DOI:
10.1039/D3QM00586K
(Review Article)
Mater. Chem. Front., 2024,
8, 159-178
Sub-nanometric materials for hydrogen evolution reaction
Received
23rd May 2023
, Accepted 21st September 2023
First published on 22nd September 2023
Abstract
With the development of renewable and clean energy, hydrogen energy is booming as an alternative to fossil energy. Hydrogen energy has the highest mass-energy density of all fuels and is versatile enough to be considered as the ultimate energy source for the planet. Among them, electrolytic hydrogen production technology is considered as one of the most promising strategies for large-scale hydrogen production. Among the current electrocatalytic hydrogen evolution catalysts, sub-nanometric materials (SNMs) have become the focus of research in recent years due to their atomic size and different compositions. In contrast to nanometric materials (NMs), SNMs have essentially fully exposed atoms, in addition to having different active sites for different reaction intermediates, which facilitate their enhanced electrocatalytic performance. In this review, firstly, we affirm the absolute advantages of SNM catalysts in the hydrogen evolution reaction (HER), and then outline the mechanism of the HER and the methods to evaluate the catalyst performance, and introduce the strategies to enhance the catalyst performance, such as synergistic effects, support anchoring, doping and vacancy defects. Next, the application of high-performance noble metal-based Pt, Pd and Ru-based SNMs in the HER is illustrated, and finally the prospects and challenges of SNMs development as HER electrocatalysts are foreseen.
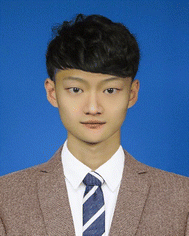
Yaodong Yu
| Yaodong Yu is currently a PhD student under the supervision of Prof. Jianping Lai and Lei Wang in Qingdao University of Science and Technology. He received his Bachelor of Engineering degree from the School of Chemical and Molecular Engineering, Qingdao University of Science and Technology in 2020, where he pursued his PhD degree in 2022. His current research interests are in the synthesis of nanomaterials and their applications in electrocatalysis. |
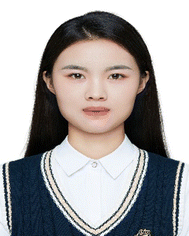
Ruixin Liu
| Ruixin Liu is currently pursuing her Bachelor's degree in the College of Chemical and Molecular Engineering at Qingdao University of Science and Technology. Her major is Molecular Science and Engineering. |
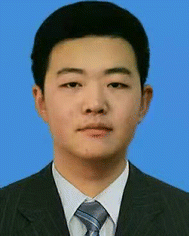
Yuyao Sun
| Yuyao Sun is a PhD candidate under the supervision of Prof. Jianping Lai and Prof. Lei Wang at Qingdao University of Science and Technology. He received his Master degree from the College of Chemical and Molecular Engineering, Qingdao University of Science and Technology in 2022. His current research interests include the synthesis of micro- and nanostructured materials and their applications in electrocatalysis. |
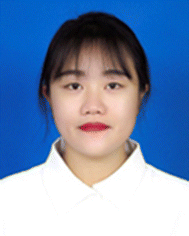
Ziyi Liu
| Ziyi Liu is currently studying for her master's degree at Qingdao University of Science and Technology under the supervision of professors Jianping Lai and Wang Lei. The current research direction is clean energy electrocatalytic materials. |
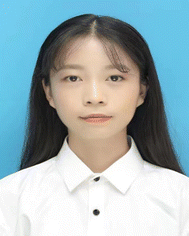
Xinrong Shi
| Xinrong Shi is currently an undergraduate at Qingdao University of Science and Technology. The tutor is Professor Jianping Lai. Entered School of Chemistry and Molecular Engineering, Qingdao University of Science and Technology, in 2021. |
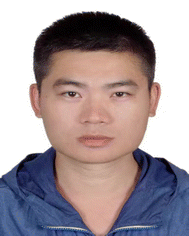
Jianping Lai
| Jianping Lai is currently a professor at Qingdao University of Science and Technology. He received a postdoctoral at Peking University. He received his BS degree from College of Chemistry in Fuzhou University (2011) and PhD degree from Changchun Institute of Applied Chemistry, Chinese Academy of Sciences (2017). His research interests focus on the synthesis and electrocatalytic applications of nanomaterials. |
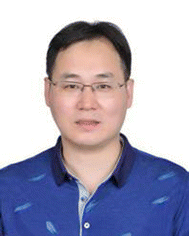
Lei Wang
| Lei Wang is currently a professor at Qingdao University of Science and Technology. He obtained his PhD degree from the Jilin University in 2006 under the direction of Prof. Shouhua Feng. And then, he went to Shandong University to work as a postdoctoral. His main research interests are focused on energy storage and conversion materials, electrocatalysis, photocatalysis, supramolecular chemistry, metal–organic coordination polymers and organic–inorganic hybrid materials. |
1. Introduction
As the global population grows every year, the demand for fossil fuels continues to grow rapidly.1,2 The consumption of fossil fuels today brings satisfaction to mankind, but also poses a great threat to the planet on which mankind depends: global warming, atmospheric pollution, etc.3–5 Therefore, we urgently need to develop clean and sustainable energy sources to gradually reduce the use of fossil energy.6–9 Hydrogen energy has been considered as one of the cleanest and most promising modes of energy utilization, since the only combustion product is water, which can be recycled.10–13 However, unlike energy sources such as oil and natural gas, which are already present on the earth, hydrogen energy is not naturally occurring and therefore it needs to be produced or converted from other sources to be used.14,15 There are many ways to obtain hydrogen energy, such as: electrochemical hydrocracking, hydrogen production from water gas, and hydrogen production from decomposing water from renewable energy sources (e.g. solar, wind, tidal and geothermal).16–19 Among them, Electrocatalytic HER is considered to be a clean, efficient and sustainable alternative strategy for hydrogen production from fossil fuels.20–22 The process of hydrogen production from electrolytic water, like other chemical reactions, requires overcoming the energy barriers from reactants to activation products for the reaction to proceed smoothly.23–25 Development of high performance HER electrocatalysts has become an important strategy for hydrogen production.26,27
Today, NMs have become a hot topic in chemistry because of their unique size effect.28–31 NMs are materials with at least one dimension on the scale of 1–100 nm.32,33 SNMs are located in the transition zone between NMs and molecules, with dimensions around or even less than 1 nm, leading to significant changes in many properties.34–36 Compared to NMs, SNMs exhibit different properties, with their atoms almost completely exposed due to the size of the atomic scale. In addition, different reaction intermediates have different active sites, so the construction of sub-nanometre-scale nanoclusters helps to enhance their HER electrocatalytic performance.34,37 The current widely used ones are noble metal-based SNMs, but the high cost of noble metals limits their large-scale application.38–41 Optimization of the SNMs by strategies such as support anchoring and doping, their activity is comparable to that of Pt/C catalysts with high stability, which also results in lower loading of precious metals Pt, Pd, and Ru in the catalysts, which greatly improved their utilization.16,39,42–44
In this review, we focus on strategies to improve the catalyst performance of SNMs and their application in HER. Firstly, we summarize the HER mechanism and the methods to evaluate the performance of HER catalysts, and explain in detail the instrumental strategies to optimize the catalyst performance. In the next section, we present the progress of Pt, Pd and Ru-based SNMs for HER applications by elemental species. Finally, the challenges and trends in the future development of sub-nanoscale HER catalysts are envisioned. It is hoped that this review will provide new perspectives and insights into the application of SNMs in electrocatalysis.
2. HER mechanism and catalyst evaluation methods
2.1 HER mechanism
Depending on the reaction medium, the HER mechanism (Fig. 1(a)) can be divided into two categories: acidic and alkaline. In the acidic medium there is a large amount of free proton H+, and H+ acquires an electron on the electrode material to form a hydrogen intermediate in the adsorbed state (H*), this step is called electrochemical adsorption (Volmer reaction), and the electrochemical desorption step (Heyrovsky step) is the combination of H+ and the resulting adsorbed state H* on the electrode material to acquire an electron reaction to form H2; the combination of H* and H* to form H2 is the chemical resolution step (Tafel step).45,46 In alkaline medium, there is no large amount of free H+, so water becomes the source of supply of protons in the reaction, and H2O gains an electron to dissociate at the electrode material to produce H* and OH−, this step is called Volmer reaction, Heyrovsky step is H* and H2O combine one more electron to form H2 and OH−; the produced H* combines itself to produce H2, which is called Tafel step.16,47–49
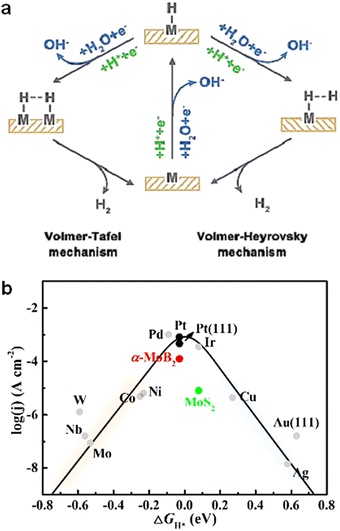 |
| Fig. 1 (a) The mechanism of HER in acidic (green route) and alkaline (blue route) electrolytes. (b) The volcano plot of acidic HER.51 | |
Regardless of the mechanism by which the HER reaction proceeds, the reaction rate and efficiency are determined by the formation and desorption processes of H*.50 From a physicochemical point of view, the adsorption and desorption of H* can be evaluated by measuring the free energy of hydrogen adsorption (ΔGH*) during HER.50,51 According to Sabatier principle, the interaction between the catalyst and the active intermediate should be moderate. If the interaction is too weak, the amount of active intermediate bound to the catalyst is too small and the reaction rate is slow, while if the interaction is too strong, the reaction product cannot be desorbed from the catalyst and its occupies more active sites and the reaction is terminated.52 A “volcano-type” diagram was created by relating the exchange current density j0. A larger ΔGH* value indicates weaker adsorption of H* and difficulty in H* production; a smaller ΔGH* value indicate stronger adsorption of H* and difficulty in H2 desorption.53 According to Fig. 1(b), the platinum group metals are located near the top of the volcano curve and have better HER reaction activity. Meanwhile, we can observe that the noble metals such as Pt, Pd and Rh are still some distance away from the top of the volcano-type curve, which indicates that the hydrogen adsorption energy of this type of catalyst can be regulated to achieve the purpose of enhancing the HER reaction activity.53
2.2 Catalyst performance evaluation methods
2.2.1 Overpotential.
Overpotential is the part of an electrochemical reaction where the potential observed in actual experiments exceeds the thermodynamically determined equilibrium potential.42 Depending on the cause of overpotential generation, overpotential can be classified into activation overpotential, concentration overpotential and resistance overpotential, of which only activation overpotential is directly related to catalytic activity of the catalyst, while concentration overpotential and resistance overpotential are caused by concentration polarization and series resistance of solutions, wires, respectively. Therefore, the activation overpotential is an important parameter to evaluate the catalytic performance. In order to obtain the accurate activation overpotential of a catalyst, a rotating electrode system is usually used to reduce the concentration overpotential and IRs compensation is used to eliminate the resistance overpotential.54–56 Where I is the current flowing through the circuit and Rs is the series resistance, the magnitude of this value can be obtained from the electrochemical impedance spectrum. Currently, the overpotential corresponding to a current density of 10 mA cm−2 is used as a benchmark for a better assessment of the catalyst activity level.
2.2.2 Tafel slope and conversion current density.
The Tafel slope is an inherent property of catalysts. It visualizes the kinetic mechanism of the catalytic decomposition reactions occurring in the electrode material. The simplified Tafel equation is η = b
log
j + a, where η, b, j and a are the overpotential,57 Tafel slope, current density and regulation constant, respectively. The unit is mV dec−1, which indicates the amount of change required in overpotential for a tenfold change in current. It is seen that the smaller the value of b, the faster the reaction kinetics. Importantly, the Tafel slope values provide insight into the reaction mechanism of HER at the catalyst surface. The theoretical Tafel slopes for the Volmer, Heyrovsky and Tafel steps in the HER reaction mechanism are 120, 40 and 30 mV dec−1, respectively.58
2.2.3 Turnover frequency.
The turnover frequency (TOF) refers to the total number of hydrogen molecules released from each active site per unit time, and it is a method to evaluate the catalytic rate of a catalyst. The common formula for calculating the TOF value of HER catalysts is
, where A is the area of the working electrode and n is the number of moles of active material calculated from the electrochemically active area of the catalyst used.42,59 The TOF can usually be obtained by cyclic voltammetry (CV) of neutral solutions, “current–voltage” absolute area integration, and related equations. Obviously, the higher the TOF, the higher the catalytic activity of the catalyst itself.60–62
2.2.4 Electrochemically active area.
The electrochemical surface area is the effective area of the catalyst exposed to the active component at the electrode surface, not simply the specific surface area of the material, which determines the kinetic parameters of the reaction. Since it is proportional to the double-layer capacitance (Cdl) in the non-Faraday interval, the electrochemically active area is usually estimated from the Cdl of the catalytic surface, which in turn reveals the number of catalyst active sites.63,64
2.2.5 Stability.
Stability is an important parameter for evaluating the catalytic performance of electrocatalysts, which is usually expressed in terms of catalyst lifetime, and refers to the usage time of a catalyst to maintain a certain reaction activity and selectivity under certain reaction conditions. Usually, electrocatalyst stability is tested by two types of methods: cyclic voltammetry and constant current methods. Cyclic voltammetry method is usually not less than 1000 times. The constant current method is usually a false current density of not less than 10 mA cm−2 and a duration of not less than 10 h. A higher number of cycles or a longer duration with no change in potential or current of the electrocatalyst indicates a better catalyst stability.59,65
3. Strategies to enhance catalyst performance
To make nanomaterial catalysts with better catalytic activity in HER, some strategies are usually used for modulation, such as heterometallic synergies, anchoring in supports, heteroatomic doping, vacancies and defects (Fig. 2).39
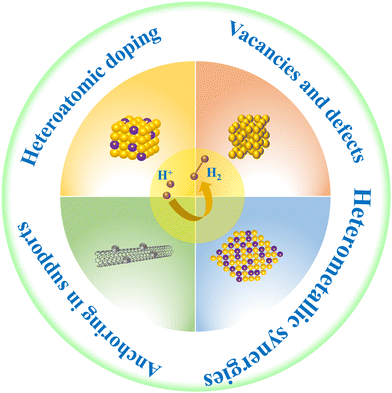 |
| Fig. 2 Strategies to enhance catalyst performance. | |
3.1 Heterometallic synergies
Synergistic effect refers to the effect of two or more components added or mixed together, which is greater than the sum of the effects of each component when applied individually. It is due to the synergistic effect of polymetallic catalysts that they exhibit excellent catalytic reaction performance. Polymetallic catalysts have attracted much attention in recent years and become a hot research topic.66,67
Heterogeneous metals have synergistic effects with each other to enhance the catalytic activity of catalysts. Ultrasmall high-entropy alloy (us-HEA) nanoparticles (NPs) with five NiCoFePtRh elements have been synthesized by an ultra-fast and powerful chemical co-reduction method (Fig. 3(a)).68 The average particle size of US-HEA is 1.68 nm, which is the smallest hydrogen evolution high-entropy alloy catalyst reported so far (Fig. 3(b)). At a current density of 10 mA cm−2, us-HEA exhibits a small overpotential of 27 mV and can maintain its stability for 100 h (Fig. 3(c) and (f)). Based on DFT theory calculations, it was found that the five elements of us-HEA exhibit synergistic effects among themselves, where Pt/Rh atoms are the main and direct active sites for HER, and Fe/Co/Ni atoms can effectively modulate the active sites (Fig. 3(d) and (e)). The five elements exhibit extremely superior performance and stability in acidic media through synergistic interaction.
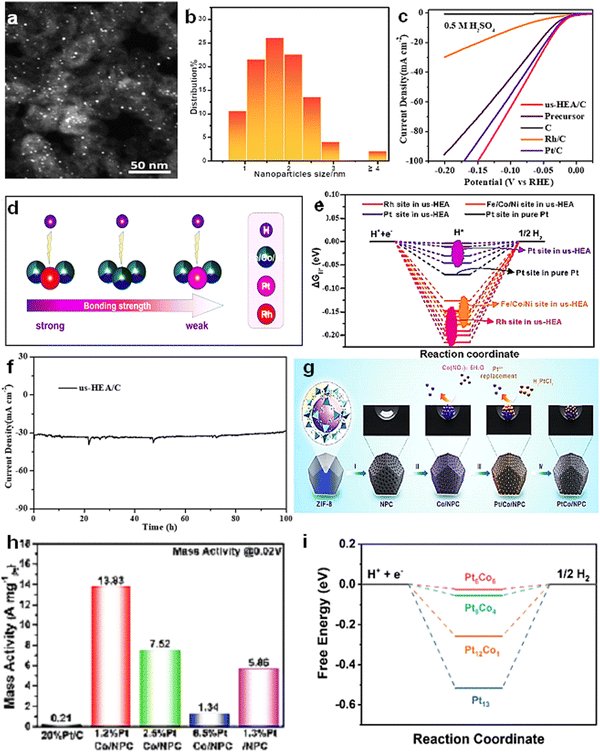 |
| Fig. 3 (a) Representative HAADF-STEM image of us-HEA NPs. (b) Size distribution histogram of us-HEA NPs. (c) Linear sweep voltammetry (LSV) curves of us-HEA/C, the precursor, C, commercial Rh/C, and commercial Pt/C (0.5 M H2SO4). (d) Schematic illustration of the binding strength between H and the Rh site, Fe/Co/Ni site, and Pt site of us-HEA, respectively. (e) Calculated hydrogen-adsorption free energy (ΔGH*) profiles for three representative sites on the us-HEA (111) surface and Pt site on pure Pt (111) surface. (f) Constant applied potential for 100 h.68 Reprinted with permission from J. Am. Chem. Soc., 2021, 143, 17117–17127. Copyright 2021, American Chemical Society. (g) Schematic diagram of the synthesis of sub-nanometer PtCo clusters confined into the small pores of ZIF-8 derived N-doped NMs. (h) Corresponding mass activity at 20 mV vs. RHE. (i) Calculated free-energy diagram of the HER on Pt6Co6, Pt9Co4, Pt12Co1 and Pt13.69 Reproduced with permission. Copyright 2021, The Royal Society of Chemistry. | |
Recently, SNMs PtCo clusters have been prepared in small holes of nitrogen-doped NMs derived from ZIF-8 by electrogenic substitution (Fig. 3(g)).69 The synthesis of SNMs-PtCo clusters in small pore channels and the synergistic interaction between Pt and Co, the catalysts showed excellent catalytic activity at low loading (1.2% of Pt). Its highest mass activity at 20 mV overpotential was 13.83 A mg−1 (Fig. 3(h)). The DFT theoretical calculations show that the enhanced d-orbital electronic coupling between Pt and Co, charge redistribution around Pt. Lower d-band center of Pt reduces the Gibbs free energy of H* adsorption, thus improving HER kinetics and activity (Fig. 3(i)).
3.2 Anchoring in supports
Some metal nanocatalysts are prone to aggregation during synthesis due to their high surface free energy. By using support anchoring as an optimization strategy, highly dispersed metal nanoclusters can be obtained to ensure more active sites for the catalysts, thus greatly enhancing the catalytic performance. The essence of support anchoring is through strong metal-support interaction (SMSI), thus significantly improving the catalytic activity and stability.70–72
By confining SNM-Pt clusters in hollow mesoporous carbon spheres (Pt5/HMCS), active and stable Pt-based hydrogen precipitation electrocatalysts were synthesized (Fig. 4(a)).73 Subsequent analysis showed that the Pt clusters were anchored in the mesoporous channels of the carbon supports. With the removal of ligand and electrochemical hydrogen production, the Pt cluster was effectively stabilized, resulting in excellent electrocatalytic performance for HER in both acidic and alkaline solutions. The overpotential was only 20.7 mV (acidic) and 46.2 mV (alkaline) at 10 mA cm−2 current density for the same Pt loading (Fig. 4(b) and (c)).
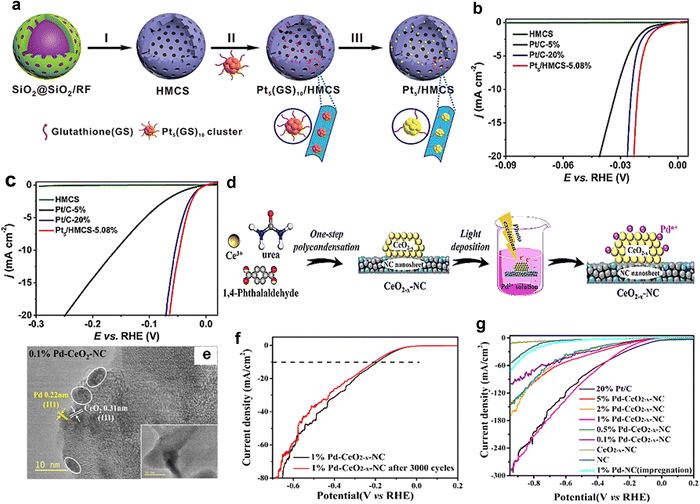 |
| Fig. 4 (a) Schematic illustration of the symthetic procedure of Pt5/HMCS. (b), (c) LSV curves of Pt5/HMCS-5.08% and commercial Pt/C (5 and 20 wt%) catalysts based on geometric area of the working electrode in: (b) 0.5 M H2SO4 and (c) 1.0 M KOH.73 Reproduced with permission. Copyright 2019, Wiley VCH. (d) Scheme illustration of the preparation process of Pd–CeO2−x-NC. (e) The HRTEM images of 0.1% Pd–CeO2−x-NC. (f) LSV curves of 1% Pd–CeO2−x-NC. (g) LSV of NC, CeO2−x-NC, 20% Pt/C and x% Pd-CeO2−x-NC.74 Reproduced with permission. Copyright 2021, Elsevier. | |
In addition, sub-nanometer Pd–CeO2−x-NC catalysts were prepared by polymerization and photodeposition (Fig. 4(d)).74 The catalyst made full use of SMSI effect to accurately anchor Pd and CeO2−x, resulting in a higher degree of uniform dispersion of Pd and CeO2−x, preventing the self-aggregation of small particles, and significantly improving the stability of the catalyst (Fig. 4(e) and (f)). It is noteworthy that the SMSI effect leads to the electron transfer from Pd to CeO2−x, forming the Pdn+ in the highly oxidized state of the dominant active species. The HER performance of Pd–CeO2−x-NC is closely related to the percentage of Pdn+, and only 1% Pd–CeO2−x-NC (overpotential and Tafel slope of 115 mV and 58 mV dec−1, respectively) exhibited HER activity comparable to 20 wt% Pt/C (overpotential and Tafel slope of 72 mV and 65 mV dec−1, respectively) (Fig. 4(g)).
3.3 Heteroatomic doping
Catalyst doping refers to the alteration of the catalyst structure by adding new substances to the catalyst, thus creating new active sites to change the catalytic performance of the catalyst. Currently, a range of metal/non-metal elements are used as dopants for catalyst materials to modulate their HER catalytic activity.75,76
In electrocatalytic reactions, doping modulation is not only considered as an effective method to modulate the active sites of catalysts, but also seems to be an important means to obtain high performance catalysts. In 2021, Wang et al.77 found that through the guidance of highly dispersed Cu atoms derived from MOF, Cu–Pt alloy nanoparticles (C-ZIF-CuPt) were prepared on N-doped porous carbon (Fig. 5(a)). The overpotential was only 46 mV at a current density of 10 mA cm−2, and the stability of C-ZIF-CuPt was found to be significantly better than that of commercial Pt/C after 1000 cycles of CV accelerated durability tests, and the strong interaction between the carbon support and the CuPt alloy enhanced the stability of the catalyst (Fig. 5(b) and (c)). The DFT calculations showed that the Cu doping enhanced the d-band center of Pt and lowered the activation energy barrier of the molecule, thus improving the HER catalytic performance of the material (Fig. 5(d)).
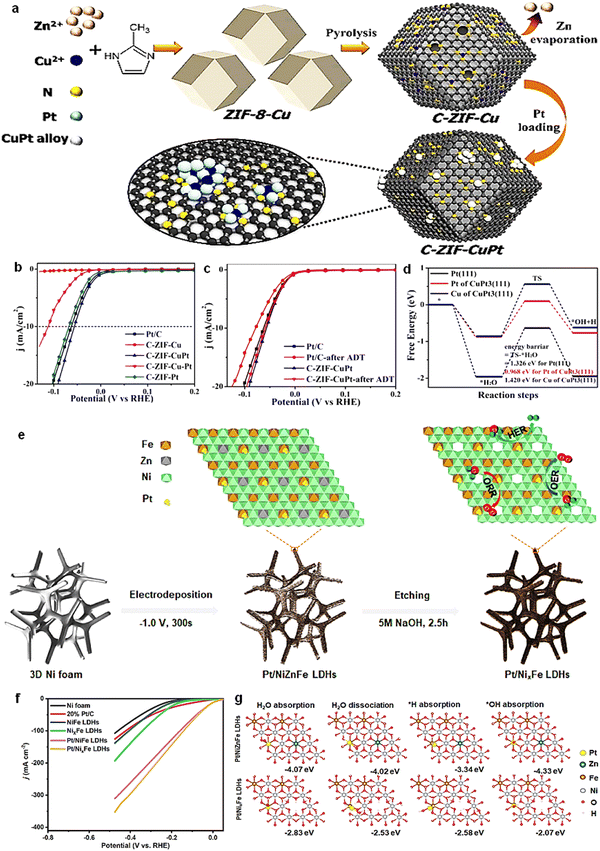 |
| Fig. 5 (a) C-ZIF-CuPt catalyst preparation process. (b) LSV curves for samples in N2-saturated 0.1 M KOH at 25 °C and 1600 rpm at a scan rate of 5 mV s−1. (c) The HER polarization curves of C-ZIF-CuPt and Pt/C before and after the ADT test by 1000 cycles. (d) The activation energy barriers of H2O molecules in the HER of three model structures.77 Reproduced with permission. Copyright 2021, Elsevier. (e)Schematic illustration of the formation of Pt/NixFe LDHs. (f) LSV curves for the HER of Pt/NixFe LDHs, Pt/NiFe LDHs, NixFe LDHs, NiFe LDHs, commercial 20% Pt/C, RuO2, and 20% Ir/C catalysts in 1 M KOH solution. (g) Optimized model structures of intermediates adsorbed on Pt/NixFe LDHs and Pt/NiZnFe LDHs surfaces.80 Reproduced with permission. Copyright 2021, American Chemical Society. | |
3.4 Vacancies and defects
Surface defects are usually formed during the crystal growth process. Adding surface defects to electrocatalysts is one of the effective ways to enhance the performance, including vacancy defects, gap filling defects, etc.78,79
Sub-nanometer Pt clusters (Pt-NCs) were prepared by simple electrodeposition and strong alkaline etching. And they were immobilized on defect-rich NiFe layered double hydroxide (NixFe-LDHs) nanosheets (Fig. 5(e)).80 The NixFe-LDHs have the best HER activity relative to the other samples in the 1 M KOH electrolyte (Fig. 5(f)). The DFT calculations showed that after selective etching of Zn sites on LDHs by strong alkaline solutions, the generation of M(II) vacancies effectively changed the surface electronic structure around the active sites, providing abundant ligand-unsaturated active sites. Their absorption energies on Pt/NixFe LDHs are lower than those on pristine Pt/NiZnFe LDHs (Fig. 5(g)), leading to more rapid Had and hydroxyl adsorption and reduction/oxidation processes, suggesting that the H2 generated on the surface of Pt/NixFe LDHs is more readily desorbed to avoid accumulation, thus promoting the exposure of active sites and further accelerating the hydrolysis process.
4. Electrocatalysis of SNMs in HER
Novel SNMs can be extended to the quantum level due to their extremely small particle size. Thus, they have unique properties and functions. The noble metals Pt, Pd, and Ru exhibit excellent catalytic activity in HER due to their unique electronic structures. However, the widespread use of noble metals is limited due to their small reserves and high costs. Therefore, related researchers have designed and prepared sub-nanometer noble metal HER catalysts by associating noble metals with sub-nanometer scales, which not only greatly reduces the loading of noble metals, but also exposes the active sites on the catalyst surface more fully and enhances the catalytic performance of the catalysts. In the following, we will discuss in detail the recent developments of precious metal Pt, Pd and Ru-based SNMs in HER according to their applications in different electrolytes.
4.1 Acidic HER
4.1.1 Pt-Based SNMs in acidic HER.
Compared to other metals, Pt is considered to be the most catalytically active and efficient metal for HER, but its high cost and low reserves have limited its widespread use.81–84
Rational design and fabrication of low Pt content and high utilization Pt-based SNMs catalysts using synergistic effects is a feasible route. It has been reported that the synergistic effect of sub-nanoparticles is greater than that of nanoparticles.67 The atomic scale mixing phase of sub-nanometer size is observed to be higher than that of nanometer size by annulardark-field scanning transmission electron microscopy (ADF-STEM), The enhancement of mixing phase improves the synergistic effect between different elements (Fig. 6(a) and (b)). Among them, Pt and Zr have the largest synergistic effect, especially Pt4Zr2 (67% atomic percentage of Pt), which has the largest synergistic effect between elements and exhibits the highest exchange current (Fig. 6(c)–(f)). The results indicate that SNMs catalysts are an attractive class of materials for applications.
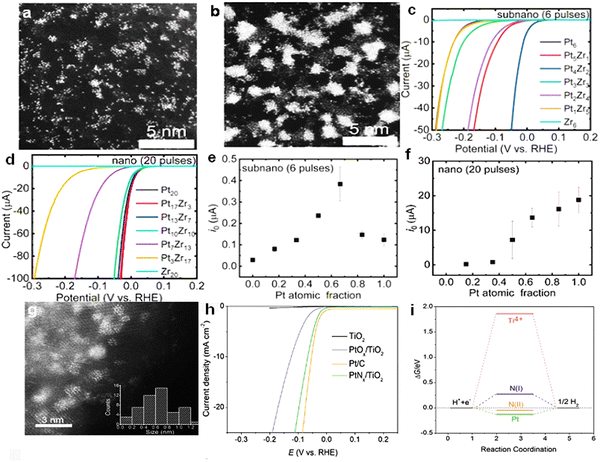 |
| Fig. 6 (a) ADF-STEM images of Pt4Zr2 SNPs. (b) ADF-STEM images of Pt13Zr7 NPs. (c), (d) Original linear sweep voltammogram (LSV) curves of PtZr alloy SNPs and NPs in the aqueous argon-saturated electrolyte (0.05 M H2SO4). (e), (f) Exchange currents (i0) of HER derived by the Tafel plots.67 Reproduced with permission. Copyright 2022, Wiley VCH. (g) High annular dark field (HAADF)-STEM images of PtNx clusters (bright spots) in the PtNx/TiO2 specimen with 3 nm scale bars. (h) HER polarization curves for PtNx/TiO2, PtOx/TiO2, Pt/C and TiO2 in H2-saturated 0.5 M H2SO4 acquired at a scan rate of 2 mV s−1. (i) Free-energy diagram for different H* adsorption sites on the PtNx/TiO2 structure.85 Reproduced with permission. Copyright 2020, Elsevier. | |
In addition, the control of the central atomic coordination environment can also greatly improve the catalytic performance. In 2020 Cheng et al.85 synthesized sub-nano raft-like PtNx clusters on TiO2 supports (PtNx/TiO2) (Fig. 6(g)). The Pt–N coordination structure was analyzed and found to have a significant enhancement of HER performance. At a current density of 10 mA cm−2, the overpotential was only 67 mV (0.5 M H2SO4), which was much lower than that of PtOx/TiO2 (Fig. 6(h)). The DFT calculations show that when hydrogen is adsorbed on the nitrogen atom, the charge is transferred from the nitrogen atom to the adjacent platinum atom. The charge redistribution in the Pt–N coordination structure leads to a reduction in the H* free energy of the activated N atom (Fig. 6(i)). Thus, the catalyst has a higher HER activity.
Support anchoring is an effective means to modulate the properties of the active phase, which is essential to obtain optimal performance in multiphase catalysis.86–88 Recently, Huang et al.89 reported for the first time heat-treated tannic acid (TA) fragments, which are stably anchored to the carbon surface to form highly heat-resistant Ptc/C catalysts (Fig. 7(a)). The TA monolayer functionalizes the nanoscale carbon surface through non-covalent interactions, thereby maintaining high electrical conductivity. This promotes the dispersion of nanoscale carbon. In addition, controlled adsorption of PtCl62− monolayer on the nanoscale carbon during impregnation. This resulted in a high transition frequency (TOF) of 154.5 A mg−1/156.1 s−1 for Ptc/C at 60 mV (Fig. 7(b) and (c)), indicating an excellent activity and high thermal stability.
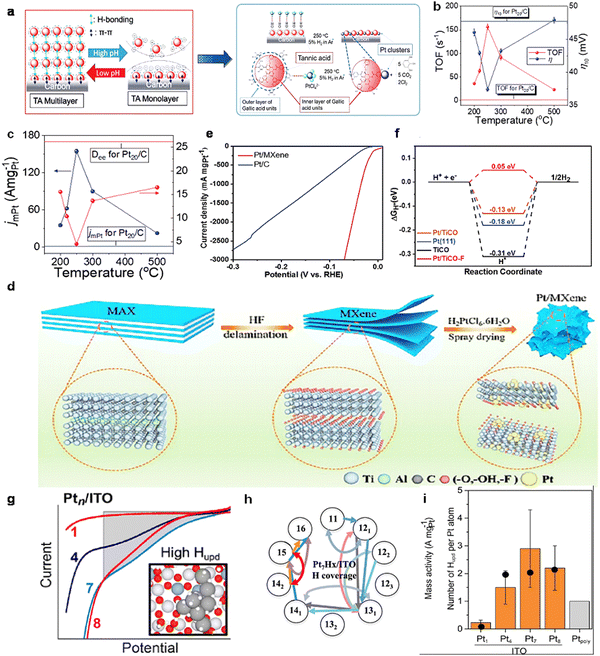 |
| Fig. 7 (a) Illustration of the TA monolayer adsorbed on carbon, the PtCl42− monolayer adsorption on TAi/C,and thermal-treated TA fragments stabilizing Ptcs anchored on the carbon. (b) TOF/η10 and (c) jmPt/Dee of Ptc(T)/C vs. thermal treatment temperature (red and blue parallel straight lines present the corresponding parameters of Pt20/C).89 Reproduced with permission. Copyright 2021, The Royal Society of Chemistry. (d) Schematic illustration for the preparation of Pt/MXene. (e) Mass activity of Pt/MXene and Pt/C. (f) The calculated free energy diagram for hydrogen evolution on Pt/TiCO, Pt (111), TiCO, and Pt/TiCO-F.90 Reproduced with permission. Copyright 2022, Wiley VCH. (g) Negative sweep of the voltammograms for 0.03 ML Ptn/ITO (n = 1, 4, 7, and 8) after subtracting the capacitive background current from ITO, ic. The subtracted currents were integrated to calculate the total charge transferred in the Hupd potential region, assumed to extend to −0.036 V. (h) Network of various elementary reactions on Pt7Hx/ITO where energetically accessible structures of the Pt7Hx cluster are connected by curved arrows representing two-step Volmer–Heyrovsky reactions, and pairs of straight arrows represent three-step Volmer–Tafel reactions. (i) Bars give the size-dependent numbers of H atoms per Pt atom adsorbed in the UPD region, estimated from the integrated Hupd currents from (i).91 Reproduced with permission. Copyright 2023, American Chemical Society. | |
In addition, three-dimensional folded Ti3C2Tx MXene containing sub-nanometer Pt clusters (Pt/MXene) was prepared by a fast continuous spray drying (Fig. 7(d)).90 The analysis revealed a strong interaction between the active component Pt and the three-dimensional folded MXene, which led to the anchoring of Pt clusters on the substrate. Even with 2.9 wt% Pt loading, the prepared Pt/MXene showed a high mass activity of 1847 mA mgPt−1 at an overpotential of 50 mV, which was much better than commercial Pt/C catalysts with a Pt loading of 20 wt% (Fig. 7(e)). DFT calculations demonstrated that the increased Pt/MXene activity could be attributed to the charge transfer from Pt clusters to MXene, which facilitated hydrogen desorption (Fig. 7(f)).
Sautet et al.91 combined density functional theory (DFT), and experiments with atom-size-selective Ptn clusters deposited on indium tin oxide (ITO) electrodes, for examining the effect of applied potential and Ptn size on the electrocatalytic HER activity of Ptn (n = 1, 4, 7 and 8) (Fig. 7(g)). The rapid increase with increasing Ptn size resulted in an approximate doubling of the activity per Pt atom for Pt7/ITO compared to atoms in the polycrystalline Pt surface layer (Fig. 7(h)). Both DFT and experiments revealed that hydrogen underpotential deposition (Hupd) resulted in the adsorption of ∼2H atoms/Pt atom by Ptn/ITO (n = 4, 7 and 8) at the HER threshold potential. This corresponds to approximately twice the Hupd of Pt blocks or nanoparticles (Fig. 7(i)).
Furthermore, in 2022, Wu et al.92 developed a solvent-free microwave synthesis method for subnanoclusters (SNCs) by designing the anchor point and reaction time (Fig. 8(a)). This method is fast, green, and most importantly, the prepared catalysts are highly loaded and have excellent stability. The SMSI was used to enable the SNCs to be firmly anchored on the support, which enhanced the stability of the catalyst. The HER catalyst Pt0.6/KB-OS5-40 was synthesized by this method with an overpotential as low as 22 mV at a current density of 10 mA cm−2 (0.5 M H2SO4), which is significantly better than that of commercial Pt/C (36 mV) (Fig. 8(b)). It is worth mentioning that after 10
000 CV cycles, the overpotential did not change significantly (Fig. 8(c)).
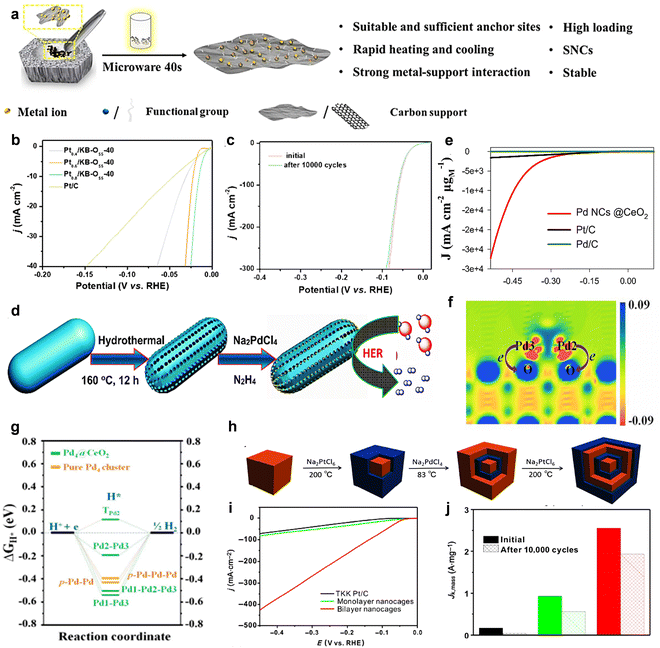 |
| Fig. 8 (a) The solvent-free microwave method, which is a scalable synthesis of high loading and stable metal SNCs. (b) LSV curves (the inset is a comparison of η10 values) of Pt0.4/KB-OS5-40, Pt0.6/KB-OS5-40, Pt0.8/KB-OS5-40 and commercial Pt/C. (c) LSV of HER of Pt0.6/KB-OS5-40 before and after 10 000 CV cycles.92 Reproduced with permission. Copyright 2022, The Royal Society of Chemistry. (d) The charge density difference Δρ of the Pd4@CeO2 model for the slice cut along the Pd2–Pd3 bond. (e) Schematic illustration of the preparation of CeO2 porous nanorods and the subsequent confinement of Pd clusters for HER. (f) Polarization curves of HER on the Pd NCs@CeO2, commercial Pt/C, and Pd/C. (g) The calculated free-energy diagram of HER for the Pd4@CeO2 and pure Pd4 cluster at the equilibrium potential.95 Reproduced with permission. Copyright 2023, American Chemical Society. (h) Schematic illustration of the transformation from cubic Pd to the final Pd–Pt tripleshelled nanocube via layer-by-layer epitaxial approach. (i) HER polarization curves of the bilayer and monolayer nanocages in comparison with a commercial Pt/C catalyst. (j) Mass activities (at −0.05 VRHE) of these three catalysts before and after durability test.96 Reproduced with permission. Copyright 2019, Springer International Publishing. | |
4.1.2 Pd-Based SNMs in acidic HER.
In addition to Pt-based nano-HER catalysts, Pd-based NMs also have excellent HER electrocatalytic properties.93,94
In recent years, Gao et al.95 successfully subnanometer-sized palladium clusters (PdNCs@CeO2) accommodated in porous cerium dioxide (Fig. 8(d)). In 0.5 M H2SO4, HER showed an onset potential at −0.036 V vs. RHE (Fig. 8(e)). DFT calculations showed that the catalyst activity was centered on the subunits of the Pd clusters, and there was a synergistic effect between the Pd and CeO2 supports in Pd NCs@CeO2 compared to the displaced Pd nanoclusters, allowing efficient electron transfer between the Pd atoms to the O atoms, facilitating H* with a suitable adsorption strength at the Pd site, which results in excellent HER activity and stability of Pd NCs@CeO2 (Fig. 8(f) and (g)).
It is worth noting that in 2019, Wang et al.96 prepared bimolecular Pd–Pt alloy nanocages catalyst by regulating various reagent components (Fig. 8(h)). This method effectively avoids the problem of spontaneous nucleation of metals. The successive formation of Pd and Pt shells, which fully expose the active sites and the existence of synergistic effects between Pd and Pt, greatly enhanced the HER performance and stability of the catalysts (Fig. 8(d)). The prepared catalysts exhibited more than twice the electrocatalytic activity compared with the monolayer nanocages. And it only decreased to 1.93 A mgPt−1 after 10
000 cycles, while the other two catalysts both had a greater loss of mass activity (Fig. 8(j)).
4.1.3 Ru-Based SNMs in acidic HER.
Recently, by a one-pot hydrothermal method, Pi et al. synthesized SNM Ru cluster HER catalysts (Ru SNC/W18O49 NWs) loaded with W18O49 nanowires (Fig. 9(a)).97 It was characterized and theoretically calculated that charge transfer between the Ru clusters and the support W18O49 would take place to make Ru positively charged, thus optimizing the binding strength of H* to Ru and ensuring excellent HER activity and durability of the catalyst (Fig. 9(b)). The catalyst overpotential is 21 mV at a current density of 10 mA cm−2, which is only 2 mV higher than that of commercial Pt/C (19 mV), and remains stable at a current of 10 mA cm−2 for 20 h continuously (Fig. 9(c) and (d)).
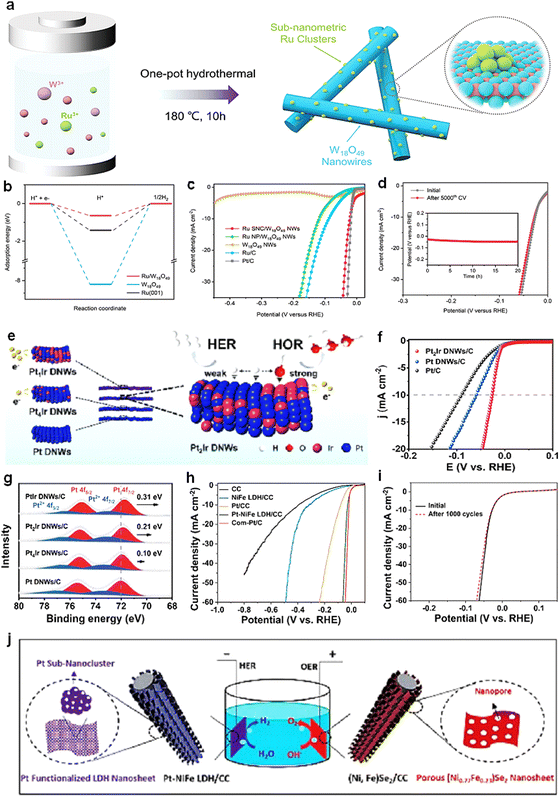 |
| Fig. 9 (a) Schematic illustration for the synthesis of Ru SNC/W18O49 NWs. (b) Comparison of the hydrogen adsorption on different surface models. (c) Polarization curves of Ru SNC/W18O49 NWs, Ru NP/W18O49 NWs, W18O49 NWs, Ru/C, and Pt/C (0.5 M H2SO4). (d) Durability test of Ru SNC/W18O49 NWs. The polarization curves were recorded before and after 5000 potential cycles from 0.1 to −0.2 V (versus RHE).97 Reproduced with permission. Copyright 2023, Wiley VCH. (e) Schematic illustration of HER/HOR for different catalysts. (f) HER polarization curves (0.1 M KOH). (g) Pt 4f XPS spectra of PtIr DNWs/C, Pt2Ir DNWs/C, Pt4Ir DNWs/C, and Pt DNWs/C.102 Reproduced with permission. Copyright 2022, The Royal Society of Chemistry. (h) LSV curves of various electrodes at a sweep rate of 1 mV s−1. (i) LSV curves of the Pt–NiFe LDH/CC electrode before and after 1000 cycles. (j) Schematic illustration of the full water splitting system assembled with the Pt–NiFe LDH/CC and (Ni0.77Fe0.23)Se2/CC electrodes.103 Reproduced with permission. Copyright 2019, The Royal Society of Chemistry. | |
4.2 Alkaline HER
Most HER is performed in alkaline electrolytes. This is because acidic media can corrode and damage equipment, which is not compatible with green and sustainable requirements.98,99 The catalyst activity in alkaline media is 2–3 orders of magnitude lower than in acidic media due to insufficient proton supply. Therefore, the development of alkaline HER catalysts with high activity is an important mission.100,101
4.2.1 Pt-Based SNMs in alkaline HER.
Recently, Wang et al.102 reported a class of sub-nanoscale Pt–Ir twisted nanowires (Pt–Ir DNWs) (Fig. 9(e)), which have excellent catalytic performance in alkaline media. The overpotential is only 26 mV even at a current density of 10 mA cm−2 (Fig. 9(f)). Analysis by X-ray photoelectron spectroscopy (XPS) showed that the binding energies of different Pt–Ir DNWs/C showed a gradual increase with increasing Ir content, indicating the transfer of electrons from Ir to Pt (Fig. 9(g)). For alkaline HER, attenuated hydrogen adsorption can accelerate hydrogen dissociation. The catalytic activity is ultimately enhanced due to the change in electronic structure of Ir and the balanced Had and OHad adsorption energies.
In addition, the design and development of a cost-effective electrolytic water electrolyzer is a major challenge. Yan et al.103 designed and developed an efficient and durable electrolytic water system for electrolysis of water in alkaline solution. The cathode material NiFe layered double hydroxide nanosheets (Pt/NixFe LDH) were prepared by hydrothermal synthesis. For the cathode material, Pt sub-nanoclusters were uniformly distributed on the NiFe LDH nanosheets. According to the analysis, Pt atoms react with Ni and Fe atoms by redox reaction of [PtCl6]2−, thus forming strong bonds with the anions of NiFe LDH nanosheets, which results in a good dispersion of Pt clusters in NiFe LDH nanosheets. It ensures sufficient exposure of Pt active sites and prevents Pt agglomeration, so that Pt-NiFe LDH/CC exhibits high activity and stability.
4.2.2 Ru-Based SNMs in alkaline HER.
Ru is expected to replace Pt material in alkaline HER as a highly promising HER catalyst material.104–106 To explore the size-activity relationship at sub-nanometer size of catalysts, in 2022, Hu et al.107 synthesized a series of ruthenium nanocrystals (Fig. 10(a)), ranging from single atoms and sub-nanoclusters to larger nanoparticles, aiming to investigate the size-dependent activity of HER in alkaline media. DFT calculations revealed that Ru19 clusters are highly active for water dissociation with an energy barrier of only 0.03 eV (Fig. 10(b)). This accelerates the production of H* and speeds up the reaction kinetics of alkaline HER. The particle size of Ru shows a negative correlation trend with the d energy band center energy level, and subnanoclusters of Ru with d-energy band centers closer to the Femi energy level exhibit a stronger water dissociation ability. Therefore, its HER activity is superior to that of single atoms and larger nanoparticles. Comparing the HER activity of Ru-1.0, Ru-2.3, and Ru-3.1, it was learned that Ru-1.0 overpotential was as low as 13 mV at 10 mA cm−2 generation, which is consistent with the above findings, and the subnanometer Ru-clusters also showed excellent HER durability in 100 h stability test (Fig. 10(c) and (d)).
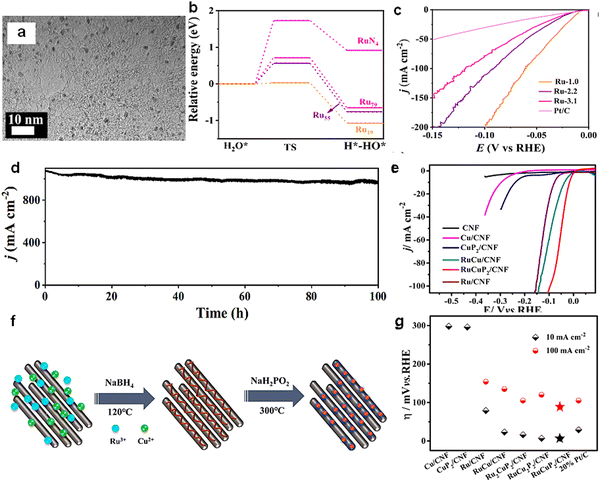 |
| Fig. 10 (a) TEM images of Ru crystals in size 1.0 nm. (b) Kinetic barriers of water dissociation for different Ru cluster models. (c) LSV curves of Ru-1.0, Ru-2.3, Ru-3.1, and commercial Pt/C for HER in 1 M KOH. (d) Stability test of Ru-1.0 at the current density of 1000 mA cm−2 for 100 h.107 Reproduced with permission. Copyright 2022, Springer International Publishing. (e) LSV curves of Ru-1.0, Ru-2.3, Ru-3.1, and commercial Pt/C for HER in 1 M KOH. (f) The formation process of RuxCuyP2/CNF. (g) Comparison of overpotential at current density of 10 mA cm−2 and 100 mA cm−2.108 Reproduced with permission. Copyright 2021, Elsevier. | |
Bimetallic phosphide materials have received much attention due to their potentially excellent HER properties. In 2021, Yang et al.108 prepared sub-nanometer Cu and Ru bimetallic phosphide nanoclusters (RuxCuyP2) in graphitized carbon nanofibers (CNF) by introducing uniformly dispersed Ru (Fig. 10(f)). The comparative analysis of CNF anchored on different metal clusters showed that the doping of Ru significantly enhanced the HER activity. And the RuCuP2/CNF catalysts showed the best HER performance (Fig. 10(e)). The overpotential was only 10 mV and 88 mV at current densities of 10 mA cm−2 and 100 mA cm−2 (Fig. 10(g)).
4.3 Both acidic and alkaline HER
During the electrolysis of water, hydrogen is continuously produced and the concentration of protons in the electrolyte is constantly changing.109 Therefore, there is a great trend to design and prepare HER catalysts with wide pH range.110,111
4.3.1 Pt-Based SNMs in acidic and alkaline HER.
An ultrafine (∼1.9 nm) trimetallic nanocatalyst (PtRhNi) was developed using the advantage of synergistic effects, using NiOx as the support and composed of high-density PtRh nanoalloys.112 It was found that the addition of Ni increased the catalyst electrochemical active sites (Fig. 11(a)), Rh in PtRh nanoalloys could change the Pt d-band electronic structure. Importantly, the Rh and Pt sites synergistically promoted the proton adsorption and desorption steps in HER (Fig. 11(b)). The trimetallic synergy allows PtRhNi to exhibit excellent HER performance in acidic (0.5 M H2SO4) and alkaline (1.0 M KOH) media, with an overpotential of only 28 mV for both even at a cathodic current density of 10 mA cm−2 (Fig. 11(c) and (d)).
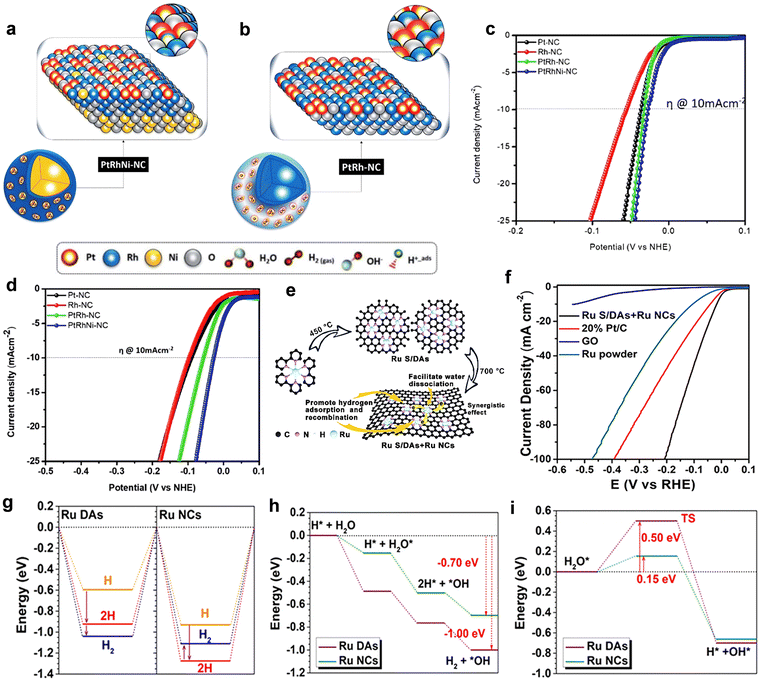 |
| Fig. 11 Schematic representation for the nanostructure of (a) PtRh and (b) PtRhNi NCs. (c) LSV of the experimental NCs (0.5 M H2SO4). (d) LSV of the experimental NCs (1 M KOH).112 Reproduced with permission. Copyright 2021, The Royal Society of Chemistry. (e) The enhanced mechanism of Ru S/DAs + Ru NCs hybrid nanocatalysts for HER. (f) Polarization curves of Ru S/DAs + Ru NCs, GO, commercial Ru powder and commercial Pt/C. (g) H adsorption energies in Ru DAs and Ru NCs. (h) Energetic diagram for the HER of Ru DAs and Ru NCs in an alkaline environment. (i) The calculated energy barrier for water dissociation in Ru DAs and Ru NCs.113 Reproduced with permission. Copyright 2021, Wiley VCH. | |
4.3.2 Ru-Based SNMs in acidic and alkaline HER.
In 2021, Wang et al.113 synthesized hybrid HER catalysts of Ru single/dual atoms (Ru S/DAs) supported by carbon dots with Ru nanoclusters (Ru NCs) (Fig. 11(e)). The team demonstrated for the first time that Ru S/DAs + Ru NCs exhibit better HER performance than commercial Pt/C catalysts. At a current density of 10 mA cm−2, the overpotential was 15 mV and 40 mV in alkaline (1 M KOH) and acidic (0.5 M H2SO4), respectively, exhibiting excellent HER performance (Fig. 11(f)). This is due to the synergistic interaction between Ru S/DAs and Ru NCs. DFT calculations showed that charge rearrangement occurred in single-atom Ru, Ru double-site promoted the adsorption and complexation of active hydrogen (Fig. 11(g) and (h)). In KOH solution, the HER performance was significantly enhanced due to the increased efficiency of water dissociation (Fig. 11(i)).
5. Conclusion and perspectives
Hydrogen energy will be one of the most desirable energy sources in the future, and the development of high-performance and long-lived catalysts is the key to achieve hydrogen energy substitution for fossil fuels. This paper reviews the recent advances in high-performance noble metal SNMs for HER, focusing on the design synthesis strategies, performance optimization methods, and applications. The activity of SNMs catalysts in HER is listed in Table 1. Overall, the superiority of sub-nano HER catalysts can be attributed to the almost complete exposure of atoms and multiple active sites. The above properties make the synergistic inter-component interaction, doping, defects and other strategies particularly obvious and important for the performance enhancement. While sub-nanomaterials have advantages in the field of HER, they also face many challenges. In order to have a better future of sub-nanomaterials in the field of HER, we need to consider the following challenges reasonably.
Table 1 Comparison of electrocatalytic HER performances of SNMs-based catalysts
Metal SNMs |
Electrolyte |
Current density (mA cm−2) |
Overpotential (mV) |
Ref. |
Pt4Zr2 |
0.05 M H2SO4 |
— |
— |
67
|
NiCoFePtRh |
0.5 M H2SO4 |
10 |
27 |
68
|
1.2% PtCo/NPC |
0.5 M H2SO4 |
10 |
14 |
69
|
Pt5/HMCS |
0.5 M H2SO4/1.0 M KOH |
10 |
20.7/46.2 |
73
|
1% Pd–CeO2−x-NC |
1 M KOH |
10 |
115 |
74
|
C-ZIF-CuPt |
0.1 M KOH |
10 |
46 |
77
|
Pt/NixFe LDHs |
1 M KOH |
10 |
240 |
80
|
PtNx/TiO2 |
0.5 M H2SO4 |
10 |
67 |
85
|
Ptc(250)/C |
0.5 M H2SO4 |
10 |
37.4 |
89
|
Pt/MXene |
0.5 M H2SO4 |
10 |
34 |
90
|
Ptn/ITO |
0.1 M HClO4 |
— |
— |
91
|
Pt0.6/KB-OS5-40 |
0.5 M H2SO4 |
10 |
22 |
92
|
Pd NCs@CeO2 |
0.5 M H2SO4 |
— |
— |
95
|
Pd–Pt nanocages |
0.5 M H2SO4 |
10 |
46 |
96
|
Ru SNC/W18O49 NWs |
0.5 M H2SO4 |
10 |
21 |
97
|
Pt2Ir DNWs/C |
0.1 M KOH |
10 |
26 |
102
|
3D Pt–NiFe LDH/CC |
1.0 M KOH |
10 |
28 |
103
|
Ru-1.0 |
1 M KOH |
10/100 |
13/60 |
107
|
RuCuP2/CNF |
1.0 M NaOH |
10/100 |
10/88 |
108
|
PtRhNi-NC |
0.5 M H2SO4/1.0 M KOH |
10 |
28/28 |
112
|
Ru S/DAs + Ru NCs |
0.5 M H2SO4/1.0 M KOH |
10 |
40/15 |
113
|
1. Although a large number of studies have shown that sub-nano HER electrocatalysts have high catalytic activity, only the performance tolerance of the catalysts under operating conditions has been discussed. Therefore, in order to meet the demand of high current and long time uninterrupted operation work in practical applications, our related researchers should next work on optimizing the activity and stability of sub-nano HER catalysts, and show the direction for developing sub-nano HER catalysts with environmental adaptability and long-term stability.114–116
2. In the practical application of SNMs, the bulk synthesis of catalysts is also a tricky challenge we face. Although the currently developed SNMs have shown excellent reactivity in HER, most of the catalyst synthesis processes are still limited to the laboratory level, therefore, the development of SNMs with high cost performance and mass production is our primary challenge.117–121
3. Establishing a unified test and evaluation standard to compare the performance of different groups of SNMs. It is difficult to avoid ambiguous or inaccurate information about catalysts due to the differences in various conditions such as preparation methods, composition and structure of HER catalysts, and the lack of details provided in the discussion of catalytic performance.122,123 Therefore, in future studies, researchers are encouraged to be able to establish uniform test evaluation criteria, which will provide a strong basis for determining and comparing the activity of newly developed electrocatalysts in the future.
In conclusion, we believe that SNMs are a very promising and challenging direction and strategy for the design of efficient HER catalysts for synthesis. We hope that this review will inform the further development of SNMs in the field of electrocatalysis.
Author contributions
L. W. and J. L. supervised the research. J. L. conceived the review. Y. Y. and R. L. completed the references and wrote the manuscript. Y. S. checked and revised the manuscript. X. S. and Z. L. helped answer some questions. All authors discussed the results and commented on the manuscript.
Conflicts of interest
There are no conflicts to declare.
Acknowledgements
This work was supported by the National Natural Science Foundation of China (52272222, 52072197), Outstanding Youth Foundation of Shandong Province, China (ZR2019JQ14), Youth Innovation and Technology Foundation of Shandong Higher Education Institutions, China (2019KJC004), the Natural Science Foundation of Shandong Province, China (ZR2021MB061), Major Scientific and Technological Innovation Project (2019JZZY020405), Taishan Scholar Young Talent Program (tsqn201909114), Major Basic Research Program of Natural Science Foundation of Shandong Province under Grant (ZR2020ZD09).
Notes and references
- M. Zhong, K. Tran, Y. Min, C. Wang, Z. Wang, C. T. Dinh, P. De Luna, Z. Yu, A. S. Rasouli, P. Brodersen, S. Sun, O. Voznyy, C. S. Tan, M. Askerka, F. Che, M. Liu, A. Seifitokaldani, Y. Pang, S. C. Lo, A. Ip, Z. Ulissi and E. H. Sargent, Accelerated discovery of CO2 electrocatalysts using active machine learning, Nature, 2020, 581, 178–183 CrossRef CAS PubMed
.
- Z. Zhang, Y. Zheng, L. Qian, D. Luo, H. Dou, G. Wen, A. Yu and Z. Chen, Emerging trends in sustainable CO2-management materials, Adv. Mater., 2022, 34, e2201547 CrossRef
.
- G. Chen, R. Lu, C. Li, J. Yu, X. Li, L. Ni, Q. Zhang, G. Zhu, S. Liu, J. Zhang, U. I. Kramm, Y. Zhao, G. Wu, J. Xie and X. Feng, Hierarchically porous carbons with highly curved surfaces for hosting single metal FeN4 sites as outstanding oxygen reduction catalysts, Adv. Mater., 2023, 35, 2300907 CrossRef CAS PubMed
.
- T. Asset and P. Atanassov, Iron-nitrogen-carbon catalysts for proton exchange membrane fuel cells, Joule, 2020, 4, 33–44 CrossRef CAS
.
- C. Lin, Y. Liu, X. Kong, Z. Geng and J. Zeng, Electrodeposited highly-oriented bismuth microparticles for efficient CO2 electroreduction into formate, Nano Res., 2022, 15, 10078–10083 CrossRef CAS
.
- G. Glenk and S. Reichelstein, Economics of converting renewable power to hydrogen, Nat. Energy, 2019, 4, 216–222 CrossRef CAS
.
- H. Li, Y. Han, H. Zhao, W. Qi, D. Zhang, Y. Yu, W. Cai, S. Li, J. Lai, B. Huang and L. Wang, Fast site-to-site electron transfer of high-entropy alloy nanocatalyst driving redox electrocatalysis, Nat. Commun., 2020, 11, 5437 CrossRef CAS PubMed
.
- H. Yin, Y. Dou, S. Chen, Z. Zhu, P. Liu and H. Zhao, 2D electrocatalysts for converting earth-abundant simple molecules into value-added commodity chemicals: Recent progress and perspectives, Adv. Mater., 2020, 32, e1904870 CrossRef
.
- Z. W. Seh, J. Kibsgaard, C. F. Dickens, I. Chorkendorff, J. K. Norskov and T. F. Jaramillo, Combining theory and experiment in electrocatalysis: Insights into materials design, Science, 2017, 355, eaad4998 CrossRef PubMed
.
- Z. Song, K. Wang, Q. Sun, L. Zhang, J. Li, D. Li, P.-W. Sze, Y. Liang, X. Sun, X.-Z. Fu and J.-L. Luo, High-performance ammonium cobalt phosphate nanosheet electrocatalyst for alkaline saline water oxidation, Adv. Sci., 2021, 8, 2100498 CrossRef CAS
.
- L. Yu, Q. Zhu, S. Song, B. McElhenny, D. Wang, C. Wu, Z. Qin, J. Bao, Y. Yu, S. Chen and Z. Ren, Non-noble metal-nitride based electrocatalysts for high-performance alkaline seawater electrolysis, Nat. Commun., 2019, 10, 5106 CrossRef PubMed
.
- X. Wu, S. Zhou, Z. Wang, J. Liu, W. Pei, P. Yang, J. Zhao and J. Qiu, Engineering multifunctional collaborative catalytic interface enabling efficient hydrogen evolution in all pH range and seawater, Adv. Energy Mater., 2019, 9, 1901333 CrossRef
.
- J. Lin, P. Wang, H. Wang, C. Li, X. Si, J. Qi, J. Cao, Z. Zhong, W. Fei and J. Feng, Defect-rich heterogeneous MoS2/nis2 nanosheets electrocatalysts for efficient overall water splitting, Adv. Sci., 2019, 6, 1900246 CrossRef
.
- Y. Zheng, Y. Jiao, A. Vasileff and S. Z. Qiao, The hydrogen evolution reaction in alkaline solution: From theory, single crystal models, to practical electrocatalysts, Angew. Chem., Int. Ed., 2018, 57, 7568–7579 CrossRef CAS
.
- Y.-Y. Ma, C.-X. Wu, X.-J. Feng, H.-Q. Tan, L.-K. Yan, Y. Liu, Z. Kang, E. Wang and Y.-G. Li, High efficient hydrogen evolution from seawater by a low-cost and stable CoMoP@C electrocatalyst superior to Pt/C, Energy Environ. Sci., 2017, 10, 788–798 RSC
.
- G. Zhao, K. Rui, S. X. Dou and W. Sun, Heterostructures for electrochemical hydrogen evolution reaction: A review, Adv. Funct. Mater., 2018, 28, 1803291 CrossRef
.
- C. Wang, M. Zhu, Z. Cao, P. Zhu, Y. Cao, X. Xu, C. Xu and Z. Yin, Heterogeneous bimetallic sulfides based seawater electrolysis towards stable industrial-level large current density, Appl. Catal., B, 2021, 291, 120071 CrossRef CAS
.
- W. Tong, M. Forster, F. Dionigi, S. Dresp, R. Sadeghi Erami, P. Strasser, A. J. Cowan and P. Farràs, Electrolysis of low-grade and saline surface water, Nat. Energy, 2020, 5, 367–377 CrossRef CAS
.
- S. H. Hsu, J. Miao, L. Zhang, J. Gao, H. Wang, H. Tao, S. F. Hung, A. Vasileff, S. Z. Qiao and B. Liu, An earth-abundant catalyst-based seawater photoelectrolysis system with 17.9% solar-to-hydrogen efficiency, Adv. Mater., 2018, 30, e1707261 CrossRef PubMed
.
- H. Jin, M. Ha, M. G. Kim, J. H. Lee and K. S. Kim, Engineering Pt coordination environment with atomically dispersed transition metal sites toward superior hydrogen evolution, Adv. Energy Mater., 2023, 13, 2204213 CrossRef CAS
.
- S. M. Younan, Z. Li, X. Yan, D. He, W. Hu, N. Demetrashvili, G. Trulson, A. Washington, X. Xiao, X. Pan, J. Huang and J. Gu, Zinc single atom confinement effects on catalysis in 1T-phase molybdenum disulfide, ACS Nano, 2023, 17, 1414–1426 CrossRef CAS
.
- F. Li, D. H. Kweon, G.-F. Han, H.-J. Noh, W. Che, I. Ahmad, H. Y. Jeong, Z. Fu, Y. Lu and J.-B. Baek, Merging platinum single atoms to achieve ultrahigh mass activity and low hydrogen production cost, ACS Nano, 2023, 17, 2923–2931 CrossRef CAS
.
- J. T. Bender, A. S. Petersen, F. C. Østergaard, M. A. Wood, S. M. J. Heffernan, D. J. Milliron, J. Rossmeisl and J. Resasco, Understanding cation effects on the hydrogen evolution reaction, ACS Energy Lett., 2023, 8, 657–665 CrossRef CAS
.
- W. Yu, H. Huang, Y. Qin, D. Zhang, Y. Zhang, K. Liu, Y. Zhang, J. Lai and L. Wang, The synergistic effect of Pyrrolic-N and Pyridinic-N with Pt under strong metal-support interaction to achieve high-performance alkaline hydrogen evolution, Adv. Energy Mater., 2022, 12, 2200110 CrossRef CAS
.
- X. Wu, Z. Wang, D. Zhang, Y. Qin, M. Wang, Y. Han, T. Zhan, B. Yang, S. Li, J. Lai and L. Wang, Solvent-free microwave synthesis of ultra-small Ru-Mo2C@CNT with strong metal-support interaction for industrial hydrogen evolution, Nat. Commun., 2021, 12, 4018 CrossRef CAS PubMed
.
- M. Jin, X. Zhang, S. Niu, Q. Wang, R. Huang, R. Ling, J. Huang, R. Shi, A. Amini and C. Cheng, Strategies for designing high-performance hydrogen evolution reaction electrocatalysts at large current densities above 1000 mA cm−2, ACS Nano, 2022, 16, 11577–11597 CrossRef CAS PubMed
.
- Z. Lu, M. Sun, T. Xu, Y. Li, W. Xu, Z. Chang, Y. Ding, X. Sun and L. Jiang, Superaerophobic electrodes for direct hydrazine fuel cells, Adv. Mater., 2015, 27, 2361–2366 CrossRef CAS PubMed
.
- Y. Zhao, J. Yan, J. Yu and B. Ding, Advances in nanofibrous materials for stable lithium-metal anodes, ACS Nano, 2022, 16, 17891–17910 CrossRef CAS PubMed
.
- T. Yang, X. Li, Y. Guo, J. Zhao and Y. Qu, Preparation of nanocellulose crystal from bleached pulp with an engineering cellulase and co-production of ethanol, Carbohydr. Polym., 2023, 301, 120291 CrossRef CAS PubMed
.
- L. Wang, H. Liu, J. Zhuang and D. Wang, Small-scale big science: From nano-to atomically dispersed catalytic materials, Small Sci., 2022, 2, 2200036 CrossRef CAS
.
- C. Liu, S. Wang, N. Wang, J. Yu, Y. T. Liu and B. Ding, From 1D nanofibers to 3D nanofibrous aerogels: A marvellous evolution of electrospun SiO2 nanofibers for emerging applications, Nano-Micro Lett., 2022, 14, 194 CrossRef CAS PubMed
.
- M. Zhao, Z. Chen, Z. Lyu, Z. D. Hood, M. Xie, M. Vara, M. Chi and Y. Xia, Ru octahedral nanocrystals with a face-centered cubic structure, 111 facets, thermal stability up to 400 °C, and enhanced catalytic activity, J. Am. Chem. Soc., 2019, 141, 7028–7036 CrossRef CAS PubMed
.
- I. Rezic, Nanoparticles for biomedical application and their synthesis, Polymers, 2022, 14, 4961 CrossRef CAS PubMed
.
- Q. Lu and X. Wang, Recent progress of sub-nanometric materials in photothermal energy conversion, Adv. Sci., 2022, 9, e2104225 CrossRef
.
- B. Ni, Y. Shi and X. Wang, The sub-nanometer scale as a new focus in nanoscience, Adv. Mater., 2018, 30, e1802031 CrossRef PubMed
.
- B. Ni and X. Wang, Chemistry and properties at a sub-nanometer scale, Chem. Sci., 2016, 7, 3978–3991 RSC
.
- J. Niu, J. Liang, A. Gao, M. Dou, Z. Zhang, X. Lu and F. Wang, Micropore-confined amorphous SnO2 subnanoclusters as robust anode materials for Na-ion capacitors, J. Mater. Chem. A, 2019, 7, 21711–21721 RSC
.
- Y. Yang, L. Zhao, X. Gao and Y. Zhao, Constructing ultrafine monodispersed Co2P/(0.59-Cu3P) on Cu doped CoZn-ZIF derived porous N-doped carbon for highly efficient dehydrogenation of ammonia borane, Nano Res., 2023, 16, 6687–6700 CrossRef CAS
.
- J. Ding, H. Yang, S. Zhang, Q. Liu, H. Cao, J. Luo and X. Liu, Advances in the electrocatalytic hydrogen evolution reaction by metal nanoclusters-based materials, Small, 2022, 18, e2204524 CrossRef PubMed
.
- J. Wang, H. Zhang and X. Wang, Recent methods for the synthesis of noble-metal-free hydrogen-evolution electrocatalysts: From nanoscale to sub-nanoscale, Small Methods, 2017, 1, 1700118 CrossRef
.
- H. Li, J. Lai, Z. Li and L. Wang, Multi-sites electrocatalysis in high-entropy alloys, Adv. Funct. Mater., 2021, 31, 2106715 CrossRef CAS
.
- J. Zhu, L. Hu, P. Zhao, L. Y. S. Lee and K. Y. Wong, Recent advances in electrocatalytic hydrogen evolution using nanoparticles, Chem. Rev., 2020, 120, 851–918 CrossRef CAS PubMed
.
- T. Tang, Z. Wang and J. Guan, A review of defect engineering in two-dimensional materials for electrocatalytic hydrogen evolution reaction, Chin. J. Catal., 2022, 43, 636–678 CrossRef CAS
.
- B. Zhou, R. Gao, J. J. Zou and H. Yang, Surface design strategy of catalysts for water electrolysis, Small, 2022, 18, e2202336 CrossRef PubMed
.
- Y. Li, H. Wang, L. Xie, Y. Liang, G. Hong and H. Dai, MoS2 nanoparticles grown on graphene: An advanced catalyst for the hydrogen evolution reaction, J. Am. Chem. Soc., 2011, 133, 7296–7299 CrossRef CAS PubMed
.
- J. Hou, Y. Wu, B. Zhang, S. Cao, Z. Li and L. Sun, Rational design of nanoarray architectures for electrocatalytic water splitting, Adv. Funct. Mater., 2019, 29, 1808367 CrossRef
.
- H. Chen, X. Ai, W. Liu, Z. Xie, W. Feng, W. Chen and X. Zou, Promoting subordinate, efficient ruthenium sites with interstitial silicon for Pt-like electrocatalytic activity, Angew. Chem., Int. Ed., 2019, 58, 11409–11413 CrossRef CAS PubMed
.
- Y. Yang, Y. Yu, J. Li, Q. Chen, Y. Du, P. Rao, R. Li, C. Jia, Z. Kang, P. Deng, Y. Shen and X. Tian, Engineering ruthenium-based electrocatalysts for effective hydrogen evolution reaction, Nano-Micro Lett., 2021, 13, 160 CrossRef CAS PubMed
.
- M. Lao, P. Li, Y. Jiang, H. Pan, S. X. Dou and W. Sun, From fundamentals and theories to heterostructured electrocatalyst design: An in-depth understanding of alkaline hydrogen evolution reaction, Nano Energy, 2022, 98, 107231 CrossRef CAS
.
- T. F. Jaramillo, K. P. Jørgensen, J. Bonde, J. H. Nielsen, S. Horch and I. Chorkendorff, Identification of active edge sites for electrochemical H2 evolution from MoS2 nanocatalysts, Science, 2007, 317, 100–102 CrossRef CAS PubMed
.
- Y. Chen, G. Yu, W. Chen, Y. Liu, G. D. Li, P. Zhu, Q. Tao, Q. Li, J. Liu, X. Shen, H. Li, X. Huang, D. Wang, T. Asefa and X. Zou, Highly active, nonprecious electrocatalyst comprising borophene subunits for the hydrogen evolution reaction, J. Am. Chem. Soc., 2017, 139, 12370–12373 CrossRef CAS
.
- S. Hu and W. X. Li, Sabatier principle of metal-support interaction for design of ultrastable metal nanocatalysts, Science, 2021, 374, 1360–1365 CrossRef CAS PubMed
.
- E. Skúlason, V. Tripkovic, M. E. Bjorketun, S. Gudmundsdottir, G. Karlberg, J. Rossmeisl, T. Bligaard, H. Jonsson and J. K. Nørskov, Modeling the electrochemical hydrogen oxidation and evolution reactions on the basis of density functional theory calculations, J. Phys. Chem. C, 2010, 114, 18182–18197 CrossRef
.
- Y. Guo, T. Park, J. W. Yi, J. Henzie, J. Kim, Z. Wang, B. Jiang, Y. Bando, Y. Sugahara, J. Tang and Y. Yamauchi, Nanoarchitectonics for transition-metal-sulfide-based electrocatalysts for water splitting, Adv. Mater., 2019, 31, e1807134 CrossRef
.
- J. Wang, X. Yue, Y. Yang, S. Sirisomboonchai, P. Wang, X. Ma, A. Abudula and G. Guan, Earth-abundant transition-metal-based bifunctional catalysts for overall electrochemical water splitting: A review, J. Alloys Compd., 2020, 819, 153346 CrossRef CAS
.
- Y. Shi and B. Zhang, Correction: Recent advances in transition metal phosphide nanomaterials: Synthesis and applications in hydrogen evolution reaction, Chem. Soc. Rev., 2016, 45, 1781 RSC
.
- H. Vrubel, T. Moehl, M. Gratzel and X. Hu, Revealing and accelerating slow electron transport in amorphous molybdenum sulphide particles for hydrogen evolution reaction, Chem. Commun., 2013, 49, 8985–8987 RSC
.
- Y. Li, Y. Sun, Y. Qin, W. Zhang, L. Wang, M. Luo, H. Yang and S. Guo, Recent advances on water-splitting electrocatalysis mediated by noble-metal-based nanostructured materials, Adv. Energy Mater., 2020, 10, 1903120 CrossRef CAS
.
- Z. Chen, H. Qing, K. Zhou, D. Sun and R. Wu, Metal-organic framework-derived nanocomposites for electrocatalytic hydrogen evolution reaction, Prog. Mater. Sci., 2020, 108, 100618 CrossRef CAS
.
- T. Naresh Kumar, S. Sivabalan, N. Chandrasekaran and K. L. Phani, Synergism between polyurethane and polydopamine in the synthesis of Ni–Fe alloy monoliths, Chem. Commun., 2015, 51, 1922–1925 RSC
.
- S. X. Guo, Y. Liu, A. M. Bond, J. Zhang, P. Esakki Karthik, I. Maheshwaran, S. Senthil Kumar and K. L. Phani, Facile electrochemical co-deposition of a graphene-cobalt nanocomposite for highly efficient water oxidation in alkaline media: Direct detection of underlying electron transfer reactions under catalytic turnover conditions, Phys. Chem. Chem. Phys., 2014, 16, 19035–19045 RSC
.
- P. E. Karthik, K. A. Raja, S. S. Kumar, K. L. N. Phani, Y. Liu, S.-X. Guo, J. Zhang and A. M. Bond, Electroless deposition of iridium oxide nanoparticles promoted by condensation of [Ir(OH)6]2− on an anodized Au surface: Application to electrocatalysis of the oxygen evolution reaction, RSC Adv., 2015, 5, 3196–3199 RSC
.
- M. R. Gao, J. X. Liang, Y. R. Zheng, Y. F. Xu, J. Jiang, Q. Gao, J. Li and S. H. Yu, An efficient molybdenum disulfide/cobalt diselenide hybrid catalyst for electrochemical hydrogen generation, Nat. Commun., 2015, 6, 5982 CrossRef CAS PubMed
.
- X. Sun, X. Gao, J. Chen, X. Wang, H. Chang, B. Li, D. Song, J. Li, H. Li and N. Wang, Ultrasmall Ru nanoparticles highly dispersed on sulfur-doped graphene for HER with high electrocatalytic performance, ACS Appl. Mater. Interfaces, 2020, 12, 48591–48597 CrossRef CAS
.
- S. Anantharaj, M. Jayachandran and S. Kundu, Unprotected and interconnected Ru0 nano-chain networks: Advantages of unprotected surfaces in catalysis and electrocatalysis, Chem. Sci., 2016, 7, 3188–3205 RSC
.
- J. Zhang, Z. Gao, S. Wang, G. Wang, X. Gao, B. Zhang, S. Xing, S. Zhao and Y. Qin, Origin of synergistic effects in bicomponent cobalt oxide-platinum catalysts for selective hydrogenation reaction, Nat. Commun., 2019, 10, 4166 CrossRef PubMed
.
- Q. Zou, Y. Akada, A. Kuzume, M. Yoshida, T. Imaoka and Y. Kimihisa, Alloying at a subnanoscale maximizes the synergistic effect on the electrocatalytic hydrogen evolution, Angew. Chem., Int. Ed., 2022, 61, e202209675 CrossRef CAS PubMed
.
- G. Feng, F. Ning, J. Song, H. Shang, K. Zhang, Z. Ding, P. Gao, W. Chu and D. Xia, Sub-2 nm ultrasmall high-entropy alloy nanoparticles for extremely superior electrocatalytic hydrogen evolution, J. Am. Chem. Soc., 2021, 143, 17117–17127 CrossRef CAS
.
- F. Guo, Z. Zou, Z. Zhang, T. Zeng, Y. Tan, R. Chen, W. Wu, N. Cheng and X. Sun, Confined sub-nanometer PtCo clusters as a highly efficient and robust electrocatalyst for the hydrogen evolution reaction, J. Mater. Chem. A, 2021, 9, 5468–5474 RSC
.
- H. Xiong, D. Kunwar, D. Jiang, C. E. García-Vargas, H. Li, C. Du, G. Canning, X. I. Pereira-Hernandez, Q. Wan, S. Lin, S. C. Purdy, J. T. Miller, K. Leung, S. S. Chou, H. H. Brongersma, R. ter Veen, J. Huang, H. Guo, Y. Wang and A. K. Datye, Engineering catalyst supports to stabilize PdOx two-dimensional rafts for water-tolerant methane oxidation, Nat. Catal., 2021, 4, 830–839 CrossRef CAS
.
- Y. Wang, Z. Li, X. Zheng, R. Wu, J. Song, Y. Chen, X. Cao, Y. Wang and Y. Nie, Renovating phase constitution and construction of Pt nanocubes for electrocatalysis of methanol oxidation via a solvothermal-induced strong metal-support interaction, Appl. Catal., B, 2023, 325, 122383 CrossRef CAS
.
- Z. Luo, G. Zhao, H. Pan and W. Sun, Strong metal–support interaction in heterogeneous catalysts, Adv. Energy Mater., 2022, 12, 2201395 CrossRef CAS
.
- X. K. Wan, H. B. Wu, B. Y. Guan, D. Luan and X. W. D. Lou, Confining sub-nanometer Pt clusters in hollow mesoporous carbon spheres for boosting hydrogen evolution activity, Adv. Mater., 2020, 32, e1901349 CrossRef
.
- Y. Yu, Z. Dong, L. Tan, N. He, R. Tang, J. Fang and H. Chen, Enhanced hydrogen evolution reaction in alkaline solution by constructing strong metal-support interaction on Pd–CeO2−x–NC hybrids, J. Colloid Interface Sci., 2022, 611, 554–563 CrossRef CAS PubMed
.
- A. Zhang, Y. Liang, H. Zhang, Z. Geng and J. Zeng, Doping regulation in transition metal compounds for electrocatalysis, Chem. Soc. Rev., 2021, 50, 9817–9844 RSC
.
- W. Peng, A. Deshmukh, N. Chen, Z. Lv, S. Zhao, J. Li, B. Yan, X. Gao, L. Shang, Y. Gong, L. Wu, M. Chen, T. Zhang and H. Gou, Deciphering the dynamic structure evolution of Fe- and Ni-Codoped CoS2 for enhanced water oxidation, ACS Catal., 2022, 12, 3743–3751 CrossRef CAS
.
- C. Wang, L. Kuai, W. Cao, H. Singh, A. Zakharov, Y. Niu, H. Sun and B. Geng, Highly dispersed Cu atoms in MOF-derived N-doped porous carbon inducing Pt loads for superior oxygen reduction and hydrogen evolution, Chem. Eng. J., 2021, 426, 130749 CrossRef CAS
.
- X. Zheng, P. Li, S. Dou, W. Sun, H. Pan, D. Wang and Y. Li, Non-carbon-supported single-atom site catalysts for electrocatalysis, Energy Environ. Sci., 2021, 14, 2809–2858 RSC
.
- P. Wang and B. Wang, Designing self-supported electrocatalysts for electrochemical water splitting: Surface/interface engineering toward enhanced electrocatalytic performance, ACS Appl. Mater. Interfaces, 2021, 13, 59593–59617 CrossRef CAS PubMed
.
- X. Yu, J. Guo, B. Li, J. Xu, P. Gao, K. S. Hui, K. N. Hui and H. Shao, Sub-nanometer Pt clusters on defective NiFe LDH nanosheets as trifunctional electrocatalysts for water splitting and rechargeable hybrid sodium-air batteries, ACS Appl. Mater. Interfaces, 2021, 13, 26891–26903 CrossRef CAS
.
- N. Nie, D. Zhang, Z. Wang, S. Ge, Y. Gu, B. Yang, J. Lai and L. Wang, Stable p-block metals electronic perturbation in PtM@CNT (M = Ga, In, Pb and Bi) for acidic seawater hydrogen production at commercial current densities, Appl. Catal., B, 2023, 322, 122100 CrossRef CAS
.
- H. Yang, Y. Ji, Q. Shao, W. Zhu, M. Fang, M. Ma, F. Liao, H. Huang, Y. Zhang, J. Yang, Z. Fan, Y. Li, Y. Liu, M. Shao and Z. Kang, Metastable-phase platinum oxide for clarifying the Pt–O active site for the hydrogen evolution reaction, Energy Environ. Sci., 2023, 16, 574–583 RSC
.
- Z. Wu, P. Yang, Q. Li, W. Xiao, Z. Li, G. Xu, F. Liu, B. Jia, T. Ma, S. Feng and L. Wang, Microwave synthesis of Pt clusters on black TiO2 with abundant oxygen vacancies for efficient acidic electrocatalytic hydrogen evolution, Angew. Chem., Int. Ed., 2023, 62, e202300406 CrossRef CAS
.
- Z. Li, K. Wang, X. Tan, X. Liu, G. Wang, G. Xie and L. Jiang, Defect-enriched multistage skeleton morphology Ni–Fe–P–Ni3S2 heterogeneous catalyst on Ni foam for efficient overall water splitting, Chem. Eng. J., 2021, 424, 130390 CrossRef CAS
.
- X. Cheng, Y. Lu, L. Zheng, Y. Cui, M. Niibe, T. Tokushima, H. Li, Y. Zhang, G. Chen, S. Sun and J. Zhang, Charge redistribution within platinum–nitrogen coordination structure to boost hydrogen evolution, Nano Energy, 2020, 73, 104739 CrossRef CAS
.
- M. Zhang, C. Lai, F. Xu, D. Huang, S. Liu, Y. Fu, L. Li, H. Yi, L. Qin and L. Chen, Atomically dispersed metal catalysts confined by covalent organic frameworks and their derivatives for electrochemical energy conversion and storage, Coord. Chem. Rev., 2022, 466, 214592 CrossRef CAS
.
- W. Zhang, Q. Yao, G. Jiang, C. Li, Y. Fu, X. Wang, A. Yu and Z. Chen, Molecular trapping strategy to stabilize subnanometric Pt clusters for highly active electrocatalysis, ACS Catal., 2019, 9, 11603–11613 CrossRef CAS
.
- M. Fan, L. Cui, X. He and X. Zou, Emerging heterogeneous supports for efficient electrocatalysis, Small Methods, 2022, 6, e2200855 CrossRef PubMed
.
- J. Huang, R. Zeng and J. Chen, Thermostable carbon-supported subnanometer-sized (<1 nm) Pt clusters for the hydrogen evolution reaction, J. Mater. Chem. A, 2021, 9, 21972–21980 RSC
.
- Y. Wu, W. Wei, R. Yu, L. Xia, X. Hong, J. Zhu, J. Li, L. Lv, W. Chen, Y. Zhao, L. Zhou and L. Mai, Anchoring sub-nanometer Pt clusters on crumpled paper-like MXene enables high hydrogen evolution mass activity, Adv. Funct. Mater., 2022, 32, 2110910 CrossRef CAS
.
- S. Kumari, T. Masubuchi, H. White, A. Alexandrova, S. Anderson and P. Sautet, Electrocatalytic hydrogen evolution at full atomic utilization over ITO-supported sub-nano-Ptn clusters: High, size-dependent activity controlled by fluxional Pt hydride species, J. Am. Chem. Soc., 2023, 145, 5834–5845 CrossRef CAS PubMed
.
- D. Wu, D. Zhang, Z. Wang, J. Xu, X. Chen, J. Lai and L. Wang, Engineering of anchor sites and reaction time to efficiently synthesize high loading and stable sub-nanocluster catalysts, Mater. Chem. Front., 2022, 6, 3033–3041 RSC
.
- H. Li and G. Li, Novel palladium-based nanomaterials for multifunctional ORR/OER/HER electrocatalysis, J. Mater. Chem. A, 2023, 11, 9383–9400 RSC
.
- Y. Jia, T. H. Huang, S. Lin, L. Guo, Y. M. Yu, J. H. Wang, K. W. Wang and S. Dai, Stable Pd-Cu hydride catalyst for efficient hydrogen evolution, Nano Lett., 2022, 22, 1391–1397 CrossRef CAS PubMed
.
- X. Gao, G. Yu, L. Zheng, C. Zhang, H. Li, T. Wang, P. An, M. Liu, X. Qiu, W. Chen and W. Chen, Strong electron coupling from the sub-nanometer Pd clusters confined in porous ceria nanorods for highly efficient electrochemical hydrogen evolution reaction, ACS Appl. Energy Mater., 2019, 2, 966–973 CrossRef CAS
.
- Y. Wang, L. Zhang, C. Hu, S. Yu, P. Yang, D. Cheng, Z.-J. Zhao and J. Gong, Fabrication of bilayer Pd-Pt nanocages with sub-nanometer thin shells for enhanced hydrogen evolution reaction, Nano Res., 2019, 12, 2268–2274 CrossRef CAS
.
- Y. Pi, Z. Qiu, Y. Sun, H. Ishii, Y. F. Liao, X. Zhang, H. Y. Chen and H. Pang, Synergistic mechanism of sub-nanometric Ru clusters anchored on tungsten oxide nanowires for high-efficient bifunctional hydrogen electrocatalysis, Adv. Sci., 2023, 10, e2206096 CrossRef
.
- C. Cai, K. Liu, L. Zhang, F. Li, Y. Tan, P. Li, Y. Wang, M. Wang, Z. Feng, D. Motta Meira, W. Qu, A. Stefancu, W. Li, H. Li, J. Fu, H. Wang, D. Zhang, E. Cortes and M. Liu, Atomically local electric field induced interface water reorientation for alkaline hydrogen evolution reaction, Angew. Chem., Int. Ed., 2023, 62, e202300873 CrossRef CAS
.
- L. Li, P. Wang, Q. Shao and X. Huang, Metallic nanostructures with low dimensionality for electrochemical water splitting, Chem. Soc. Rev., 2020, 49, 3072–3106 RSC
.
- K. Wang, B. Huang, F. Lin, F. Lv, M. Luo, P. Zhou, Q. Liu, W. Zhang, C. Yang, Y. Tang, Y. Yang, W. Wang, H. Wang and S. Guo, Wrinkled Rh2P nanosheets as superior pH-universal electrocatalysts for hydrogen evolution catalysis, Adv. Energy Mater., 2018, 8, 1801891 CrossRef
.
- T. Zhang, J. Jin, J. Chen, Y. Fang, X. Han, J. Chen, Y. Li, Y. Wang, J. Liu and L. Wang, Pinpointing the axial ligand effect on platinum single-atom-catalyst towards efficient alkaline hydrogen evolution reaction, Nat. Commun., 2022, 13, 6875 CrossRef CAS
.
- M. Wang, M. Wang, C. Zhan, H. Geng, Y. Li, X. Huang and L. Bu, Ultrafine platinum-iridium distorted nanowires as robust catalysts toward bifunctional hydrogen catalysis, J. Mater. Chem. A, 2022, 10, 18972–18977 RSC
.
- Q. Yan, P. Yan, T. Wei, G. Wang, K. Cheng, K. Ye, K. Zhu, J. Yan, D. Cao and Y. Li, A highly efficient and durable water splitting system: Platinum sub-nanocluster functionalized nickel–iron layered double hydroxide as the cathode and hierarchical nickel–iron selenide as the anode, J. Mater. Chem. A, 2019, 7, 2831–2837 RSC
.
- D. H. Kweon, M. S. Okyay, S. J. Kim, J. P. Jeon, H. J. Noh, N. Park, J. Mahmood and J. B. Baek, Ruthenium anchored on carbon nanotube electrocatalyst for hydrogen production with enhanced faradaic efficiency, Nat. Commun., 2020, 11, 1278 CrossRef CAS PubMed
.
- C. Cai, K. Liu, Y. Zhu, P. Li, Q. Wang, B. Liu, S. Chen, H. Li, L. Zhu, H. Li, J. Fu, Y. Chen, E. Pensa, J. Hu, Y. R. Lu, T. S. Chan, E. Cortes and M. Liu, Optimizing hydrogen binding on Ru sites with RuCo alloy nanosheets for efficient alkaline hydrogen evolution, Angew. Chem., Int. Ed., 2022, 61, e202113664 CrossRef CAS PubMed
.
- D. Zhang, H. Zhao, B. Huang, B. Li, H. Li, Y. Han, Z. Wang, X. Wu, Y. Pan, Y. Sun, X. Sun, J. Lai and L. Wang, Advanced ultrathin RuPdM (M = Ni, Co, Fe) nanosheets electrocatalyst boosts hydrogen evolution, ACS Cent. Sci., 2019, 5, 1991–1997 CrossRef CAS PubMed
.
- Q. Hu, K. Gao, X. Wang, H. Zheng, J. Cao, L. Mi, Q. Huo, H. Yang, J. Liu and C. He, Subnanometric Ru clusters with upshifted D band center improve performance for alkaline hydrogen evolution reaction, Nat. Commun., 2022, 13, 3958 CrossRef CAS PubMed
.
- B. Yang, D. Bin, Q. Zhong, B. Li, Y. Liu, H. Lu and B. Liu, Highly efficient sub-nanometer RuxCuyP2 nanoclusters designed for hydrogen evolution under alkaline media, J. Colloid Interface Sci., 2021, 602, 222–231 CrossRef CAS PubMed
.
- Q. Wang, C. Q. Xu, W. Liu, S. F. Hung, H. Bin Yang, J. Gao, W. Cai, H. M. Chen, J. Li and B. Liu, Coordination engineering of iridium nanocluster bifunctional electrocatalyst for highly efficient and pH-universal overall water splitting, Nat. Commun., 2020, 11, 4246 CrossRef CAS PubMed
.
- Y. Liu, X. Li, Q. Zhang, W. Li, Y. Xie, H. Liu, L. Shang, Z. Liu, Z. Chen, L. Gu, Z. Tang, T. Zhang and S. Lu, A general route to prepare low-ruthenium-content bimetallic electrocatalysts for pH-universal hydrogen evolution reaction by using carbon quantum dots, Angew. Chem., Int. Ed., 2020, 59, 1718–1726 CrossRef CAS
.
- H. Yang, P. Guo, R. Wang, Z. Chen, H. Xu, H. Pan, D. Sun, F. Fang and R. Wu, Sequential phase conversion-induced phosphides heteronanorod arrays for superior hydrogen evolution performance to Pt in wide pH media, Adv. Mater., 2022, 34, e2107548 CrossRef
.
- D. Bhalothia, Y. Yu, Y. Lin, T. Huang, C. Yan, J. Lee, K. Wang and T. Chen, NiOx-supported PtRh nanoalloy enables high-performance hydrogen evolution reaction under universal pH conditions, Sustainable Energy Fuels, 2021, 5, 5490–5504 RSC
.
- Y. Liu, N. Chen, W. Li, M. Sun, T. Wu, B. Huang, X. Yong, Q. Zhang, L. Gu, H. Song, R. Bauer, J. S. Tse, S. Q. Zang, B. Yang and S. Lu, Engineering the synergistic effect of carbon dots-stabilized atomic and subnanometric ruthenium as highly efficient electrocatalysts for robust hydrogen evolution, SmartMat, 2021, 3, 249–259 CrossRef
.
- D. Qi, F. Lv, T. Wei, M. Jin, G. Meng, S. Zhang, Q. Liu, W. Liu, D. Ma, M. S. Hamdy, J. Luo and X. Liu, High-efficiency electrocatalytic NO reduction to NH3 by nanoporous VN, Nano Res. Energy, 2022, 1, e9120022 CrossRef
.
- M. Yang, S. Liu, J. Sun, M. Jin, R. Fu, S. Zhang, H. Li, Z. Sun, J. Luo and X. Liu, Highly dispersed Bi clusters for efficient rechargeable Zn−CO2 batteries, Appl. Catal., B, 2022, 307, 121145 CrossRef CAS
.
- L. Han, P. Ou, W. Liu, X. Wang, H.-T. Wang, R. Zhang, C.-W. Pao, X. Liu, W.-F. Pong, J. Song, Z. Zhuang, M. V. Mirkin, J. Luo and H. L. Xin, Design of Ru–Ni diatomic sites for efficient alkaline hydrogen oxidation, Sci. Adv., 2022, 8, eabm3779 CrossRef CAS PubMed
.
- G. Meng, H. Cao, T. Wei, Q. Liu, J. Fu, S. Zhang, J. Luo and X. Liu, Highly dispersed Ru clusters toward an efficient and durable hydrogen oxidation reaction, Chem. Commun., 2022, 58, 11839–11842 RSC
.
- S. Gao, S. Chen, Q. Liu, S. Zhang, G. Qi, J. Luo and X. Liu, Bifunctional BiPd alloy particles anchored on carbon matrix for reversible Zn–CO2 battery, ACS Appl. Nano Mater., 2022, 5, 12387–12394 CrossRef CAS
.
- S. Ge, L. Zhang, J. Hou, S. Liu, Y. Qin, Q. Liu, X. Cai, Z. Sun, M. Yang, J. Luo and X. Liu, Cu2O-derived PtCu nanoalloy toward energy-efficient hydrogen production via hydrazine electrolysis under large current density, ACS Appl. Energy Mater., 2022, 5, 9487–9494 CrossRef CAS
.
- W. Zhang, M. Jiang, S. Yang, Y. Hu, B. Mu, Z. Tie and Z. Jin, In-situ grown CuOx nanowire forest on copper foam: A 3D hierarchical and freestanding electrocatalyst with enhanced carbonaceous product selectivity in CO2 reduction, Nano Res. Energy, 2022, 1, e9120033 CrossRef
.
- F. Gao, J. He, H. Wang, J. Lin, R. Chen, K. Yi, F. Huang, Z. Lin and M. Wang, Te-mediated electro-driven oxygen evolution reaction, Nano Res. Energy, 2022, 1, e9120029 CrossRef
.
- E. Jiang, J. Li, X. Li, A. Ali, G. Wang, S. Ma, P. Kang Shen and J. Zhu, MoP-Mo2C quantum dot heterostructures uniformly hosted on a heteroatom-doped 3D porous carbon sheet network as an efficient bifunctional electrocatalyst for overall water splitting, Chem. Eng. J., 2022, 431, 133719 CrossRef CAS
.
- J. Zeng, L. Bi, Y. Cheng, B. Xu and A. K. Y. Jen, Self-assembled monolayer enabling improved buried interfaces in blade-coated perovskite solar cells for high efficiency and stability, Nano Res. Energy, 2022, 1, e9120004 CrossRef
.
Footnote |
† The two authors contribute equal to the paper. |
|
This journal is © the Partner Organisations 2024 |
Click here to see how this site uses Cookies. View our privacy policy here.