DOI:
10.1039/C5RA08009F
(Paper)
RSC Adv., 2015,
5, 49759-49764
Highly sensitive SERS sensor for mercury ions based on the catalytic reaction of mercury ion decorated Ag nanoparticles†
Received
1st May 2015
, Accepted 26th May 2015
First published on 26th May 2015
Abstract
A surface-enhanced Raman scattering (SERS) sensor of mercury ions based on the coordinated catalytic reaction of Hg2+–Ag nanoparticles (NPs) has been designed and constructed for water quality monitoring. We combined Ag NPs (average particle size is 49 nm) with mercury ions to form Hg2+–Ag NPs in aqueous phase by electrostatic interactions. The formed Hg2+–Ag particles can catalyze a redox reaction between o-phenylendiamine (OPD) and dissolved oxygen to form 2,3-diaminephenazine (DAP), which is Raman-active and possesses a strong SERS signal due to the adherence to Ag NPs. Therefore we can trace the SERS intensity of DAP to determine mercury ions and the lowest detectable concentration of mercury is 1.0 nM using this method. This sensor displays higher sensitivity and selectivity and it possesses a certain value in terms of water quality detection.
1 Introduction
Mercury ions are toxic heavy metal ions. They can easily combine with negatively charged groups (e.g. sulfydryl of enzymes and proteins in the body),1–6 and alter or destroy physiological activities by changing their chemical structures. For living cells, mercury ions can cause metabolic disorder.7–9 Mercury ion poisoning is an accumulation process of mercury ions in the body through the food chain and drinking water.10,11 The United States of America Environmental Protection Bureau (EPA) standard code states that inorganic mercury can't exceed 2.0 nM in drinking water.10–12 Therefore, to develop a simple and selective quantitative determination approach of mercury in water is of significance. There are many methods to detect mercury ions, such as dithizone colorimetric method,13,14 atomic emission spectrometry,15,16 absorption spectrometry,17,18 stripping voltammetry,19,20 and so on. These methods have disadvantages on that they require long cycle and the complex pre-treatment of samples. The lowest detectable concentration is 2.0 nM for dithizone colorimetric method21 and is 11 nM for stripping voltammetry method.22 So a huge challenge is put forward to develop a fast respond, high selectivity and sensitivity method for the trace detection of mercury ions.
Surface-enhanced Raman spectroscopy (SERS) that employs molecules adsorbed on the surface of noble metal nanoparticle (NP) to enhance their Raman signals has extensive applications in various fields such as drug identification, catalysis monitoring the reaction process, the treatment of disease and so on.23 SERS not only can realize qualitative research, but also can be used for quantitative study in the field of analytical chemistry.24,25 It is quite applicable in aqueous phase and easily identifiable due to the fingerprint characters of probes.
In this work, we propose an indirect SERS sensing method for mercury ions by a catalytic oxidation reaction. Hg2+–Ag NPs composed of Ag NPs and mercury ions were used as catalysts and prepared in water through the electrostatic assembly (A in Scheme 1), which can catalyze a redox reaction of o-phenylendiamine (OPD) and dissolved oxygen to produce 2,3-diaminophenazineand (DAP). The SERS sensing of the growing DAP can indirectly response for the amount of mercury ions (B in Scheme 1). The results show that the lowest detectable concentration of mercury ion is as low as 1.0 nM and this sensor displays a good selectivity for mercury ions.
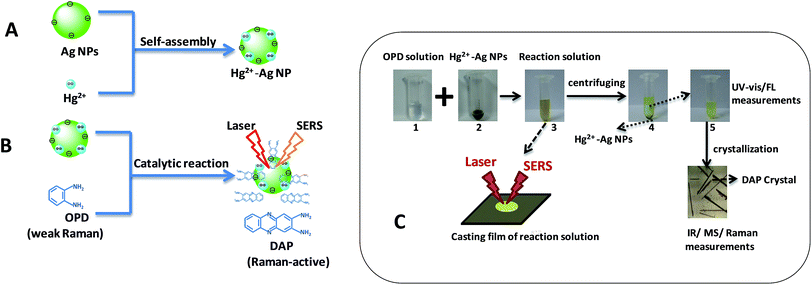 |
| Scheme 1 Schematic diagram of the formation of Hg2+–Ag NPs (A) and the SERS sensing for mercury ions by a catalytic oxidation reaction (B). (C) shows the experimental procedures. | |
2 Experimental section
2.1 Material
OPD (reagent grade) was obtained from Tianjin Guang Fu Fine Chemical Research Institute. Mercury nitrate (reagent grade) was purchased from Taixing Chemical Reagent Factory. Sodium citrate (C6H5Na3O7·2H2O, reagent grade) and citric acid (C6H8O7·H2O, reagent grade) were purchased from Beijing Chemical Corp. Silver nitrate (reagent grade) was purchased from Shanghai Chemical company. Calcium acetate, barium acetate, lead acetate, nickelous acetate, tin acetate, cupric acetate, cobalt acetate, zinc acetate, manganese acetate and cadmium acetate were obtained from Beijing Chemical Corp. Deionized water used throughout the experiments was purified by a Millipore system.
2.2 Synthesis of Hg2+–Ag NPs and their catalytic reaction
First, Ag NPs were synthesized using Lee's method as described previously.26 From transmission electron microscopic (TEM) image and size distribution (Fig. 1(a)), we can find the Ag NPs synthesized is basically spherical and the average particles size is 49 nm (Fig. S1b in ESI†). The maximum of the absorbance wavelength of the Ag NPs is 418 nm (Fig. S1a†). Second, we mixed 100 μL of Ag NPs and 100 μL of mercury ions solution for 10 min incubated time at room temperature to prepare the Hg2+–Ag NPs. The Hg2+–Ag NPs were formed via the electrostatic assembly of mercury ions on the surface of Ag NPs (Scheme 1A) and their TEM images are shown in Fig. 1(b). It can be found that the shape and size of the Hg2+–Ag NP has no obvious difference to the Ag NPs.
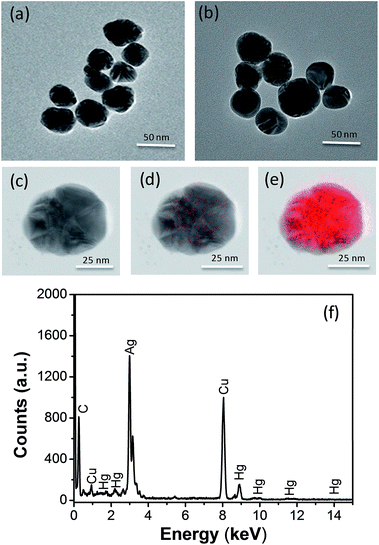 |
| Fig. 1 (a) and (b) TEM images of the Ag NPs and Hg2+–Ag NP. (c)–(e) TEM mappings of Hg2+–Ag NP prepared by adding 100 μL of Ag NPs and 100 μL of Hg2+ ion solution (1.0 × 10−6 M). (d) and (e) show Hg and Ag element distribution in this individual Hg2+–Ag nanoparticle (c), respectively. (f) The elements analysis of an Hg2+–Ag NPs above a copper grid under TEM. | |
To carry out the catalytic reaction, the synthesized Hg2+–Ag NPs (200 μL with the particle concentration is 3.0 nM) were added to 100 μL of the freshly prepared 0.02 M OPD in citrate buffer and then 700 μL of citrate buffer solution (0.1 M) were added above solution. The mixed solution was heated to 50 °C in water bath for 35 min to complete the transformation of target OPD.
In order to identify the product of reaction between the OPD and dissolved oxygen, we centrifuged the reaction solution at a speed of 10
000 rpm. The precipitates were Hg2+–Ag NPs and the suspension solution obtained through cooling crystallization, centrifugation and desiccation (Scheme 1C). There are many needle-like crystals formed in the suspension solution and they were identified by Fourier transform infrared (FT-IR), mass (MS) and SERS spectroscopies.
2.3 Characterization
A Shimadzu UV-3600 spectrophotometer was used to record ultraviolet-visible (UV-vis) spectra of the produced DAP solutions in a 1.0 cm light path quartz cuvette. The fluorescence emission spectrum of the DAP solutions was obtained on a Shimadzu RF-5301PC spectrophotometer. To avoid the interference from the Hg2+–Ag NPs, we centrifuged the reaction solution at a speed of 10
000 rpm. The precipitates of Hg2+–Ag NPs were removed before we carried out UV-vis and fluorescence measurements (the results are shown in Fig. 2).
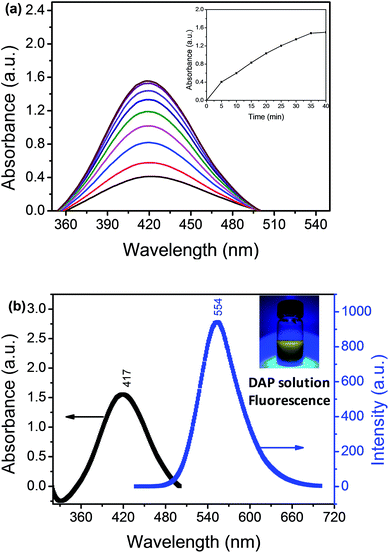 |
| Fig. 2 (a) Time-resolved extinction spectra of DAP solution without the Hg2+–Ag NPs while 1.0 × 10−5 M of Hg2+ was used. Inset in (a) shows a plot of UV-vis absorption intensity with the reaction time. (b) UV-vis and fluorescent emission spectra of the produced DAP solution without the Hg2+–Ag NPs while 1.0 × 10−5 M of Hg2+ was used. Inset (b) is a photograph of DAP solution under UV light (365 nm). | |
FT-IR spectrum was measured with a Bruker Vertex 80v spectrometer. TEM images were obtained by a JEM-2100 F field-emission transmission electron microscope (JEOL, Tokyo, Japan). The sample was loaded above a polymer film modified copper grid for TEM measurement. Mass spectrum (MS) was recorded on a Q-Trap MS (Applied Biosystems/MDS Sciex, Concord, ON, Canada) which equipped with an electrospray ionization source. The product was dissolved by methanol and introduced into the MS detector at a flow rate of 0.01 mL min−1. The analyte was monitored in positive mode, and the detection parameters were as follows: collision gas; N2 (medium), curtain gas; N2 (30 psi), gas 1; N2 (55 psi), gas; N2 (50 psi), ion spray voltage; 5000 V, and temperature; 400 °C. The data collection was carried out in enhanced MS (EMS) mode. For the product, the protonated molecular ion, [M + H]+, and two confirming ions were obtained.
2.4 SERS measurements
The reaction solutions were dropped on the 1 cm × 1 cm silicon pellet to form a spot with a diameter of 1.5 μm (Scheme 1C and Fig. S4†).
Then, SERS spectra of the dried solutions were measured by a confocal Raman system (LabRAM ARAMIS, HORIBA Jobin Yvon, USA) with a 5 mW/785 nm laser as an excitation source and actualized by Synapse Thermoelectric cooled charge-coupled device (CCD) camera (HORIBA Jobin Yvon, USA). Since the produced DAP has fluorescence in the range of 450–700 nm,27 we chose 785 nm wavelength as the excitation laser to avoid the fluorescence interference (see a comparison of SERS spectra of DAP under different excitation wavelength, Fig. S3 in ESI†). Laser excitation and Raman scattering light collection were through a ×50 microscope objective lens (numerical aperture = 0.5, LMLFLN, Olympus, Japan). The outstretched scan spectra with a spectral range from 550 to 1600 cm−1 were obtained with an integration time of 20 s and two accumulations. The 520.7 cm−1 vibrational band of silicon wafer was used as a reference for the wave number calibration. The SERS spectra of the DAP produced from the redox reaction between OPD and dissolved oxygen were detected. The signal intensities of DAP at 997 cm−1 were recorded for different concentrations of mercury ions to make a working curve.
2.5 Selectivity
We selected other metal ions, such as, Ca2+, Ba2+, Pb2+, Ni2+, Sn2+, Cu2+, Ag+, Co2+, Zn2+, Mn2+ and Cd2+ to investigate the selectivity of Ag NPs to Hg2+ (Fig. S2†). Under the optimal conditions that 6.0 of pH, 50 °C of temperature, 100 μL of Ag NP volume and 35 min of reaction time, the concentrations of the interference ions including Ca2+, Ba2+, Pb2+, Ni2+, Sn2+, Co2+, Zn2+, Mn2+, Cd2+ were all 1.0 × 10−3 M except the concentrations of Cu2+ and Ag+ were 1.0 × 10−4 M and concentration of the target (Hg2+ ions) was 1.0 × 10−6 M. The intensities of the peak at 997 cm−1 were obtained from the SERS spectra of all the metal ions and they were plotted for comparison.
3 Result and discussion
3.1 Characterization of Hg2+–Ag NPs
As we known, Ag NPs synthesized by the citrate reduction are coated with a layer of citrate ions, displaying negative charges. Moreover, mercury ions own two positive charges, which can attach to Ag NP surface due to the electrostatic force. As shown in the Fig. 1(a) and (b), the TEM images of Ag NPs and Hg2+–Ag which show the shape and size of the Hg2+–Ag NPs has no obvious difference to the Ag NP. Fig. 1(c)–(e) shows the TEM mapping of Hg2+–Ag NP. From Fig. 1(c)–(e), we can find that mercury ions have been assembled on the surface of Ag NP to form a Hg2+–Ag NP. At the same time, we can find mercury ions only occupy a very limited surface area of an Ag NP, which can leave enough large surface area for DAP adsorption. Fig. 1(f) shows the elemental analysis, in which we also can identify a certain amount of mercury in the combined NPs.
3.2 The OPD-oxidized reaction under the catalysis of Hg2+–Ag NPs
We used the Hg2+–Ag NPs to catalyze the reaction between the OPD solution and dissolved oxygen. The OPD solution was colourless before the Hg2+–Ag NPs added (optical photograph 1 in Scheme 1C). When the Hg2+–Ag NPs were added into the OPD solution, the solution colour became yellow at 35 min (optical photograph 3 in Scheme 1C) and the UV-vis maximum absorption intensity of the product after the Hg2+–Ag NPs were separated from solution gradually increased with time from bottom to top as shown in Fig. 2(a). There is a strong absorption peak at 417 nm, which is in accordance with previously reported that this band belongs to DAP.28 The inserted spectrum of Fig. 2(a) shows the relationship of UV-vis maximum absorption intensity with reaction time, indicating the growth of the coloured product. Fig. 2(b) records the UV-vis and fluorescent emission spectra of the produced solution after removing the Hg2+–Ag NPs (optical photograph 5 in Scheme 1C). At the same time, we also find a strong fluorescent emission band is located at 554 nm when the production solution is excited at 440 nm, also proving that the product of reaction is DAP.2 The product solution under UV light (365 nm) shows an obvious yellow colour (inset in Fig. 2(b), revealing that the DAP exhibits yellow fluorescence. This OPD-oxidized reaction can be expressed as follows:
3.3 Identification of the OPD-oxidized product
The oxidized product of OPD is still amphibolous in the reported literatures. For example, C. Hempen's and many reports27–31 referred that the product was DAP. However, in W. Z. Sheng's paper,32 they stated that the main product was azoaniline. To confirm our product, we characterized it by IR, MS, and Raman spectroscopies. Before characterizations, the final product that was separated from reaction solution by centrifugation was carried out through cooling crystallization, centrifugation and desiccation to form a needle-like crystal (Scheme 1C). Fig. 3(a) shows the IR spectrum of the crystal. The characteristic absorption band at 3434, 3414, 3180 and 2996 cm−1 are assigned to the N–H stretching vibration of the adjacent two amino group on phenazine. The band of C
N stretching vibration and primary amine deformation vibration are at 1643 and 1562 cm−1. The band at the 1498 cm−1 is aromatic ring. Four adjacent hydrogens of ring deformation vibration are at 763 cm−1 and the peak at 586 cm−1 attributes to aromatic plane and out-of-plane bending vibration. From Fig. 3(a), it proves that the product of the OPD-oxidized reaction is DAP, which is similar to the previously reported DAP molecules.33,34 Fig. 3(b) displays the MS of the product. A strong ion peak at 211.3 shows that the DAP has formed an OPD dimer (the mass of DAP plus a H+) in this method.2 To further confirm the structure of the oligomer, the Raman spectrum of the needle-like crystal was recorded. Fig. 3(c) shows the Raman spectrum of the product and the band assignments are listed in Table 1.34 Based on above characterizations, we can identify that the obtained product is DAP.
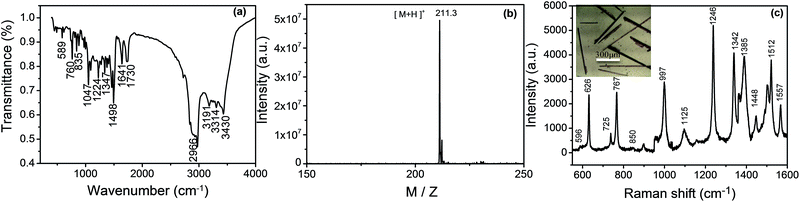 |
| Fig. 3 The IR (a), MS (b) and Raman spectra (c) of the product of needle-like DAP. Inset in (c); a photograph of needle-like DAP. | |
Table 1 The assignments of Raman vibration modes of the producta
Wave number (cm−1) |
Vibrational assignments |
β, in plane bending; γ, out plane bending; ω, wagging; ν, stretching vibration; δ, scissoring. |
596 |
βC–N–C + βC–C–C |
626 |
βC–C–C |
725 |
γC–C–C + γC–N–C |
767 |
ωNH2 + γC–C–C |
850 |
γC–H |
997 |
βC–C–C + νC–C |
1125 |
βC–H + νC–N + νC–C |
1246 |
βC–H + νC–N |
1342 |
νC–N + βC–H + νC–C |
1385 |
νC–N + νC–C |
1448 |
νC–C + νC–NH2 |
1512 |
νC–N + βC–H + νC–C |
1557 |
νC–C + δNH2 |
3.4 Detection of mercury ions
We employed this Hg2+–Ag NPs catalyzed redox reaction between OPD and dissolved oxygen to trace the concentration of mercury ions (B in Scheme 1). Since Hg2+–Ag NPs play a role of a catalyst, more Hg2+–Ag NPs will produce more DAP in the same reaction time. OPD has a weak Raman behaviour, however, the produce DAP is Raman-active. In addition, the Raman signal of the produced DAP can be greatly enhanced by the contained Ag NPs (Fig. S6†). Therefore we can trace the SERS intensity of the produced DAP to determine mercury ions and the detection sensitivity in this “turn on” sensing mechanism can also be improved by the enhancement activity of Ag NPs. The different concentrations of mercury ions were measured from 10 μM to 1 nM and the SERS spectra of produced DAP in different concentrations of mercury ions in casting films over a silicon slide are shown in Fig. 4(a). Fig. 4(b) shows the linear relationship between the concentration of mercury ions and the intensities of the peak at 997 cm−1 after subtracting the blank. The linear relationship is y = 7.4 × 108x + 673.7 with a correlation coefficient of 0.988. The minimum detection concentration is 1.0 nM with the noise to signal ratio is 4
:
1. We also have researched the reproducibility of SERS detection (Fig. S5†) and the relative standard deviations (RSDs) of SERS intensities are less than 10%, which can meet the standard of a high-performance SERS substrate (RSDs < 20%).35,36
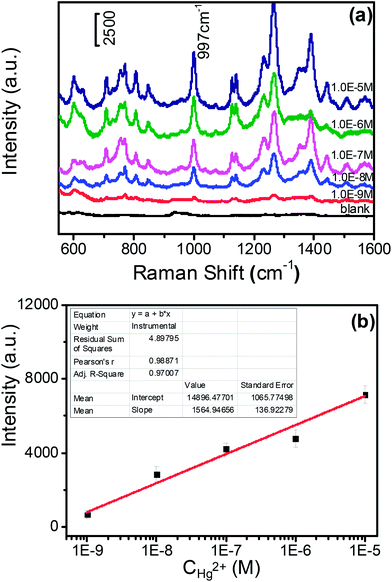 |
| Fig. 4 (a) SERS spectra for sensing different concentrations of Hg2+ (from top to bottom 1.0 × 10−5, 1.0 × 10−6, 1.0 × 10−7, 1.0 × 10−8 and 1.0 × 10−9 M, respectively). (b) The dependence of SERS intensity at 997 cm−1 on the concentration of Hg2+ probed by this designed sensor. | |
3.5 Selectivity
As shown in Fig. 5, the SERS intensity of DAP produced by the catalysis of the Hg2+–Ag NPs is much stronger while Ca2+, Ba2+, Pb2+, Ni2+, Sn2+, Co2+, Zn2+, Mn2+ and Cd2+ provide much weaker signals. In addition, we find the Ag+ and Cu2+ cause a little stronger interference than the other cations, because OPD can be oxidized by Cu2+ and Ag+ in presence of Ag NPs, which is in accordance with previous literature.31 These results indicate that this sensor has excellent selectivity for mercury ions among heavy metal ions.
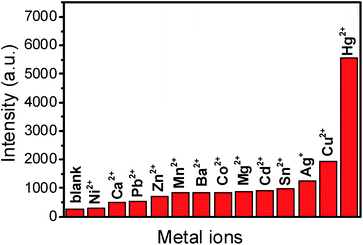 |
| Fig. 5 SERS intensities at 997 cm−1 response to common metal ions (1.0 × 10−3 M) apart from Hg2+ (1.0 × 10−6 M), Ag+ (1.0 × 10−4 M) and Cu2+ (1.0 × 10−4 M) under the optimal conditions. | |
3.6 SERS detection of real sample
In order to test the application of our sensor for Hg2+ in real sample, we adopted tap water from the campus of Jilin University with standard addition of mercury ions.
The results are listed in Table 2. We can find the recovery rate of mercury ion range from 90–95%, which proves that our sensor is practical in real samples and it displays strong anti-interference ability.
Table 2 The recovery rate of Hg2+ in local drinking water. Each sample was measured five times
Sample no. |
Real value |
Measured value |
Recovery (%) |
1 |
2.00 μM |
1.90 ± 0.400 μM |
94.5 ± 2.00 |
2 |
40.0 μM |
36.5 ± 0.300 μM |
91.2 ± 0.800 |
3 |
8.00 nM |
7.30 ± 0.100 nM |
90.6 ± 1.30 |
4 Conclusions
In summary, we adopted electrostatic assembly method to form Hg2+–Ag NPs, which can be used not only for accelerating a redox reaction between OPD and dissolved oxygen, but also for enhancing the Raman signal of the produced DAP. We used the good catalytic ability of the Hg2+–Ag NPs to develop a “turn on” SERS detection method for tracing Hg2+. The lowest concentration of mercury ion is as low as 1.0 nM. Compared with other mercury ion sensing approaches, our sensor has merits not only in the aspects of simple operation and low cost, but also in its sensing behaviours of high sensitivity and good selectivity. These merits indicate that this SERS sensor has applicable potential in water samples.
Acknowledgements
This work was supported by the National Instrumentation Program (NIP) of the Ministry of Science and Technology of China (Grant no. 2011YQ03012408), National Natural Science Foundation of China (Grant nos 21373096) and Innovation Program of the State Laboratory of Supramolecular Structure and Materials, Jilin University.
Notes and references
- H. Tan, Q. Li, C. Ma, Y. Song, F. Xu and S. Chen, Biosens. Bioelectron., 2015, 63, 566 CrossRef CAS PubMed.
- S. Liu, X. Qin, J. Tian, L. Wang and X. Sun, Sens. Actuators, B, 2012, 171–172, 886 CrossRef CAS PubMed.
- M. Yang, W. Meng, X. Liu, N. Su, J. Zhou and B. Yang, RSC Adv., 2014, 4, 22288 RSC.
- S. J. L. Billinge, E. J. McKimmy, M. Shatnawi, H. J. Kim, V. Petkov and D. Wermeille, J. Am. Chem. Soc., 2005, 127, 8492 CrossRef CAS PubMed.
- S. Yoon, A. E. Alber, A. P. Wong and C. J. Chang, J. Am. Chem. Soc., 2005, 127, 16030 CrossRef CAS PubMed.
- G. K. Darbha, A. Ray and P. C. Ray, ACS Nano, 2007, 1, 208 CrossRef CAS PubMed.
- M. Zhu, Y. Wang, Y. Deng, L. Yao, S. B. Adeloju and D. Pan, Biosens. Bioelectron., 2014, 61, 14 CrossRef CAS PubMed.
- Z. Mohammadpour, A. Safavi and M. Shamsipur, Chem. Eng. J., 2014, 255, 1 CrossRef CAS PubMed.
- Y. J. Long, Y. F. Li, Y. Liu, J. J. Zheng, J. Tang and C. Z. Huang, Chem. Commun., 2011, 47, 11939 RSC.
- V. Bhalia, R. Tejpal, M. Kumar and A. Sethi, Inorg. Chem., 2009, 48, 11677 CrossRef PubMed.
- S. Chen, D. Liu, Z. Wang, X. Sun, D. Cui and X. Chen, Nanoscale, 2013, 5, 6731 RSC.
- Y. Du, R. Liu, B. Liu, S. Wang, M. Y. Han and Z. Zhang, Anal. Chem., 2013, 85, 3160 CrossRef CAS PubMed.
- Y. Takahashi, S. Danwittayakul and T. M. Suzuki, Analyst, 2009, 134, 1380 RSC.
- J. Zhang, L. Mao, G. Yang, X. Gao and X. Tang, Spectrosc. Spectral Anal., 2010, 30, 1979 CAS.
- Q. Li, Z. Zhang and Z. Wang, Anal. Chim. Acta, 2014, 845, 7 CrossRef CAS PubMed.
- H. Matusiewicz, J. Fish and T. Malinski, Anal. Chem., 1987, 59, 2264 CrossRef CAS.
- A. Q. Shah, T. G. Kazi, J. A. Baig, H. I. Afridi and M. B. Arain, Food Chem., 2012, 134, 2345 CrossRef CAS PubMed.
- I. Serafimovski, I. Karadjova, T. Stafilov and J. Cvetkovic, Microchem. J., 2008, 89, 42 CrossRef CAS PubMed.
- R. Fukai and L. Huynh-Ngoc, Anal. Chim. Acta, 1976, 83, 375 CrossRef CAS.
- M. Hatle, Talanta, 1987, 34, 1001 CrossRef CAS.
- Y. Takahashi, S. Danwittayakul and S. M. Toshishige, Analyst, 2009, 134, 1380 RSC.
- E. Punrat, S. Chuanwatanakwl, T. Kaneta, S. Motomizu and O. Chailapakul, J. Electroanal. Chem., 2014, 727, 78 CrossRef CAS PubMed.
- S. Qiao, Y. Ding, D. Tian, Y. Li and G. Yang, Appl. Spectrosc. Rev., 2014, 9, 11746 Search PubMed.
- Y. Du, R. Liu, B. Liu, S. Wang, M. Y. Han and Z. Zhang, Anal. Chem., 2013, 85, 3160 CrossRef CAS PubMed.
- L. Zhang, H. Chang, A. Hirata, H. Wu, Q. K. Xue and M. Chen, ACS Nano, 2013, 7, 4595 CrossRef CAS PubMed.
- P. C. Lee and D. Meisel, J. Phys. Chem. C, 1982, 86, 3391 CrossRef CAS.
- L. Yan, Z. Chen, Z. Zhang, C. Qu, L. Chen and D. Shen, Analyst, 2013, 138, 4280 RSC.
- S. Fornera and P. Walde, Anal. Biochem., 2010, 407, 293 CrossRef CAS PubMed.
- G. Roman-Gusetu and K. C. Waldron, J. Chromatogr. A, 2009, 1216, 8270 CrossRef CAS PubMed.
- H. Wang, W. Jiang, Y. Wang, X. Liu, J. Yao and L. Yuan, Langmuir, 2013, 29, 3 CrossRef CAS PubMed.
- X. Yang and E. Wang, Anal. Chem., 2011, 83, 5005 CrossRef CAS PubMed.
- Z. Wu, G. Zhou, J. Jiang, G. Shen and R. Yu, Talanta, 2006, 70, 533 CrossRef CAS PubMed.
- P. D. Zhou, H. Liu, S. C. Chen, L. Lucia, H. Y. Zhan and S. Y. Fu, Molbank., 2011, M730 CrossRef CAS.
- S. Sylvestre, S. Sebastian, K. Oudayakumar, T. Jayavarthanan and N. Sundaraganesan, Spectrochim. Acta, Part A, 2012, 96, 401 CrossRef CAS PubMed.
- M. J. Natan, Faraday Discuss., 2006, 132, 321 RSC.
- X.-M. Lin, Y. Cui, Y.-H. Xu, B. Ren and Z.-Q. Tian, Anal. Bioanal. Chem., 2009, 394, 1729 CrossRef CAS PubMed.
Footnote |
† Electronic supplementary information (ESI) available. See DOI: 10.1039/c5ra08009f |
|
This journal is © The Royal Society of Chemistry 2015 |
Click here to see how this site uses Cookies. View our privacy policy here.