DOI:
10.1039/D1AN01432C
(Paper)
Analyst, 2022,
147, 55-65
A bifunctional immunosensor based on osmium nano-hydrangeas as a catalytic chromogenic and tinctorial signal output for folic acid detection†
Received
9th August 2021
, Accepted 9th November 2021
First published on 12th November 2021
Abstract
As a neglected member of the platinum group elements, osmium, the metal with the highest density in the earth, is very suitable for the preparation of a peroxidase with high catalytic activity and stability, and can also be associated with the development of a sensor. In this study, we accessed Os nano-hydrangeas (OsNHs) with one-pot synthesis and utilized them in a bifunctional immunosensor that can present both catalytic chromogenic and tinctorial signal for nanozyme-linked immunosorbent assay (NLISA) and lateral flow immunoassay (LFIA) for use in folic acid (FA) detection. In the OsNHs-NLISA, the linear range is from 9.42 to 167.53 ng mL−1. The limit of detection (LOD) is 4.03 ng mL−1 and the IC50 value is 39.73 ng mL−1. In OsNHs-LFIA, the visual cut-off value and limit of detection (v-LOD) are 100 ng mL−1 and 0.01 ng mL−1, respectively. Additionally, the outcome from the specificity and spiked sample analysis offered recovery from the spiked milk powder sample ranging from 93.9 to 103.6% with a coefficient of variation under 4.9%, compared with UPLC-MS/MS for a correlation of R2 = 0.999 and admirable validation. The promising application of the OsNHs can also be used in other bioprobes, and this bifunctional immunosensor analysis mode is suitable for diversified analytes.
1. Introduction
Noble metal elements, often with their extraordinary properties,1,2 are widely used in nanotechnology as catalysts,3,4 signal enhancement,5 medical treatment,6 and novel sensors with surface-enhanced Raman spectroscopy (SERS),7–9 and photothermal10–12 and electrochemical13,14 signal output. Quoting present reports,15–17 classic noble metal element studies are excessively focused on the development of Au and Pt, which are overly expensive for commercial manufacturing, and lack sufficient development for new elements. The pursuit of a relatively inexpensive, durable alternative with prominent performance is still a trendy topic of research on noble metals today. As a member of the Pt group of elements, osmium (Os) has attracted attention for being the densest natural element existing in the earth.18 Furthermore, it possesses superior properties of inferior compressibility, high melting point, and outstanding bulk modulus, as reported by recent work.19,20 In spite of the fact that Os is usually mined with Pt group element ores,21,22 it is barely employed for industrial and commercial purposes as much as Pt and other platinum group elements.23–25 Recently, along with the development and application of noble metal nanoparticles, Os nanomaterials have gradually emerged in application for some organic synthesis reactions26,27 or oxidases mimics.28 However, the peroxidase-like activity of OS as a platinum group element has been scarcely investigated. This is partly because the development of nanoparticles, such as Pt and Pd NPs, to enhance the peroxidase-like activity has been affected by the activity of the oxidase.29,30 In addition, for some developed Os nanoparticles, it is imperative to constitute the alloys with other metal elements such as Pt, Ru and Ir or in its oxide form to achieve their catalytic properties.31–33 Os nanoparticles in elementary substance have been barely reported. Recently, with the emerging attention of the study about the peroxidase-like activity and other properties of the Os nanomaterials in sensing34 and cancer therapy,35 there is increasing interest to exploit the novel Os nanomaterials for a wider range of applications at the same time. Inspired by the present work, we have designed osmium nano-hydrangeas (OsNHs) by a one-pot synthesis to employ a peroxidase mimic with distinguished catalytic activity and chemical stability. These easily prepared OsNHs can be used as a nanozyme to catalyze the decomposition of H2O2. At the same time, there will be free form O2 and utilized to correspond to a colorimetric sensor.
The enzyme-linked immunosorbent assay (ELISA) has contributed to progress in applications, such as clinical diagnosis, food quality control, and environmental monitoring.36–38 Traditional ELISA is based on the specific binding of the antigen and antibody with enzyme catalysis, while the catalytic activity of the enzyme and its catalytic effect prevent it from higher sensitive detection.39 Moreover, the properties of the natural nanozymes, including the narrow thermophilic scope, short shelf life, and easy inactivation, seriously diminish the capabilities of ELISA.40,41 In recent years, novel types of nanomaterials have emerged with a view to their unique optical, electrical, magnetic and catalytic properties, giving the opportunity for application to ELISA enhancement.42 An increasing number of studies have shown that the introduction of nanomaterials-based ELISA can significantly improve the sensitivity of the assay, and also reduce the operational costs by introducing novel multiple signal outputs such as colorimetric, fluorescent, electrochemical, photothermal, and Raman scattering sensing.43 However, the overly homogeneous assay mode can only solve one problem in ELISA. At the same time, there are the excessive testing time costs, making it difficult to apply those techniques industrially. It is noteworthy that the point-of-care (POC) technique has aroused the attention of immunoassay for rapid detection, which meets the demands of affordable, sensitive, specific, rapid, user-friendly and equipment-free methods for the ultimate-users.44 One such tool is lateral flow immunoassay (LFIA), which is commonly used in the medical diagnostics, food toxicity testing and chemical contamination for environment.45–47 Recently, the dual-function detection mode developed with new nanomaterials has attracted attention, in which the nanozyme-based ELISA can achieve the quantitative analysis, while the nanomaterial-labeled LFIA is applied for qualitative analysis.48,49
In this study, we introduce a bifunctional immunosensor based on OsNHs, which creates a catalytic chromogenic signal on TMB for nanozyme-linked immunoassay (NLISA) as a quantitative assay and tinctorial signal for LFIA as a qualitative assay (Scheme 1). To the best of our knowledge, it was the first report of the osmium nano-hydrangeas and its application in immunoassays. As a model analyte, folic acid (FA), one of the most important classes of water-soluble vitamins in the human body was determined in biological samples.50,51 The activity of the proposed OsNHs as peroxidase mimetics was evaluated. Moreover, the OsNHs was a promising tool and applied in two typical types of immunoassays, which can be widely used in environmental-containment monitoring, disease diagnosis, and other applications.
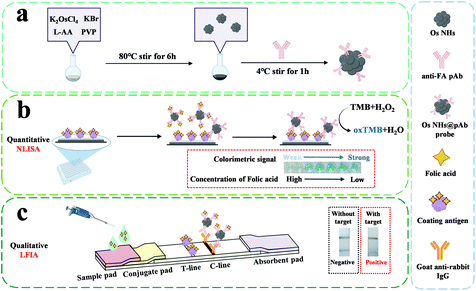 |
| Scheme 1 Illustration of the synthesis for OsNHs (a) and OsNHs-based difunctional immunosensor for folic acid detection: (b) OsNHs-based nanozyme-linked immunosorbent assay (NLISA) for quantitative assay; (c) OsNHs-based lateral flow immunoassay (LFIA) for qualitative detection. | |
2. Methods
2.1 Materials and reagents
The particular chemicals and apparatuses are described in ESI.† All reagents were of analytical quality.
New Zealand white rabbits were obtained from Guangdong Medical Animal Center (Foshan, China). All animal procedures were performed according to the Guidelines for Care and Use of Laboratory Animals of Guangdong University of Technology (Guangzhou, China), and experiments were approved by the Animal Ethics Committee of Guangdong University of Technology (Guangzhou, China).
2.2 Synthesis and characterization of OsNHs
Based on the previous reported procedure,52 OsNHs were assessed using the one-pot synthesis as follows with minor modifications. Briefly, 16 mL of an aqueous solution including 210 mg of PVP, 180 mg of L-AA, and 600 mg of KBr was hosted in a round flask and ultrasonically dissolved for 5 min. Then, the mixture was preheated at 80 °C in an oil bath under vigorous magnetic stirring for 10 min. Subsequently, 6 mL of a K2OsCl6 aqueous solution (17.4 mM) was added to a tube under ultrasonic dissolution for 5 min, and slowly added to the mixture with a pipet. After the flask had been clamped, the mixture was continued at 80 °C for 6 h under intense agitating. The OsNHs were obtained after centrifugation, washed with DI water for three times, and redispersed in 40 mL water for future use.
The detailed characterization and instruments information for OsNHs can be obtained from the ESI.†
2.3 Evaluation of the peroxidase-like activity of OsNHs
As one kind of chromophoric substrate, TMB was selected for the oxidation catalysis of OsNHs at room temperature. In an exemplary experiment, 300 μL of TMB (0.4 mM in phosphoric–citric acid buffer, 0.05 M, pH 4.0), 600 μL of H2O2 (10 mM in water), and 300 μL of OsNHs dispersion (diluted 500 times in ultrapure water) were added to a tube and mixed for 5 min. After a while, the mixture was collected into a clean cuvette and the absorbance at 652 nm was tested by an ultraviolet spectrophotometer. To further demonstrate the serviceability of the OsNHs as a peroxidase mimic, other chromophoric substrates such as o-phenylenediamine (OPD) and 3,3′,4,4′-biphenyltetraminetetrahydrochloride (DAB) were used for comparison. A photograph of the reaction solutions was recorded.
To discover the effect of the working environment and make a contrast, different pH (2 to 13) environments were pre-processed for both HRP and OsNHs for 30 min. After the pretreatment, the OsNHs samples were centrifuged, precipitated and washed several times before being redissolved in ultrapure water. Both HRP and OsNHs samples were diluted 1000 times to catalytic TMB for chromogenic signal, and the absorbance at 450 nm was tested after using 2 M H2SO4 to stop the reaction. Temperature stability tests were applied by adjusting different temperatures (20–90 °C) for OsNHs and HRP for 30 min incubation, and testing after 5 min cooling to room temperature for the samples.
The study of the steady-state kinetics experiment was employed based on the peroxidase-like activity of OsNHs. During the experiment, the substrate (TMB or H2O2) was alternatively changed, while the other stayed invariantly. The elaborate steady-state kinetics experiment procedure and the achieved data were fitted to the Michaelis–Menten equation using Origin 8.5, and the detailed calculation of the enzyme kinetic parameters are further discussed in ESI.†
2.4 Preparation for antigens and antibody against folic acid
The tailored immunogen was made by the glutaraldehyde method with inconsequential modification. Concurrently, the designed coating antigen (FA-OVA) was conducted while using OVA as the carrier protein for substitution. The more specific information and acquisition of the polyclonal antibody (pAb) against FA is displayed in the ESI.†
2.5 Conjugation of OsNHs@pAb probe
The OsNHs@pAb probe conjugation was prepared by electrostatic adsorption.53 In short, 1 mL of OsNHs was added into a tube, and 2 μL of K2CO3 (0.2 M) was successively added and expeditiously agitated for 5 min. Secondly, the above-mentioned system was introduced with 5 μL of purified pAb against FA and moderately mixed for 1 h at 4 °C. Aiming to intercept the redundant sites of the OsNHs, 100 μL of BSA blocking solution (100 mg mL−1) was reacted with the system for an additional 1 h. Superfluous free antibody and BSA may influence the subsequent work. Therefore, the mixture was fully centrifuged at 11
000 rpm for 10 min in low temperature conditions. The final precipitate was assembled and resuspended in 50 μL reconstitution solution. The OsNHs@pAb probe was preserved at 4 °C before use.
2.6 Indirect competition based on OsNHs-NLISA
Under the optimal conditions shown in ESI,† an indirect competitive OsNHs-NLISA was prepared as follows: (1) 100 μL of FA-OVA (0.25 μg mL−1) diluted in carbonate buffer was successively added into individual wells of microplates and incubated at 37 °C for 2 h; (2) 280 μL of 3% skim milk powder solution (m/v) was added to block the plates at 37 °C for 1 h after it had been washed five times with PBST buffer (PBS including 0.05% Tween-20); (3) the microplates were sequentially washed with PBST for five times again, and 50 μL of the OsNHs@pAb probe was diluted at 1
:
250 in PBS and mingled together with 50 μL FA standards with different concentrations, either with its analogs (0.1, 1, 10, 100, 1000, 10
000, 100
000 ng mL−1) for 1 h; (4) after a thorough washing, 100 μL substrate solution (consisting of H2O2 and TMB) was rapidly added and incubated at 37 °C for 10 min; (5) before assessing the absorbance at 450 nm, the chromogenic reaction was accomplished by 50 μL 2 M H2SO4. The LOD of the Os NHs-NLISA was determined as the standard concentration of FA that contributed a 10% inhibition rate (IC10). Meanwhile, the linear range of this quantitative analysis mode was formulated between the values of IC20 and IC80.54
2.7 Fabrication of the OsNPs-LFIA immunosensor
As shown in Scheme 1, the OsNHs-based immunochromato-graphic test strip was agonized with four parts: sample, conjugate and absorbent pad, as well as the indispensable NC membrane. On the NC membrane, the control line (C-line) was scribed with goat anti-rabbit antibody (1 mg mL−1) with the amount of 1 μL cm−1, while the FA-OVA (0.5 mg mL−1) was scribed on the NC membrane with the amount of 1 μL cm−1 as the test line (T-line). The OsNHs@pAb probe conjugation was attached on the conjugate pad with the amount of 8.5 μL cm−1. Then, these four parts were adhered to the base plate in sequence. After the fabrication, the whole strip was dried at 37 °C for 2 h, and then cut into smaller strips with the width of 5 mm, which was preserved in a sealed plastic bag at 4 °C for future use. The assembly of the AuNPs-LFIA immunostrip was the same as mentioned above. Detailed results of the optimization for the OsNHs-LFIA and AuNPs-LFIA experiments can be obtained from ESI.†
During an integrated analysis experiment, a series of concentrations of FA standard solutions or samples were beaded onto the sample pad with the amount of 100 μL, and the sample flowed through the strips for a duration of 10 min. The test result could be visually read by naked eyes and treated as a record by means of a smartphone (Honor V20) camera, which was used to take a photograph for each strip.
2.8 Specificity of the Os NHs-NLISA and OsNHs-LFIA immunosensor
In order to define the specificity performance for the OsNHs bifunctional immunosensor, cross-reactions were completed with different structural and functional analogical chemicals by a competitive method. For OsNHs-NLISA, the cross-reactivity (CR) was formulated as reported by the equation of CR % = [IC50 (FA)/IC50 (structural analogue)] × 100%.
For the OsNHs-LFIA immunoassay, the selectivity determination was assessed by adding two adjoining concentrations (102 and 103 ng mL−1) of FA and other structurally related analogues. Results were recorded by naked eyes and as a photograph by smartphone.
2.9 Spiked sample analysis and validation achievement
Milk powder samples were collected from the supermarket in Guangzhou, China. The pretreatment procedure was cited from the previous literature. The milk powder samples (1 g) were dissolved in a methanol–water mixture at 1
:
1 solution (v/v, 10 mL) and thoroughly mixed for 10 min. The solution was then centrifuged (10
000 rpm, 30 min, 4 °C) and the supernatant was collected. To eliminate the matrix interference, dilution was always considered as an effective way.55 Herein, each sample supernatant was diluted 100 times with PBS before being spiked. Triplet samples were reinforced by the addition of a standard solution of FA to collect the spiked samples with the ultimate concentration of 50, 100, and 150 ng mL−1 and analyzed straight-forwardly without any supplementary treatment. The statistical recovery results were determined by OsNHs-NLISA, while OsNHs-LFIA was employed as a qualitative evaluation.
Further verification of the validity of OsNHs-NLISA was based on the analysis by UPLC-MS/MS. The same preprocessing spiked milk powder samples with added FA standard solution to final concentrations of 50, 100, and 150 ng mL−1 were analyzed by UPLC-MS/MS.
3. Results and discussion
3.1 Characterization of the OsNHs and OsNHs@pAb probes
It is worth noting that the successful synthesis of nanoparticles is the key to the whole research project, especially the proper size of the nanoparticles, which is relevant to the subsequent research and applications. To demonstrate the morphology and size information of the OsNHs, TEM (Fig. 1a) was performed to observe the OsNHs, which has a hydrangea-like appearance. From the statistics of 100 random OsNHs, it can be seen that the average particle size is about 27.65 ± 0.21 nm (Fig. 1b). We have also investigated the impact by adjusting the reaction times on the morphology size of the nano-hydrangeas. As shown in Fig. 2, the average diameter of the nano-hydrangeas was depreciated apparently from 79 nm to 27 nm and 23 nm from the SEM images, when the reaction time was prolonged from 3 h to 6 h and 12 h. It is noteworthy that the involvement of the KBr exhibits an imperative action in the formation of nano-hydrangeas, for the reason that the surface of the nano-hydrangeas is most likely encompassed by a relatively thin layer of Br− ions.52 In this case, the Os surface can be protected from the oxidative etching derived from the reductant. However, this protection around the Os surface has become flimsy owing to the increment of the reaction time, wherein the oxidative etching shows no restraint to the collapse of the diameter of OsNHs. Additionally, many studies have pointed out that the oversized nanoparticles induce the exaggerated size for formation of the nanomaterials-antibody probe, which can gravely affect the results of LFIA in particular, as the exaggerated size of the probe hangs in the conjugate pad and cannot successfully proceed on the test strip.56 Nanoparticles of the ideal diameter (20–50 nm) would be principally suitable for NLISA and LFIA.43,57 In conclusion, the reaction time was defined at 6 h for time-cost and subsequent application considerations.
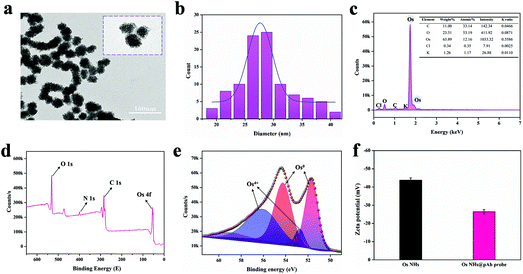 |
| Fig. 1 Characterization of the OsNHs and OsNHs@pAb probes. (a) The TEM image of OsNHs. (b) The size distribution analysis of 100 random OsNHs. (c) EDS spectra of OsNHs. (d) XPS spectrum of the OsNHs. (e) The high-resolution XPS spectra of the Os 4f region. (f) Zeta potential result of OsNHs and OsNHs@pAb. | |
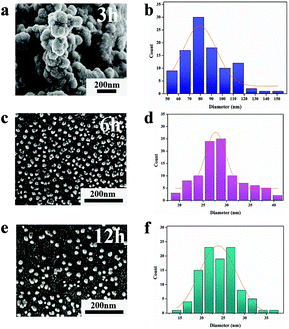 |
| Fig. 2 Investigating the effect of the reaction time of 3 h (a and b), 6 h (c and d) and 12 h (e and f) on the particle size of the morphology produced by OsNHs though the SEM image. | |
Energy dispersive X-ray spectroscopy (EDS) spectral analysis results reveal that the Os element predominate the surface of the OsNHs. Moreover, the EDS results (Fig. 1c) show that many other elements may come from the raw material, such as the K and Cl may be derived from K2OsCl6 and KBr, and C and O may originate in the PVP and L-AA. For studying the states of OsNHs, X-ray photoelectron spectroscopy (XPS) results (Fig. 1d) were conducted to evaluate the chemical components of the OsNHs, which show the detection of the activity of the O 1s, C 1s, N 1s and Os 4f atomic orbitals. Further research of the Os 4f (Fig. 1e) indicates that the Os species of the OsNHs exist in the states of Os0 and Os4+. Accordingly, referring to previous research,58 the Os0 signals (4f5/2 and 4f7/2) appeared at about 51.7 and 54 eV, while Os4+ forms as a chelate and the binding energy is located at 52.7 and 56.1 eV (4f5/2 and 4f7/2). In evidence, the signals of the peak of Os0 are more intensive than the Os4+ one, which expound that Os0 occupies the majority of the presented OsNHs. Moreover, the typical absorbance between K2OsCl6 and OsNHs (Fig. S1a†) demonstrated that the absorption peak of K2OsCl6 was not detectable in the aqueous system of OsNHs in the dispersed state, indicating that the OS4+ present in K2OsCl6 only occupies a minuscule fraction of OsNHs. Therefore, the actively catalytic performance of OsNHs may result from the Os0 on the surface. Quoting the previous report,59 noble metal nanoparticles are surrounded by charge on the surface in aqueous colloid solution, which means that it can bond with the polyclonal antibodies though electrostatic interaction while the antibodies has an opposite charge. The zeta potential results (Fig. 1f) can provide a good demonstration, indicating that the OsNHs was −43.79 mV and the OsNHs@pAb probe conjugation reached −26.41 mV, which showed that the polyclonal antibody and OsNHs were successfully connected in consequence of the electrostatic interaction. To take it even further, the higher absolute value of the zeta potential indicated that the nanoparticles were thoroughly distributed, suggesting that the well-prepared OsNHs can be thoroughly dispersed in water and maintain desirable stabilization for 30 days (Fig. S1b†). The concentration of Os in the OsNHs determined by ICP-MS was 277.54 μg mL−1.
3.2 Assessment of peroxidase-like performance for OsNHs
Generally, TMB is usually considered as a chromophoric substrate in enzyme-based assays for its unexceptionable and steady performance in chromogenic reaction, especially in the moderate protein-friendly environment. Therefore, the characteristic absorption at 652 nm (A652 nm) of the chromogenic signal for TMB was surveyed to convey the peroxidase-like activity. Evidently, the TMB can be catalyzed by OsNHs with H2O2 into ox-TMB that exhibited the color of a sapphire blue signal with a representative absorbance summit at 652 nm. As shown in Fig. 3a, the A652 nm signal of the OsNHs-TMB-H2O2 system was more evident than that of the OsNHs-TMB and TMB-H2O2 systems, announcing an encouraging peroxidase-like performance of the OsNHs instead of the oxidase-like activity. Reportedly, the oxidase-like activity is ordinarily performed by the compositions and uncovered facets of the OsNHs.60 In Shen's opinion, the oxidase activity of some noble metal elements is usually related to the activation (Eact) and reaction energies (Er) of catalyzing the dissociation of O2, using the density functional theory.61 The typical Pt and Pd among the platinum group elements have prominent oxidase-like activity on their facets because their dissociation performance for O2 is thermodynamically and kinetically supportive (Eact < 1.0 eV, Er < 1.9 eV). Therefore, by comparison, it can be inferred that one of the reasons for the unsatisfactory oxidase activity of Os is that Os has high Eact on the facet dissociation of O2, which is theoretically undesirable. Another considerable reason may be related to the weak adsorption of O2 on the surface of Os, which may be related to the outcome that Os is easily bound to other ligands or another Os, resulting in the vast majority of O2 in the dissociative state on the Os facet.60 In conclusion, the OsNHs feature an indisputable peroxidase-like performance and scarcely present any oxidase-like activity.
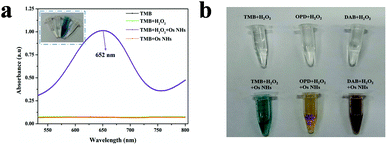 |
| Fig. 3 Peroxidase-like activity performance of OsNHs. (a) The UV-Vis spectrogram of OsNHs, TMB + H2O2, TMB + OsNHs, and TMB + OsNHs + H2O2 (insert shows the image of each solution). (b) A photograph taken at the reaction time of 5 min, showing the capability of the OsNHs in catalyzing the oxidations of various peroxidase substrates (i.e., TMB, OPD and DAB). | |
In contrast, the investigation between the OsNHs and K2OsCl6-TMB-H2O2 indicate that Os4+ barely showed an absorbance signal at 652 nm (Fig. S2†), which means its effect on A652 nm can be ignored. Combined with the results of the previous section, it can easily be said that there are large numbers of the Os0 on the surface of the nanoparticle that are responsible for the catalytic activities as a peroxidase mimic. Additionally, different chromophoric substrates such as OPD and DAB were employed to manifest the viability for peroxidase activities of OsNHs (Fig. 3b). As shown in the graphic, with the catalytic performance of OsNHs, the OPD offered a yellow color and DAB produced brown sediments, demonstrating that OsNHs can contribute peroxidase activities to diverse substrates in different environments.
Ordinarily, HRP enzymes suffer from severe temperature and pH effects. Meanwhile, the inorganic nanoparticle-based peroxidases are able to surpass the HRP enzymes in this regard. The outcomes from Fig. 4 revealed that OsNHs maintain higher and more stable activity than HRP at different temperatures and pH, especially in the working environment for the immunoassay (pH 7.0, T = 37 °C). Moreover, an overly acidic or alkaline environment can lead to a decrease in OsNHs activity (Fig. 4a), due to the partial dissolution of the metal nanoparticles. In contrast, OsNHs were more stable to temperature (Fig. 4b), with a scarcely significant decrease in activity as the temperature ascended, and maintained a relative activity level above 60% even at higher temperature (90 °C). Additionally, by conducting comparisons between the short and long-time storage stabilities, the peroxidase-like activity and morphological characteristics of OsNHs remain the same as when the nanozyme was original prepared (Fig. S9†), which is unmatchable to HRP, and indirectly leads to an exceptional sensing performance of OsNHs compared to HRP.
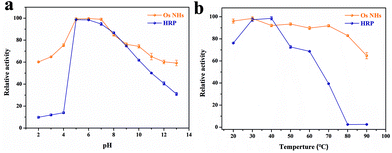 |
| Fig. 4 Stability studies of HRP vs. OsNHs under different pH (a) and temperature (b). | |
On further study of the mechanisms of the peroxidase-like activity of OsNHs, an experiment was carried out to study the intermediates produced in the catalytic oxidation reaction by OsNHs. A range of radical scavengers were used to capture the relevant reactive oxygen species (ROS), such as O2˙− (trapped by PBQ), ˙OH (trapped by thiourea and TA), and 1O2 (trapped by NaN3).62 The results from Fig. S10a† indicated that ˙OH and 1O2 were distinct intermediates. Such forceful and effective analytical method of ROS was electron spin resonance (ESR) spectroscopy. Two spin trapping agents were therefore selected for the detection of the radical signal generated by OsNHs in the catalytic reaction in ESR.63 As shown in Fig. S10b and c,† it is evidently revealing that the intensity of the ESR spectra of DMPO/˙OH indicated the formation of ˙OH in the H2O2 solution through the addition of OsNHs, as well as the formation of 1O2 by sensing the ESR signal through adding TEMP in the OsNHs-H2O2 system, which has compellingly illustrated that the decomposition of H2O2 by OsNHs generates mainly ˙OH and 1O2 as radicals.
The steady-state kinetic parameters for the OsNHs were also surveyed to expose the peroxidase-like performance of the OsNHs with TMB and H2O2. The enzyme kinetic parameters containing the Michaelis–Menten constant (Km) and maximum reaction rate (υmax) were settled. Furthermore, the turnover number (Kcat) and Kcat/Km value were determined. As shown in Fig. 4, after fitting the typical curve for OsNHs employed by the Michaelis–Menten equation under the advisable ranges of TMB and H2O2, the Km value of TMB is obviously lower than the H2O2 one, indicating the more formidable affinity of OsNHs to TMB compared to H2O2 (Fig. 5b and d). In addition, the Km value of TMB towards OsNHs was much lower than the other metal and metal oxide peroxidase mimics, especially HRP, Pt, and Pd, which implied that OsNHs showed excellent affinity performance for the TMB (Table S1†). For further demonstration, the OsNHs can provide higher Kcat and Kcat/Km value, revealing an extraordinary catalytic performance for the substrates in per unit volume concentration, which is much closer to the Pt- and Pd-based nanomaterials. This turns out to clarify the exceptional sensing performance.
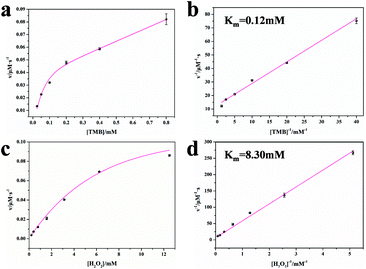 |
| Fig. 5 Steady-state kinetic assays of OsNHs as catalysts of the oxidation of TMB by H2O2. Kinetic assays toward TMB: (a) plot of the initial reaction velocity (v) against the TMB concentration, in which the H2O2 concentration was fixed at 10 mM; (b) double-reciprocal plot generated from (a). Kinetic assays toward H2O2: (c) plot of the initial reaction velocity (v) against the H2O2 concentration, in which the H2O2 concentration was fixed at 0.4 mM; (d) double-reciprocal plot generated from (c). | |
3.3 Characterization of the antigen and antibody against FA
Reportedly, the excellent performance and specificity of the antibodies often depends on the successful synthesis of antigens. Due to the lack of immunogenicity, hapten molecules often need to attach to carrier proteins. The connection of the haptens to the carrier proteins to assemble as antigens depends on the interaction of their amino group and carboxyl group. Fortunately, the structure of the FA target molecules has both amino group and carboxyl group, which means the carrier proteins can bind to the target molecules from these two different sites.64 To distinguish from the previous works, we performed a glutaraldehyde method to combine carrier proteins and FA target molecules from its amino group site. The successful synthesis for the antigen is presented in Fig. S3.† In virtue of the SDS-PAGE, we can observe that the bands of the antigenic protein were significantly higher than those of BSA. Furthermore, we used MALDI-TOF-MS to determine the molecular weight of the antigen, and the result from Fig. S3b† shows the molecular weight of FA-BSA was 72035, which was higher than that of the BSA, and the FA on the successful coupling are about 13. Those well-prepared antigens were applied to immunized rabbits.
For further assay, FA-OVA was applied to become a coating antigen. Generally, different kinds of modifications of the coating antigen may lead to the effect of homologous and heterologous assays.65 To explore this difference, we coupled OVA to both amino group and carboxyl group to FA. As the SDS-PAGE result showed in Fig. S3c,† both bands of FA-NH2-OVA and FA-COOH-OVA were higher than the OVA one, which indicate the successful preparation of two kinds of coating antigens for future assay.
3.4 Analytical performance
Inspired by the satisfactory catalysis performance of the OsNHs, we designed a NLISA to replace the conventional ELISA, which can economize the time of the incubation of the secondary antibody. To achieve the best NLISA performance, we optimized the dilution ratio and the amount of the coating antigen. As shown in Fig. S4a,† by determining the chessboard assay, the dilution ratio of 1
:
1000 was applied for the corresponding absorption value near 1.0, while 0.25 μg mL−1 of coating antigen was enough for sufficient colorimetric signal. Moreover, the lower amount of coating antigen would bring the higher sensitivity for NLISA, thus 0.25 μg mL−1 of coating antigen was considered as the optimal condition. Furthermore, different kinds of coating antigen will bring diverse effects for the sensitivity for NLISA, especially for the homologous and heterologous effects. For this reason, we had designed an experiment of competitive NLISA to explore the different sensitivities of FA-NH2-OVA and FA-COOH-OVA. From Fig. S4b,† the results from setting FA-NH2-OVA as a coating antigen shows the IC50 value is 398.55 ng mL−1, while the FA-COOH-OVA used as a coating antigen brings the lower IC50 value of 39.73 ng mL−1, which has improved by approximately 10 times. This use of FA-COOH-OVA as the coating antigen brings the heterologous effect, which results in the antibodies having more deficient affinity than the homologous one. Hence, during the competitive NLISA, most of the antibodies were inclined to the target FA rather than the heterologously modified one combined with OVA. Eventually, the improvement of the sensitivity turns out to be the inadequate affinity of both antibodies and heterologous coating antigens.
Under the above improvement, the OsNHs-based NLISA was studied and contrasted with the conventional HRP-ELISA. As show in Fig. 6a, the standard curve of the OsNHs-based NLISA presents the IC50 value of 39.73 ng mL−1. The LOD of the assay is 4.03 ng mL−1, and the linear detection was in the range of 9.42–167.53 ng mL−1. By contrast, the values of IC50 and LOD of the conventional HRP-ELISA are 249.09 ng mL−1 and 12.28 ng mL−1, respectively. Based on this outcome, the OsNHs-based NLISA shows an unexceptionable improvement of sensitivity and detection limit compared to the conventional HRP-ELISA, which is nearly 6.3-fold and 3 times for the promotion, respectively.
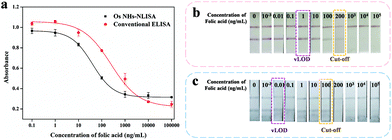 |
| Fig. 6 Sensitivity of the OsNHs-NLISA, OsNPs-LFIA and AuNPs-LFIA immunosensors for the detection of FA in PBS. (a) Comparation of the standard curves of OsNHs-NLISA and the conventional HRP-ELISA. OsNHs-LFIA by naked eyes (b), AuNPs-LFIA by naked eyes (c). | |
Generally, the OsNHs can be dispersed in the water condition, showing a dark-grey color and the conjugation of the antibodies nearly makes a difference. Therefore, the OsNHs@pAb probe can be applied to become a tinctorial signal probe to replace the tradition colloidal AuNPs for the lateral flow immunoassay. As show in Fig. S5,† optimization of the OsNHs-LFIA was conducted from the pH and ionic strength environment, volume of both polyclonal antibody and OsNHs, as well as the T-line concentration. Integrated with those desirable conditions, a competitive assay was applied to the OsNHs-LFIA and AuNPs-LFIA by contrast. FA as a diminutive target molecule is suitable for analysis in LFIA using a competitive method, so we defined samples without FA as negative (both T- and C-line present) and samples with FA as positive (only C-line present). Here, we determine the visual limit of detection (vLOD) as the minimum FA concentration at which the signal of T-line is more light-colored than the negative control strip of FA. Furthermore, the cut-off value is considered as the minimum FA concentration at which the color signal of T-line is nearly observed.66 The OsNHs-LFIA and AuNPs-LFIA results can be obtained from Fig. 6b, in which it can be notably found that the T-line tinctorial signal gradually weakened with the decrease by the standard concentration of FA. Originated from the definition, the cut-off and vLOD value of OsNHs-LFIA for FA are 100 ng mL−1 and 0.01 ng mL−1, respectively, while the AuNPs-LFIA shows these two index values at 200 ng mL−1and 1 ng mL−1. Generally speaking, the OsNHs-LFIA can become a satisfactory substitution of traditional AuNPs-LFIA and shows higher sensitivity performance.
Universally, the analytical performance of the OsNHs bifunctional immunoassay was compared to the other conventional methods and advanced methods for FA detection (Table S2†). Although those conventional methods are stable and sensitive, they require the medium of large instruments and specially trained operators, which is cost prohibitive. In addition, those advanced methods, though innovative, take longer to detect and are relatively homogeneous detection methods. In contrast, the OsNHs bifunctional immunoassay exhibits extraordinary stability and increased detection sensitivity, and also saves time in testing, relatively. The previous work has distinctly pointed out that the desirable analytical performance derives from its excellent sensing performance, especially compared to other work. On the one hand, the excellent catalytic properties of OsNHs allow for better sensing per unit volume, resulting in increased detection sensitivity due to the sensitivity of the OsNHs@pAb probe to the chromogenic substrate. On the other hand, the abundant electrical charge on the OsNHs surface attracts more antibodies to bind electrostatically, allowing the OsNHs@pAb probe to recognize the target more efficiently, thus improving the sensitivity and range of the target detection. What is more, the dual-function detection mode achieves both fast and easy qualitative analysis and accurate and sensitive quantitative analysis, while the traditional ELISA and other nanomaterials based-NLISA or LFIA can only achieve one detection way.
3.5 Specificity of the bifunctional immunoassay
To demonstrate the level of specificity of the method, both OsNHs-NLISA and OsNHs-LFIA were applied as bifunctional immunoassays for FA detection at the same time as some structural analogues (such as components of FA such as pteroic acid (PA) and 4-aminobenzoic acid (PABA)), products of FA in the human body (such as dihydrofolic acid (DHFA) and tetra-hydrofolic acid (THFA)), and structural resemblance in the human body (such as methotrexate ((MTX), N-(4-aminobenzoyl)-L-glutamic acid (FIGLU) and hydrocortisone (H-CORT).67,68 As shown in Fig. 7a, OsNHs-NLISA performed absolute obvious specificity for FA, while the other analogues exhibited lower CR value. It is noteworthy that the CR values of DHFA and THFA (0.18% and 0.17%) indicate that the OsNHs-NLISA performed a slight inhibiting effect towards them. This is because the DHFA has 2 more hydrogen atoms, while THFA has 4 more than FA, indicating that the antibodies show little affinity to them. Additionally, PA and MTX showed low affinity given their structural resemble outlook. After all, the heterologous coating antigen FA-COOH-OVA has pteridine side exposure, which means that the antibodies have a slight affinity for this site. Meanwhile, the outcome from Fig. 7b showed that the T-line disappears, while the FA concentration achieved 1000 ng mL−1, but other analogues showed obvious T-lines through the detection, presenting the exceptional specificity level for OsNHs-LFIA.
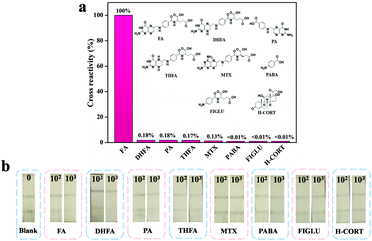 |
| Fig. 7 Specificity research results of the OsNHs dual immunosensor. Cross-reactivity of the antibody to FA and analogue by OsNHs-NLISA (a). 102 and 103 ng mL−1 of FA and its structural analogues were used in the OsNHs-LFIA (b). PBS buffer was used as a negative control. All experiments were performed in triplicate. | |
3.6 Recovery study of the milk powder samples
To evaluate the effectiveness of the OsNHs bifunctional immuno-assay in a real sample context, FA was spiked into real milk powder, for powdered milk is the FA supplement of choice for pregnant women and infants.69,70 As FA is more abundant in regular commercially available infant formula, the milk powder samples were all diluted 100 times to reduce the impact. These three commercially available samples of pre-treated milk powder were spiked with FA at final concentrations of 50, 100, and 150 ng mL−1 and each one was detected by the OsNHs bifunctional immunosensor in triplicate. In the OsNHs-NLISA performance (Table 1), the average recovery levels (n = 3) ranged from 93.9 to 103.6% with coefficient variation values (CV) of 0.9–4.9%, which is within a satisfactory field. The recovery levels determine the desirable precision outcome of the OsNHs-NLISA. The sample was also testified by OsNHs-LFIA for qualitative assay. As the result show in Fig. S7,† the T-line on the strip still shows a sensitive response to FA within the milk powder samples at the level of 50, 100, and 150 ng mL−1. The tinctorial signal of the T-line is divided into three levels: positive (“++”) indicated that the T-line completely disappeared; medi-positive (“+−”) indicated that the T-line almost disappeared; negative (“−−”) indicated that the T-line was obviously observed by naked eyes.49 Herein, the bifunctional immunoassay of OsNHs-NLISA for quantitative analysis and OsNHs-LFIA for qualitative analysis exhibits an exceptional correlation (Fig. 8a). What is more, the same pre-treated spiked samples were determined by UPLC-MS/MS for quantitative analysis as well. Linearization of the results obtained by those two methods gives a very good correlation between each other and the R2 value is 0.999, revealing that the OsNHs-NLISA has a pleasant accuracy and stability (Fig. 8b). In that event, the OsNHs bifunctional immunoassay is a competent, accurate and efficient immunoassay suitable for the detection of real samples.
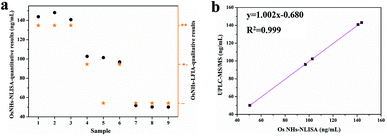 |
| Fig. 8 (a) Difunctional detection performance between OsNHs-NLISA vs. OsNHs-LFIA results for spiked milk powder samples. (b) Correlation between the result from OsNHs-NLISA and that from UPLC-MS/MS for spiked milk powder samples. In OsNHs-LFIA: “++” indicates that the T-line completely disappeared (positive): “+−” indicates that the T-line almost disappeared (medi-positive); “−−” indicates that the T-line was obviously observed (negative). | |
Table 1 Recovery of FA from spiked milk powder samples by the OsNHs-based bifunctional immunosensor
Samples |
Spiked level (ng mL−1) |
OsNHs-NLISA |
OsNHs-LFIA |
Founded (ng mL−1) |
Recovery (ng mL−1) |
CV (%) |
Qualitative results |
Each sample was repeated in triplicate (n = 3). |
Sample 1 |
150 |
143.8 ± 5.9 |
95.9 ± 3.9 |
4.1 |
++ |
100 |
102.7 ± 2.0 |
102.7 ± 2.0 |
1.9 |
+− |
50 |
51.8 ± 1.1 |
103.6 ± 2.2 |
2.1 |
−− |
Sample 2 |
150 |
148.1 ± 1.4 |
98.7 ± 0.9 |
0.9 |
++ |
100 |
101.4 ± 2.7 |
101.4 ± 2.7 |
2.6 |
−− |
50 |
50.2 ± 1.9 |
100.4 ± 3.8 |
3.7 |
−− |
Sample 3 |
150 |
140.8 ± 7.0 |
93.9 ± 4.7 |
4.9 |
++ |
100 |
96.9 ± 3.0 |
96.9 ± 3.0 |
3.1 |
+− |
50 |
50.1 ± 0.9 |
100.2 ± 1.8 |
1.8 |
−− |
4. Conclusions
In summary, we have conducted a one-pot synthesis method to present Os nano-hydrangeas as a nanozyme with prominent peroxidase-like catalytic properties and desirable stability, compared to the natural peroxidase and other nanomaterial-based peroxidase mimics. Based on this exceptional performance, a bifunctional immunosensor based on the OsNHs with both was applied to detect FA. Under a series of optimization parameters, the sensitivity of OsNHs-NLISA improved by 6.3-fold compared to the conventional HRP-ELISA, while the vLOD of OsNHs-LFIA reached 0.01 ng mL−1. Additionally, the outcome from the specificity and spiked sample analysis demonstrates that this method is widely applicable, specific, sensitive, and capable of handling real samples, which provides a new way for the detection of folic acid in the food and pharmaceutical industries. Overall, this Os nano-hydrangeas trademark can also be applied to other bioprobes, such as protein and miroRNA, and the bifunctional immunosensor analysis mode is suitable for some targets of cancers, pathogenic bacteria and epidemic viruses.
Author contributions
Junkang Pan: conceptualization, formal analysis, data curation, methodology, writing – original draft, and investigation. Oiyi He: data curation, methodology, writing – review & editing. Zhiting Lao, Jingyi Su, Yikui Zou, Zekai Chen: data curation. Suqing Zhao: project administration, resources, supervision, and writing – review & editing. Xiping Cui, Qinglan Li, Yanfei Cai: supervision, and writing – review & editing.
Conflicts of interest
There are no conflicts to declare.
Acknowledgements
This work was financially supported by the Guangzhou Science and Technology Foundation (201903010034), Natural Science Foundation of Guangdong Province (2018A030313926), Science and Technology Foundation Key R&D Program of Guangdong Province (2019B020209009; 2019B020218009), and R&D Program of Guangdong Province Drug Administration (2021TDZ09; 2021YDZ06).
Notes and references
- Z. Fan and H. Zhang, Chem. Soc. Rev., 2016, 45, 63–82 RSC.
- K. A. Stancheva, Oxid. Commun., 2012, 35, 662–673 CAS.
- J.-L. Liu, B. Jiang and G.-Z. Han, Curr. Org. Chem., 2020, 24, 855–869 CrossRef CAS.
- C.-q. Zhou, J. Han and R. Guo, Acta Polym. Sin., 2020, 51, 517–529 CAS.
- K. Shah, N. K. Sharma and V. Sajal, Opt. Quantum Electron., 2018, 50, 265 CrossRef.
- E. Boisselier and D. Astruc, Chem. Soc. Rev., 2009, 38, 1759–1782 RSC.
- T. T. Bich Quyen, W.-N. Su, C.-H. Chen, J. Rick, J.-Y. Liu and B.-J. Hwang, J. Mater. Chem. B, 2014, 2, 5550–5557 RSC.
- Q. Cui, G. Shen, X. Yan, L. Li, H. Moehwald and M. Bargheer, ACS Appl. Mater. Interfaces, 2014, 6, 17075–17081 CrossRef CAS PubMed.
- Z. Y. Bao, D. Y. Lei, R. Jiang, X. Liu, J. Dai, J. Wang, H. L. W. Chan and Y. H. Tsang, Nanoscale, 2014, 6, 9063–9070 RSC.
- D. Kumar, R. K. Soni and D. P. Ghai, Nanotechnology, 2020, 31, 035704 CrossRef CAS.
- W.-E. Hong, I. L. Hsu, S.-Y. Huang, C.-W. Lee, H. Ko, P.-J. Tsai, D.-B. Shieh and C.-C. Huang, J. Mater. Chem. B, 2018, 6, 5689–5697 RSC.
- J. He, J. Dong, Y. Hu, G. Li and Y. Hu, Nanoscale, 2019, 11, 6089–6100 RSC.
- T. Xiao, J. Huang, D. Wang, T. Meng and X. Yang, Talanta, 2020, 206, 120210 CrossRef CAS PubMed.
- Y. Wei, H. Ma, X. Ren, C. Ding, H. Wang, X. Sun, B. Du, Y. Zhang and Q. Wei, Sens. Actuators, B, 2018, 256, 504–511 CrossRef CAS.
- T. K. Sau, A. L. Rogach, F. Jaeckel, T. A. Klar and J. Feldmann, Adv. Mater., 2010, 22, 1805–1825 CrossRef CAS PubMed.
- L. L. Tan, M. Y. Wei, L. Shang and Y. W. Yang, Adv. Funct. Mater., 2021, 31, 2007277 CrossRef CAS.
- J. Wu, K. Qin, D. Yuan, J. Tan, L. Qin, X. Zhang and H. Wei, ACS Appl. Mater. Interfaces, 2018, 10, 12954–12959 CrossRef CAS PubMed.
- H. Cynn, J. E. Klepeis, C. S. Yoo and D. A. Young, Phys. Rev. Lett., 2002, 88, 135701 CrossRef PubMed.
- U. Nithiyanantham, S. R. Ede and S. Kundu, J. Mater. Chem. C, 2014, 2, 3782–3794 RSC.
- S. R. Ede, U. Nithiyanantham and S. Kundu, Phys. Chem. Chem. Phys., 2014, 16, 22723–22734 RSC.
- S. Varghese, R. P. Ramesh, R. Pillai, S. R. Joseph, B. Gopakumar, R. Sathikumar, R. K. Joshi, P. D. Guha, R. V. Manoj and M. Nagasundaram, Curr. Sci. India, 2021, 120, 1740–1748 CAS.
- P. Sahu, M. S. Jena, N. R. Mandre and R. Venugopal, Miner. Process. Extr. Metall. Rev., 2020, 42, 521–534 CrossRef.
- S. B. He, L. Yang, M. T. Lin, P. Balasubramanian, H. P. Peng, Y. Kuang, H. H. Deng and W. Chen, Biomed. Mater., 2021, 16, 032001 CAS.
- Q. Li, S. S. Zheng, Y. X. Xu, H. G. Xue and H. Pang, Chem. Eng. J., 2018, 333, 505–518 CrossRef CAS.
- J. Zhang, G. Wang, Z. Q. Liao, P. P. Zhang, F. X. Wang, X. D. Zhuang, E. Zschech and X. L. Feng, Nano Energy, 2017, 40, 27–33 CrossRef CAS.
- K. Fujita, S. Umeki and H. Yasuda, Synlett, 2013, 947–950 CrossRef CAS.
- T. J. Donohoe, R. M. Harris, S. Butterworth, J. N. Burrows, A. Cowley and J. S. Parker, J. Org. Chem., 2006, 71, 4481–4489 CrossRef CAS.
- M. Kirthiga, L. Rajendran and C. Fernandez, Electrochim. Acta, 2017, 230, 89–97 CrossRef CAS.
- S. B. He, Q. Q. Zhuang, L. Yang, M. Y. Lin, Y. Kuang, H. P. Peng, H. H. Deng, X. H. Xia and W. Chen, Anal. Chem., 2020, 92, 1635–1642 CrossRef CAS PubMed.
- S. B. He, R. T. Chen, Y. Y. Wu, G. W. Wu, H. P. Peng, A. L. Liu, H. H. Deng, X. H. Xia and W. Chen, Microchim. Acta, 2019, 186, 778 CrossRef CAS PubMed.
- J. J. Huang, H. Yang, Q. H. Huang, Y. W. Tang, T. H. Lu and D. L. Akins, J. Electrochem. Soc., 2004, 151, A1810–A1815 CrossRef CAS.
- K. Fujita, T. Ainoya, T. Tsuchimoto and H. Yasuda, Tetrahedron Lett., 2010, 51, 808–810 CrossRef CAS.
- Y. M. Alyousef, M. K. Datta, K. Kadakia, S. C. Yao and P. N. Kumta, J. Alloys Compd., 2010, 506, 698–702 CrossRef CAS.
- S. B. He, M. T. Lin, L. Yang, H. A. A. Noreldeen, H. P. Peng, H. H. Deng and W. Chen, ACS Appl. Mater. Interfaces, 2021, 13, 44541–44548 CrossRef CAS.
- S. Kang, Y. G. Gil, G. Yim, D. H. Min and H. Jang, ACS Appl. Mater. Interfaces, 2021, 13, 44124–44135 CrossRef CAS.
- C. Y. Zhao, Y. Si, B. F. Pan, A. Y. Taha, T. R. Pan and G. Sun, Talanta, 2020, 217, 121054 CrossRef CAS.
- L. Wu, G. H. Li, X. Xu, L. Zhu, R. M. Huang and X. Q. Chen, TrAC, Trends Anal. Chem., 2019, 113, 140–156 CrossRef CAS.
- C. Esen, J. Czulak, T. Cowen, E. Piletska and S. A. Piletsky, Anal. Chem., 2019, 91, 958–964 CrossRef CAS.
- W. S. Zheng and X. Y. Jiang, Analyst, 2016, 141, 1196–1208 RSC.
- D. V. Vokhmyanina, K. D. Andreeva, M. A. Komkova, E. E. Karyakina and A. A. Karyakin, Talanta, 2020, 208, 120393 CrossRef CAS PubMed.
- C. Wang, Y. Sun and Q. Zhao, Talanta, 2020, 207, 120280 CrossRef CAS PubMed.
- Y. Gao, Y. Z. Zhou and R. Chandrawati, ACS Appl. Nano Mater., 2020, 3, 1–21 CrossRef CAS.
- Q. Zhao, D. Lu, G. Y. Zhang, D. Zhang and X. B. Shi, Talanta, 2021, 223, 121722 CrossRef CAS.
- R. Banerjee and A. Jaiswal, Analyst, 2018, 143, 1970–1996 RSC.
- X. X. Yan and K. C. Persaud, IEEE Sens. J., 2019, 19, 404–412 CAS.
- C. W. Wang, R. Xiao, S. Wang, X. S. Yang, Z. K. Bai, X. Y. Li, Z. Rong, B. F. Shen and S. Q. Wang, Biosens. Bioelectron., 2019, 146, 111754 CrossRef CAS PubMed.
- N. Jiang, R. Ahmed, M. Damayantharan, B. Unal, H. Butt and A. K. Yetisen, Adv. Healthcare Mater., 2019, 8, e1900244 CrossRef.
- H. Y. Yang, Q. Y. He, Y. S. Chen, D. Shen, H. X. Xiao, S. A. Eremin, X. P. Cui and S. Q. Zhao, Microchim. Acta, 2020, 187, 592 CrossRef CAS.
- Q. Y. He, H. Y. Yang, Y. S. Chen, D. Shen, X. P. Cui, C. G. Zhang, H. X. Xiao, S. A. Eremin, Y. X. Fang and S. Q. Zhao, J. Pharm. Biomed., 2020, 187, 113317 CrossRef CAS PubMed.
- M. Yaman, J. Catak, H. Ugur, M. Gurbuz, I. Belli, S. N. Tanyildiz, H. Yildirim, S. Cengiz, B. B. Yavuz, C. Kismiroglu, B. Ozgur and M. C. Yaldiz, Trends Food Sci. Technol., 2021, 109, 552–563 CrossRef CAS.
- P. K. Borah, A. Sarkar and R. K. Duary, Food Chem., 2019, 288, 395–404 CrossRef CAS PubMed.
- B. Lim, M. J. Jiang, J. Tao, P. H. C. Camargo, Y. M. Zhu and Y. N. Xia, Adv. Funct. Mater., 2009, 19, 189–200 CrossRef CAS.
- X. M. Li, X. M. Chen, X. Z. Wu, J. Wang, Z. W. Liu, Y. M. Sun, X. Shen and H. T. Lei, Food Agric. Immunol., 2019, 30, 123–139 CrossRef CAS.
- X. P. Cui, N. Vasylieva, P. P. Wu, B. Barnych, J. Yang, D. Shen, Q. Y. He, S. J. Gee, S. Q. Zhao and B. D. Hammock, Anal. Chem., 2017, 89, 11091–11097 CrossRef CAS PubMed.
- X. Q. Tao, H. Y. Jiang, J. H. Zhu, X. Wang, Z. H. Wang, L. L. Niu, X. P. Wu, W. M. Shi and J. Z. Shen, Food Agric. Immunol., 2014, 25, 137–148 CrossRef CAS.
- Z. L. Mei, W. Qu, Y. Deng, H. Q. Chu, J. X. Cao, F. Xue, L. Zheng, H. S. El-Nezamic, Y. C. Wu and W. Chen, Biosens. Bioelectron., 2013, 49, 457–461 CrossRef CAS PubMed.
- V. T. Nguyen, S. Song, S. Park and C. Joo, Biosens. Bioelectron., 2020, 152, 112015 CrossRef CAS PubMed.
- V. W. S. Lam and E. L. Gyenge, J. Electrochem. Soc., 2008, 155, B1155–B1160 CrossRef CAS.
- M. Samari-Kermani, S. Jafari, M. Rahnama and A. Raoof, Colloid
Interface Sci. Commun., 2021, 42, 100389 CrossRef CAS.
- S. He, L. Yang, P. Balasubramanian, S. Li, H. Peng, Y. Kuang, H. Deng and W. Chen, J. Mater. Chem. A, 2020, 8, 25226–25234 RSC.
- X. M. Shen, W. Q. Liu, X. J. Gao, Z. H. Lu, X. C. Wu and X. F. Gao, J. Am. Chem. Soc., 2015, 137, 15882–15891 CrossRef CAS PubMed.
- Q. M. Chen, X. D. Zhang, S. Q. Li, J. K. Tan, C. J. Xu and Y. M. Huang, Chem. Eng. J., 2020, 395, 10 Search PubMed.
- L. Su, W. P. Dong, C. K. Wu, Y. J. Gong, Y. Zhang, L. Li, G. J. Mao and S. L. Feng, Anal. Chim. Acta, 2017, 951, 124–132 CrossRef CAS PubMed.
- F. Abyar and I. Novak, J. Mol. Liq., 2019, 276, 819–825 CrossRef CAS.
- S. M. Moore, T. A. Ricke, R. D. Davis and D. J. Briggs, Biologicals, 2005, 33, 269–276 CrossRef CAS PubMed.
- S. J. Liu, L. N. Dou, X. L. Yao, W. T. Zhang, M. Zhao, X. C. Yin, J. Sun, D. H. Zhang and J. L. Wang, Biosens. Bioelectron., 2020, 169, 112610 CrossRef CAS PubMed.
- N. Paksoy, S. K. Altun and H. Durmaz, S. Afr. J. Anim. Sci., 2018, 48, 1110–1114 CAS.
- R. Iyer and S. K. Tomar, J. Dairy Res., 2013, 80, 233–239 CrossRef CAS PubMed.
- M. T. T. Nguyen, J. Kim, H. Lee, S. Won, Y. Kim, J. A. Jung, D. Li, X. H. M. To, K. T. N. Huynh, T. V. Le, B. Israr, H. J. An and J. Kim, Nutrients, 2020, 12, 1794 CrossRef CAS PubMed.
- F. C. Celik, C. Aygun, S. Gulten, A. Bedir, E. Cetinoglu, S. Kucukdoduk and Y. Bek, Turk. Arch. Pediatr., 2016, 51, 210–216 CrossRef PubMed.
Footnote |
† Electronic supplementary information (ESI) available: Chemicals and apparatus-es; preparation and characterization results of antigens; preparation of polyclonal antibody (pAb) against FA; the steady-state kinetic experiment; preparation of colloidal Au nanoparticles; validation study and results for UPLC-MS/MS; optimization of the experimental parameters of OsNHs-LFIA and AuNPs-LFIA; typical absorbance spectra between K2OsCl6 and OsNHs; photographs of suspensions of ordinary OsNPs and OsNHs; the UV-Vis spectrogram of OsNHs + TMB + H2O2 and K2OsCl6 + TMB + H2O2; optimization results of the experimental parameters of OsNHs-NLISA immunosensor; recovery of FA from spiked milk powder samples by OsNHs-LFIA; the chromatogram of FA from spiked milk powder samples analyzed by UPLC-MS/MS; a short and long-time storage stability study between OsNHs and HRP; the study of mechanisms of peroxidase-like activity for OsNHs; apparent steady-state kinetic parameters for OsNHs and other metallic NPs peroxidase mimics; comparison between different methods used for the determination of FA; elaborate information of the mobile phase composition and gradient elution; mass spectrometry parameters setting. See DOI: 10.1039/d1an01432c |
|
This journal is © The Royal Society of Chemistry 2022 |
Click here to see how this site uses Cookies. View our privacy policy here.