A comprehensive review on the removal of antibiotics from water and wastewater using carbon nanotubes: synthesis, performance, and future challenges
Received
9th December 2021
, Accepted 23rd August 2022
First published on 14th November 2022
Abstract
Recently, various emerging pollutants have been detected in water and wastewater, among which antibiotics can be considered a real threat to human life and environment because of their unique properties (bio-accumulation, bio-magnification, resistance, and bio-transformation). Therefore, it is urgent to apply methods for the detection and removal of these pollutants. Given that antibiotics cannot be completely removed by conventional methods, developing alternative methods to enhance their removal efficiency should be the focus of researchers. Over the past decades, carbon nanotubes (CNTs) have gained considerable attention owing to their remarkable merits (high surface area, low density and high thermal conductivity). However, despite the numerous advantages of CNTs, they suffer from some limitations, which can be solved by surface modification. Hence, this comprehensive review aims to help readers to improve their fundamental knowledge regarding the main characteristics of common antibiotics and their environmental and health effects, their fate in the environment, common methods for their removal, physicochemical methods for the modification of CNTs, identifying the effects of various parameters (pH, dosage, initial concentration, and time) on the removal process, and the reusability and major mechanism for the adsorption of antibiotics by CNTs. Finally, we summarize the isotherm and kinetic models for the removal of antibiotics. Our results exhibit that all the data fitted the Langmuir isotherm model, which reveals that the adsorption process on modified-CNTs is homogenous and monolayer. Furthermore, herein, the adsorption kinetics is well described by the pseudo-second-order model. Overall, this review provides useful insight for young researchers to understand the adsorption of antibiotics and achieve the future goal of using CNTs as promising adsorbents.
Water impact
This review aimed for the first time to fill the knowledge gap on the efficiency of modified-CNTs for the removal of various common antibiotics as one of the major environmental pollutants. It is hoped that this review will provide useful approaches for the development and wide application of CNTs and their modification in the environmental engineering field in the future on a large scale. Herein, we focused on the structure of the common antibiotics, their health and environmental effects, the conventional methods (advantages and disadvantages) for their removal, the methods for the synthesis and preparation of pristine and modified CNTs, the effect of various experimental conditions on the system, the adsorption mechanism and the isotherm-kinetic models.
|
1 Introduction
Antibiotics are one of the most important drugs used for humans and veterinary medicine.1 It has been reported that the consumption of antibiotics has increased significantly from 100
000 to 200
000 tons per year due to the global economic and population growth.2,3 More than 50 million pounds of antibiotics (40% for animal farming and 60% for humans) are produced yearly in the United States.4 Moreover, based on statistical data, China is considered one the countries with the largest antibiotic consumption rate from 2009 to 2013 (248
000 tons).5
Antibiotics are classified based on their chemical structure and mechanism of action, and therefore, they can be subdivided into different subgroups with complex molecules such as β-lactams, tetracyclines (TC), quinolones, sulphonamides, glycopeptides, macrolides, and aminoglycosides, which may have different properties.6–8
Medicinal chemicals enter the water through two main routes, i.e., industrial and domestic activities. The most medicinal chemicals come from the pharmaceutical industry through fermentation, chemical synthesis, extraction and formulation, which contain a high concentration of antibiotics, while domestic route includes the metabolised and non-metabolised forms of antibiotics, which are excreted by patients into wastewater.9 Studies have shown that conventional wastewater treatment plants (WWTPs) only remove certain drugs by about 20–90%, indicating that antibiotics are not completely removed during biological treatment, and consequently enter the receiving waters. Moreover, because of their low biodegradability, it has reported that the concentration of antibiotics in raw domestic wastewater varies in the range from 100 ng L−1 to 6 mg L−1.10
Thus, the removal of antibiotics from wastewaters before their discharge into receiving water systems is important.11,12 It has been confirmed that the presence of antibiotics in waters is a global concern for suppling water to industries (focusing on water reuse) because of their severe ability to disrupt the microbial ecology, increase the proliferation of antibiotic-resistant pathogens, and endanger human health.13
Nowadays, researchers have used a wide variety of technologies (physical, chemical, and biological) such as ozone and UV,14 Fenton and photo-Fenton,15 coagulation and flocculation,16 nanofiltration,17 and adsorption18 for the removal of antibiotics and pharmaceutical residues from water and wastewater. Based on the results of previous research, conventional water and wastewater treatment methods cannot remove antibiotics according to the established standards.19 Therefore, researchers in the field of environmental chemistry need to develop alternative methods to overcome the shortcomings of the traditional methods.
Among the aforementioned methods, the adsorption process can be widely applied in the elimination of pollutants because of its merits such as high selectivity, low energy consumption, high reliability, low cost, simple design, design flexibility, non-toxic nature, and simple operation.20,21 However, it also has some drawbacks such as the adsorption efficiency can be restricted by various factors such as mass transport resistance, it is difficult to separate the adsorbent, the production of adsorbents is expensive and the spent adsorbents need to be disposed.22–24 Hence, researchers have attempted to develop new adsorbents, namely, nano adsorbents, to improve the efficiency of conventional adsorbents and overcome their shortcomings.25 In recent decades, nanostructured materials (dimensions of less than 100 nm) have gradually become a hot research topic. Among the new and novel materials, carbonaceous and porous materials especially carbon nanotubes (CNTs) have attracted attention from environmental scientists.26 CNTs as emerging carbonaceous materials are one of the most promising carbon adsorbents used in water and wastewater treatment for the removal of antibiotics.27 Generally, carbon nanotubes are a one-dimensional structures with a micron axial length and nanometer diameter, which includes one or more concentric graphite-like layers (0.4–10 nm in terms of diameter).28,29 Therefore, based on the number of graphite layers, CNTs can be mainly categorized into single-walled carbon nanotubes (SWCNTs) and multi-walled carbon nanotubes (MWCNTs).30 Basically, only a single hexagonal sheet of carbon atoms is rolled into a tube (with a diameter of 0.4–3 nm) in SWCNTs, whereas several hexagonal sheets of carbon atoms are rolled up and each hexagonal sheet of carbon atoms is separated by ∼0.34 nm in MWCNTs.31 Due to the outstanding properties of these promising materials such as good electrical and thermal conductivity, high specific surface area, high strength, large pore capacity,32 specific structural and functional properties (e.g., high aspect ratio, high electron emission, high optical absorption, and low energy loss),33 high adsorption performance because of the presence of oxygen-containing functional groups on their surface,34 and strong interaction with organic molecules via non-covalent forces35 play a very useful role in the field of environmental engineering. For example, CNT-activated carbon (as a high-performance suspension electrode for the electrochemical desalination of wastewater),36 ZnO/CNT nanocomposite as an electrochemical sensor,37 ruthenium/MWCNT as a catalyst,38 heavy metal uptake by MWCNTs,39 and hydrogen storage using SWCNTs.40 The results of previous studies confirmed that CNTs exhibit certain limitations such as weak dispersion in aqueous solutions,41,42 few active sites, difficult recycling,43 hydrophobicity and high production cost for their total synthesizes,27 prompting extensive research on the modification of the structure of CNTs. For instance, the poor dispersion of pristine CNTs can be solved by surface modification with the different functional groups such as hydroxide (OH), amine (NH2), and thiol (SH),41,42 and therefore functionalized or modified CNTs can lead to increase adsorption efficiency.44
Besides, it is interesting to mention that various CNTs integrated with the other types of treatment systems have been investigated recently. Briefly, some of these integration processes include pre-coagulation by adsorptive filtration of pharmaceutical and personal care products using carbon nanotube membranes,45 degradation of the common aqueous antibiotic tetracycline using a CNT electrochemical filter,46 bacterial filtration using CNT/antibiotic buckypaper membranes,47 covalent binding of SWCNTs to polyamide membranes for antimicrobial surface properties,48 improvement of antifouling and antimicrobial abilities on silver-CNT-based membranes under electrochemical assistance,49 study on the mechanism of tetracycline removal in electrocoagulation coupled with electro-Fenton reaction system with Fe anode and carbon nanotube cathode,50 Al-doped carbon nanotubes with adsorption and coagulation,51 and integration of coagulation/flocculation and sedimentation with carbon nanotubes ‘sandwiched’ in a granular filter bed.52 To date, several studies have been conducted on the modification of CNTs in various ways for the removal of antibiotics from aqueous solution. Balarak et al. (2016)163 applied MWCNTs modified with alumina (Al2O3) for the removal of tetracycline from aqueous solution in batch systems. Their results showed that the modified CNTs have potential to remove the tetracycline antibiotic.53 Shao et al. (2010)73 showed that the adsorption capacity of CNTs could be increased by modifying their surface with KOH, which improved the removal of sulfamethoxazole, tetracycline, and aromatic compounds compared to graphene sheets.44 Modification of the surface of CNTs by physical adsorption or chemical bonding, in addition to increasing the removal efficiency, can enable their use as a suitable adsorbent for water treatment.54
To the best of our knowledge, there is no comprehensive review on this hot topic; hence, this review aims to fill the knowledge gap for the first time on the efficiency of modified CNTs for the removal of various common antibiotics as major environmental pollutants. It is hoped that this review will provide a useful approach for the development and wide application of CNTs and their modifications for environmental engineering in the future on a large scale. Herein, the different sections are organized as follows:
1) Reviewing the structure of the common antibiotics.
2) Describing the health and environmental effects of antibiotics.
3) Summarizing the conventional methods (advantages and disadvantages) for the removal of antibiotics.
4) Investigating the methods for the synthesis and preparation of pristine CNTs and their modification process.
5) Focusing on the removal of various antibiotics using CNTs.
6) Identifying the effect of experimental conditions (dosage, initial concentration, contact time, and pH) on the system.
7) Describing the adsorption isotherm and kinetic models.
8) Characterization of the physical and chemical properties of CNTs.
9) Finally, suggesting the major points for the future use of CNTs and their derivatives for large-scale application in water and wastewater treatment.
2 Structure and classification of antibiotics
Antibiotics are chemical compounds that are completely or partially synthesized by living microorganisms, which inhibit or kill the growth of other microorganisms.15 In particular, they prevent or treat infections caused by bacteria or fungi, which lead to diseases in humans and animals, with different action mechanisms such as inhibition of nucleic acid and protein synthesis, cell membrane replacement, and suppression of the cell wall.55 They are divided into various subgroups including β-lactams, quinolones, tetracyclines (TCs), macrolides, sulfonamides (SAs),3 aminoglycosides7 and glycopeptides8 (Table 1). Among the aforementioned classes, the β-lactam group (specifically amoxicillin (AMX)) is the most widely used (more than 65%) antibiotics in the world,56 where β-lactam antibiotics, including the sub-groups of penicillins, cephalosporins, and carbapenems, are the most widely used antibiotics by humans. They are a group of antibiotics that have a typical four-membered β-lactam ring. However, the presence of this ring in their structure causes unstable thermal property. Also, these antibiotics are easily affected by light, extreme pH, metal ions, reducing and oxidizing agents, and solvents such as water and methanol.3,55 Besides, the presence of β-lactam residues in aqueous solution leads to various issues such as antibiotic resistance and public health and environmental problems even at very low concentrations.57
Table 1 The main classes and properties of common antibiotics with their applications
Class |
Applications |
Compound |
Molecular formula |
Chemical structure |
Molecular weight (g mol−1) |
Ref. |
Tetracycline |
Acne and rosacea, infections of respiratory tract, urinary tract and intestinal tract, and treatment of chlamydia |
Chlortetracycline |
C22H24Cl2N2O8 |
|
515.3 |
59 and 62 |
Oxytetracycline |
C22H25Cl N2O9 |
|
496.9 |
β-Lactams |
Severe sepsis and septic shock, genitourinary tract infections, infections of ear, nose, and throat, lower respiratory tract infections, Helicobacter pylori infections, pharyngitis, and tonsillitis |
Amoxicillin |
C16H19N3O5S |
|
365.4 |
63–66
|
Piperacillin |
C23H27N5O7S |
|
517.6 |
Aminoglycoside |
Treatment and prevention of severe enterococcal Gram-negative bacterial infections, and mycobacterial infections, which is the principal drug in the treatment of tuberculosis |
Gentamicin |
C21H43N5O7 |
|
477.596 |
65 and 67 |
|
Rifamycin |
C43H58N4O12 |
|
822.94 |
Macrolides |
To treat upper and lower respiratory infection caused by Gram-positive bacteria, feed additives in livestock and poultry farming to promote animals' growth |
Erythromycin |
C37H67O13N |
|
733.9 |
68–70
|
Pikromycin |
C28H47O8N |
|
525.32 |
Glycopeptide |
The treatment of aerobic Gram-positive infections, the treatment of methicillin-resistant Staphylococcus aureus, and the treatment of infections in patients intolerant of β-lactam antibiotics |
Vancomycin |
C66H75Cl2N9O24 |
|
1449.25 |
71 and 72 |
Sulfonamides |
The treatment of urinary tract infections, sinusitis, and toxoplasmosis, and to promote livestock growth |
Sulfamethoxazole |
C10H11N3O3S |
|
253.279 |
73
|
Quinolones |
The treatment of pneumonia, urinary tract infections, sexually transmitted diseases (gonorrhea and chancroid), skin, bone, and joint infections, gastrointestinal infections, prostatitis, anthrax, typhoid fever, plague, and salmonellosis |
Ciprofloxacin |
C17H18FN3O3 |
|
331.346 |
74 and 75 |
The tetracycline (TC) group is another commonly used antibiotic, which has been widely applied in the livestock industry and treatment of humans, but its metabolism is difficult and approximately 72% of TC can be excreted by humans and animals in its non-metabolized form.53 The TC group includes oxytetracycline hydrochloride (OTC), tetracycline hydrochloride (TCN) and chlortetracycline hydrochloride (CTC), which are obtained from a common hydro-naphthalene core with four fused rings to prevent infection.55,58 This type of antibiotics has unique properties such as high polarity and solubility in water, insoluble nature in saturated hydrocarbons, and degradation in bases, acids, and polar organic solvents especially alcohols.55
Quinolones, especially fluoroquinolones (FQs) are commonly used in human and veterinary medicine with high antimicrobial activity because of their two-ring nucleus structure, which is related to 4-quinolone.55,59 FQs as one of the largest groups of antibiotics, have strong chemical stability, and thus they are not fully metabolized in the body.58 It is estimated that due to this, more than 80% of FQs in their original form or as metabolites is eventually released into the soil and water, and consequently may cause resistance in pathogens or toxicity.60 FQs are soluble in lipids, with high polarity and poor water solubility at pH 6–8 and can be destroyed by UV light but are resistant to acid and high temperature.55 Another type of antibiotics is sulfonamides, which consist of a p-amino-benzene sulfonamide functional group in which the acidic sulfonamide group (–SO2NH–) is attached to a basic amine group (–NH2) and can be classified as polar molecules with high water solubility, low volatility and mobility properties, making them easily transported and dispersed in water.55,61
3 The fate of antibiotics in the environment
In recent decades, the rapid development of the pharmaceutical industry has led to the production of a high level of contamination especially antibiotics, causing severe environmental concerns. Because of their persistence and off-site migration,26 antibiotics have become severe pollutants of various environmental compartments such as surface and groundwater, municipal sewage, soil, vegetables, sludge sewage effluent, ground water, and even drinking water (to the level of g L−1) even at low concentrations,12,53 resulting in negative ecological effects and antibiotic resistance.76 Antibiotics enter the environment in two main ways (Fig. 1), as follows: 1) they are discharged into the sewage after human use (studies in Germany indicated that three-quarters of the volume of used drugs are disposed through sewage) and 2) they are used as growth stimulants or for veterinary purposes, which are excreted by animals, and eventually converted into fertilizer.3
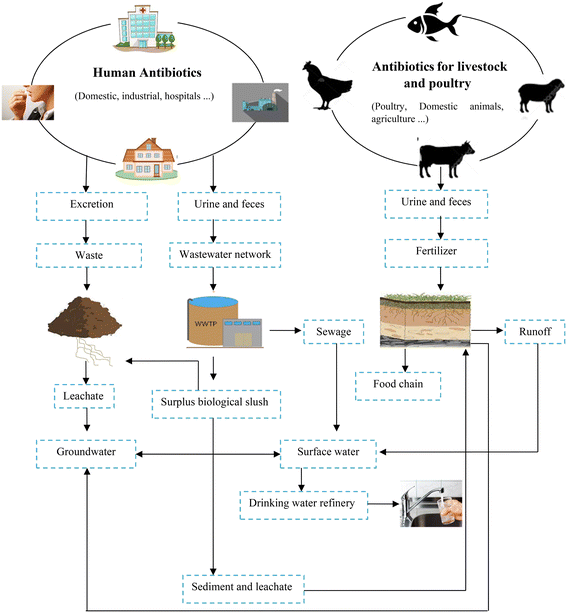 |
| Fig. 1 Main sources of antibiotics contamination in the environment.83 | |
Based on the structure of different antibiotics, their extend in animal farming causes them to be partially absorbed in the animal gut and poorly metabolized, and consequently antibiotic compounds are transferred to liquid manure (as soil fertilization) and feces, eventually leading to their presence in soil samples.77 Consequently, the presence of residual antibiotics in agricultural soils has significant negative effects on the farming process such as delaying germination or reducing the content of biomass, eventually having a negative effect on the yield in fertilized farmland.78 Therefore, the residual antibiotics in environmental compartments have different transportation pathways, for instance, they can be present in different concentrations in agricultural soil due to their leaching, sorption, and degradation.79 Tetracyclines have the soil adsorption coefficient (Kd) of 400 to 1147 (1 kg−1) in clay loam, where the highest adsorption ability in clay loam is related to chlortetracycline with a Kd in the range of 1280 to 2386 (1 kg−1), leading to its longer degradation time in manure or soil (9 to 180 days).79 As stated earlier, antibiotics react with soil minerals to various degrees, where it has been proven that OTC is stable in alkaline clays, whereas chlortetracycline is unstable.80 Moreover, several parameters affect the adsorption of antibiotics including cation exchange capacity (CEC), clay content, organic carbon (OC), and soil pH.81
Although the treatment of wastewaters reduces the concentration of antibiotics, it does not completely eliminate them, and consequently the residual concentration of antibiotics in the discharged effluent of WWTPs enters the receiving surface water.82 Generally, each type of antibiotics has a different fate in the environment. For example, among the groups of antibiotics, sulfonamide, fluoroquinolone, and macrolide antibiotics exhibit the highest half-life, while aminoglycoside antibiotics and β-lactam have the lowest.13 Furthermore, the rate of adsorption of antibiotics in soil, sediment, and/or sewage sludge varies, which can be classified into different groups. For instance, TCs and FQs are easily adsorbed, macrolides are moderately adsorbed, sulfonamides are moderately to poorly adsorbed, and aminoglycosides and β-lactams are poorly adsorbed in the aforementioned environments.4
4 The effects of antibiotics on human health and the environment
Recently, the environmental pollution by antibiotics compounds due to their overuse has become a significant concern because of their properties including low absorption after consumption and high solubility in water.84 The presence of antibiotics and their byproducts in natural ecosystems poses a serious risk to human health and the environment even at low concentrations.6,85 As stated previously, high exposure to antibiotics can cause allergic reactions in people with hypersensitivity, which is one of the adverse drug reactions. The main symptoms of allergies include angioedema, urticaria, aplastic anemia, gastrointestinal reactions, severe shock, and even death if serious.86,87 However, assessing the behavior of antibiotics in the environment is usually difficult due to their complex chemical structures. The release of antibiotics in the aquatic environment can disrupt the aquatic ecosystem, create antibiotic resistance, and make water reuse impossible. In fact, they may reach drinking water and even affect non-target and target organisms in the environment.3 For example, high concentrations of antibiotics in the aquatic environment can cause disturbances in the fish population, body deformities, DNA damage, microbiota dysfunction, suppressed immunity, and the extinction of endangered species.84 Also, the excretion of some antibiotics through urine and animal feces, such as fluoroquinolones, has toxic effects on microbial activity, and thus often exhibit poor biodegradability in biological treatment systems.26 Some antibiotics such as TCs can cause liver damage, gastrointestinal disorders, and yellowing of the teeth, even at low concentrations.1
Besides, antibiotic resistance is becoming another significant threat to human health (e.g., can cause prolonged morbidity and mortality) and the environment.88,89 Based on statistical data, it has been reported that antibiotic resistance will result in 10 million deaths by 2050, with a financial burden of approximately $100 trillion.89 As an example, a 50% detection frequency has been observed for Klebsiella pneumoniae, indicating its resistance to third-generation carbapenems and cephalosporins, in Southeast Asia.88
5 Removal of antibiotics from environmental waters
Conventional wastewater treatment systems cannot properly treat wastewater containing antibiotics, and thus antibiotics eventually leach into receiving water such as surface water resources.90 Therefore, it is essential to apply new, effective, and environmentally friendly approaches to remove antibiotics. To date, several water and wastewater treatment technologies including reverse osmosis (RO),91 coagulation–flotation,92 advanced oxidation processes (AOPs),90,93 ozonation (O3),94,95 coagulation–flocculation,96 and adsorption97 as chemical processes and moving bed biofilm reactor (MBBR),98 microbial fuel cell (MFC),99 constructed wetlands (CW),100 and conventional activated sludge (CAS)101 as the most prevalent biological treatment processes have been developed. Besides, physical processes such as membrane filtration have also been widely used for the reduction or purification of antibiotic compounds.61,102 However, although some of them have attracted attention because of their effectiveness, their applications are restricted due to some issues, as will be discussed below.
Advanced oxidation processes (AOPs) as a one of the chemical and emerging technologies for the removal of antibiotics can be broadly divided into various groups such as photochemical (photo-Fenton, sonochemical, UV/H2O2, UV/O3, photocatalysis, sono-photocatalysis, and sonocatalysis) and non-photochemical (chlorination, ozonation, Fenton, and electrochemical), which have attracted interest as promising and reliable treatment methods for the removal of various antibiotics.24,103 Specifically, these processes are highly effective for the mineralization and degradation of organic pollutants and their conversion into harmless compounds,90,93 and some of them are summarized as follows. Fenton reactions as a type of AOP process is based on hydroxyl radicals (˙OH), which react with organics and destroy them. In this process, hydroxyl radicals are formed by the catalytic decomposition of hydrogen peroxide (H2O2) upon reaction with iron ions (Fe2+).104 The Fenton reaction is an economical and environmentally friendly method for the treatment of water and wastewater with remarkable advantages such as highly oxidative and non-selective nature,72 but this process requires acidic conditions (pH range between 2–4) and produces a large amount of iron sludge.105 Ozone oxidation is a complex process, which takes place through direct reaction with soluble ozone (O3) or indirect oxidation through the formation of radicals. Both methods depend on factors such as the nature of the pollutant, the pH of the medium and the dose of ozone. For example, the production of hydroxyl radicals can be increased by increasing the pH or by combining ozone with hydrogen peroxide or UV-irradiation.94,95 O3 can be produced in situ, requiring the storage of H2O2 solution, iron salt or other chemicals. However, the main problem associated with this method is its high energy consumption and the relatively high cost of equipment and maintenance.94 Furthermore, during AOP processes, some harmful metabolites may be generated, which must be removed. Therefore, the main goal of treatment processes should be the production of non-harmful products, not just the removal of the main compounds.53,106
Another promising method for removing antibiotics is membrane filtration via reverse osmosis (RO), nanofiltration (NF), ultrafiltration (UF), and microfiltration (MF). However, little information is available on the rejection of antibiotics by RO and NF membranes, especially in wastewater treatment systems, where different species may interact on the membrane surface and in solution.61 Also, membranes require expensive equipment and significant energy.68
Among these processes, the adsorption process is the most recommended process for water and wastewater treatment, which is very effective and usually less expensive than other removal methods.71,107 Among the known adsorbents, activated carbon (AC) has been widely used in various fields due to its excellent properties such as high adsorption capacity.108 However, despite the widespread use of AC in the adsorption process, it has some limitation such as difficult production and regeneration, and hence researchers need to find new adsorbents with high adsorption capacity.109 Among the different types of carbon materials, CNTs (from the carbon family) have been widely used in water and wastewater treatment, nanotechnology, electronics, optics and other fields of materials sciences. Besides, the adsorption capacity of CNTs, as a special adsorbent for organic pollutants, can be enhanced by coating or modifying them. This modification can increase the reaction between organic pollutants and CNTs, thus increasing the adsorption rate on the surface of CNTs.110,111 However, despite the remarkable merits of all the aforementioned methods, they suffer from some limitations. In Table 2, we summarize the advantages and disadvantages of the different methods.
Table 2 Advantages and disadvantages of common antibiotics removal technologies
Methods |
Advantages |
Disadvantages |
Ref. |
Fenton reaction |
Wide application range, strong anti-interference ability, simple operation, rapid degradation and mineralization |
Iron sludge production, and limited pH range |
112 and 113 |
Heterogeneous Fenton reaction |
Low iron ion leaching, efficient cycling of Fe3+ and Fe2+, low iron sludge production, wide working pH range, reusability and long-term stability of the catalysts |
Relatively harsh synthetic conditions, complicated synthesis routes, high costs for the synthesis of heterogeneous Fenton catalysts and reactor design |
114 and 115 |
Ozone |
Elimination of many organic compounds, volume of effluent remains constant throughout the process, no sludge formation, simple installation, and usability in flow fluctuations or compounds |
Low stability of ozone in the reaction medium, low solubility, low stability in water, and high costs |
94, 95 and 116 |
Membrane filtration |
The removal of a multitude of emerging contaminants, continuous operation, no formation of by-products or metabolites, the high adaptability to conventional water treatments |
Reduction of removal efficiencies due to adsorption capacity saturation, the high cost, problem of water loss, fouling, and high energy consumption |
56, 61 and 102 |
Adsorption |
Low operational and maintenance costs, great efficiency, no production of relevant by-products |
Difficult production, difficult reconstruction of some adsorbents |
102 and 109 |
CF
|
Low energy consumption, relatively simple design and easy operation |
Sludge production |
117
|
RO |
High removal rate, effective, easy to use, easy operation, high purity of water quality |
High cost, high energy, relatively high pressure, electrical necessity, repeated erosion |
118 and 119 |
Electrochemical |
Effective, versatile, cost-effective, easy and clean technology, suitable for the treatment of toxic wastewater that contains high-concentration antibiotics |
Low flow rates, and high operating costs |
120
|
MFC |
High antibiotic removal, it generates electricity, produce less sludge than conventional treatment process, can reduce the costs and challenges associated with sludge treatment and disposal, promising method for renewable energy production, simultaneous removal of nitrogen components and carbon |
Operation problems such as short life span, high cost, low production rates, limited efficiencies, membrane fouling, instability, and inconvenience in maintaining microbe-based systems, many factors affect the performance of MFCs, including the chemical substrate, ionic concentration, proton exchange material, catalyst, internal resistance, electrode spacing, and electrode material |
121–125
|
Constructed wetlands |
Low cost and nature-based treatment technologies, can be used for the removal of organic matter and nutrients (nitrogen and phosphorus) as well as pharmaceuticals |
Requirement of large land area, the unpleasant odor, requires a shallow basin for the collection of effluent, a water control structure to sustain a shallow water depth, and a water surface above the substrate |
126
|
6 Methods for the synthesis of carbon nanotubes
CNTs are carbon allotropes composed of sp2-hybridized carbons such as graphene, fullerene and graphite. Carbon nanotubes were discovered by Iijima (1991), which based on their applications can be classified as single-walled carbon nanotubes (SWCNT), double-walled carbon nanotubes (DWCNT), and multi-walled carbon nanotubes (MWCNT).127 The typical diameter of SWCNT and MWCNT is 0.8–2 nm and 10–40 nm, respectively. These graphite structures are formed after carbon combustion and placement processes in the form of a tube.66,67
CNTs as highly efficient carbonaceous materials can be produced through various routes including arc discharge (Fig. 2), laser ablation, chemical vapor deposition (CVD), and flame treatment for their industrial development.65 Among them, the synthesis of CNTs via CVD (Fig. 3) of the preferred carbon precursor on an appropriate catalyst surface has been successfully examined.128 It should be noted that one of the critical parameters that can impact the growth of CNTs is the selection an appropriate catalyst because the composition and morphology of the catalyst nanoparticles influence the formation of CNTs.129 Iron (Fe), cobalt (Co), nickel (Ni), and yttrium (Y), either alone or incorporated on suitable supporting materials (alumina, zeolite, silica, and other metal oxides) have been shown to be suitable for the growth of CNTs.130 It is interested to note that in the process for the synthesis of SWCNTs, various catalysts as precursors can be employed including Gd, Co, Ni, Fe, Ag, Pt, and Pd, or mixtures of the aforementioned precursors such as Co, Ni, and Fe with different components including Co–Pt, Co–Ru, Ni–Y, Fe–Ni, Co–Ni, Co–Cu, Ni–Cu, Fe–No, Ni–Ti, and Ni–Y, whereas for the synthesis of MWNTs, it is not necessary to apply catalyst precursors.131 Moreover, the use of other CVD techniques has been reported, such as radiofrequency CVD (RF-CVD), microwave plasma (MPECVD) water-assisted CVD, oxygen-assisted CVD, and hot-filament CVD (HFCVD).129 The attractive use of the CVD method is due to its more controllable and cost-efficient process than other methods (arc-discharge and laser ablation), which allows the tailored control of the CNT architecture for application in highly advanced electronics and optoelectronics, while being easily scalable.128,132 In the second type of CNT synthesis (laser ablation technique, Fig. 4), a laser is used to vaporize graphite inside a quartz reactor, which was used for the first time in 1996 by Thess et al.133 Another method for the preparation of CNTs is using a flame to simultaneously provide chemical species and energy, where various fuels such as acetylene (C2H2), ethylene (C2H4), and methane (CH4) can be used with an oxidizer to generate a mixture of gases such as carbon dioxide (CO2), water vapor (H2O), carbon monoxide (CO), and hydrogen (H2) as well as saturated and unsaturated hydrocarbons (C2H2, C2H4, and C2H6).134Table 3 lists the advantages, disadvantages, and operating conditions of each method.
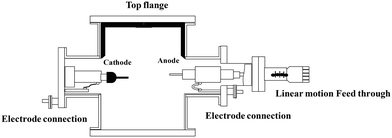 |
| Fig. 2 Schematic representation of the typical arc-discharge apparatus. Reproduced from135 with permission from AIP Publishing. | |
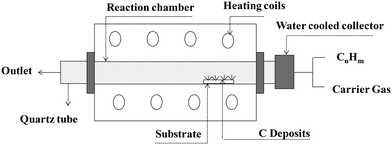 |
| Fig. 3 Schematic representation of CVD apparatus. Reproduced from134 with permission from IntechOpen, the world's leading publisher of open access books built by scientists, for scientists. | |
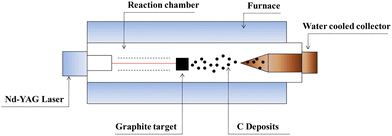 |
| Fig. 4 Laser ablation apparatus for the production of CNTs. Reproduced from134 with permission from IntechOpen, the world's leading publisher of open access books built by scientists, for scientists. | |
Table 3 Comparison of the operational conditions, advantages, and disadvantages of the most common CNT synthesis methods
Method |
Operational conditions |
Advantages |
Disadvantages |
Ref. |
CVD |
Decomposition of hydrocarbon (on cobalt, methane and ethylene) with metal catalysts at 300 °C to 1200 °C |
Large-scale usage, producing high-quality MWCNTs, relatively low cost, low power input, lower temperature range, control of CNT structural characteristics, and production of nanotubes with a controlled diameter, length and number of layers |
High density of defects in CNT structure and generation of by-products, graphitic debris, catalyst particles and fullerenes |
8, 59, 62, 65 and 136 |
Arc discharge |
Direct current passing through a gaseous atmosphere (inert gas) between two electrodes (at least one electrode is made of graphite) and uses high temperature (>3000 °C) for evaporating carbon atoms into plasma |
Production of nanotubes with low defects and high quality, with CNT yield exceeding 50% |
Existence of by-products, graphitic debris, catalyst particles and fullerenes and the reaction product always contains a mixture of different types of CNTs |
8, 59, 62 and 65 |
Laser ablation |
Use of intense laser beams for the ablation/vaporization of a target consisting of a mixture of graphite and metal catalysts in an inert gas stream and involves the vaporization of graphite in an electrical furnace heated at 1200 °C |
Production of nanotubes with low defects and high quality, environmentally benign method, producing large volumes of uniform, high-yield single-wall nanotubes SWNTs with minimal post processing, no catalyst needed, and fair control of size and morphology |
Existence of by-products and graphitic debris, small yield of ablated nanoparticles, and large particle aggregations |
8, 59, 62–64 and 137 |
7 Modified CNTs
CNTs are known as superior adsorbents because they have a unique one-dimensional tubular structure, high surface area, and tendency to create strong π–π electrostatic interactions.7 They also have various advantages such as flexibility, high strength, light weight, and high conductivity due to the arrangement of the graphite sheets, including carbon atoms in a hexagon template, which are wound and overlapped.66 However, the hydrophobicity and chemically neutral nature of the outer wall of the raw nanotubes are the main disadvantages of CNTs, which hinder their wide application.138 Accordingly, on strategy to address this problem is to coat the carbon nanotubes. The coating of CNTs extends their application to various fields and improves their properties.139 The modification of CNTs can be roughly classified into two categories including covalent and non-covalent methods.138 Between them, the non-covalent modification of CNTs is highly recommended because the structures and properties of the nanotubes can be preserved after the modification reactions.140 One of the sub-methods of non-covalent modification is the bonding of polymers, which occurs through the weak interactions between the polymer and the sp2 hybrid network of CNTs. This method is also recommended by researchers because it can preserve the sp2 hybridization of carbon atoms, preserving their mechanical and electronic properties, and consequently can has effect on their covalent performance.141 However, despite the significant merits of non-covalent modification, one of its major disadvantages is related to the weak forces between the nanotube and the wrapping molecule, resulting in a low charge transfer efficiency.142
Besides, various functional groups can be incorporated on the surface of CNTs according to their application and the chemical nature of the target contaminants, where non-polar organic contaminants have high affinity to CNTs due to their interactions with the aromatic rings of the CNTs.143
Covalent coating involves the use of a combination of modifying molecules and CNTs, or in other words, functional groups such as carboxyl attached by covalent bonds to the outer side walls and ends of CNTs.127 In this method, by changing the carbon atoms from sp2 to sp3, the translation symmetry of CNTs is disturbed, and consequently their properties such as electronics and transport properties are affected.142 The covalent coating methods include oxidation, cycloaddition, electrophilic addition, fluorination, nucleophilic addition, and radical addition.127
Coating CNTs with polymer molecules (polymer bonding) is of particular importance because it causes CNTs to dissolve in a wide range of solvents, even at low levels of functionality, depending on the nature of the bonded polymer, and increases the affinity of CNT-g-polymer in polymer matrices.141,144,145 There are two methods for the covalent bonding of polymers to CNTs, i.e., “bonding to” and “bonding from”. Polymer brushes can be made by “bonding to” via covalent tethering of preformed or end-coated polymer chains to a coated surface, and “bonding from” via polymerization on a substrate previously modified with originators to provide polymerizable sites.146 However, a problem encountered with covalent coating is that during functionalization, many defects are created on the wall of the carbon nanotubes and the nanotubes split into smaller portions. These defects result in the degradation of the mechanical properties and disruption of the π electrons of carbon nanotubes.147
7.1 Chemical coating
Chemical coating has attracted much attention as an important method for modifying the physical and chemical properties of CNTs.44 This method is based on covalent bonding, which involves the combination of hydrophilic groups in the outer CNT side walls.148 In fact, the goal of chemical methods is to create a surface coating on CNTs, which improves their chemical reaction with a solvent or polymer as well as further dispersion.149
There are various methods for the chemical coating of CNTs, including sidewall fluorination, chemical oxidation, defect coating, cycloaddition, Diels–Alder reaction, chlorination, addition of carbene and nitrene, use of azomethine ylides, bromination and hydrogenation.2,138 Fluorination is the starting point in many processes for the modification of SWCNTs, making fluorite tubes (F-SWCNTs) one of the basic precursors in the broad field of CNT chemistry.150 Some properties of the F2 molecule, such as high reactivity, oxidation resistance, and maximum electronegativity, result in the formation of very strong bonds between fluorine and many elements in the periodic table. Therefore, fluorine, fluorinated gases and fluoridated plasma are very useful for modifying the surface properties of materials.151
Chemical oxidation is another method for the covalent coating of CNTs. In this method, the ends and walls of the nanotubes are covered with oxygen-containing groups such as ether groups and carboxyl groups.152 Wet chemical methods, photo-oxidation, oxygen plasma and gas phase treatment are some of the chemical oxidation methods that have received significant attention due to the increased chemical reaction of the graphic network.138 Oxidation processes are divided into two stages of defect production and defect consumption. In the production of defects, oxidants attack the graphene structure with electrophilic reactions, producing active sites such as hydroxyl (–OH) and carbonyl (–C
O). In the defect consumption stage, the graphene structure of the tube is destroyed due to the oxidation of the active sites produced in the previous stage (defect production). This step depends on the ability of the oxidant to degrade the graphite structure around –C
O and the previously formed surrounding groups.152
Defect coating is also one of the methods of chemical coating. The defects produced by oxidants in CNTs are stabilized by binding to –OH or carboxylic acid (–COOH) groups. Because these functional groups have rich chemistry, the CNTs coated with them can be used as precursors for subsequent chemical reactions such as esterification, silanization, polymer bonding, arylation, alkylation, and even with some biological molecules.2 Defects can be heptagons in the hexagonal graphene frame, open ends or side wall holes, and oxygenated sites.147
Chemical coatings are divided into two categories, as follows: (1) aggressive method, especially oxidation process, which causes structural defects due to the use of concentrated acids and disturbs the intrinsic properties of CNTs. (2) Although some milder coating processes, such as plasma and UV/ozone treatment, have been developed, followed by silane, amine or fluorine treatment, the CNT-surface active sites are limited (mostly at the end caps and defects), causing low efficiency, and thus altering the dispersability of the CNTs in the polymer to a certain extent.149 This type of method causes the coated CNTs to dissolve in many organic solvents because the hydrophobic nature of the CNTs changes to hydrophilic (due to the bonding of polar groups). Furthermore, these methods are often time-consuming and involve several steps. Therefore, it is necessary to develop simple and cost-effective chemical methods in terms of basic and practical research.2
7.2 Physical coating
The non-covalent coating (physical) method is based on weak interactions such as π–π stacking interactions, van der Waals forces, and hydrophobic interactions between CNTs and modifying molecules (organic molecules).127 Non-covalent treatments, such as the use of polymer coatings and surfactants, followed by surfactant treatment, rarely damage the structure of CNTs because they are based on physical adsorption, i.e., they do not disrupt the intrinsic π-bonds of CNTs and do not interfere with their electrical properties.149 They change the properties of CNTs by adsorption on their surface.127
The suspension of CNTs in the presence of polymers (such as polystyrene and polyphenylene vinylene) results in the wrapping of the polymer around the CNTs and the formation of supramolecular complexes.147 The polymer wrapping method preserves the intrinsic sp2 composition and electronic structure of CNTs.153 Natural polymers, including DNA, proteins, and some polysaccharides, have been used for the modification of SWCNTs, but their use is limited due to their high cost and limited availability.154 One of the important problems related to polymer/CNT systems is the relative incompatibility between CNTs and polymer matrices, which causes problems in the homogeneous dispersion, phase separation and accumulation of CNTs at high loadings.155
In addition to polymers, surfactants have also been used for the coating of CNTs. Surfactants can be divided into three categories, i.e., non-ionic, anionic and cationic surfactants.156 Surfactants can overcome the problem of CNT instability in polar solvents such as water by creating non-covalent bonds.157 Also, the physical adsorption of surfactants on the surface of CNTs overcomes the van der Waals absorption (using electrostatic/steric repulsion forces), reducing the surface tension and preventing the formation of aggregates.149 However, surfactants also exhibit disadvantages. For example, some surfactant bonds may break at high temperatures, reducing the efficiency of the suspension.157
Another non-covalent method for coating CNTs is the endohedral method. In this method, the nanotubes are opened initially, and then filled with special materials. The simplest method is to use nitric acid, which is very selective in attacking the carbon pentagon at the end of the tube.158 Metal nanoparticles, salts and organic compounds (dyes such as β-carotene and phthalocyanines, metallocenes, coronene and carbon nanostructures such as graphene and C60 derivatives) are among the materials that have been successfully trapped in CNTs through various pathways.159 Recently, successful methods have been discovered to fill open multi-walled CNTs with fullerenes and other organic molecules, enabling endohedral inclusion in a soft low-temperature manner.158
8 Antibiotic removal using coated CNTs
Table 4 shows the coated CNTs in various forms of SWCNTs and MWCNTs used to remove antibiotics. Despite the unique properties of CNTs, the high removal efficiency of antibiotics by coated CNTs compared to other adsorbents has caused researchers to use CNTs in the coated form to achieve the optimal removal of contaminants. Although the coating process is complex, in some cases, the use of these complex processes to eliminate some antibiotics and reduce their risks may be necessary, and thus can be justified from an environmental and health perspective.160
Table 4 Summary of the results of literature reviews for the removal of antibiotics using coated CNTs
Antibiotics |
Coated-CNTs |
Results |
Ref. |
SMZ |
Fe3O4/MWCNTs |
✓ Adsorption of organic pollutants and ozone in Fe3O4/MWCNTs is an effective step for the degradation of SMZ |
75
|
✓ Acidic conditions are more suitable for the degradation and mineralization of SMZ |
✓ Catalytic ozonation of SMZ by Fe3O4/MWCNTs is influenced by its adsorption, ozone decomposition rate, and Fe3O4 state |
Cu–TiO2@SWCNT |
✓ SMZ can be completely destroyed with Cu content of 4%, SWCNT content of 10%, pH of 7, catalyst loading of 0.9 g L−1, and initial sulfamethazine concentration of 30 mg L−1 after 135 min solar irradiation |
70
|
TC |
MWCNT/TiO2 |
✓ Completely removal of TC was observed at a TC concentration of 10 mg L−1, pH 5, photocatalyst dose of 0.2 g L−1, and irradiation time of 100 minutes |
161
|
✓ Real drug wastewater was efficiently treated by UVC/MWCNT–TiO2 photocatalytic process |
Graphitized-MWCNTs, OH–MWCNTs, COOH–MWCNTs, and NH2–MWCNTs |
✓ Adsorption dependence followed the order of G–MWCNTs > OH–MWCNTs > COOH–MWCNTs > NH2–MWCNTs, showing that the adsorbent interactions between MWCNT and TC could be influenced by the surface properties of MWCNTs |
162
|
Magnetic CNTs/C@Fe/chitosan |
✓ CNTs/C@Fe/CS had good adsorption capacity for TC (104 mg g−1) |
111
|
✓ The reusability of the adsorbent was excellent, where after 10 times use the sorption capacity was still 99.3% |
Al2O3–MWCNT |
✓ Langmuir adsorption isotherms best matched the experimental data (maximum adsorption capacity: 855.6 mg g−1) |
163
|
✓ The adsorption kinetics followed the pseudo-second-order kinetics |
MWCNT/MIL-53(Fe) |
✓ Maximum adsorption capacity of TCN, CTC and OTC on MWCNT/MIL-53(Fe) was 364.37, 180.68, 325.59 mg g−1 at 25 °C, which was 1.25, 3.34, and 8.28 times than that of a single MWCNT, respectively (pH = 7.0) |
74
|
✓ Excellent reusability and great water stability indicated the potential use of MWCNT/MIL-53 (Fe) for the removal of TC antibiotics from aqueous solutions |
CIP |
CNTs/FeS |
✓ The Fenton-like catalyst CNTs/FeS has good catalytic activity to remove antibiotics (due to the synergistic effect of adsorption- advanced oxidation) |
72
|
✓ The CNTs/FeS catalyst was recoverable with a high catalytic capacity (>75%) even after four uses |
AMX |
8-Hydroxyquinoline–MWCNT and COOH–MWCNT |
✓ Maximum AMX removal efficiencies were obtained at pH = 5 for virgin-MWCNT and 8-HQ–MWCNT as well as pH = 3 for COOH–MWCNT |
164
|
✓ The adsorption equilibrium time was 4 hours for virgin-MWCNT and 2 hours for coated CNTs |
✓ The maximum adsorption capacity of 8-HQ–MWCNT was nearly 50% higher than that of virgin-MWCNT |
According to the study by Xiong et al., a new adsorbent composite was synthesized by combining amino-functionalized MIL-53(Fe) with MWCNTs, which was used to adsorb chlortetracycline hydrochloride (CTC) and tetracycline hydrochloride (TCN). The maximum adsorption capacities of CTC and TCN over MWCNT/NH2-MIL-53(Fe) were 254.04 and 368.49 mg g−1, which are 8.37 and 1.79 times higher than that of chaff biochar, respectively. Upon the combination of MWCNT and NH2-MIL-53 (Fe), the mesoporosity of MWCNT/NH2-MIL-53 (Fe) increased significantly, which is suitable for the production of active adsorption sites. The hydrogen bonding between the hydroxyl functional groups in TCN or CTC and amino functional groups in MWCNT/NH2-MIL-53 (Fe) caused an increase in adsorption capacity. Also, the π–π interaction between the adsorbate and adsorbent is the main reason for the adsorption of these two antibiotics.10 In another study, the catalytic ozonation of sulfamethazine (SMT) by Fe3O4/MWCNTs was investigated. The results showed that Fe3O4/MWCNT-catalyzed ozonation could increase the degradation and mineralization of SMT compared to ozonation alone.75 Other results regarding the removal of antibiotics by coated CNTs are described in Table 4.
9 Characteristics of modified CNTs
Herein, we summarize the major characteristics of modified CNT adsorbents for the removal of common antibiotics based on various analyses such as BET, SEM or FESEM, FTIR, and XRD. Generally, XRD, as a crystallographic technique, can be used to identify the main details about the nanocrystal structure, size, and particle shape.165 Another important analysis that can be applied for the characterization of CNTs is SEM, where using this technique, the surface morphological properties and cross-sections of nanocomposites containing CNTs can be determined by getting electron pictures of the sample at the nanoscale level.166 Also, BET analysis is very useful for predicting the specific surface area of the synthesized CNTs.20 Furthermore, the major functional groups of the adsorbents before and after the adsorption process can be determined by FTIR analysis.97 The chemical and physical characteristics of different coated-CNTs are presented in Table 5. It can be observed that the surface area of the prepared adsorbents is in the range of 60 to 1228 m2 g−1,74,167 where this high surface area of the synthesized CNTs demonstrate that these adsorbents are preferable and environmentally friendly for the removal of various common antibiotics. According to the XRD results, it can be concluded that structure of the prepared CNTs had crystalline phases, and also the coating agents successfully covered the CNTs.43,167 The results of SEM analysis indicated that coated-CNTs had varying morphological properties depending on the modifying agents, as can be seen in Table 5. Furthermore, should be noted that different functional groups were observed on the surface of CNTs. In brief, the main functional groups can be summarized as follows: 1) different peaks at 3278–3444 cm−1, 1549–1570 cm−1, 1372–1376 cm−1, 3465, 2350, and 1400 cm− 1 associated with the hydroxyl group (O–H) and 2) peaks located at 2856–2922 and 2800–3100 cm−1 due to asymmetric C–H vibrations.34,43,70,167
Table 5 The main characteristics of coated CNTs
Coated-CNTs |
Antibiotics |
BET (m2 g−1) |
SEM |
FTIR |
XRD |
Ref. |
CNTs/β-cyclodextrin/MnFe2O4 |
TC |
166.13 |
Particles with high size and density |
3278–3444 cm−1, 1549–1570 cm−1, and 1372–1376 cm−1: –OH vibrations and 2856–2922 cm−1: asymmetric C–H vibrations. |
Crystalline structure |
43
|
MnFe2O4/MWCNT |
TC |
220.62 |
The surface of the composites was successfully covered with iron oxide nanoparticles, and the new MMWCNTs were about 10–50 nm in size |
3465, 2350, and 1400 cm−1: O–H, 2800–3100 cm−1: C–H, and 1600–1650 cm−1: C C |
Purity and crystallinity of modified CNT structure |
34
|
MWCNT/MIL-53(Fe) |
TC |
60.17 |
Intimate contact between the original MOFs and MWCNT (attached on the surface and in the original MOF structures) |
After incorporation of MWCNT, two peaks of O–H (2360 cm−1) and O–H (stretch from H-bonded-COOH, 3450 cm−1) |
The characteristic diffraction peaks of both MWCNT and MIL-53(Fe), attesting the presence of MWCNT and MIL-53(Fe) |
74
|
MIL-100(Fe)-CNTs |
OTC |
1228 |
Relatively smoother than MIL-100(Fe) |
3460 cm−1: stretching vibration of the O–H group, 1720 cm−1 may be attributed to C O stretching, 1618 cm−1: frame stretching vibration of benzene ring, and vibration at 1378 cm−1 assigned to the COO– group stretch of carbohydrates |
The successful preparation of the material |
167
|
MWCNTs-AC |
CIP |
708.16 |
MWCNTs are attached to the surface of AC sheets, and the agglomeration of MWCNTs is detectable on AC |
2922.39 and 1720.53 cm−1: C–H band and C C stretching of AC, respectively, and 462.60 cm−1: Mn–O band in MnO4 |
— |
168
|
Magnetic-MWCNTs |
CIP |
— |
Iron oxide nanoparticles were effectively coated on the surface of MWCNTs |
580 cm−1: the vibrational properties of Fe–O bond and 3300–3500 cm−1: –OH group |
Good crystallinity of Fe phases |
169
|
Magnetic-graphene/CNT |
OTC |
394 |
Scrolled and hierarchical architecture of thin graphene layers |
1500–1600 cm−1: skeletal vibration of aromatic ring, 580 cm−1: Fe–O vibration, 1710 cm−1: C O, 1300 cm−1: C–O, 3600 cm−1: O–H, and 910 cm−1: epoxy C–O |
— |
170
|
Cu–TiO2@functionalized SWCNT |
SMZ |
138.82 |
Uniform distribution of Cu-doped TiO2 particles on the surface of SWCNTs |
3000 to 3800 cm−1: –OH stretching of surface-adsorbed water molecules and carboxylic bands, 1580 and 1344 cm−1: –OH deformation of –COOH functional groups and adsorbed water molecules, respectively, 1699 to 1651 cm−1: stretching vibrations of carbonyl, carboxyl, and aldehyde functional groups, and 1625 and 3300−3400 cm−1: hydroxyl functional groups and H2O molecules, respectively |
Crystallinity phases |
70
|
10 Adsorption mechanisms of antibiotics on CNTs
Among the separation technologies, adsorption is a method in which the separation of some special components or pollutants from the fluid phase on the surface of a solid phase (adsorbent) can occur.171 The steps predicted for the adsorption process for any adsorbent material are as follows: 1) contaminants are dispersed or accumulated on the external surface of the adsorbent, 2) with sufficient time, the pollutants move deep in the interlayers of the adsorbent by internal diffusion (the transfer of contaminated mass to the interior surfaces), 3) and finally, the process continues until a layer is formed on the surface of the active pores, which is deemed as the end of the adsorption process.172 Furthermore, it should be noted that the adsorption efficiency depends on the major characteristics of the applied adsorbents including porosity (macro or micro porosity), pore diameter, specific surface area (SSA), and functional groups.173 The adsorption of pollutants in CNT materials can be predicted based on the four regions in CNTs, i.e., hollow interiors of nanotubes, which are open ended, between the tube bundles at the interstitial pore spaces, at the groves present at the boundary of the nanotube bundles and the outermost CNT external surface.174,175 Moreover, one of the critical steps in the design of adsorbents based on carbon materials is determining the reaction mechanism of pollutants (with focus on antibiosis) on their surface because it can provide significant information on their adsorptive capacity, regeneration efficiency, and finally their economic viability.176
Based on previous studies, it has been proven that the adsorption of antibiotics on carbon-based materials such as activated carbon and CNTs can be accomplished via various mechanisms such as pore filling, surface complexation, covalent bonding, electrostatic interactions (cation and anion attractions), hydrophobic effects (hydrophobic interaction), π–π electron donor–acceptor interactions (EDA mechanism), hydrogen bonding, and partition into uncarbonized fractions, and other processes (surface precipitation).176–178 As previously mentioned, various adsorption mechanisms can be dominant in the adsorption of organic compounds given that the internal characteristics of adsorbents play a significant role in this process because of their unique properties (heterogeneous surface area due to the co-existence of carbonized and non-carbonized fractions).179 For instance, electrostatic interactions greatly control the adsorption of ionic compounds, which highly depend on the pH, where at pH values lower than pHzpc (zero point of charge), the surface of all CNTs will be positively charged, whereas at pH values higher than pHzpc, the surface if CNTs is negatively charged.177 Besides, because antibiotic compounds differ based on their hydrophobicity (such as polar and nonpolar), the dominant adsorption mechanism of antibiotics vary.179 Previous works have demonstrated that the adsorption of highly hydrophilic antibiotics such as SMX and lincomycine on SWCNTs may be related to various mechanisms including electrostatic interactions, hydrogen bonds, and hydrophobic interactions.180 Also, considering the simultaneous effects of the properties of antibiotics and CNTs, together with the environmental conditions (temperature, solution pH, and matrix) is very helpful to better understand the dominant adsorption mechanism of antibiotics on CNTs.181
11 Effective parameters for the removal of antibiotics
11.1 Effect of pH
pH is an important and fundamental parameter in the adsorption process, which has a significant effect on the physicochemical properties of the adsorbent and adsorbate and determines the effect of the sorption process.74,182 CNTs are often coated with a variety of materials, presenting an array of surface properties such as carboxyl, hydroxyl, and amino groups. Most of these surface properties depend on the pH, where at a relatively low pH, the amino group has a stronger catalytic surface yield than carboxyl and phenolic groups.183 Alternatively, at a solution pH below pHpzc, the functional groups of the surface are deprotonated due to the presence of OH− ions in solution, whereas at a solution pH above pHpzc, the functional groups on the surface of the adsorbent are protonated by additional H+ ions.164
Ma et al. (2015) found that the removal of ciprofloxacin from aqueous solution by FeS/CNTs increased with an increase in pH and remained stable at high pH levels from 3.0 to 7.0. When the pH was <3.0, the adsorption of ciprofloxacin increased with an increase in the pH of the solution, because when the pH of the solution was below 3.0, the surface of FeS/CNTs was positively charged, thus repelling ciprofloxacin (cationic at pH < 6.0), consequently resulting in less adsorption. Also, when the pH of the solution was above 7.0, the surface of FeS/CNTs was negatively charged, and thus ciprofloxacin (zwitterionic at 6.0 < pH < 8.7) was strongly ionized, resulting in less adsorption.72 In the study by Bazregari et al. (2018), maximum amoxicillin removal efficiencies were obtained for virgin-MWCNT and 8-HQ-MWCNT at pH = 5 and for COOH–MWCNT at pH = 2. The decrease in amoxicillin removal by increasing the pH to more than 5 can be due to two reasons, as follows: 1) increased formation of OH– in solution and 2) its competition with COO− anions on amoxicillin molecules to occupy the CNT adsorption sites. Alternatively, in very acidic conditions, the removal of amoxicillin was reduced due to interference from H+. In this study, the pHpzc for virgin-MWCNT, 8-HQ–MWCNT and COOH–MWCNT was 6, 5.2 and 3, respectively, which is greater than the pH and caused a positive charge on their surface. Thus, in acidic solution (pH = 2–6), the COOH group in the amoxicillin molecule is isolated in the form of COO−. Consequently, electrostatic adsorption was predominant between the amoxicillin (anionic) molecules via their COO− functional group and the CNT surface.164 According to Payan et al. (2019), the sorption of sulfamethazine on Cu–TiO2–SWCNT depends on the pH. At pH 7, sulfamethazine was almost completely eliminated. At this pH, the surface of the catalyst had a negative charge and the neutral form of sulfamethazine was detected as the dominant species. However, at pH values above 7.4, the removal efficiency decreased due to the electrostatic driving force occurring between the surface of the catalyst and the main form of sulfamethazine.70
11.2 Effect of initial antibiotic concentration
The effect of initial concentration has been investigated in studies as an important parameter affecting the antibiotic removal efficiency. High concentrations of antibiotics produce many intermediates compounds, which compete with the active radicals. Consequently, the number of active species is significantly reduced, decreasing the rate of antibiotic removal.70
In the study by Balarak et al. (2016), they described the effect of the tetracycline initial concentration on its percentage removal by Al2O3–MWCNTs. The results showed that the removal percentage of tetracycline using Al2O3–MWCNTs decreased with an increase in its initial concentration from 10 to 100 mg L−1. This may be ascribed to the filling of the empty spaces, preventing further adsorption from occurring.163 In another study by Kang et al. (2018), Ni@NCNTs was used as an adsorbent and catalyst to activate persulfate to remove sulfachloropyridazine as an emerging contaminant. According to the results, high concentrations of antibiotics reduced the degradation efficiency. This is because the sulfachloropyridazine molecules and intermediates occupied most of the active sites of Ni@NCNTs and inhibited the activation of persulfate. Also, an insufficient amount of persulfate can be a limiting factor for the degradation of high concentrations of sulfachloropyridazine.93
11.3 Effect of coated CNT dosage
The dosage of coated CNTs is another factor affecting the removal of antibiotics. Increasing the absorbent dosage leads to more active sites and increase in surface area. Consequently, antibiotics are more efficiently adsorbed on the adsorbent surface.70 According to Balarak et al. (2016), increasing the dosage of Al2O3–MWCNTs increased the adsorption percentage of TC, which is due to the increase in adsorption sites and adsorbent surface area. However, a further increase in adsorbent dosage did not result in the complete adsorption of tetracycline because the excessive condensation of the adsorbent particles caused the adsorption sites to overlap.163 In another study conducted by Ahmadi et al. (2017), the effect of different dosages of MWCNT/TiO2 (0.1–0.4 g L−1) on the photocatalytic degradation of tetracycline at a concentration of 5 mg L−1, pH 5 and irradiation time of 120 min was investigated. According to the obtained results, with an increase in the adsorbent dosage, the amount of antibiotic removed increased, and at a dosage of 0.2 g L−1 TC, it was completely removed after 100 min. By increasing the adsorbent dosage to 0.4 g L−1, no improvement in the removal process was achieved. At high dosages, the particles accumulated, which interfered with the homogeneous structure of the suspension and reduced the number of active sites.161 In the study by Payan et al. (2019), the effect of different dosages of Cu–TiO2/SWCNT (0.1–1.2 g L−1) on the photodecomposition of SMZ at pH 7, initial SMZ concentration of 30 mg L−1, copper content of 4%, SWCNT content of 10%, and contact time of 135 min was checked. The results showed that with an increase in the adsorbent dose from 0.1 to 0.6 g L−1 and 0.9 g L−1, the removal rate increased from 51% to 79% and 100%, respectively.70
11.4 Effect of contact time
One of the most important parameters in the adsorption process is the contact time between the adsorbate and adsorbent. According to studies, the amount of adsorption is very high early in the adsorption process, and then it reaches equilibrium. After the equilibrium phase, the adsorption rate decreases. The contact times for different adsorbents vary, which may be due to the differences in their chemical structure, availability of active surface sites, surface area and adsorption binding constants.164,184
Balarak et al. (2016) investigated the effect of contact time for the removal of TC by Al2O3–MWCNTs. Based on the results at Co = 10 to 100 mg L−1 for an adsorbent dosage of 1 g L−1, tetracycline was removed rapidly in the first 30 min due to the large number of empty surface sites for adsorption. However, the adsorption rate gradually decreased and the adsorption reached equilibrium within about 60 min. The repulsive force of the TC molecules on the solid surface and in the bulk phase made it difficult to occupy the empty space of the remaining surface.163 Bazregari et al. (2018) investigated the removal of AMX using coated CNTs, where the time required to reach equilibrium in the adsorption process on the virgin and coated CNTs (8-HQ–MWCNT and COOH–MWCNT) was approximately 240 and 120 min, respectively. The coated CNTs showed an increase in adsorption kinetics due to the presence of functional groups on their surface. Also, the amount of adsorbed antibiotics in the coated CNTs increased, which can be attributed to the high rate of adsorption at the beginning of the process due to the presence of more adsorption sites on the adsorbent.164
12 Reusability of CNTs
In the adsorption process, the reusability or recovery of adsorbents saturated by pollutants is one of the critical parameters that must be considered for the commercial application of the selected adsorbents.10 Generally, three main thermal methods are employed for the regeneration of carbonaceous materials (such as AC and CNT) including pyrolysis, pyrolysis–gasification, and gasification.179,185 For example, previous works were conducted on the regeneration of AC by the pyrolysis and gasification techniques using air or CO2 (200 cm3 min−1) under an N2 flow. It was shown that between these two methods, the best result was obtained by air gasification with the minimum time contact of 3 h.179,185 Besides the thermal regeneration of the adsorbents, it was recently demonstrated that they can be regenerated by wet air oxidation, ultrasonication, chemical treatment, and washing with a solvent such as sodium hydroxide (NaOH) and hydrochloric acid (HCl), and also sodium chloride (NaCl).186 Therefore, in recycling or estimating the reusability of the selected adsorbents, two steps (regenerating the adsorbent and maintain its stable adsorption capacity) are very important, where recycling of CNTs can be better performed at a stable temperature below 400 °C because of their thermal stability.187 Various studies have been conducted on the performance and regeneration of CNTs and modified-CNTs. Xiong et al. (2018) used MWCNT/NH2-MIL-53(Fe), and after it was dried at 100 °C in a vacuum oven, there was no significant decrease in adsorption for up to four recycling cycles.10 Wang et al. (2015) used CNTs/CoFe2O4 as a promising adsorbent for the removal of SMZ. The obtained data from the regeneration process under heating at 300 °C demonstrated that this adsorbent can be reused for five consecutive cycles.187 Sharifpour et al. (2020) performed the recovery of MWCNTs-AC for the removal of CIP using three different washing methods (NaOH, distilled water, and H2SO4), and found that the highest (96.7%) adsorbent recovery was obtained using NaOH followed by distilled water (94.1%), and H2SO4 (86.5%).168
13 Management and disposal of CNTs
As mentioned before, nanotechnology is deemed as remarkable and fascinating technology, which can be widely applied in many fields, but the severe risk related to the use of nanoparticles and their disposal have not been well studied, and thus this topic is increasingly becoming a public concern.188 According to the available data, the production rate of nanoparticles (NPs) increased (from 2000 tons to 58
000 tons) from 2004 to 2020, and thus it is urgent to comprehensively investigate the hazardous effects of various NPs, and consequently applying the appropriate management to prevent the aforementioned effects.189 Various studies have been reported on the synthesis of several types of NPs based on carbonaceous materials, but there is little information on applying feasible and professional approaches for the management of nanowaste streams generated in different phases of nanotechnology-based products and the life cycle of these materials.190 Thus, new guidelines and protocols for the safe handling and disposal of nanowastes must be further developed in the future.190
14 Adsorption modeling: kinetics and isotherms
14.1 Adsorption kinetics
To design an adsorption system, it is necessary to have sufficient knowledge about the equilibrium and kinetics of adsorption. The theoretical complexity of adsorption mechanisms is recognized as a limiting factor in the development of kinetic understanding.191 Kinetics is the basis for determining the efficiency of a fixed bed or any other flow-through system, determining the solute uptake rate (which determines the residence time to complete the adsorption reaction), and knowing the scale of the adsorption device.192 For the kinetic study of the adsorption of antibiotics on coated-CNTs, recently, various kinetic models such as pseudo-first-order (PFO) based on solid capacity, pseudo-second-order (PSO) based on solid-phase adsorption, intra-particle diffusion model (IPD) describing the diffusion mechanism, and Elovich model193 have been applied. Also, the correlation coefficient (R2) is used to measure the agreement between the measured data and the proposed model.111 The intraparticle diffusion equation is used to identify the adsorption mechanism and predict the rate of adsorption kinetic control.163 Among them, PFO and PSO have been widely used for the adsorption of antibiotics using coated-CNTs in previous works. Furthermore, the equations and major application of the aforementioned kinetic models are summarized in Table 6.
Table 6 Kinetic models, equations, and applications
Kinetic model |
Model equations |
Constant parameters |
Application |
Ref. |
PFO |
|
qt is the amount of adsorbed at any time (mg g−1) |
To estimate the initial stage of the adsorption mechanism |
194 and 195 |
|
K
1 is the adsorption rate constant |
PSO |
|
Suggests chemisorption process |
196 and 197 |
K
2 is the adsorption rate constant |
k
i
is the intraparticle diffusion rate constant |
IPD |
|
To detect the pathway involved in the adsorption system |
194 and 198 |
α is the initial sorption rate (mg g−1 min−1) |
Elovich |
|
To examine chemisorption |
193 and 197 |
β is the de-sorption constant (g mg−1) |
Ma et al. (2015) noted that CNT/C@Fe/CS had a good adsorption capacity (qe) for TC (104 mg g−1). The results of their adsorption experiments showed that the adsorption kinetics in this study followed the PSO model (qe = 117.6 mg g−1, K2 = 0.004 min−1, and R2 = 0.99).111 In the study by Bazregari et al. (2018) on the removal of AMX with coated and virgin CNTs, it was observed that the PSO model was the most compatible with the adsorption kinetics data (R2 > 0.97). Also, the results showed that the kinetic constant (k) was greater for the coated CNTs than their virgin form (k2 virgin-MWCNT = 0.0000196 g mg−1 min−1, k2 8-HQ–MWCNT = 0.0000223 g mg−1 min−1 and k2 COOH–MWCNT = 0.0000207 g mg−1 min−1) and led to the adsorption of more pollutants in less time.164Table 7 shows the parameters of the adsorption kinetic models in studies on the removal of antibiotics with coated CNTs.
Table 7 Parameters of adsorption kinetic models
Antibiotic |
Coated-CNTs |
Kinetic model |
PFO |
PSO |
IPD |
Ref. |
q
e
|
K
1
|
R
2
|
q
e
|
K
2
|
R
2
|
k
id
|
C
|
R
2
|
TC |
CNTs/C@Fe/CS |
69.4 |
0.004 |
0.88 |
117.6 |
0.004 |
0.99 |
8.2 |
58.9 |
0.97 |
111
|
Al2O3–MWCNTs |
56.24 |
0.094 |
0.841 |
94.46 |
0.0041 |
0.997 |
0.06 |
0.80 |
0.73 |
163
|
214.7 |
0.042 |
0.823 |
249.8 |
0.0059 |
0.998 |
0.12 |
1.24 |
0.75 |
402.8 |
0.035 |
0.796 |
474.2 |
0.0074 |
0.998 |
0.17 |
1.51 |
0.78 |
745.2 |
0.019 |
0.803 |
889.5 |
0.0088 |
0.997 |
0.25 |
1.98 |
0.80 |
TCH |
MWCNT/MIL-53(Fe) |
83.16 |
0.0098 |
0.98 |
89.21 |
0.00015 |
0.99 |
— |
— |
— |
74
|
OTC |
55.96 |
0.0057 |
0.94 |
60.83 |
0.00015 |
0.98 |
— |
— |
— |
CTC |
39.54 |
0.0063 |
0.91 |
43.08 |
0.00018 |
0.98 |
— |
— |
— |
TCH |
NH2-MIL-53(Fe) |
69.86 |
0.0095 |
0.96 |
76.92 |
0.00019 |
0.99 |
— |
— |
— |
10
|
MWCNT/NH2-MIL-53(Fe) |
85.62 |
0.0097 |
0.97 |
90.09 |
0.00018 |
0.99 |
— |
— |
— |
CTC |
NH2-MIL-53(Fe) |
34.43 |
0.0064 |
0.90 |
35.97 |
0.00033 |
0.98 |
— |
— |
— |
MWCNT/NH2-MIL-53(Fe) |
41.99 |
0.0062 |
0.91 |
44.31 |
0.00023 |
0.99 |
— |
— |
— |
AMX |
8-HQ–MWCNT |
1145 |
0.358 |
0.99 |
556 |
0.0000223 |
0.99 |
— |
— |
— |
164
|
COOH–MWCNT |
825 |
0.272 |
0.96 |
526 |
0.0000207 |
0.98 |
— |
— |
— |
CIP |
CNTs/FeS |
— |
0.06 |
0.93 |
— |
— |
— |
— |
— |
— |
72
|
14.2 Adsorption isotherms
An adsorption isotherm is an important tool for evaluating the adsorbent distribution on solid/liquid boundaries and can be used to estimate the adsorption capacity.199 Previous research works confirmed that the interaction between the adsorbate and adsorbent can be determined using adsorption isotherm models.200 Applying isotherms (commonly named equilibrium relationships) to the adsorption processes can be useful for several purposes, as follows: (1) to represent the physicochemical characteristics (especially surface properties) and capacities of adsorbents; (2) to optimize the adsorption process pathways; and (3) to develop efficient designs of adsorption systems.201 To date, several isotherm models including Langmuir,199,202 Freundlich,203,204 Temkin,196 Sips,205 and Dubinin–Radushkevich (D–R)206 models have been applied to describe the equilibrium relations. Notably, among the aforementioned isotherm models, the two well-known isotherm models of Freundlich (Freundlich, 1906) and Langmuir (Langmuir, 1918) were applied to the equilibrium data of coated-CNTs.203 Furthermore, the derivation of the Temkin isotherm assumes that the adsorption heat loss is linear rather than logarithmic.207 This isotherm shows a linear decrease in the adsorption energy with an increase in the number of adsorption centers in an adsorbent. When an adsorbent is coated, the adsorption heat of all the molecules in the layer is reduced due to the adsorbent–adsorbate interaction. In this isotherm, the adsorption is determined by the uniform distribution of the binding energies.163 This model is suitable for chemical adsorption based on strong electrostatic reaction between negative and positive loading.111 The different isotherm models and their main applications are listed in Table 8.
Table 8 Isotherms models, equations, and applications
Isotherms models |
Equations models |
Constant parameters |
Application |
Ref. |
Langmuir |
|
q
e is the amount of adsorbed at equilibrium (mg g−1) |
To express the single-layer adsorption process |
199, 202 and 208 |
|
C
e is the metal concentration in the aqueous phase (mg L−1) |
Freundlich |
|
b is Langmuir equilibrium constant (L g adsorbent−1) |
To suggest the heterogeneous structure of the adsorbent surface |
203, 204 and 209 |
|
q
0 is maximum monolayer adsorption (mg g adsorbent−1) |
Temkin |
q
e = B ln A × Ce |
k
L is the Langmuir constant (L mg−1) |
Assumes the heat of adsorption and effects of indirect interaction between adsorbent molecules have predictive power in a wide concentration range |
196, 204 and 210 |
K
F and n are Freundlich coefficients |
q
e = B ln A + B ln Ce |
Temkin constant is B = RT/b and b (J mol−1) is the adsorption heat |
A (L g−1) is Temkin isotherm constant |
D–R |
ln Qe = ln QD–R – βε2 |
R (3314 J Mol−1 K−1) is the global gas constant in which T (K) is an absolute |
Is used for the isotherm analysis with a high degree of regularity |
206 and 211 |
|
Q
D–R (lg/g) is the theoretical saturation capacity of the adsorbent |
Sips |
|
Is an alternative empirical approach with the characteristics of the Freundlich and Langmuir isotherms |
205
|
b is a constant related to the mean free energy of adsorption per mole of adsorbate |
ε is a logarithmic function of concentration called Polanyi potential. R and T are gas constant and temperature in Kelvin scale, respectively |
The results from the study by Ma et al. (2015) on the adsorption of TC at 25 °C by CNTs/C@Fe/CS showed that the adsorption followed the Freundlich isotherm (R2 = 0.98). The high value of KF (30.30 L g−1) indicates the good affinity between TC and CNTs/C@Fe/CS and its high adsorption capacity. Alternatively, because the value of 1/n is less than 1 (0.78), the adsorption is desirable.111 Also, based on the results from the study by Xiao et al. (2018) on the removal of TC antibiotics using MWCNT/NH2-MIL-53(Fe), the R2 of the Langmuir model was higher than that of the Freundlich and Temkin isotherm models, which indicated the adsorption of a monolayer on its surface. Alternatively, low the RL values showed the strong interaction between the adsorbates and NH2–MWCNT/MIL-53 (Fe).10Table 9 shows the parameters of the adsorption isotherm models in studies on the removal of antibiotics with coated CNTs.
Table 9 Parameters of adsorption isotherm models
Antibiotic |
Coated-CNTs |
Isotherm model |
Ref. |
Langmuir |
Freundlich |
Temkin |
q
m
|
B
|
R
2
|
K
F
|
n
|
R
2
|
A
|
B
|
R
2
|
TC |
CNTs/C@Fe/CS |
103 |
0.05 |
0.82 |
4.55 |
2.88 |
0.96 |
2.49 |
18.65 |
0.69 |
111
|
104 |
0.21 |
0.95 |
30.30 |
1.28 |
0.98 |
4.29 |
23.35 |
0.84 |
117 |
0.35 |
0.94 |
33.87 |
4.04 |
0.96 |
22.09 |
14.86 |
0.89 |
Al2O3–MWCNTs |
833.4 |
0.012 |
0.998 |
59.36 |
2.81 |
0.84 |
12.84 |
47.69 |
0.79 |
163
|
855.6 |
0.031 |
0.997 |
72.11 |
3.17 |
0.85 |
9.424 |
53.46 |
0.80 |
876.8 |
0.056 |
0.999 |
84.48 |
3.64 |
0.81 |
7.436 |
61.78 |
0.82 |
TCH |
MWCNT/MIL-53(Fe) |
364.37 |
0.044 |
0.98 |
47.74 |
0.40 |
0.97 |
— |
— |
— |
74
|
OTC |
325.59 |
0.011 |
0.99 |
9.16 |
0.62 |
0.97 |
— |
— |
— |
CTC |
180.68 |
0.042 |
0.97 |
24.82 |
0.38 |
0.98 |
— |
— |
— |
TCH |
NH2-MIL-53(Fe) |
302.63 |
0.042 |
0.99 |
36.03 |
2.38 |
0.96 |
49.12 |
1.13 |
0.97 |
10
|
MWCNT/NH2-MIL-53(Fe) |
368.49 |
0.064 |
0.98 |
60.73 |
2.70 |
0.96 |
49.01 |
3.95 |
0.90 |
CTC |
NH2-MIL-53(Fe) |
152.29 |
0.024 |
0.99 |
11.74 |
2.12 |
0.94 |
25.03 |
0.56 |
0.91 |
MWCNT/NH2-MIL-53(Fe) |
254.04 |
0.044 |
0.99 |
33.58 |
2.56 |
0.95 |
39.97 |
1.34 |
0.91 |
AMX |
8-HQ–MWCNT |
784 |
0.006 |
0.99 |
10.62 |
1.33 |
0.99 |
— |
— |
— |
164
|
COOH–MWCNT |
647 |
0.007 |
0.97 |
8.23 |
1.27 |
0.98 |
— |
— |
— |
15 Future challenges and perspectives
As mentioned above, recently many experimental studies have been performed regarding the use of carbon-based NPs for the removal of antibiotics. Among the carbonaceous martials, CNTs have gained considerable attention in the environmental engineering field (adsorbents, sensors, membrane modifiers, catalysts, gene therapy, and cancer treatments) due to their unique physicochemical, mechanical, thermal, and electrical properties. However, it worth mentioning that despite their numerous advantages, the use of CNTs on a large scale is hindered because of some limitations such as their high synthesis cost, low hydrophilicity, complex interactions with environmental colloids and microorganisms, toxicity and effect on the eco-system, and difficult recovery and regeneration. Therefore, it is urgent that researchers design new CNTs that are safe and non-toxic (synthesized through green methods), with fewer impurities, easy regeneration, and also lower costs. Herein, based on the above overview and lack of important information on the use of CNTs, we propose some points that should be considered for their future research and real-world applications, as follows:
• Study of the feasibility of CNTs on an industrial and large scale.
• Study of the optimization of various parameters affecting the synthesis route of CNTs.
• Study of the accurate human health, environmental, and eco-toxicological risk assessments related to the toxicity of CNTs.
• The development of new CNT-based remediation technologies.
• Evaluating the cost-effective synthesis of CNTs.
• Study of proper, economic, safe, and environmentally friendly options for the disposal of CNTs.
• Study of the by-products produced after using CNTs and their derivatives during the removal of antibiotics.
• Integrating CNTs with the other systems.
16 Conclusions
Examination of the literature showed that the surface of single-walled and multi-walled CNTs can be modified with different materials to overcome the hydrophobicity and chemical neutrality of the outer wall of conventional CNTs for the removal of antibiotics from aqueous solutions. Similar to many other adsorbents, the adsorption capacity of coated CNTs depends on factors such as adsorbent dosage, antibiotic initial concentration, pH, and contact time. In terms of equilibrium study, in most of the studies mentioned in the present study, the adsorption follows the Langmuir model, which represents monolayer adsorption. Studies have used the intra-particle diffusion, pseudo-first-order and pseudo-second-order models to model the adsorption kinetics, where in most of them, the pseudo-second-order model was in full agreement with the experimental data. Furthermore, this comprehensive review highlighted that the major mechanisms for the adsorption of antibiotics on coated-CNTs are π–π interaction, electrostatic interaction, hydrophobic interaction, and hydrogen bonding.
Abbreviation
AMX
| Amoxicillin |
CIP
| Ciprofloxacin |
TC
| Tetracycline |
SA
| Sulfonamide |
FQ
| Fluoroquinolone |
OTC
| Oxytetracycline |
SMX
| Sulfamethoxazole |
SMZ
| Sulfamethazine |
TCN
| Tetracycline hydrochloride |
CTC
| Chlortetracycline hydrochloride |
AC
| Activated carbon |
CNT
| Carbon nanotube |
SWCNT
| Single-wall CNT |
MWCNT
| Multi-wall CNT |
CVD
| Chemical vapor deposition |
MMWCNT
| Magnetic multi-wall CNT |
RO
| Reverse osmosis |
MFC
| Microbial fuel cell |
CW
| Constructed wetland |
CF
| Coagulation-flocculation |
MBBR
| Moving bed biofilm reactor |
AOP
| Advance oxidation process |
XRD
| X-Ray diffraction |
SEM
| Scanning electron microscopy |
EDX
| Energy dispersive X-ray analysis |
FTIR
| Fourier transform infrared spectroscopy |
WWTP
| Wastewater treatment plant |
PFO
| Pseudo-first-order |
PSO
| Pseudo-second-order |
IPD
| Intra particle diffusion |
D-R
| Dubinin-Radushkevich |
Author contributions
P. Darvishi: writing first draft, review, editing; S. A. Mousavi: supervision, conceptualization, investigation, and writing—review, editing, and project administration; A. Mahmmoudi: writing first draft, review, editing; D. Nayeri: writing–original draft preparation, reviewing, and editing.
Conflicts of interest
The authors have declared no conflict of interest.
Acknowledgements
The authors gratefully acknowledge the Research Council of Kermanshah University of Medical Sciences for the financial support (Grant number: 4010455) under ethics approval number: IR.KUMS.REC.1401.081.
References
- Y. Zhou, N. Zhu, W. Guo, Y. Wang, X. Huang, P. Wu, Z. Dang, X. Zhang and J. Xian, Simultaneous electricity production and antibiotics removal by microbial fuel cells, J. Environ. Manage., 2018, 217, 565–572 CrossRef CAS PubMed.
- S. Mallakpour and A. Zadehnazari, A facile, efficient, and rapid covalent functionalization of multi-walled carbon nanotubes with natural amino acids under microwave irradiation, Prog. Org. Coat., 2014, 77, 679–684 CrossRef CAS.
-
R. Alexy and K. Kümmerer, Antibiotics for human use, Partition. Adsorp. Organic. Contm. Environ. Syst., 2006 Search PubMed.
- K. D. Brown, J. Kulis, B. Thomson, T. H. Chapman and D. B. Mawhinney, Occurrence of antibiotics in hospital, residential, and dairy effluent, municipal wastewater, and the Rio Grande in New Mexico, Sci. Total Environ., 2006, 366, 772–783 CrossRef CAS PubMed.
- J. Lyu, L. Yang, L. Zhang, B. Ye and L. Wang, Antibiotics in soil and water in China–a systematic review and source analysis, Environ. Pollut., 2020, 115147 CrossRef PubMed.
- K. Kümmerer, Antibiotics in the aquatic environment–a review–part I, Chemosphere, 2009, 75, 417–434 CrossRef PubMed.
- S. K. Malek, M. A. Gabris, B. H. Jume, R. Baradaran, M. Aziz, K. J. B. A. Karim and H. R. Nodeh, Adsorption and in vitro release study of curcumin form polyethyleneglycol functionalized multi walled carbon nanotube: kinetic and isotherm study, Daru, J. Pharm. Sci., 2019, 27, 9–20 CrossRef PubMed.
- M. A. Motchelaho, H. Xiong, M. Moyo, L. L. Jewell and N. J. Coville, Effect of acid treatment on the surface of multiwalled carbon nanotubes prepared from Fe–Co supported on CaCO3: correlation with Fischer–Tropsch catalyst activity, J. Mol. Catal. A: Chem., 2011, 335, 189–198 CrossRef CAS.
- Z. Aksu and Ö. Tunç, Application of biosorption for penicillin G removal: comparison with activated carbon, Process Biochem., 2005, 40, 831–847 CrossRef CAS.
- W. Xiong, Z. Zeng, X. Li, G. Zeng, R. Xiao, Z. Yang, Y. Zhou, C. Zhang, M. Cheng and L. Hu, Multi-walled carbon nanotube/amino-functionalized MIL-53 (Fe) composites: remarkable adsorptive removal of antibiotics from aqueous solutions, Chemosphere, 2018, 210, 1061–1069 CrossRef CAS PubMed.
- A. Watkinson, E. Murby and S. Costanzo, Removal of antibiotics in conventional and advanced wastewater treatment: implications for environmental discharge and wastewater recycling, Water Res., 2007, 41, 4164–4176 CrossRef CAS PubMed.
- E. S. Elmolla and M. Chaudhuri, The feasibility of using combined TiO2 photocatalysis-SBR process for antibiotic wastewater treatment, Desalination, 2011, 272, 218–224 CrossRef CAS.
- C.-H. Huang, J. E. Renew, K. L. Smeby, K. Pinkston and D. L. Sedlak, Assessment of potential antibiotic contaminants in water and preliminary occurrence analysis, Journal of Contemporary Water Research and Education, 2011, 120, 4 Search PubMed.
- A. Ghauch, A. Tuqan and H. A. Assi, Antibiotic removal from water: elimination of amoxicillin and ampicillin by microscale and nanoscale iron particles, Environ. Pollut., 2009, 157, 1626–1635 CrossRef CAS PubMed.
- M. C. Ncibi and M. Sillanpää, Optimized removal of antibiotic drugs from aqueous solutions using single, double and multi-walled carbon nanotubes, J. Hazard. Mater., 2015, 298, 102–110 CrossRef CAS PubMed.
- A. Längin, R. Alexy, A. König and K. Kümmerer, Deactivation and transformation products in biodegradability testing of β-lactams amoxicillin and piperacillin, Chemosphere, 2009, 75, 347–354 CrossRef PubMed.
- N. Torimiro, A. Moshood and S. Eyiolawi, Analysis of Beta-lactamase production and antibiotics resistance in Staphylococcus aureus strains, J. Infect. Dis. Immun., 2013, 5, 24–28 CrossRef.
- T. H. Grossman, Tetracycline antibiotics and resistance, Cold Spring Harbor Perspect. Med., 2016, 6, a025387 CrossRef PubMed.
- A. S. Adeleye, J. R. Conway, K. Garner, Y. Huang, Y. Su and A. A. Keller, Engineered nanomaterials for water treatment and remediation: Costs, benefits, and applicability, J. Chem. Eng., 2016, 286, 640–662 CrossRef CAS.
- D. Shahbazi, S. Mousavi and D. Nayeri, Low-cost activated carbon: characterization, decolorization, modeling, optimization and kinetics, Int. J. Environ. Sci. Technol., 2020, 17, 3935–3946 CrossRef CAS.
- S. A. Mousavi, H. Zangeneh, A. Almasi, D. Nayeri, M. Monkaresi, A. Mahmoudi and P. Darvishi, Decolourization of aqueous Methylene Blue solutions by corn stalk: modeling and optimization, Desalin. Water Treat., 2020, 197, 335–344 CrossRef CAS.
- S. Mehdizadeh, S. Sadjadi, S. J. Ahmadi and M. Outokesh, Removal of heavy metals from aqueous solution using platinum nanopartcles/Zeolite-4A, J. Environ. Health Sci. Eng., 2014, 12, 1–7 CrossRef PubMed.
- S. Moosavi, C. W. Lai, S. Gan, G. Zamiri, O. Akbarzadeh Pivehzhani and M. R. Johan, Application of efficient magnetic particles and activated carbon for dye removal from wastewater, ACS Omega, 2020, 5, 20684–20697 CrossRef CAS PubMed.
- V. Choudhary, K. Vellingiri, M. I. Thayyil and L. Philip, Removal of antibiotics from aqueous solutions by electrocatalytic degradation, Environ. Sci.: Nano, 2021, 8, 1133–1176 RSC.
- G. Gangadhar, U. Maheshwari and S. Gupta, Application of nanomaterials for the removal of pollutants from effluent streams, Nanosci. Nanotechnol.--Asia, 2012, 2, 140–150 CrossRef CAS.
- M. Yoosefian, S. Ahmadzadeh, M. Aghasi and M. Dolatabadi, Optimization of electrocoagulation process for efficient removal of ciprofloxacin antibiotic using iron electrode; kinetic and isotherm studies of adsorption, J. Mol. Liq., 2017, 225, 544–553 CrossRef CAS.
- A. M. Mebert, C. Aimé, G. S. Alvarez, Y. Shi, S. A. Flor, S. E. Lucangioli, M. F. Desimone and T. Coradin, Silica core–shell particles for the dual delivery of gentamicin and rifamycin antibiotics, J. Mater. Chem. B, 2016, 4, 3135–3144 RSC.
- J. Peng, Y. He, C. Zhou, S. Su and B. Lai, The carbon nanotubes-based materials and their applications for organic pollutant removal: A critical review, Chin. Chem. Lett., 2021, 32, 1626–1636 CrossRef CAS.
- A. Mamalis, L. Vogtländer and A. Markopoulos, Nanotechnology and nanostructured materials: trends in carbon nanotubes, Precis. Eng., 2004, 28, 16–30 CrossRef.
-
M. Rahmandoust and M. R. Ayatollahi, Characterization of carbon nanotube based composites under consideration of defects, Springer, 2016, pp. 5–63 Search PubMed.
- R. H. Baughman, A. A. Zakhidov and W. A. De Heer, Carbon nanotubes--the route toward applications, Science, 2002, 297, 787–792 CrossRef CAS PubMed.
- J. Wang, H.-L. Duan, L. Fan, Y.-M. Lin, J.-N. Sun and Z.-Q. Zhang, Magnetic tetraethylenepentamine modified multi-walled carbon nanotubes as matrix clean-up materials for organophosphorus pesticide residues analysis in cucumber, Food Control, 2021, 124, 107904 CrossRef CAS.
- L. Lavagna, R. Nisticò, S. Musso and M. Pavese, Functionalization as a way to enhance dispersion of carbon nanotubes in matrices: A review, Mater. Today Chem., 2021, 20, 100477 CrossRef CAS.
- W. Zhao, Y. Tian, X. Chu, L. Cui, H. Zhang, M. Li and P. Zhao, Preparation and characteristics of a magnetic carbon nanotube adsorbent: Its efficient adsorption and recoverable performances, Sep. Purif. Technol., 2021, 257, 117917 CrossRef CAS.
- K. M. Lee, C. P. P. Wong, T. L. Tan and C. W. Lai, Functionalized carbon nanotubes for adsorptive removal of water pollutants, Mater. Sci. Eng., B, 2018, 236, 61–69 Search PubMed.
- K.-Y. Chen, Y.-Y. Shen, D.-M. Wang and C.-H. Hou, Carbon nanotubes/activated carbon hybrid as a high-performance suspension electrode for the electrochemical desalination of wastewater, Desalination, 2022, 522, 115440 CrossRef CAS.
- H. Karimi-Maleh, M. Moazampour, A. A. Ensafi, S. Mallakpour and M. Hatami, An electrochemical nanocomposite modified carbon paste electrode as a sensor for simultaneous determination of hydrazine and phenol in water and wastewater samples, Environ. Sci. Pollut. Res., 2014, 21, 5879–5888 CrossRef CAS PubMed.
- J. Garcia, H. Gomes, P. Serp, P. Kalck, J. Figueiredo and J. Faria, Carbon nanotube supported ruthenium catalysts for the treatment of high strength wastewater with aniline using wet air oxidation, Carbon, 2006, 44, 2384–2391 CrossRef CAS.
- S. Yang, Z. Guo, G. Sheng and X. Wang, Investigation of the sequestration mechanisms of Cd (II) and 1-naphthol on discharged multi-walled carbon nanotubes in aqueous environment, Sci. Total Environ., 2012, 420, 214–221 CrossRef CAS PubMed.
- A. C. Dillon, K. Jones, T. Bekkedahl, C. Kiang, D. Bethune and M. Heben, Storage of hydrogen in single-walled carbon nanotubes, Nature, 1997, 386, 377–379 CrossRef CAS.
- A. Farghali, H. Abdel Tawab, S. Abdel Moaty and R. Khaled, Functionalization of acidified multi-walled carbon nanotubes for removal of heavy metals in aqueous solutions, J. Nanostruct. Chem., 2017, 7, 101–111 CrossRef CAS.
- M. Vesali-Naseh, Y. Mortazavi, A. A. Khodadadi, P. Parsaeian and A. A. Moosavi-Movahedi, Plasma thiol-functionalized carbon nanotubes decorated with gold nanoparticles for glucose biosensor, Sens. Actuators, B, 2013, 188, 488–495 CrossRef CAS.
- R. Foroutan, S. J. Peighambardoust, P. Latifi, A. Ahmadi, M. Alizadeh and B. Ramavandi, Carbon nanotubes/β-cyclodextrin/MnFe2O4 as a magnetic nanocomposite powder for tetracycline antibiotic decontamination from different aqueous environments, J. Environ. Chem. Eng., 2021, 9, 106344 CrossRef CAS.
- C. Wang, G. Zhou, H. Liu, J. Wu, Y. Qiu, B.-L. Gu and W. Duan, Chemical functionalization of carbon nanotubes by carboxyl groups on Stone-Wales defects: a density functional theory study, J. Phys. Chem. B, 2006, 110, 10266–10271 CrossRef CAS PubMed.
- Y. Wang, H. Huang and X. Wei, Influence of wastewater precoagulation on adsorptive filtration of pharmaceutical and personal care products by carbon nanotube membranes, J. Chem. Eng., 2018, 333, 66–75 CrossRef CAS.
- Y. Liu, H. Liu, Z. Zhou, T. Wang, C. N. Ong and C. D. Vecitis, Degradation of the common aqueous antibiotic tetracycline using a carbon nanotube electrochemical filter, Environ. Sci. Technol., 2015, 49, 7974–7980 CrossRef CAS PubMed.
- L. J. Sweetman, L. J. Alcock, J. D. McArthur, E. M. Stewart, G. Triani and S. F. Ralph, Bacterial filtration using carbon nanotube/antibiotic buckypaper membranes, J. Nanomater., 2013, 2013, 1–11 CrossRef.
- A. Tiraferri, C. D. Vecitis and M. Elimelech, Covalent binding of single-walled carbon nanotubes to polyamide membranes for antimicrobial surface properties, ACS Appl. Mater. Interfaces, 2011, 3, 2869–2877 CrossRef CAS PubMed.
- X. Fan, Y. Liu, X. Wang, X. Quan and S. Chen, Improvement of antifouling and antimicrobial abilities on silver–carbon nanotube based membranes under electrochemical assistance, Environ. Sci. Technol., 2019, 53, 5292–5300 CrossRef CAS PubMed.
- C. Dai, S. Shi, D. Chen, J. Liu, L. Huang, J. Zhang and Y. Feng, Study on the mechanism of tetracycline removal in electrocoagulation coupled with electro-fenton reaction system with Fe anode and carbon nanotube cathode, J. Chem. Eng., 2022, 428, 131045 CrossRef CAS.
- D. Kang, X. Yu, M. Ge, F. Xiao and H. Xu, Novel Al-doped carbon nanotubes with adsorption and coagulation promotion for organic pollutant removal, J. Environ. Sci., 2017, 54, 1–12 CrossRef CAS PubMed.
- G. S. Simate, The treatment of brewery wastewater for reuse by integration of coagulation/flocculation and sedimentation with carbon nanotubes ‘sandwiched’ in a granular filter bed, J. Ind. Eng. Chem., 2015, 21, 1277–1285 CrossRef CAS.
- D. Nasuhoglu, A. Rodayan, D. Berk and V. Yargeau, Removal of the antibiotic levofloxacin (LEVO) in water by ozonation and TiO2 photocatalysis, J. Chem. Eng., 2012, 189, 41–48 CrossRef.
- G. P. Dinos, The macrolide antibiotic renaissance, Br. J. Pharmacol., 2017, 174, 2967–2983 CrossRef CAS PubMed.
- E. Jafari Ozumchelouei, A. H. Hamidian, Y. Zhang and M. Yang, Physicochemical properties of antibiotics: A review with an emphasis on detection in the aquatic environment, Water Environ. Res., 2020, 92, 177–188 CrossRef CAS PubMed.
- M. Shakak, R. Rezaee, A. Maleki, A. Jafari, M. Safari, B. Shahmoradi, H. Daraei and S.-M. Lee, Synthesis and characterization of nanocomposite ultrafiltration membrane (PSF/PVP/SiO2) and performance evaluation for the removal of amoxicillin from aqueous solutions, Environ. Technol. Innovation, 2020, 17, 100529 CrossRef.
- A. Almasi, F. Karami, S. A. Mousavi and R. Khodarahmi, Immobilization of IMP-1 metallo-beta-lactamase on Fe3O4@ SiO2 as nanobiocatalyst for degradation of beta-lactam antibiotics in wastewater, Water Sci. Technol., 2022, 85, 2189–2207 CrossRef.
- T.-H. Kim, S. D. Kim, H. Y. Kim, S. J. Lim, M. Lee and S. Yu, Degradation and toxicity assessment of sulfamethoxazole and chlortetracycline using electron beam, ozone and UV, J. Hazard. Mater., 2012, 227, 237–242 CrossRef PubMed.
- X. Ling, Y. Wei, L. Zou and S. Xu, The effect of different order of purification treatments on the purity of multiwalled carbon nanotubes, Appl. Surf. Sci., 2013, 276, 159–166 CrossRef CAS.
- O. Rozas, D. Contreras, M. A. Mondaca, M. Pérez-Moya and H. D. Mansilla, Experimental design of Fenton and photo-Fenton reactions for the treatment of ampicillin solutions, J. Hazard. Mater., 2010, 177, 1025–1030 CrossRef CAS PubMed.
- I. Koyuncu, O. A. Arikan, M. R. Wiesner and C. Rice, Removal of hormones and antibiotics by nanofiltration membranes, J. Membr. Sci., 2008, 309, 94–101 CrossRef CAS.
- P.-X. Hou, C. Liu and H.-M. Cheng, Purification of carbon nanotubes, Carbon, 2008, 46, 2003–2025 CrossRef CAS.
- W. Jiang, P. Molian and H. Ferkel, Rapid production of carbon nanotubes by high-power laser ablation, J. Eng. Ind., 2005, 127, 703–707 Search PubMed.
- R. A. Ismail, M. H. Mohsin, A. K. Ali, K. I. Hassoon and S. Erten-Ela, Preparation and characterization of carbon nanotubes by pulsed laser ablation in water for optoelectronic application, Phys. E, 2020, 119, 113997 CrossRef CAS.
-
Y. Shunin, S. Bellucci, A. Gruodis and T. Lobanova-Shunina, Nonregular Nanosystems, Springer, 2018, pp. 207–251 Search PubMed.
- Y. Kim, E. Nishikawa and Y. Watanabe, Carbon nanotube synthesis and dispersion using arc discharge in foam made with a surfactant, e-J. Surf. Sci. Nanotechnol., 2018, 16, 382–386 CrossRef CAS.
- E. Nativ-Roth, R. Shvartzman-Cohen, C. Bounioux, M. Florent, D. Zhang, I. Szleifer and R. Yerushalmi-Rozen, Physical adsorption of block copolymers to SWNT and MWNT: a nonwrapping mechanism, Macromolecules, 2007, 40, 3676–3685 CrossRef CAS.
- V. Homem and L. Santos, Degradation and removal methods of antibiotics from aqueous matrices–a review, J. Environ. Manage., 2011, 92, 2304–2347 CrossRef CAS PubMed.
- K. B. Shelimov, R. O. Esenaliev, A. G. Rinzler, C. B. Huffman and R. E. Smalley, Purification of single-wall carbon nanotubes by ultrasonically assisted filtration, Chem. Phys. Lett., 1998, 282, 429–434 CrossRef CAS.
- A. Payan, A. A. Isari and N. Gholizade, Catalytic decomposition of sulfamethazine antibiotic and pharmaceutical wastewater using Cu-TiO2@ functionalized SWCNT ternary porous nanocomposite: influential factors, mechanism, and pathway studies, J. Chem. Eng., 2019, 361, 1121–1141 CrossRef CAS.
- M. Asgher and H. N. Bhatti, Evaluation of thermodynamics and effect of chemical treatments on sorption potential of Citrus waste biomass for removal of anionic dyes from aqueous solutions, Ecol. Eng., 2012, 38, 79–85 CrossRef.
- J. Ma, M. Yang, F. Yu and J. Chen, Easy solid-phase synthesis of pH-insensitive heterogeneous CNTs/FeS Fenton-like catalyst for the removal of antibiotics from aqueous solution, J. Colloid Interface Sci., 2015, 444, 24–32 CrossRef CAS PubMed.
- L. Ji, Y. Shao, Z. Xu, S. Zheng and D. Zhu, Adsorption of monoaromatic compounds and pharmaceutical antibiotics on carbon nanotubes activated by KOH etching, Environ. Sci. Technol., 2010, 44, 6429–6436 CrossRef CAS PubMed.
- W. Xiong, G. Zeng, Z. Yang, Y. Zhou, C. Zhang, M. Cheng, Y. Liu, L. Hu, J. Wan and C. Zhou, Adsorption of tetracycline antibiotics from aqueous solutions on nanocomposite multi-walled carbon nanotube functionalized MIL-53 (Fe) as new adsorbent, Sci. Total Environ., 2018, 627, 235–244 CrossRef CAS PubMed.
- Z. Bai, Q. Yang and J. Wang, Catalytic ozonation of sulfamethazine antibiotics using Fe3O4/multiwalled carbon nanotubes, Environ. Prog. Sustainable Energy, 2018, 37, 678–685 CrossRef CAS.
- N. Hanna, P. Sun, Q. Sun, X. Li, X. Yang, X. Ji, H. Zou, J. Ottoson, L. E. Nilsson and B. Berglund, Presence of antibiotic residues in various environmental compartments of Shandong province in eastern China: its potential for resistance development and ecological and human risk, Environ. Int., 2018, 114, 131–142 CrossRef CAS PubMed.
- A. Spielmeyer, Occurrence and fate of antibiotics in manure during manure treatments: a short review, Sustainable Chem. Pharm., 2018, 9, 76–86 CrossRef.
- V. Minden, A. Deloy, A. M. Volkert, S. D. Leonhardt and G. Pufal, Antibiotics impact plant traits, even at small concentrations, AoB Plants, 2017, 9, 1–19 CrossRef PubMed.
- M. Pan and L. Chu, Fate of antibiotics in soil and their uptake by edible crops, Sci. Total Environ., 2017, 599, 500–512 CrossRef PubMed.
- L. A. Pinck, W. F. Holton and F. E. Allison, Antibiotics in soils: 1. Physico-chemical studies of antibiotic-clay complexes, Soil Sci., 1961, 91, 22–28 CrossRef CAS.
- M. J. Ahmed, Adsorption of quinolone, tetracycline, and penicillin antibiotics from aqueous solution using activated carbons, Environ. Toxicol. Pharmacol., 2017, 50, 1–10 CrossRef CAS PubMed.
- T. Christian, R. J. Schneider, H. A. Färber, D. Skutlarek, M. T. Meyer and H. E. Goldbach, Determination of antibiotic residues in manure, soil, and surface waters, Acta Hydrochim. Hydrobiol., 2003, 31, 36–44 CrossRef CAS.
- Z. Derakhshan, M. Mokhtari, F. Babaei, R. Malek Ahmadi, M. H. Ehrampoush and M. Faramarzian, Removal methods of antibiotic compounds from aqueous environments–a review, J. Environ. Health Sustainable Dev., 2016, 1, 43–62 Search PubMed.
- S. M. Limbu, L. Zhou, S.-X. Sun, M.-L. Zhang and Z.-Y. Du, Chronic exposure to low environmental concentrations and legal aquaculture doses of antibiotics cause systemic adverse effects in Nile tilapia and provoke differential human health risk, Environ. Int., 2018, 115, 205–219 CrossRef CAS PubMed.
- L. Wang, Y. Liu, J. Ma and F. Zhao, Rapid degradation of sulphamethoxazole and the further transformation of 3-amino-5-methylisoxazole in a microbial fuel cell, Water Res., 2016, 88, 322–328 CrossRef CAS PubMed.
- X. Liu, J. C. Steele and X.-Z. Meng, Usage, residue, and human health risk of antibiotics in Chinese aquaculture: a review, Environ. Pollut., 2017, 223, 161–169 CrossRef CAS PubMed.
- P. Macheboeuf, C. Contreras-Martel, V. Job, O. Dideberg and A. Dessen, Penicillin binding proteins: key players in bacterial cell cycle and drug resistance processes, FEMS Microbiol. Rev., 2006, 30, 673–691 CrossRef CAS PubMed.
- T.-H. Le, C. Ng, N. H. Tran, H. Chen and K. Y.-H. Gin, Removal of antibiotic residues, antibiotic resistant bacteria and antibiotic resistance genes in municipal wastewater by membrane bioreactor systems, Water Res., 2018, 145, 498–508 CrossRef CAS PubMed.
- E. Sanganyado and W. Gwenzi, Antibiotic resistance in drinking water systems: Occurrence, removal, and human health risks, Sci. Total Environ., 2019, 669, 785–797 CrossRef CAS PubMed.
- M. Pirsaheb, H. Hossaini and H. Janjani, Reclamation of hospital secondary treatment effluent by sulfate radicals based–advanced oxidation processes (SR-AOPs) for removal of antibiotics, Microchem. J., 2020, 153, 104430 CrossRef CAS.
- J. J. S. Alonso, N. El Kori, N. Melián-Martel and B. Del Río-Gamero, Removal of ciprofloxacin from seawater by reverse osmosis, J. Environ. Manage., 2018, 217, 337–345 CrossRef CAS PubMed.
- T. Saitoh, K. Shibata, K. Fujimori and Y. Ohtani, Rapid removal of tetracycline antibiotics from water by coagulation-flotation of sodium dodecyl sulfate and poly (allylamine hydrochloride) in the presence of Al (III) ions, Sep. Purif. Technol., 2017, 187, 76–83 CrossRef CAS.
- J. Kang, X. Duan, C. Wang, H. Sun, X. Tan, M. O. Tade and S. Wang, Nitrogen-doped bamboo-like carbon nanotubes with Ni encapsulation for persulfate activation to remove emerging contaminants with excellent catalytic stability, J. Chem. Eng., 2018, 332, 398–408 CrossRef CAS.
- E. M. Cuerda-Correa, M. F. Alexandre-Franco and C. Fernández-González, Advanced oxidation processes for the removal of antibiotics from water. An overview, Water, 2020, 12, 102 CrossRef CAS.
- M. del Mar Gómez-Ramos, M. Mezcua, A. Agüera, A. R. Fernández-Alba, S. Gonzalo, A. Rodríguez and R. Rosal, Chemical and toxicological evolution of the antibiotic sulfamethoxazole under ozone treatment in water solution, J. Hazard. Mater., 2011, 192, 18–25 Search PubMed.
- G. Kooijman, M. de Kreuk, C. Houtman and J. van Lier, Perspectives of coagulation/flocculation for the removal of pharmaceuticals from domestic wastewater: A critical view at experimental procedures, J. Water Process. Eng., 2020, 34, 101161 CrossRef.
- D. Nayeri, S. A. Mousavi and A. Mehrabi, Oxytetracycline removal from aqueous solutions using activated carbon prepared from corn stalks, J. Appl. Res. Water Wastewater, 2019, 6, 67–72 Search PubMed.
- D. Hui Liang, Y. Hu, D. Liang, J. Chenga and Y. Chena, Bioaugmentation of Moving Bed Biofilm Reactor (MBBR) with Achromobacter JL9 for enhanced sulfamethoxazole (SMX) degradation in aquaculture wastewater, Ecotoxicol. Environ. Saf., 2021, 207, 111258 CrossRef PubMed.
- X.-L. Yang, Q. Wang, T. Li, H. Xu and H.-L. Song, Antibiotic removal and antibiotic resistance genes fate by regulating bioelectrochemical characteristics in microbial fuel cells, Bioresour. Technol., 2022, 126752 CrossRef CAS PubMed.
- L. Zhang, C. Yan, R. Qi and F. Yang, Quantifying the contribution rates of sulfonamide antibiotics removal mechanisms in constructed wetlands using multivariate statistical analysis, Environ. Pollut., 2022, 292, 118463 CrossRef CAS PubMed.
- D. G. Kim, D. Choi, S. Cheon, S.-O. Ko, S. Kang and S. Oh, Addition of biochar into activated sludge improves removal of antibiotic ciprofloxacin, J. Water Process. Eng., 2020, 33, 101019 CrossRef.
- W. T. Vieira, M. B. de Farias, M. P. Spaolonzi, M. G. C. da Silva and M. G. A. Vieira, Removal of endocrine disruptors in waters by adsorption, membrane filtration and biodegradation. A review, Environ. Chem. Lett., 2020, 18, 1113–1143 CrossRef CAS.
- E. A. Serna-Galvis, J. Silva-Agredo, A. L. Giraldo-Aguirre, O. A. Flórez-Acosta and R. A. Torres-Palma, High frequency ultrasound as a selective advanced oxidation process to remove penicillinic antibiotics and eliminate its antimicrobial activity from water, Ultrason. Sonochem., 2016, 31, 276–283 CrossRef CAS PubMed.
- A. C. Affam and M. Chaudhuri, Optimization of Fenton treatment of amoxicillin and cloxacillin antibiotic aqueous solution, Desalin. Water Treat., 2014, 52, 1878–1884 CrossRef CAS.
- P. Shukla, S. Wang, H. Sun, H.-M. Ang and M. Tadé, Adsorption and heterogeneous advanced oxidation of phenolic contaminants using Fe loaded mesoporous SBA-15 and H2O2, J. Chem. Eng., 2010, 164, 255–260 CrossRef CAS.
- F. J. Beltran, A. Aguinaco, J. F. García-Araya and A. Oropesa, Ozone and photocatalytic processes to remove the antibiotic sulfamethoxazole from water, Water Res., 2008, 42, 3799–3808 CrossRef CAS PubMed.
- E. K. Putra, R. Pranowo, J. Sunarso, N. Indraswati and S. Ismadji, Performance of activated carbon and bentonite for adsorption of amoxicillin from wastewater: mechanisms, isotherms and kinetics, Water Res., 2009, 43, 2419–2430 CrossRef CAS PubMed.
- S. Parlayici, V. Eskizeybek, A. Avcı and E. Pehlivan, Removal of chromium (VI) using activated carbon-supported-functionalized carbon nanotubes, J. Nanostruct. Chem., 2015, 5, 255–263 CrossRef CAS.
- R. Crisafully, M. A. L. Milhome, R. M. Cavalcante, E. R. Silveira, D. De Keukeleire and R. F. Nascimento, Removal of some polycyclic aromatic hydrocarbons from petrochemical wastewater using low-cost adsorbents of natural origin, Bioresour. Technol., 2008, 99, 4515–4519 CrossRef CAS PubMed.
- M. A. Atieh, Removal of chromium (VI) from polluted water using carbon nanotubes supported with activated carbon, Procedia Environ. Sci., 2011, 4, 281–293 CrossRef CAS.
- J. Ma, Y. Zhuang and F. Yu, Facile method for the synthesis of a magnetic CNTs–C@ Fe–chitosan composite and its application in tetracycline removal from aqueous solutions, Phys. Chem. Chem. Phys., 2015, 17, 15936–15944 RSC.
- L. Chen, J. Ma, X. Li, J. Zhang, J. Fang, Y. Guan and P. Xie, Strong enhancement on Fenton oxidation by addition of hydroxylamine to accelerate the ferric and ferrous iron cycles, Environ. Sci. Technol., 2011, 45, 3925–3930 CrossRef CAS PubMed.
- M.-h. Zhang, H. Dong, L. Zhao, D.-x. Wang and D. Meng, A review on Fenton process for organic wastewater treatment based on optimization perspective, Sci. Total Environ., 2019, 670, 110–121 CrossRef CAS PubMed.
- X. Li, X. Wang, D. Liu, S. Song and H. Zhang, Multifunctional nanostructures based on porous silica covered Fe3O4@ CeO2–Pt composites: a thermally stable and magnetically-recyclable catalyst system, Chem. Commun., 2014, 50, 7198–7201 RSC.
- S. O. Ganiyu, M. Zhou and C. A. Martínez-Huitle, Heterogeneous electro-Fenton and photoelectro-Fenton processes: a critical review of fundamental principles and application for water/wastewater treatment, Appl. Catal., B, 2018, 235, 103–129 CrossRef CAS.
- C. C. Loures, M. A. Alcântara, H. J. Izário Filho, A. Teixeira, F. T. Silva, T. C. Paiva and G. R. Samanamud, Advanced oxidative degradation processes: fundamentals and applications, Int. Rev. Chem. Eng., 2013, 5, 102–120 Search PubMed.
- N. Ozairi, S. A. Mousavi, M. T. Samadi, A. Seidmohammadi and D. Nayeri, Removal of fluoride from water using coagulation-flocculation process: a comparative study, Desalin. Water Treat., 2020, 180, 265–270 CrossRef CAS.
- M. Belkacem, S. Bekhti and K. Bensadok, Groundwater treatment by reverse osmosis, Desalination, 2007, 206, 100–106 CrossRef CAS.
- S. J. Wimalawansa, Purification of contaminated water with reverse osmosis: effective solution of providing clean water for human needs in developing countries, Int. J. Emerging Technol. Adv. Eng., 2013, 3, 75–89 Search PubMed.
- P. Liu, Z. Wu, A. V. Abramova and G. Cravotto, Sonochemical processes for the degradation of antibiotics in aqueous solutions: a review, Ultrason. Sonochem., 2021, 74, 105566 CrossRef CAS PubMed.
- X. Song, C. Jo, L. Han and M. Zhou, Recent advance in microbial fuel cell reactor configuration and coupling technologies for removal of antibiotic pollutants, Curr. Opin. Electrochem., 2022, 31, 100833 CrossRef CAS.
- N. K. Raad, F. Farrokhi, S. A. Mousavi, P. Darvishi and A. Mahmoudi, Simultaneous power generation and sewage sludge stabilization using an air cathode-MFCs, Biomass Bioenergy, 2020, 140, 105642 CrossRef CAS.
- A. Mahmoudi, S. A. Mousavi and P. Darvishi, Effect of ammonium and COD concentrations on the performance of fixed-bed air-cathode microbial fuel cells treating reject water, Int. J. Hydrogen Energy, 2020, 45, 4887–4896 CrossRef CAS.
- F. Yu, C. Wang and J. Ma, Applications of graphene-modified electrodes in microbial fuel cells, Materials, 2016, 9, 807 CrossRef PubMed.
- Y. Guo, J. Wang, S. Shinde, X. Wang, Y. Li, Y. Dai, J. Ren, P. Zhang and X. Liu, Simultaneous wastewater treatment and energy harvesting in microbial fuel cells: an update on the biocatalysts, RSC Adv., 2020, 10, 25874–25887 RSC.
- H. Ilyas, I. Masih and E. D. van Hullebusch, Pharmaceuticals' removal by constructed wetlands: a critical evaluation and meta-analysis on performance, risk reduction, and role of physicochemical properties on removal mechanisms, J. Water Health, 2020, 18, 253–291 CrossRef PubMed.
- X.-L. Ling, Y.-Z. Wei, L.-M. Zou and S. Xu, Preparation and characterization of hydroxylated multi-walled carbon nanotubes, Colloids Surf., A, 2013, 421, 9–15 CrossRef CAS.
- M. Endo, T. Hayashi and Y.-A. Kim, Large-scale production of carbon nanotubes and their applications, Pure Appl. Chem., 2006, 78, 1703–1713 CrossRef CAS.
- J. Prasek, J. Drbohlavova, J. Chomoucka, J. Hubalek, O. Jasek, V. Adam and R. Kizek, Methods for carbon nanotubes synthesis, J. Mater. Chem., 2011, 21, 15872–15884 RSC.
- A. R. Harutyunyan, G. Chen, T. M. Paronyan, E. M. Pigos, O. A. Kuznetsov, K. Hewaparakrama, S. M. Kim, D. Zakharov, E. A. Stach and G. U. Sumanasekera, Preferential growth of single-walled carbon nanotubes with metallic conductivity, Science, 2009, 326, 116–120 CrossRef CAS PubMed.
-
P. P. R. A. Donald, Thomson/Brooks–Cole, Sci. Emerg. Mater., Australia, 4th Edition, 2003 Search PubMed.
- M. L. Terranova, V. Sessa and M. Rossi, The world of carbon nanotubes: an overview of CVD growth methodologies, Chem. Vap. Deposition, 2006, 12, 315–325 CrossRef CAS.
- A. Thess, R. Lee, P. Nikolaev, H. Dai, P. Petit, J. Robert, C. Xu, Y. H. Lee, S. G. Kim and A. G. Rinzler, Crystalline ropes of metallic carbon nanotubes, Science, 1996, 273, 483–487 CrossRef CAS PubMed.
-
J. P. Gore and A. Sane, Flame synthesis of carbon nanotubes, Carbon Nanotubes-Synthesis, Characterization, Applications, 2011, vol. 1, p. 16801 Search PubMed.
- Y. Saito, K. Nishikubo, K. Kawabata and T. Matsumoto, Carbon nanocapsules and single-layered nanotubes produced with platinum-group metals (Ru, Rh, Pd, Os, Ir, Pt) by arc discharge, J. Appl. Phys., 1996, 80, 3062–3067 CrossRef CAS.
- S. Shokry, A. El Morsi, M. Sabaa, R. Mohamed and H. El Sorogy, Study of the productivity of MWCNT over Fe and Fe–Co catalysts supported on SiO2, Al2O3 and MgO, Egypt. J. Pet., 2014, 23, 183–189 CrossRef.
- N. Anzar, R. Hasan, M. Tyagi, N. Yadav and J. Narang, Carbon nanotube-A review on Synthesis, Properties and plethora of applications in the field of biomedical science, Sensors International, 2020, 1, 100003 CrossRef.
- V. Datsyuk, M. Kalyva, K. Papagelis, J. Parthenios, D. Tasis, A. Siokou, I. Kallitsis and C. Galiotis, Chemical oxidation of multiwalled carbon nanotubes, Carbon, 2008, 46, 833–840 CrossRef CAS.
-
L. Chen, H. Xie and W. Yu, Functionalization Methods of Carbon Nanotubes and Its Applications, in Carbon Nanotubes, ed. J. M. Marulanda, IntechOpen, Rijeka, 2011, ch. 9, pp. 213–232 Search PubMed.
- Y. Bai, I. S. Park, S. J. Lee, T. S. Bae, F. Watari, M. Uo and M. H. Lee, Aqueous dispersion of surfactant-modified multiwalled carbon nanotubes and their application as an antibacterial agent, Carbon, 2011, 49, 3663–3671 CrossRef CAS.
- P. Bilalis, D. Katsigiannopoulos, A. Avgeropoulos and G. Sakellariou, Non-covalent functionalization of carbon nanotubes with polymers, RSC Adv., 2014, 4, 2911–2934 RSC.
- N. G. Sahoo, S. Rana, J. W. Cho, L. Li and S. H. Chan, Polymer nanocomposites based on functionalized carbon nanotubes, Prog. Polym. Sci., 2010, 35, 837–867 CrossRef CAS.
- B. Sarkar, S. Mandal, Y. F. Tsang, P. Kumar, K.-H. Kim and Y. S. Ok, Designer carbon nanotubes for contaminant removal in water and wastewater: a critical review, Sci. Total Environ., 2018, 612, 561–581 CrossRef CAS PubMed.
- Z. Jin, X. Sun, G. Xu, S. H. Goh and W. Ji, Nonlinear optical properties of some polymer/multi-walled carbon nanotube composites, Chem. Phys. Lett., 2000, 318, 505–510 CrossRef CAS.
- J.-B. Baek, C. B. Lyons and L.-S. Tan, Grafting of vapor-grown carbon nanofibers via in-situ polycondensation of 3-phenoxybenzoic acid in poly (phosphoric acid), Macromolecules, 2004, 37, 8278–8285 CrossRef CAS.
- M. U. Khan, K. R. Reddy, T. Snguanwongchai, E. Haque and V. G. Gomes, Polymer brush synthesis on surface modified carbon nanotubes via in situ emulsion polymerization, Colloid Polym. Sci., 2016, 294, 1599–1610 CrossRef CAS.
- P.-C. Ma, N. A. Siddiqui, G. Marom and J.-K. Kim, Dispersion and functionalization of carbon nanotubes for polymer-based nanocomposites: a review, Composites, Part A, 2010, 41, 1345–1367 CrossRef.
- K. A. Wepasnick, B. A. Smith, K. E. Schrote, H. K. Wilson, S. R. Diegelmann and D. H. Fairbrother, Surface and structural characterization of multi-walled carbon nanotubes following different oxidative treatments, Carbon, 2011, 49, 24–36 CrossRef CAS.
- Y. Geng, M. Y. Liu, J. Li, X. M. Shi and J. K. Kim, Effects of surfactant treatment on mechanical and electrical properties of CNT/epoxy nanocomposites, Composites, Part A, 2008, 39, 1876–1883 CrossRef.
- D. Claves, H. Li, M. Dubois and Y. Ksari, An unusual weak bonding mode of fluorine to single-walled carbon nanotubes, Carbon, 2009, 47, 2557–2562 CrossRef CAS.
- A. Tressaud, E. Durand and C. Labrugère, Surface modification of several carbon-based materials: comparison between CF4 rf plasma and direct F2-gas fluorination routes, J. Fluorine Chem., 2004, 125, 1639–1648 CrossRef CAS.
- J. Zhang, H. Zou, Q. Qing, Y. Yang, Q. Li, Z. Liu, X. Guo and Z. Du, Effect of chemical oxidation on the structure of single-walled carbon nanotubes, J. Phys. Chem. B, 2003, 107, 3712–3718 CrossRef CAS.
- W. Yang, Y. Wang, J. Li and X. Yang, Polymer wrapping technique: an effective route to prepare Pt nanoflower/carbon nanotube hybrids and application in oxygen reduction, Energy Environ. Sci., 2010, 3, 144–149 RSC.
- L. Li, L. Meng, X. Zhang, C. Fu and Q. Lu, The ionic liquid-associated synthesis of a cellulose/SWCNT complex and its remarkable biocompatibility, J. Mater. Chem., 2009, 19, 3612–3617 RSC.
- H.-X. Wu, X.-Q. Qiu, W.-M. Cao, Y.-H. Lin, R.-F. Cai and S.-X. Qian, Polymer-wrapped multiwalled carbon nanotubes synthesized via microwave-assisted in situ emulsion polymerization and their optical limiting properties, Carbon, 2007, 45, 2866–2872 CrossRef CAS.
-
L. Dai and J. Sun, Mechanical properties of carbon nanotubes-polymer composites, Carbon nanotubes–current progress of their polymer composites, 2016, pp. 155–194 Search PubMed.
- M. Shanbedi, S. Z. Heris and A. Maskooki, Experimental investigation of stability and thermophysical properties of carbon nanotubes suspension in the presence of different surfactants, J. Therm. Anal. Calorim., 2015, 120, 1193–1201 CrossRef CAS.
- W. Orellana and S. O. Vásquez, Endohedral terthiophene in zigzag carbon nanotubes: Density functional calculations, Phys. Rev. B: Condens. Matter Mater. Phys., 2006, 74, 125419 CrossRef.
- R. Marega and D. Bonifazi, Filling carbon nanotubes for nanobiotechnological applications, New J. Chem., 2014, 38, 22–27 RSC.
- S. A. Mousavi and H. Janjani, Antibiotics adsorption from aqueous solutions using carbon nanotubes: a systematic review, Toxin Rev., 2018, 87–98 Search PubMed.
- M. Ahmadi, H. R. Motlagh, N. Jaafarzadeh, A. Mostoufi, R. Saeedi, G. Barzegar and S. Jorfi, Enhanced photocatalytic degradation of tetracycline and real pharmaceutical wastewater using MWCNT/TiO2 nano-composite, J. Environ. Manage., 2017, 186, 55–63 CrossRef CAS PubMed.
- Z.-y. Wang, L. Duan, D.-q. Zhu and W. Chen, Effects of Cu (II) and Ni (II) ions on adsorption of tetracycline to functionalized carbon nanotubes, J. Zhejiang Univ., Sci., A, 2014, 15, 653–661 CrossRef CAS.
- D. Balarak, Y. Mahdavi and F. K. Mostafapour, Application of alumina-coated carbon nanotubes in removal of tetracycline from aqueous solution, J. Pharm. Res. Int., 2016, 1–11 Search PubMed.
- M. Bazregari and N. Farhadian, Improvement of amoxicillin removal from aqueous environment by applying functionalized carbon nanotube, Environ. Technol., 2018, 39, 2231–2242 CrossRef CAS PubMed.
-
J. Drenth, Principles of protein X-ray crystallography, Springer. Sci. Bus. Media, 2007 Search PubMed.
-
H. Butt, K. Graf and M. Kappl, Physics and Chemistry of Interfaces, Wiley-VCH, Weinheim, 2003 Search PubMed.
- S. Chen, Z. Zang, S. Zhang, G. Ouyang and R. Han, Preparation of MIL-100 (Fe) and multi-walled carbon nanotubes nanocomposite with high adsorption capacity towards Oxytetracycline from solution, J. Environ. Chem. Eng., 2021, 9, 104780 CrossRef CAS.
- N. Sharifpour, F. M. Moghaddam, G. Mardani and M. Malakootian, Evaluation of the activated carbon coated with multiwalled carbon nanotubes in removal of ciprofloxacin from aqueous solutions, Appl. Water Sci., 2020, 10, 1–17 CrossRef.
- R. Shokoohi, A. Dargahi, R. A. Gilan, H. Z. Nasab, D. Zeynalzadeh and M. M. Mahmoudi, Magnetic multi-walled carbon nanotube as effective adsorbent for ciprofloxacin (CIP) removal from aqueous solutions: isotherm and kinetics studies, Int. J. Chem. React. Eng., 2020, 18, 1–15 Search PubMed.
- Y. Sun, J. Tian, L. Wang, H. Yan, F. Qiao and X. Qiao, One pot synthesis of magnetic graphene/carbon nanotube composites as magnetic dispersive solid-phase extraction adsorbent for rapid determination of oxytetracycline in sewage water, J. Chromatogr. A, 2015, 1422, 53–59 CrossRef CAS PubMed.
- S. Egbuna, C. Mbah and J. Okoye, Optimal process parameters for the adsorption of methylene blue on thermally activated Enugu white clay as a local adsorbent, International Journal of Science and Engineering Invention, 2015, 4, 32–42 Search PubMed.
- D. Nayeri and S. A. Mousavi, Dye removal from water and wastewater by nanosized metal oxides-modified activated carbon: a review on recent researches, J. Environ. Health Sci. Eng., 2020, 18, 1671–1689 CrossRef CAS PubMed.
- B. N. Estevinho, I. Martins, N. Ratola, A. Alves and L. Santos, Removal of 2, 4-dichlorophenol and pentachlorophenol from waters by sorption using coal fly ash from a Portuguese thermal power plant, J. Hazard. Mater., 2007, 143, 535–540 CrossRef CAS PubMed.
- S. Agnihotri, J. P. Mota, M. Rostam-Abadi and M. J. Rood, Structural characterization of single-walled carbon nanotube bundles by experiment and molecular simulation, Langmuir, 2005, 21, 896–904 CrossRef CAS PubMed.
- S. Kang, M. Herzberg, D. F. Rodrigues and M. Elimelech, Antibacterial effects of carbon nanotubes: size does matter!, Langmuir, 2008, 24, 6409–6413 CrossRef CAS PubMed.
- F. Yu, Y. Li, S. Han and J. Ma, Adsorptive removal of antibiotics from aqueous solution using carbon materials, Chemosphere, 2016, 153, 365–385 CrossRef CAS PubMed.
- Q. Cong, X. Yuan and J. Qu, A review on the removal of antibiotics by carbon nanotubes, Water Sci. Technol., 2013, 68, 1679–1687 CrossRef CAS PubMed.
- X. Tan, Y. Liu, G. Zeng, X. Wang, X. Hu, Y. Gu and Z. Yang, Application of biochar for the removal of pollutants from aqueous solutions, Chemosphere, 2015, 125, 70–85 CrossRef CAS PubMed.
- M. B. Ahmed, J. L. Zhou, H. H. Ngo and W. Guo, Adsorptive removal of antibiotics from water and wastewater: progress and challenges, Sci. Total Environ., 2015, 532, 112–126 CrossRef CAS PubMed.
- H. Kim, Y. S. Hwang and V. K. Sharma, Adsorption of antibiotics and iopromide onto single-walled and multi-walled carbon nanotubes, Chem. Eng. J., 2014, 255, 23–27 CrossRef CAS.
- B. Pan and B. Xing, Adsorption mechanisms of organic chemicals on carbon nanotubes, Environ. Sci. Technol., 2008, 42, 9005–9013 CrossRef CAS PubMed.
- M. Anggraini, A. Kurniawan, L. K. Ong, M. A. Martin, J.-C. Liu, F. E. Soetaredjo, N. Indraswati and S. Ismadji, Antibiotic detoxification from synthetic and real effluents using a novel MTAB surfactant-montmorillonite (organoclay) sorbent, RSC Adv., 2014, 4, 16298–16311 RSC.
- W. Chen, D. Zhu, S. Zheng and W. Chen, Catalytic effects of functionalized carbon nanotubes on dehydrochlorination of 1, 1, 2, 2-tetrachloroethane, Environ. Sci. Technol., 2014, 48, 3856–3863 CrossRef CAS PubMed.
- N. Soliman and A. Moustafa, Industrial solid waste for heavy metals adsorption features and challenges; a review, J. Mater. Res. Technol., 2020, 9, 10235–10253 CrossRef CAS.
- E. Sabio, E. González, J. González, C. González-García, A. Ramiro and J. Ganan, Thermal regeneration of activated carbon saturated with p-nitrophenol, Carbon, 2004, 42, 2285–2293 CrossRef CAS.
- H. Tamon, T. Saito, M. Kishimura, M. Okazaki and R. Toei, Solvent regeneration of spent activated carbon in wastewater treatment, J. Chem. Eng. Jpn., 1990, 23, 426–432 CrossRef CAS.
- F. Wang, W. Sun, W. Pan and N. Xu, Adsorption of sulfamethoxazole and 17β-estradiol by carbon nanotubes/CoFe2O4 composites, Chem. Eng. J., 2015, 274, 17–29 CrossRef CAS.
- M. Moore, Do nanoparticles present ecotoxicological risks for the health of the aquatic environment?, Environ. Int., 2006, 32, 967–976 CrossRef CAS PubMed.
- S. M. Taghavi, M. Momenpour, M. Azarian, M. Ahmadian, F. Souri, S. A. Taghavi, M. Sadeghain and M. Karchani, Effects of nanoparticles on the environment and outdoor workplaces, Electron. Physician, 2013, 5, 706 Search PubMed.
- N. Musee, Nanowastes and the environment: Potential new waste management paradigm, Environ. Int., 2011, 37, 112–128 CrossRef CAS PubMed.
- K. Tan and B. Hameed, Insight into the adsorption kinetics models for the removal of contaminants from aqueous solutions, J. Taiwan Inst. Chem. Eng., 2017, 74, 25–48 CrossRef CAS.
- H. Qiu, L. Lv, B.-c. Pan, Q.-j. Zhang, W.-m. Zhang and Q.-x. Zhang, Critical review in adsorption kinetic models, J. Zhejiang Univ., Sci., A, 2009, 10, 716–724 CrossRef CAS.
- M. El-Sadaawy and O. Abdelwahab, Adsorptive removal of nickel from aqueous solutions by activated carbons from doum seed (Hyphaenethebaica) coat, Alexandria Eng. J., 2014, 53, 399–408 CrossRef.
- M. C. Somasekhara Reddy and V. Nirmala, Bengal Gram Seed Husk As An Adsorbent For The Removal Of Dye From Aqueous Solutions–Batch Studies, Arabian J. Chem., 2014, 1695–1706 Search PubMed.
- K. Yaghmaeian, R. K. Mashizi, S. Nasseri, A. H. Mahvi, M. Alimohammadi and S. Nazmara, Removal of inorganic mercury from aquatic environments by multi-walled carbon nanotubes, J. Environ. Health Sci. Eng., 2015, 13, 1–9 CrossRef CAS PubMed.
- K. Foo and B. Hameed, Preparation, characterization and evaluation of adsorptive properties of orange peel based activated carbon via microwave induced K2CO3 activation, Bioresour. Technol., 2012, 104, 679–686 CrossRef CAS PubMed.
- A. K. Prajapati and M. K. Mondal, Comprehensive kinetic and mass transfer modeling for methylene blue dye adsorption onto CuO nanoparticles loaded on nanoporous activated carbon prepared from waste coconut shell, J. Mol. Liq., 2020, 307, 112949 CrossRef CAS.
- S. Nethaji, A. Sivasamy and A. Mandal, Adsorption isotherms, kinetics and mechanism for the adsorption of cationic and anionic dyes onto carbonaceous particles prepared from Juglans regia shell biomass, Int. J. Environ. Sci. Technol., 2013, 10, 231–242 CrossRef CAS.
- P. Nanta, K. Kasemwong and W. Skolpap, Isotherm and kinetic modeling on superparamagnetic nanoparticles adsorption of polysaccharide, J. Environ. Chem. Eng., 2018, 6, 794–802 CrossRef CAS.
- E. Altıntıg, H. Altundag, M. Tuzen and A. Sarı, Effective removal of methylene blue from aqueous solutions using magnetic loaded activated carbon as novel adsorbent, Chem. Eng. Res. Des., 2017, 122, 151–163 CrossRef.
- M. I. El-Khaiary, Least-squares regression of adsorption equilibrium data: comparing the options, J. Hazard. Mater., 2008, 158, 73–87 CrossRef CAS PubMed.
- R. F. Fard, M. E. K. Sar, M. Fahiminia, N. Mirzaei, N. Yousefi, H. J. Mansoorian, N. Khanjani, S. Rezaei and S. K. Ghadiri, Efficiency of multi walled carbon nanotubes for removing Direct Blue 71 from aqueous solutions, Eurasian J. Anal. Chem., 2018, 13, 123–146 Search PubMed.
- Y. Bao and G. Zhang, Study of adsorption characteristics of methylene blue onto activated carbon made by Salix psammophila, Energy Procedia, 2012, 16, 1141–1146 CrossRef CAS.
- A. R. Kaveeshwar, S. K. Ponnusamy, E. D. Revellame, D. D. Gang, M. E. Zappi and R. Subramaniam, Pecan shell based activated carbon for removal of iron (II) from fracking wastewater: adsorption kinetics, isotherm and thermodynamic studies, Process Saf. Environ. Prot., 2018, 114, 107–122 CrossRef CAS.
- A. E. Pirbazari, E. Saberikhah and S. H. Kozani, Fe3O4–wheat straw: preparation, characterization and its application for methylene blue adsorption, Water Resour. Ind., 2014, 7, 23–37 CrossRef.
- S. A. Chaudhry, T. A. Khan and I. Ali, Zirconium oxide-coated sand based batch and column adsorptive removal of arsenic from water: isotherm, kinetic and thermodynamic studies, Egypt. J. Pet., 2017, 26, 553–563 CrossRef.
- R. Han, J. Zhang, P. Han, Y. Wang, Z. Zhao and M. Tang, Study of equilibrium, kinetic and thermodynamic parameters about methylene blue adsorption onto natural zeolite, Chem. Eng. J., 2009, 145, 496–504 CrossRef CAS.
- H. You, J. Chen, C. Yang and L. Xu, Selective removal of cationic dye from aqueous solution by low-cost adsorbent using phytic acid modified wheat straw, Colloids Surf., A, 2016, 509, 91–98 CrossRef CAS.
- A. Nasrullah, A. Bhat, A. Naeem, M. H. Isa and M. Danish, High surface area mesoporous activated carbon-alginate beads for efficient removal of methylene blue, Int. J. Biol. Macromol., 2018, 107, 1792–1799 CrossRef CAS PubMed.
- V. Njoku, K. Foo, M. Asif and B. Hameed, Preparation of activated carbons from rambutan (Nephelium lappaceum) peel by microwave-induced KOH activation for acid yellow 17 dye adsorption, Chem. Eng. J., 2014, 250, 198–204 CrossRef CAS.
- M. Dubinin, The potential theory of adsorption of gases and vapors for adsorbents with energetically nonuniform surfaces, Chem. Rev., 1960, 60, 235–241 CrossRef CAS.
|
This journal is © The Royal Society of Chemistry 2023 |