Simultaneous prediction of trihalomethanes, haloacetic acids, haloacetonitriles and haloacetamides using simulated distribution system tests†
Received
10th November 2021
, Accepted 25th February 2022
First published on 25th February 2022
Abstract
This study analysed the spatial and temporal occurrence of 29 disinfection by-products (DBPs) formed by chlorination and chloramination. Four full-scale treatment works, and distribution system locations were sampled, and the results were compared with laboratory-based simulated distribution system (SDS) tests. The DBPs monitored incorporated 4 trihalomethanes (THMs), 9 haloacetic acids (HAAs), 7 haloacetonitriles (HANs) and 9 haloacetamides (HAcAms). For the first time, SDS tests were shown to successfully simulate the levels and speciation of HANs and HAcAms in both chlorinated and chloraminated systems. While THM and HAA concentrations generally increased with water age, HAN and HAcAm concentrations fluctuated and resulted in less pronounced overall increases. To explore the impact of switching the disinfectant in distribution, free chlorine and chloramines were applied in the SDS tests, which showed that chloramination not only reduces the yields of THMs (by 34%) and HAAs (by 49%), but also HANs (by 61%) and HAcAms (by 51%), although it shifts speciation towards more brominated HAAs, HANs and HAcAms species when compared against chlorination. Overall, the aim of the study was to demonstrate that SDS tests can be recommended for the simultaneous estimation of THM, HAA, HAN and HAcAm concentrations in distribution systems and to assess the effect of potential DBP minimisation strategies, such as switching the disinfectant in distribution.
Water impact
Nitrogenous disinfection by-products (N-DBPs) result from unintended reactions between natural organic matter and disinfectants used in drinking water treatment and are known or suspected to pose certain risks to public health. This research demonstrates that simulated distribution system tests are a useful tool to predict their spatial and temporal occurrence in distribution systems, so that water treatment plants are adapted and operated in a way to control N-DBP formation alongside regulated DBPs.
|
1. Introduction
Disinfection of drinking water in treatment and distribution aims to safeguard consumers against the occurrence of waterborne diseases related to microbial contaminants and has been characterised as ‘one of the most significant public health advancements’ of the last century.1 However, research has shown that disinfectants, such as chlorine, interact to varying degrees with natural organic matter (NOM), inorganic compounds and anthropogenic constituents present in drinking water supplies to form disinfection by-products (DBPs).
Disinfection by-products have been a significant area of investigation since the mid-1970s following two publications2,3 that reported the relationship between chloroform formation and the reactions of natural organic matter (NOM) and chlorine. To date, the literature refers to more than 700 DBPs from the main disinfection methods currently implemented, as well as their combinations,4,5 while ∼40% of the mass of organic halogens in water remains unknown.6,7 From this identified amount of DBPs, an even smaller percentage has been quantified in drinking waters and distribution systems.8 Due to their known or suspected health risks,6,9 a number of countries have published regulations and guidelines to control the formation of some species in drinking water.10–15 In England and Wales, the Drinking Water Inspectorate (DWI) in 2012 advised water companies to ‘design, operate and maintain the disinfection process so as to keep DBPs as low as possible, without compromising the effectiveness of disinfection.16 As of January 2021, the revised Drinking Water Directive entered in force maintaining a maximum regulatory level for THMs at 100 μg L−1 and introduced for the first time a regulatory limit for HAA5 at 60 μg L−1.17
To comply and control the formation of regulated THMs and HAAs, water utilities may consider switching from chlorination to chloramination, since it has been widely reported to minimise DBP formation.18–21 Despite the observed positive effects of chloramines, many studies report that the implementation of chloramines may encourage the formation of certain nitrogenous DBPs (N-DBPs)4 or induce other side effects (e.g., promote Br-HAAs).21,22 In fact, further knowledge on occurrence and formation mechanisms of N-DBPs is needed since they are suspected to be more toxic than their non-nitrogenous counterparts.23,24 Furthermore, much of the published research has focused on regulated and unregulated DBP formation within water treatment works, yet there is only sparse information about their ultimate fate in distribution systems, their typical concentrations in tap water and their most important formation factors (Table 1). Indeed, DBPs are known to exhibit significant spatial and temporal variability due to raw water quality fluctuations (e.g. NOM composition, bromide levels) and/or due to the conditions in the distribution networks (corrosion materials, biofilms, disinfectant residuals, pH, water age and temperature). The levels of the most abundant DBP class, THMs, are known to increase with increasing water age and temperature in distribution, whereas some HAAs have been reported to chemically and biologically degrade.25–27 On the contrary, HANs and HAcAms appear to be minimally affected by both water age and temperature.28,29
Table 1 Main formation factors of DBPs of water utilities interest and their expected corresponding occurrence trends (adapted from ref. 4, 28, 60, 62, 74, 81 and 82)
DBPs formation factors |
THMs |
HAAs |
N-DBPs |
HANs |
HAcAms |
Biodegradation.69,82
Depends on the NOM content (algae, blended with treated wastewater effluent).28
Decreases at higher pH (>7)/less stable at lower pH.28,72
Decreases at lower pH (<5)/less stable at higher pH.28,72
|
Concentration of disinfectant |
↑ |
↑ |
∼ |
|
∼ |
Disinfectant contact time |
↑ |
↑↓a |
∼ |
∼ |
∼ |
Concentration of organic/inorganic matter |
↑ |
↑ |
↑b |
↑ |
↑ |
Bromide ions (Br−) |
↑ |
↑ |
∼ |
↑ |
↑ |
Water temperature |
↑ |
↑ |
∼ |
∼ |
∼ |
pH |
↑ |
↓ |
↑↓ |
↑↓c |
↑↓d |
Water age (distribution size) |
↑ |
↑↓a |
∼ |
∼ |
∼ |
↑: increase ↓: decrease |
: no effect |
∼: sparse evidence |
Water utilities and scientists require an evaluating tool to predict DBP occurrence and proactively adapt their water supply processes. As such, the trihalomethane formation potential test (FP), which is a standardised method (5710B),30 was initially implemented to estimate the formation of THMs, and later of HAAs. However, FP tests were mainly used for the purposes of evaluating the impact of precursors and efficiency of treatment processes, rather than predicting the actual concentrations of DBPs in distribution systems, i.e., at consumer taps. The FP test's characteristics – long incubation time (7 days), high incubation temperature (25 ± 2 °C) and excess of chlorine residual (3–5 mg L−1) – mean that it may overestimate actual DBP concentrations.31,32 Furthermore, the relative formation of brominated species was reported to be lower in FP tests compared to reality, due to the lower Br/Cl ratio in the excess chlorine conditions of the FP test.33 Therefore, in the early 1990s, simulated distribution system tests (SDS) where developed and validated as the most appropriate method to realistically predict the formation of trihalomethanes (THMs) in real distribution systems.25,30,81 In the first study by Koch et al.,25 filtered effluent water samples from two different plants were collected, which were then dosed in the lab with chlorine (representative of plant dosage) and incubated in typical distribution temperatures for up to 5 days (6 intermediates). Since then, these tests are recommended by the US EPA to American water utilities and have been implemented in many US studies, and to a lesser extent in Europe, to collect abundant DBP information.
The SDS tests are implemented using a simple and inexpensive laboratory-based technique (batch incubation in bottles), simulating three major parameters: water conditions (pH, temperature), disinfectant residuals and water ages of a distribution system, allowing sampling at prescribed time intervals for analysis of temporal DBP formation trends. Moreover, the SDS test provides key advantages over sampling programmes from real distribution systems, namely:34
• It allows realistic DBP concentration assessment in site specific location, even before commissioning, so that necessary operational adjustments can be done proactively.
• It allows DBPs prediction that would result from proposed operational changes in distribution practices (e.g., switching from chlorination to chloramination).
• It allows quantification of a range of different DBPs and other relevant water quality parameters (e.g., pH, temperature, bromide, chlorine residual) in the same sample taken at the same time.
• It gives an accurate knowledge of and control over water age when determining the DBPs.
• It allows evaluation of the effect of booster chloramination on DBPs.
• It is more cost-effective, less time intensive, and involves less manpower than extensive sampling from the real distribution system.
However, information to-date argues that SDS tests do not account for all the in situ conditions of a distribution system, which may be relevant for some DBPs.35,36 Reducing agents present in pipe materials, such as iron and/or sulphite, result in HAA degradation.37 In addition, degradation of halogenated compounds may be triggered by biotic reactions, typically increased with the presence of bacteria found in biofilms.35,38 Metallic pipe corrosion products and biofilm growth may also exert significant chlorine demand and thus leave less residual disinfectant to react with NOM to form DBPs.39–41 Finally, bottle incubation may not fully represent mixing and hydraulic conditions of a distribution and thus simulate the reaction rates.36 Nonetheless, Krasner et al.42 refers to SDS tests as a method that ‘mimics’ operations and water quality conditions in distribution systems, with reported correlations against real water samples of 0.91, 0.81, 0.98 (R2 value) for THMs, di-halogenated HAAs and tri-halogenated HAAs, respectively. Previous studies have shown that SDS estimated the levels of THMs, HAAs, 3 HANs, HKs and NDMA, either in chlorinated, chloraminated or UV/H2O2 systems,27,31,36,43–47 but otherwise there is little information about whether SDS tests can be used as predictive tools for estimating more N-DBP concentrations in distribution. AWWA studies, using nationally collected data, have reported that the SDS method was a reasonably accurate method of also predicting intermediate fluctuations of THM and HAA concentrations between the treatment plant and distribution system locations.27,48,49 Apart from these, SDS test have also been used as evaluation tool to understand the impact of potential changes to distribution practices; Andrews et al.50 performed SDS tests by using real water matrices to investigate the changes in THMs and HAAs concentrations when replacing chlorine with chlorine dioxide in selected water works.
To the authors' knowledge this study is the first time SDS tests are used to simultaneously predict the formation of 9 HAcAms, 7 HANs, THMs and HAAs in both chlorinated and chloraminated systems. This resulted in one of the most comprehensive databases in Europe of DBP occurrence and behaviour in distribution systems of the commonly regulated trihalomethanes (THMs), the just regulated in EU – haloacetic acids (HAAs), as well as the unregulated haloacetonitriles (HANs) and haloacetamides (HAcAms) of potential health significance, and their individual species. Therefore, the overall aim of the study is to provide a comprehensive insight into the monitoring, prediction and control of these DBPs in distribution systems.
2. Materials and methods
2.1 Selection & sampling of water works and downstream locations
To validate the performance of SDS tests and address the spatial/seasonal variation of DBPs in distribution systems, four water treatment works in England and 12 downstream locations were sampled in four seasonal rounds over a period of one year. The criteria by which the four lowland reservoir water supply systems (Table 2) were selected to obtain fundamental knowledge for the fate of DBPs were the following; i) frequent reported formation of ‘moderate to high’ levels of total THMs (>30 μg L−1), from the assessment of historical data (2007–2015) in final waters and at storage points, ii) data availability to run a distribution system hydraulic model to determine water age variations in distribution, iii) inclusion of both chlorinated and chloraminated systems, iv) variation of geographical locations (varying bromide levels and NOM patterns), v) presence of storage locations (reservoirs, water towers etc.) equipped with sampling taps, vi) no evidence of system nitrification, vii) absence final water blending and absence of booster chlorination in distribution.
Table 2 Specifications of selected water treatment works for simulation distribution system monitoring
Water Works (WTW) |
Pre-oxidant |
Treatment processes |
Final disinfectant |
Bromidea [μg L−1] – avg. |
Non-purgeable Organic carbona [mg L−1 C] – avg. |
pHbavg. |
Total THMsc [μg L−1] – avg. |
Network size [m] |
Water age [hours] |
Historical data from 2007–2018 (final water).
In situ measurement.
Historical data of distribution network from 2007–2018.
|
A |
Ozone |
DAF clarification, rapid gravity filtration, GAC adsorption |
Chlorine |
[10–45] – 21.2 |
[3.1–3.7] – 3.3 |
7.3
|
[6–82] – 27.6 |
1 150 800 |
6 → 144 |
B |
Ozone |
DAF clarification, rapid gravity filtration, GAC adsorption |
Chlorine |
[9.4–48.1] – 25.85 |
[2.9–4.2] – 3.5 |
7.3
|
[5–72] – 26.6 |
698 700 |
6 → 106 |
C |
Ozone |
Clarification, rapid gravity filtration, GAC adsorption |
Chloramines |
[75.7–135] – 97.05 |
[2.1–4.0] – 3.1 |
7.4
|
[5–75] – 32.5 |
6 140 000 |
6 → 144 |
D |
n/a |
Rapid gravity filtration (GAC), Slow sand filtration, aeration |
Chloramines |
[14.5–40.6] – 23.4 |
[1.8–3.4] – 2.6 |
7.3
|
[14–45] – 26.4 |
264 000 |
6 → 72 |
During each seasonal sampling round, two sets of samples were taken from each WTW; water from the treatment works (SDS tests) and from actual distribution locations to compare against the SDS predictions. In the chlorinated WTW, approximately 15 litres of filtered non-disinfected water were collected and preserved until their spiking and incubation as SDS tests. However, in-line chloramination was unable to be simulated in the lab due to health and safety limitations, and therefore the anticipated mimicking of the process was achieved by sampling the final disinfected water. Thus, in chloraminated WTW, SDS tests would initiate at the ammonisation step in the treatment works (contact time was monitored with online telemetry) and quenched at the pre-determined water age intervals during their transport. Following the sampling in the works, water samples were collected and quenched in duplicate from 3 selected downstream locations in each distribution system. All samples were collected in 1–5 L amber glass bottles with PBT (polybutylene terephthalate) screw caps with PTFE (Teflon) protected seal and stored in coolers with ice packs for transportation to the laboratory.
2.2 Water age modelling
The water age in the distribution systems at the sampling locations was modelled for each WTW separately. The software package used was SynerGEE v4.6.0 (DNV GL, Norway). The models were built from the water utility GIS data, which were then calibrated against real time telemetry data and field pressure logging to build a representative simulation of the water distribution systems, with all details of pumps, storage (towers and reservoirs), pressure regulators and mains. The hydraulic simulations were solved using the Darcy–Weisbach equations. The prediction of water age required the continuous input of real time pressures throughout the model run time (>10 days) until stable water age values were calculated, which led to the generation of maps of the water ages ranges (between 6 and 144 hours) across the distribution systems (Fig. 1).
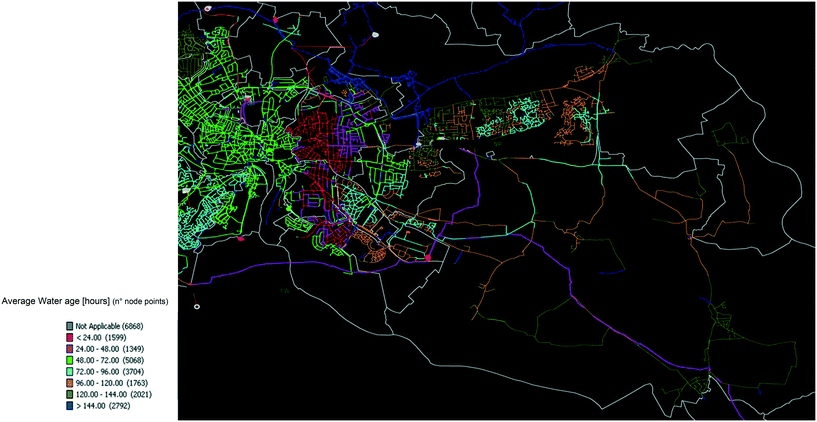 |
| Fig. 1 Water age map designed via SynerGEE v4.6.0 for the selection of water ages in distribution (WTW A). | |
2.3 Experimental design of SDS tests
The SDS test is a bench scale technique that involves incubating water samples at simulated distribution conditions (i.e., disinfectant residuals, temperatures, water ages) [5710 C].30 Prior to disinfectant dosing and preparation, the collected water was incubated to reach the in situ temperature of the sampling date. On the day of the disinfectant spiking, fresh chlorine and monochloramine solutions (N/Cl molar ratio: 1.4/1) were prepared from stock solutions, with concentrations of these oxidants determined and monitored by DPD-FAS titration [4500-Cl F].30 The chlorine/chloramine demand during each round was evaluated with trial-and-error titration experiments on the day of the spiking, to ensure residual disinfectant concentration of 1.0 ± 0.2 mg L−1. Since the results of the SDS tests were to be compared against those of the real distribution system sampling locations, representative water ages ranges in distribution were simulated as exact time intervals (6, 12, 24, 48, 72 and 106 hours) in SDS tests in duplicate.
The preparation of the SDS tests is summarised in the following steps:
a. The water sample was spiked with pre-determined volume of chlorine or chloramine solution (magnet stir plate), to achieve the in situ residual disinfectant concentration.
b. The SDS bottle was filled up to the top (avoid overflowing) until the water-air interface became convex.
c. The bottles were capped headspace free, inverted to mix and checked for trapped air bubbles. If air bubbles were present, the bottle was refilled until no air was observed, to ensure the detection of volatile DBPs.
d. Finally, the SDS bottles were stored in the incubator at the on-site measured temperature and selected contact time to simulate the water ages in distribution.
After the completion of the pre-determined incubation times (6, 12, 24, 48, 72, 106 hours), the samples were quenched with 100 mg L−1 of ammonium chloride (chlorinated samples) and 50 mg L−1 of ascorbic acid (chloraminated samples), to preserve the individual analytes.51–55 Prior to their quenching, residual disinfectant, pH (Mettler-Toledo, UK), UV absorbance at 254 nm (Camspec M550/1 double beam scanning, UK), non-purgeable organic carbon (NPOC) (Shimadzu TOC-V, Japan) were measured and SUVA was calculated (S4†). It has to be noted that if a SDS sample post-incubation had a residual concentration of <0.2 mg L−1 of chloramine, it was disposed and excluded from further assessment.
2.4 DBP analyses
The determination of all target DBPs in this study was performed by liquid–liquid extraction and gas chromatography – electron capture detector (GC-ECD) (Perkin Elmer Clarus 500), using two separate methods; a modified EPA method 551.1 (for THMs, HANs and HAcAms) with a fused silica capillary column (RXi 5Sil MS, 30 m × 0.25 mm ID, 0.25 μm film thickness, Restek, USA) and added temperature increase rates up to 280 °C (Table S1†), and EPA method 552.3 (for HAAs) (Table S2†).51,52 With the analytical methods selected six HANs (MBAN, DCAN, TCAN, BCAN, DBAN, DBCAN) and eight HAcAms (MBAcAm, DCAcAm, TCAcAm, BCAcAm, DBAcAm, BDCAcAm, DBCAcAm, TBAcAm) were able to be quantified in water. For supplementary information on the standards, analytes method detection limits, recovery rates and median values, the reader is referred to Table S3.†
For the purposes of this study, >500 L of water were collected from water treatment works and downstream locations. In total 480 bottles were filled headspace-free for incubation and approximately 1000 extraction vials were taken for solvent extraction and GC-ECD analysis for DBP quantification.
2.5 Data analysis
The data analysis and correlations were performed in Excel and SPSS Statistics 25 (IBM, UK), with the implementation of t-tests and ANOVA packages. The specific tests and Pearson product moment correlation coefficient (r), with 95% confidence interval, were used to assess the statistical relation between the individual DBP yields in SDS tests, actual distribution systems and water quality variables, in chlorinated and chloraminated systems, separately and combined.
3. Results and Discussion
3.1 Occurrence of regulated and unregulated DBPs in SDS tests
3.1.1 In chlorinated water supply systems.
3.1.1.1 Occurrence of THMs and HAAs.
In general, it was observed that SDS tests predicted to a high extent the formation patterns and speciation of THMs (r = 0.97) and HAAs (r = 0.95) in the chlorinated systems (A & B WTW), while illustrating the various spatial and seasonal trends. THM concentrations increased linearly with the increase of water age and decrease of chlorine residual (from 1.0 ± 0.2 to 0.2 mg L−1 Cl2) in the incubation bottles. This trend was consistent with the results obtained from the distribution locations (Fig. 2a and S1a†) and with literature.25,36,56 More specifically, the lowest THM concentrations were observed after 6 hours (minimum incubation time), while the highest were identified after 106 hours (maximum incubation time), during all four seasonal samplings. Between 6 and 106 hours, the THM increases were significant, averaging 60% in the SDS tests (avg. 50% in distribution system). Generally, the concentration of the distribution samples would fall between the concentrations of the simulated samples of the exact minimum and maximum hours of each range. However, in several cases in WTW B, particularly in low water ages (6–12 hours), it was observed that the THMs from the distribution locations exceeded those from the SDS tests of 6 and 12 hours. This was possibly related more to the complex hydraulic conditions that enhanced chlorine reactions in the actual network than in the bottles. It is noteworthy that concentrations increased more rapidly in lower (6–24 hours) than in higher water ages (48–106), which indicated rapid THM generation in the early stages of distribution, possibly even immediately after the disinfection step.57 Even though the increased rates had minimal correlation with water temperature or TOC fluctuation, this was not the case with total mass concentrations in both WTWs, since THM yields varied between the seasonal rounds. In fact, the highest THM concentration reported was 75.4 ± 1.2 μg L−1 after 106 hours during summer (22°), while the concentration of the relative distribution system at 72–106 hours was 71.0 ± 3.2 μg L−1 (Fig. 2a). Likewise, during the winter sampling round (8 °C) simulated tests presented the lowest total concentrations, ranging from 17.0 (±1.4) – 45 (±3.6) μg L−1 (21.0 (±0.2) – 42.0 (±0.2) μg L−1 in distribution system). In addition, SDS tests simulated the formation pattern of the individual THM species of chloroform and brominated THMs in the chlorinated systems.25 Similarly, to the distribution locations, brominated species, and predominantly dibromochloromethane (DBCM), represented the majority of THM occurrence. This indicated that bromine incorporation in the incubation bottles occurred in similar levels as in the actual networks; while bromide levels were approx. 12 μg L−1, chloroform and bromine containing THMs represented 15 and 85% of TTHM, respectively (16 and 84% in distribution system, respectively).
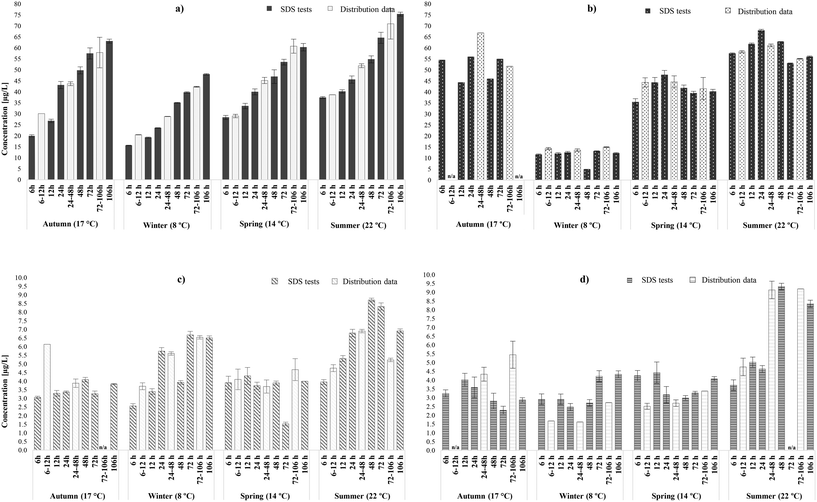 |
| Fig. 2 DBP occurrence of a) THMs, b) HAAs, c) HANs and d) HAcAms from SDS tests (6, 12, 24, 48, 72, 106 hours) and actual distribution samples (6–12, 24–48, 72–106 hours) in chlorinated water treatment works A (4 seasonal rounds). | |
Furthermore, as seen in Fig. 2b and S1b,† SDS tests also simulated HAAs in chlorinated systems during the four seasonal samplings. Overall, it can be noted that the concentrations generated at 6 hours were statistically similar or slightly higher than those occurred in the highest incubation time (106 hours), regardless of the intermediate variations. In any case, the magnitude of HAA yields increase during chlorination (avg. 9%, from 6 to106 hours) was lower than that observed for THMs (avg. 60%). In the SDS tests of WTW A, total HAAs presented an increase from 6 to 24 hours (avg. 15%), where they peaked. After 24 hours, HAAs presented a decrease until 48 hours (avg. 18%), and a subsequent increase until 72 and 106 hours (avg. 5%). The increases were associated with continuous oxidation reactions in the presence of chlorine residual, in low water ages, or the accumulative effect of other DBPs decomposition, in higher water ages.58 On the other hand, the hydrolysis of tri-HAA to their corresponding THMs at the pH reported (pH range: 7.2–7.9)59 functioned as the primary HAA degeneration mechanism. Also, abiotic degradation might have occurred in the SDS samples; hydrolysis or reductive dehalogenation of HAAs37,58 induced with the pH increases observed in two rounds. The likelihood of microbial carry over in the SDS tests was high, due to the sampling of pre-disinfected water, which may also be responsible for biodegradation.38 Additionally, the formation of total HAAs in SDS samples had a strong correlation with water temperature, since significantly lower concentrations were reported when the chlorinated samples were incubated at 8 °C; mean HAA levels were approximately 80%, 71% and 78% greater in summer (22 °C), autumn (14 °C) and spring (17 °C), respectively, than this of winter (8 °C) (downstream location presenting 75%, 67% and 76% greater levels, respectively). These percentages between seasonal samplings are consistent will previous HAAs occurrence studies in the UK,44 but not as dramatic as these reported in Canada,60 that saw four fold lower concentrations during winter. Concerning HAA speciation, the dominant species was DCAA, followed by DBAA, same as those reported in the selected distribution locations. In the selected chlorinated systems, the most prevalent HAAs species are regulated as HAA5. In general, the weight ratios of bromine containing HAAs species identified were higher than that of the chlorinated ones, averaging 60% (58% in downstream locations) (Table S5†). In addition, the weight ratios of regulated (HAA5) to total HAAs were similar in both free chlorinated systems investigated, averaging 69% (68% in downstream locations). This suggested that the non-regulated HAAs comprise just over 1/3 of the HAAs in the selected systems, regardless of the low bromide levels.
3.1.1.2 Occurrence of N-DBPs.
The accurate simulation of the trends and speciation of the THMs and HAAs described above, already known to be well-predicted by SDS tests, validated the performance of the tests in our laboratory. Therefore, the same samples were further assessed to investigate their potential in predicting the occurrence and speciation of nitrogenous DBPs; HANs and HAcAms, for which there was uncertainty about whether the SDS test would be a good predictor. Fig. 2c (A WTW) and S1c† (B WTW) demonstrate that most of total HAN concentrations identified in actual systems were found to be between the concentrations identified in the lower and upper limits of their water age ranges (r = 0.83). However, few exceptions were observed especially in low water ages, where total HANs levels in simulated samples were 2.5–20% lower (max mass difference 2.5 μg L−1) than those in the actual networks. From a practical viewpoint these deviations were minimal, when taking in account the order of magnitude for the WHO guideline values for DCAN and DBAN, 20 and 70 μg L−1, respectively,14 which are significantly higher than the variations observed here. In this case, the underestimation was not linked to determining factors, such as pH (r = −0.05) and chlorine residual (r = −0.41), since these were not statistically different between the two set of samples and was more likely to occur due to the low circulation and mixing conditions in the bottles that may have limited reactions. Total HANs reported by chlorinated SDS tests and downstream locations were always <9 μg L−1, with mean values of 4.8 (±1.7) and 4.9 (±1.5) μg L−1, respectively. Generally, HANs increased between 6 hours and 106 hours, in both chlorinated SDS tests, with varying increase rates; by on average 35%. This has been previously reported by Bond et al.61 and Weinberg et al.8 in nationwide occurrence studies where HAN levels presented increases (by 7–50%) within the initial and final location of real and simulated distribution networks. Regardless of the overall mass increases, decays were also observed in intermediate incubation times. However, these decays were not significant enough to reset the total HAN concentrations to their minimum levels, which were reported only in 6 hours during all four sampling rounds. Even though clear formation/degradation patterns could not be extracted, the simulations data analysis indicated that HANs presented an increase trend until 24 and 48 hours, then decreased at 48 and 72 hours respectively, followed by another increase thereafter. The decreases observed are related to HANs hydrolysis to form their HAcAms counterparts (only chlorinated species), confirmed in correlation analysis (r = 0.74). The above trend is in agreement with Koch et al.25 in chlorinated SDS tests that reported the same water age trend, with HAN levels increased until 24 hours, decreased until 72 hours and then increased back again to be very similar to the initial concentrations of 3 hours. HAN occurrence was not characterised by any seasonal variability, since similar levels were identified in low and high water temperature incubations. This observation suggested that HANs formation was mainly affected by the constituents of the water matrix, rather than the environmental conditions, of each sampling round.28,29,62 Similar to what was observed in distribution, the most predominant HAN species in SDS tests were DBAN, followed by BCAN (brominated species represented 60–100%). An exception was observed in summer, especially during low water ages; chlorine-containing species, and particularly TCAN, were abundant, representing a mean weight fraction of 67%. This was possibly due to the algal blooms and high water temperatures during that period that led to increased chlorine demand,63 thus shifted the chlorine to bromine ratio in the water samples.
Apart from the HAN simulation, SDS tests acted as an equally accurate prediction tool for HAcAms (r = 0.83) in chlorinated systems (Fig. 2d and S1d†). However, several downstream location samples reported higher HAcAm yields than the SDS bottles over the same amount of time (24–106 hours). This was potentially due to the presence of reservoirs of organic materials associated with pipe walls in distribution, which may have acted as supplementary HAcAm precursors at high water ages. The statistically significant deviations between the SDS and distribution samples ranged between 0.5–2.5 μg L−1 (avg. 1.1 μg L−1), therefore total HAcAms in SDS were always <10 μg L−1 in both chlorinated systems, with mean values of 3.6 μg L−1 (3.4 μg L−1 in distribution samples). From the results there was no consistent relationship between HAcAms occurrence and/or the magnitude of intermediate increases/decays, and water age in both chlorinated systems. There was an overall pattern, though, for HAcAms to degrade between 12 and 24 hours, likely linked to HAcAm hydrolysis and subsequent formation of the relative HAAs, confirmed from HAAs levels in the same incubation time during all sampling rounds for WTW A. Then, average concentrations would either increase, partially related to HAN hydrolysis, or would continue to degrade until 106 hours. It is noteworthy that the HAcAm increases in WTW B did not necessarily coincide with the hydrolysis of HANs (r = 0.54), highlighting the potential independent formation of HAcAms from separate precursors.20,64 These different patterns within the same system during the various sampling rounds were not influenced by water temperature (r = 0.2), or any other measured water quality parameter [e.g., pH (r = −0.04), TOC (r = 0.09)], but were more related to the nature of HAcAms precursors of the water matrix. NOM quality is characterised by seasonal variations and therefore changes in molecular weight, solubility and functional group composition.65,66 Concerning HAcAm speciation, the weight ratios of bromine containing HAcAm species were similar, averaging 68% (64% in distribution) and 61% (55% in distribution) during the various incubation times, in A and B WTW SDS samples, respectively (Table S5†). Similar to distribution systems, the most dominant HAcAm species in chlorinated SDS samples was BCAcAm, followed by TCAcAm and DCAcAm. Overall, it was observed that SDS test encompassed the ranges of HANs and HAcAms concentrations and speciation expected to be identified during the different water ages within the chlorinated distribution systems. SDS tests predicted, even conservatively, the total HAcAms levels to be expected in the given water ages.
3.1.2 In chloraminated water supply systems.
3.1.2.1 Occurrence of THMs and HAAs.
Similarly to the chlorinated systems, THM concentrations increased linearly as water age increased and chlorine residual decreased (Table S4†); a trend that was observed during all seasonal sampling rounds at both chloraminated systems (Fig. 3a and S2a†). Total THMs varied between the seasonal sampling rounds, though not as much as in the chlorinated systems, and presented the same seasonal and temporal dependency as their relative distribution samples (r = 0.96). In the chloraminated systems THMs were always <40 μg L−1 even at 106 h, where concentrations were expected to be found in their peak. The increases were noteworthy between 6 and 106 hours, averaging 52% during the four sampling rounds (avg. 51% in distribution system). It has to be noted that unlike the chlorinated samples, the yields here followed higher increase patterns between 48 and 106 hours, than between 6 and 24 hours. This is possibly related to the fact that chloramines are weaker oxidants than free chlorine and therefore initially promote substitution and then oxidation reactions, with increasing formation rates as water age progressed.27 As seen in Fig. S2a† during the autumn tests of D WTW, THMs presented an unexpected decrease (by approx. 23%) at the incubation time of 72 hours, while chloramine residual was <0.5 mg L−1. This was attributable to the decrease of THM species containing chlorine molecules and has been previously observed in SDS tests,27 where anoxic conditions occurring in high water ages were responsible.67 In addition, THM biodegradation may have occurred from the combining effect of nitrifying bacteria and disinfectant decrease.68
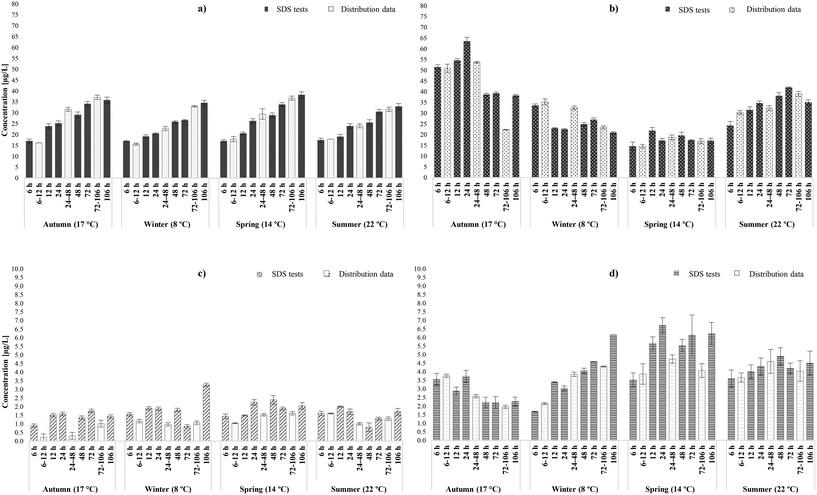 |
| Fig. 3 DBP occurrence of a) THMs, b) HAAs, c) HANs and d) HAcAms from SDS tests (6, 12, 24, 48, 72, 106 hours) and actual distribution samples (6–12, 24–48, 72–106 hours) in chloraminated water treatment works C (4 seasonal rounds). | |
In the case of chloraminated supply systems, THM speciation was also predicted; in WTW C was characterised mainly by brominated THM species (i.e., DBCM and BDCM), whereas SDS samples of WTW D were dominated by BDCM and chloroform. In fact, bromide levels in WTW C (avg. 82 μg L−1) were approximately four times higher than those of WTW D (avg. 20 μg L−1) (Table 2), thus it was expected that the fraction of brominated species would be significantly higher than that of chloroform. Indeed, in WTW C the bromide-containing species constituted 82–94% of the total THMs (76–94% in distribution network). On the other hand, in WTW D the weight fraction ratio of chloroform and bromide-containing was similar (48
:
52), as bromide incorporation was reduced due to relatively low bromide levels (avg. 20 μg L−1). The data agreements between the two set of samples indicated that SDS tests simulated bromine incorporation to a high extent.
As far as the HAAs were concerned, similarly to the actual chloraminated systems (r = 0.91), yields followed both overall increases (∼25%) and decreases (∼60%) trends from 6 to 106 hours amongst the seasonal sampling events, regardless of the intermediate fluctuations, which is consistent with previous studies.19,26,69,70 These trends are related to the pronounced HAA degradation in high temperatures, and the high disinfectant residuals and SUVA (approx. 2.8) found in the systems.18 Other parameters, pH, HAcAms hydrolysis or NOM nature may have acted as equally important triggers for HAA behaviour.27 Overall, it was expected that the yields of the chloraminated downstream locations would fall into the extremities of those identified by the relative SDS tests (r = 0.91). In the few cases that there were statistical deviations between the two set of samples, the average overestimation and underestimation in SDS tests was 8% (max deviation: 6 μg L−1) and 35% (max deviation:15 μg L−1), respectively. The uncertainty of the exact water ages in distribution and inability of SDS to mimic the exact mixing and hydraulic conditions of the water pipes, may have acted as limiting factors in the enhanced prediction of the formation/ decay of HAAs. As in actual chloraminated distribution systems, the dominant HAA species was the currently unregulated TBAA, followed by DBAA and DCAA. Unlike in the chlorinated systems, other unregulated species, such as DBCAA and DBCAA were also present in relative high levels in the chloraminated systems. In general, the weight ratios of bromine containing HAAs species identified were significantly higher than that of the chlorinated ones, averaging 62% (60% in downstream locations) and 59% (57% in downstream locations) in WTW C and WTW D, respectively. In addition, the SDS results predicted with precision the weight ratios of regulated (HAA5) to total HAAs, averaging 28% (33% in downstream locations) and 40% (40% in downstream locations), for C and D WTW, respectively. Greater bromide concentrations, as in WTW C, lead to even lower HAA5-to-HAA9 mass ratios, as the bromine-containing species are not adequately represented by HAA5. These results highlighted the regulatory deficiency to cover the health hazard profile of these chloraminated systems, since the identification of HAA5 is only representative of one third of the total HAAs. From a practical standpoint, the implementation of SDS tests by water utilities will provide a useful insight on the expected HAA levels and speciation in chloraminated systems if further optimised, since contrary to THMs the prediction of HAAs was more water-specific and water age-dependent.
3.1.2.2 Occurrence of N-DBPs.
Overall, it was observed that SDS test encompassed the ranges of HAN (r = 0.74) and HAcAm (r = 0.73) concentrations and speciation expected to be identified within the chloraminated distribution systems. Total HANs were lower than those generated in chlorinated systems28,71 and the levels predicted to generate in the early stages of distribution were similar or slightly higher (by 0.1–1.7 μg L−1) at the final stages, thus increasing with time. In more detail, total HANs were <4 μg L−1 with mean yields of 1.7 μg L−1 (avg. in distr.: 1.9 μg L−1) (Fig. 3c and S2c†). Unlike in chlorinated samples, several chloraminated SDS samples overestimated the occurrence of HANs; especially in low and medium water ages. Nonetheless, these deviations were minimal from a practical viewpoint; maximum deviation reported was just 0.8 μg L−1 (WTW C-Winter). This overestimation was likely due to the different circulation conditions in the bottles that may have enhanced formation and/or delayed hydrolysis. The potential impact of other parameters for this overestimation was possible but not wholly confirmed by the data; low pH (<7) has been reported to enhance HANs stability,72 and lower disinfectant residual in distribution enhances HANs hydrolysis.42 This tendency has been previously reported in chloraminated SDS tests, and SDS bottles characterised by nitrification phenomena.8 Same as in chlorinated SDS tests, HANs increased between 6 and 106 hours in all chloraminated SDS samples, with varying increase rates; 6–52%. This agreed with chloramination experiments by Yang et al.,62 where the effect of contact time was investigated and concluded that HANs increased between 0 and 170 hours of incubation. Regardless of the overall increases in the current study, HAN levels presented decays during the intermediate incubation times. These decays were mainly identified around 48–72 hours, due to hydrolysis,61,73 and were not significant to influence the overall occurrence. The same pattern was observed by Chen et al.,74 where HAN levels (3 species) were reported to increase until 24 hours, with a subsequent decrease until 72 hours. Also, HAN occurrence was not characterised by any seasonal variability upon chloramination, since similar levels were identified amongst the seasonal samplings. Concerning HANs speciation, the most predominant species were DBAN, followed by DCAN and BCAN. During the four sampling rounds the brominated species represented in average 53% and 16% of the total HANs, in WTW C and D water samples, respectively.
Furthermore, Fig. 3d and S2d† illustrate that chloraminated SDS tests succeeded in representing well the different scenarios over the same amount of time and temperature in the case of HAcAms (r = 0.73). If an exception was to be noted, would be the tendency of some SDS samples to minimally overestimate the levels of HAcAms, with deviations ranging 0.2–2 μg L−1 between the different sample sets. Even though literature does not provide any explanation to this tendency, and the incubation bottles were isolated from the known HAcAms triggering factors of distribution pipes, it was possible that inorganic nitrogen incorporation was enhanced during the mixing in confined bottles.75 This assumption was not evaluated during this study since isotopically labelled monochloramine was exempted from the experiments. Total HAcAms in SDS samples were always <7 μg L−1 in both chloraminated systems, with mean values of 4.0 μg L−1 (3.9 μg L−1 in distribution) and 3.6 μg L−1 (3.7 μg L−1 in distribution), in WTW C and D, respectively. Generally, HAcAm concentrations increased between 6 and 106 hours, in three of four sampling rounds (winter, spring, summer) by rates that were inversely proportional to water temperature; partially confirmed from distribution samples. More specifically, in WTW C the increase rate (between 6–106 hours) was significantly higher (73%) in winter, against those in spring (43%) and summer (20%). From the average reported levels in each water age during all sampling rounds, it was observed that total HAcAms followed an increase until 48 hours, with a subsequent degradation around 72 hours followed by a final increase until 106 hours. This HAcAms degradation, though, was not necessarily linked with consequent formation of their relative HAAs, while HAcAms increase was partially linked with HAN hydrolysis. Since this was observed in both chlorinated and chloraminated systems, it indicated that the formation of HAcAms was associated with both nitrogen and non-nitrogen organic precursors found in the samples water matrices,75–77 and could form independently from HANs.64 Furthermore, HAcAm formation in chloraminated waters was not characterised by any seasonal pattern, since the occurrence in 8 °C was similar with that observed in 22 °C.28,72 The weight ratios of bromine containing HAcAms species (Table S5†) were also similar, averaging 81% (86% in distribution) and 66% (70% in distribution) during the various incubation times, in WTW C and D SDS samples, respectively. The above percentages highlighted once more the importance of bromide, even in low concentrations as found in WTW D, in the preponderance of brominated HAcAms in distribution systems. Same as in downstream samples, the most abundant HAcAms species were DBAcAm, DCAcAm and BCAcAm. Finally, Krasner et al.4 had reported that upon chloramination DCAcAm and DCAN usually occur in similar levels. It was noteworthy that observation was reported in this study in both chloraminated systems; DCAcAm and DCAN were on average 0.7 μg L−1 and 0.6 μg L−1 (WTW C), and 1.1 μg L−1 and 1.3 μg L−1 (WTW D), respectively.
3.2 The impact of switching from chlorine to chloramines
A fundamental question posed by this research was the simultaneous impact assessment of a potential disinfection process alteration on the formation and fate of the selected DBPs in distribution which was allowed through the SDS validation. Therefore, water samples from WTW A and B, usually chlorinated by the water utility, were also chloraminated (with pre-formed chloramines) and incubated in the laboratory under the same conditions and water ages as the initial SDS tests. Overall, switching from chlorine to chloramines in both systems, formed lower THMs, HAAs, HANs and HAcAms levels during all the seasonal sampling rounds (Fig. 4). Chloramination is known to control THM and HAA formation when compared with chlorination.20,70,78 The novelty of our findings lay in the fact that chloramination was proven to also limit the formation of both HANs and HAcAms. Therefore, this was an indication that the nitrogen atom in N-DBPs came primarily from the organic nitrogen present in the water matrices, rather than from the ammonia added during chloramination. In more detail, switching from chlorine to chloramines in WTW A, reported average decreases of 30% in THMs, 47% in HAAs, 72% in HANs and 55% in HAcAms. Switching from chlorine to chloramines in WTW B, reported average decreases of 36% in THM, 50% in HAAs, 50% in HANs and 47% in HAcAms. When chlorine was used as the disinfectant, considerable variation was observed between the increase levels of THMs during each water age; with mean yield 45 μg L−1 (range: 20–75 μg L−1) between 6 to 106 hours during all sampling rounds. Whilst when chloramines were applied to the same water matrix mean THMs were 30 μg L−1 and ranged between 16–55 μg L−1. Practically, this means that a potential switch to chloramines will not only decrease the total THMs in specific water ages but also delay their formation; crucial to achieve regulatory compliance in large water networks with water ages >106 hours. Furthermore, the percentage of incorporated bromide in THMs was similar during both chlorination (avg: 86%) and chloramination (avg 87%) of the selected water matrices. The minimisation percentage in total HAAs upon shifting to chloramines was more significant (avg. reduction: 49%) than that of THMs (avg. reduction: 34%). Namely, mean HAA levels during chlorination were 41 μg L−1 and 28 μg L−1 in WTW A and B, respectively, whereas during chloramination were 14.5 μg L−1 and 13 μg L−1 (Fig. 4b). The impact on HAA speciation during chloramination has been widely studied with researchers suggesting that switching from chlorine to chloramines will be followed by a switch in speciation towards dihalogenated HAAs.22,79 The data obtained in this study agreed partially with literature since, especially in WTW A samples, tri-HAAs became the major species in two of the three sampling rounds. This unusual behaviour may be attributable to the ability of ozonation in the treatment works to have efficiently removed important di-HAA precursors,78 mainly of non humic and hydrophilic character.80 Even so, di-HAAs formed during chlorination were substantially higher than those formed during chloramination, by approximately 57%, in line with a similar study by Hua et al.78 where this difference was calculated to be 30%. In addition, the percentage of brominated HAAs was more elevated in chloraminated samples than in chlorinated by 10%, which indicated that the implementation of chloramines enhanced bromide incorporation in total HAAs.
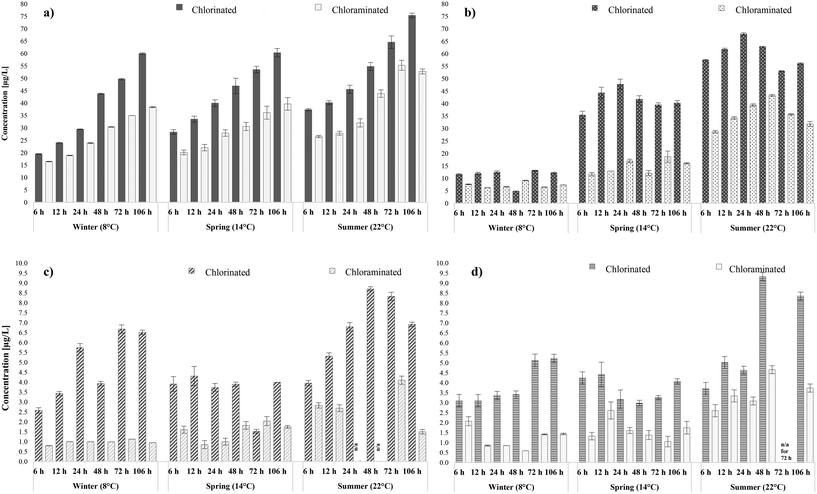 |
| Fig. 4 Comparison of total DBP concentrations in chlorination and chloramination of a) THMs, b) HAAs, c) HANs and d) HAcAms in WTW A. | |
The effect of applying chloramines as a disinfectant was similarly pronounced for HANs (Fig. 4c) and HAcAms (Fig. 4d), and all N-DBP levels were lower than those identified with chlorination during all sampling events. In WTW A, mean HAN yields changed from 4.1 μg L−1 upon chlorination to 1.1 μg L−1 when applying chloramines, whereas mean HAcAms yields changed from 4.1 μg L−1 to 1.6 μg L−1. The maximum total HANs observed during chloramination (4.1 μg L−1) was approximately two times less than the maximum identified during chlorination (8.7 μg L−1), both identified in summer at 72 hours. Likewise, in WTW B the average concentration of HANs changed from 4.3 μg L−1 in chlorinated to 1.8 μg L−1 when using chloramines, whereas mean concentration of HAcAms changed from 4.1 μg L−1 to 2.5 μg L−1. Dominant species quantified during chlorination, such as DCAN and DBAN, were below the detection limits in several water ages during chloramination, which explained the low occurrence of N-DBPs. Overall, the results were comparable to the English nationwide occurrence survey where chloramines were reported to limit N-DBPs formation with similar values of 1.5 μg L−1 for HAN and 3.8 μg L−1 for HAcAm.28 A study by a different research group in the UK by Goslan et al.70 reported HANs mean levels of 1.3 μg L−1 in chloraminated waters. Furthermore, knowledge on the impact of HANs and HAcAms speciation during chlorination is sparse61 and during chloramination is even more limited. In this study the speciation leaned towards the di-HANs and di-HAcAms during both chlorination and chloramination, whereas tri-N-DBPs always occurred in lower levels. Thus, switching from chlorine to chloramines is expected to form less N-DBPs but without any considerable alteration in halogenation levels. In any case, the occurrence of HANs was in fact low and safely below the recommended guidelines values for DBAN (70 μg L−1), and DCAN (20 μg L−1)14 during both disinfection processes. The percentage of bromine-containing HAcAms species was higher than that of chlorine-containing species during all water ages, with minimal variances between the two disinfection practices but with a tendency of higher bromide incorporation during chloramination. In more detail, bromine containing HAcAms represented approx. 50% of total HAcAms yields in chlorination and 62% in chloramination.
4. Conclusions
The key conclusions of this study are:
• Simulated distribution system tests are able to accurately and simultaneously predict the occurrence and speciation of THMs, HAAs, HANs and HAcAms in both chlorinated (r = 0.97, 0.95, 0.87 and 0.83) and chloraminated systems (r = 0.96, 0.87, 0.74 and 0.73).
• SDS tests are recommended to water utilities not only to estimate the levels of the regulated and unregulated DBPs in distribution systems, but also to proactively design and adjust operational distribution disinfection practices.
• Whereas THM concentrations significantly increase with water age in both chlorinated and chloraminated systems, generally, HAA, HAN, and HAcAm concentrations increase to a lesser extent, with levels identified in the early stages of distribution either similar or only slightly higher at the final stages, and with intermediate fluctuations observed. Upon chlorination THMs, HAAs, HANs and HAcAms increased by 62%, 13%, 35% and 3% by mass between the treatment works and the final distribution sampling point, respectively. Upon chloramination THMs, HAAs, HANs and HAcAms increased by 48%, 5%, 43% and 20%, respectively.
• THMs and HAAs showed high seasonal dependence whereas HANs and HAcAms were relatively unaffected by the range of water temperatures tested.
• Switching from chlorine to chloramines resulted in average decreases of 34% in THMs, 49% in HAAs, 61% in HANs and 51% in HAcAms by mass.
• Switching to chloramines highlighted a tendency for higher bromide incorporation into HAAs, HANs and HAcAms.
Author contributions
CS, TB and MT contributed to the conceptualization and methodology of the study. CS then conducted the investigation, formal analysis, data curation and visualisation, while RK provided the validation and resources. The original draft was written by CS, whereas TB, RK and MT contributed further to the writing, reviewing and editing the manuscript.
Conflicts of interest
There are no conflicts to declare.
Acknowledgements
Anglian Water and AW Innovation, UK are gratefully acknowledged for funding this work, providing supply system information, and facilitating seasonal sampling. The authors would also like to specifically thank Barrie Holden and Toni Holtby for their support and guidance.
References
- Centers for Disease Control and Prevention (CDC), A Century of U.S. Water Chlorination and Treatment: One of the Ten Greatest Public Health Achievements of the 20th Century, Morb. Mortal. Wkly. Rep., 1999, 48(29), 621–629 Search PubMed
.
- J. Rook, Formation of haloforms during chlorination of natural waters, Water Treat. Exam., 1974, 23, 234–243 Search PubMed
.
- T. A. Bellar, J. J. Lichtenberg and R. C. Kroner, The occurence of organohalides in chlorinated drinking waters, J. – Am. Water Works Assoc., 1974, 66, 703–706 CrossRef CAS
.
- S. W. Krasner, H. S. Weinberg, S. D. Richardson, S. J. Pastor, R. Chinn and M. J. Sclimenti,
et al., Occurrence of a new generation of disinfection byproducts, Environ. Sci. Technol., 2006, 40(23), 7175–7185 CrossRef CAS
.
- S. D. Richardson and M. J. Plewa, To regulate or not to regulate? What to do with more toxic disinfection by-products?, J. Environ. Chem. Eng., 2020, 8(4), 103939 CrossRef CAS
.
- S. D. Richardson, M. J. Plewa, E. D. Wagner, R. Schoeny and D. M. DeMarini, Occurrence, genotoxicity, and carcinogenicity of regulated and emerging disinfection by-products in drinking water: A review and roadmap for research, Mutat. Res., Rev. Mutat. Res., 2007, 636(1–3), 178–242 CrossRef CAS PubMed
.
- J. G. Pressman, S. D. Richardson, T. F. Speth, R. J. Miltner, M. G. Narotsky and E. S. Hunter,
et al., Concentration, chlorination, and chemical analysis of drinking water for disinfection byproduct mixtures health effects research: U.S. EPAs four lab study, Environ. Sci. Technol., 2010, 44(19), 7184–7192 CrossRef CAS PubMed
.
-
H. S. Weinberg, S. W. Krasner, S. D. Richardson and J. A. D. Thruston, The Occurrence of Disinfection By-Products (DBPs) of Health Concern in Drinking Water: Results of a Nationwide DBP Occurrence Study [Internet], US Environmental Protection Agency, 2002 Search PubMed.
- C. M. Villanueva, S. Cordier, L. Font-Ribera, L. A. Salas and P. Levallois, Overview of Disinfection By-products and Associated Health Effects, Curr. Environ. Health Rep., 2015, 2(1), 107–115 CrossRef CAS PubMed
.
-
EU, Standards and Strategies in the European Union to Control Trihalomethanes (THMs) in Drinking Water, 1997 Search PubMed.
-
US Environmental Protection Agency, Stage 2 D/DBP Rule, Federal Register US Environemental Protection Agency, 2006, vol. 40 CFR 9 Search PubMed.
- Health Canada, Guidelines for Canadian Drinking Water Quality: Guideline Technical Document – Simazine, Heal Living, 2009 Search PubMed.
-
Japanese Ministry of Health L and W. Drinking Water Quality Standards, 2010, (1), pp. 27–28 Search PubMed.
- WHO, WHO guidelines for drinking-water quality, WHO Chron., 2011, 38(3), 104–108 Search PubMed
.
-
NHMRC and NRMMC, Australian Drinking Water Guidelines Paper 6 National Water Quality Management Strategy [Internet], National Health and Medical Research Council, National Resource Management Ministerial Council, Commonwealth of Australia, 2011, p. 1244 Search PubMed.
-
DWI, Guidance on the implementation of the water supply (water quality) regulations 2000 (as amended) in England, Drink Water Insp., 2012, p. 98 Search PubMed.
-
EU, Directive (EU) 2020/2184 of the European Parliament and the Council of the European Union on the quality of water intended for human consumption, Official Journal of the European Communities, 2020 Search PubMed.
- C. M. Bougeard, E. H. Goslan, B. Jefferson and S. A. Parsons, Comparison of the disinfection by-product formation potential of treated waters exposed to chlorine and monochloramine, Water Res., 2010, 44(3), 729–740 CrossRef CAS PubMed
.
-
S. A. Parsons and E. H. Goslan, Evaluation of haloacetic acids concentrations in treated drinking waters (Project No.WT1236), London, UK, 2009 Search PubMed.
- C. Sfynia, T. Bond, R. Kanda and M. R. Templeton, The formation of disinfection by-products from the chlorination and chloramination of amides, Chemosphere, 2020, 248, 125940 CrossRef CAS PubMed
.
- G. A. Cowman and P. C. Singer, Effect of bromide ion on haloacetic acid speciation resulting from chlorination and chloramination of aquatic humic substances, Environ. Sci. Technol., 1996, 30(1), 16–24 CrossRef CAS
.
- A. C. Diehl, S. E. Gerald, J. M. Symons, S. W. Krasner, C. J. Hwang and S. E. Barrett, DBPs during Chloramination, J. – Am. Water Works Assoc., 2000, 92(6), 76–90 CrossRef CAS
.
- M. J. Plewa, M. G. Muellner, S. D. Richardson, F. Fasano, K. M. Buettner and Y.-T. Woo,
et al., Occurrence, synthesis, and mammalian cell cytotoxicity and genotoxicity of haloacetamides: an emerging class of nitrogenous drinking water disinfection byproducts, Environ. Sci. Technol., 2008, 42(3), 955–961 CrossRef CAS PubMed
.
- J. Le Roux, M. J. Plewa, E. D. Wagner, M. Nihemaiti, A. Dad and J. P. Croué, Chloramination of wastewater effluent: Toxicity and formation of disinfection byproducts, J. Environ. Sci., 2017, 58, 135–145 CrossRef PubMed
.
- B. Koch, S. W. Kramer, W. K. Schimpf and M. J. Sclimenti, Predicting the formation of DBPs by the simulated distribution system, J. – Am. Water Works Assoc., 1991, 83(10), 62–70 CrossRef CAS
.
-
P. C. Singer, H. S. Weinberg, K. Brophy, L. Liang, M. Roberts and I. Grisstede, et al., Relative Dominance of HAAs and THMs in Treated Drinking, AWWA Research Foundation, 2002, p. 344 Search PubMed.
-
H. Baribeau, L. Boulos, H. Haileselassie, G. Crozes, P. C. Singer and C. Nichols, et al., Formation and decay of disinfection by-products in the distribution system, AWWA Res Found., 2006, pp. 1–360 Search PubMed
.
-
M. R. Templeton, R. Kanda, N. Graham, H. M. Kamal and T. Bond, Monitoring of nitrogenated DBPs in drinking water, Dep Environ Food Rural Aff., DWI 70/2/2:90, 2012 Search PubMed.
- K. E. Furst, J. Bolorinos and W. A. Mitch, Use of trihalomethanes as a surrogate for haloacetonitrile exposure introduces misclassification bias, Water Res.: X, 2021, 11, 100089 CAS
.
-
APHA, Water Environment Federation and American Water Works Association, Standard Methods for the Examination of Water and Wastewater, Washington DC, 20th edn, 1999, p. 733 Search PubMed
.
- J. A. Brereton and D. S. Mavinic, Field and material-specific simulated distribution system testing as aids to understanding trihalomethane formation in distribution systems, Can. J. Civ. Eng., 2002, 29, 17–26 CrossRef CAS
.
- D. A. Reckhow and J. K. Edzwald, Bromoform and Iodoform Formation Potential Tests as Surrogates for THM Formation Potential, J. – Am. Water Works Assoc., 1991, 83(5), 67–73 CrossRef CAS
.
- B. Chen and P. Westerhoff, Predicting disinfection by-product formation potential in water, Water Res., 2010, 44(13), 3755–3762 CrossRef CAS PubMed
.
-
US Environmental Protection Agency, ICR Treatment Study Fact Sheet: The Simulated Distribution System Test, EPA/815-F-97-002, 1997 Search PubMed.
-
D. A. Reckhow, W. Mitch and C. Park, Fate of non-regulated DBPs in distribution systems, Water Resarch Foundation, 2016 Search PubMed.
- L. A. Rossman, R. A. Brown, P. C. Singer and J. R. Nuckols, DBP formation kinetics in a simulated distribution system, Water Res., 2001, 35(14), 3483–3489 CrossRef CAS
.
-
R. M. Hozalski, W. A. Arnold, C. Chun, T. M. Lapara, J.-Y. Lee and C. R. Pearson, Degradation of Halogenated Disinfection Byproducts in Water Distribution Systems, in ACS Symposium Series, 2008, pp. 334–348 Search PubMed
.
- B. M. McRae, T. M. Lapara and R. M. Hozalski, Biodegradation of haloacetic acids by bacterial enrichment cultures, Chemosphere, 2004, 55(6), 915–925 CrossRef CAS PubMed
.
- J. J. Vasconcelos, L. A. Rossman, W. M. Grayman, P. F. Boulos and R. M. Clark, Kinetics of chlorine decay, J. – Am. Water Works Assoc., 1997, 89(7), 54–65 CrossRef CAS
.
- I. Frateur, C. Deslouis, L. Kiene, Y. Levi and B. Tribollet, Free chlorine consumption induced by cast iron corrosion in drinking water distribution systems, Water Res., 1999, 33(8), 1781–1790 CrossRef CAS
.
- T. Zeng and W. A. Mitch, Impact of Nitrification on the Formation of N-Nitrosamines and Halogenated Disinfection Byproducts within Distribution System
Storage Facilities, Environ. Sci. Technol., 2016, 50(6), 2964–2973 CrossRef CAS PubMed
.
-
S. W. Krasner, C. F. T. Lee, E. Garcia and W. A. Mitch, A simulated distribution system test to predict the formation of nitrosamines, THMs, and HAAs in drinking water, in Water Quality Technology Conference and Exposition, 2011, pp. 2264–2285 Search PubMed.
-
S. W. Krasner, M. J. Sclimenti and E. G. Means, Quality degradation: Implications for DBP formation, American Water Works Association, 1994 Search PubMed.
-
N. Graham, C. Collins, M. J. Nieuwenhuijsen and M. R. Templeton, The Formation and Occurrence of Haloacetic Acids in Drinking Water, Final Report, Anglian Water, Scotish Water and United Utilities, 2009 Search PubMed.
- Y. Zhang, D. Martinez, C. Collins, N. Graham, M. R. Templeton and J. Huang,
et al., Modelling of haloacetic acid concentrations in a United Kingdom drinking water system, J. Water Supply: Res. Technol.--AQUA, 2011, 60(5), 275 CrossRef CAS
.
- I. C. Chien, S. P. Wu, H. C. Ke, S. L. Lo and H. H. Tung, Comparing ozonation and biofiltration treatment of source water with high cyanobacteria-derived organic matter: The case of a water treatment plant followed by a small-scale water distribution system, Int. J. Environ. Res. Public Health, 2018, 15(12), 2633 CrossRef CAS PubMed
.
- D. H. Metz, M. Meyer, A. Dotson, E. Beerendonk and D. D. Dionysiou, The effect of UV/H2O2 treatment on disinfection by-product formation potential under simulated distribution system conditions, Water Res., 2011, 45(13), 3969–3980 CrossRef CAS PubMed
.
-
AWWA, Effects of Water Age on Distribution System Water Quality, United States Environ Prot Agency, 2002 Search PubMed.
-
M. J. McGuire, J. McLain and A. Obolensky, Information Collection Rule Data Analysis, AWWA Res Found, 2002, vol. 628 Search PubMed.
-
R. C. Andrews, Z. Alam, R. Hofmann, L. Lachuta, R. Cantwell and S. Andrews, et al., Impact of Chlorine Dioxide on Transmission, Treatment, and Distribution System Performance: Project #2843, San Francisco, CA, 2005 Search PubMed.
-
D. J. Munch and D. P. Hautman, Method 551.1: Determination of chlorination disinfection byproducts, chlorinated solvents , and halogenated pesticides/ herbicides in drinking water by liquid-liquid extraction and gas chromatography with electron-capture detection, US Environ Prot Agency, 1995, pp. 1–61 Search PubMed.
-
M. M. Domino, B. V. Pepich, D. J. Munch, P. S. Fair, Y. Xie and J. W. Munch, et al., Method 552.3: Determination of Haloacetic Acids and Dalapon in Drinking Water by Liquid-Liquid Microextraction, Derivatization, and Gas Chromatography with Electron Capture Detection, US Environ Prot Agency, 2003, pp. 1–55 Search PubMed.
- D. Liew, K. L. Linge, C. A. Joll, A. Heitz and J. W. A. Charrois, Determination of halonitromethanes and haloacetamides: an evaluation of sample preservation and analyte stability in drinking water, J. Chromatogr. A, 2012, 1241, 117–122 CrossRef CAS PubMed
.
- I. Kristiana, A. Lethorn, C. Joll and A. Heitz, To add or not to add: The use of quenching agents for the analysis of disinfection by-products in water samples, Water Res., 2014, 59, 90–98 CrossRef CAS PubMed
.
- S. Ding, W. Chu, S. W. Krasner, Y. Yu, C. Fang and B. Xu,
et al., The stability of chlorinated, brominated, and iodinated haloacetamides in drinking water, Water Res., 2018, 142, 490–500 CrossRef CAS PubMed
.
- M. J. Rodriguez and J.-B. B. Sérodes, Spatial and temporal evolution of trihalomethanes in three water distribution systems, Water Res., 2001, 35(6), 1572–1586 CrossRef CAS PubMed
.
-
US Environmental Protection Agency, EPA Drinking Water Guidance on Disinfection By-Products Advice Note No. 4. Version 2. Disinfection By-Products in Drinking Water, Environmental Protection Agency Office of Environmental Enforcement, 2010 Search PubMed.
- X. Zhang and R. A. Minear, Decomposition of trihaloacetic acids and formation of the corresponding trihalomethanes in drinking water, Water Res., 2002, 36(14), 3665–3673 CrossRef CAS PubMed
.
- D. A. Reckhow, P. C. Singer and R. L. Malcolm, Chlorination of humic materials: byproduct formation and chemical interpretations, Environ. Sci. Technol., 1990, 24(11), 1655–1664 CrossRef CAS
.
- R. Sadiq and M. J. Rodriguez, Disinfection by-products (DBPs) in drinking water and predictive models for their occurrence: A review, Sci. Total Environ., 2004, 321(1–3), 21–46 CrossRef CAS PubMed
.
- T. Bond, M. R. Templeton, N. H. Mokhtar Kamal, N. Graham and R. Kanda, Nitrogenous disinfection byproducts in English drinking water supply systems: Occurrence, bromine substitution and correlation analysis, Water Res., 2015, 85, 85–94 CrossRef CAS PubMed
.
- X. Yang, C. Shang and P. Westerhoff, Factors affecting formation of haloacetonitriles, haloketones, chloropicrin and cyanogen halides during chloramination, Water Res., 2007, 41(6), 1193–1200 CrossRef CAS PubMed
.
-
D. R. U. Knappe, C. R. Belk, D. S. Briley, S. R. Gandy, N. Rastogi and A. H. Rike, Algae Detection and Removal Strategies for Drinking Water Treatment Plants, American W., Denver, CO, 2004, p. 470 Search PubMed
.
- H. Huang, Q. Y. Wu, H. Y. Hu and W. A. Mitch, Dichloroacetonitrile and dichloroacetamide can form independently during chlorination and chloramination of drinking waters, model organic matters, and wastewater effluents, Environ. Sci. Technol., 2012, 46(19), 10624–10631 CrossRef CAS PubMed
.
- E. L. Sharp, S. A. Parsons and B. Jefferson, Seasonal variations in natural organic matter and its impact on coagulation in water treatment, Sci. Total Environ., 2006, 363(1–3), 183–194 CrossRef CAS PubMed
.
- E. H. Goslan, C. Seigle, D. Purcell, R. Henderson, S. A. Parsons and B. Jefferson,
et al., Carbonaceous and nitrogenous disinfection by-product formation from algal organic matter, Chemosphere, 2017, 170, 1–9 CrossRef CAS PubMed
.
- P. C. Singer, D. R. Pyne, M. Avs, C. T. Miller and C. Mojonnier, Examining the Impact of Aquifer Storage and Recovery on DBPs, J. – Am. Water Works Assoc., 1993, 85, 85–94 CrossRef CAS
.
- D. G. Wahman, A. E. Henry, L. E. Katz and G. E. Speitel, Cometabolism of trihalomethanes by mixed culture nitrifiers, Water Res., 2006, 40(18), 3349–3358 CrossRef CAS PubMed
.
- W. Bayless and R. C. Andrews, Biodegradation of six haloacetic acids in drinking water, J. Water Health, 2008, 6(1), 15–22 CrossRef CAS PubMed
.
- E. H. Goslan, S. W. Krasner, M. Bower, S. A. Rocks, P. Holmes and L. S. Levy,
et al., A comparison of disinfection by-products found in chlorinated and chloraminated drinking waters in Scotland, Water Res., 2009, 43(18), 4698–4706 CrossRef CAS PubMed
.
- C. Sfynia, T. Bond, N. Ganidi, R. Kanda and M. R. Templeton, Predicting the formation of haloacetonitriles and haloacetamides by simulated distribution system tests, Procedia Eng., 2017, 186, 186–192 CrossRef CAS
.
-
UKWIR, Disinfection By-Product Risk Assessment: DBPs of Concern (Final Report), UK Water Ind Res Ltd., 2014, vol. 87 Search PubMed.
- V. Glezer, B. Harris, N. Tal, B. Iosefzon and O. Lev, Hydrolysis of haloacetonitriles: Linear free energy relationship, kinetics and products, Water Res., 1999, 33(8), 1938–1948 CrossRef CAS
.
- W. J. Chen and C. P. Weisel, Halogenated DBP Concentrations in a Distribution System, J. – Am. Water Works Assoc., 1998, 90(4), 151–163 CrossRef CAS
.
- X. Yang, C. Fan, C. Shang and Q. Zhao, Nitrogenous disinfection byproducts formation and nitrogen origin exploration during chloramination of nitrogenous organic compounds, Water Res., 2010, 44(9), 2691–2702 CrossRef CAS PubMed
.
- Y. H. Chuang, D. L. McCurry, H. H. Tung and W. A. Mitch, Formation pathways and trade-offs between haloacetamides and haloacetaldehydes during combined chlorination and chloramination of lignin phenols and natural waters, Environ. Sci. Technol., 2015, 49(24), 14432–14440 CrossRef CAS PubMed
.
- W. Chu, X. Li, T. Bond, N. Gao and D. Yin, The formation of haloacetamides and other disinfection by-products from non-nitrogenous low-molecular weight
organic acids during chloramination, Chem. Eng. J., 2016, 285, 164–171 CrossRef CAS
.
- G. Hua and D. A. Reckhow, Comparison of disinfection byproduct formation from chlorine and alternative disinfectants, Water Res., 2007, 41(8), 1667–1678 CrossRef CAS PubMed
.
-
S. W. Krasner, C. F. T. Lee, R. Chinn, S. Hartono, H. S. Weinberg and S. D. Richardson, et al., Bromine incorporation in regulated and emerging DBPs and the relative predominance of mono-, di-, and trihalogenated DBPs, Proc AWWA WQTC Denver, Color AWWA, 2008, pp. 1–16 Search PubMed.
- N. Ates, M. Kitis and U. Yetis, Formation of Chlorination by-Products in Waters with Low SUVA—correlations with SUVA and Differential UV Spectroscopy, Water Res., 2007, 41(18), 4139–4148 CrossRef CAS PubMed
.
-
S. E. Hrudey and J. W. A. Charrois, Disinfection By-Products and Human Health, IWA Publishing, UK, 2012, p. 324 Search PubMed
.
- P. Zhang, T. M. LaPara, E. H. Goslan, Y. Xie, S. A. Parsons and R. M. Hozalski, Biodegradation of Haloacetic Acids by Bacterial Isolates and Enrichment Cultures from Drinking Water Systems, Environ. Sci. Technol., 2009, 43(9), 3169–3175 CrossRef CAS PubMed
.
Footnote |
† Electronic supplementary information (ESI) available. See DOI: 10.1039/d1ew00824b |
|
This journal is © The Royal Society of Chemistry 2022 |
Click here to see how this site uses Cookies. View our privacy policy here.