Oxidative potential of fine particulate matter emitted from traditional and improved biomass cookstoves†
Received
10th September 2023
, Accepted 17th December 2023
First published on 18th January 2024
Abstract
Exposure to PM2.5 emitted from traditional biomass cookstoves is a significant health risk for nearly one-third of the global population. Improved cookstoves aim to reduce pollutant emissions, but there is limited evidence of whether PM2.5 toxicity is also reduced. Using the dithiothreitol (DTT) assay to measure the potential for PM2.5 chemical components to induce oxidative stress through antioxidant depletion and/or oxidant generation, we characterized the mass- and volume-normalized DTT activity of PM2.5 emitted from a traditional three-stone fire cookstove and three improved cookstoves burning wood or charcoal fuels. Although improved cookstoves typically yield lower PM2.5 mass concentrations compared to traditional three-stone cookstove, exposure to DTT active PM2.5 is not always reduced due to increases in mass-normalized DTT activity. A notable decrease in DTT active PM2.5 exposure (by 67%) was only observed for a forced-draft improved cookstove burning wood, where low PM2.5 mass concentration offsets the increased mass-normalized DTT activity. Additionally, elemental carbon and water-soluble organic matter were identified as key predictors of volume-normalized DTT activity. Compared to wood, the use of charcoal led to a 61–86% reduction in exposure to DTT active PM2.5, owing to both lower PM2.5 mass concentration and mass-normalized DTT activity. This further supports a proposed strategy whereby biomass fuel treatment can potentially reduce household exposure to toxic PM2.5. Collectively, our findings emphasized the need to consider not only the mass concentration but also the toxic properties of PM2.5 when evaluating the health impacts of cookstoves and fuels.
Environmental significance
Household PM2.5 pollution from traditional biomass cookstoves is a leading global health risk. Although improved cookstoves aim to reduce PM2.5 concentration, increased toxic potency of chemical components can offset these benefits, potentially leading to increased exposure to toxic PM2.5. In this study, we evaluated the mass concentration, chemical composition, and intrinsic toxicity of PM2.5 emitted from various cookstove/fuel combinations. We found that only certain improved cookstoves or the use of charcoal fuels can significantly reduce exposure to toxic PM2.5. We emphasize the importance of assessing the toxic properties of PM2.5 in addition to mass concentration when evaluating the impacts of cookstove design and fuel treatment on air quality and human health.
|
1 Introduction
Approximately 2.4 billion people living in low- and middle-income countries rely on the combustion of solid biomass fuels, such as wood and charcoal, for daily heating and cooking needs.1 The inefficient combustion of these fuels in traditional cookstoves emits harmful gaseous pollutants and fine particulate matter (PM2.5) that are associated with multiple adverse human health outcomes, including acute and chronic pulmonary cardiovascular effects.2 Moreover, exposure to household air pollution, including those from solid-fuel combustion, is estimated to cause 4.3 million annual pre-mature deaths worldwide.3 This health burden disproportionately affects women and children who spend more time near cooking activities and consequently have increased cookstove pollutant exposure.4
One key mitigation strategy to reduce pollutant exposure involves the development and deployment of biomass cookstoves with improved combustion efficiency and reduced pollutant emissions.5 These improved cookstove are designed to enhance thermal insulation and optimize airflow for efficient fuel combustion.6 Despite diverse improved cookstove designs and configurations (e.g., natural vs. forced draft; rocket vs. gasifier), laboratory and field studies have shown that improved cookstoves typically yield reductions in PM2.5 emission compared to traditional stoves when burning solid biomass fuels.7–17 However, a reduction in PM2.5 mass concentration may not correspond to a reduction in adverse health impacts if the intrinsic toxicity or potency (i.e., toxicity per mass) of PM2.5 is increased. Given the complexity of cookstove PM2.5, which is a mixture of chemical components at varying concentrations,15–18 each with potentially differing toxicities, it is critical to characterize the toxic properties of PM2.5 emitted from biomass cookstoves. This will allow for a comprehensive evaluation of their health impacts and potential health benefits of improved cookstove technologies.
While a growing number of studies have characterized the concentration of carcinogenic polycyclic aromatic hydrocarbons (PAH) in cookstove PM2.5,16,19–22 other toxicity metrics of PM2.5 emitted from improved biomass cookstoves remain less explored. Early studies have shown that for wood combustion, mutagenic potency,23 and inflammation levels24 were reduced for PM2.5 emitted from forced- and natural-draft improved cookstoves compared to a traditional three-stone stove. Fuel type also influences cookstove PM2.5 toxicity. In particular, cells exposed to PM2.5 emitted from improved cookstoves that used briquettes and charcoal processed from wood showed lower concentrations of an inflammation biomarker compared to PM2.5 emitted from the combustion of the unprocessed wood fuel.25 A recent study by Champion et al. reported significantly lower mutagenic potency (up to two orders of magnitude) for PM2.5 emitted from three different forced-drafted improved cookstoves burning two types of wood pellets compared to traditional stoves burning cut hardwood.26 These research efforts collectively suggest that the toxicity of cookstove PM2.5 depends on both the type of cookstove and fuel used. Further characterization of PM2.5 toxicity for additional cookstoves and fuel types is warranted, to explore the generality of previously reported toxicity and PM2.5 mass reduction for improved cookstoves.
Previous studies have established connections between cookstove PM2.5 and toxicological/biological endpoints such as mutagenicity, carcinogenicity, and inflammation. However, the current understanding of cookstove PM2.5 toxicity can be expanded by evaluating its oxidative potential (OP)—the capacity of PM to induce oxidative stress, which is a cellular condition of antioxidant–oxidant imbalance. Moreover, oxidative stress is considered a central mechanism responsible for many adverse health effects associated with PM2.5 exposure.27,28 Studies have also indicated that OP is a health relevant metric for acute PM2.5 health effects.29–31 A recent study examined the OP of PM2.5 emitted from the combustion of coal and various biomass fuels in common household cookstoves used in Northwest China, where the combustion of biomass in different stoves influenced the amount of oxidant formed by PM2.5.32 As such, characterizing the OP of PM2.5 emitted from different cookstoves burning different fuel types can further contribute to the assessment of their potential health risks.
This study aims to address the aforementioned knowledge gaps by characterizing the OP of PM2.5 emitted from the combustion of two biomass fuel types (wood and charcoal) in both traditional and improved cookstoves (a total of 12 cookstove–fuel combinations). Of particular interest are three key objectives: (1) determining whether the transition from a traditional stove to improved cookstoves leads to a reduction in exposure to toxic PM2.5; (2) examining the impact of wood and charcoal fuels on exposure to toxic PM2.5; and (3) identifying the cookstove PM2.5 chemical components that are associated with OP.
2 Methods
2.1 Burn events: biomass cookstove and fuel types
The tested cookstoves span a range of biomass burning cookstove technology (pictures of stoves and additional information are shown and described in Fig. S1 and Section S1†): a “minimally tended” traditional three-stone fire cookstove (TSF); two natural-draft rocket elbow improved cookstoves produced by EcoZoom (models: Dura and Versa); and a forced-draft gasifier improved cookstove produced by African Clean Energy (ACE; model: One). The following biomass fuel types were used for each cookstove (more information on fuel types is provided in Section S2†): split dry hardwood, charcoal lumps, and charcoal briquettes. Each stove and fuel combination were tested in triplicates (total of 36 burn events). All combustion experiments were conducted in a constant displacement exhaust hood (∼2.8 m3 min−1). Manufacture ignition instructions for the ACE cookstove include lighting two kerosene-soaked ceramic blocks (3 × 2 × 2 cm). For consistency, this ignition protocol was employed for all cookstove–fuel combinations.
The collection of PM (described below) only took place during the low power ‘simmer phase’, where water was maintained within ∼3 °C below its boiling temperature, which is inline with the temperature criteria of the standardized water boiling test protocol.33 The simmer phase simulates long cooking methods (e.g., cooking legumes) that are common throughout the world.33 PM was only collected during the simmering phase to prevent the contribution of the ignition material on the collected sample and that the long duration of this cooking period likely represents the longest exposure duration. A small aluminum pot containing 2.5 L of water was used for all experiments. Additional information regarding stove operation, such as fuel consumption, is presented in Table S1.†
2.2 PM2.5 sample collection, OC/EC, and inorganic ion analysis
The emitted PM was sampled through a PM2.5 cyclone inlet (URG, 2000-30EH) located ∼4 cm above the cookstove (but below the top of the pot to avoid sampling of water vapour) at a constant flow rate (18 slpm) controlled by a mass-flow controller (Alicat Scientific) for 30 to 120 minutes to ensure the collection of sufficient PM2.5 mass for the DTT assay. Integrated PM2.5 samples were collected via two parallel 47 mm-diameter filter holders, each containing a front and back quartz filter (prebaked Tissuquartz, Pall Laboratory). The back filter was used to account for any positive artifact due to the adsorption of semi-volatile gases from biomass combustion;34,35 all reported data were corrected for this artifact. The collected PM2.5 mass was determined gravimetrically with a microbalance (Satorius BCE1241-1S or Mettler Toledo XS105) and stored at −20 °C until analysis.
The filters were divided into portions for the determination of various chemical components. A portion of the filter (1–1.5 cm2 punch) was analyzed for organic carbon (OC) and elemental carbon (EC) using an OC/EC analyzer by Concord Analytical Services Ltd (Concord, Canada) and Sunset Laboratory (North Carolina, USA) following the IMPROVED-A thermal/optical method.36 OC was converted to organic matter (OM) using conversion factors of 1.5 and 1.2 for hardwood and charcoal, respectively.37 A separate 1.5 cm2 filter punch was used to determine water-soluble inorganic ions; the detailed ion chromatography (IC) analysis procedure is described in Section S3.† In brief, an IC system (940 Professional IC Vario, Metrohm) was used to measure the filtered sample extracts for anions (F−, Cl−, NO2−, Br−, NO3−, SO42−, and PO43−) and cations (Li+, Na+, K+, Ca2+, Mg2+, NH4+) using A Supp 5 column (150 × 4.0 mm, Metrohm) and C4 column (150 × 4.0 mm, Metrohm), respectively. Quality assurance and quality control (QA/QC) procedures for the OC, EC, and inorganic ion data are described in Section S4.†
The combined mass of OM, EC, and measured inorganic ion mass was compared to the gravimetrically determined PM2.5 mass in a mass balance analysis (Fig. S2†). The analysis indicated the presence of an unknown component in PM2.5 (i.e., not EC, OM, or measured inorganic ions), where the reconstructed mass from EC, OM, and inorganic ions represented, on average, 85% and 73% of the gravimetrically determined PM2.5 mass concentration for wood and charcoal fuels, respectively. We refer to the difference between the reconstructed OM + EC + measured inorganic mass and gravimetrically determined mass as the unknown component of PM2.5.
2.3 DTT activity
The DTT assay is a commonly used acellular technique, in part owing to its ease of operation and rapid measurements, to quantify the potential of PM2.5 components to induce oxidative stress by monitoring the rate by which DTT decays due to the presence of reactive PM2.5 components.38 DTT activities of the water-soluble (WS) and total (i.e., water-soluble and -insoluble) components were measured using a modified protocol outlined in Wong et al.,39 and Gao et al.;40,41 the detailed DTT assay procedure is described in Section S5.† Briefly, the WS-DTT activity represents the sample extract that was filtered (0.45 μm PTFE syringe filter, VWR) to remove insoluble material, while the total-DTT activity represents the unfiltered aliquot, with the PM2.5 filter punch remaining in solution throughout the reaction with DTT. We note that Gao et al. have compared the performance of various methods to characterize total-DTT activity and determined the approach taken by the current study (unfiltered aliquot with the PM2.5 filter punch remaining in solution) to be the most effective and precise method.41 The decay rate of DTT due to reaction with PM2.5 components in pH 7.4 phosphate buffer and at 37 °C was determined by monitoring the concentration of unreacted DTT using UV-Vis absorption spectroscopy. The WS- and total-DTT activities for the back filter were also determined and accounted for; this correction was, on average, 17% of the uncorrected DTT activities. Using the corrected WS- and total-DTT activities and following the procedure of Fang et al.,42 the corrected DTT activities were normalized by the volume of air, to represent the volume-normalized WS- and total-DTT activity of PM2.5 (OPWS-DTTvol and OPTotal-DTTvol; nmol min−1 m−3). The difference between OPWS-DTTvol and OPTotal-DTTvol represents volume-normalized water-insoluble (WI) DTT activity (OPWI-DTTvol).41,43–45 We note that this approach assumes WS- and WI-DTT activities are additive and does not account for potential (synergistic or antagonistic) interactions between the WS and WI components, which remain unknown and warrant future investigation. For intrinsic (potency) DTT activity, the corrected total-DTT activities were normalized by the PM2.5 mass on the filter punch (OPTotal-DTTmass, pmol min−1 μg−1). We did not determine the mass-normalized WS- or WI-DTT activities as the mass concentrations of WS and WI PM2.5 components were not determined.
2.4 Data analysis
Analysis was conducted from different perspectives to examine the complex interactions between cookstove design and fuel types46 on PM2.5 DTT activity: comparisons of different cookstoves for the same fuel type (e.g., wood combustion in traditional vs. improved cookstoves) and different fuel types for the same cookstove type (e.g., wood vs. charcoal combustion in a traditional stove). Following the approach of Champion et al.,18 two-way Wilcoxon rank-sum tests were used in evaluating statistical significance of comparisons. This statistical test was selected due to the limited number of burn events per stove/fuel combination and non-normal distribution of some datasets as indicated by Shapiro–Wilk test. Statistical significance was defined as p ≤ 0.05.
To identify the component(s) that influence mass-normalized OP, Spearman rank correlations between OPTotal-DTTmass and the mass fraction of measured chemical components were conducted. Since the mass concentrations of WS and WI components were not characterized, partial least squares regression (PLSR) models were developed to assess the influence of measured chemical components on OPWS-DTTvol and OPWI-DTTvol. Additionally, univariate correlations (e.g., Spearman or Pearson correlations) of volume-normalized DTT activity are susceptible to multicollinearity effects as strong correlations of PM2.5 mass concentrations to chemical components can make assessments of individual chemical component's contribution to volume-normalized DTT challenging.47,48 PLSR can account for multicollinearity effects49 and it is well suited for the identification of which predictors (chemical components) are best associated with the response (DTT activity). A OPTotal-DTTvol PLSR model was also developed to compare with the Spearman correlation analysis.
To build the PLSR models, the input data were scaled and mean-normalized to remove effects related to differences in data magnitude. A 10-fold cross-validation approach was used, and the optimal number of components (latent variables) was selected when the root mean squared error of prediction (RMSEP) was minimized. The number of components selected for each PLSR model and evaluation of model robustness using various metrics are described in Section S6 and Table S2.† PLSR models were developed using R (version 4.3.1) implemented in R Studio (+524), using packages pls and plsVarSel.50
3 Results and discussion
All figures presented in the main text are pooled by broad fuel category (i.e., wood and charcoal), with results for each stove/fuel combination shown in Section S7 (Fig. S3, S4, and S5).†
3.1 PM2.5 mass concentration
Out of all cookstove–fuel combinations tested, the PM2.5 mass concentration (Fig. 1a) was the highest for TSF burning wood at 41.8 ± 25.6 mg m−3 (mean ± SD). For wood combustion, improved cookstoves led to reduced mean PM2.5 compared to TSF: by 27–41% for natural-draft stoves (Dura and Versa) and 89% for forced-draft stove (ACE). The reduction in PM2.5 for improved cookstoves can be attributed to more complete fuel combustion as a result of enhanced airflow and heat loss reduction.51 ACE demonstrated a greater reduction than the two natural draft cookstoves due to the ability of forced-draft cookstoves to maintain a constant air flow, thereby enhancing combustion efficiency and combustion temperature which in turn results in reduced pollution emissions.52,53 While only the reduction in PM2.5 for ACE was statistically significant compared to TSF, the percentage reductions for improved cookstoves are consistent with real-world measurements. For example, a transition from traditional biomass cookstoves to Dura led to 46.4–51.1% reduction in household/personal PM2.5 emission54,55 and transition to ACE resulted in a ∼25% reduction.15
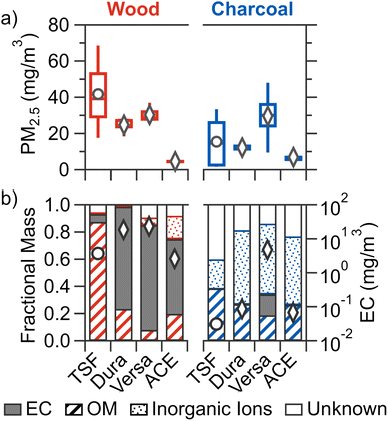 |
| Fig. 1 For wood (red) and charcoal (blue) fuel combustion using each stove type: (a) box and whisker plots of PM2.5 mass concentration. The horizontal line within the box indicates the median value; the dark grey circle and diamond markers are the means for traditional and improved cookstoves, respectively; the lower and upper box boundaries represent the 25th and 75th percentiles; and the whiskers indicate the 5th and 95th percentiles. (b) Mean fractional mass contribution of OM (striped bar), EC (dark grey bar), inorganic ions (dotted bars), and unknown component (colourless bar). Note that while the mass contribution of EC cannot be easily seen in this figure for charcoal combustion, the mean EC mass concentrations (circle and diamond markers for traditional and improved cookstoves, respectively) are also shown to highlight the low, but non-zero concentrations. | |
For charcoal fuel combustion, improved cookstoves did not lead to statistically significant reductions in mean PM2.5 compared to TSF, as TSF exhibited substantial variability. This variability is potentially due to the non-uniform charcoal fuel structure that results in variable airflow in the less controlled fire of a traditional stove.12 However, significant differences were observed among all improved stoves burning charcoal, with ACE demonstrating a lower mean PM2.5 by 43–77% compared to natural draft improved stoves. The results for both wood and charcoal combustion align with previous research, where improved cookstoves generally emit less PM2.5 compared to TSF, and amongst improved cookstoves, forced-draft stove emit the lowest PM2.5.12–14,16,17
Not all stoves resulted in significantly different PM2.5 from the change in fuel type. Mean PM2.5 for Versa was similar for wood and charcoal. While mean PM2.5 from charcoal combustion increased by 53% for ACE and reduced by 65% for TSF, these changes were not statistically significant. Only Dura exhibited a significant 51% reduction for charcoal fuel compared to wood. Of the limited number of previous studies that compared charcoal and wood combustion using the same stove, lower PM2.5 emissions for charcoal fuels by 7–39% were observed for a traditional stove,56 19–83% for a clean (semi-gasifier) stove,25 and ∼36% for a forced-draft gasifier stove.17 This reduction in PM2.5 from charcoal combustion is attributed to lower volatile content of charcoal fuels,16,25,56 as volatile matter is removed during the carbonization process in charcoal production.56 Collectively, results from previous work and the current study suggest that compared to wood fuels, charcoal combustion generally results in lower PM2.5. However, the magnitude of this reduction varies significantly due to differences in stove technologies, which highlights the complex interaction of fuel and stove on PM2.5 emission.46
3.2 PM2.5 chemical composition
Variations in the chemical composition of PM2.5 were observed across the different stove–fuel combinations tested (Fig. 1b). For all tested stove–fuel combinations, TSF burning wood resulted in PM2.5 with the highest mean OM mass fraction (0.86 ± 0.13), with some contribution from EC (0.07 ± 0.05) and unknown component (0.06 ± 0.06), as well as negligible contribution from inorganic ions (0.01 ± 0.01). This is consistent with previous laboratory and field studies of traditional stoves burning wood fuels, where the organic carbon constitutes 0.40–0.94 of the total carbon mass of PM2.5.14–17,57
For wood fuel combustion, compared to TSF, all improved cookstoves showed significantly higher mean fractional contributions by EC, ranging from 0.56 to 0.78, which aligns with previous studies.14–17 The higher contribution by EC is due to the higher combustion temperatures in insulated improved cookstoves favours EC formation, whereas the lower combustion temperature in a non-insulated traditional stove favour OM formation.58
For charcoal combustion, the combined mass fraction of EC and OM to PM2.5 ranges from 0.26–0.38 across all cookstoves. In particular, with the exception of Versa burning charcoal briquettes, low elemental carbon-to-total carbon ratios (EC
:
TC ratios are shown in Fig. S3f†) ranging from 0.01 to 0.07 indicated the carbon is predominately organic with comparatively low EC, consistent with previous studies of charcoal combustion.16,17,57 No significant differences in fractional contribution of EC, OM, and non-carbon were observed across most cookstoves except for lower contribution of inorganic ions for TSF and higher EC contribution for Versa, which is further discussed in Section S7.†
Amongst each cookstove burning different fuel types, wood fuel resulted in significantly higher fractional contributions of EC compared to charcoal fuels for all cookstoves except for Versa. The lower contribution of EC (and higher OM) by charcoal fuels can be attributed to two reasons: (1) lower EC emissions for charcoal fuels as combustion occurs primarily in heterogeneous matter (i.e., the surface of charcoal), in contrast to the gas-phase combustion of wood fuels that can result in PM-forming products;16,57 and (2) higher moisture content for wood fuel, which can result in lower combustion efficiency that favours OM formation in gas-phase combustion.58,59 In addition to the low EC observed with charcoal combustion, all stoves resulted in PM2.5 that was predominately non-carbon (combined inorganic ion and unknown fraction ranges from 0.64–0.75), whereas wood combustion resulted in PM2.5 that was mostly carbonaceous (combined inorganic ion and unknown fraction ranging from 0.07 to 0.28). For charcoal combustion, with exception to TSF, the non-carbon fraction is dominated by inorganic ions (ranging from 0.50 to 0.55) of which potassium and phosphate are typically the main inorganic ions (Fig. S5†). Previous studies have also reported reduced contribution of carbonaceous material to PM2.5 and greater inorganic ion emissions for charcoal combustion compared to wood.16,56,60 The unknown fraction can be composed of metals such as aluminum, copper, and iron that have been detected in cookstove PM2.5.56 Both the current study and existing literature suggest that a significant portion of PM2.5 from charcoal fuel combustion may not be carbonaceous.
3.3 Mass-normalized total-DTT activity
The mass-normalized total-DTT activity (OPTotal-DTTmass; i.e., total-DTT activity per mass of PM2.5) was characterized to examine whether different stove–fuel combinations resulted in PM2.5 of varying intrinsic toxicities. Shown in Fig. 2a, for all cookstove–fuel combinations tested, mean values of OPTotal-DTTmass ranged from (0.2 to 1.4) × 102 pmol min−1 μg−1. These are comparable to biomass burning PM [(0.17–1.50) × 102 pmol min−1 μg−1]61–63 and to PM2.5 measured in the kitchens of rural households in the Tibetan plateau that predominately use biomass fuels for cooking [(7.92–8.89) × 101 pmol min−1 μg−1] as reported by Brehmer et al.64
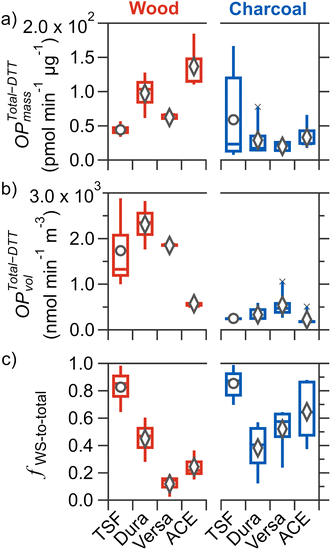 |
| Fig. 2 Box and whisker plots of (a) mass-normalized total-DTT activity (OPTotal-DTTmass), (b) air volume-normalized total-DTT activity (OPTotal-DTTvol), and (c) fraction of water-soluble (WS) to total volume-normalized DTT activity for wood (red) and charcoal (blue) fuel combustion in each stove type. The horizontal line within the box indicates median values; the lower and upper box boundaries represent the 25th and 75th percentiles; the whiskers indicate the 5th and 95th percentiles, the dark grey circle and diamond markers indicates the mean for traditional cookstove and improved cookstoves, respectively. The grey cross markers indicate outliers. | |
For wood fuel, the mean OPTotal-DTTmass for improved cookstoves increased by 40–210% [(0.6–1.4) × 102 pmol min−1 μg−1] relative to that of TSF (4.4 × 101 pmol min−1 μg−1). However, only OPTotal-DTTmass for ACE and Dura was statistically higher than that of TSF, indicating that an equivalent exposure of PM2.5 mass from these two improved stoves will result in a faster decay of a model antioxidant (DTT) compared to TSF. Mutlu et al. previously reported that PM2.5 from a forced-draft cookstove burning wood was the most mutagenic on a per mass basis compared to emissions from a three-stone and a natural-draft cookstove.23 Among improved cookstoves, differences in intrinsic toxicity were observed, with ACE having the highest mean OPTotal-DTTmass [(1.4 ± 0.4) × 102 pmol min−1 μg−1], followed by Dura [(9.8 ± 3.4) × 101 pmol min−1 μg−1], then Versa [(6.2 ± 1.0) × 101 pmol min−1 μg−1]; however only the differences between ACE and Versa are statistically significant. In contrast to wood fuel, a narrow range of OPTotal-DTTmass [(2.0–5.9) × 101 pmol min−1 μg−1] was observed for charcoal fuel combustion in all tested cookstoves, and no statistically significant differences were found between any two cookstoves burning charcoal, despite statistically different PM2.5 mass among improved cookstoves. These contrasting effects further highlight the role of cookstove technology and fuel type in the intrinsic toxicity for PM2.5 emitted.
Considering each cookstove burning different fuel types, no statistically significant differences were observed for TSF and Dura, whereas the use of charcoal fuel led to a statistically significant reduction of OPTotal-DTTmass by 68–76% for Versa and ACE. Notably, for Versa, the use of charcoal fuel compared to wood fuel yielded no reduction in PM2.5 yet a 68% decrease in OPTotal-DTTmass. In contrast, for ACE, there was a 53% increase in PM2.5 but a 76% decrease in OPTotal-DTTmass. These contrasting effects further indicate that changes in PM2.5 mass do not necessarily correlate with the intrinsic toxicity of PM2.5. In fact, these two metrics (PM2.5 and OPTotal-DTTmass), when used independently, do not accurately represent the exposure to toxic components in PM2.5, as exposure to higher PM2.5 does not necessarily indicate exposure to a greater mass of toxic PM2.5 components. Indeed, to evaluate whether changes in the type of cookstove and fuel used will lead to a reduction in toxic PM2.5 exposure, both PM2.5 mass concentration and intrinsic toxicity need to be considered.
3.4 Volume-normalized DTT activity
To evaluate the potential adverse health risks associated with exposure to toxic chemicals in PM2.5 emitted from biomass cookstoves, the total-DTT activity was normalized by the volume of air sampled (OPTotal-DTTvol; nmol min−1 m−3). This metric better represents the overall exposure to toxic PM2.5 compared to OPTotal-DTTmass because in addition to OPTotal-DTTmass, it also considers PM2.5 mass concentration in the air. The OPTotal-DTTvol of PM2.5 emitted by different cookstoves burning wood and charcoal fuels are shown on Fig. 2b. Here, the OPTotal-DTTvol represents contribution from both water-soluble and -insoluble components.
The mean OPTotal-DTTvol for all tested cookstoves burning wood and charcoal fuels ranges from (0.2–2.3) × 103 nmol min−1 m−3. These OPTotal-DTTvol values are remarkably high compared to typical literature OPTotal-DTTvol values. Only one other study has reported high OPTotal-DTTvol values of (0.089–3.500) × 103 nmol min−1 m−3 for PM2.5 collected close to garbage burning piles (∼0.5 m above) in urban India.65 In contrast, for the aforementioned study by Brehmer et al., OPTotal-DTTvol for PM2.5 collected over a 48 hours period in the kitchens of rural households in the Tibetan plateau that cook with biomass span (0.86–1.10) × 101 nmol min−1 m−3.64 The much lower OPTotal-DTTvol values reported by Brehmer et al. can be attributable to indoor dilution and that cooking activities were unlikely to occur throughout the entire 48 hours sampling period. Given that OPTotal-DTTmass values of the current study are comparable to those reported by Brehmer et al., the higher OPTotal-DTTvol values are a result of sampling relatively undiluted PM2.5 emissions. Hence, the OPTotal-DTTvol values reported in this study represent the worst-case exposure scenario for a person who remains close to the cookstove when cooking (i.e., when tending to the stove). Nonetheless, we note that comparisons of OPTotal-DTTvol values under identical sampling conditions provide insights into the impacts of cookstove technology and fuel type.
Considering wood fuel alone, mean OPTotal-DTTvol for both natural-draft stoves (Dura and Versa) were 6–33% higher than that of TSF [(1.7 ± 1.0) × 103 nmol min−1 m−3], but not statistically so. The forced-draft stove (ACE) exhibited the lowest OPTotal-DTTvol [(5.7 ± 1.2) × 102 nmol min−1 m−3], which was a statistically significant reduction of 67–75% from the three other stoves. Despite PM2.5 from ACE-wood being the most DTT active per mass, the OPTotal-DTTvol was the lowest among cookstoves burning wood burning, which is owed to the low PM2.5 mass emitted. These results indicate that not all improved cookstoves burning wood result in reduced exposure to DTT active PM2.5 compared to TSF. For charcoal fuels, Versa had the highest mean OPTotal-DTTvol [(5.4 ± 2.9) × 102 nmol min−1 m−3] which was 60–140% greater than that of other tested stoves; however, it was only statistically different from ACE and TSF [(2.2 ± 1.4) × 102 and (2.5 ± 0.5) × 102 nmol min−1 m−3], respectively.
The most prominent result was that for each cookstove, the combustion of charcoal led to significant reductions of 61–86% for OPTotal-DTTvol compared to wood. The reduction of OPTotal-DTTvol for each cookstove due to the use of charcoal instead of wood is consistent with the study by Niu et al., where for PM2.5 emitted from a clean cookstove, combustion of charcoal fuel led to a reduction in cell injury by 10–20% and cellular inflammatory response by 62.7% compared to the use of (unprocessed) wood fuel.25 Moreover, the results from the current study extend this reduction effect from the use of charcoal fuel instead of wood for other cookstoves (including the traditional three-stone stove), except for the use of ACE-wood to Dura-charcoal or Versa-charcoal, where the reductions were not significant. Since charcoal is a processed form of wood, results from the current study and those by Niu et al. suggest that the chemical components in wood that are responsible for the higher OPTotal-DTTvol are removed or converted to a less DTT active form during the production of charcoal.
3.5 WS and WI volume-normalized DTT activity
The contribution of WS fraction to total-DTT activity offers valuable insights into the nature of toxic components capable of reacting with DTT. In particular, the difference in OPWS-DTTvol to OPTotal-DTTvol represents the contribution of WI components to OPTotal-DTTvol. There is growing evidence that insoluble material in PM2.5 contributes to oxidative stress and different PM2.5 sources have varying proportions of WS and WI components contributing to overall toxicity.40,66–68
The fractional contribution of OPWS-DTTvol to OPTotal-DTTvol (Fig. 2c; hereafter referred to as fws-to-Total) varied tremendously for the tested stove–fuel combinations, with mean values ranging from 0.12 to 0.85. Traditional stove burning wood or charcoal fuels both resulted in significant contributions of WS fraction (0.85 ± 0.13), which is statistically greater compared to most improved cookstoves, suggesting the impacts of cookstove technology and fuel type on the variable contribution of WS and WI components to total-DTT activity for cookstove PM2.5. Detailed comparison of fws-to-Total across cookstove and fuel type is provided in Section S7.†
3.6 Association of OPDTT with PM2.5 chemical components
To investigate differences in the contribution of chemical components to OPDTT, univariate linear regression (Spearman rank correlation coefficient, rs) was initially used to examine correlations of OPTotal-DTTmass to the mass fraction of EC, OM, and inorganic ions for all cookstove/fuel combinations (see Table S3†). OPTotal-DTTmass was best correlated with the mass fraction of EC (rs: 0.62, p < 0.01), whereas OPTotal-DTTmass had weak correlations with the mass fraction of OM (rs: −0.20, p > 0.05), and all measured inorganic ions (−0.30 ≤ rs ≤ 0.19, p > 0.05). The positive moderate correlation between the mass fraction of EC and OPTotal-DTTmass is in line with previous studies of household biomass combustion, in which EC is correlated with mutagencity,26 inflammatory response,25 and environmental persistent free radicals.69 The lack of a positive correlation between the mass fraction of OM to OPTotal-DTTmass may be attributed to different intrinsic OPDTT between WSOM and WIOM,70 where aggregate measures of OM and total-DTT activity (both metrics include WSOM and WIOM) may mask the variable contribution of WSOM and WIOM to OPTotal-DTTmass.
PLSR models were developed to assess the importance of measured chemical components (EC, OM, and inorganic ions) on OPTotal-DTTvol, OPWS-DTTvol, and OPWI-DTTvol. The three models demonstrated good explanatory power; each explaining 89%, 68%, and 87% of the variation in OPDTTvol, respectively (see Table S2† for a summary of model performance assessments and related discussion in Section S6†). Table 1 shows the variable importance in the projection (VIP) scores and the regression coefficients for key predictors whose VIP scores are greater than one (see Table S4† for all predictors). The VIP is a measure to rank the relative contributions of each predictor in the model. A VIP score greater than one indicates that the predictor significantly contributes to the model predictive ability. The sign of the regression coefficient indicates the direction of influence of each predictor on OPDTTvol.71 For the OPTotal-DTTvol PLSR model, EC emerged as the most important predictor, consistent with the Spearman rank correlation analysis. In particular, EC is not only an important predictor in the OPWI-DTTvol model but also has larger regression coefficient in this model compared to the OPWS-DTTvol model. This aligns with the inherent insolubility of EC. Though EC is inherently insoluble, the enhanced importance of EC as a key predictor in OPWI-DTTvol model compared to the OPWS-DTTvol model could arise from DTT-active insoluble components associated with EC. For example, it has been previously suggested that soot, which can be detected as EC in OC/EC analysis,72,73 can have water-insoluble DTT active species on its surface.66
Table 1 Key predictors (i.e., VIP scores greater than one) and their corresponding VIP score and regression coefficient in PLSR models of OPDTTvol
OPTotal-DTTvol |
OPWS-DTTvol |
OPWI-DTTvol |
Predictor |
VIP |
Coefficient |
Predictor |
VIP |
Coefficient |
Predictor |
VIP |
Coefficient |
EC |
2.25 |
+0.72 |
OM |
2.51 |
+0.64 |
EC |
2.69 |
+0.88 |
OM |
1.33 |
+0.52 |
PO43− |
1.15 |
−0.17 |
PO43− |
1.01 |
+0.03 |
PO43− |
1.20 |
−0.07 |
NO2− |
1.10 |
+0.02 |
Mg2+ |
1.00 |
−0.06 |
NO2− |
1.10 |
−0.05 |
NO3− |
1.04 |
+0.03 |
|
|
|
Ca2+ |
1.02 |
−0.12 |
EC |
1.03 |
+0.26 |
|
|
|
NO3− |
1.01 |
+0.14 |
|
|
|
|
|
|
OM is the most significant predictor of OPWS-DTTvol, with OM increasing with OPWS-DTTvol as indicated by the positive regression coefficient coupled with the observation that OM was not a significant predictor for OPWI-DTTvol, suggest that DTT active OM are predominately water-soluble. Strong positive correlations of water-soluble organic carbon to DTT have been reported previously for biomass burning.74–76 DTT active WS humic-like substances (HULIS) are abundant in biomass burning PM62 and can accounted for 50 ± 7% of OPWS-DTTvol for PM2.5 generated from the combustion of five different biomass fuels.77 We note that certain organic compounds, which may be present in the water extract of cookstove PM2.5 and have been identified in solid fuel combustion PM2.5,63,78 could have contributed to the observed WS-DTT activity. Specifically, compounds such as quinones and oxygenated PAHs are known to be DTT active,79,80 and nitro-PAHs have been postulated to exhibit DTT activity.81,82 Future studies to investigate the contribution of these oxygenated and nitro-PAHs on the DTT activity of cookstove PM2.5 are warranted. In fact, a deeper understanding of the molecular composition of WSOM emitted by different fuel types and cookstoves can shed light on the specific organic compounds that drive the observed OPDTT.
Although OM was the most significant predictor for OPWS-DTTvol, its reduced importance in predicting OPTotal-DTTvol can be attributed to OM being a combined measure of WSOM and WIOM. This is further supported by the relatively low VIP score (0.47) with a positive regression coefficient (+0.14) for OM in the OPWI-DTTvol PLSR model, suggesting that the WIOM fraction may have considerably lower DTT activity compared to WSOM. As a result, the lower DTT activity of WIOM would reduce the influence that DTT-active WSOM has on OM in the OPTotal-DTTvol PLSR model. Therefore, the results from this study further emphasize that sample preparation approaches that separate PM2.5 component based on solubility provide important insights into the relative contribution of various toxic WSOM components in PM2.5 that might be otherwise overlooked.41,62
Notably, certain inorganic ions, such as PO43−, NO2−, NO3−, Ca2+, and Mg2+, which are not known to directly react with DTT, emerged as significant predictors of OPDTTvol. These inorganic ions may be co-emitted with species influencing DTT activity. The varying signs of their regression coefficients indicated associations with both increased and reduced DTT activities. However, compared to dominant predictors such as EC, the coefficients of these inorganic ions are smaller in magnitude, suggesting that they play a lesser role in DTT activity of cookstove PM2.5.
While PLSR models can predict 68–89% of the variation in OPDTTvol using directly measured chemical components, the contributions of DTT-active transition metals are not considered in these models. For example, DTT-active metals copper and manganese,79 may be present in the unknown component and have been identified in biomass burning PM2.5 in minute fractions (typically <1% of PM2.5 mass).83,84 As such, they may have contributed to the measured DTT activities in this study. To gain additional insights, more comprehensive chemical characterization of cookstove PM2.5, coupled with OPDTT measurements, is warranted. This will enable the evaluation of the impact of cookstove and fuel type on the emission of DTT-active transition metals.
4 Implications
The results of this study, along with previous research, have shown that various improved cookstove designs can result in lower PM2.5 mass concentrations and change the chemical composition of the PM2.5 compared to traditional cookstoves. For an improved forced-draft cookstove, the decrease in PM2.5 mass concentration effectively offsets the higher OPTotal-DTTmass DTT activity compared to a traditional stove, leading to a net reduction in the exposure to DTT active PM2.5. However, improved natural-draft cookstoves resulted in slightly lower or similar PM2.5 mass concentrations compared to a traditional stove, but the increase in OPTotal-DTTmass resulted in a similar or even greater exposure to DTT active PM2.5. As such, reduced PM2.5 emissions of improved cookstoves do not necessarily equate to reduced toxic PM2.5 exposure. Although this study focused on the OPDTT of primary PM2.5 emitted from biomass cookstoves, oxidation of gaseous emissions from wood fuel combustion in a traditional three-stone cookstove, improved natural- and forced-drafted cookstoves can give rise to secondary organic aerosol formation.85,86 Over time, as these secondary organic aerosol form and emissions disperse throughout the household, their contribution may become more significant, potentially leading to differences in exposure levels between the kitchen and the rest of the household. The health impacts of this secondary organic aerosol remain unknown and should be examined.
This study also suggests that the switch to charcoal fuels from wood fuel (without the switch in cookstove technology) can potentially lead to a reduction of 61–86% in OPTotal-DTTvol, which further supports recommendations by previous studies to consider the use of processed fuel as an alternative strategy to reduce cookstove emissions.18,25,87 However, several caveats associated with the use of charcoal should be considered. Compared to wood, charcoal combustion produces higher carbon monoxide concentrations;12,16,17 given the documented health impacts associated with carbon monoxide,2 this represents a pollutant trade-off for the decreased exposure to DTT active PM2.5. Additionally, the production of charcoal emits a significant amount of PM2.5, resulting in greater emissions than wood over their lifecycle, thus the use of charcoal fuel instead of wood represents a trade-off between exposure and climate impacts associated with biomass cookstoves.5,88
Furthermore, the results from this study suggest that insoluble EC and WSOM are the DTT active components of PM2.5 emitted from the tested cookstove–fuel combinations. Therefore, variations in the emissions of these DTT active components, which are likely driven by differences in cookstove technology and fuel properties, can significantly influence the overall toxic impacts of biomass cookstove PM2.5. From this perspective, reductions in PM2.5 will not necessarily lead to reduced toxicity if the reduction in mass concentration is driven by lower emissions of non-toxic components. As such, this study emphasizes the need to consider not only reductions in PM2.5 emissions, but also the changes in chemical composition and toxicity of PM2.5 when evaluating the potential health impacts of biomass cookstoves.
Data availability
Experimental data and codes (in R language) used to develop the PLSR models are available on request.
Author contributions
Conceptualization, JPSW; methodology, JPSW, MMF, and BHI; investigation, BHI, JPD, SAW, and JPSW; writing – original draft, BHI and JPSW; writing – review and editing, BHI and JPSW; funding acquisition, JPSW; supervision, JPSW. All authors have given approval to the final version of the manuscript.
Conflicts of interest
There are no conflicts to declare.
Acknowledgements
Funding for this work was provided by Natural Sciences and Engineering Research Council of Canada (NSERC Discovery Grant, RGPIN-2020-06890), Canadian Innovation Foundation (John R. Evans Leaders Funds, Project 41318), New Brunswick Innovation Foundation (NBIF) Talent Recruitment Fund, Mount Allison University President Creative Activity Fund and Start-Up Fund. BHI was supported in part by Marjorie Young Bell Endowment Independent Student Research Grant (Mount Allison University). We thank Concord Analytical Services Ltd and Sunset Laboratories Inc. for carrying out the OC/EC analysis, and Bill Cameron for assistance with wood fuel preparation.
References
-
IEA, IRENA, UNSD, World Bank and WHO, Tracking SDG 7: the Energy Progress Report, World Bank, Washington DC, 2023 Search PubMed.
- R. Pratiti, D. Vadala, Z. Kalynych and P. Sud, Health effects of household air pollution related to biomass cook stoves in resource limited countries and its mitigation by improved cookstoves, Environ. Res., 2020, 186, 109574 CrossRef CAS PubMed.
-
World Health Organization, Burning Opportunity: Clean Household Energy for Health, Sustainable Development, and Wellbeing of Women and Children, World Health Organization, 2016 Search PubMed.
- N. Bruce, D. Pope, E. Rehfuess, K. Balakrishnan, H. Adair-Rohani and C. Dora, WHO indoor air quality guidelines on household fuel combustion: Strategy implications of new evidence on interventions and exposure–risk functions, Atmos. Environ., 2015, 106, 451–457 CrossRef CAS.
- A. P. Grieshop, J. D. Marshall and M. Kandlikar, Health and climate benefits of cookstove replacement options, Energy Policy, 2011, 39, 7530–7542 CrossRef CAS.
- M. P. Kshirsagar and V. R. Kalamkar, A comprehensive review on biomass cookstoves and a systematic approach for modern cookstove design, Renewable Sustainable Energy Rev., 2014, 30, 580–603 CrossRef.
- C. V. Preble, O. L. Hadley, A. J. Gadgil and T. W. Kirchstetter, Emissions and Climate-Relevant Optical Properties of Pollutants Emitted from a Three-Stone Fire and the Berkeley-Darfur Stove Tested under Laboratory Conditions, Environ. Sci. Technol., 2014, 48, 6484–6491 Search PubMed.
- V. H. Rapp, J. J. Caubel, D. L. Wilson and A. J. Gadgil, Reducing Ultrafine Particle Emissions Using Air Injection in Wood-Burning Cookstoves, Environ. Sci. Technol., 2016, 50, 8368–8374 CrossRef CAS PubMed.
- A. P. Grieshop, G. Jain, K. Sethuraman and J. D. Marshall, Emission factors of health- and climate-relevant pollutants measured in home during a carbon-finance-approved cookstove intervention in rural India, GeoHealth, 2017, 1, 222–236 CrossRef PubMed.
- G. Shen, C. K. Gaddam, S. M. Ebersviller, R. L. Vander Wal, C. Williams, J. W. Faircloth, J. J. Jetter and M. D. Hays, A Laboratory Comparison of Emission Factors, Number Size Distributions, and Morphology of Ultrafine Particles from 11 Different Household Cookstove-Fuel Systems, Environ. Sci. Technol., 2017, 51, 6522–6532 CrossRef CAS PubMed.
- S. Rose Eilenberg, K. R. Bilsback, M. Johnson, J. K. Kodros, E. M. Lipsky, A. Naluwagga, K. M. Fedak, M. Benka-Coker, B. Reynolds, J. Peel, M. Clark, M. Shan, S. Sambandam, C. L'Orange, J. R. Pierce, R. Subramanian, J. Volckens and A. L. Robinson, Field measurements of solid-fuel cookstove emissions from uncontrolled cooking in China, Honduras, Uganda, and India, Atmos. Environ., 2018, 190, 116–125 Search PubMed.
- J. Jetter, Y. Zhao, K. R. Smith, B. Khan, T. Yelverton, P. DeCarlo and M. D. Hays, Pollutant Emissions and Energy Efficiency under Controlled Conditions for Household Biomass Cookstoves and Implications for Metrics Useful in Setting International Test Standards, Environ. Sci. Technol., 2012, 46, 10827–10834 CrossRef CAS PubMed.
- B. Just, S. Rogak and M. Kandlikar, Characterization of Ultrafine Particulate Matter from Traditional and Improved Biomass Cookstoves, Environ. Sci. Technol., 2013, 47, 3506–3512 CrossRef CAS PubMed.
- C. A. Roden, T. C. Bond, S. Conway, A. B. Osorto Pinel, N. MacCarty and D. Still, Laboratory and field investigations of particulate and carbon monoxide emissions from traditional and improved cookstoves, Atmos. Environ., 2009, 43, 1170–1181 CrossRef CAS.
- R. Wathore, K. Mortimer and A. P. Grieshop, In-Use Emissions and Estimated Impacts of Traditional, Natural- and Forced-Draft Cookstoves in Rural Malawi, Environ. Sci. Technol., 2017, 51, 1929–1938 Search PubMed.
- K. R. Bilsback, J. Dahlke, K. M. Fedak, N. Good, A. Hecobian, P. Herckes, C. L'Orange, J. Mehaffy, A. Sullivan, J. Tryner, L. Van Zyl, E. S. Walker, Y. Zhou, J. R. Pierce, A. Wilson, J. L. Peel and J. Volckens, A Laboratory Assessment of 120 Air Pollutant Emissions from Biomass and Fossil Fuel Cookstoves, Environ. Sci. Technol., 2019, 53, 7114–7125 CrossRef CAS PubMed.
- E. R. Coffey, D. Muvandimwe, Y. Hagar, C. Wiedinmyer, E. Kanyomse, R. Piedrahita, K. L. Dickinson, A. Oduro and M. P. Hannigan, New Emission Factors and Efficiencies from in-Field Measurements of Traditional and Improved Cookstoves and Their Potential Implications, Environ. Sci. Technol., 2017, 51, 12508–12517 CrossRef CAS PubMed.
- W. M. Champion, M. D. Hays, C. Williams, L. Virtaranta, M. Barnes, W. Preston and J. J. Jetter, Cookstove Emissions and Performance Evaluation Using a New ISO Protocol and Comparison of Results with Previous Test Protocols, Environ. Sci. Technol., 2021, 55, 15333–15342 CrossRef CAS PubMed.
- S. Gupta, S. Saksena, V. R. Shankar and V. Joshi, Emission factors and thermal efficiencies of cooking biofuels from five countries, Biomass Bioenergy, 1998, 14, 547–559 CrossRef CAS.
- N. T. Kim Oanh, D. O. Albina, L. Ping and X. Wang, Emission of particulate matter and polycyclic aromatic hydrocarbons from select cookstove–fuel systems in Asia, Biomass Bioenergy, 2005, 28, 579–590 Search PubMed.
- G. Shen, W. Wang, Y. Yang, J. Ding, M. Xue, Y. Min, C. Zhu, H. Shen, W. Li, B. Wang, R. Wang, X. Wang, S. Tao and A. G. Russell, Emissions of PAHs from Indoor Crop Residue Burning in a Typical Rural Stove: Emission Factors, Size Distributions, and Gas–Particle Partitioning, Environ. Sci. Technol., 2011, 45, 1206–1212 CrossRef CAS PubMed.
- A. Leavey, S. Patel, R. Martinez, D. Mitroo, C. Fortenberry, M. Walker, B. Williams and P. Biswas, Organic and inorganic speciation of particulate matter formed during different combustion phases in an improved cookstove, Environ. Res., 2017, 158, 33–42 Search PubMed.
- E. Mutlu, S. H. Warren, S. M. Ebersviller, I. M. Kooter, J. E. Schmid, J. A. Dye, W. P. Linak, M. I. Gilmour, J. J. Jetter, M. Higuchi and D. M. DeMarini, Mutagenicity and Pollutant Emission Factors of Solid-Fuel Cookstoves: Comparison with Other Combustion Sources, Environ. Health Perspect., 2016, 124, 974–982 CrossRef CAS PubMed.
- B. Hawley and J. Volckens, Proinflammatory effects of cookstove emissions on human bronchial epithelial cells, Indoor Air, 2013, 23, 4–13 Search PubMed.
- X. Niu, X. Liu, B. Zhang, Q. Zhang, H. Xu, H. Zhang, J. Sun, K.-F. Ho, H.-C. Chuang, Z. Shen and J. Cao, Health benefits from substituting raw biomass fuels for charcoal and briquette fuels: In vitro toxicity analysis, Sci. Total Environ., 2023, 866, 161332 CrossRef CAS PubMed.
- W. M. Champion, S. H. Warren, I. M. Kooter, W. Preston, Q. T. Krantz, D. M. DeMarini and J. J. Jetter, Mutagenicity- and pollutant-emission factors of pellet-fueled gasifier cookstoves: Comparison with other combustion sources, Sci. Total Environ., 2020, 739, 139488 CrossRef CAS PubMed.
- N. Li, M. Hao, R. F. Phalen, W. C. Hinds and A. E. Nel, Particulate air pollutants and asthma. A paradigm for the role of oxidative stress in PM-induced adverse health effects, Clin. Immunol., 2003, 109, 250–265 Search PubMed.
- A. Valavanidis, K. Fiotakis and T. Vlachogianni, Airborne particulate matter and human health: toxicological assessment and importance of size and composition of particles for oxidative damage and carcinogenic mechanisms, J. Environ. Sci. Health,
Part C: Environ. Carcinog. Ecotoxicol. Rev., 2008, 26, 339–362 Search PubMed.
- S. Weichenthal, E. Lavigne, G. Evans, K. Pollitt and R. T. Burnett, Ambient PM2.5 and risk of emergency room visits for myocardial infarction: impact of regional PM2.5 oxidative potential: a case-crossover study, Environ. Health, 2016, 15, 46 CrossRef PubMed.
- J. Y. Abrams, R. J. Weber, M. Klein, S. E. Samat, H. H. Chang, M. J. Strickland, V. Verma, T. Fang, J. T. Bates, J. A. Mulholland, A. G. Russell and P. E. Tolbert, Associations between Ambient Fine Particulate Oxidative Potential and Cardiorespiratory Emergency Department Visits, Environ. Health Perspect., 2017, 125, 107008 CrossRef PubMed.
- J. Korsiak, E. Lavigne, H. You, K. Pollitt, R. Kulka, M. Hatzopoulou, G. Evans, R. T. Burnett and S. Weichenthal, Air Pollution and Pediatric Respiratory Hospitalizations: Effect Modification by Particle Constituents and Oxidative Potential, Am. J. Respir. Crit. Care Med., 2022, 206, 1370–1378 CrossRef CAS PubMed.
- S. Huang, Y. Luo, X. Wang, T. Zhang, Y. Lei, Y. Zeng, J. Sun, H. Che, H. Xu, J. Cao and Z. Shen, Optical properties, chemical functional group, and oxidative activity of different polarity levels of water-soluble organic matter in PM2.5 from biomass and coal combustion in rural areas in Northwest China, Atmos. Environ., 2022, 283, 119179 CrossRef CAS.
-
The Water Boiling Test Version 4.2.3: Cookstove Emissions and Efficiency in a Controlled Laboratory Setting, Clean Cooking Alliance, 2014 Search PubMed.
- R. Subramanian, A. Y. Khlystov, J. C. Cabada and A. L. Robinson, Positive and Negative Artifacts in Particulate Organic Carbon Measurements with Denuded and Undenuded Sampler Configurations Special Issue of Aerosol Science and Technology on Findings from the Fine Particulate Matter Supersites Program, Aerosol Sci. Technol., 2004, 38, 27–48 CrossRef CAS.
- E. M. Lipsky and A. L. Robinson, Effects of Dilution on Fine Particle Mass and Partitioning of Semivolatile Organics in Diesel Exhaust and Wood Smoke, Environ. Sci. Technol., 2006, 40, 155–162 CrossRef CAS PubMed.
- J. C. Chow, J. G. Watson, L.-W. A. Chen, M. C. O. Chang, N. F. Robinson, D. Trimble and S. Kohl, The IMPROVE_A Temperature Protocol for Thermal/Optical Carbon Analysis: Maintaining Consistency with a Long-Term Database, J. Air Waste Manage. Assoc., 2007, 57, 1014–1023 Search PubMed.
- J. C. Chow, D. H. Lowenthal, L.-W. A. Chen, X. Wang and J. G. Watson, Mass reconstruction methods for PM2.5: a review, Air Qual., Atmos. Health, 2015, 8, 243–263 CrossRef CAS PubMed.
- A. K. Cho, E. D. Stefano, Y. You, C. E. Rodriguez, D. A. Schmitz, Y. Kumagai, A. H. Miguel, A. Eiguren-Fernandez, T. Kobayashi, E. Avol and J. R. Froines, Determination of Four Quinones in Diesel Exhaust Particles, SRM 1649a, and Atmospheric PM2.5 Special Issue of Aerosol Science and Technology on Findings from the Fine Particulate Matter Supersites Program, Aerosol Sci. Technol., 2004, 38, 68–81 CrossRef CAS.
- J. P. S. Wong, M. Tsagkaraki, I. Tsiodra, N. Mihalopoulos, K. Violaki, M. Kanakidou, J. Sciare, A. Nenes and R. J. Weber, Effects of Atmospheric Processing on the Oxidative Potential of Biomass Burning Organic Aerosols, Environ. Sci. Technol., 2019, 53, 6747–6756 CrossRef CAS PubMed.
- D. Gao, J. A. Mulholland, A. G. Russell and R. J. Weber, Characterization of water-insoluble oxidative potential of PM2.5 using the dithiothreitol assay, Atmos. Environ., 2020, 224, 117327 CrossRef CAS.
- D. Gao, T. Fang, V. Verma, L. Zeng and R. J. Weber, A method for measuring total aerosol oxidative potential (OP) with the dithiothreitol (DTT) assay and comparisons between an urban and roadside site of water-soluble and total OP, Atmos. Meas. Tech., 2017, 10, 2821–2835 CrossRef CAS.
- T. Fang, V. Verma, H. Guo, L. E. King, E. S. Edgerton and R. J. Weber, A semi-automated system for quantifying the oxidative potential of ambient particles in aqueous extracts using the dithiothreitol (DTT) assay: results from the Southeastern Center for Air Pollution and Epidemiology (SCAPE), Atmos. Meas. Tech., 2015, 8, 471–482 CrossRef CAS.
- D. Gao, K. J. Godri Pollitt, J. A. Mulholland, A. G. Russell and R. J. Weber, Characterization and comparison of PM2.5 oxidative potential assessed by two acellular assays, Atmos. Chem. Phys., 2020, 20, 5197–5210 CrossRef CAS.
- T. Fang, L. Zeng, D. Gao, V. Verma, A. B. Stefaniak and R. J. Weber, Ambient Size Distributions and Lung Deposition of Aerosol Dithiothreitol-Measured Oxidative Potential: Contrast between Soluble and Insoluble Particles, Environ. Sci. Technol., 2017, 51, 6802–6811 CrossRef CAS PubMed.
- M. A. Frezzini, G. Di Iulio, C. Tiraboschi, S. Canepari and L. Massimi, A New Method for the Assessment of the Oxidative Potential of Both Water-Soluble and Insoluble PM, Atmos, 2022, 13, 349 CrossRef CAS.
- H. Shen, Z. Luo, R. Xiong, X. Liu, L. Zhang, Y. Li, W. Du, Y. Chen, H. Cheng, G. Shen and S. Tao, A critical review of pollutant emission factors from fuel combustion in home stoves, Environ. Int., 2021, 157, 106841 CrossRef CAS PubMed.
- J. V. Puthussery, A. Singh, P. Rai, D. Bhattu, V. Kumar, P. Vats, M. Furger, N. Rastogi, J. G. Slowik, D. Ganguly, A. S. H. Prevot, S. N. Tripathi and V. Verma, Real-Time Measurements of PM2.5 Oxidative Potential Using a Dithiothreitol Assay in Delhi, India, Environ. Sci. Technol. Lett., 2020, 7, 504–510 CrossRef CAS.
- S. J. Campbell, K. Wolfer, B. Utinger, J. Westwood, Z.-H. Zhang, N. Bukowiecki, S. S. Steimer, T. V. Vu, J. Xu, N. Straw, S. Thomson, A. Elzein, Y. Sun, D. Liu, L. Li, P. Fu, A. C. Lewis, R. M. Harrison, W. J. Bloss, M. Loh, M. R. Miller, Z. Shi and M. Kalberer, Atmospheric conditions and composition that influence PM2.5 oxidative potential in Beijing, China, Atmos. Chem. Phys., 2021, 21, 5549–5573 CrossRef CAS PubMed.
- S. Wold, M. Sjöström and L. Eriksson, PLS-regression: a basic tool of chemometrics, Chemom. Intell. Lab. Syst., 2001, 58, 109–130 CrossRef CAS.
- T. Mehmood, K. H. Liland, L. Snipen and S. Sæbø, A review of variable selection methods in Partial Least Squares Regression, Chemom. Intell. Lab. Syst., 2012, 118, 62–69 CrossRef CAS.
-
M. Bryden, D. Still, P. Scott, G. Hoffa, D. Ogle, R. Balis and K. Goyer, Design Principles for Wood Burning Cook Stoves, Aprovecho Research Centre, 2005 Search PubMed.
-
Handbook for Biomass Cookstove Research, Design, and Development: A Practical Guide to Implementing Recent Advances, Global Clean Cooking Alliance, Washington, DC, 2021 Search PubMed.
- M. Kumar, S. Kumar and S. K. Tyagi, Design, development and technological advancement in the biomass cookstoves: A review, Renewable Sustainable Energy Rev., 2013, 26, 265–285 Search PubMed.
- G. Rosa, F. Majorin, S. Boisson, C. Barstow, M. Johnson, M. Kirby, F. Ngabo, E. Thomas and T. Clasen, Assessing the Impact of Water Filters and Improved Cook Stoves on Drinking Water Quality and Household Air Pollution: A Randomised Controlled Trial in Rwanda, PLoS One, 2014, 9, e91011 CrossRef PubMed.
- M. A. Kirby, C. L. Nagel, G. Rosa, L. D. Zambrano, S. Musafiri, J. de D. Ngirabega, E. A. Thomas and T. Clasen, Effects of a large-scale distribution of water filters and natural draft rocket-style cookstoves on diarrhea and acute respiratory infection: A cluster-randomized controlled trial in Western Province, Rwanda, PLoS Med., 2019, 16, e1002812 CrossRef PubMed.
- Q. Li, J. Qi, J. Jiang, J. Wu, L. Duan, S. Wang and J. Hao, Significant reduction in air pollutant emissions from household cooking stoves by replacing raw solid fuels with their carbonized products, Sci. Total Environ., 2019, 650, 653–660 Search PubMed.
- W. M. Champion and A. P. Grieshop, Pellet-Fed Gasifier Stoves Approach Gas-Stove Like Performance during in-Home Use in Rwanda, Environ. Sci. Technol., 2019, 53, 6570–6579 CrossRef CAS PubMed.
- G. Shen, M. Xue, S. Wei, Y. Chen, B. Wang, R. Wang, Y. Lv, H. Shen, W. Li, Y. Zhang, Y. Huang, H. Chen, W. Wei, Q. Zhao, B. Li, H. Wu and S. TAO, The Influence of Fuel Moisture, Charge Size, Burning Rate and Air Ventilation Conditions on Emissions of PM, OC, EC, Parent PAHs, and Their Derivatives from Residential Wood Combustion, J. Environ. Sci., 2013, 25, 1808–1816 CrossRef CAS PubMed.
- L. van Zyl, J. Tryner, K. R. Bilsback, N. Good, A. Hecobian, A. Sullivan, Y. Zhou, J. L. Peel and J. Volckens, Effects of Fuel Moisture Content on Emissions from a Rocket-Elbow Cookstove, Environ. Sci. Technol., 2019, 53, 4648–4656 Search PubMed.
- J. Sun, Z. Shen, Y. Zhang, Q. Zhang, Y. Lei, Y. Huang, X. Niu, H. Xu, J. Cao, S. S. H. Ho and X. Li, Characterization of PM2.5 source profiles from typical biomass burning of maize straw, wheat straw, wood branch, and their processed products (briquette and charcoal) in China, Atmos. Environ., 2019, 205, 36–45 CrossRef CAS.
- A. Patel, R. Satish and N. Rastogi, Remarkably High Oxidative Potential of Atmospheric PM2.5 Coming from a Large-Scale Paddy-Residue Burning over the Northwestern Indo-Gangetic Plain, ACS Earth Space Chem., 2021, 5, 2442–2452 CrossRef CAS.
- V. Verma, T. Fang, L. Xu, R. E. Peltier, A. G. Russell, N. L. Ng and R. J. Weber, Organic Aerosols Associated with the Generation of Reactive Oxygen Species (ROS) by Water-Soluble PM2.5, Environ. Sci. Technol., 2015, 49, 4646–4656 Search PubMed.
- W. Y. Tuet, F. Liu, N. de Oliveira Alves, S. Fok, P. Artaxo, P. Vasconcellos, J. A. Champion and N. L. Ng, Chemical Oxidative Potential and Cellular Oxidative Stress from Open Biomass Burning Aerosol, Environ. Sci. Technol. Lett., 2019, 6, 126–132 Search PubMed.
- C. Brehmer, A. Lai, S. Clark, M. Shan, K. Ni, M. Ezzati, X. Yang, J. Baumgartner, J. J. Schauer and E. Carter, The Oxidative Potential of Personal and Household PM2.5 in a Rural Setting in Southwestern China, Environ. Sci. Technol., 2019, 53, 2788–2798 Search PubMed.
- H. Vreeland, J. J. Schauer, A. G. Russell, J. D. Marshall, A. Fushimi, G. Jain, K. Sethuraman, V. Verma, S. N. Tripathi and M. H. Bergin, Chemical characterization and toxicity of particulate matter emissions from roadside trash combustion in urban India, Atmos. Environ., 2016, 147, 22–30 CrossRef CAS.
- R. D. McWhinney, K. Badali, J. Liggio, S.-M. Li and J. P. D. Abbatt, Filterable Redox Cycling Activity: A Comparison between Diesel Exhaust Particles and Secondary Organic Aerosol Constituents, Environ. Sci. Technol., 2013, 47, 3362–3369 CrossRef CAS PubMed.
- A. M. Knaapen, T. Shi, P. J. A. Borm and R. P. F. Schins, Soluble metals as well as the insoluble particle fraction are involved in cellular DNA damage induced by particulate matter, Mol. Cell. Biochem., 2002, 234, 317–326 CrossRef PubMed.
- S. Yi, F. Zhang, F. Qu and W. Ding, Water-insoluble fraction of airborne particulate matter (PM10) induces oxidative stress in human lung epithelial A549 cells, Environ. Toxicol., 2014, 29, 226–233 CrossRef CAS PubMed.
- J. Zhao, G. Shen, L. Shi, H. Li, D. Lang, L. Zhang, B. Pan and S. Tao, Real-World Emission Characteristics of Environmentally Persistent Free Radicals in PM2.5 from Residential Solid Fuel Combustion, Environ. Sci. Technol., 2022, 56, 3997–4004 CrossRef CAS PubMed.
- T. Cao, M. Li, C. Zou, X. Fan, J. Song, W. Jia, C. Yu, Z. Yu and P. Peng, Chemical composition, optical properties, and oxidative potential of water- and methanol-soluble organic compounds emitted from the combustion of biomass materials and coal, Atmos. Chem. Phys., 2021, 21, 13187–13205 CrossRef CAS.
- I.-G. Chong and C.-H. Jun, Performance of some variable selection methods when multicollinearity is present, Chemom. Intell. Lab. Syst., 2005, 78, 103–112 CrossRef CAS.
- J. C. Corbin, H. Czech, D. Massabò, F. B. de Mongeot, G. Jakobi, F. Liu, P. Lobo, C. Mennucci, A. A. Mensah, J. Orasche, S. M. Pieber, A. S. H. Prévôt, B. Stengel, L.-L. Tay, M. Zanatta, R. Zimmermann, I. El Haddad and M. Gysel, Infrared-absorbing carbonaceous tar can dominate light absorption by marine-engine exhaust, npj Clim. Atmos. Sci., 2019, 2, 1–10 CrossRef CAS.
- R. E. Pileci, R. L. Modini, M. Bertò, J. Yuan, J. C. Corbin, A. Marinoni, B. Henzing, M. M. Moerman, J. P. Putaud, G. Spindler, B. Wehner, T. Müller, T. Tuch, A. Trentini, M. Zanatta, U. Baltensperger and M. Gysel-Beer, Comparison of co-located refractory black carbon (rBC) and elemental carbon (EC) mass concentration measurements during field campaigns at several European sites, Atmos. Meas. Tech., 2021, 14, 1379–1403 Search PubMed.
- V. Verma, A. Polidori, J. J. Schauer, M. M. Shafer, F. R. Cassee and C. Sioutas, Physicochemical and toxicological profiles of particulate matter in Los Angeles during the October 2007 southern California wildfires, Environ. Sci. Technol., 2009, 43, 954–960 CrossRef CAS PubMed.
- M. Hakimzadeh, E. Soleimanian, A. Mousavi, A. Borgini, C. De Marco, A. A. Ruprecht and C. Sioutas, The impact of biomass burning on the oxidative potential of PM2.5 in the metropolitan area of Milan, Atmos. Environ., 2020, 224, 117328 CrossRef CAS.
- V. Verma, T. Fang, H. Guo, L. King, J. T. Bates, R. E. Peltier, E. Edgerton, A. G. Russell and R. J. Weber, Reactive oxygen species associated with water-soluble PM2.5 in the southeastern United States: spatiotemporal trends and source apportionment, Atmos. Chem. Phys., 2014, 14, 12915–12930 CrossRef.
- X. Fan, M. Li, T. Cao, C. Cheng, F. Li, Y. Xie, S. Wei, J. Song and P. Peng, Optical properties and oxidative potential of water- and alkaline-soluble brown carbon in smoke particles emitted from laboratory simulated biomass burning, Atmos. Environ., 2018, 194, 48–57 Search PubMed.
- J. Wang, W. Du, Y. Chen, Y. Lei, L. Chen, G. Shen, B. Pan and S. Tao, Nitrated and oxygenated polycyclic aromatic hydrocarbons emissions from solid fuel combustion in rural China: Database of 12 real-world scenarios for residential cooking and heating activities, Sci. Total Environ., 2022, 852, 158501 CrossRef CAS PubMed.
- J. G. Charrier and C. Anastasio, On dithiothreitol (DTT) as a measure of oxidative potential for ambient particles: evidence for the importance of soluble transition metals, Atmos. Chem. Phys., 2012, 12, 9321–9333 Search PubMed.
- R. D. McWhinney, S. Zhou and J. P. D. Abbatt, Naphthalene SOA: redox activity and naphthoquinone gas–particle partitioning, Atmos. Chem. Phys., 2013, 13, 9731–9744 CrossRef.
- L. Ntziachristos, J. R. Froines, A. K. Cho and C. Sioutas, Relationship between redox activity and chemical speciation of size-fractionated particulate matter, Part. Fibre Toxicol., 2007, 4, 5 CrossRef PubMed.
- W. Y. Tuet, Y. Chen, L. Xu, S. Fok, D. Gao, R. J. Weber and N. L. Ng, Chemical oxidative potential of secondary organic aerosol (SOA) generated from the photooxidation of biogenic and anthropogenic volatile organic compounds, Atmos. Chem. Phys., 2017, 17, 839–853 CrossRef CAS.
- J. J. Schauer, M. J. Kleeman, G. R. Cass and B. R. T. Simoneit, Measurement of Emissions from Air Pollution Sources. 3. C1–C29 Organic Compounds from Fireplace Combustion of Wood, Environ. Sci. Technol., 2001, 35, 1716–1728 CrossRef CAS PubMed.
- I. Seo, K. Lee, M.-S. Bae, M. Park, S. Maskey, A. Seo, L. J. S. Borlaza, E. M. R. Cosep and K. Park, Comparison of physical and chemical characteristics and oxidative potential of fine particles emitted from rice straw and pine stem burning, Environ. Pollut., 2020, 267, 115599 Search PubMed.
- S. M. Reece, A. Sinha and A. P. Grieshop, Primary and Photochemically Aged Aerosol Emissions from Biomass Cookstoves: Chemical and Physical Characterization, Environ. Sci. Technol., 2017, 51, 9379–9390 Search PubMed.
- A. Sinha, M. M. Islam and A. Grieshop, Influence of Stove, Fuel, and Oxidation Flow Reactor Conditions on Aging of Laboratory-Generated Cookstove Emissions, ACS Earth Space Chem., 2021, 5, 1575–1590 CrossRef CAS.
- G. Zhi, C. Peng, Y. Chen, D. Liu, G. Sheng and J. Fu, Deployment of Coal Briquettes and Improved Stoves: Possibly an Option for both Environment and Climate, Environ. Sci. Technol., 2009, 43, 5586–5591 CrossRef CAS PubMed.
-
Comparative Analysis of Fuels for Cooking: Life Cycle Environmental Impacts and Economic and Social Considerations, Global Alliance for Clean Cookstoves, 2017 Search PubMed.
|
This journal is © The Royal Society of Chemistry 2024 |