DOI:
10.1039/D0SC04699J
(Edge Article)
Chem. Sci., 2021,
12, 552-558
Synthesis, crystal structure and charge transport characteristics of stable peri-tetracene analogues†
Received
26th August 2020
, Accepted 13th October 2020
First published on 14th October 2020
Abstract
peri-Acenes have shown great potential for use as functional materials because of their open-shell singlet biradical character. However, only a limited number of peri-acene derivatives larger than peri-tetracene have been synthesized to date, presumably owing to the low stability of the target compounds in addition to the complicated synthesis scheme. Here, a very simple synthesis route for the tetrabenzo[a,f,j,o]perylene (TBP) structure enables the development of highly stable peri-tetracene analogues. Despite a high degree of singlet biradical character, the compounds with four substituents at the zigzag edge show a remarkable stability in solution under ambient conditions, which is better than that of acene derivatives with a closed-shell electronic configuration. The crystal structures of the TBP derivatives were obtained for the first time; these are valuable to understand the relationship between the structure and biradical character of peri-acenes. The application of peri-acenes in electronic devices should also be investigated. Therefore, the semiconducting properties of the TBP derivative were investigated by fabricating the field-effect transistors.
Introduction
Acenes and functionalized acenes, which are a class of polycyclic aromatic hydrocarbons (PAHs) with laterally fused benzene rings, have received considerable attention because of their attractive properties that include high mobility and efficient multi-excitonic processes like singlet fission and triplet–triplet upconversion.1–3 Thus, they have been applied to various organic electronic devices such as organic field-effect transistors (OFETs)4 and organic light-emitting diodes (OLEDs).5 Larger acenes having more than six benzene rings are known to have an open-shell (OS) singlet biradical character and are also promising for use in optoelectronic devices. However, their intrinsically low stability has hindered a detailed study of these materials.6
Additionally, interest in peri-fused systems, so-called peri-acenes and acenoacenes, has been increasing because such zigzag-edged PAHs are considered to be nanographenes.7 Examples of peri-acenes are shown in Fig. 1, where the compounds have a conjugated system with two rows of acenes. Although perylene8 and bisanthene,9 as the smaller π-systems, have been known for a long time, teranthene,10 tetrabenzo[a,f,j,o]perylene (TBP)11 and peri-tetracene12,13 were synthesized only very recently, and peri-pentacenes have only been obtained in ultrahigh vacuum conditions.14 These larger π-systems can exhibit a significant singlet biradical feature and unique properties. The OS singlet biradical contributions in their resonance structures result from the additional Clar's aromatic sextets in the biradical form. Fig. 1b shows the resonance structures of TBP, where the closed-shell (CS) form has two aromatic sextets while the OS form has five aromatic sextets, which indicates a large biradical character for TBP. Note that the radicals in the allylic positions can be delocalized and stabilized, which results in a large spin density at the four carbon atoms found at the zigzag edges. The resonance structures of peri-tetracene are essentially similar to that of TBP, therefore referred to as peri-tetracene analogue for TBP in this paper, although the biradical character of peri-tetracene is higher than that of TBP because of the larger conjugation in the planar structure of peri-tetracene. To date, there have been few examples of the precisely synthesized and isolated derivatives of TBP and peri-tetracene (Fig. 1c).11,12 Because singlet biradical materials are generally unstable, these three molecules also decompose quickly in solution. Therefore, the stability needs to be improved for their further applications in electronic devices. However, their complicated synthesis schemes hinder the preparation of derivatives with various substituents. Consequently, the properties of peri-tetracene analogues are not fully understood.
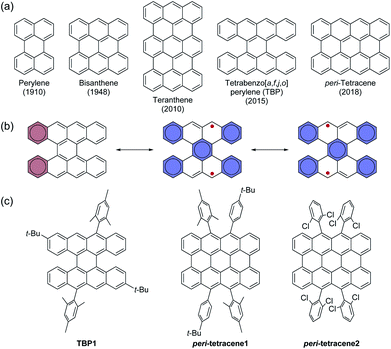 |
| Fig. 1 (a) Chemical structures of peri-acenes. (b) Resonance structures of TBP and peri-tetracene. (c) Structures of the reported TBP and peri-tetracene derivatives. | |
In this study, we developed a simple synthetic route for various TBP derivatives, which can potentially be used for the synthesis of peri-tetracenes. The synthesis scheme allows us to obtain TBP derivatives with two or four substituents at the 9,20- or 9,10,19,20-positions, respectively, which results in a significant improvement of the stability. In addition, OFET devices comprising the TBP derivatives have been investigated and show a good bipolar charge transport property despite the non-planar structure of the TBP core.
Results and discussion
Synthesis
The synthesis procedures for the new TBP derivatives (TBP2–TBP5) are shown in Scheme 1 and Method S1 (see the ESI†). The key intermediate in our strategy was compound 4 (tetrabenzo[a,f,j,o]perylene-9,20-dione) based on the fact that many acene derivatives can be synthesized from acene quinones.15 This also enabled the introduction of various substituents/protecting groups in the last step, in contrast to the synthesis methods for the previously reported TBP1 and peri-tetracenes, which require the introduction of substituents in the early steps. We found that 4 was formed as a trace byproduct under the reaction conditions (at 100 °C for 5 h) for the synthesis of 2 and 3,16 and 4 could be obtained as the main product by optimizing the reaction temperature and time (at 120 °C for 48 h). Compound 4 was also obtained from an isolated mixture of 2 and 3 (inseparable E and Z isomers), which suggested a sequential two-step reaction from 1. According to Clar's rule,174 is considered to have a large contribution of the resonance structure with five aromatic sextets, which results in a clear α,β-unsaturated carbonyl structure (see Crystal structure section below). For the reaction between 4 and organolithium reagents, both 1,2-addition (a direct nucleophilic attack at the carbonyl group) and 1,4-addition (Michael addition) were observed depending on the nucleophiles involved. Similar to the synthesis of TIPS-pentacene from pentacenequinone,18 TIPS-acetylene-substituted TBP (TBP2) was synthesized by the nucleophilic 1,2-addition of lithium acetylide, followed by reduction using SnCl2 in low yield (<1%), where the products of the 1,4-addition were obtained in trace amounts. Although lithium and Grignard reagents tend to promote a 1,2-addition, aryl lithium and aryl Grignard reagents with 4 lead to Michael addition rather than 1,2-addition to give compounds 5a and 5b as the main products with a small amount of by-products from a 1,2-addition, probably owing to the steric congestion from the rigid core structure.19 Therefore, four-substituted TBP (TBP3–TBP5) could be synthesized through a second nucleophilic addition.18 Therefore, we successfully obtained new TBP derivatives in a few short steps. Although we focused on TBP derivatives in this work, 4 will undergo intramolecular coupling by a Scholl reaction to give a fully planar fused compound (see the ESI†), which can be used as a precursor of peri-tetracene.
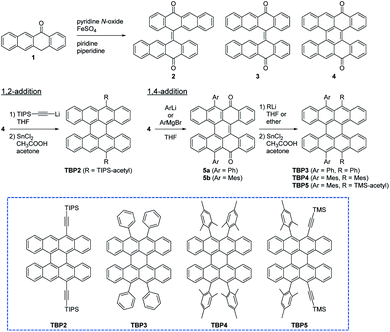 |
| Scheme 1 Chemical structures of new TBP derivatives (TBP2–5) and synthesis routes. | |
Optical and electrochemical properties and stability test
Because all of the new compounds (TBP2–TBP5) are based on the TBP core, their properties, which include the biradical character, should be similar to those of TBP1, which has already been determined to have an OS singlet biradical nature by UV-vis absorption spectroscopy, theoretical calculations, electron spin resonance (ESR) spectroscopy and variable-temperature (VT) NMR spectroscopy.11 To realize stable singlet biradical compounds, it is important to protect the position with a high spin density (9,10,19,20-positions of the TBP core). TBP2 has a thermodynamic stabilization that extends the conjugation and delocalizes radicals by the introduction of substituents, such as acetylene, although only two positions are protected. On the other hand, TBP1, TBP3 and TBP4 are kinetically stabilized by bulky protecting groups. A mesityl group is bulkier than a phenyl group, which indicates a better protection for the singlet biradical of TBP4. TBP5 is expected to be stabilized by both a thermodynamic and kinetic approach.
Fig. 2 shows UV-vis absorption spectra in dichloromethane (DCM). The solutions of TBP2–TBP5 were green because of the absorption band in the range of the red to near-infrared (NIR) region. The results clearly showed a red shift of the lowest energy band in the NIR region for the compounds with acetyl groups. The absorption maxima (λmax) were 765, 733, 729 and 783 nm for TBP2–TBP5, respectively, which indicated a narrow HOMO–LUMO gap and high biradical character for these compounds that were similar to those for TBP1 with an absorption maximum at 727 nm. In fact, the theoretical calculations of the biradical character (y0) using Yamaguchi's scheme at the UHF/6-31G(d,p) level were 0.60, 0.62, 0.60, 0.59 and 0.62 for TBP1–TBP5, respectively (full data of the calculations can be found in the ESI, Methods S2, Tables S1–S4, and Fig. S1–S4†).20 However, these singlet biradical features generally led to a fast decomposition of the compounds by photooxidation in air. In fact, the absorption spectra of most of the acenes quickly changed, which indicated a low stability. The changes of the absorption intensity at λmax under white room light in ambient air are summarized in Fig. 2b and each spectrum is shown in Fig. 2c–f. These results demonstrated the significant difference in stability of these compounds. The decompositions of TBP2 and TBP3 took place on a similar time scale, which was faster than that of rubrene,21 because of the higher singlet biradical character of the peri-acenes. Thus, the absorption intensity almost disappeared after several hours, as shown in Fig. 2c and d. The colour of the solution changed to yellow, then red-purple, likely owing to the addition of oxygen and the formation of carbonyl groups (Fig. S5 in the ESI†). Because TBP2 had only two substituents and TBP3 had less sterically crowded phenyl rings, the protection of the radicals was expected to be limited. Although the experimental conditions were not exactly the same as those in previous reports by keeping the samples under ambient light, the photostability of TBP1, which showed a ca. 50% absorption intensity after 20 min, is likely to be similar to that of TBP2 and TBP3. On the other hand, TBP4 with four bulky mesityl groups showed a much better stability than rubrene with only a slight degradation in ambient conditions. In addition, the spectra of TBP5 exhibited no significant changes of the intensity even after a week. These results indicated that the trialkylsilylacetylene groups have a better protection property than the mesityl groups. Therefore, a highly stable molecule with an OS singlet ground state could be obtained. This improvement enables an easy handling of the solution for fabricating organic electronic devices that require high purity.
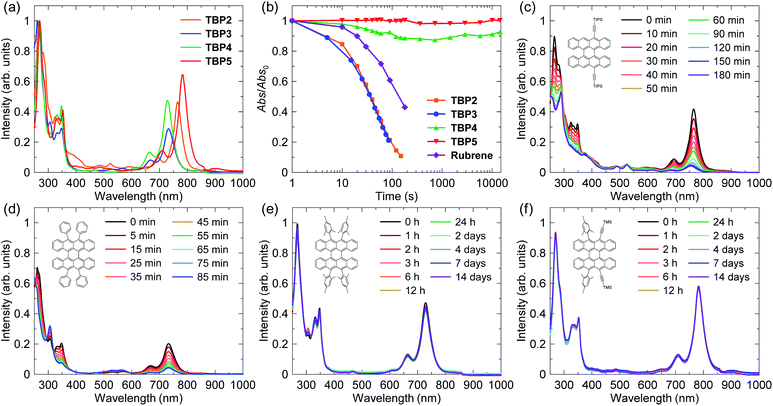 |
| Fig. 2 UV-vis absorption spectra for (a) TBP2–TBP5 in DCM. (b) Absorbance normalized to the initial absorbance for TBP2–TBP5 at their λmax (765, 733, 729, 783 and 527 nm, respectively) and rubrene as a function of time under ambient white light in air saturation. UV-vis absorption spectra as a function of time for (c) TBP2, (d) TBP3, (e) TBP4 and (f) TBP5. | |
The emission spectra of TBP4 and TBP5 are shown in Fig. S6 in the ESI.† The compounds showed NIR emission with emission maxima of 888 nm and 934 nm and a small full width at half maximum (FWHM) of 127 nm (0.19 eV) and 80 nm (0.11 eV) for TBP4 and TBP5, respectively. However, the quantum yields were very low (<0.5%) for both compounds.
The results of the cyclic voltammetry (CV) for TBP4 and TBP5 showed two reversible oxidation and reduction peaks (Fig. S7 and Table S5 in the ESI†). The first oxidation and reduction peaks vs. ferrocene/ferrocenium were at −0.07 V and −1.64 V for TBP4 and 0 V and −1.37 V for TBP5, respectively. The energy gaps of 1.57 eV and 1.37 eV agreed well with optical gaps of these compounds.
Single crystal structure
The single crystals of compounds 5b, TBP4 and TBP5 were obtained by the slow recrystallization from DCM layered with MeOH (Fig. 3, Methods S3, Tables S6 and S7, Fig. S8 and S9 in the ESI†). The crystals of 5b and TBP5 included DCM solvent molecules. Unfortunately, the quality of data for TBP4 were not sufficiently high (R > 20%) because of the very weak reflections. Therefore, the data are provided in the ESI† without the detailed discussions of the bond lengths. However, we were able to confirm the structure and packing motif.
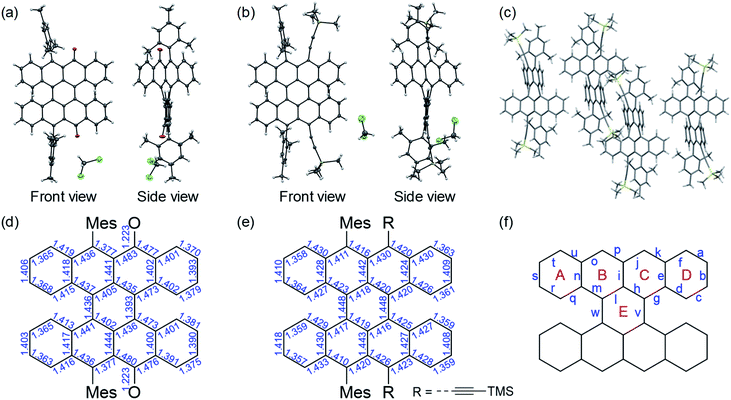 |
| Fig. 3 X-ray crystal structures of (a) 5b and (b) TBP5. (c) Packing structure of TBP5. Bond lengths in the crystals of (d) 5b and (e) TBP5. (f) Positions of bonds and rings. | |
All the compounds showed an intersecting conformation of two tetracene subunits (Fig. S1†), where the central ring E was twisted (Fig. 3f). The bond lengths of compound 5b indicated the contribution of α,β-unsaturated carbonyl structures, where α,β-unsaturated C
C bond (bond p) is short compared with a tetracene quinone derivative,22 as observed in a peri-pentacene derivative that undergoes Michael addition.19 In addition, the slightly increased bond lengths for bonds i, m and o suggested a decrease of the aromaticity in ring B. These features enabled a Michael addition reaction to compound 4. Although the crystal structure of peri-tetracene2 shown in Fig. 1 has been investigated,12b there has been no report for the crystal structure of the TBP derivatives. Therefore, the crystal data of TBP5 would be important to confirm the biradical characters of TBP derivatives. Although the torsion angle for the two tetracene units were relatively large for TBP5 (ca. 40°), the bond lengths for bonds v and w were still short (1.448 Å) and only slightly longer than that of peri-tetracene2 (1.442 Å), which indicated a biradical contribution owing to electronic coupling between two tetracene units. The degree of bond alternation was similar to that of peri-tetracene2. These results strongly support the high biradical character of TBP derivatives. Note that the calculated bond lengths well agreed with the experimental result (Fig. S8†).
The packing structure, which may give some insight to the charge transport property, is shown in Fig. 3c for TBP5. The packing structure of TBP4 is shown in Fig. S9,† where the interactions between TBP cores seemed to be very small. For TBP5, a herringbone packing with a tilt angle of 42° was observed in the horizontal direction. The distance of the CH–π interaction was 2.785 Å, which is comparable to that for pentacene in the peri-direction. Although the intermolecular interactions are limited because of the relatively large substitution groups, the results proved that efficient charge transport in TBP5 is possible.
VT NMR and ESR
The 1H NMR spectra of TBP4 in DCM-d2 showed broad peaks at room temperature (Fig. 4a), which is often observed for OS singlet biradicals because triplet biradicals are thermally accessible. Therefore, the peaks became sharper by decreasing the temperature and were assigned to the structure of TBP4 with the help of 2D-COSY NMR spectroscopy. The broad peaks at room temperature were found to be from H3 (7.08 ppm, double doublet at 193 K) and H1 (7.26 ppm, doublet at 193 K). This result agrees well with the relatively high spin density distribution at these positions calculated by density functional theory (DFT) at the UB3LYP/6-311G(d,p) level. The singlet biradical feature was further characterized by an ESR measurement in the solid state, which gave a typical ESR signal with a g-value of 2.003 for TBP4 (Fig. S10 in the ESI†). On the other hand, TBP5 showed a weaker ESR signal and negligible broadening of the NMR peaks at room temperature, which indicated a larger singlet–triplet energy gap (ΔES–T) (Fig. 4b). The theoretical calculations showed a smaller ΔES–T, calculated by geometry optimization for the singlet and triplet states, for TBP5 than TBP4, which was not consistent with the NMR results. However, the time-dependent (TD)-DFT calculations showed a larger vertical excitation energy of TBP5 than TBP4 for the triplet in the singlet ground state geometry, suggesting that the different activation energies for the triplet caused the different degrees of broadening. Because some recent papers have reported a high biradical character with a large ΔES–T,23,24 this should be investigated in more detail in future works.
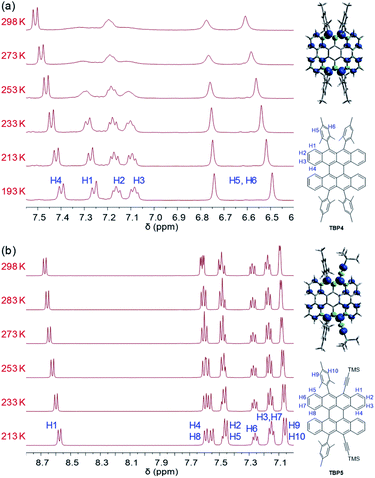 |
| Fig. 4 VT 1H-NMR spectra of (a) TBP4 and (b) TBP5 in DCM-d2 in the aromatic region. | |
Charge transport properties
The OFET devices using TBP5 were investigated because of its higher stability and better interactions. The devices with bottom-gate/top-contact (BG/TC) configurations were fabricated for the drop-coated films from an o-dichlorobenzene (ODCB) solution on a cross-linked poly-4-vinylphenol (PVP) dielectric layer (see Methods S4 and Fig. S11 in the ESI†). Some recent reports used several types of singlet biradical compounds for the active layer of OFETs, which revealed their bipolar transport owing to small energy gaps.24–26 The TBP-based devices also demonstrated a capability of bipolar transport for peri-acene derivatives. Fig. 5 shows the transfer and output characteristics with hole and electron mobilities of 1.6 × 10−3 and 6.1 × 10−5 cm2 V−1 s−1, respectively. The devices obtained from the different drop-casting conditions, which have different degree of crystalline domains, showed a higher hole mobility of 1.2 × 10−2 cm2 V−1 s−1, although the electron transport was not clear because of a largely unbalanced hole and electron currents (Fig. S12 in the ESI†). The crystallinity of the thin-films was evaluated by X-ray diffraction (XRD), polarized optical microscopy (POM) and atomic force microscopy (AFM) (see Fig. S13 and 14 in the ESI†). The out-of-plane XRD showed a small peak at 2θ = 9.28° (d = 9.5 Å). This was shorter than the molecular length (ca. 11 Å for the tetracene length and 19 Å in the substitution direction). Therefore, the molecules may have had declined orientations on the substrate. The microscopy images also confirmed polycrystalline domains in the channel.
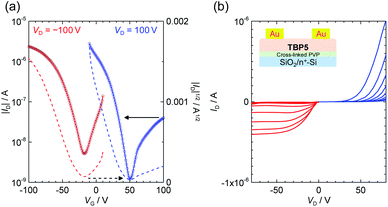 |
| Fig. 5 OFET devices of TBP5. (a) Transfer and (b) output characteristics of a BG/TC device with a drop-casted film from 0.3 wt% ODCB solution. | |
Overall, peri-acene derivatives show a high potential for use as organic semiconducting materials.
Conclusions
In this work, we have developed a new synthesis route for TBP derivatives of a peri-tetracene analogue, which is much simpler and shorter than the previously reported methods. The newly developed TBP derivatives with four substituents showed a higher stability than rubrene in solution under ambient conditions despite a higher singlet biradical character. Thus, the simple protection approach is found to be an effective method to improve the stability of peri-acenes. The crystal structures of TBP derivatives were obtained for the first time and clearly revealed a large biradical contribution in the TBP structure. The charge transport properties of the TBP derivative were also investigated for the first time and the results clearly demonstrated a bipolar nature for the peri-tetracenes.
Conflicts of interest
There are no conflicts to declare.
Acknowledgements
We thank Ms K. Kusuhara and Ms N. Nakamura for the materials characterization, Mr T. Matsumoto for the measurements of X-ray single crystal analysis. This work was financially supported by JST ERATO Grant Number JPMJER1305, JSPS KAKENHI Grant Number 19H02790, and the JSPS Core-to-Core Program.
Notes and references
-
(a) Q. Ye and C. Chi, Chem. Mater., 2014, 26, 4046–4056 CrossRef CAS;
(b) Z. Sun, Q. Ye, C. Chi and J. Wu, Chem. Soc. Rev., 2012, 41, 7857–7889 RSC.
-
(a) W. Warta and N. Karl, Phys. Rev. B: Condens. Matter Mater. Phys., 1985, 32, 1172–1182 CrossRef CAS;
(b) J. E. Anthony, Chem. Rev., 2006, 106, 5028–5048 CrossRef CAS;
(c) C. Wang, H. Dong, W. Hu, Y. Liu and D. Zhu, Chem. Rev., 2012, 112, 2208–2267 CrossRef CAS.
-
(a) M. B. Smith and J. Michl, Chem. Rev., 2010, 110, 6891–6936 CrossRef CAS;
(b) D. N. Congreve, J. Lee, N. J. Thompson, E. Hontz, S. R. Yost, P. D. Reusswig, M. E. Bahlke, S. Reineke, T. Van Voorhis and M. A. Baldo, Science, 2013, 340, 334–337 CrossRef CAS;
(c) E. G. Fuemmeler, S. N. Sanders, A. B. Pun, E. Kumarasamy, T. Zeng, K. Miyata, M. L. Steigerwald, X.-Y. Zhu, M. Y. Sfeir, L. M. Campos and N. Ananth, ACS Cent. Sci., 2016, 2, 316–324 CrossRef CAS;
(d) R. G. Kepler, J. C. Caris, P. Avakian and E. Abramson, Phys. Rev. Lett., 1963, 10, 400–402 CrossRef CAS.
-
(a) A. N. Aleshin, J. Y. Lee, S. W. Chu, J. S. Kim and Y. W. Park, Appl. Phys. Lett., 2004, 84, 5383–5385 CrossRef CAS;
(b) T. W. Kelley, D. V. Muyres, P. F. Baude, T. P. Smith and T. D. Jones, Mater. Res. Soc. Symp. Proc., 2003, 771, 169–179 CrossRef CAS;
(c) K. Ito, T. Suzuki, Y. Sakamoto, D. Kubota, Y. Inoue, F. Sato and S. Tokito, Angew. Chem., Int. Ed., 2003, 42, 1159–1162 CrossRef CAS;
(d) A. Afzali, C. D. Dimitrakopoulos and T. L. Breen, J. Am. Chem. Soc., 2002, 124, 8812–8813 CrossRef CAS.
-
(a) D. Y. Kondakov, T. D. Pawlik, T. K. Hatwar and J. P. Spindler, J. Appl. Phys., 2009, 106, 124510 CrossRef;
(b) B. H. Wallikewitz, D. Kabra, S. Gélinas and R. H. Friend, Phys. Rev. B: Condens. Matter Mater. Phys., 2010, 85, 045209 CrossRef;
(c) R. Ieuji, K. Goushi and C. Adachi, Nat. Commun., 2019, 10, 5283 CrossRef;
(d) R. Nagata, H. Nakanotani, W. J. Potscavage Jr and C. Adachi, Adv. Mater., 2018, 30, 1801484 CrossRef.
-
(a) M. M. Payne, S. R. Parkin and J. E. Anthony, J. Am. Chem. Soc., 2005, 127, 8028–8029 CrossRef CAS;
(b) R. Mondal, R. M. Adhikari, B. K. Shah and D. C. Neckers, Org. Lett., 2007, 9, 2505–2508 CrossRef CAS;
(c) M. Watanabe, Y. J. Chang, S.-W. Liu, T.-H. Chao, K. Goto, M. M. Islam, C.-H. Yuan, Y.-T. Tao, T. Shinmyozu and T. J. Chow, Nat. Chem., 2012, 4, 574–578 CrossRef CAS;
(d) D. Chun, Y. Cheng and F. Wudl, Angew. Chem., Int. Ed., 2008, 47, 8380–8385 CrossRef CAS;
(e) H. Qu and C. Chi, Org. Lett., 2010, 12, 3360–3363 CAS;
(f) B. Purushothaman, S. R. Parkin and J. E. Anthony, Org. Lett., 2010, 12, 2060–2063 CAS.
-
(a) L. A. Stevens, K. P. Goetz, A. Fonari, Y. Shu, R. M. Williamson, J.-L. Brédas, V. Coropceanu, O. D. Jurchescu and G. E. Collis, Chem. Mater., 2015, 27, 112–118 CAS;
(b) L. Zhang, A. Fonari, Y. Liu, A. L. Hoyt, H. Lee, D. Granger, S. Parkin, T. P. Russell, J. E. Anthony, J.-L. Brédas, V. Coropceanu and A. L. Briseno, J. Am. Chem. Soc., 2014, 136, 9248–9251 CrossRef CAS;
(c) K. Sbargoud, M. Mamada, T. Jousselin-Oba, Y. Takeda, S. Tokito, A. Yassar, J. Marrot and M. Frigoli, Chem.–Eur. J., 2017, 23, 5076–5080 CrossRef CAS;
(d) C. Reus, M. P. Lechner, M. Schulze, D. Lungerich, C. Diner, M. Gruber, J. M. Stryker, F. Hampel, N. Jux and R. R. Tykwinski, Chem.–Eur. J., 2016, 22, 9097–9101 CrossRef CAS;
(e) Z. Wang, R. Li, Y. Chen, Y. Tan, Z. Tu, X. Gao, H. Dong, Y. Yi, Y. Zhang, W. Hu, K. Müllen and L. Chen, J. Mater. Chem. C, 2017, 5, 1308–1312 RSC;
(f) X. Liu, M. Chen, C. Xiao, N. Xue and L. Zhang, Org. Lett., 2018, 20, 4512–4515 CrossRef CAS;
(g) T. Dumslaff, B. Yang, A. Maghsoumi, G. Velpula, K. S. Mali, C. Castiglioni, S. D. Feyter, M. Tommasini, A. Narita, X. Feng and K. Müllen, J. Am. Chem. Soc., 2016, 138, 4726–4729 CrossRef CAS;
(h) Q. Wang, T. Y. Gopalakrishna, H. Phan, T. S. Herng, S. Dong, J. Ding and C. Chi, Angew. Chem., Int. Ed., 2017, 56, 11415–11419 CrossRef CAS;
(i) Q. Chen, D. Wang, M. Baumgarten, D. Schollmeyer, K. Müllen and A. Narita, Chem.–Asian J., 2019, 14, 1703–1707 CrossRef CAS;
(j) X. Xu, K. Müllen and A. Narita, Bull. Chem. Soc. Jpn., 2020, 93, 490–506 CrossRef CAS.
-
(a) R. Scholl and J. Mansfeld, Ber. Dtsch. Chem. Ges., 1910, 43, 1734–1746 CrossRef CAS;
(b) R. Scholl, C. Seer and R. Weitzenböck, Ber. Dtsch. Chem. Ges., 1910, 43, 2202–2209 CrossRef CAS.
-
(a) E. Clar, Chem. Ber., 1948, 81, 52–63 CrossRef CAS;
(b) E. Clar, Chem. Ber., 1949, 82, 46–60 CrossRef CAS;
(c) E. H. Fort, P. M. Donovan and L. T. Scott, J. Am. Chem. Soc., 2009, 131, 16006–16007 CrossRef CAS;
(d) H. Hayashi, N. Aratani and H. Yamada, Chem.–Eur. J., 2017, 12, 7000–7008 CrossRef.
-
(a) A. Konishi, Y. Hirao, M. Nakano, A. Shimizu, E. Botek, B. Champagne, D. Shiomi, K. Sato, T. Takui, K. Matsumoto, H. Kurata and T. Kubo, J. Am. Chem. Soc., 2010, 132, 11021–11023 CrossRef CAS;
(b) A. Konishi, Y. Hirao, K. Matsumoto, H. Kurata, R. Kishi, Y. Shigeta, M. Nakano, K. Tokunaga, K. Kamada and T. Kubo, J. Am. Chem. Soc., 2013, 135, 1430–1437 CrossRef CAS.
- J. Liu, P. Ravat, M. Wagner, M. Baumgarten, X. Feng and K. Müllen, Angew. Chem., Int. Ed., 2015, 54, 12442–12446 CrossRef CAS.
-
(a) M. R. Ajayakumar, Y. Fu, J. Ma, F. Hennersdorf, H. Komber, J. J. Weigand, A. Alfonsov, A. A. Popov, R. Berger, J. Liu, K. Müllen and X. Feng, J. Am. Chem. Soc., 2018, 140, 6240–6244 CrossRef CAS;
(b) Y. Ni, T. Y. Gopalakrishna, H. Phan, T. S. Herng, S. Wu, Y. Han, J. Ding and J. Wu, Angew. Chem., Int. Ed., 2018, 57, 9697–9701 CrossRef CAS.
-
(a) S. Mishra, T. G. Lohr, C. A. Pignedoli, J. Liu, R. Berger, J. I. Urgel, K. Müllen, X. Feng, P. Ruffieux and R. Fasel, ACS Nano, 2018, 12, 11917–11927 CrossRef CAS;
(b) M. R. Ajayakumar, Y. Fu, F. Liu, H. Komber, V. Tkachova, C. Xu, S. Zhou, A. A. Popov, J. Liu and X. Feng, Chem.–Eur. J., 2020, 26, 7497–7503 CrossRef CAS;
(c) X. Liu, M. Chen, C. Xiao, N. Xue and L. Zhang, Org. Lett., 2018, 20, 4512–4515 CrossRef CAS.
-
(a) C. Rogers, C. Chen, Z. Pedramrazi, A. A. Omrani, H.-Z. Tsai, H. S. Jung, S. Lin, M. F. Crommie and F. R. Fischer, Angew. Chem., Int. Ed., 2015, 54, 15143–15146 CrossRef CAS;
(b) F. Plasser, H. Pašalić, M. H. Gerzabek, F. Libisch, R. Reiter, J. Burgdörfer, T. Müller, R. Shepard and H. Lischka, Angew. Chem., Int. Ed., 2013, 52, 2581–2584 CrossRef CAS;
(c) L. Zöphel, R. Berger, P. Gao, V. Enkelmann, M. Baumgarten, M. Wagner and K. Müllen, Chem.–Eur. J., 2013, 19, 17821–17826 CrossRef.
-
(a) J. G. Laquindanum, H. E. Katz and A. J. Lovinger, J. Am. Chem. Soc., 1998, 120, 664–672 CrossRef CAS;
(b) Q. Miao, X. Chi, S. Xiao, R. Zeis, M. Lefenfeld, T. Siegrist, M. L. Steigerwald and C. Nuckolls, J. Am. Chem. Soc., 2006, 128(4), 1340–1345 CrossRef CAS;
(c) P. Natarajan and M. Schmittel, J. Org. Chem., 2013, 78, 10383–10394 CrossRef CAS;
(d) C. F. H. Allen and A. Bell, J. Am. Chem. Soc., 1942, 64, 1253–1260 CrossRef CAS;
(e) K. Tanaka, N. Aratani, D. Kuzuhara, S. Sakamoto, T. Okujima, N. Ono, H. Uno and H. Yamada, RSC Adv., 2013, 3, 15310–15315 RSC;
(f) Z. Sun, Q. Ye, C. Chi and J. Wu, Chem. Soc. Rev., 2012, 41, 7857–7889 RSC.
-
(a) S. Toyota, R. Miyaji, Y. Yamamoto, M. Inoue, K. Wakamatsu and T. Iwanaga, Eur. J. Org. Chem., 2015, 7648–7651 CrossRef CAS;
(b) I. Agranat and Y. Tapuhi, J. Org. Chem., 1979, 44, 1941–1948 CrossRef CAS.
-
(a)
The Aromatic Sextet, ed. E. Clar, John Wiley & Sons, London, 1972, pp. 81–82 Search PubMed;
(b)
E. Clar, Polycyclic Hydrocarbons, I, Academic Press, New York, 1964, p. 46 Search PubMed.
-
(a) J. E. Anthony, D. L. Eaton and S. R. Parkin, Org. Lett., 2002, 4, 15–18 CrossRef CAS;
(b) M. L. Tang, A. D. Reichardt, T. Siegrist, S. C. B. Mannsfeld and Z. Bao, Chem. Mater., 2008, 20, 4669–4676 CrossRef CAS.
- X. Zhang, J. Li, H. Qu, C. Chi and J. Wu, Org. Lett., 2010, 12, 3946–3949 CrossRef CAS.
-
(a) K. Yamaguchi, Chem. Phys. Lett., 1975, 33, 330–335 CrossRef CAS;
(b) K. Yamaguchi, T. Kawakami, Y. Takano, Y. Kitagawa, Y. Yamashita and H. Fujita, Int. J. Quantum Chem., 2002, 90, 370–385 CrossRef CAS.
- M. Mamada, H. Katagiri, T. Sakanoue and S. Tokito, Cryst. Growth Des., 2015, 15, 442–448 CrossRef CAS.
- T. Wombacher, S. Foro and J. J. Schneider, Eur. J. Org. Chem., 2016, 569–578 CrossRef CAS.
-
(a) J. J. Dressler, M. Teraoka, G. L. Espejo, R. Kishi, S. Takamuku, C. J. Gómez-García, L. N. Zakharov, M. Nakano, J. Casado and M. M. Haley, Nat. Chem., 2018, 10, 1134–1140 CrossRef CAS;
(b) Y.-C. Hsieh, C.-F. Wu, Y.-T. Chen, C.-T. Fang, C.-S. Wang, C.-H. Li, L.-Y. Chen, M.-J. Cheng, C.-C. Chueh, P.-T. Chou and Y.-T. Wu, J. Am. Chem. Soc., 2018, 140, 14357–14366 CrossRef CAS.
- T. Jousselin-Oba, M. Mamada, A. Okazawa, J. Marrot, T. Ishida, C. Adachi, A. Yassar and M. Frigoli, Chem. Sci., 2020 10.1039/D0SC04583G.
-
(a) G. E. Rudebusch, J. L. Zafra, K. Jorner, K. Fukuda, J. L. Marshall, I. Arrechea-Marcos, G. L. Espejo, R. Ponce Ortiz, C. J. Gómez-García, L. N. Zakharov, M. Nakano, H. Ottosson, J. Casado and M. M. Haley, Nat. Chem., 2016, 8, 753–759 CrossRef CAS;
(b) Y.-C. Hsieh, C.-F. Wu, Y.-T. Chen, C.-T. Fang, C.-S. Wang, C.-H. Li, L.-Y. Chen, M.-J. Cheng, C.-C. Chueh, P.-T. Chou and Y.-T. Wu, J. Am. Chem. Soc., 2018, 140, 14357–14366 CrossRef CAS;
(c) A. M. Zeidell, L. Jennings, C. K. Frederickson, Q. Ai, J. J. Dressler, L. N. Zakharov, C. Risko, M. M. Haley and O. D. Jurchescu, Chem. Mater., 2019, 31, 6962–6970 CrossRef CAS;
(d) T. Jousselin-Oba, M. Mamada, J. Marrot, A. Maignan, C. Adachi, A. Yassar and M. Frigoli, J. Am. Chem. Soc., 2019, 141(23), 9373–9381 CrossRef.
- J. G. Champlain, Appl. Phys. Lett., 2011, 99, 123502 CrossRef.
Footnote |
† Electronic supplementary information (ESI) available: Synthesis, characterization, computational, photophysical, electrochemical and thin-film transistor data. CCDC 2025243 and 2025244. For ESI and crystallographic data in CIF or other electronic format see DOI: 10.1039/d0sc04699j |
|
This journal is © The Royal Society of Chemistry 2021 |