DOI:
10.1039/D3NA00327B
(Review Article)
Nanoscale Adv., 2023,
5, 5426-5434
Functional nucleic acids for the treatment of diabetic complications
Received
14th May 2023
, Accepted 26th August 2023
First published on 28th August 2023
Abstract
In recent decades, diabetes mellitus (DM) has become a major global health problem owing to its high prevalence and increased incidence of diabetes-associated complications, including diabetic wounds (DWs), diabetic nephropathy, metabolic syndrome, diabetic retinopathy, and diabetic neuropathy. In both type 1 and type 2 diabetes, tissue damage is organ-specific, but closely related to the overproduction of reactive oxygen species (ROS) and hyperglycaemia-induced macrovascular system damage. However, existing therapies have limited effects on complete healing of diabetic complications. Fortunately, recent advances in functional nucleic acid materials have provided new opportunities for the treatment and diagnosis of diabetic complications. Functional nucleic acids possess independent structural functions that can replace traditional proteases and antibodies and perform specific biological non-genetic functions. This review summarises the current functional nucleic acid materials reported for the treatment of diabetic complications, including tetrahedral framework nucleic acids (tFNAs), short interfering RNA (siRNA), micorRNA (miRNA), locked nucleic acids, antisense oligonucleotides (ASOs), and DNA origami, which may assist in the development of novel nucleic acids with new functions and capabilities for better healing of diabetic complications.
Introduction
Diabetes mellitus (DM) is the most common metabolic disorder, and more than 475 million people worldwide are reported to suffer from DM globally.1,2 DM seriously endangers the quality of life and health and is characterised by glucose intolerance and insulin resistance. Based on its pathogenesis, diabetes is divided into type I (T1DM; insulin deficiency) and type II (T2DM; insulin resistance). The prevalence of diabetes continues to increase annually due to unhealthy eating habits and sedentary lifestyles.
It is widely known that DM has adverse effects on multiple organs, including the skin, kidney, eye, and nerves. Sustained hyperglycaemia causes generalised vascular damage that can lead to metabolic disturbance and diabetic complications, which cause more patient deaths than diabetes itself.3,4 According to the statistics of the prevalence of these complications in patients with diabetes, diabetic wounds (DW) accounted for 42%,5 diabetic nephropathy 21.8%,6 metabolic syndrome 20–25%,7 diabetic retinopathy 3.4–12.3% (ref. 8) and diabetic neuropathy 23%.9 Despite diagnostic tools and the continuing treatment improvement, diabetic complications still exert a great burden on patients' health and the economy. Traditional general systemic and local treatments focus on insulin secretion and insulin sensitisation which cause unwanted side effects to patients and lead to incompliance as well as treatment failure, while other treatments such as gene therapy and induced β-cell regeneration have not been widely introduced to manage diabetes.10 Recent new treatment targets and novel functional nucleic acid materials bring about new hope to DM and its complications. Functional nucleic acid is a general term for nucleic acids and nucleic acid analogue molecules, including unconventional types of nucleic acids, DNAzymes, aptamers, DNA tiles, and DNA origami, and the research has developed rapidly in recent years.11
In this review, we briefly describe the pathogenesis of and existing therapies for DM complications. We then elucidate novel functional nucleic acids as new treatment targets that have been reported to exert their actions, such as improving cellular oxidative tolerance, proliferation, migration, anti-apoptosis and anti-inflammation.12 Moreover, we emphasize the potential therapeutic and preventive action, as well as the diagnostic role of different functional nucleic acids in diabetic complications. Finally, we discuss current challenges and promising directions for improvement in this field. This overview of nucleic acid-assisted therapeutic materials for diabetic complications provides readers and fellow researchers with practical solutions and applications for combating diabetic complications.
Functional nucleic acid materials and diabetic foot ulcers (DFUs)
DFUs are the most common type of DW which can occur anywhere in the body and present as open ulcers. Sustained hyperglycaemia and the release of reactive oxygen species (ROS) lead to anoxia, neuropathy, impaired angiogenesis, and cellular damage, which then progress into generalised vascular and neurological lesions.13,14 Since DFUs are susceptible to repeated bacterial infection, they easily develop into ulcers, cellulitis, and deep abscesses, resulting in amputation.15 The traditional treatments of DFUs encompass the application of antibiotics, vasodilators, neurotrophic drugs, hyperbaric oxygen, and surgical debridement.16 However, sustained high morbidity and disability rates indicate that, in most cases, general systemic or local treatments cannot effectively control the development. Therefore, there is an urgent need to explore novel therapeutic strategies.
Recently, research on the biomedical applications of materials has been developing rapidly, and several nucleic acid materials have been found to be remarkably effective in tissue rejuvenation (Fig. 1).17–19 Tetrahedral framework nucleic acids (tFNAs), a simple three-dimensional structure, possess long-term stability and biocompatibility.20 In a hyperglycaemic environment, excessive glucose and protein synthesise advanced glycation end products (AGEs) which accumulate in the body, leading to ROS generation and advancement of the illness. Lin et al. discovered that tFNAs can activate the protein kinase B (Akt)/nuclear factor erythroid 2-related factor 2 (Nrf2)/haeme oxygenase-1 (HO-1) signalling pathway in type I diabetic animal models.12 Akt is an important downstream kinase of vascular endothelial growth factor (VEGF) signalling, whose activation leads to upregulated gene expression of VEGF and neovascularization.21 While AGE pretreatment lowers Akt phosphorylation, the subsequent tFNA treatment upregulates Akt phosphorylation. Akt phosphorylates endothelial nitric oxide synthase (enos) and subsequently regulates NO release. NO can elevate the level of Nrf2 signalling and a battery of antioxidative proteins, such as haeme oxygenase-1 HO-1, which protect cells from oxidative stress. Research has also revealed that tFNAs can improve the proliferation and migratory ability of endothelial cells which are attenuated by AGEs.12 Therefore, tFNAs can promote human diabetic wound healing by accelerating epithelialisation, vascularisation, collagen deposition, and collagen alignment, and enhancing oxidation resistance.
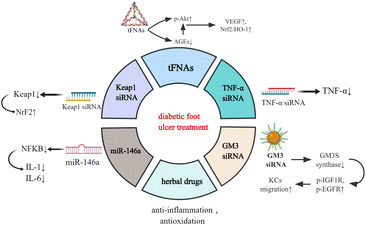 |
| Fig. 1 The role of functional nucleic acids in diabetic foot ulcer treatment. | |
Some traditional Chinese herbal medicines, such as Aloe vera,22 curcumin23 and acheflan,24 have also been reported to possess anti-inflammatory and antioxidant effects, which have therapeutic potential in diabetic wounds.25 Nevertheless, most of them are rarely used owing to their lability, poor water solubility, short biological half-life, and poor systemic bioavailability. It has been reported that Cur-tFNAs have a significant therapeutic effect on acute gouty arthritis.26 Thus, the tFNA-based delivery system may be an excellent approach for treating DW with herbal drugs.
Studies have revealed that the Nrf2/Kelch-like erythroid cell-derived protein 1 (Keap1) pathway, an important pathway associated with wound healing, is disrupted in DW owing to ROS accumulation. Diabetic skin has low Nrf2 activation and subsequently has low antioxidant capacity, resulting in delayed wound healing.27 Using nucleic acid nanomaterial short interfering RNA (siRNA) can decrease the level of Keap1, activate the expression of NrF2 and enhance cellular oxidation resistance capacity, which contributes to wound healing.28 Tumour necrosis factor (TNF)-α siRNA helps in DW healing by similar mechanisms. The inflammatory factor TNF-α is beneficial to fibroblast migration, proliferation, and remodelling at a low concentration, whereas its overproduction in DM deteriorates DFUs by promoting cellular apoptosis, ROS, and matrix degradation. Thus, TNF-α siRNA is used against TNF-α to alleviate DW.29 Another functional nucleic acid material is miR-146a, which can protect DW skin from the overexpression of proinflammatory cytokines such as interleukin (IL)-6 and IL-8 by knocking down the nuclear factor kappa B (NFKB).30
Moreover, GM3 synthase (GM3S), a known target that contributes to insulin resistance and impedes wound healing, is overexpressed in diabetic foot skin.31 Furthermore, siRNA-based GM3S spherical nucleic acids (SNAs) effectively and specifically downregulate the expression of GM3S mRNA in cultured keratinocytes (KCs) as well as intact and wounded skin in type 2 diabetic mice by activating both antisense and RNAi pathways and proteins. This treatment promotes KC migration into the wound bed, increases Insulin-like growth factor (IGF1)R and epidermal growth factor (EGFR) phosphorylation, and in turn accelerates the growth of granulation tissue and blood vessels and foot ulcer closures in DM mice.32
Functional nucleic acid materials and diabetic nephropathy (DNep)
As one of the leading causes of renal failure, DNep increasingly occurs in patients with diabetes, which is characterised by persistent albuminuria (>300 mg day−1 or >200 μg min−1), progressive decrease in the glomerular filtration rate and progressive high arterial blood pressure.33,34 Although its complex pathogenesis has not been well studied so far, scientists have found that oxidative stress, angiotensin II (Ang-II), and inflammatory processes are closely associated with the disease's development.35 Moreover, there are some functional nucleic acid materials that are effective for the treatment of Dnep by maintaining podocyte homeostasis and associated renal functions, and ameliorating fibrosis in the remnant kidney (Fig. 2).36,37
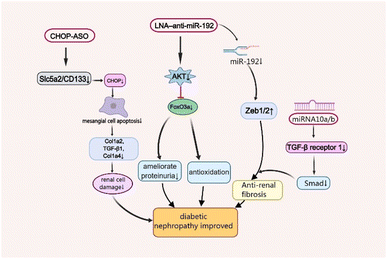 |
| Fig. 2 Promoting effects of various functional nucleic acids in diabetic nephropathy. | |
A master target of DNep, transforming growth factor (TGF)-β1 not only promotes cell growth and extracellular matrix (ECM) production, but also increases collagen expression by upregulating microRNA-192 (miR-192) and other renal miRNAs in kidney cells in culture. The specific inhibition of miR-192 increases the E-box repressors Zeb1/2 which can reduce renal fibrosis and ameliorate proteinuria.38 Locked nucleic acids (LNA)1 are a kind of DNA analogs which possess high affinity towards complementary DNA and RNA, and LNA-modified antisense oligonucleotides (ASOs) are widely used in the field of siRNA and miRNA therapeutics.39 The LNA–anti-miR-192 efficiently suppresses the expression of endogenous miR-192, specifically in both the renal cortex and glomeruli in the Type 1 diabetes mouse model by dramatically enhancing the levels of Zeb1/Zeb2 (targets of miR-192). Therefore, LNA–anti-miR-192 treatment improves the symptoms of proteinuria and albuminuria in the DNep group. Moreover, LNA–anti-miR-192 also exerts protective effects against renal hypertrophy and oxidative stress by inhibiting the Akt/FoxO3a signalling pathway to treat DNep.40
The nucleic acid nanomaterial miRNA-10a/b also targets TGF-β/Smad signalling in DNep.41 Mechanistically, miR-10a/b played an anti-fibrotic role by binding to 3′UTR of TGFBR1 mRNA. It suppresses renal fibrosis by targeting TGF-β receptor 1, a vital protein in TGF-β/Smad signalling which is upregulated in kidneys from patients with DNep and DNep mouse models.42 Manipulation of miR-10a/b mimic in podocytes or tubular cells decreased the expression of TGFBR1 protein, TGF-β/Smad signalling activation and downstream profibrotic gene expression including fibronectin43 and α-smooth muscle actin (α-SMA).41
The C/EBP homologous protein (CHOP), a marker of endoplasmic reticulum stress, also plays a vital role in DNep progression, which significantly increases in the DM mouse model.44 As a cell-death-promoting transcription factor, CHOP sharply reduces the expression of collagen-related factors Col1a2, TGF-β1 and Col1a4 by inducing mesangial cell apoptosis, leading to kidney damage.45 Recently, Shahzad et al. revealed that CHOP-ASOs can effectively decrease glucose-induced CHOP in type 2 DM mice and downregulate the top genes sodium-glucose transport protein 2 (Slc5a2) and PROM1 (CD133), associated with tubular dedifferentiation after acute renal injury.46 This showed that CHOP-ASOs remained effective in human renal cells and inhibited glucose-induced cell damage.
Functional nucleic acid materials and diabetic neuropathy
Diabetic neuropathy is one of the most common diabetic complications and is associated with hyperglycemia, metabolic disorders, microvascular injury, and inflammation.47–49 Diabetic neuropathy mainly manifests as distal symmetric dysesthesia, motor nerve lesion, and autonomic neuropathy, which includes the gastrointestinal, urogenital, and cardiovascular autonomic nervous systems.50–52 The typical symptom of diabetic neuropathy is neuropathic pain which principally results in burning pain and numbness of the lower extremities. The occurrence of pain is involved in dorsal root ganglia (DRG) dysfunction. Currently, the drugs used in diabetic neuropathy have various side-effects and lack tissue specificity. Therefore, it is necessary to explore novel therapies that release drugs regionally to ensure that they can play a specific role.
Nucleic acid nanomaterials have been found to play a vital role in the treatment of diabetic neuropathy, and presently, they are mainly used in RNA nanotechnology to relieve symptoms (Fig. 3). The miR-146a-5p is differentially expressed in the sciatic nerve of T2DM and diabetic neuropathy mice, and it efficiently inhibits the inflammatory response.53,54 In diabetic neuropathy mice, the down-regulation of miR-146a-5p plays along with the up-regulation of TNF-α, IL-1β, and NF-κB, indicating that miR-146a-5p can influence diabetic neuropathy through regulating inflammatory response.54 Luo et al. successfully prepared nano-miR-146a-5p by mixing TPSP solution and miR-146a-5p. This study demonstrated that although nano-miR-146a-5p has no effect on regulating blood glucose, it contributes to the protection of nerve injury.55 In diabetic neuropathy mice, the myelinated nerve fibres show irregularly arranged myelin sheaths, vacuolar-like defects, and axonal atrophy. Interestingly, using nano-miR-146a-5p significantly improved axonal atrophy and myelin sheath abnormalities. Moreover, nano-miR-146a-5p is able to inhibit the expression of IL-1β, IL-6, caspase-3 and cleaved caspase-3, which are associated with apoptosis, so it plays a role in reducing inflammation and cell apoptosis to improve diabetic neuropathy.55
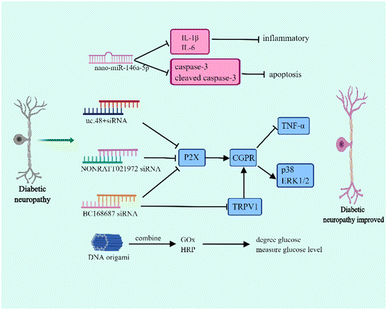 |
| Fig. 3 Summary of the mechanisms of action of functional nucleic acids in diabetic neuropathy. | |
In addition to nano-miRNAs, nucleic acid nanomaterial siRNAs affect diabetic neuropathy. The P2X receptor family (P2X1–7) is considered as a potential target for the treatment of diabetic peripheral neuropathic pain.56 Several studies have demonstrated that siRNA can increase the hyperpathia threshold value and relieve pain through regulating P2X.57–60 It had been reported that uc.48+ siRNA is able to decrease the expression of P2X, TNF-α and the activation of extracellular signal-regulated kinase (ERK1/2) in DRG.57 Further study found that uc.48+ siRNA contributes to the release of calcitonin gene related peptide (CGRP), thereby reducing the expression of proinflammatory cytokines and the phosphorylation of p38 and ERK1/2 and finally improving hyperpathia.58 In addition, NONRATT021972 siRNA and BC168687 siRNA are similar to uc.48+ siRNA.59,60 Transient receptor potential vanilloid type 1 (TRPV1) participates in pain signal transduction which is mainly distributed in DRG.61 The activation of TRPV1 can enhance the sensitivity of pain and the up-regulation of TRPV1 has been found to be involved in diabetic neuropathic pain.62 In fact, BC168687 siRNA does not only influence P2X but also regulates TRPV1. BC168687 siRNA has an effect on decreasing the expression of TRPV1 and then also inhibits proinflammatory cytokine expression and p38 and ERK1/2 phosphorylation.63 However, diabetic cardiovascular autonomic neuropathy is one of the reasons for diabetic death and the prevalence of cardiovascular autonomic neuropathy in patients with T1DM can reach up to 90%.64 In the early stage of diabetic cardiovascular autonomic neuropathy, the vagus nerve is impaired and leads to resting tachycardia whereas in the advanced diabetic cardiovascular autonomic neuropathy, the sympathetic nerve becomes abnormal.65 Notably, uc.48+ siRNA also affects the improvement of cardiac function and reduces the symptoms of diabetic cardiovascular autonomic neuropathy through affecting P2X.66
DNA origami is also a nanoscale reactor and DNA origami has been demonstrated to be a promising candidate for drug delivery. The size of the DNA origami functional devices ranges from 50 nm to 400 nm which can protect the loaded drugs from damage.67 DNA origami has shown great potential in delivering anti-cancer drugs to increase drug sensitivity and reverse drug resistance.68 Interestingly, DNA origami can combine with glucose oxidase (GOx) and horseradish peroxidase (HRP). The GOx degrades glucose and measures the level of blood glucose.69 Thus, this DNA origami is a potential tool to use in metabolic syndrome to diagnose hyperglycemia.70 However, different from other DNA nanomaterials, DNA origami carries more negative charges and is dependent on its size and shape in the process of interacting with cells.71 Actually, compared with vimineous DNA origami nanoparticles, the larger DNA origami nanoparticles which have a greater compactness were preferentially internalized.72 Thus, the larger DNA origami nanoparticles have a greater cell uptake rate. Furthermore, the shape of DNA origami nanoparticles influences the interaction way between DNA origami nanoparticles and cells.72,73 Therefore, further studies are still needed to promote DNA origami to work more effectively.
Diabetic neuropathy is a complex regional disease with diverse symptoms that may greatly influence the health of patients with diabetes and cause great suffering. Nucleic acid nanomaterials have been found to be beneficial for improving nervous lesions. As the only nucleic acid nanomaterials used are RNA nanomaterials, more studies are needed to investigate the application of DNA nanomaterials in diabetic neuropathy and provide new insights into the treatment of diabetic neuropathy.
Functional nucleic acid materials and diabetic retinopathy
Diabetic retinopathy is a familiar microangiopathy of diabetes as well as a neurodegenerative disease which is involved in metabolic disturbance resulting in hyperglycemia, vascular endothelial cell abnormity, and retinal ischemia-reperfusion injuries.74,75 Diabetic retinopathy generally leads to macular oedema, amotio retinae, and vitreous haemorrhage. It may ultimately result in visual decrease, blurred vision, and even blindness.76,77 The prevalence of diabetic retinopathy is increasing with the aggravation of diabetes; therefore, regulating the level of blood glucose or avoiding the transportation of glucose to the retina and angiogenesis are necessary.78,79 At present, using nucleic acid nanomaterials in diabetic retinopathy has achieved great progress to reduce the risk of vision diminution and blindness (Fig. 4).
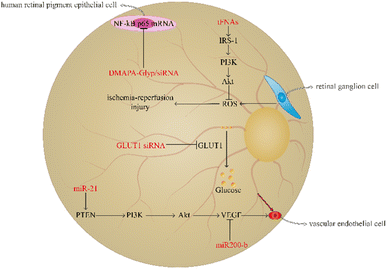 |
| Fig. 4 Functional nucleic acids improving diabetic retinopathy. | |
Retinal ganglion cells (RGCs) are able to participate in the formation of visual sensation by converting the visual pattern into an action potential to transport to a specific area of the cerebrum; therefore, the injury of RGCs is closely associated with severe vision loss, and ROS overproduction of RGCs can result in retinal ischaemia-reperfusion injuries.80,81 Qin et al. demonstrated that tFNA plays a prominent role in protecting the retina from ischaemia-reperfusion injuries. The tFNA serves as an antioxidant to reverse apoptosis induced by oxidative stress by activating the Akt/pAkt signalling pathway to block the ROS-mediated mitochondrial apoptosis pathway.82 Besides, tFNAs have been found to activate the insulin receptor substrate (IRS-1)/phosphoinositide 3 kinase (PI3K)/Akt signalling pathway to improve the glucose uptake ability and promote the transcription of glucose transporter-2 (GLUT2) to increase the utilisation rate of glucose and strengthen the sensitivity to insulin. In addition, in mice with T2DM, tFNAs can also decrease the blood glucose level.83 Resveratrol (RSV) is a traditional Chinese medicine, which plays an important role in anti-inflammation.84 However, RSV is unstable and has a bad bioavailability.85 Yanjing Li et al. have prepared tFNA nanoparticles to deliver RSV.86 The study showed that the combination of tFNA and RSV can vastly enhance the role of tFNA in insulin resistance. RSV-loaded tFNA promotes the transformation of M1 macrophages to the M2 phenotype to improve glycogen synthesis and insulin sensitivity in the liver. Therefore, tFNA is a potential tool in the improvement of insulin resistance.86
miRNA has been found to play a pivotal key in angiogenesis of diabetic retinopathy. miR-21 can target phosphatase and tensin homolog (PTEN) to activate the PI3K/Akt/VEGF signalling pathway to promote vascular endothelial cell proliferation and angiogenesis.87 In contrast to miR-21, miR200 plays a role in inhibiting the angiogenic factor and decreasing vascular endothelial cell growth.88 In the induction of the CAG promoter, the plasmid pCAG-miR200-b-IRES-eGFP can be prepared through the amplification and clone of miR200-b and its native flanking sequences into the pCAG-eGFP carrier and then contraction into DNA nanoparticles to deliver to the diseased region. The study demonstrated that miR200-b DNA nanoparticles can inhibit VEGF receptor 2 to decrease angiogenesis in mice with diabetic retinopathy.89
The transportation of glucose from the blood to the retina can result in hyperglycaemia-induced diabetic retinopathy, and GLUT1 is the only glucose carrier which can mediate the glucose shuttle from the blood to the retina.90,91 GLUT1 siRNA can inhibit GLUT1, and the retinal glucose level of diabetic retinopathy mice using GLUT1 siRNA is significantly lower than that in mice using diabetic scrambled siRNA, suggesting that GLUT1 siRNA has a positive effect on inhibiting glucose transport. Furthermore, GLUT1 siRNA can reduce rod cell death and the number of cone cells, thereby improving the retinal microenvironment.92 In addition, siRNA is able undergo condensation reactions with DMAPA-Glyp ramifications and then form DMAPA-Glyp/siRNA nanoparticles. Liu et al. found that these nanoparticles contributed to decreasing the expression of NF-κB p65 mRNA in human retinal pigment epithelial cells.93 Therefore, these nanoparticles could serve as potential candidates for diabetic retinopathy treatment.
Notably, oligonucleotide nanomaterials can be used to monitor the degree of angiogenesis in diabetic retinopathy. Leucine-rich alpha-2-glycoprotein 1 (Lrg1) promotes vessel growth induced by TGF-β1 signalling.94 MBs are single stranded hairpin shaped oligonucleotides which have the ability to recognise the exon of Lrg1 mRNA. MBs-gold nanorod nanosensors have been found to play a role in determining the severity of diabetic retinopathy by observing the expression of Lrg1.95
Therefore, nucleic acid nanomaterials are beneficial to inhibit the vascular endothelial cell growth, retinal ischemia-reperfusion injuries, and monitor angiogenesis degree. Therefore, nucleic acid nanomaterials may be potential tools that can be applied in the treatment and diagnosis of diabetic retinopathy.
Conclusions
Studies on the use of functional nucleic acids in the treatment of diabetic complications are rapidly developing. In this review, we have listed most of the latest antidiabetic functional nucleic acids, including tFNAs, siRNAs, miRNAs, LNA, ASOs, and DNA origami, which can limit ROS overproduction and alleviate tissue damage caused by hyperglycaemia. The advantages of functional nucleic acids including biocompatibility, biodegradability, renewability at the damaged organs and less toxicity make them unique materials to be used for diabetic complication treatments.11,32,37,96,97
Although most of these have been tested for effectiveness in DM animal models, few have been tested in clinical trials, and their physicochemical properties remain imperfect. Further experiments are needed, such as reliable toxicological studies in the human body and the discovery of unique antidiabetic properties. Currently, the efficient delivery of functional nucleic acids to the target tissue is still the main bottleneck for the development of nucleic acid-based therapeutics. Although delivery system great progress has been made in the field of novel drug delivery such as liposomes, niosomes, transfersomes, exosomes, nano-hydrogels and SLNs, there are still a number of limitations.98–100 Their clinical translation is hindered by their storage instability, toxicity, poor drug loading and yield, poor site specificity and expensive manufacturing procedures and so on. Therefore, researchers need to identify better strategies to ensure that these functional nucleic acids are efficiently delivered to the appropriate cells. In addition, at present, studies of functional nucleic acids for diabetic complications mostly focus on tFNA, miRNA, and siRNA; therefore, more research is needed to design additional functional nucleic acids which may have a superior role in diabetic complications. Furthermore, comparing the effects and mechanisms of existing functional nucleic acids and identifying the most suitable drugs loaded into functional nucleic acids may provide a more effective therapeutic regimen for diabetic complications. We discovered that drug-loaded tFNA can also play a role in diverse diabetic complications; therefore, subsequent studies can further explore the application of tFNA to simultaneously treat multiple diabetic complications.86 The novel functional nucleic acids presented in this article, as well as the existing challenges and future trends, will help researchers develop unique functional nucleic acids for novel diabetic therapeutic applications.
Diabetic complication |
Functional nucleic acid material |
Mechanism |
Reference |
Diabetic foot ulcer |
tFNAs |
Activate the Akt/Nrf2/HO-1 signalling pathway; deliver traditional Chinese herbal medicines |
101
|
Keap1 siRNA |
Decrease Keap1 and activate NrF2 |
28
|
TNF-α siRNA |
Against TNF-α to alleviate DW |
102
|
miR-146a |
Knock down the NFKB and decrease IL-6, IL-8 |
103
|
GM3 siRNA |
Down-regulate GM3S mRNA |
104
|
Diabetic nephropathy |
LNA-anti-miR-192 |
Suppress the expression of endogenous miR-192; enhance Zeb1/Zeb2 |
39
|
Inhibit the Akt/Foxo3a signalling pathway |
40
|
miRNA-10a/b |
Inhibit TGF-β receptor 1 |
105
|
CHOP-ASOs |
Down-regulate Slc5a2/CD133 |
106
|
Diabetic neuropathy |
MiR-146a-5p |
Inhibit inflammatory response |
54
|
Nano-miR-146a-5p |
Improve axonal atrophy and myelin sheath abnormalities; inhibit the expression of IL-1β, IL-6, caspase-3 and cleaved caspase-3 |
55
|
Uc.48+ siRNA |
Decrease the expression of P2X and TNF-α and the activation of ERK1/2 |
57
|
Promote the release of CGRP and reduce the expression of proinflammatory cytokines and the phosphorylation of p38 and ERK1/2 |
58
|
BC168687 siRNA |
Decrease the expression of TRPV1 |
61
|
NONRATT021972 siRNA |
Regulate P2X |
60
|
DNA origami |
Combine with Gox and HRP |
70
|
Diabetic retinopathy |
tFNAs |
Activate the Akt/pAkt signalling pathway |
82
|
Activate the PI3K/Akt signalling pathway |
83
|
Promote the transformation of M1 macrophages to the M2 phenotype |
86
|
MiR-21 |
Activate the PI3K/Akt/VEGF signalling pathway |
87
|
miR-200 |
Inhibit the angiogenic factor and decreasing vascular endothelial cell growth |
88
|
miR200-b DNA nanoparticles |
Inhibit VEGF receptor 2 to decrease angiogenesis |
89
|
GLUT1 siRNA |
Inhibit CLUT1; reduce rod cell death and the number of cone cells |
92
|
DMAPA-Glyp/siRNA nanoparticles |
Decrease the expression of NF-κB p65 mRNA |
93
|
MBs-gold nanorod nanosensors |
Recognise the exon of Lrg1 mRNA |
95
|
Author contributions
Wen Wen: conceptualization; writing – original draft. Yuzi Wei: writing – review and editing; conceptualization. Shaojingya Gao: writing – review and editing; conceptualization.
Conflicts of interest
There are no conflicts to declare.
Acknowledgements
This work was financially supported by the Sichuan University Innovation and Entrepreneurship Charity Fund (C2023123104), Sichuan University Postdoctoral Innovation Fund (2023SCU12040) and China Postdoctoral Science Foundation Funded Project (2022TQ0222 & 2023M732441). Fig. 2–4 were drawn using MedPeer.
Notes and references
- K. M. El-Say,
et al., Pairing 3D-Printing with Nanotechnology to Manage Metabolic Syndrome, Int. J. Nanomed., 2022, 17, 1783–1801 CrossRef PubMed.
- P. Saeedi,
et al., Global and regional diabetes prevalence estimates for 2019 and projections for 2030 and 2045: Results from the International Diabetes Federation Diabetes Atlas, Diabetes Res. Clin. Pract., 2019, 157, 107843 CrossRef PubMed.
- R. Najafi,
et al., Neuroprotective effect of cerium oxide nanoparticles in a rat model of experimental diabetic neuropathy, Brain Res. Bull., 2017, 131, 117–122 CrossRef CAS PubMed.
- C. Faselis,
et al., Microvascular complications of type 2 diabetes mellitus, Curr. Vasc. Pharmacol., 2020, 18(2), 117–124 CrossRef CAS PubMed.
- A. Pfannkuche,
et al., Prevalence and risk factors of diabetic peripheral neuropathy in a diabetics cohort: register initiative “diabetes and nerves”, Endocr. Metab. Sci., 2020, 1(1–2), 100053 CrossRef.
- X.-X. Zhang, J. Kong and K. Yun, Prevalence of diabetic nephropathy among patients with type 2 diabetes mellitus in China: a meta-analysis of observational studies, J. Diabetes Res., 2020, 2020 Search PubMed.
- N. C. do Vale Moreira,
et al., Prevalence of Metabolic Syndrome by different definitions, and its association with type 2 diabetes, pre-diabetes, and cardiovascular disease risk in Brazil, Diabetes Metabol. Syndr., 2020, 14(5), 1217–1224 CrossRef PubMed.
- C. Sabanayagam,
et al., Incidence and progression of diabetic retinopathy: a systematic review, Lancet Diabetes Endocrinol., 2019, 7(2), 140–149 CrossRef PubMed.
- G. Ponirakis,
et al., Prevalence and management of diabetic neuropathy in secondary care in Qatar, Diabetes Metabol. Res. Rev., 2020, 36(4), e3286 CrossRef CAS PubMed.
- S. Y. Tan,
et al., Type 1 and 2 diabetes mellitus: a review on current treatment approach and gene therapy as potential intervention, Diabetes, Metab. Syndrom, 2019, 13(1), 364–372 CrossRef PubMed.
- W. Xu,
et al., Functional Nucleic Acid Nanomaterials: Development, Properties, and Applications, Angew Chem. Int. Ed. Engl., 2021, 60(13), 6890–6918 CrossRef CAS PubMed.
- S. Lin,
et al., Antioxidative and angiogenesis-promoting effects of tetrahedral framework nucleic acids in diabetic wound healing with activation of the Akt/Nrf2/HO-1 pathway, ACS Appl. Mater. Interfaces, 2020, 12(10), 11397–11408 CrossRef CAS PubMed.
- S. Guo and L. A. DiPietro, Factors Affecting Wound Healing, J. Dent. Res., 2010, 89(3), 219–229 CrossRef CAS PubMed.
- C. Wang,
et al., Engineering bioactive self-healing antibacterial exosomes hydrogel for promoting chronic diabetic wound healing and complete skin regeneration, Theranostics, 2019, 9(1), 65 CrossRef CAS PubMed.
- X. Zhou,
et al., The signaling pathways of traditional Chinese medicine in promoting diabetic wound healing, J. Ethnopharmacol., 2022, 282, 114662 CrossRef CAS PubMed.
-
D. F. Bandyk, The diabetic foot: pathophysiology, evaluation, and treatment, in Seminars in vascular surgery, Elsevier, 2018 Search PubMed.
- J. Zhu,
et al., Tetrahedral framework nucleic acids promote scarless healing of cutaneous wounds via the AKT-signaling pathway, Signal Transduct. Targeted Ther., 2020, 5(1), 1–11 CrossRef PubMed.
- T. Tian,
et al., A framework nucleic acid based robotic nanobee for active targeting therapy, Adv. Funct. Mater., 2021, 31(5), 2007342 CrossRef CAS.
- C. Liu,
et al., Nanobooster-encapsulated hybrid RNA as anti-tumor viral mimicry, Nano Today, 2021, 38, 101211 CrossRef CAS.
- S. Li,
et al., Advances in biological applications of self-assembled DNA tetrahedral nanostructures, Mater. Today, 2019, 24, 57–68 CrossRef CAS.
- M. Simons, E. Gordon and L. Claesson-Welsh, Mechanisms and regulation of endothelial VEGF receptor signalling, Nat. Rev. Mol. Cell Biol., 2016, 17(10), 611–625 CrossRef CAS PubMed.
- M. S. Hotkar,
et al., Preliminary investigation of topical nitroglycerin formulations containing natural wound healing agent in diabetes-induced foot ulcer, Int. Wound J., 2015, 12(2), 210–217 CrossRef PubMed.
- B. C. Ren,
et al., Curcumin alleviates oxidative stress and inhibits apoptosis in diabetic cardiomyopathy via Sirt1-Foxo1 and PI3K-Akt signalling pathways, J. Cell. Mol. Med., 2020, 24(21), 12355–12367 CrossRef CAS PubMed.
- J. A. Perini,
et al., Topical application of Acheflan on rat skin injury accelerates wound healing: a histopathological, immunohistochemical and biochemical study, BMC Compl. Alternative Med., 2015, 15(1), 1–8 CrossRef PubMed.
- R. Bhikha and J. Glynn, The pharmacological action of common herbal remedies, Am. J. Intern. Med., 2018, 6(5), 99–107 Search PubMed.
- M. Zhang,
et al., Anti-inflammatory activity of curcumin-loaded tetrahedral framework nucleic acids on acute gouty arthritis, Bioact. Mater., 2022, 8, 368–380 CrossRef CAS PubMed.
- M. A. Soares,
et al., Restoration of Nrf2 Signaling Normalizes the Regenerative Niche, Diabetes, 2016, 65(3), 633–646 CrossRef CAS PubMed.
- P. S. Rabbani,
et al., Dysregulation of Nrf2/Keap1 Redox Pathway in Diabetes Affects Multipotency of Stromal Cells, Diabetes, 2019, 68(1), 141–155 CrossRef CAS PubMed.
- L. N. Kasiewicz and K. A. Whitehead, Lipid nanoparticles silence tumor necrosis factor α to improve wound healing in diabetic mice, Bioeng. Transl. Med., 2019, 4(1), 75–82 CrossRef CAS PubMed.
- A. Rebane,
et al., MicroRNA-146a alleviates chronic skin inflammation in atopic dermatitis through suppression of innate immune responses in keratinocytes, J. Allergy Clin. Immunol., 2014, 134(4), 836–847 CrossRef CAS PubMed.
- X. Q. Wang,
et al., Ganglioside GM3 depletion reverses impaired wound healing in diabetic mice by activating IGF-1 and insulin receptors, J. Invest. Dermatol., 2014, 134(5), 1446–1455 CrossRef CAS PubMed.
- P. S. Randeria,
et al., siRNA-based spherical nucleic acids reverse impaired wound healing in diabetic mice by ganglioside GM3 synthase knockdown, Proc. Natl. Acad. Sci. U.S.A., 2015, 112(18), 5573–5578 CrossRef CAS PubMed.
- N. Samsu, Diabetic Nephropathy: Challenges in Pathogenesis, Diagnosis, and Treatment, BioMed Res. Int., 2021, 2021, 1497449 Search PubMed.
- L. Wang,
et al., TGF-beta as a master regulator of diabetic nephropathy, Int. J. Mol. Sci., 2021, 22(15), 7881 CrossRef CAS PubMed.
- J. Donate-Correa,
et al., Inflammatory targets in diabetic nephropathy, J. Clin. Med., 2020, 9(2), 458 CrossRef CAS PubMed.
- M. L. Alvarez and J. K. DiStefano, Towards microRNA-based therapeutics for diabetic nephropathy, Diabetologia, 2013, 56(3), 444–456 CrossRef CAS PubMed.
- T. Zhang,
et al., Progress in Biomedical Applications of Tetrahedral Framework Nucleic Acid-Based Functional Systems, ACS Appl. Mater. Interfaces, 2020, 12(42), 47115–47126 CrossRef CAS PubMed.
- M. Kato,
et al., MicroRNA-192 in diabetic kidney glomeruli and its function in TGF-beta-induced collagen expression via inhibition of E-box repressors, Proc. Natl. Acad. Sci. U. S. A., 2007, 104(9), 3432–3437 CrossRef CAS PubMed.
- K. Fluiter,
et al., In vivo tumor growth inhibition and biodistribution studies of locked nucleic acid (LNA) antisense oligonucleotides, Nucleic Acids Res., 2003, 31(3), 953–962 CrossRef CAS PubMed.
- S. Putta,
et al., Inhibiting microRNA-192 ameliorates renal fibrosis in diabetic nephropathy, J. Am. Soc. Nephrol., 2012, 23(3), 458–469 CrossRef CAS PubMed.
- J. Li,
et al., MicroRNA-10a/b inhibit TGF-β/Smad-induced renal fibrosis by targeting TGF-β receptor 1 in diabetic kidney disease, Mol. Ther. Nucleic Acids, 2022, 28, 488–499 CrossRef CAS PubMed.
- D. Wang,
et al., Sitagliptin ameliorates diabetic nephropathy by blocking TGF-β1/Smad signaling pathway, Int. J. Mol. Med., 2018, 41(5), 2784–2792 CAS.
- S. A. Gale, D. Acar and K. R. Daffner, Dementia, Am. J. Med., 2018, 131(10), 1161–1169 CrossRef PubMed.
- Y. Fan,
et al., Rtn1a-mediated endoplasmic reticulum stress in podocyte injury and diabetic nephropathy, Sci. Rep., 2017, 7(1), 1–13 CrossRef PubMed.
- Y. Li,
et al., Altered DNA methylation of TRIM13 in diabetic nephropathy suppresses mesangial collagen synthesis by promoting ubiquitination of CHOP, EBioMedicine, 2020, 51, 102582 CrossRef PubMed.
- K. Shahzad,
et al., CHOP-ASO ameliorates glomerular and tubular damage on top of ACE inhibition in diabetic kidney disease, J. Am. Soc. Nephrol., 2021, 32(12), 3066–3079 CrossRef CAS PubMed.
- L. M. Hinder,
et al., Transcriptional networks of progressive diabetic peripheral neuropathy in the db/db mouse model of type 2 diabetes: an inflammatory story, Exp. Neurol., 2018, 305, 33–43 CrossRef CAS PubMed.
- E. L. Feldman,
et al., Diabetic neuropathy, Nat. Rev. Dis. Prim., 2019, 5(1), 42 CrossRef PubMed.
- R. Straube,
et al., Metabolic and Non-Metabolic Peripheral Neuropathy: Is there a Place for Therapeutic Apheresis?, Horm. Metab. Res., 2019, 51(12), 779–784 CrossRef CAS PubMed.
- D. Ziegler,
et al., Polyneuropathy is inadequately treated despite increasing symptom intensity in individuals with and without diabetes (PROTECT follow-up study), J. Diabetes Investig., 2020, 11(5), 1272–1277 CrossRef CAS PubMed.
- K. Ozaki,
et al., Effect of combined dyslipidemia and hyperglycemia on diabetic peripheral neuropathy in alloxan-induced diabetic WBN/Kob rats, J. Toxicol. Pathol., 2018, 31(2), 125–133 CrossRef CAS PubMed.
- J. K. Sharma, A. Rohatgi and D. Sharma, Diabetic autonomic neuropathy: a clinical update, J. Roy. Coll. Phys. Edinb., 2020, 50(3), 269–273 CrossRef PubMed.
- W. Y. Lo, C. T. Peng and H. J. Wang, MicroRNA-146a-5p Mediates High Glucose-Induced Endothelial Inflammation via Targeting Interleukin-1 Receptor-Associated Kinase 1 Expression, Front. Physiol., 2017, 8, 551 CrossRef PubMed.
- Y. Feng,
et al., Involvement of microRNA-146a in diabetic peripheral neuropathy through the regulation of inflammation, Drug. Des. Devel. Ther., 2018, 12, 171–177 CrossRef CAS PubMed.
- Q. Luo,
et al., Nanoparticle-microRNA-146a-5p polyplexes ameliorate diabetic peripheral neuropathy by modulating inflammation and apoptosis, Nanomedicine, 2019, 17, 188–197 CrossRef CAS PubMed.
- L. P. Bernier, A. R. Ase and P. Séguéla, P2X receptor channels in chronic pain pathways, Br. J. Pharmacol., 2018, 175(12), 2219–2230 CrossRef CAS PubMed.
- S. Wang,
et al., LncRNA uc.48+ is involved in diabetic neuropathic pain mediated by the P2X3 receptor in the dorsal root ganglia, Purinergic Signal., 2016, 12(1), 139–148 CrossRef CAS PubMed.
- W. Xiong,
et al., Effects of lncRNA uc.48+ siRNA on the release of CGRP in the spinal cords of rats with diabetic neuropathic pain, Int. J. Clin. Exp. Pathol., 2017, 10(9), 9960–9969 Search PubMed.
- C. Liu,
et al., Effects of LncRNA BC168687 siRNA on Diabetic Neuropathic Pain Mediated by P2X(7) Receptor on SGCs in DRG of Rats, BioMed Res. Int., 2017, 2017, 7831251 Search PubMed.
- H. Peng,
et al., lncRNA NONRATT021972 siRNA Decreases Diabetic Neuropathic Pain Mediated by the P2X(3) Receptor in Dorsal Root Ganglia, Mol. Neurobiol., 2017, 54(1), 511–523 CrossRef CAS PubMed.
- Y. Takayama and M. Tominaga, Involvement of TRPV1-ANO1 Interactions in Pain-Enhancing Mechanisms, Adv. Exp. Med. Biol., 2018, 1099, 29–36 CrossRef CAS PubMed.
- Akhilesh,
et al., Unlocking the potential of TRPV1 based siRNA therapeutics for the treatment of chemotherapy-induced neuropathic pain, Life Sci., 2022, 288, 120187 CrossRef CAS PubMed.
- C. Liu,
et al., Long Non-coding RNA BC168687 is Involved in TRPV1-mediated Diabetic Neuropathic Pain in Rats, Neuroscience, 2018, 374, 214–222 CrossRef CAS PubMed.
- M. Matta,
et al., Predictors of Cardiovascular Autonomic Neuropathy Onset and Progression in a Cohort of Type 1 Diabetic Patients, J. Diabetes Res., 2018, 2018, 5601351 CAS.
- S. Agashe and S. Petak, Cardiac Autonomic Neuropathy in Diabetes Mellitus, Methodist Debakey Cardiovasc. J., 2018, 14(4), 251–256 CrossRef PubMed.
- B. Wu,
et al., LncRNA uc.48+ siRNA improved diabetic sympathetic neuropathy in type 2 diabetic rats mediated by P2X7 receptor in SCG, Auton. Neurosci., 2016, 197, 14–18 CrossRef CAS PubMed.
- P. W. Rothemund, Folding DNA to create nanoscale shapes and patterns, Nature, 2006, 440(7082), 297–302 CrossRef CAS PubMed.
- S. Pal and T. Rakshit, Folate-Functionalized DNA Origami for Targeted Delivery of Doxorubicin to Triple-Negative Breast Cancer, Front. Chem., 2021, 9, 721105 CrossRef CAS PubMed.
- S. H. Khatami,
et al., Glucose oxidase: applications, sources, and recombinant production, Biotechnol. Appl. Biochem., 2022, 69(3), 939–950 CrossRef CAS PubMed.
- V. Linko, M. Eerikäinen and M. A. Kostiainen, A modular DNA origami-based enzyme cascade nanoreactor, Chem. Commun., 2015, 51(25), 5351–5354 RSC.
- Q. Jiang,
et al., Rationally Designed DNA-Origami Nanomaterials for Drug Delivery In Vivo, Adv. Mater., 2019, 31(45), e1804785 CrossRef PubMed.
- M. M. C. Bastings,
et al., Modulation of the Cellular Uptake of DNA Origami through Control over Mass and Shape, Nano Lett., 2018, 18(6), 3557–3564 CrossRef CAS PubMed.
- P. Wang,
et al., Visualization of the Cellular Uptake and Trafficking of DNA Origami Nanostructures in Cancer Cells, J. Am. Chem. Soc., 2018, 140(7), 2478–2484 CrossRef CAS PubMed.
- A. A. Thomas,
et al., lncRNA H19 prevents endothelial-mesenchymal transition in diabetic retinopathy, Diabetologia, 2019, 62(3), 517–530 CrossRef CAS PubMed.
- N. Jiang,
et al., Laquinimod exerts anti-inflammatory and antiapoptotic effects in retinal ischemia/reperfusion injury, Int. Immunopharmacol., 2020, 88, 106989 CrossRef CAS PubMed.
- J. Lange,
et al., Region-specific ischemia, neovascularization and macular oedema in treatment-naïve proliferative diabetic retinopathy, Clin. Exp. Ophthalmol., 2018, 46(7), 757–766 CrossRef PubMed.
- A. N. Antoszyk,
et al., Effect of Intravitreous Aflibercept vs Vitrectomy With Panretinal Photocoagulation on Visual Acuity in Patients With Vitreous Hemorrhage From Proliferative Diabetic Retinopathy: A Randomized Clinical Trial, JAMA, 2020, 324(23), 2383–2395 CrossRef CAS PubMed.
- T. Takao,
et al., Effect of postprandial hyperglycemia at clinic visits on the incidence of retinopathy in patients with type 2 diabetes: an analysis using real-world long-term follow-up data, J Diabetes Investig, 2020, 11(4), 930–937 CrossRef CAS PubMed.
- K. Y. Lin,
et al., Update in the epidemiology, risk factors, screening, and treatment of diabetic retinopathy, J Diabetes Investig, 2021, 12(8), 1322–1325 CrossRef PubMed.
- P. B. Detwiler, Phototransduction in Retinal Ganglion Cells, Yale J. Biol. Med., 2018, 91(1), 49–52 CAS.
- M. Liu,
et al., GSK872 and necrostatin-1 protect retinal ganglion cells against necroptosis through inhibition of RIP1/RIP3/MLKL pathway in glutamate-induced retinal excitotoxic model of glaucoma, J. Neuroinflammation, 2022, 19(1), 262 CrossRef CAS PubMed.
- X. Qin,
et al., Tetrahedral framework nucleic acids prevent retina ischemia-reperfusion injury from oxidative stress via activating the Akt/Nrf2 pathway, Nanoscale, 2019, 11(43), 20667–20675 RSC.
- Y. Li,
et al., Tetrahedral Framework Nucleic Acids Ameliorate Insulin Resistance in Type 2 Diabetes Mellitus via the PI3K/Akt Pathway, ACS Appl. Mater. Interfaces, 2021, 13(34), 40354–40364 CrossRef CAS PubMed.
- T. Meng,
et al., Anti-Inflammatory Action and Mechanisms of Resveratrol, Molecules, 2021, 26(1) DOI:10.3390/molecules26010229.
- B. Tian and J. Liu, Resveratrol: a review of plant sources, synthesis, stability, modification and food application, J. Sci. Food Agric., 2020, 100(4), 1392–1404 CrossRef CAS PubMed.
- Y. Li,
et al., Tetrahedral Framework Nucleic Acid-Based Delivery of Resveratrol Alleviates Insulin Resistance: From Innate to Adaptive Immunity, Nanomicro Lett., 2021, 13(1), 86 CrossRef CAS PubMed.
- J. M. Lu,
et al., Repression of microRNA-21 inhibits retinal vascular endothelial cell growth and angiogenesis via PTEN dependent-PI3K/Akt/VEGF signaling pathway in diabetic retinopathy, Exp. Eye Res., 2020, 190, 107886 CrossRef CAS PubMed.
- C. Y. Wang,
et al., Elevated miR-200a and miR-141 inhibit endocrine gland-derived vascular endothelial growth factor expression and ciliogenesis in preeclampsia, J. Physiol., 2019, 597(12), 3069–3083 CrossRef CAS PubMed.
- R. N. Mitra,
et al., Nanoparticle-mediated miR200-b delivery for the treatment of diabetic retinopathy, J. Control Release, 2016, 236, 31–37 CrossRef CAS PubMed.
- S. Cao,
et al., GLUT1 biological function and inhibition: research advances, Future Med. Chem., 2021, 13(14), 1227–1243 CrossRef CAS PubMed.
- N. C. Holoman,
et al., Reduction of Glut1 in the Neural Retina But Not the RPE Alleviates Polyol Accumulation and Normalizes Early Characteristics of Diabetic Retinopathy, J. Neurosci., 2021, 41(14), 3275–3299 CrossRef CAS PubMed.
- Z. P. You,
et al., Suppression of diabetic retinopathy with GLUT1 siRNA, Sci. Rep., 2017, 7(1), 7437 CrossRef PubMed.
- Z. Liu,
et al., Efficient delivery of NF-κB siRNA to human retinal pigment epithelial cells with hyperbranched cationic polysaccharide derivative-based nanoparticles, Int. J. Nanomed., 2015, 10, 2735–2749 CAS.
- M. Singhal,
et al., Temporal multi-omics identifies LRG1 as a vascular niche instructor of metastasis, Sci. Transl. Med., 2021, 13(609), eabe6805 CrossRef PubMed.
- D. C. S. Lio,
et al., Molecular Beacon Gold Nanosensors for Leucine-Rich Alpha-2-Glycoprotein-1 Detection in Pathological Angiogenesis, ACS Sens., 2018, 3(9), 1647–1655 CrossRef CAS PubMed.
- R. Regazzi, MicroRNAs as therapeutic targets for the treatment of diabetes mellitus and its complications, Expert Opin. Ther. Targets, 2018, 22(2), 153–160 CrossRef CAS PubMed.
- Q. Bai,
et al., Potential Applications of Nanomaterials and Technology for Diabetic Wound Healing, Int. J. Nanomed., 2020, 15, 9717–9743 CrossRef CAS PubMed.
- V. T. Arantes,
et al., Retinoic acid-loaded solid lipid nanoparticles surrounded by chitosan film support diabetic wound healing in in vivo study, Colloids Surf. B Biointerfaces, 2020, 188, 110749 CrossRef CAS PubMed.
- X. He,
et al., Emerging roles of exosomal miRNAs in diabetes mellitus, Clin. Transl. Med., 2021, 11(6), e468 CrossRef CAS PubMed.
- A. Awasthi,
et al., Expanding arsenal against diabetic wounds using nanomedicines and nanomaterials: success so far and bottlenecks, J. Drug Delivery Sci. Technol., 2022, 74, 103534 CrossRef CAS.
- S. Lin,
et al., Antioxidative and Angiogenesis-Promoting Effects of Tetrahedral Framework Nucleic Acids in Diabetic Wound Healing with Activation of the Akt/Nrf2/HO-1 Pathway, ACS Appl. Mater. Interfaces, 2020, 12(10), 11397–11408 CrossRef CAS PubMed.
- L. N. Kasiewicz and K. A. Whitehead, Lipid nanoparticles silence tumor necrosis factor α to improve wound healing in diabetic mice, Bioeng. Transl. Med., 2019, 4(1), 75–82 CrossRef CAS PubMed.
- A. Rebane,
et al., MicroRNA-146a alleviates chronic skin inflammation in atopic dermatitis through suppression of innate immune responses in keratinocytes, J. Allergy Clin. Immunol., 2014, 134(4), 836–847 CrossRef CAS PubMed.
- P. S. Randeria,
et al., siRNA-based spherical nucleic acids reverse impaired wound healing in diabetic mice by ganglioside GM3 synthase knockdown, Proc. Natl. Acad. Sci. U. S. A., 2015, 112(18), 5573–5578 CrossRef CAS PubMed.
- J. Li,
et al., MicroRNA-10a/b inhibit TGF-β/Smad-induced renal fibrosis by targeting TGF-β receptor 1 in diabetic kidney disease, Mol. Ther. Nucleic Acids, 2022, 28, 488–499 CrossRef CAS PubMed.
- K. Shahzad,
et al., CHOP-ASO Ameliorates Glomerular and Tubular Damage on Top of ACE Inhibition in Diabetic Kidney Disease, J. Am. Soc. Nephrol., 2021, 32(12), 3066–3079 CrossRef CAS PubMed.
Footnote |
† These authors contributed equally to this work. |
|
This journal is © The Royal Society of Chemistry 2023 |