DOI:
10.1039/C5RA08076B
(Paper)
RSC Adv., 2015,
5, 52726-52736
Preparation of silver decorated silica nanocomposite rods for catalytic and surface-enhanced Raman scattering applications†
Received
2nd May 2015
, Accepted 10th June 2015
First published on 10th June 2015
Abstract
This paper describes a straightforward approach for the decoration of Ag nanoparticles onto the surface of mesoporous silica nanorods (denoted as SiO2@Ag nanocomposite rods), in which polyvinylpyrrolidone (PVP) serves as both a reductant and stabilizer. In this approach, mesoporous silica nanorods are initially synthesized through a binary surfactant template method by using CTAB and F127 in a basic aqueous solution. Subsequently, through the electrostatic attraction between the negatively charged silanol groups and the positively charged [Ag(NH3)2]+ ions, the silver precursor-[Ag(NH3)2]+ ions can be adsorbed onto the surfaces of mesoporous silica nanorods. Then, those [Ag(NH3)2]+ are in situ reduced to metallic Ag nanoparticles and stay there with the protection of PVP, consequently, SiO2@Ag nanocomposite rods are formed. By adjusting the concentration of [Ag(NH3)2]+ ions, the size of Ag nanoparticles and the surface coverage of mesoporous silica nanorods by Ag nanoparticles can be easily tailored. During the synthesis, neither additional reductants nor surface modifications are necessary. These as-synthesized SiO2@Ag nanocomposite rods show excellent catalytic activity for the reduction of organic dyes, which may be useful for wastewater treatment. Furthermore, these SiO2@Ag nanocomposite rods are ideal candidates as surface-enhanced Raman spectroscopy (SERS) active substrates for the trace detection of antibiotics, i.e., penicillin G sodium and chloramphenicol. This SERS feature may be applicable for organic residue detection in food.
Introduction
Metal nanoparticles have attracted great scientific interests in the fields of chemistry, physics and biotechnology during the past few decades. Especially, a lot of attention on noble metal nanoparticles (e.g. Au, Ag, Pd and Pt) has been paid because of their unique optical, electronic and catalytic properties originating from their nanoscale effects, which are markedly different with their counterpart bulk phase.1,2 Therefore, these size-induced quantum effects of metal nanoparticles have been widely exploited for a huge diversity of novel applications as sensors, catalysts, optical switches, nanoelectronic devices, biological reagents, etc.1–3 However, these metal nanoparticles are prone to coalesce due to van der Waals forces and a high surface energy, which can lead to an easy aggregation and deactivation in practical applications. To overcome these shortcomings, many different approaches have been developed, which are mainly based on the incorporation of metal nanoparticles into/onto various stable host matrices1–5 or coating nanoparticles with a protective shell.6–9 The later strategy is efficient from the protection view point, but the unique features that only nanoparticles demonstrate are partly suppressed or even completely diminished because of the barrier layer. In addition, the homogenously coating of single nanoparticle is not easy, the multi-encapsulation of the nanoparticles or the formation of a thick shell would only deteriorate the unique activity of the nanoparticles. Compared with the challenges aforementioned for the later strategy, the deposition of metal nanoparticles into/onto a stable substrate is relatively easier to achieve. Although a number of host matrices have been used so for, few of them truly meets the requirements of chemical inertness, large surface area, possible modification for specific demands and commercial available.10,11 Among these host matrices, silica is an excellent candidate used for supporting metal nanoparticles against aggregation, not only because of its chemical inactiveness and controllable surface area and property, but also because of the rich diverse selection in its shape and morphology. Spherical (0D), film (2D) and aerogel (3D) shaped silica materials have been successfully fabricated and used as the substrates for supporting metal nanoparticles.7,12–14 However, one dimensional silica materials have not been used yet to the best of our knowledge.
Silica nanorods are of a great interest not only because of their analogues of molecular liquid crystal phases behaviors, but also as the templates for the formation of rod-like composite particles with distinct optical, electrical and mechanical properties which are not realized by spherical particles alone.15–18 Serving as the templates, silica nanorods decorated with zero-dimensional metal nanoparticles can combine the materials with distinctly different physical and chemical properties to yield unique hybrid systems with promising tunable properties for the new generation of applications in sensors, optics and catalysis.19–21 In addition, compared with zero-dimensional silica materials, silica nanorods have more surface area, which may lead to an increase in the amount of nanoparticles loaded. Therefore, it is worthwhile to use silica nanorods as the matrices for the preparation of nanocomposite rods which may perform advanced properties for future applications.
Up to now, many different approaches have been demonstrated to successfully prepare silica–metal nanocomposites, e.g., surface seeding methods and/or electroless plating, surface functionalization deposition, interfacial reaction, layer-by-layer (LbL) technology, self-assembly, and so on.6,22,23 Among these impressive success, surface functionalization or modification of host matrices is crucial to achieve the decoration of uniform metallic nanoparticle coating on the host matrices. Basically, one typical surface functionalization process involves the usage of some functional linkers or metals (such as organosilanes,24–27 SnCl2,28 palladium24 and gold29) so as to activate the surfaces of the host matrices; the others are achieved by the layer-by-layer (LbL) self-assembly of polyelectrolytes and metal nanoparticles alternatively on the surfaces of the host matrices.26 In addition, some reductants (such as hydrazine, KBH4, sodium citrate or ascorbic acid), reducing solvents (dimethylformamide, polyols), or external energies (γ-ray, ultrasound, ultraviolet or microwave) have to apply to reduce the metallic precursors to their zero-valence states.30–34 In spite of the success of these approaches, it is still worth to develop a facile and feasible way which does not suffer from the impurities existing in the final nanocomposites system, the complicated preparation process and the usage of additional reductants that cannot be avoided in the above approaches.
Herein, we proposed a simple method for the decoration of silver nanoparticles onto mesoporous silica nanorods (denoted as SiO2@Ag nanocomposite rods) aided by polyvinylpyrrolidone (PVP) for the catalytic and surface enhanced Raman spectroscopy application. In this approach, initially, mesoporous silica nanorods were prepared by using CTAB and F127 as the binary surfactant templates, and then, after the extraction of CTAB and F127, the surface of mesoporous silica nanorods was saturated with opposite charged [Ag(NH3)2]+ ions. The presence of PVP promotes the [Ag(NH3)2]+ ions in situ reduced to Ag nanoparticles home-fixed on the surface of silica nanorod substrate. The other function of PVP is to maintain the stability of the system during the reaction, in other words, results in an aggregate-free system. The Ag nanoparticle size and the coverage degree of silica nanorods can be easily tailored via the precise adjustment of Ag precursor content. Compared with the approaches aforementioned, neither extra reductants, linker nor pre-surface activation were necessary in this approach. These SiO2@Ag nanocomposite rods were ideal catalysts for the catalytic degradation of an organic dye (Rhodamine B) and as the active substrates for the surface-enhanced Raman spectroscopy (SERS) test of trace amount of antibiotics (penicillin G sodium and chloramphenicol).
Experimental section
Materials
Polyvinylpyrrolidone (PVP, MW = 40
000 g mol−1) and triblock copolymer Pluronic F-127 (EO100PO70EO100, EO = ethylene oxide, PO = propylene oxide) were purchased from Sigma-Aldrich without further purification. Tetraethyl orthosilicate (TEOS, ≥99.8%), hexadecyltrimethyl-ammonium bromide (CTAB, ≥99.8%), hydrochloric acid (HCl, 36.0–38.0%), silver nitrate (AgNO3, ≥99.8%), aqueous ammonia (28 wt% aqueous solution), absolute ethanol were purchased from Sinopharm Chemical Reagent Co., Ltd (China) and used as received. Penicillin G sodium and chloramphenicol were bought from Aladdin Industrial Corporation (China) and stored at 4 °C until use. Potassium borohydride (KBH4, ≥99.8%), Rhodamine B were used in the experiment. Ultrapure water (>17 MΩ cm−1) from a GZY-P10 water system was used throughout the experiments.
Synthesis of mesoporous silica nanorods
Mesoporous silica nanorods were synthesized by using CTAB and F127 as the binary templates in a basic aqueous solution at room temperature according to the procedures in the ref. 20 and 21. Typically, first, all of F127 (0.1573 g), CTAB (12.5 mL aqueous solution, 0.04 M) and aqueous ammonia solution (15 mL, 2.5 wt%) were mixed together to form a colorless and transparent mixture at room temperature. Subsequently, 0.6 mL of TEOS was added into the above mixture under a stir. After about 5 min, the mixture was allowed to stay standing for 3 h at room temperature. The mixture gradually turned to become cloudy. The SiO2 nanorods were collected by centrifugation and washed with ethanol and deionized water three times. Upon heating the as-prepared SiO2 nanorods to reflux in an ethanol/hydrochloric acidic solution at 90 °C for 24 h, the template surfactant molecules inside the mesoporous channels were removed. After the extraction, the mesoporous SiO2 nanorods were washed three times with deionized water, and finally, dried 24 h in a vacuum oven at room temperature.
Preparation of SiO2@Ag nanocomposite rods
The typical strategy used for the fabrication of SiO2@Ag nanocomposite rods is illustrated in Scheme 1. Typically, first, the stock dispersion was prepared by dispersing mesoporous SiO2 nanorods powder (0.15 g) in ethanol (15 g). Meanwhile, the PVP solution was prepared by dissolving PVP (0.1 g) in ethanol (4 g) at room temperature, which was then mixed with the as-prepared SiO2 nanorods dispersion. Subsequently, 1 mL of freshly prepared [Ag(NH3)2]+ (0.588 to 1.76 M) aqueous solution was quickly fed into the SiO2 nanorods dispersion, which was magnetically stirred at a speed of 300 rpm for 1 h. Later on, the mixture was heated up to 70 °C and the temperature was kept for 7 h. The SiO2@Ag nanocomposite rods were collected by centrifugation and washed with ethanol and ultrapure water several times. The samples were stored in the ultrapure water at room temperature for further use.
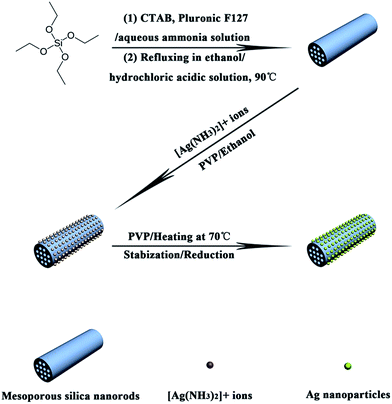 |
| Scheme 1 Schematic diagram illustrating the formation of SiO2@Ag nanocomposite rods. | |
Catalytic property of SiO2@Ag nanocomposite rods
For the catalytic examination, in general, a certain amount of SiO2@Ag nanocomposite rods were first dispersed in ultrapure water. On the other hand, both Rhodamine B (1 × 10−5 M) and KBH4 (1 × 10−2 M) aqueous solutions were freshly prepared, separately. Then, a given amount of SiO2@Ag nanocomposite rods dispersion was mixed with 10 mL of Rhodamine B solution, and subsequently, 1 mL of KBH4 aqueous solutions was rapidly injected into the above mixture under a stir. With the experiment proceeding, the color of the mixture was gradually fading, indicating a gradual reduction of Rhodamine B. During this period, UV-Vis absorption spectra were used to study the catalytic performance of SiO2@Ag nanocomposite rods by monitoring the variation in optical density at the wavelength of the absorbance maximum (λmax) of Rhodamine B.
SERS property of SiO2@Ag nanocomposite rods
Penicillin G sodium and chloramphenicol were selected as the model probe molecules for the SERS measurement. During the measurement, the as-prepared SiO2@Ag nanocomposite rods (20 mg) were first dispersed in 10 g of ethanol by sonication for 30 min, and then, dried onto the glass slides as the substrates. Subsequently, 200 μL of penicillin G sodium aqueous solutions (from 1 × 10−2 to 1 × 10−10 M) and chloramphenicol aqueous solutions (from 1 × 10−3 to 1 × 10−11 M) were dropped onto the prepared SERS-active substrates (10 × 10 mm), separately, which were then dried under ambient conditions before tests. The Raman measurements were performed at 25 °C on an inVia Raman Microscope equipped with a 532 nm He–Ne laser. 1 mW of laser irradiation intensity was used to excite the samples throughout the experiments. The data acquisition time was 10 s for one accumulation. To obtain the reproducible SERS data, all the SERS measurement were carried out under the same conditions iteratively: e.g. each sample was measured 3 times within 10 min, with 6 randomly chosen areas on the sample, which was focused for 10 s at 25 °C.
Characterization
TEM observation. The morphologies of SiO2 nanorods and SiO2@Ag nanocomposite rods were examined with transmission electron microscopy (TEM, JEOL JEM-2100, Japan). All samples were diluted with ethanol, and ultrasonically treated at 25 °C for 10 min prior to the deposition onto the carbon-coated copper grids for observation.
BET analysis. Nitrogen-adsorption–desorption isotherms of mesoporous SiO2 nanorods were recorded at 77 K on a QUADRASORB SI-4 Analyzer (Quantachrome Instruments, USA). Samples were degassed at 200 °C for 6 h prior to the measurement. The specific surface area of mesoporous SiO2 nanorods was determined by using a multipoint BET method, and the pore-size distribution was calculated from desorption isotherms by using Barrett–Joyner–Halenda method.
X-ray photoelectron spectroscopy. XPS measurement was performed on an AXIS Ultra X-ray photoelectron spectrometer (Kratos Analytical Ltd. UK) equipped with a monochromatized Al Kα X-ray source (1486.6 eV). All binding energies were calibrated by using the containment carbon (C1s = 284.6 eV).
X-ray diffraction. Powder X-ray diffraction of SiO2@Ag nanocomposite rods was performed on a DX-2700 X-ray diffractometer equipped with a Cu tube and a diffracted beam curved graphite monochromator operated at 40 kV and 30 mA. The XRD patterns were recorded by scanning the SiO2@Ag powders deposited on a glass substrate with a scanning rate of 0.02 degrees (2θ) per second in the range of 30° and 80° (2θ).
UV-visible spectrum. UV-visible spectrophotometer (Lambda 35, PerkinElmer) was used to record the UV-visible absorption spectra of the samples. All samples were placed in the 1 cm × 1 cm × 3 cm quartz cuvettes, and recorded at room temperature.
SERS measurement. SERS spectra of penicillin G sodium and chloramphenicol enhanced by the SiO2@Ag based SERS-active substrates were recorded on an inVia Raman Microscope (Renishaw, UK) equipped with a 532 nm He–Ne laser, separately. 1 mW of laser irradiation intensity was used to excite the samples throughout the experiments. The signals were collected in 10 s.
Results and discussion
Preparation and characterization of mesoporous silica nanorods
To synthesize mesoporous silica nanorods, two functional surfactants, in our case, hexadecyltrimethyl-ammonium bromide (CTAB) and a triblock copolymer Pluronic F127 were used as the templates in a basic aqueous environment. The silica precursor tetraethyl orthosilicate (TEOS) was hydrolyzed and polymerized through a classic ammonia-catalyzed approach. The absence of externally vigorous disturbance, e.g., stirring, would guarantee the formation of uniform mesoporous silica nanorods rather than a polydisperse system. Note, without F 127, only silica nanospheres were formed.35 These binary surfactant templates (CTAB and F127) are not permanently connected to silica, which can be easily removed by rinsing the as-synthesized silica nanorods in the ethanol/hydrochloric acidic environment at an elevated temperature (90 °C). TEM image in Fig. 1a shows that the result silica nanorods are uniform in size with an average length of 479 nm and diameter of 96 nm. A close inspection of single silica rod as shown in Fig. 1b demonstrates a highly ordered channel-like pore structure inside the silica nanorods, which directly confirms the formation of mesoporous structure through this soft binary surfactant templates method.
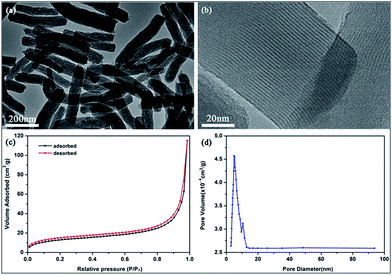 |
| Fig. 1 Transmission electron microscopy (TEM) images of (a) mesoporous silica nanorods and (b) samples in (a) at a high magnification; (c) nitrogen-adsorption–desorption isotherms and (d) Barrett–Joyner–Halenda (BJH) pore-size-distribution of mesoporous silica nanorods. | |
In order to quantitatively analysis the mesoporous structures of SiO2 nanorods, N2 adsorption/desorption measurements were carried out after the removal of the surfactant templates. As shown in Fig. 1c, the as-synthesized SiO2 nanorods show a hysteresis loops indicating the hierarchical characteristics. The Barrett–Joyner–Halenda (BJH) pore size distribution curve derived from N2 adsorption/desorption isotherms was plotted in Fig. 1d. A sharp peak in the size distribution curve present indicates the uniformity of the channel-like pores inside the SiO2 nanorods. The average diameter of the mesopores is 4.9 nm, which may be suitable for the loading of silver nanoparticles with a size smaller than 4.9 nm. Correspondingly, their Brunauer–Emmett–Teller (BET) surface area is as high as 785 m2 g−1. Taking advantages of their uniform mesoporous structure, high specific surface area and one-dimensional nanostructure, these mesoporous silica nanorods have a great potential as the versatile substrate to prepare a variety of one-dimensional nanocomposite materials used for sensors, optics and catalysis applications.20,21
Preparation and characterization of SiO2@Ag nanocomposite rods
The detailed preparation process of SiO2@Ag nanocomposite rods has been illustrated in Scheme 1 and Experimental section. As demonstrated, mesoporous silica nanorods as the templates were dispersed in ethanol. The selection of polar reaction media would offer a good water dispersity of the products for the potential environmental remediation application, e.g., decomposition of pollutant and antibiotic detection as demonstrated in the following sections. [Ag(NH3)2]+ ions were served as the silver source, which were adsorbed by the surfaces of mesoporous silica nanorods via the electrostatic attraction between Si–OH groups and [Ag(NH3)2]+ ions.17 In the synthesis, PVP was the key ingredient which played two roles. During the introduction of [Ag(NH3)2]+ ions, PVP served as the steric stabilizer protected those mesoporous silica nanorods against aggregation. Otherwise, some aggregations would be formed in the dispersion because the negatively charged silica nanorods (Si–OH groups) were rapidly neutralized by [Ag(NH3)2]+ ions. When the dispersion was heated up to 70 °C, PVP exhibited its reductive nature which enables to reduce [Ag(NH3)2]+ ions to Ag nanoparticles in situ captured by mesoporous silica nanorods. These preliminary Ag nanoparticles would serve as the seeds, nanoparticle growth took place.17 In addition, it is not clear but still worth mentioning here that PVP show an attraction with silica materials, which may contribute to the in situ fixation of Ag nanoparticles on the surface of silica nanorods.19,36,37
TEM images in Fig. 2 show the morphologies of SiO2@Ag nanocomposite rods prepared by using various concentrations of [Ag(NH3)2]+ ions. In comparison with the pristine mesoporous silica nanorods (see Fig. 1a), the relatively rough surface of nanocomposite rods was observed, indicating a successful deposition of Ag nanoparticles on the mesoporous silica nanorods (see Fig. 2a, c, e, g and i). When the concentration of [Ag(NH3)2]+ ions added was low, for example, 0.588 M, a small amount of Ag nanoparticles sparsely covered on the surfaces of mesoporous silica nanorods was observed (see Fig. 2a). As the concentration of [Ag(NH3)2]+ ions was increased from 0.882 M to 1.47 M, the amount of Ag nanoparticles decorated on the mesoporous silica nanorods was correspondingly increased (see Fig. S1 in ESI†). Although the covering of Ag nanoparticles on the silica nanorods were observed homogeneously, the size of the Ag particles were not uniform. This is probably caused by the low concentration of silver precursor.38 Provided the concentration of [Ag(NH3)2]+ ions increased, the polydispersity of the result Ag nanoparticles will decrease. As expected, when the concentration of [Ag(NH3)2]+ ions was further increased to 1.76 M, the uniform Ag nanoparticles were obtained. Both the size and polydispersity of the result Ag nanoparticles were measured and calculated based on their TEM observation. As the concentration of [Ag(NH3)2]+ ions increased from 0.588 to 1.76 M, the average size of Ag nanoparticles on SiO2@Ag nanocomposite rods increased from 7.63 nm to 12.24 nm, while the polydispersity were decreased from 28.2 to 18.1%, correspondingly. These results are consistence with our previous work.38 Since the sizes of the result Ag nanoparticles are bigger than the diameter of the mesopores of silica nanorods, the fill of Ag nanoparticles inside the silica nanorods, unfortunately, is failed, which has been confirmed by the results shown in Fig. 2. In addition, the coverage of the Ag nanoparticles on the surface of silica nanoparticles increased as more Ag precursor was fed. Further increase the content of [Ag(NH3)2]+ ions would finally lead to a complete Ag shell (see Fig. S2 in ESI†).
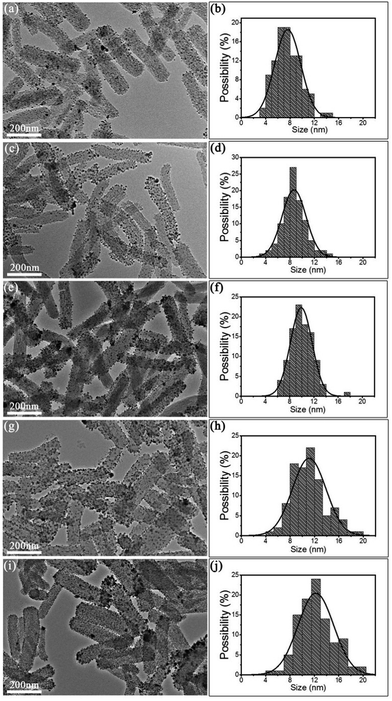 |
| Fig. 2 Transmission electron microscopy (TEM) images of SiO2@Ag nanocomposite rods prepared by using various concentrations of [Ag(NH3)2]+ ions: (a) 0.588 M; (c) 0.882 M; (e) 1.16 M; (g) 1.47 M and (i) 1.76 M; (b, d, f, h, j) their related size distribution histograms of Ag nanoparticles on SiO2@Ag nanocomposite rods, respectively. | |
Additionally, X-ray photoelectron spectroscopy (XPS) was employed to analyze the surface chemical composition of mesoporous silica nanorods and SiO2@Ag nanocomposite rods. As shown in Fig. 3a, prior to the decoration with Ag nanoparticles, only silicon (Si2p, Si2s), carbon (C1s) and oxygen (O1s) signal peaks were observed in the XPS spectrum. In contrast, after the decoration, the surface chemical elements varied due to the introduction of Ag nanoparticles. As shown in Fig. 3b, besides the peaks of Si2p, Si2s, C1s, N1s and O1s, new signal peaks emerged, that is, Ag3d (Ag3d5/2, Ag3d3/2) and Ag3p (Ag3p5/2, Ag3p3/2) which are strong proof for the successful employment of Ag element. A close inspection of Ag3d was shown in Fig. 3c, which demonstrates the two peaks at 368.2 eV and 374.2 eV with a spin–orbit separation of 6.0 eV, corresponding to the binding energies of Ag3d5/2 and Ag3d3/2, respectively. These two characteristic peaks of Ag3d5/2 and Ag3d3/2 are attributed to the Ag0 species, which again certainly indicate the presence of zero-valent metallic silver nanoparticles. XRD is the other powerful tool with which the element specie will be determined with the position of the pattern. As shown in Fig. 3d, a typical XRD characterization of SiO2@Ag nanocomposite rods exhibits the peaks at 2θ angles of 37.9°, 44.1°, 64.3° and 77.2°, corresponding to the reflections of (111), (200), (220) and (311) crystalline planes of the fcc structure of Ag (JCPDS no. 04-0783), respectively. Combined the results from XRD, XPS and TEM, the result of the successful preparation of mesoporous SiO2 nanorods decorated with fcc Ag nanoparticles assisted by PVP can be concluded.
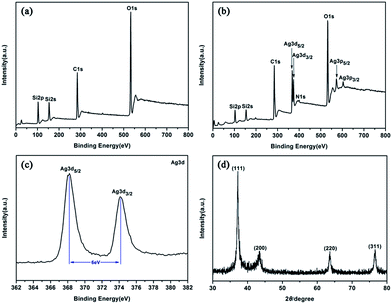 |
| Fig. 3 X-ray photoelectron spectroscopy (XPS) scans of (a) mesoporous silica nanorods; (b) SiO2@Ag nanocomposite rods (c) Ag3d core-level spectrum of SiO2@Ag nanocomposite rods (d) X-ray diffraction (XRD) patterns of SiO2@Ag nanocomposite rods. | |
Generally, PVP, a kind of non-ionic polymer, was widely used as the steric stabilizers or capping agents in the chemical synthesis of colloidal polymer39–42 and nanocomposite particles, because of its high chemical stability, nontoxicity and excellent solubility in many polar solvents.17,38,43–46 Recently, PVP as the mild reductant has been demonstrated to successfully reduce some noble metal (e.g., Au, Ag, Pd and Pt) salts to their metal nanoparticles in aqueous media, where the reductive native of PVP was mostly related to its hydroxyl end groups.47–49 Here, again, PVP was proved to be efficient as the reductant and stabilizer in our system. [Ag(NH3)2]+ ions were in situ reduced and protected by PVP, forming stable SiO2@Ag nanocomposite particles in single step, neither the additional reducing agents nor the surface modifications were necessary during this synthesis process. The versatileness of this method may allow it to extend applicable to the other similar system, for instance, titania materials.
Optical property of SiO2@Ag nanocomposite rods
The UV-visible absorption spectra have been recorded for mesoporous silica nanorods and SiO2@Ag nanocomposite rods prepared with various concentrations of [Ag(NH3)2]+ ions, as shown in Fig. 4. There was no distinct absorption peak shown in the UV-visible absorption spectra (300–800 nm) of bare silica nanorods (curve a). But after the decoration of Ag nanoparticles, a strong absorption peak appeared at around 412 nm (curve b), which is owed to the Mie plasmonic resonance excitation from Ag nanoparticles attached.50,51 As the concentration of [Ag(NH3)2]+ ions increased (curves c–f), the position of plasmonic resonance peak were gradually redshift, and the peaks broadened as well, which indicated the growth of Ag nanoparticles in size and the incremental surface coverage of Ag nanoparticles on the surfaces of mesoporous silica nanorods. These are consistent with the TEM results shown in Fig. 2.
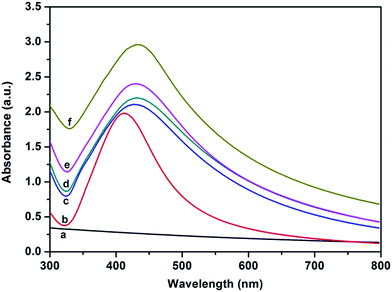 |
| Fig. 4 UV-visible absorption spectra of (a) mesoporous silica nanorods and SiO2@Ag nanocomposite rods prepared by using various concentrations of [Ag(NH3)2]+ ions: (b) 0.588 M; (c) 0.882 M; (d) 1.16 M; (e) 1.47 M and (f) 1.76 M. | |
Catalytic property of SiO2@Ag nanocomposite rods
Metal nanoparticles can exhibit unexpectedly high catalytic activities towards different types of reactions, e.g., hydrogenation, hydroformylation, carbonylation.52,53 Unfortunately, in most cases, the high catalytic activities are hampered or even lost during the practical catalytic processes because these active metal nanoparticles are prone to coalesce due to the strong van der Waals forces and their high surface energy. This problem is usually figured out by either coating nanoparticles with a protective layer or depositing nanoparticles onto a stable yet inert substrate. The later manner is much straightforward and efficient since the protective layer may suppress the catalytic activity performed. This way, the silica nanorods are the ideal substrates because of their inert and stable nature, and high surface area. Therefore, the as-synthesized SiO2@Ag nanocomposite rods are able to exert a high performance in various practical applications which mostly likely takes place in aqueous environments, e.g., waste water treatment, antibiotics detection in food, etc.
Generally, most of dyes are an abundant class of synthetic, colored organic compounds, which represent an increasing environmental danger due to their toxicity, potentially carcinogenic nature and resistant to the aerobic degradation.54,55 Herein, we introduce these SiO2@Ag nanocomposite rods as the dye decomposer catalyst to mimic the remediation of organic chemical polluted environment. In order to easily monitor the progress of the experiment, Rhodamine B was selected as the model organic dye contaminant, while the KBH4 as the reductant. The decoloration of the dye-nanocomposite aqueous mixture demonstrated the degradation of the dye quantitatively reflected with the help of UV-visible spectra. The evolution of the UV-visible spectra of Rhodamine B reduced by variable concentrations of SiO2@Ag nanocomposite rod at the wavelength of absorbance maximum (λmax) in the presence of KBH4 has been illustrated in Fig. 5. Meanwhile, the reaction systems in the absence of SiO2@Ag nanocomposite rods and Ag nanoparticles were also present for the sake of comparison. As shown in Fig. 5a, the absence of SiO2@Ag nanocomposite rods allowed the color of Rhodamine B solution remaining for 24 h. While, the absence of Ag nanoparticles only resulted in a slight decrease of the absorbance at λmax of Rhodamine B (see Fig. 5b), which was caused by the physical adsorption of Rhodamine B by mesoporous silica nanorods. The significantly changes were observed when the reaction system contained SiO2@Ag nanocomposite rods. The absorbance at the λmax of Rhodamine B was quickly decreased in a relatively short reaction time (see Fig. 5c–f). As increasing the concentration of SiO2@Ag nanocomposite rods, the degradation of Rhodamine B was accelerated, as shown the two comparisons in Fig. 5c and d (1.16 M of [Ag(NH3)2]+), and Fig. 5e and f (1.76 M of [Ag(NH3)2]+), that is, the complete degradation time of the dye was shortened from 36 to 27 min as the concentration of SiO2@Ag nanocomposite rods increased from 0.1 to 0.15 wt% (see Fig. 5c and d); the similar phenomenon was observed with the high content Ag nanoparticles loaded system used as well (see Fig. 5e and f). On the other hand, increase the concentration of Ag nanoparticles loaded would also increase the degradation of the dye, as compared with the samples in Fig. 5c and e and 5d and f, respectively. These results hint that the catalytic activities of SiO2@Ag nanocomposite rods are mainly drawn from those Ag nanoparticles attached on the surfaces of mesoporous silica nanorods.
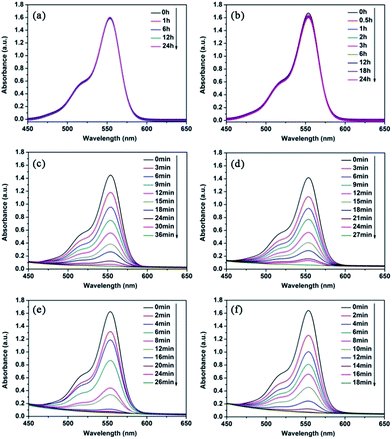 |
| Fig. 5 UV-visible spectra of Rhodamine B aqueous solution reduced by KBH4 (a) in the absence of SiO2@Ag nanocomposite rods; (b) in the presence of mesoporous silica nanorods: 0.15 wt%, 0.5 mL; (c–f) in the presence of SiO2@Ag nanocomposite rods prepared at the concentration of (c and d) 1.16 M or (e and f) 1.76 M of [Ag(NH3)2]+ ions as functions of reaction time and catalyst. SiO2@Ag nanocomposite rods dispersions: (c and e) 0.10 wt% and (d and f) 0.15 wt%, 0.5 mL; Rhodamine B: 1 × 10−5 M, 10 mL; KBH4: 1 × 10−2 M, 1 mL. | |
In addition, the mechanism of the catalytic reduction of dyes by metal nanoparticles can be explained as follows: the Ag nanoparticles deposited on the mesoporous silica nanorods can be served as the electron relay in the reaction system for an oxidant and a reductant. The electron transfer occurs via these supported metallic Ag nanoparticles. In principle, dyes are electrophilic and BH4− ions are nucleophilic with respect to Ag nanoparticles. In this reaction, the nucleophile-KBH4 donates electrons to Ag nanoparticles, and the electrophile-dyes would capture those electrons from Ag nanoparticles. As a result, these Ag nanoparticles were served as an electron relay for the catalytic reduction of dyes in KBH4 solution.17
To further study the influence of SiO2@Ag nanocomposite rods on the reduction of Rhodamine B, the catalytic degradation of the process can be directly read from those curves (Fig. 5c–f), as the ratio of the concentration ct of Rhodamine B at the time t to its value c0 at t = 0 is directly given by the ratio of their respective absorbance A/A0. Since the concentration of KBH4 largely exceeded the concentration of Rhodamine B as indicated in the Experimental section, the rates of reduction were assumed to be independent with the concentration of KBH4 concentration. Therefore, the kinetic data can be fitted by a first-order rate law, by which a first-order rate kinetics with regard to the Rhodamine B concentration were used to study the catalytic rates as follows:54,55
|
 | (1) |
where
ct is the concentration of Rhodamine B at the time
t and
kapp is the apparent reaction rate constant.
In this case, the ratio of ct (the concentration of Rhodamine B at the time t) to c0 at t = 0 was directly given by the ratio of their respective absorbance at λmax of Rhodamine B (554 nm). Hence, the apparent reaction rate constant kapp was obtained as the ratio of ln(ct/c0) to time t. This allows us to compare the reaction systems with SiO2@Ag nanocomposite rods at different concentrations. As shown in Fig. 6, the linear relations between ln(ct/c0) versus time t have been obtained in all cases illustrated in Fig. 5. The absence of Ag nanoparticles resulted in the extreme small values (0.000645 and 0.00146 h−1 for the reactions without SiO2@Ag nanocomposite rods and Ag nanoparticles, respectively) of kapp, which reveal that both KBH4 and mesoporous silica nanorods have a very poor reductive capability to the dye in the absence of Ag nanoparticles. As the Ag nanoparticles had been decorated onto the silica nanorods, the degradation was vastly enhanced. In detail, when the SiO2@Ag nanocomposite rods prepared at the concentration of 1.16 M of [Ag(NH3)2]+ ions were used as the catalysts, the kapp increased from 1.5586 to 1.5736 h−1 as the amount of SiO2@Ag nanocomposite rods dispersions increased from 0.10 wt% to 0.15 wt%, correspondingly. Similarly, for the SiO2@Ag nanocomposite rods with a high Ag content (e.g., 1.76 M [Ag(NH3)2]+ ions were used), the kapp increased from 2.3203 to 2.3684 h−1, respectively. Apart from that, it is also observed that the kapp increased rapider in the system with a high concentration of Ag nanoparticles than that in the system with a low concentration of Ag nanoparticles. This is also applicable for the system with the same amount of silica nanorods but different amount of Ag nanoparticles as compared the samples in Fig. 5c and e and 5d and f. In consistence with the conclusions gained from Fig. 5, these results further demonstrated that the increase either of the concentration of SiO2@Ag nanocomposite rods or the amount of Ag nanoparticles in SiO2@Ag nanocomposite rods would lead to a rapid gain in the rate constant.
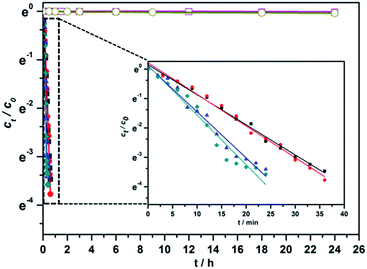 |
| Fig. 6 Influence of SiO2@Ag nanocomposite rods with different concentrations on the degradation of Rhodamine B, which corresponds to the data in Fig. 5. The concentrations of the reactants are as follows: Rhodamine B (1 × 10−5 M) and KBH4(1 × 10−2 M) in absence of SiO2@Ag nanocomposite rods (□); in the presence of mesoporous silica nanorods(○); and with the SiO2@Ag nanocomposite rods prepared at the concentration of 1.16 M of [Ag(NH3)2]+ ions (■: 0.10 wt%; ●: 0.15 wt%); and 1.76 M of [Ag(NH3)2]+ ions (▲: 0.10 wt%; ◆: 0.15 wt%); solid lines are their correspondingly linear fittings. | |
Besides its catalytic efficiency, the recyclability is the other key property for the catalyst used in applications, because it will allow the catalyst for a multiple use and correspondingly reduce its economic cost. To demonstrate the renewable catalytic activity of SiO2@Ag nanocomposite rods, the recycling experiment was carried out by repeating the dye (Rhodamine B) degradation eight times. As shown in Fig. 7, it is found that after eight recycling reactions, SiO2@Ag nanocomposite rods were still stable in the reaction media. The degradation efficiency was still as high as 89.8%. The slight drop in degradation efficiency of Rhodamine B was probably ascribed to the loss of SiO2@Ag nanocomposite rods during the centrifuge. Base on the results above, these SiO2@Ag nanocomposite rods were proven to be a potent recyclable catalyst for the wastewater treatment applications.
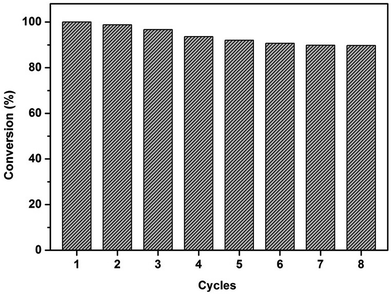 |
| Fig. 7 Recyclable catalytic activity of SiO2@Ag nanocomposite rods as a catalyst for the degradation of Rhodamine B with KBH4. | |
SERS property of SiO2@Ag nanocomposite rods
As well known, antibiotics (such as penicillin, kanamycin, chloramphenicol, cephalosporin) have been widely used as the efficient antibacterial drugs for the clinical treatments of various infectious diseases, saving a enormous number of human lives as well as animals. Some of them have been proved with severely adverse effects when they are overloaded by live entities, but still used in clinical medicine, veterinary medicine and food additive because of their high antibacterial efficiency and low cost. This drug abuse and irregularity in the use of antibiotics are often causing the drug residues in animal foods and animal themselves, which will bring the potential health risks to human beings and the ecological environment as well. Therefore, it is eagerly desired to develop a sensitive, reliable and simple method for the detection of the antibiotics.
Surface-enhanced Raman scattering (SERS) has been considered as a sensitive and powerful analytical technique for the detection and identification of molecules, especially, the signals of which will be vastly enhanced when the molecules are adsorbed on the surfaces of plasmonic nanostructured materials. It has been demonstrated that the large enhancement is attributed to the highly concentrated electromagnetic fields associated with the strong localized surface plasmonic resonances at the interstitial sites (so-called SERS “hot spots”) in those nanostructures consisting of two or more coupled plasmonic metal (e.g., Au or Ag) nanoparticles or nanostructured surfaces with closely spaced features.56 Here, by making use of these novel SiO2@Ag nanocomposite rods as the “hot spots”, the effectively SERS-active substrates have been prepared for the trace detection of the antibiotics. The SERS performances of the as-synthesized SiO2@Ag based substrates were evaluated by using two kinds of antibiotics-penicillin G sodium and chloramphenicol as the probe molecules, separately.
In detail, SiO2@Ag nanocomposite rods with the uniform Ag nanoparticles coating (the sample as shown in Fig. 2i) were used as the model system to prepare the effective SERS-active substrates so as to investigate the trace detection of penicillin G sodium and chloramphenicol, respectively. The corresponding SERS spectra at the different concentrations of penicillin G sodium and chloramphenicol were obtained, as shown in Fig. 8. As a comparison, the spectra of two antibiotics deposited on the glass slides in the absence of SiO2@Ag nanocomposite rods were also scanned, and shown in Fig. 8. In Fig. 8a–10, it was clearly shown that no Raman peak was identified at 1 × 10−2 M of penicillin G sodium on the glass slides in the absence of SiO2@Ag nanocomposite rods. While, under the same condition (1 × 10−2 M of penicillin G sodium), the strong patterns of penicillin G sodium molecule emerged solely in the presence of SiO2@Ag nanocomposite rods. The main Raman peaks are assigned to the out-of-plane deformation vibrations of beta-lactam and thiazolidine rings (676.6 cm−1), the deformation vibrations of the CH groups from beta-lactam and thiazolidine rings (1379.9 cm−1), the stretching vibration of C–O in the carboxyl (1556.3 cm−1), the asymmetric stretching vibration of the CH groups from beta-lactam ring (2939.7 cm−1), respectively.57 In order to test the sensitivity of the measurement, the concentration of penicillin G sodium was decreased from 1 × 10−2 to 1 × 10−10 M by a factor of 10−1. As the curves 1–9 in shown Fig. 8a, the pattern intensity of penicillin G sodium decreased with less penicillin G sodium present. All characteristic peaks of penicillin G sodium molecule were distinguishable, even when the penicillin G sodium aqueous solution was diluted to a level as low as 1 × 10−10 M, as shown in Fig. 8c. Although the concentration of penicillin G sodium was extremely low (1 × 10−10 M), the signals of SERS were still strong, demonstrating the high sensitivity of the measurement aided by the as-synthesized SiO2@Ag nanocomposite rods.
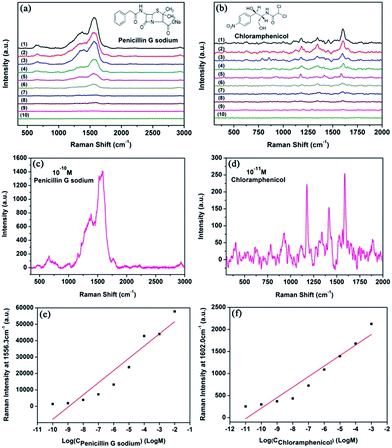 |
| Fig. 8 SERS spectra of penicillin G sodium (a and c) and chloramphenicol (b and d) at different concentrations: (a) curves 1–9: 1 × 10−2 to 1 × 10−10 M, in the presence of SiO2@Ag nanocomposite rods; curve 10: 1 × 10−2 M, no SiO2@Ag nanocomposite rod was involved. (b) Curves 1–9: 1 × 10−3 to 1 × 10−11 M, in the presence of SiO2@Ag nanocomposite rods; curve 10: 1 × 10−3 M, no SiO2@Ag nanocomposite rod was involved. (c) and (d) The detailed SERS spectra of penicillin G sodium at 1 × 10−10 M and chloramphenicol at 1 × 10−11 M, respectively. (e and f) The relationship between SERS intensity at 1556.3 cm−1, 1602.0 cm−1 and the concentrations of penicillin G sodium, chloramphenicol in solution, respectively. | |
To further confirm the high sensitivity also applicable for other antibiotics, the as-synthesized SiO2@Ag nanocomposite rods based SERS-active substrates were also used for the trace detection of the other commercial antibiotic chloramphenicol. The corresponding SERS spectra of chloramphenicol at different concentrations ranging from 1 × 10−3 to 1 × 10−11 M were shown in Fig. 8b. The three main peaks at 1184.3, 1346.7, 1602.0 cm−1 were attributed to the aromatic C–H in-plane bending, NO2 symmetric stretching, and ring stretching vibration of chloramphenicol molecule, respectively.58 An obvious enhanced effect is shown in comparison with the curve 1 and 10 in Fig. 8b. It is hard to distinguish the characteristic patterns of chloramphenicol at the concentration of 10−3 M in the absence of SERS-active substrates (see Fig. 8b–10). While in the presence of SERS-active substrates, the results were vastly enhanced (see Fig. 8b–1). Similar to the results of penicillin G sodium, as the concentration of the chloramphenicol decreased, the intensity of the peaks was declined. The signals were still detectable when as low as 1 × 10−11 M of chloramphenicol was used (see Fig. 8d).
In order to quantitatively study the detection performance, the SERS intensity (I) of penicillin G sodium emerged at 1556.3 cm−1 was plotted as a function of log(C), where C is the concentration of penicillin G sodium used. As shown in Fig. 8e, the SERS intensity seems linearly increased with increasing the concentration of penicillin G sodium, which can be fitted in the first order.59 This fitting may help to roughly determine the concentration limit in SERS, lower than which the characteristic patterns of the chemicals would not be detected. For example, if the penicillin G sodium aqueous solution was diluted to 10−11 M, the SERS would not detect the penicillin G sodium anymore. A similar treatment was carried out with the results of chloramphenicol in Fig. 8b. As summarized in Fig. 8f, the intensity of chloramphenicol was decreased with the dilution of chloramphenicol solution. The concentration limit was observed at 10−11 M. Based on these results, we believed that the detection limits for penicillin G sodium and chloramphenicol on the SiO2@Ag nanocomposite rods based SERS-active substrates were 10−10 and 10−11 M, respectively, which were lower than those detected on the pure Ag nanoparticles based SERS-active substrates from literatures.60,61
According to previous studies,62,63 the sensitivity of a SERS substrate can be characterized by the enhancement factors (EF) for each given molecule. The SERS enhancement factors (EF) for penicillin G sodium and chloramphenicol deposited on the SiO2@Ag nanocomposite rods can be described by:
|
EF = (ISERS/Ibulk)(Nbulk/Nads)
| (2) |
where
ISERS and
Ibulk are the signal intensities of SERS and normal Raman spectra, respectively;
Nbulk and
Nads are the number of molecules in the cross section of the laser beam from the concentrated sample and the number of adsorbed molecules in the cross section of laser beam, respectively. In our experiment, the cross sections of the laser bean were identical for the Raman and SERS performances because the same Raman spectrometer was used. Thus, the
Nbulk and
Nads may be replaced by the concentrations of the bulk and SERS samples, respectively.
64 In this experiment, the main Raman bands at 1556.3 cm
−1 and 1602.0 cm
−1 were selected to estimate the EF values for penicillin G sodium and chloramphenicol, respectively. Therefore, the EF was calculated to be about 3.17 × 10
8 and 2.50 × 10
8 for the SERS detection of penicillin G sodium and chloramphenicol, respectively, which is higher than the reported results where only nanoparticles were used.
60,65
Conclusions
In summary, we presented a facile approach to fabricate SiO2@Ag nanocomposite rods with the aid of PVP served both as the reductant and stabilizer. Neither additional reductants nor surface modification were needed during the synthesis. The formation of SiO2@Ag nanocomposite rods has been fully investigated with a rich variety of characterizations (TEM, XPS and XRD). Upon adjusting the concentration of silver precursor-[Ag(NH3)2]+ ions, the size of Ag nanoparticle decorated and the surface coverage of mesoporous silica nanorods can be easily tailored. In addition, these as-synthesized SiO2@Ag nanocomposite rods have been proved to have a good catalytic activity with a recyclable feature in the degradation of Rhodamine B dye. On the other hand, the preliminary SERS measurements indicated that these as-synthesized SiO2@Ag nanocomposite rods can be used as the ultrasensitive SERS substrates for the trace detection of antibiotics, which may be useful for the food testing. Based on these results, we believe these SiO2@Ag nanocomposite rods can be broadly used as the recyclable catalysts for reducing organic dyes in the wastewater treatment applications and as the SERS-active substrates for biomedical and environmental applications.
In addition, this approach also presents a versatile paradigm for the preparation of many types of materials with complex shapes. On the basis of this technique, a number of nanocomposite materials coated with a diversity of metallic (Au, Ag, Pd and Pt, etc.) nanoparticles could be achieved, which may show the unique promising feature for biological and materials applications.
Acknowledgements
The financial supports from the National Natural Science Foundation of China (no. 51203087, 51473089), the Scientific Research Foundation for the Returned Overseas Chinese Scholars, State Education Ministry, the Natural Science Basic Research Plan in Shaanxi Province of China (no. 2013JQ2006) and the Fundamental Research Funds for the Central Universities(no. GK201503039, GK201501002, GK201301002, GK201101003) are appreciated.
Notes and references
- Y. Sun and Y. Xia, Science, 2002, 298, 2176–2179 CrossRef CAS PubMed.
- Y. N. Xia, Y. J. Xiong, B. Lim and S. E. Skrabalak, Angew. Chem., Int. Ed., 2009, 48, 60–103 CrossRef CAS PubMed.
- A. R. Tao, S. Habas and P. D. Yang, Small, 2008, 4, 310–325 CrossRef CAS PubMed.
- T. Liu, D. Li, D. Yang and M. Jiang, Chem. Commun., 2011, 47, 5169–5171 RSC.
- T. Liu, D. Li, D. Yang and M. Jiang, Colloids Surf., A, 2011, 387, 17–22 CrossRef CAS PubMed.
- X. Peng, J. Chen, J. A. Misewich and S. S. Wong, Chem. Soc. Rev., 2009, 38, 1076–1098 RSC.
- R. M. Crooks, M. Zhao, L. Sun, V. Chechik and L. K. Yeung, Acc. Chem. Res., 2000, 34, 181–190 CrossRef PubMed.
- X. Sun and Y. Li, Angew. Chem., Int. Ed., 2004, 43, 597–601 CrossRef PubMed.
- S. H. Joo, S. J. Choi, I. Oh, J. Kwak, Z. Liu, O. Terasaki and R. Ryoo, Nature, 2001, 412, 169–172 CrossRef CAS PubMed.
- N. R. Jana, C. Earhart and J. Y. Ying, Chem. Mater., 2007, 19, 5074–5082 CrossRef CAS.
- K. Aslan, M. Wu, J. R. Lakowicz and C. D. Geddes, J. Am. Chem. Soc., 2007, 129, 1524–1525 CrossRef CAS PubMed.
- H. Lu, L. Fan, Q. Liu, J. Wei, T. Ren and J. Du, Polym. Chem., 2012, 3, 2217–2227 RSC.
- S. S. Bale, P. Asuri, S. S. Karajanagi, J. S. Dordick and R. S. Kane, Adv. Mater., 2007, 19, 3167–3170 CrossRef CAS PubMed.
- W. P. Xu, L. C. Zhang, J. P. Li, Y. Lu, H. H. Li, Y. N. Ma, W. D. Wang and S. H. Yu, J. Mater. Chem., 2011, 21, 4593–4597 RSC.
- H. Qi, K. E. Shopsowitz, W. Y. Hamad and M. J. MacLachlan, J. Am. Chem. Soc., 2011, 133, 3728–3731 CrossRef CAS PubMed.
- J. H. Zhang, J. B. Liu, S. Z. Wang, P. Zhan, Z. L. Wang and N. B. Ming, Adv. Funct. Mater., 2004, 14, 1089–1096 CrossRef CAS PubMed.
- Z. W. Deng, M. Chen and L. M. Wu, J. Phys. Chem. C, 2007, 111, 11692–11698 CAS.
- N. C. Bigall, A. K. Herrmann, M. Vogel, M. Rose, P. Simon, W. Carrillo Cabrera, D. Dorfs, S. Kaskel, N. Gaponik and A. Eychmüller, Angew. Chem., Int. Ed., 2009, 48, 9731–9734 CrossRef CAS PubMed.
- A. Kuijk, A. van Blaaderen and A. Imhof, J. Am. Chem. Soc., 2011, 133, 2346–2349 CrossRef CAS PubMed.
- J. F. Ye, H. J. Zhang, R. Yang, X. G. Li and L. M. Qi, Small, 2010, 6, 296–306 CrossRef CAS PubMed.
- L. You, T. Wang and J. Ge, Chem.–Eur. J., 2013, 19, 2142–2149 CrossRef CAS PubMed.
- S. E. Habas, P. D. Yang and T. Mokari, J. Am. Chem. Soc., 2008, 130, 3294–3295 CrossRef CAS PubMed.
- S. E. Habas, H. Lee, V. Radmilovic, G. A. Somorjai and P. Yang, Nat. Mater., 2007, 6, 692–697 CrossRef CAS PubMed.
- Y. Kobayashi, V. Salgueirino-Maceira and L. M. Liz-Marzan, Chem. Mater., 2001, 13, 1630–1633 CrossRef CAS.
- B. Sadtler and A. Wei, Chem. Commun., 2002, 1604–1605 RSC.
- T. Cassagneau and F. Caruso, Adv. Mater., 2002, 14, 732–736 CrossRef CAS.
- Z. J. Jiang and C. Y. Liu, J. Phys. Chem. B, 2003, 107, 12411–12415 CrossRef CAS.
- B. J. Jankiewicz, D. Jamiola, J. Choma and M. Jaroniec, Adv. Colloid Interface Sci., 2012, 170, 28–47 CrossRef CAS PubMed.
- A. Warshawsky and D. A. Upson, J. Polym. Sci., Part A: Polym. Chem., 1989, 27, 2963–2994 CrossRef CAS PubMed.
- F. Gao, Q. Lu and S. Komarneni, Chem. Mater., 2005, 17, 856–860 CrossRef CAS.
- A. Henglein and M. Giersig, J. Phys. Chem. B, 1999, 103, 9533–9539 CrossRef CAS.
- H. H. Huang, X. P. Ni, G. L. Loy, C. H. Chew, K. L. Tan, F. C. Loh, J. F. Deng and G. Q. Xu, Langmuir, 1996, 12, 909–912 CrossRef CAS.
- I. Pastoriza-Santos and L. M. Liz-Marzán, Langmuir, 2002, 18, 2888–2894 CrossRef CAS.
- A. L. Rogach, G. P. Shevchenko, Z. M. Afanas'ev and V. V. Sviridov, J. Phys. Chem. B, 1997, 101, 8129–8132 CrossRef CAS.
- X. Hu, Y. Wang and B. Peng, Chem.–Asian J., 2013, 9, 319–327 CrossRef PubMed.
- C. Graf, D. L. J. Vossen, A. Imhof and A. van Blaaderen, Langmuir, 2003, 19, 6693–6700 CrossRef CAS.
- B. Peng, G. Soligno, M. Kamp, B. de Nijs, J. de Graaf, M. Dijkstra, R. van Roij, A. van Blaaderen and A. Imhof, Soft Matter, 2014, 10, 9644–9650 RSC.
- Y. Cong, T. Xia, M. Zou, Z. N. Li, B. Peng, D. Z. Guo and Z. W. Deng, J. Mater. Chem. B, 2014, 2, 3450–3461 RSC.
- B. Peng, A. van Blaaderen and A. Imhof, ACS Appl. Mater. Interfaces, 2013, 5, 4277–4284 CAS.
- B. Peng, E. van der Wee, A. Imhof and A. van Blaaderen, Langmuir, 2012, 28, 6776–6785 CrossRef CAS PubMed.
- B. Peng, H. R. Vutukuri, A. van Blaaderen and A. Imhof, J. Mater. Chem., 2012, 22, 21893–21900 RSC.
- B. Peng and A. Imhof, Soft Matter, 2015, 11, 3589–3598 RSC.
- Z. W. Deng, M. Chen, G. X. Gu and L. M. Wu, J. Phys. Chem. B, 2008, 112, 16–22 CrossRef CAS PubMed.
- Z. W. Deng, M. Chen, S. X. Zhou, B. You and L. M. Wu, Langmuir, 2006, 22, 6403–6407 CrossRef CAS PubMed.
- Z. W. Deng, Z. P. Zhen, X. X. Hu, S. L. Wu, Z. S. Xu and P. K. Chu, Biomaterials, 2011, 32, 4976–4986 CrossRef CAS PubMed.
- Z. N. Li, C. J. Wu, K. Zhao, B. Peng and Z. W. Deng, Colloids Surf., A, 2015, 470, 80–91 CrossRef CAS PubMed.
- I. Washio, Y. Xiong, Y. Yin and Y. Xia, Adv. Mater., 2006, 18, 1745–1749 CrossRef CAS PubMed.
- Y. Xiong, I. Washio, J. Chen, H. Cai, Z. Li and Y. Xia, Langmuir, 2006, 22, 8563–8570 CrossRef CAS PubMed.
- C. E. Hoppe, M. Lazzari, I. Pardiñas-Blanco and M. A. López-Quintela, Langmuir, 2006, 22, 7027–7034 CrossRef CAS PubMed.
- A. B. R. Mayer, W. Grebner and R. Wannemacher, J. Phys. Chem. B, 2000, 104, 7278–7285 CrossRef CAS.
- K. Kim, H. S. Kim and H. K. Park, Langmuir, 2006, 22, 8083–8088 CrossRef CAS PubMed.
- T. S. Ahmadi, Z. L. Wang, T. C. Green, A. Henglein and M. A. El-Sayed, Science, 1996, 272, 1924–1925 CAS.
- Z. Jiang, C. Liu and L. Sun, J. Phys. Chem. B, 2005, 109, 1730–1735 CrossRef CAS PubMed.
- Y. Lu, Y. Mei, M. Drechsler and M. Ballauff, Angew. Chem., Int. Ed., 2006, 45, 813–816 CrossRef CAS PubMed.
- Y. Lu, Y. Mei, M. Ballauff and M. Drechsler, J. Phys. Chem. B, 2006, 110, 3930–3937 CrossRef CAS PubMed.
- P. L. Stiles, J. A. Dieringer, N. C. Shah and R. R. Van Duyne, Annu. Rev. Anal. Chem., 2008, 1, 601–626 CrossRef CAS PubMed.
- T. Iliescu, M. Baia and I. Pavel, J. Raman Spectrosc., 2006, 37, 318–325 CrossRef CAS PubMed.
- D. Sajan, G. D. Sockalingum, M. Manfait, I. Hubert Joe and V. S. Jayakumar, J. Raman Spectrosc., 2008, 39, 1772–1783 CrossRef CAS PubMed.
- J. Huang, F. Chen, Q. Zhang, Y. Zhan, D. Ma, K. Xu and Y. Zhao, ACS Appl. Mater. Interfaces, 2015, 7, 5725–5735 CAS.
- X. Jiang, X. Qin, D. Yin, M. Gong, L. Yang, B. Zhao and W. Ruan, Spectrochim. Acta, Part A, 2015, 140, 474–478 CrossRef CAS PubMed.
- L. He, M. Lin, H. Li and N. Kim, J. Raman Spectrosc., 2010, 41, 739–744 CAS.
- E. C. Le Ru, E. Blackie, M. Meyer and P. G. Etchegoin, J. Phys. Chem. C, 2007, 111, 13794–13803 CAS.
- B. K. Jena, B. K. Mishra and S. Bohidar, J. Phys. Chem. C, 2009, 113, 14753–14758 CAS.
- Y. Liu, L. L. Liu and R. Guo, Langmuir, 2010, 26, 13479–13485 CrossRef CAS PubMed.
- W. Ji and W. Yao, Spectrochim. Acta, Part A, 2015, 144, 125–130 CrossRef CAS PubMed.
Footnote |
† Electronic supplementary information (ESI) available: The amount of Ag nanoparticles loaded on SiO2@Ag nanocomposite rods; TEM images of SiO2@Ag nanocomposite rods with complete Ag shells. See DOI: 10.1039/c5ra08076b |
|
This journal is © The Royal Society of Chemistry 2015 |