DOI:
10.1039/D2NA00700B
(Review Article)
Nanoscale Adv., 2023,
5, 27-45
Engineering functional natural polymer-based nanocomposite hydrogels for wound healing
Received
11th October 2022
, Accepted 17th November 2022
First published on 17th November 2022
Abstract
Skin injury occurs due to acute trauma, chronic trauma, infection, and surgical intervention, which can result in severe dysfunction and even death in humans. Therefore, clinical intervention is critical for the treatment of skin wounds. One idealized method is to use wound dressings to protect skin wounds and promote wound healing. Among these wound dressings, nanocomposite natural polymer hydrogels (NNPHs) are multifunctional wound dressings for wound healing. The combination of nanomaterials and natural polymer hydrogels avoids the shortcomings of a single component. Moreover, nanomaterials could provide improved antibacterial, anti-inflammatory, antioxidant, stimuli-responsive, electrically conductive and mechanical properties of hydrogels to accelerate wound healing. This review focuses on recent advancements in NNPHs for skin wound healing and repair. Initially, the functions and requirements of NNPHs as wound dressings were introduced. Second, the design, preparation and capacities of representative NNPHs are classified based on their nanomaterial. Third, skin wound repair applications of NNPHs have been summarized based on the types of wounds. Finally, the potential issues of NNPHs are discussed, and future research is proposed to prepare idealized multifunctional NNPHs for skin tissue regeneration.
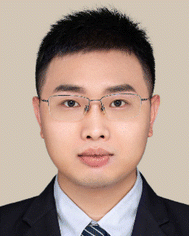 Min Wang | Min Wang received his PhD degree in materials science and engineering under the supervision of Prof. Bo Lei from Xi'an Jiaotong University. He is now working as a post-doctoral researcher at Honghui Hospital, Xi'an Jiaotong University. His research focuses on engineering multifunctional bioactive citric acid-based biomaterials for skin or cartilage tissue engineering. |
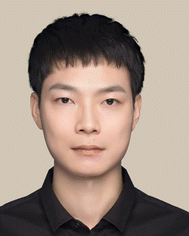 Zexing Deng | Zexing Deng received his PhD degree in materials science and engineering under the supervision of Prof. Baolin Guo from Xi'an Jiaotong University. He was also a visiting scholar at the Department of Biologic and Materials Sciences & Prosthodontics, University of Michigan. He is currently investigating conductive hydrogels for biomedical applications, and is also involved in developing biodegradable conductive scaffolds for tissue engineering. |
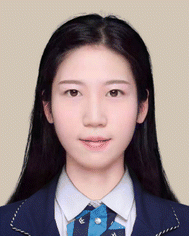 Yi Guo | Yi Guo received her PhD degree in Biomedical Engineering from Xi'an Jiaotong University. She was also a visiting scholar at the Department of Biologic and Materials Sciences & Prosthodontics, University of Michigan. She is now working as a lectorate at the Institute of Basic and Translational Medicine, Xi'an Medical University. Her research mainly focuses on developing bioactive materials for bone or muscle regeneration and cancer therapy. |
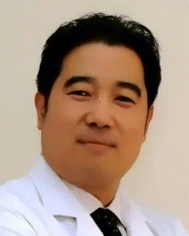 Peng Xu | Prof. Peng Xu is a first-level chief physician, professor, and doctoral supervisor. He is currently the vice president of Honghui Hospital, Xi'an Jiaotong University, president and subject leader of Arthropathy Hospital of Honghui Hospital, Xi'an Jiaotong University, and leading science and technology innovation talent of Shaanxi Province High-level Talents Special Support Program. His research is focused on the treatment of femoral head necrosis and hip-related diseases, osteoarthritis, and cartilage repair. |
1. Introduction
Skin serves as the largest organ, protects and covers us from external injury (mechanical, heat, and UV radiation), maintains temperature and moisture, excretes waste from humans and senses stimuli.1,2 However, both trauma and physiological environment can cause harm to skin tissue, making skin wounds become a common healthcare problem.3,4 Skin usually exhibits excellent regenerative properties, and normal skin wound healing involves a series of highly ordered processes including hemostasis, inflammation, proliferation, and extracellular matrix (ECM) remodeling,5–8 as shown in Fig. 1. These four stages of skin wound healing involve interactions among cells, bioactive factors, and ECM.9–13 However, some of the skin wounds are difficult to heal by themselves on account of infectious, chronic diseases and severe injuries.14–18 As a result, skin wound healing has been a major social and financial burden.
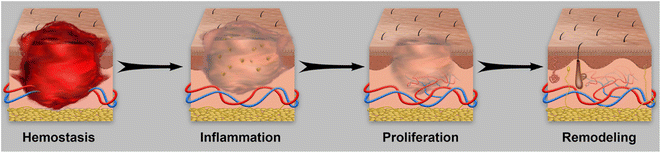 |
| Fig. 1 Illustration of four consecutive stages of wound healing. | |
The most successful strategies to date are the use of wound dressings or skin substitutes, which can serve as temporary substrates to protect the skin wound surface and provide a therapeutic environment, thereby promoting skin wound healing.19–21 Consequently, researchers have been developing various types of wound dressings or skin substitutes for many years, and trying to design and fabricate corresponding wound dressings such as nanomaterials,22,23 microspheres,24,25 films,26,27 fibers,28,29 and hydrogels30,31 through advanced technology to mimic the microenvironment of skin to promote wound healing. To date, different types of medical products such as HemCon bandage, Tegaderm™ film, and QuikClot bandage have been approved by the Food and Drug Administration (FDA) for hemostasis and wound healing.32 However, these products have limitations that cannot be ignored, including the difficulty of simultaneous application on external/internal wounds, and the external pressure when applying the product may accompany the secondary injury.5,32 To address these concerns, hydrogels have been developed by researchers for wound healing due to their good biocompatibility, moisturizing ability, similar structure to ECM and tunable functions.3 In addition, hydrogels can not only prevent bleeding of external and internal wounds because of their shape adapting ability and hemostatic performance, but also maintain the moisture environment of wounds and absorb exudate to accelerate wound healing and skin tissue regeneration.32,33
Hydrogels can be classified based on their source of raw materials, degradability, electrical conductivity, crosslinking method, and stimuli-responsive properties.34,35 According to the source of raw materials, hydrogels can be divided into natural polymer hydrogels (NPHs), synthetic polymer hydrogels, and hybrid hydrogels.36 Natural polymers coming from animals or plants include gelatin,37,38 agarose,39 collagen,40 polypeptide,41 pullulan,42 cellulose,43 chitosan,44 and hyaluronic acid45 and so on, as shown in Fig. 2. Natural polymers can be easily functionalized/modified due to their abundance of –NH2, –OH, and –COOH groups, and generally exhibit good biocompatibility, biodegradability, and gel-forming ability.46,47 Therefore, many natural polymers such as chitosan, gelatin, hyaluronic acid, alginate and dextran have been investigated for wound healing. Their functions and limitations in wound healing are summarized in Table 1.
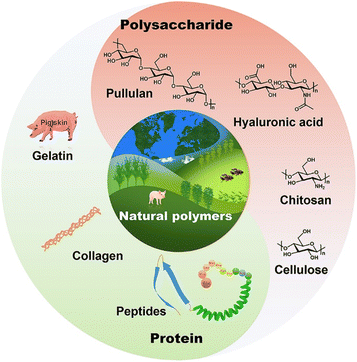 |
| Fig. 2 Illustration of representative natural polymers for building wound dressings. | |
Table 1 Functions and limitations of typical natural polymers for wound healing
Natural polymers |
Functions and advantages |
Limitations |
Ref. |
Hyaluronic acid |
Hemostasis; maintaining wound moisture |
High price |
12, 62, 68, 70, 73 and 74 |
Gelatin |
Hemostasis; promoting cell proliferation |
High price and easy degradation |
58, 62, 80, 82, 87, 98 and 102 |
Chitosan |
Hemostasis; antibacterial ability; promoting granulation tissue formation |
Slow degradation rate |
49, 57, 60, 61, 93, 172, 178 and 207 |
Alginate |
Hemostasis; maintaining wound moisture; promoting granulation tissue formation |
Low chemical stability and mechanical properties |
75, 90, 99, 102, 103 and 210 |
Dextran |
Hemostasis; promoting cell proliferation |
— |
61, 93, 95 and 96 |
Cellulose |
Hemostasis; antibacterial ability |
Slow degradation rate |
67, 84 and 203 |
However, the disadvantages of weak mechanical properties, poor antioxidant properties, and undesirable stimuli-responsive properties of NPHs greatly block their application in skin wound healing.48–51 To address these limitations, some functional nanomaterials were incorporated into the hydrogel network to improve antibacterial, antioxidant, and mechanical properties, and electrical conductivity of NPHs as wound dressings to accelerate wound healing.52,53 In addition, the combination also reduces the limitations of nanomaterials in wound healing, such as causing inflammation on wounds due to a large amount of abrupt release of nanomaterials with a small size.52,54 Moreover, some nanomaterials can be easily removed due to their undesirable adhesive performance to biological tissue.55 Representative nanomaterials commonly used in NNPHs for wound healing mainly include carbon-based,56–62 metal-based,63–70 MXene-based71–75 and silicon-based76–78 nanomaterials, as shown in Table 2 and Fig. 3. Specifically, typical carbon-based nanomaterials involve multiwalled carbon nanotubes (MWCNTs),79 pluronic F127 (F127)-dispersed MWCNTs,49 polydopamine (PDA)-coated MWCNTs,80,81 graphene oxide (GO)82 and PDA reduced graphene oxide (rGO).83 MXene-based nanomaterials contain MXene nanosheets,84 PDA-coated MXene nanosheets,73 and cerium dioxide (CeO2)-loaded MXene nanosheets.71 The representative metal-based nanomaterials include silver (Ag) nanoparticles,85–89 Ag nanowires,90 Ag nanoclusters,91 gold (Au) nanorods,92 molybdenum disulfide (MoS2) nanosheets,93–95 copper sulfide (CuS) nanoparticles,96 zinc oxide (ZnO) nanomaterials,97–99 CeO2 nanoparticles,100 and metal–organic frameworks (MOFs).101–103 Moreover, silicon-based nanomaterials include silica nanoparticles,104,105 polyhedral oligomeric silsesquioxane (POSS),106 bioactive glass nanoparticles (BGNs)107 and nanoclay.108
Table 2 Functions and limitations of typical nanomaterials for wound healing
Nanomaterials |
Functions and advantages |
Limitations |
Ref. |
Carbon-based |
Light-induced antibacterial properties; stimuli-responsive properties; electrical conductivity |
Undesirable dispersion |
12, 49, 57, 58, 60–62, 80, 82, 83, 172 and 178 |
Metal-based |
Antibacterial properties; stimuli-responsive properties; electrical conductivity |
Potential toxicity |
67–70, 85, 87, 90, 91, 93, 95–99, 102 and 103 |
MXenes-based |
Good dispersion; stimuli-responsive properties; electrical conductivity |
Dangerous chemicals during production |
71, 73–75 and 84 |
Silicon-based |
Stability; mechanical reinforcement; cost-effectiveness |
Undesirable dispersion and multifunctionality |
203, 205, 207, 209 and 210 |
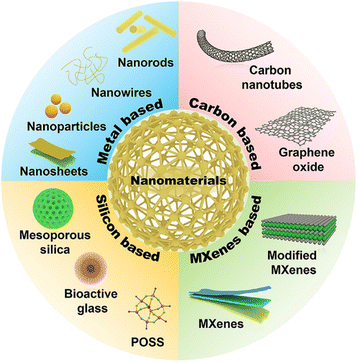 |
| Fig. 3 Classification of NNPHs based on their nanomaterials. | |
Representative preparation methods of nanocomposite hydrogels were divided into three categories: (1) nanomaterials were not grafted with chemical functional groups and then dispersed in hydrogel precursor solution, followed by physical crosslinking to form nanocomposite hydrogels. For example, Haraguchi et al. first used nanoclay as a physical crosslinker to fabricate nanocomposite hydrogels with superior mechanical performance in 2002, which was a significant study for nanocomposite hydrogels;109 (2) nanomaterials are embedded in the hydrogel matrix through physical interactions including hydrogen bonding, hydrophobic interactions, and electrostatic interactions;110–112 (3) nanomaterials are first functionalized and loaded into the hydrogel matrix through chemical interactions, including imine bonds (Schiff-base interaction), disulfide bonds, and borate–catechol.32 The physical interaction and chemical interaction between natural polymers and nanomaterials are shown in Fig. 4.
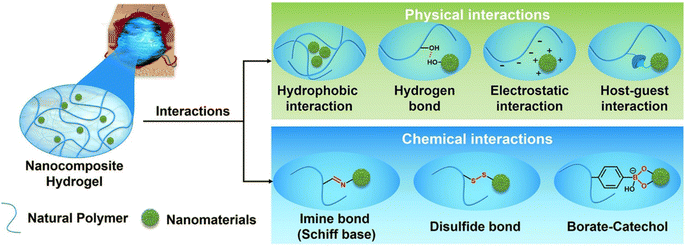 |
| Fig. 4 Illustration of representative interactions between natural polymers and nanomaterials. | |
Numerous achievements of NNPHs for skin wound healing have been made by scientists and researchers in recent years. This review highlights and focuses recent publications related to skin wound healing of NNPHs. Initially, the functions and requirements of NNPHs as wound dressings are introduced and discussed. Second, NNPHs are classified based on the types of nanomaterials. Finally, the potential issues of NNPHs are concluded, and future research is proposed to prepare idealized multifunctional NNPH wound dressings.
2. Functions and requirements of NNPHs as wound dressings for skin wound healing
In this section, the functions and requirements of NNPHs as wound dressings are introduced and discussed in detail, including biocompatibility, hemostatic and adhesive performance, antibacterial properties, anti-inflammatory and antioxidant properties, moisturizing ability and air permeability, stimuli-responsive properties, electrical conductivity, and self-healing, as shown in Fig. 5.
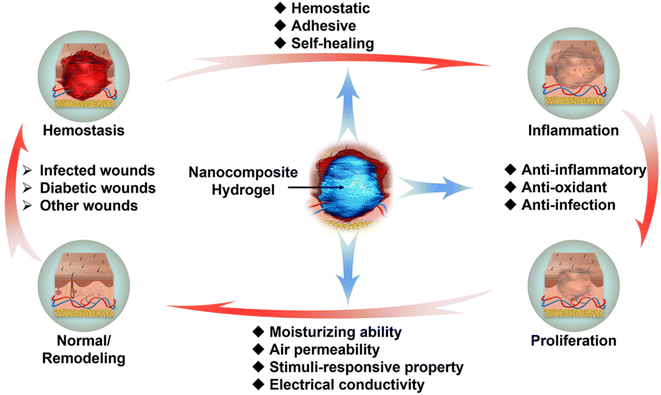 |
| Fig. 5 Illustration of functions and requirements of NNPHs as wound dressings for four consecutive stages of wound healing. | |
2.1 Biocompatibility
As biomaterials, the basic requirement of NNPHs should be biocompatibility so that they would not cause adverse effects on the human body.113 The composition, structure, surface properties, stability, and mechanical properties can affect the biocompatibility of NNPHs.114–116 NNPHs serve as wound dressings that come into contact with healthy human skin, damaged tissue and blood, and they might cause inflammation, hyperplasia, thrombosis, and calcification in surrounding tissues and blood.117 Therefore, it is significant to evaluate the biocompatibility including histocompatibility, cytocompatibility and hemocompatibility of NNPHs before clinical trials. The good histocompatibility of NNPHs ensures that they will not cause severe toxicity, inflammation, or immune response during use.118 The good cytocompatibility of NNPHs means that they can maintain cell communication, viability, proliferation, and differentiation.119,120 Blood plays an important role in the transport of nutrients, oxygen, and waste, so it is necessary to maintain the stability of the blood environment.121,122 The good blood compatibility of NNPHs requires that NNPHs will not cause damage to blood, such as changing the composition of blood and destabilization of the blood environment, leading to blood coagulation.123,124 It is worth noting that the composition of blood is very complex, including blood cells, genetic components, proteins, electrolytes, enzymes, and nutrients, so NNPHs should also undergo strict in vivo blood compatibility evaluation before clinical use.125,126
2.2 Hemostatic and adhesive performance
Hemostasis is the first stage of wound healing. Specifically, hemostasis controls bleeding at the injury site, closing ruptured blood vessels through the formation of blood clots, thereby preventing bleeding.127–129 When injury occurs, platelets in the blood aggregate and become activated at the bleeding site, and subsequently participate in all stages of hemostasis including: (1) activated platelets secreting chemicals that induce vasoconstriction, thereby reducing blood loss; (2) activated platelets forming adhesions with each other and clumping together to form hemostatic platelet plugs and attracting other nearby platelets through secreted substances to accelerate the formation and spread of hemostasis by forming a positive feedback loop; (3) the surface of activated platelets acting as coagulation sites to form blood clots and stop bleeding.5,121,130
NNPH dressings with hemostatic effects have positive effect on wound healing. Studies have shown that hemostatic properties of NNPHs can be achieved not only by physical sealing, but also by enriching coagulation factors through the absorption of wound exudates.49 Notably, adhesive NNPHs can be attached to the wound site seamlessly, which can avoid the potential infection risk caused by the external environment. Moreover, they can also seal the wound quickly to achieve hemostasis.131,132 Moreover, in the past 10 years, inspired by the excellent dry and wet adhesive properties of mussels, biomimetic NNPHs were designed and fabricated to mimic the 3,4-dihydroxyphenyl chemical structure of mussel foot proteins, which was considered to be the origin of strong adhesiveness due to strong interfacial interactions with skin tissues through hydrogen bonding, cation–π interactions, and Schiff-base bonding.133 In addition to mussel inspired chemistry, Schiff-base interactions between the aldehyde groups of NNPHs and –NH2 groups of native skin tissues are believed to be another adhesive mechanism.5
2.3 Antibacterial properties
Although bacteria are a normal part of the skin and wound, it has been suggested that a value of 105 bacteria should be considered as a critical point between normal and clinical infections.3,134,135 Bacteria can cause persistent inflammation at the site of infection after wound infection, delaying the wound healing process, and severe inflammation can even result in non-healing wounds and serious complications.136,137 Physical or chemical methods have been applied to kill or hinder the growth of bacteria, and reduce their activity.137,138 Owing to the abuse of antibiotics, drug-resistant bacteria induced wound infection is a great challenge for wound healing.139 Although clinical antibacterial drugs can control infection effectively, the drug resistance of bacteria is becoming an increasing problem.135 Thence, it is of great significance to propose better antibacterial strategies. Integration of bioactive antibacterial components in NNPHs has been utilized to address drug resistance of bacteria and accelerate wound healing.17,48 Representative bioactive antibacterial materials include metal-based nanomaterials (Ag, Zn, Au, and Cu, etc.),140,141 photothermal antibacterial agents (PDA, CNTs, and GO, etc.),142,143 photodynamic antibacterial agents (MoS2 and TiO2, etc.),144,145 antibacterial polymers (chitosan, polyethyleneimine (PEI) and ε-polylysine (EPL), etc.),146–149 and extracts from natural products (honey and essential oil, etc.).150,151
2.4 Anti-inflammatory and antioxidant performances
The second stage of wound healing is inflammation.152 Inflammatory cells would kill bacteria or other harmful agents, followed by healing injured wounds.153 Inflammation causes redness, pain, warmth, and swelling, and can lead to an increase and accumulation of reactive oxygen species (ROS), which cause damage to skin tissue.154 However, low levels of ROS are believed to have a positive effect on wound healing.155 Antioxidant agents can consume accumulated ROS and restrain the production of ROS, reducing inflammation to protect cells and promote wound repair.156 The representative natural antioxidants are mercaptan compounds, vitamins, and enzymes.157
2.5 Moisturizing and air permeability
Gauze is often used as a wound dressing to simply cover the wound for traditional wound healing in a dry environment.158 Because gauze cannot prevent the evaporation of water, the wound surface would be dehydrated easily, causing large numbers of cell death and scab of wound, which cannot provide an ideal environment for wound healing.159 In addition, gauze cannot effectively prevent bacterial infection, and it can cause pain when changing gauze due to its adherence to granulation tissue, which even causes multiple injuries and hinders the wound healing process.160–162
Since scientists first discovered that wounds show a faster healing rate in a wet environment than in a dry environment in 1962, many scientific studies and clinical trials have been carried out to verify the wound healing mechanism in a wet environment.163 Research has shown that wound healing in a wet environment can mimic and maintain the physiological state of wounds, which can prevent wounds from drying up, avoid scab formation and scarring, and reduce tissue necrosis.164 In addition, it is beneficial to maintain the enzyme activity in wound exudate, clear necrotic tissue, stimulate growth factor activity, promote angiogenesis, and fibroblast and epithelial cell growth, accelerate epithelialization and collagen generation, reduce bacterial infection, maintain wound temperature, and reduce pain, and finally accelerate wound healing.165 NNPHs are one of the wet wound dressings, which could maintain a moist healing environment and absorb exudate for wound healing.
Apart from their moisturizing ability, the air permeability of NNPHs is also important for skin tissue regeneration because healthy skin possesses respiratory functions that can absorb oxygen and release carbon dioxide.166 While oxygen plays an important role in skin repair and regeneration processes, oxygen can interact with a variety of cytokines, promote cell proliferation, participate in the synthesis of collagen, and accelerate angiogenesis and epithelialization.167 Oxygen supply affects the rate of wound healing. Researchers have reported that oxygen-permeable dressings can significantly promote wound healing compared to oxygen-impermeable dressings.168 NNPHs were engineered to create a moist environment with gas exchange performance mimicking the physiological environment of wounds.
2.6 Stimuli-responsive properties
Stimuli-responsive properties of NNPHs, which can respond to environmental stimuli such as temperature, light, and pH are also an interesting function for wound healing.35,169–171 This section mainly highlights the heat, light, and pH responsiveness of NNPHs because of their interesting performance in smart wound dressings.
Some polymer solutions can undergo a phase transition from sol to gel at the physiological temperature of 37 °C, and they can be injected into wound and form hydrogels immediately, which greatly simplifies the application of wound dressings and makes treatment simple and convenient.83 On the other hand, some thermoresponsive hydrogels exhibit shrinking behavior at body temperature, causing pre-shrink stress of skin tissue to promote wound closure and healing.172 The typical thermoresponsive polymers include agar, gelatin, poly(N-isopropylacrylamide) and F127.173
A variety of nanomaterials (MXenes, Au nanomaterials, MWCNTs, rGO, and PDA nanomaterials) can demonstrate photothermal properties, which can convert light to heat.174–176 Taking advantage of this phenomenon, NNPHs could enhance their antibacterial performance by scavenging bacteria at high temperature for photothermal therapy.175–177 In order to avoid damage to the skin due to photothermal therapy at high temperature, photodynamic therapy was also proposed, where photosensitizers such as TiO2 and ZnO nanomaterials could transform photons to ROS and free radicals for photodynamic therapy at normal temperature.4 It should be noted that the ROS level for photodynamic therapy should be on-demand controlled, because overloaded ROS might have a negative impact on wound healing.4
The pH responsiveness of NNPHs is also involved in wound healing owing to pH changes during infected and/or diabetic wound tissue healing processes; for example, the pH of healed uninfected wounds is between 5.5 and 6.5, while the pH of unhealed infected wounds is higher than 7.0.3,178 The typical pH responsive natural polymers of NNPHs are hyaluronic acid, chitosan, and sodium alginate on account of rich –NH2 and/or –COOH groups on their structures.179 Scientists usually load drugs in pH responsive NNPHs for pH-controlled drug release for wound healing.
2.7 Electrical conductivity
Electrically conductive NNPH dressings are beneficial for wound healing and repair, because skin tissue is electrically conductive, enabling function and communication of skin.50 In addition, the proliferation and differentiation of cells is associated with the electrically conductive environment.180,181 As mentioned before, the third stage of wound healing is proliferation, which can be verified by granulation tissue formation, where newly granulation tissue will replace damaged tissue.3 Lots of nanomaterials such as MXenes, nanoscale metallic materials, nanoclay, CNTs, and rGO are electrically conductive, and adequate studies have shown that electrically conductive nanomaterials can optimize the electrical conductivity of NNPHs.50
2.8 Self-healing properties
Another requirement of NNPHs is to have good self-healing ability to avoid structural and functional failure under external tension and pressure at the wound.182 NNPHs with self-healing properties were carefully designed and fabricated to achieve structural integrity.183 NNPHs can heal the network structure by themselves to maintain their functions and protect wounds from the outside invasion of harmful components.184 To date, the self-healing chemistry of NNPHs has focused on physical interactions and/or chemical interactions; for instance, hydrogen bonding, electrostatic interactions, π–π interactions, host–guest interactions and hydrophobic interactions are representative physical interactions, while Schiff-base bonds, S–S bonds and borate bonds are typical chemical interactions.185
All in all, specific functions and requirements for NNPHs were summarized. Although it is a challenge to integrate all the functions in one system of NNPH, researchers have been dedicated to preparing multifunctional NNPH platforms for idealized wound dressings in the past 10 years.
3. Preparation and performance of NNPHs according to their nanomaterials
In this section, the design, preparation and capacities of representative NNPHs based on different nanomaterials for skin wound healing and repair are highlighted. The categories of NNPHs are classified as carbon-based, metal-based, MXene-based and silicon-based, as demonstrated in Tables 3–6 according to recent studies.
3.1 Carbon-based NNPHs for wound healing
Due to the decent biocompatibility, and photothermal and electrical conductivity of CNTs, GO and rGO, they have been widely applied in NNPHs for wound healing.3 Representative research on carbon-based NNPHs for wound healing is summarized in Table 3. CNTs, tubes composed of carbon with a nanometer diameter, high electrical conductivity, astonishing tensile strength and modulus, and desirable thermal conductivity, have attracted much interest in the biomedical field, including wound healing.186–188 However, the hydrophobic and insoluble limitations of CNTs lead to poor dispersion in various solvents.81 Therefore, NNPHs with homogeneous performance were fabricated by chemical modification with hydrophilic groups such as –OH, –COOH, and –NH2 or using surfactants such as F127 to increase the dispersion of CNTs.57,80 For example, He et al. prepared an NNPH network through a Schiff-base reaction using aldehyde F127-dispersed CNTs and N-carboxyethyl chitosan for infected full-thickness wound healing (Fig. 6A and B). The NNPH exhibited the highest electrical conductivity of 8.45 × 10−3 S m−1, the largest adhesive strength of 8.5 kPa, photothermal antibacterial properties with 100% of killing ratio of bacteria in just 10 minutes and pH-responsive drug release behavior to kill bacteria, and the lowest blood loss of 143.9 ± 60.9 mg, 140 ± 39.7 mg, and 207 ± 19.3 mg for the mouse liver trauma model, mouse tail cutout model, and mouse liver incision model, respectively. Besides, the multifunctional NNPH was further applied in bacterial infected skin wound healing, where bacteria can cause persistent inflammation at the infected site and postpone wound healing. The macroscopic wound contraction of NNPHs was 100% after 14 days of healing. Microscopic wound healing results were also presented, and the thicknesses of granulation tissue and epithelium were 778 μm and 110 μm after 7 days of healing, respectively. After 14 days of healing, the dermis was formed, and blood vessels, hair follicles, etc., in the NNPH group were similar to those of normal healthy skin.57 In addition, Liang et al. optimized the dispersity and bioactivity of CNTs by coating with PDA, and further prepared the NNPH using dopamine functionalized gelatin, chitosan and PDA coated CNTs through self-polymerization. The NNPH displayed antioxidant properties by scavenging 86.5% and 100% of free radicals when the concentration of NNPH was 3 mg mL−1 and 5 mg mL−1, respectively. Moreover, NNPHs also showed electrical conductivity from 0.062 to 0.072 S m−1, adhesive strength from 3.9 kPa to 6.5 kPa, the lightest blood loss of 170 mg in the liver bloodletting model, and photothermal antibacterial properties with a killing ratio of nearly 100% in just 5 minutes. Furthermore, the infected skin wound healing study was also performed, where a significant wound closure and granulation tissue thickness could be observed in the NNPH group after 7 and 14 days of healing. Histomorphological observation was further performed to evaluate wound healing, and the NNPH group demonstrated obvious dermis and epidermis tissue regeneration.80
Table 3 Composition of NNPHs based on carbon-based nanomaterials for wound healinga
Nanomaterials |
Polymers and/or monomers |
Wound healing model |
Healing period |
Ref. |
rGO: reduced graphene oxide, PDA: polydopamine, HA: hyaluronic acid, DA: dopamine, PF127: poly(ethylene oxide)–poly(propylene oxide)–poly(ethylene oxide), CNTs: carbon nanotubes, QCSG: glycidyl methacrylate functionalized quaternized chitosan, CEC: N-carboxyethyl chitosan, GelMA: gelatin methacryloyl, AM: acrylamide, GO: graphene oxide, CD: β-cyclodextrin, QCS: quaternized chitosan, AD: amantadine, CQD: carbon quantum dots, CMCS: carboxymethyl chitosan, ODex: oxidized dextran, BNN6: N,N′-di-sec-butyl-N,N′-dinitroso-1,4-phenylenediamine, GT: gelatin, CS: chitosan, EPL: ε-polylysine, NIPAM: N-isopropylacrylamide, CS–DHCA–LAG: chitosan–dihydrocaffeic acid–L-arginine, PEG: polyethylene glycol, PEGS–PBA–BA: PEG2000–sebacic acid–glycerol–phenylboronic acid–benzaldehyde.
|
rGO@PDA |
HA–DA |
Full-thickness skin defect wound |
14 days |
12
|
PF127/CNTs |
QCSG and PF127 |
Full-thickness skin defect wound |
15 days |
49
|
PF127/CNTs |
CEC and PF127 |
S. aureus infected wound |
14 days |
57
|
GelMA/CNTs |
GelMA and AM |
S. aureus infected wound |
12 days |
58
|
GO–CD |
QCS–CD and QCS–AD |
Full-thickness skin defect wound |
14 days |
60
|
CQD |
CMCS and ODex |
S. aureus infected wound |
14 days |
61
|
GO–β-CD–BNN6 |
GelMA and HA–DA |
S. aureus infected wound |
14 days |
62
|
PDA@CNTs |
GT–DA and CS |
S. aureus infected wound |
14 days |
80
|
GO |
QCSG and GelMA |
MRSA infected wound |
14 days |
82
|
rGO@PDA |
F127–EPL |
Diabetic wound |
21 days |
83
|
rGO@PDA |
QCS and NIPAM |
Full-thickness skin defect wound |
14 days |
172
|
rGO@PDA |
CS–DHCA–LAG and PEGS–PBA–BA |
Diabetic foot wound |
21 days |
178
|
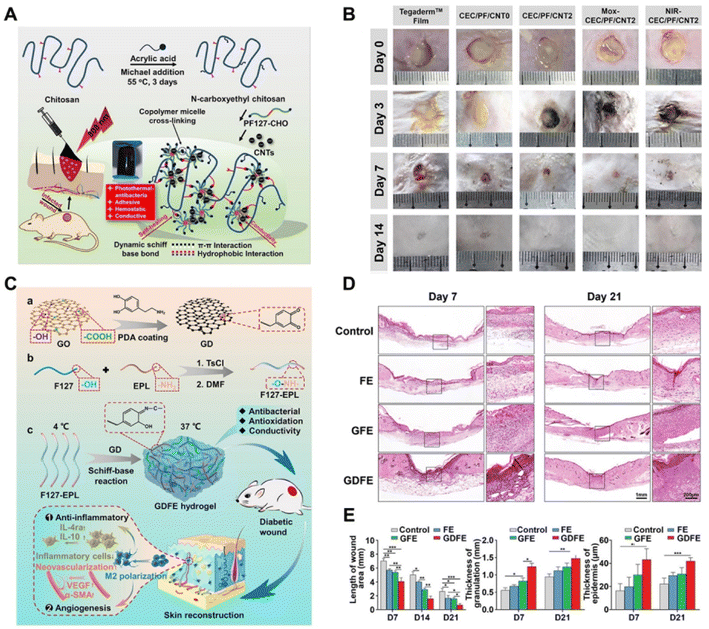 |
| Fig. 6 Carbon-based NNPHs for wound healing. (A) Synthesis schematic of a CNT-based NNPH for wound healing; (B) the photograph of wound closure on the 0th, 3rd, 7th and 14th days;57 Copyright 2020, Elsevier. (C) Synthesis schematic of a GO-based NNPH for diabetic wound healing; (D) hematoxylin–eosin staining of wound on the 7th and 21st days; (E) quantification of length, granulation and epidermis thickness of wounds.83 Copyright 2021, John Wiley and Sons. | |
Another fascinating carbon-based nanomaterial is graphene, research on which was awarded the Nobel Prize in 2010; graphene is made of carbon atoms with a two-dimensional hexagonal lattice structure.189 Due to its high electrical conductivity, mechanical strength, and thermal conductivity, graphene is an ideal candidate for application in composite materials, biomedical engineering, electronic industry, and so forth.190 However, graphene suffers from limitations such as poor water solubility, non-biodegradability, potential long-term toxicity, and inefficient preparation processes.189 To overcome these challenges, researchers fabricated GO and rGO to ameliorate the dispersity of graphene related nanomaterials in water and solvents.82,119 For instance, Liang et al. prepared an injectable NNPH through free radical polymerization using well-dispersed GO, methacrylate gelatin and methacrylate quaternized chitosan to promote the MRSA-infected mouse full-thickness wound healing. The NNPH had electrical conductivity from 0.0097 to 0.1007 S m−1, inherent antibacterial properties against normal bacteria with a 95% killing ratio and drug resistant bacteria with a >90% killing ratio, and photothermal antibacterial properties against all types of bacteria with a 100% killing ratio after exposure to NIR light for 10 minutes. A skin wound healing model for drug-resistant bacterial infection was studied, in which wound closure and collagen content in the NNPH group were significantly better than those in the control group. Besides, obvious hair follicles and blood vessels could be observed after 14 days in the NNPH group, indicating the repair of skin tissue.82 GO with hydrophilic functional groups increased its dispersity but sacrificed its electrical conductivity; thus, reduced GO (rGO) was fabricated to optimize its electrical conductivity without affecting its dispersity.3 Coating PDA on GO is a typical method to prepare rGO with antioxidant performance and excellent dispersity in water.83,172 For example, Li et al. prepared an NNPH using PDA coated GO, quaternized chitosan, and N-isopropylacrylamide. The NNPH exhibited self-healing performance within 2 hours through Schiff-base dynamic chemical interactions, hydrogen bonding and cation–π physical interactions, antioxidant performance with 98% free radicals consumed with only 5 mg mL−1 of NNPH, electrical conductivity of 5.0–5.6 mS cm−1, innate antibacterial performance with a killing ratio more than 85% for Staphylococcus aureus (S. aureus) and Escherichia coli (E. coli), NIR light induced antibacterial performance with entirely killing of bacteria within 10 minutes, decent in vitro hemocompatibility with a small hemolysis ratio of ∼1.6, good air permeability compared to Tegaderm™ film, and an adhesive strength of 9.68 kPa. Interestingly, the NNPH was built for temperature induced wound closure at 37 °C because of the self-shrinking behavior of PNIPAM with 51% of the wound area contracted on the second day, while the control group had only 20% of the contracted wound area. In addition, an acute full-thickness skin defect model was further studied for wound healing. Conventional acute wound models are usually similar to surgery or trauma wounds, where wounds occur and heal in just a few days or weeks. In addition, the NNPH group showed complete wound closure on day 14, while wounds in the control group were still not 100% closured.172
In addition to acute wound healing and chronic infected wound healing, a NNPH with PDA coated rGO was also synthesized for chronic diabetic wound healing, in which wounds would take a longer time to repair for diabetic patients.83,178 Microvascular dysfunction caused by hyperglycemia in diabetic patients can lead to wound ischemia and delayed healing, which has become a serious threat to the health of patients.149 Therefore, it is important to propose advanced strategies to accelerate diabetic wound healing.83 Among diabetic wounds, diabetic foot wounds commonly occur in diabetic patients, usually at the bottom of the foot.178 For example, Liang et al. prepared an NNPH using dihydrocaffeic acid and L-arginine grafted chitosan, benzaldehyde groups and phenylboronic acid functionalized sebate and PDA coated rGO through the double dynamic bonds of Schiff base and phenylboronate ester for athletic diabetic foot wound healing. The NNPH demonstrated desirable biocompatibility and biodegradability (<30% of remaining weight after 15 days of degradation), water absorption (a swelling ratio of 1081%), self-healing properties, on-demand removal ability (NNPH can be removed using solutions containing acid and/or glucose), pH and glucose dual responsive characteristics (highest metformin release at a pH of 5.5 and better metformin release within glucose), electrical conductivity (∼10−1 S m−1), antibacterial properties (killing ratio of >99% with drug resistant bacteria), adhesive and hemostatic ability (an adhesive strength of 17.51 kPa), and anti-oxidation properties (>90% of free radicals eradicated with a NNPH concentration of 2 mg mL−1, and nearly complete free radicals consumed when NNPH concentration is 4 mg mL−1). In addition, the synthesized NNPH exhibited an obvious positive effect on diabetic foot wound healing, where 98% of the wound was closured after 3 weeks of healing. In addition, the thickest granulation tissue, best angiogenesis results, and highest number of follicles could be found in the NNPH group.178 Apart from diabetic foot wound healing, Tu et al. prepared an injectable and self-healing NNPH at 37 °C using EPL grafted F127 and PDA coated GO through a Schiff base reaction for diabetic wound healing (Fig. 6C and E). The NNPH possessed antioxidant properties with an antioxidant rate of 92%, broad-spectrum antibacterial capacity with a 99% killing ratio for E. coli, S. aureus, and methicillin-resistant Staphylococcus aureus (MRSA), electrical conductivity of 0.04 S m−1, and promoting M2 polarization of macrophages and proliferation, migration, and angiogenesis of endothelial cells. In addition, the NNPH can promote the full-thickness diabetic wound repair with a fastest period of 17.5 days for completed wound closure.83
3.2 Metal-based NNPHs for wound healing
Ag, Au, ZnO, TiO2, MoS2, CuS, and ZnS based nanomaterials are representative materials for synthesizing NNPHs for wound healing because of their biocompatibility, electrical conductivity, photothermal and/or photodynamic properties and antibacterial performance.3,93,95,191 The typical studies of metal-based NNPHs for wound healing are shown in Table 4.
Table 4 Composition of NNPHs based on metal-based nanomaterials for wound healinga
Nanomaterials |
Polymers and/or monomers |
Wound healing model |
Healing period |
Ref. |
CMC: carboxymethyl cellulose, NPs: nanoparticles, CMCS: carboxymethyl chitosan, OHA: oxidized hyaluronic acid, NCs: nanoclusters, SA: sodium alginate, NRs: nanorods, HA–AL: hyaluronic acid–alendronate sodium, PF127: poly(ethylene oxide)–poly(propylene oxide)–poly(ethylene oxide), FA: fumaric acid, GT–DA: gelatin–dopamine, GG: guar gum, MA-ALG: methacrylated alginate, MF: mangiferin, Lip: liposome, CS: chitosan, PEG: polyethylene glycol, BSA: bovine serum albumin, ODex: oxidized dextran, TA: tannic acid, PVA: poly(vinyl alcohol), QDs: quantum dots, GelMA: gelatin methacryloyl, CSO: chitosan oligosaccharide, ZIF: zeolitic imidazolate framework, OSA: oxidized sodium alginate, GelMA-CDH: gelatin methacryloyl-carbohydrazide, PCN: zirconium-based porphyrin metal–organic frameworks, CG: cationic guar gum.
|
Ag/Ag@AgCl/ZnO |
CMC |
Full-thickness skin defect wound |
14 days |
67
|
Au–Pt NPs |
CMCS and OHA |
Diabetic wound |
15 days |
68
|
MoS2 NCs |
SA |
Diabetic wound |
14 days |
69
|
Eu2O3 NRs |
HA–AL and PF127 |
Full-thickness skin defect wound |
14 days |
70
|
Ag NPs |
Agar and FA |
Full-thickness skin defect wound |
16 days |
85
|
Ag NPs |
GT–DA and GG |
S. aureus infected wound |
10 days |
87
|
Ag nanowires |
MA-ALG |
E. coli and S. aureus infected wound |
2 days |
90
|
Ag NCs and MF@Lip |
CS and PEG |
S. aureus infected wound |
8 days |
91
|
MoS2@Au@BSA |
ODex and glycol chitosan |
Diabetic wound |
8 days |
93
|
MoS2@TA/Fe |
ODex and PVA |
S. aureus infected wound |
8 days |
95
|
CuS NPs |
ODex and PEG with amino end groups |
Full-thickness skin defect wound |
14 days |
96
|
ZnO QDs@GO |
CS |
S. aureus infected wound |
14 days |
97
|
Tetrapodal ZnO |
GelMA |
Full-thickness skin defect wound |
14 days |
98
|
ZnO NPs |
SA and CSO |
Deep second-degree scald wound |
19 days |
99
|
Au@ZIF-8 |
OSA and GelMA-CDH |
S. aureus infected wound |
14 days |
102
|
Au NCs@PCN |
PVA and ALG |
Diabetic wound |
21 days |
103
|
PDA@Ag |
CG |
S. aureus infected wound |
12 days |
191
|
Ag-based nanomaterials have been used in biomedical applications for many years due to their antibacterial, anticancer, and accelerated wound healing performances.191 Qi et al. fabricated an NNPH using Ag-decorated polydopamine nanoparticles and cationic guar gum to combat bacterial infection and promote wound healing. The addition of Ag can effectively improve the photothermal conversion efficiency of PDA nanoparticles. The NNPH displayed self-healing and injectable properties, excellent photothermal-assisted antibacterial properties with >99% of E. coli and S. aureus being eliminated after exposure to NIR light for 3 minutes, good hemocompatibility and cytocompatibility with a hemolysis ratio less than 5% and ∼100% cell viability, respectively. Furthermore, the bacteria-infected skin wound model was used to study the wound healing efficiency of the NNPH. The NNPH with the assistance of NIR light (NNPH + NIR) possessed superior bactericidal activity and a rapid wound healing rate with wound closure after 12 days. Besides, the wounds treated with NNPH + NIR also showed the most collagen deposition and the highest CD31 expression but the lowest IL-6 expression, indicating that the NNPH + NIR can accelerate the healing of infected wounds.191 In addition, other precious metal Au-based nanomaterials were also developed for skin wound healing because of their chemical stability, biocompatibility and photothermal properties.68
MoS2, ZnS, CuS, ZnO, and TiO2 based nanomaterials are typical metal oxides and metal sulfides that have been prepared to develop NNPH platforms for wound healing on account of their photodynamic properties and/or photothermal properties, antibacterial properties, and promoting ability.63,95–99 Li et al. constructed a multifunctional NNPH platform based on tannic acid-chelated Fe-decorated MoS2 nanosheets with dual enzyme activities and a multifunctional hydrogel including polyvinyl alcohol (PVA), dextran (Dex) and borax for bacteria-infected wound healing. The MoS2@TA/Fe nanosheet had a peroxidase-like ability to catalyze H2O2 to ·OH under an acid environment and catalase-like ability to catalyze H2O2 to O2 under a neutral environment. The NNPH showed excellent antibacterial properties with a 100% killing ratio through the combined effect of ·OH, loss of glutathione, and photothermal therapy. The NNPH also showed self-healing properties and shape adaptability due to dynamic boron ester bonds and hydrogen bonds, adhesive properties because of abundant phenolic hydroxyl groups, good blood compatibility and cell compatibility with low hemolytic ratio and high cell viability in vitro, anti-inflammatory performance with high expression of IL-10 and low expression of TNF-α in vitro, antioxidant performance with scavenging of >80% reactive oxygen species (ROS) and reactive nitrogen species (RNS) with 15 mg mL−1 of MoS2@TA/Fe nanosheets. Finally, S. aureus-infected wounds were created to investigate the ability of the NNPH to promote wound healing. With the assistance of H2O2 and NIR light, there were negligible bacteria in the NNPH group, which exhibited an excellent ability to prevent infection in vivo. In addition, the NNPH demonstrated the best wound healing after 8 days, displaying the smallest wound area, mature epidermal layer formation, more well-defined collagen fibers, highest expression of VEGF and IL-10, and lowest expression of TNF-α in vivo.95 Furthermore, Li et al. further designed an injectable NNPH using defect-rich MoS2 nanosheets (MoS2@Au@BSA) loaded with bovine serum albumin (BSA) modified with Au nanoparticles and a hydrogel prepared using oxidized dextran and glycol chitosan for diabetic wound healing through an oxygen self-supplying cascade reaction (Fig. 7). The MoS2@Au@BSA nanosheets had catalytic abilities similar to those of glucose oxidase, which is able to oxidize glucose to H2O2 and gluconic acid, along with the peroxidase-like and catalase-like abilities of MoS2. Compared to the previously mentioned work, this NNPH could eradicate bacteria and promote infected diabetic wound healing in just 8 days without the help of external H2O2 and NIR light due to the accumulated glucose in the diabetic wounds being automatically converted to H2O2.93 ZnO based nanomaterials are another well-known nanofillers to develop NNPHs for wound healing due to their biocompatibility, antibacterial properties, and photoactive properties.98 In addition, Zn elements have vital significance for cell functions, including growth, metabolism, immunity, and so on.192 For example, Siebert et al. prepared a ZnO based 3D printable NNPH using vascular endothelial growth factor (VEGF) modified antibacterial tetrapodal ZnO and methacrylated gelatin (GelMa). The 3D-printed NNPH demonstrated excellent cytocompatibility of endothelial cells due to the sustainable release of VEGF and antibacterial performance against S. aureus and P. aeruginosa mainly due to the ROS release. The 3D-printed NNPH also promoted angiogenesis with the highest expression of the marker CD31 after 4 weeks because of the combined effect of VEGF and Zn2+. Finally, the 3D-printed NNPH can inhibit inflammation and promote angiogenesis and cell proliferation to accelerate wound healing. After 2 weeks, the 3D-printed NNPH group showed the smallest wound size with only 3.48% of wound area remaining, the thickest epidermis with 101.2 μm of thickness, and the densest dermis with 95.3% per size.98
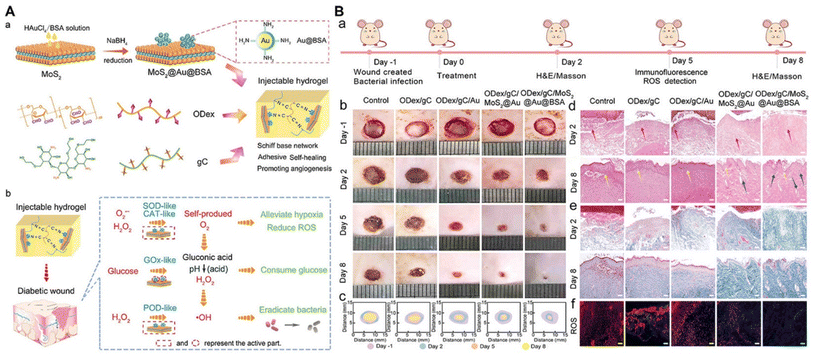 |
| Fig. 7 Metal-based NNPHs for wound healing. (A) Synthesis schematic of a MoS2-based NNPH for wound healing; (B) the photograph of wound closure and the histologic analyses of wound on the 2nd and 8th days.93 Copyright 2022, John Wiley and Sons. | |
Metal–organic frameworks (MOFs), a class of hybrid materials, consist of central metals and organic struts.193 MOF-based nanomaterials have ultra-high surface areas, high porosities with uniform pore size, and superior thermal stability.193 Due to the bactericidal ability of metal ions such as Ag+, Zn2+, and Cu2+, NNPHs with MOF-based nanomaterials serving as nanofillers have also been studied for wound healing applications.194–196 Deng et al. reported an injectable biomimetic NNPH using metal–organic frameworks (MOFs) with encapsulated Au nanoparticles (Au@ZIF-8) and hydrogels prepared using carbohydrazide-modified GelMa and oxidized sodium alginate through a Schiff-base as well as photo crosslinking. The NNPH displayed photodynamic bactericidal performance, with >99.0% of bacteria eradicated after light exposure (generating ROS) for 20 minutes. Besides, in vivo S. aureus infected wound healing studies were conducted to test the wound healing efficiency of NNPHs, where 97.6% of wound area was closured for the NNPH group after 2 weeks.102
Taken together, metal-based nanomaterials are good candidates for the manufacturing of multifunctional NNPHs. To achieve integrated functions and performances such as dispersion, photodynamic/photothermal properties, and antibacterial properties, for different types of wound healing, they were elaborately designed and often chemically modified with other functional components. However, there remain potential challenges and limitations for metal-based nanomaterials in wound healing applications due to their potential toxicity.197
3.3 MXene-based NNPHs for wound healing
MXenes are a class of 2D inorganic materials consisting of carbides and/or nitrides with transition metals.198 Compared to the hydrophobicity of previously mentioned nanomaterials, MXenes have desirable hydrophilicity due to the presence of hydrophilic –OH groups on their surface, as well as good biocompatibility, facile functionalization properties, photothermal properties, electrical conductivity and stability.199 The primary papers of MXene-based NNPHs for wound healing are shown in Table 5.
Table 5 Composition of NNPHs based on MXene-based nanomaterials for wound healinga
Nanomaterials |
Polymers and/or monomers |
Wound healing model |
Healing period |
Ref. |
OSA: oxidized sodium alginate, PF127–PEI: poly(ethylene oxide)–poly(propylene oxide)–poly(ethylene oxide)–polyethyleneimine, PDA: polydopamine, OHA: oxidized hyaluronic acid, GTA: glycerol modified with –C C–, HA–DA: hyaluronic acid–dopamine, MNPs: Fe3O4@SiO2 magnetic nanoparticles, NPs: nanoparticles, PNIPAM: poly(N-isopropylacrylamide), ALG: alginate, rBC: regenerated bacterial cellulose.
|
MXene@CeO2 |
OSA and PF127–PEI |
MRSA infected wound |
14 days |
71
|
MXene@PDA |
OHA and PEI–GTA |
MRSA infected wound |
14 days |
73
|
MXene@PDA |
HA–DA |
S. aureus infected wound |
9 days |
74
|
MNPs@MXene and Ag NPs |
PNIPAM and ALG |
Deep diabetic wound |
12 days |
75
|
MXenes |
rBC |
Full-thickness skin defect wound |
14 days |
84
|
In recent years, MXene-based NNPHs have been extensively studied in skin wound repair.71,73,84 For example, Mao et al. developed a biodegradable and electroactive NNPH using regenerated bacterial cellulose and MXenes (Ti3C2Tx) to accelerate skin wound healing under electrical stimulation. The NNPH displayed compressive-recoverable flexible mechanical capacity, electrical conductivity from 0.00283 S m−1 to 0.0704 S m−1, and degradability with a degradation period of 210 minutes. Moreover, the full-thickness wound treated with the NNPH under electrical stimulation was closured 93.8% after 2 weeks, which exhibited the best wound closure.84 In addition, Zhou et al. prepared a conductive antibacterial NNPH using PDA coated MXenes (Ti3C2Tx), poly(glycerol-ethylenimine) and oxidized hyaluronic acid through a Schiff-base reaction to promote MRSA-infected wound healing. The NNPH exhibited excellent self-healing properties, an electrical conductivity of 2.89 S m−1, competitive bactericidal properties against E. coli, S. aureus, and MRSA with a killing ratio of ∼99.0%. The NNPH also possessed biodegradability with a degradation period of 7 days in vivo and good biocompatibility with the best cell proliferation and the highest collagen III, α-actin, and VEGF gene expression. In addition, the NNPH exhibited a wound closure rate of approximately 96% after 14 days for MRSA-infected skin wounds, and the thickest granulation tissue (∼120 μm), the best collagen deposition (∼70%) and the highest gene expression of α-actin and CD31, indicating that the NNPH can accelerate the MRSA-infected wound healing.73 Furthermore, Zheng et al. prepared an anti-inflammatory, antibacterial and conductive NNPH using CeO2-loaded MXene, PEI grafted F127 and oxidized sodium alginate for infection-impaired skin multimodal therapy. The NNPH can promote cell proliferation, cell migration and collagen deposition to accelerate multidrug-resistant (MDR) bacterial infected-wound healing.71 In addition, Li et al. developed an injectable NNPH through oxyhemoglobin/H2O2 crosslinking using dopamine-grafted hyaluronic acid and PDA coated MXene nanosheets for the mild photothermal-controlled release of oxygen to promote diabetic wound healing (Fig. 8). The oxyhemoglobin served as a catalyst to fabricate a network of NNPH and oxygen vehicles to release O2 under exposure to NIR light. The NNPH possessed tissue adhesion ability, self-healing performance induced by hydrogen bonding and π–π stacking, photothermal properties with a maximum temperature of 40.0 °C, controlled O2 release with a maximum O2 release of 22.7 mg mL−1 under exposure to NIR light, ROS and RNS scavenging performances (∼90%), and good hemostatic ability. For the infected diabetic wounds, the NNPH exposed to NIR light exhibited a maximum wound closure rate of about 98.8%, a minimum epidermal thickness of 26 μm, and the highest expression of CD31, collagen and M2 macrophages after 9 days.74
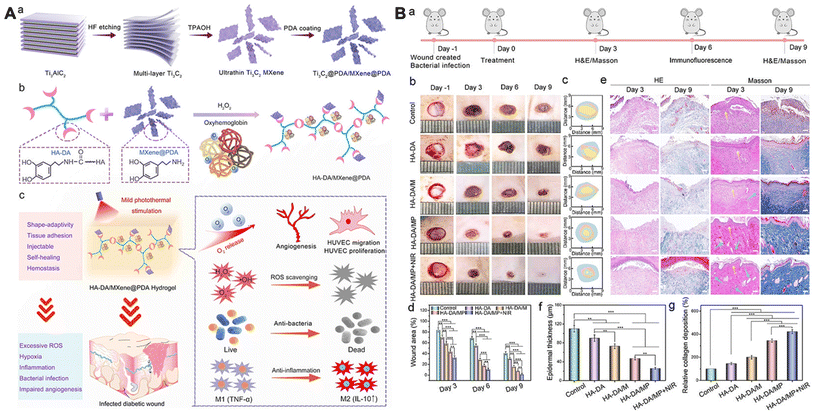 |
| Fig. 8 MXene-based NNPHs for wound healing. (A) Synthesis schematic of an MXene-based NNPH for wound healing; (B) the photograph of wound closure, the histologic analyses, epidermis thickness and collagen deposition of wound on the 3rd and 9th days.74 Copyright 2022, American Chemical Society. | |
Tremendous achievements have been made related to MXene-based nanomaterials, but there are some limitations that should be noted. The MAX etching process might bring in highly toxic and dangerous hydrofluoric acid, which can cause permanent harm and even death to lives easily. In addition, the large scale production of MXenes should be further studied. The hydrofluoric acid free etching method is highly anticipated and meaningful, but few hydrofluoric acid free methods have been reported.
3.4 Silicon-based NNPHs for wound healing
Silicon-based nanomaterials including silica, nanoclay, polyhedral oligomeric silsesquioxane (POSS), and bioactive glass nanoparticles (BGNs) have been widely applied in hemostasis, bone tissue engineering and wound healing due to their cost-effectiveness, biocompatibility, mechanical reinforcement, and stable capacities.200–202 The main studies of silicon-based NNPHs for wound healing are displayed in Table 6.
Table 6 Composition of NNPHs based on silicon-based nanomaterials for wound healinga
Nanomaterials |
Polymers and/or monomers |
Wound healing model |
Healing period |
Ref. |
MCF: mesocellular silica foam, HEC: quaternized hydroxyethyl cellulose, MATMA: 2-(methacryloyloxy)-ethyltrimethylammonium chloride, PEGDA: poly(ethylene glycol)diacrylate, Col: collagen, POSS: polyhedral oligomeric silsesquioxane, PDA: polydopamine, TCS: thiolated chitosan, BGN: bioactive glass nanoparticle, PF127–CHO: poly(ethylene oxide)–poly(propylene oxide)–poly(ethylene oxide) functionalized with –CHO groups, EPL: ε-polylysine, ALG: alginate.
|
MCF |
HEC, MATMA and PEGDA |
Bacteria infected wound |
15 days |
203
|
Nanoclay |
Col |
Diabetic wound |
21 days |
205
|
POSS@PDA |
TCS and PEGDA |
Full-thickness skin defect wound |
18 days |
207
|
BGN@PDA |
PF127–CHO and PF127–EPL |
Full-thickness skin defect wound |
14 days |
209
|
BGN–Cu |
PEGDA and ALG |
Diabetic wound |
21 days |
210
|
Wang et al. developed a bioinspired injectable NNPH using quaternized hydroxyethyl cellulose (HEC) and mesocellular silica foam (MCF) for rapid hemostasis without compression and accelerated wound healing. The NNPH possessed excellent swelling and water absorption, excellent bionic hemostatic effects, and good antibacterial properties. In addition, in full-thickness infected wounds, the NNPH can effectively inhibit bacterial infection and the inflammatory response and promote wound healing.203 Nanoclay is an ultra-fine polar nanomaterial composed of various nanoparticles, which possess diverse mineral components, and good binding ability and dispersibility compared to silica nanoparticles.204 Ordeghan et al. developed an antibacterial NNPH comprised of nanoclay, collagen and tadalafil, which can effectively accelerate the healing time of wounds and promote hair follicle formation and collagen deposition for diabetic wounds under high-intensity interval training.205 POSS is a biocompatible hybrid nanostructure comprised of silicon and oxygen, which can achieve multifunctionality through covalent chemical modification of the organic groups.206 Yang et al. developed an Ag-loaded NNPH using PEGDA, thiolated chitosan and PDA-modified POSS to accelerate wound healing. The NNPH exhibited excellent mechanical properties, and good adhesive and antibacterial properties, and facilitated cell attachment and proliferation. The NNPH can effectively inhibit the inflammatory response and promote the formation of blood vessels and granulation tissue to promote the full-thickness wound healing.207
BGN is a silicate-based inorganic amorphous nanomaterial, which possesses good hemostatic properties and angiogenesis ability, and can promote the expression of bioactive factors related to wound healing.208 Zhou et al. prepared a bioactive polypeptide-based NNPH using PDA coated BGNs, benzaldehyde-modified F127 and EPL-modified F127 through a Schiff-base reaction for the skin-tumor therapy and MRSA-infected wound healing (Fig. 9). The NNPH exhibited good injectability and self-healing ability, excellent broad spectrum antibacterial activity, and photothermal performance to inhibit tumor growth. Furthermore, the NNPH exhibited a wound closure rate of approximately 98.8% and regenerated granulation tissue of approximately 240 μm after 14 days for full-thickness skin wounds.209 Li et al. developed an antibacterial NNPH using copper-doped BGN, sodium alginate and polyethylene glycol diacrylate (PEGDA) to promote diabetic wound healing. The NNPH possessed good self-healing performance due to dynamic electrostatic interactions between Ca2+/Cu2+ and sodium alginate, excellent antibacterial capacity with an ∼100% killing ratio due to the existence of Cu2+, and enhanced endothelial progenitor cell proliferation and angiogenic ability. For full-thickness diabetic wounds, the NNPH can promote the deposition of collagen and the expression of HIF-α/VEGF to restore blood vessel networks, which further accelerated the wound healing.210 In addition, Chen et al. developed hierarchical functionalization of strontium-doped BGN nanohybrids with polypyrrole, PDA and EPL, which exhibited enhanced antioxidant, electrical conductivity, broad-spectrum antibacterial and anti-infective properties. The nanohybrids can not only promote collagen deposition and angiogenesis to accelerate normal wound healing, but also promote MRSA-infected and tumor-impaired wound healing.211 Niu et al. also prepared different element-doped BGNs, including molybdenum-doped or europium and gadolinium-codoped BGN nanoplatforms, which can also efficiently promote MRSA-infected and tumor-impaired skin regeneration.212 Therefore, NNPHs based on functionalized modified BGN will have important research prospects in various complex skin repair.
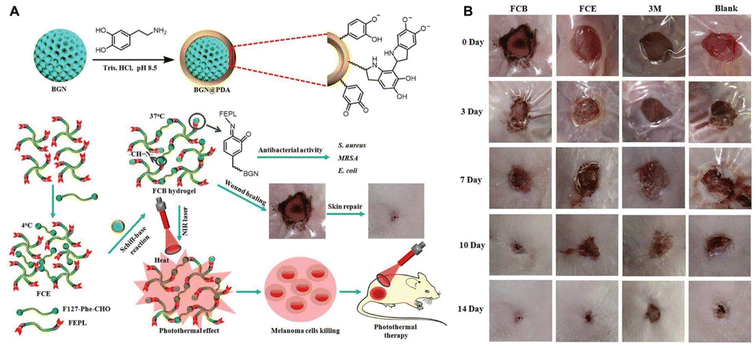 |
| Fig. 9 Silicon-based NNPHs for wound healing. (A) Synthesis schematic of a BGN-based NNPH for wound healing; (B) the photograph of wound closure on the 0th, 3rd, 7th, 10th and 14th days.209 Copyright 2019, John Wiley and Sons. | |
Although some of the silicon based materials have been approved by the FDA for clinical use, their nanoscale counterparts with undesirable dispersity should be further considered. Chemical modification with other components, such as PDA, might ameliorate their dispersity and functionality. Similar to other nanomaterials, the system mass production is difficult to implement.
4. Conclusion and outlook
In this review, requirements and functions for wound dressings of NNPHs were first proposed and highlighted, including biocompatibility, hemostatic, tissue adhesive, bactericidal, anti-inflammatory and antioxidant, stimuli-responsive, self-healing, and electrically conductive properties and so forth. Thereafter, NNPHs for wound healing were classified and summarized based on their nanofillers. Although great and ever-increasing NNPHs have been elaborately created to address different types of wounds, NNPHs still have some roadblocks for further investigation. The remaining potential problems are highlighted as follows: (1) cost-effectiveness with high-volume mass production and eco-friendly preparation methods of NNPHs are major challenges, because the production of nanomaterials usually demonstrates complicated time-consuming procedures along with harmful chemicals, moreover, the costs of natural polymers and some chemicals are expensive; (2) stability of performances of NNPHs from batch to batch should be further ameliorated because of the instability of natural polymers, for example, molecular weights of natural polymers are different from batch to batch production; (3) long-term biosafety of NNPHs should be carefully considered because nanomaterials would be accumulated in creatures, which might cause undesirable side effects and toxicity; (4) the exchange and removal of NNPHs might cause secondary damage to wounds because they can adhere to the injury site; (5) wound healing studies for large mammals such as pigs and sheep are rarely reported, and the majority of animal studies are focused on rats skin despite lots of difference between rat skin and human skin. Based on the above-mentioned limitations, the future outlooks of NNPHs for wound healing are proposed: (1) advanced technology and facile eco-friendly methods should be developed to engineer NNPHs with mass production; (2) synthetic polymers are anticipated to be combined with natural polymers to develop NNPHs with better performance for wound healing; (3) further and long-term in vivo biocompatibility studies should be put into practice before clinical utilization; (4) design and manufacture of smart wound dressings with detection and monitoring ability for wound conditions without incurring harm during peeled off and exchange is highly expected; (5) it would be better to add large animal skin wound models for wound healing investigation to address the limitations of rat skin.
Although numerous studies and great efforts have been made to engineer NNPHs for wound healing, clinical trials and commercial products still need to be developed. There are some considerations that should be taken into account before clinical translation. First, the therapeutic effect of NNPHs for wound healing should be prioritized. Second, the biosafety, stability and environmental tolerance of NNPHs are anticipated to be further investigated and ameliorated. Last, the cost of products should be reasonable so patients can afford them. At the intersection of materials science and biomedical fields, NNPHs have a promising future with the ever-developing science and technologies. We believe that NNPHs will be in clinical use soon after they are elaborately designed and fabricated for wound healing.
Author contributions
Min Wang: investigation, writing – original draft, visualization. Zexing Deng: investigation, writing – original draft. Yi Guo: investigation, writing – editing. Peng Xu: supervision, conceptualization.
Conflicts of interest
The authors declare no conflict of interest.
Acknowledgements
We acknowledge the financial support of the Natural Science Foundation of Shaanxi Province (grant no. 2022JQ-384), the High-level Talents Foundation for Scientific Research of Xi'an University of Science and Technology (grant no. 2050122015), the Fundamental Research Funds for the Central Universities (grant no. xzy012021075), and the China Postdoctoral Science Foundation (grant no. 2021M702644).
References
- Y. Xi, J. Ge, M. Wang, M. Chen, W. Niu, W. Cheng, Y. Xue, C. Lin and B. Lei, ACS Nano, 2020, 14, 2904–2916 CrossRef CAS PubMed.
- Y. Huang, L. Mu, X. Zhao, Y. Han and B. Guo, ACS Nano, 2022, 16, 13022–13036 CrossRef CAS PubMed.
- Y. Liang, J. He and B. Guo, ACS Nano, 2021, 15, 12687–12722 CrossRef CAS PubMed.
- A. Maleki, J. He, S. Bochani, V. Nosrati, M. A. Shahbazi and B. Guo, ACS Nano, 2021, 15, 18895–18930 CrossRef CAS PubMed.
- B. Guo, R. Dong, Y. Liang and M. Li, Nat. Rev. Chem., 2021, 5, 773–791 CrossRef CAS.
- P. A. Shiekh, A. Singh and A. Kumar, Biomaterials, 2020, 249, 120020 Search PubMed.
- Y. Yuan, S. Shen and D. Fan, Biomaterials, 2021, 276, 120838 Search PubMed.
- Z. Zhang, J. Xie, J. Xing, C. Li, T. M. Wong, H. Yu, Y. Li, F. Yang, Y. Tian, H. Zhang, W. Li, C. Ning, X. Wang and P. Yu, Adv. Healthcare Mater., 2022, 2201565 CrossRef.
- Z. Xu, S. Han, Z. Gu and J. Wu, Adv. Healthcare Mater., 2020, 9, 1901502 CrossRef CAS PubMed.
- X. Zhao, H. Wu, B. Guo, R. Dong, Y. Qiu and P. X. Ma, Biomaterials, 2017, 122, 34–47 CrossRef CAS PubMed.
- J. Qu, X. Zhao, Y. Liang, T. Zhang, P. X. Ma and B. Guo, Biomaterials, 2018, 183, 185–199 CrossRef CAS PubMed.
- Y. Liang, X. Zhao, T. Hu, B. Chen, Z. Yin, P. X. Ma and B. Guo, Small, 2019, 15, 1900046 CrossRef PubMed.
- Y. Xi, J. Ge, Y. Guo, B. Lei and P. X. Ma, ACS Nano, 2018, 12, 10772–10784 Search PubMed.
- J. Qu, X. Zhao, Y. Liang, Y. Xu, P. X. Ma and B. Guo, Chem. Eng. J., 2019, 362, 548–560 Search PubMed.
- X. Zhao, Y. Liang, Y. Huang, J. He, Y. Han and B. Guo, Adv. Funct. Mater., 2020, 30, 1910748 Search PubMed.
- Y. Liang, Z. Li, Y. Huang, R. Yu and B. Guo, ACS Nano, 2021, 15, 7078–7093 CrossRef CAS.
- S. Du, N. Zhou, G. Xie, Y. Chen, H. Suo, J. Xu, J. Tao, L. Zhang and J. Zhu, Nano Energy, 2021, 85, 106004 Search PubMed.
- F. Bao, G. Pei, Z. Wu, H. Zhuang, Z. Zhang, Z. Huan, C. Wu and J. Chang, Adv. Funct. Mater., 2020, 30, 2005422 CrossRef CAS.
- R. Yu, Y. Yang, J. He, M. Li and B. Guo, Chem. Eng. J., 2021, 417, 128278 CrossRef CAS.
- Y. Yang, Y. Liang, J. Chen, X. Duan and B. Guo, Bioact. Mater., 2022, 8, 341–354 CrossRef CAS.
- M. Li, Y. Liang, Y. Liang, G. Pan and B. Guo, Chem. Eng. J., 2022, 427, 132039 Search PubMed.
- Q. Zeng, X. Qi, G. Shi, M. Zhang and H. Haick, ACS Nano, 2022, 16, 1708–1733 CrossRef CAS PubMed.
- A. Kushwaha, L. Goswami and B. S. Kim, Nanomaterials, 2022, 12, 618 CrossRef CAS PubMed.
- L. Lei, X. Wang, Y. Zhu, W. Su, Q. Lv and D. Li, Mater. Des., 2022, 215, 110478 CrossRef CAS.
- L. Lei, Y. Zhu, X. Qin, S. Chai, G. Liu, W. Su, Q. Lv and D. Li, Chem. Eng. J., 2021, 425, 130671 Search PubMed.
- M. Li, J. Chen, M. Shi, H. Zhang, P. X. Ma and B. Guo, Chem. Eng. J., 2019, 375, 121999 CrossRef CAS.
- Q. Tang, T. Lim, X.-J. Wei, Q.-Y. Wang, J.-C. Xu, L.-Y. Shen, Z.-Z. Zhu and C.-Q. Zhang, Biomaterials, 2020, 255, 120138 CrossRef CAS PubMed.
- S. Homaeigohar and A. R. Boccaccini, Acta Biomater., 2020, 107, 25–49 CrossRef CAS.
- X. Chen, X. Wang, S. Wang, X. Zhang, J. Yu and C. Wang, Mater. Sci. Eng., C, 2020, 110, 110624 CrossRef CAS PubMed.
- X. Zhang, D. Yao, W. Zhao, R. Zhang, B. Yu, G. Ma, Y. Li, D. Hao and F.-J. Xu, Adv. Funct. Mater., 2021, 31, 2009258 CrossRef CAS.
- J. Cao, P. Wu, Q. Cheng, C. He, Y. Chen and J. Zhou, ACS Appl. Mater. Interfaces, 2021, 13, 24095–24105 Search PubMed.
- S. K. Das, T. Parandhaman and M. D. Dey, Green Chem., 2021, 23, 629–669 Search PubMed.
- R. Dong and B. Guo, Nano Today, 2021, 41, 101290 CrossRef CAS.
- Z. Deng, Y. Guo, X. Zhao, T. Du, J. Zhu, Y. Xie, F. Wu, Y. Wang and M. Guan, Gels, 2022, 8, 280 CrossRef CAS PubMed.
- Z. Deng, R. Yu and B. Guo, Mater. Chem. Front., 2021, 5, 2092–2123 RSC.
- Z. Q. Li and J. J. Guan, Polymers, 2011, 3, 740–761 CrossRef CAS.
- X. Zhao, Q. Lang, L. Yildirimer, Z. Y. Lin, W. Cui, N. Annabi, K. W. Ng, M. R. Dokmeci, A. M. Ghaemmaghami and A. Khademhosseini, Adv. Healthcare Mater., 2016, 5, 108–118 CrossRef CAS.
- F. Gao, Z. Xu, Q. Liang, H. Li, L. Peng, M. Wu, X. Zhao, X. Cui, C. Ruan and W. Liu, Adv. Sci., 2019, 6, 1900867 Search PubMed.
- T. Su, M. Zhang, Q. Zeng, W. Pan, Y. Huang, Y. Qian, W. Dong, X. Qi and J. Shen, Bioact. Mater., 2021, 6, 579–588 Search PubMed.
- J. Yoo, J. H. Park, Y. W. Kwon, J. J. Chung, I. C. Choi, J. J. Nam, H. S. Lee, E. Y. Jeon, K. Lee, S. H. Kim, Y. Jung and J. W. Park, Biomater. Sci., 2020, 8, 6261–6271 RSC.
- A. L. Wollenberg, T. M. O'Shea, J. H. Kim, A. Czechanski, L. G. Reinholdt, M. V. Sofroniew and T. J. Deming, Biomaterials, 2018, 178, 527–545 CrossRef CAS PubMed.
- X. Pan, S. Cheng, C. Zhang, Y. Jiao, X. Lin, W. Dong and X. Qi, Chem. Eng. J., 2021, 409, 128203 CrossRef CAS.
- S. H. Zainal, N. H. Mohd, N. Suhaili, F. H. Anuar, A. M. Lazim and R. Othaman, J. Mater. Res. Technol., 2021, 10, 935–952 Search PubMed.
- Q. Xu, Y. Ji, Q. Sun, Y. Fu, Y. Xu and L. Jin, Nanomaterials, 2019, 9, 253 Search PubMed.
- J. Shin, S. Choi, J. H. Kim, J. H. Cho, Y. Jin, S. Kim, S. Min, S. K. Kim, D. Choi and S.-W. Cho, Adv. Funct. Mater., 2019, 29, 1903863 Search PubMed.
- H. Samadian, H. Maleki, Z. Allahyari and M. Jaymand, Coord. Chem. Rev., 2020, 420, 213432 CrossRef CAS.
- Z. J. Shi, X. Gao, M. W. Ullah, S. X. Li, Q. Wang and G. Yang, Biomaterials, 2016, 111, 40–54 Search PubMed.
- R. Yu, M. Li, Z. Li, G. Pan, Y. Liang and B. Guo, Adv. Healthcare Mater., 2022, e2102749 CrossRef PubMed.
- X. Zhao, B. Guo, H. Wu, Y. Liang and P. X. Ma, Nat. Commun., 2018, 9, 2784 CrossRef PubMed.
- R. Yu, H. Zhang and B. Guo, Nano-Micro Lett., 2021, 14, 1 Search PubMed.
- S. Pourshahrestani, E. Zeimaran, N. A. Kadri, N. Mutlu and A. R. Boccaccini, Adv. Healthcare Mater., 2020, 9, e2000905 CrossRef PubMed.
- Z. Zhang, W. Jiang, X. Xie, H. Liang, H. Chen, K. Chen, Y. Zhang, W. Xu and M. Chen, ChemistrySelect, 2021, 6, 12358–12382 Search PubMed.
- A. K. Gaharwar, N. A. Peppas and A. Khademhosseini, Biotechnol. Bioeng., 2014, 111, 441–453 Search PubMed.
- S. Rafieian, H. Mirzadeh, H. Mahdavi and M. E. Masoumi, Sci. Eng. Compos. Mater., 2019, 26, 154–174 CAS.
- Z. B. Xiao, Q. X. Zhao, Y. W. Niu and D. Zhao, Soft Matter, 2022, 18, 3447–3464 Search PubMed.
- S. R. U. Rehman, R. Augustine, A. A. Zahid, R. Ahmed, M. Tariq and A. Hasan, Int. J. Nanomed., 2019, 14, 9603–9617 Search PubMed.
- J. He, M. Shi, Y. Liang and B. Guo, Chem. Eng. J., 2020, 394, 124888 CrossRef CAS.
- M. Xu, Q. Li, Z. Fang, M. Jin, Q. Zeng, G. Huang, Y. G. Jia, L. Wang and Y. Chen, Biomater. Sci., 2020, 8, 6957–6968 RSC.
- W. Shi, N. Song, Y. Huang, C. He, M. Zhang, W. Zhao and C. Zhao, Biomacromolecules, 2022, 23, 889–902 CrossRef CAS PubMed.
- B. Zhang, J. He, M. Shi, Y. Liang and B. Guo, Chem. Eng. J., 2020, 400, 125994 CrossRef CAS.
- P. Li, S. Liu, X. Yang, S. Du, W. Tang, W. Cao, J. Zhou, X. Gong and X. Xing, Chem. Eng. J., 2021, 403, 126387 CrossRef CAS.
- S. Huang, H. Liu, K. Liao, Q. Hu, R. Guo and K. Deng, ACS Appl. Mater. Interfaces, 2020, 12, 28952–28964 CAS.
- Y. Lin, X. Liu, Z. Liu and Y. Xu, Small, 2021, 17, e2103348 Search PubMed.
- A. A. Shefa, T. Sultana, M. K. Park, S. Y. Lee, J.-G. Gwon and B.-T. Lee, Mater. Des., 2020, 186, 108313 Search PubMed.
- H. Deng, Z. Yu, S. Chen, L. Fei, Q. Sha, N. Zhou, Z. Chen and C. Xu, Carbohydr. Polym., 2020, 230, 115565 CrossRef CAS PubMed.
- W. C. Huang, R. Ying, W. Wang, Y. Guo, Y. He, X. Mo, C. Xue and X. Mao, Adv. Funct. Mater., 2020, 30, 2000644 Search PubMed.
- C. Mao, Y. Xiang, X. Liu, Z. Cui, X. Yang, K. W. K. Yeung, H. Pan, X. Wang, P. K. Chu and S. Wu, ACS Nano, 2017, 11, 9010–9021 CrossRef CAS PubMed.
- B. Zhang, Y. Lv, C. Yu, W. Zhang, S. Song, Y. Li, Y. Chong, J. Huang and Z. Zhang, Biomater. Adv., 2022, 137, 212869 CrossRef CAS PubMed.
- B. Ma, W. Dang, Z. Yang, J. Chang and C. Wu, Appl. Mater. Today, 2020, 20, 100735 CrossRef.
- M. Luo, M. Wang, W. Niu, M. Chen, W. Cheng, L. Zhang, C. Xie, Y. Wang, Y. Guo, T. Leng, X. Zhang, C. Lin and B. Lei, Chem. Eng. J., 2021, 412, 128471 Search PubMed.
- H. Zheng, S. Wang, F. Cheng, X. He, Z. Liu, W. Wang, L. Zhou and Q. Zhang, Chem. Eng. J., 2021, 424, 130148 CrossRef CAS.
- Y. Li, M. Han, Y. Cai, B. Jiang, Y. Zhang, B. Yuan, F. Zhou and C. Cao, Biomater. Sci., 2022, 10, 1068–1082 Search PubMed.
- L. Zhou, H. Zheng, Z. Liu, S. Wang, Z. Liu, F. Chen, H. Zhang, J. Kong, F. Zhou and Q. Zhang, ACS Nano, 2021, 15, 2468–2480 Search PubMed.
- Y. Li, R. Fu, Z. Duan, C. Zhu and D. Fan, ACS Nano, 2022, 16, 7486–7502 Search PubMed.
- X. Yang, C. Zhang, D. Deng, Y. Gu, H. Wang and Q. Zhong, Small, 2022, 18, 2104368 CrossRef CAS PubMed.
- S. Zhu, Q. Dai, L. Yao, Z. Wang, Z. He, M. Li, H. Wang, Q. Li, H. Gao and X. Cao, Composites, Part B, 2022, 231, 109569 CrossRef CAS.
- G. Lokhande, J. K. Carrow, T. Thakur, J. R. Xavier, M. Parani, K. J. Bayless and A. K. Gaharwar, Acta Biomater., 2018, 70, 35–47 CrossRef CAS.
- L. Han, X. Lu, K. Liu, K. Wang, L. Fang, L. T. Weng, H. Zhang, Y. Tang, F. Ren, C. Zhao, G. Sun, R. Liang and Z. Li, ACS Nano, 2017, 11, 2561–2574 CrossRef CAS PubMed.
- A. Khalid, A. Madni, B. Raza, M. ul Islam, A. Hassan, F. Ahmad, H. Ali, T. Khan and F. Wahid, Int. J. Biol. Macromol., 2022, 203, 256–267 CrossRef CAS PubMed.
- Y. Liang, X. Zhao, T. Hu, Y. Han and B. Guo, J. Colloid Interface Sci., 2019, 556, 514–528 CrossRef CAS PubMed.
- M. Wang, C. G. Wang, M. Chen, M. Luo, Q. X. Chen and B. Lei, Chem. Eng. J., 2022, 439, 135629 CrossRef CAS.
- Y. Liang, B. Chen, M. Li, J. He, Z. Yin and B. Guo, Biomacromolecules, 2020, 21, 1841–1852 CrossRef CAS.
- Z. Tu, M. Chen, M. Wang, Z. Shao, X. Jiang, K. Wang, Z. Yao, S. Yang, X. Zhang, W. Gao, C. Lin, B. Lei and C. Mao, Adv. Funct. Mater., 2021, 31, 2100924 Search PubMed.
- L. Mao, S. Hu, Y. Gao, L. Wang, W. Zhao, L. Fu, H. Cheng, L. Xia, S. Xie, W. Ye, Z. Shi and G. Yang, Adv. Healthcare Mater., 2020, 9, 2000872 CrossRef CAS.
- S. I. Basha, S. Ghosh, K. Vinothkumar, B. Ramesh, P. H. P. Kumari, K. V. M. Mohan and E. Sukumar, Mater. Sci. Eng., C, 2020, 111, 110743 Search PubMed.
- J. Yang, Y. Chen, L. Zhao, Z. Feng, K. Peng, A. Wei, Y. Wang, Z. Tong and B. Cheng, Composites, Part B, 2020, 197, 108139 CrossRef CAS.
- H. Zhang, X. Sun, J. Wang, Y. Zhang, M. Dong, T. Bu, L. Li, Y. Liu and L. Wang, Adv. Funct. Mater., 2021, 31, 2100093 CrossRef CAS.
- Z. Fan, B. Liu, J. Wang, S. Zhang, Q. Lin, P. Gong, L. Ma and S. Yang, Adv. Funct. Mater., 2014, 24, 3933–3943 Search PubMed.
- K. Liu, L. Dai and C. Li, Int. J. Biol. Macromol., 2021, 191, 1249–1254 CrossRef CAS PubMed.
- C. Wang, X. Jiang, H.-J. Kim, S. Zhang, X. Zhou, Y. Chen, H. Ling, Y. Xue, Z. Chen, M. Qu, L. Ren, J. Zhu, A. Libanori, Y. Zhu, H. Kang, S. Ahadian, M. R. Dokmeci, P. Servati, X. He, Z. Gu, W. Sun and A. Khademhosseini, Biomaterials, 2022, 285, 121479 CrossRef CAS PubMed.
- X. Wang, Z. Wang, S. Fang, Y. Hou, X. Du, Y. Xie, Q. Xue, X. Zhou and X. Yuan, Chem. Eng. J., 2021, 420, 127589 Search PubMed.
- J. Li, Y. Wang, J. Yang and W. Liu, Chem. Eng. J., 2021, 420, 127638 CrossRef CAS.
- Y. Li, R. Fu, Z. Duan, C. Zhu and D. Fan, Small, 2022, 18, 2200165 CrossRef CAS PubMed.
- X. Zhang, G. Zhang, H. Zhang, X. Liu, J. Shi, H. Shi, X. Yao, P. K. Chu and X. Zhang, Chem. Eng. J., 2020, 382, 122849 CrossRef CAS.
- Y. Li, R. Fu, Z. Duan, C. Zhu and D. Fan, Bioact. Mater., 2022, 9, 461–474 Search PubMed.
- L. Zhou, F. Chen, Z. Hou, Y. Chen and X. Luo, Chem. Eng. J., 2021, 409, 128224 CrossRef CAS.
- Y. Liang, M. Wang, Z. Zhang, G. Ren, Y. Liu, S. Wu and J. Shen, Chem. Eng. J., 2019, 378, 122043 CrossRef CAS.
- L. Siebert, E. Luna-Cerón, L. E. García-Rivera, J. Oh, J. Jang, D. A. Rosas-Gómez, M. D. Pérez-Gómez, G. Maschkowitz, H. Fickenscher, D. Oceguera-Cuevas, C. G. Holguín-León, B. Byambaa, M. A. Hussain, E. Enciso-Martínez, M. Cho, Y. Lee, N. Sobahi, A. Hasan, D. P. Orgill, Y. K. Mishra, R. Adelung, E. Lee and S. R. Shin, Adv. Funct. Mater., 2021, 31, 2007555 Search PubMed.
- M. Zhang, X. Qiao, W. Han, T. Jiang, F. Liu and X. Zhao, Carbohydr. Polym., 2021, 266, 118100 CrossRef CAS PubMed.
- X. Gong, M. Luo, M. Wang, W. Niu, Y. Wang and B. Lei, Regener. Biomater., 2022, 9, rbab074 Search PubMed.
- L. Yang, F. Liang, X. Zhang, Y. Jiang, F. Duan, L. Li and F. Ren, Chem. Eng. J., 2022, 427, 131506 CrossRef CAS.
- Z. Deng, M. Li, Y. Hu, Y. He, B. Tao, Z. Yuan, R. Wang, M. Chen, Z. Luo and K. Cai, Chem. Eng. J., 2021, 420, 129668 Search PubMed.
- X. Zhao, L. Chang, Y. Hu, S. Xu, Z. Liang, X. Ren, X. Mei and Z. Chen, ACS Appl. Mater. Interfaces, 2022, 14, 18194–18208 CrossRef CAS PubMed.
- H. Wu, F. Li, S. Wang, J. Lu, J. Li, Y. Du, X. Sun, X. Chen, J. Gao and D. Ling, Biomaterials, 2018, 151, 66–77 Search PubMed.
- Z. Pan, K.-R. Zhang, H.-L. Gao, Y. Zhou, B.-B. Yan, C. Yang, Z.-y. Zhang, L. Dong, S.-M. Chen, R. Xu, D.-H. Zou and S.-H. Yu, Nano Res., 2020, 13, 373–379 CrossRef CAS.
- C. Li, T. Jiang, C. Zhou, A. Jiang, C. Lu, G. Yang, J. Nie, F. Wang, X. Yang and Z. Chen, Carbohydr. Polym., 2023, 299, 120198 CrossRef CAS.
- W. Niu, M. Chen, Y. Guo, M. Wang, M. Luo, W. Cheng, Y. Wang and B. Lei, ACS Nano, 2021, 15, 14323–14337 CrossRef CAS PubMed.
- M. Long, Q. Liu, D. Wang, J. Wang, Y. Zhang, A. Tang, N. Liu, B. Bui, W. Chen and H. Yang, Mater. Today Adv., 2021, 12, 100190 Search PubMed.
- K. Haraguchi, T. Takehisa and S. Fan, Macromolecules, 2002, 35, 10162–10171 Search PubMed.
- Z. Deng, Y. Guo, X. Zhao, P. X. Ma and B. Guo, Chem. Mater., 2018, 30, 1729–1742 Search PubMed.
- Z. Deng, T. Hu, Q. Lei, J. He, P. X. Ma and B. Guo, ACS Appl. Mater. Interfaces, 2019, 11, 6796–6808 CrossRef CAS PubMed.
- Z. Deng, H. Wang, P. X. Ma and B. Guo, Nanoscale, 2020, 12, 1224–1246 RSC.
- B. Grigoryan, S. J. Paulsen, D. C. Corbett, D. W. Sazer, C. L. Fortin, A. J. Zaita, P. T. Greenfield, N. J. Calafat, J. P. Gounley, A. H. Ta, F. Johansson, A. Randles, J. E. Rosenkrantz, J. D. Louis-Rosenberg, P. A. Galie, K. R. Stevens and J. S. Miller, Science, 2019, 364, 458–464 CrossRef CAS PubMed.
- H. Yi, M. Seong, K. Sun, I. Hwang, K. Lee, C. Cha, T.-i. Kim and H. E. Jeong, Adv. Funct. Mater., 2018, 28, 1706498 CrossRef.
- K. Škrlová, K. Malachová, A. Muñoz-Bonilla, D. Měřinská, Z. Rybková, M. Fernández-García and D. Plachá, Nanomaterials, 2019, 9, 1548 CrossRef PubMed.
- Z. Deng, Y. Guo, X. Zhao, L. Li, R. Dong, B. Guo and P. X. Ma, Acta Biomater., 2016, 46, 234–244 CrossRef CAS PubMed.
- P. Lavrador, M. R. Esteves, V. M. Gaspar and J. F. Mano, Adv. Funct. Mater., 2021, 31, 2005941 Search PubMed.
- W. Peng, D. Li, K. L. Dai, Y. X. Wang, P. Song, H. R. Li, P. Tang, Z. Y. Zhang, Z. Y. Li, Y. C. Zhou and C. C. Zhou, Int. J. Biol. Macromol., 2022, 208, 400–408 Search PubMed.
- X. Zhao, Z. Zhang, J. Luo, Z. Wu, Z. Yang, S. Zhou, Y. Tu, Y. Huang, Y. Han and B. Guo, Appl. Mater. Today, 2022, 26, 101365 Search PubMed.
- T. Hu, M. Shi, X. Zhao, Y. Liang, L. Bi, Z. Zhang, S. Liu, B. Chen, X. Duan and B. Guo, Chem. Eng. J., 2022, 428, 131017 Search PubMed.
- M. Li, Z. Zhang, Y. Liang, J. He and B. Guo, ACS Appl. Mater. Interfaces, 2020, 12, 35856–35872 CrossRef CAS PubMed.
- W. Huang, Y. Wang, Y. Chen, Y. Zhao, Q. Zhang, X. Zheng, L. Chen and L. Zhang, Adv. Healthcare Mater., 2016, 5, 2813–2822 CrossRef CAS PubMed.
- Y. Ma, J. Yao, Q. Liu, T. Han, J. Zhao, X. Ma, Y. Tong, G. Jin, K. Qu, B. Li and F. Xu, Adv. Funct. Mater., 2020, 30, 2001820 CrossRef CAS.
- X. Xia, X. Xu, B. Wang, D. Zhou, W. Zhang, X. Xie, H. Lai, J. Xue, A. Rai, Z. Li, X. Peng, P. Zhao, L. Bian and P. W.-Y. Chiu, Adv. Funct. Mater., 2022, 32, 2109332 CrossRef CAS.
- Y. Huang, X. Zhao, C. Wang, J. Chen, Y. Liang, Z. Li, Y. Han and B. Guo, Chem. Eng. J., 2022, 427, 131977 CrossRef CAS.
- Y. Hong, F. Zhou, Y. Hua, X. Zhang, C. Ni, D. Pan, Y. Zhang, D. Jiang, L. Yang, Q. Lin, Y. Zou, D. Yu, D. E. Arnot, X. Zou, L. Zhu, S. Zhang and H. Ouyang, Nat. Commun., 2019, 10, 2060 Search PubMed.
- E. E. Leonhardt, N. Kang, M. A. Hamad, K. L. Wooley and M. Elsabahy, Nat. Commun., 2019, 10, 2307 CrossRef PubMed.
- Z. Qiao, X. Lv, S. He, S. Bai, X. Liu, L. Hou, J. He, D. Tong, R. Ruan, J. Zhang, J. Ding and H. Yang, Bioact. Mater., 2021, 6, 2829–2840 CrossRef CAS PubMed.
- S. An, E. J. Jeon, J. Jeon and S.-W. Cho, Mater. Horiz., 2019, 6, 1169–1178 RSC.
- Y. Huang, X. Zhao, Z. Zhang, Y. Liang, Z. Yin, B. Chen, L. Bai, Y. Han and B. Guo, Chem. Mater., 2020, 32, 6595–6610 CrossRef CAS.
- Z. Chen, H. Wu, H. Wang, D. Zaldivar-Silva, L. Agüero, Y. Liu, Z. Zhang, Y. Yin, B. Qiu, J. Zhao, X. Lu and S. Wang, Mater. Sci. Eng., C, 2021, 129, 112422 CrossRef CAS.
- W. Huang, S. Cheng, X. Wang, Y. Zhang, L. Chen and L. Zhang, Adv. Funct. Mater., 2021, 31, 2009189 CrossRef CAS.
- H. Lee, S. M. Dellatore, W. M. Miller and P. B. Messersmith, Science, 2007, 318, 426–430 CrossRef CAS PubMed.
- S. Li, S. Dong, W. Xu, S. Tu, L. Yan, C. Zhao, J. Ding and X. Chen, Adv. Sci., 2018, 5, 1700527 Search PubMed.
- Y. Liang, Y. Liang, H. Zhang and B. Guo, Asian J. Pharm. Sci., 2022, 17, 353–384 Search PubMed.
- Z. Yang, R. Huang, B. Zheng, W. Guo, C. Li, W. He, Y. Wei, Y. Du, H. Wang, D. Wu and H. Wang, Adv. Sci., 2021, 8, 2003627 Search PubMed.
- D. Gan, T. Xu, W. Xing, X. Ge, L. Fang, K. Wang, F. Ren and X. Lu, Adv. Funct. Mater., 2019, 29, 1805964 Search PubMed.
- K. Wang, J. Wang, L. Li, L. Xu, N. Feng, Y. Wang, X. Fei, J. Tian and Y. Li, Chem. Eng. J., 2019, 372, 216–225 CrossRef CAS.
- W. Liu, W. Ou-Yang, C. Zhang, Q. Wang, X. Pan, P. Huang, C. Zhang, Y. Li, D. Kong and W. Wang, ACS Nano, 2020, 14, 12905–12917 CrossRef CAS.
- C. Shuai, G. Liu, Y. Yang, F. Qi, S. Peng, W. Yang, C. He, G. Wang and G. Qian, Nano Energy, 2020, 74, 104825 CrossRef CAS.
- X. Qu, H. Yang, B. Jia, Z. Yu, Y. Zheng and K. Dai, Acta Biomater., 2020, 117, 400–417 CrossRef CAS PubMed.
- Q. Xin, H. Shah, A. Nawaz, W. Xie, M. Z. Akram, A. Batool, L. Tian, S. U. Jan, R. Boddula, B. Guo, Q. Liu and J. R. Gong, Adv. Mater., 2019, 31, 1804838 Search PubMed.
- Y. Fu, L. Yang, J. Zhang, J. Hu, G. Duan, X. Liu, Y. Li and Z. Gu, Mater. Horiz., 2021, 8, 1618–1633 RSC.
- X. Zhang, C. Zhang, Y. Yang, H. Zhang, X. Huang, R. Hang and X. Yao, Chem. Eng. J., 2019, 374, 596–604 CrossRef CAS.
- J. Sun, L. Song, Y. Fan, L. Tian, S. Luan, S. Niu, L. Ren, W. Ming and J. Zhao, ACS Appl. Mater. Interfaces, 2019, 11, 26581–26589 CrossRef CAS PubMed.
- J. Chi, X. Zhang, C. Chen, C. Shao, Y. Zhao and Y. Wang, Bioact. Mater., 2020, 5, 253–259 CrossRef PubMed.
- J. Li, S. Yuan, J. Zhu and B. Van der Bruggen, Chem. Eng. J., 2019, 373, 275–284 CrossRef CAS.
- L. Ren, G. He, Y. Zhou, J. Dai, W. Miao, C. Ouyang, J. Liu and G. Chen, Biomater. Sci., 2022, 10, 3174–3187 RSC.
- M. Wang, C. G. Wang, M. Chen, Y. W. Xi, W. Cheng, C. Mao, T. Z. Xu, X. X. Zhang, C. Lin, W. Y. Gao, Y. Guo and B. Lei, ACS Nano, 2019, 13, 10279–10293 CrossRef CAS PubMed.
- Y. Tang, X. Lan, C. Liang, Z. Zhong, R. Xie, Y. Zhou, X. Miao, H. Wang and W. Wang, Carbohydr. Polym., 2019, 219, 113–120 CrossRef CAS PubMed.
- M. Ghodrati, M. R. Farahpour and H. Hamishehkar, Colloids Surf., A, 2019, 564, 161–169 CrossRef CAS.
- M. Luo, K. Shaitan, X. Qu, A. P. Bonartsev and B. Lei, Appl. Mater. Today, 2022, 26, 101304 CrossRef.
- P. Wang, S. Huang, Z. Hu, W. Yang, Y. Lan, J. Zhu, A. Hancharou, R. Guo and B. Tang, Acta Biomater., 2019, 100, 191–201 CrossRef CAS PubMed.
- N. Lohmann, L. Schirmer, P. Atallah, E. Wandel, R. A. Ferrer, C. Werner, J. C. Simon, S. Franz and U. Freudenberg, Sci. Transl. Med., 2017, 9, eaai9044 Search PubMed.
- C. Dunnill, T. Patton, J. Brennan, J. Barrett, M. Dryden, J. Cooke, D. Leaper and N. T. Georgopoulos, Int. Wound J., 2017, 14, 89–96 CrossRef PubMed.
- Z. Xu, G. Liu, J. Huang and J. Wu, ACS Appl. Mater. Interfaces, 2022, 14, 7680–7689 CrossRef CAS PubMed.
- N. Gupta, B. F. Lin, L. M. Campos, M. D. Dimitriou, S. T. Hikita, N. D. Treat, M. V. Tirrell, D. O. Clegg, E. J. Kramer and C. J. Hawker, Nat. Chem., 2010, 2, 138–145 Search PubMed.
- A. Singh, S. Halder, S. Chumber, M. C. Misra, L. K. Sharma, A. Srivastava and G. R. Menon, Asian J. Surg., 2004, 27, 326–332 CrossRef PubMed.
- V. J. Jones, Int. Wound J., 2006, 3, 79–88 CrossRef PubMed.
- D. B. Hom, G. Adams, M. Koreis and R. Maisel, Otolaryngol.--Head Neck Surg., 1999, 121, 591–598 CrossRef CAS PubMed.
- A. Z. K. MD, N. T. MD and M. A. MD, J. Wound Care, 2005, 14, 42–44 CrossRef PubMed.
- J. Xiao, Y. J. Zhou, M. Q. Ye, Y. An, K. N. Wang, Q. J. Wu, L. W. Song, J. W. Zhang, H. C. He, Q. W. Zhang and J. Wu, Adv. Healthcare Mater., 2021, 10, 2001591 CrossRef CAS PubMed.
- G. D. Winter, Nature, 1962, 193, 293–294 CrossRef CAS PubMed.
- J. P. E. Junker, R. A. Kamel, E. J. Caterson and E. Eriksson, Adv. Wound Care, 2013, 2, 348–356 CrossRef.
- S. Metzger, Home Healthc Now, 2004, 22, 586–590 CrossRef.
- S. Koosehgol, M. Ebrahimian-Hosseinabadi, M. Alizadeh and A. Zamanian, Mater. Sci. Eng., C, 2017, 79, 66–75 CrossRef CAS.
- S. Park and K. M. Park, Biomaterials, 2018, 182, 234–244 CrossRef CAS.
- A. S. Pandit and D. S. Feldman, Wound Repair Regen., 1994, 2, 130–137 CrossRef CAS.
- H. Ma, Q. Zhou, J. Chang and C. Wu, ACS Nano, 2019, 13, 4302–4311 CrossRef CAS PubMed.
- S. O. Blacklow, J. Li, B. R. Freedman, M. Zeidi, C. Chen and D. J. Mooney, Sci. Adv., 2019, 5, eaaw3963 CrossRef CAS PubMed.
- Y. Zhu, J. Zhang, J. Song, J. Yang, Z. Du, W. Zhao, H. Guo, C. Wen, Q. Li, X. Sui and L. Zhang, Adv. Funct. Mater., 2020, 30, 1905493 CrossRef CAS.
- M. Li, Y. Liang, J. He, H. Zhang and B. Guo, Chem. Mater., 2020, 32, 9937–9953 Search PubMed.
- D. Roy, W. L. A. Brooks and B. S. Sumerlin, Chem. Soc. Rev., 2013, 42, 7214–7243 RSC.
- H. S. Jung, P. Verwilst, A. Sharma, J. Shin, J. L. Sessler and J. S. Kim, Chem. Soc. Rev., 2018, 47, 2280–2297 RSC.
- D. Zhi, T. Yang, J. O'Hagan, S. Zhang and R. F. Donnelly, J. Controlled Release, 2020, 325, 52–71 CrossRef CAS PubMed.
- J. Chen, C. Ning, Z. Zhou, P. Yu, Y. Zhu, G. Tan and C. Mao, Prog. Mater. Sci., 2019, 99, 1–26 CrossRef CAS PubMed.
- C. Tong, X. Zhong, Y. Yang, X. Liu, G. Zhong, C. Xiao, B. Liu, W. Wang and X. Yang, Biomaterials, 2020, 243, 119936 CrossRef CAS PubMed.
- Y. Liang, M. Li, Y. Yang, L. Qiao, H. Xu and B. Guo, ACS Nano, 2022, 16, 3194–3207 CrossRef CAS.
- G. Kocak, C. Tuncer and V. Bütün, Polym. Chem., 2017, 8, 144–176 Search PubMed.
- L. Wang, Y. Wu, B. Guo and P. X. Ma, ACS Nano, 2015, 9, 9167–9179 CrossRef CAS PubMed.
- Y. Wu, L. Wang, B. Guo and P. X. Ma, ACS Nano, 2017, 11, 5646–5659 CrossRef CAS PubMed.
- T. Chen, Y. Chen, H. U. Rehman, Z. Chen, Z. Yang, M. Wang, H. Li and H. Liu, ACS Appl. Mater. Interfaces, 2018, 10, 33523–33531 CrossRef CAS.
- M. Chen, J. Tian, Y. Liu, H. Cao, R. Li, J. Wang, J. Wu and Q. Zhang, Chem. Eng. J., 2019, 373, 413–424 Search PubMed.
- A. Zhang, Y. Liu, D. Qin, M. Sun, T. Wang and X. Chen, Int. J. Biol. Macromol., 2020, 164, 2108–2123 CrossRef CAS PubMed.
- D. L. Taylor and M. in het Panhuis, Adv. Mater., 2016, 28, 9060–9093 CrossRef CAS PubMed.
- T. W. Ebbesen, Annu. Rev. Mater. Sci., 1994, 24, 235–264 Search PubMed.
- R. C. Haddon, Acc. Chem. Res., 2002, 35, 997 CrossRef CAS PubMed.
- H. Dai, Surf. Sci., 2002, 500, 218–241 CrossRef CAS.
- L. Feng and Z. Liu, Nanomedicine, 2011, 6, 317–324 CrossRef CAS PubMed.
- A. K. Geim, Science, 2009, 324, 1530–1534 CrossRef CAS PubMed.
- X. Qi, Y. Huang, S. You, Y. Xiang, E. Cai, R. Mao, W. Pan, X. Tong, W. Dong, F. Ye and J. Shen, Adv. Sci., 2022, 9, 2106015 Search PubMed.
- V. R. Askary, N. A. Jahan, A. Sabbagh, F. S. Jahani, N. Dourandish and A. R. K. Kamachali, Clin. Biochem., 2011, 44, S323–S324 Search PubMed.
- B. Li, H. M. Wen, Y. J. Cui, W. Zhou, G. D. Qian and B. L. Chen, Adv. Mater., 2016, 28, 8819–8860 CrossRef CAS PubMed.
- F. Hu, S. S. Xia, Y. He, Z. L. Huang, H. Ke and J. Z. Liao, Colloids Surf., B, 2022, 213, 112425 CrossRef CAS PubMed.
- Y. Chen, J. Cai, D. Liu, S. Liu, D. Lei, L. Zheng, Q. Wei and M. Gao, Regener. Biomater., 2022, 9, rbac019 Search PubMed.
- L. Q. Fu, X. Y. Chen, M. H. Cai, X. H. Tao, Y. B. Fan and X. Z. Mou, Front. Bioeng. Biotechnol., 2020, 8, 576348 CrossRef PubMed.
- H. Wang, Z. Xu, Q. Li and J. Wu, Engineered Regeneration, 2021, 2, 137–153 CrossRef.
- M. Naguib, M. Kurtoglu, V. Presser, J. Lu, J. J. Niu, M. Heon, L. Hultman, Y. Gogotsi and M. W. Barsoum, Adv. Mater., 2011, 23, 4248–4253 Search PubMed.
- Y. Gogotsi and B. Anasori, ACS Nano, 2019, 13, 8491–8494 Search PubMed.
- B. Song and Y. He, Nano Today, 2019, 26, 149–163 CrossRef CAS.
- F. Peng, Y. Su, Y. Zhong, C. Fan, S.-T. Lee and Y. He, Acc. Chem. Res., 2014, 47, 612–623 CrossRef CAS PubMed.
- M. Mesa and N. Y. Becerra, Int. J. Biomater., 2021, 2021, 6857204 Search PubMed.
- C. Wang, H. Niu, X. Ma, H. Hong, Y. Yuan and C. Liu, ACS Appl. Mater. Interfaces, 2019, 11, 34595–34608 CrossRef CAS PubMed.
- A. M. Villalba-Rodriguez, S. Martinez-Gonzalez, J. E. Sosa-Hernandez, R. Parra-Saldivar, M. Bilal and H. M. N. Iqbal, Gels, 2021, 7, 59 CrossRef CAS PubMed.
- A. N. Ordeghan, D. Khayatan, M. R. Saki, M. Alam, K. Abbasi, H. Shirvani, M. Yazdanian, R. S. Soufdoost, H. T. Raad, A. Karami and H. Tebyaniyan, Adv. Mater. Sci. Eng., 2022, 2022, 9222003 Search PubMed.
- L. F. Fan, X. Wang and D. C. Wu, Chin. J. Chem., 2021, 39, 757–774 CrossRef CAS.
- C. Yang, H. Huang, S. Fan, C. Yang, Y. Chen, B. Yu, W. Li and J. Liao, Adv. Mater. Technol, 2021, 6, 2001012 Search PubMed.
- U. Pantulap, M. Arango-Ospina and A. R. Boccaccini, J. Mater. Sci.: Mater.
Med., 2022, 33, 3 Search PubMed.
- L. Zhou, Y. Xi, Y. Xue, M. Wang, Y. Liu, Y. Guo and B. Lei, Adv. Funct. Mater., 2019, 29, 1806883 CrossRef.
- Y. Li, T. Xu, Z. Tu, W. Dai, Y. Xue, C. Tang, W. Gao, C. Mao, B. Lei and C. Lin, Theranostics, 2020, 10, 4929–4943 CrossRef CAS PubMed.
- M. Chen, D. D. Winston, M. Wang, W. Niu, W. Cheng, Y. Guo, Y. Wang, M. Luo, C. Xie, T. Leng, X. Qu and B. Lei, Mater. Today, 2022, 53, 27–40 CrossRef CAS.
- W. Niu, Y. Guo, Y. Xue, M. Wang, M. Chen, D. D. Winston, W. Cheng and B. Lei, Nano Today, 2021, 38, 101137 CrossRef CAS.
Footnote |
† M. Wang, Z. Deng, and Y. Guo contributed equally to this paper. |
|
This journal is © The Royal Society of Chemistry 2023 |