DOI:
10.1039/D2TB00683A
(Review Article)
J. Mater. Chem. B, 2022,
10, 7384-7396
Recent advances in biomedical applications of bacterial outer membrane vesicles
Received
28th March 2022
, Accepted 14th June 2022
First published on 16th June 2022
Abstract
Nanoscale and non-self-replicating outer membrane vesicles (OMVs) are naturally secreted by some bacteria with their structures and compositions similar to that of the outer membrane of parental bacteria. With some specific bacterial physiological characteristics, e.g., immunomodulations and intercellular communications, OMVs have been intensively studied and extensively used and engineered as vaccines, immunotherapeutic drugs, anti-bacterial agents, and drug delivery carriers. In this review, we describe the extraction, characterization, and functionalization of OMVs with emphasis on the latest progress and prospects in biomedical applications.
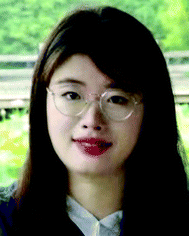
Chen Haiyan
| Haiyan Chen is currently a postgraduate at Soochow University. In 2019 she obtained her bachelor's degree in science also at Soochow University. Chen's research focuses on the bacterial outer membrane vesicle-based brain-targeted drug delivery systems for brain metastases treatment, and related research findings have been published in Advanced Science. |
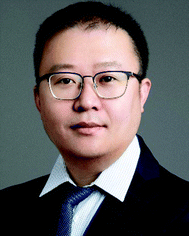
Liang Han
| Liang Han is a professor at Soochow University. He obtained his PhD degree at Fudan University in 2012 under the supervision of Prof. Chen Jiang. From 2012 to 2015, he worked at Yale School of Medicine as a postdoctoral associate. Prof. Han's research focuses on overcoming the blood–brain barrier, and designing and constructing brain-targeting drug delivery systems for therapy of brain diseases including breast cancer brain metastases and Alzheimer's disease. He has published over 40 peer-reviewed papers in journals, including Advanced Materials, Advanced Science, Advanced Functional Materials, ACS Nano, Small, Biomaterials, Journal of Controlled Release, etc. |
1. Introduction
Outer membrane vesicles (OMVs) are natural spherical vesicles that are mainly produced by Gram-negative bacteria.1–3 OMVs are 20–250 nm in diameter and are composed of a bilayer lipid, outer membrane proteins, lipopolysaccharide (LPS), adhesins, and encapsulated cargoes (e.g., DNA, enzymes, toxins, and periplasmic proteins (Fig. 1)),2,4 which are all inherited from parental bacteria. Although the production mechanisms have not been elucidated, lipoproteins, misfolded proteins (and envelope components), and lipids are thought to be involved in the OMV biosynthesis.2 Presumably, OMVs are released through (1) the detachment of the outer membrane from peptidoglycan via the disintegration of the connective lipoproteins,5 (2) the disrupted crosslinks between the outer membrane and peptidoglycan via accumulated misfolded proteins and envelope components,2 and (3) the enhanced membrane fluidity and curvature by enriched LPS, phospholipids and LPS-associated molecules,2 respectively.
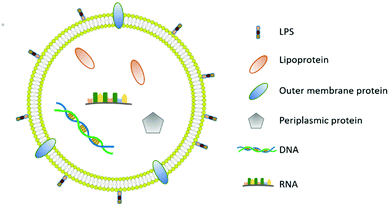 |
| Fig. 1 OMVs are vesicles composed of a lipid bilayer, bacterial proteins, lipids, multiple PAMPs, and other molecules. | |
OMVs play significant roles in bacterial physiological processes, e.g., intercellular communications and stress responses. OMVs mediate communications with host cells and bacteria by transporting virulence factors and DNA.6,7 For example, OMVs from Moraxella catarrhalis deliver outer-membrane-bound super antigen Moraxella immunoglobulin D-binding protein into B cells to activate Toll-like receptor 9 signalling.8 In addition, bacteria clean up misfolded proteins and environmental harmful components by releasing OMVs (stress responses) for bacterial survival.9
Based on the inherent bacterial components and characteristics, OMVs have been widely developed for biomedical applications. First, OMVs can be efficiently recognized and internalized by immune cells through their robust pathogen-associated molecular patterns (PAMPs) including LPS and lipoprotein.2 Thus, OMVs can produce strong humoral and cellular immunity,10 leading to the direct use or engineering of OMVs as vaccines. In addition, OMVs can be used as a bacterial simulant to competitively inhibit the adhesion of bacteria to host cells via adhesins.11 Recently, OMVs were explored for mediating antitumor therapy because of the induced production of interferon-γ (IFN-γ) and subsequent long-term antitumor immune responses.12 Finally, OMVs can be used as efficient drug delivery carriers. In this case, drug can be covalently connected to the vesicle surface or encapsulated in the lumen for targeted drug delivery to specific sites, e.g., tumor and brain.13,14 Therefore, OMVs have shown great potential in biomedical fields.
In this review, we introduce recent advances regarding extractions, functionalization, and characterization of OMVs with emphasis on the latest progresses and prospects on biomedical applications. Based on the discussion in this review, we hope that the production and transformation of OMVs can be upgraded and they can be optimized as vaccines, immunotherapeutic drugs, anti-bacterial agents, and drug delivery carriers for broader clinical applications.
2. Extraction of OMVs
OMVs are usually isolated from supernatants of bacterial cultures unless otherwise specified. For various applications, different extraction methods (e.g., ultrafiltration plus centrifugation, immunoaffinity chromatography, and detergent-based extraction) have been reported and can be chosen to successfully collect highly purified OMVs with specific characteristics (Fig. 2a).
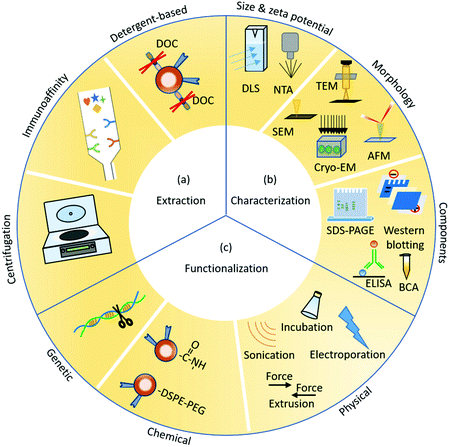 |
| Fig. 2 The extraction, characterization and functionalization of OMVs. | |
2.1. Ultrafiltration plus centrifugation
Ultrafiltration plus centrifugation is the most commonly used method for separating and extracting OMVs.15 First, the supernatants of bacterial cultures were acquired by centrifugation at 4000–6000 × g for 10 minutes.12,16 Then, residual large impurities (e.g., bacteria and/or bacterial debris) in the supernatant can be removed by passing through 0.22 or 0.45 μm filters. Using 0.22 μm filters may reduce the yield of OMVs by blocking the passage of OMVs larger than 220 nm. Then the supernatant was ultrafiltered to concentrate OMVs. After the ultrafiltration, OMVs can be obtained by ultracentrifugation, with the centrifugal force ranging from 38
400 × g to 150
000 × g17,18 and the time ranging from 1 h to 4 h (ultrafiltration plus centrifugation method).19,20 OMVs can be obtained by only ultracentrifugation without ultrafiltration as well (centrifugation method). Reimer et al. compared these two OMV isolation methods, ultracentrifugation with or without ultrafiltration, with respect to the final OMV quantities, size distributions, and morphologies using a hypervesiculating Escherichia coli K12 ΔtolA mutant. For ΔtolA OMVs extracted by ultrafiltration plus ultracentrifugation, the average vesicle size and the yield were 125.2 nm and 1945.6 particles per mL, while that for ultracentrifugation-extracted ΔtolA OMVs were 116.7 nm and 4870.7 particles per mL, respectively. The reason for these differences may be that ultracentrifugation promotes aggregation of vesicles due to repeated pelleting steps, while ultrafiltration prefers to select smaller and more uniform particles.15
2.2. Immunoaffinity chromatography extraction
Immunoaffinity chromatography extraction is based on the specific interaction of target molecules and immobilized ligands (such as the interaction between antigen and antibody) to separate specific molecules in a mixture or remove a specific component from reaction systems.21 After bioengineering, the surface of OMVs can bear a variety of specific tags or ligands that can be used as markers for immunoaffinity chromatographic isolation. For example, OMVs with a 6x His-tag fused to outer membrane protein A can be obtained directly from the culture medium by affinity chromatography.22
2.3. Detergent-based extraction for attenuated OMVs
Detergent-based attenuated OMVs (dOMVs) are traditionally prepared via detergent-based-extraction techniques to remove endotoxins and improve biocompatibility. Detergents, such as deoxycholate (DOC), which have a high affinity for hydrophobic components such as LPS lipid A and thus can replace LPS to form vesicles, have been used to extract dOMVs from bacteria themselves.23 First, precipitated bacteria (after centrifugation at 5000 × g at 4 °C for 20 min) are resuspended in PBS, homogenized by sonication in an ice water bath, and centrifuged at 2900 × g at 4 °C. The precipitate is resuspended in buffer with DOC to form dOMVs and centrifuged at 20
000 × g at 4 °C to remove the precipitated cell debris. The addition of DOC replaced LPS to stabilize dOMVs. Then, dOMVs in the supernatant are further precipitated at 125
000 × g at 4 °C to finally obtain the dOMV pellets.14
Lysozyme can also be used to prepare attenuated OMVs. Lysozyme has a high affinity with LPS, and the complex formed by lysozyme and LPS can reduce the LPS-mediated inflammatory response.24 Therefore, OMVs can be detoxified after incubation with lysozyme.25 Although these attenuated OMVs may have improved biocompatibility, these OMV preparation approaches may also result in the loss of lipoproteins and other compositions and further weaken the adjuvant properties of OMVs.26
2.4. Discussion
There are some problems to be addressed with regard to OMV extraction. The compositions of OMVs are susceptible to various methods, e.g., detergents will remove the lipoprotein and LPS in OMVs while other mentioned methods do not,26 so they are supposed to be further investigated. Besides, the extraction methods require optimization to improve the OMV yield for better clinical translation. Despite the markedly increased yield by DOC, the stability of OMVs declines a lot simultaneously for the lack of phospholipid.27 Additionally, in consideration of OMV biosafety, nonpathogenic bacteria are more recommended to be the source of OMVs.
3. Characterization of OMVs
3.1. Size-based characterization
OMVs are nanoscale and spherical vesicles. Dynamic light scattering (DLS) analysis is often used to simultaneously measure the hydrodynamic diameter, size distribution and zeta potential of extracted or engineered OMVs. For OMV size characterization, DLS analysis has the advantages of high accuracy, rapidity and repeatability. Grande et al. performed DLS analysis and determined that the OMVs from the Helicobacter pylori planktonic phase had an average diameter of 123.7 nm, the polydispersity index of 0.217, and a zeta potential of −25 mV.28
Nanoparticle tracking analysis (NTA) utilizes the characteristics of light scattering and Brownian motion of particles to characterize size information of particles in liquid suspensions. NTA can not only measure the size of OMVs, but also has the capacity to count the number of particles, which can be used to reflect the yield of OMVs.29,30 The mean hydrodynamic diameter of OMVs from Haemophilus influenzae was found between 80 and 100 nm by NTA,19 and that of OMVs from Escherichia coli strains was found in the range of 118–156 nm.31
It is worth noting that NTA can measure extremely small vesicles (∼50 nm).32 If the isolated OMVs contain extremely small impurities such as undissolved salt, medium components or cell debris carried in the bacterial culture medium, the measured size would be less accurate (Fig. 2b).15
3.2. Morphology-based characterization
The morphology of OMVs can be observed by various optical microscopes, such as transmission electron microscopy (TEM), scanning electron microscopy (SEM), cryo-electron microscopy (cryo-EM) and atomic force microscopy (AFM).33–36 TEM is the most commonly used method to analyze the morphology and structure of OMVs, which can provide high-resolution morphological, size and structural information. The typical spherical structure of OMVs can be observed through TEM images. OMVs can be used to encapsulate solid nanoparticles (NPs) constructed from polymers, e.g., poly(lactic-co-glycolic acid).37–39 Under TEM, OMV-coated NPs were found to present a core–shell structure with increased diameter compared with that of uncoated NPs.16 SEM can display the three-dimensional structure of OMVs. For example, the spherical budding OMVs out of rod-shaped Enterobacter cloacae can be observed through SEM.18 However, the resolution of SEM is lower than TEM. In addition, both TEM and SEM need the samples to be fixed and stained before observation, which may damage the morphology of OMVs. However, cryo-EM does not require additional sample processing, which can avoid morphological changes caused by dehydration and chemical fixation, preserving the original structure of OMVs.17 Cryo-EM can directly determine the morphology of OMVs and measure the size and total number of OMVs. Unlike the electron microscope, which needs to be operated in a high vacuum environment, AFM can use the probe to visualize OMVs in air or fluid in real time without any special treatment of the sample, minimize the impact of sample preparation on the original characteristics of OMVs, and obtain the exact three-dimensional structure information of the OMV surface.40 The disadvantage of AFM is that the imaging range is narrow, the speed is slow, and it is easily affected by the probe.41 According to different research goals, all these technologies can be used to better characterize the morphology of OMVs (Fig. 2b).
3.3. Composition-based characterization
The qualitative and quantitative analysis of protein in OMVs mainly include bicinchoninic acid (BCA) assay, Sodium Dodecyl Sulfate (SDS)-Polyacrylamide Gel Electrophoresis (PAGE), western blotting, enzyme-linked immunosorbent assay (ELISA) and mass spectrometry analysis, etc. For the LPS-mediated toxicity analysis of OMVs, photometry methods (e.g., colorimetric analysis and limulus reagent chromogenic endotoxin quantitative assay) and gel methods are usually adopted (Fig. 2b).
The BCA method was mainly used to determine the total amount of protein in OMVs, which can be used to measure the overall extraction efficiency.11,16 For example, according to the BCA measurement, the OMV yield of Haemophilus parasuis culture was 6.8 mg protein per liter of bacterial medium.20
OMVs can be qualitatively analyzed by SDS-PAGE gel electrophoresis to ensure the extraction of the desired OMVs.42 Kothary et al. utilized SDS-PAGE to analyze OMVs obtained from Cronobacter sakazakii, Cronobacter malonaticus, and Cronobacter turicensis, and showed the existence of approximately 11 major protein bands with the molecular weight ranging from 18 kDa to 100 kDa.43 After further analysis, these protein bands were identified as Outer membrane protein X, MltA-interacting protein, contaminated flagellin, Outer membrane protein A, Outer membrane protein C, Outer membrane protein E, Outer membrane protein F, conjugated plasmid transfer protein and OM autotransporter.
Western blotting and ELISA are mainly used to specify target proteins in OMVs.44 For example, Soltani et al. used western blotting analysis and demonstrated the expression of all three main protective immunogens (pertussis toxin, pertactin, and filamentous hemagglutinin) in Bordetella pertussis.45
Mass spectrometry can perform proteomic analysis of OMVs due to its high throughput, to reveal the biogenesis and pathophysiological functions of OMVs.46 Bhar et al. determined protein cargoes of Enterobacter cloacae OMVs by using shotgun mass spectrometry, obtaining the sequence of a total of 7179 different peptides, which mapped to 229 proteins of E. cloacae. 47 out of 229 proteins were membrane-bound and worked as molecule transporters, signal factors, and receptors. The second abundant class of proteins was enzymes (21 proteins), including 13 hydrolases.18
The inherent toxicity of OMVs mainly comes from LPS components. The OMV toxicity is often characterized by determining LPS content. At present, methods for measuring the LPS content are mainly divided into photometry and gel methods. Photometry methods include colorimetric analysis and limulus reagent chromogenic endotoxin quantitative assay, etc. The colorimetric assay determines LPS concentration by measuring 2-keto-3-deoxyoctonate, which forms the oligosaccharide core of LPS molecules.47 For example, through the colorimetric assay, the content of LPS in OMVs from Escherichia coli JC8031 was 0.35 nmol LPS μg−1 total protein.48 The principle of the limulus chromogenic endotoxin quantitative method is that LPS can react with an enzyme in Limulus amebocyte lysate to release yellow p-nitroaniline. The release of p-nitroaniline can be stopped by the addition of glacial acetic acid or SDS, and the concentration of the substrate can be calculated by measuring the absorbance at 545 nm.49 By using this method, the OMV endotoxin contents of Cystobacter velatus CBV34 and Sorangianeae specialties SBSR073 were found to be 0.75 EU mL−1 and 0.5 EU mL−1 respectively.49 The gel method utilizes SDS-PAGE to visualize LPS and to perform quantitative analysis by silver staining. For example, in OMVs of Neisseria meningitidis serogroup X,50 LPS was determined through monoclonal antibody and quantified by SDS-PAGE and silver staining. The percent of LPS in OMVs was from 2.1% to 4.5% in relation to the total amount of protein.
3.4. Discussion
There are several points that deserve attention. For size measurement, DLS can be utilized to detect the hydrodynamic diameter while an electron microscope is required to determine the true diameter. The compositions of OMVs are still uncertain, e.g., periplasmic proteins exist in OMVs by chance without a convincing mechanism,3 thus thorough analyses are needed. Moreover, not only LPS induces OMV toxicity, but also other virulence factors like lipoproteins are threatening. Further removal of these components will be conducive to enhancing the biosafety and broadening the biomedical applications of OMVs.
4. Functionalization of OMVs
OMVs have become a unique platform for biomedical applications due to the inherent biological characteristics of OMVs. Further functional modification can not only help overcome the toxicity problems of bacterial OMVs, but also enable fine control of OMV performance as required, greatly expanding the application of OMVs. This section introduces several major OMV functionalization methods, some of which rely on traditional physicochemical methods, while others rely on genetic engineering (Fig. 2c).
4.1. Physical functionalization
Physical functionalization is commonly used to load drugs into the OMVs lumen. Incubation with drugs during bacteria culturing or after extraction of OMVs is the easiest loading strategy. For example, Huang et al. physically loaded antibiotics into OMVs by directly adding drugs into the culture.51 However, this method often requires large quantities of drugs and has very high requirements for drug stability in the incubation environment. In addition, the physical drug loading by simple incubation is usually low.52 For example, the loading capacity of Gemcitabine by OMVs was only 2.79 ± 0.72% through simple incubation.53 Other physical methods, such as electroporation, sonication and extrusion, can also be applied in drug loading and OMV functionalization.54,55 Electroporation is usually used to load exogenous substances including therapeutic macromolecules and gold NPs into OMVs. Higher loading capacity, e.g., 11.68 ± 3.68% for the sonication method,53 15% for electroporation44 and 7.13% for extrusion,54 and other advantages endow these methods with wider applications than simple incubation.
In general, the methods of physical functionalization can be used for the loading of small molecules, but may be not efficient enough for macromolecules.
4.2. Chemical functionalization
Besides physical methods, chemical coupling reactions are also often used for OMV functionalization. The 1-ethyl-3-[3-dimethylaminopropyl]-carbodiimide hydrochloride/N-hydroxysuccinimide (EDC/NHS) reaction is one of the classical coupling reactions to achieve biological conjugation. This reaction is commonly used for OMV labeling. For example, OMVs were labelled by Cy7 through this reaction.56
OMVs can be functionalized with targeting ligands through 1,2-distearoyl-sn-glycero-3-phosphoethanolamine-N-polyethylene glycol (DSPE-PEG) to endow OMVs with the targeting function. DSPE-PEG could readily fuse into the lipid structure of OMVs because of the similar lipid nature of OMVs to that of DSPE. For example, OMVs from attenuated Salmonella were functionalized with DSPE-PEG and tumor-targeting RGD peptide for a tumor-targeting ability.54
These physical or chemical methods should be carefully selected according to the chemical and biological properties of the materials to be functionalized. The need of further purification often limits the application of physical and chemical methods in OMV functionalization.
4.3. Genetic engineering functionalization
With the help of recombinant DNA technology, genetically engineered Escherichia coli can be viewed as a micro-factory to efficiently produce OMVs with robust modifications.57–59 Compared with physical and chemical methods, genetic engineering methods have obvious advantages in OMV functionalization. First, this method is more environmentally friendly and cost-effective. Genetic modification of OMVs allows extremely high controllability and preciseness, and functionalized OMVs can be produced on a large scale in a simple bacterial culture process, which is economical and efficient. Second, genetic engineering does not require additional purification. Besides, genetic engineering enables the indicated placement of the desired modification materials, providing flexibility for specific applications. For example, Li et al. generated OMVs with expression of programmed death-1 (PD-1) ectodomain on their surface by fusing the coding region for the mouse PD-1 ectodomain with that of ClyA (an OMV surface protein).60 This modification did not affect the immunostimulatory abilities of OMVs. The surface-modified OMVs were able to bind with programmed death-L1 (PD-L1) on the tumor cell membrane, and to be further internalized by tumor cells, thereby blocking the inhibition of T cell proliferation by tumor cells.
Genetic engineering can also be applied to detoxify OMVs by knocking out the genes encoding toxic proteins. At present, modifying the genes responsible for LPS synthesis or LPS acyl chains and phosphate groups, e.g., msbA, msbB, lpxL1, and lpxM,12,61–65 is a common and general method to attenuate LPS-mediated endotoxicity.66 After mutation of the relA gene and spot gene, which regulate the expression of virulence factors in Salmonella typhimurium, the pathogenicity of the source bacteria decreased.67 The extracted OMVs from these engineered bacteria didn’t cause an obvious inflammatory response and systemic toxicity with normal biochemical indexes and histological tests after intravenous injection, indicating the biocompatibility of these OMVs.68
This detoxification by genetic engineering can remove LPS more efficiently than detergent-based methods with little effect on the immunogenicity of OMVs. For OMVs obtained from Neisseria meningitidis, the LPS content was found to be 24.3 ± 6.6% for wild-type OMVs, 2.8 ± 0.4% for dOMVs,26 and 0.011% for ΔmsbB Escherichia coli OMVs.60 The detoxification by genetic engineering may be better than other methods.
4.4. Discussion
Despite the significant effort devoted to functionalization of OMVs, there still exist certain problems. For physical functionalization, simple incubation is limited by low drug loading; other physical methods, e.g., electroporation, sonication and extrusion will enhance the drug loading but may alter the surface properties of OMVs. For chemical functionalization, the chemical linkage sites possibly conflict with the active sites, thus affecting the function of OMVs; besides, the density of surface-modified ligands is supposed to be adjusted for the most appropriate affinity between ligands and receptors, which is not only sufficient for targeted delivery of the drug, but also prevents the OMV entrapment due to a low degree of dissociation.69,70 The development of genetic detoxification methods may slow down due to different virulence genes of various bacteria. In summary, the functionalization methods of OMVs require further optimization.
5. OMV-related biomedical applications
OMVs are promising for various biomedical applications based on their various properties.
5.1. Vaccines
5.1.1. OMV vaccine.
Vaccines activate innate and adaptive immunity by triggering the immune system to produce strong pathogen-specific immunity in a certain period of time. Non-self-replicating OMVs carry a large amount of virulence factors of bacteria. Through lymphatic drainage and phagocytosis, OMVs reach lymph nodes, enter antigen presenting cells (APCs),71 and then activate the immune system, which can specifically remove the cells infected with related bacteria (Fig. 3a).66
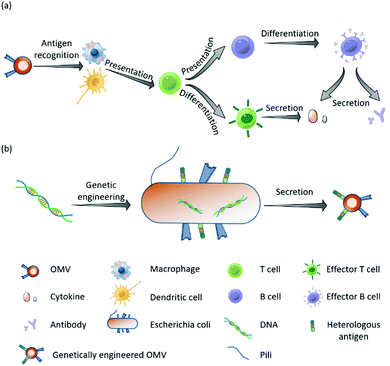 |
| Fig. 3 OMVs with surface PAMPs have been used as powerful vaccines. (a) OMVs can be recognized by APCs including macrophages and dendritic cells, causing adaptive immunity and activating cellular and humoral immunity with the production of cytokines and antibodies. (b) OMVs have been engineered to express heterologous antigens for mediating multiple immune responses. | |
At present, OMV vaccines have been developed from initial inactivated vaccines, live attenuated vaccines to the emerging genetic engineering vaccines and subunit vaccines. Among them, the most representative and successful vaccine is the attenuated OMV vaccine against meningitis.10 Attenuated OMV vaccines for the prevention of epidemic cerebrospinal meningitis caused by Neisseria meningitidis group B were approved as early as the 20th century. This OMV vaccine has been put into production and promotion in Cuba, Norway, New Zealand and China, etc., proving the practical and effective use of OMV vaccines.72,73 With reduced LPS and subsequent weakened toxicity, detoxified OMVs (e.g., DOC-based dOMVs) may show reduced immunogenicity and effectiveness.74 In order to overcome this limitation, OMV vaccines containing multiple membrane antigens by a non-detergent detoxification process have been evaluated under clinical research.75
OMV vaccines for Helicobacter pylori and Vibrio cholerae are already at an advanced stage of development. OMV vaccines for other bacteria such as Mycobacterium tuberculosis and Staphylococcus aureus are also in the development stage as well.76,77
Besides the retained immunogenicity of bacteria, OMV vaccines also have advantages such as non-self-replication, safety, effectiveness and practicality, endowing OMVs with the potential for being ideal vaccines.
5.1.2. OMV vaccine platform.
OMVs have also been engineered to express heterologous antigens for designing novel OMV vaccines. For example, the matrix protein M2 of influenza A virus has been successfully transfected into the exons of Escherichia coli. The newly-prepared OMV vaccine was shown to be able to resist the invasion of homotype influenza virus.78 In addition, heterologous OMV vaccines such as Neisseria meningitidis OMVs for Lyme disease,79Vibrio cholerae OMVs for enterotoxigenic Escherichia coli,80 and Salmonella typhimurium OMVs for pneumonia,81 and pulmonary tuberculosis82 are also in different stages of research and development (Fig. 3b).
5.2. Anti-bacterial agents
Bacterial infection is still one of the major causes of human illness and death.83,84 Due to the spread of resistant bacteria, the effectiveness of antibiotics in treating bacterial diseases is being challenged. OMVs from Pseudomonas aeruginosa could inhibit the growth of Escherichia coli, Bacillus subtilis, Enterococcus flatus and Staphylococcus aureus by autolysin and peptidoglycan hydrolases in Pseudomonas aeruginosa OMVs.85,86 Autolysins and peptidoglycan hydrolases are able to hydrolyze the N-acetyltericin-N-acetylglucosamine peptidoglycan bone frame and further degrade bacteria, exhibiting antibacterial properties without the development of drug resistance.87,88
Bacterial infections often begin with bacterial adhesion to host cells and tissues.89 Competitively inhibiting the initial adhesion of bacteria has become a promising approach to combat bacterial infection.90,91 This strategy can exert antibacterial effects without inducing bacterial resistance.92 A combination of anti-adhesion therapy with antibiotics can act synergistically to inhibit bacterial growth more effectively.93
The anti-adhesive agents have been developed increasingly. Some agents exercise the anti-adhesive function by targeting specific adhesins on the surface of bacteria. Some agents act as analogues of bacterial adhesins and competitively weaken the adhesion of bacteria.94,95 OMVs inherit multiple bacterial adhesins and thus can inhibit bacterial binding to the host with the efficiency higher than synthetic compounds. For example, Helicobacter pylori OMV-coated NPs have been reported to block the adhesion of Helicobacter pylori to gastric epithelial cells and mouse gastric tissues in a dose-dependent manner,11 suggesting the potential of OMVs to inhibit bacterial adhesion to host tissue and thus prevent bacterial infection (Fig. 4).
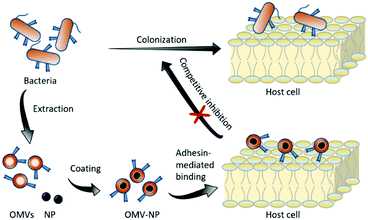 |
| Fig. 4 OMVs competitively inhibit the adhesion of parental bacteria to host cells and tissues. | |
Genetic engineering can further make OMVs express specific adhesins and enhance the antibacterial effect.96 Moreover, with the advantage of loading drugs, OMVs can simultaneously inhibit adhesion and achieve targeted drug delivery for an enhanced curative effect.97,98 OMVs have great potential in the field of antibacterial agents.
5.3. Tumor immunotherapy
In recent years, with the rapid development of molecular biology and tumor immunology, immunotherapy has become one of the most potential tumor treatment methods. OMVs can induce the body to produce some inflammatory factors to enhance the innate protective immune response. In addition, there is an important relationship between tumor biology and chronic inflammation, endowing inflammation-targeted bacteria and related carriers with the ability to realize tumor immunotherapy.99 For example, the injection of partially attenuated bacteria was shown to be able to induce the body's spontaneous immune response to identify and kill tumors.100–102 However, live bacteria have severe safety issues because of a variety of pathogens and the strong self-replication ability, which limits related applications. With the advantages of non-self-replication, immunogenicity, biosafety, and tumor targeting, OMVs possess enormous potential to effectively and safely activate the immune response of the body.103
5.3.1. OMVs regulating tumor immunosuppressive microenvironments.
The highly immunosuppressive microenvironment in solid tumors often reduces the immunotherapy efficiency.104,105 Recently, Qing et al. realized the successful regulation of the tumor immunosuppressive microenvironment via stealth OMVs, which were covered by a calcium phosphate shell to avoid the toxicity and side effects of OMVs.106 When the covered OMVs reach tumor tissues, the slightly acidic tumor environment can dissolve the calcium phosphate shell to expose OMVs to promote the infiltration of cytotoxic T cells by upregulating immune activation-related genes and downregulating immune-suppressive genes. Meanwhile, the dissolution of the calcium phosphate shell neutralizes H+ in the tumor microenvironment, leading to the polarization of M2-type macrophages to M1-type macrophages. The transformed OMVs effectively inhibited the tumor growth via changing the immunosuppressive microenvironment and enhancing the effect of immune cells (e.g., macrophages) (Fig. 5a).
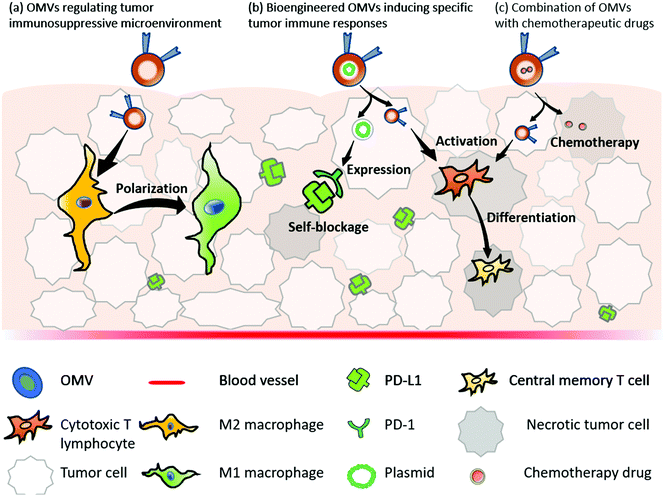 |
| Fig. 5 OMVs play a critical role in tumor immunotherapy. (a) OMVs effectively improve the tumor immunosuppressive microenvironment by promoting the polarization of M2-type macrophages to M1-type macrophages. (b) Bioengineered OMVs deliver PD-1 plasmid into tumor cells, and then the self-expressed PD-1 bind to the PD-L1 expressed by itself or adjacent tumor cells, inducing the self-blocking mediated tumor death. OMVs are able to differentiate CTLs into central memory T cells to produce long-lasting immunity as well. (c) Bacterial OMVs deliver chemotherapeutic drug to tumor cells and meanwhile promote CTL infiltration, achieving dual anti-tumor effects. | |
5.3.2. OMVs inducing specific tumor immune responses.
OMVs can be genetically engineered to express specific peptides or antigens to target tumor cells and to trigger specific tumor immune responses. Recently, Pan et al. developed LyP1-modified OMVs to deliver PD-1 plasmids.107 These PD-1 plasmid-loaded LyP1-modified OMVs were obtained by extracting OMVs from LyP1-expressed Escherichia coli and encapsulating the PD-1 plasmid. After intravenous injection, these engineered OMVs were efficiently accumulated in tumor tissues through the targeting ability of OMVs LyP1, leading to the expression of PD-1 in tumor cells. The expressed PD-1 by tumor cells was found to bind with tumoral PD-L1 to achieve self-blocking and reactivate cytotoxic T lymphocytes (CTLs) to eliminate tumor cells. In addition, OMV membrane proteins can recruit cytotoxic lymphocytes and natural killer cells (NK cells) to tumor tissues, to stimulate the secretion of IFN-γ and improve the antitumor activity of PD-1/PD-L1 self-blocking therapy. OMVs were also found to differentiate CTLs into central memory T cells to produce long-lasting immunity (Fig. 5b).
This strategy significantly improved the therapeutic effect of tumors, and provided a new idea for the development of tumor immunotherapy nanomedicines. OMVs can also induce specific anti-tumor immune responses by expressing antigens on the surface of OMVs.108 Tumor antigens were fused with OMV surface ClyA protein to mediate the transfer of tumor antigens to the OMV surface. These tumor antigens on the surface of OMVs were proved to be able to induce specific antitumor immunity mediated by T cells. In addition, different tumor antigens can be simultaneously displayed on the surface of OMVs with the plug and play technologies, leading to synergistic anti-tumor immune responses. This strategy can tailor the specific immune response according to different immunogenicity and provides the possibility for the development of various tumor-targeted vaccines in the future (Fig. 5b).
In addition to genetic engineering, membrane fusion can also contribute to inducing specific tumor immune effects. Chen et al. prepared a co-delivery nanovaccine composed of antigen and adjuvant separately from tumor cell membranes (TCMs) of autologous tumor tissue and Escherichia coli cytomembrane (Ems).109 Ems can induce dendritic cells to mature, thus activating splenetic T cells, finally eliciting robust innate and tumor-specific adaptive immune responses against tumors with the help of TCMs. Similarly, Li et al. designed a vesicular cancer nanovaccine with PLGA NPs as the core whose surface was coated with the fused bacterial OMVs and TCMs, namely BTs.110 The bacterial PAMPs in BTs could target dendritic cells, and promote the complete maturation of dendritic cells and antigen presentation by dendritic cells. TCMs in BTs provided tumor endogenous antigens, which can produce multi-antigenic anti-tumor immunity. The nanovaccine showed an excellent tumor prevention effect in vivo. Besides, Chen et al. fused melanoma cell membrane vesicles (CMVs) with attenuated Salmonella OMVs.111 The fused vesicles inherited and enhanced the immunogenicity of the two parental components, and worked as a preventive vaccine which could stimulate the immune system, trigger an anti-tumor immune response and inhibit tumor occurrence. In conclusion, the combination of tumor antigens and immunogenic bacterial membranes is a promising strategy to induce effective anti-tumor immunity.
5.3.3. Combination of OMVs with chemotherapeutic drugs to synergistically improve tumor immunotherapeutic efficacy.
The combination of OMVs and chemotherapeutic drugs can simultaneously initiate immunotherapy and chemotherapy, with the potential to synergistically improve the therapeutic effect.54,112 For example, OMVs of Escherichia coli BL21 (ΔmsbB) modified with mannose, and loaded with paclitaxel and Redd1 siRNA were designed by Guo et al. to regulate tumor cell growth and immune cell metabolism in tumor microenvironments (TME).113 Redd1 can promote tumor progression by inhibiting the mTOR signaling pathway, and the deficiency of Redd1 in tumor associated macrophages (TAMs) can prevent vessel leakiness and tumor metastasis.114–116 After injection, the chemotherapeutic drug paclitaxel was released first, directly killing tumor cells. The rest of the system regulated the metabolism of macrophages by Redd1 siRNA, promoting TAM repolarization and TME remolding. In addition, OMVs promoted the infiltration of immune cells in tumor tissues by stimulating innate immunity, playing a synergistic role in inhibiting tumor growth. In summary, TME remodeling, tumor immune activation and chemotherapeutic effects were found to contribute to the tumor therapy (Fig. 5c).113
Attenuated Salmonella OMVs were functionalized with polyethylene glycol and targeting peptides to deliver Tegafur-loaded nano-micelles. The drugs loaded inside micelles can simultaneously play chemotherapeutic and immunomodulatory effects by directly killing cancer cells and sensitizing cancer cells to CTLs, which synergically enhanced the therapeutic effects via immunotherapy and chemotherapy, providing a very useful reference for the clinical trial and transformation of OMVs for tumor treatment.54
5.4. Drug delivery
With the vesicle-like structure, OMVs are supposed to possess enormous drug delivery potential.117
The natural existence of OMVs may simplify the preparation and characterization technologies of OMV-based drug delivery systems (DDSs). With the property of nanoscale dimension, OMVs can be passively accumulated in tumor regions through the enhanced permeability and retention effect. OMVs also have an outstanding targeting ability for bacteria or inflammatory sites by modification with the surface functional proteins.11 The expression of specific targeting ligands on the surface of OMVs by genetic engineering can further strengthen the targeting effects.107 PAMPs on OMVs make OMVs more easily recognized and ingested by immune cells, which can be used for targeted drug delivery toward immune cells. Thus, OMVs hold great potential for drug delivery.
5.4.1. Tumor-targeted drug delivery.
Ning et al. developed αvβ3 integrin targeting and indocyanine green loaded OMVs to induce phototherapy against melanoma. The developed OMVs showed excellent stratum corneum penetration and melanoma targeting. Under near-infrared light stimulation, the developed OMVs induced robust photothermal and photodynamic responses, and activated TRAIL-induced apoptosis in diffused tumor cells, thus eliminating melanoma completely. This OMV-based DDS may have wide application prospects in cancer treatment (Fig. 6a).25
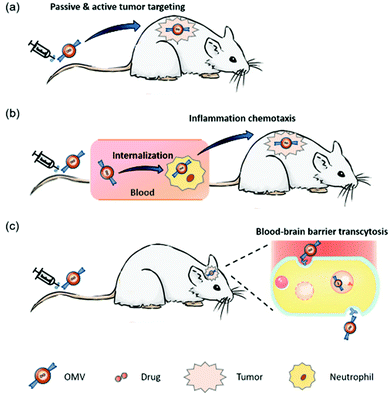 |
| Fig. 6 OMVs are promising drug delivery carriers for target sites, including (a) tumor tissues, (b) immune cells and (c) brain. | |
5.4.2. Immune cell-targeted drug delivery.
OMVs inherit the surface antigen from parental bacteria, so it is easy to activate an immune response, be recognized and ingested by immune cells, and realize targeted delivery. It has been reported that neutrophils have chemotactic effects and can spontaneously return to the inflammatory area, which has a profound and lasting effect and therefore is one of the ideal targets.118 The efficiency of DDSs is hampered by a variety of biological barriers, such as the mononuclear phagocyte system and vascular and interstitial barriers. To overcome these barriers, Li et al. reported a nano-pathogen (NPN) system that can target neutrophils and enhance the therapeutic effect in combination with photothermal therapy.119 The surface of NPNs encapsulated by OMVs carried the PAMPs of natural bacteria, which can be effectively recognized and internalized by neutrophils to promote their migration to the inflammatory area of the tumor. After penetrating the blood vessels to the deep tumor, the released NPNs played a role in killing tumor cells. The combination of photothermal therapy and NPNs can completely cure the tumor-bearing mice, showing strong potential for clinical transformation (Fig. 6b).
5.4.3. Brain-targeted drug delivery.
Brain-targeted DDSs are deeply troubled by the blood–brain barrier (BBB). The BBB strictly controls the material exchange between blood and brain, and hinders the delivery and accumulation of therapeutic drugs for intracranial diseases, making it difficult for brain-targeted drug therapies to achieve the expected effect.120,121
In recent years, many nanotechnology-related studies have been devoted to the effective intracerebral delivery of therapeutic drugs for brain diseases,122 such as the DDS based on low density lipoprotein receptor associated protein (LRP1)123 and prostate-specific membrane antigen (PSMA) that can mediate endocytosis,124 the DDS based on activating endothelial cell KATP to promote BBB permeability,125 the DDS that regulates endothelial Mfsd2a to antagonize its inhibition of transcytosis,126,127 and the DDS that avoids basal LRP1 mediated brain clearance, etc.128 However, the brain targeting efficiency is still not enough to achieve efficient treatment. Thus, new strategies urgently need to be explored.
Recently, a new brain-targeted DDS based on the bacterial OMVs has been reported. Inspired by the contribution of the bacterial outer membrane to Escherichia coli K1 (EC-K1) binding to and invasion of BBB endothelial cells in bacterial meningitis, Chen et al. utilized the BBB invasion ability of the EC-K1 outer membrane for brain-targeted drug delivery and constructed a biomimetic self-assembled nanoparticle with the surface featuring an LPS-free EC-K1 outer membrane. This biomimetic nanoengineering strategy endowed the loaded drugs with prolonged circulation, high intracranial accumulation more than most brain-targeted DDSs, and extremely high biocompatibility, which reveal the great potential of OMVs in brain and brain tumor DDS (Fig. 6c).14
5.5. Discussion
OMVs have been extensively utilized in biomedical applications, while there are many challenges in OMV development. (1) OMVs from various bacteria, e.g., pathogenic bacteria and symbiotic bacteria, may possess completely different functions. There is a need to clarify the forming and action mechanisms of different OMVs, which can endow these OMVs with more accurate usage for biomedical application. (2) When exploiting the immunogenicity of OMVs for treatment, e.g., vaccines and immunotherapeutic agents, how to induce a sufficient immune response but few potential side effects is an important issue to be addressed. It is necessary to avoid the excessive production or consumption of the immune response, because the former can induce inflammation and the latter will weaken the therapeutic effect. (3) Many OMV-related reports are still in the stage of laboratory research. This is supposed to enlarge the scale of samples for future clinical translation. (4) The preparation of OMVs should be optimized, e.g., enhancing the drug loading, maintaining the biological activity, conducting thorough safety tests and other operations, to ensure the consistency between batches and quality stability of products. (5) Additionally, the targeting efficiency of OMV-based brain-targeted DDS compared with parental bacteria demands more investigation.
6. Conclusions
In this review, the extraction, characterization, functionalization, detoxifications and the most recent application cases of bacterial OMVs are comprehensively updated. Meanwhile, we clarify the strengths and weaknesses of the aforementioned methods, providing a more reliable theoretical basis for the subsequent improvement of OMV production.
Furthermore, the applications of OMVs in the biomedical field are illustrated as well, including vaccines, anti-bacterial agents, immunotherapeutic drugs and drug delivery carriers. In particular, we put emphasis on the breakthrough of OMV-based brain-targeted DDS, which helps broaden the mind in the clinical treatment. In summary, OMVs have made encouraging progress in biomedical applications. Despite a few challenges, OMVs show significant application prospects and deserve further exploration.
Author contributions
Liang Han: conceptualization, funding acquisition, project administration, writing-review & editing; Haiyan Chen: investigation, writing – original draft, writing – review & editing; Mengyuan Zhou: drawing & review; Yuteng Zeng: writing – review & editing; Ziyan Lv: review; Pan Wang: review.
Conflicts of interest
There are no conflicts to declare.
Acknowledgements
This work was supported by the National Natural Science Foundation of China (81973254 and 32171381), the Natural Science Foundation of Jiangsu Province (BK20191421), the Suzhou Science and Technology Development Project (SYS2019033) and the Priority Academic Program Development of the Jiangsu Higher Education Institutes (PAPD).
Notes and references
- A. Kulp and M. J. Kuehn, Annu. Rev. Microbiol., 2010, 64, 163–184 CrossRef CAS PubMed.
- C. Schwechheimer and M. J. Kuehn, Nat. Rev. Microbiol., 2015, 13, 605–619 CrossRef CAS PubMed.
- M. Toyofuku, N. Nomura and L. Eberl, Nat. Rev. Microbiol., 2019, 17, 13–24 CrossRef CAS PubMed.
- M. Kaparakis-Liaskos and R. L. Ferrero, Nat. Rev. Immunol., 2015, 15, 375–387 CrossRef CAS PubMed.
- L. M. Mashburn-Warren and M. Whiteley, Mol. Microbiol., 2006, 61, 839–846 CrossRef CAS PubMed.
- J. C. Caruana and S. A. Walper, Front. Microbiol., 2020, 11, 432 CrossRef PubMed.
- L. M. Mashburn and M. Whiteley, Nature, 2005, 437, 422–425 CrossRef CAS PubMed.
- M. L. Vidakovics, J. Jendholm, M. Mörgelin, A. Månsson, C. Larsson, L. O. Cardell and K. Riesbeck, PLoS Pathog., 2010, 6, e1000724 CrossRef PubMed.
- A. J. McBroom and M. J. Kuehn, Mol. Microbiol., 2007, 63, 545–558 CrossRef CAS PubMed.
- L. van der Pol, M. Stork and P. van der Ley, Biotechnol. J., 2015, 10, 1689–1706 CrossRef CAS PubMed.
- Y. Zhang, Y. Chen, C. Lo, J. Zhuang, P. Angsantikul, Q. Zhang, X. Wei, Z. Zhou, M. Obonyo, R. Fang, W. Gao and L. Zhang, Angew. Chem., Int. Ed., 2019, 58, 11404–11408 CrossRef CAS PubMed.
- O. Y. Kim, H. T. Park, N. T. H. Dinh, S. J. Choi, J. Lee, J. H. Kim, S. W. Lee and Y. S. Gho, Nat. Commun., 2017, 8, 626 CrossRef PubMed.
- S. Jain and J. Pillai, Int. J. Nanomed., 2017, 12, 6329–6341 CrossRef CAS PubMed.
- H. Chen, M. Zhou, Y. Zeng, T. Miao, H. Luo, Y. Tong, M. Zhao, R. Mu, J. Gu, S. Yang and L. Han, Adv. Sci., 2022, 9, 2105854 CrossRef CAS PubMed.
- S. L. Reimer, D. R. Beniac, S. L. Hiebert, T. F. Booth, P. M. Chong, G. R. Westmacott, G. G. Zhanel and D. C. Bay, Front. Microbiol., 2021, 12, 628801 CrossRef PubMed.
- W. Gao, R. Fang, S. Thamphiwatana, B. T. Luk, J. Li, P. Angsantikul, Q. Zhang, C. Hu and L. Zhang, Nano Lett., 2015, 15, 1403–1409 CrossRef CAS PubMed.
- C. Perez-Cruz, O. Carrion, L. Delgado, G. Martinez, C. Lopez-Iglesias and E. Mercade, Appl. Environ. Microbiol., 2013, 79, 1874–1881 CrossRef CAS PubMed.
- S. Bhar, M. J. Edelmann and M. K. Jones, J. Proteomics, 2021, 231, 103994 CrossRef CAS PubMed.
- S. Roier, T. Blume, L. Klug, G. E. Wagner, W. Elhenawy, K. Zangger, R. Prassl, J. Reidl, G. Daum, M. F. Feldman and S. Schild, Int. J. Med. Microbiol., 2015, 305, 298–309 CrossRef CAS PubMed.
- W. D. McCaig, C. L. Loving, H. R. Hughes and S. L. Brockmeier, PLoS One, 2016, 11, e0149132 CrossRef PubMed.
- J. Fitzgerald, P. Leonard, E. Darcy, S. Sharma and R. O'Kennedy, Methods Mol. Biol., 2017, 1485, 27–51 CrossRef CAS PubMed.
- N. J. Alves, K. B. Turner, K. A. DiVito, M. A. Daniele and S. A. Walper, Res. Microbiol., 2017, 168, 139–146 CrossRef CAS PubMed.
- C. E. Frasch and M. S. Peppler, Infect. Immun., 1982, 37, 271–280 CrossRef CAS PubMed.
- K. Takada, N. Ohno and T. Yadomae, Infect. Immun., 1994, 62, 1171–1175 CrossRef CAS PubMed.
- L. Peng, M. Wang, Y. Chu, L. Zhang, J. Niu, H. Shao, T. Yuan, Z. Jiang, J. Gao and X. Ning, Sci. Adv., 2020, 6, eaba2735 CrossRef CAS PubMed.
- B. van de Waterbeemd, M. Streefland, P. van der Ley, B. Zomer, H. van Dijken, D. Martens, R. Wijffels and L. van der Pol, Vaccine, 2010, 28, 4810–4816 CrossRef CAS PubMed.
- I. Claassen, J. Meylis, P. van der Ley, C. Peeters, H. Brons, J. Robert, D. Borsboom, A. van der Ark, I. van Straaten, P. Roholl, B. Kuipers and J. Poolman, Vaccine, 1996, 14, 1001–1008 CrossRef CAS.
- R. Grande, M. C. Di Marcantonio, I. Robuffo, A. Pompilio, C. Celia, L. Di Marzio, D. Paolino, M. Codagnone, R. Muraro, P. Stoodley, L. Hall-Stoodley and G. Mincione, Front. Microbiol., 2015, 6, 1369 Search PubMed.
- G. De Benedetto, P. Cescutti, C. Giannelli, R. Rizzo and F. Micoli, ACS Omega, 2017, 2, 8282–8289 CrossRef CAS PubMed.
- M. J. H. Gerritzen, D. E. Martens, R. H. Wijffels and M. Stork, J. Extracell. Vesicles, 2017, 6, 1333883 CrossRef PubMed.
- J. Hong, P. Dauros-Singorenko, A. Whitcombe, L. Payne, C. Blenkiron, A. Phillips and S. Swift, J. Extracell. Vesicles, 2019, 8, 1632099 CrossRef CAS PubMed.
- R. A. Dragovic, C. Gardiner, A. S. Brooks, D. S. Tannetta, D. J. Ferguson, P. Hole, B. Carr, C. W. Redman, A. L. Harris, P. J. Dobson, P. Harrison and I. L. Sargent, Nanomedicine, 2011, 7, 780–788 CrossRef CAS PubMed.
- M. S. Soltani, F. Eftekhar, M. Noofeli, S. R. Banihashemi and F. Shahcheraghi, Arch. Razi. Inst., 2021, 76, 411–419 CAS.
- D. Chatterjee and K. Chaudhuri, FEBS Lett., 2011, 585, 1357–1362 CrossRef CAS PubMed.
- C. M. Hampton, R. C. Guerrero-Ferreira, R. E. Storms, J. V. Taylor, H. Yi, P. A. Gulig and E. R. Wright, Front. Microbiol., 2017, 8, 2177 CrossRef PubMed.
- C. Pérez-Cruz, L. Delgado, C. López-Iglesias and E. Mercade, PLoS One, 2015, 10, e0116896 CrossRef PubMed.
- H. Zhang, S. Dong, Z. Li, X. Feng, W. Xu, C. M. S. Tulinao, Y. Jiang and J. Ding, Asian J. Pharm. Sci., 2020, 15, 397–415 CrossRef PubMed.
- H. Liu, Z. Miao and Z. Zha, Chin. Chem. Lett., 2021, 33, 1673–1680 CrossRef.
- X. Liu, X. Zhong and C. Li, Chin. Chem. Lett., 2021, 32, 2347–2358 CrossRef CAS.
- X. Zhang, Y. Chen and Y. Chen, Biochem. Biophys. Res. Commun., 2014, 446, 375–379 CrossRef CAS PubMed.
- W. Fu and W. Zhang, Small, 2017, 13, 1700611 CrossRef PubMed.
- E. Y. Lee, J. Y. Bang, G. W. Park, D. S. Choi, J. S. Kang, H. J. Kim, K. S. Park, J. O. Lee, Y. K. Kim, K. H. Kwon, K. P. Kim and Y. S. Gho, Proteomics, 2007, 7, 3143–3153 CrossRef CAS PubMed.
- M. H. Kothary, G. R. Gopinath, J. Gangiredla, P. V. Rallabhandi, L. M. Harrison, Q. Yan, H. R. Chase, B. Lee, E. Park, Y. Yoo, T. Chung, S. B. Finkelstein, F. J. Negrete, I. R. Patel, L. Carter, V. Sathyamoorthy, S. Fanning and B. D. Tall, Front. Microbiol., 2017, 8, 134 Search PubMed.
- V. Gujrati, S. Kim, S. H. Kim, J. J. Min, H. E. Choy, S. C. Kim and S. Jon, ACS Nano, 2014, 8, 1525–1537 CrossRef CAS PubMed.
- M. S. Soltani, M. Noofeli, S. R. Banihashemi, F. Shahcheraghi and F. Eftekhar, Iran. Biomed. J., 2021, 25, 399–407 CrossRef PubMed.
- J. Lee, O. Y. Kim and Y. S. Gho, Proteomics Clin Appl, 2016, 10, 897–909 CrossRef CAS PubMed.
- V. Herlax, M. J. de Alaniz and L. Bakás, Chem. Phys. Lipids, 2005, 135, 107–115 CrossRef CAS PubMed.
- D. Chen, N. Osterrieder, S. M. Metzger, E. Buckles, A. M. Doody, M. P. DeLisa and D. Putnam, Proc. Natl. Acad. Sci. U. S. A., 2010, 107, 3099–3104 CrossRef CAS PubMed.
- E. Schulz, A. Goes, R. Garcia, F. Panter, M. Koch, R. Müller, K. Fuhrmann and G. Fuhrmann, J. Controlled Release, 2018, 290, 46–55 CrossRef CAS PubMed.
- R. Acevedo, C. Zayas, G. Norheim, S. Fernández, B. Cedré, Y. Aranguren, M. Cuello, Y. Rodriguez, H. González, A. Mandiarote, M. Pérez, M. Hernández, M. Hernández-Cedeño, D. González, S. H. Brorson, E. Rosenqvist, L. Naess, G. Tunheim, D. Cardoso and L. García, Pharmacol. Res., 2017, 121, 194–201 CrossRef CAS PubMed.
- W. Huang, Q. Zhang, W. Li, M. Yuan, J. Zhou, L. Hua, Y. Chen, C. Ye and Y. Ma, J. Controlled Release, 2020, 317, 1–22 CrossRef CAS PubMed.
- M. Somiya, Y. Yoshioka and T. Ochiya, Aims Bioeng., 2017, 1, 73–92 Search PubMed.
- Y. Li, J. Wu, J. Wang, X. Hu, J. Cai and D. Xiang, Acta Biomater., 2020, 101, 519–530 CrossRef CAS PubMed.
- Q. Chen, H. Bai, W. Wu, G. Huang, Y. Li, M. Wu, G. Tang and Y. Ping, Nano Lett., 2020, 20, 11–21 CrossRef CAS PubMed.
- Z. Ayed, L. Cuvillier, G. Dobhal and R. V. Goreham, SN Appl. Sci., 2019, 1, 1600 CrossRef CAS.
- S. C. Jang, S. R. Kim, Y. J. Yoon, K. S. Park, J. H. Kim, J. Lee, O. Y. Kim, E. J. Choi, D. K. Kim, D. S. Choi, Y. K. Kim, J. Park, D. Di Vizio and Y. S. Gho, Small, 2015, 11, 456–461 CrossRef CAS PubMed.
- N. J. Alves, K. B. Turner and S. A. Walper, J. Visualized Exp., 2016, 3, 54458 Search PubMed.
- Y. Ojima, T. Sawabe, K. Konami and M. Azuma, Biotechnol. Bioeng., 2020, 117, 701–709 CrossRef CAS PubMed.
- Q. Chen, S. Rozovsky and W. Chen, Chem. Commun., 2017, 53, 7569–7572 RSC.
- Y. Li, R. Zhao, K. Cheng, K. Zhang, Y. Wang, Y. Zhang, Y. Li, G. Liu, J. Xu, J. Xu, G. J. Anderson, J. Shi, L. Ren, X. Zhao and G. Nie, ACS Nano, 2020, 14, 16698–16711 CrossRef CAS PubMed.
- J. E. Somerville, Jr., L. Cassiano, B. Bainbridge, M. D. Cunningham and R. P. Darveau, J. Clin. Invest., 1996, 97, 359–365 CrossRef PubMed.
- P. van der Ley, L. Steeghs, H. J. Hamstra, J. ten Hove, B. Zomer and L. van Alphen, Infect. Immun., 2001, 69, 5981–5990 CrossRef CAS PubMed.
- J. Arenas, H. van Dijken, B. Kuipers, H. J. Hamstra, J. Tommassen and P. van der Ley, Clin. Vaccine Immunol., 2010, 17, 487–495 CrossRef CAS PubMed.
- J. Geurtsen, L. Steeghs, H. J. Hamstra, J. Ten Hove, A. de Haan, B. Kuipers, J. Tommassen and P. van der Ley, Infect. Immun., 2006, 74, 5574–5585 CrossRef CAS PubMed.
- L. Chen, J. L. Valentine, C. Huang, C. E. Endicott, T. D. Moeller, J. A. Rasmussen, J. R. Fletcher, J. M. Boll, J. A. Rosenthal, J. Dobruchowska, Z. Wang, C. Heiss, P. Azadi, D. Putnam, M. S. Trent, B. D. Jones and M. P. DeLisa, Proc. Natl. Acad. Sci. U. S. A., 2016, 113, E3609–E3618 CrossRef CAS PubMed.
- B. D. Needham, S. M. Carroll, D. K. Giles, G. Georgiou, M. Whiteley and M. S. Trent, Proc. Natl. Acad. Sci. U. S. A., 2013, 110, 1464–1469 CrossRef CAS PubMed.
- S. Jiang, S. H. Park, H. J. Lee, J. Zheng, H. S. Kim, H. S. Bom, Y. Hong, M. Szardenings, M. G. Shin, S. C. Kim, V. Ntziachristos, H. E. Choy and J. J. Min, Mol. Ther., 2013, 21, 1985–1995 CrossRef CAS PubMed.
- Q. Zhuang, J. Xu, D. Deng, T. Chao, J. Li, R. Zhang, R. Peng and Z. Liu, Biomaterials, 2021, 268, 120550 CrossRef CAS PubMed.
- K. B. Johnsen, M. Bak, F. Melander, M. S. Thomsen, A. Burkhart, P. J. Kempen, T. L. Andresen and T. Moos, J. Controlled Release, 2019, 295, 237–249 CrossRef CAS PubMed.
- A. M. Alkilany, L. Zhu, H. Weller, A. Mews, W. J. Parak, M. Barz and N. Feliu, Adv. Drug Delivery Rev., 2019, 143, 22–36 CrossRef CAS PubMed.
- M. F. Bachmann and G. T. Jennings, Nat. Rev. Immunol., 2010, 10, 787–796 CrossRef CAS PubMed.
- C. L. Byington, Y. A. Maldonado, E. D. Barnett, H. Dele Davies, K. M. Edwards, R. Lynfield, F. M. Munoz, D. L. Nolt, A. C. Nyquist, M. H. Rathore, M. H. Sawyer, W. J. Steinbach, T. Q. Tan and T. E. Zaoutis, Pediatrics, 2016, 138, e20161890 CrossRef PubMed.
- Y. Masforrol, J. Gil, D. García, J. Noda, Y. Ramos, L. Betancourt, O. Guirola, S. González, B. Acevedo, V. Besada, O. Reyes and L. J. González, Hum. Vaccin. Immunother., 2017, 13, 2548–2560 CrossRef PubMed.
- J. Holst, D. Martin, R. Arnold, C. C. Huergo, P. Oster, J. O'Hallahan and E. Rosenqvist, Vaccine, 2009, 27(Suppl 2), B3–B12 CrossRef CAS PubMed.
- P. B. Keiser, S. Biggs-Cicatelli, E. E. Moran, D. H. Schmiel, V. B. Pinto, R. E. Burden, L. B. Miller, J. E. Moon, R. A. Bowden, J. F. Cummings and W. D. Zollinger, Vaccine, 2011, 29, 1413–1420 CrossRef CAS PubMed.
- R. Prados-Rosales, L. J. Carreño, A. Batista-Gonzalez, A. Baena, M. M. Venkataswamy, J. Xu, X. Yu, G. Wallstrom, D. M. Magee, J. LaBaer, J. M. Achkar, W. R. Jacobs, Jr., J. Chan, S. A. Porcelli and A. Casadevall, mBio, 2014, 5, e01921–01914 CrossRef CAS PubMed.
- S. J. Choi, M. H. Kim, J. Jeon, O. Y. Kim, Y. Choi, J. Seo, S. Hong, W. H. Lee, S. G. Jeon, Y. S. Gho, Y. K. Jee and Y. K. Kim, PLoS One, 2015, 10, e0136021 CrossRef PubMed.
- C. G. Rappazzo, H. C. Watkins, C. M. Guarino, A. Chau, J. L. Lopez, M. P. DeLisa, C. A. Leifer, G. R. Whittaker and D. Putnam, Vaccine, 2016, 34, 1252–1258 CrossRef CAS PubMed.
- M. L. Salverda, S. M. Meinderts, H. J. Hamstra, A. Wagemakers, J. W. Hovius, A. van der Ark, M. Stork and P. van der Ley, Vaccine, 2016, 34, 1025–1033 CrossRef CAS PubMed.
- D. R. Leitner, S. Lichtenegger, P. Temel, F. G. Zingl, D. Ratzberger, S. Roier, K. Schild-Prüfert, S. Feichter, J. Reidl and S. Schild, Front. Microbiol., 2015, 6, 823 Search PubMed.
- K. Kuipers, M. H. Daleke-Schermerhorn, W. S. Jong, C. M. ten Hagen-Jongman, F. van Opzeeland, E. Simonetti, J. Luirink and M. I. de Jonge, Vaccine, 2015, 33, 2022–2029 CrossRef CAS PubMed.
- M. H. Daleke-Schermerhorn, T. Felix, Z. Soprova, C. M. Ten Hagen-Jongman, D. Vikström, L. Majlessi, J. Beskers, F. Follmann, K. de Punder, N. N. van der Wel, T. Baumgarten, T. V. Pham, S. R. Piersma, C. R. Jiménez, P. van Ulsen, J. W. de Gier, C. Leclerc, W. S. Jong and J. Luirink, Appl. Environ. Microbiol., 2014, 80, 5854–5865 CrossRef PubMed.
- T. F. Murphy and G. I. Parameswaran, Clin. Infect. Dis., 2009, 49, 124–131 CrossRef PubMed.
- J. Simon and K. Kotloff, Curr. Opin. Gastroenterol., 2010, 26, 12–16 CrossRef CAS PubMed.
- J. L. Kadurugamuwa and T. J. Beveridge, J. Bacteriol., 1996, 178, 2767–2774 CrossRef CAS PubMed.
- K. L. MacDonald and T. J. Beveridge, Can. J. Microbiol., 2002, 48, 810–820 CrossRef CAS PubMed.
- Z. Li, A. J. Clarke and T. J. Beveridge, J. Bacteriol., 1998, 180, 5478–5483 CrossRef CAS PubMed.
- S. R. Watt and A. J. Clarke, Can. J. Microbiol., 1997, 43, 1054–1062 CrossRef CAS PubMed.
- C. Berne, C. K. Ellison, A. Ducret and Y. V. Brun, Nat. Rev. Microbiol., 2018, 16, 616–627 CrossRef CAS PubMed.
- D. A. Rasko and V. Sperandio, Nat. Rev. Drug Discovery, 2010, 9, 117–128 CrossRef CAS PubMed.
- A. Asadi, S. Razavi, M. Talebi and M. Gholami, Infection, 2019, 47, 13–23 CrossRef PubMed.
- I. Ofek, D. L. Hasty and N. Sharon, FEMS Immunol. Med. Microbiol., 2003, 38, 181–191 CrossRef CAS PubMed.
- C. K. Cusumano, J. S. Pinkner, Z. Han, S. E. Greene, B. A. Ford, J. R. Crowley, J. P. Henderson, J. W. Janetka and S. J. Hultgren, Sci. Transl. Med., 2011, 3, 109–115 Search PubMed.
- I. W. DeVoe and J. E. Gilchrist, J. Exp. Med., 1975, 141, 297–305 CrossRef CAS PubMed.
- S. N. Chatterjee and J. Das, J. Gen. Microbiol., 1967, 49, 1–11 CrossRef CAS PubMed.
- G. Gasperini, M. Biagini, V. Arato, C. Gianfaldoni, A. Vadi, N. Norais, G. Bensi, I. Delany, M. Pizza, B. Aricò and R. Leuzzi, Mol. Cell. Proteomics, 2018, 17, 205–215 CrossRef CAS PubMed.
- M. Lundqvist, J. Stigler, G. Elia, I. Lynch, T. Cedervall and K. A. Dawson, Proc. Natl. Acad. Sci. U. S. A., 2008, 105, 14265–14270 CrossRef CAS PubMed.
- L. Turner, J. Praszkier, M. L. Hutton, D. Steer, G. Ramm, M. Kaparakis-Liaskos and R. L. Ferrero, Helicobacter, 2015, 20, 269–283 CrossRef CAS PubMed.
- L. M. Coussens and Z. Werb, Nature, 2002, 420, 860–867 CrossRef CAS PubMed.
- M. A. Richardson, T. Ramirez, N. C. Russell and L. A. Moye, Altern. Ther. Health Med., 1999, 5, 42–47 CAS.
- E. F. McCarthy, Iowa Orthop. J., 2006, 26, 154–158 Search PubMed.
- R. Acevedo, S. Fernández, C. Zayas, A. Acosta, M. E. Sarmiento, V. A. Ferro, E. Rosenqvist, C. Campa, D. Cardoso, L. Garcia and J. L. Perez, Front. Immunol., 2014, 5, 121 Search PubMed.
- M. Li, H. Zhou, C. Yang, Y. Wu, X. Zhou, H. Liu and Y. Wang, J. Controlled Release, 2020, 323, 253–268 CrossRef CAS PubMed.
- H. Wang, J. Li, Z. Wang, Y. Wang, X. Xu, X. Gong, J. Wang, Z. Zhang and Y. Li, Biomaterials, 2021, 269, 120609 CrossRef CAS PubMed.
- M. Binnewies, E. W. Roberts, K. Kersten, V. Chan, D. F. Fearon, M. Merad, L. M. Coussens, D. I. Gabrilovich, S. Ostrand-Rosenberg, C. C. Hedrick, R. H. Vonderheide, M. J. Pittet, R. K. Jain, W. Zou, T. K. Howcroft, E. C. Woodhouse, R. A. Weinberg and M. F. Krummel, Nat. Med., 2018, 24, 541–550 CrossRef CAS PubMed.
- S. Qing, C. Lyu, L. Zhu, C. Pan, S. Wang, F. Li, J. Wang, H. Yue, X. Gao, R. Jia, W. Wei and G. Ma, Adv. Mater., 2020, 32, e2002085 CrossRef PubMed.
- J. Pan, X. Li, B. Shao, F. Xu, X. Huang, X. Guo and S. Zhou, Adv. Mater., 2022, 34, e2106307 CrossRef PubMed.
- K. Cheng, R. Zhao, Y. Li, Y. Qi, Y. Wang, Y. Zhang, H. Qin, Y. Qin, L. Chen, C. Li, J. Liang, Y. Li, J. Xu, X. Han, G. J. Anderson, J. Shi, L. Ren, X. Zhao and G. Nie, Nat. Commun., 2021, 12, 2041 CrossRef CAS PubMed.
- L. Chen, H. Qin, R. Zhao, X. Zhao, L. Lin, Y. Chen, Y. Lin, Y. Li, Y. Qin, Y. Li, S. Liu, K. Cheng, H. Chen, J. Shi, G. J. Anderson, Y. Wu, Y. Zhao and G. Nie, Sci. Transl. Med., 2021, 13, eabc2816 CrossRef CAS PubMed.
- M. Li, H. Zhou, W. Jiang, C. Yang, H. Miao and Y. Wang, Nano Today, 2020, 35, 101007 CrossRef CAS.
- Q. Chen, G. Huang, W. Wu, J. Wang, J. Hu, J. Mao, P. K. Chu, H. Bai and G. Tang, Adv. Mater., 2020, 32, e1908185 CrossRef PubMed.
- S. M. Sagnella, L. Yang, G. E. Stubbs, E. Boslem, E. Martino-Echarri, K. Smolarczyk, S. L. Pattison, N. Vanegas, E. Clair, S. Clarke, J. Boockvar, J. A. MacDiarmid and H. Brahmbhatt, Cancer Cell, 2020, 37(354–370), e357 Search PubMed.
- Q. Guo, X. Li, W. Zhou, Y. Chu, Q. Chen, Y. Zhang, C. Li, H. Chen, P. Liu, Z. Zhao, Y. Wang, Z. Zhou, Y. Luo, C. Li, H. You, H. Song, B. Su, T. Zhang, T. Sun and C. Jiang, ACS Nano, 2021, 15, 13826–13838 CrossRef CAS PubMed.
- M. Wenes, M. Shang, M. Di Matteo, J. Goveia, R. Martín-Pérez, J. Serneels, H. Prenen, B. Ghesquière, P. Carmeliet and M. Mazzone, Cell Metab., 2016, 24, 701–715 CrossRef CAS PubMed.
- V. Byles, A. J. Covarrubias, I. Ben-Sahra, D. W. Lamming, D. M. Sabatini, B. D. Manning and T. Horng, Nat. Commun., 2013, 4, 2834 CrossRef PubMed.
- C. Zhu, J. M. Kros, C. Cheng and D. Mustafa, Neuro-Oncology, 2017, 19, 1435–1446 CrossRef CAS PubMed.
- S. Wang, J. Gao and Z. Wang, Wiley Interdiscip. Rev.: Nanomed. Nanobiotechnol., 2019, 11, e1523 Search PubMed.
- E. Kolaczkowska and P. Kubes, Nat. Rev. Immunol., 2013, 13, 159–175 CrossRef CAS PubMed.
- M. Li, S. Li, H. Zhou, X. Tang, Y. Wu, W. Jiang, Z. Tian, X. Zhou, X. Yang and Y. Wang, Nat. Commun., 2020, 11, 1126 CrossRef CAS PubMed.
- L. Han and C. Jiang, Acta Pharm. Sin. B, 2021, 11, 2306–2325 CrossRef CAS PubMed.
- L. Han, Med. Drug Discovery, 2022, 13, 100111 CrossRef CAS.
- R. Liu, C. Luo, Z. Pang, J. Zhang, S. Ruan, M. Wu, L. Wang, T. Sun, N. Li, L. Han, J. Shi, Y. Huang, W. Guo, S. Peng, W. Zhou and H. Gao, Chin. Chem. Lett., 2022 DOI:10.1016/j.cclet.2022.05.032.
- Q. Guo, Q. Zhu, T. Miao, J. Tao, X. Ju, Z. Sun, H. Li, G. Xu, H. Chen and L. Han, J. Controlled Release, 2019, 303, 117–129 CrossRef CAS PubMed.
- J. Ni, T. Miao, M. Su, N. U. Khan, X. Ju, H. Chen, F. Liu and L. Han, J. Controlled Release, 2021, 329, 934–947 CrossRef CAS PubMed.
- T. Miao, X. Ju, Q. Zhu, Y. Wang, Q. Guo, T. Sun, C. Lu and L. Han, Adv. Funct. Mater., 2019, 29, 1900259 CrossRef.
- X. Ju, T. Miao, H. Chen, J. Ni and L. Han, Adv. Healthcare Mater., 2021, 10, e2001997 CrossRef PubMed.
- X. Ju, H. Chen, T. Miao, J. Ni and L. Han, Mol. Pharm., 2021, 18, 2694–2702 CrossRef CAS PubMed.
- N. U. Khan, J. Ni, X. Ju, T. Miao, H. Chen and L. Han, Acta Pharm. Sin. B, 2021, 11, 1341–1354 CrossRef CAS PubMed.
|
This journal is © The Royal Society of Chemistry 2022 |