DOI:
10.1039/D2SC00952H
(Edge Article)
Chem. Sci., 2022,
13, 5846-5853
CPL on/off control of an assembled system by water soluble macrocyclic chiral sources with planar chirality†
Received
15th February 2022
, Accepted 28th March 2022
First published on 30th March 2022
Abstract
Herein, we report the synthesis and planar chiral properties of a pair of water-soluble cationic pillar[5]arenes with stereogenic carbons. Interestingly, although units of the molecules were rotatable, only one planar chiral diastereomer existed in water in both cases. As a new type of chiral source, these molecules transmitted chiral information from the planar chiral cavities to the assembly of a water-soluble extended π-conjugated compound, affording circularly polarized luminescence (CPL). The chirality transfer process and resulting CPL were extremely sensitive to the feed ratio of the chiral pillar[5]arenes owing to the combined action of their planar chirality, bulkiness, and strong binding properties. When a limited amount of chiral source was added, further assembly of the extended π-conjugated compound into helical fibers with CPL was triggered. Unexpectedly, larger amounts of chiral source destroyed the helical fiber assemblies, resulting in elimination of the chirality and CPL properties from the assembled structures.
Introduction
Chirality is a widespread physical phenomenon in nature that is not only essential for various sophisticated biological activities, but has also been applied extensively in the field of materials science.1 Among all research on chiral systems, functional materials with circularly polarized luminescence (CPL) have received extensive attention owing to their potential photonic applications, including sensors,2 photoelectric devices,3 chiroptical materials,4 and 3D imaging.5 In general, the fabrication of CPL materials can be achieved by two strategies. The first involves covalently connecting the chiral and fluorescent units to generate chiral luminescent small molecules6 or polymers7 and their assemblies.8 This results in the two units being close together and enables the systems to possess relatively high dissymmetry values (|glum|). Extended π-conjugated moieties were widely present in such systems and successfully produced CPL materials with high |glum|.9 In especial, pyrene moieties were frequently used as the fluorescent units.10 Pyrene can show excimer fluorescence. It was demonstrated that the excimer of pyrene gave rise to higher CPL, promising CPL control by controlling the emission modes of pyrene derivatives. However, these systems sometimes suffer from cumbersome synthetic routes to introduce chiral units covalently. The other strategy uses the concept of chirality transfer to connect the chiral unit and fluorescent unit non-covalently and has produced various of successful CPL materials,11 despite that the binding affinity of noncovalent bonds might turn to be low compared with covalent bonds.
Chiral macrocyclic molecules12 with an intrinsic cavity might provide new candidates for a different mode of chirality transfer to fluorescent units owing to their bulky size, special chirality, and generally strong and specific host–guest complexing properties. This is promising for the fabrication of new supramolecular CPL materials.13 However, this field remains underexplored owing to difficulties in the synthesis of chiral macrocycles and establishing effective chiral cavities. Only a few CPL materials based on host–guest interactions between fluorophores and cyclodextrins have been reported.14 In contrast to cyclodextrins, which have only one chirality, and most other macrocyclic molecules, which lack intrinsic chirality in general, pillar[n]arenes15 are pillar-shaped macrocyclic hosts that exhibit planar chirality originating from the direction of substituents on their rims. These compounds possess two stable planar chiral isomers (pR and pS), and consequently produce two types of stable chiral cavity (Fig. 1).16 These remarkable features have led to a few early-stage examples of CPL materials based on the single-molecule luminescence of functional pillar[n]arenes.17
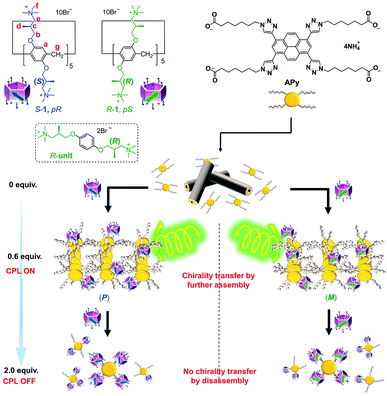 |
| Fig. 1 Rational design of new supramolecular CPL on/off controllable materials using water-soluble planar chiral pillar[5]arenes S-1 and R-1. | |
Despite promising applications as supramolecular chiral sources, pillar[n]arenes with highly planar chiral purity have rarely been explored because flipping of their phenyl units leads to fast inversion of the planar chiral isomers.18 Bulky groups on rims can inhibit the unit flip and generate two unconvertable enantiomers.19 However, their isolation using chiral column chromatography is usually time-consuming and labor-intensive. Chiral control and regulation of pillar[5]arenes have also been performed by introducing stereogenic carbons onto their rims to form diastereomers.20 However, the diastereomeric excess (de) was often low. Therefore, obtaining pillar[5]arenes with high planar chiral purity in a facile manner is among the most intriguing pillar[n]arene research areas. Herein, we report the first examples of cationic water-soluble planar chiral pillar[5]arenes with stereogenic carbons (S-1 and R-1 in Fig. 1), which were, unexpectedly, diastereomerically pure in water. By controlling the on/off transmission of planar chiral information from S-1 and R-1 to APy, a dendritic molecule fusing four linear alkyl acids with a π-conjugated core, using the feed amount of chiral pillar[5]arenes, CPL on/off-control of the assembly of APy was achieved. To our knowledge, this is the first example of a CPL on/off-control system dependent on the feed amount of the planar chiral sources.
Results and discussion
Synthesis, planar chiral properties, and host–guest properties of 1 in water
Synthesis of S-1 and R-1.
Cationic water-soluble chiral pillar[5]arenes S-1 and R-1 (Fig. 1) were obtained by refluxing a solution of trimethylamine in ethanol with chiral pillar[5]arene precursors bearing 1-bromo-2-methylpropoxy groups on both rims (Scheme S1†). In principle, both S-1 and R-1 bearing ten stereogenic carbons on the rims possessed two stable planar chiral conformers, namely (S, pS)- and (S, pR)-conformers for S-1, and (R, pS)- and (R, pR)-conformers for R-1.
Solvent-dependent planar chiral expression of S-1 and R-1.
The UV-vis spectra of 1 showed an absorption band at approx. 295 nm, which was attributed to π–π* transitions in the pillar[5]arene backbones (Fig. 2a and S1a†).21 In the CD spectra, the positive Cotton effect in the π–π* transition region suggested the pR-rich planar chirality of S-1 induced by the stereogenic carbon centers on the rims (Fig. 2a and S1b†).22 Interestingly, the CD spectra of S-1 were critically affected by the solvent employed. For example, the CD intensity of S-1 in water was four times that in DMSO (Fig. S1b†). This indicated the unit-flip ability of S-1, meaning that conversion between the pR and pS planar chiral conformers of S-1 could occur at room temperature.
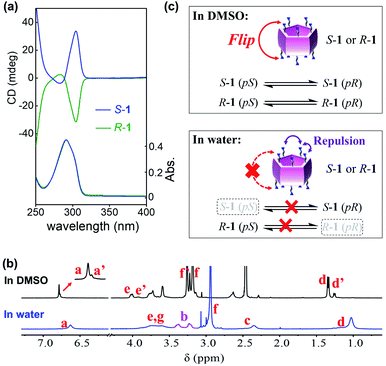 |
| Fig. 2 (a) CD and UV-vis spectra of S-1 and R-1 (2 × 10−5 M) in water. (b) 1H NMR spectra of S-1 (400 MHz) in D2O and DMSO-d6. All spectra were recorded at 25 °C. (c) Illustration of the unit flip of S-1 and R-1 in DMSO and inhibition of the unit flip in water. | |
1H NMR spectra of S-1 in aprotic solvents afforded two clear sets of resonance signals (Fig. 2b, S2 and S3†), indicating that S-1 possessed both pS and pR conformations in these solvents.23 For example, the de values of S-1 in DMSO-d6 and acetonitrile-d3 were determined to be approx. 60% and 26%, respectively. Unexpectedly, all protons on S-1 showed only one set of resonance signals in D2O (Fig. 2b).24 Furthermore, the resonance signal of proton Hb in proximity to the pillar[5]arene core was split and hardly coalesced in D2O, even at 80 °C (Fig. S5†), indicating that flipping of the pillar[5]arene units was inhibited or slow on the NMR timescale, and that interconversion between conformers was difficult in D2O. Additionally, the dissymmetry value (g) of the induced CD intensity of S-1 in water was 5.9 × 10−3 (Fig. S6†), which was around twice the g value of the previously reported water-soluble planar chiral pillar[5]arene with almost 100% diastereomeric purity,25 and closer to those of enantiopure pillar[5]arenes in organic solvents (Table S1†).19b,c,26 These observations implied that only the conformer with pR planar chirality ((S, pR)-conformer) existed in the aqueous solution of S-1. The CD spectra of R-1 and S-1 were mirror images (Fig. 2a), suggesting that R-1 was diastereomerically pure in water with pS planar chirality (the (R, pS)-conformer).
The above results demonstrated that the bulkiness of the substituents on rims was turned out to be not enough to prevent the unit flip of the pillar[5]arenes, so the expression of planar chirality of S-1 and R-1 was critically affected by the solvent employed. Such solvent-dependent planar chiral expression was first caused by the solvation of the substituents on both rims. In water, the relatively strong repulsion between the hydrated trimethyl ammonium groups inhibited flipping of the pillar[5]arene units (Fig. 2c). In contrast, as an organic solvent, DMSO might not only fail to fully ionize the trimethylammoniums and their counter anions (Br−), but also reduced the repulsion between the solvated trimethylammonium salts, which led to easier flipping of the pillar[5]arene units. In addition, the different orientations of substituents of 1 in different solvents also contributed to the solvent-dependent planar chiral expression according to our previous findings.20a,23 There are two branches on each stereogenic carbon of 1, one is the trimethylammonium, which is larger in polarity and volume, and the other is the methyl group, which is smaller in polarity and volume. In water, the branched methyl groups probably favored being located in the pillar[5]arene cavity, while the trimethylammoniums favored being outside due to hydration, leading to highly selective orientation of the side chains of 1, which resulted in the high de value. Furthermore, this “open”-form with small groups inside and large groups outside often results in the pR chirality of S-1 and pS chirality of R-1.20a,23 In contrast, the difference in the location of the methyl branch and trimethylammonium branch decreased in aprotic solvent (such as DMSO), which further decreased the de value of 1.
Host–guest properties of S-1 and R-1.
Pillar[5]arenes with flippable units usually show low de values in solution.20 The high planar chiral purity of S-1 and R-1 in water made them perfect candidates as chiral sources with effective chiral cavities that might show strong host–guest interactions with specific guest molecules.
Both S-1 and R-1 were able to form complexes with linear carboxylic acids C4–C9 (Fig. 3 and S7–S15, and detailed discussion in the ESI†), because the pillar[5]arene cavity bound the alkyl moieties via multiple C–H/π interactions,27 and the trimethyl ammonium groups of 1 interacted with the acid groups of C4–C9.28 The binding affinity increased significantly with increasing length of the guest molecules (Table S2†). For example, the association constants (Ka) of the complexes of S-1 and R-1 with C8 reached 6.3 × 10−5 M−1 and 5.3 × 10−5 M−1, respectively.
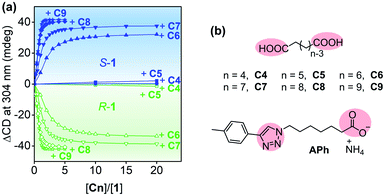 |
| Fig. 3 (a) Plots of CD increase at 304 nm for S-1 and R-1 (2 × 10−5 M) upon addition of C4–C9. All spectra were recorded in water at 25 °C. (b) Molecular structures of C4–C9 and APh. | |
Chirality transfer on/off control from chiral 1 to APy in water
Molecular design and self-assembly of APy.
Based on the binding properties of 1 in water, compound APy bearing four peripheral aliphatic acid chains and an extended π-conjugated core was synthesized (Fig. 1 and Scheme S3†). The aliphatic acid chains with the same linker as C8 were expected to enable strong complexation between APy and 1. The triazole moieties of APy also aid complexation according to previous reports.29
The assembly properties of APy in water due to π-oligomerization were initially investigated by concentration-variable fluorescence measurements (Fig. S16 and S17†). The critical assembly concentration (CAC) of APy was determined to be approx. 2 × 10−5 M in water, which was accordant with the concentration-dependent and temperature-variable 1H NMR measurements (Fig. S18 and S19†).30,31 All subsequent experiments were performed with an APy concentration of 6 × 10−5 M, which was higher than the CAC.
Two-step complexing process of Apy with 1.
Complexation between APy and S-1 was confirmed by 1H NMR titration (Fig. S20†). Although the spectra were complicated, the signals corresponding to the linear alkyl chains of APy clearly showed dramatic upfield shift to around −1 to −2 ppm upon addition of S-1. This observation indicated the strong shielding effect resulting from the inclusion of these protons in the cavity of S-1. The binding mode and affinity between APy and S-1 were estimated by investigating the binding between S-1 and APh (Fig. 3b), which was the unit model of APy (Fig. S21–S24†). The association constant between S-1 and APh was 8.0 × 105 M−1 (Fig. S24†), suggesting a strong binding.
Carefully examination of the 1H NMR spectra of APy upon addition of S-1 showed a two-step complexing process (Fig. S20†). With less than 0.6 equiv. of S-1, all resonance signals of APy underwent an upfield shift, while a gradual downfield shift was observed with further addition of S-1. UV titration also suggested the same two-step process. In the aqueous solution, APy gave rise to absorption maxima at 253, 299, and 385 nm in a wide concentration range (Fig. S25†). The absorption band at approx. 385 nm decreased, broadened, and underwent a red-shift when less than 0.6 equiv. of planar chiral 1 was added, while adding more 1 resulted in the same band intensifying again (Fig. S26 and S27†).
Two-step chirality transfer from chiral 1 to Apy.
As both S-1 and R-1 possess pure planar chirality in water, the chiral effect of 1 on APy was investigated (Fig. 4). Gratifyingly, both S-1 and R-1 induced the chirality in APy, as evidenced by the appearance of a CD signal at approx. 400 nm from the pyrene moiety upon addition of 1 (Fig. 4a). The CD signals indicated that S-1 induced a positive Cotton effect on APy, while R-1 induced a negative Cotton effect. Interestingly, the chiral induction ability of 1 exactly followed the trend of the two-step complexing process of APy (Fig. 4b and S28†), which was confirmed by 1H NMR and UV titrations. Upon addition of less than 0.6 equiv. of chiral 1 to the aqueous solution of APy, the CD signals emerged gradually. Conversely, further addition of 1 caused a decrease in the chirality of APy previously acquired. When 2.0 equiv. of chiral 1 was added, the induced CD signals disappeared completely.
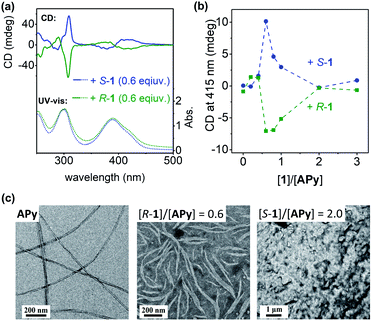 |
| Fig. 4 (a) UV-vis and CD spectra of APy (6 × 10−5 M) with 0.6 equiv. of S-1 and R-1. (b) Plots of CD at 415 nm for APy (6 × 10−5 M) upon addition of S-1 and R-1. All spectra were recorded in water at 25 °C. (c) TEM images of Apy (left), and mixtures of APy with chiral 1 ([R-1]/[APy] = 0.6 (middle); [S-1]/[APy] = 2.0 (right)). | |
Such a two-step chiral induction process was beyond our expectation, because it behaved differently from most chirality transfer systems, where additional feeding of chiral sources usually improves the chirality transfer efficiency. As control experiments, the unit model of 1 (R-unit in Fig. 1) and l-valine methyl ester hydrochloride (L-Val) only caused a continuous decrease in the absorption at approx. 385 nm in the UV spectrum upon addition (Fig. S29†). Furthermore, these additives failed to transfer chirality to APy, even when added in large excess, except for causing random signals at approx. 400 nm that could be considered noise (Fig. S30 and S31†). These observations indicated that planar chiral 1 was effective compared with non-macrocyclic chiral sources and ensured successful two-step chirality transfer from the chiral source to APy in this system.
Transmission electron microscopy (TEM) images more clearly showed the effect of 1 on chirality transfer “on and off” control (Fig. 4c and S32†). Monolayered nanotubes of APy with a thickness of around 2.5 nm were changed to short bundle structures with partial helicity by adding 0.6 equiv. of chiral 1, while further addition of 1 completely destroyed the assemblies of APy and only amorphous aggregates were observed.
Mechanism of the two-step complexation and chirality transfer between chiral 1 and APy in water
To further clarify the two-step complexation and chirality transfer phenomena, the fluorescence change of APy caused by chiral 1 was investigated. On the fluorescence spectrum of APy, there are two emissions at approx. 426 nm and 531 nm (Fig. 5a), whose lifetimes were determined to be approx. 2.6 ns and 94 ns, respectively, which verified that the two emissions represented the monomeric APy and the excimer formed by stacking, respectively (Fig. S34†). Interestingly, upon adding chiral 1 to the aqueous solution of APy, a drastic spectral change was observed (Fig. 5a and S33†). Only 0.4 equiv. of S-1 led to disappearance of the fluorescence band at approx. 426 nm and broadened the fluorescence band at approx. 531 nm (Fig. 5a). When S-1 was further increased to 0.6 equiv., the emission maximum underwent a red-shift to approx. 545 nm, and the emission lifetime increased to 135 ns (Fig. S34†). The emission color gradually changed from light green to yellow during this process (Fig. 5b). These observations clearly suggested that S-1 triggered oligomerization of APy through π–π stacking of extended pyrene moieties effectively. However, the emission at approx. 545 nm was gradually decreased and blue-shifted, accompanied by emergence of the band at approx. 426 nm upon addition of more than 0.6 equiv. of S-1. When 2.0 equiv. of S-1 was added, the emission at approx. 545 nm disappeared and the emission color changed to blue, which indicated complete disassembly of APy to the monomeric state. The two-step complexation between 1 and APy were fast and efficient, as evidenced by that the fluorescent spectra of each titration were hardly changed within 9 hours at room temperature.
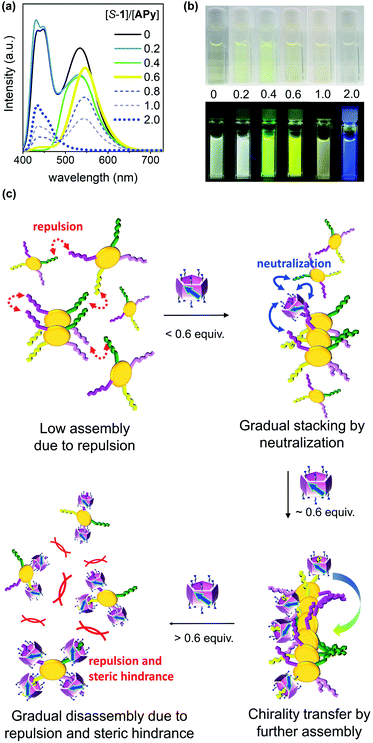 |
| Fig. 5 (a) Fluorescence spectra (λex = 385 nm) and (b) images of APy (6 × 10−5 M) upon addition of S-1 under visible (top) and UV light (365 nm). All spectra were recorded in water at 25 °C. (c) Schematic illustration of the proposed two-step chirality transfer between S-1 and APy upon changing the feed amount of S-1. The four peripheral chains of APy are shown in different colors for clarifying. | |
As shown in Fig. 5c, the neutralization of anions on APy by cations on 1 upon initial addition of 1 decreased repulsion between the molecules of APy, which had weakened the stacking of pyrene moieties, resulting in the assembly of APy. Theoretically, 0.4 equiv. of 1 possessed equal and opposite charges to APy. This was likely the reason for the monomeric emission disappearing in the fluorescence spectra of APy when 0.4 equiv. of 1 was added. A slight excess of 1 (0.6 equiv.) might regulate the assembly of APy and cause longer-range assembly. Taking advantage of the strong binding between 1 and APy, the assembly of APy obtained chiral information from planar chiral 1. The CD signals shown in Fig. 4a indicated that S-1 induced P-helicity in the assembly of APy, while R-1 induced M-helicity. Further addition of 1 caused repulsion between the stable complex (APy·1n) owing to excess cations and steric hindrance between the bulky pillar[5]arenes, leading APy to depolymerize and the previously acquired chirality to gradually disappear.
CPL on/off control of the assembled system of APy by chiral 1 in water
The acquired helical assemblies of APy (6 × 10−5 M) possessed relatively strong fluorescence emission. For example, the quantum yields in the presence of 0.6 equiv. of S-1 and R-1 were 0.43 and 0.47, respectively (Table S3†). Therefore, the unusual chiral-source-concentration-dependent CPL property of APy was investigated. In the presence of 0.6 equiv. of chiral 1, CPL activity with emission maxima consistent with those in the fluorescence spectra were observed (Fig. 6). The |glum| value reached approx. 1.7 × 10−3 at 550 nm (Fig. S37†). To our knowledge, this is the highest glum value reported to date for pillar[n]arene-containing systems capable of CPL properties in solution.17a,c Furthermore, further feeding with the chiral source eliminated the CPL of APy owing to decreased CD induction and emission, indicating that CPL on/off control was achieved by continuous addition of planar chiral pillar[5]arenes.
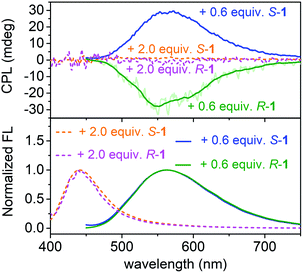 |
| Fig. 6 CPL (top) and fluorescence (bottom) spectra of Apy (6 × 10−5 M) in the presence of 0.6 equiv. (solid lines) and 2.0 equiv. (dashed lines) of chiral 1. All spectra were recorded in water at 25 °C. | |
Conclusions
In conclusion, we synthesized cationic water-soluble planar-chiral pillar[5]arenes S-1 and R-1, which were diastereomerically pure in water (pR for S-1, pS for R-1). Compared with simple chiral molecules (such as R-unit and L-Val), planar chiral pillar[5]arene 1 readily transferred chiral information to guest molecule APy through host–guest interactions. Interestingly, the chirality transfer efficiency and subsequent CPL properties were sensitive to the host–guest feed ratio owing to the bulkiness of planar chiral macrocycle 1. Adding a small amount of 1 (0.6 equiv.) resulted in CPL of APy. The strategy of using readily obtained chiral macrocyclic molecules as the chiral source makes the chirality transfer process more diverse and interesting, and can be finely regulated, which might provide new possibilities in the area of supramolecular CPL materials. In particular, planar chiral pillar[5]arenes strongly bound the linear aliphatic chains of fluorescent guest molecules, with only a minor impact on the physical properties of the fluorophores. However, complexation of linear aliphatic chains in the supramolecular assemblies of Apy resulted in effective chiral transmission from planar chiral pillar[5]arenes to the fluorescent guest molecules. Various fluorescent supramolecular assemblies composed of linear aliphatic chains and fluorophore(s) have been reported,32 so whether pillar[5]arenes 1 can transfer chiral information to them should be investigated. This is currently under investigation in our group.
Data availability
All analytical data is provided in the narrative and in the ESI.†
Author contributions
S. F. and T. O.: conceptualization, methodology, formal analysis, investigation, writing-original draft and editing. T. O.: project administration, funding acquisition and supervision. S. F., S. O., K. K.: resources and validation. T. T., K. W., K. Y., M. G., K. T., T. K., T. Y.: formal analysis and resources.
Conflicts of interest
There are no conflicts to declare.
Acknowledgements
This work was supported by JSPS KAKENHI Grant Numbers JP15H00990, JP17H05148, JP18H04510, and JP20H04670 (Scientific Research on Innovative Areas, T. O.), JP19H00909 (Scientific Research (A), T. O.), JP21K14612 (Early-Career Scientists, S. F.), JP20K22528 (Research Activity Start-up, K. K.) and JP21K14611 (Early-Career Scientists, K.K.), JST CREST Grant Number JPMJCR18R3 (T. O.), the Nanotechnology Platform Program of the Ministry of Education, Culture, Sports, Science and Technology (MEXT), and MEXT World Premier International Research Center Initiative (WPI), Japan.
Notes and references
-
(a) J. R. Brandt, F. Salerno and M. J. Fuchter, Nat. Rev. Chem., 2017, 1, 0045 CrossRef CAS;
(b) D. Zhao, T. van Leeuwen, J. Cheng and B. L. Feringa, Nat. Chem., 2017, 9, 250–256 CrossRef CAS PubMed;
(c) C. Kulkarni, A. K. Mondal, T. K. Das, G. Grinbom, F. Tassinari, M. F. J. Mabesoone, E. W. Meijer and R. Naaman, Adv. Mater., 2020, 32, 1904965 CrossRef CAS PubMed;
(d) K. Shimomura, T. Ikai, S. Kanoh, E. Yashima and K. Maeda, Nat. Chem., 2014, 6, 429–434 CrossRef CAS PubMed;
(e) P. Duan, H. Cao, L. Zhang and M. Liu, Soft Matter, 2014, 10, 5428–5448 RSC.
-
(a) F. Zinna and L. Di Bari, Chirality, 2015, 27, 1–13 CrossRef CAS PubMed;
(b) H. Maeda, Y. Bando, K. Shimomura, I. Yamada, M. Naito, K. Nobusawa, H. Tsumatori and T. Kawai, J. Am. Chem. Soc., 2011, 133, 9266–9269 CrossRef CAS PubMed;
(c) Y. Zhao, N. A. A. Rahirn, Y. Xia, M. Fujiki, B. Song, Z. Zhang, W. Zhang and X. Zhu, Macromolecules, 2016, 49, 3214–3221 CrossRef CAS.
-
(a) M. Grell, M. Oda, K. S. Whitehead, A. Asimakis, D. Neher and D. D. C. Bradley, Adv. Mater., 2001, 13, 577–580 CrossRef CAS;
(b) Y. Geng, A. Trajkovska, S. W. Culligan, J. J. Ou, H. M. P. Chen, D. Katsis and S. H. Chen, J. Am. Chem. Soc., 2003, 125, 14032–14038 CrossRef CAS PubMed;
(c) M. Li, S.-H. Li, D. Zhang, M. Cai, L. Duan, M.-K. Fung and C.-F. Chen, Angew. Chem., Int. Ed., 2018, 57, 2889–2893 CrossRef CAS PubMed;
(d) M. Sun, L. Xu, A. Qu, P. Zhao, T. Hao, W. Ma, C. Hao, X. Wen, F. M. Colombari, A. F. de Moura, N. A. Kotov, C. Xu and H. Kuang, Nat. Chem., 2018, 10, 821–830 CrossRef CAS PubMed.
-
(a) H. K. Bisoyi and Q. Li, Angew. Chem., Int. Ed., 2016, 55, 2994–3010 CrossRef CAS PubMed;
(b) Y. Kim, B. Yeom, O. Arteaga, S. J. Yoo, S. G. Lee, J. G. Kim and N. A. Kotov, Nat. Mater., 2016, 15, 461–468 CrossRef CAS PubMed.
-
(a) M. Schadt, Annu. Rev. Mater. Sci., 1997, 27, 305–379 CrossRef CAS;
(b) M. C. Heffern, L. M. Matosziuk and T. J. Meade, Chem. Rev., 2014, 114, 4496–4539 CrossRef CAS PubMed;
(c) J. Han, S. Guo, H. Lu, S. Liu, Q. Zhao and W. Huang, Adv. Opt. Mater., 2018, 6, 1800538 CrossRef.
-
(a) E. M. Sánchez-Carnerero, A. R. Agarrabeitia, F. Moreno, B. L. Maroto, G. Muller, M. J. Ortiz and S. de la Moya, Chem.–Eur. J., 2015, 21, 13488–13500 CrossRef PubMed;
(b) J. F. Koegel, S. Kusaka, R. Sakamoto, T. Iwashima, M. Tsuchiya, R. Toyoda, R. Matsuoka, T. Tsukamoto, J. Yuasa, Y. Kitagawa, T. Kawai and H. Nishihara, Angew. Chem., Int. Ed., 2016, 55, 1377–1381 CrossRef CAS PubMed;
(c) K. Takaishi, K. Iwachido, R. Takehana, M. Uchiyama and T. Ema, J. Am. Chem. Soc., 2019, 141, 6185–6190 CrossRef CAS PubMed;
(d) Z.-B. Sun, J.-K. Liu, D.-F. Yuan, Z.-H. Zhao, X.-Z. Zhu, D.-H. Liu, Q. Peng and C.-H. Zhao, Angew. Chem., Int. Ed., 2019, 58, 4840–4846 CrossRef CAS PubMed;
(e) Y. Saito, M. Satake, R. Mori, M. Okayasu, H. Masu, M. Tominaga, K. Katagiri, K. Yamaguchi, S. Kikkawa, H. Hikawa and I. Azumaya, Org. Biomol. Chem., 2020, 18, 230–236 RSC;
(f) K. Nakamura, S. Furumi, M. Takeuchi, T. Shibuya and K. Tanaka, J. Am. Chem. Soc., 2014, 136, 5555–5558 CrossRef CAS PubMed;
(g) J. Zhu, S. Chen and C. He, J. Am. Chem. Soc., 2021, 143, 5301–5307 CrossRef CAS PubMed.
-
(a) M. Oda, H. G. Nothofer, G. Lieser, U. Scherf, S. C. J. Meskers and D. Neher, Adv. Mater., 2000, 12, 362–365 CrossRef CAS;
(b) S.-T. Wu, Z.-W. Cai, Q.-Y. Ye, C.-H. Weng, X.-H. Huang, X.-L. Hu, C.-C. Huang and N.-F. Zhuang, Angew. Chem., Int. Ed., 2014, 53, 12860–12864 CrossRef CAS PubMed;
(c) B. A. San Jose, J. L. Yan and K. Akagi, Angew. Chem., Int. Ed., 2014, 53, 10641–10644 CrossRef CAS PubMed;
(d) S. T. Duong and M. Fujiki, Polym. Chem., 2017, 8, 4673–4679 RSC;
(e) B. Zhao, X. Gao, K. Pan and J. Deng, ACS Nano, 2021, 15, 7463–7471 CrossRef CAS PubMed;
(f) Y. Ru, L. Sui, H. Song, X. Liu, Z. Tang, S.-Q. Zang, B. Yang and S. Lu, Angew. Chem., Int. Ed., 2021, 60, 14091–14099 CrossRef CAS PubMed.
-
(a) S. H. Chen, D. Katsis, A. W. Schmid, J. C. Mastrangelo, T. Tsutsui and T. N. Blanton, Nature, 1999, 397, 506–508 CrossRef CAS;
(b) Q. Ye, D. Zhu, L. Xu, X. Lu and Q. Lu, J. Mater. Chem. C, 2016, 4, 1497–1503 RSC;
(c) C.-T. Yeung, K.-H. Yim, H.-Y. Wong, R. Pal, W.-S. Lo, S.-C. Yan, M. Y.-M. Wong, D. Yufit, D. E. Smiles, L. J. McCormick, S. J. Teat, D. K. Shuh, W.-T. Wong and G.-L. Law, Nat. Commun., 2017, 8, 1128 CrossRef PubMed;
(d) R. Aoki, R. Toyoda, J. F. Kögel, R. Sakamoto, J. Kumar, Y. Kitagawa, K. Harano, T. Kawai and H. Nishihara, J. Am. Chem. Soc., 2017, 139, 16024–16027 CrossRef CAS PubMed;
(e) X.-M. Chen, Y. Chen, L. Liang, Q.-J. Liu and Y. Liu, Macromol. Rapid Commun., 2018, 39, 1700869 CrossRef PubMed;
(f) Y. Shi, G. Q. Yin, Z. Yan, P. Sang, M. Wang, R. Brzozowski, P. Eswara, L. Wojtas, Y. Zheng, X. Li and J. Cai, J. Am. Chem. Soc., 2019, 141, 12697–12706 CrossRef CAS PubMed;
(g) T. Zhao, J. Han, X. Jin, Y. Liu, M. Liu and P. Duan, Angew. Chem., Int. Ed., 2019, 58, 4978–4982 CrossRef CAS PubMed;
(h) H.-T. Feng, C. Liu, Q. Li, H. Zhang, J. W. Y. Lam and B. Z. Tang, ACS Mater. Lett., 2019, 1, 192–202 CrossRef CAS.
-
(a) J.-L. Ma, Q. Peng and C.-H. Zhao, Chem.–Eur. J., 2019, 25, 15441–15454 CrossRef CAS PubMed;
(b) F. Song, Z. Zhao, Z. Liu, J. W. Y. Lam and B. Z. Tang, J. Mater. Chem. C, 2020, 8, 3284–3301 RSC;
(c) S. Wang, D. Hu, X. Guan, S. Cai, G. Shi, Z. Shuai, J. Zhang, Q. Peng and X. Wan, Angew. Chem., Int. Ed., 2021, 60, 21918–21926 CrossRef CAS PubMed.
-
(a) K. Nakabayashi, T. Amako, N. Tajima, M. Fujiki and Y. Imai, Chem. Commun., 2014, 50, 13228–13230 RSC;
(b) Y. Hashimoto, T. Nakashima, D. Shimizu and T. Kawai, Chem. Commun., 2016, 52, 5171–5174 RSC;
(c) X. Bai, Y. Jiang, G. Zhao, J. Jiang, C. Yuan and M. Liu, Soft Matter, 2021, 17, 4328–4334 RSC;
(d) T. Ikai, Y. Wada, S. Awata, C. Yun, K. Maeda, M. Mizuno and T. M. Swager, Org. Biomol. Chem., 2017, 15, 8440–8447 RSC;
(e) A. Homberg, E. Brun, F. Zinna, S. Pascal, M. Górecki, L. Monnier, C. Besnard, G. Pescitelli, L. Di Bari and J. Lacour, Chem. Sci., 2018, 9, 7043–7052 RSC;
(f) Y. Imai and J. Yuasa, Chem. Commun., 2019, 55, 4095–4098 RSC.
-
(a) K. Maeda, M. Nozaki, K. Hashimoto, K. Shimomura, D. Hirose, T. Nishimura, G. Watanabe and E. Yashima, J. Am. Chem. Soc., 2020, 142, 7668–7682 CrossRef CAS PubMed;
(b) T. Goto, Y. Okazaki, M. Ueki, Y. Kuwahara, M. Takafuji, R. Oda and H. Ihara, Angew. Chem., Int. Ed., 2017, 56, 2989–2993 CrossRef CAS PubMed;
(c) Z. Shen, T. Wang, L. Shi, Z. Tang and M. Liu, Chem. Sci., 2015, 6, 4267–4272 RSC;
(d) J. Han, J. You, X. Li, P. Duan and M. Liu, Adv. Mater., 2017, 29, 1606503 CrossRef PubMed;
(e) A. H. G. David, R. Casares, J. M. Cuerva, A. G. CampaÇa and V. Blanco, J. Am. Chem. Soc., 2019, 141, 18064–18074 CrossRef CAS PubMed;
(f) Q. Jiang, X. Xu, P.-A. Yin, K. Ma, Y. Zhen, P. Duan, Q. Peng, W.-Q. Chen and B. Ding, J. Am. Chem. Soc., 2019, 141, 9490–9494 CrossRef CAS PubMed;
(g) Y. Li, Q. Li, X. Miao, C. Qin, D. Chu and L. Cao, Angew. Chem., Int. Ed., 2021, 60, 6744–6951 CrossRef CAS PubMed.
-
(a) A. Szumna, Chem. Soc. Rev., 2010, 39, 4274–4285 RSC;
(b) Y. Shoji, K. Tashiro and T. Aida, J. Am. Chem. Soc., 2006, 128, 10690–10691 CrossRef CAS PubMed;
(c) G.-W. Zhang, P.-F. Li, Z. Meng, H.-X. Wang, Y. Han and C.-F. Chen, Angew. Chem., Int. Ed., 2016, 55, 5304–5308 CrossRef CAS PubMed;
(d) S. Tong, J.-T. Li, D.-D. Liang, Y.-E. Zhang, Q.-Y. Feng, X. Zhang, J. Zhu and M.-X. Wang, J. Am. Chem. Soc., 2020, 142, 14432–14436 CrossRef CAS PubMed;
(e) H. Chai, Z. Chen, S.-H. Wang, M. Quan, L.-P. Yang, H. Ke and W. Jiang, CCS Chem., 2020, 2, 440–452 CrossRef CAS;
(f) R. Ning, H. Zhu, S.-X. Nie, Y.-F. Ao, D.-X. Wang and Q.-Q. Wang, Angew. Chem., Int. Ed., 2020, 59, 10894–10898 CrossRef CAS PubMed;
(g) T. Miura, T. Nakamura, Y. Ishihara, Y. Nagata and M. Murakami, Angew. Chem., Int. Ed., 2020, 59, 20475–20479 CrossRef CAS PubMed;
(h) N. Luo, Y.-F. Ao, D.-X. Wang and Q.-Q. Wang, Angew. Chem., Int. Ed., 2021, 60, 20650–20655 CrossRef CAS PubMed;
(i) X.-N. Han, Y. Han and C.-F. Chen, J. Am. Chem. Soc., 2020, 142, 8262–8269 CrossRef CAS PubMed.
- J. Li, H.-Y. Zhou, Y. Han and C.-F. Chen, Angew. Chem., Int. Ed., 2021, 60, 21927–21933 CrossRef CAS PubMed.
-
(a) M. Inouye, K. Hayashi, Y. Yonenaga, T. Itou, K. Fujimoto, T.-a. Uchida, M. Iwamura and K. Nozaki, Angew. Chem., Int. Ed., 2014, 53, 14392–14396 CrossRef CAS PubMed;
(b) Y. Zhang, D. Yang, J. Han, J. Zhou, Q. Jin, M. Liu and P. Duan, Langmuir, 2018, 34, 5821–5830 CrossRef CAS PubMed;
(c) C. Yang, W. Chen, X. Zhu, X. Song and M. Liu, J. Phys. Chem. Lett., 2021, 12, 7491–7496 CrossRef CAS PubMed;
(d) K. Hayashi, Y. Miyaoka, Y. Ohishi, T.-a. Uchida, M. Iwamura, K. Nozaki and M. Inouye, Chem.–Eur. J., 2018, 24, 14613–14616 CrossRef CAS PubMed.
-
(a) T. Ogoshi, S. Kanai, S. Fujinami, T. Yamagishi and Y. Nakamoto, J. Am. Chem. Soc., 2008, 130, 5022–5023 CrossRef CAS PubMed;
(b) M. Xue, Y. Yang, X. Chi, Z. Zhang and F. Huang, Acc. Chem. Res., 2012, 45, 1294–1308 CrossRef CAS PubMed;
(c) N. L. Strutt, H. Zhang, S. T. Schneebeli and J. F. Stoddart, Acc. Chem. Res., 2014, 47, 2631–2642 CrossRef CAS PubMed;
(d) T. Ogoshi, T. Yamagishi and Y. Nakamoto, Chem. Rev., 2016, 116, 7937–8002 CrossRef CAS PubMed;
(e) S. Fa, T. Kakuta, T. Yamagishi and T. Ogoshi, CCS Chem., 2019, 1, 50–63 CAS;
(f) E. Li, K. Jie, M. Liu, X. Sheng, W. Zhu and F. Huang, Chem. Soc. Rev., 2020, 49, 1517–1544 RSC;
(g) Z. Li and Y.-W. Yang, Acc. Mater. Res., 2021, 2, 292–305 CrossRef CAS;
(h) K. Kato, S. Ohtani, S. Fa and T. Ogoshi, Bull. Chem. Soc. Jpn., 2021, 94, 2319–2328 CrossRef CAS.
-
(a) S. Fa, T. Kakuta, T. Yamagishi and T. Ogoshi, Chem. Lett., 2019, 48, 1278–1287 CrossRef CAS;
(b) J.-F. Chen, J.-D. Ding and T.-B. Wei, Chem. Commun., 2021, 57, 9029–9039 RSC.
-
(a) J.-F. Chen, X. Yin, B. Wang, K. Zhang, G. Meng, S. Zhang, Y. Shi, N. Wang, S. Wang and P. Chen, Angew. Chem., Int. Ed., 2020, 59, 11267–11272 CrossRef CAS PubMed;
(b) W.-J. Li, Q. Gu, X.-Q. Wang, D.-Y. Zhang, Y.-T. Wang, X. He, W. Wang and H.-B. Yang, Angew. Chem., Int. Ed., 2021, 60, 9507–9515 CrossRef CAS PubMed;
(c) J.-F. Chen, X. Yin, K. Zhang, Z. Zhao, S. Zhang, N. Zhang, N. Wang and P. Chen, J. Org. Chem., 2021, 86, 12654–12663 CrossRef CAS PubMed;
(d) H. Zhu, Q. Li, B. Shi, H. Xing, Y. Sun, S. Lu, L. Shangguan, X. Li, F. Huang and P. J. Stang, J. Am. Chem. Soc., 2020, 142, 17340–17345 CrossRef CAS PubMed.
-
(a) T. Ogoshi, K. Kitajima, T. Aoki, S. Fujinami, T. Yamagishi and Y. Nakamoto, J. Org. Chem., 2010, 75, 3268–3273 CrossRef CAS PubMed;
(b) H. Zhu, Q. Li, Z. Gao, H. Wang, B. Shi, Y. Wu, L. Shangguan, X. Hong, F. Wang and F. Huang, Angew. Chem., Int. Ed., 2020, 59, 10868–10872 CrossRef CAS PubMed;
(c) Y. Chen, L. Fu, B. Sun, C. Qian, R. Wang, J. Jiang, C. Lin, J. Ma and L. Wang, Org. Lett., 2020, 22, 2266–2270 CrossRef CAS PubMed;
(d) J. Ji, Y. Li, C. Xiao, G. Cheng, K. Luo, Q. Gong, D. Zhou, J. J. Chruma, W. Wu and C. Yang, Chem. Commun., 2020, 56, 161–164 RSC;
(e) K. Du, P. Demay-Drouhard, K. Samanta, S. Li, T. U. Thikekar, H. Wang, M. Guo, B. van Lagen, H. Zuihof and A. C.-H. Sue, J. Org. Chem., 2020, 85, 11368–11374 CrossRef CAS PubMed;
(f) S. Fa, K. Egami, K. Adachi, K. Kato and T. Ogoshi, Angew. Chem., Int. Ed., 2020, 59, 20353–20356 CrossRef CAS PubMed.
-
(a) T. F. Al-Azemi, M. Vinodh, F. H. Alipour and A. A. Mohamod, RSC Adv., 2019, 9, 23295–23301 RSC;
(b) N. L. Strutt, H. Zhang and J. F. Stoddart, Chem. Commun., 2014, 50, 7455–7458 RSC;
(c) T. Ogoshi, K. Masaki, R. Shiga, K. Kitajima and T. Yamagishi, Org. Lett., 2011, 13, 1264–1266 CrossRef CAS PubMed;
(d) T. Ogoshi, R. Shiga, M. Hashizume and T. Yamagishi, Chem. Commun., 2011, 47, 6927–6929 RSC;
(e) Q. Li, X. Li, L. Ning, C.-H. Tan, Y. Mu and R. Wang, Small, 2019, 15, 1804678 CrossRef PubMed;
(f) S. Fa, M. Mizobata, S. Nagano, K. Suetsugu, T. Kakuta, T. Yamagishi and T. Ogoshi, ACS Nano, 2021, 15, 16794–16801 CrossRef CAS PubMed.
-
(a) Y. Nagata, M. Suzuki, Y. Shimada, H. Sengoku, S. Nishida, T. Kakuta, T. Yamagishi, M. Suginome and T. Ogoshi, Chem. Commun., 2020, 56, 8424–8427 RSC;
(b) T. Ogoshi, R. Shiga, T. Yamagishi and Y. Nakamoto, J. Org. Chem., 2011, 76, 618–622 CrossRef CAS PubMed.
- T. Ogoshi, K. Umeda, T. Yamagishi and Y. Nakamoto, Chem. Commun., 2009, 45, 4874–4876 RSC.
- J. Yao, W. Wu, W. Liang, Y. Feng, D. Zhou, J. J. Chruma, G. Fukuhara, T. Mori, Y. Inoue and C. Yang, Angew. Chem., Int. Ed., 2017, 56, 6869–6873 CrossRef CAS PubMed.
- S. Fa, K. Adachi, K. Egami, K. Kato and T. Ogoshi, Chem. Sci., 2021, 12, 3483–3488 RSC.
- One set of signals was also observed in other protic solvents such as methanol-d4. Details were shown in the ESI.†.
- J. Park, Y. Choi, S. S. Lee and J. H. Jung, Org. Lett., 2019, 21, 1232–1236 CrossRef CAS PubMed.
- Although the g value of S-1 in water was smaller than those enantio-pure examples, a high value was reached after complexation with guests due to stop of the unit swing.
-
(a) X. Hu, H. Deng, J. Li, X. Jia and C. Li, Chin. Chem. Lett., 2013, 24, 707–709 CrossRef CAS;
(b) X. Shu, S. Chen, J. Li, Z. Chen, L. Weng, X. Jia and C. Li, Chem. Commun., 2012, 48, 2967–2969 RSC.
- Y. Ma, M. Xue, Z. Zhang, X. Chi and F. Huang, Tetrahedron, 2013, 69, 4532–4535 CrossRef CAS.
- K. Han, Y. Zhang, J. Li, Y. Yu, X. Jia and C. Li, Eur. J. Org. Chem., 2013, 2013, 2057–2060 CrossRef CAS.
-
(a) H. Anetai, Y. Wada, T. Takeda, N. Hoshino, S. Yamamoto, M. Mitsuishi, T. Takenobu and T. Akutagawa, J. Phys. Chem. Lett., 2015, 6, 1813–1818 CrossRef CAS PubMed;
(b) Y.-Y. Chen, H. Wang, D.-W. Zhang, J.-L. Hou and Z.-T. Li, Chem. Commun., 2015, 51, 12088–12091 RSC;
(c) Y. Ohishi and M. Inouye, Tetrahedron Lett., 2019, 60, 151232 CrossRef CAS.
- At low concentration, the fluorescence maximum was clearly observed at ca. 426 nm, corresponding to the emission of monomeric APy. As concentration increased, a new fluorescence band emerged at ca. 530 nm and gradually red-shift, indicating the formation of the π-oligomer of APy. For detailed discussion, see the ESI.†.
-
(a) J. Zhao and P. Xing, ChemPlusChem, 2020, 85, 1511–1522 CrossRef CAS PubMed;
(b) J. Zhao and P. Xing, ChemPhotoChem, 2022, 6, e202100124 CrossRef CAS.
Footnote |
† Electronic supplementary information (ESI) available: Chemicals, general methods, characterization, procedures and additional figures. See DOI: 10.1039/d2sc00952h |
|
This journal is © The Royal Society of Chemistry 2022 |
Click here to see how this site uses Cookies. View our privacy policy here.