|
XAD-PAS |
7 sites on King George Island, Antarctica |
Dec. 2010 to Jan. 2018, 7 consecutive 1 year periods |
PCBs, OCPs, PBDEs |
539
|
LDPE |
1 site at Cape Bounty, Melville Island, Nunavut, Canada |
Aug. 2015, 21 days, Jun. to Aug. 2016, 59 days |
PCBs, HCB, α-HCH, p,p′-DDE |
90
|
SIP-PAS |
6 sites, Terra Nova Bay, East Antarctica |
Dec. 2010 to Jan. 2011, One 1 month period |
OCPs, PCBs |
542
|
XAD-PAS |
5 sites, Ny-Ålesund and London Island, Svalbard |
Aug. 2014 to Aug. 2015, 1 year |
OPEs |
549
|
G.7 Time trend monitoring
Long term time trends in the air concentrations of SVOCs are an important method to assess the effectiveness of measures to reduce emissions of those compounds. Traditionally, this is being done using active air sampling campaigns. However, such campaigns require a long-term commitment of fairly significant resources in terms of personnel and finances. With the Stockholm Convention, there is a greatly increased need for such time trend monitoring in the context of the Convention's effectiveness evaluation. An important motivation for developing PASs for SVOCs therefore was the prospect to determine long-term, interannual temporal trends in air concentrations much cheaper and with less effort. One of the advantages of PASs in this regard is that they can provide time-averaged concentrations over long time periods. The challenge of deriving long term trends from active sampling campaigns is that the latter usually sample only during a small fraction of time and therefore the time periods of active sampling need to be representative, which requires a relatively large number of samples.448
On the other hand, atmospheric concentration changes over time may be quite subtle and, considering the uncertainty of SRs for many PASs, it may be questioned whether the precision of PASs is sufficient to detect such changes. Shunthirasingham et al. have argued that the uncertainty of the SR is of diminished concern when the main interest is in the trend and not in the absolute concentration levels and if the PAS sampling sites are not changed.448 The reason is that the factors that result in SR variability (most notably wind speed) should be reasonably constant from year to year at the same site. Therefore, the uncertainty of the SR is not propagated to the uncertainty of the temporal trend. In fact, time trends can be derived from time-normalised sequestered amounts, i.e., no SRs are required at all.448,515,516
Kalina et al. investigated to what extent PAS-derived long-term trends agree with those derived from active air sampling campaigns.515,517 The first comparison involved 534 active air samples and 132 PUF-PASs taken over a period of more than 10 years in Košetice in the Czech Republic.515 The trends obtained from the two sampling methods generally agreed well, except when break-through of lighter SVOCs in the active sampling occurred or when levels of high molecular weight PAHs were too uncertain because of low levels close to the detection limit. In particular, the study confirmed that time-normalised sequestered amounts can be used for trend determination from PASs and suggested that it can be advantageous to use annually averaged PAS values. Kalina et al. expanded on this work by comparing time trends from active and passive air sampling at five additional sites in Northern Europe.517 While again good agreement in the trends from both sampling method was noted, the comparison was limited somewhat by the shorter time periods of side-by-side sampling.
By now several multi-year trends obtained by PASs have been reported. Table 27 lists the studies that have derived temporal trends from at least four consecutive years of passive sampling. The longest such trend so far could be obtained from the transect of SPMD deployments at 11 sites in the UK and Norway, which comprises 12 years of sampling between 1994 and 2008.150 This study clearly established declining trends of both PCBs and PBDEs in Northwestern Europe. Interannual time trends could also be derived from the XAD-PAS deployed within the global GAPS project for pesticides448 and neutral perfluoroalkyl substances.197 Somewhat confusingly, there are two national networks in Spain deriving temporal trends of SVOCs in the atmosphere. These networks, which have been sampling over the same time frame, using the same PUF-PAS, the same seasonal resolution, the same target compounds and even at a similar number of urban and rural locations, reported preliminary results from five years of sampling in 2016.545,546 More recently, one of the networks has reported on seven years of continuous monitoring for PCBs, PBDEs, PCDD/Fs and selected OCPs at 12 locations across the country.548 Among the findings are divergent trends for different PBDE congeners belonging to different technical mixtures,547 as well as significant declines in the levels of dioxin-like PCBs and PCDD/Fs.548
Table 27 Studies deriving interannual time trends in atmospheric SVOC contamination from PASs
Sampler |
Location, no. of sites |
Time frame |
Compounds |
Ref. |
SPMDs (n = 66) |
11 sites along UK-Norway transect |
1994–2008, 14 years, two-year resolution, gap: 1996–1998 |
PCBs, PBDEs |
45
|
XAD-PAS (n = 60) |
15 sites distributed globally |
2005–2008, 4 years, annual resolution |
OCPs, CUPs |
448
|
XAD-PAS (n = 78) |
13 sites distributed globally |
2006–2011, 6 years, annual resolution |
PFAS |
197
|
XAD-PAS (n = 80) |
16 background sites in Tibet, China |
2007–2012, 5 years, annual resolution |
PCBs, OCPs |
520
|
PUF-PAS (n = 17) |
1 urban site in São Paulo, Brazil |
2010–2015, 5 years, seasonal resolution |
PCBs, PCDD/Fs, OCPs |
550
|
PUF-PAS (n = 211) |
4 urban and 7 background sites across Spain |
2008–2013, 5 years, seasonal resolution |
DDTs, HCHs, HCB, PCBs, PBDEs, PCDD/Fs |
545
|
PUF-PAS |
5 urban and 7 background sites across Spain |
2008–2013, 5 years, seasonal resolution |
DDTs, HCHs, HCB, PCBs, PBDEs, PCDD/Fs |
546
|
PUF-PAS (n = 132) |
1 site in the Czech Republic |
2003–2013, 10 years, monthly resolution (28 days) |
OCPs, PCBs, PAHs |
515
|
PUF-PAS |
14 sites in the Czech Republic |
At most: 2004–2014 |
OCPs, PCBs, PAHs |
516
|
At least: 2007–2010, monthly resolution (28 days) |
PUF-PAS |
5 sites in Northern Europe |
2012–2016, min. of 5 years, seasonal resolution |
PCBs, OCPs, PBDEs |
517
|
FTS (n = 33) |
1 site in Antarctica |
Dec. 2009 to Nov. 2014 |
PCBs, OCPs |
181
|
PUF-PAS (n = 321) |
5 urban and 7 background sites across Spain |
2008–2015, 7 years, seasonal resolution |
PBDEs |
547
|
dlPCBs, PCDD/Fs |
548
|
XAD-PAS (n = 44) |
6 to 7 sites on the Fildes Peninsula, Antarctica |
2007–2017, 7 years, annual resolution |
PCBs, PBDEs, HCB, HCHs DDTs, endosulfans |
539
|
PUF-PAS (n = 35) |
1 site at Lake Hovsgol, Mongolia |
2008–2015, 8 years, three to five 2 month periods per year |
PCBs, OCPs |
549
|
Time trends of OCPs, PAHs, and PCBs have been derived from PUF-PASs deployed for multiple years at 14 sampling sites across the Czech Republic.516 Levels for most PCBs and HCHs declined significantly, but those of p,p′-DDE did not show trends and HCB and the PAH chrysene even showed increasing levels. Having time trends from multiple sites within a relatively small area allowed for the investigation of the spatial variability in those trends: three types of sampling sites could be identified which differ from each other in terms of the absolute levels and time trends. For example, four remote mountain sites were characterised by low levels of all SVOC types and decreasing levels of PAHs.516
PASs have particularly been useful for time trend determination in remote regions, where active sampling campaigns are often not feasible. Examples are Antarctica,181,539 Tibet520 or Mongolia.549 In Antarctic air, concentrations of PCBs, HCHs, DDTs and endosulfans declined over a seven-year period, whereas those of HCB did not.539 At a site in Mongolia, levels of HCB even increased, those of HCHs and DDTs declined, whereas there was no significant trend for the PCBs.549 Over a five-year period, concentrations of HCB and HCHs declined on the Tibetan plateau, but no such trend was apparent for DDT-related substances.520
In some cases, while there may not be an extended period of continuous sampling, it can be possible to infer time trends if sampling sites are revisited after a hiatus of several years. For example, levels of pesticides measured with PASs in Brazilian mountains in 2014/15 could be compared475 with those measured with the same technique in 2008.474 Similarly, time trends of cVMS and PFAS could be derived443 by comparing levels in PASs from 21 GAPS sites in 2013 and 2015 with those reported at the same sites in 2009.139,147
G.8 PASs in studies of air–surface exchange
Quite regularly, surface compartments, such as soil and water, are sampled at PAS deployment sites, often with the intention to learn something about the equilibrium status of SVOCs between the atmosphere and these compartments and possibly estimate air–surface exchange fluxes.
G.8.1 PASs in studies of air–water exchange.
The very first field study of a PAS involved the placement of SPMDs in the water and the overlying atmosphere of a coastal lagoon.35 Another early study also deployed SMPDs in air and water in the vicinity of a chemical factory to record the levels of PAHs36 and while both these studies estimated concentrations, no attempt was made to relate the concentration in different media with each other. On the other hand, Booij and van Drooge used four simultaneous deployments of SPMDs in air, water and within the sea surface microlayer to probe the equilibrium status of HCB and PCBs in the Western Wadden Sea of the Netherlands.37 The study indicated no enrichment of these SVOC at the water surface and a phase distribution close to equilibrium.
PE was first used to sample both air and water at the same location and derive an activity or fugacity gradient for PCBs and PAHs in Narragansett Bay.72,77 Differences in the activity or fugacity of a chemical between different compartments indicate deviations from thermodynamic equilibrium and therefore the potential for diffusive exchange between those compartments. Another early study using this approach was for PCDD/Fs in Newark Bay, New Jersey, USA, and revealed very strong fugacity gradients favouring volatilisation from the bay to the atmosphere.78 The approach is also suitable for sampling from a moving ship by deploying the PE-PAS on the ship's mast and sampling the water either by towing a PE strip behind the ship or pumping sea water through a pipe containing the PE strip.79 This approach was applied to study the air–water exchange of PBDEs along a cruise track across the tropical Atlantic Ocean.79
PE has been deployed in paired air and water passive samplers along the shores and on several on-lake locations of Lakes Ontario and Erie (number of sites varied from 13 to 22) during several ∼2 month long periods in 2011 and 2012 (number of deployments varied from 3 to 4 depending on location). Those samples have been analyzed for PAHs,82 OCPs,80 PCBs,81,85 PBDEs and other BFRs83 and musk compounds.84 In a similar set-up, PE samplers were deployed at eleven coastal and three open-lake sites on Lake Superior during three deployments in 2011, with quantification of PAHs, PBDEs87 and PCBs and OCPs.88 Error propagation analysis and Monte Carlo simulations have been used to estimate the uncertainty of air–water exchange fluxes that have been calculated from the air and water concentration obtained from PE-based passive samplers.86
PE-PASs have more recently been used to confirm the net deposition of OCPs and PAHs from the atmosphere to mountain lakes in Brazilian National Parks89,484 and to assess the air–water equilibrium of PCBs and OCPs in the Canadian High Arctic.90 While studies involving paired air and water samples have been dominated by PE-based PAS, not all studies used them. PUF-PAS-derived air concentrations have been combined with water concentrations obtained from a passive water sampler based on PDMS sheets to derive air–water fugacity ratios for selected PAHs, PCBs, OCPs, and PBDEs at three sites in the Aegean Sea.103 Accounting for the gas-phase fraction of the air concentration measured by PUF-PAS was possible, because active air sampling conducted at the same time allowed for the derivation of that fraction. Atmospheric concentrations measured by PUF-PAS have also been combined with grab water samples to estimate air–water fugacity ratios of several OCPs in the lower Ganga River.359
G.8.2 PASs in studies of air–soil exchange.
It is quite common to analyze soil samples taken at or in the vicinity of passive air sampling sites,301,353,364,377,459,460,476,551 for example to compare the relative composition of PCB mixtures in different media.387 In particular, spatial trends of concentrations in air and soil along elevation gradients have been used to study the occurrence of mountain cold trapping.390,391,452,471
Measured air–soil concentration ratios can be compared with those that might be expected if air and soil were in equilibrium.280 It is also possible to calculate fugacity gradients between soil and air and infer the likely flux direction from that gradient. Daly et al. were the first to do this with air concentrations derived from PASs and also explored the considerable uncertainty inherent in this approach.390 Not only is the estimation of a fugacity from a soil concentration dependent on numerous assumptions and possibly quite uncertain partition coefficients, the uncertainty of the SR of a PAS directly propagates to the uncertainty of the air fugacity value. Therefore, only calculated fugacity gradients that deviate strongly from equilibrium should be interpreted as indicating the potential for volatilization or deposition.390 This approach has since been adopted repeatedly,340,341,344,346,347,356,363,375,376,394,543 not always with full awareness of the significant uncertainties it entails. It is also important to stress that only the gas-phase concentration contributes to a chemical's fugacity in air. Since there is now considerable evidence that the PUF-PAS samples particle-bound substances (Section F.6), an air concentration derived from a PUF-PAS should only be used to calculate a fugacity if the chemical is entirely in the gas-phase.
Sometimes, generic mass transfer coefficients are applied to the fugacity gradient to estimate fluxes across the air–soil interface.346,394 This is quite questionable, as (1) air concentration averaged over extended periods of time may not be appropriate for flux estimation, considering that the fugacity gradient will undergo significant changes as air concentration and temperature are variable at a time scale shorter than the PAS deployment period,464 (2) the mass transfer coefficients are highly site-specific, and (3) the uncertainty of the fugacity ratio is directly propagated to the flux. Such flux values should therefore not be interpreted as anything but rough order of magnitude estimates.
Another approach to measuring soil–air exchange fluxes is not based on the analysis of soil samples, but on recording a gradient of air concentrations above the soil. These studies were reviewed in Section G.3.1.
H. Recommendations and outlook regarding PASs for SVOCs
H.1 Comprehensive and quantitative evaluation of PASs for SVOCs
It is now more than 25 years since the first PAS for SVOCs has been introduced34 and since then well in excess of 300 studies have used PASs for SVOCs in a wide range of applications (see Section G). Some of these applications include governmental monitoring programs, such as the programs for Global Atmospheric Passive Sampling (GAPS)208 and Joint Oilsands Monitoring (JOSM),292 as well as large epidemiological investigations such as the Airborne Exposure to Semi-volatile Organic Pollutants (AESOP) study.492 One would think that this represents a mature field, where the community of PAS developers and users has a solid understanding of the strengths, limitations and uncertainties of this sampling technique. However, it is only as a result of a number of comprehensive evaluation studies conducted during the past few years254,262,514,552 that increased awareness and a better quantitative understanding of these limitations and uncertainties have emerged.
Holt et al. used the long time series of concurrent air samples taken in Košetice in the Czech Republic to assess the agreement between a PUF-PAS deployed for one month and four one-day HiVol samples taken during the same time.514 For most PCBs, OCPs and PAHs, concentrations obtained from the two sampling techniques were statistically significantly different from each other, irrespective of whether the effective air volume Vair was derived using site-specific or generic SRs and what assumptions were made concerning the relative SR of particle-bound substances. Correlations between concentrations obtained from passive and active sampling were often poor and linear regressions hardly ever showed a slope close to 1. While the average of 12 monthly PUF-PASs and the average of the 48 active air samples taken during the same year were generally within the same order of magnitude, for less volatile PAHs discrepancies larger than an order of magnitude were common. Some of these discrepancies may be attributed to uncertainties introduced during analytical quantification and the different temporal coverage of the two sampling approaches (14% overlap), but they are too large to be fully explained by these sources of uncertainty.514
When trying to calibrate the PUF-PAS for a number of perfluorinated substances, Karásková et al. often failed to observe an increase in the sequestered amount with increasing deployment time indoors.552 Also, the uptake curves obtained for outdoor deployments showed poor replicate precision and a very large range in SRe (0.7 to 30 m3 per day). Because many of these perfluorinated compounds are charged and have extremely limited volatility, these results hint at the large variability in the uptake of non-gaseous substances in PAS, especially indoors, where air turbulence is very limited and/or highly spatially variable. Melymuk et al. found much lower levels of several PAHs and BFRs in PUF-PASs deployed for one month than were taken up cumulatively in four PUF-PASs deployed sequentially for one week each at the same time and place.262 Together the studies by Holt et al.,514 Melymuk et al.262 and Karásková et al.552 are a sobering illustration of the large uncertainties one might expect even from one of the most thoroughly characterised PAS when deployed and analyzed by a highly experienced group.
Melymuk et al. summarised many of the challenges besetting the passive air sampling for SVOCs and highlighted the appreciable uncertainties and biases to which they give rise.284 It is important that the community of PAS researchers and users is responding appropriately to the repercussions of the findings of these evaluation studies. For the community of PAS researchers, it means that future effort should be dedicated to quantifying and reducing the uncertainties of the passive air sampling of SVOCs based on a comprehensive understanding of how it works. New sampler designs and modifications of existing designs should be targeted to address the identified shortcomings of the existing designs. This is further discussed in Section H.2. For the community of PAS users, it implies the need to become aware of, and knowledgeable about, the limitations of the tools they apply and consequently to become more transparent and explicit in reporting the uncertainties of the results they report. Section H.3 expands on this further.
H.2 Recommendations and outlook regarding the design of PASs for SVOCs
This review revealed a surprisingly large number of designs for PASs for SVOCs. Tables 1, 2, 4 and 5 list almost 40 different designs that have been proposed. Many of these designs are only the subject of one or two publications, i.e. these designs remain for the most part poorly characterised and find only very limited, if any, applications. On the other hand, there are a number of PAS designs for which a large body of work exists, that created valuable information on SRs and their variability, e.g. with respect to the influence of wind or to the differences between different types of SVOCs (see Section F), as well empirical quantitative data and estimation techniques on the uptake capacity of their sorbent materials (see Section E). Some of these have also been rigorously evaluated (Section H.1). As a result, it is possible to apply these PASs with a reasonably clear understanding of their limitations and the expected uncertainty of the data they yield.
This is not to say that these PAS designs could not be improved upon. On the contrary, some of the most frequently used PAS designs are quite compromised. The first PAS for SVOCs, the SPMD, has turned out to have too complex and too variable an uptake kinetic and to pose too many analytical challenges that it has ceased to be a competitive choice for most applications, despite a fairly substantive body of work characterising it. Even the PUF-PAS, which has become the default choice of many PAS studies, leans heavily on the side of high SRs in the balancing of the conflicting objectives of high vs. tightly controlled SRs and therefore these SRs are variable and highly uncertain (see Section C.1). While PUF is a popular choice as a sorbent because of its ease of handling and low cost, its low uptake capacity necessitates complex SR estimations for more volatile SVOCs that introduce considerable uncertainty (see Section F.3).
Introducing a new sampler design only makes sense, if two conditions are fulfilled. There has to be a:
(1) Clear motivation for proposing a new design, i.e. it has to fundamentally improve on the existing, well-characterised PAS designs for at least one type of application. Sadly, the evidence suggests that some of the researchers proposing new designs are not even always aware of the full slate of PAS designs that have been described in the literature and therefore may be ill-equipped to make a genuinely useful contribution.
(2) Commitment to undertake the experiments that are required for comprehensive characterisation of a new design. At a minimum that needs to include (i) a calibration study with replicated samplers being retrieved after various lengths of time and with simultaneous measurement of air concentration with active air sampler, as this is what is necessary to obtain information on tlinear and tequilibrium for SVOCs of different volatility and at different temperatures, (ii) an exploration of the variability in SRs that can be expected in different types of deployments.
There is certainly a need for a PAS for SVOCs that can generate air concentrations with a much smaller uncertainty than the current slate of sampler designs. Such a PAS would be useful for applications where small concentration differences need to be discerned (e.g. when measuring vertical gradients, see Section G.3) or when highly accurate numbers are required (e.g. when the potential for diffusive air–surface exchange of SVOCs is to be deduced, see Section G.8). Such a sampler would almost certainly need to apply effective wind sheltering and a diffusive barrier in order to achieve the tightly controlled SRs necessary for high precision (see Section B.4). Because that would inevitably lead to small SRs, the analysis of the amount of SVOC on the sorbent of such a sampler will need to achieve very low LODs. There are now several studies that have shown that an analytical approach that thermally desorbs the sequestered chemical from a sorbent onto a GC-MS is capable of delivering such low LODs. This is most easily achieved by a sorbent that can be placed in the tubes used by commercial thermal desorption instruments. Sorbents that have been thermally desorbed include silicone coated stir bars,52,55,108–110 Tenax TA-coated GFFs,199,200 and commercially available Tenax TA filled cartridges.201
Often and maybe surprisingly, there has been no attempt to control the diffusion distance to these sorbents. In studies where this was attempted, e.g. by using different types of PE-based diffusive barriers,55 this has not been successful, as evidenced by high and unexplained SR variability. Interestingly, some of the thermally stable sorbents that have been proposed, such as the commercially available Tenax TA-filled cartridges and silicone rods, would be compatible with the commercial Radiello diffusive barriers, which for example finds use for passively sampling VOCs.273 Yet, there appears to have been no study that explored the feasibility of a PAS for SVOCs that combines a thermally stable sorbent in rod-shaped form with a Radiello diffusive barrier. It is possible, and maybe even likely, that the sorptive capacity of the PE of the Radiello is itself too large and would interfere with the diffusion of the SVOC to the sorbent. However, it may be feasible to construct a radial diffusive barrier from material that has less capacity to sorb SVOCs than PE.
Apart from their potential analysis by thermal desorption, PDMS-based PASs show considerable promise for indoor applications, because of the ease of handling and because fast diffusion within the polymer should assure that diffusion across the air boundary layer is rate-limiting uptake. A hurdle to their credibility currently is a general disregard of the need to constrain the thickness of that air boundary layer to a small range, which even in indoor settings would be required to increase precision, and the failure to observe the theoretically expected quantitative relationship between surface area and uptake rate, at least when the results of various studies are compared (see Table 3). If that issue could be resolved, it should be quite easily possible to modify the surface area to volume ratio of the PDMS sorbent in order to tailor a PAS for a particular set of deployment conditions (i.e. a specific set of SVOCs sampled for a particular length of time, given a particular LOD of the detection technique).
H.3 Recommendations and outlook regarding applications of PASs for SVOCs
As Section G illustrates, PASs for SVOCs now find use for a wide variety of applications. A perusal of those studies reveals that the considerable uncertainty of results obtained with most currently used PASs is often profoundly underappreciated. Partly, this may be attributable to an overestimation of the performance capability of a PAS. More typically, it is a result of the reluctance of many PAS users to quantify or even consider that uncertainty. Going forward, there should be an expectation that certain elements are present in a PAS-based study, if it is to be published in the peer-reviewed literature or if the generated data serve regulatory purposes.
A good PAS study will replicate at least some of the sampler deployments and have a large number of field blanks. When targeting SVOCs that are known to be susceptible to blank contamination (e.g. cVMS) and/or when sampling very low concentrations (see e.g. Section G.6), it may be advisable to have a field blank for every deployed sampler.
Importantly, the uncertainty of the reported results needs to be explicitly stated. If results are reported in sequestered amounts (normalised to time), it may be sufficient to estimate that uncertainty from the standard deviation of replicated sampler deployments. If volumetric air concentrations are reported, the uncertainty of the applied SRs or effective sampling volumes needs to be considered in addition to the sampling and analytical uncertainty. As Section F.3 illustrates the uncertainty of SRs is often very large. If an effective sampling volume is estimated for a PAS operating in the curvi-linear uptake regime, the uncertainty of the variables going into that estimation need to be propagated to the final reported air concentration. These variables include the KSG value, which is often estimated from a highly uncertain empirical regression equation and then adjusted to the temperature of deployment using an equally uncertain energy of sorption,263 and it includes the intrinsic SR determined from the loss of depuration compounds. The study by Liu et al. illustrates how this can be done.86
An honest and comprehensive appraisal of uncertainty will most likely reveal that in many instances a PAS-derived air concentration for SVOCs may essentially be an order of magnitude estimate. This is especially true for particle-bound substances. It is important to stress that this does not invalidate the usefulness of PASs as in many applications even highly uncertain data are perfectly sufficient to meet the project objectives. As was discussed in Sections G.5.1 and G.7, amounts sequestered in a PAS have much less uncertainty than air concentrations and are often appropriate to answer the questions related to spatial variability and temporal trends that PASs are frequently called upon to address. However, honest uncertainty estimates will also reveal that some of the interpretations of PAS-based studies will have to be questioned. Small differences in levels measured at forested and open sites may be caused by SRs that depend on wind and not necessarily be an indication of the forest filter effect.65,468,478 The same applies to small differences in paired indoor/outdoor PAS deployments. Even air–soil or air–water fugacity fractions of 0.1 or 0.9 may not be confidently distinguished from equilibrium, if the air concentration is derived from a PAS. If efforts to develop a high precision PAS for SVOCs, as advocated in Section H.2, should succeed it will be time to revisit those PAS applications that rely on measured air concentrations with small uncertainty.
I. Acronyms, abbreviations, variables
I.1 Variables
A
| Geometric surface area of the sorbent exposed to the gas-phase |
A
S
| Specific surface area of an adsorbent |
C
G
| Concentration of chemical in the gas-phase |
C
S
| Concentration of chemical in the sorbent of a passive air sampler |
D
| Molecular diffusivity in the gas-phase |
ΔHSG | Enthalpy of transfer from the sorbed phase to the gas-phase |
k
l
| Rate constant describing chemical loss from a passive air sampler |
k
u
| Rate constant describing chemical uptake in a passive air sampler |
K
SG
| Equilibrium sorption coefficient between a sorbent and the gas-phase |
m
S
| Amount of chemical taken up in the sorbent of a passive air sampler |
R
| Ideal gas constant |
SR | Inherent sampling rate of a passive air sampler |
SRe | Empirical sampling rate of a passive air sampler |
Δt | Deployment time of a passive air sampler |
t
linear
| Length of the linear uptake period of a passive air sampler |
t
equilibrium
| Time needed to approach equilibrium between sorbent and gas-phase |
T
| Temperature |
ΔUSG | Internal energy of transfer from the sorbed phase to the gas-phase |
V
air
| Effective sampling volume of a passive air sampler |
V
S
| Geometric bulk volume of a passive sampling sorbent |
Δz | Thickness of the stagnant air boundary layer surrounding a sorbent |
I.2 Abbreviations for different samplers and sorbent materials
ACF | Activated carbon felt |
AL | Artificial leaf |
EVA | Ethyl vinyl acetate |
FTS | Flow through sampler |
GFF | Glass fibre filter |
HiVol | High volume sampler |
LoVol | Low volume sampler |
MECOP | Membrane-enclosed copolymer |
PAS | Passive air sampler |
PAS-DD | Passive dry deposition sampler |
PDMS | Polydimethylsiloxane |
PE, LDPE | Polyethylene, low-density polyethylene |
PES | Passive emission sampler |
POG | Polymer-coated glass |
PUF | Polyurethane foam |
SBSE | Stir bar sorptive extraction |
SIP | Sorbent impregnated polyurethane |
SPMD | Semi-permeable membrane device |
SPME | Solid phase micro extraction |
VERAM | Versatile, easy and rapid atmospheric monitor |
XAD | Styrene–divinylbenzene co-polymer |
I.3 Abbreviations for compounds and compound groups
SVOCs | Semi-volatile organic chemicals |
VOCs | Volatile organic chemicals |
BFRs | Brominated flame retardants |
CUPs | Current use pesticides |
HFR | Halogenated flame retardants |
MCCPs | Medium chain chlorinated paraffins |
OCPs | Organochlorine pesticides |
OPE | Organophosphate esters |
OPFRs | Organophosphate flame retardants |
PAHs | Polycyclic aromatic hydrocarbons |
PBDEs | Polybrominated diphenyl ethers |
PCBs | Polychlorinated biphenyls |
PCDD/Fs | Polychlorinated dibenzo-p-dioxins and dibenzofurans |
PCNs | Polychlorinated naphthalenes |
PFAS | Perfluorinated and polyfluorinated alkyl substances |
SCCPs | Short-chain chlorinated paraffins |
VMS | Volatile methyl siloxanes |
ADBI | Celestolide |
AHTN | Tonalide |
ATE | 2,4,6-Tribromophenylallyl ether |
ATII | Traseolide |
BATE | 2-Bromoallyl-2,4,6-tribromo-phenyl ether |
BTBPE | 1,2-Bis(2,4,6-tribromo-phenoxy)ethane |
D3 | Hexamethylcyclotrisiloxane |
D4 | Octamethylcyclotetrasiloxane |
D5 | Decamethylcyclopentasiloxane |
DBDPE | Decabromodiphenylethane |
DDT | Dichlorodiphenyltrichloroethane |
DDE | Dichlorodiphenyldichloroethylene |
DPTE | 2,3-Dibromopropyl-2,4,6-tribromophenyl ether |
EHTBB | 2-Ethylhexyl-2,3,4,5-tetrabromobenzoate |
EtFOSA |
N-Ethyl perfluorooctane sulfonamide |
EtFOSE |
N-Ethyl perfluorooctane sulfonamidoethanol |
8:2 FTOH | Perfluorooctyl ethanol |
10:2 FTOH | Perfluorodecyl ethanol |
GEM | Gaseous elemental mercury |
HBB | Hexabromobenzene |
HBCD | Hexabromocyclododecane |
HCBD | Hexchlorobutadiene |
HCB | Hexachlorobenzene |
HCH | Hexachlorocyclohexane |
HHCB | Galaxolide |
MCPA | 2-Methyl-4-chlorophenoxyacetic acid |
MDM | Octamethyltrisiloxane |
MD2M | Decamethyltetrasiloxane |
MD3M | Dodecamethylpentasiloxane |
MeFOSA |
N-Methyl perfluorooctane sulfonamide |
MeFOSEA |
N-Methylperfluorooctane sulfonamidethylacry-late |
MeFOSE |
N-Methyl perfluorooctane sulfonamidoethanol |
PBEB | Pentabromoethylbenzene |
PBT | 2,3,4,5,6-Pentabromotoluene |
PCA | Pentachloroanisole |
PeCBz | Pentachlorobenzene |
TBBP-A | Tetrabromobisphenol-A |
TBE | 1,2-Bis(2,4,6-tribromophenoxy)ethane |
TBECH | Tetrabromoethyl-cyclohexane |
TBCO | 1,2,5,6-Tetrabromocyclooctane |
p-TBX | 2,3,5,6-Tetrabromo-p-xylene |
TBPH | Bis-(2-ethylhexyl)tetrabromophthalate |
Conflicts of interest
There are no conflicts of interest to declare.
Acknowledgements
We are grateful to the Natural Sciences and Engineering Research Council of Canada for funding most of our work on passive air sampling of SVOCs over the past 20 years.
References
- R. M. Cox, The use of passive sampling to monitor forest exposure to O3, NO2 and SO2: a review and some case studies, Environ. Pollut., 2003, 126, 301–311 CrossRef CAS PubMed.
- G. R. Carmichael, M. Ferm, N. Thongboonchoo, J.-H. Woo, L. Y. Chan, K. Murano, P. H. Viet, C. Mossberg, R. Bala, J. Boonjawat, P. Upatum, M. Mohan, S. P. Adhikary, A. B. Shrestha, J. J. Pienaar, E. B. Brunke, T. Chen, T. Jie, D. Guoan, L. C. Peng, S. Dhiharto, H. Harjanto, A. M. Jose, W. Kimani, A. Kirouane, J.-P. Lacaux, S. Richard, O. Barturen, J. C. Cerda, A. Athayde, T. Tavares, J. S. Cotrina and E. Bilici, Measurements of sulfur dioxide, ozone and ammonia concentrations in Asia, Africa, and South America using passive samplers, Atmos. Environ., 2003, 37, 1293–1308 CrossRef CAS.
- S. Mukerjee, L. A. Smith, G. A. Norris, M. T. Morandi, M. Gonzales, C. A. Noble, L. M. Neas and A. H. Özkaynak, Field method comparison between passive air samplers and continuous monitors for VOCs and NO2 in El Paso, Texas, J. Air Waste Manage. Assoc., 2004, 54, 307–319 CrossRef CAS PubMed.
- M. A. Puchalski, M. E. Sather, J. T. Walker, C. M. B. Lehmann, D. A. Gay, J. Mathew and W. P. Robarge, Passive ammonia monitoring in the United States: Comparing three different sampling devices, J. Environ. Monit., 2011, 13, 3156–3167 RSC.
- D. S. McLagan, M. E. E. Mazur, C. P. J. Mitchell and F. Wania, Passive air sampling of gaseous elemental mercury: A critical review, Atmos. Chem. Phys., 2016, 16, 3061–3076 CrossRef CAS.
- G. Zhang and X. Liu, Passive atmospheric sampling of persistent organic pollutants (POPs), Prog. Chem., 2009, 21, 297–306 Search PubMed.
- L. Tuduri, M. Millet, O. Briand and M. Montury, Passive air sampling of semi-volatile organic compounds, TrAC, Trends Anal. Chem., 2012, 31, 38–49 CrossRef CAS.
- M. Partyka, B. Zabiegała, J. Namieśnik and A. Przyjazny, Application of passive samplers in monitoring of organic constituents of air, Crit. Rev. Anal. Chem., 2007, 37, 51–78 CrossRef CAS.
- A. Kot-Wasik, B. Zabiegała, M. Urbanowicz, E. Dominiak, A. Wasik and J. Namieśnik, Advances in passive sampling in environmental studies, Anal. Chim. Acta, 2007, 602, 141–163 CrossRef CAS PubMed.
- S. Seethapathy, T. Górecki and X. Li, Passive sampling in environmental analysis, J. Chromatogr. A, 2008, 1184, 234–253 CrossRef CAS PubMed.
- F. A. Esteve-Turrillas and A. Pastor, Passive air sampling, Compr. Anal. Chem., 2016, 73, 203–232 CAS.
- F. A. Esteve-Turrillas, V. Yusá, A. Pastor and M. de la Guardia, New perspectives in the use of semipermeable membrane devices as passive samplers, Talanta, 2008, 74, 443–457 CrossRef CAS PubMed.
-
C. Qu, A. L. Doherty, X. Xing, W. Sun, S. Albanese, A. Lima, S. Qi and B. De Vivo, Polyurethane foam-based passive air samplers in monitoring persistent organic pollutants: Theory and application, in Environmental Geochemistry: Site Characterization, Data Analysis and Case Histories, ed. B. De Vivo, H. Belkin and A. Lima, Elsevier, 2nd edn, 2018, ch. 20, pp. 521–542 Search PubMed.
- F. Salim and T. Górecki, Theory and modelling approaches to passive sampling, Environ. Sci.: Processes Impacts, 2019, 21, 1618–1641 RSC.
- J. R. Aboal, E. Concha-Graña, F. De Nicola, S. Muniategui-Lorenzo, P. López-Mahía, S. Giordano, F. Capozzi, A. Di Palma, R. Reski, H. Zechmeister, J. Martínez-Abaigar and J. A. Fernández, Testing a novel biotechnological passive sampler for monitoring atmospheric PAH pollution, J. Hazard. Mater., 2020, 381, 120949 CrossRef CAS PubMed.
- S. E. Gingrich, M. L. Diamond, G. A. Stern and B. E. McCarry, Atmospherically derived organic surface films along an urban-rural gradient, Environ. Sci. Technol., 2001, 35, 4031–4037 CrossRef CAS PubMed.
- M. Unger and O. Gustafsson, PAHs in Stockholm window films: Evaluation of the utility of window film content as indicator of PAHs in urban air, Atmos. Environ., 2008, 42, 5550–5557 CrossRef CAS.
- E. Terzaghi, M. Scacchi, B. Cerabolini, K. C. Jones and A. Di Guardo, Estimation of polycyclic aromatic hydrocarbon variability in air using high volume, film, and vegetation as samplers, Environ. Sci. Technol., 2015, 49, 5520–5528 CrossRef CAS PubMed.
- C.-Y. Huo, Y. Sun, L.-Y. Liu, E. Sverko, Y.-F. Li, W.-L. Li, W.-L. Ma, Z.-F. Zhang and W.-W. Song, Assessment of human indoor exposure to PAHs during the heating and non-heating season: Role of window films as passive air samplers, Sci. Total Environ., 2019, 659, 293–301 CrossRef CAS PubMed.
- M. E. Bartkow, K. Booij, K. E. Kennedy, J. F. Müller and D. W. Hawker, Passive air sampling theory for semivolatile organic compounds, Chemosphere, 2005, 60, 170–176 CrossRef CAS PubMed.
- J. M. Armitage, S. J. Hayward and F. Wania, Modeling the uptake of neutral organic chemicals on XAD passive air samplers under variable temperatures, external wind speeds and ambient air concentrations (PAS-SIM), Environ. Sci. Technol., 2013, 47, 13546–13554 CrossRef CAS PubMed.
- D. S. McLagan, C. P. J. Mitchell, H. Huang, Y. D. Lei, A. S. Cole, A. Steffen, H. Hung and F. Wania, A high precision passive air sampler for gaseous mercury, Environ. Sci. Technol. Lett., 2016, 3, 24–29 CrossRef CAS.
-
M. A. Snow, Development, characterization and field testing of a passive air sampler for monitoring occupational exposure to mercury vapors, MSc thesis, University of Toronto, Department of Chemistry, April 2020, p. 108.
- M. Shoeib and T. Harner, Characterization and comparison of three passive air samplers for persistent organic pollutants, Environ. Sci. Technol., 2002, 36(19), 4142–4151 CrossRef CAS PubMed.
- L. Melymuk, M. Robson, P. A. Helm and M. L. Diamond, Evaluation of passive air sampler calibrations: Selection of sampling rates and implications for the measurement of persistent organic pollutants in air, Atmos. Environ., 2011, 45, 1867–1875 CrossRef CAS.
- F. Wania, L. Shen, Y. D. Lei, C. Teixeira and D. C. G. Muir, Development and calibration of a resin-based passive sampling system for persistent organic pollutants in the atmosphere, Environ. Sci. Technol., 2003, 37, 1352–1359 CrossRef CAS.
- T. Gouin, F. Wania, C. Ruepert and L. E. Castillo, Field testing passive air samplers for current use pesticides in a tropical environment, Environ. Sci. Technol., 2008, 42, 6625–6630 CrossRef CAS PubMed.
- I. S. Krogseth, X. Zhang, Y. D. Lei, F. Wania and K. Breivik, Calibration and application of a passive air sampler (XAD-PAS) for volatile methyl siloxanes, Environ. Sci. Technol., 2013, 47, 4463–4470 CrossRef CAS PubMed.
- L. Shen, F. Wania, Y. D. Lei, C. Teixeira, D. C. G. Muir and T. F. Bidleman, Atmospheric distribution and long-range transport behavior of organochlorine pesticides in North America, Environ. Sci. Technol., 2005, 39, 409–420 CrossRef CAS PubMed.
- J. E. Balmer, H. Hung, K. Vorkamp, R. J. Letcher and D. C. G. Muir, Hexachlorobutadiene (HCBD) contamination in the Arctic environment: A review, Emerging Contam., 2019, 5, 116–122 CrossRef.
- C. Rauert, T. Harner, J. K. Schuster, A. Eng, G. Fillmann, L. E. Castillo, O. Fentanes, M. Villa Ibarra, K. S. B. Miglioranza, I. Moreno Rivadeneira, K. Pozo and B. H. Aristizábal Zuluaga, Atmospheric concentrations of new persistent organic pollutants and emerging chemicals of concern in the Group of Latin America and Caribbean (GRULAC) region, Environ. Sci. Technol., 2018, 52, 7240–7249 CrossRef CAS PubMed.
- Y.-G. Ma, Y. D. Lei, H. Xiao, F. Wania and W.-H. Wang, Critical review and recommended values for the physical-chemical property data of 15 polycyclic aromatic hydrocarbons at 25 °C, J. Chem. Eng. Data, 2010, 55, 819–825 CrossRef CAS.
- N. Li, F. Wania, Y. D. Lei and G. L. Daly, A comprehensive and critical compilation, evaluation and selection of physical chemical property data for selected polychlorinated biphenyls, J. Phys. Chem. Ref. Data, 2003, 32, 1535–1590 CrossRef.
- J. D. Petty, J. N. Huckins and J. L. Zajicek, Application of semipermeable membrane devices (SPMDs) as passive air samplers, Chemosphere, 1993, 27, 1609–1624 CrossRef CAS.
- H. F. Prest, L. A. Jacobson and J. N. Huckins, Passive sampling of water and coastal air via semipermeable membrane devices, Chemosphere, 1995, 30, 1351–1362 CrossRef CAS.
- J. Caslavsky, Z. Zdrahal and M. Vytopilova, Application of SPMDs for PAH sampling in the DEZA chemical factory, Polycyclic Aromat. Compd., 2000, 20, 123–141 CrossRef CAS.
- K. Booij and B. L. van Drooge, Polychlorinated biphenyls and hexachlorobenzene in atmosphere, sea-surface microlayer, and water measured with semi-permeable membrane devices (SPMDs), Chemosphere, 2001, 44, 91–98 CrossRef CAS PubMed.
- W. A. Ockenden, H. F. Prest, G. O. Thomas, A. Sweetman and K. C. Jones, Passive air sampling of PCBs: Field calculation of atmospheric sampling rates by triolein-containing semipermeable membrane devices, Environ. Sci. Technol., 1998, 32, 1538–1543 CrossRef CAS.
- R. Lohmann, B. P. Corrigan, M. Howsam, K. C. Jones and W. A. Ockenden, Further developments in the use of semipermeable membrane devices (SPMDs) as passive air samplers for persistent organic pollutants: Field application in a spatial survey of PCDD/Fs and PAHs, Environ. Sci. Technol., 2001, 35, 2576–2582 CrossRef CAS PubMed.
- W. A. Ockenden, A. Sweetman, H. F. Prest, E. Steinnes and K. C. Jones, Toward an understanding of the global atmospheric distribution of persistent organic pollutants: The use of semipermeable membrane devices as time-integrated passive samplers, Environ. Sci. Technol., 1998, 32, 2795–2803 CrossRef CAS.
- W. A. Ockenden, B. P. Corrigan, M. Howsam and K. C. Jones, Further developments in the use of semipermeable membrane devices as passive air samplers: Application to PCBs, Environ. Sci. Technol., 2001, 35, 4536–4543 CrossRef CAS PubMed.
- S. N. Meijer, W. A. Ockenden, E. Steinnes, B. P. Corrigan and K. C. Jones, Spatial and temporal trends of POPs in Norwegian and UK background air: Implications for global cycling, Environ. Sci. Technol., 2003, 37, 454–461 CrossRef CAS PubMed.
- F. Jaward, S. N. Meijer, E. Steinnes, G. Thomas and K. C. Jones, Further studies on the latitudinal and temporal trends of persistent organic pollutants in Norwegian and U.K. background air, Environ. Sci. Technol., 2004, 38, 2523–2530 CrossRef CAS PubMed.
- R. Gioia, E. Steinnes, G. O. Thomas, S. N. Mejier and K. C. Jones, Persistent organic pollutants in European background air: derivation of temporal and latitudinal trends, J. Environ. Monit., 2006, 8, 700–710 RSC.
- J. K. Schuster, R. Gioia, K. Breivik, E. Steinnes, M. Scheringer and K. C. Jones, Trends in European background air reflect reductions in primary emissions of PCBs and PBDEs, Environ. Sci. Technol., 2010, 44, 6760–6766 CrossRef CAS PubMed.
- H. S. Söderström and P.-A. Bergqvist, Polycyclic aromatic hydrocarbons in a semiaquatic plant and semipermeable membrane devices exposed to air in Thailand, Environ. Sci. Technol., 2003, 37, 47–52 CrossRef PubMed.
- H. Söderström, J. Hajslova, V. Kocourek, B. Siegmund, A. Kocan, M. W. Obiedzinski, M. Tysklind and P.-A. Bergqvist, PAHs and nitrated PAHs in air of five European countries determined using SPMDs as passive samplers, Atmos. Environ., 2005, 39, 1627–1640 Search PubMed.
- H. Söderström and P.-A. Bergqvist, Passive air sampling using semipermeable membrane devices at different wind-speeds in situ calibrated by performance reference compounds, Environ. Sci. Technol., 2004, 38, 4828–4834 CrossRef PubMed.
- B. Strandberg, P. Gustafson, H. Söderström, L. Barregard, P.-A. Bergqvist and G. Sällsten, The use of semipermeable membrane devices as passive samplers to determine persistent organic compounds in indoor air, J. Environ. Monit., 2006, 8, 257–262 RSC.
- W. L. Cranor, D. Á. Álvarez, J. N. Huckins and J. D. Petty, Uptake rate constants and partition coefficients for vapor phase organic chemicals using semipermeable membrane devices (SPMDs), Atmos. Environ., 2009, 43, 3211–3219 CrossRef CAS.
- A. Cicenaite, J. N. Huckins, D. Á. Álvarez, W. L. Cranor, R. W. Gale, V. Kauneliene and P.-A. Bergqvist, Feasibility of a simple laboratory approach for determining temperature influence on SPMD-air partition coefficients of selected compounds, Atmos. Environ., 2007, 41, 2844–2850 CrossRef CAS.
- L. Wennrich, P. Popp and C. Hafner, Novel integrative passive samplers for the long-term monitoring of semivolatile organic air pollutants, J. Environ. Monit., 2002, 4, 371–376 RSC.
- S.-D. Choi, S.-Y. Baek and Y.-S. Chang, Influence of a large steel complex on the spatial distribution of volatile polycyclic aromatic hydrocarbons (PAHs) determined by passive air sampling using membrane-enclosed coplolmer (MECOP), Atmos. Environ., 2007, 41, 6255–6264 CrossRef CAS.
- F. A. Esteve-Turrillas, S. Ly-Verdú, A. Pastor and M. de la Guardia, Development of a versatile, easy and rapid atmospheric monitor for benzene, toluene, ethylbenzene and xylenes determination in air, J. Chromatogr. A, 2009, 1216, 8549–8556 CrossRef CAS PubMed.
- H. Paschke and P. Popp, New passive samplers for chlorinated semivolatile organic pollutants in ambient air, Chemosphere, 2005, 58, 855–863 CrossRef CAS PubMed.
- Y. Wang, A. J. Easteal and X. D. Chen, Ethylene and oxygen permeability through polyethylene packaging films, Packag. Technol. Sci., 1998, 11, 169–178 CrossRef CAS.
- K. Booij, H. E. Hofmans, C. V. Fischer and E. M. Van Weerlee, Temperature-dependent uptake rates of nonpolar organic compounds by semipermeable membrane devices and low-density polyethylene membranes, Environ. Sci. Technol., 2003, 37(2), 361–366 CrossRef CAS PubMed.
- W. Levy, B. Henkelmann, G. Pfister, M. Kirchner, G. Jakobi, A. Niklaus, J. Kotalik, S. Bernhöft, N. Fischer and K.-W. Schramm, Monitoring of PCDD/Fs in a mountain forest by means of active and passive sampling, Environ. Res., 2007, 105, 300–306 CrossRef CAS PubMed.
- M. T. Piccardo, A. Stella, M. Pala, D. Balducci and F. Valerio, Field use of semipermeable membrane devices (SPMDs) for passive air sampling of polycyclic aromatic hydrocarbons: Opportunities and limitations, Atmos. Environ., 2010, 44, 1947–1951 CrossRef CAS.
- W. Levy, B. Henkelmann, G. Pfister, S. Bernhoft, M. Kirchner, G. Jakobi, R. Bassan, N. Kräuchi and K.-W. Schramm, Long-term air monitoring of organochlorine pesticides using Semi Permeable Membrane Devices (SPMDs) in the Alps, Environ. Pollut., 2009, 157, 3272–3279 CrossRef CAS PubMed.
- S. Fiedler, G. Pfister and K.-W. Schramm, Partitioning of fluorotelomer alcohols (FTOH) to semipermeable membrane devices (SPMD), Environ. Sci. Pollut. Res., 2010, 17, 420–428 CrossRef CAS PubMed.
- X. Zhu, C. Zhou, B. Henkelmann, Z. Wang, X. Ma, G. Pfister, K.-W. Schramm, J. Chen, Y. Ni, W. Wang and J. Mu, Monitoring of PAHs profiles in the urban air of Dalian, China with
active high-volume sampler and semipermeable membrane devices, Polycyclic Aromat. Compd., 2013, 33, 265–288 CrossRef CAS.
- Q. Xu, X. Zhu, B. Henkelmann, K.-W. Schramm, J. Chen, Y. Ni, W. Wang, G. Pfister, J. Mu, S. Qin and Y. Li, Simultaneous monitoring of PCB profiles in the urban air of Dalian, China with active and passive samplings, J. Environ. Sci., 2013, 25, 133–143 CrossRef CAS.
- X. Zhu, T. Shi, B. Henkelmann, G. Pfister, W. Wang, J. Mu, X. Li, L. Han and K.-W. Schramm, Monitoring of organochlorine pesticides in the urban air of Dalian, China with semipermeable membrane devices, Chin. Sci. Bull., 2014, 59, 2957–2965 CrossRef CAS.
- N. Zhu, K.-W. Schramm, T. Wang, B. Henkelmann, X. Zheng, J. Fu, Y. Gao, Y. Wang and G. Jiang, Environmental fate and behavior of persistent organic pollutants in Shergyla Mountain, southeast of the Tibetan Plateau of China, Environ. Pollut., 2014, 191, 166–174 CrossRef CAS PubMed.
- J. F. Müller, K. Manomanii, M. R. Mortimer and M. S. McLachlan, Partitioning of polycyclic aromatic hydrocarbons in the polyethylene/water system, Fresenius. J. Anal. Chem., 2001, 371, 816–822 CrossRef PubMed.
- M. E. Bartkow, D. W. Hawker, K. E. Kennedy and J. F. Müller, Characterizing uptake kinetics of PAHs from the air using polyethylene-based passive air samplers of multiple surface area-to volume ratios, Environ. Sci. Technol., 2004, 38, 2701–2706 CrossRef CAS PubMed.
- M. E. Bartkow, K. C. Jones, K. E. Kennedy, N. Holling, D. W. Hawker and J. F. Müller, Evaluation of performance reference compounds in polyethylene-based passive air samplers, Environ. Pollut., 2006, 144, 365–370 CrossRef CAS PubMed.
- L. B. Paulik, C. E. Donald, B. W. Smith, L. G. Tidwell, K. A. Hobbie, L. Kincl, E. N. Haynes and K. A. Anderson, Emissions of polycyclic aromatic hydrocarbons from natural gas extraction into air, Environ. Sci. Technol., 2016, 50, 7921–7929 CrossRef CAS PubMed.
- C. E. Donald and K. A. Anderson, Assessing soil-air partitioning of PAHs and PCBs with a new fugacity passive sampler, Sci. Total Environ., 2017, 596–597, 293–302 CrossRef CAS PubMed.
- C. E. Donald, R. P. Scott, G. Wilson, P. D. Hoffman and K. A. Anderson, Artificial turf: chemical flux and development of silicone wristband partitioning coefficients, Air Qual. Atmos. Hlth., 2019, 12, 597–611 CrossRef CAS PubMed.
- E. J. Morgan and R. Lohmann, Detecting air–water and surface-deep water gradients of PCBs using polyethylene passive samplers, Environ. Sci. Technol., 2008, 42, 7248–7253 CrossRef CAS PubMed.
- M. A. Khairy and R. Lohmann, Field validation of polyethylene passive air samplers for parent and alkylated PAHs in Alexandria, Egypt, Environ. Sci. Technol., 2012, 46, 3990–3998 CrossRef CAS PubMed.
- M. A. Khairy and R. Lohmann, Feasibility of using low density polyethylene sheets to detect atmospheric organochlorine pesticides in Alexandria, Egypt, Environ. Pollut., 2013, 181, 151–158 CrossRef CAS PubMed.
- M. A. Khairy and R. Lohmann, Field calibration of low-density polyethylene passive samplers for gaseous POPs, Environ. Sci.: Processes Impacts, 2014, 16, 414–421 RSC.
- Y. Liu, S. Xie, L. Zheng, T. Li, Y. Sun, L. Ma, Z. Lin, P. Grathwohl and R. Lohmann, Air–soil diffusive exchange of PAHs in an urban park of Shanghai based on polyethylene passive sampling: Vertical distribution, vegetation influence and diffusive flux, Sci. Total Environ., 2019, 689, 734–742 CrossRef CAS PubMed.
- R. Lohmann, M. Dapsis, E. J. Morgan, V. Dekany and P. J. Luey, Determining air–water exchange, spatial and temporal trends of freely dissolved PAHs in an urban estuary using passive polyethylene samplers, Environ. Sci. Technol., 2011, 45, 2655–2662 CrossRef CAS PubMed.
- C. L. Friedman, M. G. Cantwell and R. Lohmann, Passive sampling provides evidence for Newark Bay as a source of polychlorinated dibenzo-p-dioxins and furans to the New York/New Jersey, USA, atmosphere, Environ. Toxicol. Chem., 2012, 31, 253–261 CrossRef CAS PubMed.
- R. Lohmann, J. Klánová, P. Kukučka, S. Yonis and K. Bollinger, Concentrations, fluxes, and residence time of PBDEs across the tropical Atlantic Ocean, Environ. Sci. Technol., 2013, 47, 13967–13975 CrossRef CAS PubMed.
- M. Khairy, D. Muir, C. Teixeira and R. Lohmann, Spatial trends, sources, and air–water exchange of organochlorine pesticides in the Great Lakes basin using low density polyethylene passive samplers, Environ. Sci. Technol., 2014, 48, 9315–9324 CrossRef CAS PubMed.
- M. Khairy, D. Muir, C. Teixeira and R. Lohmann, Spatial distribution, air-water fugacity ratios and source apportionment of polychlorinated biphenyls in the lower Great Lakes basin, Environ. Sci. Technol., 2015, 49, 13787–13797 CrossRef CAS PubMed.
- C. A. McDonough, M. A. Khairy, D. C. G. Muir and R. Lohmann, Significance of population centers as sources of gaseous and dissolved PAHs in the lower Great Lakes, Environ. Sci. Technol., 2014, 48, 7789–7797 CrossRef CAS PubMed.
- C. A. McDonough, G. Puggioni, P. A. Helm, D. Muir and R. Lohmann, Spatial distribution and air–water exchange of organic flame retardants in the lower Great Lakes, Environ. Sci. Technol., 2016, 50, 9133–9141 CrossRef CAS PubMed.
- C. A. McDonough, P. A. Helm, D. Muir, G. Puggioni and R. Lohmann, Polycyclic musks in the air and water of the lower Great Lakes: Spatial distribution and volatilization from surface waters, Environ. Sci. Technol., 2016, 50, 11575–11583 CrossRef CAS PubMed.
- Y. Liu, S. Wang, C. A. McDonough, M. Khairy, D. Muir, P. A. Helm and R. Lohmann, Gaseous and freely-dissolved PCBs in the lower Great Lakes based on passive sampling: Spatial trends and air-water exchange, Environ. Sci. Technol., 2016, 50, 4932–4939 CrossRef CAS PubMed.
- Y. Liu, S. Wang, C. A. McDonough, M. Khairy, D. Muir and R. Lohmann, Estimation of uncertainty in air-water exchange flux and gross volatilization loss of PCBs: A case study based on passive sampling in the lower Great Lakes, Environ. Sci. Technol., 2016, 50, 10894–10902 CrossRef CAS PubMed.
- Z. Ruge, D. Muir, P. Helm and R. Lohmann, Concentrations, trends, and air-water exchange of PAHs and PBDEs derived from passive samplers in Lake Superior in 2011, Environ. Sci. Technol., 2015, 49, 13777–13786 CrossRef CAS PubMed.
- Z. Ruge, D. Muir, P. Helm and R. Lohmann, Concentrations, trends, and air-water exchange of PCBs and organochlorine pesticides derived from passive samplers in Lake Superior in 2011, Environ. Sci. Technol., 2018, 52, 14061–14069 CrossRef PubMed.
- R. O. Meire, M. Khairy, A. C. Targino, P. M. A. Galvão, J. P. M. Torres, O. Malm and R. Lohmann, Use of passive samplers to detect organochlorine pesticides in air and water at wetland mountain region sites (S-SE Brazil), Chemosphere, 2016, 144, 2175–2182 CrossRef CAS PubMed.
- Y. Ma, D. A. Adelman, E. Bauerfeind, A. Cabrerizo, C. A. McDonough, D. Muir, T. Soltwedel, C. Sun, C. C. Wagner, E. M. Sunderland and R. Lohmann, Concentrations and water mass transport of legacy POPs in the Arctic Ocean, Geophys. Res. Lett., 2018, 45, 12972–12981 CAS.
- J. B. Wilcockson and F. A. P. C. Gobas, Thin-film solid-phase extraction to measure fugacities of organic chemicals with low volatility in biological samples, Environ. Sci. Technol., 2001, 35(7), 1425–1431 CrossRef CAS PubMed.
- T. Harner, N. J. Farrar, M. Shoeib, K. C. Jones and F. A. P. C. Gobas, Characterization of polymer-coated glass as a passive air sampler for persistent organic pollutants, Environ. Sci. Technol., 2003, 37, 2486–2493 CrossRef CAS PubMed.
- N. J. Farrar, T. Harner, A. J. Sweetman and K. C. Jones, Field calibration of rapidly equilibrating thin-film passive air samplers
and their potential application for low-volume air sampling studies, Environ. Sci. Technol., 2005, 39, 261–267 CrossRef CAS PubMed.
- N. J. Farrar, T. Harner, A. J. Sweetman and K. C. Jones, Field deployment of thin film passive air samplers for persistent organic pollutants: A study in the urban atmospheric boundary layer,, Environ. Sci. Technol., 2005, 39, 42–48 CrossRef CAS PubMed.
- N. J. Farrar, K. Prevedouros, T. Harner, A. J. Sweetman and K. C. Jones, Continental scale passive air sampling of persistent organic pollutants using rapidly equilibrating thin films (POGs), Environ. Pollut., 2006, 144, 423–433 CrossRef CAS PubMed.
- T. St. George, P. Vlahos, T. Harner, P. Helm and B. Wilford, A rapidly equilibrating, thin film, passive water sampler for organic contaminants; characterization and field testing, Environ. Pollut., 2011, 159, 481–486 CrossRef CAS PubMed.
- K. E. Kennedy, D. W. Hawker, J. F. Willer, M. E. Bartkow and R. W. Truss, A field comparison of ethylene vinyl acetate and low-density polyethylene thin films for equilibrium phase passive air sampling of polycyclic aromatic hydrocarbons, Atmos. Environ., 2007, 41, 5778–5787 CrossRef CAS.
- S. Genualdi and T. Harner, Rapidly equilibrating micrometer film sampler for priority pollutants in air, Environ. Sci. Technol., 2012, 17, 7661–7668 CrossRef PubMed.
- T. P. Rusina, F. Smedes, J. Klánová, K. Booij and I. Holoubek, Polymer selection for passive sampling: A comparison of critical properties, Chemosphere, 2007, 68, 1344–1351 CrossRef CAS PubMed.
- J. F. Narváez Valderrama, K. Baek, F. J. Molina and I. J. Allan, Implications of observed PBDE diffusion coefficients in low density polyethylene and silicone rubber, Environ. Sci.: Processes Impacts, 2016, 18, 87–94 RSC.
- T. P. Rusina, F. Smedes, M. Koblizkova and J. Klánová, Calibration of silicone rubber passive samplers: Experimental and modeled relations between sampling rate and compound properties, Environ. Sci. Technol., 2010, 44, 362–367 CrossRef CAS PubMed.
- M. T. O. Jonker, S. A. van der Heijden, M. Kotte and F. Smedes, Quantifying the effects of temperature and salinity on partitioning of hydrophobic organic chemicals to silicone rubber passive samplers, Environ. Sci. Technol., 2015, 49, 6791–6799 CrossRef CAS PubMed.
- G. Lammel, O. Audy, A. Besis, C. Efstathiou, K. Eleftheriadis, J. Kohoutek, P. Kukučka, M. D. Mulder, P. Přibylová, R. Prokeš, T. P. Rusina, C. Samara, A. Sofuoglu, S. C. Sofuoglu, Y. Taşdemir, V. Vassilatou, D. Voutsa and B. Vrana, Air and seawater pollution and air–sea gas exchange of persistent toxic substances in the Aegean Sea: spatial trends of PAHs, PCBs, OCPs and PBDEs, Environ. Sci. Pollut. Res., 2015, 22, 11301–11313 CrossRef CAS PubMed.
- P. A. Martos and J. Pawliszyn, Time-weighted average sampling with solid-phase micro-extraction device: implications for enhanced personal exposure monitoring to airborne pollutants, Anal. Chem., 1999, 71, 1513–1520 CrossRef CAS PubMed.
- A. Khaled and J. Pawliszyn, Time-weighted average sampling of volatile and semi-volatile airborne organic compounds by the solid-phase microextraction device, J. Chromatogr. A, 2000, 892, 455–467 CrossRef CAS PubMed.
- P. A. Martos and J. Pawliszyn, Calibration of solid phase microextraction for air analyses based on physical chemical properties of the coating, Anal. Chem., 1997, 69, 206–215 CrossRef CAS.
- J. Wang, L. Tuduri, M. Mercury, M. Millet, O. Briand and M. Montury, Sampling atmospheric pesticides with SPME: Laboratory developments and field study, Environ. Pollut., 2009, 157, 365–370 CrossRef CAS PubMed.
- K. Desmet, N. De Coensel, T. Gorecki and P. Sandra, Profiling the spatial concentration of allethrin and piperonyl butoxide using passive sorptive sampling and thermal desorption capillary GC-MS, Chemosphere, 2008, 71, 2193–2198 CrossRef CAS PubMed.
- X.-M. Li, Q.-H. Zhang, P. Wang, Y.-M. Li and G.-B. Jiang, Determination of polycyclic aromatic hydrocarbons in air by stir bar sorptive extraction-thermal desorption-gas chromatography tandem mass spectrometry, Chin. J. Anal. Chem., 2011, 39, 1641–1646 CrossRef CAS.
- J. Matsiko, H. Li, P. Wang, H. Sun, S. Zheng, D. Wang, W. Zhang, Y. Hao, Y. Li, Q. Zhang and G. Jiang, Stir bar sorptive extraction and thermal desorption – gas chromatography/mass spectrometry for determining phosphorus flame retardants in air samples, Anal. Methods, 2018, 10, 1918–1927 RSC.
- J. O. Okeme, A. Saini, C. Yang, J. Zhu, F. Smedes, J. Klánová and M. L. Diamond, Calibration of polydimethylsiloxane and XAD-Pocket passive air samplers (PAS) for measuring gas- and particle-phase SVOCs, Atmos. Environ., 2016, 143, 202–208 CrossRef CAS.
- J. O. Okeme, C. Yang, A. Abdollahi, S. Dhal, S. A. Harris, L. M. Jantunen, D. Tsirlin and M. L. Diamond, Passive air sampling of flame retardants and plasticizers in Canadian homes using PDMS, XAD-coated PDMS and PUF samplers, Environ. Pollut., 2018, 239, 109–117 CrossRef CAS PubMed.
- J. O. Okeme, L. V. Nguyen, M. Lorenzo, S. Dhal, Y. Pico, V. H. Arrandale and M. L. Diamond, Polydimethylsiloxane (silicone rubber) brooch as a personal passive air sampler for semi-volatile organic compounds, Chemosphere, 2018, 208, 1002–1007 CrossRef CAS PubMed.
- K. Vorkamp, L. Odsbjerg, M. Langeland and P. Mayer, Utilizing the partitioning properties of silicone for the passive sampling of polychlorinated biphenyls (PCBs) in indoor air, Chemosphere, 2016, 160, 280–286 CrossRef CAS PubMed.
- J. N. Huckins, M. W. Tubergen and G. K. Manuweera, Semipermeable membrane devices containing model lipid: A new approach to monitoring the bioavailability of lipophilic contaminants and estimating their bioconcentration potential, Chemosphere, 1990, 20, 533–552 CrossRef CAS.
- G. Eriksson, S. Jensen, H. Kylin and W. Strachan, The pine needle as a monitor of atmospheric pollution, Nature, 1989, 341, 42–44 CrossRef CAS.
- P. Kömp and M. S. McLachlan, Interspecies variability of the plant/air partitioning of polychlorinated biphenyls, Environ. Sci. Technol., 1997, 31, 2944–2948 CrossRef.
- F. Böhme, K. Welsch-Pausch and M. S. McLachlan, Uptake of airborne semivolatile organic compounds in agricultural plants:
field measurements of interspecies variability, Environ. Sci. Technol., 1999, 33, 1805–1813 CrossRef.
- H. Kylin, K. Söderkvist, A. Undeman and R. Franich, Seasonal variation of the terpene content, an overlooked factor in the determination of environmental pollutants in pine needles, Bull. Environ. Contam. Toxicol., 2002, 68, 155–160 CrossRef CAS PubMed.
- J. F. Müller, D. W. Hawker, D. W. Connell, P. Kömp and M. S. McLachlan, Passive sampling of atmospheric SOCs using tristearin-coated fibreglass sheets, Atmos. Environ., 2000, 34, 3525–3534 CrossRef.
- M. Stracquadanio, D. Bergamini, E. Massaroki and C. Trombini, Field evaluation of a passive sampler of polycyclic aromatic hydrocarbons (PAHs) in an urban atmosphere (Bologna, Italy), J. Environ. Monit., 2005, 7, 910–915 RSC.
- D. Gilbert, G. Witt, F. Smedes and P. Mayer, Polymers as reference partitioning phase: polymer calibration for an analytically operational approach to quantify multimedia phase partitioning, Anal. Chem., 2016, 88, 5818–5826 CrossRef CAS PubMed.
- T. P. Rusina, F. Smedes and J. Klánová, Diffusion coefficients of polychlorinated biphenyls and polycyclic aromatic hydrocarbons in polydimethylsiloxane and low-density polyethylene polymers, J. Appl. Polym. Sci., 2010, 116, 1803–1810 CAS.
- R. Lohmann, Critical review of low-density polyethylene's partitioning and diffusion coefficients for trace organic contaminants and implications for its use as a passive sampler, Environ. Sci. Technol., 2012, 46, 606–618 CrossRef CAS PubMed.
- T. F. Bidleman and C. E. Olney, High-volume collection of atmospheric polychlorinated biphenyls, Bull. Environ. Contam. Toxicol., 1974, 11, 442–450 CrossRef CAS PubMed.
- N. J. Herkert, S. N. Spak, A. Smith, J. K. Schuster, T. Harner, A. Martinez and K. C. Hornbuckle, Calibration and evaluation of PUF-PAS sampling rates across the Global Atmospheric Passive Sampling (GAPS) network, Environ. Sci.: Processes Impacts, 2018, 20, 210–219 RSC.
- K. Pozo, T. Harner, S. C. Lee, F. Wania, D. C. G. Muir and K. C. Jones, Seasonally resolved concentrations of persistent organic pollutants in the global atmosphere from the first year of the GAPS study, Environ. Sci. Technol., 2009, 43, 796–803 CrossRef CAS PubMed.
- L. Tuduri, T. Harner and H. Hung, Polyurethane foam (PUF) disks passive air samplers: Wind effect on sampling rates, Environ. Pollut., 2006, 144, 377–383 CrossRef CAS PubMed.
- J. Klánová, P. Eupr, J. Kohoutek and T. Harner, Assessing the influence of meteorological parameters on the performance of polyurethane foam-based passive air samplers, Environ. Sci. Technol., 2008, 42, 550–555 CrossRef PubMed.
- C. Moeckel, T. Harner, L. Nizzetto, B. Strandberg, A. Lindroth and K. C. Jones, Use of depuration compounds in passive air samplers: Results from active sampling-supported field deployment, potential uses, and recommendations, Environ. Sci. Technol., 2009, 43, 3227–3232 CrossRef CAS PubMed.
- C. Chaemfa, E. Wild, B. Davison, J. L. Barber and K. C. Jones, A study of aerosol entrapment and the influence of wind speed, chamber design and foam density of polyurethane foam passive air samplers used persistent organic pollutants, J. Environ. Monit., 2009, 11, 1135–1139 RSC.
- J. Thomas, T. M. Holsen and S. Dhaniyala, Computational fluid dynamic modeling of two passive samplers, Environ. Pollut., 2006, 144, 384–392 CrossRef CAS PubMed.
- A. A. May, P. Ashman, J. Huang, S. Dhaniyala and T. M. Holsen, Evaluation of the polyurethane foam (PUF) disk passive air sampler: Computational modeling and experimental measurements, Atmos. Environ., 2011, 45, 4354–4359 CrossRef CAS.
- S. Hazrati and S. Harrad, Calibration of polyurethane foam (PUF) disk passive air samplers for quantitative measurement of polychlorinated biphenyls (PCBs) and polybrominated diphenyl ethers (PBDEs): Factors influencing sampling rates, Chemosphere, 2007, 67, 448–455 CrossRef CAS PubMed.
- C. Chaemfa, J. L. Barber, T. Gocht, T. Harner, I. Holoubek, J. Klánová and K. C. Jones, Field calibration of polyurethane foam (PUF) disk passive air samplers for PCBs and OC pesticides, Environ. Pollut., 2008, 156, 1290–1297 CrossRef CAS PubMed.
- M. Shoeib, T. Harner, S. C. Lee, D. Lane and J. Zhu, Sorbent-impregnated polyurethane foam disk for passive air sampling of volatile fluorinated chemicals, Anal. Chem., 2008, 80, 675–682 CrossRef CAS PubMed.
- L. Ahrens, T. Harner, M. Shoeib, M. Koblizkova and E. J. Reiner, Characterization of two passive air samplers for per- and polyfluroalkyl substances, Environ. Sci. Technol., 2013, 47, 14024–14033 CrossRef CAS PubMed.
- R. Liu, Y. Lin, R. Liu, F. Hu, T. Ruan and G. Jiang, Evaluation of two passive samplers for the analysis of organophosphate esters in the ambient air, Talanta, 2016, 147, 69–75 CrossRef CAS PubMed.
- S. Genualdi, S. C. Lee, M. Shoeib, A. Gawor, L. Ahrens and T. Harner, Global pilot study of legacy and emerging persistent organic pollutants using sorbent-impregnated polyurethane foam disk passive air samplers, Environ. Sci. Technol., 2010, 44, 5534–5539 CrossRef CAS PubMed.
- A. Dreyer, M. Shoeib, S. Fiedler, J. Barber, T. Harner, K.-W. Schramm, K. C. Jones and R. Ebinghaus, Field intercomparison on the determination of volatile and semivolatile polyfluorinated compounds in air, Environ. Chem., 2010, 7, 350–358 CrossRef CAS.
- V. Langer, A. Dreyer and R. Ebinghaus, Polyfluorinated compounds in residential
and non-residential indoor air, Environ. Sci. Technol., 2010, 44, 8075–8081 CrossRef CAS PubMed.
- L. Vierke, A. Lutz, M. Shoeib, E. J. Reiner, R. Guo, W.-U. Palm, R. Ebinghaus and T. Harner, Air concentrations and particle–gas partitioning of polyfluoroalkyl compounds at a wastewater treatment plant, Environ. Chem., 2011, 8, 363–371 CrossRef CAS.
- J. Li, S. Del Vento, J. Schuster, G. Zhang, P. Chakraborty, Y. Kobara and K. C. Jones, Perfluorinated compounds in the Asian atmosphere, Environ. Sci. Technol., 2011, 45, 7241–7248 CrossRef CAS PubMed.
- L. Ahrens, M. Shoeib, T. Harner, S. C. Lee, R. Guo and E. J. Reiner, Wastewater treatment plant and landfills as sources of polyfluoroalkyl compounds to the atmosphere, Environ. Sci. Technol., 2011, 45, 8098–8105 CrossRef CAS PubMed.
- M. Shoeib, T. Harner, G. M. Webster and S. C. Lee, Indoor sources of poly- and perfluorinated compounds (PFCS) in Vancouver, Canada: Implications for human exposure, Environ. Sci. Technol., 2011, 45, 7999–8005 CrossRef CAS PubMed.
- E. Goosey and S. Harrad, Perfluoroalkyl substances in UK indoor and outdoor air: Spatial and seasonal variation, and implications for human exposure, Environ. Int., 2012, 45, 86–90 CrossRef CAS PubMed.
- S. Genualdi, T. Harner, Y. Cheng, M. MacLeod, K. M. Hansen, R. van Egmond, M. Shoeib and S. C. Lee, Global distribution of linear and cyclic volatile methyl siloxanes in air, Environ. Sci. Technol., 2011, 45, 3349–3354 CrossRef CAS PubMed.
- Y. Cheng, M. Shoeib, L. Ahrens, T. Harner and J. Ma, Wastewater treatment plants and landfills emit volatile methyl siloxanes (VMSs) to the atmosphere: Investigations using a new passive air sampler, Environ. Pollut., 2011, 159, 2380–2386 CrossRef CAS PubMed.
- L. Ahrens, T. Harner and M. Shoeib, Temporal variations of cyclic and linear volatile methyl- siloxanes in the atmosphere using passive samplers and high-volume air samplers, Environ. Sci. Technol., 2014, 48, 9374–9381 CrossRef CAS PubMed.
- J. K. Schuster, R. Gioia, T. Harner, S. C. Lee, K. Breivik and K. C. Jones, Assessment of sorbent impregnated PUF disks (SIPs) for long-term sampling of legacy POPs, J. Environ. Monit., 2012, 14, 71–78 RSC.
- M. Koblizkova, S. Genualdi, S. C. Lee and T. Harner, Application of sorbent impregnated polyurethane foam (SIP) disk passive air samplers for investigating organochlorine pesticides and polybrominated diphenyl ethers at the global scale, Environ. Sci. Technol., 2012, 46, 391–396 CrossRef CAS PubMed.
- M. Koblizkova, S. C. Lee and T. Harner, Sorbent impregnated polyurethane foam disk passive air samplers for investigating current–use pesticides at the global scale, Atmos. Pollut. Res., 2012, 3, 456–462 CrossRef CAS.
- C. Rauert, M. Shoeib, J. K. Schuster, A. Eng and T. Harner, Atmospheric concentrations and trends of poly- and perfluoroalkyl substances (PFAS) and volatile methyl siloxanes (VMS) over 7 years of sampling in the Global Atmospheric Passive Sampling (GAPS) network, Environ. Pollut., 2018, 238, 94–102 CrossRef CAS PubMed.
- Y. Li, J. O. Okeme, H. Liu, M. Diamond and J. Zhu, Fixation of XAD-4 power on filter paper using methyl cellulose for the passive air sampling of semi-volatile organic compounds in indoor air, Int. J. Environ. Anal. Chem., 2016, 96, 1145–1155 CrossRef CAS.
- S. Tao, Y. Liu, W. Xu, C. Lang, S. Liu, H. Dou and W. Liu, Calibration of a passive sampler for both gaseous and particulate phase polycyclic aromatic hydrocarbons, Environ. Sci. Technol., 2007, 41, 568–573 CrossRef CAS PubMed.
- M. A.-E. Abdallah and S. Harrad, Modification and calibration of a passive air sampler for monitoring vapor and particulate phase brominated flame retardants in indoor air: Application to car interiors, Environ. Sci. Technol., 2010, 44, 3059–3065 CrossRef CAS PubMed.
- S. Tao, J. Cao, W. T. Wang, J. Y. Zhao, W. Wang, Z. H. Wang, H. Y. Cao and B. Xing, A passive sampler with improved performance for collecting gaseous and particulate phase polycyclic aromatic hydrocarbons in air, Environ. Sci. Technol., 2009, 43, 4124–4129 CrossRef CAS PubMed.
- A. Eng, T. Harner and K. Pozo, A prototype passive air sampler for measuring dry deposition of polycyclic aromatic hydrocarbons, Environ. Sci. Technol. Lett., 2014, 1, 77–81 CrossRef CAS.
- J. Dotel, P. Gong, X. Wang, B. Pokhrel, C. Wang and J. Nawab, Determination of dry deposition velocity of polycyclic aromatic hydrocarbons under the sub-tropical climate and its implication for regional cycling, Environ. Pollut., 2020, 261, 114–143 CrossRef PubMed.
- M. J. Climent, C. Coscollà, A. López, R. Barra and R. Urrutia, Legacy and current-use pesticides (CUPs) in the atmosphere of a rural area in central Chile, using passive air samplers, Sci. Total Environ., 2019, 662, 646–654 CrossRef CAS PubMed.
- B. H. Wilford, T. Harner, J. Zhu, M. Shoeib and K. C. Jones, Passive sampling survey of polybrominated diphenyl ether flame retardants in indoor and outdoor air in Ottawa, Canada: Implications for sources and exposure, Environ. Sci. Technol., 2004, 38, 5312–5318 CrossRef CAS PubMed.
- M. Shoeib, T. Harner, B. H. Wilford, K. C. Jones and J. Zhu, Perfluorinated sulfonamides in indoor and outdoor air and indoor dust: Occurrence, partitioning, and human exposure, Environ. Sci. Technol., 2005, 39, 6599–6606 CrossRef CAS PubMed.
- X. Wang, A. P. W. Banks, C. He, D. S. Drage, C. L. Gallen, Y. Li, Q. Li, P. K. Thai and J. F. Müller, Polycyclic aromatic hydrocarbons, polychlorinated biphenyls and legacy and current pesticides in indoor environment in Australia – occurrence, sources and exposure risks, Sci. Total Environ., 2019, 693, 133588 CrossRef CAS PubMed.
- R. E. Dodson, V. Bessonneau, J. O. Udesky, M. Nishioka, M. McCauley and R. A. Rudel, Passive indoor air sampling for consumer product chemicals: a field evaluation study, J. Exposure Sci. Environ. Epidemiol., 2019, 29, 95–108 CrossRef CAS PubMed.
- N. J. Herkert, J. C. Jahnke and K. C. Hornbuckle, Emissions of tetrachlorobiphenyls (PCBs 47, 51, and 68) from polymer resin on kitchen cabinets as a non-Aroclor source to residential air, Environ. Sci. Technol., 2018, 52, 5154–5160 CrossRef CAS PubMed.
- P. Bohlin, K. C. Jones, L. O. Levin, R. Lindahl and B. Strandberg, Field evaluation of a personal passive air sampler for PAH exposure in workplaces, J. Environ. Monit., 2010, 12, 1437–1444 RSC.
- B. Strandberg, A. Julander, M. Sjöström, M. Lewné, H. Koca Akdeva and C. Bigert, Evaluation of polyurethane foam passive air sampler (PUF) as a tool for occupational PAH measurements, Chemosphere, 2018, 190, 35–42 CrossRef CAS PubMed.
- M. Sorais, A. Rezaei, J. O. Okeme, M. L. Diamond, R. Izquierdo, J.-F. Giroux and J. Verreault, A miniature bird-borne passive air sampler for monitoring halogenated flame retardants, Sci. Total Environ., 2017, 599/600, 1903–1911 CrossRef PubMed.
- Y. Zhang, S. Deng, Y. Liu, G. Shen, X. Li, J. Cao, X. Wang, B. Reid and S. Tao, A passive air sampler for characterizing the vertical concentration profile of gaseous phase polycyclic aromatic hydrocarbons in near soil surface air, Environ. Pollut., 2011, 159, 694–699 CrossRef CAS PubMed.
- B. Yang, B. Han, N. Xue, L. Zhou and F. Li, Air–soil exchange of organochlorine pesticides in a sealed chamber, J. Environ. Sci., 2015, 27, 241–250 CrossRef CAS PubMed.
- C. F. Wang, X. P. Wang, J. Ren, P. Gong and T. D. Yao, Using a passive air sampler to monitor air–soil exchange of organochlorine pesticides in the pasture of the central Tibetan plateau, Sci. Total Environ., 2017, 580, 958–965 CrossRef CAS PubMed.
- S. Tao, Y. N. Liu, C. Lang, W. T. Wang, H. S. Yuan, D. Y. Zhang, W. X. Qiu, J. M. Liu, Z. G. Liu, S. Z. Liu, R. Yi, M. Ji and X. X. Liu, A directional passive air sampler for monitoring polycyclic aromatic hydrocarbons (PAHs) in air mass, Environ. Pollut., 2008, 156, 435–441 CrossRef CAS PubMed.
- H. Xiao, H. Hung, T. Harner, Y. D. Lei, G. W. Johnston and F. Wania, A flow-through sampler for semi-volatile organic compounds in air, Environ. Sci. Technol., 2007, 41, 250–256 CrossRef CAS PubMed.
- H. Xiao, H. Hung, T. Harner, Y. D. Lei and F. Wania, Field testing a flow-through sampler for semi-volatile organic compounds in air, Environ. Sci. Technol., 2008, 42, 2970–2975 CrossRef CAS PubMed.
- H. Xiao, H. Hung, Y. D. Lei and F. Wania, Validation of a flow-through sampler for pesticides and polybrominated diphenyl ethers in air, Atmos. Environ., 2009, 43, 2401–2409 CrossRef CAS.
- H. Xiao, L. Shen, Y. Su, E. Barresi, M. DeJong, H. Hung, Y. D. Lei, F. Wania, E. J. Reiner, E. Sverko and S.-C. Kang, Atmospheric concentrations of halogenated flame retardants at two remote locations: The Canadian High Arctic and the Tibetan Plateau, Environ. Pollut., 2012, 161, 154–161 CrossRef CAS PubMed.
- H. Xiao, H. Hung, F. Wania, R. Lao, E. Sablic, E. Sverko, Y. D. Lei, P. Fellin and E. Barresi, Field evaluation of a flow-through sampler for measuring pesticides and brominated flame retardants in the Arctic atmosphere, Environ. Sci. Technol., 2012, 46, 7669–7676 CrossRef CAS PubMed.
- C. L. Davie-Martin, K. J. Hageman, Y.-P. Chin, B. J. Nistor and H. Hung, Concentrations, gas–particle distributions, and source indicator analysis of brominated flame retardants in air at Toolik Lake, Arctic Alaska, Environ. Sci.: Processes Impacts, 2016, 18, 1274–1284 RSC.
- Y. Yu, H. Hung, N. Alexandrou, P. Roach and K. Nordin, Multiyear measurements of flame retardants and organochlorine pesticides in air in Canada's Western sub-Arctic, Environ. Sci. Technol., 2015, 49, 8623–8630 CrossRef CAS PubMed.
- H. Xiao, S. C. Kang, Q. G. Zhang, W. W. Han, M. Loewen, F. Wong, H. Hung, Y. D. Lei and F. Wania, Transport of semivolatile organic compounds to the Tibetan Plateau: Monthly resolved air concentrations at Nam Co, J. Geophys. Res., 2010, 115, D16310 CrossRef.
- S. Bengtson Nash, S. Wild, D. Hawker, R. Cropp, A. Bignert, H. Hung, F. Wania, H. Xiao, P. Bohlin-Nizzetto and S. Broomhall, Persistent organic pollutants in the East Antarctic atmosphere: Inter-annual observations from 2010–2015 using high flow-through passive sampling, Environ. Sci. Technol., 2017, 51, 13929–13937 CrossRef CAS PubMed.
- T. S. Geoghegan, K. J. Hageman and A. J. Hewitt, Testing flow-through air samplers for use in near-field vapour drift studies by measuring pyrimethanil in air after spraying, Environ. Sci.: Processes Impacts, 2014, 16, 422–432 RSC.
- F. Esen, Development of a passive sampling device using polyurethane foam (PUF) to measure polychlorinated biphenyls (PCBs) and organochlorine pesticides (OCPs) near landfills, Environ. Forensics, 2013, 14, 1–8 CrossRef CAS.
- F. Esen, Y. M. Evci and Y. Taşdemir, Evaluation and application of a passive air sampler for polycylic aromatic hydrocarbons (PAHs), J. Environ. Sci. Health, Part A: Toxic/Hazard. Subst. Environ. Eng., 2017, 52, 1022–1029 CrossRef CAS PubMed.
- S. Oono, K. H. Harada, M. A. M. Mahmoud, K. Inoue and A. Koizumi, Current levels of airborne polyfluorinated telomers in Japan, Chemosphere, 2008, 73, 932–937 CrossRef CAS PubMed.
- J.-O. Levin, C.-A. Nilsson and K. Andersson, Sampling of organic substances in workroom air using amberlite XAD-2 resin, Chemosphere, 1977, 6, 595–598 CrossRef CAS.
- J. E. Woodrow, J. N. Seiber, D. G. Crosby, K. W. Moilanen, C. J. Soderquist and C. Mourer, Airborne and surface residues of parathion and its conversion products in a treated plum orchard environment, Arch. Environ. Contam. Toxicol., 1977, 6, 175–191 CrossRef CAS PubMed.
- J. C. Chuang, S. W. Hannan and N. K. Wilson, Field comparison of polyurethane foam and XAD-2 resin for air sampling for polynuclear aromatic hydrocarbons, Environ. Sci. Technol., 1987, 21, 798–804 CrossRef CAS PubMed.
- R. Dobson, A. Scheyer, A. Laure Rizet, P. Mirabel and M. Millet, Comparison of the efficiencies of different types of adsorbents at trapping currently used pesticides in the gaseous phase using the technique of high-volume sampling, Anal. Bioanal. Chem., 2006, 386, 1781–1789 CrossRef CAS PubMed.
- A. Ramírez Restrepo, S. J. Hayward, J. M. Armitage and F. Wania, Evaluating the PAS-SIM model using a passive air sampler calibration study for pesticides, Environ. Sci.: Processes Impacts, 2015, 17, 1228–1237 RSC.
- X. Zhang, T. N. Brown, A. Ansari, B. Yeun, K. Kitaoka, A. Kondo, Y. D. Lei and F. Wania, Effect of wind on the chemical uptake kinetics of a passive air sampler, Environ. Sci. Technol., 2013, 47, 7868–7875 CrossRef CAS PubMed.
- X. Zhang, C. Wong, Y. D. Lei and F. Wania, Influence of sampler configuration on the uptake kinetics of a passive air sampler, Environ. Sci. Technol., 2012, 46, 397–403 CrossRef CAS PubMed.
- X. Zhang, M. Hoang, Y. D. Lei and F. Wania, Exploring the role of the sampler housing in limiting uptake of semivolatile organic compounds in passive air samplers, Environ. Sci.: Processes Impacts, 2015, 17, 2006–2012 RSC.
- W. J. Liu, D. Chen, X. D. Liu, X. Y. Zheng, W. Yang, J. N. Westgate and F. Wania, Transport of semivolatile organic compounds to the Tibetan Plateau: Spatial and temporal variation in air concentrations in mountainous Western Sichuan, China, Environ. Sci. Technol., 2010, 44, 1559–1565 CrossRef CAS PubMed.
- C. Shunthirasingham, R. Barra, G. Mendoza, M. Montory, C. E. Oyiliagu, Y. D. Lei and F. Wania, Spatial variability of semivolatile organic compounds in the Chilean atmosphere, Atmos. Environ., 2011, 45, 303–309 CrossRef CAS.
- P. Gong, X. Wang, X. Liu and F. Wania, Field calibration of XAD-based passive air sampler on the Tibetan Plateau: wind influence and configuration improvement, Environ. Sci. Technol., 2017, 51, 5642–5649 CrossRef CAS PubMed.
- A. Gawor, C. Shunthirasingham, S. J. Hayward, Y. D. Lei, T. Gouin, B. Mmereki, W. Masamba, C. Ruepert, L. E. Castillo, S. C. Lee, M. Shoeib, T. Harner and F. Wania, Neutral perfluoroalkyl substances in the global atmosphere, Environ. Sci.: Processes Impacts, 2014, 16, 404–413 RSC.
- J. Wagner and D. Leith, Passive aerosol sampler. Part I: Principle of operation, Aerosol Sci. Technol., 2001, 34, 186–192 CrossRef CAS.
- K. Arnoldsson, R. Magnusson, L. Hägglund, C. Lejon and H. Wingfors, Initial evaluation of an axial passive sampler for PAHs and OPAHs using substrates with and without gas sampling capacity and varying diffusion distances, Atmos. Pollut. Res., 2015, 6, 673–681 CrossRef CAS.
- R. Magnusson, K. Arnoldsson, C. Lejon, L. Hägglund and H. Wingfors, Field evaluation and calibration of a small axial passive air sampler for gaseous and particle bound polycyclic aromatic hydrocarbons (PAHs) and oxygenated PAHs, Environ. Pollut., 2016, 216, 235–244 CrossRef CAS PubMed.
- A. Sonnette, M. Millet, R. Ocampo, L. Alleman and P. Coddeville, Tenax-TA spiking approach
of thermal desorption coupled to GC–MSMS for the quantification of PAHs in indoor air and dust, Polycyclic Aromat. Compd., 2017, 37, 170–177 CrossRef CAS.
- T. Primbs, S. Genualdi and S. M. Simonich, Solvent selection for pressurized liquid extraction of polymeric sorbents used in air sampling, Environ. Toxicol. Chem., 2008, 27, 1267–1272 CrossRef CAS PubMed.
- G. E. Arias-Loaiza, E. Beristain-Montiel, S. Gómez-Arroyo and O. A. Amador-Muñoz, A thermo-cavitation method to determine organochlorine pesticides in gas and particle phases collected in polyurethane foam used in passive air samplers, Water, Air, Soil Pollut., 2018, 229–282 Search PubMed.
- N. J. Herkert, A. Martinez and K. C. Hornbuckle, A model using local weather data to determine the effective sampling volume for PCB congeners collected on passive air samplers, Environ. Sci. Technol., 2016, 50, 6690–6697 CrossRef CAS PubMed.
- X. Zhang, M. Tsurukawa, T. Nakano, Y. D. Lei and F. Wania, Sampling medium side resistance to uptake of semi-volatile organic compounds in passive air samplers, Environ. Sci. Technol., 2011, 45, 10509–10515 CrossRef CAS PubMed.
- J. O. Okeme, E. M. Webster, J. M. Parnis and M. L. Diamond, Approaches for estimating PUF-air partitions coefficient for semi-volatile organic compounds: A critical comparison, Chemosphere, 2017, 168, 199–204 CrossRef CAS PubMed.
- X. M. Zhang and F. Wania, Modeling the uptake of semi-volatile organic compounds by passive air samplers: Importance of mass transfer processes within the porous sampling media, Environ. Sci. Technol., 2012, 46, 9563–9570 CrossRef CAS PubMed.
- K. Pozo, T. Harner, F. Wania, D. C. G. Muir, K. C. Jones and L. A. Barrie, Toward a global network for persistent organic pollutants in air: Results from the GAPS study, Environ. Sci. Technol., 2006, 40, 4867–4873 CrossRef CAS PubMed.
- C. Persoon and K. C. Hornbuckle, Calculation of passive sampling rates from both native PCBs and depuration compounds in indoor and outdoor environments, Chemosphere, 2009, 74, 917–923 CrossRef CAS PubMed.
- C. Shunthirasingham, B. T. Mmereki, W. Masamba, C. E. Oyiliagu, Y. D. Lei and F. Wania, Fate of pesticides in the arid subtropics, Botswana, Southern Africa, Environ. Sci. Technol., 2010, 44, 8082–8088 CrossRef CAS PubMed.
- L. Sprunger, A. Proctor, W. E. Acree and M. H. Abraham, Characterization of the sorption of gaseous and organic solutes onto polydimethyl siloxane solid-phase microextraction surfaces using the Abraham model, J. Chromatogr. A, 2007, 1175, 162–173 CrossRef CAS PubMed.
- J. O. Okeme, J. M. Parnis, J. Poole, M. L. Diamond and L. M. Jantunen, Poly-dimethylsiloxane–air partition ratios for semi-volatile organic compounds by GC-based measurement and COSMO-RS estimation: rapid measurements and accurate modelling, Chemosphere, 2016, 156, 204–211 CrossRef CAS PubMed.
- P. C. Tromp, H. Beeltje, J. O. Okeme, R. Vermeulen, A. Pronk and M. L. Diamond, Calibration of polydimethylsiloxane and polyurethane foam passive air samplers for measuring semi volatile organic compounds using a novel exposure chamber design, Chemosphere, 2019, 227, 435–443 CrossRef CAS PubMed.
- D. Zhao, J. C. Little and S. S. Cox, Characterizing polyurethane foam as a sink for or source of volatile organic compounds in indoor air, J. Environ. Eng., 2004, 130, 984–989 CrossRef.
- I. Kamprad and K.-U. Goss, Systematic investigation of the sorption properties of polyurethane foams for organic vapors, Anal. Chem., 2007, 79, 4222–4227 CrossRef CAS PubMed.
- T. F. Bidleman, O. Nygren and M. Tysklind, Field estimates of polyurethane foam air partition coefficients for hexachlorobenzene, alpha-hexachlorocyclohexane and bromoanisoles, Chemosphere, 2016, 159, 126–131 CrossRef CAS PubMed.
- J. M. Parnis, D. Mackay and T. Harner, Modelling PUF passive air samplers: Temperature dependence of polyurethane foam–air partitioning of PAHs and their O-, N-, and S-derivatives computed by COSMO-RS, Atmos. Pollut. Res., 2016, 7, 155–161 CrossRef.
- J. M. Parnis, A. Eng, D. Mackay and T. Harner, Characterizing PUF disk passive air samplers for alkyl-substituted PAHs: measured and modelled PUF-AIR partition coefficients with COSMO-RS, Chemosphere, 2016, 145, 360–364 CrossRef CAS PubMed.
- A. P. Francisco, T. Harner and A. Eng, Measurement of polyurethane foam–air partition coefficients for semivolatile organic compounds as a function of temperature: application to passive air sampler monitoring, Chemosphere, 2017, 174, 638–642 CrossRef CAS PubMed.
- A. Saini, J. Clarke and T. Harner, Direct measurements of polyurethane foam (PUF)–air partitioning coefficients for chemicals of emerging concern capable of equilibrating in PUF disk samplers, Chemosphere, 2019, 234, 925–930 CrossRef CAS PubMed.
- J. F. Pankow, W. Luo, L. M. Isabelle, K. M. Hart and D. F. Hagen, Gas-solid retention volumes of organic compounds on styrene-divinylbenzene and ethylvinylbenzene–divinylvenzene copolymer sorbent beads, J. Chromatogr. A, 1996, 732, 317–326 CrossRef CAS.
- L. Shen, Y. D. Lei and F. Wania, Sorption of chlorobenzene vapors on styrene-divinylbenzene polymer, J. Chem. Eng. Data, 2002, 47, 944–949 CrossRef CAS.
- S. J. Hayward, Y. D. Lei and F. Wania, Sorption of a diverse set of organic chemical vapors onto XAD-2 resin: Measurement, prediction and implications for air sampling, Atmos. Environ., 2011, 45, 296–302 CrossRef CAS.
- K.-U. Goss and R. P. Schwarzenbach, Linear free energy relationships used to evaluate equilibrium partitioning of organic compounds, Environ. Sci. Technol., 2001, 35, 1–9 CrossRef CAS PubMed.
- S. Endo and K.-U. Goss, Applications of polyparameter linear free energy relationships in environmental chemistry, Environ. Sci. Technol., 2014, 48, 12477–12491 CrossRef CAS PubMed.
- T. H. Uber, T. Hüffer, S. Planitz and T. C. Schmidt, Characterization of sorption properties of high-density polyethylene using the poly-parameter linearfree-energy relationships, Environ. Pollut., 2019, 248, 312–319 CrossRef CAS PubMed.
- Y. Choi, Y.-M. Cho and R. G. Luthy, Polyethylene–water partitioning coefficients for parent- and alkylated-polycyclic aromatic hydrocarbons and polychlorinated biphenyls, Environ. Sci. Technol., 2013, 47(13), 6943–6950 CrossRef CAS PubMed.
- M. Schneider and K.-U. Goss, Systematic investigation of the sorption properties of Tenax TA, Chromosorb 106, Porapak N, and Carbopak F, Anal. Chem., 2009, 81, 3017–3021 CrossRef CAS PubMed.
- H. C. Tülp, K.-U. Goss, R. P. Schwarzenbach and K. Fenner, Experimental determination of LSER parameters for a set of 76 diverse pesticides and pharmaceuticals, Environ. Sci. Technol., 2008, 42(6), 2034–2040 CrossRef PubMed.
- A. Stenzel, K.-U. Goss and S. Endo, Determination of polyparameter linear free energy relationship (pp-LFER) substance descriptors for established and alternative flame retardants, Environ. Sci. Technol., 2013, 47(3), 1399–1406 CAS.
- A. Stenzel, K.-U. Goss and S. Endo, Experimental determination of polyparameter linear free energy relationship (pp-LFER) substance descriptors for pesticides and other contaminants: New measurements and recommendations, Environ. Sci. Technol., 2013, 47(24), 14204–14214 CrossRef CAS PubMed.
-
N. Ulrich, S. Endo, T. N. Brown, N. Watanabe, G. Bronner, M. H. Abraham and K.-U. Goss, UFZ-LSER database v. 3.2.1, Helmholtz Centre for Environmental Research-UFZ, Leipzig, Germany, 2017, accessed on 27.01.2020, http://www.ufz.de/lserd Search PubMed.
- K.-U. Goss, Predicting equilibrium sorption of neutral organic chemicals into various polymeric sorbents with COSMO-RS, Anal. Chem., 2011, 83, 5304–5308 CrossRef CAS PubMed.
- C. Loschen and A. Klamt, Prediction of solubilities and partition coefficients in polymers using COSMO-RS, Ind. Eng. Chem. Res., 2014, 53, 11478–11487 CrossRef CAS.
-
Y. Chen, C. Wang and F. Wania, Prediction of partitioning coefficients between XAD-2 and air for organic compounds using COSMOtherm, CGCS internship report, University of Toronto, 2016, p. 27 Search PubMed.
- M. Mari, M. Schuhmacher, J. Feliubadalo and J. L. Domingo, Air concentrations of PCDD/Fs, PCBs and PCNs using active and passive air samplers, Chemosphere, 2008, 70, 1637–1643 CrossRef CAS PubMed.
- A. Carratalá, R. Moreno-González and V. M. León, Occurrence and seasonal distribution of polycyclic aromatic hydrocarbons and legacy and current-use pesticides in air from a Mediterranean coastal lagoon (Mar Menor, SE Spain), Chemosphere, 2017, 167, 382–395 CrossRef PubMed.
- J. He and R. Balasubramanian, A comparative evaluation of passive and active samplers for measurements of gaseous semi-volatile organic compounds in the tropical atmosphere, Atmos. Environ., 2010, 44, 884–891 CrossRef CAS.
- S.-K. Kim, M. Shoeib, K.-K. Kim and J.-E. Park, Indoor and outdoor poly- and perfluoroalkyl substances (PFASs) in Korea determined by passive air sampler, Environ. Pollut., 2012, 162, 144–150 CrossRef CAS PubMed.
- A. Saini, J. O. Okeme, E. Goosey and M. L. Diamond, Calibration of two passive air samplers for monitoring phthalates and brominated flame-retardants in indoor air, Chemosphere, 2015, 137, 166–173 CrossRef CAS PubMed.
- D. T. Waite, N. R. Droneck, L. Tuduri, J. F. Sproul, D. F. Chau and D. V. Quiring, Comparison of active versus passive atmospheric samplers for some current-use pesticides, Bull. Environ. Contam. Toxicol., 2005, 74, 1011–1018 CrossRef CAS PubMed.
- C. Chaemfa, J. L. Barber, C. Moeckel, T. Gocht, T. Harner, I. Holoubek, J. Klánová and K. C. Jones, Field calibration of polyurethane foam disk passive air samplers for PBDEs, J. Environ. Monit., 2009, 11, 1859–1865 RSC.
- C. Chaemfa, J. L. Barber, K.-S. Kim, T. Harner and K. C. Jones, Further studies on the uptake of persistent organic pollutants (POPs) by polyurethane foam disk passive air samplers, Atmos. Environ., 2009, 43, 3843–3849 CrossRef CAS.
- J. L. Armstrong, M. G. Yost and R. A. Fenske, Development of a passive air sampler to measure airborne organophosphorus pesticides and oxygen analogs in an agricultural community, Chemosphere, 2014, 111, 135–143 CrossRef CAS PubMed.
- P. Bohlin, O. Audy, L. Škrdlíková, P. Kukučka, P. Přibylová, R. Prokeš, Š. Vojta and J. Klańová, Outdoor passive air monitoring of semi volatile organic compounds (SVOCs): a critical evaluation of performance and limitations of polyurethane foam (PUF) disks, Environ. Sci.: Processes Impacts, 2014, 16, 433–444 RSC.
- J. Heo and G. Lee, Field-measured uptake rates of PCDDs/Fs and dl-PCBs using PUF-disk passive air samplers in Gyeonggi-do, South Korea, Sci. Total Environ., 2014, 491/492, 42–50 CrossRef PubMed.
- T. Harner, K. Su, S. Genualdi, J. Karpowicz, L. Ahrens, C. Mihele, J. Schuster, J.-P. Charland and J. Narayan, Calibration and application of PUF disk passive air samplers for tracking polycyclic aromatic compounds (PACs), Atmos. Environ., 2013, 75, 123–128 CrossRef CAS.
- Y. M. Evci, F. Esen and Y. Taşdemir, Monitoring of long-term outdoor concentrations of PAHs with passive air samplers and comparison with meteorological data, Arch. Environ. Contam. Toxicol., 2016, 71, 246–256 CrossRef CAS PubMed.
- A. E. Sakin, F. Esen and Y. Taşdemir, Effects of sampling interval on the passive air sampling of atmospheric PCBs levels, J. Environ. Sci. Health, Part A: Toxic/Hazard. Subst. Environ. Eng., 2017, 52, 673–679 CrossRef CAS PubMed.
- A. Abdollahi, A. Eng, L. M. Jantunen, L. Ahrens, M. Shoeib, J. M. Parnis and T. Harner, Characterization of polyurethane foam (PUF) and sorbent impregnated PUF (SIP) disk passive air samplers for measuring organophosphate flame retardants, Chemosphere, 2017, 167, 212–219 CrossRef CAS PubMed.
- H. Jiang, G. Zhong, J. Wang, H. Jiang, C. Tian, J. Li, S. Zhao, Z. Yu, L. Morawska and G. Zhang, Using polyurethane foam-based passive air sampling technique to monitor monosaccharides at a regional scale, Environ. Sci. Technol., 2018, 52, 12546–12555 CrossRef CAS PubMed.
- Y. Wang, X. Zhu, Y. Gao, H. Bai, P. Wang, J. Chen, H. Yuan, L. Wang, X. Li and W. Wang, Monitoring gas- and particulate-phase short-chain polychlorinated paraffins in the urban air of Dalian by a self-developed passive sampler, J. Environ.
Sci., 2019, 80, 287–295 CrossRef PubMed.
- K. M. Ellickson, C. M. McMahon, C. Herbrandson, M. J. Krause, C. M. Schmitt, C. J. Lippert and G. C. Pratt, Analysis of polycyclic aromatic hydrocarbons (PAHs) in air using passive sampling calibrated with active measurements, Environ. Pollut., 2017, 231, 487–496 CrossRef CAS PubMed.
- M. E. Bartkow, J. N. Huckins and J. F. Müller, Field-based evaluation of semipermeable membrane devices (SPMDs) as passive air samplers of polyaromatic hydrocarbons (PAHs), Atmos. Environ., 2004, 38, 5983–5990 CrossRef CAS.
- G. Liu, G. Zhang, J. Lia, X. Li, X. Peng and S. Qi, Spatial distribution and seasonal variations of polycyclic aromatic hydrocarbons (PAHs) using semi-permeable membrane devices (SPMD) and pine needles in the Pearl River Delta, South China, Atmos. Environ., 2006, 40, 3134–3143 CrossRef CAS.
- S. Harrad and M. A.-E. Abdallah, Calibration of two passive air sampler configurations for monitoring concentrations of hexabromocyclododecanes in indoor air, J. Environ. Monit., 2008, 10, 527–531 RSC.
- P. Bohlin, K. C. Jones and B. Strandberg, Field evaluation of polyurethane foam passive air samplers to assess airborne PAHs in occupational environments, Environ. Sci. Technol., 2010, 44, 749–754 CrossRef CAS PubMed.
- S.-K. Kim and J.-E. Park, Comparison of two different passive air samplers (PUF-PAS versus SIP-PAS) to determine time-integrated average air concentration of volatile hydrophobic organic pollutants, Ocean Sci. J., 2014, 49, 137–150 CrossRef CAS.
- P. Bohlin, O. Audy, L. Škrdlíková, P. Kukučka, Š. Vojta, P. Přibylová, R. Prokeš, P. Čupr and J. Klánová, Evaluation and guidelines for using polyurethane foam (PUF) passive air samplers in double-dome chambers to assess semi-volatile organic compounds (SVOCs) in non-industrial indoor environments, Environ. Sci.: Processes Impacts, 2014, 16, 2617–2626 RSC.
- J. L. Armstrong, R. A. Fenske, M. G. Yost, M. Tchong-French and J. Yu, Comparison of polyurethane foam and XAD-2 sampling matrices to measure airborne organophosphorus pesticides and their oxygen analogs in an agricultural community, Chemosphere, 2013, 92, 451–457 CrossRef CAS PubMed.
- N. Jariyasopit, Y. Liu, J. Liggio and T. Harner, Stability of polycyclic aromatic compounds in polyurethane foam-type passive air samplers upon O3 exposure, Atmos. Environ., 2015, 120, 200–204 CrossRef CAS.
- L. Melymuk, P. Bohlin-Nizzetto, R. Prokeš, P. Kukučka, P. Přibylová, Š. Vojta, J. Kohoutek, G. Lammel and J. Klánová, Uncertainties in monitoring of SVOCs in air caused by within-sampler degradation during active and passive air sampling, Atmos. Environ., 2017, 167, 553–565 CrossRef CAS.
-
T. Harner, Template for calculating PUF and SIP disk sample air volumes, Version 2, April 6, 2020, https://www.researchgate.net/profile/Tom_Harner/publications Search PubMed.
- S. J. Hayward, T. Gouin and F. Wania, Levels and seasonal variability of pesticides in the rural atmosphere of Southern Ontario, J. Agric. Food Chem., 2010, 58, 1077–1084 CrossRef CAS PubMed.
- K. Prevedouros, E. Brorström-Lundén, C. J. Halsall, K. C. Jones, R. G. M. Lee and A. J. Sweetman, Seasonal and long-term trends in atmospheric PAH concentrations: evidence and implications, Environ. Pollut., 2004, 128, 17–27 CrossRef CAS PubMed.
- F. Wania, J.-E. Haugen, Y. D. Lei and D. Mackay, Temperature dependence of atmospheric concentrations of semivolatile organic compounds, Environ. Sci. Technol., 1998, 32, 1013–1021 CrossRef CAS.
- I. S. Krogseth, A. Kierkegaard, M. S. McLachlan, K. Breivik, K. M. Hansen and M. Schlabach, Occurrence and seasonality of cyclic volatile methyl siloxanes in Arctic air, Environ. Sci. Technol., 2013, 47, 502–509 CrossRef CAS PubMed.
- K. Kennedy, D. W Hawker, M. E Bartkow, S. Carter, Y. Ishikawa and J. F Müller, The potential effect of differential ambient and deployment chamber temperatures on PRC derived sampling rates with polyurethane foam (PUF) passive air samplers, Environ. Pollut., 2010, 158, 142–147 CrossRef CAS PubMed.
- N. Domínguez-Morueco, M. Schuhmacher, J. Sierra, M. Nadal and J. L. Domingo, Assessment of PAH loss in passive air samplers by the effect of temperature, Atmos. Pollut. Res., 2016, 7, 142–146 CrossRef.
- K. Booij and F. Smedes, An improved method for estimating in situ sampling rates of nonpolar passive samplers, Environ. Sci. Technol., 2010, 44, 6789–6794 CrossRef CAS PubMed.
- C. A. McDonough, D. G. Franks, M. E. Hahn and R. Lohmann, Aryl hydrocarbon receptor-mediated activity of gas-phase ambient air derived from passive sampling and an in vitro bioassay, Environ. Toxicol. Chem., 2019, 38, 748–759 CrossRef CAS PubMed.
- N. T. Petrich, S. N. Spak, G. R. Carmichael, D. Hu, A. Martinez and K. C. Hornbuckle, Simulating and explaining passive air sampling rates for semivolatile compounds on polyurethane foam passive samplers, Environ. Sci. Technol., 2013, 47, 8591–8598 CAS.
- A. Pennequin-Cardinal, H. Plaisance, N. Locoge, O. Ramalho, S. Kirchner and J.-C. Galloo, Performances of the Radiello® diffusive sampler for BTEX measurements: influence of environmental conditions and determination of modelled sampling rates, Atmos. Environ., 2005, 39, 2535–2544 CrossRef CAS.
- H. Skov, B. T. Sørensen, M. S. Landis, M. S. Johnson, P. Sacco, M. E. Goodsite, C. Lohse and K. S. Christiansen, Performance of a new diffusive sampler for Hg0 determination in the troposphere, Environ. Chem., 2007, 4, 75–80 CrossRef CAS.
- H. Plaisance, The effect of the wind velocity on the uptake rates of various diffusive samplers, Int. J. Environ. Anal. Chem., 2011, 91, 1341–1352 CrossRef CAS.
- D. S. McLagan, C. P. J. Mitchell, H. Huang, B. Abdul Hussain, Y. D. Lei and F. Wania, The effects of meteorological parameters and diffusive barrier reuse on the sampling rate of a passive air sampler for gaseous mercury, Atmos. Meas. Tech., 2017, 10, 3651–3660 CrossRef CAS.
- L. M. van Mourik, X. Wang, C. Paxman, P. E. G. Leonards, F. Wania, J. de Boer and J. F. Müller, Spatial variation of short- and medium-chain chlorinated paraffins in ambient air across Australia, Environ. Pollut., 2020, 261, 114141 CrossRef CAS PubMed.
- X. H. Zhu, G. H. Ding, W. Levy, G. Jakobi, I. Offenthaler, W. Moche, P. Weiss and K.-W. Schramm, QSPR study about sampling rates of semipermeable membrane devices for monitoring of organochlorine pesticides in Alps air, Chin. Sci. Bull., 2011, 56, 1884–1889 CrossRef CAS.
- X. H. Zhu, G. H. Ding, W. Levy, G. Jakobi and K.-W. Schramm, Relationship of air sampling rates of semipermeable membrane devices with the properties of organochlorine pesticides, J. Environ. Sci., 2011, 23(supplement), S40–S44 CrossRef.
- G. L. Daly, Y. D. Lei, L. E. Castillo, D. C. G. Muir and F. Wania, Polycyclic aromatic hydrocarbons in Costa Rican air and soil: A tropical/temperate comparison, Atmos. Environ., 2007, 41, 7339–7350 CrossRef CAS.
- M. Z. Markovic, S. Prokop, R. M. Staebler, J. Liggio and T. Harner, Evaluation of the particle infiltration efficiency of three passive samplers and the PS-1 active air sampler, Atmos. Environ., 2015, 112, 289–293 CrossRef CAS.
- Q. Li, K. Yang, J. Li, X. Zeng, Z. Yu and G. Zhang, An assessment of polyurethane foam passive samplers for atmospheric metals compared with active samplers, Environ. Pollut., 2018, 236, 498–504 CrossRef CAS PubMed.
- E. O. Gaga, T. Harner, E. Dabek-Zlotorzynska, V. Celo, G. Evans, C.-H. Jeong, S. Halappanavar, N. Jariyasopit and Y. Su, Polyurethane Foam (PUF) disk samplers for measuring trace metals in ambient air, Environ. Sci. Technol. Lett., 2019, 6, 545–550 CrossRef CAS.
- L. Melymuk, P. Bohlin, O. Sáńka, K. Pozo and J. Klańová, Current challenges in air sampling of semivolatile organic contaminants: Sampling artifacts and their influence on data comparability, Environ. Sci. Technol., 2014, 48, 14077–14091 CrossRef CAS PubMed.
- L. Vilavert, M. Nadal, M. Schuhmacher and J. L. Domingo, Seasonal surveillance of airborne PCDD/Fs, PCBs and PCNs using passive samplers to assess human health risks, Sci. Total Environ., 2014, 466/467, 733–740 CrossRef PubMed.
- L. Gao, Q. Zhang, L. Liu, C. Li and Y. Wang, Spatial and seasonal distributions of polychlorinated dibenzo-p-dioxins and dibenzofurans and polychlorinated biphenyls around a municipal solid waste incinerator, determined using polyurethane foam passive air samplers, Chemosphere, 2014, 114, 317–326 CrossRef CAS PubMed.
- M. Shoeib, J. Schuster, C. Rauert, K. Su, S.-A. Smyth and T. Harner, Emission of poly and perfluoroalkyl substances, UV-filters and siloxanes to air from wastewater treatment plants, Environ. Pollut., 2016, 218, 595–604 CrossRef CAS PubMed.
- F. Wong, M. Robson, L. Melymuk, C. Shunthirasingham, N. Alexandrou, M. Shoeib, E. Luk, P. Helm, M. L. Diamond and H. Hung, Urban sources of synthetic musk compounds to the environment, Environ. Sci.: Processes Impacts, 2019, 21, 74–88 RSC.
- J. Klánová, J. Kohoutek, L. Hamplova, P. Urbanova and I. Holoubek, Passive air sampler as a tool for long-term air pollution monitoring: Part 1. Performance assessment for seasonal and spatial variations, Environ. Pollut., 2006, 144, 393–405 CrossRef PubMed.
- N. Domínguez-Morueco, S. Augusto, L. Trabalón, E. Pocurull, F. Borrull, M. Schuhmacher, J. L. Domingo and M. Nadal, Monitoring PAHs in the petrochemical area of Tarragona County, Spain: comparing passive air samplers with lichen transplants, Environ. Sci. Pollut. Res., 2017, 24, 11890–11900 CrossRef PubMed.
- S.-Y. Baek, S.-D. Choi, H. Park, J.-H. Kang and Y.-S. Chang, Spatial and seasonal distribution of polychlorinated biphenyls (PCBs) in the vicinity of an iron and steel making plant, Environ. Sci. Technol., 2010, 44, 3035–3040 CrossRef CAS PubMed.
- J. K. Schuster, T. Harner, K. Su, C. Mihele and A. Eng, First results from the oil sands passive air monitoring network for polycyclic aromatic compounds, Environ. Sci. Technol., 2015, 49, 2991–2998 CrossRef CAS PubMed.
- J. K. Schuster, T. Harner, K. Su, A. Eng, A. Wnorowski and J.-P. Charland, Temporal and spatial trends of polycyclic aromatic compounds in air across the Athabasca Oil Sands Region reflect inputs from open pit mining and forest fires, Environ. Sci. Technol. Lett., 2019, 6, 178–183 CrossRef CAS.
- N. Jariyasopit, Y. Zhang, J. W. Martin and T. Harner, Comparison of polycyclic aromatic compounds in air measured by conventional passive air samplers and passive dry deposition samplers and contributions from petcoke and oil sands ore, Atmos. Chem. Phys., 2018, 18, 9161–9171 CrossRef CAS.
- J. Hu, M. Zheng, W. Liu, Z. Nie, C. Li, G. Liu and K. Xiao, Characterization of polychlorinated dibenzo-p-dioxins and dibenzofurans, dioxin-like polychlorinated biphenyls, and polychlorinated naphthalenes in the environment surrounding secondary copper and aluminum metallurgical facilities in China, Environ. Pollut., 2014, 193, 6–12 CrossRef CAS PubMed.
- T. H. Nøst, A. K. Halse, S. Randall, A. R. Borgen, M. Schlabach, A. Paul, A. Rahman and K. Breivik, High concentrations of organic contaminants in air from ship breaking activities in Chittagong, Bangladesh, Environ. Sci. Technol., 2015, 49, 11372–11380 CrossRef PubMed.
- H. Sun, Y. Li, P. Wang, S. Zheng, J. Matsiko, D. Wang, W. Zhang, Y. Hao, Q. Zhang and G. Jiang, Atmospheric levels and distribution of Dechlorane Plus in an E-waste dismantling region of East China, Sci. China: Chem., 2017, 60, 305–310 CrossRef CAS.
- A. De la Torre, I. Navarro, P. Sanz, M. A. Arjol, J. Fernández and M. A. Martínez, HCH air levels derived from Bailín dumpsite dismantling (Sabiñánigo, Spain), Sci. Total Environ., 2018, 626, 1367–1372 CrossRef CAS PubMed.
- M. Petrovic, M. Sremacki, J. Radonic, I. Mihajlovic, B. Obrovski and M. Vojinovic Miloradov, Health risk assessment of PAHs, PCBs and OCPs in atmospheric air of municipal solid waste landfill in Novi Sad, Serbia, Sci. Total Environ., 2018, 644, 1201–1206 CrossRef CAS PubMed.
- P. Wang, N. Zhao, Y. Cui, W. Jiang, L. Wang, Z. Wang, X. Chen, L. Jiang and L. Ding, Short-chain chlorinated paraffin (SCCP) pollution from a CP production plant in China: Dispersion, congener patterns and health risk assessment, Chemosphere, 2018, 211, 456–464 CrossRef CAS PubMed.
- M. M. Gune, W.-L. Ma, S. Sampath, W. Li, Y.-F. Li, H. N. Udayashankar, K. Balakrishna and Z. Zhang, Occurrence of polycyclic aromatic hydrocarbons (PAHs) in air and soil surrounding a coal-fired thermal power plant in the south-west coast of India, Environ. Sci. Pollut. Res., 2019, 26, 22772–22782 CrossRef CAS PubMed.
- N. A. O. Morin, P. L. Andersson, S. E. Hale and H. P. H. Arp, The presence and partitioning behavior of flame retardants in waste, leachate, and air particles from Norwegian waste-handling facilities, J. Environ. Sci., 2017, 62, 115–132 CrossRef PubMed.
- H. P. Arp, N. A. Morin, P. L. Andersson, S. E. Hale, F. Wania, K. Breivik and G. D. Breedveld, The presence, emission and partitioning behavior of polychlorinated biphenyls in waste, leachate and aerosols from Norwegian waste-handling facilities, Sci. Total Environ., 2020, 136824 CrossRef CAS PubMed.
- H. Q. Anh, K. Tomioka, N. M. Tue, L. H. Tuyen, N. K. Chi, T. B. Minh, P. H. Viet and S. Takahashi, A preliminary investigation of 942 organic micro-pollutants in the atmosphere in waste processing and urban areas, northern Vietnam: Levels, potential sources, and risk assessment, Ecotoxicol. Environ. Saf., 2019, 167, 354–364 CrossRef CAS PubMed.
- H. Q. Anh, I. Watanabe, T. N. Tue, L. H. Tuyen, P. H. Viet, N. K. Chi, T. B. Minh and S. Takahashi, Polyurethane foam-based passive air sampling for simultaneous determination of POP- and PAH-related compounds: A case study in informal waste processing and urban areas, northern Vietnam, Chemosphere, 2020, 247, 125991 CrossRef CAS PubMed.
- S. Du, S. J. Wall, L. A. Cacia and L. A. Rodenburg, Passive air sampling for polychlorinated biphenyls in the Philadelphia metropolitan area, Environ. Sci. Technol., 2009, 43, 1287–1292 CrossRef CAS PubMed.
- C. Persoon, T. M. Peters, N. Kumar and K. C. Hornbuckle, Spatial distribution of airborne polychlorinated biphenyls in Cleveland, Ohio and Chicago, Illinois, Environ. Sci. Technol., 2010, 44, 2797–2802 CrossRef CAS PubMed.
- A. A. Peverly, Y. Ma, M. Venier, Z. Rodenburg, S. N. Spak, K. C. Hornbuckle and R. A. Hites, Variations of flame retardant, polycyclic aromatic hydrocarbon, and pesticide concentrations in Chicago's atmosphere measured using passive sampling, Environ. Sci. Technol., 2015, 49, 5371–5379 CrossRef CAS PubMed.
- Y. Hao, Y. Li, T. Wang, Y. Hu, H. Sun, J. Matsiko, S. Zheng, P. Wang and Q. Zhang, Distribution, seasonal variation and inhalation risks of polychlorinated dibenzo-p-dioxins and dibenzofurans, polychlorinated biphenyls and polybrominated diphenyl ethers in the atmosphere of Beijing, China, Environ. Geochem. Health, 2018, 40, 1907–1918 CrossRef CAS PubMed.
- X. Y. Wang, Q. B. Li, Y. M. Luo, Q. Ding, L. M. Xi, J. M. Ma, Y. Li, Y. P. Liu and C. L. Cheng, Characteristics and sources of atmospheric polycyclic aromatic hydrocarbons (PAHs) in Shanghai, China, Environ. Monit. Assess., 2010, 165, 295–305 CrossRef CAS PubMed.
- X. Zheng, D. Chen, X. Liu, Q. Zhou, Y. Liu, W. Yang and G. Jiang, Spatial and seasonal variations of organochlorine compounds in air on an urban–rural transect across Tianjin, China, Chemosphere, 2010, 78, 92–98 CrossRef CAS PubMed.
- L. Ding, Y. M. Li, P. Wang, X. M. Li, Z. S. Zhao, T. Ruan and Q. A. Zhang, Spatial concentration, congener profiles and inhalation risk assessment of PCDD/Fs and PCBs in the atmosphere of Tianjin, China, Chin. Sci. Bull., 2013, 58, 971–978 CrossRef CAS.
- Y. Liang, H. Wang, Q. Yang, S. Cao, C. Yan, L. Zhang and N. Tang, Spatial distribution and seasonal variations of atmospheric organophosphate esters (OPEs) in Tianjin, China based on gridded field observations, Environ. Pollut., 2020, 263, 114460 CrossRef CAS PubMed.
- W.-L. Ma, H. Qi, Y.-F. Li, L.-Y. Liu, D.-Z. Sun, D.-G. Wang, Z. Zhang, C.-G. Tian and J.-M. Shen, Seasonal and spatial variations of air concentrations of polycyclic aromatic hydrocarbons in Northeastern Chinese urban region, Bull. Environ. Contam. Toxicol., 2011, 86, 43–49 CrossRef CAS PubMed.
- E. C. Santiago and M. G. Cayetano, Polycyclic aromatic hydrocarbons in ambient air in the Philippines derived from passive sampler with polyurethane foam disk, Atmos. Environ., 2007, 41, 4138–4147 CrossRef CAS.
- J. Nasir, X. Wang, B. Xu, C. Wang, D. R. Joswiak, S. Rehman, A. Lodhi, S. Shafiq and R. Jilani, Selected organochlorine pesticides and polychlorinated biphenyls in urban atmosphere of Pakistan: Concentration, spatial variation and sources, Environ. Sci. Technol., 2014, 48, 2610–2618 CrossRef CAS PubMed.
- J. Cortés, C. M. González, L. Morales, M. Abalos, E. Abad and B. H. Aristizábal, PCDD/PCDF and dl-PCB in the ambient air of a tropical Andean city: Passive and active sampling measurements near industrial and vehicular pollution sources, Sci. Total Environ., 2014, 491/492, 67–74 CrossRef PubMed.
- J. Cortés, M. Cobo, C. M. González, C. D. Gómez, M. Abalos and B. H. Aristizábal, Environmental variation of PCDD/Fs and dl-PCBs in two tropical Andean Colombian cities using passive samplers, Sci. Total Environ., 2016, 568, 614–623 CrossRef PubMed.
- S.-D. Choi, H.-O. Kwon, Y.-S. Lee, E.-J. Park and J.-Y. Oh, Improving the spatial resolution of atmospheric polycyclic aromatic hydrocarbons using passive air samplers in a multi-industrial city, J. Hazard. Mater., 2012, 241/242, 252–258 CrossRef PubMed.
- Q. T. Vuong, S. J. Kim, T. N. T. Nguyen, P. Q. Thang, S.-J. Lee, T. Ohura and S.-D. Choi, Passive air sampling of halogenated polycyclic aromatic hydrocarbons in the largest industrial city in Korea: Spatial distributions and source identification, J. Hazard. Mater., 2020, 382, 121238 CrossRef CAS PubMed.
- Y. M. Aydin, M. Kara, Y. Dumanoglu, M. Odabasi and T. Elbir, Source apportionment of polycyclic aromatic hydrocarbons (PAHs) and polychlorinated biphenyls (PCBs) in ambient air of an industrial region in Turkey, Atmos. Environ., 2014, 97, 271–285 CrossRef CAS.
- P. Čupr, J. Klánová, T. Bartos, Z. Flegrova, J. Kohoutek and I. Holoubek, Passive air sampler as a tool for long-term air pollution monitoring: Part 2. Air genotoxic potency screening assessment, Environ. Pollut., 2006, 144, 406–413 CrossRef PubMed.
- O. Sanka, L. Melymuk, P. Čupr, A. Dvorska and J. Klánová, Dispersion modeling of selected PAHs in urban air: A new approach combining dispersion model with GIS and passive air sampling, Atmos. Environ., 2014, 96, 88–95 CrossRef CAS.
- P. S. Diefenbacher, A. C. Gerecke, C. Bogdal and K. Hungerbühler, Spatial distribution of atmospheric PCBs in Zürich, Switzerland: Do joint sealants still matter?, Environ. Sci. Technol., 2016, 50, 232–239 CrossRef CAS PubMed.
- M. Lévy, J. Al-Alam, C. Ridacker, S. Massemin and M. Millet, Use of XAD®-2 passive air samplers for monitoring environmental trends of PAHs, PCBs and pesticides in three different sites in Strasbourg and its vicinity (east of France), Atmos. Environ., 2018, 195, 12–23 CrossRef.
- T. Gouin, D. Wilkinson, S. Hummel, B. Meyer and A. Culley, Polycyclic aromatic hydrocarbons in air and snow from Fairbanks, Alaska, Atmos. Pollut. Res., 2010, 1, 9–15 CrossRef CAS.
- N. Jariyasopit, T. Harner, D. Wu, A. Williams, S. Halappanavar and K. Su, Mapping indicators of toxicity for polycyclic aromatic compounds in the atmosphere of the Athabasca oil sands region, Environ. Sci. Technol., 2016, 50(20), 11282–11291 CrossRef CAS PubMed.
- K. Pozo, T. Harner, A. Rudolph, G. Oyola, V. H. Estellano, R. Ahumada-Rudolpha, M. Garrido, K. Pozo, R. Mabilia and S. Focardi, Survey of persistent organic pollutants (POPs) and polycyclic aromatic hydrocarbons (PAHs) in the atmosphere of rural, urban and industrial areas of Concepción, Chile, using passive air samplers, Atmos. Pollut. Res., 2012, 3, 426–434 CrossRef CAS.
- K. Pozo, V. H. Estellano, T. Harner, L. Diaz-Robles, F. Cereceda-Balic, P. Etcharren, K. Pozo, V. Vidal, F. Guerrero and A. Vergara-Fernández, Assessing Polycyclic Aromatic Hydrocarbons (PAHs) using passive air sampling in the atmosphere of one of the most wood-smoke-polluted cities in Chile: The case study of Temuco, Chemosphere, 2015, 134, 475–481 CrossRef CAS PubMed.
- K. Pozo, G. Oyola, V. H. Estellano, T. Harner, A. Rudolph, P. Přibylová, P. Kukučka, O. Audy, J. Klánová, A. Metzdorff and S. Focardi, Persistent Organic Pollutants (POPs) in the atmosphere of three Chilean cities using passive air samplers, Sci. Total Environ., 2017, 586, 107–114 CrossRef CAS PubMed.
- Á. L. Álvarez, K. Pozo, M. I. Paéz, V. H. Estellano, Y. Llanos and S. Focardi, Semivolatile Organic Compounds (SVOCs) in the atmosphere of Santiago de Cali, Valle del Cauca, Colombia along north-south transect using polyurethane foam disk as passive air samplers, Atmos. Pollut. Res., 2016, 7, 945–953 CrossRef.
- M. F. Ruggeri, N. B. Lana, J. C. Altamirano and S. E. Puliafito, Spatial distribution, patterns and source contributions of POPs in the atmosphere of Great Mendoza using the WRF/CALMET/CALPUFF modelling system, Emerging Contam., 2020, 6, 103–113 CrossRef.
- N. L. Devi, S. Qi, P. Chakraborty, G. Zhang and I. C. Yadav, Passive air sampling of organochlorine pesticides in a northeastern state of India, Manipur, J. Environ. Sci., 2011, 23, 808–815 CrossRef CAS.
- N. L. Devi, S. Qi, P. Chakraborty, G. Zhang and I. C. Yadav, Atmospheric polycyclic aromatic hydrocarbons (PAH) in Manipur of the Northeast India: Monitoring on urban, rural, and mountain sites, Polycyclic Aromat. Compd., 2014, 34, 12–34 CrossRef CAS.
- K. Pozo, S. K. Sarkar, V. H. Estellano, S. Mitra, O. Audy, P. Kukučka, P. Přibylová, J. Klánová and S. Corsolini, Passive air sampling of persistent organic pollutants (POPs) and emerging compounds in Kolkata megacity and rural mangrove wetland Sundarban in India: An approach to regional monitoring, Chemosphere, 2017, 168, 1430–1438 CrossRef CAS PubMed.
- B. Pokhrel, P. Gong, X. Wang, C. Wang and S. Gao, Polycyclic aromatic hydrocarbons in the urban atmosphere of Nepal: Distribution, sources, seasonal trends, and cancer risk, Sci. Total Environ., 2018, 618, 1583–1590 CrossRef CAS PubMed.
- B. Pokhrel, P. Gong, X. Wang, S. N. Khanal, J. Ren, C. Wang, S. Gao and T. Yao, Atmospheric organochlorine pesticides and polychlorinated biphenyls in urban areas of Nepal: Spatial variation, sources, temporal trends, and long-range transport potential, Atmos. Chem. Phys., 2018, 18, 1325–1336 CrossRef CAS.
- B. Li, S. Wu, S. Zhou, T. Wang and C. Wang, Spatiotemporal distribution and dynamic modeling of atmospheric gaseous polycyclic aromatic hydrocarbons in a rapidly urbanizing city: Nanjing, China, Environ. Geochem. Health, 2018, 40, 2603–2616 CrossRef CAS PubMed.
- L. Tong, C.-H. Peng, Z.-W. Huang, J.-J. Zhang, X.-R. Dai, H. Xiao, N.-B. Xu and J. He, Identifying the pollution characteristics of atmospheric polycyclic aromatic hydrocarbons associated with functional districts in Ningbo, China, Bull. Environ. Contam. Toxicol., 2019, 103, 34–40 CrossRef CAS PubMed.
- D. Wu, H. Liu, Z. Wang, J. Zhang, C. Zhan, S. Liu, T. Liu, J. Zheng, R. Yao and J. Cao, Atmospheric concentrations and air–soil exchange of polycyclic aromatic hydrocarbons (PAHs) in typical urban-rural fringe of Wuhan-Ezhou region, Central China, Bull. Environ. Contam. Toxicol., 2020, 104, 96–106 CrossRef CAS PubMed.
- Y. Wang, M. Bao, Y. Zhang, F. Tan, H. Zhao, Q. Zhang and Q. Li, Polycyclic aromatic hydrocarbons in the atmosphere and soils of Dalian, China: Source, urban-rural gradient, and air–soil exchange, Chemosphere, 2020, 244, 125518 CrossRef CAS PubMed.
- P. Q. Thang, S.-J. Kim, S.-J. Lee, C. H. Kim, H.-J. Lim, S.-B. Lee, J. Y. Kim, Q. T. Vuong and S.-D. Choi, Monitoring of polycyclic aromatic hydrocarbons using passive air samplers in Seoul, South Korea: Spatial distribution, seasonal variation, and source identification, Atmos. Environ., 2020, 229, 117460 CrossRef CAS.
- X. Jiang, L. Gao, G. Liu, C. Li and M. Zheng, A case study on the long-term influence of historical intensive usage of organochlorine pesticides on current ambient air, Aerosol Air Qual. Res., 2014, 14, 1047–1054 CrossRef CAS.
- B. Cetin, F. Ozturk, M. Keles and S. Yurdakul, PAHs and PCBs in an Eastern Mediterranean megacity, Istanbul: Their spatial and temporal distributions, air–soil exchange and toxicological effects, Environ. Pollut., 2017, 220, 1322–1332 CrossRef CAS PubMed.
- A. Birgül, P. B. Kurt-Karakuş, H. Alegria, E. Gungormus, H. Celik, T. Cicek and E. C. Güven, Polyurethane foam (PUF) disk passive samplers derived polychlorinated biphenyls (PCBs) concentrations in the ambient air of Bursa-Turkey: Spatial and temporal variations and health risk assessment, Chemosphere, 2017, 168, 1345–1355 CrossRef PubMed.
- W. Wang, S. Simonich, B. Giri, Y. Chang, Y. Zhang, Y. Jia, S. Tao, R. Wang, B. Wang, W. Li, J. Cao and X. Lu, Atmospheric concentrations and air–soil gas exchange of polycyclic aromatic hydrocarbons (PAHs) in remote, rural village and urban areas of Beijing–Tianjin region, North China, Sci. Total Environ., 2011, 409, 2942–2950 CrossRef CAS PubMed.
- C. Qu, X. Xing, S. Albanese, A. Doherty, H. Huang, A. Lima, S. Qi and B. De Vivo, Spatial and seasonal variations of atmospheric organochlorine pesticides along the plain-mountain transect in central China: Regional source vs. long-range transport and air–soil exchange, Atmos. Environ., 2015, 122, 31–40 CrossRef CAS.
- X. Mao, Z. Yu, Z. Ding, T. Huang, J. Ma, G. Zhang, J. Li and H. Gao, Sources and potential health risk of gas phase PAHs in Hexi Corridor, Northwest China, Environ. Sci. Pollut. Res., 2016, 23, 2603–2612 CrossRef CAS PubMed.
- V. H. Estellano, K. Pozo, T. Harner, S. Corsolini and S. Focardi, Using PUF disk passive samplers to simultaneously measure air concentrations of persistent organic pollutants (POPs) across the Tuscany Region, Italy, Atmos.
Pollut. Res., 2012, 3, 88–94 CrossRef CAS.
- V. H. Estellano, K. Pozo, C. Silibello, M. D. Mulder, C. Efstathiou, M. P. Tomasino, F. Funaro, I. Donadio and S. Focardi, Characterization of urban pollution in two cities of the Puglia region in Southern Italy using field measurements and air quality (AQ) model approach, Atmos. Pollut. Res., 2014, 5, 34–41 CrossRef.
- V. H. Estellano, K. Pozo, P. Přibylová, J. Klánová, O. Audy and S. Focardi, Assessment of seasonal variations in persistent organic pollutants across the region of Tuscany using passive air samplers, Environ. Pollut., 2017, 222, 609–616 CrossRef CAS PubMed.
- S.-Y. Baek, S.-D. Choi, S.-J. Lee and Y.-S. Chang, Assessment of the spatial distribution of coplanar PCBs, PCNs, and PBDEs in a multi-industry Region of South Korea using passive air samplers, Environ. Sci. Technol., 2008, 42, 7336–7340 CrossRef CAS PubMed.
- O. Roots, A. Roose, A. Kull, I. Holoubek, P. Čupr and J. Klánová, Distribution pattern of PCBs, HCB and PeCB using passive air and soil sampling in Estonia, Environ. Sci. Pollut. Res., 2010, 17, 740–749 CrossRef CAS PubMed.
- O. Roots, T. Lukki, P. Přibylová, J. Borůvková, P. Kukučka, O. Audy, J. Kalina, J. Klánová, I. Holoubek, A. Sweetman and O. Schleicher, Measurements of persistent organic pollutants in Estonian ambient air (1990–2013), Proc. Est. Acad. Sci., 2015, 64, 184–199 CrossRef.
- A. Adu-Kumi, R. Kares, J. Literak, J. Boruvkova, P. O. Yeboah, D. Carboo, O. Akoto, G. Darko, S. Osae and J. Klánová, Levels and seasonal variations of organochlorine pesticides in urban and rural background air of southern Ghana, Environ. Sci. Pollut. Res., 2012, 19, 1963–1970 CrossRef PubMed.
- J. Sultana, J. H. Syed, A. Mahmood, U. Ali, M. Y. A. Rehman, R. N. Malik, J. Li and G. Zhang, Investigation of organochlorine pesticides from the Indus Basin, Pakistan: Sources, air–soil exchange fluxes and risk assessment, Sci. Total Environ., 2014, 497/498, 113–122 CrossRef PubMed.
- M. F. Silva-Barni, M. Gonzalez, F. Wania, Y. D. Lei and K. S. B. Miglioranza, Spatial and temporal distribution of pesticides and PCBs in the atmosphere using XAD-resin based passive air sampler: The case study of Quequén Grande River watershed (Argentina), Atmos. Pollut. Res., 2018, 9, 238–245 CrossRef CAS.
- B. M. Sharma, L. Melymuk, G. K. Bharat, P. Přibylová, O. Sáňka, J. Klánová and L. Nizzetto, Spatial gradients of polycyclic aromatic hydrocarbons (PAHs) in air, atmospheric deposition, and surface water of the Ganges River basin, Sci. Total Environ., 2018, 627, 1495–1504 CrossRef CAS PubMed.
- S. N. Khuman and P. Chakraborty, Air-water exchange of pesticidal persistent organic pollutants in the lower stretch of the transboundary river Ganga, India, Chemosphere, 2019, 233, 966–974 CrossRef CAS PubMed.
- S. Niu, R. Chen, Y. Zou, L. Dong, R. Hai and Y. Huang, Spatial distribution and profile of atmospheric short-chain chlorinated paraffins in the Yangtze River Delta, Environ. Pollut., 2020, 259, 113958 CrossRef CAS PubMed.
- S. Sampath, G. Shanmugam, K. K. Selvaraj and B. R. Ramaswamy, Spatio-temporal distribution of polycyclic aromatic hydrocarbons (PAHs) in atmospheric air of Tamil Nadu, India, and human health risk assessment, Environ. Forensics, 2015, 16, 76–87 CrossRef CAS.
- S. Niu, L. Dong, L. Zhang, C. Zhu, R. Hai and Y. Huang, Temporal and spatial distribution, sources, and potential health risks of ambient polycyclic aromatic hydrocarbons in the Yangtze River Delta (YRD) of eastern China, Chemosphere, 2017, 172, 72–79 CrossRef CAS PubMed.
- Y. Dumanoglu, E. O. Gaga, E. Gungormus, S. C. Sofuoglu and M. Odabasi, Spatial and seasonal variations, sources, air–soil exchange, and carcinogenic risk assessment for PAHs and PCBs in air and soil of Kutahya, Turkey, the province of thermal power plants, Sci. Total Environ., 2017, 580, 920–935 CrossRef CAS PubMed.
- U. Ali, A. J. Sweetman, K. C. Jones and R. N. Malik, Higher atmospheric levels and contribution of black carbon in soil-air partitioning of organochlorines in Lesser Himalaya, Chemosphere, 2018, 191, 787–798 CrossRef CAS PubMed.
- K. Pozo, M. Palmeri, V. Palmeri, V. H. Estellano, M. D. Mulder, C. I. Efstathiou, G. Sará, T. Romeo, G. Lammel and S. Focardi, Assessing persistent organic pollutants (POPs) in the Sicily island atmosphere, Mediterranean, using PUF disk passive air samplers, Environ. Sci. Pollut. Res., 2016, 23, 20796–20804 CrossRef CAS PubMed.
- S. Loppi, K. Pozo, V. H. Estellano, S. Corsolini, G. Sardella and L. Paoli, Accumulation of polycyclic aromatic hydrocarbons by lichen transplants: Comparison with gas-phase passive air samplers, Chemosphere, 2015, 134, 39–43 CrossRef CAS PubMed.
- T. F. Bidleman, H. Laudon, O. Nygren, S. Svanberg and M. Tysklind, Chlorinated pesticides and natural brominated anisoles in air at three northern Baltic stations, Environ. Pollut., 2017, 225, 381–389 CrossRef CAS PubMed.
- C. Qu, S. Albanese, A. Lima, D. Hope, P. Pond, A. Fortelli, N. Romano, P. Cerino, A. Pizzolante and B. De Vivo, The occurrence of OCPs, PCBs, and PAHs in the soil, air, and bulk deposition of the Naples metropolitan area, southern Italy: Implications for sources and environmental processes, Environ. Int., 2019, 124, 84–97 CrossRef PubMed.
- I. Navarro, A. de la Torre, P. Sanz, M. A. Arjol, J. Fernández and M. A. Martínez, Organochlorine pesticides air monitoring near a historical lindane production site in Spain, Sci. Total Environ., 2019, 670, 1001–1007 CrossRef CAS PubMed.
- Y. Moussaoui, L. Tuduri, Y. Kerchich, B. Y. Meklati and G. Eppe, Atmospheric concentrations of PCDD/Fs, dl-PCBs and some pesticides in northern Algeria using passive air sampling, Chemosphere, 2012, 88, 270–277 CrossRef CAS PubMed.
- Z. J. Katima, O. I. Olukunle, O. L. Kalantzi, A. P. Daso and J. O. Okonkwo, The occurrence of brominated flame retardants in the atmosphere of Gauteng Province, South Africa using polyurethane foam passive air samplers and assessment of human exposure, Environ. Pollut., 2018, 242, 1894–1903 CrossRef CAS PubMed.
- H. A. Alegria, F. Wong, L. M. Jantunen, T. F. Bidleman, M. S. Figueroa, G. G. Bouchot, V. C. Moreno, S. M. Waliszewski and R. Infanzon, Organochlorine pesticides and PCBs in air of southern Mexico (2002-2004), Atmos. Environ., 2008, 42, 8810–8818 CrossRef CAS.
- N. Tombesi, K. Pozo and T. Harner, Persistent Organic Pollutants (POPs) in the atmosphere of agricultural and urban areas in the province of Buenos Aires in Argentina using PUF disk passive air samplers, Atmos. Pollut. Res., 2014, 5, 170–178 CrossRef CAS.
- C. N. Pegoraro and E. D. Wannaz, Occurrence of persistent organic pollutants in air at different sites in the province of Córdoba, Argentina, Environ. Sci. Pollut. Res., 2019, 26, 18379–18391 CrossRef CAS PubMed.
- M. M. Orazi, A. H. Arias, A. L. Oliva, A. C. Ronda and J. E. Marcovecchio, Characterization of atmospheric and soil polycyclic aromatic hydrocarbons and evaluation of air–soil relationship in the Southwest of Buenos Aires province (Argentina), Chemosphere, 2020, 240, 124847 CrossRef CAS PubMed.
- J. H. Syed, R. N. Malik, D. Liu, Y. Xu, Y. Wang, J. Li, G. Zhang and K. C. Jones, Organochlorine pesticides in air and soil and estimated air–soil exchange in Punjab, Pakistan, Sci. Total Environ., 2013, 444, 491–497 CrossRef CAS PubMed.
- U. Ali, J. H. Syed, A. Mahmood, J. Li, G. Zhang, K. C. Jones and R. N. Malik, Influential role of black carbon in the soil-air partitioning of polychlorinated biphenyls (PCBs) in the Indus River Basin, Pakistan, Chemosphere, 2015, 134, 172–180 CrossRef CAS PubMed.
- A. Kamal, J. H. Syed, J. Li, G. Zhang, A. Mahmood and R. N. Malik, Profile of atmospheric PAHs in Rawalpindi, Lahore and Gujranwala districts of Punjab province (Pakistan), Aerosol Air Qual. Res., 2016, 16, 1010–1021 CrossRef CAS.
- Y. Min, J. Heo and M. Lee, Determination of toxic congeners of 17 PCDDs/PCDFs and 12 dl-PCBs using polyurethane foam passive air samplers in ten cities around Seoul, Sci. Total Environ., 2014, 491/492, 17–27 CrossRef PubMed.
- T. N. T. Nguyen, H. O. Kwon, G. Lammel, K. S. Jung, S. J. Lee and S. D. Choi, Spatially high-resolved monitoring and risk assessment of polycyclic aromatic hydrocarbons in an industrial city, J. Hazard. Mater., 2020, 393, 122409 CrossRef CAS PubMed.
- E. Can-Güven, K. Gedik and P. B. Kurt-Karakuş, Polyurethane foam disk passive sampler derived air concentrations of persistent organic pollutants in an agricultural region with hot climate, Atmos. Pollut. Res., 2019, 10, 1913–1920 CrossRef.
- B. Cetin, S. Yurdakul, E. Gungormus, F. Ozturk and S. Sofuoglu, Source apportionment and carcinogenic risk assessment of passive air sampler-derived PAHs and PCBs in a heavily industrialized region, Sci. Total Environ., 2018, 633, 30–41 CrossRef CAS PubMed.
- L. Wang, Y. Zhao, X. Yi, Z. Wang, Y. Yi, T. Huang, H. Gao and J. Ma, Spatial distribution of atmospheric PAHs and their genotoxicity in petrochemical industrialized Lanzhou valley, northwest China, Environ. Sci. Pollut. Res., 2017, 24, 12820–12834 CrossRef CAS PubMed.
- P. Liu, Y. Ju, Y. Li, Z. Wang, X. Mao, H. Cao, C. Jia, T. Huang, H. Gao and J. Ma, Spatiotemporal variation of atmospheric nitrated polycyclic aromatic hydrocarbons in semi-arid and petrochemical industrialized Lanzhou City, Northwest China, Environ. Sci. Pollut. Res., 2019, 26, 1857–1870 CrossRef CAS PubMed.
- C. Schummer, L. Tuduri, O. Briand, B. M. Appenzeller and M. Millet, Application of XAD-2 resin-based passive samplers and SPME-GC-MS/MS analysis for the monitoring of spatial and temporal variations of atmospheric pesticides in Luxembourg, Environ. Pollut., 2012, 170, 88–94 CrossRef CAS PubMed.
- C. Schummer, B. M. Appenzeller and M. Millet, Monitoring of polycyclic aromatic hydrocarbons (PAHs) in the atmosphere of southern Luxembourg using XAD-2 resin-based passive samplers, Environ. Sci. Pollut. Res., 2014, 21, 2098–2107 CrossRef CAS PubMed.
- Z. Zhang, L. Liu, Y.-F. Li, D. Wang, H. Jia, T. Harner, E. Sverko, X. Wan, D. Xu, N. Ren, J. Ma and K. Pozo, Analysis of polychlorinated biphenyls in concurrently sampled Chinese air and surface soil, Environ. Sci. Technol., 2008, 42, 6514–6518 CrossRef CAS PubMed.
- Y. Zhao, J. Ma, X. Qiu, Y. Lin, Q. Yang and T. Zhu, Gridded field observations of polybrominated diphenyl ethers and decabromodiphenyl ethane in the atmosphere of North China, Environ. Sci. Technol., 2013, 47, 8123–8129 CAS.
- B. Gevao, A. Al-Omair, A. J. Sweetman, L. Al-Ali, M. Al-Bahloul, M. Helaleh and J. Zafar, Passive sampler-derived air concentrations for polybrominated diphenyl ethers and polycyclic aromatic hydrocarbons in Kuwait, Environ. Toxicol. Chem., 2006, 25, 1496–1502 CrossRef CAS PubMed.
- G. L. Daly, Y. D. Lei, C. Teixeira, D. C. G. Muir, L. E. Castillo, L. M. M. Jantunen and F. Wania, Organochlorine pesticides in the soils and atmosphere of Costa Rica, Environ. Sci. Technol., 2007, 41, 1124–1130 CrossRef CAS PubMed.
- G. L. Daly, Y. D. Lei, C. Teixeira, D. C. G. Muir, L. E. Castillo and F. Wania, Accumulation of current-use pesticides in neotropical montane forests, Environ. Sci. Technol., 2007, 41, 1118–1123 CrossRef CAS PubMed.
- J. N. Hogarh, N. Seike, Y. Kobara and S. Masunaga, Atmospheric polychlorinated naphthalenes in Ghana, Environ. Sci. Technol., 2012, 46, 2600–2606 CrossRef CAS PubMed.
- J. N. Hogarh, N. Seike, Y. Kobara, G. K. Ofosu-Budu, D. Carboo and S. Masunaga, Atmospheric burden of organochlorine pesticides in Ghana, Chemosphere, 2014, 102, 1–5 CrossRef CAS PubMed.
- G. Aliyeva, R. Kurkova, I. Hovorkova, J. Klánová and C. Halsall, Organochlorine pesticides and polychlorinated biphenyls in air and soil across Azerbaijan, Environ. Sci. Pollut. Res., 2012, 19, 1953–1962 CrossRef CAS PubMed.
- P. Chakraborty, G. Zhang, J. Li, Y. Xu, X. Liu, S. Tanabe and K. C. Jones, Selected organochlorine pesticides in the atmosphere of major Indian cities: Levels, regional versus local variations and sources, Environ. Sci. Technol., 2010, 44, 8038–8043 CrossRef CAS PubMed.
- J. E. Schrlau, L. Geiser, K. J. Hageman, D. H. Landers and S. M. Simonich, Comparison of lichen, conifer needles, passive air sampling devices, and snowpack as passive sampling media to measure semi-volatile organic compounds in remote atmospheres, Environ. Sci. Technol., 2011, 45, 10354–10361 CrossRef CAS PubMed.
- Y. Yao, T. Harner, J. Ma, L. Tuduri and P. Blanchard, Sources and occurrence of dacthal in the Canadian atmosphere, Environ. Sci. Technol., 2007, 41, 688–694 CrossRef CAS PubMed.
- P. G. Messing, A. Farenhorst, D. T. Waite and J. F. Sproull, Current-use herbicides in air as influenced by their estimated agricultural use at various distances from six sampling locations, Water, Air, Soil Pollut., 2014, 225, 2013–2022 CrossRef.
- N. Ren, E. Sverko, Y.-F. Li, Z. Zhang, T. Harner, D. Wang, X. Wan and B. E. McCarry, Levels and isomer profiles of dechlorane plus in Chinese air, Environ. Sci. Technol., 2008, 42, 6476–6480 CrossRef CAS PubMed.
- K. Pozo, T. Harner, M. Shoeib, R. Urrutia, R. Barra, O. Parra and S. Focardi, Passive-sampler derived air concentrations of persistent organic pollutants on a North-South transect in Chile, Environ. Sci. Technol., 2004, 38, 6529–6537 CrossRef CAS PubMed.
- C. Shunthirasingham, T. Gouin, Y. D. Lei, C. Ruepert, L. E. Castillo and F. Wania, Current-use pesticides transport to Costa Rica's high-altitude tropical cloud forest, Environ. Toxicol. Chem., 2011, 30, 2709–2717 CrossRef CAS PubMed.
- F. Wong, H. A. Alegria, T. F. Bidleman, V. Alvarado, F. Angeles, A. A. Galarza, B. Bandala, I. D. L. C. Hinojosa, I. G. Estrada, G. G. Ryes, G. Gold-Bouchot, J. V. M. Zamora and E. R. Esinoza, Passive air sampling of organochlorine pesticides in Mexico, Environ. Sci. Technol., 2009, 43, 704–710 CrossRef CAS PubMed.
- X. Liu, G. Zhang, J. Li, H.-R. Chang, S.-H. Qi, X.-D. Li and K. C. Jones, Polycyclic aromatic hydrocarbons (PAHs) in the air of Chinese cities, J. Environ. Monit., 2007, 9, 1092–1098 RSC.
- X. Liu, G. Zhang, J. Li, L.-L. Yu, Y. Xu, X.-D. Li, Y. Kobara and K. C. Jones, Seasonal patterns and current sources of DDTs, chlordanes, hexachlorobenzene, and endosulfan in the atmosphere of 37 Chinese cities, Environ. Sci. Technol., 2009, 43, 1316–1321 CrossRef CAS PubMed.
- Y. Lin, Y. Zhao, X. Qiu, J. Ma, Q. Yang, M. Shao and T. Zhu, Spatial distribution of polychlorinated naphthalenes
in the atmosphere across North China based on gridded field observations, Environ. Pollut., 2013, 180, 27–33 CrossRef CAS PubMed.
- Y. Lin, X. Qiu, Y. Zhao, J. Ma, Q. Yang and T. Zhu, Polybromobenzene pollutants in the atmosphere of North China: Levels, distribution, and sources, Environ. Sci. Technol., 2013, 47, 12761–12767 CrossRef CAS PubMed.
- Y. Lin, X. Qiu, Y. Ma, J. Ma, M. Zheng and M. Shao, Concentrations and spatial distribution of polycyclic aromatic hydrocarbons (PAHs) and nitrated PAHs (NPAHs) in the atmosphere of North China, and the transformation from PAHs to NPAHs, Environ. Pollut., 2015, 196, 164–170 CrossRef CAS PubMed.
- G. Zhang, P. Chakraborty, J. Li, P. Sampathkumar, T. Balasubramanian, K. Kathiresan, S. Takahashi, A. Subramanian, S. Tanabe and K. C. Jones, Passive atmospheric sampling of organochlorine pesticides, polychlorinated biphenyls, and polybrominated diphenyl ethers in urban, rural, and wetland sites along the coastal length of India, Environ. Sci. Technol., 2008, 42, 8218–8223 CrossRef CAS PubMed.
- C. Chaemfa, Y. Xu, J. Li, P. Chakraborty, J. H. Syed, R. N. Malik, Y. Wang, C. Tian, G. Zhang and K. C. Jones, Screening of atmospheric short- and medium-chain chlorinated paraffins in India and Pakistan using polyurethane foam based passive air sampler, Environ. Sci. Technol., 2014, 48, 4799–4808 CrossRef CAS PubMed.
- Y. Xu, J. Li, P. Chakraborty, J. H. Syed, R. N. Malik, Y. Wang, C. Tian, C. Luo, G. Zhang and K. C. Jones, Atmospheric polychlorinated naphthalenes (PCNs) in India and Pakistan, Sci. Total Environ., 2014, 466/467, 1030–1036 CrossRef PubMed.
- P. Chakraborty, G. Zhang, H. Cheng, P. Balasubramanian, J. Li and K. C. Jones, Passive air sampling of polybrominated diphenyl ethers in New Delhi, Kolkata, Mumbai and Chennai: Levels, homologous profiling and source apportionment, Environ. Pollut., 2017, 231, 1181–1187 CrossRef CAS PubMed.
- K. Pozo, T. Harner, S. C. Lee, R. K. Sinha, B. Sengupta, M. Loewen, V. Geethalakshmi, K. Kannan and V. Volp, Assessing seasonal and spatial trends of persistent organic pollutants (POPs) in Indian agricultural regions using PUF disk passive air samplers, Environ. Pollut., 2011, 159, 646–653 CrossRef CAS PubMed.
- K. Kennedy, M. Macova, M. E. Bartkow, D. W. Hawker, B. Zhao, M. S. Dension and J. F. Müller, Effect based monitoring of seasonal ambient air exposures in Australia sampled by PUF passive air samplers, Atmos. Pollut. Res., 2010, 1, 50–58 CrossRef CAS PubMed.
- X. Wang, K. Kennedy, J. Powell, M. Keywood, R. Gillett, P. Thai, P. Bridgen, S. Broomhall, C. Paxman, F. Wania and J. Müller, Spatial distribution of selected persistent organic pollutants (POPs) in Australia's atmosphere, Environ. Sci.: Processes Impacts, 2015, 17, 525–532 RSC.
- J. N. Hogarh, N. Seike, Y. Kobara and S. Masunaga, Seasonal variation of atmospheric polychlorinated biphenyls and polychlorinated naphthalenes in Japan, Atmos. Environ., 2013, 80, 275–280 CrossRef CAS.
- J. N. Hogarh, N. Seike, Y. Kobara, D. Carboo, J. N. Fobil and S. Masunaga, Source characterization and risk of exposure to atmospheric polychlorinated biphenyls (PCBs) in Ghana, Environ. Sci. Pollut. Res., 2018, 25, 16316–16324 CrossRef CAS PubMed.
- W. Wang, Y. Wang, R. Zhang, S. Wang, C. Wei, C. Chaemfa, J. Li, G. Zhang and K. Yu, Seasonal characteristics and current sources of OCPs and PCBs and enantiomeric signatures of chiral OCPs in the atmosphere of Vietnam, Sci. Total Environ., 2016, 542, 777–786 CrossRef CAS PubMed.
- I. C. Yadav, N. L. Devi, J. Li, G. Zhang and K. Breivik, Possible emissions of POPs in plain and hilly areas of Nepal: Implications for source apportionment and health risk assessment, Environ. Pollut., 2017, 220, 1289–1300 CrossRef CAS PubMed.
- P. B. Kurt-Karakuş, T. Ugranli-Cicek, S. C. Sofuoglu, H. Celik, E. Gungormus, K. Gedik, A. Sofuoglu, H. E. Okten, A. Birgul, H. Alegria and K. C. Jones, The first countrywide monitoring of selected POPs: Polychlorinated biphenyls (PCBs), polybrominated diphenyl ethers (PBDEs) and selected organochlorine pesticides (OCPs) in the atmosphere of Turkey, Atmos. Environ., 2018, 177, 154–165 CrossRef.
- S. Zhao, K. C. Jones, J. Li, A. J. Sweetman, X. Liu, Y. Xu, Y. Wang, T. Lin, S. Mao, K. Li, J. Tang and G. Zhang, Evidence for major contributions of unintentionally produced PCBs in the air of China: Implications for the national source inventory, Environ. Sci. Technol., 2020, 54, 2163–2171 CrossRef CAS PubMed.
- R. Gioia, S. Eckhardt, K. Breivik, F. M. Jaward, A. Prieto, L. Nizzetto and K. C. Jones, Evidence for major emissions of PCBs in the west African region, Environ. Sci. Technol., 2011, 45, 1349–1355 CrossRef CAS PubMed.
- N. Isogai, J. N. Hogarh, N. Seike, Y. Kobara, F. Oyediran, M. J. Wirmvem, S. N. Ayonghe, J. Fobil and S. Masunaga, Atmospheric monitoring of organochlorine pesticides across some West African countries, Environ. Sci. Pollut. Res., 2018, 25, 31828–31835 CrossRef CAS PubMed.
- S.-Y. Baek, J. Jurng and Y.-S. Chang, Spatial distribution of polychlorinated biphenyls, organochlorine pesticides, and dechlorane plus in Northeast Asia, Atmos. Environ., 2013, 64, 40–46 CrossRef CAS.
- Q. Li, J. Li, C. Chaemfa, G. Zhang, Y. Kobara, J.-J. Nam and K. C. Jones, The impact of polybrominated diphenyl ether prohibition: A case study on the atmospheric levels in China, Japan and South Korea, Atmos. Res., 2014, 143, 57–63 CrossRef CAS.
- T. Gouin, T. Harner, P. Blanchard and D. Mackay, Passive and active air samplers as complementary methods for investigating persistent organic pollutants in the Great Lakes Basin, Environ. Sci. Technol., 2005, 39, 9115–9122 CrossRef CAS PubMed.
- T. Harner, M. Shoeib, T. Gouin and P. Blanchard, Polychlorinated naphthalenes in Great Lakes air: Assessing spatial trends and combustion inputs using PUF disk passive air samplers, Environ. Sci. Technol., 2006, 40, 5333–5339 CrossRef CAS PubMed.
- T. Gouin, L. Jantunen, T. Harner, P. Blanchard and T. F. Bidleman, Spatial and temporal trends of chiral organochlorine signatures in Great Lakes air using passive air samplers, Environ. Sci. Technol., 2007, 41, 3877–3883 CrossRef CAS PubMed.
- R. A. Gioia, A. J. Sweetman and K. C. Jones, Coupling passive air sampling with emission estimates and chemical fate modeling for persistent organic pollutants (POPs): A feasibility study for Northern Europe, Environ. Sci. Technol., 2007, 41, 2165–2171 CrossRef CAS PubMed.
- P. Bohlin, K. C. Jones, H. Tovalin and B. Strandberg, Observations on persistent organic pollutants in indoor and outdoor air using passive polyurethane foam samples, Atmos. Environ., 2008, 42, 7234–7241 CrossRef CAS.
- P. Přibylová, R. Kares, J. Borůvková, P. Čupr, R. Prokeš, J. Kohoutek, I. Holoubek and J. Klánová, Levels of persistent organic pollutants and polycyclic aromatic hydrocarbons in ambient air of Central and Eastern Europe, Atmos. Pollut. Res., 2012, 3, 494–505 CrossRef.
- C. S. Kwan, H. Takada, R. Boonyatumanond, Y. Kato, K. Mizukawa, M. Ito, L. Q. Dung, M. P. Zakaria and E. C. Santiago, Historical
occurrences of polybrominated diphenyl ethers and polychlorinated biphenyls in Manila Bay, Philippines, and in the upper Gulf of Thailand, Sci. Total Environ., 2014, 470/471, 427–437 CrossRef PubMed.
- L. Shen, F. Wania, Y. D. Lei, C. Teixeira, D. C. G. Muir and T. F. Bidleman, Hexachlorocyclohexanes in the North American atmosphere, Environ. Sci. Technol., 2004, 38, 965–975 CrossRef CAS PubMed.
- L. Shen, F. Wania, Y. D. Lei, C. Teixeira, D. C. G. Muir and H. Xiao, Polychlorinated biphenyls and polybrominated diphenyl ethers in the North American atmosphere, Environ. Pollut., 2006, 144, 434–444 CrossRef CAS PubMed.
- F. M. Jaward, N. J. Farrar, T. Harner, A. J. Sweetman and K. C. Jones, Passive air sampling of PCBs, PBDEs, and organochlorine pesticides across Europe, Environ. Sci. Technol., 2004, 38, 34–41 CrossRef CAS PubMed.
- F. M. Jaward, N. J. Farrar, T. Harner, A. J. Sweetman and K. C. Jones, Passive air sampling of polycyclic aromatic hydrocarbons and polychlorinated naphthalenes across Europe, Environ. Toxicol. Chem., 2004, 23, 1355–1364 CrossRef CAS PubMed.
- A. K. Halse, M. Schlabach, S. Eckhardt, A. J. Sweetman, K. C. Jones and K. Breivik, Spatial variability of POPs in European background air, Atmos. Chem. Phys., 2011, 11, 1549–1564 CrossRef CAS.
- F. M. Jaward, G. Zhang, J. J. Nam, A. J. Sweetman, J. P. Obbard, Y. Kobara and K. C. Jones, Passive air Sampling of polychlorinated biphenyls, organochlorine compounds, and polybrominated diphenyl ethers across Asia, Environ. Sci. Technol., 2005, 39, 8638–8645 CrossRef CAS PubMed.
- J. N. Hogarh, N. Seike, Y. Kobara, A. Habib, J.-J. Nama, J.-S. Lee, Q. Li, X. Liu, J. Li, G. Zhang and S. Masunaga, Passive air monitoring of PCBs and PCNs across East Asia: A comprehensive congener evaluation for source characterization, Chemosphere, 2012, 86, 718–726 CrossRef CAS PubMed.
- W.-J. Hong, H. Jia, W.-L. Ma, R. K. Sinha, H.-B. Moon, H. Nakata, N. H. Minh, K. H. Chi, W.-L. Li, K. Kannan, E. Sverko and Y.-F. Li, Distribution, fate, inhalation exposure and lung cancer risk of atmospheric polycyclic aromatic hydrocarbons in some Asian countries, Environ. Sci. Technol., 2016, 50, 7163–7174 CrossRef CAS PubMed.
- J. Klánová, P. Čupr, I. Holoubek, J. Borůvková, P. Přibylová, R. Kares, T. Tomsej and T. Ocelka, Monitoring of persistent organic pollutants in Africa. Part 1: Passive air sampling across the continent in 2008, J. Environ. Monit., 2009, 11, 1952–1963 RSC.
- S. Fuhrimann, J. Klánová, P. Přibylová, J. Kohoutek, M. A. Dalvie, M. Röösli and C. Degrendele, Qualitative assessment of 27 current-use pesticides in air at 20 sampling sites across Africa, Chemosphere, 2020, 258, 127333 CrossRef CAS.
- C. Rauert, T. Harner, J. K. Schuster, K. Quinto, G. Fillmann, L. E. Castillo, O. Fentanes, M. V. Ibarra, K. S. B. Miglioranza, I. M. Rivadeneira, K. Pozo, A. P. Puerta and B. H. A. Zuluaga, Towards a regional passive air sampling network and strategy for new POPs in the GRULAC region: Perspectives from the GAPS Network and first results for organophosphorus flame retardants, Sci. Total Environ., 2016, 573, 1294–1302 CrossRef CAS PubMed.
- C. Rauert, T. Harner, J. K. Schuster, A. Eng, G. Fillmann, L. E. Castillo, O. Fentanes, M. Villa Ibarra, K. S. B. Miglioranza, I. Moreno Rivadeneira, K. Pozo and B. H. Aristizábal Zuluaga, Air monitoring of new and legacy
POPs in the Group of Latin America and Caribbean (GRULAC) region, Environ. Pollut., 2018, 243, 1252–1262 CrossRef CAS PubMed.
- T. Harner, K. Pozo, T. Gouin, A.-M. Macdonald, H. Hung, J. Cainey and A. Peters, Global pilot study for persistent organic pollutants (POPs) using PUF disk passive air samplers, Environ. Pollut., 2006, 144, 445–452 CrossRef CAS PubMed.
- S. C. Lee, T. Harner, K. Pozo, M. Shoeib, F. Wania, D. C. G. Muir, L. A. Barrie and K. C. Jones, Polychlorinated naphthalenes in the global atmospheric passive sampling (GAPS) study, Environ. Sci. Technol., 2007, 41, 2680–2687 CrossRef CAS PubMed.
- S. C. Lee, E. Sverko, T. Harner, K. Pozo, E. Barresi, J. A. Schachtschneider, D. Zaruk, M. DeJong and J. Narayan, Retrospective analysis of “new” flame retardants in the global atmosphere under the GAPS Network, Environ. Pollut., 2016, 217, 62–69 CrossRef CAS PubMed.
- C. Rauert, J. K. Schuster, A. Eng and T. Harner, Global atmospheric concentrations of brominated and chlorinated flame retardants and organophosphate esters, Environ. Sci. Technol., 2018, 52, 2777–2789 CrossRef CAS PubMed.
- C. Shunthirasingham, C. E. Oyiliagu, X. Cao, T. Gouin, F. Wania, S. C. Lee, K. Pozo, T. Harner and D. C. G. Muir, Spatial and temporal pattern of pesticides in the global atmosphere, J. Environ. Monit., 2010, 12, 1650–1657 RSC.
- J. N. Westgate, C. Shunthirasingham, C. E. Oyiliagu, H. von Waldow and F. Wania, Three methods for quantifying proximity of air sampling sites to spatially resolved emissions of semivolatile organic contaminants, Atmos. Environ., 2010, 44, 4380–4387 CrossRef CAS.
- C. Bogdal, E. Abad, M. Abalos, B. van Bavel, J. Hagberg, M. Scheringer and H. Fiedler, Worldwide distribution of persistent organic pollutants in air, including results of air monitoring by passive air sampling in five continents, Trends Anal. Chem., 2013, 46, 151–161 CrossRef.
- P. Barthel, S. Thüns, C. Shunthirasingham, J. N. Westgate, F. Wania and M. Radke, Application of XAD-resin based passive air samplers to assess local (roadside) and regional patterns of persistent organic pollutants, Environ. Pollut., 2012, 166, 218–225 CrossRef CAS PubMed.
- S.-D. Choi, C. Shunthirasingham, G. L. Daly, H. Xiao, Y. D. Lei and F. Wania, Levels of polycyclic aromatic hydrocarbons in Canadian mountain air and soil are controlled by proximity to roads, Environ. Pollut., 2009, 157, 3199–3206 CrossRef CAS PubMed.
- T. Harner, M. Shoeib, M. Diamond, G. Stern and B. Rosenberg, Using passive air samplers to assess urban-rural trends for persistent organic pollutants. 1. polychlorinated biphenyls and organochlorine pesticides, Environ. Sci. Technol., 2004, 38, 4474–4483 CrossRef CAS PubMed.
- A. Motelay-Massei, T. Harner, M. Shoeib, M. L. Diamond, G. Stern and B. Rosenberg, Using passive air samplers to assess urban-rural trends for persistent organic pollutants and polycyclic aromatic hydrocarbons. 2. Seasonal trends for PAHs, PCBs, and organochlorine pesticides, Environ. Sci. Technol., 2005, 39, 5763–5773 CrossRef CAS PubMed.
- T. Gouin, T. Harner, G. L. Daly, F. Wania, D. Mackay and K. C. Jones, Variability of concentrations of polybrominated diphenyl ethers and polychlorinated biphenyls in air: Implications for monitoring, modeling and control, Atmos. Environ., 2005, 39, 151–166 CrossRef CAS.
- T. Harner, M. Shoeib, M. L. Diamond, M. Ikonomou and G. Stern, Passive sampler derived air concentrations of PBDEs along an urban–rural transect: Spatial and temporal trends, Chemosphere, 2006, 64, 262–267 CrossRef CAS PubMed.
- T. Gouin, M. Shoeib and T. Harner, Atmospheric concentrations of current-use pesticides across south-central Ontario using monthly-resolved passive air samplers, Atmos. Environ., 2008, 42, 8096–8104 CrossRef CAS.
- L. Melymuk, M. Robson, P. A. Helm and M. L. Diamond, PCBs, PBDEs, and PAHs in Toronto air: Spatial and seasonal trends and implications for contaminant transport, Sci. Total Environ., 2012, 429, 272–280 CrossRef CAS PubMed.
- S. Harrad and S. Hunter, Concentrations of polybrominated diphenyl ethers in air and soil on a rural-urban transect across a major UK conurbation, Environ. Sci. Technol., 2006, 40, 4548–4553 CrossRef CAS PubMed.
- A. Jamshidi, S. Hunter, S. Hazrati and S. Harrad, Concentrations and chiral signatures of polychlorinated biphenyls in outdoor and indoor air and soil in a major U.K. conurbation, Environ. Sci. Technol., 2007, 41, 2153–2158 CrossRef CAS PubMed.
- H. von Waldow, M. MacLeod, M. Scheringer and K. Hungerbühler, Quantifying remoteness from emission sources of persistent organic pollutants on a global scale, Environ. Sci. Technol., 2010, 44, 2791–2796 CrossRef CAS PubMed.
- A. Paschke, B. Vrana, P. Popp and G. Schüürmann, Comparative application of solid-phase microextraction fibre assemblies and semi-permeable membrane devices as passive air samplers for semi-volatile chlorinated organic compounds. A case study on the landfill “Grube Antonie”’ in Bitterfeld, Germany, Environ. Pollut., 2006, 144, 414–422 CrossRef CAS PubMed.
- Y. Wang, S. Wang, C. Luo, J. Li, L. Ming, G. Zhang and X. Li, The effects of rice canopy on the air–soil exchange of polycyclic aromatic hydrocarbons and organochlorine pesticides using paired passive air samplers, Environ. Pollut., 2015, 200, 35–41 CrossRef CAS PubMed.
- D. J. Bolinius, A. Jahnke and M. MacLeod, Comparison of eddy covariance and modified Bowen ratio methods for measuring gas fluxes and implications for measuring fluxes of persistent organic pollutants, Atmos. Chem. Phys., 2016, 16, 5315–5322 CrossRef CAS.
- E. Moreau-Guigon, A. Motelay-Massei, T. Harner, K. Pozo, M. L. Diamond, M. C. Chevreuil and H. Blanchoud, Vertical and temporal distribution of persistent organic Pollutants in Toronto. 1. Organochlorine pesticides, Environ. Sci. Technol., 2007, 41, 2172–2177 CrossRef CAS PubMed.
- Y. Li, Q. Zhang, D. Ji, T. Wang, Y. Wang, P. Wang, L. Ding and G. Jiang, Levels and vertical distributions of PCBs, PBDEs, and OCPs in the atmospheric boundary layer: Observation from the Beijing 325-m meteorological tower, Environ. Sci. Technol., 2009, 43, 1030–1035 CrossRef CAS PubMed.
- B. L. van Drooge, J. O. Grimalt, K. Booij, L. Camarero and J. Catalan, Passive sampling of atmospheric organochlorine compounds by SPMDs in a remote high mountain area, Atmos. Environ., 2005, 39, 5195–5204 CrossRef CAS.
- F. M. Jaward, A. Di Guardo, L. Nizzetto, C. Cassani, F. Raffaele, R. Ferretti and K. C. Jones, PCBs and selected organochlorine compounds in Italian mountain air: the influence of altitude and forest ecosystem type, Environ. Sci. Technol., 2005, 39, 3455–3463 CrossRef CAS PubMed.
- G. L. Daly, Y. D. Lei, C. Teixeira, D. C. G. Muir and F. Wania, Pesticides in Western Canadian mountain air and soil, Environ. Sci. Technol., 2007, 41, 6020–6025 CrossRef CAS PubMed.
- M. Loewen, F. Wania, F. Wang and G. Tomy, Altitudinal transect of atmospheric and aqueous fluorinated organic compounds in Western Canada, Environ. Sci. Technol., 2008, 42, 2374–2379 CrossRef CAS PubMed.
- B. Abdul Hussain, J. N. Westgate, S. J. Hayward, C. Shunthirasingham, T. N. Brown, H. Hung, Y. D. Lei and F. Wania, Polycyclic aromatic hydrocarbons and polychlorinated biphenyls in soils and atmosphere of Western Canadian mountains: The role of source proximity, precipitation, forest cover and mountain cold-trapping, Atmos. Environ. X, 2019, 100004 Search PubMed.
- V. H. Estellano, K. Pozo, T. Harner, M. Franken and M. Zaballa, Altitudinal and seasonal variations of persistent organic pollutants in the Bolivian Andes mountains, Environ. Sci. Technol., 2008, 42, 2528–2534 CrossRef CAS PubMed.
- R. O. Meire, S. C. Lee, A. C. Targino, J. P. M. Torres and T. Harner, Air concentrations and transport of persistent organic pollutants (POPs) in mountains of southeast and southern Brazil, Atmos. Pollut. Res., 2012, 3, 417–425 CrossRef CAS.
- R. O. Meire, S. C. Lee, Y. Yao, A. C. Targino, J. P. M. Torres and T. Harner, Seasonal and altitudinal variations of legacy and current-use pesticides in the Brazilian tropical and subtropical mountains, Atmos. Environ., 2012, 59, 108–116 CrossRef CAS.
- Y. D. S. Guida, R. O. Meire, T. P. M. Torres and O. Malm, Air contamination by legacy and current-use pesticides in Brazilian mountains: An overview of national regulations by monitoring pollutant presence in pristine areas, Environ. Pollut., 2018, 242, 19–30 CrossRef CAS PubMed.
- P. Gong, X.-P. Wang, S.-H. Li, W.-S. Yu, J.-I. Li, D. B. Kattel, W.-C. Wang, L. P. Devkota, T.-D. Yao and D. R. Joswiak, Atmospheric transport and accumulation of organochlorine compounds on the southern slopes of the Himalayas, Nepal, Environ. Pollut., 2014, 192, 44–51 CrossRef CAS PubMed.
- B. Pokhrel, P. Gong, X. Wang, S. Gao, C. Wang and T. Yao, Sources and environmental processes of polycyclic aromatic hydrocarbons and mercury along a southern slope of the central Himalayas, Nepal, Environ. Sci. Pollut. Res., 2016, 23, 13843–13852 CrossRef CAS PubMed.
- J. Ren, X. Wang, Y. Xue, P. Gong, D. R. Joswiak, B. Xu and T. Yao, Persistent organic pollutants in mountain air of the southeastern Tibetan Plateau: Seasonal variations and implications for regional cycling, Environ. Pollut., 2014, 194, 210–216 CrossRef CAS PubMed.
- P. Gong, X.-P. Wang, B. Pokhrel, H. Wang, X. Liu, X. Liu and F. Wania, Trans-Himalayan transport of organochlorine compounds: three-years of observations and model-based flux estimation, Environ. Sci. Technol., 2019, 53, 6773–6783 CrossRef CAS PubMed.
- J. Wu, W. Gao, Y. Liang, J. Fu, Y. Gao, Y. Wang and G. Jiang, Spatiotemporal distribution and alpine behavior of short chain chlorinated paraffins in air at Shergyla Mountain and Lhasa on the Tibetan Plateau of China, Environ. Sci. Technol., 2017, 51, 11136–11144 CrossRef CAS PubMed.
- J. Wu, D. Cao, W. Gao, K. Lv, Y. Liang, J. Fu, Y. Gao, Y. Wang and G. Jiang, The atmospheric transport and pattern of medium chain chlorinated paraffins at Shergyla Mountain on the Tibetan Plateau of China, Environ. Pollut., 2019, 245, 46–52 CrossRef CAS PubMed.
- X. Zhang, J. Barnes, Y. D. Lei and F. Wania, Semivolatile organic contaminants in the Hawaiian atmosphere, Environ. Sci. Technol., 2017, 51, 11634–11642 CrossRef CAS PubMed.
- C. Shunthirasingham, F. Wania, M. MacLeod, Y. D. Lei, C. L. Quinn, X. Zhang, M. Scheringer, F. Wegmann, K. Hungerbühler, S. Ivemeyer, F. Heil, P. Klocke, G. Pacepavicius and M. Alaee, Mountain cold-trapping increases transfer of persistent organic pollutants from atmosphere to cow's milk, Environ. Sci. Technol., 2013, 47, 9175–9181 CrossRef CAS PubMed.
- R. O. Meire, M. Khairy, D. Aldeman, P. M. A. Galvão, J. P. M. Torres, O. Malm and R. Lohmann, Passive sampler-derived concentrations of PAHs in air and water along Brazilian mountain transects, Atmos. Pollut. Res., 2019, 10, 635–641 CrossRef CAS.
- L. Li, J. A. Arnot and F. Wania, Revisiting the contributions of far- and near-field routes to human aggregate exposure to polychlorinated biphenyls (PCBs), Environ. Sci. Technol., 2018, 52, 6974–6984 CrossRef CAS PubMed.
- R. E. Dodson, D. E. Camann, R. Morello-Frosch, J. G. Brody and R. A. Rudel, Semivolatile organic compounds in homes: Strategies for efficient and systematic exposure measurement based on empirical and theoretical factors, Environ. Sci. Technol., 2015, 49, 113–122 CrossRef CAS PubMed.
- P. Bohlin, K. C. Jones and B. Strandberg, Occupational and indoor air exposure to persistent organic pollutants: a review of passive sampling techniques and needs, J. Environ. Monit., 2007, 9, 501–509 RSC.
- R. W. Gale, W. L. Cranor, D. Á. Álvarez, J. A. Huckins, J. D. Petty and G. L. Robertson, Semivolatile organic compounds in residential air along the Arizona-Mexico border, Environ. Sci. Technol., 2009, 43, 3054–3060 CrossRef CAS PubMed.
- S. Harrad, S. Hazrati and C. Ibarra, Concentrations of polychlorinated biphenyls in indoor air and polybrominated diphenyl ethers in indoor air and dust in Birmingham, United Kingdom:
Implications for human exposure, Environ. Sci. Technol., 2006, 40(15), 4633–4638 CrossRef CAS PubMed.
- K. P. Messier, L. G. Tidwell, C. C. Ghetu, D. Rohlman, R. P. Scott, L. M. Bramer, H. M. Dixon, K. M. Waters and K. A. Anderson, Indoor versus outdoor air quality during wildfires, Environ. Sci. Technol. Lett., 2019, 6, 696–701 CrossRef CAS PubMed.
- M. D. Ampleman, A. Martinez, J. DeWall, D. F. K. Rawn, K. C. Hornbuckle and P. S. Thorne, Inhalation and dietary exposure to PCBs in urban and rural cohorts via congener-specific measurements, Environ. Sci. Technol., 2015, 49, 1156–1164 CrossRef CAS PubMed.
- R. F. Marek, P. S. Thorne, N. J. Herkert, A. M. Awad and K. C. Hornbuckle, Airborne PCBs and OH-PCBs inside and outside urban and rural U.S. schools, Environ. Sci. Technol., 2017, 51, 7853–7860 CrossRef CAS PubMed.
- A.-L. Rantalainen, T. Hyötyläinen, M. Saramo and I. Niskanen, Passive sampling of PAHs in indoor air in Nepal, Toxicol. Environ. Chem., 1999, 68, 335–348 CrossRef CAS.
- M. Khairy and R. Lohmann, Organophosphate flame retardants in the indoor and outdoor dust and gas-phase of Alexandria, Egypt, Chemosphere, 2019, 220, 275–285 CrossRef CAS PubMed.
- B. Sha, A.-K. Dahlberg, K. Wiberg and L. Ahrens, Fluorotelomer alcohols (FTOHs), brominated flame retardants (BFRs), organophosphorus flame retardants (OPFRs) and cyclic volatile methylsiloxanes (cVMSs) in indoor air from occupational and home environments, Environ. Pollut., 2018, 241, 319–330 CrossRef CAS PubMed.
- Y. Wan, M. L. Diamond and J. A. Siegel, Elevated concentrations of semivolatile organic compounds in social housing multiunit residential building apartments, Environ. Sci. Technol. Lett., 2020, 7, 191–197 CrossRef CAS.
- L. Melymuk, P. Bohlin-Nizzetto, Š. Vojta, M. Krátká, P. Kukučka, O. Audy, P. Přibylová and J. Klánová, Distribution of legacy and emerging semivolatile organic compounds in five indoor matrices in a residential environment, Chemosphere, 2016, 153, 179–186 CrossRef CAS PubMed.
- X. Zhang, M. L. Diamond, M. Robson and S. Harrad, Sources, emissions, and fate of polybrominated diphenyl ethers and polychlorinated biphenyls indoors in Toronto, Canada, Environ. Sci. Technol., 2011, 45, 3268–3274 CrossRef CAS PubMed.
- H. H. Abd Hamid, L. W. Wan, I. P. Ren Kai, M. T. Latif and N. Kannan, Distribution of gas phase polycyclic aromatic hydrocarbons (PAHs) in selected indoor and outdoor air samples of Malaysia: A case study in Serdang, Selangor and Bachang, Malacca, EnvironmentAsia, 2017, 10, 80–85 Search PubMed.
- M. Venier, O. Audy, Š. Vojta, J. Bečanová, K. Romanak, L. Melymuk, M. Krátká, P. Kukučka, J. Okeme, A. Saini, M. L. Diamond and J. Klánová, Brominated flame retardants in the indoor environment - Comparative study of indoor contamination from three countries, Environ. Int., 2016, 94, 150–160 CrossRef CAS PubMed.
- M. Vykoukalová, M. Venier, Š. Vojta, L. Melymuk, J. Bečanová, K. Romanak, R. Prokeš, J. O. Okeme, A. Saini, M. L. Diamond and J. Klánová, Organophosphate esters flame retardants in the indoor environment, Environ. Int., 2017, 106, 97–104 CrossRef PubMed.
- O. Audy, L. Melymuk, M. Venier, S. Vojta, J. Bečanová, K. Romanak, M. Vykoukalová, R. Prokeš, P. Kukučka, M. L. Diamond and J. Klánová, PCBs and organochlorine pesticides in indoor environments - A comparison of indoor contamination in Canada and Czech Republic, Chemosphere, 2018, 206, 622–631 CrossRef CAS PubMed.
- W. Han, T. Fan, B. Xu, J. Feng, G. Zhang, M. Wu, Y. Yu and J. Fu, Passive sampling of polybrominated diphenyl ethers in indoor and outdoor air in Shanghai, China: seasonal variations, sources, and inhalation exposure, Environ. Sci. Pollut. Res., 2016, 23, 5771–5781 CrossRef CAS PubMed.
- A. Boudehane, A. Lounas, Y. Moussaoui, C. Balducci and A. Cecinato, Levels of organic compounds in interiors (school, home, university and hospital) of Ouargla city, Algeria, Atmos. Environ., 2016, 144, 266–273 CrossRef CAS.
- I. C. Yadav, N. L. Devi, J. Li and G. Zhang, Occurrence and source apportionment of halogenated flame retardants in the indoor air of Nepalese cities: Implication on human health, Atmos. Environ., 2017, 161, 122–131 CrossRef CAS.
- J. Sun, Q. Wang, S. Zhuang and A. Zhang, Occurrence of polybrominated diphenyl ethers in indoor air and dust in Hangzhou, China: Level, role of electric appliances, and human exposure, Environ. Pollut., 2016, 218, 942–949 CrossRef CAS PubMed.
- S. Newton, U. Sellström, S. Harrad, G. Yu and C. A. de Wit, Comparisons of indoor active and passive air sampling methods for emerging and legacy halogenated flame retardants in Beijing, China offices, Emerging Contam., 2016, 2, 80–88 CrossRef.
- K. Winkens, J. Koponen, J. Schuster, M. Shoeib, R. Vestergren, U. Berger, A. M. Karvonen, J. Pekkanen, H. Kiviranta and I. T. Cousins, Perfluoroalkyl acids and their precursors in indoor air sampled in children's bedrooms, Environ. Pollut., 2017, 222, 423–432 CrossRef CAS PubMed.
- W. Gao, D. Cao, Y. Wang, J. Wu, Y. Wang, Y. Wang and G. Jiang, External exposure to short- and medium-chain chlorinated paraffins for the general population in Beijing, China, Environ. Sci. Technol., 2018, 52, 32–39 CrossRef CAS PubMed.
- H.-L. Li, L.-Y. Liu, Z.-F. Zhang, W.-L. Ma, E. Sverko, Z. Zhang, W.-W. Song, Y. Sun and Y.-F. Li, Semi-volatile organic compounds in infant homes: Levels, influence factors, partitioning, and implications for human exposure, Environ. Pollut., 2019, 251, 609–618 CrossRef CAS PubMed.
- M. F. Sari, F. Esen, D. A. C. Del Aguila and P. B. Kurt Karakus, Passive sampler derived polychlorinated biphenyls (PCBs) in indoor and outdoor air in Bursa, Turkey: Levels and an assessment of human exposure via inhalation, Atmos. Pollut. Res., 2020, 11, 71–80 CrossRef CAS.
- B. Strandberg, C. Österman, H. K. Akdeva, J. Moldanová and S. Langer, The use of polyurethane foam (PUF) passive air samplers in exposure studies to PAHs in Swedish seafarers, Polycyclic Aromat. Compd., 2020 DOI:10.1080/10406638.2020.1739084.
- Y.-X. Feng, N.-X. Feng, L.-J. Zeng, X. Chen, L. Xiang, Y.-W. Li, Q.-Y. Cai and C.-H. Mo, Occurrence and human health risks of phthalates in indoor air of laboratories, Sci. Total Environ., 2020, 707, 135609 CrossRef CAS PubMed.
- E. Holt, P. Bohlin-Nizzetto, J. Borůvková, T. Harner, J. Kalina, L. Melymuk and J. Klánová, Using long-term air monitoring of semi-volatile organic compounds
to evaluate the uncertainty in polyurethane-disk passive sampler-derived air concentrations, Environ. Pollut., 2017, 220, 1100–1111 CrossRef CAS PubMed.
- J. Kalina, M. Scheringer, J. Borůvková, P. Kukučka, P. Přibylová, P. Bohlin-Nizzetto and J. Klánová, Passive air samplers as a tool for assessing long-term trends in atmospheric concentrations of semivolatile organic compounds, Environ. Sci. Technol., 2017, 51, 7047–7054 CrossRef CAS PubMed.
- J. Kalina, M. Scheringer, J. Borůvková, P. Kukučka, P. Přibylová, O. Sáňka, L. Melymuk, M. Váňa and J. Klánová, Characterizing spatial diversity of passive sampling sites for measuring levels and trends of semivolatile organic chemicals, Environ. Sci. Technol., 2018, 52(18), 10599–10608 CrossRef CAS PubMed.
- J. Kalina, K. B. White, M. Scheringer, P. Přibylová, P. Kukučka, O. Audy and J. Klánová, Comparability of long-term temporal trends of POPs from co-located active and passive air monitoring networks in Europe, Environ. Sci.: Processes Impacts, 2019, 21, 1132–1142 RSC.
- M. Sorais, M. J. Mazerolle, J.-F. Giroux and J. Verreault, Landfills represent significant atmospheric sources of exposure to halogenated flame retardants for urban-adapted gulls, Environ. Int., 2020, 135, 105387 CrossRef PubMed.
- X. Liu and F. Wania, Cluster analysis of passive air sampling data based on the relative composition of persistent organic pollutants, Environ. Sci.: Processes Impacts, 2014, 16, 453–463 RSC.
- X. Wang, J. Ren, P. Gong, C. Wang, Y. Xue, T. Yao and R. Lohmann, Spatial distribution of the persistent organic pollutants across the Tibetan Plateau and its linkage with the climate systems: A 5-year air monitoring study, Atmos. Chem. Phys., 2016, 16, 6901–6911 CrossRef CAS.
- S. J. Hayward, T. Gouin and F. Wania, Comparison of four active and passive sampling techniques for pesticides in air, Environ. Sci. Technol., 2010, 44, 3410–3416 CrossRef CAS PubMed.
- C. Cocheo, P. Sacco, P. Perez Ballesta, E. Donato, S. Garcia, M. Gerboles, D. Gombert, B. McManus, R. Fernandez Patier, C. Roth, E. de Saeger and E. Wright, Evaluation of the best compromise between the urban air quality monitoring resolution by diffusive sampling and resource requirements, J. Environ. Monit., 2008, 10, 941–950 RSC.
- G. Hoek, R. Beelen, K. de Hoogh, D. Vienneau, J. Gulliver, P. Fischer and D. Briggs, A review of land-use regression models to assess spatial variation of outdoor air pollution, Atmos. Environ., 2008, 42, 7561–7578 CrossRef CAS.
- L. Melymuk, M. Robson, P. A. Helm and M. L. Diamond, Application of land use regression to identify sources and assess spatial variation in urban SVOC concentrations, Environ. Sci. Technol., 2013, 47, 1887–1895 CrossRef CAS PubMed.
- R. Liard, M. Zureik, Y. Le Moullec, D. Soussan, M. Glorian, A. Grimfeld and F. Neukirch, Use of personal passive samplers for measurement of NO2, NO, and O3 levels in panel studies, Environ. Res., 1999, 81, 339–348 CrossRef CAS PubMed.
- N. Jariyasopit, P. Tung, K. Su, S. Halappanavar, G. J. Evans, Y. Su, S. Khoomrung and T. Harner, Polycyclic aromatic compounds in urban air and associated inhalation cancer risks: A case study targeting distinct source sectors, Environ. Pollut., 2019, 252, 1882–1891 CrossRef CAS PubMed.
- A. P. Francisco, A. C. Nardocci, M. Y. Tominaga, C. R. da Silva and J. V. de Assunção, Spatial and seasonal trends of polychlorinated dioxins, furans and dioxin-like polychlorinated biphenyls in air using passive and active samplers and inhalation risk assessment, Atmos. Pollut. Res., 2017, 8, 979–987 CrossRef.
- M. Isidori, M. Ferrara, M. Lavorgna, A. Nardelli and A. Parrella,
In situ monitoring of urban air in Southern Italy with the Tradescantia micronucleus bioassay and semipermeable membrane devices (SPMDs), Chemosphere, 2003, 52, 121–126 CrossRef CAS PubMed.
- G. Slapšytė, E. Lastauskiene and J. Mierauskiene, Genotoxicity of airborne hydrophobic pollutants sampled by semipermeable membrane devices (SPMDs) in Vilnius city, Biologija, 2006, 1, 41–46 Search PubMed.
- K. Kennedy, M. Macova, F. Leusch, M. E. Bartkow, D. W. Hawker, B. Zhao, M. S. Denison and J. F. Müller, Assessing indoor air exposures using passive sampling with bioanalytical methods for estrogenicity and aryl hydrocarbon receptor activity, Anal. Bioanal. Chem., 2009, 394, 1413–1421 CrossRef CAS PubMed.
- S. Bonetta, E. Carraro, S. Bonetta, C. Pignata, I. Pavan, C. Romano and G. Gilli, Application of semipermeable membrane device (SPMD) to assess air genotoxicity in an occupational environment, Chemosphere, 2009, 75, 1446–1452 CrossRef CAS PubMed.
- A. Érseková, K. Hilscherová, J. Klánová, J. P. Giesy and J. Novák, Effect-based assessment of passive air samples from four countries in Eastern Europe, Environ. Monit. Assess., 2014, 186, 3905–3916 CrossRef PubMed.
- S. J. Wallace, S. R. de Solla, P. J. Thomas, T. Harner, A. Eng and V. S. Langlois, Airborne polycyclic aromatic compounds contribute to the induction of the tumour-suppressing P53 pathway in wild double-crested cormorants, Ecotoxicol. Environ. Saf., 2018, 150, 176–189 CrossRef CAS PubMed.
- S.-D. Choi, S.-Y. Baek, Y.-S. Chang, F. Wania, M. G. Ikonomou, Y.-Y. Yoon, B.-K. Park and S. Hong, Passive air sampling of polychlorinated biphenyls and organochlorine pesticides at the Korean Arctic and Antarctic research stations: Implications for long-range transport local pollution, Environ. Sci. Technol., 2008, 42, 7125–7131 CrossRef CAS PubMed.
- S.-Y. Baek, S.-D. Choi and Y.-S. Chang, Three-year atmospheric monitoring of organochlorine pesticides and polychlorinated biphenyls in polar regions and the South Pacific, Environ. Sci. Technol., 2011, 45, 4475–4482 CrossRef CAS PubMed.
- X.-P. Wang, P. Gong, T.-D. Yao and K. C. Jones, Passive air sampling of organochlorine pesticides, polychlorinated biphenyls, and polybrominated diphenyl ethers across the Tibetan Plateau, Environ. Sci. Technol., 2010, 44, 2988–2993 CrossRef CAS PubMed.
- K. L. Foster, L. E. Kimpe, S. K. Brimble, H. Liu, M. L. Mallory, J. P. Smol, R. W. Macdonald and J. M. Blais, Effects of seabird vectors on the fate, partitioning, and signatures of contaminants in a High Arctic ecosystem, Environ. Sci. Technol., 2011, 45, 10053–10060 CrossRef CAS PubMed.
- Y. Li, D. Geng, Y. B. Hu, P. Wang, Q. Zhang and G. Jiang, Levels and distribution of polychlorinated biphenyls in the atmosphere close to Chinese Great Wall Station, Antarctica: Results from XAD-resin passive air sampling, Chin. Sci. Bull., 2012, 57, 1499–1503 CrossRef CAS.
- Y. Hao, Y. Li, X. Han, T. Wang, R. Yang, P. Wang, K. Xiao, W. Li, H. Lu, J. Fu, Y. Wang, J. Shi, Q. Zhang and G. Jiang, Air monitoring of polychlorinated biphenyls, polybrominated diphenyl ethers and organochlorine pesticides in West Antarctica during 2011–2017: Concentrations, temporal trends and potential sources, Environ. Pollut., 2019, 249, 381–389 CrossRef CAS PubMed.
- X. Han, Y. Hao, Y. Li, R. Yang, P. Wang, G. Zhang, Q. Zhang and G. Jiang, Occurrence and distribution of organophosphate esters in the air and soils of Ny-Ålesund and London Island, Svalbard, Arctic, Environ. Pollut., 2020, 263, 114495 CrossRef CAS PubMed.
- Y. Li, D. Geng, F. Liu, T. Wang, P. Wang, Q. Zhang and G. Jiang, Study of PCBs and PBDEs in King George Island, Antarctica,
using PUF passive air sampling, Atmos. Environ., 2012, 51, 140–145 CrossRef CAS.
- K. Pozo, T. Martellini, S. Corsolini, T. Harner, V. H. Estellano, P. Kukučka, M. D. Mulder, G. Lammel and A. Cincinelli, Persistent organic pollutants (POPs) in the atmosphere of coastal areas of the Ross Sea, Antarctica: Indications for long-term downward trends, Chemosphere, 2017, 178, 458–465 CrossRef CAS PubMed.
- C. Wang, X. Wang, P. Gong and T. Yao, Polycyclic aromatic hydrocarbons in surface soil across the Tibetan Plateau: Spatial distribution, source and air–soil exchange, Environ. Pollut., 2014, 184, 138–144 CrossRef CAS PubMed.
- X. Wang, J. Schuster, K. C. Jones and P. Gong, Occurrence and spatial distribution of neutral perfluoroalkyl substances and cyclic volatile methylsiloxanes in the atmosphere of the Tibetan Plateau, Atmos. Chem. Phys., 2018, 18, 8745–8755 CrossRef CAS.
- A. De la Torre, P. Sanz, I. Navarro and M. Á. Martínez, Time trends of persistent organic pollutants in Spanish air, Environ. Pollut., 2016, 217, 26–32 CrossRef CAS PubMed.
- J. Muñoz-Arnanz, J. L. Roscales, M. Ros, A. Vicente and B. Jiménez, Towards the implementation of the Stockholm Convention in Spain: Five-year monitoring (2008–2013) of POPs in air based on passive sampling, Environ. Pollut., 2016, 217, 107–113 CrossRef PubMed.
- J. L. Roscales, J. Muñoz-Arnanz, M. Ros, A. Vicente, L. Barrios and B. Jiménez, Assessment of POPs in air from Spain using passive sampling from 2008 to 2015. Part I: Spatial and temporal observations of PBDEs, Sci. Total Environ., 2018, 634, 1657–1668 CrossRef CAS PubMed.
- J. Muñoz-Arnanz, J. L. Roscales, A. Vicente, M. Ros, L. Barrios, L. Morales, E. Abad and B. Jiménez, Assessment of POPs in air from Spain using passive sampling from 2008 to 2015. Part II: Spatial and temporal observations of PCDD/Fs and dl-PCBs, Sci. Total Environ., 2018, 634, 1669–1679 CrossRef PubMed.
- E. A. Mamontova, E. N. Tarasova, A. A. Mamontov, A. V. Goreglyad and L. L. Tkachenko, Variations in the concentration of polychlorinated biphenyls and organochlorine pesticides in air over the Northern Hovsgol Region in 2008–2015, Russ. Meteorol. Hydrol., 2019, 44, 78–85 CrossRef.
- M. Y. Tominaga, C. R. Silva, J. P. Melo, N. A. Niwa, D. Plascak, C. A. M. Souza and M. I. Z. Sato, PCDD, PCDF, DL-PCB and organochlorine pesticides monitoring in São Paulo City using passive air sampler as part of the Global Monitoring Plan, Sci. Total Environ., 2016, 571, 323–331 CrossRef CAS PubMed.
- F. Wong, A. Alegria and T. F. Bidleman, Organochlorine pesticides in soils of Mexico and the potential for soil-air exchange, Environ. Pollut., 2010, 158, 749–755 CrossRef CAS PubMed.
- P. Karásková, G. Codling, L. Melymuk and J. Klánová, A critical assessment of passive air samplers for per- and polyfluoroalkyl substances, Atmos. Environ., 2018, 185, 186–195 CrossRef.
Footnote |
† Current affiliation and address: Air Quality Processes Research Section, Environment and Climate Change Canada, 4905 Dufferin St, Ontario, M3H 5T4, Canada. |
|
This journal is © The Royal Society of Chemistry 2020 |