DOI:
10.1039/D4BM00709C
(Review Article)
Biomater. Sci., 2024,
12, 4103-4116
Splittable systems in biomedical applications
Received
22nd May 2024
, Accepted 7th July 2024
First published on 16th July 2024
Abstract
Splittable systems have emerged as a powerful approach for the precise spatiotemporal control of biological processes. This concept relies on splitting a functional molecule into inactive fragments, which can be reassembled under specific conditions or stimuli to regain activity. Several binding pairs and orthogonal split fragments are introduced by fusing with other modalities to develop more complex and robust designs. One of the pillars of these splittable systems is modularity, which involves decoupling targeting, activation, and effector functions. Challenges, such as off-target effects and overactivation, can be addressed through precise control. This review provides an overview of the design principles, strategies, and applications of splittable systems across diverse fields including immunotherapy, gene editing, prodrug activation, biosensing, and synthetic biology.
 Sichen Yuan | Sichen Yuan is a Ph.D. student under the supervision of Professor Hu at the University of Wisconsin–Madison School of Pharmacy. She received a bachelor's degree in Pharmaceutical Sciences from Zhejiang University in 2022. Sichen's research interests lie in the field of cell-based drug delivery systems, particularly focusing on their application in immunotherapy. |
 Quanyin Hu | Quanyin Hu is an Assistant Professor at School of Pharmacy, University of Wisconsin–Madison (UW-Madison). He received his Ph. D. degree in Biomedical Engineering at University of North Carolina at Chapel Hill (UNC-CH) and North Carolina State University. Before he joined UW-Madison, he was a postdoc associate at Koch Institute for Integrative Cancer Research at Massachusetts Institute of Technology (MIT). His research group focuses on drug delivery, cell therapy, immunotherapy and personalized therapy. |
1. Introduction
The ability to precisely control and modulate biological processes in a spatiotemporal manner is a longstanding goal in various research fields, ranging from therapeutic development to synthetic biology. Splittable systems have emerged as a powerful approach for achieving this level of control, offering unique advantages over traditional methods. The concept of splitting relies on dividing a functional molecule into two or more inactive fragments that can reassemble and regain activity under specific conditions or in response to stimuli. The principle of modularity lies at the core of splittable systems, where the functions of targeting, activation, and effector domains can be effectively decoupled and reassembled in a controlled manner. This strategy circumvents several challenges associated with conventional approaches and offers many advantages, such as minimizing off-target effects, enhancing specificity, and enabling the conditional activation or deactivation of biological processes on demand.
Splittable systems have found widespread applications in diverse fields, including immunotherapy, gene editing, prodrug activation, biosensing, and synthetic biology.1–4 In the realm of cancer immunotherapy, splitting strategies have been employed to develop chimeric antigen receptor (CAR) T-cell therapies with improved tumor specificity and reduced off-tumor toxicity.5,6 Furthermore, the engineering of bispecific antibodies has benefited from split designs, enabling enhanced target recognition and recruitment of cytotoxic effector cells.7
In this review, we aim to provide an overview of the current state of research on splittable systems for biomedical applications, with a focus on their design principles, strategies, and diverse applications across various domains (Fig. 1). We highlight key examples, discuss the advantages and limitations of different approaches, and explore potential avenues for future development and translation of these innovative technologies.
 |
| Fig. 1 Schematic illustration of technologies for splittable designs and their applications. | |
2. Unifying principles and common strategies in split systems
When fabricating split systems, one purpose is splitting the original moiety to deprive it of function and maintain suitable biophysical properties, including solubility and stability. Another purpose is to ensure that the function can be restored upon reassembly. There is a lack of clear criteria and principles to guide the design; in most cases, several split sites were chosen, and the optimal structure was screened out. However, empirical principles, such as domain boundaries, solvent exposure, and intramolecular interactions, guide structural considerations and site selection. We expect that certain engineering principles can be applied to various biomolecules. A splittable protein always has multiple domains, or at least recognizable structures, to be nicked. The split sites can be within the active sites, which will dramatically abrogate the activity when segments are separated. They can also be distal to the functional sites, avoiding the disruption of their original function during engineering.8 The splitting site should be fully exposed to the solvent to enable dimerization and prevent the formation of protein aggregates when the hydrophobic regions are exposed after splitting.9 It should not be involved in extensive intramolecular or extra-molecular interactions that may affect the secondary structures of segments.10 With the development of computational technologies that facilitate the understanding of protein structure, these tools aid in the design process for split-site selection.11 Furthermore, strategies for improving the stability, solubility, and reassembly efficiency can be applied once suboptimal splitting structures are determined.12 Efforts have also been made to minimize background activity by preventing premature assembly without induction. For example, Dagliyan et al. developed a scoring function called split energy as a predictor for split sites and successfully applied it to split proteins, such as tyrosine kinase and tobacco etch virus (TEV) protease.13 Dolberg et al. performed an in silico scan to obtain the interaction energy across the interface and total stability.14 This demonstrated that based on the energy partition, effective selection of a split site can be achieved by predicting and evaluating the energy perturbation of the mutations.
After splitting biomolecules, approaches for controlled reassembly can be employed to achieve better performance. Strategies for controlled reassembly include chemical-induced dimerization (CID), trans-splicing systems, biological orthogonal pairs, facilitating proximity-induced functions, and precise control. In CID, the most commonly used pairs are FK506 binding protein (FKBP) and FK506-rapamycin binding (FRB),15 which form a ternary complex in the presence of rapamycin. The small size of the FKPB and FRB makes it amenable to split systems to aid the proximity of split agents. A variety of inducible dimerization systems have been developed that can be controlled by chemicals, light, etc.16,17 Other orthogonal pairs with high affinity have been fused to split agents and promote recognition through direct binding, such as the SpyTag and SpyCatcher.18 In addition to direct binding, a trans-splicing approach such as split inteins can promote the reassembly of split units by self-splicing the reconstitution.19
3. Biomedical applications
3.1 Immunotherapy
Split strategies have been employed in chimeric antigen receptor (CAR) T-cell therapies and bispecific antibodies, to improve tumor specificity, reduce off-tumor toxicity, and enhance target recognition and effector cell recruitment.
3.1.1 CAR-T therapy.
CARs are typically composed of a single-chain antibody variable domain (scFv) coupled with a hinge and transmembrane domain, and are further linked to intracellular costimulatory and activation domains. Splitting CARs can offer multiple targeting properties and restrict the activation selectivity of CAR-T cells for reduced on-target, off-tumor side effects and toxicity. Because of the modularity of CAR, the splitting sites vary in different designs. Strategies include introducing dual antigen recognition for CAR cell activation, decoupling the antigen recognition and downstream signaling, and masking activation domains for conditional activation. The reconstitution of CAR subunits can also be regulated by peptides, small molecules like biological orthogonal pairs, and light-sensitive elements.
Improved selectivity of activation.
The selection of scFv, with varying affinity for antigens, significantly impacts antigen specificity and potential side effects in CAR design. High-affinity scFvs can recognize cells at a lower level of antigen density but also allow on-target, off-tumor recognition; while low-affinity scFv allows for better antigen density discrimination; however, its specificity is compromised. This dilemma related to CAR-T cell targeting can be rectified using split strategies.
A simple way is to split CAR by separating the CD3ζ and co-stimulatory domains to improve the safety profile of CAR-T therapy. Those domains are reconstituting with antigen recognition domains that have various specificities. When confronting antigens, the suboptimal activation of CARs upon binding of one antigen leads to a mild effect while dual recognitions facilitate the maximum response.20 Innovatively, He et al. developed the bispecific and split (Biss) CAR-T cells targeting CD13+ TIM3+ tumor cells with high activation efficiency, while showing relatively low activation towards CD13+ TIM3− cells. This differentiation between acute myeloid leukemia (AML) stem cells and hematopoietic stem cells facilitates selective tumor eradication.5 The bispecific and split CAR-T cells expressed a nanobody-CD3ζ domain and an antiTIM3scFv-CD28-4-1BB domain. The nanobody against CD13 was screened for directing CAR-T cells to induce target cell death, specifically in CD13+ tumor cells. The antiTIM3scFv-CD28-41BB domain promoted the function of CAR-T cells by inducing cytokine secretion, including IFN-γ and TNF-α, towards CD13+ TIM3+ cancer cells. The induced cytokine production, however, maintained a relatively lower killing effect on cells that only express CD13 recognized by anti-CD13 nanobodies. In the humanized mouse model, these BissCAR-T cells effectively eradicated AML in the patient-derived xenograft mouse model, but reduced hematopoietic toxicity. However, because the single CD3ζ-CAR activation was sufficient for cell killing, the issue of the on-target off-tumor effect was mitigated, but not fully addressed. Recently, a study applied the proximal signaling molecules, LAT (linker for activation of T cell) and SLP-76, in split CARs to bypass the reliance of CAR-T cell activation on CD3ζ.21 This is the first system that has achieved a truly AND-logic gate for CAR-T cell activation and restricted the activity in a dual-targeting manner. Instead of selecting double-positive targets, split CARs can inactivate T cells when they recognize proteins in normal cells. This CAR design consists of two split subunits, Signal-CAR and Scissors-CAR. Signal-CAR recognizes one protein in tumor cells and activates CAR-T cells to perform cell-killing functions. However, when Scissors-CAR recognizes another protein that is mainly expressed in normal cells, the protease domain in Scissors-CAR is activated, cleaving the peptide sequence between the co-stimulatory domain and CD3ζ domain in Signal-CAR for inactivation.22 The split CARs described above would improve the safety profile of CAR-T cell therapy by enhancing its specificity.
Switchable and tunable control over CAR function.
Continuous activation of CAR-T cells in the presence of antigens is one of the factors contributing to severe cytokine release syndrome. After recognizing tumor antigens, CAR-T cells secrete inflammatory cytokines and induce tumor cell death, during which a large number of damage-associated molecular pattern molecules (DAMPs) are released to further stimulate macrophages for cytokine production.23 Persistent antigen stimulation may also result in the exhaustion of CAR-T cells, causing them to lose effector function and develop defective memory formation.24 Splittable systems provide alternatives to address the aforementioned challenges caused by overactivation.
The antigen recognition and signal transduction of CARs can be dissociated, and are regulated temporally and spatially by biological orthogonal pairs or CIDs. Various switches tune the CAR-T cells from “ON” to “OFF” status to avoid continuous activation. For example, the constitutive antigen-dependent activation of T cells was circumvented in a design called split, universal, and programmable (SUPRA) CAR.25,26 The SUPRA CAR incorporating leucine zippers for split CARs was based on the interaction between the EE-leucine zipper and the RR-leucine zipper. The EE-leucine zipper was fused to the scFv for antigen targeting. After EE-leucine zipper-scFv is bound to the tumor antigens, zipCAR-T cells expressing a recombinant motif consisting of RR-leucine zipper and intracellular signaling domains can recognize EE-leucine zipper and activate zipCAR-T cells (Fig. 2a). The antitumor efficacy of SUPRA CAR was evaluated in vitro and in vivo. This study further investigated how cytokine secretion and killing capability were affected by changing four parameters: scFv affinity, zipper pair affinity in between, zipper-scFv concentration, and zipCAR expression. While high-affinity zipper-scFv often resulted in overactivation, the maximum level of cytokine secretion and killing ability was correlated with zipper pair affinity. In addition, zipCAR-T activity can be controlled by adding competitive zipFvs or redirected by adding zipFvs that target different antigens. Adjustable dosing of zipFvs is amenable to regulating and controlling the overactivation of CAR-T cells. In addition, SUPRA CAR could be applied to many other cells, including Th1, Th2, Treg, γδ T cells, NK cells, and macrophages, for different functions in cell therapy.27 A NOT gate and three-input (A AND B) AND NOT C logic were developed through rational design, enabling more complex computations in immune cells with increased versatility.
 |
| Fig. 2 Split-CAR designs for immunotherapy. (a) Schematic illustration of zipCAR-T. The heatmap indicates cytokine release at varying parameters. Reproduced from ref. 25 with permission from Elsevier, copyright 2018. (b) Schematic illustration of SpyTag and SpyCatcher in CAR-T cell design. Reproduced from ref. 6 with permission from Xuan Liu et al., copyright 2020. (c) Small molecule-induced, titratable tumor killing of split CAR. Reproduced from ref. 34 with permission from The American Association for the Advancement of Science, copyright 2015. Reproduced with permission (d) Safety switch for irreversible CAR-T deactivation. Reproduced from ref. 35 with permission from Gargett and Brown, copyright 2018. Reproduced from ref. 36 with permission from Elsevier, copyright 2021. | |
Other biological orthogonal pairs for switch-mediated activation and retargeting include 14-amino acid PNE sequence and high-affinity antibody against PNE, SpyTag, and SpyCatcher.28,29 The pair SpyTag and SpyCatcher interact via an isopeptide bond; moreover, this pair has been used for antibody discovery in phage display and have been explored for splitting CARs.30,31 The distinct high affinity of pairs to their counterparts elicits more precise tuning of cytokine. For example, SpyCatcher CAR-T cells can bind to SpyTag fused with scFv, which recognizes antigens6 (Fig. 2b). The pair showed a significant killing effect on hGPC3+ cells in the presence of anti-hGPC3 scFv-SpyTag. While maintaining cytotoxicity against target cells comparable to conventional CAR-T cells, only about 20% of CAR-T cells that produce IFN-γ were detected in vitro. The potency of SpyCatcher CAR-T cells and cytokine release could be tuned by changing the concentration of anti-hGPC3 scFv-SpyTag. As demonstrated in the in vivo study, cytokine release, including IFN-γ, IL-2, and IL-6, was reduced. Interestingly, it is postulated that the binding intensity between CAR-T cells and cancer cells regulates CAR-T functions. In a similar study, the in vitro lytic capability of SpyCatcher CAR-T cells was dependent on the dose of scFv-SpyTag.32 And this universal platform of SpyTag and SpyCatcher CAR-T cells can redirect CAR-T cells by administering different scFv-SpyTags without reengineering CAR-T cells in vivo.
Applying biological orthogonal pairs can benefit from the high affinity between these pairs, indicating a better therapeutic index of the original therapeutic agents as a relatively lower dose of CAR-T cells might be sufficient to elicit killing functions. However, the killing capability of CAR-T cells can be compromised especially when there are low effector-to-T cell ratios. Moreover, as Spytag and Spycatcher are derived from the bacteria strain Streptococcus pyogenes, the potential immune response after administration and the requirement of continued dosing for prolonged CAR-T cell function may limit the translation of some pairs in split approaches. There are also concerns about the auto-aggregation of recombinant scFvs, which may lead to the exhaustion of CAR-T cells, even though no aggregation of SpyCatcher was observed in the studies mentioned above. Nevertheless, because of the optimal performance of the SpyCatcher CAR, this split system has also been applied in CAR-NK cell immunotherapy.33
CARs can also be divided at the intracellular domains and combined with CID for switchable control over functions. CAR subunits can reassemble in the presence of small molecules such as rapamycin to reconnect the co-stimulatory domain and CD3ζ for the following signaling transduction after antigen recognition. While the activation of T cells is still dependent on antigen recognition, the rapamycin-controlled binding of FKPB12 and FRB provided an additional requirement and control for CAR-T cell activation, as the concentration of small molecules correlated with the tumor-killing effect34 (Fig. 2c). Also, the inducible dimerization of CARs did not affect the cytotoxicity kinetics of CAR-T cells. By changing and tailoring the dosing of small molecules after CAR-T cell infusion, the continuous activation of CAR-T cells is abrogated by suspending small-molecule administration, whereas, robust CAR-T cell activity can be reversed. To minimize the immunosuppressive activity of rapamycin and prevent the impact of endogenous FKBP12, FKPB12 and FRB domains can be inserted into the extracellular domain to decrease the dose of rapamycin for regulation.37 In this design, the antigen-targeting domains included CD19-targeting scFv, FKPB12, and the CD4 transmembrane domain. And the activation domain is composed of the FRB domain, CD8α transmembrane domain, and signaling domains of 4-1BB and CD3ζ. Interestingly, adding scFv-FKBP12 that targets another antigen (B-cell maturation antigen, BCMA but not CD19) after preincubation with rapamycin following anti-CD19 CAR-T therapy led to the recognition and killing of BCMA+ CD19− cells. In this way, the targeting reactivity of CAR-T cells is expanded towards a second antigen without the need to re-engineer the infused CAR-T cells. In another study, FKBP-FRB pairs were fused with Caspase-9 to trigger cell death in CAR-T cells and prevent overactivation35,36 (Fig. 2d). These switches in conjugation with other functional biomolecules explore the versatility of split systems.1
In addition to small-molecule-mediated CID, CAR-T split designs can also be light-inducible for transcriptional and translational regulation. For instance, blue light triggers the unfolding of the Jα helix of the light–oxygen–voltage-sensing (LOV2) domain to expose the nuclear localization sequence and translocate LexA-CIB1 from the cytoplasm to the nucleus. Thus, the LexA-CIB1 and CRY2-VPR complex localized to the LexA BS region in the plasmid and activated the transcription of anti-CD19 CAR. Because of the fast dissociation of CRY2-CIB1 with a half-life of approximately 5.5 minutes, the optical control of anti-CD19 CAR expression is dependent on the turnover of CAR and is reversible with high spatial resolution.38 This compatible and noninvasive method provides temporal control over CAR-T activity for tumor killing and enables on-demand activation.
The field of cell immunotherapy, particularly CAR-T treatment, has shown great success. One research strategy for increasing the overall safety and efficacy of CAR-T therapy is to divide the CAR design into distinct modules. CAR-T supports Boolean logic operations (AND gate, OR gate, NOT gate, etc.), and more “off-the-shelf” designs have been developed. The use of inducible and inhibitory CARs has allowed for greater flexibility and control over functions. However, additional efforts are needed to overcome the constraints and challenges posed by the tumor microenvironment and heterogenicity in solid tumors.
3.1.2 Bispecific antibody.
Bispecific antibodies are regarded as a new type of immunotherapy that helps prime the immune system against cancer. Tumor-specific antigens suitable for targeting purposes are rare. A split strategy can be applied to antibody therapy to obtain better specificity, addressing the limitations of antigen expression in normal cells. Banaszek et al. designed a tri-specific antibody split into two domains that can align and reconstitute to activate CD3-positive T lymphocytes for tumor cell lysis with improved specificity.39 The split system addressed the challenge of distinguishing healthy tissues that are human leukocyte antigens (HLA) A2 positive from diseased hematopoiesis which is HLA-A2 and CD45 positive. In their design, the single-chain variable fragment (scFv) of anti-HLA-A2 was fused to the variable light (VL) chain domain of an anti-CD3 antibody, whereas the scFv of anti-CD45 was fused to the variable heavy (VH) chain domain of the same anti-CD3 antibody (Fig. 3). While having negligible binding via VH/VL interaction at μM concentration, the split two fragments can resemble each other only when binding to their target antigens on the same cell surface, achieving dual antigen-restricted V-fragment complementation. Tri-specific antibodies have achieved antitumor efficacy comparable to bispecific antibodies in AML but maintained low adverse bystander toxicity in vivo. The antitumor effect was further evaluated in breast cancer, showing promising applications in solid tumors. By applying the split system in antibody design, the choice of antigens is not limited to tumor-associated antigens with an ideal distribution. For bispecific antibody production, an intein-based protein splicing system helps to overcome the issues of light/heavy chain mispairing.7 For example, two antibody fragments can be expressed separately in mammalian cells, and the trans-splicing of intein recombines the fragments to form bispecific antibodies.40 The generated antibody has a natural human IgG architecture with fewer chain mispairings. It has also been further adapted for automatic antibody reconstitution at a high-throughput screening level.41
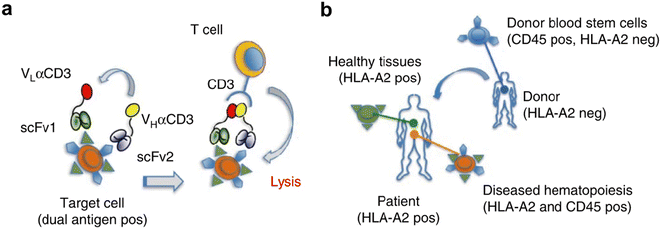 |
| Fig. 3 Schematic illustration of a dual-targeting antibody for T cell engaging. (a) Reconstitution of bi-specific antibody on target cells to engage T cells. (b) Split antibody spares single antigen-positive cells from double antigen-positive hematopoietic cells in an allogeneic mismatch transplantation model. Reproduced from ref. 39 with permission from Shuyu Huang at al., copyright 2020. | |
3.2 Gene therapy and synthetic biology
Several split systems have been designed and used for monitoring protein–protein interactions, genome engineering, and controlling gene transcription and translation in living cells. A typical idea in the field of synthetic biology is to create gene circuits in which the transcriptional regulation of gene expression is controlled by split regulators. Split regulators typically comprise of DNA a binding domain and a regulatory domain that can be fused to complementary pairs. While the focus of synthetic gene regulation has been put in the transcription process of gene of interest, increasing efforts have also been made to examine novel approaches to translation and protein degradation.42 The subsequent sections detail how the split principle has been implemented in diverse applications, with a focus on gene editing and tool development in the field of synthetic biology.
3.2.1 Split Cas9.
The purposes of splitting Cas9 include improving the therapeutic index of CRISPR technology, enhancing the compatibility of Cas9 with virus vectors, and enabling temporal and spatial precision control. Currently, the CRISPR/Cas system is generally delivered using an adeno-associated virus (AAV), which is the most frequently used delivery system for gene therapy.43 The maximum payload of the AAV vector restricts the size of the gene delivered, limiting the application of some CRISPR/Cas systems. The size of Cas9 is about 3–4.2 kb, depending on their various strains, and the size of which after combining with the necessary promoters and other components will reach up to 5 kb.44 Simply splitting the components of the CRISPR/Cas system, especially Cas9, will enhance compatibility with delivery virus vectors by diminishing their size. Upon delivery of each component into cells, it can reconstruct its full-length function and overcome the limitations of the loading capacity of the virus.
The feasibility of splitting Cas9 is based on its structure consisting of two lobes: a recognition lobe (REC) and a nuclease lobe (NUC).45 Conformational rearrangement of the two lobes through interaction with nucleic acids provides the foundation for splitting Cas9. The combined use of CID, light-induced dimerization pairs, and other biomolecules has expanded the scope of CRISPR/Cas9 applications. One split-Cas9 system takes advantage of FKBP and FRB pairs, as discussed in the previous sections. The two split fragments of Cas9 were fused to FKBP and FRB domains, and rapamycin regulated the reconstitution of separate Cas9 fragments.46 The most active version of split-Cas9 was selected from among 11 potential sites. At approximately the same time, two other studies have applied a split strategy for the Cas9 system. Similarly, Truong et al. chose the same split site, but split-Cas9 was combined with the intein system. The design includes the N-terminal DNA polymerase III DnaE fused to the N-intein and C-termini DnaE fused to C-intein47 (Fig. 4a). The split site of DnaE was carefully selected between Glu573 and Cys574 to generate inactive fragments. The ligated recombinant Cas9 recovered its wild-type function by leveraging the recognition and self-splicing of inteins. Gene editing efficiency and targeted gene editing were restored, which was comparable to that of wild-type Cas9. The intein-mediated split-Cas9 could be successfully delivered by AAV, and demonstrated maintenance of activity with an even higher level than that delivered by a single AAV.
 |
| Fig. 4 Split-Cas9 systems for gene therapy. (a) Split intein-mediated reassemble of two DnaE fragments via trans-splicing reaction. Reproduced from ref. 47 with permission from Oxford University Press, copyright 2015. (b) Schematic illustration of reconstitution and transportation of Cas9 into the nucleus upon addition of 4-hydroxytamoxifen (4OHT). Reproduced from ref. 50 with permission from Springer Nature, copyright 2016. (c) Far-red light-controlled split-Cas9 system. Far-red light (∼730 nm) triggers the transcription and translation of Cas9(N)-Coh2, which will bind with its constitutively expressed counterpart. Reproduced from ref. 51 with permission from Yuanhuan Yu et al., copyright 2020. | |
However, the activation of Cas9 was irreversible, as replacing the treatment medium after 2 h of treatment led to continuous activation. Other problems also exist. Wright et al. split Cas9 into the NUC lobe and the α-helical lobe in efforts of reassembling when both are recognized by the sgRNA/DNA complex.48 Although the in vitro gene editing function of reassembled Cas9 was verified in this study, the efficiency of split-Cas9 without further modification was significantly lower, with 0.6% to 2% indel efficiency compared to 22% of WT Cas9 targeting the EMX1 locus in HEK-293T cells. This indicates that the limited affinity after splitting and lower kinetics of DNA cleavage are potential obstacles when designing split Cas9. Also, moderate levels of background activity can be detected in the absence of an inducing agent.46,48,49 Constitutive expression of Cas9 also contributes to the undesired outcomes such as off-target effect, which remains to be resolved.46 Accordingly, optimization of the split system has strived to address the problems of compromised robustness; however, more importantly, these systems have achieved higher precision and specificity.
Advances in novel bioengineering technologies have promoted the development of inducible split-Cas9 for in vivo activation. The estrogen receptor (ER) ligand-binding domain, which binds to Hsp90, was applied for the cytoplasmic localization of split Cas9 fragments fused to achieve spatial control.50 By adding the synthetic ligand 4-hydroxytamoxifen (4OHT), the release of Hsp90 triggers nuclear translocation of both Cas9 fragments, enabling the formation of a reassembled Cas9-sgRNA complex in the nucleus. This modification and spatial regulation of split-Cas9 decreased background activity without 4OHT, thus improving performance (Fig. 4b). Furthermore, reversible and remote regulation of split-Cas9 CRISPR has been developed using far-red light to control the transcription and translation of a split-Cas9 fragment.51 The split pairs used in this study were Coh2 and DocS derived from Clostridium thermocellum fused to N-terminal Cas9 and C-terminal Cas9, respectively, which were encoded in DNA. DocS-Cas9 (C) is regulated by the human cytomegalovirus promoter (PhCMV) for constitutive expression. Far-red light regulated the transcription of Cas9(N)-Coh2 by activating bacteriophytochromes (BphS) to synthesize cyclic-di-GMP for the translocation of the regulator p65-VP64-BldD into the nucleus, inciting the activation of the far-red light-inducible (FRL) promoter (Fig. 4c). After expression, the high-affinity interaction between Cho2 and DocS facilitated the reconstitution of the nuclease-active form of Cas9. The gene editing effect was reversed after shifting the transfected cells to the dark, and there was a minimum off-target effect. Due to the experimental design, the long period between the two tests for gene editing efficiency may allow the turnover of related proteins within the cell. Nevertheless, the results still support a reusable split-Cas9 system. The split-Cas9 has also been explored and designed into a synthetic AND-logic gate and used as a biosensor to detect cellular events, such as phenotype transition and cell–cell fusion.52 Efforts have been made to optimize CRISPR/Cas9 for therapeutic applications in mammalian cells.53
3.2.2 Split RNA polymerase.
The possibility of split T7 RNA polymerase was found during the purification process when the site between amino acids 179 and 180 in the H-loop domain was nicked. Disrupting the refolding of the H-loop domain after T7 RNAP binding to the promoter abrogated its ability to synthesize full-length RNA. Accordingly, the C-terminal and N-terminal fragments of T7 RNAP have been developed.54 Binding between two subunits does not require additional binding pairs for functional restoration. Fused to points of interest separately, split T7 RNA polymerase was developed into a versatile biosensor platform in mammalian cells and was able to control the transcription process.55–57
3.2.3 Split-Cre.
Cre is one of the most extensively developed agents in gene therapy and synthetic biology. The ability of Cre recombinase to catalyze the site-specific recombination of DNA has been utilized for many biological applications in prokaryotic and eukaryotic cells. Cre has been split into two segments, which were able to dimerize, rendering the regulation over recombination activity.58 To develop more biologically relevant systems, the two fragments of Cre can be fused with other moieties. Split-Cre recombinase has been used to investigate the protein–protein interactions in bacterial cells as well as controllable genome engineering in vivo.8,59
3.2.4 Split-protease.
Two proteases have been investigated for splitting: tobacco etch virus (TEV) protease and human rhinovirus 3C (HRV 3C) protease.60,61 N-TEV(1–118)/C-TEV(119–242) pairs of TEV proteases were used first for protein–protein interactions and were later used for the design of fast proteolysis-based signaling and logic circuits in mammalian cells.4,60 Considering the crucial role of proteases in various biological processes, programmable protease-based systems have been developed for therapeutic protein secretion.62
3.3 Prodrug activation and targeted drug delivery
The purpose of using prodrugs as therapeutic agents is to minimize side effects when the drug is in normal cells by remaining nonactive and nontoxic. However, the difference between target and non-target cells is usually not significant enough to have ideal outcomes and leads to failure in preclinical or clinical studies due to premature activation of the prodrug. In this case, the split system provides a compensation strategy to achieve targeted delivery. There are several ways to achieve prodrug activation in split systems.
3.3.1 Split enzyme for prodrug activation.
Enzymes required to metabolize the prodrug into the active form or uncage small molecules in situ can be split. For example, Nervig et al. employed split β-lactamase for prodrug cleavage activation, which could potentially diminish the systemic side effect2 (Fig. 5a). Split β-lactamase segments were fused using two anti-HER2 (human epidermal growth factor receptor 2) antibody binders. The binding epitopes of the two binders were different, indicating that the two segments can be simultaneously displayed on HER2. The positions of the two binding sites allow the correct orientation, facilitating the refolding of β-lactamase for proximity-dependent gain-of-function. The cytotoxicity of this strategy was tested in an in vitro model using a high-HER2-expressing cancer cell line. Compared to the intact enzyme, split β-lactamase fused to HER2 binders showed a comparable IC50 and increased the toxicity to 7-fold over the prodrug treatment alone.
 |
| Fig. 5 “Tag-and-play” in split systems for prodrug activation. (a) Split β-lactamase catalyzes prodrug activation on HER2-positive cancer cells. Reproduced from ref. 2 with permission from American Chemical Society, copyright 2022. (b) In situ uncaging of trans-cyclooctene (TCO) protected antibiotic. Reproduced from ref. 65 with permission from American Chemical Society, copyright 2018. (c) tumor-targeting and membrane inserting ligand for redirection of CAR-T cells against solid tumors. The endogenous T cells are infiltrated in the tumor and involved in the anti-tumor immune response. Reproduced from ref. 67 with permission from Springer Nature, copyright 2023. | |
3.3.2 Tag-and-play strategy.
This idea of “tag-and-play” has been demonstrated in many treatment strategies, ranging from small molecules like chemical prodrugs to antibodies and cell therapy, as discussed briefly in section 3.1.1. Based on the activation of chemical prodrugs via an enzyme-mediated process, the targeting property of prodrugs has been enhanced by utilizing antibody-directed enzyme to initiate a two-stage system.63 The amplification of specificity overcomes the concerns of the cross-targeting of normal cells in antibody-directed enzyme prodrug therapy (ADEPT).64 To activate the systemically delivered prodrug, the capturing agents can also be locally injected. For example, biocompatible materials modified with bio-orthogonal chemical groups have been utilized for click-activated prodrugs in antibiotic treatment and chemotherapy65,66 (Fig. 5b). The concept of tag-and-play for CAR-T therapy in cancer treatment could potentially address antigenic heterogeneity by introducing artificial antigens for CAR-T cell targeting. Similarly, other tagging agents include amphiphilic poly (ethylene glycol) lipids which are specifically inserted into the tumor cell membrane to introduce a new target (FITC). Anti-FITC CAR-T cells can then preferentially recognize artificial antigens and elicit selective cytotoxicity toward cancer cells67 (Fig. 5c). Importantly, this study discusses the mechanism by which de novo endogenous T cells are actively involved in the immune response following CAR-T therapy. Metabolic glycan labeling of cancer is another modification method to introduce tags for CAR-T cells to target different types of cancer.68
3.4 Cytokine monotherapy
Therapeutic recombinant cytokines including IL-2, IL-11, TNF, and others have been approved for various immune-related diseases, but the dose-dependent side effects by activation of immune cells still limit their clinical use.69–72 Some technologies have been applied such as directed evolution that introduces mutations to increase the affinity of therapeutic cytokines to their receptor.73,74 PEGylation of cytokine molecules can also optimize the pharmacokinetics property of cytokines with a longer circulation half-life and diminished immunogenicity or even lead to sustained activation of regulatory T cells.75 Nevertheless, PEGylation failed to reduce the toxicity of IL-2.76 Antibody-cytokine fusion proteins, also called immuno-cytokines, have also been developed to promote cytokine infiltration at disease sites.77 However, due to their pleiotropic nature and broad distribution of receptors, the regulation of systemic cytotoxicity of therapeutic cytokines was still demanded.
To improve the therapeutic index of therapeutic cytokines, a splitting strategy has been applied to selectively generate the activity of cytokines on demand in the disease sites but not when they are in circulation. The proof-of-concept study was done by Venetz et al., in which an IL-12 subunit p35S (C92S mutation) was fused to the bivalent single-chain variable fragment (scFv) against the fibronectin, facilitating its accumulation on tumor neovasculature.78 The counterpart subunit p40S (C197S mutation) was associated with p35S and formed a heterodimeric IL-12 moiety, inducing in vitro IFN-γ production in freshly isolated mouse splenocytes. However, the single p35S subunit alone remained a partial function of IL-12 activity, limiting the further application of split IL-12 for the in situ cytokine reassembly. Besides IL-12, other cytokines including IL-6 and IL-11 have also applied similar principles of split system for split-cytokine-precursors that have demonstrated the feasibility and robustness of this concept.79 Selective mimics of IL-2 and IL-15 have been generated by Silva et al. based on the crystal structures called neoleukin-2/15 (Neo-2/15), which showed a superior therapeutic activity to IL-2.80
The therapeutic relevance of this spit-cytokine strategy was evaluated in the following study, in which they generated a controlled and conditionally activated mimetic of IL-2/IL-15 to optimize the activation of immune cells by multiple selectivity.81 The activation of immune cells was achieved through two methods, trans-activation and cis-activation by fusing split interleukin-2/15 (Neo-2/15), which stimulates the IL-2Rβ and IL-2Rγ to different targeting domains. In trans-activation, two fragments (Neo2A and Neo2B) have been fused to tumor-antigen targeting domains against EGFR and HER2 respectively (Neo2A-aEGFR and Neo2B-aHER2) (Fig. 6a). After the binding of two domains to their targets on HER2+/EGFR+ cells, colocalization of Neo2A and Neo2B elicit an AND-logic gate activity for effectively recruiting IL-2 Rβ and IL-2Rγ for trans-activation of NK cells. This trans-activation is dependent on the surface density of split Neo2A and Neo2B. Different from binding to tumor cells and recruiting immune cells through IL-2/receptor interaction, in cis-activation, two fragments (Neo2A-aCD8 and Neo2B-aCD8) were selectively targeting CD8+ T cells and the complemented Neo-2/15 directly activate the IL-2 receptor on the same recognized CD8+T cell for subsequent proliferation and tumor killing function. Administration of split Neo-2/15 through subcutaneous injection in the opposite flank of tumor-implanted mice has successfully demonstrated robust anti-tumor efficacy and significantly reduced the toxicity.
 |
| Fig. 6 Splittable cytokines for conditional immunotherapy. (a) trans-Activation of T cells by targeting single or dual antigens. Reproduced from ref. 81 with permission from Springer Nature, copyright 2022. (b) Schematic illustration of chain-exchange mechanism for generating therapeutic IL-4. Reproduced from ref. 82 with permission from Steffen Dickopf et al., copyright 2022. | |
The function of in situ recombinant therapeutic cytokines can be further controlled by proximity-induced chain exchange on target cells. Vasic et al. developed a PACE (Prodrug-Activating Chain Exchange) system and was applied for conditionally active IL-4 prodrug.82 Similarly, IL-4, consisting of four alpha helices was split into two fragments, and the separated helices are denoted as A and BCD, which were further fused to the C-termini of TriFabs. TriFabs are a type of CH3-dimer capable of exchanging their heavy chains. When the two entities are in close proximity, they convert from destabilized CH3-dimers into stable CH3–CH3 products.83 Either mutation in helix A or helix C was introduced to the recombinant protein respectively (denoted as purple color in Fig. 6b), generating two reactants. The exchange of a heavy chain of two reactants, when they recognized the same target cells via binding domains against the tumor-associated antigen (TAA), mediated the activation of IL-4 without mutations (denoted as Product 1). However, another product containing mutated IL-4 showed no activity and is removed from the cells due to a lack of TAA binding domains. By changing the binding domains on recombinant protein against different TAAs, a TAA-mediated targeting and activation of IL-4 prodrug was achieved, eliciting robust activation of IL-4 receptors on targeted cells and neighboring cells. Nevertheless, it is innovative to apply the prodrug concept in developing a new antibody-cytokine system, and evaluation of in vivo treatment efficacy and their performance in reducing side effects is necessary. The therapeutic window between on-target activation and premature activation necessitates further optimization. Moreover, the fate of cleared inactive and mutated side products after change exchange and recombination within circulation should be investigated.
3.5 Biosensing
3.5.1 Split fluorophore.
Split fluorescent proteins provide the foundation for the development of biosensors. Currently, there are a variety of fluorescent proteins with different excitation/emission maxima, offering flexible and diverse choices.84 By fusing with target protein or coupled with other split pairs, they have demonstrated broad applications in protein labeling,85,86 protein visualization,87 detection of protein delivery, and other biological processes,88–90 generally working as a reporter.3
3.5.2 Split aptamers.
Split aptamers are two or more short nucleic acid strands that can regain the binding ability with high specificity and affinity after reconstitution. These split aptamers are usually small, usually from 10 to 50 nucleotides.91 Currently, only a few split aptamers exist, including thrombin, adenosine, ATP, and cocaine split aptamers.92–96 To develop split aptamers, the parent aptamers are required to have suitable original structures that are easy to divide, such as stem region.97 After splitting, neither of the aptamer fragments can bind to the target protein alone but the binding ability is restored after reassembly. For example, in the presence of thrombin, the split aptamers will form the G-quadruplex structure, losing or gaining the conformation-based functions, such as the Förster resonance energy transfer (FRET) phenomenon.92 Most applications of split aptamers are in the field of biosensors. Various strategies based on the split aptamers have been brought up to detect proteins or small molecules.91 There are ongoing efforts to further increase the specificity and sensitivity of these biosensors.
3.5.3 Split DNAzyems.
DNAzymes or deoxyribozymes, synthetic DNA oligonucleotides that can catalyze certain reactions, can be split into multi-components that are sometimes called MNAzymes (Multicomponent Nucleic Acid enzymes).98 They have also shown great potential as a therapeutic intervention involved in chemotherapy, gene therapy, and cancer immunotherapy against different diseases.99,100 Similar to split aptamers, the applications of split DNAzymes are focused on developing biosensors for protein detection and RNA detection.101–103
3.6 Other applications
Split systems have been explored for intracellular applications, such as split toxins for selective cell inhibition and conditional activation of immune cells. The reassembly and complementation always occur on the cell surface in these designs. It can also be programmed to cross cell membranes and function in cytoplasm. For example, split Pseudomonas exotoxin A (PE3) that can inhibit cell synthesis and cause cell death by ADP-ribosylation of eukaryotic elongation factor 2 (eEF2) after complementation has been restructured into a split toxin system.104 It showed the cell inhibition effect in HEK-293 cells after transfection comparably as intact PE3. The intracellular complementation of split PE3 takes place in the cytoplasm; however, the delivery approach is still reliant on cell transfection which lacks selectivity. In another study, a similar split toxin system consisting of diphtheria toxin (DT) catalytic domain (DTA) as a split-effector was developed by using two different delivery machineries.105 One fragment encoded the N-terminal split DTA (toxinN) in a gene was delivered via transfection or viral transduction. Another fragment containing C-terminal split DTA (toxinC) was designed to enter the cell cytoplasm through the anthrax lethal toxin system (LeTx). The targeting and selectivity of the second delivery route are due to the targeting of modified pore-forming protective antigen (PA) towards HER2, EGFR, and TEM8, which are all overexpressed oncoproteins that can serve as targeting markers. By leveraging the translocation-priming lethal factor N-terminal fragment (LFN) that binds to the PAs, the translocation of fragments fused with LFN can be translocated to the endosomes.
4. Challenges and future perspectives
The broad applications of splittable systems range from investigating basic biological processes at an early stage of drug discovery, to optimizing therapeutics for translational purposes. We have discussed the advantages of utilizing splittable strategies in numerous scenarios, either to enhance the targeting specificity or provide a controllable handle. However, the challenges of premature assembly of split subunits lead to high background signals and the off-target effect, compromising the utilization of split systems for precision medicine. Further optimization is required to increase the stability of fragments and prevent potential self-aggregation within single fragments. Moreover, efforts should also be attributed into to evaluating the immunogenicity of current splitting systems and fully investigating the safety profile of available methods. To discover more splittable agents, a better understanding of the function-structure relationship is necessary. Computational techniques are advancing the development of protein design and engineering, particularly for selecting splitting sites, improving the solubility, and identifying binding pairs. Protein–protein interaction prediction and modeling are important in the development of splittable drug delivery systems. In recent years, deep learning algorithms like AlphaFold have revolutionized this procedure by providing atomic-level accurate prediction without known similar structures.106 The predication of protein interaction sites and folding patterns is vital for identifying optimal split sites and ensuring effective reassembly under physiological conditions.107 Prediction algorithms that are highly customized for specific splittable protein types have also been used to produce more efficient modeling and docking structure predictions. For example, automated tools were developed for SPELL (split proteins regulated by a ligand or by light) to predict these sites with minimal spontaneous assembly.13 Although accurate structural information is needed, traditional biomolecular modeling algorithms could provide more reliable outcomes, which are predictions of the behavior and stability of protein components before they are reassembled in vivo, compared to deep learning algorithms. The toolbox of splitting systems for more complex bio functions can be fulfilled by discovering more dimerization domains and conditional inducers. Systemic approaches for split system optimization via computational design advance their applications. Advanced geometric and network concepts can contribute to the effective design of splittable systems. For example, Ricci Curvature-based algorithms, including Ollivier-Ricci Curvature and Forman-Ricci Curvature, can predict interactions of protein complexes and protein-inorganic nanoparticles with high-performance accuracy.108,109 Those machine learning algorithms have provided powerful tools for system biology and synthetic biology and can boost the novel designing of splittable systems. Through those computation-guided optimizations, it is possible to understand and interpret why specific mutations work while the rest cannot, thus transferring these generalizable rules for tuning other split systems. For multi-component systems, determining how each component impacts the output of whole systems will help guide the tuning of functions in real situations. Moving forward, the novelty should not rely on the complexity of the system rather more focus should be put on their translational potential and clinic relevance.
Data availability
No primary research results, software or code have been included and no new data were generated or analysed as part of this review.
Conflicts of interest
We declare no conflict of interest.
Acknowledgements
This work was supported, in part, by METAVIVOR Foundation Early Career Research Grant Award, American Cancer Society Research Scholar Grant (grant number: RSG-23-1140821-01-ET), the University of Wisconsin Carbone Cancer Center Research Collaborative and the Pancreas Cancer Task Force, and the start-up package from the University of Wisconsin–Madison.
References
- L. Bouquet, E. Bôle-Richard, W. Warda, M. Neto Da Rocha, R. Trad, C. Nicod, R. Haderbache, D. Genin, C. Ferrand and M. Deschamps, Gene Ther., 2023, 30, 706–713 CrossRef CAS PubMed
.
- C. S. Nervig, S. T. Hatch and S. C. Owen, ACS Med. Chem. Lett., 2022, 13, 1769–1775 CrossRef CAS PubMed
.
- S. K. Elledge, X. X. Zhou, J. R. Byrnes, A. J. Martinko, I. Lui, K. Pance, S. A. Lim, J. E. Glasgow, A. A. Glasgow, K. Turcios, N. S. Iyer, L. Torres, M. J. Peluso, T. J. Henrich, T. T. Wang, C. M. Tato, K. K. Leung, B. Greenhouse and J. A. Wells, Nat. Biotechnol., 2021, 39, 928–935 CrossRef CAS PubMed
.
- T. Fink, J. Lonzarić, A. Praznik, T. Plaper, E. Merljak, K. Leben, N. Jerala, T. Lebar, Ž. Strmšek, F. Lapenta, M. Benčina and R. Jerala, Nat. Chem. Biol., 2019, 15, 115–122 CrossRef CAS PubMed
.
- X. He, Z. Feng, J. Ma, S. Ling, Y. Cao, B. Gurung, Y. Wu, B. W. Katona, K. P. O'Dwyer, D. L. Siegel, C. H. June and X. Hua, Blood, 2020, 135, 713–723 CrossRef PubMed
.
- X. Liu, J. Wen, H. Yi, X. Hou, Y. Yin, G. Ye, X. Wu and X. Jiang, Ther. Adv. Med. Oncol., 2020, 12, 1–16 CAS
.
- R. Yamada, I. Nakahara, I. Kumagai, R. Asano, T. Nakanishi and K. Makabe, Sci. Rep., 2023, 13, 15961 CrossRef CAS PubMed
.
- N. Jullien, F. Sampieri, A. Enjalbert and J. Herman, Nucleic Acids Res., 2003, 31, e131 CrossRef PubMed
.
- K. Camacho-Soto, J. Castillo-Montoya, B. Tye, L. O. Ogunleye and I. Ghosh, J. Am. Chem. Soc., 2014, 136, 17078–17086 CrossRef CAS PubMed
.
-
S. A. Lim and J. A. Wells, in Methods in Enzymology, ed. D. S. Tawfik, Academic Press, 2020, vol. 644, pp. 275–296 Search PubMed
.
- K. Zhu, H. Su, Z. Peng and J. Yang, Bioinformatics, 2023, 39, 1–7 CrossRef
.
- A. S. Dixon, M. K. Schwinn, M. P. Hall, K. Zimmerman, P. Otto, T. H. Lubben, B. L. Butler, B. F. Binkowski, T. Machleidt, T. A. Kirkland, M. G. Wood, C. T. Eggers, L. P. Encell and K. V. Wood, ACS Chem. Biol., 2016, 11, 400–408 CrossRef CAS PubMed
.
- O. Dagliyan, A. Krokhotin, I. Ozkan-Dagliyan, A. Deiters, C. J. Der, K. M. Hahn and N. V. Dokholyan, Nat. Commun., 2018, 9, 4042 CrossRef PubMed
.
- T. B. Dolberg, A. T. Meger, J. D. Boucher, W. K. Corcoran, E. E. Schauer, A. N. Prybutok, S. Raman and J. N. Leonard, Nat. Chem. Biol., 2021, 17, 531–539 CrossRef CAS PubMed
.
- D. M. Spencer, T. J. Wandless, S. L. Schreiber and G. R. Crabtree, Science, 1993, 262, 1019–1024 CrossRef CAS PubMed
.
- R. DeRose, T. Miyamoto and T. Inoue, Pflugers Arch., 2013, 465, 409–417 CrossRef CAS PubMed
.
- L. Klewer and Y. Wu, Chemistry, 2019, 25, 12452–12463 CrossRef CAS PubMed
.
- D. Hatlem, T. Trunk, D. Linke and J. C. Leo, Int. J. Mol. Sci., 2019, 20, 2129 CrossRef CAS PubMed
.
- N. H. Shah and T. W. Muir, Chem. Sci., 2014, 5, 446–461 RSC
.
- C. C. Kloss, M. Condomines, M. Cartellieri, M. Bachmann and M. Sadelain, Nat. Biotechnol., 2013, 31, 71–75 CrossRef CAS PubMed
.
- A. M. Tousley, M. C. Rotiroti, L. Labanieh, L. W. Rysavy, W.-J. Kim, C. Lareau, E. Sotillo, E. W. Weber, S. P. Rietberg, G. N. Dalton, Y. Yin, D. Klysz, P. Xu, E. L. de la Serna, A. R. Dunn, A. T. Satpathy, C. L. Mackall and R. G. Majzner, Nature, 2023, 615, 507–516 CrossRef CAS PubMed
.
- S. Aoyama, S. Yasuda, H. Li, D. Watanabe, Y. Umezawa, K. Okada, A. Nogami, O. Miura and N. Kawamata, Int. J. Mol. Med., 2022, 49, 1–11 Search PubMed
.
- X. Xiao, S. Huang, S. Chen, Y. Wang, Q. Sun, X. Xu and Y. Li, J. Exp. Clin. Cancer Res., 2021, 40, 367 CrossRef CAS PubMed
.
- M. Poorebrahim, J. Melief, Y. Pico de Coaña, S. L. Wickström, A. Cid-Arregui and R. Kiessling, Oncogene, 2021, 40, 421–435 CrossRef CAS PubMed
.
- J. H. Cho, J. J. Collins and W. W. Wong, Cell, 2018, 173, 1426–1438 CrossRef CAS PubMed
.
- Y. Y. Chen, Cell, 2018, 173, 1316–1317 CrossRef CAS PubMed
.
- J. H. Cho, A. Okuma, K. Sofjan, S. Lee, J. J. Collins and W. W. Wong, Nat. Commun., 2021, 12, 792 CrossRef CAS PubMed
.
- D. T. Rodgers, M. Mazagova, E. N. Hampton, Y. Cao, N. S. Ramadoss, I. R. Hardy, A. Schulman, J. Du, F. Wang, O. Singer, J. Ma, V. Nunez, J. Shen, A. K. Woods, T. M. Wright, P. G. Schultz, C. H. Kim and T. S. Young, Proc. Natl. Acad. Sci. U. S. A., 2016, 113, E459–E468 CrossRef CAS PubMed
.
- Y. Cao, D. T. Rodgers, J. Du, I. Ahmad, E. N. Hampton, J. S. Y. Ma, M. Mazagova, S. Choi, H. Y. Yun, H. Xiao, P. Yang, X. Luo, R. K. V. Lim, H. M. Pugh, F. Wang, S. A. Kazane, T. M. Wright, C. H. Kim, P. G. Schultz and T. S. Young, Angew. Chem., Int. Ed., 2016, 55, 7520–7524 CrossRef CAS PubMed
.
- L. Li, J. O. Fierer, T. A. Rapoport and M. Howarth, J. Mol. Biol., 2014, 426, 309–317 CrossRef CAS PubMed
.
- J. K. Fierle, J. Abram-Saliba, M. Brioschi, M. deTiani, G. Coukos and S. M. Dunn, Sci. Rep., 2019, 9, 12815 CrossRef PubMed
.
- N. G. Minutolo, P. Sharma, M. Poussin, L. C. Shaw, D. P. Brown, E. E. Hollander, A. Smole, A. Rodriguez-Garcia, J. Z. Hui, F. Zappala, A. Tsourkas and D. J. Powell Jr., J. Am. Chem. Soc., 2020, 142, 6554–6568 CrossRef CAS PubMed
.
- C. Guo, X. Guo, X. Li, M. Dong, X. Wang, S. Cheng, L. Zhi, Z. Niu and W. Zhu, Mol. Immunol., 2024, 165, 11–18 CrossRef CAS PubMed
.
- C.-Y. Wu, K. T. Roybal, E. M. Puchner, J. Onuffer and W. A. Lim, Science, 2015, 350, aab4077 CrossRef PubMed
.
- T. Gargett and M. P. Brown, Front. Pharmacol., 2014, 5, 235 Search PubMed
.
- M. T. Duong, M. R. Collinson-Pautz, E. Morschl, A. Lu, S. P. Szymanski, M. Zhang, M. E. Brandt, W.-C. Chang, K. L. Sharp, S. M. Toler, K. M. Slawin, A. E. Foster, D. M. Spencer and J. H. Bayle, Mol. Ther.–Oncolytics, 2019, 12, 124–137 CrossRef PubMed
.
- W.-H. Leung, J. Gay, U. Martin, T. E. Garrett, H. M. Horton, M. T. Certo, B. R. Blazar, R. A. Morgan, P. D. Gregory, J. Jarjour and A. Astrakhan, JCI Insight, 2019, 4, e124430 CrossRef PubMed
.
- Z. Huang, Y. Wu, M. E. Allen, Y. Pan, P. Kyriakakis, S. Lu, Y.-J. Chang, X. Wang, S. Chien and Y. Wang, Sci. Adv., 2020, 6, eaay9209 CrossRef CAS PubMed
.
- A. Banaszek, T. G. P. Bumm, B. Nowotny, M. Geis, K. Jacob, M. Wölfl, J. Trebing, K. Kucka, D. Kouhestani, T. Gogishvili, B. Krenz, J. Lutz, L. Rasche, D. Hönemann, H. Neuweiler, J. C. Heiby, R. C. Bargou, H. Wajant, H. Einsele, G. Riethmüller and G. Stuhler, Nat. Commun., 2019, 10, 5387 CrossRef PubMed
.
- L. Han, J. Chen, K. Ding, H. Zong, Y. Xie, H. Jiang, B. Zhang, H. Lu, W. Yin, J. Gilly and J. Zhu, Sci. Rep., 2017, 7, 8360 CrossRef PubMed
.
- T. Hofmann, J. Schmidt, E. Ciesielski, S. Becker, T. Rysiok, M. Schütte, L. Toleikis, H. Kolmar and A. Doerner, mAbs, 2020, 12, 1731938 CrossRef PubMed
.
- J. Shao, S. Li, X. Qiu, J. Jiang, L. Zhang, P. Wang, Y. Si, Y. Wu, M. He, Q. Xiong, L. Zhao, Y. Li, Y. Fan, M. Viviani, Y. Fu, C. Wu, T. Gao, L. Zhu, M. Fussenegger, H. Wang and M. Xie, Cell Res., 2024, 34, 31–46 CrossRef CAS PubMed
.
- C. L. Xu, M. Z. C. Ruan, V. B. Mahajan and S. H. Tsang, Viruses, 2019, 11, 28 CrossRef CAS PubMed
.
- A. Cebrian-Serrano and B. Davies, Mamm. Genome, 2017, 28, 247–261 CrossRef CAS PubMed
.
- H. Nishimasu, F. A. Ran, P. D. Hsu, S. Konermann, S. Shehata, N. Dohmae, R. Ishitani, F. Zhang and O. Nureki, Cell, 2014, 156, 935–949 CrossRef CAS PubMed
.
- B. Zetsche, S. E. Volz and F. Zhang, Nat. Biotechnol., 2015, 33, 139–142 CrossRef CAS PubMed
.
- D.-J. J. Truong, K. Kühner, R. Kühn, S. Werfel, S. Engelhardt, W. Wurst and O. Ortiz, Nucleic Acids Res., 2015, 43, 6450–6458 CrossRef CAS PubMed
.
- A. V. Wright, S. H. Sternberg, D. W. Taylor, B. T. Staahl, J. A. Bardales, J. E. Kornfeld and J. A. Doudna, Proc. Natl. Acad. Sci. U. S. A., 2015, 112, 2984–2989 CrossRef CAS PubMed
.
- J. Zhang, L. Chen, J. Zhang and Y. Wang, Comput. Struct. Biotechnol. J., 2019, 17, 1171–1177 CrossRef CAS PubMed
.
- D. P. Nguyen, Y. Miyaoka, L. A. Gilbert, S. J. Mayerl, B. H. Lee, J. S. Weissman, B. R. Conklin and J. A. Wells, Nat. Commun., 2016, 7, 12009 CrossRef CAS PubMed
.
- Y. Yu, X. Wu, N. Guan, J. Shao, H. Li, Y. Chen, Y. Ping, D. Li and H. Ye, Sci. Adv., 2020, 6, eabb1777 CrossRef CAS PubMed
.
- A. Przybyszewska-Podstawka, J. Czapiński, J. Kałafut and A. Rivero-Müller, Sci. Rep., 2023, 13, 14988 CrossRef CAS PubMed
.
- D. Ma, S. Peng and Z. Xie, Nat. Commun., 2016, 7, 13056 CrossRef CAS PubMed
.
- D. L. Shis and M. R. Bennett, Proc. Natl. Acad. Sci. U. S. A., 2013, 110, 5028–5033 CrossRef CAS PubMed
.
- J. Pu, J. Zinkus-Boltz and B. C. Dickinson, Nat. Chem. Biol., 2017, 13, 432–438 CrossRef CAS PubMed
.
- J. Pu, J. A. Dewey, A. Hadji, J. L. LaBelle and B. C. Dickinson, J. Am. Chem. Soc., 2017, 139, 11964–11972 CrossRef CAS PubMed
.
- S. Komatsu, H. Ohno and H. Saito, Nat. Commun., 2023, 14, 7256 CrossRef PubMed
.
- S. P. O'Brien and M. P. DeLisa, Biotechnol. J., 2014, 9, 355–361 CrossRef PubMed
.
- J. Wu, M. Wang, X. Yang, C. Yi, J. Jiang, Y. Yu and H. Ye, Nat. Commun., 2020, 11, 3708 CrossRef CAS PubMed
.
- M. C. Wehr, R. Laage, U. Bolz, T. M. Fischer, S. Grünewald, S. Scheek, A. Bach, K.-A. Nave and M. J. Rossner, Nat. Methods, 2006, 3, 985–993 CrossRef CAS PubMed
.
- S. Wang, F. Zhang, M. Mei, T. Wang, Y. Yun, S. Yang, G. Zhang and L. Yi, Commun. Biol., 2021, 4, 841 CrossRef CAS PubMed
.
- X. Wang, L. Kang, D. Kong, X. Wu, Y. Zhou, G. Yu, D. Dai and H. Ye, Nat. Chem. Biol., 2024, 20, 432–442 CrossRef CAS PubMed
.
- A. Mayer, R. J. Francis, S. K. Sharma, B. Tolner, C. J. Springer, J. Martin, G. M. Boxer, J. Bell, A. J. Green, J. A. Hartley, C. Cruickshank, J. Wren, K. A. Chester and R. H. J. Begent, Clin. Cancer Res., 2006, 12, 6509–6516 CrossRef CAS PubMed
.
- S. K. Sharma and K. D. Bagshawe, Adv. Drug Delivery Rev., 2017, 118, 2–7 CrossRef CAS PubMed
.
- M. Czuban, S. Srinivasan, N. A. Yee, E. Agustin, A. Koliszak, E. Miller, I. Khan, I. Quinones, H. Noory, C. Motola, R. Volkmer, M. Di Luca, A. Trampuz, M. Royzen and J. M. Mejia Oneto, ACS Cent. Sci., 2018, 4, 1624–1632 CrossRef CAS PubMed
.
- K. Wu, N. A. Yee, S. Srinivasan, A. Mahmoodi, M. Zakharian, J. M. M. Oneto and M. Royzen, Chem. Sci., 2021, 12, 1259–1271 RSC
.
- A. Q. Zhang, A. Hostetler, L. E. Chen, V. Mukkamala, W. Abraham, L. T. Padilla, A. N. Wolff, L. Maiorino, C. M. Backlund, A. Aung, M. Melo, N. Li, S. Wu and D. J. Irvine, Nat. Biomed. Eng., 2023, 7, 1113–1128 CrossRef CAS PubMed
.
- J. H. Cha, E. Kim, H. J. Lee, Y.-H. Lee, J. Lee, E. Kim and C. H. Kim, J. Med. Chem., 2023, 66, 7804–7812 CrossRef CAS PubMed
.
- S. A. Rosenberg, M. T. Lotze, J. C. Yang, P. M. Aebersold, W. M. Linehan, C. A. Seipp and D. E. White, Ann. Surg., 1989, 210, 474–484 CrossRef CAS PubMed
.
- X. Du and D. A. Williams, Blood, 1997, 89, 3897–3908 CrossRef CAS PubMed
.
- B. A. Baldo, Drug Saf., 2014, 37, 921–943 CrossRef CAS PubMed
.
- G. van Loo and M. J. M. Bertrand, Nat. Rev. Immunol., 2023, 23, 289–303 CrossRef CAS PubMed
.
- A. M. Levin, D. L. Bates, A. M. Ring, C. Krieg, J. T. Lin, L. Su, I. Moraga, M. E. Raeber, G. R. Bowman, P. Novick, V. S. Pande, C. G. Fathman, O. Boyman and K. C. Garcia, Nature, 2012, 484, 529–533 CrossRef CAS PubMed
.
- J. Deckers, T. Anbergen, A. M. Hokke, A. de Dreu, D. P. Schrijver, K. de Bruin, Y. C. Toner, T. J. Beldman, J. B. Spangler, T. F. A. de Greef, F. Grisoni, R. van der Meel, L. A. B. Joosten, M. Merkx, M. G. Netea and W. J. M. Mulder, Nat. Rev. Bioeng., 2023, 1, 286–303 CrossRef PubMed
.
- B. Zhang, J. Sun, Y. Wang, D. Ji, Y. Yuan, S. Li, Y. Sun, Y. Hou, P. Li, L. Zhao, F. Yu, W. Ma, B. Cheng, L. Wu, J. Hu, M. Wang, W. Song, X. Li, H. Li, Y. Fei, H. Chen, L. Zhang, G. C. Tsokos, D. Zhou and X. Zhang, Nat. Biomed. Eng., 2021, 5, 1288–1305 CrossRef CAS PubMed
.
- V. Mattijssen, P. H. De Mulder, A. De Graeff, P. S. Hupperets, F. Joosten, D. J. Ruiter, H. Bier, P. A. Palmer and P. Van den Broek, Ann. Oncol., 1994, 5, 957–960 CrossRef CAS PubMed
.
- P. Murer and D. Neri, Nat. Biotechnol., 2019, 52, 42–53 CAS
.
- D. Venetz, D. Koovely, B. Weder and D. Neri, J. Biol. Chem., 2016, 291, 18139–18147 CrossRef CAS PubMed
.
- J. M. Moll, M. Wehmöller, N. C. Frank, L. Homey, P. Baran, C. Garbers, L. Lamertz, J. H. Axelrod, E. Galun, H. D. Mootz and J. Scheller, ACS Synth. Biol., 2017, 6, 2260–2272 CrossRef CAS PubMed
.
- D.-A. Silva, S. Yu, U. Y. Ulge, J. B. Spangler, K. M. Jude, C. Labão-Almeida, L. R. Ali, A. Quijano-Rubio, M. Ruterbusch, I. Leung, T. Biary, S. J. Crowley, E. Marcos, C. D. Walkey, B. D. Weitzner, F. Pardo-Avila, J. Castellanos, L. Carter, L. Stewart, S. R. Riddell, M. Pepper, G. J. L. Bernardes, M. Dougan, K. C. Garcia and D. Baker, Nature, 2019, 565, 186–191 CrossRef CAS PubMed
.
- A. Quijano-Rubio, A. M. Bhuiyan, H. Yang, I. Leung, E. Bello, L. R. Ali, K. Zhangxu, J. Perkins, J.-H. Chun, W. Wang, M. J. Lajoie, R. Ravichandran, Y.-H. Kuo, S. K. Dougan, S. R. Riddell, J. B. Spangler, M. Dougan, D.-A. Silva and D. Baker, Nat. Biotechnol., 2023, 41, 532–540 CrossRef CAS PubMed
.
- V. Vasic, C. Buldun, M. Ritz, S. Dickopf, G. J. Georges, C. Spick, A. Peuker, T. Meier, K. Mayer and U. Brinkmann, mAbs, 2023, 15, 2245111 CrossRef PubMed
.
- S. Dickopf, C. Buldun, V. Vasic, G. Georges, C. Hage, K. Mayer, M. Forster, U. Wessels, K.-G. Stubenrauch, J. Benz, A. Ehler, M. E. Lauer, P. Ringler, S. Kobold, S. Endres, C. Klein and U. Brinkmann, Biol. Chem., 2022, 403, 495–508 CrossRef CAS PubMed
.
- M. G. Romei and S. G. Boxer, Annu. Rev. Biophys., 2019, 48, 19–44 CrossRef CAS PubMed
.
- S. Feng, S. Sekine, V. Pessino, H. Li, M. D. Leonetti and B. Huang, Nat. Commun., 2017, 8, 370 CrossRef PubMed
.
- S. Makhija, D. Brown, R. M. Rudlaff, J. K. Doh, S. Bourke, Y. Wang, S. Zhou, R. Cheloor-Kovilakam and B. Huang, ACS Chem. Biol., 2021, 16, 671–681 CrossRef CAS PubMed
.
- S. Feng, A. Varshney, D. Coto Villa, C. Modavi, J. Kohler, F. Farah, S. Zhou, N. Ali, J. D. Müller, M. K. Van Hoven and B. Huang, Commun. Biol., 2019, 2, 1–12 CrossRef CAS PubMed
.
- H. Ishikawa, F. Meng, N. Kondo, A. Iwamoto and Z. Matsuda, Protein Eng., Des. Sel., 2012, 25, 813–820 CrossRef CAS PubMed
.
- N. Milech, B. A. Longville, P. T. Cunningham, M. N. Scobie, H. M. Bogdawa, S. Winslow, M. Anastasas, T. Connor, F. Ong, S. R. Stone, M. Kerfoot, T. Heinrich, K. M. Kroeger, Y.-F. Tan, K. Hoffmann, W. R. Thomas, P. M. Watt and R. M. Hopkins, Sci. Rep., 2015, 5, 18329 CrossRef CAS PubMed
.
- A. Yudenko, A. Smolentseva, I. Maslov, O. Semenov, I. M. Goncharov, V. V. Nazarenko, N. L. Maliar, V. Borshchevskiy, V. Gordeliy, A. Remeeva and I. Gushchin, ACS Synth. Biol., 2021, 10, 72–83 CrossRef CAS PubMed
.
- X. Qi, X. Yan, Y. Zhao, L. Li and S. Wang, TrAC, Trends Anal. Chem., 2020, 133, 116069 CrossRef CAS
.
- W. Duan, X. Wang, H. Wang and F. Li, Talanta, 2018, 180, 76–80 CrossRef CAS PubMed
.
- T. Fan, Y. Du, Y. Yao, J. Wu, S. Meng, J. Luo, X. Zhang, D. Yang, C. Wang, Y. Qian and F. Gao, Sens. Actuators, B, 2018, 266, 9–18 CrossRef CAS
.
- Y. Ma, F. Geng, Y. Wang, M. Xu, C. Shao, P. Qu, Y. Zhang and B. Ye, Biosens. Bioelectron., 2019, 134, 36–41 CrossRef CAS PubMed
.
- J. You, Z. You, X. Xu, J. Ji, T. Lu, Y. Xia, L. Wang, L. Zhang and S. Du, Microchim. Acta, 2018, 186, 43 CrossRef PubMed
.
- M. Esmaelpourfarkhani, N. Mohammad Danesh, M. Ramezani, M. Alibolandi, A. Khakshour Abdolabadi, K. Abnous and S. M. Taghdisi, Microchem. J., 2023, 190, 108630 CrossRef CAS
.
- M. Debiais, A. Lelievre, M. Smietana and S. Müller, Nucleic Acids Res., 2020, 48, 3400–3422 CrossRef CAS PubMed
.
- E. Mokany, S. M. Bone, P. E. Young, T. B. Doan and A. V. Todd, J. Am. Chem. Soc., 2010, 132, 1051–1059 CrossRef CAS PubMed
.
- L. Sun, F. Shen, L. Tian, H. Tao, Z. Xiong, J. Xu and Z. Liu, Adv. Mater., 2021, 33, 2007910 CrossRef CAS PubMed
.
- L. Meng, W. Ma, S. Lin, S. Shi, Y. Li and Y. Lin, ACS Appl. Mater. Interfaces, 2019, 11, 6850–6857 CrossRef CAS PubMed
.
- Y. Cao, H. Zhang and X. C. Le, Anal. Chem., 2021, 93, 15712–15719 CrossRef CAS PubMed
.
- X. Luo, H. Wu, M. Xiong, L. Jiang, Z. Jiang and M. Gong, J. Anal. Sci. Technol., 2022, 13, 33 CrossRef CAS
.
- C. Shi, D. Yang, X. Ma, L. Pan, Y. Shao, G. Arya, Y. Ke, C. Zhang, F. Wang, X. Zuo, M. Li and P. Wang, Angew. Chem., Int. Ed., 2024, 63, e202320179 CrossRef CAS PubMed
.
- E. L. Boland, C. M. Van Dyken, R. M. Duckett, A. J. McCluskey and G. M. K. Poon, J. Mol. Biol., 2014, 426, 645–655 CrossRef CAS PubMed
.
- V. Purde, E. Kudryashova, D. B. Heisler, R. Shakya and D. S. Kudryashov, Proc. Natl. Acad. Sci. U. S. A., 2020, 117, 22090–22100 CrossRef CAS PubMed
.
- J. Jumper, R. Evans, A. Pritzel, T. Green, M. Figurnov, O. Ronneberger, K. Tunyasuvunakool, R. Bates, A. Žídek, A. Potapenko, A. Bridgland, C. Meyer, S. A. A. Kohl, A. J. Ballard, A. Cowie, B. Romera-Paredes, S. Nikolov, R. Jain, J. Adler, T. Back, S. Petersen, D. Reiman, E. Clancy, M. Zielinski, M. Steinegger, M. Pacholska, T. Berghammer, S. Bodenstein, D. Silver, O. Vinyals, A. W. Senior, K. Kavukcuoglu, P. Kohli and D. Hassabis, Nature, 2021, 596, 583–589 CrossRef CAS PubMed
.
- F. Liu, X. Jiang, J. Yang, J. Tao and M. Zhang, Briefings Bioinf., 2022, 23, bbac365 CrossRef PubMed
.
- J. Sia, W. Zhang, E. Jonckheere, D. Cook and P. Bogdan, Sci. Rep., 2022, 12, 10883 CrossRef CAS PubMed
.
- M. Cha, E. S. T. Emre, X. Xiao, J.-Y. Kim, P. Bogdan, J. S. VanEpps, A. Violi and N. A. Kotov, Nat. Comput. Sci., 2022, 2, 243–252 CrossRef PubMed
.
|
This journal is © The Royal Society of Chemistry 2024 |
Click here to see how this site uses Cookies. View our privacy policy here.