DOI:
10.1039/D1TB02316K
(Paper)
J. Mater. Chem. B, 2022,
10, 2628-2636
Phospholipid polymer hydrogels with rapid dissociation for reversible cell immobilization†
Received
22nd October 2021
, Accepted 20th December 2021
First published on 21st December 2021
Abstract
A reversible and cytocompatible cell immobilization polymer matrix with a rapid dissociation rate was prepared using a zwitterionic phospholipid polymer bearing phenylboronic acid and poly(vinyl alcohol) (PVA). A reversible and spontaneously forming phospholipid polymer hydrogel is reported for use as a cell immobilization matrix which caused no invasive damage to the cells. To improve the possibility of applying the hydrogels as a reversible cell immobilization matrix, the stimuli-responsive dissociation rate of polymer hydrogels was designed to have a more rapid rate to ease the recovery of the immobilized cells. In this study, a phospholipid polymer containing 3-methacrylamide phenylboronic acid (MAPBA) as the phenylboronic acid unit was synthesized. The water-soluble phospholipid polymer (PMB–MAPBA) can spontaneously form polymer hydrogels after mixing with PVA solution under normal pressure, room temperature, and neutral pH conditions. Also, the dissociation of the hydrogels after the addition of D-sorbitol completely occurred within 10 minutes. The cells were easily immobilized on the hydrogels during the preparation process. Also, the recovery ratio of the immobilized cells was improved due to the rapid dissociation of the hydrogels. The reversible and spontaneously formed phospholipid polymer hydrogels are promising for use as soft materials for platforms for cell engineering.
1. Introduction
Zwitterionic polymer materials are promising platform biomaterials for medical and pharmaceutical applications including cell engineering.1–4 Recently, a lot of studies have been carried out to investigate the interactions between zwitterionic biointerfaces and biological compounds.5–7 Cells are used not only as biological models, but also as living biomaterials for applications in tissue engineering and regenerative medicine. Various cell handling techniques have been developed to control cell culture including proliferation and differentiation. However, these functional cells are eventually cryopreserved as part of the usual production process. However, the cryopreservation process is invasive and affects the cell functions. It is desirable to maintain the cell function in the polymer matrix without any significant damage of the cells when they are to be used for cell engineering.8,9
Cytocompatible phospholipid polymers, such as 2-methacryloyloxyethyl phosphorylcholine (MPC) polymers, are used as polymer biomaterials for multi-purpose biomedical applications including implantable medical devices, cell culture substrates, and pharmaceutical applications.10–15 The cytocompatibility of MPC polymers are based on the weak interactions of biological compounds, typically proteins.16,17 In particular, the biointerfaces of MPC polymers have a high free-water content and are weakly affected by the water structure in bulk water.18 This is the major difference between conventional hydrophilic polymer materials such as poly(2-hydroxyethyl methacrylate) (PHEMA) and poly(ethylene glycol) (PEG).
Polymer hydrogels are promising platform biomaterials for use in 3D cell engineering systems. Various polymer hydrogels have been proposed as 3D cell scaffolds.19–28 However, conventional polymer hydrogels are molecularly designed to enhance cell proliferation including cell adhesion. The cells adhered on conventional materials expressed inflammatory cytokines during the cell culture period.29 The expression of inflammatory cytokines indicated that the conventional polymers are not cytocompatible for cell engineering. Recently, polymer hydrogels, characterized by the ability to perform reversible formation have attracted much attention, and have been applied to various biomedical applications.29 In particular, polymer hydrogels with phenylboronic acid as a functional group can be reversibly gelled under neutral conditions and are expected to be used in the field of cell engineering.29–32 It has already been reported that a phospholipid polymer containing p-vinylphenylboronic acid (VPBA) can spontaneously form polymer hydrogels after mixing with poly(vinyl alcohol) (PVA) solution.33 The hydrogels can be various cells in the network that can be mildly immobilized without any significant damage.34–41 In addition, the cell cycle of the immobilized cells was gradually dominated by the G1 phase during the immobilization period, and thus, the cell differentiation ability can be enhanced in the hydrogels.37,39 The polymer hydrogels can be dissociated by the addition of D-sorbitol which changes the reaction between the diol groups in PVA and the sugar moiety.41 However, the dissociation rate is a relatively long period, and the recovery rate of the immobilized cells is hardly improved. The signal-responsivity of this hydrogel should be improved and it should be suitable to use as the matrix for cell engineering.
In this study, a phospholipid polymer containing 3-methacrylamide phenylboronic acid (MAPBA) was synthesized for use as a functional phenylboronic acid unit. The water-soluble MPC polymer containing MAPBA also spontaneously formed polymer hydrogels after mixing with PVA solution. The dissociation rate of the PMB–MAPBA hydrogels is extremely quick when compared to that of conventional hydrogels. The degree of polymerization of PVA is 500. Although this degree of polymerization is relatively low, the PMB–MAPBA can covalently bind to PVA, and hydrogels with the cell immobilization ability can be achieved. It is expected that the cytocompatible phospholipid polymer with a rapid dissociation ability might be useful as a reversible cell immobilization matrix for use in the cell engineering field.
2. Materials and methods
2.1 Materials
The MPC was purchased from the NOF Company (Tokyo, Japan), and was synthesized using a previously reported method.10 The n-butyl methacrylate (BMA) was purchased from FUJIFILM Wako Pure Chemical Corporation (Osaka, Japan). The α,α′-azobisisobutyronitrile (AIBN) were purchased from Kanto Chemical, (Tokyo, Japan). A phospholipid polymer containing VPBA, poly(MPC-co-BMA-co-VPBA) (PMB–VPBA) was synthesized by a method reported previously.33 HeLa cells were obtained from the RIKEN cell bank (Saitama, Japan). Dulbecco's modified Eagle medium (DMEM), fetal bovine serum (FBS), and phosphate-buffered saline (PBS) were purchased from Thermo Fisher Scientific (Waltham, MA, USA). Other solvents and reagents were of extra-pure grade and used without further purification.
2.2 Monomer synthesis
3-Aminophenylboronic acid (5.08 g, 32.8 mmol, 1.09 eq.) was dissolved in 46.0 mL of 3.0 M sodium hydroxide, and methacryloyl chloride (3.16 g, 30.2 mmol, 1.00 eq.) was added dropwise at 0 °C to the mixture placed in an ice bath. After stirring for 2 h at room temperature, 12 M hydrochloric acid was added dropwise to acidify the mixture to a pH of 1. The precipitated solid was filtered off using a glass filter, and dissolved in ethyl acetate, and washed three times with distilled water, and saturated brine. After dehydration with sodium sulfate, the solvent was removed using a rotary evaporator, and an oily compound was precipitated. The obtained compound was purified by hot filtration in about 200 mL of hot water at 80 °C, and cooled overnight in a refrigerator, filtered through a glass filter, and dried under reduced pressure to obtain the desired methacrylamide-type phenylboronic acid, MAPBA. The yield was 41%, and the 1H-nuclear magnetic resonance (1H-NMR) spectrum of the product is shown in Fig. S1 (ESI†).
2.3 Polymer synthesis
The PMB–MAPBA was synthesized using conventional radical polymerization. The reaction was carried out in ethanol as the polymerization solvent using AIBN as an initiator. All the monomers were dissolved in ethanol and the total monomer concentration was adjusted to 1.0 mol L−1. The mole fractions of MPC, BMA, and MAPBA in the feed solution were 0.60, 0.30, and 0.10, respectively. The AIBN was also dissolved in the monomer solution at a concentration of 10 mmol L−1. The solution was transferred to a test-tube, and argon gas was bubbled through the solution for 15 min to eliminate oxygen. Following this, the test-tube was sealed and heated at 60 °C for 3 h. The reacted solution was removed, and the polymer which had formed was collected by reprecipitation in diethyl ether. The precipitated polymer was filtered and dried in vacuo. The white solid obtained by reprecipitation was dried under reduced pressure, and then the polymer was dissolved in distilled water and dialyzed for 6 d using a dialysis membrane with a fractional molecular weight of 3.5 kDa. After dialysis, the PMB–MAPBA631 was lyophilized to obtain the desired polymer.
The composition of each monomer unit of PMB–MAPBA was determined using NMR with deuterated ethanol (C2D5OD) as a solvent. The 1H-NMR spectrum of PMB–MAPBA is shown in Fig. 1.
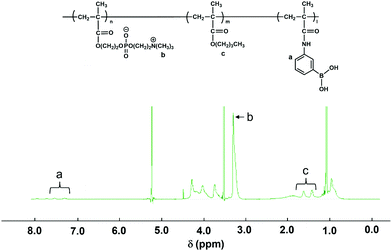 |
| Fig. 1 The 1H-NMR spectrum of PMB–MAPBA in C2D5OD. | |
The weight-averaged molecular weight (Mw) and number-averaged molecular weight (Mn) of the polymer were determined using gel permeation chromatography (GPC, JASCO, Tokyo, Japan). A mixture of methanol and water (70/30 by volume) containing 10 mmol L−1 of LiBr and 1.0 mg mL−1 of D-sorbitol was used as an eluent. The flow rate was 0.4 mL min−1. The differential refractive index (RI) detection was performed. The calibration curve was prepared using a standard sample of poly(ethylene oxide). The chemical structure of PMB–MAPBA is shown in Fig. 1 and the results of its synthesis and characterization are summarized in Table 1.
Table 1 Synthetic result of PMB–MAPBA
Abbreviation |
In feed (mole fraction) MPC/BMA/MAPBA |
In polymer (mole fraction)a MPC/BMA/MAPBA |
Polimerization time (h) |
Yield (%) |
Mwb |
Mw/Mnb |
Determined by 1H-NMR (solvent: ethanol-d6).
Determined by GPC (solvent: methanol/H2O = 7/3, in the presence of 10 mM LiBr, 1 mg mL−1D-sorbitol).
|
PMB–MAPBA |
0.60/0.30/0.10 |
0.69/0.19/0.12 |
2.5 |
55.6 |
1.42 × 105 |
1.05 |
2.4 The pKa measurement of PMB–MAPBA
To estimate the pKa value of PMB–MAPBA, UV spectra were obtained at various pH values. The UV spectra of PMB–MAPBA631 and PMBV631 (measurement range 230–350 nm) were measured by preparing a 1 mg mL−1 polymer solution using a buffer solution with a pH 6.0–12.0. The absorbance of each polymer at 286.0 nm and 267.5 nm was measured to determine the pKa, and the effect of the change in the phenylboronic acid in the polymer was confirmed.
2.5 Spontaneous gelation of the PMB–MAPBA hydrogels
The PMB–MAPBA and completely saponified PVA500 (degree of polymerization was 500) were dissolved in PBS at pH 7.4 to achieve the desired concentration. Each polymer solution (200 μL) was mixed in a microtube. After gently shaking the tube for 10 s, the tube was inverted, and the hydrogel formation was confirmed.
2.6 Rheological measurements
The PMB–MAPBA was dissolved in PBS at pH 7.4 to give the desired concentration. A 5 wt% completely saponified PVA500 solution was prepared by dissolving PVA in hot water (80 °C) and adjusting the concentration to 5 wt% of PVA solution dissolved in PBS at pH 7.4. A portion (200 μL) of each of PMB–MAPBA solution and PVA solution were added into a microtube, and gently shaken for 10 s. The prepared hydrogel was scraped out with a spatula and set on a circular plate with a radius of 1 cm on a rheometer (Rheolograph-Micro, Toyo Seiki Kogyo, Tokyo, Japan). The hydrogel was sandwiched between circular plates with a 1 cm radius and 1 mm in height, and oscillated at a frequency of 20 Hz and an amplitude of 200 μm.
2.7 Dissociation of PMB–MAPBA hydrogels in the presence of sugar molecules
The dissociation behavior of the hydrogel was evaluated by determining the weight ratio of the hydrogel before and after the addition of the dissociation solution. A completely saponified PVA500 solution (5 wt%) were prepared. The gels were prepared by adding 200 μL of PMB–MAPBA (5 wt%) aqueous solution and 200 μL of the PVA aqueous solution into a microtube and gently tapping it for 10 s.
The dissociation behaviors of the PMB–MAPBA hydrogels were evaluated by the following method. Dissociation solution (1 mL of 1 M D-sorbitol or D-glucose solution) was added into a microtube containing the hydrogel, and the dissociation solution was removed by decanting at the desired time. The fraction that remained in the microtube was weighed, and this was the residual hydrogel after the dissociation operation. The weight ratio of the hydrogel before and after dissociation was calculated each time to evaluate the dissociation properties.
2.8 Cell immobilization in the PMB–MAPBA hydrogels
The HeLa cells were cultured in DMEM medium containing 10% FBS and 1% penicillin–streptomycin at 37 °C and 5% CO2. After trypsin treatment, the cells were collected and resuspended in DMEM medium (FBS+, Ab+) containing 5 wt% PMB–MAPBA. The PMBV–MAPBA solution containing the cells was mixed with 5 wt% PVA solution (DMEM medium), and the PMB–MAPBA hydrogels were prepared by pipetting several times. The volume ratio of PMB–MAPBA solution to PVA solution was set at 1
:
1.
After 3 d of incubation, 1 mL of D-sorbitol solution (1.0 M) was added, and the hydrogels were collapsed by pipetting. The cells were collected from the dissociated gels. The number of recovered cells was counted using a hemocytometer, and the recovery rate of immobilized cells was calculated. The recovered cells were replated under normal conditions. The cells were seeded at 7.5 × 103 cells per mL per well and 1 mL per well. The collected cells were cultured at 37 °C and 5% CO2, and after washing with PBS on days 1, 2, and 3, the cells were observed with a phase-contrast microscope.
3. Results and discussion
3.1. Synthesis and characterization of the PMB–MAPBA polymer
The MAPBA was successfully synthesized as shown in the 1H-NMR spectrum (Fig. S1, ESI†). The polymer, PMB–MAPBA was obtained using conventional radical polymerization with ethanol and AIBN as solvent and initiator, respectively. The 1H-NMR spectrum of PMB–MAPBA is shown in Fig. 1 and the characterization of the polymers is summarized in Table 1. The copolymer composition was calculated from the integral values of the following peaks: 3.25 ppm (–N + (CH3)3, 9H) for the MPC unit, 1.30–1.60 ppm (–CH2–, 4H) for the BMA unit, and 7.30–8.00 ppm (aromatic ring, 4H) for the MAPBA unit. The copolymer compositions were almost the same as those of the monomer feed, and the molar ratios of the MPC unit and BMA unit and the MAPBA unit in the polymer were 0.69, 0.19, 0.11, respectively. The molecular weight of PMB–MAPBA was 1.42 × 105 and the polydispersity index (Mw/Mn) was determined by GPC and found to be 1.05. This polymer was water-soluble, and the polymer solution was low viscosity because the solution could be treated with a micropipette.
Next, the properties of PMB–MAPBA and PMB–VPBA in solution were compared. These copolymer compositions were almost the same. The pKa of the copolymers were determined by measuring the UV-Vis spectra using buffers adjusted to pHs of 6–12 as shown in Fig. 2. The maximum absorbances at 286.0 nm and 267.5 nm depended on the ratio of the trigonal acidic form of the phenylboronic acid groups in the polymers. The changes in absorbance of the copolymers are shown in Fig. 3. The pKa was calculated from the inflection point of a pH-absorbance function by KaleidaGraph software (Hulinks, Tokyo, Japan) and and the results are summarized in Table S1 (ESI†). The pKa value of PMB–MAPBA (pKa = 9.34) was lower than that of PMB–VPBA (pKa = 10.0). This result indicated that PMB–MAPBA easily formed a boronate anionic structure in physiological pHs. Generally, the phenylboronic acid groups having a lower pKa is advantageous to for it to bind to cis-diol compounds. Therefore, PMB–MAPBA is expected to form hydrogels with polyol compounds via reversible and dynamic covalent bonds.
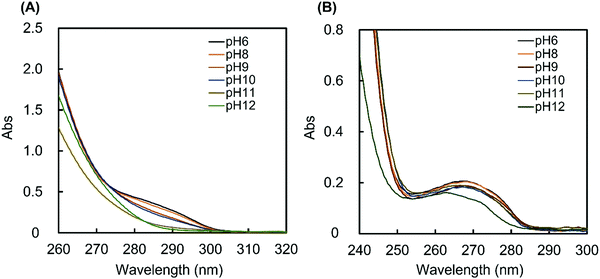 |
| Fig. 2 The UV spectra of (A) PMB–MAPBA and (B) PMB–VPBA solution. The UV spectra of 1 mg mL−1 polymer solutions were measured at 25 °C using buffers with pH values of 6–12. | |
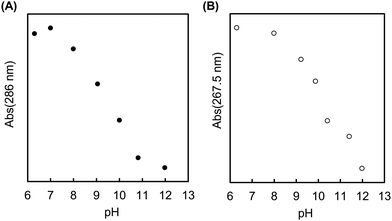 |
| Fig. 3 The changes in absorbance of (A) PMB–MAPBA (286 nm) and (B) PMB–VPBA (267.5 nm) in buffer solutions with pH values of 6–12. | |
3.2 Preparation of the PMB–MAPBA hydrogels
Next, PMB–MAPBA/PVA hydrogels were prepared. The PMB–MAPBA solution was mixed with PVA solution by pipetting, and hydrogels were formed immediately at room temperature, ambient pressure, and neutral pH. As shown in Fig. 4, the phenylboronic acid groups in PMB–MAPBA formed crosslinking with the diols of PVA. Firstly, the effect of polymer concentration on hydrogel formation using PMB–MAPBA and completely saponified PVA was investigated. Analysis of the sol–gel transition behavior was determined by the inverted tube method. Tables 2 and 3 show that PVA with a high degree of polymerization (DP) was easily able to form a hydrogel with a low concentration of PMB–MAPBA solution. This result suggested that PVA having a high DP formed more crosslinking points with PMB–MAPBA than PVA which had a low DP. Fig. 5 shows that PMB–MAPBA more easily formed hydrogels with PVA which had a lower DP than PMB–VPBA. This was because the boronic acid unit in PMB–MAPBA has a methacrylamide-type structure, which increased the hydrophilicity of the polymer association, and can thus, facilitate crosslinking. These results suggested that the DP of the polyol unit and type of bonding between the phenylboronic acid moiety and the main chain of polymers were important for the hydrogel formation.
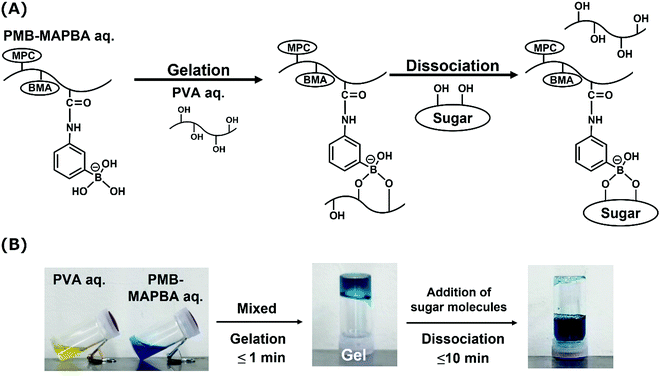 |
| Fig. 4 (A) A schematic illustration of the gelation and dissociation mechanisms of the PMB–MAPBA/PVA hydrogel. (B) Photographs of PMB–MAPBA and PVA aqueous media (labeled with dye) and the PMB–MAPBA/PVA hydrogel. The addition of sugar molecules immediately induced the dissociation of the hydrogel (in 10 min). | |
Table 2 Combination of hydrogel formation between PMB–MAPBA and PVA500
|
Concentration of PMB–MAPBA |
5 wt% |
2 wt% |
1 wt% |
0.5 wt% |
○: Gel. ×: Sol. |
Concentration PVA500 |
5 wt% |
○ |
× |
× |
× |
2 wt% |
○ |
× |
× |
× |
1 wt% |
× |
× |
× |
× |
0.5 wt% |
× |
× |
× |
× |
Table 3 Combination of hydrogel formation between PMB–MAPBA and PVA1000
|
Concentration of PMB–MAPBA |
5 wt% |
2 wt% |
1 wt% |
0.5 wt% |
○: Gel. ×: Sol. |
Concentration PVA1000 |
5 wt% |
○ |
× |
× |
× |
2 wt% |
○ |
× |
× |
× |
1 wt% |
○ |
× |
× |
× |
0.5 wt% |
○ |
× |
× |
× |
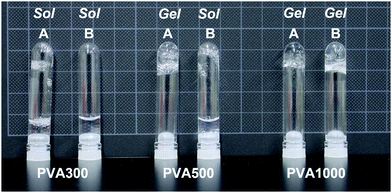 |
| Fig. 5 A photograph of hydrogels formed in test tubes. (A) PMB–MAPBA (5 wt%) or (B) PMB–VPBA (5 wt%) was mixed with 5 wt% completely saponified PVA with different three molecular weights (PVA300, PVA500, and PVA1000). | |
3.3 Rheological properties of the PMB–MAPBA hydrogels
Fig. 6 shows the results of the dynamic viscoelasticity measurements of the PMB–MAPBA/PVA hydrogels. The storage modulus (G′) was higher than the loss modulus when the concentration of PMB–MAPBA was more than 4%. The modulus increased with the increasing the concentration of PMB–MAPBA. This was thought to be due to the increase in the number of gel crosslinking points as the concentration increased. These results showed that changing the concentration of the PMB–MAPBA polymer controlled the storage modulus and loss modulus of the hydrogels.
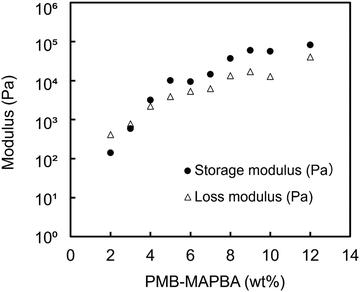 |
| Fig. 6 The rheological properties of the PMB–MAPBA/PVA hydrogels. Completely saponified PVA500 (5 wt%) was mixed with PMB–MAPBA at various concentrations. | |
3.4 The dissociation behavior of the PMB–MAPBA hydrogels
In addition to immediate hydrogel formation, the PMB–MAPBA hydrogel also shows good reversibility in response to sugar molecules. The dissociation properties of the PMB–MAPBA/PVA hydrogels were evaluated using gravimetric measurements. Fig. 7 shows that the PMB–MAPBA hydrogel was more quickly dissociated (in 10 min) than then PMB–VPBA in response to the addition of D-sorbitol. This conventional polymer hydrogel system based on VPBA as a boronic acid moiety took more than 60 min for dissociation. The MAPBA was a more hydrophilic unit than VPBA because the MAPBA unit and VPBA unit combine with phenylboronic acid moiety by an amide bond and a C–C bond, respectively. Hence, the results suggest that the hydrophilicity of the crosslinking points in the hydrogel affected the dissociation behavior of the hydrogel. From this quick dissociation in response to the addition of D-sorbitol, is was expected that the PMB–MAPBA hydrogel would achieve good cell recovery from the hydrogel. Therefore, the PMB–MAPBA gel is suitable as a material for cell immobilization. On the other hand, Fig. 8 demonstrates that the DP of PVA hardly affected the dissociation behavior. Fig. 9 shows that the dissociation of hydrogel was due to the exchange reaction between the diol group of PVA and the sugar moiety at the gel crosslinking point. Because the phenylboronic acid group had a methacrylamide-type structure, the pKa of PMB–MAPBA was lowered, which may have enabled the rapid dissociation. On the other hand, after the addition of D-glucose, no dissociation was observed within the measurement time. This was thought to be due to the weak binding constant between phenylboronic acid and D-glucose. It was also found that the binding constants between boronic acid and sugar molecules affected the dissociation behavior of the hydrogel.
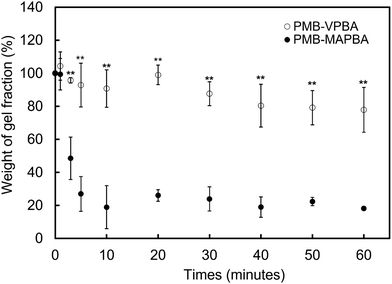 |
| Fig. 7 Stimuli-responsive dissociation behavior of PMB–MAPBA/PVA and PMB–VPBA/PVA hydrogels after the addition of D-sorbitol (1.0 M). The gels were prepared by adding 5 wt% PMB–MAPBA or PMB–VPBA solution and PVA1000 solution into a microtube. The data represent mean ± SD (n = 5, **p < 0.01, student's t-test). | |
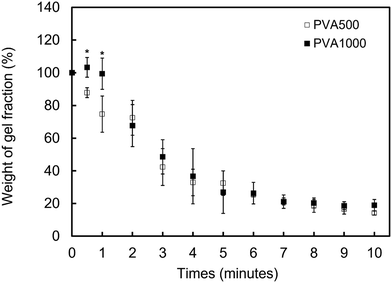 |
| Fig. 8 The stimuli-responsive dissociation behavior of the PMB–MAPBA/PVA hydrogels after the addition of D-sorbitol (1.0 M). The gels were prepared by adding 5 wt% PMB–MAPBA solution and PVA with two different degrees of polymerization into a microtube. The data represent mean ± SD (n = 5, *p < 0.05, student's t-test). | |
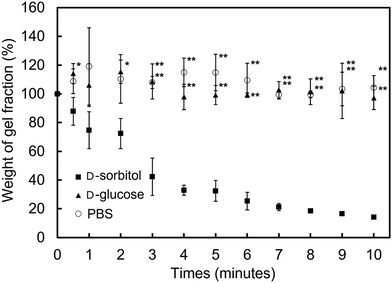 |
| Fig. 9 The stimuli-responsive dissociation of the PMB–MAPBA/PVA hydrogels in the presence of sugar molecules (1.0 M). The gels were prepared by adding 5 wt% PMB–MAPBA solution and PVA500 solution into a microtube. The data are mean ± SD (n = 5, *p < 0.05, **p < 0.01, student's t-test). | |
From these results, it was confirmed that the PMB–MAPBA/PVA hydrogel could be dissociated quickly within 10 min by the addition of D-sorbitol. This dissociation behavior was desirable for deployment of the hydrogel in the cell culture systems.
3.5 Observation of HeLa cells immobilized on PMB–MAPBA/PVA hydrogels
To estimate the feasibility of using PMB–MAPBA/PVA hydrogels as a cell culture substrate, HeLa cells were encapsulated and cultured in the hydrogel. Fig. 10 shows the phase-contrast microscopy images of the HeLa cells cultured in the PMB–MAPBA/PVA hydrogel or on tissue-culture polystyrene (TCPS) or polystyrene (PS). On PS, which is a non-treated hydrophobic polystyrene, the cells were aggregated to form spheroids. On the other hand, the cells were dispersed singly, immobilized, and showed a round shape in the PMB–MAPBA/PVA hydrogel. In addition, after 3 d, some cells were observed to be clustered together. This result suggested that cell division had occurred in the PMB–MAPBA/PVA hydrogel. Therefore, this hydrogel had good cytocompatibility as a matrix for cell culture. The recovery rate of immobilized cells by dissociation of the hydrogel was 121% (n = 3, standard deviation ±9.17%). These results indicated that cell proliferation occurred in the hydrogel and the rapid dissociation of the gel enabled efficient recovery of cells. Therefore, PMB–MAPBA/PVA hydrogels could be a promising material to immobilize cells used for biochemical assays, and drug screening.
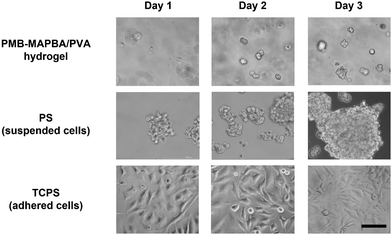 |
| Fig. 10 Phase-contrast microscope images of HeLa cells immobilized on PMB–MAPBA/PVA500 hydrogels, PS (polystyrene dish), and TCPS (tissue-cultured polystyrene dish). Scale bars represent 100 μm. | |
3.6 Recultivation of HeLa cells recovered from PMB–MAPBA hydrogels
Finally, the cell proliferation behavior of replated cells recovered from the hydrogel was investigated. Fig. 11 shows that the phase-contrast microscopy images of the cells recultured on TCPS under normal conditions for 3 d. On day 1, many round shaped cells were observed from images of the cells precultured in PMB–MAPBA/PVA hydrogels. In contrast, on days 2 and 3, the cells extended and proliferated, and the morphology of the cells was similar to that of cells in a normal culture. This suggested that the quick dissociation of the PMB–MAPBA/PVA hydrogel enabled non-invasive cell recovery of the immobilized cells to be achieved. From the previous results, it was confirmed that the cells recovered from the PMB–MAPBA/PVA hydrogel could be cultured without any adverse effects on their morphology and cell proliferation. Therefore, this hydrogel, which is capable of spontaneous formation and rapid dissociation, is useful as a substrate for cell immobilization.
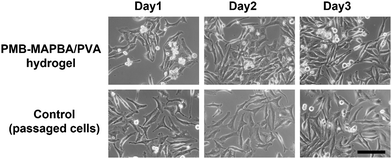 |
| Fig. 11 Phase-contrast microscope images of HeLa cells recultured on TCPS. The cells were immobilized on the PMB–MAPBA/PVA500 hydrogel and incubated for 3 d. Then, the cells were collected from the hydrogel and 7.5 × 103 cells were replated on TCPS. The scale bars represent 100 μm. | |
4. Conclusions
PMB–MAPBA was synthesized using MAPBA as the phenylboronic acid group unit. The polymer composition was almost the same as the initial monomer composition. The obtained polymer was water-soluble and formed a hydrogel spontaneously when mixing with PVA aqueous solution. PMB–MAPBA showed better performance during hydrogel formation and dissociation than PMB–VPBA did. The chain length of PVA and the type of bonding between phenylboronic acid and the main chain of the polymer played a crucial role in the formation of the hydrogel. The PMB–MAPBA/PVA hydrogels could be dissociated within 10 minutes after the addition of D-sorbitol. This quick dissociation was very effective for the recovery of immobilized cells and their re-cultivation. The immobilized cells could be recovered without any invasive damage. It was concluded that this hydrogel would be effective as a biomaterial platform for conducting three-dimensional cell engineering.
Conflicts of interest
The authors declare no conflicts of interest.
References
- K. Ishihara, Revolutionary advances in 2-methacryloyloxyethyl phosphorylcholine polymers as biomaterials, J. Biomed. Mater. Res., Part A, 2019, 105A, 933–943 CrossRef PubMed.
- K. Ishihara, Blood compatible surfaces with phosphorylcholine-based polymers for cardiovascular medical devices, Langmuir, 2019, 35(5), 1778–1787 CrossRef CAS PubMed.
- K. Ishihara, H. Oda and T. Konno, Spontaneously and reversibly forming phospholipid polymer hydrogels as a matrix for cell engineering, Biomaterials, 2020, 230, 119628 CrossRef CAS PubMed.
- K. Ishihara, M. Mu and T. Konno, Water-soluble and amphiphilic phospholipid polymers having 2-methacryloyloxyethyl phosphorylcholine unit for solubilization of bioactive compounds, J. Biomater. Sci., Polym. Ed., 2018, 29(7–9), 844–862 CrossRef CAS PubMed.
- Q. Shao and S. Jiang, Molecular understanding and design of zwitterionic materials, Adv. Mater., 2015, 27, 15–26 CrossRef CAS PubMed.
- A. L. Lewis, L. A. Tolhurst and P. W. Stratford, Analysis of a phosphorylcholine-based polymer coating on a coronary stent pre- and post-implantation, Biomaterials, 2002, 23, 1697–1706 CrossRef CAS PubMed.
- J. B. Schlenoff, Zwitteration: Coating surfaces with zwitterionic functionality to reduce nonspecific adsorption, Langmuir, 2014, 30, 9625–9636 CrossRef CAS PubMed.
- R. M. Adams, M. Wang, A. M. Crane, B. Brown, G. J. Darlington and F. D. Ledley, Effective cryopreservation and long-term storage of primary human hepatocytes with recovery of viability, differentiation, and replicative potential, Cell Transplant., 1995, 4, 579–586 CrossRef CAS PubMed.
- S. Jitraruch, A. Dhawan, R. D. Hughes, C. Filippi, S. C. Lehec, L. Glover and R. R. Mitry, Cryopreservation of hepatocyte microbeads for clinical transplantation, Cell Transplant., 2017, 26, 1341–1354 Search PubMed.
- K. Ishihara, T. Ueda and N. Nakabayashi, Preparation of phospholipid polymers and their properties as polymer hydrogel membranes, Polym. J., 1990, 22, 355–360 CrossRef CAS.
- T. Moro, Y. Takatori, K. Ishihara, T. Konno, Y. Takigawa, T. Matsushita, U. I. Chung, K. Nakamura and H. Kawaguchi, Surface grafting of artificial joints with a biocompatible polymer for preventing periprosthetic osteolysis, Nat. Mater., 2004, 3, 829–836 CrossRef CAS PubMed.
- T. Konno, J. Watanabe and K. Ishihara, Enhanced solubility of paclitaxel using water-soluble and biocompatible 2-methacryloyloxyetyl phosphorylcholine polymers, J. Biomed. Mater. Res., Part A, 2003, 65, 209–214 CrossRef PubMed.
- K. Ishihara, K. Fukazawa, V. Sharma, S. Liang, A. Shows, D. C. Dunbar, Y. Zheng, J. Ge, S. Zhang, Y. Hong, X. Shi and J. Y. Wu, Antifouling silicon hydrogel contact lenses with a bioinspired 2-methacryloyloxyethyl phosphorylcholine polymer surface, ACS Omega, 2021, 6, 7058–7067 CrossRef CAS PubMed.
- M. Kinoshita, Y. S. Kikkawa, T. Sakamoto, T. Kondo, K. Ishihara, T. Konno, N. Pawsey and T. Tamasoba, Safety, reliability, and operatability of cochlear implant electrode assays coated with biocompatible polymer, Acta Oto-Laryngol., 2015, 135, 320–327 CrossRef CAS PubMed.
- K. Ishihara, M. Mu and T. Konno, Water-soluble and amphiphilic phospholipid polymers having 2-methacryloyloxyethyl phosphorylcholine unit for solubilization of bioactive compounds, J. Biomater. Sci., Polym. Ed., 2018, 29, 844–862 CrossRef CAS PubMed.
- K. Ishihara, M. Mu, T. Konno, Y. Inoue and K. Fukazawa, The unique hydration state of poly(2-methacryloyloxyethyl phosphorylcholine), J. Biomater. Sci., Polym. Ed., 2017, 28, 884–899 CrossRef CAS PubMed.
- K. Ishihara, H. Nomura, T. Mihara, K. Kurita, Y. Iwasaki and N. Nakabayashi, Why do phospholipid polymers reduce protein adsorption?, J. Biomed. Mater. Res., 1998, 39, 323–330 CrossRef CAS PubMed.
- H. Kitano, K. Sudo, K. Ichikawa, M. Ide and K. Ishihara, Raman spectroscopic study on the structure of water in aqueous polyelectrolyte solutions, J. Phys. Chem. B, 2000, 104, 11425–11429 CrossRef CAS.
- A. S. Hoffman, Hydrogels for biomedical applications, Ann. N. Y. Acad. Sci., 2001, 944, 62–73 CrossRef CAS PubMed.
- J. L. Drury and D. J. Mooney, Hydrogels for tissue engineering: scaffold design variables and applications, Biomaterials, 2003, 24, 4337–4351 CrossRef CAS PubMed.
- W. He, M. Reaume, M. Hennenfent, B. P. Lee and R. Rajachar, Biomimetic hydrogels with sparial-and temporal-controlled chemical cues for tissue engineering, Biomater. Sci., 2020, 8, 3248–3269 RSC.
- S. C. Tang, B. M. Richardson and K. S. Anseth, Dynamic covalent hydrogels as biomaterials to mimic the viscoelasticity of soft tissues, Prog. Mater. Sci., 2021, 120, 100738 CrossRef CAS.
- V. Yesilyurt, A. M. Ayoob, E. A. Appel, J. T. Borenstein, R. Langer and D. G. Anderson, Mixed reversible covalent crosslink kinetics enable precise, hierarchical mechanical tuning of hydrogel networks, Adv. Mater., 2017, 29, 1605947 CrossRef PubMed.
- J. S. Boateng, K. H. Matthews, H. N. E. Stevens and G. M. Eccleston, Wound healing dressings and drug delivery systems: A Review, J. Pharm. Sci., 2008, 97, 2892–2923 CrossRef CAS PubMed.
- T. Vermonden, R. Censi and W. E. Hennink, Hydrogels for protein delivery, Chem. Rev., 2012, 112, 2853–2888 CrossRef CAS PubMed.
- D. Seliktar, Designing cell-compatible hydrogels for biomedical applications, Science, 2012, 336, 1124–1128 CrossRef CAS PubMed.
- Y. Yoshizaki, H. Takai, N. Mayumi, S. Fujiwara, A. Kuzuya and Y. Ohya, Cellular therapy for myocardial ischemia using a temperature-responsive biodegradable injectable polymer system with adipose-derived stem cells, Sci. Technol. Adv. Mater., 2021, 22, 627–642 CrossRef CAS PubMed.
- N. Ishiyama, T. Moro, K. Ishihara, T. Ohe, T. Miura, T. Konno, T. Ohyama, M. Kimura, M. Kyomoto, N. Nakamura and H. Kawaguchi, The prevention of peritendinous adhesions by a phospholipid polymer hydrogel formed in situ by spontaneous intermolecular interactions, Biomaterials, 2010, 31, 4009–4016 CrossRef CAS PubMed.
- S. Sawada, Y. Iwasaki, N. Nakabayashi and K. Ishihara, Stress response of adherent cells on a polymer blend surface composed of a segmented polyurethane and MPC copolymers, J. Biomed. Mater. Res., Part A, 2006, 79A, 476–484 CrossRef CAS PubMed.
- J. N. Cambre and B. S. Sumerlin, Biomedical applications of boronic acid polymers, Polymer, 2011, 52, 4631–4643 CrossRef CAS.
- D. Shiino, A. Kubo, Y. Murata, Y. Koyama, K. Kataoka, A. Kikuchi, Y. Sakurai and T. Okano, Amine effect on phenylboronic acid complex with glucose under physiological pH in aqueous solution, J. Biomater. Sci., Polym. Ed., 1996, 7, 697–705 CrossRef CAS PubMed.
- A. Kikuchi, K. Suzuki, O. Okabayashi, H. Hoshino, K. Kataoka, Y. Sakurai and T. Okano, Glucose-sensing electrode coated with polymer complex gel containing phenylboronic acid, Anal. Chem., 1996, 68, 823–828 CrossRef CAS PubMed.
- T. Konno and K. Ishihara, Temporal and spatially controllable cell encapsulation using a water-soluble phospholipid polymer with phenylboronic acid moiety, Biomaterials, 2007, 28, 1770–1777 CrossRef CAS PubMed.
- B. Gao, T. Konno and K. Ishihara, A simple procedure for the preparation of precise spatial multicellular phospholipid polymer hydrogels, Colloids Surf., B, 2013, 108, 345–351 CrossRef CAS PubMed.
- B. Gao, T. Konno and K. Ishihara, Fabrication of a live cell-containing multilayered polymer hydrogel membrane with micrometer-scale thickness to evaluate phar- maceutical activity, J. Biomater. Sci., Polym. Ed., 2015, 26, 1372–1385 CrossRef CAS PubMed.
- J. Choi, T. Konno, R. Matsuno, M. Takai and K. Ishihara, Surface immobilization of biocompatible phospholipid polymer multilayered hydrogel on titanium alloy, Colloids Surf., B, 2008, 67, 216–223 CrossRef CAS PubMed.
- T. Aikawa, T. Konno and K. Ishihara, Phospholipid polymer hydrogel microsphere modulates the cell cycle profile of encapsulated cells, Soft Matter, 2013, 9, 4628–4634 RSC.
- X. Lin, K. Nishio, T. Konno and K. Ishihara, The effect of the encapsulation of bacteria in redox phospholipid polymer hydrogels on electron transfer efficiency in living cell- based devices, Biomaterials, 2012, 33, 8221–8227 CrossRef CAS PubMed.
- H. Oda, T. Konno and K. Ishihara, The use of the mechanical microenvironment of phospholipid polymer hydrogels to control cell behavior, Biomaterials, 2013, 34, 5891–5896 CrossRef CAS PubMed.
- H. Oda, T. Konno and K. Ishihara, Efficient differentiation of stem cells encapsulated in a cytocompatible phospholipid polymer hydrogel with tunable physical properties, Biomaterials, 2015, 56, 86–91 CrossRef CAS PubMed.
- H. Oda and K. Ishihara, Determination of association constants between water-soluble phospholipid polymer bearing phenylboronic acid group and polyol compounds for reversible formation of three-dimensional networks, React. Funct. Polym., 2019, 135, 112–120 CrossRef.
Footnote |
† Electronic supplementary information (ESI) available. See DOI: 10.1039/d1tb02316k |
|
This journal is © The Royal Society of Chemistry 2022 |