Efficient and effective removal of toluene from aqueous solution using MIL-100(Fe)†
Received
15th June 2024
, Accepted 4th July 2024
First published on 10th July 2024
Abstract
The MIL-100(Fe) was employed for the remediation of toluene-contaminated water. The MIL-100(Fe) samples synthesised for this work exhibit high thermal (300 °C) and chemical (pH range 2–10) stability. Adsorption kinetics and isotherms were fitted to the Elovich and Temkin models. The pH of the aqueous sample containing Toluene impacted the adsorption capacity of MIL-100(Fe) through modulation of the MOF ζ potential. As a result, we concluded that MIL-100(Fe) is most effective at adsorbing toluene in the 6–10 pH range, a finding that underscores its potential in water treatment. The maximum Langmuir adsorption capacity of 318.48 mg g−1 was determined. MIL-100(Fe) showed excellent adsorption–desorption performance and stability; hence, it can be used repeatedly without losing toluene adsorption capacity. FT-IR spectra suggest that π–π interactions serve a crucial role during toluene adsorption, further confirming the effectiveness of MIL-100 (Fe) in water treatment.
Water impact
Due to the lack of research focused on removing VOCs from aqueous solutions, this study presents the application of MIL-100(Fe), an advanced adsorbent, for toluene removal from water, an aromatic VOC of high toxicity. MIL-100(Fe) is a promising system for VOC adsorption and illustrates how these systems need to be further explored in applications that impact our environment.
|
Introduction
Water pollution, a pressing environmental issue that arises from the uncontrolled release of wastewater from the vast industrial and municipal sectors,1 has escalated due to the burgeoning human population. This pollution has a profound impact on aquatic ecosystems. Generally, the main pollutants are organic dyes, heavy metals, nutrients, pharmaceuticals, and volatile organic compounds (VOCs).2 Furthermore, VOCs are chemical complexes based on aromatic and hydrocarbon structures.2 VOCs are highly persistent in the environment and are classified as harmful and toxic pollutants. VOCs participate in atmospheric photochemical reactions that promote ozone formation,3 due to their usually being found in the vapor phase. However, VOCs can be located in soil4 and water5 mainly due to anthropogenic activities, such as refineries,6 pharmaceuticals7 and textiles manufacturers,8 and natural (biogenic) sources.9 In this context, the presence of VOCs pollutants in water ecosystems has emerged as a problem. The main concern is the lack of monitoring of VOCs in water systems. The family of VOC aromatics (benzene, toluene, and xylenes) are the most persistent in water.10 Specifically, toluene is a widely known raw material for diverse solvents, inks, and adhesives applications.11 Toluene molecules have displayed high toxicity for human health, even at low concentrations.12
Thus, developing methodologies to remove toluene from the water matrix is necessary. Adsorbents such as zeolites13 and activated carbon14 have been employed to remove VOCs from water, but they have been demonstrated to be ineffective. As an alternative, Metal–Organic Frameworks (MOFs) are crystal materials formed of metal ion centers linked by organic ligands.15 They are highly porous and structurally stable, making them excellent adsorbents for removing pollutants from water.
Different MOF materials have been applied to remediate toluene in the gas phase. UiO-66-NH2, UiO-66, MOF-199, and ZIF-67 show high adsorption capacity involving hydrogen bonding and π–π complexation as the main interactions.16 Based on this outstanding result of applying MOF materials for toluene adsorption, the evaluation of MOF in aqueous media is analyzed. Furthermore, the implementation of iron-containing MOFs, such as MIL-100(Fe), is desirable for water remediation due to their low cost, high stability in water, high porosity compared to conventional adsorbents, excellent performance, reusability, and low toxicity (compared to chromium, cobalt, and cadmium analogues), as well as their excellent performance in removing contaminants.
Thus, this study explores the application of an MOF material in the adsorption process for removing toluene from an aqueous solution using a Fe-based MOF. MIL-100(Fe) was characterized by different analytical techniques such as powder X-ray diffraction (PXRD), fourier-transform infrared spectroscopy (FTIR), thermogravimetric analysis (TGA), N2 adsorption, scanning electron microscope (SEM), and X-ray photoelectron spectroscopy (XPS). The toluene quantification was performed using gas chromatography (GC) combined with an appropriate sample pretreatment. The effect of dosage, pH, contact time, concentration, selectivity, and reusability was studied. The main adsorption mechanism was determined by FTIR and XPS techniques. Hence, this research corroborates the prospective application of MOF materials for toluene remediation in water.
Experimental
Chemicals
Iron(III) chloride hexahydrate (FeCl3·6H2O, ≥98%), benzene-1,3,5-tricarboxylic acid (H3BTC, 95%), toluene (C6H5CH3, 99.5%), benzene (C6H6, 99.8%), xylene (C6H4(CH3)2, 98%), chlorobenzene (C6H5Cl, 99.8%), methanol (CH3OH, 99.8%), ethanol (CH3CH2OH, 95%), acetone (CH3COCH3, 99.5%), n-hexane (CH3(CH2)4CH3, 99.8%), nitric acid (HNO3, 70%), sodium hydroxide pellets (NaOH, 97%), anhydrous magnesium sulfate (MgSO4, 97%) and hydrofluoric acid (HF, 48%) were supplied by Sigma-Aldrich. High-purity deionized water with a specific resistance of 18.2 mΩ cm−1 was obtained from a Mili-Q system Simplicity®. All reagents and solvents were used as received from commercial suppliers without further purification.
Synthesis of MIL-100(Fe)
MIL-100(Fe) synthesis (Fig. S1†) was performed as reported by Guo and collaborators.17 H3BTC linker (0.656 g, 3.94 mmol) and FeCl3·6H2O (0.972 g, 3.6 mmol) were dissolved in deionized water (20 mL) with HF (150 μL, 8.62 mmol) modulator. The mixed solution was transferred to a Teflon liner placed in an autoclave (50 mL), then heated to 150 °C for 24 h. Subsequently, the powder was centrifugated and washed with water, ethanol, and acetone (three times) and dried at 100 °C overnight.
Instruments
Detailed information on the instrumental techniques is available in section S1.†
Adsorption experiments
Experiments were carried out in triplicates. Toluene adsorption evaluations were performed by adding a known mass (1–30 mg) of MIL-100(Fe) in a fixed volume (20 mL) with a toluene initial concentration (5–150 mg L−1) under stirring at a specific time (1440 min), at room temperature, under a controlled pH (2–10), at the end of the experiments through gas chromatography was analysed the residual amount of toluene by liquid–liquid extraction. More detailed information is available in the S2† section.
Results and discussion
Characterization of MIL-100(Fe)
On account of the promising characteristic of MIL-100(Fe) for aqueous remediation applications, we prepared samples of this MOF as per the previously reported synthesis by Simon et al.18 The Powder X-ray Diffraction (PXRD) pattern obtained from our MIL-100(Fe) sample is similar to the simulated pattern (Fig. 1a) and previously reported data. Then, the Fourier Transform Infra-Red (FT-IR) spectrum (Fig. 1b) possesses the characteristic bands expected for MIL-100(Fe). The band at 484 cm−1 corresponds to the Fe–O vibration, while the bands at 713, 758, 1383, and 1448 cm−1 are related to the C–H stretching signals from the aromatic ring and the symmetric and asymmetric stretching vibrations of the carboxylate from the BTC ligand, respectively. Moreover, the band at 1576 cm−1 indicates the vibration generated from C
C aromatics groups. The coordination of the C
O carbonyl group with the metal center is shown at the band at 1631 cm−1. Finally, the band at 3482 cm−1 corresponds to the stretching of the –OH group. These bands agree with those reported previously for this material.19,20 Further, the pH stability test conducted in the 2–10 interval indicates that MIL-100(Fe) retains its characteristic signals and bands as identified through FT-IR and PXRD analysis, without any modification in this interval.
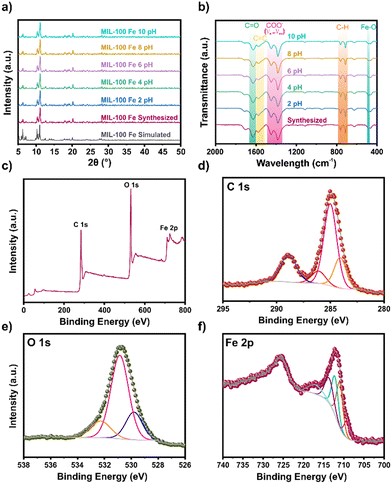 |
| Fig. 1 a) PXRD pattern; b) FT-IR spectrum; c) XPS survey spectrum; high-resolution spectra of d) C 1s; e) O 1s; and f) Fe 2p of synthesized MIL-100(Fe). | |
The thermogravimetric analysis (TGA) (Fig. S2a†) shows thermal stability up to 300 °C. The weight loss is divided into three stages.21,22 The first is related to moisture release. The second stage is associated with the breakdown of the MOF, with the decomposition of the unreacted H3BTC molecules, forming and detaching CO2 from organic molecules' carbonization. And a third stage, above 550 °C, in which the structure collapses, forming residual Fe2O3.
The surface morphology of the MIL-100(Fe) obtained was analysed by SEM (Fig. S3a†). Particles of irregular superficial morphology were obtained.23 The surface element distribution analysis was also performed (Fig. S3b†). A homogeneous distribution of carbon, oxygen, and iron distribution was observed on the surface. The nitrogen adsorption measurements were performed at 77 K (Fig. S2b†). The isotherm obtained is similar to those reported previously for this material.24 The calculated BET surface area was 743 m2 g−1, with a volume pore of 0.750 cm3 g−1. This surface area was similar to that reported by Nehra and collaborators.25 X-ray photoelectron spectroscopy (XPS) analysis (Fig. 1c–f) was performed to obtain details of the chemical environment. The results of the survey spectra (Table S1†) corroborated C, O, and Fe presence in MIL-100(Fe). The high-resolution XPS spectra of C 1s (Fig. 1d) fit show peaks at 284.1, 285.0, 286.1, and 288.9 eV, attributed to the aromatic C
C of the linker, C–C/C–H, C–O due to OH groups, and –C
O for the carboxylic moieties,26 respectively (Table S2†). The O 1s high-resolution (Fig. 1e) signals fit showed peaks at 529.8, 530.9, and 532.3 eV, related to Fe–O, C
O, and O-chemisorbed,27 respectively (Table S3†). The Fe 2p high-resolution signals (Fig. 1f) fit showed peaks at 709.2, 711.0, 712.2, 713.6, and 717.0 eV, related to Fe+2, and three different Fe+3 contributions, and satellite Fe+3 respectively (Table S4†).28
Effect of parameters on toluene adsorption
Having thoroughly characterised the chemical and physical properties of MIL-100(Fe), we turned our attention towards assessing the toluene adsorption performance of the material. Gas chromatography analysis was carried out to measure the amount of residual toluene. The sample was subjected to liquid–liquid extraction pretreatment to obtain an accurate analysis, which included preconcentration and isolation steps. The extraction process utilized n-hexane as the organic phase, chlorobenzene as the internal standard, and saturated magnesium sulphate as a dry agent. More detailed information can be found in section S2.†
This study investigated several factors that affect the adsorption of toluene by MIL-100(Fe). The impact of the amount of adsorbent dose used was examined by adding 1–30 mg of MIL-100(Fe) (Fig. 2a). It can be noticed an adsorption capacity decreases when the mass of the adsorbent increases. However, an increment of removal efficiency was observed from 57% to 80% from 1 to 30 mg, respectively (Fig. S6†). This can be attributed to the interaction sites increment as the mass increases.
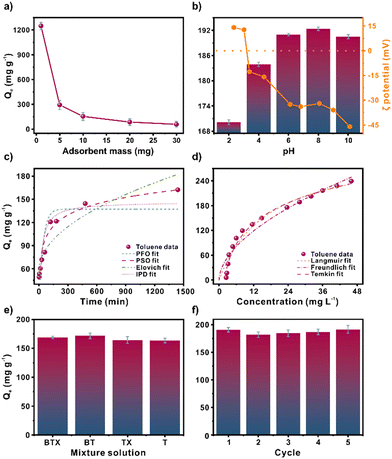 |
| Fig. 2 a) Adsorbent mass effect [1–30 mg, 20 mL, 100 mg L−1, 6.5 pH, 24 h]; b) pH solution effect [10 mg, 20 mL, 100 mg L−1, 2–10 pH, 24 h]; c) kinetic fits [10 mg, 20 mL, 100 mg L−1, 6.5 pH, 24 h]; d) isotherms fits [10 mg, 20 mL, 5–150 mg L−1, 6.5 pH, 24 h], e) toluene (T) adsorption in presence of benzene (B) and xylene (X) [10 mg, 20 mL, 100 mg L−1, 6.5 pH, 24 h], and f) toluene adsorption in regenerated MIL-100(Fe) [10 mg, 20 mL, 100 mg L−1, 6.5 pH, 24 h]. | |
We next considered the effect of pH on adsorption behaviour. The results reveal reduced adsorption capacity at pH 2–4 (Fig. 2b). Based on the ζ potential analysis, we could attribute this behaviour to the agglomeration of particles close to the MIL-100(Fe) isoelectric point (pH 3.2), thereby reducing the absorbent-adsorbate interaction. When the ζ potential ranges between −30 to +30 mV, the particles tend to agglomerate and become less stable in dispersion.29 Above pH 6, the ζ potential is above −30 mV. Thus, the entire surface area and interaction sites are exposed and available. The ideal pH operates in ranges of 6–10, in which the MIL-100(Fe) particles are dispersed in the solution. The variation in adsorption capacity is negligible, with a maximum adsorption capacity of 192.36 mg g−1 at pH 8 and a minimum adsorption capacity of 190.47 mg g−1 at pH 10.
Adsorption kinetics and adsorption isotherms
Furthermore, non-linear fitting to the pseudo-first-order (PFO), pseudo-second-order (PSO), Elovich, and Intra-particle diffusion (IPD) kinetic models were analysed to study the adsorption kinetics of toluene over MIL-100(Fe) (Fig. 2c). The main parameters for each model are listed in Table S7.† The Elovich model displayed the best fit with a correlation coefficient (R2) of 0.950. This model often considers a slow adsorption rate based on chemical adsorption. Conversely, in an effort to better understand the interactions between MIL-100(Fe) and toluene molecules, a non-linear fitting of the Freundlich, Langmuir, and Temkin models was performed (Fig. 2d and Table S9). The fitting order concerning the correlation coefficient, R2, was Temkin > Langmuir > Freundlich with values of 0.982, 0.963, and 0.937 R2, respectively. The Temkin model considers the indirect adsorbate–adsorbent interaction due to the heat of adsorption decreasing linearly with increasing adsorbent surface coverage.30 It assumes a uniform distribution of interaction sites, similar to the Langmuir model.31 The maximum adsorption capacity obtained was 318.48 mg g−1. Compared to other reported toluene adsorbents (Table S5†), the material is highly competitive.
Effect of coexistence pollutants and reusability
The ability of MIL-100(Fe) to adsorb toluene in the presence of other pollutants, such as benzene and a mixture of xylenes, was evaluated (Fig. 2e). Three experiments were conducted to assess the impact of these pollutants on toluene adsorption. The first experiment involved exposing MIL-100(Fe) to a mixture of benzene, toluene, and xylenes, while the second and third experiments involved a mixture of benzene and toluene, and xylenes, respectively. The results showed a negligible variation in the toluene adsorption capacity compared to the toluene adsorption alone. Hence, xylenes and benzene have minimal impact on toluene adsorption.
The ability to regenerate an adsorbent is highly beneficial since it increases the lifetime adsorption capacity of the absorbent. Five toluene adsorption–desorption cycles were performed using MIL-100(Fe) to assess the reusability of the material (Fig. 2f). Due to the high boiling temperature of toluene (110 °C), the desorption was performed at an elevated temperature of 110 °C to ensure that adsorbed toluene was fully released from MIL-100(Fe). Across the adsorption–desorption cycles, negligible adsorption capacity variation was noted. The structural stability of MIL-100(Fe) over the course of multiple adsorption–desorption cycles was evaluated (Fig. S5†) by PXRD analysis, which confirmed that the MIL-100(Fe) structure was preserved.
Adsorption mechanism
To investigate the possible adsorption mechanism, we turned our attention to FT-IR spectroscopy of MIL-100(Fe) samples before and after toluene adsorption (Fig. 3a). The appearance of new bands at 1045 and 1090 cm−1 is attributed to C–H bending in the plane, and a variation in 760 cm−1 corresponds to C–H bending out of a plane from toluene.31,32 Additionally, the appearance of a shoulder around 1320 cm−1 assigned to the C–C originated from the toluene aromatic ring adsorption in MIL-100(Fe).33 The above and the changes in the FT-IR spectrum in the region related to the C
C and C
O stretching vibration from the aromatic ring and carboxyl groups may suggest a π–π interaction among the aromatic toluene ring and the MIL-100(Fe).34
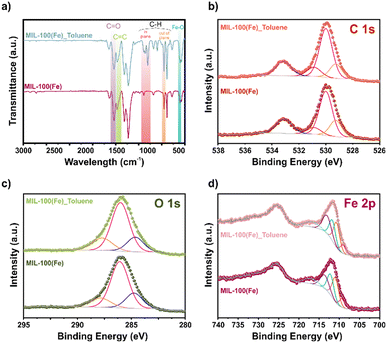 |
| Fig. 3 a) FT-IR spectrum and high-resolution spectra of b) C 1s; c) O 1s; and d) Fe 2p for MIL-100(Fe). | |
In order to analyse the adsorption mechanism within MIL-100(Fe), XPS analysis was performed. The high-resolution XPS spectra of C 1s fit before and after toluene adsorption show unaltered peaks (Fig. 3b). However, the high-resolution XPS spectra of O 1s (Fig. 3c) display slight shifts (0.1 eV) from the Fe–O and –C
O peaks. This change was at lower energies from 529.8 to 529.7 eV and 530.9 to 530.8 eV, respectively. Similarly, the high-resolution XPS spectra of Fe 2p (Fig. 3d) show a shift to lower binding energies after the toluene adsorption in the Fe3+ contributions, from 711.0, 712.2, and 713.6 to 710.7, 711.7, and 713.1 eV.
These changes could be related to the coordination of water molecules through the open metal sites of MIL-100(Fe) since the adsorption process was via an aqueous solution.35 In this scenario, the toluene molecule could enter the MIL-100(Fe) pore and interrelate via π–π interaction with the organic linkers due to the low availability of the metal centre for water coordination.36 The above analysis proposed the possible interaction mechanism (Fig. 4) between the toluene molecules and MIL-100(Fe).
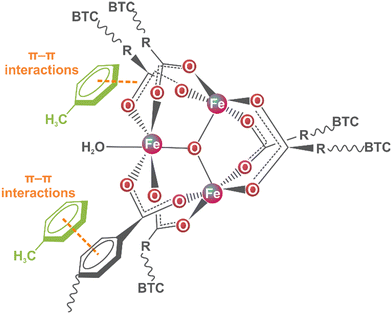 |
| Fig. 4 Possible adsorption mechanism for toluene adsorption using MIL-100(Fe). | |
Conclusion
In summary, we have evaluated the effect of several relevant factors on the toluene adsorption behaviour of MIL-100(Fe), including kinetic models, adsorption isotherms, mass dosage, pH of the polluted sample, the presence of coexisting pollutants, adsorption–desorption cycles, and the characterization of MIL-100(Fe). The optimal pH range was determined to be pH 6–10 since the ζ potential of MIL-100(Fe) at lower pH promotes agglomeration of the MIL-100(Fe) particles in the solution, which is detrimental to toluene adsorption capacity. The Langmuir maximum adsorption capacity was estimated to be 318.48 mg g−1, a highly competitive value considering the alternative adsorbents used for toluene adsorption. MIL-100(Fe) exhibits outstanding adsorption–desorption performance over multiple cycles. FT-IR and XPS characterizations suggest a π–π interaction, along the C
C and C
O in MIL-100(Fe) with the toluene ring, as the possible adsorption mechanism. Overall, this work demonstrates that MIL-100(Fe) is a promising system for toluene adsorption and illustrates how these systems need to be further explored in applications that impact our environment.
Data availability
All data is available in the main manuscript and ESI.†
Conflicts of interest
There are no conflicts to declare.
Acknowledgements
The authors thank U. Winnberg (Euro Health) for scientific discussions and G. Ibarra-Winnberg for scientific encouragement. C. V. F and J. L. O. thank CONAHCYT for the Ph.D. fellowships (1040318 and 1003953). C. L. thanks to the IPN projects (20241189 and 20242829).
References
- J. Mikosz, Int. J. Environ. Sci. Technol., 2015, 12, 827–836 CrossRef CAS
.
-
S. Madhav, A. Ahamad, A. K. Singh, J. Kushawaha, J. S. Chauhan, S. Sharma and P. Singh, in Sensors in Water Pollutants Monitoring: Role of Material, ed. D. Pooja, P. Kumar, P. Singh and S. Patil, Springer Singapore, Singapore, 2020, pp. 43–62 Search PubMed
.
- Y. Zhang, T. Zang, B. Yan and C. Wei, Int. J. Environ. Res. Public Health, 2020, 17, 553 CrossRef CAS PubMed
.
- K. Schulz-Bohm, S. Geisen, E. R. J. Wubs, C. Song, W. De Boer and P. Garbeva, ISME J., 2017, 11, 817–820 CrossRef CAS PubMed
.
- A. Nikolaou, Water Res., 2002, 36, 2883–2890 CrossRef CAS PubMed
.
- S. Saikomol, S. Thepanondh and W. Laowagul, J. Environ. Health Sci. Eng., 2019, 17, 561–570 CrossRef CAS PubMed
.
- V. S. Priya and L. Philip, Chem. Eng. J., 2015, 266, 309–319 CrossRef CAS
.
- Z. Liang, J. Wang, Y. Zhang, C. Han, S. Ma, J. Chen, G. Li and T. An, J. Cleaner Prod., 2020, 253, 120019 CrossRef CAS
.
- P. K. Padhy and C. K. Varshney, Chemosphere, 2005, 59, 1643–1653 CrossRef CAS PubMed
.
- C. Wang, W. Wang, S. Shao, W. Deng, C. Wang, X. Liu, H. Li, M. Wen, X. Zhang, G. Li and T. An, Sci. Total Environ., 2024, 917, 170407 CrossRef CAS PubMed
.
- S. L. Cruz, M. T. Rivera-García and J. J. Woodward, J. Drug Alcohol Res., 2014, 3, 1–8 CrossRef PubMed
.
- M. Rooseboom, N. A. Kocabas, C. North, R. J. Radcliffe and L. Segal, Regul. Toxicol. Pharmacol., 2023, 141, 105387 CrossRef CAS PubMed
.
- Z. Mamaghanifar, A. Heydarinasab, A. Ghadi and E. Binaeian, Water Conserv. Sci. Eng., 2020, 5, 1–13 CrossRef
.
- H. Anjum, K. Johari, N. Gnanasundaram, A. Appusamy and M. Thanabalan, J. Mol. Liq., 2019, 280, 238–251 CrossRef CAS
.
- S. L. James, Chem. Soc. Rev., 2003, 32, 276 RSC
.
- K. Vellingiri, P. Kumar, A. Deep and K.-H. Kim, Chem. Eng. J., 2017, 307, 1116–1126 CrossRef CAS
.
- X.-Z. Guo, S.-S. Han, J.-M. Yang, X.-M. Wang, S.-S. Chen and S. Quan, Ind. Eng. Chem. Res., 2020, 59, 2113–2122 CrossRef CAS
.
- M. A. Simon, E. Anggraeni, F. E. Soetaredjo, S. P. Santoso, W. Irawaty, T. C. Thanh, S. B. Hartono, M. Yuliana and S. Ismadji, Sci. Rep., 2019, 9, 16907 CrossRef PubMed
.
- B. T. Le, D. D. La and P. T. H. Nguyen, ACS Omega, 2023, 8, 1262–1270 CrossRef CAS PubMed
.
- S. Rostamnia and H. Alamgholiloo, Catal. Lett., 2018, 148, 2918–2928 CrossRef CAS
.
- S. Huang, K.-L. Yang, X.-F. Liu, H. Pan, H. Zhang and S. Yang, RSC Adv., 2017, 7, 5621–5627 RSC
.
- C. R. Quijia, C. Lima, C. Silva, R. C. Alves, R. Frem and M. Chorilli, J. Drug Deliv. Sci. Technol., 2021, 61, 102217 CrossRef CAS
.
- W. Li, T. Zhang, L. Lv, Y. Chen, W. Tang and S. Tang, Colloids Surf., A, 2021, 624, 126791 CrossRef CAS
.
- M. Ahmad, S. Chen, F. Ye, X. Quan, S. Afzal, H. Yu and X. Zhao, Appl. Catal., B, 2019, 245, 428–438 CrossRef CAS
.
- M. Nehra, N. Dilbaghi, N. K. Singhal, A. A. Hassan, K.-H. Kim and S. Kumar, Environ. Res., 2019, 169, 229–236 CrossRef CAS PubMed
.
- F. Zhang, J. Shi, Y. Jin, Y. Fu, Y. Zhong and W. Zhu, Chem. Eng. J., 2015, 259, 183–190 CrossRef CAS
.
- J.-H. Kim, H.-Y. Jang, S.-B. Kim, J.-W. Choi and J.-A. Park, Water, Air, Soil Pollut., 2022, 233, 480 CrossRef CAS
.
- Y. Meng, Y. Huang, G. Huang and Y. Song, RSC Adv., 2023, 13, 28148–28157 RSC
.
-
A. Barhoum, M. L. García-Betancourt, H. Rahier and G. Van Assche, in Emerging Applications of Nanoparticles and Architecture Nanostructures, Elsevier, 2018, pp. 255–278 Search PubMed
.
- N. Ayawei, A. N. Ebelegi and D. Wankasi, J. Chem., 2017, 2017, 1–11 CrossRef
.
- H. Wang, D. J. Grant, P. C. Burns and C. Na, Langmuir, 2015, 31, 5820–5826 CrossRef CAS PubMed
.
- L. Heredia, E. Colombo, P. Quaino and S. Collins, Top. Catal., 2022, 65, 934–943 CrossRef CAS
.
- O. Baytar, Ö. Şahin, S. Horoz and S. Kutluay, Environ. Sci. Pollut. Res., 2020, 27, 26191–26210 CrossRef CAS PubMed
.
- Y. Hou, Z. Li, S. Ren and W. Wu, Fuel Process. Technol., 2015, 135, 99–104 CrossRef CAS
.
- S.-H. Huo and X.-P. Yan, J. Mater. Chem., 2012, 22, 7449 RSC
.
- X. Ma, W. Wang, C. Sun, H. Li, J. Sun and X. Liu, Sci. Total Environ., 2021, 793, 148622 CrossRef CAS PubMed
.
Footnote |
† Electronic supplementary information (ESI) available: Instrumental techniques, characterization, and computational chemistry. See DOI: https://doi.org/10.1039/d4ew00503a |
|
This journal is © The Royal Society of Chemistry 2024 |