Preparation, modification and antifouling properties of polyaniline conductive membranes for water treatment: a comprehensive review
Received
26th September 2023
, Accepted 14th November 2023
First published on 24th November 2023
Abstract
Membrane separation has made significant contributions to effective water treatment technology in the fields of resource recovery, desalination and wastewater treatment in recent years. However, problems such as concentration polarisation and membrane fouling limit its selectivity and permeability in separation. The rapid development of electrochemical technology and emerging preparation methods for conductive membranes have enhanced membrane separation technology. Polyaniline (PANI) is a conductive polymer, and it has shown great potential for use in water treatment due to its flexible tunability, acceptable electrochemical properties and low cost, which necessitate a comprehensive review. In this paper, the preparation and doping of PANI conductive membranes are described in detail from the introduction of PANI. The effects of preparation and doping methods on membrane properties are evaluated. In addition, the research on PANI conductive membranes for water treatment and antifouling applications is summarised. Finally, the main remaining problems are presented, and directions for further research are envisaged.
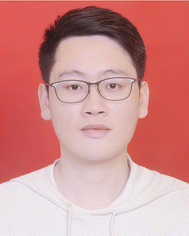 Jiajin Hao | Jiajin Hao received his B.S. degree in Environmental Science and Engineering in 2020 from Shaanxi University of Science and Technology. He is currently pursuing a Ph.D. degree in Prof. Wang's group. His research focuses on electrically assisted conductive nanofiltration membranes in desalination. |
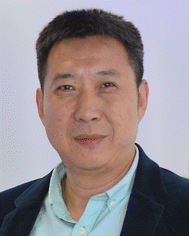 Lei Wang | Lei Wang received his Ph.D. degree in 2002 from the Hachinohe Institute of Technology (Japan). He is currently a full professor at Xi'an University of Architecture & Technology and the director of the Institute of Membrane Separation Technology of Shaanxi Province. His research focuses on the fundamental science and industrialization of membrane separation. |
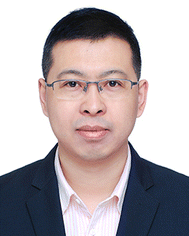 Xudong Wang | Xudong Wang received his Ph.D. degree from Xi'an University of Architecture and Technology in 2007. He is currently a professor at Xi'an University of Architecture and Technology, mainly engaged in the research of membrane science and technologies. |
Water impact
The emergence of conductive membranes has improved the application of membrane separation technology in the field of water treatment. Conductive membranes prepared from polyaniline, a common conductive polymer have great potential for wastewater treatment, desalination and antifouling. Therefore, this review focuses on research on polyaniline conductive membranes for water treatment.
|
1. Introduction
The rapid development of global industry and the dramatic increase in population have led to increased demand for water resources year after year, while improvements in production technology and quality of life have also brought higher requirements for the quality of water resources. With limited freshwater resources, the water needed for human consumption and production can be provided through wastewater treatment and desalination. Membrane separation technologies, mainly microfiltration (MF), ultrafiltration (UF), nanofiltration (NF) and reverse osmosis (RO), remove target ions and contaminants from water based on pressure or concentration differences, and they have provided satisfactory results in terms of the environment and cost.1–4 However, due to the “trade-off” effect, membranes often must be prepared at the expense of permeate flux to achieve high selectivity, and vice versa.5 Hydrophilic modifications of membrane surfaces,6–8 optimisation of the interfacial polymerisation processes,9,10 construction of asymmetric membrane structures11,12 and modulation of the two-dimensional (2D) membrane d-spacing13,14 have proven useful in breaking the upper limit of the membrane “trade-off”, but there is still a large gap between these methods and the desired effects. In addition, membrane fouling affects the selectivity and permeability of membranes.15 Membrane fouling can be mitigated via water pretreatment,16,17 physical and chemical cleaning,18,19 hydrophilic modification of the membrane surface20,21 and addition of antimicrobial materials.22,23 However, the pretreatment and cleaning processes make the overall operation cumbersome. Additionally, although the modified membranes exhibit antifouling capabilities, they still must be cleaned periodically.
The rapid development of electrochemical technology has brought new possibilities to the field of membrane separation. Electrically assisted membrane separation systems can be classified into two types according to the different modes of operation. One type involves placing the membrane material between two electrodes and relying on electric field forces such as electrophoresis and electroosmotic flow to achieve selective separation of the target material (Fig. 1a), such as in electrodialysis.24,27,39 However, the long transmission distance and complex solution system require a high external driving voltage, which consumes large amounts of energy; furthermore, electrode corrosion and generation of harmful byproducts under high potential are inevitable.25 In addition, the external electric field can play a limited role in the selective separation of substances and membrane fouling, which still depend on the performance of the membrane material itself. Another model has been proposed to effectively solve these problems. Conductive membrane separation systems that directly use membrane materials as electrodes significantly improve the efficiency of energy utilisation (Fig. 1b). In addition to the driving force of the electric field, the polarised membrane surface increases the electrostatic repulsion with the charged material, further enhancing the selective separation of the target material.26,28–30 Moreover, in situ electrochemical redox effectively slows down the accumulation of contaminants on the membrane, and the system can operate for a long period of time.31–38,40
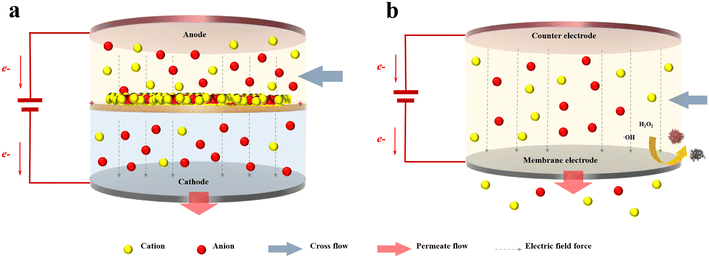 |
| Fig. 1 Schematic diagrams of two operational models in an electrically assisted membrane separation system: (a) a membrane between electric fields and (b) a conductive membrane as an electrode material. | |
In principle, membranes serving as electrodes must have good conductivities and electrochemical stabilities, especially those acting as anodes. Conventional polymer membranes are not conductive, even after the incorporation of highly conductive nanomaterials such as carbon nanotubes (CNTs) and reduced graphene oxide (rGO), and they exhibit poor electron conductivity through the polymer network.41,42 In addition, the instability of carbon materials at anodic potentials limits practical application. Fortunately, the emergence of conductive polymers has effectively remedied these problems. These polymers have many π–π or p–π conjugated systems in their structures, allowing electrons to move freely through the backbone chains. Representative substances include PANI,43 polyacetylene (PAC),44 polypyrrole (PPy),45 and polythiophene (PTh).46 Among them, PANI is widely used in the preparation of conductive membrane materials due to its tunable chemistry, stable electrode properties and low preparation cost.43,47–49 Previous reports on PANI membranes used in water treatment were mainly focused on physical adsorption,50–52 structural modification43,53 and antifouling properties.54 However, there is no literature detailing the preparation and doping of PANI conductive membranes and their properties in electrically assisted water treatment. Therefore, it is necessary to summarise the current research.
In the next few sections, we systematically introduce research on PANI conductive membranes used for water treatment. We first give a brief introduction to PANI. Subsequently, we analyse and summarise the utility of different preparation methods in synthesising PANI conductive membranes and evaluate the effects of different nanomaterials and protonic acid doping on the performance of PANI conductive membranes. In addition, this paper reviews the use of PANI conductive membranes in water treatment and analyses and summarises their positive effects in mitigating and monitoring membrane fouling. Finally, this paper summarises the problems and looks ahead to the future development of PANI conductive membranes in water treatment applications, and it is believed that this review provides useful references and guidance for related research.
2. Polyaniline
PANI is a macromolecular conjugated chain polymer formed by the oxidation of aniline monomers. Since its discovery in 1826, it has been used in a wide range of applications, such as photothermal medicine,55 biomimetic materials,56 anticorrosive devices57 and electromagnetic shielding.58Fig. 2a illustrates the molecular structural models of the three redox states of PANI. Unlike conventional polymers, PANI changes the properties of polymers through doping and dedoping reactions with protonic acids, which provides a strong advantage based on tunability.59 PANI doped with protonic acid undergoes significant changes in both its colour and conductivity properties, but only protonic acid doping of emeraldine base PANI confers conductivity. The doped PANI has a typical emeraldine colour and a metallic luster. Fig. 2b demonstrates the mechanism by which PANI becomes conductive after being doped with protonic acids. Briefly, after acid dissociation, H+ first forms a quinoid double-polaron by binding to the benzoquinone imine nitrogen in the native PANI chain. To keep the polymer chain structure stable, the polaron promotes enables the conjugated π-electrons on the benzene ring to leave the domain, causing them to undergo rearrangement to form the benzenoid double-polaron. With stabilisation of the environment, the PANI chain eventually assumes the form of a single-polaron, and the overall chemical environment shows homogeneity. The unique conductivity of PANI results because the electrons in the PANI single-polaron structure are excited very easily, which allows them to move freely between the half-filled conduction bands.60
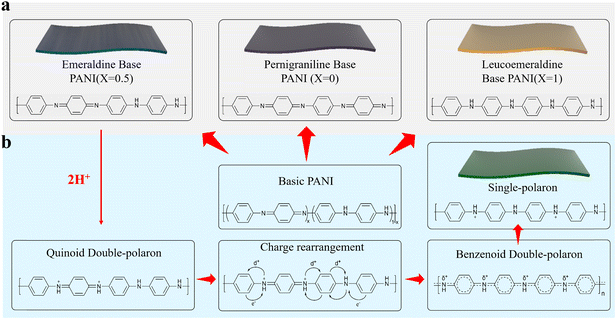 |
| Fig. 2 (a) Structural diagrams of PANI molecular chains in different redox states. (b) Mechanism for protonation of emeraldine base PANI. | |
3. Preparation of PANI conductive membranes
As with conventional polymer membranes, PANI conductive membranes can be prepared via layer-by-layer (LBL) self-assembly and non-solvent induced phase separation (NIPS). Due to the unique nature of PANI, some auxiliary preparation techniques are also required to ensure that the membrane has a uniform surface pore size and stable properties. Therefore, we classify the preparation of PANI conductive membranes into two categories: (1) membrane forming processes and (2) post-treatment processes. In addition, the advantages and disadvantages of each process are discussed in detail and summarised in Table 1.
Table 1 Advantages and disadvantages of different methods to prepare PANI conductive membranes
Preparation method |
Advantages |
Disadvantages |
Membrane forming processes |
LBL self-assembly |
Easy operation and mature process; flexible and controllable membrane thickness and structural properties |
Significant material loss; PANI needs to be prepared in advance, which increases operational complexity; difficult to prepare on a large scale |
NIPS |
Easy operation, low material loss rate and high membrane stability |
Low PANI addition makes the membrane poorly conductive; preparation conditions need to be optimised |
ISEO |
Membranes have a high crosslinking degree and good conductivity; avoids complex preparation processes and has potential for industrial application |
The membrane preparation process has many influencing factors and is poorly controllable |
ISCO |
Avoid the use of initiators; high purity of the produced PANI; simple and controllable reaction conditions and high experimental reproducibility |
Low product; low separation accuracy of the prepared membrane; difficult to prepare on a large scale |
Post-treatment process |
Thermal crosslinking |
Convenient; high membrane stability; avoids the use of additional chemicals |
High temperatures may damage the PANI structure |
Chemical crosslinking |
Low energy consumption; controllable; no damage to membrane structure |
Additional crosslinking agents are required |
Flash welding |
Fast reaction speed and high efficiency |
Poor control; may cause irreversible damage to membranes |
3.1 Membrane forming processes
3.1.1 LBL self-assembly.
LBL self-assembly has been used in the development of membrane materials for NF, RO and forward osmosis (FO) and is a simple and efficient preparation method.61,62 The physical and chemical stabilities of the membranes are ensured by tight bonding between the functional materials and the substrate through electrostatic and van der Waals forces under pressure and other effects.63–65 In the preparation of PANI conductive membranes by the LBL self-assembly method, presynthesised PANI powder is usually combined with the surface of the base membrane by filtration or spraying to form a functional layer with a stable separation structure (Fig. 3a).66 For example, Xu et al. formed a composite layer by vacuum filtration of negatively charged multiwall carbon nanotubes with carboxylic groups (MWNT–COOH) and positively charged PANI onto a polyethersulfone (PES) base membrane, and different thicknesses of PANI/MWNT–COOH conductive membranes were prepared by controlling the number of stacked layers in the composite layers.67 Stronger electrostatic interactions assembled PANI and MWNT–COOH on the membrane surface. The membrane exhibited the best conductivity (732.0 S m−1) when the number of stacked layers reached 10. In addition, Jeon et al. prepared a variety of PANI electrode membranes by sequentially spraying PANI and polyacrylic acid (PAA) onto indium-tin-oxide-coated glass surfaces by electrospray.68 Electrospraying effectively reduced the operation time and avoided the dedoping reactions of PANI. As products of a simple and mature preparation process, PANI conductive membranes prepared by the LBL self-assembly method are flexible and controllable in thickness, which are particularly suitable properties for the preparation of nanomaterial doped PANI conductive membranes. The structure and properties of the membranes can be controlled at the molecular level as they are not limited by the size and shape of the substrate. The prepared membranes are typically at the NF or UF level and are often used for ion rejection or dye removal. However, the weaker intermolecular forces make it inevitable that the conductive membrane will undergo material loss during operation. Moreover, using the LBL self-assembly method to prepare PANI conductive membranes usually requires the polymerisation of aniline to be completed in advance, which increases the complexity of the operation. In addition, the LBL self-assembly technique, which is mainly based on vacuum filtration, always faces difficulties in large-scale preparation.
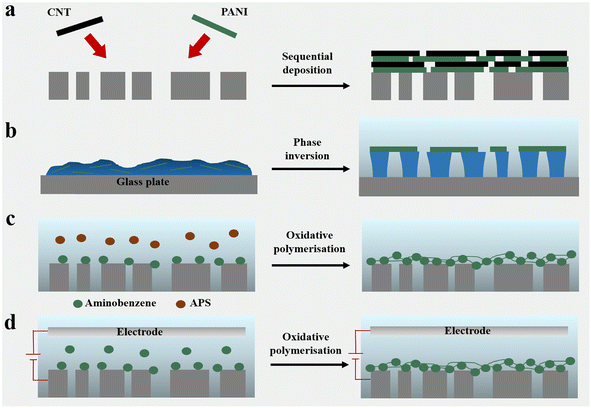 |
| Fig. 3 Strategies for the preparation of PANI conductive membranes: (a) layer-by-layer self-assembly, (b) non-solvent induced phase separation, (c) in situ chemical polymerisation, and (d) in situ electrochemical polymerisation. | |
3.1.2 Non-solvent induced phase separation.
NIPS, also known as the phase inversion method, relies on the principle that a polymer-containing solvent has higher solubility when in contact with another non-solvent phase to achieve membrane formation.69–73 Due to diffusive exchange between the solvent and the non-solvent phase, the prepared membranes usually show asymmetric structures. The top of the membrane generally exhibits a homogeneous and dense structure due to hydrophilic diffusion of the functional material, while the bottom forms a finger-like or spongy structure (Fig. 3b). Notably, strong intermolecular hydrogen bonding by PANI makes it soluble only in several organic solvents, such as chloroform, N-methyl-2-pyrrolidone (NMP) and dimethyl sulfoxide (DMSO), and then in low amounts.54 To improve the overall performance of PANI conductive membranes, dopant acids and highly conductive nanomaterials are usually added into the casting solution. The addition of these materials increases the viscosity of the casting solution, decreases the diffusion rate of the underlying solvent during phase inversion and forms larger volumes of finger pores at the bottom of the membrane.74 However, the hydrophilicity of PANI in turn increases the degree of crosslinking of the polymer and promotes the formation of dense and elongated pores within the membrane.75 In addition, the pH of the aqueous phase in the coagulation bath also affects the conformations of the PANI conductive membranes, since the morphology of PANI varies at different pHs. When the coagulation bath solution is alkaline, intermolecular hydrogen bonding is more prevalent, the PANI tends to form independent spherical particles, which are more likely to generate pores during the phase transition process, and the prepared membranes have larger pore sizes and pore densities. In contrast, the hydrogen bonding between PANI molecules is weakened by acidic environments, and the surfaces of the prepared PANI membranes are usually flatter.76 The preparation of PANI conductive membranes by the NIPS method has the advantages of a mature process, convenient operations and controllable membrane structures. In the preparation of PANI conductive membranes by the NIPS method, aniline polymerisation, material doping and membrane preparation can be performed simultaneously, which makes the operation more convenient. In addition, PANI conductive membranes prepared by the NIPS method have higher hydrophilicity and stability and a lower material dissolution rate than those prepared by the LBL self-assembly method, and they can be used in conventional water treatment operations such as UF and NF.77,78 However, the prepared membranes had poor conductivity due to the role of PANI's own hydrogen bonding, which limits its solubility in the casting solution.79 In addition, the dopant and solvent properties strongly affect the final properties of the conductive membranes, thus the preparation conditions of the membranes need to be optimised.
3.1.3
In situ chemical oxidation.
Unlike conventional polymers, aniline monomers must be oxidised to form chain structures. Therefore, it is usually necessary to synthesise the required PANI in advance when preparing conductive membranes by the LBL self-assembly and NIPS methods, which increases the complexity of the membrane preparation operation. In contrast, the ISCO method is completed by immersing the membrane material directly in an acidic aniline solution, and in situ growth of the PANI on the membrane surface is achieved by the addition of ammonium persulfate (APS) (Fig. 3c).80–82 For example, Cruz-Tato et al. immersed a polysulfone (PSF) supported membrane in an acidic aniline monomer solution and accomplished in situ oxidative polymerisation of PANI by slow dropwise addition of an APS solution.83 Scanning electron microscopy (SEM) confirmed crosslinking growth of the PANI network on the membrane surface. The ISCO method avoids prepolymerisation, multiple filtration and cleaning of the PANI, which simplifies the preparation process and shows potential for industrial application. In addition, PANI conductive membranes prepared by the ISCO method have better crosslinked degree networks, which improves the electron conductivities of the membranes. Notably, various factors, such as the concentration ratio of aniline to oxidant, polymerisation temperature, polymerisation time, pH and type of dopant acid make the prepared conductive membranes less controllable in terms of morphology and structure, but this also provides a possibility for the accurate separation of substances by PANI conductive membranes.
3.1.4
In situ electrochemical oxidation.
DeBerry et al. first prepared PANI conductive membranes by electrochemical oxidation in 1985,57 and since then, electrochemical syntheses of PANI conductive membranes have been studied extensively.84,85 The ISEO of PANI conductive membranes is similar to the electroplating process on metal surfaces. Under an applied voltage, aniline monomers are oxidised on the electrode surface to form aniline radicals, and in situ polymerisation to PANI is occurs as the reaction proceeds (Fig. 3d).86,87 Recently, Li et al. prepared a GO/S-PANI composite conductive membrane via a modified electrochemical method.88 Graphite intercalation compound (GIC) were first obtained by intercalating graphite foil in a concentrated sulfuric acid environment. Then the GO/S-PANI membrane was prepared by electrochemical oxidation in a (NH4)2SO4 electrolyte solution containing CuSO4 with a GIC as the anode and a PANI membrane as the cathode. When an external voltage was applied, water molecules entered and reacted between the GIC layers, and the oxygen produced contributed to stripping of the GO. Cu2+ in the solution combined with the negatively charged GO and moved towards the cathode under the applied electric. Finally, the GO/S-PANI membrane was assembled on the surface of the PANI membrane by electrostatic forces, hydrogen bonding and π–π stacking. Compared with the traditional ISEO method, this method enables the stripping and assembly of GO and acid doping of the PANI to be carried out simultaneously, which has the advantages of easy operation and high safety, and provides a new electrochemical synthesis of PANI conductive membranes. The greatest advantage of the ISEO method is the high purity of the prepared PANI and the fact that no additional initiator needs to be added. Moreover, the fast reaction speed and easy operation result in good reproducibility. However, due to the limitations of the operating conditions, PANI conductive membranes prepared by the ISEO method usually need to be based on conductive materials, and the prepared membrane performs poorly in terms of separation accuracy, which is generally relevant for oil–water separation or mitigation of membrane fouling. Furthermore, the limited electrode size restricts the possibility of large-scale preparation.
3.2 Post-treatment process
3.2.1 Thermal crosslinking.
Thermal crosslinking is widely used in the post-treatment stages of membrane materials such as UF, NF and RO due to its simplicity and high efficiency. At higher temperatures, the polymer backbone chain swells, and the membrane becomes denser.89–92 After thermal crosslinking, the PANI conductive membranes exhibit significantly reduced structural defects and show good mechanical and electrochemical stabilities in the presence of applied voltages, acids, alkalis, salts, and oxidants.67 Sarihan et al. investigated the changes occurring in the structural properties of PANI/poly(2-acrylamido-2-methyl-1-propanesulfonic acid) (PAMPSA) conductive membranes after heat treatment at a temperature lower than that of the thermal transition temperature of PANI (180 °C).93 SEM showed that the upper structure of the membranes was progressively denser and more homogeneous and that the surface pore sizes were reduced with higher heat treatment temperatures and longer times (Fig. 4a). In addition, Wang et al. reported that heat treatment affected the conductivities of PANI membranes.94 The moisture content in the polymer structure gradually decreased with increasing temperature, which increased the degree of crosslinking in the polymeric network and thus improved the electron transfer efficiency. However, excessively high temperatures lead to phase changes in the PANI, which destroys the membrane structure and thus reduces the conductivity.
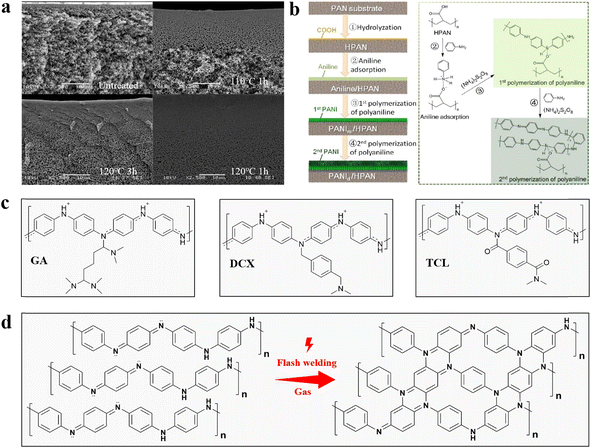 |
| Fig. 4 (a) Cross-section of a PANI/MWNT–COOH conductive membrane after heat treatment with different temperatures and times.93 Reprinted with permission from ref. 93, copyright 2019 Elsevier. (b) Mechanism for secondary polymerisation of PANI on a HPAN substrate.80 Reprinted with permission from ref. 80, copyright 2021 Elsevier. (c) Mechanism for chemical crosslinking of PANI by GA, DCX and TCL. (d) Mechanistic diagram for polymer network formation by flash welding of PANI conductive membranes. | |
3.2.2 Chemical crosslinking.
Chemical crosslinking is a post-treatment process that relies on interactions such as covalent bond formation between the crosslinkers and the membrane material to strengthen the membrane structure and is common in NF and FO.91,95–97 Chemical crosslinking of PANI conductive membranes is accomplished by immersing the membrane in a solution containing a crosslinking agent for a certain period. The crosslinkers can form a strong polymer network by covalent bonding with the imine nitrogen atoms on the PANI backbone chain. Zhang et al. chemically crosslinked prepared PANI/CNTs membranes with GA, and the crosslinked membranes showed strong mechanical stability during ultrasonic irradiation for 30 min.81 Amura et al. chemically crosslinked sulfonated polyaniline (SPANI) membranes using three crosslinkers, glutaraldehyde (GA), terephthaloyl chloride (TCL) and α,α′-dichloro-p-xylene (DCX) (Fig. 4c).98 The analyses showed that all three crosslinkers treated SPANI membranes were stable during immersion in DMF. Organic solvent NF experiments showed that the prepared membranes operated stably at a pressure of 20 bar, and the GA crosslinked membranes had the smallest membrane pore size. Compared with thermal crosslinking, chemical crosslinking has the advantage of low energy consumption and avoids damage to the membrane structure caused by high temperatures. However, the use of crosslinkers inevitably has an impact on the environment. Recently, He et al. achieved secondary polymerisation of PANI on the membrane surface by immersing HPAN-based membranes twice in PANI and APS solutions (Fig. 4b), and the composite conductive membranes with double-layered PANI structure showed high stability by keeping the permeation flux unchanged after 200 h of immersion in 2 M HCl or after 6 h of ultrasonication.80 The in situ secondary growth of PANI effectively avoids the use of traditional chemical crosslinkers and reduces secondary pollution to the environment.
3.2.3 Flash welding.
Flash welding utilises the photoresponsiveness of PANI to post-treat the membranes. Due to the strong photothermal reactivity of PANI, high intensity photothermal pulses can be used to rapidly weld PANI to achieve crosslinking between the polymer backbone chain. During the welding process, crosslinking between C and N atoms in the PANI backbone chain occurs to form a denser and more stable structure (Fig. 4d).99 In addition, after the welding treatment, the morphologies, functionalities and conductivities of the PANI membranes were altered to different degrees. Liao et al. investigated the combined effect of flash welding post-treatment on PANI UF membranes and found that the surface of the welded PANI/CNTs membranes was homogeneous and smooth.100 In addition, the low welding power did not cause an overall breakdown of the membrane thickness, so part of the membrane showed an asymmetric structure. Flash welding exposed of some CNTs on the membrane surface, leading to a nearly 10-fold increase in conductivity compared to the non-welded PANI conductive membrane. Additionally, the retention of SiO2 nanoparticles by the welded membrane was increased by a factor of 10, indicating that flash welding can be used to improve the retention performance of PANI conductive membranes. However, research on the post-processing of PANI conductive membranes using flash welding is relatively sparse, because the excessively fast photothermal reaction rate cannot be well controlled.
4. Doping modification of PANI conductive membranes
Although PANI has good electron-conducting ability, its conductivity only ranges within 1–10 S cm−1.101 The conductivity of the membrane can be improved by doping with highly conductive nanomaterials. In addition, the dopant acids also affect the overall performance of PANI conductive membranes. Therefore, in this section, we explore the effects of multiple dopants, which mainly include nanomaterials and protonic acids, on the performance of PANI conductive membranes. The former emphasises the compatibility between nanomaterials and PANI and the effects on the electrochemical properties of the membranes, while the latter focuses on the effects of dopant acids with different molecular weights on the performance of PANI conductive membranes during the polymerisation process, which are summarised in Table 2.
Table 2 Advantages and disadvantages of different doping materials used to prepare PANI conductive membranes
Doping material |
Advantages |
Disadvantages |
Nanomaterials |
CNT |
Large specific surface area, strong resistance to anodic oxidation; strong degree of crosslinking, high adjustability |
Excessive doping may damage the membrane structure |
Graphite derivatives |
High conductivity and adjustability |
There is a trade-off between material conductivity and stability |
MXene |
High compatibility and strong dispersion, the prepared membrane is more homogeneous and has higher flux; crosslinking can occur with PANI to stabilise the nanolayer structure |
Easily oxidised |
TiO2 |
Can form a heterojunction with PANI to improve the photoelectric efficiency of the membrane |
Conductivity is strongly influenced by temperature and other factors |
Protonic acid |
Small-molecule inorganic acids |
High conductivity; can be doped multiple times |
Volatile and prone to de-doping; large membrane pores, not suitable for accurate substance separation; poor stability |
Macromolecular organic acids |
Can serve as template for aniline polymerisation, and achieve unique and diverse morphology and properties of the prepared products; the membrane is homogeneous and dense with high separation performance; high mechanical and chemical stability |
It is not volatile and the residual acid is not easy to remove completely; the low degree of proton dissociation makes the membrane less conductive and usually necessitates the addition of strong acid reagents |
4.1 Nanomaterial doping modification
4.1.1 Carbon nanotubes.
CNTs have been used in membrane separations due to their large specific surface areas and abundant surface functional group.102–105 Their ultrahigh current density (4 × 109 A cm−2) are advantageous in the preparation of PANI conductive membranes.75,106,107 As shown in Fig. 5a, PANI bonded with O–CNTs via electrostatic forces, hydrogen bonding and π–π interactions. Connections were formed between adjacent CNTs. This enabled free conduction of electrons within the membrane while stabilising the membrane structure. It was shown that charge transfer resistance within the PANI/CNTs membrane was decreased by a factor of 5 compared to a CNTs membrane that was not crosslinked with PANI.108 In addition, the 1D structure of CNTs provides an excellent specific surface area for aniline polymerisation because of the polymerisation of aniline and its dependence on the morphology and structure of the base membrane and dopant. Therefore, the doping of CNTs can effectively improve the overall conductivity of PANI membranes, but the irregular arrangement of CNTs and excessive doping may lead to the formation of defects on the membrane surface, which affects the water treatment performance of the membrane. Furthermore, excess CNTs functionalisation may have an effect on the conductivity of PANI membranes.
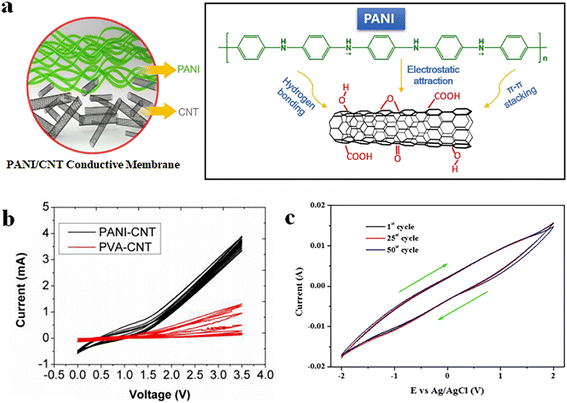 |
| Fig. 5 (a) Diagram showing the mechanism for mutual bonding between O–CNTs and PANI backbone chain via multiple forces.108 Reprinted with permission from ref. 108, copyright 2022 Elsevier. (b) CV data for PANI/CNTs and PVA/CNTs conductive membranes after 20 cycle scans.87 Reprinted with permission from ref. 87, copyright 2016 American Chemical Society. (c) CV data for PANI/MWNT–COOH conductive membrane after 50 scans.67 Reprinted with permission from ref. 67, copyright 2022 Elsevier. | |
When conductive membranes were used as anodes, the ·OH produced by the CNTs at high anodic potentials oxidised the membrane material, causing a decrease in conductivity and cracking. Fortunately, the reversible redox properties of PANI allow conductive membranes to exhibit strong stability at the anodic potential. When the anode undergoes an oxidation reaction, PANI can serve as a sacrificial polymer to avoid electron loss on CNTs.67 Duan et al. tested the electrochemical stability of PVA/CNTs and PANI/CNTs conductive membranes at an applied voltage of +3 V.87 Their results showed that the current through the conductive membrane crosslinked by polyvinyl alcohol (PVA) decreased by 14.4%, whereas the current of the conductive membrane crosslinked by PANI decreased by only 5%. When the voltage was increased to +5 V, the current of the PVA/CNTs membrane showed a significant decrease (52%), while that of the PANI/CNTs membrane decreased by only 16% (Fig. 5b). SEM images showed that the porosity of the PVA/CNTs membrane increased by approximately 4% after 10 min of applied voltage, while that of the PANI/CNTs membrane was almost unchanged. Xu et al. also demonstrated good corrosion resistance of PANI at anodic oxidation potential.67 The prepared MWCNTs/PANI conductive membranes were subjected to 50 cyclic voltammetry (CV) scans with no obvious change in current (Fig. 5c). These results indicated that the CNTs-doped PANI conductive membrane combined the unique advantages of the two materials. The low percolation threshold of CNTs endowed the conductive membrane with excellent water flux, while the corrosion resistance of PANI improved the stability of the CNTs at the anode.
4.1.2 Graphite derivatives.
Graphene (Gr), as a graphite exfoliation product, exhibits a lower electrode resistance than CNTs due to its unique 2D structure. PANI conductive membranes doped with Gr exhibited high conductivities.109 Li et al. prepared a Gr/PANI conductive membrane via a one-step method, which showed a 1.6-fold increase in conductivity (1.51 S m−1) compared to the undoped PANI membrane.85 Gr constitutes sp2 hybridised monolayers of honeycomb nanosheets, which form π–π interactions with the sp2 hybridised imine N in PANI and bind to each other via van der Waals forces.110 Wang et al. investigated the interaction energy between Gr and PANI with density functional theory.111 Their calculations showed that the Gr and PANI combined via van der Waals forces. Moreover, due to the charge-transfer dipolar attraction, an increase in the degree of PANI protonation increased the binding strength (Fig. 6a). Notably, the hydrophobicity of Gr leads to poor dispersion in aqueous solutions, which may cause the formation of defect structures during membrane preparation. Guo et al. suppressed agglomeration of three-dimensional graphene (3D-G) in water by introducing graphene quantum dots (GQDS) (Fig. 6b).112 The addition of GQDS on 3D-G increased the π–π interactions with the PANI backbone chain and significantly improved the dispersion of 3D-G in water. The prepared GQDS/3D-G/PANI composite conductive membrane exhibited a uniform membrane structure. However, under prolonged high-pressure operation, van der Waals forces and π–π interactions alone could not maintain stable binding between the Gr nanosheets and PANI, which affected the stability of the membrane structure.
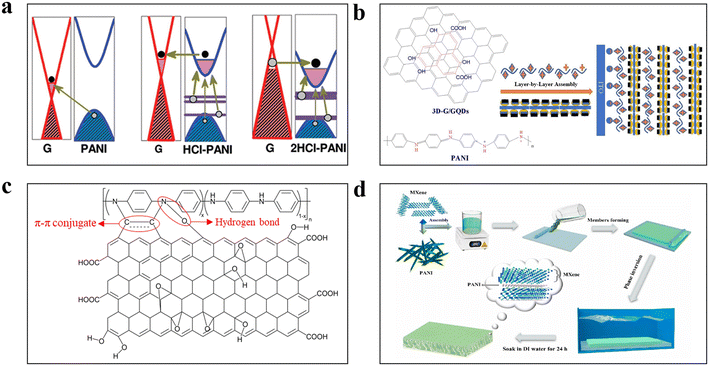 |
| Fig. 6 (a) Mechanistic diagram for charge transfer between the protonic acid, PANI and Gr.111 Reprinted with permission from ref. 111, copyright 2012 American Chemical Society. (b) Schematic diagram for the preparation of a composite conductive membrane using GQDs/3D-G/PANI.112 Reprinted with permission from ref. 112, copyright 2020 Elsevier. (c) Schematic diagram showing the interaction forces between PANI and GO. (d) Schematic diagram of a PANI composite conductive membrane doped with MXene and prepared via NIPS method.120 Reprinted with permission from ref. 120, copyright 2023 Elsevier. | |
The many oxygen-containing functional groups on the surface of graphene oxide (GO), which is an oxidation product of Gr, provided additional hydrogen bonding and electrostatic forces to strengthen the bonding with PANI (Fig. 6c), but inevitably led to a decrease in the conductivity of the membrane.78,85 To balance the conductivities and stabilities of PANI membranes, the GO was rGO.74 Karkooti et al. prepared an rGO/PANI conductive membrane by vacuum filtration,113 and rGO incorporation increased the membrane conductivity from 0.46 S m−1 to 84.53 S m−1, which was significantly higher than that of GO-doped PANI. Ko et al. explored the interfacial interaction between rGO and PANI.114 It was found that PANI grew stably on the rGO surface. In addition, the density of the spherical PANI nanoparticles grown on the rGO surface was the largest, and it was influenced by the specific surface area and spatial site resistance of the material, indicating that 0D PANI nanoparticles had the strongest interactions with rGO. Moreover, rGO improved the dispersion of PANI in the polymer solution and increase the content of PANI in the casting solution, and the synthesised membrane was denser and exhibited higher conductivity.115
As a processing product of graphite, expanded graphite (EG) with a sparse 3D structure also has a high potential for improving the conductivity of PANI membranes. Xu et al. prepared EG/PANI conductive membranes doped with two acids, dodecylbenzene sulfonic acid (DBSA) and PAMPSA.116 Their results showed that the EG increased the conductivities of both membranes from 1 × 10−6 S cm−1 to 5 × 10−4 S cm−1. However, due to the its structure and large pore size, EG is currently mostly used for antimicrobial purposes and membrane fouling mitigation.117
4.1.3 MXene.
MXene is a collective term for transition metal carbide, nitride and carbon-nitride materials, whose good electrical conductivity (6.8 × 105 S m−1) and abundant surface functional groups provide unique advantages in the preparation of PANI conductive membranes.118 Compared with carbon-based materials, MXene has better interfacial compatibility with polymers and the prepared membranes are more homogeneous. In addition, the good compatibility effectively inhibited the swelling of MXene nanosheets in water while improving the conductivity of the PANI membrane. Li et al. prepared an MXene-doped PANI conductive membrane (Fig. 6d), which exhibited good conductivity (0.5 S m−1) and excellent permeation flux (227 L m−2 h−1).120 The hydrogen bonding interactions between the N–H bonds on PANI and the –F and –O functional groups on the MXene surface anchored the two adjacent MXene lamellae, which suppressed interlayer swelling of the MXene nanosheets. Additionally, the hydrogen bonds enhanced the overall mechanical stability of the composite conductive membrane, and the tensile strength of the composite membrane increased from 2.2 MPa to 4.0 MPa with the addition of MXene. Furthermore, the recent findings of VahidMohammadi et al. demonstrated that PANI spontaneously polymerises on the surfaces of MXene nanosheets in the absence of oxidising agents as long as charge transfer occurs between the aniline monomer and the MXene.121 This method has not yet been used for the preparation of PANI composite conductive membranes, but it provides a new idea for the preparation of MXene doped PANI conductive membranes.
4.1.4 Titanium dioxide.
Titanium dioxide (TiO2) is an advanced metal oxide semiconductor and is widely used in electronic devices,122 coatings,123 and photoelectrocatalysis.124 Compared with CNT, rGO, MXene and other materials, TiO2 with semiconductor properties is slightly less conductive. However, its superior photoelectric conversion ability provides more possibilities for the application of PANI conductive membranes. Sboui et al. prepared a TiO2/PANI/PVDF composite conductive membrane via NIPS, and it server as a photoelectrocatalyst to remove the two organic contaminants, methylene blue (MB) and methyl orange (MO).134 The introduction of TiO2 increased the membrane resistance from 4.28 Ω m to 19.93 Ω m. Alternating current impedance plots showed that the membrane resistance increased approximately by a factor of 3 with increasing TiO2 content, indicating that TiO2 has poor conductivity. However, the TiO2/PANI/PVDF composite conductive membrane exhibited significant photoelectrocatalytic removal of MB and MO, which was attributed to the heterogeneous structure between TiO2 and PANI that improved the overall photoresponse range of the conductive membrane. In addition, TiO2 with photogenerated electron holes improves the antimicrobial properties of PANI conductive membranes. Farias et al. demonstrated good antibacterial properties of a TiO2/PANI/CTAB composite conductive membrane prepared from TiO2 and cetyl trimethylammonium bromide (CTAB), which effectively prevented the growth of S. aureus on its surface.125
4.2 Protonic acid doping
The unique properties of PANI require doping with a protonic acid to generate conductivity. The protonic acids currently used for doping are mainly classified into two categories: small-molecule inorganic acids and macromolecular organic acids. Different dopant acids lead to differences in the structures, morphologies, conductivities and stabilities of the synthesised PANI conductive membranes.
4.2.1 Structure and morphology.
PANI conductive membranes prepared from small, inorganic acids were usually looser and had larger free volumes, whereas the membranes doped with macromolecular acids show good densification. Shen et al. explored the surface morphologies of the three membranes, HCl/PANI, PSSA/PANI and PAMPSA/PANI.126 The SEM image Fig. 7a shows that the surface of the PANI membrane doped with HCl was the roughest. However, with increasing molecular weight of the dopant acid, the membrane surface gradually smoothed. Moreover, the long-chain structure of the macromolecular dopant acid promoted better dispersion of PANI in the casting solution, which led to a homogeneous and dense functional layer structure.74 In addition, the choice of dopant acid affected the polymerisation rate of aniline, which in turn determined the final morphologies of the PANI conductive membranes.127 Previous reports confirmed that different acidic environments affected PANI polymerisation.128 For example, in a strongly acidic environment (0.1 M H2SO4), PANI forms a typical granular structure, whereas in a weakly acidic environment (0.4 M CH3COOH), it formed a CNTs-like tubular structure.129 Pan et al. found that the surfaces of HCl- and H2C2O4-doped PANI conductive membranes prepared by the ISEO method showed distinct spherical structures at an H+ concentration of 0.5 M, whereas the surfaces of H2SO4 and HNO3 doped membranes showed network-like structures.84 Thus, PANI conductive membranes with different surface structures were prepared by choosing the correct acid, which has a positive effect in increasing membrane flux and mitigation fouling.
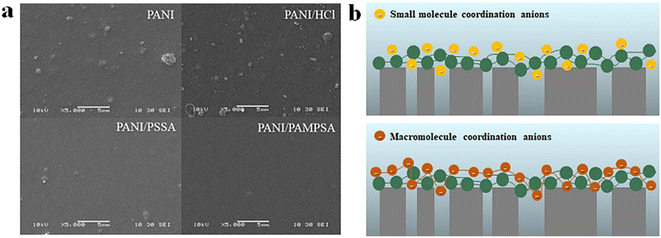 |
| Fig. 7 (a) Surface morphologies of PANI, PANI/HCl, PANI/PSSA and PANI/PAMPSA conducting membranes.126 Reprinted with permission from ref. 126, copyright 2018 Elsevier. (b) Structural diagram of PANI conducting membranes prepared by doping with macromolecule organic acids and small-molecule inorganic acids. | |
4.2.2 Conductivity.
PANI conductivity is closely linked to the strength of the protonic acid. The strong protonic acid dissociated more H+ to dope the PANI, which improved the overall doping rate of the PANI backbone chain and increased the conductivity of the membrane.66 In addition, the conductivities of PANI membranes can be improved by secondary doping. Xu et al. used DBSA for secondary doping of PAMPSA/PANI conductive membranes.116 Electrostatic bonding between the added sulfonic acid groups and the free imine nitrogens in the membrane increased the electron conduction paths in the membrane. Test results showed that the conductivity of the membranes were increased by one order of magnitude after secondary doping. SEM studies confirmed that the number of defects on the membrane surface was significantly reduced and that the membrane surface was smoother after secondary acid doping, indicating that secondary doping formed a denser and more homogeneous membrane structure. However, the macromolecular volume of the polymeric acid prevented complete entry into the membrane during the secondary doping process, resulting in a lower actual doping rate. The problem of acid diffusion was solved by adding the dopant acid directly into the casting solution, but the resulting membrane was not homogeneous. Therefore, Xu et al. prepared PA/PANI membranes directly by using a polymeric acid (PA) as a template, which enabled simultaneous oxidation of PANI and PA doping and improved the conductivity of the membrane.127 The conductivity of the PA/PANI membrane was as high as 1.2 × 10−1 S cm−1, which was three orders of magnitude higher than that of the membrane prepared by secondary doping.
4.2.3 Mechanical and chemical stability.
In terms of the mechanical and chemical stability of the membrane, the PANI doped with a small molecule acid exhibited only a single chain and had poor overall stability. When PANI was doped with macromolecular acids, the ligand anions form polymerised chains and interacted with the PANI chains to form a double-chain structure, which enhanced the physical and chemical stability of the conductive membrane due to the combined effect of electrostatic forces and hydrogen bonding (Fig. 7b). Xu et al. compared the mechanical strengths of PAMPSA- and HCl-doped PANI conducting membranes.127 The Young's modulus and tensile strength of the PAMPSA/PANI membrane were higher than those of the HCl/PANI membrane, and analyses of the membrane structure revealed that fewer finger-like structures were formed within the PAMPSA/PANI membrane, which significantly enhanced its mechanical stability. In addition, the double chain structure of the macromolecular acid also endowed PANI conductive membranes with better resistance to organic solvents and acids. Shen et al. investigated the stabilities of PANI conductive membranes doped with three acids (HCl, PSSA, PAMPSA) in organic solvents.126 Their results showed that the macromolecular acid-doped PANI conductive membranes exhibited superior stabilities in organic solvents compared to those prepared with HCl doping, and the membrane mass was essentially unchanged after one week of immersion. Subsequently, Xu et al. investigated the acid resistance of CNTs/PANI composite conductive membranes doped with HCl, DBSA and PSSA.82 They found that the HCl-doped conductive membrane formed obvious cracks during prolonged immersion, and the conductivity decreased by approximately 50%. In contrast, the DBSA- and PSSA-doped conductive membranes showed good stability.
5. Application in water treatment
The unique electrochemical properties of PANI conductive membranes show great potential for use in water treatment. With an applied voltage, the properties of the surface or entirety of PANI conductive membranes are altered. Not only can achieve efficient and rapid separation of target substances in water be achieved, but such altered membranes have also shown positive effects in membrane contamination mitigation and monitoring. In this section, we provide a comprehensive review and discussion of PANI conductive membranes used for water treatments.
5.1 Enhanced contaminant removal
Precise, rapid and efficient removal of organic and heavy metal contaminants from water bodies has been an extremely important challenge in wastewater treatment.130 Traditional technologies such as MF and NF effectively retain contaminants, but the toxic waste liquid remaining after retention still requires additional treatment.131–133 In contrast, the electrostatic adsorption, repulsion and redox processes of PANI conductive membranes subjected to an electric field lead to the removal of heavy metals and in situ degradation of organic contaminants, minimising the need for subsequent treatment of the wastewater.
5.1.1 Organic contaminant removal.
For charged organic contaminants, increased electrostatic repulsion by the PANI conductive membrane at an applied voltage provides removal efficiencies higher than those of conventional separation membranes. However, with increasing voltage, oxidation on the membrane surface dominated. Xu et al. found that the efficiency for removal of methylene blue (MB), methyl orange (MO), and phenol (PhOH) by PANI/MWNT conductive membranes at an applied voltage of +2.5 V was increased by more than 90% compared to that at +1 V.67 The removal of MB, MO, and phenol by a PANI/MWNT conductive membrane was also improved by over 90% at an applied voltage of +2.5 V. Importantly, electrochemical oxidation of the contaminant required that the relative potential at the electrode surface exceed the oxidation potential of the contaminant. In addition, an interesting study showed that CNTs entangled by PANI could significantly increase the generation of H2O2 with removal rates as high as 77.4% and 79.8% for MB and MO (Fig. 8a), suggesting that PANI played a positive role in enhancing electrochemical oxidation of the conductive membrane.108
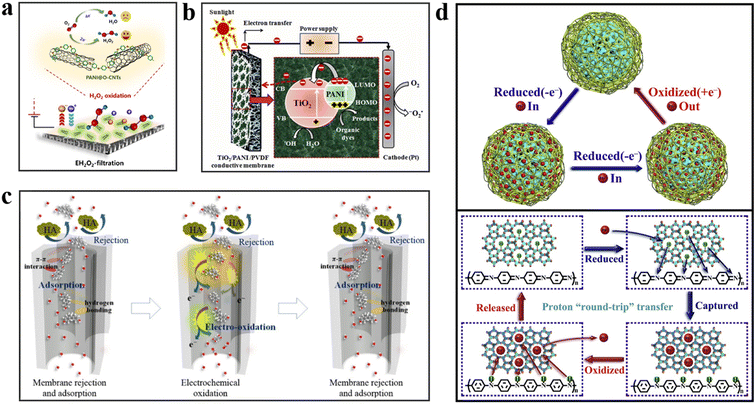 |
| Fig. 8 (a) Diagram of in situ H2O2 production and the mechanism for rejection and removal of dye molecules by CNTs/PANI membrane at cathodic voltage.108 Reprinted with permission from ref. 108, copyright 2022 Elsevier. (b) Mechanistic diagram for photoelectrocatalytic removal of MO and MB by a TiO2/PANI/PVDF composite conducting membrane.134 Reprinted with permission from ref. 134, copyright 2022 Elsevier. (c) Mechanistic diagram of adsorption and electrochemical oxidation removal for bisphenol A by PANI/CNTs.106 Reprinted with permission from ref. 106, copyright 2023 SpringerLink. (d) Mechanistic diagram for electrically enhanced adsorption of Pb2+ by a HZSM-5@PANI/PSS composite membrane.135 Reprinted with permission from ref. 135, copyright 2018 Elsevier. | |
For weakly charged or uncharged organic contaminants, the pore sizes and uniformities of conventional polymer membranes usually determine the separation performance. Synthesis of membrane materials with small pore sizes places strict requirements on the preparation method and causes some contaminants to be gradually transferred to the membrane during adsorption under pressure, which affects the permeation flux of the membrane. In contrast, the PANI conductive membrane have exhibited effective removal of uncharged organic contaminants by direct and indirect oxidation under an applied electric field. For example, Zhang et al. found that PANI/CNTs membranes oxidised bisphenol A at a voltage of +2.0 V (Fig. 8c), with a removal rate of almost 100%.106 Additionally, intermittent control of the external voltage effectively removed bisphenol A adsorbed on the surface of the membrane electrodes, resulting in a retention rate of approximately 100% during long-term operation.
The unique properties of PANI conductive membranes can also be used for the treatment of salt-containing dye wastewater. Liu et al. used polyimide (PI)/PANI conductive membranes to treat wastewater containing Congo red (CR) dye.136 The addition of Mg2+ resulted in a nearly fourfold increase in the permeate flux (204.1 L m−1 bar−1), while the CR retention rate remained unchanged. This was attributed to the formation of large ligand structures due to electrostatic interactions between Mg2+, PANI and a dissociated sulfonate on the CR. On the one hand, this provided synergistic removal of the dye and salt ions, and on the other hand, it provided more channels for diffusion of the water molecules, which significantly improved the permeate flux of the membrane. In addition, the good conductivity and photoresponsivity of PANI enable its use in photoelectrocatalytic removal of dyes. Sboui et al. reported a novel ternary conductive MF membrane based on PANI and TiO2 doping and used it for removal of azo dyes from wastewater.134 The added PANI broadened the overall light absorption range of the membrane, and its high conductivity allowed the electrons to move rapidly towards the cathode under the applied voltage while avoiding secondary binding with holes (Fig. 8b). The contaminant s and holes on the membrane surface underwent direct oxidation to form active intermediates, which were then removed by indirect oxidation of –OH.
5.1.2 Heavy metal removal.
Adsorption is a fast and efficient means of removing heavy metal contaminants. There are many reports of PANI composites used in the treatment of heavy metal-containing wastewater. For example, Eskandari et al. provided a detailed review of PANI composite adsorbent materials used for the treatment of heavy metal-containing wastewater.52 However, most of these systems relied on physical adsorption by the PANI material itself,137,138 and its electrical properties were never applied. An applied voltage can enhance the adsorption of heavy metals from water by increasing the surface charge of the PANI membrane, and rapid desorption can be achieved with the reverse voltage. Du et al. prepared the highly-crystalline protonic acid Zeolite Socony Mobil-5 (HZSM-5)@PANI/PSS composite electroresponsive membrane with the potential pulse method and used it for electrosorption and desorption of Pb(II) on the membrane by precisely modulating the electrode potential.135 When a voltage of 0.21 V was applied, the membrane underwent reduction, and to ensure the overall electroneutrality of the membrane, the Pb(II) in solution was adsorbed onto the surface of the PANI/PSS and moved towards the internal HZSM-5 as the applied voltage was decreased, where the energy required for dehydration of the Pb(II) was provided by the applied electric field. When a voltage of 0.75 V was applied, H on the PANI backbone chain were transferred to HZSM-5 by oxidation to drive the desorption of Pb(II), and the amount adsorbed reached 240.6 mg g−1; the mechanism is shown in Fig. 8d.
5.2 Ion rejection and sieving
In addition to their use in enhanced contaminant removal, PANI conductive membranes have shown satisfactory results in ion rejection and sieving. It is well known that membranes used for ion sieving always have a “trade-off” effect in terms of selection and permeability, which seriously limits their practical application. PANI's excellent conductivity, unique polymerisation method and redox reaction effectively increase the upper “trade-off” limit of conductive membranes. Previous studies have shown that ion exchange membranes (IEMs) doped with PANI exhibited superior intramembrane electron conductivity and high ion exchange capacities.139–143 Influenced by the mode of aniline polymerisation, ectopic nucleation growth of aniline on sulfonated polyphenylene ether substrates can be achieved by controlling the reaction conditions. The PANI nanopores with a conical structure showed high performance in the separation of mono- and divalent cations (Fig. 9a). During transmembrane transport, the electrostatic repulsion between the cations and the positively charged conical pores gradually increased, and more highly charged divalent cations could not pass through, allowing only monovalent cations to be transported across the membrane. In addition, the applied electric field formed a pair of opposite vortices on the inner surface of the membrane pores, which effectively prevented the generation of a concentration polarisation layer on the membrane surface and ensured the stability of the membrane flux (Fig. 9b).144 However, the applied electric field must be conducted through a variety of media, which increased the energy required for the ion sieving process. For this reason, Zhang et al. developed a PSSA-doped CNTs/PANI conductive membrane and used it directly as the cathode in, an electrically assisted NF system (Fig. 9c).81 The polymerisation of aniline on CNT effectively improved the overall conductivity of the membrane. The polarisation of the conductive membrane under voltage significantly increased the charge density on the membrane surface. In addition, the reduction of PANI at the cathodic potential further increased the charge on the membrane surface. As a result, the increased Donnan potential difference between the membrane phase and the solution phase effectively improved the separation performance of ions without affecting the membrane flux.
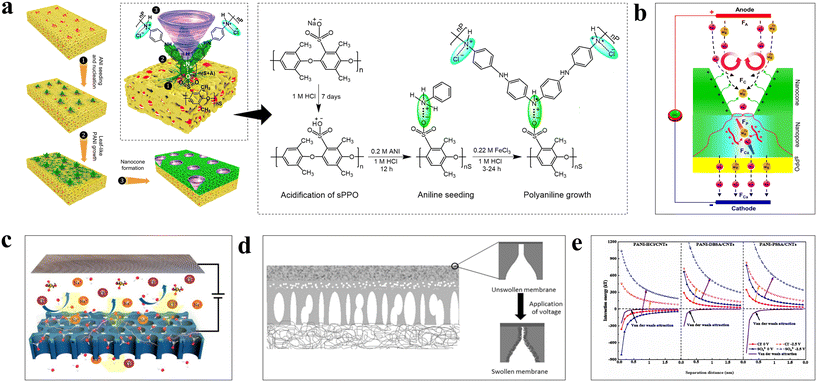 |
| Fig. 9 (a) Mechanistic diagram for PANI formation of conical pore structure on sPPO surface by ectopic nucleation.144 (b) Mechanism diagram for permselective conical pores for mono- and divalent cations under electric field.144 Reprinted with permission from ref. 144, copyright 2019 Wiley. (c) Schematic diagram of the electric field assisted nanofiltration system with PANI/CNT conductive membrane as cathode material.81 (d) Schematic diagram of the swelling effect of PANI conductive membranes under electrical stimulation.145 Reprinted with permission from ref. 145, copyright 2018 Elsevier. (e) Magnitudes of the interaction energies between PANI/CNTs conducting membranes doped by HCl, DBSA and PSSA with SO42− and Cl− in the presence or absence of an applied voltage, as calculated with DLVO theory.82 Reprinted with permission from ref. 82, copyright 2022 Elsevier. | |
A study by Xu et al. showed that the PANI backbone chains were swollen by an applied voltage, which caused the membrane pore sizes to shrink and improved the rejection performance of the substance (Fig. 9d).145 When PANI conductive membranes served as electrode materials and underwent oxidation reactions, the polymer backbone was positively charged. To ensure charge balance, the ligand anions of the dopant acid in the solution spontaneously entered the polymer together with the solvent, which ultimately led to expansion of the membrane volume. A higher applied voltage and greater membrane conductivity led to, more pronounced swelling, and the process was reversible without damage to the membrane structure. This provides a new idea for PANI conductive membranes used in electroresponsive ion sieving. With the Derjaguin–Landau–Verwey–Overbeek (DLVO) theory, Xu et al. demonstrated that the interaction force between the PANI membrane and SO42− was significantly larger than that of Cl− at an applied voltage (Fig. 9e), which led to efficient electrically responsive mono- and divalent anion separation.82 In addition, Xu et al. tested the NaCl, Na2SO4 and MgCl2 rejection efficiencies of three membranes in acid conditions.146 Rejection of the ions by the HCl-doped conductive membranes decreased with increasing acid immersion time due to dissolution and destruction of the PANI backbone chains. However, the PSSA- and DBSA-doped PANI ensured the stability of the membrane due to strong electrostatic interactions between the backbone chains, and showed stable retention of ions. Notably, the negatively charged PANI/PSSA/CNTs and PANI/DBSA/CNTs membranes showed significantly higher rejection of Mg2+ than the positively charged PANI/HCl/CNTs membranes, probably because SO3− was more readily bound to Mg2+; the Mg2+ content inside the membrane was enriched due to the binding energy, which prevented diffusion of the remaining Mg2+.147,148
5.3 Oil–water separation
Depending on the interfacial forces, current oil–water separation membranes can be classified as hydrophilic–oleophobic, lipophilic–hydrophobic and responsive. In principle, the hydrophilic N–H group and the hydrophobic phenyl group in the PANI generate amphiphilicity, but doping with the protonic acid causes hydrophilicity in most cases.149 Recently, it was found that the conductivity of PANI enabled controllable wetting properties under applied voltage. Zheng et al. used emulsion polymerisation and prepared oil–water separation membranes with a branched PANI network on a stainless steel mesh, which were superhydrophobic in air and superoleophobic under water.150 When a voltage of 170 V was applied to the membrane, the capillary electric pressure overcame the static pressure of water at the microporous interface; this allowed the water to contact and completely wet the membrane surface, and the water contact angle decreased from 146° to 70°. The underwater oil contact angle was not affected by the external voltage, which prevented the oil phase from moving downwards through the membrane surface, thus realising the electrically controlled oil–water separation with PANI membranes.
5.4 Membrane fouling mitigation
During water treatment, substances adsorbed and accumulated on the membrane surface can contaminate the membrane and affect its separation performance. It is well known that membranes with greater hydrophilicity usually resist fouling effectively. The N–H and acid-doped groups on the PANI chains provided hydrophilic conductive membranes and inhibited membrane fouling.54 In addition, electrostatic repulsion on the membrane surface was more effective in preventing membrane fouling than the hydrophilicity. For example, the increased surface charge of the cathode membrane under applied voltage enhanced the electrostatic repulsion of contaminants.85,120,151 However, in an actual separation process, diffusion and extrusion effects may be stronger than electrostatic repulsion between the contaminants and the membrane, resulting in transfer of some of the contaminants to the interior of the membrane pores. Electrochemical oxidation is an extremely effective and efficient way to mitigate membrane fouling. Liu et al. found that large amounts of –OH and OCl− were generated on the surface of a PANI/PI membrane at an applied voltage of 10, which achieved oxidative decomposition of CR molecules within the membrane pores and, a membrane flux recovery rate of 87%.136 Additionally, –OH attacked the chemical bonds of the contaminants to enhance their hydrophilicities, reduce their effective molecular volumes in water and enable adsorption on the membrane surface. In terms of reaction rate, indirect oxidation usually proceeds faster and plays a major role in mitigating membrane fouling. Shakeri et al. suggested that the removal of alginate by PANI/GO at an applied voltage was mainly accomplished through reactions with the –OH groups generated on the membrane surface, while direct oxidation only played an auxiliary role.110 In addition, the electrons provided by the external voltage facilitated the movement and reorganisation of the anions along the main chain of PANI, which changed the membrane structure and effectively reduced the retention and accumulation of contaminants in the membrane.79,119
The introduction of metal atoms is an effective was to improve the antifouling performance of PANI conductive membranes. AgNP has been widely used in water treatment as a broad-spectrum bactericidal material, and its chemical stability prevents it from generating sterilisation by-products during electrochemical processes and endows the PANI membranes with higher conductivities.152 Zhou et al. tested the antibacterial performance of AgNP-doped PANI composite conductive membranes against S. aureus.153 The results showed that the bacterial attachment on the surface of PANI/AgNP membrane was reduced by 3.5 orders of magnitude compared to that on the PSF membrane. The amount of S. aureus attachment was reduced by two orders of magnitude when a DC voltage was applied. The membrane showed almost complete resistance to contamination when an AC voltage was applied. In addition, Li et al. prepared a sulfuric acid doped GO/PANI membrane with GO and PANI, while Cu2+ was introduced to stabilise the membrane structure.85 Interestingly, the Cu2+ destroyed the cell walls of E. coli by electrostatic attraction while improving the stability of the GO/PANI membrane, and the inhibition rate of E. coli was as high as 92.1%.
5.5 Membrane fouling monitoring
Rapid in situ monitoring of membrane fouling is essential for membrane-based water treatment technologies. In the traditional methods used for monitoring membrane fouling, the long lag times and complex procedure usually do not reflect the degree of membrane fouling in a timely and accurate manner. Although changes in the membrane flux respond directly to the degree of fouling on the membrane surface, there is still a certain lag in the feedback time, which has been well demonstrated in the relevant report by Tay et al.154 The good electrochemical properties of PANI conductive membranes allow real-time monitoring with feedback from electrical signals, e.g., the accumulated metal ions or organic contaminants on the membrane surface will change the membrane resistance. Yuan et al. explored the fouling of membranes with BSA tests of the resistance and flux of PANI/MWCNTs conductive membranes.75 The membrane flux and resistance decreased with the increase of the fouling level and showed a three-stage linear relationship, corresponding to the three stages of “initial adsorption – fouling layer thickening – compaction” of BSA (Fig. 10a), and stage 2 was the optimal time to clean the membrane. This was the first report on monitoring membrane fouling of PANI conductive membranes with linear voltammetric scanning. Wang et al. performed real-time monitoring of membrane fouling by CV curves of PANI/GO/CNTs membranes.77 When the surface of the PANI/GO/CNTs was contaminated, the foulants accumulated on the membrane surface prevented direct contact between the membrane material and the electrolyte, resulting in disappearance of the redox peaks at 0.2 V, 0.3 V and 0.5 V on the CV curve (Fig. 10b). In addition, reports of PANI conductive membranes used for monitoring electrochemical membrane fouling are relatively sparse at present, and there is much room for additional research.
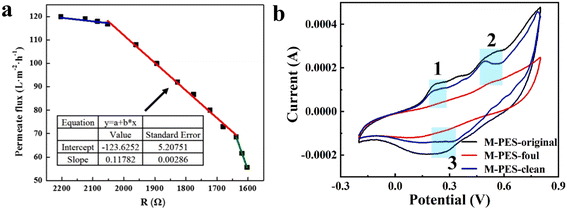 |
| Fig. 10 (a) CV plots showing different stages of contamination layer formation on the PANI/MWCNTs conductive membrane surface.75 Reprinted with permission from ref. 75, copyright 2019 Elsevier. (b) Linear plot of membrane resistance and permeation flux for the PANI/GO/CNTs conductive membrane at the applied voltage.77 Reprinted with permission from ref. 77, copyright 2021 Elsevier. | |
6. Summary and outlook
In recent years, the rapid development of electrochemical technology has created higher application value in the field of membrane separation. Among these technologies, conductive membrane systems show better performance in terms of water flux, separation accuracy, antifouling and energy consumption compared with separation system in which the membrane is placed between electric fields. Conjugated polymers, mainly PANI, are widely used in the preparation of conductive membranes due to their unique conductive properties, reversible doping properties and strong stability. In this paper, starting from the material properties of PANI, the influence of different preparation methods and doping modification on the structure and performance of PANI conductive membranes is deeply analysed, and the current application of PANI conductive membranes in water treatment is summarised. Overall, the emergence of PANI conductive membranes provides a more efficient and convenient method for treating water, which is of great value in environmental control and resource recovery. It is believed that research on PANI conductive membranes will continue to increase in the future, but there are still problems that must be solved in the current research process.
The synthesis of PANI and the convenient and controllable operating conditions have led to the preparation of PANI conductive membranes mostly by LBL self-assembly or the NIPS method, but it has always faced challenges in industrialisation. While in situ polymerisation of aniline is the most likely promising for large-scale fabrication, the membranes are more difficult to control in terms of structure and morphology due to a variety of factors, and therefore complex optimisation of the reaction conditions may be required when treating real water bodies. ISEO is one of the least commonly used preparation methods, which may be related to its lower yield and poorer separation accuracy. In addition, post-treatment as an essential process is particularly important in the preparation of PANI conductive membranes. Among post-treatment methods, chemical crosslinking is the preferred process due to its mild reaction conditions and stable performance. Thermal crosslinking and flash welding, on the other hand, are currently commonly used in water treatment processes that are focused on antifouling or do not require high separation accuracy due to their poor controllability. In conclusion, when preparing PANI conductive membranes, improving the precision of controlling the morphology and structure of the membranes is a challenge that needs to be solved. In addition, it is particularly important to take into account the comprehensive benefits of the preparation process in terms of cost, environmental protection and industrialisation.
In terms of doping modification, the functionalization of nanomaterials effectively improves the interaction force with PANI, but the formation of defects severely reduces the overall electron transport ability of the membrane. In addition, PANI conductive membranes doped with macromolecular acids have superior stability compared to those doped with small-molecule acids exhibit poor conductivity. Therefore, improving the trade-off effect between conductivity and stability of PANI membranes is an issue that urgently needs to be addressed. It has been reported that the photoelectric conversion ability of PANI has great potential to improve the wastewater treatment effect, and the good photoelectrocatalytic response effectively compensates for the insufficient conductivity of the membrane. In addition, the higher conductivity of the membrane places higher requirements on its antioxidant ability at anodic potential. PANI as a sacrificial material can effectively protect nanomaterials from corrosion under the anodic potential, but the oxidation of aniline will inevitably reduce the separation performance of the membrane. Therefore, future research should focus on the stability of PANI conductive membranes at high anodic potentials.
To date, PANI conductive membranes under the action of electric potential have been shown to be excellent for water treatment. Depending on the preparation conditions, most of the currently synthesised PANI conductive membranes are applied in antifouling, oil–water separation or organic contaminant removal, while ion separation is more difficult. In addition, the specific separation and electrical response mechanisms of PANI conductive membranes have not yet been fully investigated. For example, the expansion and contraction of PANI conductive membranes under voltage is a comprehensive process, and most of the previous studies have only considered the effects of single factors. In addition, existing studies have demonstrated that the electrochemical redox effect of PANI causes differences in the binding capacity of different ions, but how to achieve accurate sieving still needs to be further explored. In terms of membrane fouling mitigation and monitoring, the introduction of electrochemistry has endowed PANI conductive membranes with good antifouling and ultrafast membrane fouling monitoring-feedback pathways. However, the current research remains at the level of apparent phenomena and simple mechanism analysis, and the complex electrochemical redox reactions occurring between membrane electrodes and pollutants still need to be studied in depth. It should be emphasise that PANI conductive membranes can only operate in low-pH environments, whereas natural water bodies such as salt lake brines and seawater are usually weakly alkaline or basic, which may have an impact on the structure and conductivity of PANI membranes. In addition, the effect of the complex ionic environment in brine and seawater on the selective separability of PANI conductive membranes is still unclear. Therefore, broadening the applicability of PANI conductive membranes for the treatment of actual water bodies and in-depth analysis of their separation performance in mixed-salt systems are future challenges that need to be addressed.
Conflicts of interest
There are no conflicts to declare.
Acknowledgements
This research was supported by the National Key R&D Programs (2022YFC2904302) and the Research of Tibet Province program (XZ202101ZY0006G) and the Shaanxi Provincial Department of Education Service Local Special Scientific Research Project (No. 22JC041).
References
- D. J. Johnson and N. Hilal, Nanocomposite nanofiltration membranes: State of play and recent advances, Desalination, 2022, 524, 115480 CrossRef CAS.
- T. Zhang, W. Zheng, Q. Wang, Z. Wu and Z. Wang, Designed strategies of nanofiltration technology for Mg2+/Li+ separation from salt-lake brine: A comprehensive review, Desalination, 2023, 546, 116205 CrossRef CAS.
- Y. Qiu, S. Depuydt, L.-F. Ren, C. Zhong, C. Wu, J. Shao, L. Xia, Y. Zhao and B. Van Der Bruggen, Progress of Ultrafiltration-Based Technology in Ion Removal and Recovery: Enhanced Membranes and Integrated Processes, ACS ES&T Water, 2023, 3, 1702–1719 Search PubMed.
- M. Qasim, N. A. Darwish, S. Sarp and N. Hilal, Water desalination by forward (direct) osmosis phenomenon: A comprehensive review, Desalination, 2015, 374, 47–69 CrossRef CAS.
- H. B. Park, J. Kamcev, L. M. Robeson, M. Elimelech and B. D. Freeman, Maximizing the right stuff: The trade-off between membrane permeability and selectivity, Science, 2017, 356, 6343 CrossRef PubMed.
- S. A. N. Mehrabani, T. S. Rad, V. Vatanpour, A. Khataee, M. Kaya, B. Zeytuncu and I. Koyuncu, CuCr NLDH-Graphene oxide blended polyvinyl chloride ultrafiltration membrane with improved permeability and antifouling behavior, Sep. Purif. Technol., 2023, 317, 123931 CrossRef.
- T. A. Sherazi, S. Azam, S. H. Shah, S. Hussain, S. A. R. Naqvi and S. Li, Zwitterionic analog structured ultrafiltration membranes for high permeate flux and improved anti-fouling performance, J. Membr. Sci., 2022, 643, 120060 CrossRef CAS.
- F. Yao, W. Zhang, D. Hu, S. Li, X. Kong, S. Uemura, T. Kusunose and Q. Feng, Ultra-hydrophilic layered titanate nanosheet-based nanofiltration membrane with ultrafast water transport for low energy consumption desalination, Desalination, 2022, 544, 116144 CrossRef CAS.
- Y. Liang, Y. Zhu, C. Liu, K.-R. Lee, W.-S. Hung, Z. Wang, Y. Li, M. Elimelech, J. Jin and S. Lin, Polyamide nanofiltration membrane with highly uniform sub-nanometre pores for sub-1 Å precision separation, Nat. Commun., 2020, 11, 1–9 CrossRef PubMed.
- U. Baig and A. Waheed, Exploiting interfacial polymerization to fabricate hyper-cross-linked nanofiltration membrane with a constituent linear aliphatic amine for freshwater production, npj Clean Water, 2022, 5, 1–13 CrossRef.
- H.-C. Yang, Y. Xie, J. Hou, A. K. Cheetham, V. Chen and S. B. Darling, Janus Membranes: Creating Asymmetry for Energy Efficiency, Adv. Mater., 2018, 30, 1801495 CrossRef PubMed.
- H. Zhou and Z. Guo, Superwetting Janus membranes: focusing on unidirectional transport behaviors and multiple applications, J. Mater. Chem. A, 2019, 7, 12921–12950 RSC.
- J. Wang, Z. Zhang, J. Zhu, M. Tian, S. Zheng, F. Wang, X. Wang and L. Wang, Ion sieving by a two-dimensional Ti3C2Tx alginate lamellar membrane with stable interlayer spacing, Nat. Commun., 2020, 11, 1–10 CrossRef PubMed.
- J. Abraham, K. S. Vasu, C. D. Williams, K. Gopinadhan, Y. Su, C. T. Cherian, J. Dix, E. Prestat, S. J. Haigh, I. V. Grigorieva, P. Carbone, A. K. Geim and R. R. Nair, Tunable sieving of ions using graphene oxide membranes, Nat. Nanotechnol., 2017, 12, 546–550 CrossRef CAS PubMed.
- T. Xiao, Z. Zhu, L. Li, J. Shi, Z. Li and X. Zuo, Membrane fouling and cleaning strategies in microfiltration/ultrafiltration and dynamic membrane, Sep. Purif.
Technol., 2023, 318, 123977 CrossRef CAS.
- Y.-H. Cai, N. Galili, Y. Gelman, M. Herzberg and J. Gilron, Evaluating the impact of pretreatment processes on fouling of reverse osmosis membrane by secondary wastewater, J. Membr. Sci., 2021, 623, 119054 CrossRef CAS.
- R. Miao, B. Ma, P. Li, P. Wang, L. Wang and X. Li, Mitigation mechanism of ozonation in the casein fouling of ultrafiltration membranes: Possible application in dairy wastewater treatment, J. Membr. Sci., 2021, 629, 119307 CrossRef CAS.
- E. Arkhangelsky, A. Bazarbayeva, A. Kamal, J. Kim, V. Inglezakis and V. Gitis, Tangential streaming potential, transmembrane flux, and chemical cleaning of ultrafiltration membranes, Sep. Purif. Technol., 2021, 258, 118045 CrossRef CAS.
- X. Gan, T. Lin, F. Jiang and X. Zhang, Impacts on characteristics and effluent safety of PVDF ultrafiltration membranes aged by different chemical cleaning types, J. Membr. Sci., 2021, 640, 119770 CrossRef CAS.
- Y.-H. Cheng, A. Y. Kirschner, C.-C. Chang, Z. He, M. Nassr, T. Emrick and B. D. Freeman, Surface Modification of Ultrafiltration Membranes with 1,4-Benzoquinone and Polyetheramines to Improve Fouling Resistance, ACS Appl. Mater. Interfaces, 2022, 14, 52390–52401 CrossRef CAS PubMed.
- S. Huang, J. A. McDonald, R. P. Kuchel, S. J. Khan, G. Leslie, C. Tang, J. Mansouri and A. G. Fane, Surface modification of nanofiltration membranes to improve the removal of organic micropollutants: Linking membrane characteristics to solute transmission, Water Res., 2021, 203, 117520 CrossRef CAS PubMed.
- X. Zhang, Y. Zhou, F. Zhao, C. Geng, Z. Li, J. Zhang, Y. Yang and H. Chen, Anti-fouling mechanism of ultrafiltration membranes modified by graphene oxide with different charged groups under simulated seawater conditions, J. Membr. Sci., 2023, 674, 121483 CrossRef CAS.
- Q. Feng, Y. Zhan, W. Yang, H. Dong, A. Sun, L. Li, X. Chen and Y. Chen, Ultra-high flux and synergistically enhanced anti-fouling Ag@MXene lamellar membrane for the fast purification of oily wastewater through nano-intercalation, photocatalytic self-cleaning and antibacterial effect, Sep. Purif. Technol., 2022, 298, 121635 CrossRef CAS.
- L. Liu, K. Li, S. Zhao, J. Wang, H. Lan and J. Wang, The effects of electrophoresis, bubbles and electroosmosis for conductive membrane performance in the electro-filtration process, J. Membr. Sci., 2021, 620, 118955 CrossRef CAS.
- X. Zhu and D. Jassby, Electroactive Membranes for Water Treatment: Enhanced Treatment Functionalities, Energy Considerations, and Future Challenges, Acc. Chem. Res., 2019, 52, 1177–1186 CrossRef CAS PubMed.
- G. Yi, L. Du, G. Wei, H. Zhang, H. Yu, X. Quan and S. Chen, Selective molecular separation with conductive MXene/CNT nanofiltration membranes under electrochemical assistance, J. Membr. Sci., 2021, 658, 120719 CrossRef.
- J. R. Varcoe, P. Atanassov, D. R. Dekel, A. M. Herring, M. A. Hickner, P. A. Kohl, A. R. Kucernak, W. E. Mustain, K. Nijmeijer, K. Scott, T. W. Xu and L. Zhuang, Anion-exchange membranes in electrochemical energy systems, Energy Environ. Sci., 2014, 7, 3135–3191 RSC.
- X. Fan, G. Wei and X. Quan, Carbon nanomaterial-based membranes for water and wastewater treatment under electrochemical assistance, Environ. Sci.: Nano, 2023, 10, 11–40 RSC.
- G. Wei, L. Du, H. Zhang, J. Xing, S. Chen and X. Quan, Electrochemical Opening of Impermeable Nanochannels in Laminar Graphene Membranes for Ultrafast Nanofiltration, Environ. Sci. Technol., 2023, 57, 3843–3852 CrossRef CAS PubMed.
- H.-G. Zhang, X. Quan, L. Du, G.-L. Wei, S. Chen, H.-T. Yu and Y.-C. Dong, Electroregulation of graphene–nanofluid interactions to coenhance water permeation and ion rejection in vertical graphene membranes, Proc. Natl. Acad. Sci. U. S. A., 2023, 120, e2219098120 CrossRef CAS PubMed.
- X. Zhu, A. V. Dudchenko, C. M. Khor, X. He, G. Z. Ramon and D. Jassby, Field-Induced Redistribution of Surfactants at the Oil/Water Interface Reduces Membrane Fouling on Electrically Conducting Carbon Nanotube UF Membranes, Environ. Sci. Technol., 2018, 52, 11591–11600 CAS.
- X. Fan, H. Zhao, X. Quan, Y. Liu and S. Chen, Nanocarbon-based membrane filtration integrated with electric field driving for effective membrane fouling mitigation, Water Res., 2016, 88, 285–292 CrossRef CAS PubMed.
- W. Fu, X. Wang, J. Zheng, M. Liu and Z. Wang, Antifouling performance and mechanisms in an electrochemical ceramic membrane reactor for wastewater treatment, J. Membr. Sci., 2019, 570, 355–361 CrossRef.
- J. H. Lee, E.-T. Yun, S.-Y. Ham, H.-S. Kim, P.-F. Sun and H.-D. Park, Electrically conductive carbon nanotube/graphene composite membrane for self-cleaning of biofouling via bubble generation, Desalination, 2022, 535, 115841 CrossRef CAS.
- S. F. Anis, B. S. Lalia, R. Hashaikeh and N. Hilal, Ceramic nanofiltration membranes for efficient fouling mitigation through periodic electrolysis, Sep. Purif. Technol., 2022, 303, 122228 CrossRef CAS.
- L. L. Xu, L. Liu, K. P. Wang, S. Y. Zhao, Q. Y. Liu, Y. Zhang and J. Wang, Development of a novel electrocoagulation membrane reactor with electrically conductive membranes as cathode to mitigate membrane fouling, J. Membr. Sci., 2021, 618, 118713 CrossRef CAS.
- X. Xu, H. Zhang, M. Yu, Y. Wang, T. Gao and F. Yang, Conductive thin film nanocomposite forward osmosis membrane (TFN-FO) blended with carbon nanoparticles for membrane fouling control, Sci. Total Environ., 2019, 697, 134050 CrossRef CAS PubMed.
- Q. Zhang, J. Wang and C. D. Vecitis, Fouling reduction and recovery during forward osmosis of wastewater using an electroactive CNT composite membrane, J. Membr. Sci., 2021, 620, 118803 CrossRef CAS.
- Q. Li, H. Liu, B. He, W. Shi, Y. Ji, Z. Cui, F. Yan, Y. Mohammad and J. Li, Ultrahigh-efficient separation of Mg2+/Li+ using an in-situ reconstructed positively charged nanofiltration membrane under an electric field, J. Membr. Sci., 2022, 641, 119880 CrossRef CAS.
- M. Sun, X. Wang, L. R. Winter, Y. Zhao, W. Ma, T. Hedtke, J.-H. Kim and M. Elimelech, Electrified Membranes for Water Treatment Applications, ACS ES&T Engg, 2021, 1, 725–752 Search PubMed.
- Q. Zhang and C. D. Vecitis, Conductive CNT-PVDF membrane for capacitive organic fouling reduction, J. Membr. Sci., 2014, 459, 143–156 CrossRef CAS.
- N. Ghaemi, S. S. Madaeni, P. Daraei, H. Rajabi, T. Shojaeimehr, F. Rahimpour and B. Shirvani, PES mixed matrix nanofiltration membrane embedded with polymer wrapped MWCNT: Fabrication and performance optimization in dye removal by RSM, J. Hazard. Mater., 2015, 298, 111–121 CrossRef CAS PubMed.
- G. Liao, Q. Li and Z. Xu, The chemical modification of polyaniline with enhanced properties: A review, Prog. Org. Coat., 2019, 126, 35–43 CrossRef CAS.
- H. Shirakawa, The Discovery of Polyacetylene Film: The Dawning of an Era of Conducting Polymers (Nobel Lecture), Angew. Chem., Int. Ed., 2001, 40, 2574–2580 CrossRef.
- G. A. Snook, P. Kao and A. S. Best, Conducting-polymer-based supercapacitor devices and electrodes, J. Power Sources, 2011, 196, 1–12 CrossRef CAS.
- R. S. Bobade, Polythiophene composites: a review of selected applications, J. Polym. Eng., 2011, 31, 209–215 CAS.
- M. N. Naseer, K. Dutta, A. A. Zaidi, M. Asif, A. Alqahtany, N. A. Aldossary, R. Jamil, S. H. Alyami and J. Jaafar, Research Trends in the Use of Polyaniline Membrane for Water Treatment Applications: A Scientometric Analysis, Membranes, 2022, 12, 777 CrossRef CAS PubMed.
- A. Ronen, S. L. Walker and D. Jassby, Electroconductive and electroresponsive membranes for water treatment, Rev. Chem. Eng., 2016, 32, 533–550 CrossRef CAS.
- N. H. Barbhuiya, U. Misra and S. P. Singh, Synthesis, fabrication, and mechanism of action of electrically conductive membranes: a review, Environ. Sci.: Water Res. Technol., 2021, 7, 671–705 RSC.
- Y. Jiang, Z. Liu, G. Zeng, Y. Liu, B. Shao, Z. Li, Y. Liu, W. Zhang and Q. He, Polyaniline-based adsorbents for removal of hexavalent chromium from aqueous solution: a mini review, Environ. Sci. Pollut. Res., 2018, 25, 6158–6174 CrossRef CAS PubMed.
- S. Senguttuvan, P. Senthilkumar, V. Janaki and S. Kamala-Kannan, Significance of conducting polyaniline based composites for the removal of dyes and heavy metals from aqueous solution and wastewaters - A review, Chemosphere, 2021, 267, 129201 CrossRef CAS PubMed.
- E. Eskandari, M. Kosari, M. H. D. A. Farahani, N. D. Khiavi, M. Saeedikhani, R. Katal and M. Zarinejad, A review on polyaniline-based materials applications in heavy metals removal and catalytic processes, Sep. Purif. Technol., 2020, 231, 115901 CrossRef CAS.
- K. V. Kurada and S. De, Polyaniline doped ultrafiltration membranes: Mechanism of membrane formation and pH response characteristics, Polymers, 2018, 153, 201–213 CrossRef CAS.
- C.-W. Lin, S. Xue, C. Ji, S.-C. Huang, V. Tung and R. B. Kaner, Conducting Polyaniline for Antifouling Ultrafiltration Membranes: Solutions and Challenges, Nano Lett., 2021, 21, 3699–3707 CrossRef CAS PubMed.
- J.-W. Li, Y. Zhou, J. Xu, F. Gao, Q.-K. Si, J.-Y. Wang, F. Zhang and L.-P. Wang, Water-Soluble and Degradable Gelatin/Polyaniline Assemblies with a High Photothermal Conversion Efficiency for pH-Switchable Precise Photothermal Therapy, ACS Appl. Mater. Interfaces, 2022, 14, 52670–52683 CrossRef CAS PubMed.
- L. Xu, C. Liu, X. Ma, Y. Xu, W. Zhou, W. Guan, Q. Qiang, T. Lang, L. Peng, Y. Zhong, Y. A. Nikolaevich, Z. Zhou and B. Liu, Two-birds-one-stone: Flexible PANI film with bionic microstructures for multifunctional sensing of physical and chemical stimuli, Chem. Eng. J., 2023, 451, 138820 CrossRef CAS.
- D. W. DeBerry, Modification of the Electrochemical and Corrosion Behavior of Stainless Steels with an Electroactive Coating, J. Electrochem. Soc., 1985, 132, 1022 CrossRef CAS.
- P. Modak, S. B. Kondawar and D. V. Nandanwar, Synthesis and Characterization of Conducting Polyaniline/Graphene Nanocomposites for Electromagnetic Interference Shielding, Procedia Mater. Sci., 2015, 10, 588–594 CrossRef CAS.
- M.-L. Liu, L. Li, M.-J. Tang, L. Hong, S.-P. Sun and W. Xing, Multi-component separation of small molecular/ionic pollutants with smart pH-gating membranes, Chem. Eng. Sci., 2021, 245, 116854 CrossRef CAS.
- J. Stejskal, I. Sapurina and M. Trchová, Polyaniline nanostructures and the role of aniline oligomers in their formation, Prog. Polym. Sci., 2010, 35, 1420–1481 CrossRef CAS.
- X. Li, C. Liu, W. Yin, T. H. Chong and R. Wang, Design and development of layer-by-layer based low-pressure antifouling nanofiltration membrane used for water reclamation, J. Membr. Sci., 2019, 584, 309–323 CrossRef CAS.
- S. Zhao, F. Caruso, L. Däehne, G. Decher, B. G. De Geest, J. Fan, N. Feliu, Y. Gogotsi, P. T. Hammond, M. C. Hersam, A. Khademhosseini, N. Kotov, S. Leporatti, Y. Li, F. Lisdat, L. M. Liz-Marzán, S. Moya, P. Mulvaney, A. L. Rogach, S. Roy, D. G. Shchukin, A. G. Skirtach, M. M. Stevens, G. B. Sukhorukov, P. S. Weiss, Z. Yue, D. Zhu and W. J. Parak, The Future of Layer-by-Layer Assembly: A Tribute to ACS Nano Associate Editor Helmuth Möhwald, ACS Nano, 2019, 13, 6151–6169 CrossRef CAS PubMed.
- G.-M. Weng, J. Li, M. Alhabeb, C. Karpovich, H. Wang, J. Lipton, K. Maleski, J. Kong, E. Shaulsky, M. Elimelech, Y. Gogotsi and A. D. Taylor, Layer-by-Layer Assembly of Cross-Functional Semi-transparent MXene-Carbon Nanotubes Composite Films for Next-Generation Electromagnetic Interference Shielding, Adv. Funct. Mater., 2018, 28, 1803360 CrossRef.
- K. Kang, K.-H. Lee, Y. Han, H. Gao, S. Xie, D. A. Muller and J. Park, Layer-by-layer assembly of two-dimensional materials into wafer-scale heterostructures, Nature, 2017, 550, 229–233 CrossRef PubMed.
- J. Lipton, G.-M. Weng, J. A. Röhr, H. Wang and A. D. Taylor, Layer-by-Layer Assembly of Two-Dimensional Materials: Meticulous Control on the Nanoscale, Matter, 2020, 2, 1148–1165 CrossRef.
- P. B. D. Firda, Y. T. Malik, J. K. Oh, E. K. Wujcik and J.-W. Jeon, Enhanced Chemical and Electrochemical Stability of Polyaniline-Based Layer-by-Layer Films, Polymers, 2021, 13, 2992 CrossRef CAS PubMed.
- L. Xu, K. Wang, K. Li, S. Zhao and J. Wang, Development and performance of stable PANI/MWNT conductive membrane for contaminants degradation and anti-fouling behavior, Sep. Purif. Technol., 2022, 282, 120112 CrossRef CAS.
- J.-W. Jeon, S. R. Kwon, F. Li and J. L. Lutkenhaus, Spray-On Polyaniline/Poly (acrylic acid) Electrodes with Enhanced Electrochemical Stability, ACS Appl. Mater. Interfaces, 2015, 7, 24150–24158 CrossRef CAS PubMed.
- A. Wang, W. Fang, J. Zhang, S. Gao, Y. Zhu and J. Jin, Zwitterionic Nanohydrogels–Decorated Microporous Membrane with Ultrasensitive Salt Responsiveness for Controlled Water Transport, Small, 2020, 16, 1903925 CrossRef CAS PubMed.
- P. Li, H. Xie, Y. Bi, C. Miao, K. Chen, T. Xie, S. Zhao, H. Sun, X. Yang, Y. Hou and Q. J. Niu, Preparation of high flux organic solvent nanofiltration membrane based on polyimide/Noria composite ultrafiltration membrane, Appl. Surf. Sci., 2023, 618, 156650 CrossRef CAS.
- R. A. Nuaimi, R. L. Thankamony, X. Liu, L. Cao, Z. Zhou and Z. Lai, Ultrafiltration membranes prepared via mixed solvent phase separation with enhanced performance for produced water treatment, J. Membr. Sci., 2023, 670, 121375 CrossRef CAS.
- C. Ursino, F. Russo, R. M. Ferrari, M. P. D. Santo, E. D. Nicolò, T. He, F. Galiano and A. Figoli, Polyethersulfone hollow fiber membranes prepared with Polarclean® as a more sustainable solvent, J. Membr. Sci., 2020, 608, 118216 CrossRef CAS.
- A. Ilyas, M. Mertens, S. Oyaert and I. F. J. Vankelecom, Synthesis of patterned PVDF ultrafiltration membranes: Spray-modified non-solvent induced phase separation, J. Membr. Sci., 2020, 612, 118383 CrossRef CAS.
- E. L. Subtil, J. Gonçalves, H. G. Lemos, E. C. Venancio, J. C. Mierzwa, J. D. S. D. Souza, W. Alves and P. L. Clech, Preparation and characterization of a new composite
conductive polyethersulfone membrane using polyaniline (PANI) and reduced graphene oxide (rGO), Chem. Eng. J., 2020, 390, 124612 CrossRef CAS.
- X.-S. Yuan, Z.-Y. Guo, H.-Z. Geng, D. S. Rhen, L. Wang, X.-T. Yuan and J. Li, Enhanced performance of conductive polysulfone/MWCNT/PANI ultrafiltration membrane in an online fouling monitoring application, J. Membr. Sci., 2019, 575, 160–169 CrossRef CAS.
- S. Zhao, Z. Wang, J. Wang and S. Wang, The effect of pH of coagulation bath on tailoring the morphology and separation performance of polysulfone/polyaniline ultrafiltration membrane, J. Membr. Sci., 2014, 469, 316–325 CrossRef CAS.
- Q. Wang, Y. Peng, X. Ji, M. K. Hadi, S. Zhang, J. Tang and F. Ran, Conductive 3D networks in a 2D layer for high performance ultrafiltration membrane with high flux-retention and robust cyclic stability, J. Membr. Sci., 2021, 640, 119781 CrossRef CAS.
- S. M. Sontakke and V. Awate, The effect of synthesis parameters on the conductivity of PSf/PANI and PSf/PPy composite membranes, Can. J. Chem. Eng., 2018, 96, 564–572 CrossRef CAS.
- J. N. Martins, M. Kersch, V. Altstädt and R. V. B. Oliveira, Electrical conductivity of poly (vinylidene fluoride)/polyaniline blends under oscillatory and steady shear conditions, Polym. Test., 2013, 32, 862–869 CrossRef CAS.
- P. He, S. Zhao, C. Mao, Y. Wang, G. Ma, Z. Wang and J. Wang, In-situ growth of double-layered polyaniline composite membrane for organic solvent nanofiltration, Chem. Eng. J., 2021, 420, 129338 CrossRef CAS.
- H. Zhang, X. Quan, X. Fan, G. Yi, S. Chen, H. Yu and Y. Chen, Improving Ion Rejection of Conductive Nanofiltration Membrane through Electrically Enhanced Surface Charge Density, Environ. Sci. Technol., 2019, 53, 868–877 CrossRef CAS PubMed.
- L. Xu, S. Liu, L. Yu, K. Li, Y. Zhang, J. Wang and J. Wang, Tuneable ion transport by electrically responsive membranes under electrical assistance, J. Membr. Sci., 2022, 663, 121046 CrossRef CAS.
- P. Cruz-Tato, N. Rivera-Fuentes, M. Flynn and E. Nicolau, Anti-Fouling Electroconductive Forward Osmosis Membranes: Electrochemical and Chemical Properties, ACS Appl. Polym. Mater., 2019, 1, 1061–1070 CrossRef CAS.
- Z. Pan, H. Xin, S. Xu, R. Xu, P. Wang, Y. Yuan, X. Fan, Y. Song, C. Song and T. Wang, Preparation and performance of polyaniline modified coal-based carbon membrane for electrochemical filtration treatment of organic wastewater, Sep. Purif. Technol., 2022, 287, 120600 CrossRef CAS.
- B. Li, W. Tang, D. Sun, B. Li, Y. Ge, X. Ye and W. Fang, Electrochemical manufacture of graphene oxide/polyaniline conductive membrane for antibacterial application and electrically enhanced water permeability, J. Membr. Sci., 2021, 640, 119844 CrossRef CAS.
- X.-M. Feng, R.-M. Li, Y.-W. Ma, R.-F. Chen, N.-E. Shi, Q.-L. Fan and W. Huang, One-Step Electrochemical Synthesis of Graphene/Polyaniline Composite Film and Its Applications, Adv. Funct. Mater., 2011, 21, 2989–2996 CrossRef CAS.
- W. Duan, A. Ronen, S. Walker and D. Jassby, Polyaniline-Coated Carbon Nanotube Ultrafiltration Membranes: Enhanced Anodic Stability for In Situ Cleaning and Electro-Oxidation Processes, ACS Appl. Mater. Interfaces, 2016, 8, 22574–22584 CrossRef CAS PubMed.
- B. Li, D. Sun, B. Li, W. Tang, P. Ren, J. Yu and J. Zhang, One-Step Electrochemically Prepared Graphene/Polyaniline Conductive Filter Membrane for Permeation Enhancement by Fouling Mitigation, Langmuir, 2020, 36, 2209–2222 CrossRef CAS PubMed.
- L. Wang, X. Guo, F. Zhang and N. Li, Blending and in situ thermally crosslinking of dual rigid
polymers for anti-plasticized gas separation membranes, J. Membr. Sci., 2021, 638, 119668 CrossRef CAS.
- J. Li and Y. Wang, Application of Crosslinking in Gas Separation Membranes, Sci. Adv. Mater., 2016, 8, 1155–1164 CrossRef CAS.
- C. Liu, Y. Sun, Z. Chen and S. Zhang, From ultrafiltration to nanofiltration: Nanofiltration membrane fabricated by a combined process of chemical crosslinking and thermal annealing, J. Membr. Sci., 2019, 212, 465–473 CAS.
- Y. Liu, M. Zhu, Q. Zhao, Q. An, J. Qian, K. Lee and J. Lai, The chemical crosslinking of polyelectrolyte complex colloidal particles and the pervaporation performance of their membranes, J. Membr. Sci., 2011, 385–386, 132–140 CrossRef CAS.
- A. Sarihan, S. Shahid, J. Shen, I. Amura, D. A. Patterson and E. A. C. Emanuelsson, Exploiting the electrical conductivity of poly-acid doped polyaniline membranes with enhanced durability for organic solvent nanofiltration, J. Membr. Sci., 2019, 579, 11–21 CrossRef CAS.
- X. Wang, D. Liu, J. Deng, X. Duan, J. Guo and P. Liu, Improving cyclic stability of polyaniline by thermal crosslinking as electrode material for supercapacitors, RSC Adv., 2015, 5, 78545–78552 RSC.
- K. Zhou, C. Guo, F. Gan, J. H. Xin and H. Yu, Large-area ultra-thin GO nanofiltration membranes prepared by a pre-crosslinking rod coating technique, J. Colloid Interface Sci., 2023, 640, 261–269 CrossRef CAS PubMed.
- S. Shin, Y. Park, H. Park, Y. Cho, G. Won and Y. Yoo, A facile crosslinking method for polybenzimidazole membranes toward enhanced organic solvent nanofiltration performance, Sep. Purif. Technol., 2022, 299, 121783 CrossRef CAS.
- C. V. Goethem, M. M. Magboo, M. Mertens, M. Thijs, G. Koeckelberghs and I. F. J. Vankelecom, A scalable crosslinking method for PVDF-based nanofiltration membranes for use under extreme pH conditions, J. Membr. Sci., 2020, 611, 118274 CrossRef.
- I. F. Amura, S. Shahid, A. Sarihan, J. Shen, D. A. Patterson and E. A. C. Emanuelsson, Fabrication of self-doped sulfonated polyaniline membranes with enhanced antifouling ability and improved solvent resistance, J. Membr. Sci., 2021, 620, 117712 CrossRef CAS.
- J. Huang and R. B. Kaner, Flash welding of conducting polymer nanofibres, Nat. Mater., 2004, 3, 783–786 CrossRef CAS PubMed.
- Y. Liao, D.-G. Yu, X. Wang, W. Chain, X.-G. Li, E. M. V. Hoek and R. B. Kaner, Carbon nanotube-templated polyaniline nanofibers: synthesis, flash welding and ultrafiltration membranes, Nanoscale, 2013, 5, 3856–3862 RSC.
- C. O. Baker, X. Huang, W. Nelson and R. B. Kaner, Polyaniline nanofibers: broadening applications for conducting polymers, Chem. Soc. Rev., 2017, 46, 1510–1525 RSC.
- K.-Y. Chun, Y. Oh, J. Rho, J.-H. Ahn, Y.-J. Kim, H. R. Choi and S. Baik, Highly conductive, printable and stretchable composite films of carbon nanotubes and silver, Nat. Nanotechnol., 2010, 5, 853–857 CrossRef CAS PubMed.
- Y. Liu, G. Gao and C. D. Vecitis, Prospects of an Electroactive Carbon Nanotube Membrane toward Environmental Applications, Acc. Chem. Res., 2020, 53, 2892–2902 CrossRef CAS PubMed.
- M. Lokesh, S. K. Youn and H. G. Park, Osmotic Transport across Surface Functionalized Carbon Nanotube Membrane, Nano Lett., 2018, 18, 6679–6685 CrossRef CAS PubMed.
- H. Zhang, X. Quan, S. Chen, H. Yu and J. Niu, Electrokinetic Enhancement of Water Flux and Ion Rejection through Graphene Oxide/Carbon Nanotube Membrane, Environ. Sci. Technol., 2020, 54, 15433–15441 CrossRef CAS PubMed.
- H. Zhang, L. Du, J. Xing, G. Wei and X. Quan, Electro-conductive crosslinked polyaniline/carbon nanotube nanofiltration membrane for electro-enhanced removal of bisphenol A, Front. Environ. Sci. Eng., 2023, 17, 59 CrossRef CAS.
- Y. Liao, X.-G. Li, E. M. V. Hoek and R. B. Kaner, Carbon nanotube/polyaniline nanofiber ultrafiltration membranes, J. Mater. Chem. A, 2013, 1, 15390–15396 RSC.
- L. Jiang, M. Rastgar, C. Wang, S. Ke, L. He, X. Chen, Y. Song, C. He, J. Wang and M. Sadrzadeh, Robust PANI-entangled CNTs Electro-responsive membranes for enhanced In-situ generation of H2O2 and effective separation of charged contaminants, Sep. Purif. Technol., 2022, 303, 122274 CrossRef CAS.
- N. Li, L. Liu and F. Yang, Highly conductive graphene/PANi-phytic acid modified cathodic filter membrane and its antifouling property in EMBR in neutral conditions, Desalination, 2014, 338, 10–16 CrossRef CAS.
- A. Shakeri, H. Salehi and M. Rastgar, Antifouling electrically conductive membrane for forward osmosis prepared by polyaniline/graphene nanocomposite, J. Water Process. Eng., 2019, 32, 100932 CrossRef.
- R.-X. Wang, L.-F. Huang and X.-Y. Tian, Understanding the Protonation of Polyaniline and Polyaniline-Graphene Interaction, J. Phys. Chem. C, 2012, 116, 13120–13126 CrossRef CAS.
- R. Guo, Y. Jiang, Q. Jia, H. Pei, Z. Mo, N. Liu, X. Niu and Z. Liu, Improved electrocatalytic performance from graphene quantum dots/three-dimensional graphene/polyaniline doped powder to layer-by-layer self-assembled membrane materials, Mater. Today Commun., 2020, 25, 101426 CrossRef CAS.
- A. Karkooti, M. Rastgar, N. Nazemifard and M. Sadrzadeh, Graphene-based electro-conductive anti-fouling membranes for the treatment of oil sands produced water, Sci. Total Environ., 2020, 704, 135365 CrossRef CAS PubMed.
- M. K. H. K. Ko, S. P. Yeap and A. H. A. Bakar, On shape-induced interfacial interactions in graphene/polyaniline composite produced through in situ polymerization approach, J. Taiwan Inst. Chem. Eng., 2023, 144, 104735 CrossRef.
- Y. Zhang, L. Zou, Y. Wimalasiri, J.-Y. Lee and Y. Chun, Reduced graphene oxide/polyaniline conductive anion exchange membranes in capacitive deionisation process, Electrochim. Acta, 2015, 182, 383–390 CrossRef CAS.
- L. L. Xu, Y. Xu, L. Liu, K. P. Wang, D. A. Patterson and J. Wang, Electrically responsive ultrafiltration polyaniline membrane to solve fouling under applied potential, J. Membr. Sci., 2019, 572, 442–452 CrossRef CAS.
- L. Xu, K. Wang, J. Wang and D. A. Patterson, Linking the Tuneability and Defouling of Electrically Conductive Polyaniline/Exfoliated Graphite Composite Membranes, Membrane, 2021, 11, 631 CrossRef CAS PubMed.
- Z.-K. Li, Y. Liu, L. Li, Y. Wei, J. Caro and H. Wang, Ultra-thin titanium carbide (MXene) sheet membranes for high-efficient oil/water emulsions separation, J. Membr. Sci., 2019, 592, 117361 CrossRef.
- L. Ding, L. Li, Y. Liu, Y. Wu, Z. Lu, J. Deng, Y. Wei, J. Caro and H. Wang, Effective ion sieving with Ti3C2Tx MXene membranes for production of drinking water from seawater, Nat. Sustain., 2020, 3, 296–302 CrossRef.
- N. Li, T.-J. Lou, W. Wang, M. Li, L.-C. Jing, Z.-X. Yang, R.-Y. Chang, J. Li and H.-Z. Geng, MXene-PANI/PES composite ultrafiltration membranes with conductive properties for anti-fouling and dye removal, J. Membr. Sci., 2023, 668, 121271 CrossRef CAS.
- A. VahidMohammadi, J. Moncada, H. Chen, E. Kayali, J. Orangi, C. A. Carrero and M. Beidaghi, Thick and freestanding MXene/PANI pseudocapacitive electrodes with ultrahigh specific capacitance, J. Mater. Chem. A, 2018, 6, 22123–22133 RSC.
- L. Liang, Y. Liu, C. Bu, K. Guo, W. Sun, N. Huang, T. Peng, B. Sebo, M. Pan, W. Liu, S. Guo and X.-Z. Zhao, Highly Uniform, Bifunctional Core/Double-Shell-Structured β-NaYF4:Er3+, Yb3+ @ SiO2@TiO2 Hexagonal Sub-microprisms for High-Performance Dye Sensitized Solar Cells, Adv. Mater., 2013, 25, 2174–2180 CrossRef CAS PubMed.
- Q. Huang, X. Liu, T. A. Elkhooly, R. Zhang, X. Yang, Z. Shen and Q. Feng, Preparation and characterization of TiO2/silicate hierarchical coating on titanium surface for biomedical applications, Mater. Sci. Eng., C, 2016, 60, 308–316 CrossRef CAS PubMed.
- S. Kumar, R. Bhawna, R. Sharma, A. Gupta, K. K. Dubey, A. M. Khan, R. Singhal, R. Kumar, A. Bharti, P. Singh, R. Kant and V. Kumar, TiO2 based Photocatalysis membranes: An efficient strategy for pharmaceutical mineralization, Sci. Total Environ., 2022, 845, 157221 CrossRef CAS PubMed.
- E. A. O. Farias, N. A. Dionisio, P. V. Quelemes, S. H. Leal, J. M. E. Matos, E. C. Silva Filho, I. H. Bechtold, J. R. S. A. Leite and C. Eiras, Development and characterization of multilayer films of polyaniline, titanium dioxide and CTAB for potential antimicrobial applications, Mater. Sci. Eng., C, 2014, 35, 449–454 CrossRef CAS PubMed.
- J. Shen, S. Shahid, A. Sarihan, D. A. Patterson and E. A. C. Emanuelsson, Effect of polyacid dopants on the performance of polyaniline membranes in organic solvent nanofiltration, Sep. Purif. Technol., 2018, 204, 336–344 CrossRef CAS.
- L. L. Xu, S. Shahid, D. A. Patterson and E. A. C. Emanuelsson, Flexible electro-responsive in-situ polymer acid doped polyaniline membranes for permeation enhancement and membrane fouling removal, J. Membr. Sci., 2019, 578, 263–272 CrossRef CAS.
- H. Noby, A. H. El-Shazly, M. F. Elkady and M. Ohshima, Strong acid doping for the preparation of conductive polyaniline nanoflowers, nanotubes, and nanofibers, Polymers, 2019, 182, 121848 CrossRef.
- J. Stejskal, I. Sapurina, M. Trchová and E. N. Konyushenko, Oxidation of Aniline: Polyaniline Granules, Nanotubes, and Oligoaniline Microspheres, Macromolecules, 2008, 41, 3530–3536 CrossRef CAS.
- J. Cai, B. Niu, Q. Xie, N. Lu, S. Huang, G. Zhao and J. Zhao, Accurate Removal of Toxic Organic Pollutants from Complex Water Matrices, Environ. Sci. Technol., 2022, 56, 2917–2935 CrossRef CAS PubMed.
- S. Kim, K. H. Chu, Y. A. J. Al-Hamadani, C. M. Park, M. Jang, D. H. Kim, M. Yu, J. Heo and Y. Yoon, Removal of contaminants of emerging concern by membranes in water and wastewater: A review, Chem. Eng. J., 2018, 335, 896–914 CrossRef CAS.
- M. Taheran, S. K. Brar, M. Verma, R. Y. Surampalli, T. C. Zhang and J. R. Valero, Membrane processes for removal of pharmaceutically active compounds (PhACs) from water and wastewaters, Sci. Total Environ., 2016, 547, 60–77 CrossRef CAS PubMed.
- M.-Y. Zhou, P. Zhang, L.-F. Fang, B.-K. Zhu, J.-L. Wang, J.-H. Chen and H. Abdallah, A positively charged tight UF membrane and its properties for removing trace metal cations via electrostatic repulsion mechanism, J. Hazard. Mater., 2019, 373, 168–175 CrossRef CAS PubMed.
- M. Sboui, W. Niu, D. Li, G. Lu, N. Zhou, K. Zhang and J. H. Pan, Fabrication of electrically conductive TiO2/PANI/PVDF composite membranes for simultaneous photoelectrocatalysis and microfiltration of azo dye from wastewater, Appl. Catal., A, 2022, 644, 118837 CrossRef CAS.
- X. Du, D. Zhang, X. Ma, W. Qiao, Z. Wang, X. Hao and G. Guan, Electrochemical redox induced rapid uptake/release of Pb(II) ions with high selectivity using a novel porous electroactive HZSM-5@PANI/PSS composite film, Electrochim. Acta, 2018, 282, 384–394 CrossRef CAS.
- M.-L. Liu, L. Li, Y.-X. Sun, Z.-J. Fu, X.-L. Cao and S.-P. Sun, Scalable conductive polymer membranes for ultrafast organic pollutants removal, J. Membr. Sci., 2021, 617, 118644 CrossRef CAS.
- R. Li, L. Liu and F. Yang, Removal of aqueous Hg(II) and Cr(VI) using phytic acid doped polyaniline/cellulose acetate composite membrane, J. Hazard. Mater., 2014, 280, 20–30 CrossRef CAS PubMed.
- S. Singh, S. Perween and A. Ranjan, Dramatic enhancement in adsorption of congo red dye in polymer-nanoparticle composite of polyaniline-zinc titanate, J. Environ. Chem. Eng., 2021, 9, 105149 CrossRef CAS.
- M. N. Z. Abidin, M. M. Nasef and J. Veerman, Towards the development of new generation of ion exchange membranes for reverse electrodialysis: A review, Desalination, 2022, 537, 115854 CrossRef CAS.
- J. Zhao, L. Sun, Q. Chen, H. Lu and J. Wang, Modification of cation exchange membranes with conductive polyaniline for electrodialysis applications, J. Membr. Sci., 2019, 582, 211–223 CrossRef CAS.
- H. Farrokhzad, M. R. Moghbeli, T. Van Gerven and B. Van der Bruggen, Surface modification of composite ion exchange membranes by polyaniline, React. Funct. Polym., 2015, 86, 161–167 CrossRef CAS.
- H. Farrokhzad, S. Darvishmanesh, G. Genduso, T. Van Gerven and B. Van der Bruggen, Development of bivalent cation selective ion exchange membranes by varying molecular weight of polyaniline, Electrochim. Acta, 2015, 158, 64–72 CrossRef CAS.
- P. Kumar, S. Suhag, J. R. Mandal and V. K. Shahi, Polyaniline encapsulated sulphonated graphene oxide based cation exchange membrane for electrodialytic separation of mono- and bi-valent ions, Sep. Purif. Technol., 2023, 326, 124752 CrossRef CAS.
- M. A. Shehzad, Y. Wang, A. Yasmin, X. Ge, Y. He, X. Liang, Y. Zhu, M. Hu, X. Xiao, L. Ge, C. Jiang, Z. Yang, M. D. Guiver, L. Wu and T. Xu, Biomimetic Nanocones that Enable High Ion Permselectivity, Angew. Chem., Int. Ed., 2019, 58, 12646–12654 CrossRef CAS PubMed.
- L. Xu, S. Shahid, A. K. Holda, E. A. C. Emanuelsson and D. A. Patterson, Stimuli responsive conductive polyaniline membrane: In-filtration electrical tuneability of flux and MWCO, J. Membr. Sci., 2018, 552, 153–166 CrossRef CAS.
- L. Xu, S. Liu, Z. Zheng, L. Yu, K. Li, J. Wang and J. Wang, Polyaniline-based acid resistant membranes for controllable ion rejection performance, Sep. Purif. Technol., 2023, 308, 122910 CrossRef CAS.
- X. Tong, S. Liu, Y. Zhao, L. Huang, J. Crittenden and Y. Chen, MXene Composite Membranes with Enhanced Ion Transport and Regulated Ion Selectivity, Environ. Sci. Technol., 2022, 56, 8964–8974 CrossRef CAS PubMed.
- Y. Zhao, C. Zhou, J. Wang, H. Liu, Y. Xu, J. Seo, J. Shen, C. Gao and B. Van der Bruggen, Formation of morphologically confined nanospaces via self-assembly of graphene and nanospheres for selective separation of lithium, J. Mater. Chem. A, 2018, 6, 18859–18864 RSC.
- M. Shakiba, M. Abdouss, S. Mazinani and M. R. Kalaee, Super-hydrophilic electrospun PAN nanofibrous membrane modified with alkaline treatment and ultrasonic-assisted PANI in-situ polymerization for highly efficient gravity-driven oil/water separation, Sep. Purif. Technol., 2023, 309, 123032 CrossRef CAS.
- X. Zheng, Z. Guo, D. Tian, X. Zhang and L. Jiang, Electric Field Induced Switchable Wettability to Water on the Polyaniline Membrane and Oil/Water Separation, Adv. Mater. Interfaces, 2016, 3, 1600461 CrossRef.
- K. Wang, L. Xu, K. Li, L. Liu, Y. Zhang and J. Wang, Development of polyaniline conductive membrane for electrically enhanced membrane fouling mitigation, J. Membr. Sci., 2019, 570, 371–379 CrossRef.
- Z. Yang, Y. Wu, H. Guo, X.-H. Ma, C.-E. Lin, Y. Zhou, B. Cao, B.-K. Zhu, K. Shih and C. Y. Tang, A novel thin-film nano-templated composite membrane with in situ silver nanoparticles loading: Separation performance enhancement and implications, J. Membr. Sci., 2017, 544, 351–358 CrossRef CAS.
- Y. Zhou, S. Maharubin, P. Tran, T. Reid and G. Z. Tan, Anti-biofilm AgNP-polyaniline-polysulfone composite membrane activated by low intensity direct/alternating current, Environ. Sci.: Water Res. Technol., 2018, 4, 1511–1521 RSC.
- K. G. Tay and L. Song, A more effective method for fouling characterization in a full-scale reverse osmosis process, Desalination, 2005, 177, 95–107 CrossRef CAS.
|
This journal is © The Royal Society of Chemistry 2024 |