DOI:
10.1039/D2MA00945E
(Review Article)
Mater. Adv., 2023,
4, 1415-1443
Biofilm-mediated wastewater treatment: a comprehensive review
Received
1st October 2022
, Accepted 4th February 2023
First published on 13th February 2023
Abstract
Global industrialization has increased and so has the pollution generated by industries. Although all types of pollutions are a major concern for the environment, wastewater treatment has always intrigued the interest of researchers globally. Wastewater contains multiple contaminants, which can be treated via physical, biological, and chemical methods. Biological wastewater treatment technologies are seen as modern alternatives to conventional techniques that play a crucial role in improving human health and water quality. Organic pollution is one of the primary culprits of several phenomena, including eutrophication, decline in dissolved oxygen, and build-up of toxins, which impact the quality of water. Therefore, it becomes a necessity to eliminate organic pollutants from wastewater. The use of bioremediation as an environmentally benign method for the decontamination of harmful contaminants for environmental sustainability and safety has gained popularity due to the several disadvantages (expensive, operation, efficiency, and start-up) of traditional wastewater treatment plants. Bioremediation has been used to eliminate, mineralize, degrade, and neutralize many inorganic and organic contaminants from wastewater and contaminated environments using microbial communities (bacteria, fungi, and algae). The biofilm-mediated remediation method is one of the bioremediation techniques, which has been regarded as an economical and environment-friendly choice for environmental clean-up. Biofilms are aggregates of single or mixed microbial cells that adhere to a living or inert surface in an aqueous environment. In contrast to free-floating planktonic cells, biofilm-forming bacteria can compete for nutrients, show greater tolerance to contaminants, and offer a protective environment for cells. They show remarkable survival rate under extreme environmental parameters. Due to a properly regulated gene expression pattern mediated by quorum sensing, biofilm communities are therefore apt for the sorption and metabolism of organic contaminants and heavy metals. They are being used for wastewater treatment since the past few years due to their commendable ability to remove pollutants. Biodeterioration of materials such as metals, plastics, and concrete is accelerated by metabolic processes mediated by microorganisms living in biofilms. Electronic databases such as Scopus, Google Scholar, Science Direct, ACS, Wiley, Web of Science, Springer Link and, PubMed were used to collect all the appropriate information available in previously published literature related to biofilms. In this review, we briefly discuss the different types of biofilms used for wastewater treatment, factors affecting the development, structure, and function of biofilms and their use as potent biological remediation tools to accelerate the breakdown of environmental contaminants, types of bioreactors based on biofilms, and their application in removing various contaminants such as nitrate, bromate, and petroleum waste. In addition, the challenges faced while using biofilms in wastewater treatment and future perspectives have also been discussed.
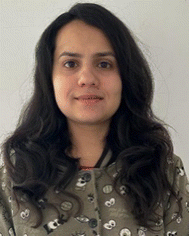
Sonia Saini
| Sonia Saini hails from Jammu, Jammu & Kashmir, India. She has received her MSc in Environmental Science from Central University, Jammu in 2018, qualified NET, and subsequently joined Banasthali Vidyapith, Rajasthan. She is currently a PhD scholar at Banasthali Vidyapith. Her core area of research is nanotechnology, and currently her research work is focused on the synthesis of nanocomposites for water remediation and sensing applications. |
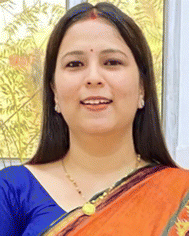
Sanjana Tewari
| Sanjana Tewari has grown up in Uttarakhand, India. She has pursued her bachelors and masters in chemistry from Banasthali Vidyapith, Rajasthan and received her MSc in 2018. She is currently a PhD student at Banasthali Vidyapith. Her core area of research is nanotechnology, and currently her research work is focused on the synthesis of novel nanocomposites for the effective sensing and removal of heavy metal ions in aqueous medium. |
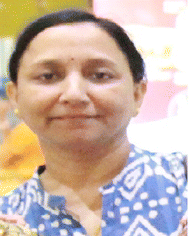
Jaya Dwivedi
| Dr Jaya Dwivedi is working in the capacity of Professor and Head, Department of Chemistry and Dean, School of life sciences at Banasthali Vidyapith, Rajasthan. Her area of specialization is organic synthesis, material development, and natural products. She has published more than 125 research papers in journals of international/national repute. She holds six patents to her credit and has authored various book chapters. She has actively contributed in uplifting the departmental research infrastructure through resource mobilization from various funding agencies, viz., UGC, WTP- DST, DST-CURIE, and FAST-MHRD, India and developed ‘State of Art’ instrumental facility at the department. In addition to institutional projects, she has successfully handled four individual projects. She has supervised 25 PhD research scholars under her direct supervision. She has delivered various keynote lectures in International/National Conferences. |
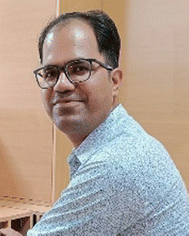
Vivek Sharma
| Vivek Sharma is currently working as Assistant Professor in Department of Chemistry at Banasthali Vidyapith, India. He received his PhD in Chemistry in 2017 from the Indian Institute of Technology Roorkee, under the supervision of Prof. Paritosh Mohanty. His current research interest is the development of multifunctional nanomaterials for energy and environmental applications. He has authored/coauthored 13 research papers published in international reputed journals and a number of book chapters. |
1 Introduction
Although water covers more than 70% of the planet, only 3% of it is safe for human consumption, with the remaining 97% being salty water.1 Globally, water scarcity affects around 4 billion people for at least one month in a year.2 Across the world, water supplies are becoming more limited as a result of rising imbalance between freshwater supply and its demand, thus making access to safe and clean water one of the primary concerns of modern society. Due to hazardous and recalcitrant chemicals, wastewater created from numerous industrial and urban activities greatly threaten public health and environment. Wastewaters containing inorganic and organic pollutants from different types of industries are dumped in the environment, thereby contaminating numerous ecosystems in the process. As a result, timely treatment of urban and industrial wastewater is an important research field for developing technology to improve wastewater quality to meet environmental regulatory requirements.3 Many researchers have focused their research toward creating innovative wastewater treatment processes in response to the environmental challenges created by water contamination.4 To date, physical methods, namely, membrane filtration, adsorption, and coagulation/flocculation, and chemical methods, namely, oxidation, ozonation, and biological treatments have been developed to reduce wastewater discharge and manage pollutant hazards (Fig. 1).5 However, the chemical and physical methods need great energy and have substantial running expenses. The employment of biological agents, such as plants, fungi, and bacteria, is an inexpensive, environment-friendly alternative to the currently used chemical and physical approaches for environmental restoration. Bacteria may be employed for wastewater treatment in a variety of ways, including dead or alive, and immobilized or suspended.6 Ugya et al. investigated the efficiency of microalgae to remediate the polluted water of River Kaduna that results in certain physicochemical factors and contaminants having substantially reduced efficiencies such as Pb (71.0%), Ni (74.0%), Cd (70.0%), BOD (33%), COD (24%), nitrate (42.9%), TSS (66.7%), TDS (9.9%), chloride (11.5%), alkalinity (62.5%), sulfate (37.5%), conductivity (9.8%), and turbidity (71%).7
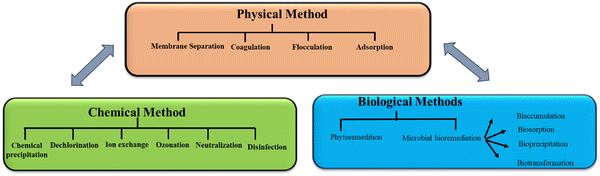 |
| Fig. 1 Water treatment methods. | |
Bioremediation has been known as an environmentally acceptable procedure for the decontamination of harmful contaminants for the safety and preservation of environment due to several limitations (start-up, cost, efficiency, and operation) of traditional wastewater treatment plants.8 Most of the time, microbial populations including algae, bacteria, and fungi are neutralized, mineralized, degraded, and often remove various inorganic and organic contaminants from contaminated environments and wastewater as a part of the bioremediation process.9 Biological remediation is a broadly used remediation method that exploits the metabolic abilities of a broad variety of bio-inoculants for the amelioration and alleviation of contaminants present in environment. It is effective, economically feasible, compatible, and environmentally safe. Diverse advanced environmental studies are being conducted based on the remediation approach and the existing contaminants to create sustainable ex situ and in situ biological remediation procedures for the effective removal and reduction of hazardous pollutants. Ex situ bioremediation treats dangerous pollutants offsite, while in situ biological remediation treats the pollutant on-site. Compared to ex situ procedures, in situ techniques are more beneficial and valuable as they cause less site disruption and have cheaper transportation costs for the amelioration and alleviation of environmental pollutants.10
2 Biofilm
A biofilm is a well-structured group of microorganisms immobilized in a synthesized matrix generated by microorganisms called extracellular polymeric substances (EPS). EPS protects from pollutants, protozoa with predatory nature, and environmental challenges on biotic or abiotic surfaces. Proteins, lipids, polysaccharides, nucleic acids, humic compounds, and surfactants are all components of EPS (Fig. 2).11
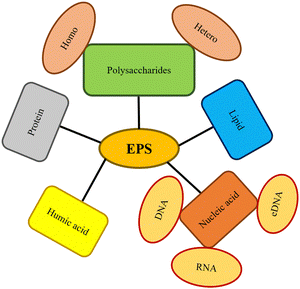 |
| Fig. 2 EPS composition in biofilms (changes from microorganism to microorganism and is impacted by their surroundings).11 | |
Water (97%) is a key element of biofilms and has a significant impact in nutrient flow within the matrix of the biofilm.12 The biofilm layer is roughly 10 to 30 mm thick with the EPS matrix layer thickness of about 0.2 to 1.0 mm. EPS makes up majority of the biofilm (65–95%), with bacteria accounting for the rest; biofilms majorly consist of polysaccharides and proteins, as evidenced from the Fourier Transform Infrared (FTIR) spectra.13 The composition of EPS is largely determined by environmental growth circumstances, bacterial strain, and nutrient supply.
One of the many strategies, biofilm-mediated remediation, has been acknowledged as an affordable and ecologically friendly alternative for cleaning up the environment. Because of their remarkable flexibility, functionality, genetic diversity, and cellular simplicity, microbial communities offer enormous promise for the repair of any damaged environment. Indigenous microbial communities and microorganisms have the capacity to detoxify dangerous metal pollution through biomineralization, biotransformation, biosorption, and bioaccumulation. When compared to the use of planktonic microorganisms, among the different biological remediation techniques, biofilm-mediated remediation has been regarded as a secure, competent, and organized option for the removal/refinement of pollutants. It is currently being used as an innovative remedial alternative for the biological degradation of environmental contaminants.14
In comparison to chemical and physical procedures, remediation techniques based on biofilms are less expensive and simpler to use. In the case of physiochemical approaches, the cost of recovering materials from waste is once again included. However, most research on biofilm-based remediation have relied on monospecies cultures, but almost all biofilm communities in nature that are connected to successful bioremediation include a range of microorganisms.15
Biofilms created from biopolymers may be used in the food sector, packaging, biomedicine, tissue engineering, and pharmaceuticals since they are biodegradable and have high biocompatibility. Because they are a source of clean energy, they are made from renewable resources, and their raw materials are widely available.16
2.1 Biofilm formation
Biofilm formation is an important microbial proliferation process, which has different methods of development, and these are different from growing planktonic organisms in the environment.17 Biofilm production occurs under a variety of conditions, but a majority of them necessitate the use of a wet surface, which can be either man-made, non-living, or natural/living. Biofilm groups that are beneficial have been discovered in a broad variety of habitats, including wastewater treatment plants, rivers, streams, and alimentary canals of mammals. Biofilms may be found in all three kinds of interactions in nature––solid/air, liquid/liquid, and solid/liquid. Hydrocarbons such as fuels, oils, and industrial coolants have been degraded using biofilms grown at liquid/liquid interfaces.18 Physicochemical bonding forces hold EPS components together to manage the architecture of the biofilm during its life cycle. Planktonic bacteria attach to the substrate via van der Waals forces and electrostatic forces during the onset of biofilm development. Then, to increase bacterial adherence, hydrogen forces or ionic interaction forces are used. The architecture of the biofilm is primarily comprised of four phases, beginning with planktonic bacterial adhesion to an unsterilized moist substratum and ending with separation. The 3-D structure of biofilm development and microcolony generation are also engaged between these processes.19 Microcolonies are made up of a single or a group community of bacterial cells that are smooth or flat, rough filamentous, mushroom-shaped, or fluffy, and are surrounded by gaps filled with water.20 The amount of EPS produced in a microcolony is determined by the number of bacteria present. Microcolonies have been discovered to contain 75–90% EPS content, with the remaining being cells. All stages are described in detail below (Fig. 3).11
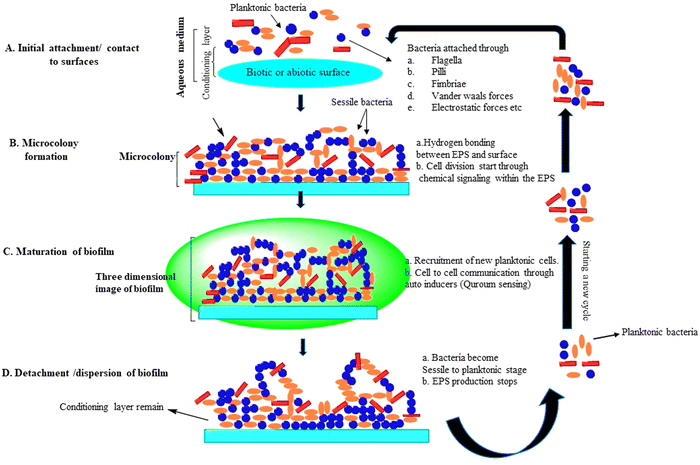 |
| Fig. 3 Life cycle of a biofilm: (A) attachment of microbes, (B) development of microcolonies, (C) maturation of biofilm, and (D) detachment.11 | |
2.2 Microorganisms adhering to the surface
An aqueous media such as blood and water initially favor bacterial adherence to the surface, which is thereafter coated by a layer of conditioning made up of the polymers from the medium. This organic conditioning layer forms within some minutes of contact and keeps expanding for a long time. At this stage of biofilm development, projections on the cell surface such as flagella and pili (structures made of fibers) encourage bacterial adhesion to the surface.21 A variety of physical stresses, such as van der Waals contact, electrostatic forces contact, and various variables, play a role in bacterial attachment to the surface as well. The attachment of bacteria to a surface is called adhesion, while cell-to-cell contact is called cohesion. Flagella, in addition to pili and fimbriae, play a significant part in bacterial adherence to the surface. Another key aspect in the interplay between bacteria with surface attachment is surface hydrophobicity. Enhanced hydrophobicity of the surface causes less repulsion between the surface and bacteria during biofilm development. Biofilm production is aided by planktonic bacteria that naturally interact with the conditioning layer.
Bacterial adherence may be affected by physical surface features such as fibrousness, porosity, texture, and surface roughness in addition to surface chemistry. In another investigation, the surface of HDPE coupons underwent physiochemical modification. It was shown that physical modification improved treatment effectiveness, live cell percentage, biofilm adhesion and development, contact angle, hydrophobicity, and surface roughness. The research that has been done to date shows that the formation of biofilms on polymer surfaces is a complex process that is influenced by extracellular proteins and polymers, initial bacterial concentrations, and the bacterias forming the biofilm.22
In a study, Zhou et al. assessed the effectiveness of the supporting media used in anaerobic attached growth wastewater remediation systems made up of polymethyl methacrylate (acrylic), polyvinylidene fluoride (PVDF), polypropylene (PP), polyvinylchloride (PVC), polycarbonate (PC), acrylonitrile butadiene styrene (ABS), and high-density polyethylene (HD). The results revealed that hydrophobic polymeric materials, such as PVDF and PP, promoted biofilm development (in less than 16 days) and initial cell attachment more effectively than hydrophilic materials, such as HDPE and ABS. The hydrophilic materials did, however, show greater mature biofilm volumes and improved COD elimination as a measure of wastewater treatment efficacy during longer-term and steady-state operation (81 days). These findings imply that the kind of polymer material has an important effect on the growth of biofilms, the variety of bacterial populations, and the efficacy of anaerobic fixed-film systems in treating wastewater. For the anaerobic wastewater treatment process, HDPE and ABS fared better than the commonly applied PVC in the industry and is considered as the most promising and appropriate medium.23
2.3 Formation of microcolonies
Bacterial cells proliferate and replicate to create a microcolony after adhering to an unsterilized surface.27 In other words, the biofilm's intricate composition and purpose is created by the spatial arrangement and cell clusters of these microcolonies in connection to one another. Different micro-communities of bacterial colonies are often involved in biofilm construction. The distribution of nutrients, supply of metabolic products, and elimination of end products are all aided by this control.24 For instance, in an anaerobic biofilm, three bacterial species break down a complex chemical substance into two end products, CH4 and CO2. In the beginning, fermentative bacteria break down complex organic substances to make alcohol and acid. These products are then used as substrates by acetogenic bacteria, and finally, methanogens produce CH4 gas using CO2, hydrogen, and acetate particles in the final stages of the reaction. Biofilms are a good habitat for forming syntrophic bacterial partnerships because it offers a complete environment for two or more bacterial species that vary metabolically to form a bond. Syntropy is the association between two or higher metabolically diverse bacteria that rely on one another for specific substrates to create energy. These polyhydroxy units in extracellular polymeric substances operate as a biofilm's anchoring and enhance bacterial contact to the surface with hydrogen. At this moment, it is difficult for the bacteria to detach from the surface. Mature biofilms have been found to remain in place until they reach the end of their development cycle.25
2.4 Biofilm architecture and maturation
The maturation of biofilms is the next stage just after biofilm creation, where auto-inducer signals are used by bacterial cells inside a biofilm to communicate with one another to achieve appropriate cell density.26 Quorum sensing (QS) is aided by these auto-inducer signals in biofilms, making it easier to produce certain gene products that are required for EPS production at this stage of biofilm development. Because the EPS matrix is such an important feature of a three-dimensional biofilm, water-filled interstitial spaces grow in the extracellular polymeric substances. They operate in biofilms as a systemic circulation, assisting in metabolic product excretion from microcolony bacterial colonies and nutrient supply.27 During the development phase, the biofilm's motive is to expand in the three dimensions. It is performed by recruiting new planktonic bacteria and collecting sand from the environment. During these stages, a biofilm develops due to reproduction, which happens at a regular basis in the microcolonies. In certain situations, biofilms may grow to reach several inches thick.11
2.5 Dispersion
Whenever detachment follows biological patterns, the biofilm's bacterial cell division and multiplication processes occur fast at the beginning of detachment, enabling sessile cells to become motile.28 Some types of bacteria do not manufacture EPS and scatter or get mechanically strained as a result. Dispersion that is passive, such as sloughing and erosion, may occur in the aquatic environment due to shear or hydrodynamic forces and is not generally intentional. Biofilm layers may be shattered due to abrasion caused by nature, shortage of nutrients, and stress caused by hydrodynamics that results from the liquid's velocity.29 A cellular layer, known as the conditioning layer, remains at the surface regardless of how dispersion happens, thus allowing the developmental process to continue or resume. Enzymes that break down sugar aid in the eradication of bacteria from the outer surfaces of the biofilm, and they are sometimes produced by bacterial populations. Some enzymes are beneficial to bacteria for colonizing a new environment, which might lead to the formation of a newly made biofilm and bacteria such as Streptococcus equi, Pseudomonas aeruginosa, and E. coli produce saccharolytic enzymes including hyaluronidase, alginate, lyase, and N-acetyl-heparosanlyase in ongoing detachment procedure for EPS matrix lysis. During this step of biofilm development, bacterial cells focus their efforts on increasing the expression of bacterial flagellum genes, which aids the bacterium in moving to a new site.11
3 Types of biofilms
3.1 Bacterial biofilms
Biofilms are microbial communities that are essential for their survival in harsh environments.30 Bacterial biofilms are described as a self-regulating community of bacteria that have colonized a surface and structured themselves into a community with a structure made up of a self-generated polymeric matrix to enhance their development in the environment.31,32
The competence of bacterial biofilms is influenced by surface proteins, extracellular proteins, capsular polysaccharides, adhesins, autolysin, anaerobicity, carbon dioxide level, glucose, osmotic levels, pH, temperature, ionic concentration, nutritional environment, and the presence of surfactants.33 Mature bacterial biofilms are geographically, temporally, and dynamically heterogeneous communities that depend on a variety of topologies depending on the surrounding environment (osmolarity, pH, shear pressures, temperature, and nutrient availability) as well as on the composition of microbial consortia.34,35
The structure of polysaccharides influences the fundamental shape of bacterial biofilms. It is made up of biomolecules such as DNA, protein, lipids, and organic compounds in addition to polysaccharides. Andersson et al. investigated biofilm formation and adhesion characteristics of thirteen bacterial strains in pure and mixed cultures.35 Turki et al. studied the structure and diversification of bacterial communities in a semi-industrial test facility employing rotating bio-disk approach (RB). They reported that by determining the dominance of bacteria, the existence of useful and beneficial species that may play a vital role in the wastewater purification process was identified. Cronobacter sakazakii, Enterobacter agglomerans, and Pantoea agglomerans were found in a majority of biofilm samples, and these species are well known for their bioremediation potential. Salmonella community detection is frequent, suggesting that the RB system had little effect on Salmonella.36
3.2 Algal biofilms
Algal biofilms are algae-dominated biofilm ecosystems that colonize illuminated surface areas in the presence of moisture and nutrients.37,38 Algal biofilms like bacterial biofilms may form a colony on a surface and separate from the surface in clumps or as a single colony and respond to changes in the environment. Despite their extensive occurrence in the environment, algal biofilms have been studied largely for their structural implications,39 largely for safety and economic reasons, with the purpose of restricting or preventing their expansion.37 However, the need for wastewater treatment solutions for alternative biofuel feedstock, efficient and inexpensive biomass harvesting, and nutrient control methods has recently rekindled interest in algal biofilms.40,41
Producing algal biofilms as a substitute for removing nutrients from wastewater might provide both nutrient remediation and a source of algal biomass for the development of bioproducts.42,43 However, the development of algal biofilm-based wastewater treatment systems faces problems owing to a lack of knowledge on biofilm area requirements, algal growth demands, standard operating procedures, and nutrient removal efficiency for field- or bench-scale testing and operations.44
Li et al. employed microalgal biofilm to extract zinc from the effluent and leachate from a mine dump. They employed Halochlorella rubescens (CCAC 2069 B) and the trebouxiophyte green alga Stichococcus bacillaris to make microalgal biofilms (strains CCAC 1898 B and CCAC 1896 B). S. bacillaris strains were selected for this work due to their effectiveness as heavy metal bioadsorbents,45–47 whilst H. rubescens has previously been employed effectively in two-layer PBRs for treating wastewater.48,49 The results showed that the S. bacillaris strain CCAC 1898 B exhibited the highest zinc resistance. Halochlorella and Stichococcus CCAC 1896 B were found in quasi environments; however, this strain was discovered at a metal waste site's drainage stream at Decazeville, France. It is commonly known that when microalgae are exposed to toxic substances, such as in polluted environments, they might acquire a degree of tolerance to them.50 Furthermore, earlier research has shown that algal strains isolated from polluted settings are capable of removing more heavy metals.51,52
3.3 Fungi biofilms
Fungi, like bacteria, adhere to the basic requirements for biofilm development, which include adhesion to a surface or to one another and encapsulation in EPS. Filamentous fungi have recently been discovered to have hydrophobic protein of amphipathic type, which aids in fungal biofilm growth by allowing the fungus to cling to the surface.53 Hydrophobins act as morphogenetic type cues, allowing fungal spores to adhere to surfaces that repel water (artificial and natural). Eight cysteine residue hydrophobic pattern is secreted by Aspergillus fumigates, creating significant attachment forces during spore adherence with the surface.54Candida albicans, Penicillium rubrum, Fusarium species, Acremonium, and Neocosmopora are examples of fungal species that can create biofilms.55 However, in diverse microbial biofilm in clean water delivery methods, fungi's variation is lower than bacterial variation. Howell and Shepperd compared C. albicans and Candida fumigates biofilms to bacterial biofilms to acquire a better knowledge of their biological function, biosynthesis, and structure.56 Despite the fact that the sequence homology of glycan materials and fungi and bacteria have different biosynthetic enzymes, both are able to construct antibiotic resistance strategies involving immune evasion and biofilm formation. Despite a range of treatment techniques, enzyme-based therapy procedures to suppress and deconstruct biofilm components are emerging as potential future options for better treatment of skin diseases.56
3.4 Symbiotic biofilms
Physiological efficiency has been consistently shown in complex multispecies biofilms.57 Biofilms with multicultures produced 70% higher cellulase than biofilms containing just one species.58,59 Because successive gastrointestinal processes are helped by the superposition of multiple species that work together within a multilayer biofilm matrix, multispecies synergy is particularly important in the case of solid substrate breakdown.60
3.4.1 Fungi and bacterial biofilms.
Fungi and bacteria biofilms have the potential to increase biological delignification. In nature, there is a lot of synergy between fungi and bacteria. Bacteria and fungi are the only species that can degrade large plant polymers such as chitin and lignin, and break down cellulose into small molecules that biota may absorb.61 Fungi are much more effective than bacteria in utilizing resources available through enzymatic hydrolysis and invasion of leaf material and lysed hyphae; hence, there is generally more fungal biomass on leaves than bacterial biomass.62,63 Bacterial activity supplies growth factors to fungi in return; for example, nitrogen-fixing bacteria enhance the quantity of nitrogen available for fungal development.64,65 Bacteria consume fungal compounds and synergy develops, thus allowing the activity of fungal enzymes to increase. It has been proven that a large number of tiny molecules induce fungi to produce enzymes that need to be suppressed, which enhance severe bacterial infection due to significant reduction in metabolite concentrations to avoid this kind of feedback regulation.60,66
3.4.2 Protozoans and bacterial biofilms.
Biofilm architecture is influenced by protozoans not only via their own structures but also through their feeding activities on biofilm microorganisms. The spatially patched feeding activity of protozoans may increase the temporal and spatial heterogeneity of the bacterial biofilm.67,68 Amoebae's behaviors (feeding on diatoms, migration between biofilm and substrate) were thought to be crucial to biofilm disintegration and sloughing. Because of their abundance and distinct characteristics, protozoans may have a significant influence on biofilm architecture.69 Because of the intricate entanglement generated by their stalks, Cereceda et al. highlighted the critical role of peritrich ciliates in sustaining the layered biofilm structure in a full-scale treatment plant.70
Protozoans can constantly modify the 3-dimensional structure of biofilms because they have extracellular structures including loricae, shells, and stalks that can be present in biofilms long after cell death. Protozoans may improve the movement of biochemically essential solutes into and through the biofilm, in addition to their impact on biofilm architecture. Benthic ciliates have been shown to increase extracellular solute transport in sediment biofilms by a factor of 1.1 to 10 due to their cilia motility.71,72
3.4.3 Microalgal and bacterial biofilms.
Water treatment may be aided by microalgal-bacterial consortiums,73,74 providing enhanced nutrient removal, minimal maintenance, and self-sustaining oxygen.75,76 Suet al. used microalgae and activated sludge to obtain a synergistic connection to enhance wastewater treatment, achieving nitrogen and phosphorus reductions of 91 and 93.5%, respectively, using a 5
:
1 algae-to-sludge ratio.77Fig. 4 illustrates the interaction of microalgal-bacterial consortia and the structural development of microalgal bacterial biofilm.
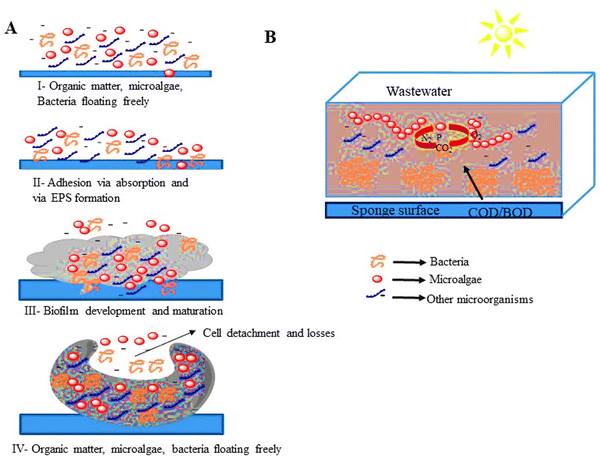 |
| Fig. 4 (A) Microalgal bacterial biofilm structure and development. (B) Main biological interactions between microalgal-bacterial consortia.86,87 | |
A xenic culture fluid was employed in another study to enhance Chlorella vulgaris flocculation; it was discovered that bacteria connected to microalgae increased floc size for better microalgal flocculation, resulting in increased sedimentation and better microalgae harvest.78 Using a symbiotic microalgal bacterial consortia in a photobioreactor to treat municipal wastewater, TKN (Total Kjeldahl Nitrogen) and COD removal efficiency was reported to be 87% and 98%, respectively.79 Gou et al. built an efficient reactor system that increased the COD and rate of removal of ammonium to 90% in a 12 h retention time.80
Microalgae for raising oxygen and nutrient reduction (organic compounds, phosphorus, and nitrogen) in polluted water may be less expensive than typical treatment procedures. Several ranges of water quality indicators, such as DO (dissolved oxygen), temperature, and pH, have been acknowledged for wastewater remediation employing a bacterium and mixed microalgae culture.74 DO profiles may affect the symbiosis between bacteria that use microalgae and oxygen that create it. For example, Mhedhbi et al. discovered that DO varied from 2–11 mg L−1 when the microalgae first started generating oxygen and gradually decreased over the dark phase.81 Microalgal growth (mixotrophic microalgal culture and/or CO2 absorption by autotrophic) and/or excretion from organic matter biodegradation elevated the pH from 7.8 to 10.0, according to Mirquez et al.82 Depending on the type of bacteria and microalgae, microalgae cultures can develop at temperatures ranging from 10 to 45 °C, with ideal conditions between 20 and 25 °C,83 while bacterial cultures may thrive at temperatures ranging from 0 to 49 °C.84 Several studies have employed immobilized biofilms of microalgal-bacterial consortia as a secondary treatment for wastewater; however, additional study is required to increase surface attachment.85
4 Types of bioreactors based on biofilms
The progression of biofilm-based bioreactors as a technology for wastewater remediation processes has shown potential. Biofilm-based systems provide a number of benefits, including improved pollutant removal effectiveness, minimal sludge generation, shorter hydraulic retention times (HRT), presence of EPS, high concentrations of active biomass, and high diversity. As a result, a variety of biofilm-based bioreactors have been widely applied to remove pollutants from highly contaminated wastewater.88 Biofilm reactors are primarily made up of five compartments, with certain additional components that are specific to a particular reactor type: (1) containment structure; (2) influent, which refers to wastewater having a specific level of the contaminant of concern; (3) biofilm substratum or carrier, which refers to the material used for microbial cell attachment and growth; (4) system for collecting wastewater; and (5) mixing system or aeration for carrier distribution and agitation.89
4.1 Membrane biofilm reactors
Membrane biofilm reactors (MBR) have long been hailed as a viable biotechnology for the removal and/or recovery of contaminants from water. It fundamentally entails the breakdown of biological waste components via physical separation and a system based on biofilms via a membrane module that replaces the secondary settlement. It is used to treat a number of urban and industrial wastewater, demonstrating excellent eradication of both inorganic as well as organic matter.90 It has specific advantages over other treatment systems.
MBRs, unlike traditional filtration membranes, do not separate liquids from solids. Rather, a gaseous substrate is transmitted across the membrane, such as oxygen or hydrogen. On the outer membrane surface, a biofilm grows naturally, triggering desired reactions. Exogenous substrates such as gaseous substrates can be ideal because they leave nothing behind in the bulk and are often affordable or produced on-site. Unfortunately, the traditional gas delivery method of aeration or bubbling is energy intensive and limited by their low solubility. Furthermore, a majority of the gas is lost to the atmosphere. Compared to suspended processes, MBR has the same advantages as other biofilm reactors (e.g., IFAS, MBBR)––higher biomass concentrations in the tank, support for slow-growing microorganisms, and generation of fewer solids (e.g., activated sludge). Infact, it does produce high effluent quality and lower sludge generation. It also has a lot of flexibility when it comes to disinfection efficiency, tremendous volumetric loading, and influent fluctuation.91,92
It is possible to use it in two ways, namely, the exterior or side stream configuration and second, the submerged or immersed configuration. Yamamoto et al. proposed the submerged configuration in 1989, which involves the membrane module being immersed in the reactor directly.93 Negative pressure on the permeate side or pressurization of the bioreactor provide the driving force in this configuration. This configuration has a number of benefits, including lower energy usage, cheaper running costs, and fewer cleaning processes. The development of this system has been aided by these benefits. Outside the bioreactor, the membrane is put in the external configuration, allowing a mixture of spirits to be recirculated. This setup makes membrane replacement and control simple. In this setup, the bioreactor's high cross-flow velocity (CFV) serves as a driving force.94,95 MBR systems have been employed successfully for a range of industrial effluents to date because of their numerous advantages, the most notable of which is their decreased energy usage. The creation of air-lift side stream MBRs has recently emerged as a new configuration.92 The basic concept behind this configuration is to use the side-stream airlift principle to make use of all of the abovementioned benefits of MBRs.96,97 For the remediation of urban and many types of industrial wastewater, this concept is highly effective and much work is still being done to improve its applicability.98
To promote the growth of biofilms, a medium may be added for moving or fixed-bed arrangements, or aerated membranes can be added to the bioreactor, which could be used to facilitate biofilm processes in MBRs. For biofilm support, a variety of materials are available. Granular activated carbon (GAC), sponge, cord media, plastic media, and RBC media have all been used in full-scale systems commercially to date.99 This approach has been shown to be successful in the removal of a variety of contaminants and it allows a degradation efficiency of more than 90%.100,101 In subsequent testing, it has demonstrated good elimination of COD and inorganic nitrogen.102
Membrane biofilm bioreactors have emerged as a popular and effective treatment solution for home and industrial wastes. However, membrane fouling and clogging layers, as well as the repercussions for plant maintenance and operating expenses, limit its broad implementation. The demand for immense energy associated with air scouring and the high cost of membranes further limit its widespread implementation. Membrane fouling is a typical occurrence in membrane-based systems, especially MBRs, which is mostly caused by soluble and colloidal organic content, such as suspended particles, EPS, or biopolymers or physical qualities.99 Controlling membrane fouling mechanisms and discovering economical membrane materials have been the focus of many studies to address these drawbacks. It also aimed to reduce energy usage, making this system more realistic and trustworthy than activated sludge and other traditional technologies.89,99 Based on the application, we can classify MBRs into 3 types, as discussed below.
4.1.1 Oxygen supplying MBRs.
MBRs are well-suited for high-strength biological oxygen demand (BOD) oxidation due to their high gas transfer rates of air or oxygen. Due to the high yields and rapid growth rates of heterotrophic bacteria, excessive biofilm formation is a key problem in these systems. A pilot-scale study found that regular biofilm washings successfully cleaned high-strength brewery effluent.103 When BOD and ammonia are supplied via the bulk liquid, oxygen-based MBRs support unique biofilm communities capable of concurrent BOD oxidation and complete nitrogen removal. Nitrifying bacteria live near the membrane, where oxygen is plentiful, and oxidize ammonia to nitrite and nitrate. They produce anoxic conditions for fast-growing, denitrifying bacteria that live in the outer biofilm layer by devouring oxygen. BOD from the bulk liquid and nitrate from the nitrifying bacteria are consumed by the denitrifying bacteria. The layered microbial community structure of this biofilm has been validated by a number of experimental investigations and modelling approaches.104–107 Researchers have also shown that oxygen concentrations may be manipulated to encourage the formation of nitrite rather than nitrate by changing intra-membrane pressure.108 Thick biofilms, unfortunately, can disrupt the community structure. If the denitrifying layer is too thick, mass transfer resistance prevents ammonia from reaching the nitrifying biofilm, resulting in poor activity or loss of nitrifying bacteria.109 Nitrification occurs along the membrane surface in the aerobic inner sections of the biofilm, while anaerobic ammonia oxidation occurs at the outside borders.110,111
4.1.2 Hydrogen-supplying MBRs.
Hydrogen is a powerful electron acceptor. Its advantages include reduced biomass yields, low toxicity to humans, and extensive utilization as an electron donor by a varied population of bacteria.112 Low solubility issues and the risk of a flammable headspace have limited the number of full-scale gas sparging installations.113 MBR provides a safe method of delivering hydrogen while also increasing gas transfer rates. Hydrogen is used within the biofilm before reaching the bulk liquid, which is supplied at high quantities by pressured membranes. Hydrogen can be produced on-site using electrolysis or methane reforming, which is a bonus.91
MBRs based on hydrogen have been shown to be effective against a wide spectrum of inorganic and organic pollutants. Perchlorate, chlorate, bromate, chromate, selenate, arsenate, and dichloromethane are among the inorganic oxidizing pollutants that can be reduced by hydrogenotrophic biofilms using nitrate or oxygen as the major electron acceptor.114–118 Hydrogen can also engage in nitro reduction and reductive dehalogenation for the treatment of chlorinated solvents, chlorophenols, and chloronitrobenzenes under anaerobic circumstances.119,120 For these applications, nitrate or sulphate could be used as the principal electron acceptor.
4.1.3 Novel MBRs.
MBRs have been studied with methane and carbon dioxide, two inexpensive and abundant gases, in addition to hydrogen and oxygen. Methane-based MBRs support methanotrophic bacteria, which can co-metabolize organic molecules and act as an electron donor by releasing decay products.121,122 To support methane gas production, Ju et al. supplied an MBR with a mixture of carbon dioxide and hydrogen (ratio 1
:
4).123
The extractive membrane reactor, for example, transports contaminated gas or liquid across the membrane's lumen. This configuration prevents the biofilm from being exposed to toxic chemicals in the waste stream by avoiding air-stripping of volatile organic compound contaminants, spatially separating competing substrates (one in the bulk liquid, the other in the membrane lumen), and preventing the biofilm from being exposed to toxic chemicals in the waste stream.91
4.2 Moving bed biofilm reactors (MBBR)
The MBBR is a wastewater treatment method based on biofilms that is currently being used in over fifty countries. It was invented in Norway between 1980s and 1990s.124 The MBBR plants have been a huge success and are employed for municipal and industrial wastewater treatment.
This technique is based on combining the greatest aspects of both the activated sludge and biofiltration processes. Aerobic, anoxic, and anaerobic processes can all be carried out in this reactor. In this method, the carriers that function as biofilm growth housing move about freely within the tank capacity. The movement of the carriers is driven by the unrest created by the gas in aerobic processes, whereas mechanical mixing keeps the carriers moving in anaerobic and anoxic processes. K1 is the most often utilized biofilm carrier in MBBRs, which are continuous-flow reactor units. They take the form of a cylinder made of polyethylene with a high density (0.95 g cm−3) featuring “fins” on the outside and a cross on the inside. With these carriers, the bioreactor does not have a filling portion; it can range from 25% to 70% of the whole volume of the tank. It is, however, suggested that it can be maintained below 70% (for K1, the effective specific area is 350 m2 m−3). On the inside of the plastic containers, biofilms flourish, where they are sheltered abrasion from the outside. The use of movable beds has the positive effect of decreasing congestion and allowing the bioreactor's entire volume to be utilized. In the reactor, there is turbulence caused by forces of shearing, which ensures not only proper compound diffusion with the biofilm but also a thin biofilm. Furthermore, this method removes or lowers the requirement for biomass recirculation, which is a major concern in activated sludge systems and fixed bed biofilms. The extra biomass must only be removed from the solution.99
Because of the carrier materials, a moving-bed biofilm reactor has larger accessible surface for growth and adhesion of microbes than a fixed or fluidized bed biofilm reactor. Furthermore, it provides effective mixing within the reactor, which favors biogas liberation and the distribution of volatile acids in an aqueous medium.125 Combining moving-bed biofilm bioreactors with other treatment procedures has already been explored for different treatment processes. Indeed, the use of this method in conjunction with flotation and coagulation for higher-rate secondary treatment has been previously documented. MBBR has been shown to be a credible, resilient, and compact wastewater treatment reactor that may be used for nitrification, denitrification, carbon oxidation, and as a single stage or in combined systems,126,127 with satisfactory operating results at both lab and larger scales. To benefit the reactor components, the carrier must be removed, which is a recognized drawback of these systems.
In MBBR, unlike most biofilm reactors, for biomass growth, the full tank capacity is used. It also has a lower probability of head loss. It does not require sludge recycling, unlike the activated sludge reactor. It is accomplished by biomass production on carriers that float freely in the reactor's water volume and a sieve structure keeps them confined at the reactor's output. As shown in Fig. 5, the reactor can be employed for aerobic, anoxic, or anaerobic operations.128
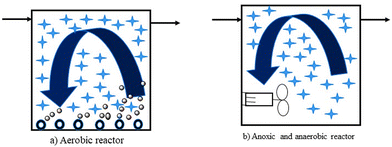 |
| Fig. 5 Principle of biofilm carriers in aerobic and anaerobic reactors.128 | |
The movement of biofilm carriers in aerobic processes is generated by the turbulence created by air, whereas in anaerobic and anoxic processes, the carriers are kept moving by a mixer (often a horizontal shaft mounted banana mixer). A specific coarse bubble aeration mechanism has been devised for aerobic reactors. Special filter arrangements have also been designed to keep the biofilm carriers within the reactors. It can be rectangular mesh sieves positioned vertically, but more recently, the sieve has been formed as a cylindrical bar sieve mounted horizontally or vertically. For the MBBR process to perform optimally, the design of aeration grids and sieves is important.128
4.3 Fluidized bed biofilm reactors
Fluidized-bed biofilm reactors (FBBRs) employ tiny carriers to build a bed within a column that is maintained in fluidized movement by running wastewaters, and the bed increases as a result. A recycling line is utilized inside this system to keep up with the vertical hydraulic velocity of the constantly introduced wastewater. Aeration occurs when influent wastewater combines with effluent recovered from the top of the bed during recycling. It is possible to introduce air into the recycling stream; however, this has been demonstrated to generate turbulence within the reactor, which might shear the adherent biofilm off the carriers.
In general, medium particles in FBBRs are distributed in a size gradient from the bioreactor's top to bottom. Depending on the size of the particles that have expanded, the bed is characterized as inflated or fluidized. There are several biofilm-supporting materials available such as silica-based compounds,129 zeolite, and GAC,130 which have been employed in this system. In pilot-scale studies, small materials (<1 mm) were used to improve the active surface area, which is a key aspect of this approach. The microscopic particles get suspended and separated when the driving power of the flow rate within the bioreactor is greater than gravity (30–50 m h−1).131
In comparison to fixed biofilm reactors, FBBR is commonly found to have greater mass transfer properties. In the instance of municipal wastewater treatment, it has demonstrated its efficacy for tertiary denitrification. FBBRs are used in wastewater treatment to remove oxidized pollutants.132
The advantage of fluidizing the media particles is that it increases the surface of contact between effluents and microbial cells. This also helps in mass transport and, as a result, efficiency of treatment. A modest extent of the bed's extension, on the other hand, is encouraged since it reduces flow velocity, saves energy, and enhances the amount of biomass that is effective. The number of connected FBBR microbial cells is critical since it indicates a high level of microbial diversity. This setting allows the system to quickly recover from changes under unstable circumstances.133,134 Despite this, due to the high biomass concentration, it increased volumetric oxygen biomass. Puhakka et al. utilized Rhodococcus sp. and Pseudomonas sp. in a lab-scale fluidized-bed biofilm reactor to remediate chlorophenol-contaminated groundwater. The treatment allowed for considerable mineralization of chlorophenols effectively at chlorophenol rates with loading of 1000 mg L−1 d−1 and hydraulic retention periods of less than 1 h (above 99.9% removal efficiency of pentachlorophenol, 2,4,6-trichlorophenol, and 2,3,4,6-tetrachlorophenol).
The actual idea behind the procedure is to pump wastewater upward through a densely packed particle bed at a high enough speed to fluidize or move the particles. As the wastewater rises through the biological bed, extremely concentrated organisms living on the surface of the bed particles devour the biodegradable waste pollutants in the wastewater. A basic unit of the process is depicted in Fig. 6 as a fluidized bed reactor with wastewater moving upward through the bed, fluidizing the particles in the liquid. A clear water zone exists above the bed, where the liquid and the particles separate.
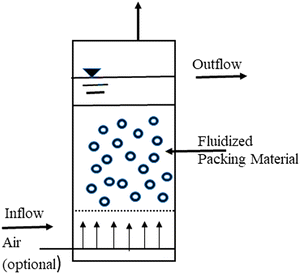 |
| Fig. 6 Fluidized bed biofilm reactor.135 | |
The associated microbes on the suspended particles could be any of the aerobic, facultative, or anaerobic organisms commonly found in trickling filters and suspended growth treatment systems from a biological standpoint. The dominant species would be determined only by the waste pollutant consumed, whether an aerobic or anaerobic environment is maintained, and other parameters affecting biological growth.135
4.4 Microbial fuel cells
Microbial fuel cells (MFCs) have the potential to solve a number of issues concerned with traditional wastewater treatment. They lower the energy input and superfluous sludge production while enabling energy recovery from wastewater. The chemical energy stored in biological materials is converted into electricity in this method, which provides for the simultaneous resolution of two current global challenges—wastewater depollution and green energy production.136
MFCs are made up of a certain microbial species that can contribute to current generation using an electrode to exchange an electron network. Electroactive biofilms (EAB) are formed when microbial cells develop a biofilm on the electrodes' surface. MFCs have been successfully used with pure cultures of several bacterial species in a number of articles. Mixed culture MFCs, on the other hand, have outperformed pure culture MFCs, generating significantly higher power densities.137 MFCs can contain a wide variety of microorganisms, according to community analysis; however, algal and bacterial species are the most used.138 MFCs are available in a variety of configurations. An anodic and cathodic MFC is made up of two chambers connected by a conductive substance including a resistor or operated under load (Fig. 7). Separate electrochemical and biochemical reactions take place in these chambers. The organic matter in the anodic chamber (negative terminal) is oxidized by the microorganisms that make up the biofilm, leading to the creation of protons and electrons. After that, the electrons move toward the anode and travel to the other side of the MFC through an external connection, which contains the cathode. Using shuttles or electron mediators, electrons can be delivered to the anode through bacteria-produced nanowires or direct membrane-associated electron transfer.139 Oxygen serves as an electron acceptor in the cathodic chamber. Electrons and protons diffuse from the anode to a separator, where they interact with oxygen under aerobic conditions to form water molecules.140
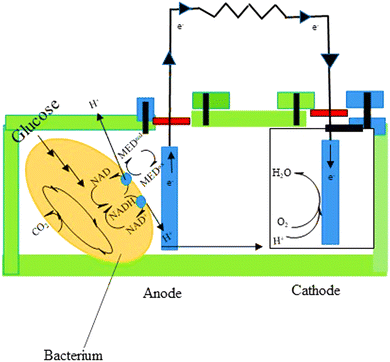 |
| Fig. 7 The principle of MFC.139 | |
Presently, MFCs are utilized to generate power and treat organic and inorganic wastes. Important influencing aspects such as design, construction materials, and voltage generating processes are currently receiving great attention.141–143 MFC devices have made significant breakthroughs in terms of the generated power density,144 which has risen dramatically after numerous research studies from a baseline less than 0.1 W m−2 to nearly 7 W m−2.145,146
MFC technology has demonstrated its efficacy in the treatment of a wide range of contaminants. Many industrial wastewaters, such as food-processing effluents147 and refinery wastewater, have been employed as inoculums in anode chambers.148 Indeed, heavy metal-rich wastewaters (with a great possibility for reduction in potential) might be utilized as an electron acceptor alternative. As a result, the inorganic stuff in the container is reduced to non-hazardous or less toxic forms. It also demonstrated its excellent capacity to denitrify without the use of a supplier of exogenous electrons as the electrons released for the oxidation of organic substances are passed to the cathodic chamber, aiding nitrate reduction.149 Furthermore, the MFC technique is suited for the treatment of high-strength wastewaters due to the anaerobic conditions in the anode chamber (COD greater than 8000 mg L−1)
Microbial fuel cells are touted as a viable biotechnology for electricity generation and removing contaminants owing to their energy efficiency, low biomass production, and COD elimination without further oxygenation. However, significant obstacles remain in the way of its practical use. To improve MFC performance, it was suggested that the reactor capacity should be increased; nevertheless, larger MFCs produced disappointing results.
These constraints are primarily encountered when laboratory-size experiments are scaled up to pilot scale. As a considerable fact, maintaining the generated power density in larger systems is difficult. With the growth in the MFC size, the volumetric power density tended to drop, but a minimum threshold volumetric power density of 1 kW m−3 should be maintained.150 This can be attributed to ohmic losses and low conductivity of wastewaters. However, there were some outstanding achievements. Indeed, the larger reactor size gave a twofold volumetric power density to the significant cathode specific area.151 These findings demonstrated that the power density may be maintained in MFC scale-up with careful control of the key elements influencing the system performance. Indeed, increasing the cathode specific area using electrodes with a three-dimensional structure showed its capacity for improving the MFC performance and overcoming this constraint. This technique proposes increasing the accessible surface area for microbial adhesion while keeping the MFC volume the same.152 For MFC scale-up, the parallel connection of microbial fuel cell in sequence is commonly used. The shortcomings of the previous method including voltage reversal and operating instability were demonstrated.153,154 Furthermore, the voltage loss caused by the substrate cross-conduction effect may severely limit full-scale operation. When two independent stacks were serially coupled with both hydraulic and electrical connections, this phenomenon was discovered.155
4.5 Trickling filter
For more than 60 years, many types of biological trickling filters (BTFs) have been employed to treat wastewater. The development of BTFs in their early stages was done on the basis of empirical knowledge of treating wastewater.156 It is a three-phase biofilm reactor with a pump station for recirculating influent, a clarification unit, and a TF (Fig. 8). It usually consists of (1) a wastewater distribution system, which is used to introduce wastewaters into the reactor, (2) a containment system, (3) a medium for support, (4) a drainage system under the ground, and (5) a system for ventilation. The removal of suspended solids from wastewater treated with TFs necessitates further liquid-solid separation since the TF treatment produces total suspended solids. Secondary clarifiers, either circular or rectangular, are commonly used for this step.157
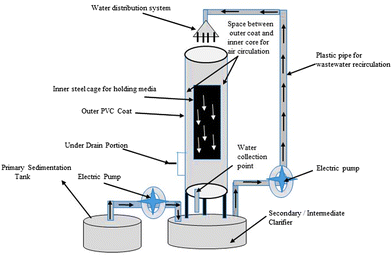 |
| Fig. 8 Setup of a biological trickling filter (BTF) for wastewater treatment in a laboratory-sized area.157 | |
For influent distribution, the rotatory distributor is ideal because it enables periodic wastewater application as well as better substratum soaking. These parameters prevent dry pockets and odour emission, as well as allow the biofilm to rest and serve as a process aeration mechanism.131 Natural ventilation may be provided by the temperature differential between air inside the trickling filter and ambient air. When there is a temperature differential between the two, the oxygen dose delivered is insufficient. In this example, the air supply could come from an underdrain system that collects the treated influent in a chamber beneath the trickling filter.
The ability of TFs to satisfy the treatment goals in terms of nitrification and carbon oxidation has been demonstrated. For carbon oxidation, the biofilm on the filter medium that is being sustained needs oxygen in the form of air. When a solid separation is included in the treatment train, TFs are ideal for carbon oxidation and combined carbon oxidation and nitrification. With a combined oxidation and nitrification, good nitrification outcomes have been reported, with ammonia concentrations less than 3 mg NH4+ N L−1 and BOD concentrations less than 10 mg L−1. When nitrification is the primary treatment goal, the ammonia concentration is significantly lower.
The selection of filter media is an important factor to consider while designing TFs. For a long time, the most often used materials in this system were gravel and stones. However, these materials restricted air movement in the filter, limiting the amount of oxygen available for microbial biofilm formation. This issue limited the amount of treated wastewater available, as well as the specific surface area available for microbial attachment that can support the reactor's BOD load. Furthermore, while handling high organic loads, stone bed trickling filters were limited by void space blockage caused by increased microbial cell development. However, at low organic loading (i.e., 1 kg BOD5 d−1 m−3), rock-media TFs were able to give excellent treatment performance.89
Other materials, such as plastic rings, zeolite, ceramsite, sponge, and others, have been employed to circumvent these constraints.158 These tiny particles improved oxygen transmission and biofilm thickness management as compared to the rock medium. TFs using plastic modules have specific surface area between 89 to 102 m−2 m−3 and are suitable for carbon oxidation and combined carbon oxidation and nitrification.131 The trickling filter, on the other hand, has the drawback of not being a volume-effective system. Table 1 illustrates various bioreactors used for the treatment of various pollutants.
Table 1 Traditional bioreactors for the removal of different pollutants
Contaminants |
Bioreactors |
Ref. |
NO3− |
Methane-based MBR |
159
|
BrO3− |
Methane-based MBR |
160
|
Tetracycline |
Hydrogen-based MBR |
161
|
Sulfur-containing organic pollutants |
Membrane aerated biofilm reactor (MABR) |
162
|
Petroleum wastewater |
Moving bed MBBR |
163
|
Industrial waste water |
Fluidized bed biofilm reactor |
164
|
Organic contaminants |
Microbial fuel cells |
165
|
p-Chloronitrobenzene |
Hydrogen-based MBR |
166
|
5 Wastewater treatment using biofilm
5.1 Pollutants of organic origin
Because of their large microbial bio-mass and immobilizing capabilities, bacterial biofilm communities are much resilient and show stronger resistance to organic contaminants. Thus, by creating extracellular enzymes via the carefully regulated gene expression profile controlled by the cell communication signalling mechanism known as quorum sensing (QS), biofilm communities may participate in the sorption and metabolism of heavy metal contamination and organic contaminants.167
Many human activities including natural gas production, crude oil extraction, and mining have resulted in a significant increase in environmental pollution. Dyes, oils, pesticides, heavy metals, herbicides, phenolic compounds, and polyaromatic hydrocarbons (PAHs) are among the most harmful and refractory xenobiotic wastes generated by these activities. Because of their negative effects on the environment and human health, these compounds are classified as toxic and carcinogenic.168 This contamination extended across 5 million places on Earth, with petrochemical chemicals polluting about 67% of them.169
Industrial wastewater contains acetonitrile, a water-soluble hazardous chemical that is metabolised to poisonous HCN and C2H4O compounds in living creatures, posing a serious health risk to aquatic and terrestrial species. Li et al. utilized a mixture of three biofilm-forming bacterial strains, B. subtilis N4, E2, and E3, along with an acetonitrile degrading bacterium to reduce acetonitrile toxicity (Rhodococcusrhodochrous BX2). In a similar investigation, the recombinant bacterium capable of generating biofilms (B. subtilis N4-pHT01-nit) was found to be extremely capable of degrading higher concentrations of acetonitrile (800 mg L−1) over the next 24 h when used in conjunction with MBBR.170 The degradation of diverse organic and inorganic contaminants is very significant to biofilms utilized in bioremediation and wastewater treatment operations (Tables 2 and 3). As a substrate, biofilm effectively decomposes or degrades organic substances. Furthermore, enzymes involved in the breakdown process can be kept in the biofilm EPS near the producing cell, increasing the likelihood that both the product and the enzyme will not be separated and utilized by other cells.28
Table 2 Biofilm-mediated bioremediation of inorganic micropollutants and organic contaminants from wastewater
Biofilms |
Wastewater type |
Contaminants |
Ref. |
Gordonia sp. H19 and P. monteilii P26 |
Artificially created seawater |
Crude oil |
171
|
Batch-dispersed biofilm reactor sequencing based on algal-bacterial interaction |
Sewage from the home |
Total phosphorous and total nitrogen |
172
|
MBBR |
Sewage from the hospitals |
Trimethoprim, propranolol, diatrizoic acid, clarithromycin, and azithromycin |
173
|
Sphingobacteriales, Flavobacteriales, Xanthomonadales, and Burkholderiales |
Sewage from the hospitals |
Drugs |
174
|
Batch biofilm reactor using algae as a sequence agent |
Sewage from the home |
Phosphorus and Nitrogen |
175
|
MBR |
Wastewater with saline conditions |
Ammonium and COD |
176
|
MBBR |
Sewage from the city |
Medicines |
177
|
MBBR |
Sewage from the city |
4-Nonylphenol, 17β-estradiol, naproxen, and diclofenac |
178
|
B. mojavensis M1+ R. rhodochrous BX2 |
Groundwater |
Organic cyanide |
179
|
Arthrobacter sp. |
— |
Chromium(VI) |
180
|
B. atrophaeus CN4 |
— |
Naphthalene |
181
|
Trichosporonasahii B1, Candida tropicalis TH4, and Candida viswanathii TH1 |
— |
Pyrene, anthracene, naphthalene, and phenol |
182
|
Microalgal biofilm |
Sewage from the city |
Phosphorus and nitrogen |
183
|
Chlorella vulgaris
|
Wastewater contaminated with aromatic hydrocarbons that was collected from petroleum storage |
Removing nutrients |
184
|
Table 3 Traditional biofilms for the removal of different contaminants
Biofilms |
Contaminants |
Ref. |
Algal-bacterial biofilm |
Nitrogen |
215
|
Phosphorous |
Kaolin-supported bacterial biofilms |
Cr(VI) |
216
|
Zn(II) |
Algal-bacterial biofilm |
Nitrogen |
217
|
Algal biofilm |
Sulfonamides |
218
|
Fungi biofilm |
Pharmaceuticals contaminants |
219
|
Microbial biofilm |
Polycyclic-aromatic hydrocarbons |
220
|
Fungal–bacterial biofilm |
Crude oil |
221
|
Bacterial biofilm |
Hydrocarbon |
222
|
Fungi biofilm |
Mercury |
223
|
Fungi biofilm |
Cr(VI) |
224
|
Fungal–bacterial biofilm |
Cd2+ and Pb2+ |
225
|
Fungal–bacterial biofilm |
Cr(VI) |
226
|
Biofilms have greater biomass than suspended cells, which defends cells from chemical damage. Furthermore, biofilm was found to be more efficient at degrading toxicants as the physicochemical structure of the biofilm matrix optimizes compound availability and sorption while allowing aerobic and anaerobic bacteria to coexist.185 It was discovered that flocks (associated biofilms) of anaerobic denitrifiers and oxygen-dependent nitrifiers coexisted in biofilm by occupying distinct levels and efficiently removed nitrogen from wastewater.186,187 Anaerobic ammonium oxidation (anammox) bacteria were found to have a similar relationship in the establishment of microenvironments with the existence of aerobic ammonia-oxidizing bacteria. Due to gradient niches, reactions with various oxygen and substrate needs can occur in the same biofilm in this form of interaction. When compared with single-species biofilms, multicomponent biofilms exhibited more efficient bioremediation. This has been noticed during the breakdown of PAHs employing multispecies biofilms and it has been linked to the addition of emulsifier makers. Emulsifiers are well-known for lowering the surface tension between surfaces, compounds, and cells, resulting in increased substrate availability on cell surface adhesion.188 The genes that code for emulsifier synthesis are found on plasmid DNA, which is routinely passed between bacteria in PAH breakdown by multispecies biofilms.189 Yoshida et al. observed that when Burkholderia sp. NK8 was cocultured in a biofilm with P. aeruginosa PAO1, the breakdown of 3-chlorobenzoate was increased. Despite its inability to degrade 3-chlorobenzoate, P. aeruginosa aids in the production of Burkholderia sp. NK8 biofilms,190 and as a consequence, deterioration was tenfold enhanced when compared to monospecies biofilms, demonstrating that multispecies biofilms may be used for biocatalysis and bioremediation.191 Another research discovered that biofilm process for energy-efficient carbon removal from wastewater was dominated by a glycogen-accumulating organism that was passively aerated.192
In the cytoplasm, EPS, and cell walls, biofilms feature distinct and variable sorption processes and binding sites (anionic and cat-ionic). Even in low concentrations, a diverse group of pollutants, including hormones, phenolics, medicines, and poisonous chemicals, can be bounded and stored for possible ingestion by biofilm cells.193–195 Despite the lack of obvious lipophilic binding sites, slightly hydrophilic chemicals such as xylene, toluene, and benzene can develop in the EPS matrix. The sorbed chemicals disintegrate or persist in the biofilm till decomposition. Higher toluene concentrations express a greater amount of anionic carboxyl groups inside the EPS of P. putida biofilm, resulting in an increase in its cation binding ability. Other ions, such as phosphate ions, also collect within the dental plaque biofilm to improve the biofilm's mechanical resilience.196 Suspended biodegradable materials, in addition to ions, can be settled in EPS and exploited as the nutrients’ source. Extracellular signalling, metabolic heterogeneity, metal immobilization, complex reactivity with siderophores, phenotypic variations, and genetic alterations are used by biofilms to detoxify metals.197 The entire EPS has a net positive charge due to the net negative charge of the sludge, which may produce flocculation.
Because of their aromatic ring structures that contain carbon, one of the most dangerous pollutants in the environment is PAHs having mutagenic and carcinogenic consequences in humans.198 For the bioremediation of PAHs, many bacterial species were used such as pollutant trapping inside EPS through biosorption, usage as a nutrition source for their development, and stimulating biofilm growth to guard against PAH toxicity. Atrazine herbicide may be digested and used as a nitrogen source by Pseudomonas sp. strain ADP bacterium.199 In one investigation, the selective concentration of PAH was discovered to help P. aeruginosa N6P6 marine bacteria produce biofilms.200 A range of insecticides and herbicides have been successfully bioremediated using biofilm-mediated reactors, including 2-propionic acid, diclofop, 2,4-dichlorophenoxyacetic acid, diclofop, 2-propionic acid, carben-dazim, choropropham, methomyl, diazinon, carbamate compounds, carbaryl, and carbofuran.201–203
5.2 Pollutants of inorganic origin
Inorganic contaminants, similar to organic contaminants, are a source of pollution in wastewater. In general, wastewater includes inorganic pollutants such as synthetic colors and toxic heavy metals, which pollute aquatic bodies and endanger human health. Furthermore, bacterial biofilms play a key role in the elimination or bioremediation of inorganic pollutants from wastewater (Table 3).
The polysaccharides in the EPS matrix, in particular, have the ability to chelate heavy metals from contaminated locations.180,198 Many bacterial biofilms, such as Pseudochrobactrummendocina NR802, Pseudochrobactrumsaccharolyticum LY10 sp., and Arthrobacter sp., have been used to eliminate harmful heavy metal ions and xenobiotic chemicals.181,204 Furthermore, certain isolates were able to tolerate larger concentrations of manganese and zinc heavy metal ions (2000 mg L−1); thus, the advantage of their effectiveness allows for the establishment of a biofilm capable of removing the greatest quantity of heavy metals.205
To counteract the toxic effects of heavy metals, indigenous microorganisms from heavy metal-contaminated locations evolve a variety of adaptive mechanisms such as heavy metal chelation, biotransformation, and metal efflux from cells. Despite these advantages, biofilms acquire resistance against heavy metal stress by forming an EPS barrier that may absorb, entrap, or immobilize metals surrounding their cell population. When it comes to some metals, EPS may act as a diffusional barrier that stops them from entering the cell.206
In all cases, a biofilm of pure bacterial species or a consortium of Cellulosimicrobium sp.207 and Halomonasaquamarina TA-04208 grown on diverse matrices (sand, stone, polyvinyl chloride, and rubber) may reduce 500 g mL−1 of chromate by more than 90%. It was discovered that the B. subtilis biofilm may accumulate many heavy metal ions, including Cu(II), Zn(II), Fe(II), Fe(III), and Al(III).209 The metal adsorption characteristics of biofilm have been employed in biotechnology to remove uranium from the groundwater.210
Periphyton-based biochar biofilm systems have also been found to remove 95.4% of 2 mg L−1 of As(III). The functional groups (aCQO and aOH) on the surface of the calcite, as well as the periphyton biofilm, were both responsible for As(III) contamination removal and binding.211 The EPS produced by the periphytic biofilm's phototrophic and heterotrophic community of microbes has the greatest potential for heavy metal sorption, which also includes arsenic.212 Metal fixation is enabled by the ionizable functional groups of EPS, while the microbial surface's anionic charge provides binding sites for several metals.
5.3 Micropollutants removal
Electronics, pharmaceuticals, and cosmetics industries, in addition to inorganic and organic pollutants, discharge dangerous micropollutants in the environment, creating water pollution. Bacteria and biofilms are two of the most promising solutions for this issue. Microbes in biofilms are beneficial for micropollutant bioremediation because they may absorb or degrade a wide range of severe contaminants via a variety of catabolic routes.198 According to Polesel et al., when predenitrifying MBBR was exposed to different concentrations of organic contaminants, it eliminated severalmantibiotic micro-contaminants including erythromycin, trimethoprim, acetyl-sulfadiazine, sulfadiazine, sulfamethizole, sulfamethoxazole, metoprolol, and atendol.177 Torresi et al. investigated the sorption of several micropollutants in MBBR carriers with varying biofilm thicknesses, and only eight micropollutants such as roxithromycin, clarithromycin, erythromycin, venlafaxine, citalopram, propranolol, metoprolol, and atenolol showed significant binding and sorption via interactions between carriers and electrostatics.213 Biofilm microbial cells have proven to be much more adaptive and resilient to stress than planktonic cells in numerous bioprocesses.
Titanium dioxide nanoparticles can be found practically in all cosmetics, paints, and a variety of other household items. As a result, a substantial number of these nanoparticles end up in wastewater discharged from residential areas all over the world. Titanium dioxide nanoparticles may represent a major hazard to the microenvironment because titania element is known to be photocatalytically activated. To minimize the toxicity of nanoparticles, biofilm-mediated absorption and removal of these particles were studied. The remediation of nanoparticles was simple due to the presence of humic and surfactants compounds in the wastewater.214 By modulating the physical trapping and diffusion transport of the substances, contaminant adhesion and absorption are regulated by the EPS matrix. The uptake of contaminants by biofilm microorganisms is influenced by nutritional, temperature, and osmotic pressure conditions, in addition to diffusion transport. The dissolved and organic matter components in wastewater act as a support for micropollutant absorption.
6 Factors affecting biofilm formation
Changes in environmental factors are known to cause a shift from planktonic toward sessile phenotype.227 The variables that aid production of biofilms are discussed below.
6.1 Environmental factors affecting biofilm formation
Biofilm production can be influenced by environmental parameters such as nutrition levels, temperature, ionic strength, and pH.228
6.1.1 Effects of nutrients.
Biofilm formation is affected by nutrient concentration, which may vary from very excessive to just about unnoticeable. They are more common and denser in a nutrient-rich habitat since it stimulates bacterial cells to transition from planktonic toward biofilm states, while a lack of nutrients leads biofilm cells to disengage from surfaces.229 Bacterial biofilms are nourished in a number of ways: (i) using extracellular polymer to concentrate minor organics onto surfaces, (ii) repurposing waste from secondary colonialists, and (iii) combining biochemical resources using variety of enzymes to decompose the food supply.230
Several studies have found that living in a nutritionally deficient environment speeds up the transition from planktonic toward sessile lifestyle.231 This finding revealed that biofilm development could be a survival strategy in a nutrient-limited environment, as surface colonization would give a number of benefits, including greater uptake of nutrients that could otherwise be absorbed to surfaces. Because the growing medium's nutritional content has been discovered to influence the establishment of biofilms by other organisms.232,233
The impact of nutrient levels on the production of bacterial biofilm has been studied in a number of ways. High nutrient concentrations, for example, increased cell counts inside the biofilms in drinking water distribution systems.234,235 The pace and quantity of P. putida biofilm in a paper mill was increased as nutrient levels increased.229 The addition of glucose as a carbon source to the medium has been observed to improve biofilm formation in several bacteria, such as E. coli236 and P. putida.229 Adding glucose to various medium, on the other hand, was reported to inhibit biofilm formation in numerous Enterobacteriaceae species, including K. pneumoniae, Citrobacter freundii, and Salmonella enterica.237
6.1.2 Effects of pH.
The pH of the surrounding environment can have a significant impact on the production of biofilms.228 Since changes in pH have the potential to overwhelm numerous processes and have fatal or negative effects on microorganisms, they have a substantial influence on bacterial growth and development as well as biofilm formation. Bacteria swiftly adjust the synthesis and activity of proteins linked with many cellular functions in response to external or internal pH variations. Some cellular functions, such as the excretion of exopolymeric compounds or polysaccharides, are less adaptive to pH changes.
This factor has been demonstrated to influence microbial adherence to surfaces, which is the first stage in biofilm development.238,239 The pH is considered a key variable in the first attachment to surfaces of bacteria such as Staphylococcus epidermidis.240 Furthermore, the pH of the medium has been demonstrated to alter the development of bacterial biofilm slime, which can affect enzyme performance because each enzyme has an ideal pH.241S. aureus biofilm development was found to be reduced at highly alkaline (pH 12) pH and acidic (pH 3) levels than at pH 7.242
Each species has a different preferred pH for polysaccharide synthesis; however, it is about 7 for most bacteria.243 Exopolysaccharide synthesis helps biofilms protect themselves from environmental stressors such as pH.244 As a result, bacterial cells within the biofilm are more resistant to pH fluctuations than free-floating cells.245 Under severely acidic conditions, for example, the gel-like structure of the bacterial biofilm can aid in reducing fast ion diffusion and allowing the establishment of a pH gradient within the extracellular matrix. Under alkaline circumstances, however, poorly organized and very thin biofilms have been seen as a result of biofilm maturation impairment. Some bacteria, such as S. epidermidis and S. aureus, are resistant to adhesion.241
6.1.3 Effect of temperature.
Microbial activity is strongly influenced by temperature. The ideal temperature for bacterial growth is correlated with an increase in food consumption.246 The presence and response rates of enzymes that control the development of several biochemical and physiological systems in bacteria are known to have an impact on food metabolism, and as a consequence, the appropriate temperature favors bacterial growth, resulting in fast biofilm creation.246 When the temperature is raised above the optimum, bacterial growth is hampered due to a decrease in response rates, and biofilm production may be hampered as a result. The physical features of substances inside and surrounding cells may be influenced by environmental temperature in addition to enzymes.247 This is related to a reduction in the rate of bacterial enzyme reaction. The ideal temperature for many bacteria found in cold water systems is about 40 °C.248
6.1.4 Effect of oxygen availability.
The ability of bacteria to cling to submerged surfaces and form biofilms is influenced by oxygen availability.249 Oxygen availability can also influence bacterial energy production, which can have an impact on biofilm development; for example, inadequate oxygen supply can reduce bacterial biofilm metabolic activity.250 As a result, oxygen supply is regarded as a critical environmental component that might influence biofilm composition and development.251,252 Active dispersal, which is crucial for the biofilm's life cycle, is mainly triggered by lower oxygen availability.253 Bacterial cells in the biofilm's base were discovered to get less oxygen than those at the top, allowing separation from the biofilm's greater depths.254 Sloughing has also been reported in biofilms growing in low-oxygen environments.255 The presence of oxygen is necessary for the formation of biofilm in some bacteria, such as E. coli, because a lack of oxygen can signal separation.256 Biofilm has been observed in other bacteria, such as P. aeruginosa, when grown anaerobically.257
6.1.5 Divalent cations.
One of the influences that bacteria may experience during biofilm-related development is calcium because divalent cations such as Ca2+ are common in aquatic and terrestrial habitats. According to recent research, eDNA chelates divalent cations, which aids in the alteration of bacterial cell surface characteristics and hence enhances biofilm resistance to antimicrobial agents and detergents.258 By bridging negatively charged sites on extracellular polymers, divalent cations, such as calcium, play a vital role in the early attachment of microbial aggregates of activated anaerobic sludge granules, sludge flocs, and biofilms.259 Recent research has found that adding more divalent cations to a biofilm increases its thickness, making it more mechanically stable and denser.260 Calcium has been discovered to play a role in alginate control, cellular extracellular product production, biofilm virulence, and cell signalling in addition to acting as a cofactor for specific proteins.261
6.2 Biological factors affecting biofilm formation
6.2.1 Quorum sensing (QS) and gene regulation.
According to a study, the downregulation and upregulation of numerous genes are involved in the earliest attachment of cells to the substratum. During the development of P. aeruginosa biofilms, about 22% of genes were upregulated and 16% were downregulated.262 Furthermore, in P. aeruginosa biofilm development, rpoS, algU, algD, and genes driving polyphosphokinase synthesis were upregulated.263 In S. aureus biofilms, genes encoding enzymes involved in the fermentation or glycolysis, such as alcohol dehydrogenase, triphosphate, and phosphoglycerate mutase, were upregulated.264 QS, or cell-to-cell signalling, has recently been shown to play an important role in biofilm cell attachment and detachment. The creation and proliferation of biofilms on diverse surfaces is triggered by a density dependent chemical signal released by bacterial cells firmly packed with an EPS matrix. QS activates target gene expression by combining a transcriptional activator protein with small autoinducers (AIs) signalling molecules, resulting in chemical behavior changes. This sort of intercellular communication is utilized to coordinate bacterial cell growth, morphological differentiation, and gene expression responses when a large number of AIs have been gathered.265
6.2.2 Extracellular polymeric substance production (EPSs).
Extracellular polymeric substances (EPSs) are a complex combination of microorganism excreted adsorbed organic compounds from wastewater, hydrolysis products, lysis, and high molecular weight polymers (Mw 10
000). Humic acids, phospholipids, DNA oligomers, glycoproteins, proteins, and polysaccharides in general have been discovered in EPSs.20 Because of their capacity to hydrogen link large quantities of water into their structure, EPSs are also very hydrated. EPSs are thought to contribute to the formation of a gel-like network that holds bacteria together in biofilms through bridging with hydrophobic contacts and multivalent cations. In addition to granulation, flocculation, and biofilm adherence to surfaces, EPSs help bacteria to acquire nutrients from the environment and protect bacteria from harmful environmental conditions. Various biofilms create different amounts of EPSs, and the quantity of EPSs produced rises as the biofilms mature.266
6.2.3 Extracellular DNA (eDNA).
Extracellular DNA (eDNA) has been discovered to be a substantial component of biofilms from both multispecies and single species. eDNA, often known as naked DNA, is a component of bacterial self-produced extracellular polymeric substances (EPSs) that shares chromosomal DNA's fundamental sequence.267 It is important for biofilm development at all phases, including early bacterial adhesion, aggregation, and the production of microcolonies that help with wastewater treatment. Biofilms benefit from eDNA because it strengthens them, protects them against detergents, antibiotics, and physical stress, and offers a plentiful source of nutrients for biofilm development.268 eDNA may also be employed in biofilm engineering for beneficial purposes such as pollution remediation and power or fuel generation in bioreactors or bio-electrochemical systems.230
6.3 Physical factors affecting biofilm formation
6.3.1 Surface topology.
Surface topography has a large impact on microbes’ capacity to cling to a surface. Surface roughness at the nanoscale and microscale levels improves bacterial adhesion to substrates during the early stages of colonization by increasing the surface area available for cell adhesion. Surface roughness reduces the shear stress on bacterial cells and communities found in moving liquids at high flow rates, such as water pipes in industrial facilities. Polymers from the medium will unavoidably condition or coat a material surface exposed in an aqueous medium, and the consequent chemical alteration will affect the rate and degree of microbial adhesion. Microbial adhesion is also influenced by other parameters such as elasticity, hydrophobicity, and charge.263
6.3.2 Turbulence, hydrodynamics, and velocity.
The boundary layer is the region where there is zero turbulent flow away from the surface. This location flow velocity has been shown to be inadequate for eliminating biofilms. Outside of this layer, there is a lot of turbulent flow, which has an impact on cell adherence to the surface. The water flow velocity determines the size of the boundary layer. At high speed, the boundary layer decreases, exposing the cells to a higher amount of turbulence. Hydrodynamic conditions may affect metabolic activities, mass, thickness, EPS production, structure, and biofilm growth.269
7 Advantages of biofilm-based wastewater treatment
Low space requirements, operational flexibility, resilience to changes in the environment, reduced hydraulic retention time, high active biomass concentration, enhanced ability to degrade recalcitrant compounds, and a slower microbial growth rate, which result in lower sludge production, are all advantages of wastewater treatment with biofilm systems.230
7.1 Operational flexibility
Wastewater treatment systems based on biofilms are quite adaptable in terms of operation. When it comes to reactors such as MBBR, they are relatively simple to run and have a straightforward design. Other treatment technologies have been utilized in conjunction with the MBBR method.270 Schneider et al. investigated how oil refinery effluent may be treated in a hybrid system that included a biologically-activated carbon column, an ozone reactor, and an MBBR.271 This demonstrates that biofilm-based reactors can operate in a hybrid environment.
7.2 Low space requirements
Varied biofilm-based water treatment reactors require different amounts of area to function. MBBR utilizes the entire tank volume for biomass growth, unlike other biofilm-based treatment technologies. The biofilm that grows on carriers has aerobic outer layers and anaerobic/anoxic interior layers. Consequently, nutrient removal may be accomplished in a single reactor, minimizing the size of the wastewater treatment facility.272
7.3 Reduced hydraulic retention time
At an organic loading rate (OLR) of 4.9 kg COD m−3 sponge per day and a hydraulic retention time (HRT) for 15.3 h, the experiment demonstrated a COD removal efficiency of over 98% on a trickling filter reactor.273 For the simultaneous removal of bromate and nitrate, the CH4-MBR employed by Lai et al. has a working capacity of 65 mL. For an influent bromate concentration of 800 g L−1 and 40 h hydraulic retention period, the biofilm process removed bromate (driven by denitrifiers) at a maximum rate of 40.7 g L−1 min−1.274
7.4 Resilience to changes in the environment
Bacteria have complex mechanisms that enable them to adjust to small pH changes in their surroundings. EPS outflow may be an adaptive response to overcome pH oscillations since cellular activities do not respond as quickly to pH variations.247 Heavy metal chelation, biotransformation, and metal efflux from cells are among the adaptation processes found by Teitzel and Parsek in indigenous bacteria from heavy metal-contaminated environments.206
In bioremediation, biofilm-forming bacteria that have been found in nutrition and oxygen competition as well as resistance to contaminants have been examined. Biofilm-forming bacteria found in nutrition and oxygen competition as well as contaminant resistance have all been studied in bioremediation. In biofilms found in profoundly polluted areas, indigenous bacteria can live, endure, and handle tough environmental conditions such as predation, nutrition, salinity, pH, changeable and high temperature, UV exposure, and pollutant concentrations.
7.5 High active biomass concentration
Various studies have revealed that the usual biomass content, when estimated on reactor volume, is in the range of 2–5 kg SSm−3, which is high when compared to other biological water treatment procedures.275–277
7.6 Low sludge production
Two pilot experiments of an immersed membrane activated sludge process were given by Cote et al. and it was discovered that the sludge production rate from a submerged MBR (0.25 kgSS kg−1 COD removed) was nearly half that of an extended aeration activated sludge process.278 Another French pilot investigation of series MBR indicated a sludge production rate of 0.23 kgSS kg−1 COD removed, backed the low sludge production.279
8 Challenges in biofilm-mediated removal of contaminants
8.1 Biofilm thickness/architecture
The thickness and cell density of a biofilm are significant variables to consider while assessing it, according to Mathur et al.214 Another crucial factor is the matrix porosity, which influences the biofilm's ability and structure. According to study, thin biofilms contain fewer holes and a greater cell density than thick biofilms. Biofilm may use these perforations as a solid–liquid partitioning substrate. Consequently, the biofilm's ability to break down phenol is related to its thickness; therefore, thickness is suggested for usage as an effective bioremediation strategy.280
8.2 Quality of pollutants/effluent nature
Positively-charged micro-contaminants (greater than 90%) have exhibited significant sorption potential in activated sludge as compared to neutrally or negatively charged micropollutants compounds at pH 7.5.213 The pH has been found to play a role in determining the charge and sorption potential of micropollutants. The protonation of the macrolides amino group causes a strong ionic contact between the negatively-charged cell surface and positively-charged macrolides.
9 Future perspectives
More research is required to create and execute economically efficient processes due to the significant use of energy and carbon sources. For the treatment of nitrogen-rich water that is related to concentrated waste streams produced by various industries, biofilm-based treatment solutions provide a viable substitute. Several biofilm-based wastewater treatment technologies have been developed during the last several decades.
Since biofilms are superior at protecting the environment, they are employed in many industrial applications, including the packaging of commercial items and food. Numerous research noted certain drawbacks of employing biofilms, including their poor mechanical qualities, susceptibility to bacterial and fungal infections, susceptibility to air permeability, and water sensitivity. Recent research has however shown that these problems may be avoided using plasticizers, nanomaterials, etc. In addition, the use of plasticizers and nanoparticles enhances the products’ structural, thermal, and chemical qualities. The use of preservative and antibacterial nanomaterials in food packaging biofilms carries a risk since human health may be adversely affected by nanoparticle movement, injection/inhalation, and exposure to food surfaces. Contemporary researchers are confronted with the significant challenge of creating regulatory standards and thorough toxicological analyses on nanomaterials. When compared to synthetic polymers, the cost of producing biofilms is excessively high. New kinds of bio-based films with simple processing that use affordable, renewable resources, and have high antibacterial, chemical, physical, and thermomechanical properties are needed to replace conventional materials. Currently, research is focusing on antibacterial edible packaging materials, but in the next few years, antibacterial nanoparticle-reinforced biofilms will become a key trend. Even though this field has seen a lot of study, there are still numerous obstacles to overcome, including gas permeability, control over drug release, adverse effects on humans, drug loading, and encapsulation ability. Therefore, in the near future, it is important to produce mechanically and chemically superior edible biofilms that have the potential to be employed in the packaging and biomedical sectors.
There is still a need for more research: (1) recovering aged biofilm using economical and ecofriendly regulatory techniques. In addition, novel biofilm reaction technologies, and principles continue to pique people's interest to meet the rising demands for lower energy consumption and pollution removal; (2) uncovering the attributes of biofilm from a more microscopic viewpoint through in situ monitoring methods and molecular biology technologies (i.e., metagenomic and macro-proteomics approaches); and (3) specific and practical methods for shortening biofilm formation in refractive processes.
The use of this technique for the treatment of industrial wastewater is constrained by the lack of techno-economic information on biofilm dependability, sustainability, and system performance. In addition, certain components of effluent wastewater, including suspended particles, have a detrimental impact on the functioning of the biofilm by obstructing the diffusion of oxygen and substrate into the biofilm. In addition, the high cost and biofouling of membranes are the main obstacles to the development of the membrane biofilm process.
10 Conclusion
With increase in number of toxic xenobiotics in the wastewater, different bioremediation approaches have been introduced to eliminate them. Biofilm-mediated reduction of pollutants from wastewater is one of the potential approaches that have been used widely since the past few years. In this approach, bacterial cells exchange signalling molecules, genetic materials, and metabolites, and this interaction between bacterial cells of various strains depends on quorum sensing. The understanding of bacterial diversity in biofilm by utilizing metagenomic approach, i.e., 16s rRNA is much essential to understand the bioremediation of contaminated water. Globally, the usage of microbiome-based bioremediation approach has tremendously increased, and the fabrication of genetically-modified biofilm thus increases the elimination of pollutants. In industries, quorum sensing technology is applied to remove contaminates from industrial wastewater, it regulates the synthesis of EPS, fabrication of biofilm, and synthesis of biosurfactants, which could significantly remove organic substances and heavy metals from wastewater. In wastewater treatment plants, quorum sensing and quorum quenching are responsible for biofouling, granulation, aggregation, colonization, metabolic of organic substances, and removal of nutrients. In a nutshell, the omics approach would provide a much-needed understanding of how the potential to form biofilms is influenced by a variety of physiological, environmental, and mutational variables, as well as how these factors interact with one another in a predictable way. The development of a database where biofilm signatures are analyzed and recognized may follow from this. However, before starting such a venture, it will be necessary to choose a high-throughput, reliable, and adaptable biofilm growth apparatus as well as the proper techniques for biofilm analysis. Although strategies similar to metabolic engineering are very necessary for controlling metabolic flux, the underlying biofilm architecture should not be disregarded. The effectiveness of any system is substantially impacted by each of the biofilm's constituent parts as well as the biofilm's overall form. Currently, the improvement of bioremediation involves the use of genome editing tools such as clustered regularly interspaced short palindromic repeats (CRISPR-Cas), transcription activators such as effector nucleases, and zinc finger nucleases. Escherichia coli and Pseudomonas are two bacteria that often employ the CRISPR-Cas system, which consists of guide RNA connected crisper generated RNA and transacting antisense RNA. In addition, the Achromobacter sp. HZ01, Comamonas testosterone, and Rhodococcusruber TH utilize the CRISPR system to express genes necessary for bioremediation. A new direction for the study of biofilm-mediated bioremediation may be opened up by genome editing, which improves the ability of bacteria to survive in hazardous environments when used with bioinformatics techniques and in silico databases.
Conflicts of interest
There are no conflicts to declare.
References
- M. Ahmad, M. Yousaf, A. Nasir, I. A. Bhatti, A. Mahmood, X. Fang, X. Jian, K. Kalantar-Zadeh and N. Mahmood, Porous eleocharis @ MnPE layered hybrid for synergistic adsorption and catalytic biodegradation of toxic Azo dyes from industrial wastewater, Environ. Sci. Technol., 2019, 53, 2161–2170 CrossRef CAS PubMed.
- P. D. Dongare, A. Alabastri, S. Pedersen, K. R. Zodrow, N. J. Hogan, O. Neumann, J. Wu, T. Wang, A. Deshmukh and M. Elimelech, Nanophotonics-enabled solar membrane distillation for off-grid water purification, Proc. Natl. Acad. Sci. U. S. A., 2017, 114, 6936–6941 CrossRef CAS PubMed.
- D. D. Trapani, G. Mannina, M. Torregrossa and G. Viviani, Quantification of kinetic parameters for heterotrophic bacteria via respirometry in a hybrid reactor, Water Sci. Technol., 2010, 61, 1757–1766 CrossRef PubMed.
- Z. Xu, C. Wei, J. Jin, W. Xu, Q. Wu, J. Gu, M. Ou and X. Xu, Development of a novel mixed titanium, silver oxide polyacrylonitrile nanofiber as a superior adsorbent and its application for MB removal in wastewater treatment, J. Braz. Chem. Soc., 2018, 29, 560–571 CAS.
- V. Sharma, P. Rekha and P. Mohanty, Nanoporous hypercrosslinked polyaniline: an efficient adsorbent for the adsorptive removal of cationic and anionic dye, J. Mol. Liq., 2016, 222, 1091–1100 CrossRef CAS.
- W. Zhang, Y. Wu, J. Wu, X. Zheng and Y. Chen, Enhanced removal of sulfur-containing organic pollutants from actual wastewater by biofilm reactor: Insights of sulfur transformation and bacterial metabolic traits, Environ. Pollut., 2022, 313, 120187 CrossRef CAS PubMed.
- A. Y. Ugya, F. O. Ajibade and X. Hua, The efficiency of microalgae biofilm in the phycoremediation of water from River Kaduna, J. Environ. Manage., 2021, 295, 113109 CrossRef CAS PubMed.
- M. N. V. Prasad and R. Prasad, Nature's cure for cleanup of contaminated environment: a review of bioremediation strategies, Rev. Environ. Health, 2012, 27, 181–189 CAS.
- A. F. Miranda, N. Ramkumar, C. Andriotis, T. Holtkemeier, A. Yasmin, S. Rochfort, D. Wlodkowic, P. Morrison, F. Roddick, G. Spangenberg and B. Lal, Applications of microalgal biofilms for wastewater treatment and bioenergy production, Biotechnol. Biofuels, 2017, 10, 1–23 CrossRef PubMed.
- S. Mishra, S. Pang, W. Zhang, Z. Lin, P. Bhatt and S. Chen, Insights into the microbial degradation and biochemical mechanisms of carbamates, Chemosphere, 2021, 279, 130500 CrossRef CAS PubMed.
-
A. Maurya and A. Raj, Recent advances in the application of biofilm in bioremediation of industrial wastewater and organic pollutants, Microorganisms for Sustainable Environment and Health, Elsevier, 2020, pp. 81–118 Search PubMed.
- H. C. Flemming, J. Wingender, U. Szewzyk, P. Steinberg, S. A. Rice and S. Kjelleberg, Biofilms: an emergent form of bacterial life, Nat. Rev. Microbiol., 2016, 14, 563 CrossRef CAS PubMed.
- M. Mosharaf, M. Tanvir, M. Haque, M. Haque, M. Khan, A. H. Molla, M. Z. Alam, M. S. Islam and M. R. Talukder, Metal-adapted bacteria isolated from wastewaters produce biofilms by expressing proteinaceous Curli Fimbriae and cellulose nanofibers, Front. Microbiol., 2018, 9, 1334 CrossRef CAS PubMed.
- M. Sandhya, Y. Huang, J. Li, X. Wu, Z. Zhou, Q. Lei and S. Chen, Biofilm-mediated bioremediation is a powerful tool for the removal of environmental pollutants, Chemosphere, 2022, 133609 Search PubMed.
-
A. Maurya and A. Raj, Recent advances in the application of biofilm in bioremediation of industrial wastewater and organic pollutants, Microorganisms for Sustainable Environment and Health, 2020, pp. 81–118 Search PubMed.
- A. Vinod, M. R. Sanjay, S. Suchart and P. Jyotishkumar, Renewable and sustainable biobased materials: An assessment on biofibers, biofilms, biopolymers and biocomposites, J. Cleaner Prod., 2020, 258, 120978 CrossRef CAS.
-
C. S. Butler and J. P. Boltz, Biofilm processes and control in water and wastewater treatment, Remediation of Polluted Waters, Elsevier Inc., 2014, pp. 90–107 Search PubMed.
-
S. L. Percival, S. Malic, H. Cruz and D. W. Williams, Introduction to biofilms, Biofilms and Veterinary Medicine, Springer, 2011, pp. 41–68 Search PubMed.
- M. Jamal, W. Ahmad, S. Andleeb, F. Jalil, M. Imran, M. A. Nawaz, T. Hussain, M. Ali, M. Rafiq and M. A. Kamil, Bacterial biofilm and associated infections, J. Chin. Med., 2018, 81, 7–11 Search PubMed.
- A. Ghanbari, J. Dehghany, T. Schwebs, M. Musken, S. Haussler and M. Meyer-Hermann, Inoculation density and nutrient level determine the formation of mushroom-shaped structures in Pseudomonas aeruginosa biofilms, Sci. Rep., 2016, 6, 32097 CrossRef CAS PubMed.
- L. Qi and G. F. Christopher, Role of flagella, Type IV Pili, biosurfactants, and extracellular polymeric substance polysaccharides on the formation of pellicles by Pseudomonas aeruginosa, Langmuir, 2019, 35, 5294–5304 CrossRef CAS PubMed.
- K. U. Mahto and S. Das, Bacterial biofilm and extracellular polymeric substances in the moving bed biofilm reactor for wastewater treatment: A review, Bioresour. Technol., 2022, 345, 126476 CrossRef CAS PubMed.
- Y. Zhou, P. D. Kiely, R. Kibbee and B. Ormeci, Effect of polymeric support material on biofilm development, bacterial population, and wastewater treatment performance in anaerobic fixed-film systems, Chemosphere, 2021, 264, 128477 CrossRef CAS PubMed.
- N. Boelee, H. Temmink, M. Janssen, C. Buisman and R. J. Wijffels, Nitrogen and phosphorus removal from municipal wastewater effluent using microalgal biofilms, Water Res., 2011, 45, 5925–5933 CrossRef CAS PubMed.
- I. Randrianjatovo-Gbalou, P. Rouquette, D. Lefebvre, E. Girbal-Neuhauser and C. E. Marcato-Romain,
In situ analysis of Bacillus licheniformis biofilms: amyloid-like polymers and eDNA are involved in the adherence and aggregation of the extracellular matrix, J. Appl. Microbiol., 2017, 122, 1262–1274 CrossRef CAS PubMed.
- R. Vasudevan, Biofilms: microbial cities of scientific significance, J. Microbiol. Exp., 2014, 1, 00014 Search PubMed.
- E. Karatan and P. Watnick, Signals, regulatory networks, and materials that build and break bacterial biofilms, Microbiol. Mol. Biol. Rev., 2009, 73, 310–347 CrossRef CAS PubMed.
- H. C. Flemming and J. Wingender, The biofilm matrix, Nat. Rev. Microbiol., 2010, 8, 623–633 CrossRef CAS PubMed.
- Y. Wang, S. Ho, C. Cheng, W. Guo, D. Nagarajan, N. Ren, D. Lee and J. Chang, Perspectives on the feasibility of using microalgae for industrial wastewater treatment, Bioresour. Technol., 2016, 222, 485–497 CrossRef CAS PubMed.
- L. R. Johnson, Micro colony and Biofilm formation as survival strategy of bacteria, J. Theor. Biol., 2008, 251, 24–34 CrossRef CAS PubMed.
- S. K. Hood and E. A. Zottola, Biofilms in food processing, Food Control, 1995, 6, 9–18 CrossRef.
- A. Raza, G. Muhammad, S. Sharif and A. Atta, Biofilm Producing Staphylococcusaureus and Bovine Mastitis, Mol. Microbiol., 2013, 3, 1–8 Search PubMed.
- A. Chowdhury, M. Nagaraja and A. G. Kumar, Potential of biofilm formation by staphylococci on polymer surface and its correlation with methicillin susceptibility, Indian J. Med. Microbiol., 2009, 27, 377–378 CrossRef PubMed.
- E. D. Pulcini, Bacterial Biofilms: a review of current research, Nephrologi, 2001, 22, 439–441 Search PubMed.
- S. Andersson, G. Dalhammar, C. J. Land and G. KuttuvaRajarao, Characterization of extracellular polymeric substances from denitrifying organism Comamonasdenitrificans, Appl. Microbiol. Biotechnol., 2009, 82, 535–543 CrossRef CAS PubMed.
- Y. Turki, I. Mehri, R. Lajnef, A. B. Rejab, A. Khessairi, H. Cherif, H. Ouzari and A. Hassen, Biofilms in bioremediation and wastewater treatment: characterization of bacterial community structure and diversity during seasons in municipal wastewater treatment process, Environ. Sci. Pollut. Res., 2017, 24, 3519–3530 CrossRef PubMed.
-
B. S. C. Leadbeater and M. E. Callow, Formation, composition and physiology of algal biofilms, in Biofilms - Science and Technology, ed. L. F. Melo, T. R. Bott, M. Fletcher and B. Capdeville, Springer, Dordrecht, 1992, pp. 149–162 Search PubMed.
- H. P. Jarvie, C. Neal, A. Warwick, J. White, M. Neal, H. D. Wickham, L. K. Hill and M. C. Andrews, Phosphorus uptake into algal biofilms in a lowland chalk river, Sci. Total Environ., 2002, 282, 353–373 CrossRef PubMed.
- N. Haubner, R. Schumann and U. Karsten, Aeroterrestrial microalgae growing in biofilms on facades - response to temperature and water stress, Microb. Ecol., 2006, 51, 285–293 CrossRef CAS PubMed.
- J. P. Hoffmann, Mini review: wastewater treatment with suspended and non-suspended algae, J. Phycol., 1998, 34, 757–763 CrossRef CAS.
- G. Roeselers, M. C. M. van Loosdrecht and G. Muyzer, Phototrophic biofilms and their potential applications, J. Appl. Phycol., 2008, 20, 227–235 CrossRef CAS PubMed.
- E. Posadas, P. Garcia-Encina, A. Soltau, A. Dominguez, I. Diaz and R. Munoz, Carbon and nutrient removal from centrates and domestic wastewater using algal-bacterial biofilm bioreactors, Bioresour. Technol., 2013, 139, 50–58 CrossRef CAS PubMed.
- L. B. Christenson and R. C. Sims, Production and harvesting of microalgae for wastewater treatment, biofuels and by-products, Biotechnol. Adv., 2011, 29, 686–702 CrossRef CAS PubMed.
- N. C. Boelee, H. Temmink, M. Janssen, C. J. N. Buisman and R. H. Wijffels, Scenario analysis of nutrient removal from municipal wastewater by microalgal biofilms, Water, 2012, 4, 460–473 CrossRef CAS.
- T. Li, G. Lin, B. Podola and M. Melkonian, Continuous removal of zinc from wastewater and mine dump leachate by a microalgal biofilm PSBR, J. Hazard. Mater., 2015, 297, 112–118 CrossRef CAS PubMed.
- T. Skowronski, Adsorption of cadmium on green microalga Stichococcusbacillaris, Chemosphere, 1986, 15, 69–76 CrossRef CAS.
- T. Skowroński and M. Przytocka-Jusiak, Cadmium removal by green alga Stichococcusbacillaris, Chemosphere, 1986, 15, 77–79 CrossRef.
- J. Shi, B. Podola and M. Melkonian, Removal of nitrogen and phosphorus from wastewater using microalgae immobilized on twin layers: an experimental study, J. Appl. Phycol., 2007, 19, 417–423 CrossRef CAS.
- J. Shi, B. Podola and M. Melkonian, Application of a prototype-scale Twin-Layer photobioreactor for effective N and P removal from different process stages of municipal wastewater by immobilized microalgae, Bioresour. Technol., 2014, 154, 260–266 CrossRef CAS PubMed.
- N. Mirghaffari, E. Moeini and O. Farhadian, Biosorption of Cd and Pb ions from aqueous solutions by biomass of the green microalga, Scenedesmus quadricauda, J. Appl. Phycol., 2015, 27, 311–320 CrossRef CAS.
- J. P. Wong, Y. Wong and N. F. Tam, Nickel biosorption by two chlorella species, C. Vulgaris (a commercial species) and C. Miniata (a local isolate), Bioresour. Technol., 2000, 73, 133–137 CrossRef CAS.
- H. Doshi, C. Seth, A. Ray and I. L. Kothari, Bioaccumulation of heavy metals by green algae, Curr. Microbiol., 2008, 56, 246–255 CrossRef CAS PubMed.
- D. Perez-Mendoza, S. J. Coulthurst, J. Sanjuan and G. P. Salmond, N-Acetylglucosamine-dependent biofilm formation in Pectobacteriumatrosepticum is cryptic and activated by elevated c-di-GMP levels, Microbiology, 2011, 157, 3340–3348 CrossRef CAS PubMed.
- V. Dupres, D. Alsteens, G. Andre and Y. F. J. Dufrene, Microbial nanoscopy: a closer look at microbial cell surfaces, Trends Microbiol., 2010, 18, 397–405 CrossRef CAS PubMed.
- I. Douterelo, K. Fish and J. J. Boxall, Succession of bacterial and fungal communities within biofilms of a chlorinated drinking water distribution system, Water Res., 2018, 141, 74–85 CrossRef CAS PubMed.
- D. C. Sheppard and P. L. Howell, Biofilm exopolysaccharides of pathogenic fungi: lessons frombacteria, J. Biol. Chem., 2016, 291, 12529–12537 CrossRef CAS PubMed.
- P. Stoodley, K. Sauer, D. G. Davies and J. W. Costerton, Biofilms as complex differentiated communities, Annu. Rev. Microbiol., 2002, 56, 187–209 CrossRef CAS PubMed.
- M. Gutierrez-Correa and R. P. Tengerdy, Xylanase production by fungal mixed culture solid substrate fermentation on sugar cane bagasse, Biotechnol. Lett., 1998, 20, 45–47 CrossRef CAS.
- R. Rajendra, L. Sherry, A. Deshpande, E. M. Johnson, M. F. Hanson, C. Williams, C. A. Munro, B. L. Jones and G. Ramage, A prospective surveillance study of candidaemia: epidemiology, risk factors, antifungal treatment and outcome in hospitalized patients, Front. Microbiol., 2016, 7, 915 Search PubMed.
- Z. W. Wang and S. Chen, Potential of biofilm-based biofuel production, Appl. Microbiol. Biotechnol., 2009, 83, 1–18 CrossRef CAS PubMed.
- R. L. Sinsabaugh and A. E. Linkins, Enzymic and chemical analysis of particulate organic matter from a boreal river, Freshwater Biol., 1990, 23, 301–309 CrossRef CAS.
- A. C. Chamier, Cell-wall-degrading enzymes of aquatic hyphomycetes: a review, Bot. J. Linn. Soc., 1985, 91, 67–81 CrossRef.
- V. Gulis and K. Suberkropp, Effect of inorganic nutrients on relative contributions of fungi and bacteria to carbon flow from submerged decomposing leaf litter, Microb. Ecol., 2003, 45, 11–19 CrossRef CAS PubMed.
- H. Greaves, The bacterial factor in wood decay, Wood Sci. Technol., 1971, 5, 6–16 CrossRef.
- M. F. Jurgensen, M. J. Larsen, M. Wolosiewicz and A. E. Harvey, A comparison of dinitrogen fixation rates in wood litter decayed by white-rot and brown-rot fungi, Plant Soil, 1989, 115, 117–122 CrossRef CAS.
- P. B. Rainey, A. L. J. Cole, T. R. Fermor and D. A. Wood, A model system for examining involvement of bacteria in basidiome initiation of Agaricusbisporus, Mycol. Res., 1990, 94, 191–195 CrossRef.
- J. R. Lawrence and R. A. Snyder, Feeding behaviour and grazing impacts of a Euplotes sp. on attached bacteria, Can. J. Microbiol., 1998, 44, 623–629 CrossRef CAS.
- J. M. Bernhard and S. S. Bowser, Bacterial biofilms as a trophic resource for certain benthic foraminifera, Mar. Ecol.: Prog. Ser., 1992, 263–272 CrossRef.
- E. Posadas, M. del Mar Morales, C. Gomez, F. G. Acien and R. Munoz, Influence of pH and CO2 source on the performance of microalgae-based secondary domestic wastewater treatment in outdoors pilot raceways, Chem. Eng. J., 2015, 265, 239–248 CrossRef CAS.
- M. Martin-Cereceda, A. Alvarez, S. Serrano and A. Guinea, Confocal and light microscope examination of protozoa and other microorganisms in the biofilms from a rotating biological contactor wastewater treatment plant, Acta Protozoa, 2001, 40, 263–272 Search PubMed.
- R. N. Glud and T. Fenchel, The importance of ciliates for interstitial solute transport in benthic communities, Mar. Ecol.: Prog. Ser., 1999, 186, 87–93 CrossRef.
- Z. She, L. Zhao, X. Zhang, C. Jin, L. Guo, S. Yang, Y. Zhao and M. Gao, Partial nitrification and denitrification in a sequencing batch reactor treating high-salinity wastewater, Chem. Eng. J., 2016, 288, 207–215 CrossRef CAS.
- J. Q. Xiong, S. Govindwar, M. B. Kurade, K. J. Paeng, H. S. Roh, M. A. Khan and B. H. Jeon, Toxicity of sulfamethazine and sulfamethoxazole and their removal by a green microalga, Scenedesmus obliquus, Chemosphere, 2019, 218, 551–558 CrossRef CAS PubMed.
-
I. Johnson, S. Girijan, B. K. Tripathy, M. A. S. Ali and M. Kumar, Algal-bacterial symbiosis and its application in wastewater treatment, Emerging Technologies in Environmental Bioremediation, 2020, pp. 341–372 Search PubMed.
- J. Yang, W. Shi, F. Fang, J. Guo, L. Lu, Y. Xiao and X. Jiang, Exploring the feasibility of sewage treatment by algal-bacterial consortia, Crit. Rev. Biotechnol., 2020, 40, 169–179 CrossRef CAS PubMed.
- P. Praveen and K. C. Loh, Photosynthetic aeration in biological wastewater treatment using immobilized microalgae-bacteria symbiosis, Appl. Microbiol. Biotechnol., 2015, 99, 10345–10354 CrossRef CAS PubMed.
- Y. Su, A. Mennerich and B. Urban, Synergistic cooperation between wastewater-born algae and activated sludge for wastewater treatment: influence of algae and sludge inoculation ratios, Bioresour. Technol., 2012, 105, 67–73 CrossRef CAS PubMed.
- J. Lee, D. H. Cho, R. Ramanan, B. H. Kim, H. M. Oh and H. S. Kim, Microalgae-associated bacteria play a key role in the flocculation of Chlorella vulgaris, Bioresour. Technol., 2013, 131, 195–201 CrossRef CAS PubMed.
- P. Foladori, S. Petrini and G. Andreottola, Evolution of real municipal wastewater treatment in photobioreactors and microalgae-bacteria consortia using real-time parameters, Chem. Eng. J., 2018, 345, 507–516 CrossRef CAS.
- Y. Gou, J. Yang, F. Fang, J. Guo and H. Ma, Feasibility of using a novel algal-bacterial biofilm reactor for efficient domestic wastewater treatment, Environ. Technol., 2020, 41, 400–410 CrossRef CAS PubMed.
- E. Mhedhbi, N. Khelifi, P. Foladori and I. Smaali, Real-time behavior of a microalgae-Bacteria consortium treating wastewater in a sequencing batch reactor in response to feeding time and agitation mode, Water, 2020, 12, 1893 CrossRef.
- L. Delgadillo-Mirquez, F. Lopes, B. Taidi and D. Pareau, Nitrogen and phosphate removal from wastewater with a mixed microalgae and bacteria culture, Biotechnol. Rep. Amst., 2016, 11, 18–26 CrossRef PubMed.
- M. Ras, J. P. Steyer and O. Bernard, Temperature effect on microalgae: a crucial factor for outdoor production, Rev. Environ. Sci. Biotechnol., 2013, 12, 153–164 CrossRef CAS.
- A. Sanchez Zurano, C. Gomez Serrano, F. G. Acien-Fernandez, J. M. Fernández-Sevilla and E. Molina-Grima, Modeling of photosynthesis and respiration rate for microalgae-bacteria consortia, Biotechnol. Bioeng., 2021, 118, 952–962 CrossRef CAS PubMed.
- S. Zimba, T. S. Kumar, N. Mohan and P. H. Rao, Evaluation of various waste substrates for biofilm formation and subsequent use in aerobic packed-bed reactor for secondary treatment of domestic wastewater, World J. Microbiol. Biotechnol., 2021, 37, 1–8 CrossRef PubMed.
- J. Yang, Y. Gou, F. Fang, J. Guo, L. Lu, Y. Zhou and H. Ma, Potential of wastewater treatment using a concentrated and suspended algal-bacterial consortium in a photo membrane bioreactor, Chem. Eng. J., 2018, 335, 154–160 CrossRef CAS.
- A. Mantzorou and F. Ververidis, Microalgal biofilms: A further step over current microalgal cultivation techniques, Sci. Total Environ., 2019, 651, 3187–3201 CrossRef CAS PubMed.
- Y. Zhao, D. Liu, W. Huang, Y. Yang, M. Ji, L. D. Nghiem and N. Tran, HInsights into biofilm carriers for biological wastewater treatment processes: Current state-of-the-art, challenges, and opportunities, Bioresour. Technol., 2019, 288, 121619 CrossRef CAS PubMed.
-
M. Asri, S. Elabed, S. I. Koraichi and N. ElGhachtouli, Biofilm-based systems for industrial wastewater treatment, Handbook of Environmental Materials Management, 2018, pp. 1–21 Search PubMed.
- S. Di Fabio, S. Lampis, L. Zanetti, F. Cecchi and F. Fatone, Role and characteristics of problematic biofilms within the removal and mobility of trace metals in a pilot-scale membrane bioreactor, Process Biochem., 2013, 48, 1757–1766 CrossRef CAS.
- K. J. Martin and R. Nerenberg, The membrane biofilm reactor (MBfR) for water and wastewater treatment: Principles, applications, and recent developments, Bioresour. Technol., 2012, 122, 83–94 CrossRef CAS PubMed.
- H. Lin, W. Gao, F. Meng, B. Q. Liao, K. T. Leung, L. Zhao, J. Chen and H. Hong, Membrane bioreactors for industrial wastewater treatment: a critical review, Crit. Rev. Environ. Sci. Technol., 2012, 42, 677–740 CrossRef CAS.
- K. Yamamoto, M. Hiasa, T. Mahmood and T. Matsuo, Direct solid–liquid separation using hollow fiber membrane in an activated sludge aeration tank, Water Pollut. Res. Control Brighton, 1988, 43–54 CAS.
- P. Le-Clech, V. Chen and T. A. Fane, Fouling in membrane bioreactors used in wastewater treatment, J. Membr. Sci., 2006, 284, 17–53 CrossRef CAS.
- B. Q. Liao, J. T. Kraemer and D. M. Bagley, Anaerobic membrane bioreactors: applications and research directions, Crit. Rev. Environ. Sci. Technol., 2006, 36, 489–530 CrossRef CAS.
- S. Chen and J. Liu, Landfill leachate treatment by MBR: performance and molecular weight distribution of organic contaminant, Sci. Bull., 2006, 51, 2831–2838 CrossRef CAS.
- F. P. Shariati, M. R. Mehrnia, B. M. Salmasi, M. Heran, C. Wisniewski and M. H. Sarrafzadeh, Membrane bioreactor for treatment of pharmaceutical wastewater containing acetaminophen, Desalination, 2010, 250, 798–800 CrossRef CAS.
- H. Futselaar, H. Schonewille, D. de Vente and L. Broens, NORIT AirLift MBR: side-stream system for municipal waste water treatment, Desalination, 2007, 204, 1–7 CrossRef CAS.
- I. Ivanovic and T. Leiknes, The biofilm membrane bioreactor (BF-MBR)-a review, Desalin. Water Treat., 2012, 37, 288–295 CrossRef CAS.
- C. C. Chang, S. K. Tseng, C. C. Chang and C. M. Ho, Reductive dechlorination of 2-chlorophenol in a hydrogenotrophic, gas-permeable, silicone membrane bioreactor, Bioresour. Technol., 2003, 90, 323–328 CrossRef CAS PubMed.
- C. C. Chang, S. K. Tseng, C. C. Chang and C. M. Ho, Degradation of 2-chlorophenol via a hydrogenotrophic biofilm under different reductive conditions, Chemosphere, 2004, 56, 989–997 CrossRef CAS PubMed.
- M. J. Semmens, K. Dahm, J. Shanahan and A. Christianson, COD and nitrogen removal by biofilms growing on gas permeable membranes, Water Res., 2003, 37, 4343–4350 CrossRef CAS PubMed.
- H. H. Ngo, M. C. Nguyen, N. G. Sangvikar, T. T. L. Hoang and W. S. Guo, Simple approaches towards the design of an attached-growth sponge bioreactor (AGSB) for wastewater treatment and reuse, Water Sci. Technol., 2006, 54, 191–197 CrossRef CAS PubMed.
- L. S. Downing and R. Nerenberg, Effect of bulk liquid BOD concentration on activity and microbial community structure of a nitrifying, membrane-aerated biofilm, Appl. Microbiol. Biotechnol., 2008, 81, 153–162 CrossRef CAS PubMed.
- T. M. LaPara, A. C. Cole, J. W. Shanahan and M. J. Semmens, The effects of organic carbon, ammoniacal-nitrogen, and oxygen partial pressure on the stratification of membrane-aerated biofilms, J. Ind. Microbiol. Biotechnol., 2006, 33, 315–323 CrossRef CAS PubMed.
- S. Matsumoto, A. Terada, Y. Aoi, S. Tsuneda, E. Alpkvist, C. Picioreanu and M. C. M. Van Loosdrecht, Experimental and simulation analysis of community structure of nitrifying bacteria in a membrane-aerated biofilm, Water Sci. Technol., 2007, 55, 283–290 CrossRef CAS PubMed.
- A. Terada, K. Hibiya, J. Nagai, S. Tsuneda and A. Hirata, Nitrogen Removal Characteristics and Biofilm Analysis of a Membrane-Aerated Biofilm Reactor Applicable to High-Strength Nitrogenous Wastewater Treatment, J. Biosci. Bioeng., 2003, 95, 170–178 CrossRef CAS PubMed.
- L. S. Downing and R. Nerenberg, Effect of Oxygen Gradients on the Activity and Microbial Community Structure of a Nitrifying, Membrane-Aerated Biofilm, Biotechnol. Bioeng., 2008, 101, 1193–1204 CrossRef CAS PubMed.
- S. Matsumoto, A. Terada and S. Tsuneda, Modeling of membrane-aerated biofilm: Effects of C/N ratio, biofilm thickness and surface loading of oxygen on feasibility of simultaneous nitrification and denitrification, Biochem. Eng. J., 2007, 37, 98–107 CrossRef CAS.
- A. Terada, S. Lackner, S. Tsuneda and B. F. Smets, Redox-stratification controlled biofilm (ReSCoBi) for completely autotrophic nitrogen removal: The effect of co- versus counter-diffusion on reactor performance, Biotechnol. Bioeng., 2007, 97, 40–51 CrossRef CAS PubMed.
- Z. Gong, S. T. Liu, F. L. Yang, H. Bao and K. Furukawa, Characterization of functional microbial community in a membrane-aerated biofilm reactor operated for completely autotrophic nitrogen removal, Bioresour. Technol., 2008, 99, 2749–2756 CrossRef CAS PubMed.
- K. C. Lee and B. E. Rittmann, Applying a novel autohydrogenotrophic hollow-fiber membrane biofilm reactor for denitrification of drinking water, Water Res., 2002, 36, 2040–2052 CrossRef CAS PubMed.
- K. A. Karanasios, I. A. Vasiliadou, S. Pavlou and D. V. Vayenas, Hydrogenotrophic denitrification of potable water: A review, J. Hazard. Mater., 2010, 180, 20–37 CrossRef CAS PubMed.
- J. Chung, R. Nerenberg and B. E. Rittmann, Bio-reduction of soluble chromate using a hydrogen-based membrane biofilm reactor, Water Res., 1996, 40, 1634–1642 CrossRef PubMed.
- J. Chung, X. Li and B. E. Rittmann, Bio-reduction of arsenate using a hydrogen-based membrane biofilm reactor, Chemosphere, 2006, 65, 24–34 CrossRef CAS PubMed.
- J. Chung, R. Nerenberg and B. E. Rittmann, Bioreduction of Selenate Using a Hydrogen- Based Membrane Biofilm Reactor, Environ. Sci. Technol., 2006, 40, 1664–1671 CrossRef CAS PubMed.
- R. Nerenberg, B. E. Rittmann and I. Najm, Perchlorate reduction in a hydrogen-based membrane-biofilm reactor, J. - Am. Water Works Assoc., 2002, 94, 103–114 CrossRef CAS.
- R. Nerenberg and B. E. Rittmann, Hydrogen-based, hollow-fiber membrane biofilm reactor for reduction of perchlorate and other oxidized contaminants, Water Sci. Technol., 2004, 49, 223–230 CrossRef CAS PubMed.
- S. Xia, H. Li, Z. Zhang, Y. Zhang, X. Yang, R. Jia, K. Xie and X. Xu, Bioreduction of para-chloronitrobenzene in drinking water using a continous stirred hydrogen-based hollow fiber membrane biofilm reactor, J. Hazard. Mater., 2011, 192, 593–598 CrossRef CAS PubMed.
- S. Xia, Z. Zhang, F. Zhong and J. Zhang, High efficiency removal of 2-chlorophenol from drinking water by a hydrogen-based polyvinyl chloride membrane biofilm reactor, J. Hazard. Mater., 2011, 186, 1367–1373 CrossRef CAS PubMed.
- O. Modin, K. Fukushi, F. Nakajima and K. Yamamoto, A membrane biofilm reactor achievesaerobic methane oxidation coupled to denitrification (AME-D) with high efficiency, Water Sci. Technol., 2008, 58, 83–87 CrossRef CAS PubMed.
- O. Modin, K. Fukushi and K. Yamamoto, Simultaneous removal of nitrate and pesticides from groundwater using a methane-fed membrane biofilm reactor, Water Sci. Technol., 2008, 58, 1273–1279 CrossRef CAS PubMed.
- D. H. Ju, J. H. Shin, H. K. Lee, S. H. Kong, J. I. Kim and B. I. Sang, Effects of pH conditions on the biological conversion of carbon dioxide to methane in a hollow-fiber membrane biofilm reactor (Hf-MBfR), Desalination, 2008, 234, 409–415 CrossRef CAS.
- H. Odegaard, B. Rusten and T. Westrum, A new moving bed biofilm reactor - applications and results, Water Sci. Technol., 1994, 29, 157–165 CrossRef.
- D. Karadag, O. E. Koroglu, B. Ozkaya, M. Cakmakci, S. Heaven, C. Banks and A. Serna-Maza, Anaerobic granular reactors for the treatment of dairy wastewater: A review, Int. J. Dairy Technol., 2015, 68, 459–470 CrossRef.
- E. M. Gilbert, S. Agrawal, S. M. Karst, H. Horn, P. H. Nielsen and S. Lackner, Low temperature partial nitritation/anammox in a moving bed biofilm reactor treating low strength wastewater, Environ. Sci. Technol., 2014, 48, 8784–8792 CrossRef CAS PubMed.
- A. Malovanyy, J. Yang, J. Trela and E. Plaza, Combination of upflow anaerobic sludge blanket (UASB) reactor and partial nitritation/anammox moving bed biofilm reactor (MBBR) for municipal wastewater treatment, Bioresour. Technol., 2015, 180, 144–153 CrossRef CAS PubMed.
- B. Rusten, B. Eikebrokk, Y. Ulgenes and E. Lygren, Design and operations of the Kaldnes moving bed biofilm reactors, Aquac. Eng., 2006, 34, 322–331 CrossRef.
- J. A. Puhakka, E. S. Melin, K. T. Jarvinen, P. M. Koro, J. A. Rintala, P. Hartikainen, W. K. Shieh and J. F. Ferguson, Fluidized-bed biofilms for chlorophenol mineralization, Water Sci. Technol., 1995, 31, 227–235 CrossRef CAS.
- K. Kida, S. Morimura, Y. Sonoda, M. Obe and T. Kondo, Support media for microbial adhesion in an anaerobic fluidized-bed reactor, J. Biosci. Bioeng., 1990, 69, 354–359 CrossRef CAS.
- M. Khraisheh, F. AlMomani, M. Inamdar, M. K. Hassan and M. A. Al-Ghouti, Ionic liquids application for wastewater treatment and biofuel production: A mini review, J. Mol. Liq., 2021, 337, 116421 CrossRef CAS.
- P. L. McCarty and T. E. Meyer, Numerical model for biological fluidized-bed reactor treatment of perchlorate-contaminated groundwater, Environ. Sci. Technol., 2005, 39, 850–858 CrossRef CAS PubMed.
- F. Malaspina, C. M. Cellamare, L. Stante and A. Tilche, Anaerobic treatment of cheese whey with a downflow-upflow hybrid reactor, Bioresour. Technol., 1996, 55, 131–139 CrossRef CAS.
- R. Borja, B. Rincon, F. Raposo, J. R. Domınguez, F. Millan and A. Martın, Mesophilic anaerobic digestion in a fluidised-bed reactor of wastewater from the production of protein isolates from chickpea flour, Process Biochem., 2004, 39, 1913–1921 CrossRef CAS.
- B. J. Brosilow, M. Schnitzer, S. Tarre and M. Green, A simple model describing nitrate and nitrite reduction in fluidized bed biological reactor, Biotechnol. Bioeng., 1997, 54, 543–548 CrossRef CAS.
- K. Rabaey, K. Van de Sompel, L. Maignien, N. Boon, P. Aelterman, P. Clauwaert, L. De Schamphelaire, H. T. Pham, J. Vermeulen, M. Verhaege and P. Lens, Microbial fuel cells for sulfide removal, Environ. Sci. Technol., 2006, 40, 5218–5224 CrossRef CAS PubMed.
- B. R. Ringeisen, R. Ray and B. Little, A miniature microbial fuel cell operating with an aerobic anode chamber, J. Power Sources, 2007, 165, 591–597 CrossRef CAS.
- B. Saba, A. D. Christy, Z. Yu, A. C. Co, R. Islam and O. H. Tuovinen, Characterization and performance of anodic mixed culture biofilms in submersed microbial fuel cells, Bioelectrochemistry, 2017, 113, 79–84 CrossRef CAS PubMed.
- B. E. Logan, B. Hamelers, R. Rozendal, U. Schroder, J. Keller, S. Freguia, P. Aelterman, W. Verstraete and K. Rabaey, Microbial fuel cells: methodology and technology, Environ. Sci. Technol., 2006, 40, 5181–5192 CrossRef CAS PubMed.
- B. Min and B. E. Logan, Continuous electricity generation from domestic wastewater and organic substrates in a flat plate microbial fuel cell, Environ. Sci. Technol., 2004, 38, 5809–5814 CrossRef CAS PubMed.
- N. Jaafarzadeh, A. Takdastan, S. Jorfi, F. Ghanbari, M. Ahmadi and G. Barzegar, The performance study on ultrasonic/Fe3O4/H2O2 for degradation of azo dye and real textile wastewater treatment, J. Mol. Liq., 2018, 256, 462–470 CrossRef CAS.
- L. Huang, X. Chai, S. Cheng and G. Chen, Evaluation of carbon-based materials in tubular biocathode microbial fuel cells in terms of hexavalent chromium reduction and electricity generation, Chem. Eng. J., 2011, 166, 652–661 CrossRef CAS.
- M. Ghasemi, S. Shahgaldi, M. Ismail, Z. Yaakob and W. R. W. Daud, New generation of carbon nanocomposite proton exchange membranes in microbial fuel cell systems, Chem. Eng. J., 2012, 184, 82–89 CrossRef CAS.
- C. Santoro, C. Arbizzani, B. Erable and I. Ieropoulos, Microbial fuel cells: From fundamentals to applications. A review, J. Power Sources, 2017, 356, 225–244 CrossRef CAS PubMed.
- T. D. Soštarić, M. S. Petrović, F. T. Pastor, D. R. Lončarević, J. T. Petrović, J. V. Milojković and M. D. Stojanović, Study of heavy metals biosorption on native and alkali-treated apricot shells and its application in wastewater treatment, J. Mol. Liq., 2018, 59, 340–349 CrossRef.
- Y. Fan, E. Sharbrough and H. Liu, Quantification of the internal resistance distribution of microbial fuel cells, Environ. Sci. Technol., 2008, 42, 8101–8107 CrossRef CAS PubMed.
- E. Blanchet, E. Desmond, B. Erable, A. Bridier, T. Bouchez and A. Bergel, Comparison of synthetic medium and wastewater used as dilution medium to design scalable microbial anodes: application to food waste treatment, Bioresour. Technol., 2015, 185, 106–115 CrossRef CAS PubMed.
- F. Zhang, Y. Ahn and B. E. Logan, Treating refinery wastewaters in microbial fuel cells using separator electrode assembly or spaced electrode configurations, Bioresour. Technol., 2014, 152, 46–52 CrossRef CAS PubMed.
- P. Clauwaert, K. Rabaey, P. Aelterman, L. De Schamphelaire, T. H. Pham, P. Boeckx, N. Boon and W. Verstraete, Biological denitrification in microbial fuel cells, Environ. Sci. Technol., 2007, 41, 3354–3360 CrossRef CAS PubMed.
- L. Zhang, J. Li, X. Zhu, D. Ye and Q. Liao, Anodic current distribution in a liter-scale microbial fuel cell with electrode arrays, Chem. Eng. J., 2013, 223, 623–631 CrossRef CAS.
- Y. Fan, S. K. Han and H. Liu, Improved performance of CEA microbial fuel cells with increased reactor size, Energy Environ. Sci., 2012, 5, 8273–8280 RSC.
- P. Aelterman, M. Versichele, M. Marzorati, N. Boon and W. Verstraete, Loading rate and external resistance control the electricity generation of microbial fuel cells with different three-dimensional anodes, Bioresour. Technol., 2008, 99, 8895–8902 CrossRef CAS PubMed.
- S. H. Shin, Y. J. Choi, S. H. Na, S. H. Jung and S. H. Kim, Development of bipolar plate stack type microbial fuel cells, Bull. Korean Chem. Soc., 2006, 27, 281–285 CrossRef CAS.
- S. E. Oh and B. E. Logan, Voltage reversal during microbial fuel cell stack operation, J. Power Sources, 2007, 167, 11–17 CrossRef CAS.
- L. Zhuang and S. Zhou, Substrate cross-conduction effect on the performance of serially connected microbial fuel cell stack, Electrochem. Commun., 2009, 11, 937–940 CrossRef CAS.
- H. R. Noormohamadi, M. R. Fathi, M. Ghaedi, S. Azizzadeh and V. Nobakht, Mechanochemically synthesized Ag (I) coordination polymer as a new adsorbent and its application to ultrasound assisted wastewater treatment via the central composite design: Isotherm and kinetic studies, J. Mol. Liq., 2018, 262, 71–77 CrossRef CAS.
- I. Naz, D. P. Saroj, S. Mumtaz, N. Ali and S. Ahmed, Assessment of biological trickling filter systems with various packing materials for improved wastewater treatment, Environ. Technol., 2015, 36, 424–434 CrossRef CAS PubMed.
- X. Zhang, J. Li, Y. Yu, R. Xu and Z. Wu, Biofilm characteristics in natural ventilation trickling filters (NVTFs) for municipal wastewater treatment: Comparison of three kinds of biofilm carriers, Biochem. Eng. J., 2016, 106, 87–96 CrossRef CAS.
- J. Yang, Y. Wang, B. Chen, Y. Chen and X. Zhang, Applications of methane-based membrane biofilm reactor for oxidized pollutants removal from wastewater: a short review, IOP Conf. Ser.: Earth Environ. Sci., 2021, 676, 012011 CrossRef.
- J. H. Luo, M. Wu, Z. Yuan and J. Guo, Biological bromate reduction driven by methane in a membrane biofilm reactor, Environ. Sci. Technol. Lett., 2017, 4, 562–566 CrossRef CAS.
- S. Bosu, N. Rajamohan, M. Rajasimman, N. Raut and Y. Vasseghian, Hydrogen based membrane bioreactor for environmental remediation of pollutants-Review on applications and mechanism, Int. J. Hydrogen Energy, 2022, 6546–6559 Search PubMed.
- W. Zhang, Y. Wu, J. Wu, X. Zheng and Y. Chen, Enhanced removal of sulfur-containing organic pollutants from actual wastewater by biofilm reactor: Insights of sulfur transformation and bacterial metabolic traits, Environ. Pollut., 2022, 313, 120187 CrossRef CAS PubMed.
- F. Qaderi, A. H. Sayahzadeh and M. Azizi, Efficiency optimization of petroleum wastewater treatment by using of serial moving bed biofilm reactors, J. Clean. Prod., 2018, 192, 665–677 CrossRef CAS.
- A. K. Swain, A. Sahoo, H. M. Jena and H. Patra, Industrial wastewater treatment by aerobic inverse fluidized bed biofilm reactors (AIFBBRs): a review, J. Water Process. Eng., 2018, 23, 61–74 CrossRef.
- M. O. Idris, H. C. Kim, A. A. Yaqoob and M. N. M. Ibrahim, Exploring the effectiveness of microbial fuel cell for the degradation of organic pollutants coupled with bio-energy generation. Sustain., Energy Technol. Assess., 2022, 52, 102183 Search PubMed.
- Y. Yuan, H. Lin, Z. Lin and Y. Wang, A review of hydrogen-based membrane biofilm reactor to remove oxidized pollutants from water, IOP Conf. Ser: Mater. Sci. Eng., 2018, 392, 042031 Search PubMed.
- W. Yin, Y. Wang, L. Liu and J. He, Biofilms: the microbial “protective clothing” in extreme environments, Int. J. Mol. Sci., 2019, 20, 3423 CrossRef CAS PubMed.
- S. Stasik, L. Y. Wick and K. Wendt-Potthoff, Anaerobic BTEX degradation in oil sands tailings ponds: impact of labile organic carbon and sulfate-reducing bacteria, Chemosphere, 2015, 138, 133–139 CrossRef CAS PubMed.
- S. M. Ibrahim and A. F. Al-Hossainy, Kinetics and mechanism of oxidation of bromothymol blue by permanganate ion in acidic medium: application to textile industrial wastewater treatment, J. Mol. Liq., 2020, 318, 114041 CrossRef CAS.
- A. Maurya and A. Raj, Recent advances in the application of biofilm in bioremediation of industrial wastewater and organic pollutants, Microorganisms Sustain, Environ. Health, 2020, 81–118 Search PubMed.
- M. J. Alessandrello, M. S. J. Tomas, E. E. Raimondo, D. L. Vullo and M. A. Ferrero, Petroleum oil removal by immobilized bacterial cells on polyurethane foam under different temperature conditions, Mar. Pollut. Bull., 2017, 122, 156–160 CrossRef CAS PubMed.
- C. C. Tang, Y. Tian, Z. W. He, W. Zuo and J. Zhang, Performance and mechanism of a novel algal-bacterial symbiosis system based on sequencing batch suspended biofilm reactor treating domestic wastewater, Bioresour. Technol., 2018, 265, 422–431 CrossRef CAS PubMed.
- G. T. Ooi, K. Tang, R. K. Chhetri, K. M. Kaarsholm, K. Sundmark, C. Kragelund, K. Litty, A. Christensen, S. Lindholst, C. Sund and M. Christensson, Biological removal of pharmaceuticals from hospital wastewater in a pilot-scale staged moving bed biofilm reactor (MBBR) utilising nitrifying and denitrifying processes, Bioresour. Technol., 2018, 267, 677–687 CrossRef CAS PubMed.
- E. Torresi, A. Gulay, F. Polesel, M. M. Jensen, M. Christensson, B. F. Smets and B. G. Plosz, Reactor staging influences microbial community composition and diversity of denitrifying MBBRs-Implications on pharmaceutical removal, Water Res., 2018, 138, 333–345 CrossRef CAS PubMed.
- E. Torresi, K. Tang, J. Deng, C. Sund, B. F. Smets, M. Christensson and H. R. Andersen, Removal of micropollutants during biological phosphorus removal: Impact of redox conditions in MBBR, Sci. Total Environ., 2019, 663, 496–506 CrossRef CAS PubMed.
- Y. Yang, Z. Shao, J. Du, Q. He and H. Chai, Enhancement of organic matter removal in an integrated
biofilm-membrane bioreactor treating high-salinity wastewater, Archaea, 2018, 2018, 2148286 CrossRef PubMed.
- F. Polesel, E. Torresi, L. Loreggian, M. E. Casas, M. Christensson, K. Bester and B. G. Plosz, Removal of pharmaceuticals in pre-denitrifying MBBR-Influence of organic substrate availability in single-and three-stage configurations, Water Res., 2018, 123, 408–419 CrossRef PubMed.
- S. M. Abtahi, M. Petermann, A. J. Flambard, S. Beaufort, F. Terrisse, T. Trotouin, C. J. Cassan and C. Albasi, Micropollutants removal in tertiary moving bed biofilm reactors (MBBRs): Contribution of the biofilm and suspended biomass, Sci. Total Environ., 2018, 643, 1464–1480 CrossRef CAS PubMed.
- X. An, Y. Cheng, M. Huang, Y. Sun, H. Wang, X. Chen, J. Wang, D. Li and C. Li, Treating organic cyanide-containing groundwater by immobilization of a nitrile-degrading bacterium with a biofilm-forming bacterium using fluidized bed reactors, Environ. Pollut., 2018, 237, 908–916 CrossRef CAS PubMed.
- S. Dey and A. K. Paul, Influence of metal ions on biofilm formation by Arthrobacter sp. SUK 1205 and evaluation of their Cr(VI) removal efficacy, Int. Biodeterior. Biodegrad., 2018, 132, 122–131 CrossRef CAS.
- E. A. Parellada, M. Igarza, P. Isacc, A. Bardón, M. Ferrero, K. L. Ameta and A. Neske, Squamocin, an annonaceousacetogenin, enhances naphthalene degradation mediated by Bacillus atrophaeus CN4, Rev. Argent. Microbiol., 2017, 49, 282–288 Search PubMed.
- L. T. Nhi Cong, C. T. Ngoc Mai, V. T. Thanh, L. P. Nga and N. N. Minh, Application of a biofilm formed by a mixture of yeasts isolated in Vietnam to degrade aromatic hydrocarbon polluted wastewater collected from petroleum storage, Water Sci. Technol., 2014, 70, 329–336 CrossRef PubMed.
- S. I. Shayan, F. A. Agblevor, L. Bertin and R. C. Sims, Hydraulic retention time effects on wastewater nutrient removal and bioproduct production via rotating algal biofilm reactor, Bioresour. Technol., 2016, 211, 527–533 CrossRef PubMed.
- F. Gao, Z. H. Yang, C. Li, G. M. Zeng, D. H. Ma and L. Zhou, A novel algal biofilm membrane photobioreactor for attached microalgae growth and nutrients removal from secondary effluent, Bioresour. Technol., 2015, 179, 8–12 CrossRef CAS PubMed.
- M. Burmølle, D. Ren, T. Bjarnsholt and S. J. Sørensen, Interactions in multispecies biofilms: do they actually matter?, Trends Microbiol., 2014, 22, 84–91 CrossRef PubMed.
- T. Kindaichi, I. Tsushima, Y. Ogasawara, M. Shimokawa, N. Ozaki, H. Satoh and S. Okabe,
In situ activity and spatial organization of anaerobic ammonium-oxidizing (anammox) bacteria in biofilms, Appl. Environ. Microbiol., 2007, 73, 4931–4939 CrossRef CAS PubMed.
- E. Syron and E. Casey, Membrane-aerated biofilms for high rate biotreatment: performance appraisal, engineering principles, scale-up, and development requirements, Environ. Sci. Technol., 2008, 42, 1833–1844 CrossRef CAS PubMed.
- N. C. M. Gomes, L. R. Borges, R. Paranhos, F. N. Pinto, E. Krögerrecklenfort, L. C. Mendonça-Hagler and K. Smalla, Diversity of ndo genes in mangrove sediments exposed to different sources of polycyclic aromatic hydrocarbon pollution, Appl. Environ. Microbiol., 2007, 73, 7392–7399 CrossRef CAS PubMed.
- D. Osterreicher-Ravid, E. Z. Ron and E. Rosenberg, Horizontal transfer of an exopolymer complex from one bacterial species to another, Environ. Microbiol., 2000, 2, 366–372 CrossRef CAS PubMed.
- S. Yoshida, N. Ogawa, T. Fujii and S. Tsushima, Enhanced biofilm formation and 3-chlorobenzoate degrading activity by the bacterial consortium of Burkholderia sp. NK8 and Pseudomonas aeruginosa PAO1, J. Appl. Microbiol., 2009, 106, 790–800 CrossRef CAS PubMed.
- B. A. Stubblefield, K. E. Howery, B. N. Islam, A. J. Santiago, W. E. Cardenas and E. S. Gilbert, Constructing multispecies biofilms with defined compositions
by sequential deposition of bacteria, Appl. Microbiol. Biotechnol., 2010, 86, 1941–1946 CrossRef CAS PubMed.
- M. I. Hossain, A. Paparini and R. Cord-Ruwisch, Rapid adaptation of activated sludge bacteria into a glycogen accumulating biofilm enabling anaerobic BOD uptake, Bioresour. Technol., 2017, 228, 1–8 CrossRef CAS PubMed.
- J. Dobor, M. Varga and G. Zaray, Biofilm controlled sorption of selected acidic drugs on river sediments characterized by different organic carbon content, Chemosphere, 2012, 87, 105–110 CrossRef CAS PubMed.
- M. Shahzad, E. Millhouse, S. Culshaw, C. A. Edwards, G. Ramage and E. Combet, Selected dietary (poly) phenols inhibit periodontal pathogen growth and biofilm formation, Food Funct., 2015, 6, 719–729 RSC.
- L. Zhang, D. Dong, X. Hua and Z. Guo, Inhibitory effects of extracellular polymeric substances on ofloxacin sorption by natural biofilms, Sci. Total Environ., 2018, 625, 178–184 CrossRef CAS PubMed.
- J. L. M. Welch, B. J. Rossetti, C. W. Rieken, F. E. Dewhirst and G. G. Borisy, Biogeography of a human oral microbiome at the micron scale, Proc. Natl. Acad. Sci. U. S. A., 2016, 113, E791–E800 CrossRef PubMed.
- J. J. Harrison, H. Ceri and R. J. Turner, Multimetal resistance and tolerance in microbial biofilms, Nat. Rev. Microbiol., 2007, 5, 928–938 CrossRef CAS PubMed.
- S. J. Edwards and B. V. Kjellerup, Applications of biofilms in bioremediation and biotransformation of persistent organic pollutants, pharmaceuticals/personal care products, and heavy metals, Appl. Microbiol. Biotechnol., 2013, 97, 9909–9921 CrossRef CAS PubMed.
- M. A. Delcau, V. A. Henry, E. R. Pattee and T. L. Peeples, Mode of Growth and Temperature Dependence on Expression of Atrazine-degrading Genes in Pseudomonas sp. strain ADP Biofilms, bioRxiv, 2018, 302877 Search PubMed.
- S. Kumari, N. Mangwani and S. Das, Synergistic effect of quorum sensing genes in biofilm development and PAHs degradation by a marine bacterium, Bioengineered, 2016, 7, 205–211 CrossRef CAS PubMed.
- C. S. Chen, T. W. Wu, H. L. Wang, S. H. Wu and C. J. Tien, The ability of immobilized bacterial consortia and strains from river biofilms to degrade the carbamate pesticide methomyl, Int. J. Environ. Sci. Technol., 2015, 12, 2857–2866 CrossRef CAS.
-
B. Horemans, P. Albers and D. Springael, The biofilm concept from a bioremediation perspective. Biofilms in Bioremediation: Current Research and Emerging Technologies, Caister Academic Press, 2015, pp. 23–40 Search PubMed.
- C. J. Tien, M. C. Lin, W. H. Chiu and C. S. Chen, Biodegradation of carbamate pesticides by natural river biofilms in different seasons and their effects on biofilm community structure, Environ. Pollut., 2013, 179, 95–104 CrossRef CAS PubMed.
- D. Long, L. Zou, M. Z. Hashmi, K. Cai, X. Tang, G. Chen and J. Shi, Determination of the accumulation, spatial distribution and reduction of Cr in unsaturated Pseudochrobactrumsaccharolyticum LY10 biofilms by X-ray fluorescence and absorption methods, Chem. Eng. J., 2015, 280, 763–770 CrossRef CAS.
- T. Pani, A. Das and J. W. Osborne, Bioremoval of zinc and manganese by bacterial biofilm: a bioreactor-based approach, J. Photochem. Photobiol., B, 2017, 175, 211–218 CrossRef CAS PubMed.
- G. M. Teitzel and M. R. Parsek, Heavy metal resistance of biofilm and planktonic Pseudomonas aeruginosa, Appl. Environ. Microbiol., 2003, 69, 2313–2320 CrossRef CAS PubMed.
- A. Naeem, R. Batool and N. Jamil, Cr(VI) reduction by Cellulosimicrobium sp. isolated from tannery effluent, Turk. J. Biol., 2013, 37, 315–322 CAS.
- S. Focardi, M. Pepi, G. Landi, S. Gasperini, M. Ruta, P. Di Biasio and S. E. Focardi, Hexavalent chromium reduction by whole cells and cell free extract of the moderate halophilic bacterial strain Halomonas sp. TA-04, Int. Biodeterior. Biodegrad., 2012, 66, 63–70 CrossRef CAS.
- S. Grumbein, M. Opitz and O. Lieleg, Selected metal ions protect Bacillus subtilis biofilms from erosion, Metallomics, 2014, 6, 1441–1450 CrossRef CAS PubMed.
- B. Cao, B. Ahmed, D. W. Kennedy, Z. Wang, L. Shi, M. J. Marshall, J. K. Fredrickson, N. G. Isern, P. D. Majors and H. Beyenal, Contribution of extracellular polymeric substances from Shewanella sp. HRCR-1 biofilms to U (VI) immobilization, Environ. Sci. Technol., 2011, 45, 5483–5490 CrossRef CAS PubMed.
- N. Zhu, J. Zhang, J. Tang, Y. Zhu and Y. Wu, Arsenic removal by periphytic biofilm and its application combined with biochar, Bioresour. Technol., 2018, 248, 49–55 CrossRef CAS PubMed.
- A. R. Lopez, D. H. Funk and D. B. Buchwalter, Arsenic (V) bioconcentration kinetics in freshwater macroinvertebrates and periphyton is influenced by pH, Environ. Pollut., 2017, 224, 82–88 CrossRef CAS PubMed.
- E. Torresi, F. Polesel, K. Bester, M. Christensson, B. F. Smets, S. Trapp, H. R. Andersen and B. G. Plósz, Diffusion and sorption of organic micropollutants in biofilms with varying thicknesses, Water Res., 2017, 123, 388–400 CrossRef CAS PubMed.
- A. Mathur, A. Parashar, N. Chandrasekaran and A. Mukherjee, Nano-TiO2 enhances biofilm formation in a bacterial isolate from activated sludge of a waste water treatment plant, Int. Biodeterior. Biodegrad., 2017, 116, 17–25 CrossRef CAS.
- C. C. Tang, Y. Tian, H. Liang, W. Zuo, Z. W. Wang, J. Zhang and Z. W. He, Enhanced nitrogen and phosphorus removal from domestic wastewater via algae-assisted sequencing batch biofilm reactor, Bioresour. Technol., 2018, 250, 185–190 CrossRef CAS PubMed.
- K. G. Quiton, B. Doma Jr, C. M. Futalan and M. W. Wan, Removal of chromium (VI) and zinc(II) from aqueous solution using kaolin-supported bacterial biofilms of Gram-negative E. coli and Gram-positive Staphylococcus epidermidis, Sustainable Environ. Res., 2018, 28, 206–213 CrossRef CAS.
- W. Han, Y. Mao, Y. Wei, P. Shang and X. Zhou, Bioremediation of aquaculture wastewater with algal-bacterial biofilm combined with the production of selenium rich biofertilizer, Water, 2020, 12, 2071 CrossRef CAS.
- Y. Y. Peng, F. Gao, H. L. Yang, C. Li, M. M. Lu and Z. Y. Yang, Simultaneous removal of nutrient and sulfonamides from marine aquaculture wastewater by concentrated and attached cultivation of Chlorella vulgaris in an algal biofilm membrane photobioreactor (BF-MPBR), Sci. Total Environ., 2020, 725, 138524 CrossRef CAS PubMed.
- G. Akerman-Sanchez and K. Rojas-Jimenez, Fungi for the bioremediation of pharmaceutical-derived pollutants: A bioengineering approach to water treatment, Environ. Adv., 2021, 4, 100071 CrossRef CAS.
- S. Balu, S. Bhunia, R. Gachhui and J. Mukherjee, Assessment of polycyclic aromatic hydrocarbon contamination in the Sundarbans, the world's largest tidal mangrove forest and indigenous microbial mixed biofilm-based removal of the contaminants., Environ. Pollut., 2020, 266, 115270 CrossRef CAS PubMed.
- X. Yuan, X. Zhang, X. Chen, D. Kong, X. Liu and S. Shen, Synergistic degradation of crude oil by indigenous bacterial consortium and exogenous fungus Scedosporium boydii, Bioresour. Technol., 2018, 264, 190–197 CrossRef CAS PubMed.
- D. M. Al-Mailem, M. K. Kansour and S. S. Radwan, Bioremediation of hydrocarbons contaminating sewage effluent using man-made biofilms: effects of some variables, Appl. Biochem. Biotechnol., 2014, 174, 1736–1751 CrossRef CAS PubMed.
- E. Hoque and J. Fritscher, A new mercury accumulating Mucor hiemalis strain EH8 from cold sulfidic spring water biofilms, MicrobiologyOpen, 2016, 5, 763–781 CrossRef CAS PubMed.
- S. Hussain, L. Quinn, J. Li, E. Casey and C. D. Murphy, Simultaneous removal of malachite green and hexavalent chromium by Cunninghamella elegans biofilm in a semi-continuous system, Int. Biodeterior. Biodegrad., 2017, 125, 142–149 CrossRef CAS.
- W. Wang, S. Zhu, N. Li, S. Xie, C. Wen and X. Luo, Enhanced Cd2+ adsorption and toxicity for microbial
biofilms in the presence of TiO2 nanoparticles, Environ. Pollut., 2022, 314, 120239 CrossRef CAS PubMed.
- H. M. L. I. Herath, A. U. Rajapaksha, M. Vithanage and G. Seneviratne, Developed fungal–bacterial biofilms as a novel tool for bioremoval of hexavelant chromium from wastewater, Chem. Ecol., 2014, 30, 418–427 CrossRef CAS.
- C. Rossi, C. Chaves-LOpez, A. Serio, E. Goffredo, B. T. C. Goga and A. Paparella, Influence of incubation conditions on biofilm formation by Pseudomonas fluorescens isolated from dairy products and dairy manufacturing plants, Ital. J. Food Saf., 2016, 5, 5793 Search PubMed.
- R. K. Agarwal, S. Singh, K. N. Bhilegaonkar and V. P. Singh, Optimization of microtitre plate assay for the testing of biofilm formation ability in different Salmonella serotypes, Int. Food Res. J., 2011, 18, 1493–1498 Search PubMed.
- A. Rochex and J. M. Lebeault, Effects of nutrients on biofilm formation and detachment of a Pseudomonas putida strain isolated from a paper machine, Water Res., 2007, 41, 2885–2892 CrossRef CAS PubMed.
- S. Sehar and I. Naz, Role of the biofilms in wastewater treatment, Microb. Biofilms: Importance Appl., 2016, 121–144 CAS.
- K. K. Jefferson, What drives bacteria to produce a biofilm?, FEMS Microbiol. Lett., 2004, 236, 163–173 CrossRef CAS PubMed.
- G. A. O'Toole and R. Kolter, Initiation of biofilm formation in Pseudomonas fluorescens WCS365 proceeds via multiple, convergent signalling pathways: a genetic analysis, Mol. Microbiol., 1998, 28, 449–461 CrossRef PubMed.
- S. L. Sanin, F. D. Sanin and J. D. Bryers, Effect of starvation on the adhesive properties of xenobiotic degrading bacteria, Process Biochem., 2003, 38, 909–914 CrossRef CAS.
- C. J. Volk and M. W. LeChevallier, Impacts of the reduction of nutrient levels on bacterial water quality in distribution systems, Appl. Environ. Microbiol., 1999, 65, 4957–4966 CrossRef CAS PubMed.
- J. Frias, F. Ribas and F. Lucena, Effects of different nutrients on bacterial growth in a pilot distribution system, Antonie van Leeuwenhoek, 2001, 80, 129–138 CrossRef CAS PubMed.
- T. Bühler, S. Ballestero, M. Desai and M. R. W. Brown, Generation of a reproducible nutrient-depleted biofilm of Escherichia coli and Burkholderiacepacia, J. Appl. Microbiol., 1998, 85, 457–462 CrossRef PubMed.
- D. W. Jackson, J. W. Simecka and T. Romeo, Catabolite repression of Escherichia coli biofilm formation, J. Bacterial, 2002, 184, 3406–3410 CrossRef CAS PubMed.
- M. Oder and K. GodicTorkar, The influence of shear stress on the adhesion capacity of Legionella pneumophila, Arh. Za Hig. Rada Toksikol., 2017, 68, 109–115 CrossRef CAS PubMed.
- A. Pompilio, R. Piccolomini, C. Picciani, D. D'Antonio, V. Savini and G. Di Bonaventura, Factors associated with adherence to and biofilm formation on polystyrene by Stenotrophomonas maltophilia: the role of cell surface hydrophobicity and motility, FEMS Microbiol. Lett., 2008, 287, 41–47 CrossRef CAS PubMed.
- A. Nostro, L. Cellini, M. Di Giulio, M. D'Arrigo, A. Marino, A. R. Blanco, A. Favaloro, G. Cutroneo and G. Bisignano, Effect of alkaline pH on staphylococcal biofilm formation, APMIS, 2012, 120, 733–742 CrossRef CAS PubMed.
- K. Chaieb, O. Chehab, T. Zmantar, M. Rouabhia, K. Mahdouani and A. Bakhrouf, In vitro effect of pH and ethanol on biofilm formation by clinicalica-positiveStaphylococcus epidermidis strains, Ann. Microbiol., 2007, 57, 431–437 CrossRef CAS.
- T. Zmantar, B. Kouidhi, H. Miladi, K. Mahdouani and A. Bakhrouf, A microtiter plate assay for Staphylococcus aureus biofilm quantification at various pH levels and hydrogen peroxide supplementation, New Microbiol., 2010, 33, 137–145 CAS.
- R. Oliveira, L. Melo, A. Oliveira and R. Salgueiro, Polysaccharide production and biofilm formation by Pseudomonas fluorescens: effects of pH and surface material, Colloids Surf., B, 1994, 2, 41–46 CrossRef CAS.
- P. C. Bogino, M. D. L. M. Oliva, F. G. Sorroche and W. Giordano, The role of bacterial biofilms and surface components in plant-bacterial associations, Int. J. Mol. Sci., 2013, 14, 15838–15859 CrossRef PubMed.
- A. Maleki, B. Hayati, F. Najafi, F. Gharibi and S. W. Joo, Heavy metal adsorption from industrial wastewater by PAMAM/TiO2 nanohybrid: preparation, characterization and adsorption studies, J. Mol. Liq., 2016, 24, 95–104 CrossRef.
- V. O. Adetunji and I. A. Odetokun, Biofilm formation in human and tropical foodborne isolates of Listeria strains, Am. J. Food Technol., 2012, 7, 517–531 CrossRef.
- T. R. Garrett, M. Bhakoo and Z. Zhang, Bacterial adhesion and biofilms on surfaces, Prog. Nat. Sci.: Mater., 2008, 18, 1049–1056 CrossRef CAS.
- T. C. Ells and L. T. Hansen, Strain and growth temperature influence Listeria spp. attach-ment to intact and cut cabbage, Int. J. Food Microbiol., 2006, 111, 34–42 CrossRef PubMed.
- Y. Chang, A. A. Fragkopoulos, S. M. Marquez, H. D. Kim, T. E. Angelini and A. Fernandez-Nieves, Biofilm formation in geometries with different surface curvature and oxygen availability, New J. Phys., 2015, 17, 033017 CrossRef.
- R. Pagan and D. García-Gonzalo, Influence of Environmental factors on bacterial biofilm formation in the food industry: a review, Postdoc J., 2015, 3, 3–13 Search PubMed.
- S. J. Ahn and R. A. Burne, Effects of oxygen on biofilm formation and the AtlA autolysin of Streptococcus mutans, J. Bacteriol., 2007, 189, 6293–6302 CrossRef CAS PubMed.
- S. Keleştemur, E. Avci and M. Culha, Raman and surface-enhanced Raman scattering for biofilm characterization, Chemosensors, 2018, 6, 5 CrossRef.
- M. Toyofuku, T. Inaba, T. Kiyokawa, N. Obana, Y. Yawata and N. Nomura, Environmental factors that shape biofilm formation, Biosci., Biotechnol., Biochem., 2016, 80, 7–12 CrossRef CAS PubMed.
- C. G. Kumar and S. K. Anand, Significance of microbial biofilms in food industry: a review, Int. J. Food Microbiol., 1998, 42, 9–27 CrossRef CAS PubMed.
- F. Ahimou, M. J. Semmens, G. Haugstad and P. J. Novak, Effect of protein, polysaccharide, and oxygen concentration profiles on biofilm cohesiveness, Appl. Environ. Microbiol., 2007, 73, 2905–2910 CrossRef CAS PubMed.
- L. A. Bjergbæk, J. A. J. Haagensen, A. Reisner, S. Molin and P. Roslev, Effect of oxygen and growth medium on in vitro biofilm formation by Escherichia coli, Biofilms, 2006, 3, 1–10 CrossRef.
- D. Worlitzsch, R. Tarran, M. Ulrich, U. Schwab, A. Cekici, K. C. Meyer, P. Birrer, G. Bellon, J. Berger, T. Weiss and K. Botzenhart, Effects of reduced mucus oxygen concentration in airway Pseudomonas infections of cystic fibrosis patients, J. Clin. Invest., 2002, 109, 317–325 CrossRef CAS PubMed.
- H. Mulcahy, L. Charron-Mazenod and S. Lewenza, Extracellular DNA chelates cations and induces antibiotic resistance in Pseudomonas aeruginosa biofilms, PLoS Pathog., 2008, 4, 1000213 CrossRef PubMed.
- A. J. DeKerchove and M. Elimelech, Calcium and magnesium cations enhance the adhesion of motile and nonmotile Pseudomonas aeruginosa on alginate films, Langmuir, 2008, 24, 3392–3399 CrossRef CAS PubMed.
- T. Das, S. Sehar, L. Koop, Y. K. Wong, S. Ahmed, K. S. Siddiqui and M. Manefield, Influence of calcium in extracellular DNA mediated bacterial aggregation and biofilm formation, PLoS One, 2014, 9, 91935 CrossRef PubMed.
- S. Sarkisova, M. A. Patrauchan, D. Berglund, D. E. Nivens and M. J. Franklin, Calcium-induced virulence factors associated with the extracellular matrix of mucoid Pseudomonas aeruginosa biofilms, J. Bacteriol., 2005, 187, 4327–4337 CrossRef CAS PubMed.
- B. Steyn, M. C. Oosthuizen, R. MacDonald, J. Theron and V. S. Brozel, The use of glass wool as an attachment surface for studying phenotypic changes in Pseudomonas aeruginosa biofilms by two-dimensional gel electrophoresis, Proteomics, 2001, 1, 871–879 CrossRef CAS PubMed.
- B. Prakash, B. M. Veeregowda and G. Krishnappa, Biofilms: a survival strategy of bacteria, Curr. Sci., 2003, 85, 1299–1307 Search PubMed.
- P. Becker, W. Hufnagle, G. Peters and M. Herrmann, Detection of different gene expression in biofilm-forming versus planktonic populations of Staphylococcus aureus using micro-representational-difference analysis, Appl. Environ. Microbiol., 2001, 67, 2958–2965 CrossRef CAS PubMed.
- Y. Xiong and Y. Liu, Biological control of microbial attachment: a promising alternative for mitigating membrane biofouling, Appl. Microbiol. Biotechnol., 2010, 86, 825–837 CrossRef CAS PubMed.
- C. U. Riedel, I. R. Monk, P. G. Casey, M. S. Waidmann, C. G. Gahan and C. Hill, AgrD-dependent quorum sensing affects biofilm formation, invasion, virulence and global gene expression profiles in Listeria monocytogenes, Mol. Microbiol., 2009, 71, 1177–1189 CrossRef CAS PubMed.
- U. B. Ockelmann, A. Janke, R. Kuhn, T. R. Neu, J. Wecke, J. R. Lawrence and U. Szewzyk, Bacterial extracellular DNA forming a defined network-like structure, FEMS Microbiol. Lett., 2006, 262, 31–38 CrossRef PubMed.
- T. Das, S. Sehar and M. Manefield, The roles of extracellular DNA in the structural integri-ty of extracellular polymeric substance and bacterial biofilm development, Environ. Microbiol. Rep., 2013, 5, 778–786 CrossRef CAS PubMed.
- M. Simoes, M. O. Pereira, S. Silankorva, J. Azeredo and M. J. Vieira, The effect of the hydrody-namic conditions of the phenotype of Pseudomonas fluorescens biofilms, Biofouling, 2007, 23, 249–258 CrossRef CAS PubMed.
- S. Pandey, A comprehensive review on recent developments in bentonite-based materials used as adsorbents for wastewater treatment, J. Mol. Liq., 2017, 241, 1091–1113 CrossRef CAS.
- E. Schneider, A. C. F. P. Cerqueira and M. Dezotti, MBBR evaluation for oil refinery wastewater treatment, with post-ozonation and BAC, for wastewater reuse, Water Sci. Technol., 2011, 63, 143–148 CrossRef CAS PubMed.
- A. Rodriguez-Sanchez, J. C. Leyva-Diaz, J. M. Poyatos and J. Gonzalez-Lopez, Influent salinity conditions affect the bacterial communities of biofouling in hybrid MBBR-MBR systems, J. Water Process. Eng., 2019, 30, 100650 CrossRef.
- M. Kornaros and G. Lyberatos, Biological treatment of wastewaters from a dye manufacturing company using a trickling filter, J. Hazard. Mater., 2006, 136, 95–102 CrossRef CAS PubMed.
- C. Y. Lai, P. L. Lv, Q. Y. Dong, S. L. Yeo, B. E. Rittmann and H. P. Zhao, Bromate and nitrate bioreduction coupled with poly-β-hydroxybutyrate production in a methane-based membrane biofilm reactor, Environ. Sci. Technol., 2018, 52, 7024–7031 CrossRef CAS PubMed.
- B. Rusten, J. G. Siljudalen and B. Nordeidet, Upgrading to nitrogen removal with the KMT moving bed biofilm process, Water Sci. Technol., 1994, 29, 185–195 CrossRef CAS.
- B. Rusten, L. J. Hem and H. Odegaard, Nitrogen removal from dilute wastewater in cold climate using moving-bed biofilm reactors, Water Environ. Res., 1995, 67, 65–74 CrossRef CAS.
- B. Rusten, M. McCoy, R. Proctor and J. G. Siljudalen, The innovative moving bed biofilm reactor/solids contact reaeration process for secondary treatment of municipal wastewater, Water Environ. Res., 1998, 70, 1083–1089 CrossRef CAS.
- P. Cote, H. Buisson, C. Pound and G. Arakaki, Immersed membrane activated sludge for the reuse of municipal wastewater, Desalination, 1997, 113, 189–196 CrossRef CAS.
- V. Urbain, R. Benoit and J. Manem, Membrane bioreactor: a new treatment tool, J. - Am. Water Works Assoc., 1996, 88, 75–86 CrossRef CAS.
- M. Crampon, J. Hellal, C. Mouvet, G. Wille, C. Michel, A. Wiener, J. Braun and P. Ollivier, Do natural biofilm impact nZVI mobility and interactions with porous media? A column study, Sci. Total Environ., 2018, 610, 709–719 CrossRef PubMed.
|
This journal is © The Royal Society of Chemistry 2023 |