DOI:
10.1039/D2DT02960J
(Paper)
Dalton Trans., 2022,
51, 16119-16125
AIE-active Ir(III) complexes functionalised with a cationic Schiff base ligand: synthesis, photophysical properties and applications in photodynamic therapy†
Received
9th September 2022
, Accepted 30th September 2022
First published on 11th October 2022
Abstract
Photodynamic therapy (PDT) is a promising cancer treatment method. Traditional small-molecule photosensitizers (PSs) suffer from low intersystem crossing (ISC) ability and aggregation-caused quenching (ACQ), which adversely affects the luminous efficiency and singlet oxygen (1O2) yield of PSs in the aggregated state. Ir(III) complexes are promising PSs with long excited-state lifetime, good photophysical and photochemical properties and large Stokes shifts. Aggregation-induced emission (AIE) characteristics could reduce the nonradiative recombination and improve the ISC ability of excited states through the restriction of the intramolecular motions in aggregated states. Accordingly, two AIE-active Ir(III) complexes Ir-1-N+ and Ir-2-N+ were successfully designed and obtained based on Schiff base ligands. Experimental results showed that Ir-1-N+ and Ir-2-N+ have good photophysical properties and the corresponding nanoparticles (NPs) have good water solubility and 1O2 generation ability. Notably, Ir-2-N+ NPs can be efficiently taken up by mouse breast cancer cells (4T1 cells) with good biocompatibility, low dark toxicity and excellent phototoxicity. This work demonstrates a versatile strategy for exploiting efficient transition metal PSs with a cationic ligand in PDT.
Introduction
Photodynamic therapy (PDT) is a new method of cancer treatment that has attracted much attention in recent years. Its main process is that photosensitizers (PSs) accumulated in the tumor produce cytotoxic reactive oxygen species (ROS) to kill cancer cells or microorganisms while irradiated by light of specific wavelengths.1–3 Nevertheless, most of the current PSs, such as boron dipyrromethene (BODIPY) and porphyrin derivatives, need long irradiation times and large amount of PSs to achieve the high generation of ROS owing to their low singlet-to-triplet intersystem crossing (ISC) ability, which limits their PDT efficacy.4–6 The ISC rate depends upon the spin–orbital coupling (SOC) interaction between the lowest triplet and one or more singlet excited states. Because the SOC constant generally increases with atomic number, large SOC interactions are found in molecules comprising heavy atoms and SOC is therefore quite large for Ir (3909 cm−1).7,8 Compared with organic small-molecule PSs, Ir(III) complexes have the benefit of larger Stokes shifts that can reduce the interference between excited and emitted states.9–11 At the same time, Ir(III) complexes also have high photochemical stability, which effectively avoids the shortcoming of photobleaching that organic small molecule PSs suffer from. In addition, long excited-state lifetime, high luminescence efficiency and tuneable emission color mean that Ir(III) complexes attract extensive attention as PSs.12–14 However, Ir(III) complexes also have several disadvantages that severely limit their efficacy in PDT such as poor water solubility, limited biocompatibility and aggregation-caused quenching (ACQ) of emission.
Many available PSs have large π-electron systems and planar molecular structures which result in ACQ.15 Meanwhile, the molecules suffer from other problems such as complicated synthetic and purification processes, poor solubility, low yield, weak absorption and low quantum efficiency.16 However, the phenomenon of aggregation-induced emission (AIE) based on restricted intramolecular motion was promoted by Ben Zhong Tang and his team in 2001
17 and has subsequently been extensively discussed and developed as an important feature in many types of luminescent molecules.18–23 PSs with AIE characteristics circumvent the above problems of traditional PSs in the aggregated state and have the following advantages: (i) improved ISC ability of excited states, (ii) high photoluminescence quantum efficiency (PLQY), (iii) high ROS generation ability.24–26 Up till now there are only very few AIE Ir(III) complexes reported as PSs, indicating that it is still a challenge to realize their potential in cell and animal imaging, bacterial therapy and anti-tumor applications.27,28
Schiff bases have the advantages of easy modification, simple and efficient synthesis method. Many Schiff bases with different properties and variable structures can be derived by changing the substituent groups.29–31 The presence of the imine bond makes Schiff bases one of the most widely used ligands in coordination chemistry with good coordination ability with most metal elements.32–34 These ligands and their complexes have a wide range of applications in the biological field, including antioxidant, anti-cancer, antibacterial and others.35–37 Therefore, Schiff bases have been used as versatile building blocks to construct AIE molecules.38–40
In this work, two AIE-active complexes Ir-1-N+ and Ir-2-N+ were obtained by a straightforward synthetic method with easy purification in high yields by using a chalcone Schiff base as auxiliary ligand.41 The cationic quaternary amine functionality in Ir-1-N+ and Ir-2-N+ was designed to enhance hydrophilicity, cellular uptake and internalization of the PSs, by analogy with other ionic transition metal complexes.42–44 The introduction of a quinolone–triphenylamine derivative as the cyclometalating ligand aims to increase the molar absorption and the singlet oxygen (1O2) generation ability of Ir-2-N+. The corresponding nanoparticles (NPs) Ir-1-N+ NPs and Ir-2-N+ NPs were prepared by self-assembly process with poloxamer (F127) as surfactant. Both Ir-1-N+ NPs and Ir-2-N+ NPs have good water solubility and 1O2 generation capacity. Spectroscopic studies and in vitro experiments show that Ir-2-N+ NPs have good biocompatibility, high photo-cytotoxicity that can be applied to PDT effects in the future.
Experimental section
Materials and instruments
Materials for organic synthesis, poloxamer (F127) and indocyanine green (ICG) were purchased from Energy Chemical Company. RPMI Medium 1640 was purchased from Solarbio Life Science company. Fetal bovine serum (FBS) was purchased from Sigma-Aldrich. 3-(4,5-Dimethylthiazol-2-yl)-2,5-diphenyltetrazolium bromide (MTT) was obtained from Aladdin. 2′,7′-Dichlorofluorescence diacetate (DCFH-DA) and the cell viability (live dead cell staining) assay kit were purchased from Shanghai Beyotime Biotechnology Co. Ltd.
1H NMR spectra were recorded at 25 °C on a Varian 600 MHz spectrometer. The high resolution mass spectra (HRMS) were obtained on a high-performance liquid chromatography-high-resolution time of flight mass spectrometer (MicroTOF II). UV-vis absorption spectra were recorded on a Shimadzu UV-3100 spectrophotometer. The photoluminescence spectra, excited state lifetimes (τ) and photoluminescence quantum yields (ΦPL) were recorded on an Edinburgh FLS920 spectrofluorimeter under air at room temperature. Transmission electron microscopy (TEM) images were taken by a TECNAI F20 microscope. Diameter and diameter distribution of the nanoparticles were determined by a Malvern Zetasizer Nano instrument for dynamic light scattering (DLS). Confocal laser scanning microscopy (CLSM) images were taken using a ZeissLSM 700 (Zurich, Switzerland).
The structures of complexes Ir-1-N+ and Ir-2-N+ are shown in Scheme 1 and the synthesis and characterization are reported in the ESI† with 13C{1H}NMR spectra (Fig. S1–S8†) and mass spectra (Fig. S9–S12†).
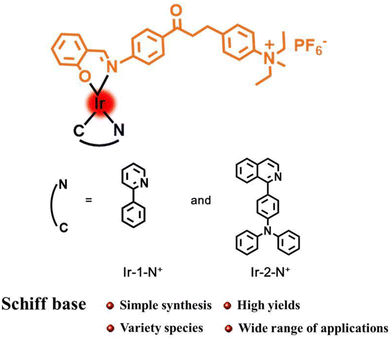 |
| Scheme 1 Structures of Ir-1-N+ and Ir-2-N+. | |
Preparation of nanoparticles (NPs)
The water-soluble NPs were prepared by the solvent exchange method. Firstly, metal iridium complex Ir-1-N+/Ir-2-N+ (1 mg) and the surfactant F127 were dissolved in THF (1 mL). The mixture was dropped into deionized water (10 mL). The solution was stirred at a constant speed to evaporate the THF; after the THF was completely volatilized, the solution was put into a dialysis bag to remove the residual THF. Further filtration was performed with a 0.22 μm filter head to obtain uniformly dispersed nanoparticles. The concentration of nanoparticles was then calculated using a standard curve. The hydrodynamic sizes and morphologies of the NPs were analyzed by dynamic light scattering (DLS) and transmission electron microscopy (TEM).
Test method for singlet oxygen generation in solution
The effect of PDT can be evaluated by the 1O2 generation capacity in the solution. In these experiments, ICG was used as an indicator to evaluate the 1O2 generation capacity of Ir-1-N+ and Ir-2-N+ and the corresponding NPs in solution. Ir-1-N+ and Ir-2-N+ solutions (15 μg mL−1 in CH3CN–water (v/v) = 4/1) and their corresponding NPs solutions (15 μg mL−1 in water) were mixed with ICG solution (5 μg mL−1) as working solutions. The working solution (3 mL) was transferred to a cuvette, and then was illuminated with a 425 nm LED at 20 mW cm−2 for 300 s.
Cell culture method
Mouse breast cancer cells (4T1 cells) were selected as the cell model for these experiments. The culture medium was prepared by RPMI Medium 1640 containing 10% (v
:
v) FBS. The cell culture flask was placed in an incubator at 37 °C and 5% CO2. To ensure adequate nutrition for the cells, the culture medium was changed every two days.
Cytotoxicity test method
The cytotoxicity of PSs was detected by MTT. 4T1 cells were seeded in 96-well plates at a density of 10
000 cells per well. The cells were cultured in an incubator at 37 °C and 5% CO2 for 24 h. After aspirating the old culture medium, RPMI Medium 1640 (100 μL) containing different concentration gradient PSs (0–10 μg mL−1) was added to each well. The original culture medium was replaced by fresh RPMI Medium 1640 (100 μL) after 6 h. The light group was irradiated with 425 nm LED at 20 mW cm−2 for 30 min, while the dark group was not illuminated. After irradiation, the cells were placed in an incubator for 24 h. MTT (10 μL) with the concentration of 5 mg mL−1 was added to each well. The cells were placed in an incubator for 4 h. After 4 h, DMSO (200 μL) was added to each well to replace the original medium. The absorbance value of the sample at 490 nm was detected by a microplate reader.
Live/dead staining test methods
4T1 cells were seeded in confocal dishes at the density of 50
000 cells per well and incubated for 24 h. After the culture medium was aspirated, RPMI Medium 1640 containing PSs (10 μg mL−1) was added to the confocal dish. After culturing for 6 h, the culture medium containing PSs was removed and fresh RPMI Medium 1640 was added. The light group was irradiated with 425 nm LED at 20 mW cm−2 for 30 min, and the dark group was treated the same without illumination. After illumination, the confocal dish was placed in the incubator and incubation was continued overnight. PBS was used to wash the confocal dishes. Detection buffer containing Calcein-AM and PI was added to the confocal dishes. This was followed by incubation in the dark in the incubator for 30 min. The fluorescence image was observed under an inverted fluorescence microscope to judge the cell survival state.
Evaluation of intracellular singlet oxygen production capacity
The ability of PSs to generate intracellular 1O2 was assessed by DCFH-DA. 4T1 cells were seeded in confocal dishes at a density of 50
000 cells per well and incubated for 24 h. The next procedure was to aspirate the original medium and add RPMI Medium 1640 (1 mL) containing PSs (10 μg mL−1) to the confocal dish. After continuing to culture in the incubator for 6 h, the supernatant was removed and RPMI 1640 was added. The light group was irradiated with 425 nm LED at 20 mW cm−2 for 15 min, and the dark group was treated in the same way without illumination. After light exposure, the original medium was aspirated, washed twice with PBS, and then DCFH-DA (1 μL) was dissolved in blank RPMI Medium 1640 without FBS and added to the confocal culture dish. After treatment in the dark for 20 min, the medium containing DCFH-DA was aspirated, washed twice with PBS (1 mL), and PBS (1 mL) was added, followed by CLSM to observe the green fluorescence intensity in the cells.
Test method for endocytic behavior
4T1 cells were seeded in confocal culture dishes at a density of 50
000 cells per well. A cell suspension (1 mL) containing RPMI Medium 1640 was added to each well. The cells were then placed in an incubator at 37 °C and 5% CO2 overnight. The original medium was replaced by RPMI Medium 1640 containing PSs (10 μg mL−1). The incubation time was 0.5 h, 2 h and 6 h, respectively. After incubation, the supernatant was withdrawn and the confocal culture dish was washed twice with PBS, and the uptake of PSs by cells was observed by CLSM.
Results and discussion
Photophysical properties
The photophysical properties of Ir-1-N+ and Ir-2-N+ were analyzed by UV-Vis absorption and emission spectroscopy. As shown in Fig. 1, both Ir-1-N+ and Ir-2-N+ have two absorption bands. The relatively strong absorption band in the range of 250–350 nm originates from the π–π* transition of the ligand center. The relatively weak absorption band in the 350–500 nm range can be attributed to spin-allowed metal-to-ligand charge-transfer transition (1MLCT), spin-allowed ligand-to-ligand charge-transfer transition (1LLCT), spin-forbidden metal-to-ligand charge-transfer transition (3MLCT) and spin-forbidden ligand-to-ligand charge transfer transition (3LLCT).10 From Fig. 1, the absorbance of Ir-2-N+ at 425 nm is significantly enhanced due to the introduction of the extended π-conjugated cyclometalated ligand (quinoline triphenylamine) in Ir-2-N+.45 Both complexes exhibit red emission at excitation wavelength 425 nm. The maximum emission peak of Ir-1-N+ is at 640 nm and Ir-2-N+ is at 660 nm, with the red-shifted emission of Ir-2-N+ ascribed to the π-extended ligand which is consistent with the changing trends of the UV-Vis absorption spectra of the two complexes. Compared with Ir-1-N+, Ir-2-N+ shows a larger Stokes shift (245 nm). This is important because PSs with a large Stokes shift can improve the signal-to-noise ratio of imaging by reducing the interference from the background.46 The photoluminescence quantum yields (PLQYs) and excited-state lifetimes of Ir-1-N+ and Ir-2-N+ were determined, and their corresponding photophysical data are summarized in Table S1.† The Ir(III) complexes exhibit high PLQYs and relatively long excited-state lifetimes. The PLQYs of Ir-1-N+ and Ir-2-N+ are 17% and 19%, respectively, and the excited-state lifetimes are 542.2 ns and 551.3 ns, respectively. Their excellent photophysical properties, especially the enhanced absorption band and large Stokes shift and long excited-state lifetime, endow the complexes with high potential as PSs.
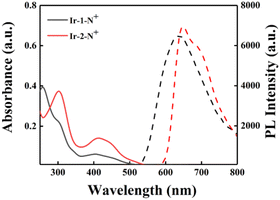 |
| Fig. 1 The UV–vis absorption spectra in CH3CN (solid line) and the PL spectra in the mixed solvent (CH3CN–H2O = 1 : 9) of Ir-1-N+ and Ir-2-N+ (10−5 M) (dashed line). | |
Research on AIE phenomenon
The AIE performance of Ir-1-N+ and Ir-2-N+ was analysed in mixed solutions of CH3CN and water, while the water content was varied in the range of 0–99%. As shown in Fig. 2, Ir-1-N+ and Ir-2-N+ show weak emission in pure CH3CN, but emit bright red phosphorescence when the water content gradually increases to 80%, which is a typical AIE characteristic.47 The AIE properties of Ir-1-N+ and Ir-2-N+ were further explored by transmission electron microscopy (TEM) and UV-Vis absorption spectroscopy. The morphology and particle size are shown in Fig. S14.† Only a very few small aggregates were observed in pure CH3CN. When the water content is 99%, the agglomerates increase significantly and become larger. From the UV-Vis absorption spectra (Fig. S15†) the tail of the absorption spectrum is enhanced with the gradual increase of the water content, indicating the Mie scattering effect.48Ir-1-N+ and Ir-2-N+ possess excellent AIE characteristics according to the above results, originating from the effective restriction of nonradiative transitions, and these properties are beneficial for the ISC process and the generation of ROS.
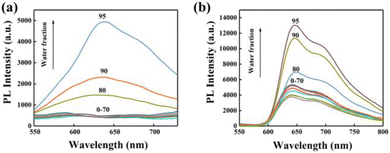 |
| Fig. 2 PL spectra of Ir-1-N+ (a) and Ir-2-N+ (b) in CH3CN–H2O mixtures (complex concentration = 1.0 × 10−5 M) with different water fractions (0–95% v/v) at room temperature. | |
Characterization of nanoparticles
Two water-soluble nanoparticles, Ir-1-N+ NPs and Ir-2-N+ NPs, were prepared by a solvent exchange method. As shown in Fig. S16,† the hydrated particle size of the NPs was measured using DLS. The data showed that the average particle sizes of Ir-1-N+ NPs and Ir-2-N+ NPs were 92 nm and 63 nm, respectively. According to the TEM images (Fig. 3), the NPs are spherical and the particle size is <100 nm. These properties make the NPs easily endocytosed by cells. Within a week, Ir-1-N+ NPs and Ir-2-N+ NPs exhibited negligible change in size and polydispersity indices (PDIs), indicating their good stability. The high stability of NPs facilitates better circulation in the blood. Therefore, Ir-1-N+ NPs and Ir-2-N+ NPs with good water solubility, suitable size and high stability are promising candidates for use in living cells and animal experiments.49
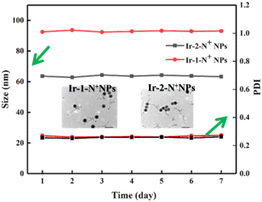 |
| Fig. 3 DLS data of Ir-1-N+ NPs and Ir-2-N+ NPs during 7 days. Inset: TEM images of Ir-1-N+ NPs and Ir-2-N+ NPs. | |
Evaluation of singlet oxygen generation capability in solution
The ability to generate 1O2 is an important parameter to assess the effect of PDT. The 1O2 generation capacity of Ir-1-N+, Ir-2-N+ and their corresponding NPs was evaluated by monitoring the change in the absorbance of ICG at 790 nm. The data are shown in Fig. 4 and S17–S20,† for (1) the unirradiated group containing ICG and PSs; (2) the irradiated group containing only PSs; and (3) the irradiated group containing only ICG. The absorbance of ICG has no change within 300 s, indicating that Ir-1-N+ and Ir-2-N+ and their corresponding NPs have good photostability. Under irradiation conditions, the absorbance of ICG in the presence of Ir-1-N+ and Ir-2-N+ decreased. This reveals that 1O2 is generated in irradiation conditions rather than in dark conditions. Compared with Ir-1-N+, Ir-2-N+ shows higher 1O2 generation ability, which could be because the absorbance of Ir-2-N+ at 425 nm is higher than that of Ir-1-N+. After Ir-1-N+ and Ir-2-N+ were fabricated into NPs, their 1O2 generation capacity was significantly higher than those of the corresponding “free” complexes. The data suggests that the ability to generate 1O2 is increased in the aggregated state (Fig. 4). The 1O2 generation capacities of Ir-1-N+ and Ir-2-N+ and their corresponding NPs conform to first-order kinetics, with the slope Ir-2-N+ NPs > Ir-2-N+ > Ir-1-N+ NPs > Ir-1-N+. The larger the slope, the faster the degradation rate of ICG, indicating the greater ability to generate 1O2. Obviously, Ir-2-N+ NPs exhibited improved singlet oxygen generation ability and they have a high potential as PSs for clinical applications in the future. The above experiments establish that charged Ir(III) complexes with AIE properties based on a rational molecular design strategy demonstrate improved 1O2 generation ability for such kind of PSs.
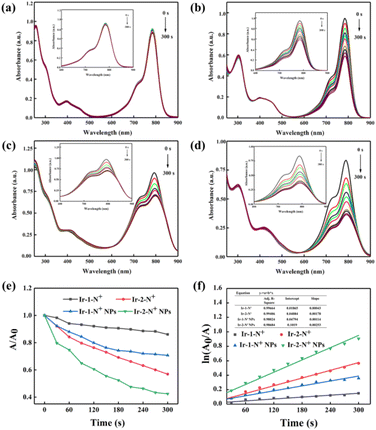 |
| Fig. 4 The UV–vis absorption spectra of ICG (5 μg mL−1) with Ir-1-N+ (a), Ir-2-N+ (b) Ir-1-N+ NPs (c) and Ir-2-N+ NPs (d). (e) The decay rates of ICG in the presence of Ir-1-N and Ir-2-N and their NPs upon exposure to light illumination (425 nm, 20 mW cm−2). (f) Time-dependent 1O2 generation kinetics. A0 = initial absorbance maximum of ICG. A = real-time absorbance maximum of ICG with various light exposure times. An enlarged inset table is shown as Table S2 in ESI.† | |
Cytotoxicity studies
The cytotoxicity of Ir-2-N+ NPs in 4T1 cells was analyzed by using the 3-(4,5-dimethylthiazol-2-yl)-2,5-diphenyltetrazolium bromide (MTT) assay,50 which is a quantitative colorimetric method. MTT is converted into purple formazan crystals by metabolizing the mitochondrial dehydrogenase of living cells, then dimethyl sulfoxide can dissolve the generated formazan. As shown in Fig. 5a, after the 4T1 cells were incubated overnight, they were treated with Ir-2-N+ NPs and had a viability of more than 90%, showing good biocompatibility and low dark toxicity. When irradiated by a 425 nm LED at 20 mW cm−2, the cell viability decreased gradually with the increasing concentration of Ir-2-N+ NPs, showing a concentration-dependent cellular phototoxicity. When the concentration of Ir-2-N+ NPs reached 10 μg mL−1, the cell viability was only 10% under irradiation. The MTT experiments therefore showed that Ir-2-N+ NPs exhibited good phototoxicity and low dark toxicity in cell experiments.
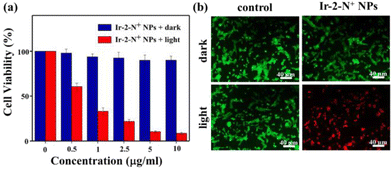 |
| Fig. 5 (a) Relative viability of 4T1 cells after 24 h co-incubation with Ir-2-N+ NPs under darkness and under irradiation (425 nm, 20 mW cm−2). (b) Confocal fluorescence images of 4T1 cells co-stained with Calcein-AM (live cells, green fluorescence) and propidium iodide (dead cells, red fluorescence) after treatment with Ir-2-N+ NPs (10 μg mL−1). | |
Live/dead cell staining experimental study
We used the Calcein-AM and propidium iodide (PI) double fluorescence staining method to detect the PDT effect of Ir-2-N+ NPs in 4T1 cells.51 Calcein-AM is a cell-permeable dye that is widely used to detect viability in most eukaryotic cells. PI cannot pass through the cell membrane of living cells and only can enter damaged cells, where it binds to nucleic acids and emits red fluorescence. Calcein-AM and PI were used simultaneously to detect live/dead cells. As shown in Fig. 5b, a strong green fluorescence signal and a very weak red fluorescence signal were observed in the dark for the blank control group and Ir-2-N+ NPs, indicating that Ir-2-N+ NPs have lower dark toxicity and good biocompatibility. However, strong red fluorescence signals and negligible green fluorescence signals were observed in 4T1 cells pre-treated with Ir-2-N+ NPs under irradiation, indicating that almost all 4T1 cells were killed. Both live/dead cell staining experiments and MTT experiments showed that the conversion of AIE iridium complexes into the corresponding NPs can effectively improve the biocompatibility and PDT effect of the complexes.
Evaluation of intracellular singlet oxygen production capacity
2,7-Dichlorodihydrofluorescein diacetate (DCFH-DA) does not emit light. However, when it enters cells, it is hydrolyzed by intracellular esterase and converted to 2,7-dichlorodihydrofluorescein (DCFH) which can react with 1O2 to generate the green fluorescent molecule 2,7-dichlorofluorescein (DCF).52 The greater the amount of singlet oxygen, the stronger the fluorescent signal generated. The intensity of the green fluorescence can be used to judge the ability of the PSs to generate 1O2 in the cell. Therefore, we used DCFH-DA to detect the formation of 1O2 in 4T1 cells after illumination. As shown in Fig. 6, no green fluorescence was produced in the blank control and Ir-2-N+ NPs under dark conditions. After 15 min of illumination, the cells in the Ir-2-N+ NPs group showed obvious green fluorescence, while the blank control group still had no fluorescence. The result shows that Ir-2-N+ NPs cannot produce 1O2 under dark conditions, but they can effectively generate 1O2 in the cells after illumination. The good 1O2 generation ability of Ir-2-N+ NPs indicates that Ir-2-N+ NPs have good application potential as PSs in PDT.
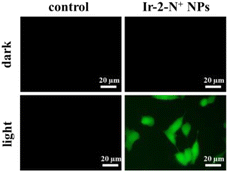 |
| Fig. 6 Confocal fluorescence images for the detection of 1O2 generation in 4T1 cells treated with Ir-2-N+ NPs under darkness and under irradiation (425 nm, 20 mW cm−2). | |
Endocytosis studies
We further characterized the cellular uptake behavior of Ir-2-N+ NPs using CLSM (Fig. 7). Since the particle size of Ir-2-N+ NPs is <100 nm, they can readily enter cells. The phosphorescence signal generated in the cells gradually increased with the extension of the culture time, indicating that the cellular uptake was time-dependent. At 6 h, 4T1 cells incubated with Ir-2-N+ NPs had a strong red phosphorescence signal due to the large Stokes shift of Ir-2-N+, which gives a good imaging effect. Endocytosis experiments demonstrated that Ir-2-N+ NPs could be efficiently taken up by 4T1 cells and had good biocompatibility. The efficient uptake of PSs by cells is a premise for good PDT.
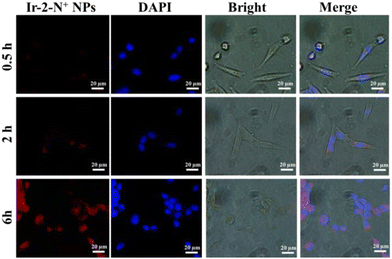 |
| Fig. 7 Cellular uptake of Ir-2-N+ NPs (10 μg mL−1) detected by CLSM at different periods of time. | |
Conclusions
In summary, two Ir(III) complexes with a pendant quaternary ammonium cation and their NPs with AIE properties were designed and synthesized utilizing a Schiff base ligand. The synthetic routes to Ir-1-N+ and Ir-2-N+ are simple and high yielding. The complexes were successfully used as PSs in the study of PDT. The introduction of quinoline triphenylamine as the cyclometalated ligands enhances the absorption of Ir-2-N+; the emission is red-shifted and the ability to generate 1O2 is significantly improved relative to the phenylpyridine analogue Ir-1-N+. Owing to the AIE features of the Ir(III) complexes, the Ir-1-N+ NPs and Ir-2-N+ NPs, which are in the aggregated state of Ir-1-N+ and Ir-2-N+, exhibit higher 1O2 generation capacity than their corresponding small molecules. Among them, Ir-2-N+ NPs which exhibit bright red emission, efficient 1O2 generation ability, good water solubility, excellent phototoxicity, and negligible dark toxicity are shown to be good PSs in vitro for PDT. This work provides a meaningful design strategy for obtaining AIE self-assembled cationic transition metal complex NPs with red light emission as efficient PSs for PDT.
Conflicts of interest
There are no conflicts to declare.
Acknowledgements
This work was funded by NSFC (Grants No. 52073045, 51773195, 62075217), the Key Scientific and Technological Project of Jilin Province (20190701010GH), Jilin Provincial Department of Science and Technology (Grants 20210101148JC), the Development and Reform Commission of Jilin Province (2020C035-5), and Changchun Science and Technology Bureau (CC202110378310002101). M. R. B. thanks EPSRC (UK) grant EP/L02621X/1 for funding. D. Zhu is grateful for the support from the Key Laboratory of Nanobiosensing and Nanobioanalysis at the Universities of Jilin Province. The authors acknowledge the support from the Jilin Provincial Department of Education.
References
- H. Zhao, L. Li, F. Li, C. Liu, M. Huang, J. Li, F. Gao, X. Ruan and D. Yang, Adv. Mater., 2022, 34, e2109920 CrossRef PubMed.
- D. Wang, H. Wang, L. Ji, M. Xu, B. Bai, X. Wan, D. Hou, Z. Y. Qiao, H. Wang and J. Zhang, ACS Nano, 2021, 15, 8694–8705 CrossRef CAS PubMed.
- M. Huo, P. Liu, L. Zhang, C. Wei, L. Wang, Y. Chen and J. Shi, Adv. Funct. Mater., 2021, 31, 2010196 CrossRef CAS.
- Z. Yu, H. Wang, Z. Chen, X. Dong, W. Zhao, Y. Shi and Q. Zhu, J. Med. Chem., 2022, 65, 3616–3631 CrossRef CAS PubMed.
- V. Ramu, P. Kundu, P. Kondaiah and A. R. Chakravarty, Inorg. Chem., 2021, 60, 6410–6420 CrossRef CAS PubMed.
- Y. X. Li, Y. Liu, H. Wang, Z. T. Li and D. W. Zhang, ACS Appl. Bio Mater., 2022, 5, 881–888 CrossRef CAS PubMed.
-
Iridium(III) in Optoelectronic and Photonics Applications, ed. E. Zysman-Colman, John Wiley and Sons, Chichester, 2017 Search PubMed.
-
M. Montalti, A. Credi, L. Prodi and M. T. Gandolfi, Handbook of Photochemistry, Taylor and Francis, Boca Raton, FL, 3rd edn, 2006 Search PubMed.
- G. Lu, Z. G. Wu, R. Wu, X. Cao, L. Zhou, Y. X. Zheng and C. Yang, Adv. Funct. Mater., 2021, 31, 2102898 CrossRef CAS.
- Y. Luo, Z. Liu, G. Yang, T. Wang, Z. Bin, J. Lan, D. Wu and J. You, Angew. Chem., Int. Ed., 2021, 60, 18852–18859 CrossRef CAS PubMed.
- H. Shin, Y. H. Ha, H. G. Kim, R. Kim, S. K. Kwon, Y. H. Kim and J. J. Kim, Adv. Mater., 2019, 31, e1808102 CrossRef PubMed.
- C. Jin, G. Li, X. Wu, J. Liu, W. Wu, Y. Chen, T. Sasaki, H. Chao and Y. Zhang, Angew. Chem., Int. Ed., 2021, 60, 7597–7601 CrossRef CAS PubMed.
- J. Shen, J. Karges, K. Xiong, Y. Chen, L. Ji and H. Chao, Biomaterials, 2021, 275, 120979 CrossRef CAS PubMed.
- J. Zhao, K. Yan, G. Xu, X. Liu, Q. Zhao, C. Xu and S. Gou, Adv. Funct. Mater., 2020, 31, 2008325 CrossRef.
- K. Zheng, G. B. Bodedla, Y. Hou, J. Zhang, R. Liang, J. Zhao, D. Lee Phillips and X. Zhu, J. Mater. Chem. A, 2022, 10, 4440–4445 RSC.
- B. M. Luby, C. D. Walsh and G. Zheng, Angew. Chem., Int. Ed., 2019, 58, 2558–2569 CrossRef CAS PubMed.
- J. Luo, Z. Xie, J. W. Lam, L. Cheng, H. Chen, C. Qiu, H. S. Kwok, X. Zhan, Y. Liu, D. Zhu and B. Z. Tang, Chem. Commun., 2001, 1740–1741 RSC.
- J. Mei, N. L. C. Leung, R. T. K. Kwok, J. W. Y. Lam and B. Z. Tang, Chem. Rev., 2015, 115, 11718–11940 CrossRef CAS PubMed.
- L. Meng, X. Ma, S. Jiang, S. Zhang, Z. Wu, B. Xu, Z. Lei, L. Liu and W. Tian, CCS Chem., 2021, 3, 2084–2094 CrossRef CAS.
- S. Du, G. Pan, S. Dai, B. Xu and W. Tian, Aggregate, 2021, 2, e96 Search PubMed.
- F. Würthner, Angew. Chem., Int. Ed., 2020, 59, 14192–14196 CrossRef PubMed.
- G. R. Suman, M. Pandey and A. S. J. Chakravarthy, Mater. Chem. Front., 2021, 5, 1541–1584 RSC.
- Y. Luo, S. Zhang, H. Wang, Q. Luo, Z. Xie, B. Xu and W. Tian, CCS Chem., 2022, 4, 456–463 CrossRef CAS.
- D. Mao and B. Liu, Matter, 2021, 4, 350–376 CrossRef CAS.
- D. Li, Y. Li, Q. Wu, P. Xiao, L. Wang, D. Wang and B. Z. Tang, Small, 2021, 17, e2102044 CrossRef PubMed.
- Q. Wan, R. Zhang, Z. Zhuang, Y. Li, Y. Huang, Z. Wang, W. Zhang, J. Hou and B. Z. Tang, Adv. Funct. Mater., 2020, 30, 2002057 CrossRef CAS.
- L. Zhang, Y. Li, W. Che, D. Zhu, G. Li, Z. Xie, N. Song, S. Liu, B. Z. Tang, X. Liu, Z. Su and M. R. Bryce, Adv. Sci., 2019, 6, 1802050 CrossRef PubMed.
- J. Zhao, S. Sun, X. Li, W. Zhang and S. Gou, ACS Appl. Bio Mater., 2020, 3, 252–262 CrossRef CAS PubMed.
- H. Chen, C. Huang, Y. Deng, Q. Sun, Q. L. Zhang, B. X. Zhu and X. L. Ni, ACS Nano, 2019, 13, 2840–2848 CrossRef CAS PubMed.
- J. Zhang, L. Xu and W.-Y. Wong, Coord. Chem. Rev., 2018, 355, 180–198 CrossRef CAS.
- T. A. J. Grell, A. P. Young, C. L. Drennan and V. Bandarian, J. Am. Chem. Soc., 2018, 140, 6842–6852 CrossRef CAS PubMed.
- M. A. Malik, S. A. Lone, M. Y. Wani, M. I. A. Talukdar, O. A. Dar, A. Ahmad and A. A. Hashmi, Bioorg. Chem., 2020, 98, 103771 CrossRef CAS PubMed.
- R. E. Malekshah, F. Shakeri, A. Khaleghian and M. Salehi, Int. J. Biol. Macromol., 2020, 152, 846–861 CrossRef CAS PubMed.
- X. Liu and J.-R. Hamon, Coord. Chem. Rev., 2019, 389, 94–118 CrossRef CAS.
- D. Ling, H. Li, W. Xi, Z. Wang, A. Bednarkiewicz, S. T. Dibaba, L. Shi and L. Sun, J. Mater. Chem. B, 2020, 8, 1316–1325 RSC.
- Y. Liang, Z. Li, Y. Huang, R. Yu and B. Guo, ACS Nano, 2021, 15, 7078–7093 CrossRef CAS PubMed.
- Y. Liang, M. Li, Y. Yang, L. Qiao, H. Xu and B. Guo, ACS Nano, 2022, 16, 3194–3207 CrossRef CAS PubMed.
- S. Guieu, F. Cardona, J. Rocha and A. M. S. Silva, Chem. – Eur. J., 2018, 24, 17262–17267 CrossRef CAS PubMed.
- D. Li, X. Liang, F. Zhang, J. Li, Z. Zhang, S. Wang, Z. Li, Y. Xing and K. Guo, J. Mater. Chem. C, 2022, 10, 11016–11026 RSC.
- S. Lin, H. Pan, L. Li, R. Liao, S. Yu, Q. Zhao, H. Sun and W. Huang, J. Mater. Chem. C, 2019, 7, 789–7899 Search PubMed.
- S. Liu, J. Han, Y. Chang, W. Wang, R. Wang, Z. Wang, G. Li, D. Zhu and M. R. Bryce, Chem. Commun., 2022, 58, 10056–10059 RSC.
- R. E. Daniels, L. K. McKenzie, J. R. Shewring, J. A. Weinstein, V. N. Kozhevnikov and H. E. Bryant, RSC Adv., 2018, 8, 9670–9676 RSC.
- Y. Ma, S. Zhang, H. Wei, Y. Dong, L. Shen, S. Liu, Q. Zhao, L. Liu and W.-Y. Wong, Dalton Trans., 2018, 47, 5582–5588 RSC.
- B. Hou, W. Zhang, C. Li, X. Sun, X. Feng and J. Liu, Appl. Organomet. Chem., 2022, 36, e6598 CrossRef CAS.
- Z. Liu, S. W. Zhang, M. Zhang, C. Wu, W. Li, Y. Wu, C. Yang, F. Kang, H. Meng and G. Wei, Front. Chem., 2021, 9, 758357 CrossRef CAS PubMed.
- W. Wang, K. J. Wu, K. Vellaisamy, C. H. Leung and D. L. Ma, Angew. Chem., Int. Ed., 2020, 59, 17897–17902 CrossRef CAS PubMed.
- Y. Wang, T. Yang, X. Liu, G. Li, W. Che, D. Zhu and Z. Su, J. Mater. Chem. C, 2018, 6, 12217–12223 RSC.
- B. Xu, W. Li, J. He, S. Wu, Q. Zhu, Z. Yang, Y. C. Wu, Y. Zhang, C. Jin, P. Y. Lu, Z. Chi, S. Liu, J. Xu and M. R. Bryce, Chem. Sci., 2016, 7, 5307–5312 RSC.
- Z. Zhang, W. Xu, M. Kang, H. Wen, H. Guo, P. Zhang, L. Xi, K. Li, L. Wang, D. Wang and B. Z. Tang, Adv. Mater., 2020, 32, e2003210 CrossRef PubMed.
- H. Lu, X. Jiang, Y. Chen, K. Peng, Y. Huang, H. Zhao, Q. Chen, F. Lv, L. Liu, S. Wang and Y. Ma, Nanoscale, 2020, 12, 14061–14067 RSC.
- N. K. Pandey, W. Xiong, L. Wang, W. Chen, B. Bui, J. Yang, E. Amador, M. Chen, C. Xing, A. A. Athavale, Y. Hao, W. Feizi and L. Lumata, Bioact. Mater., 2022, 7, 112–125 CrossRef CAS PubMed.
- J. Deng, H. Li, M. Yang and F. Wu, Photochem. Photobiol. Sci., 2020, 19, 905–912 CrossRef CAS PubMed.
Footnotes |
† Electronic supplementary information (ESI) available: Supporting figures and tables. See DOI: https://doi.org/10.1039/d2dt02960j |
‡ The authors contributed equally to the preparation of this work. |
|
This journal is © The Royal Society of Chemistry 2022 |
Click here to see how this site uses Cookies. View our privacy policy here.