DOI:
10.1039/D4TB00940A
(Paper)
J. Mater. Chem. B, 2024,
12, 6840-6846
A water-soluble luminescent tris(2,4,6-trichlorophenyl)methyl radical-carbazole dyad†
Received
1st May 2024
, Accepted 6th June 2024
First published on 7th June 2024
Abstract
Organic luminescent radicals are a new class of materials with potential applications not only in light-emitting devices but also in the biochemistry field. New tris(2,4,6-trichlorophenyl)methyl (TTM) radicals with alkoxy-substituted carbazole donors were synthesized and characterized. PEG-substituted carbazole-TTM was found to be water-soluble. The water-soluble TTM radical aqueous solution showed fluorescence at 777 nm and the ability to shorten the longitudinal relaxation time (T1) of water. The concept of water-soluble luminescent radicals is expected to be used to develop a potential fluorescence and MR dual-use imaging moiety.
Introduction
Organic luminescent radicals are attractive for various applications, such as OLEDs, bioimaging, and sensing, due to their unique luminescence properties.1–11 In general, radicals are unstable under an ambient atmosphere and are highly reactive species, and they are recognized as luminescent quenchers rather than luminophores. Recently, some stable organic radicals have been discovered as luminescent materials through ingenious molecular design strategies. Tris(2,4,6-trichlorophenyl)methyl (TTM) and perchlorotriphenylmethyl (PTM) radicals have been known as stable luminescent radicals, which are isolatable under atmospheric conditions.12,13 The stability of chlorinated trityl radicals is ascribed largely to the steric protection of the center carbon with chlorine atoms at the ortho position and the delocalization of spin density over three π-conjugated systems. However, bare luminescent trityl radicals are known to have low photostability, and several strategies to improve photostability are proposed.14,15 For example, attaching a donor molecule such as carbazole to have a charge transfer excited state character is the most widely accepted method.16–19 Another approach is to add a heterocycle, such as pyridine, to decrease the energy of the frontier orbitals (PyBTM).20 Halogenated trityl radicals are the most widely studied luminescent radicals, and a great effort has been made to improve the photoluminescence (PL) quantum yield (PLQY) and photostability.
Organic radicals have potential applications as magnetic resonance imaging (MRI) contrast agents due to their paramagnetic properties.21–24 Typical MRI is an imaging technique that detects proton nuclei in water, fat, and other substances and produces contrast images based on differences in their relaxation times. The relaxation time of protons around a paramagnetic molecule is shortened by the weak local magnetic field generated by the molecule. Currently, Gd complexes are being used as clinical imaging contrast agents because they give MRI images with good contrast due to the highest spin quantum number (S = 7/2) of the Gd(III) ion.22,25,26 However, side effects such as nephrogenic systemic fibrosis and accumulation of Gd in the brain are considered problematic. On the other hand, organic radicals have lower toxicity and are a promising candidate for electron spin resonance (ESR) or MR imaging even though they have smaller spin quantum numbers.27 Several organic radicals based on 2,2,6,6-tetramethylpiperidine 1-oxyl (TEMPO) as ESR or MR imaging agents have been reported.19–22
TTM radical derivatives have attracted much attention due to their atmospheric stability, paramagneticity, and unique luminescence properties.28TTM-Cz, which has a carbazole donor and a TTM acceptor, has been extensively studied because of the photostability, high PLQY, and ease in modification of the carbazole moiety.29–32 Due to the red to near-infrared (NIR) emission of TTM-Cz derivatives, they are potential candidates for fluorescence bioimaging.3,5,6TTM-Cz is hydrophobic, and the low water solubility limits its biological application. The currently reported strategy is to use surfactants and encapsulate radical nanoparticles to solubilize them in water.3,5,6 The radical nanoparticles have potential for application in fluorescence imaging, but there is no report on their application as MR contrast agents and magnetic interaction with solvent water. Developing intrinsically water-soluble TTM-Cz derivatives will be a more straightforward approach to utilizing them for bioimaging applications. A few TTMs attached to the water-soluble or hydrophilic group have already been reported.33–37 However, to our knowledge, a compound that shows water-solubility, luminescence, and paramagnetic relaxation enhancement (PRE) effects simultaneously has never been reported.
In this work, two methoxy substituted and two polyethylene-glycol substituted TTM-Cz radicals were designed and synthesized as water soluble luminescent radicals (Fig. 1). Their photophysical properties, solubility, and potential applications for fluorescence and MR imaging in water were investigated. The small and dual-functional imaging probe is assumed to be not harmful to biological activities. TTM-PEG3 showed water solubility and NIR emission in an aqueous solution. Moreover, TTM-PEG3 exhibited water-proton longitudinal relaxivity under aqueous conditions. This is the first water-soluble TTM-Cz radical derivative showing potential for fluorescence and MR dual imaging applications.
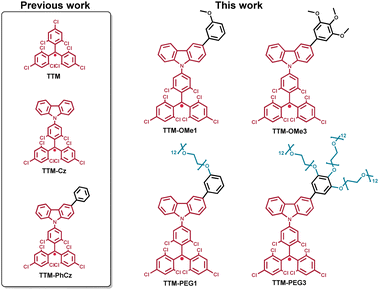 |
| Fig. 1 Chemical structures of alkoxy-substituted TTM radical derivatives. | |
Results and discussion
Synthesis and characterization
New TTM-Cz radicals were synthesized via deprotonation and one-electron oxidation of precursors: HTTM-(OMe1, OMe3, PEG1, and PEG3) (Fig. S1, ESI†),38–42 and radical precursors were synthesized by combining Suzuki–Miyaura cross-coupling and Williamson ether synthesis. The key compounds HTTM-OMe1 and HTTM-OMe3 were synthesized by Suzuki–Miyaura cross-coupling between boronated carbazole-HTTM (HTTM-BCz) and aryl halides.32,34,35 The methoxy group was converted to a hydroxyl group with BBr3 and reacted with tosylated polyethylene glycol (Ts-mPEG12) to obtain HTTM-PEG1 and HTTM-PEG3.32,33,37 Obtained precursors were treated with KOtBu and then subsequently oxidized with p-chloranil to obtain the radicals. Precursors were characterized using 1H NMR spectroscopy, 13C NMR spectroscopy, MALDI-TOF-MS, and elemental analysis. Radicals were characterized using MALDI-TOF-MS, elemental analysis, and ESR spectroscopy. The purity of the radicals was determined by ESR spectroscopy to be around 79–98% by comparing the signal strength with that of the standard reference (TEMPOL). The impurities are considered to be the radical precursors because they cannot be separated due to their structural similarities. The impurities were confirmed to be the radical precursors by 1H NMR. The new methoxylated and PEGylated TTM radical derivatives were successfully synthesized.
UV-vis absorption
The UV-vis absorption spectra of radicals were measured in toluene, chloroform, and water (Fig. 2a and Table 1). The new radicals in toluene showed identical spectral shapes with characteristic absorption bands at 377 nm attributed to the locally excited π–π* transition of the TTM moiety. Absorption bands around 625 nm (TTM-PhCz: 622 nm,28TTM-OMe1: 623 nm, TTM-OMe3: 626 nm, TTM-PEG1: 624 nm, and TTM-PEG3: 629 nm) attributed to charge transfer (CT) from the carbazole donor to the TTM acceptor were also observed. For comparison,
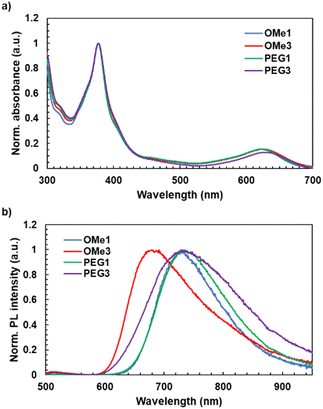 |
| Fig. 2 (a) UV-vis absorption spectra of new methoxylated and PEGylated TTM-Cz radicals measured in toluene (10−5 M) and (b) PL spectra of new methoxylated and PEGylated TTM-Cz radicals measured at an absorbance of 1.0[−] at 377 nm (excitation wavelength) in toluene. | |
Table 1 Photophysical properties of alkoxy-substituted TTM-Cz radical derivatives
Compounds |
λ
abs
(nm) |
λ
PL
(nm) |
PLQYa (%) |
τ
(ns) |
k
f
(106 s−1) |
k
nr
(106 s−1) |
λ
abs: absorption, λPL: PL peak wavelength and PLQY: photoluminescence quantum yield measured in toluene solutions (10−5 M).
τ: PL lifetime measured at absorbance of 1.0[−] at 377 nm in toluene.
k
f: radiative decay rate.
k
nr: non-radiative decay rate.
These values were cited from ref. 28.
|
TTM-PhCz
|
622e |
722e |
6.0e |
5.0 |
12 |
190 |
TTM-OMe1
|
623 |
729 |
6.8 |
4.1 |
17 |
230 |
TTM-OMe3
|
626 |
691 |
0.3 |
31 |
0.10 |
33 |
TTM-PEG1
|
624 |
735 |
3.4 |
2.1 |
16 |
463 |
TTM-PEG3
|
623 |
731 |
0.2 |
4.2 |
0.48 |
239 |
UV-vis absorption and PL spectra of TTM-PhCz were also measured. Comparing TTM-PhCz (Fig. 1) and new radicals, the CT absorption band of new radicals was slightly red-shifted due to the electron-donating effect of the methoxy group. The molar absorption coefficients (ε) were determined to be 4.8 × 103 M−1 cm−1, 608 nm (TTM-OMe1), 4.6 × 103 M−1 cm−1, 608 nm (TTM-OMe3), 4.6 × 103 M−1 cm−1, 608 nm (TTM-PEG1), and 4.4 × 103 M−1 cm−1, 610 nm (TTM-PEG3) in chloroform. Note that the ε values were corrected by the purity of radical compounds determined by ESR measurements, and the CT absorption peaks of new radicals in chloroform tend to appear at shorter wavelengths than those in toluene.28,43 PEGylated TTMs were water soluble and showed almost the same absorption spectral shape as in toluene (Fig. 3). The absorbance of TTM-PEG1 saturated aqueous solution prepared by filtration of the 10−4 M dispersion through a 0.20 μm pore size membrane filter was 0.047 (631 nm) which is about 1/10 times of TTM-PEG3 after filtration (0.42, 634 nm). ε of TTM-PEG3 in water was almost the same as that of TTM-PEG3 in chloroform. This result suggests that the water-solubility limit of TTM-PEG1 is smaller than 10−5 M, and that of TTM-PEG3 is higher than 10−5 M (later, it will be shown to be higher than 10−2 M). The CT absorption peak of TTM-PEG3 in water was located at 634 nm, and a slight bathochromic shift (5 nm) was observed compared to that in toluene. The aqueous solution of TTM-PEG3 was transparent, and no light scattering was observed. Methoxy and PEG substitutions change the absorption and emission slightly, and TTM-PEG3 is favorably water-soluble without any additives such as surfactants.
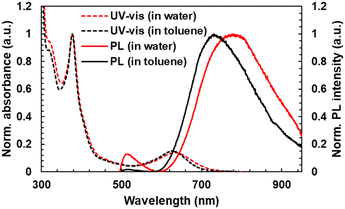 |
| Fig. 3 (dotted line) UV-vis absorption and (real line) PL spectra of TTM-PEG3 in (red) water (10−5 M) and (black) toluene (10−5 M) for comparison. | |
Dynamic light scattering (DLS) measurement of radicals was performed in toluene and water (1 mM) to understand the dissolved state of radicals in toluene and water (Fig. S14, ESI†). DLS measurements for TTM-PEG3 with average hydrodynamic diameters in water showed broad and sharp bands distributed around 6 nm and 104 nm. On the other hand, all of the radicals showed no light scattering signal in toluene, indicating that they were in a single molecular state. The DLS results indicate that TTM-PEG3 is water-soluble but tends to form aggregates in water.
Photoluminescence properties
The PL spectra, PLQY, and PL lifetime (τ) in toluene and water were measured (Fig. 2b, Table 1). New radical compounds exhibited PL in the red to NIR region in toluene at 729 nm (OMe1), 691 nm (OMe3), 735 nm (PEG1), and 731 nm (PEG3), respectively. The PLQY was determined to be 6.8% (OMe1), 0.3% (OMe3), 3.4% (PEG1), and 0.2% (PEG3), respectively. When methoxylated radicals were compared to PEGylated radicals, the PLQY of PEGylated radicals was lowered. The non-radiative decay rates (knr) of TTM-OMe1 (230 × 106 s−1), TTM-OMe3 (33 × 106 s−1), TTM-PEG1 (463 × 106 s−1), TTM-PEG3 (239 × 106 s−1) in toluene were determined using τ and PLQY (Table 1, Fig. S18, ESI†). PEGylated radicals seem to have higher flexibility, resulting in lower PLQY caused by the higher knr. The PL spectra and PLQY of TTM-PEG3 were also measured in water (Fig. 3). This water-soluble radicals exhibited detectable NIR emission with a low PLQY (777 nm, 0.006%) in water. Note that the PLQY value was determined by comparing the PL intensity of [Ru(bpy)3]Cl2 with the radical emission.44 To understand in detail the PL of TTM-PEG3 under aqueous conditions, PL spectra of TTM-PEG3 in different ratios of the water/THF (v/v%) mixture were measured (Fig. S15, ESI†).45 The PL intensity in the 10% water/THF mixture decreased compared to that in pure THF, while the PL intensities in the range from 10% to 90% were almost constant (Fig. S15, ESI†). This suggests that TTM-PEG3 forms an aggregate when water exists, resulting in concentration quenching of PL. All the radicals showed red to NIR emission in toluene, and TTM-PEG3 also showed NIR emission in water.
Solvent effect
The solvatofluorochromism was investigated to understand the details of the emission properties of radicals. The PL spectra of radical precursors were measured in toluene. HTTM-OMe3 (precursor) showed a red-shifted PL (367 and 380 nm) derived from the locally excited π*–π transition of the carbazole unit, compared to HTTM-OMe1 (356 and 373 nm), while their UV-vis absorption peak was almost identical (Fig. S16, ESI†). HTTM-OMe3 is assumed to have a higher HOMO energy level and a smaller HOMO–LUMO gap than HTTM-OMe1 due to the more electron donative methoxy groups. On the other hand, the PL peak of TTM-OMe3 (radical) (691 nm) was at a shorter wavelength than that of TTM-OMe1 (729 nm). According to the Lippert–Mataga plots (Fig. S17 and Table S1, ESI†), TTM-OMe1 was shown to be more sensitive to solvent polarity than TTM-OMe3.46,47 In cyclohexane, TTM-OMe1 showed a shorter PL wavelength than TTM-OMe3, as expected from the bandgap. TTM-OMe3 has a smaller bandgap and longer PL wavelength in cyclohexane compared to TTM-OMe1, but TTM-OMe1 has a larger solvent polarity effect that reverses the order in toluene. Similar to the comparison of TTM-OMe radicals, TTM-PEG3 has a smaller sensitivity to solvent polarity than TTM-PEG1 (Fig. S17, ESI†). However, the solvent dependence of the Stokes shift of TTM-PEG3 is rather weak, in highly polar solvents (water and ethanol) and 1,2-dimethoxyethane, which has structural similarity to the PEG chain, and the shift is almost the same around 3000 cm−1. This suggests that the dielectric environment around the TTM-PEG3 luminophore is constant. Moreover, the actual PL peak of TTM-PEG3 (777 nm) in water was much shorter than that in water extrapolated from the plot of TTM-OMe3 (around 929 nm). These results indicate that the TTM-PEG3 radicals are in a hydrophobic environment (probably aggregates in polar solvents). Thus, the formation of aggregates in water maintains the hydrophobic nature and is assumed to suppress the red-shift of PL, which results in the suppression of the quenching of the PL of TTM-PEG3 due to the energy gap law.
Photostability
The photostability of new radicals was investigated by monitoring the PL intensity during continuous irradiation with a 355 nm pulsed laser in toluene (Fig. 4). For the investigations, the absorbance of all radicals was controlled to be 0.5 at 355 nm, and the solutions were stirred during the measurement without degassing using a home-made apparatus.48 The half-lifetimes (t1/2) of radicals were determined to be 3.6 × 104 s (TTM-OMe1), 7.0 × 104 s (TTM-OMe3), 2.8 × 104 s (TTM-PEG1), respectively. These t1/2 values were slightly longer than that of TTM-PhCz (1.6 × 104 s), and drastically improved compared with that of TTM (3.3 × 101 s) measured under the same conditions. TTM-PEG3 showed an increase in PL intensity. This is assumed to be because the original PL intensity of TTM-PEG3 is weak and degraded compounds show a stronger luminescence. A distinct difference due to the laser irradiation is seen in the time-dependent PL spectra (Fig. S20, ESI†). The PL peak of TTM-PEG3 after long-term laser irradiation of 3590 seconds was blue-shifted by 18 nm from that after short-term laser irradiation of 20 seconds, namely 732 nm to 714 nm, attributed to a degraded compound, while other radicals show no or quite less blue-shift after long-term irradiation. The degraded compound of TTM-PEG3 also does not have strong luminescence intensity but it seems to be stronger than that of TTM-PEG3. As a result, some increase in the PL intensity was observed for TTM-PEG3. New radicals exhibited good photostability under atmospheric conditions.
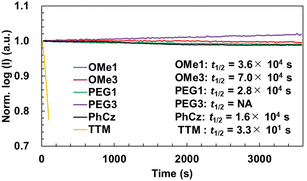 |
| Fig. 4 Photostability of TTM radical derivatives measured at an absorbance of 0.5[−] at 355 nm in toluene. t1/2 was determined by fitting the results with the equation I(t) = I0(1/2)^(t/t1/2), I(t) is the intensity at a specific time, I0 is the initial intensity, and t is the time. The plots were shown using the values of moving averages of 10 values over 200 seconds. | |
Longitudinal relaxivity
Water-proton longitudinal relaxivity (r1) of TTM-PEG3 in an aqueous solution was determined to investigate the potential of TTM-PEG3 for application as an MRI contrast agent. The longitudinal relaxation time (T1) of solvent water was measured using a 1 T NMR magnet at different concentrations (Fig. 5). The T1 relaxation time was shortened by the PRE effect due to the TTM-PEG3 radicals.19–22 This result indicates the potential of TTM-PEG3 for application as an MRI contrast agent. The compatibility of the MRI contrast agent was estimated as the relaxivity value r1, which was calculated to be 0.02 mM−1 s−1 using eqn (1).T0 and C denote the T1 relaxation time without the contrast agent and concentration of the contrast agent, respectively.20 This r1 value was smaller than those of the clinically used Gd complex (Gd-DTPA; 3.48 mM−1 s−1) and the organic radical TEMPO derivative (2-HEG; 0.41 mM−1 s−1) (Fig. S21, ESI†).21,49 In comparison with Gd, the spin quantum number of organic radicals is small (7/2 vs. 1/2). In comparison with organic radicals, the spin quantum number is the same, but the r1 value of TTM-PEG3 was 1 order of magnitude smaller than that of 2-HEG. This may originate from the hydrophobicity of the TTM unit and aggregate formation, i.e., the relatively small magnetic interactions between TTM-PEG3 and water molecules. Although r1 of TTM-PEG3 is lower than that of conventional radical contrast agents, TTM-PEG3 is the first TTM derivative to show potential for application as an MRI contrast agent.
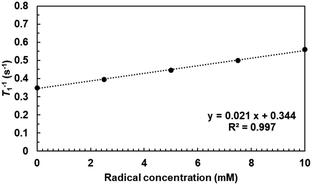 |
| Fig. 5 Plot of T1 relaxation rates of H2O protons versus the concentration of TTM-PEG3 in water. | |
Electrochemical properties
The electrochemical behavior of new radicals was investigated by cyclic voltammetry (CV) and differential pulse voltammetry (DPV) (Fig. 6). The CV of TTM-PhCz was also measured for comparison. All radicals exhibited two similar reversible redox couples at half-wave potentials of −0.96 V/0.53 V vs. Fc+/Fc (TTM-OMe1), −0.96 V/0.53 V (TTM-OMe3), −0.97 V/0.54 V (TTM-PEG1), and −0.97 V/0.52 V (TTM-PEG3). These redox potentials were also similar to that of TTM-PhCz (−0.98 V/0.53 V) which has no alkoxy group. The redox couple at low voltage is attributed to the reduction of the TTM radical and the redox couple at high voltage is attributed to the oxidation of the TTM radical.28 These potentials were nearly identical values, despite that the oxidation potential of the carbazole donor itself, as determined by the CV of the radical precursor, is affected by the alkoxy substituents (Fig. S22, ESI†). These nearly identical potentials were assumed to be due to the approximately 50° torsion between the carbazole donor and the TTM unit resulting in minimum electronic communication. All new radicals showed similar redox properties regardless of their substituents.
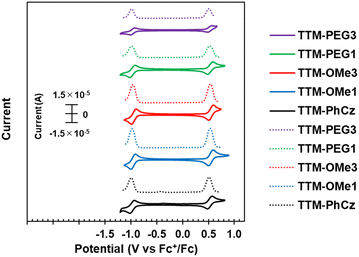 |
| Fig. 6 (Solid line) Cyclic voltammogram (CV) and (dotted line) differential pulse voltammogram (DPV) of TTM-Cz derivatives in CH2Cl2. | |
Theoretical calculations
Unrestricted density functional theory (DFT) calculations using Gaussian 16 with the UPBE0 functional and 6-31G(d,p) basic sets were performed in a vacuum to understand and compare the electronic structures of TTM-OMe1, TTM-OMe3, and TTM-PhCz (Fig. 7).50 The three radicals were chosen because the PEG chain has too much freedom for calculations, and the electronic structure was assumed to be similar to that of methoxy-substituted radicals. From calculations, the three radicals were found to exhibit similar orbital distributions. The βLUMO (TTM-PhCz: 192b, TTM-OMe1: 200b, TTM-OMe3: 216b) was localized over the TTM moiety, and the βHOMO (TTM-PhCz: 191b, TTM-OMe1: 199b, TTM-OMe3: 215b) was localized over the carbazole moiety. The βHOMO-βLUMO energies were obtained to give an orbital description of the lowest energy absorption band. The energy gaps between the βHOMO and βLUMO were estimated to be 2.20 eV (TTM-PhCz), 2.15 eV (TTM-OMe1), and 2.05 eV (TTM-OMe3). These results indicate the decrease of βHOMO → βLUMO transition energy with the introduction of the methoxy group and the trend matches the absorption maxima of the radicals (Table 1) and describes that the lowest excited state has a CT character.
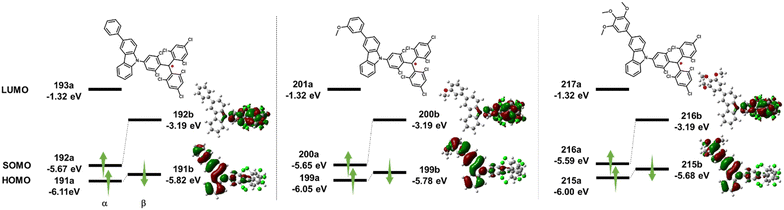 |
| Fig. 7 DFT calculation of HOMO and SOMO energy levels and distributions of molecular orbitals of TTM-Cz derivatives at the UPBE0/6-31G(d,p) level of theory. | |
Conclusions
Alkoxy-group substituted TTM-Cz radicals TTM-(OMe1, OMe3, PEG1, PEG3) exhibited red to NIR emission, and TTM-PEG3 is water soluble and showed NIR emission in water. All new radicals exhibited red to NIR emission derived from the CT excited state of the carbazole donor and TTM acceptor. TTM-PEG3 showed weak NIR emission in water (777 nm) and a water-proton longitudinal relaxivity (0.02 mM−1 s−1). To our knowledge, this is the first report of water-proton longitudinal relaxivity of luminescent radicals. These results show their potential for application in fluorescence and MR dual imaging. The simple molecular design strategy allowed the development of water-soluble and luminescent radicals that exhibit magnetic interactions with solvents and have potential applications in bioimaging.
Author contributions
K. Albrecht, and T. H. contributed to the acquisition of the funds for this project. The overall design of the investigation was done by K. Albrecht with assistance from T. H. Most measurements were performed by K. Anraku, with the assistance of K. M., K. Nakamura, K. Nakao, T. H., H. I., and S. M. Water Relaxation time was measured and analyzed by S. O. Major contributions to the data analysis and curation were undertaken by K. Anraku, and T. H. with smaller contributions from all others. The original draft of the paper was prepared by K. Anraku, and revised based on comments from K. Albrecht, T. H., and S. O. All authors have given approval to the final version of the manuscript.
Conflicts of interest
There are no conflicts to declare.
Acknowledgements
This work was supported, in part, by JSPS KAKENHI Grant No. JP23H02026 and JP23H03966, the Mitsubishi Foundation, and the Cooperative Research Program of “Network Joint Research Center for Materials and Devices”, and “Dynamic Alliance for Open Innovation Bridging Human, Environment and Materials” from MEXT, Japan. This study was also supported by the AIST Nanocharacterization Facility (ANCF) platform as a program of the “Nanotechnology Platform” (Grant Number JPMXP09A21AT0017) of MEXT, Japan. The computation was carried out using the computer resource offered under the category of General Projects by the Research Institute for Information Technology, Kyushu University. K. Albrcht thanks Transdisciplinary Energy Research (Q-PIT) through its “ModuleResearch” Program at Kyushu University. This work was supported by the Japan Science and Technology Agency (JST) as part of Adopting Sustainable Partnerships for Innovative Research Ecosystem (ASPIRE), Grant Number JPMJAP2334. K. Anraku, and K. Nakamura want to thank Ms Keiko Ideta for helping with ESR measurements. K. Anraku wants to thank Prof. Hajime Nakanotani and Ms Yuika Tamura for helping with PLQY measurements. KM thanks for the support from JST SPRING, Grant Number JPMJSP2136, and Grant-in-Aid for JSPS Research Fellow Grant No. JP24KJ1764. K. Anraku and KM thank Kyushu University Q-PIT Support Program for Young Researchers and Doctoral Students.
References
- Q. Peng, A. Obolda, M. Zhang and F. Li, Angew. Chem., Int. Ed., 2015, 54, 7091 CrossRef CAS PubMed.
- P. Murto, R. Chowdhury, S. Gorgon, E. Guo, W. Zeng, B. Li, Y. Sun, H. Francis, R. H. Friend and H. Bronstein, Nat. Commun., 2023, 14, 4147 CrossRef CAS PubMed.
- X. Bai, W. Tan, A. Abdurahman, X. Li and F. Li, Dyes Pigm., 2022, 202, 110260 CrossRef CAS.
- D. Blasi, N. Gonzalez-Pato, X. R. Rodriguez, I. Diez-Zabala, S. Y. Srinivasan, N. Camarero, O. Esquivias, M. Roldán, J. Guasch, A. Laromaine, P. Gorostiza, J. Veciana and I. Ratera, Small, 2023, 19(32), e2207806 CrossRef PubMed.
- L. Chen, T. Rudolf, R. Blinder, N. Suryadevara, A. Dalmeida, P. J. Welscher, M. Lamla, M. Arnold, U. Herr, F. Jelezko, M. Ruben and A. J. C. Kuehne, Macromolecules, 2023, 56, 2104 CrossRef CAS.
- Y. Xu, C. Teng, H. Dang, D. Yin and L. Yan, Talanta, 2024, 266, 124948 CrossRef CAS PubMed.
- T. P. Nguyen, A. D. Easley, N. Kang, S. Khan, S. M. Lim, Y. H. Rezenom, S. Wang, D. K. Tran, J. Fan, R. A. Letteri, X. He, L. Su, C. H. Yu, J. L. Lutkenhaus and K. L. Wooley, Nature, 2021, 593, 61 CrossRef CAS PubMed.
- Y. Hattori, S. Kimura, T. Kusamoto, H. Maeda and H. Nishihara, Chem. Commun., 2018, 54, 615 RSC.
- V. S. Mothika, M. Baumgarten and U. Scher, ACS Appl. Nano Mater., 2019, 2, 4832 CrossRef CAS.
- L. Zheng, W. Zhu, Z. Zhou, K. Liu, M. Gao and B. Z. Tang, Mater. Horiz., 2021, 8, 3082 RSC.
- Z. Zhou, J. Qian, K. Liu, Y. Zhang, M. Gao and B. Z. Tang, Angew. Chem., Int. Ed., 2022, 61, e2022126 Search PubMed.
- M. Ballester, Bull. Soc. Chim. Fr., 1966, 7 CAS.
- O. Armet, J. Veciana, C. Rovira, J. Riera, J. Castaner, E. Molins, J. Rius, C. Miravitlles, S. Olivella and J. Brichfeus, J. Phys. Chem., 1987, 91, 5608 CrossRef CAS.
- G. R. Luckhurst and J. N. Ockwell, Tetrahedron Lett., 1968, 9, 4123 CrossRef.
- M. A. Fox, E. Gaillard and C. C. Chen, J. Am. Chem. Soc., 1987, 109, 7088 CrossRef CAS.
- V. Gamero, D. Velasco, S. Latorre, F. Lopez-Calahorra, E. Brillas and L. Julia, Tetrahedron Lett., 2006, 47, 2305 CrossRef CAS.
- H. Guo, Q. Peng, X. Chen, Q. Gu, S. Dong, E. W. Evans, A. J. Gillett, X. Ai, M. Zhang, D. Credgington, V. Coropceanu, R. H. Friend, J. Brédas and F. Li, Nat. Mater., 2019, 18, 977 CrossRef CAS PubMed.
- H. Imahori, Y. Kobori and H. Kaji, Acc. Mater. Res., 2021, 2, 501 CrossRef CAS.
- C. Yan, D. An, W. Chen, N. Zhang, Y. Qiao, J. Fang, X. Lu, G. Zhou and Y. Liu, CCS Chem., 2022, 4, 3190 CrossRef CAS.
- Y. Hattori, T. Kusamoto and H. Nishihara, Angew. Chem., Int. Ed., 2014, 53, 11845 CrossRef CAS PubMed.
- K. Morishita, S. Murayama, T. Araki, I. Aoki and S. Karasawa, J. Org. Chem., 2016, 81, 8351 CrossRef CAS PubMed.
- K. Morishita, Y. Okamoto, S. Murayama, K. Usui, E. Ohashi, G. Hirai, I. Aoki and S. Karasawa, Langmuir, 2017, 33(31), 7810 CrossRef CAS PubMed.
- K. Morishita, S. Ueki, Y. Fuchi, S. Murayama, T. Kaneko, N. Narita, S. Kobayashi, G. Hirai, I. Aoki and S. Karasawa, ACS Appl. Nano Mater., 2018, 1, 6967 CrossRef CAS.
- R. Shiraishi, T. Kaneko, K. Usui, T. Naganuma, N. Iizuka, K. Morishita, S. Kobayashi, Y. Fuchi, Y. Matsuoka, G. Hirai, K. Yamada and S. Karasawa, ACS Omega, 2019, 4, 20715 CrossRef CAS PubMed.
- S. Okada, S. Mizukami and K. Kikuchi, ChemBioChem, 2010, 11, 785 CrossRef CAS PubMed.
- J. O. Lagerstedt, J. Petrlova, S. Hilt, A. Marek, Y. Chung, R. Sriram, M. S. Budamagunta, J. F. Desreuxd, D. Thonond, T. Jue, A. I. Smirnov and J. C. Voss, Contrast Media Mol. Imaging, 2013, 8, 252 CrossRef CAS PubMed.
- E. G. Ankel, C. Lai, L. E. Hopwood and Z. Zivkovic, Life Sci., 1987, 40(5), 495 CrossRef CAS PubMed.
- O. Armet, J. Veciana, C. Rovira, J. Riera, J. Castaner, E. Molins, J. Rius, C. Miravitlles, S. Olivella and J. Brichfeus, J. Phys. Chem., 1987, 91(22), 5608 CrossRef CAS.
- D. Velasco, S. Castellanos, M. López, F. López-Calahorra, E. Brillas and L. Juliá, J. Org. Chem., 2007, 72(20), 7523 CrossRef CAS PubMed.
- K. Matsuda, R. Xiaotian, K. Nakamura, M. Furukori, T. Hosokai, K. Anraku, K. Nakao and K. Albrecht, Chem. Commun., 2022, 58, 13443 RSC.
- K. Nakamura, K. Matsuda, R. Xiaotian, M. Furukori, S. Miyata, T. Hosokai, K. Anraku, K. Nakao and K. Albrecht, Faraday Discuss., 2024, 250, 192 RSC.
- R. Xiaotian, W. Ota, T. Sato, M. Furukori, Y. Nakayama, T. Hosokai, E. Hisamura, K. Nakamura, K. Matsuda, K. Nakao, A. P. Monkman and K. Albrecht, Angew. Chem., Int. Ed., 2023, 62, e202302550 CrossRef CAS PubMed.
- J. A. Mesa, A. Velázquez-Palenzuela, E. Brillas, J. Coll, J. L. Torres and L. Juliá, J. Org. Chem., 2012, 77(2), 1081 CrossRef CAS PubMed.
- J. A. Mesa, A. Velázquez-Palenzuela, E. Brillas, J. L. Torres and L. Juliá, Tetrahedron, 2011, 67, 3119 CrossRef CAS.
- A. Carreras, J. A. Mesa, M. Cascante, J. L. Torres and L. Juliá, New J. Chem., 2013, 37, 2043 RSC.
- A. Boś-Liedke, M. Walawender, A. Woźniak, D. Flak, J. Gapiński, S. Jurga, M. Kucińska, A. Plewiński, M. Murias, M. Elewa, L. Lampp, P. Imming and K. Tadyszak, Cell Biochem. Biophys., 2018, 76, 19 CrossRef PubMed.
- M. E. Arnold, L. Schoeneburg, M. Lamla and A. J. C. Kuehne, Molecules, 2024, 29, 995 CrossRef CAS PubMed.
- Z. S. Parr, R. B. Rashid, B. D. Paulsen, B. Poggi, E. Tan, M. Freeley, M. Palma, I. Abrahams, J. Rivnay and C. B. Nielsen, Adv. Electron. Mater., 2020, 6, 2000215 CrossRef CAS.
- T. Yasuda, T. Shimizu, F. Liu, G. Ungar and T. Kato, J. Am. Chem. Soc., 2011, 133(34), 13437 CrossRef CAS PubMed.
- B. Cheng, G. Zhu, L. Meng, G. Wu, Q. Chen and S. Ma, Eur. J. of Med. Chem., 2022, 228, 113930 CrossRef CAS PubMed.
- A. Abdurahman, Q. Peng, O. Ablikim, X. Ai and F. Li, Mater. Horiz., 2019, 6, 1265 RSC.
- H. Maeda, Y. Ito, Y. Haketa, N. Eifuku, E. Lee, M. Lee, T. Hashishin and K. Kaneko, Chem. – Eur. J., 2009, 15, 3706 CrossRef CAS PubMed.
- A. Abdurahman, T. J. H. Hele, Q. Gu, J. Zhang, Q. Peng, M. Zhang, R. H. Friend, F. Li and E. W. Evans, Nat. Mater., 2020, 19, 1224 CrossRef CAS PubMed.
- K. Nakamaru, Bull. Chem. Soc. Jpn., 1982, 55, 2697 CrossRef CAS.
- S. S. Liow, H. Zhou, S. Sugiarto, S. Guo, M. L. S. Chalasani, N. K. Verma, J. Xu and X. J. Loh, Biomacromolecules, 2017, 18, 886 CrossRef CAS PubMed.
- N. Mataga, Y. Kaifu and M. Koizumi, Bull. Chem. Soc. Jpn., 1956, 29, 465 CrossRef CAS.
- J. P. Cerón-Carrascoa, D. Jacquemina, C. Laurencea, A. Planchata, C. Reichardtc and K. Sraïdi, J. Phys. Org. Chem., 2014, 27, 512 CrossRef.
- M. Furukori, Y. Nagamune, Y. Nakayama and T. Hosokai, J. Mater. Chem. C, 2023, 11, 4357 RSC.
- P. Mi, D. Kokuryo, H. Cabral, M. Kumagai, T. Nomoto, I. Aoki, Y. Terada, A. Kishimura, N. Nishiyama and K. Kataoka, J. Controlled Release, 2014, 174, 63 CrossRef CAS PubMed.
-
M. J. Frisch, et al., Gaussian 16, Revision C.01, Gaussian, Inc., Wallingford CT, 2016 Search PubMed.
|
This journal is © The Royal Society of Chemistry 2024 |