DOI:
10.1039/D3QI01878D
(Research Article)
Inorg. Chem. Front., 2024,
11, 98-106
Heterogeneous CoS2/MS2 microspheres for an efficient oxygen evolution reaction†
Received
18th September 2023
, Accepted 8th November 2023
First published on 9th November 2023
Abstract
Delicately fabricating efficient electro-catalysts to boost the catalytic kinetics of the oxygen evolution reaction (OER) is essential for realizing the efficient performance of water splitting into hydrogen to alleviate environmental issues and the energy crisis. Here, heterogeneous and hollow microspheres (denoted as CoS2/MS2-HPMS, M = Fe, FeCo) were obtained by the sulfurization of Fe3+-decorated ZIF-67 hollow microspheres (ZIF-67-HMS). At a current density of 10 mA cm−2, the prepared CoS2/MS2-HPMS showed an overpotential of 217 mV for the OER, which is lower than that of commercial RuO2 (336 mV). Density functional theory calculations demonstrated low reaction free energies of the intermediates of the OER and significant charge accumulation at the heterojunction interface between CoS2 and FeCoS2. This work establishes a method for the assembly of nanoparticles and the construction of heterojunctions.
1. Introduction
Splitting water into hydrogen is a green and promising technology to alleviate the environmental issues and energy crises caused by the rapid consumption of non-renewable energy (natural gas, coal, and oil).1–4 However, the sluggish catalytic kinetics of the oxygen evolution reaction (OER) hinders highly efficient water splitting.5–8 Therefore, delicately fabricating efficient and stable electro-catalysts for the OER is essential for boosting water-splitting performance.9–12 Highly ordered porous coordination polymers, MOFs, have been regarded as promising candidates for the OER due to their high surface areas and rich porous structures for the exposure of more active sites and facilitation of fast mass transport.13–16 Increasing efforts have been focused on the synthesis of various MOF-based materials in recent years.17–19 Nevertheless, proposing a rapid and environmentally friendly synthetic method for the fabrication of MOF-based materials with well-defined structures remains challenging. In conventional routes for the fabrication of morphology-controllable and structure-versatile MOF-based materials, either environmentally hazardous chemicals or complicated synthetic procedures are often required,20–23 which hinders their practical applications. Therefore, developing a facile, green, and environmentally friendly approach for the fabrication of morphology-property-specific MOF-based materials is crucial for broadening their applicability.
Deep eutectic solvents (DESs) composed of Lewis/Brønsted acids and bases are a novel type of ionic liquid analog, possessing high thermal stability, tunable chemistry, etc.24–26 Relative to traditional ionic liquids, DESs possess a unique combination of various properties such as easy synthesis, economical affordability, non-toxicity, and biodegradability.27–29 As a mixture, the individual component of DESs endows the nanocrystals with a multi-self-assembly process due to its high ionic strength, making it possible to prepare functional materials with controllable morphologies and structures that are not accessible from other conventional fabrication routes.30,31 However, the use of DESs for the preparation of metal–organic frameworks (MOFs) has rarely been reported. Previous reports indicate that MOF-derived bimetallic-based sulfides are potential catalysts for OER research because of their low band gaps, strong synergistic effect, and high conductivity.32–36 The introduction of secondary metals, including Fe, Ni, V, Cu, Mn, and Mo, effectively tunes the electronic structure of the active sites,37–39 thereby lowering their adsorption to *OOH intermediates and enhancing the intrinsic catalytic activity toward the OER.40
Herein, we present a self-assembled method to prepare ZIF-67 hollow microspheres (ZIF-67-HMS) by using DES as a solvent. After vulcanizing the Fe3+-decorated ZIF-67-HMS, heterogeneous hollow and porous microspheres (denoted as CoS2/MS2-HPMS, M = Fe, FeCo) were generated. The prepared CoS2/MS2-HPMS exhibited high electrocatalytic activity and long-term durability for the OER owing to their unique heterogeneous structures and appropriate compositions. Density functional theory (DFT) calculations indicated that excellent catalytic performance originated from the lower reaction free energy of intermediates and electronic accumulation at the interface of CoS2 and FeCoS2. The current work presents a novel tactic for the assembly of nanoparticles and the construction of heterogeneous structures, which can be applied in catalytic chemistry.
2. Experimental
2.1 Materials
Ethanol (CH3CH2OH), methanol (CH3OH), and ethylene glycol (EG) were ordered from Xilong Chemical Co., Ltd, China. 2-Methylimidazole (2-MeIm) was purchased from Alfa Aesar. Choline chloride (ChCl) and urea were bought from Sinopharm Chemical Reagent Co. Ltd, China. Cobalt(II) nitrate hexahydrate (Co(NO3)2·6H2O, 99.99%), iron(III) chloride hexahydrate (FeCl3·6H2O, 99%), thioacetamide (TAA, 98%), and commercial ruthenium oxide (RuO2, 99.9%) were supplied by Aladdin. Potassium hydroxide (KOH, 95%) was provided by Maclean Biochemical Technology Co., Ltd, and the 5 wt% Nafion solution was obtained from Sigma-Aldrich. All the purchased chemicals were used directly.
2.2 Synthesis of ZIF-67 polyhedra
In a conventional preparation route, Co(NO3)2·6H2O (587.9 mg) and 2-MeIm (677.1 mg) were dissolved in 40 mL of ethanol. Then the mixture was transferred into a Teflon-lined stainless-steel autoclave (100 mL) and heated to 130 °C for 4 h. After being naturally cooled down, the obtained purple precipitate was washed with ethanol several times and then dried in a vacuum oven at 60 °C for 6 h. The resulting product was marked as ZIF-67 polyhedra.
2.3 Synthesis of ZIF-67-HMS
DESs were prepared based on the previously reported procedure.41,42 In a typical synthesis, the eutectic mixture was obtained by stirring choline chloride and urea at 80 °C until the formation of a homogeneous colorless liquid, and then it was sealed and stored for the subsequent synthesis. For the synthesis of ZIF-67-HMS, 677.1 mg of 2-MeIm (8 mmol) was first dissolved in the as-prepared DESs (40 mL) to form a homogeneous colorless solution at 75 °C, followed by the addition of 587.9 mg of Co(NO3)2·6H2O (2 mmol) and stirring for 5 min at 25 °C. Finally, the resultant mixture was poured into a Teflon-lined stainless-steel autoclave (100 mL) and heated at 130 °C for 4 h. After being naturally cooled down, the resulting purple precipitate was collected by centrifugation and washing with ethanol several times, and then it was dried in a vacuum oven at 60 °C for 6 h and finally, the obtained sample was labeled ZIF-67-HMS.
2.4 Synthesis of CoS2/MS2-HPMS and CoS/CoS2-HMS
ZIF-67-HMS (20 mg) and FeCl3·6H2O (6.1 mg) were dissolved in 20 mL of EG solution containing 90 mg of TAA under ultrasonication. Later, the obtained solution was transferred into a Teflon-lined stainless-steel autoclave (25 mL) and heated for 2 h at 180 °C. After cooling down to 25 °C, the sample was gathered by centrifugation and washing repeatedly with ethanol and then dried in a vacuum oven at 60 °C for 6 h and the obtained sample was denoted as CoS2/MS2-HPMS. CoS/CoS2-HMS was also synthesized by following the above-mentioned procedure in the absence of FeCl3·6H2O.
2.5 Electrochemical measurements
All electrochemical measurements were performed using the CHI 660E (Chenhua, Shanghai) electrochemical workstation equipped with a traditional three-electrode configuration, in which a saturated calomel electrode (SCE) was used as the reference electrode, a graphite rod was used as the counter electrode, and a carbon paper electrode coated with catalysts was used as the working electrode. All the applied potentials in OER studies were converted to the value of a reversible hydrogen electrode (RHE) by the Nernst equation of ERHE = ESCE + 0.05916pH + 0.241 (V). The overpotential (η) was obtained according to the formula, η = ERHE − 1.23 (V). All the electrochemical experiments were conducted in an N2-saturated 1.0 M KOH solution. Linear sweep voltammetry (LSV) measurements were performed at a scan rate of 5 mV s−1 with 85% iR compensation. Electrochemical impedance spectroscopy (EIS) studies were conducted at 1.54 V vs. RHE. Chronoamperometry (CA) used in the OER was conducted through galvanostatic experiments at −10 mA cm−2 for 20 h.
The working electrodes were prepared by pipetting 23 μL of catalyst ink onto pretreated carbon paper (load area: 0.5 cm2) with a mass loading of 0.275 mg cm−2. The preparation of a homogeneous catalyst ink was as follows: first, a uniform mixture was obtained by ultrasonically dispersing 1.5 mL of H2O, 1 mL of ethanol, and 15 μL of 5 wt% Nafion solution for 30 min, and then 3 mg of the as-synthesized catalyst was added into 500 μL of the above solution under ultrasonication to form the catalyst ink. The carbon paper was ultrasonically washed with a mixture of 10 wt% HNO3 and 10 wt% H2SO4, tri-distilled water, and ethanol, respectively.
2.6 Theoretical calculations
Density functional theory (DFT) implemented in the Vienna ab initio simulation package (VASP) was used to conduct the theoretical calculations using ref. 43 and 44 as references. The electron exchange and correlation energy were used to calibrate the generalized gradient approximation in the Perdew–Burke–Ernzerhof functional (GGA-PBE).45 The valence orbitals of S (3s, 3p), Fe (3d, 4s), Co (3d, 4s), O (2s, 2p), and H (1s) were described by plane-wave basis sets with a cutoff energy of 500 eV. The Monkhorst–Pack scheme with a (3 × 3 × 1) mesh for surface models with and without adsorbates was used for k-point sampling, and a (1 × 1 × 1) mesh was utilized for single-molecule adsorbates. For astringency, the values of electronic self-consistent iteration and force were set to 10−5 eV and 0.02 eV Å−1, respectively.
3. Results and discussion
Fig. 1a schematically shows the synthesis of CoS2/MS2-HPMS. In brief, the ZIF-67-HMS were synthesized by the self-assembly of smaller ZIF-67 polyhedra by using DES as the structure-directing agent and solvent. Then, the obtained ZIF-67-HMS were subjected to sulfurization in the presence of a sulfurization agent (TAA) to produce MS2 covered CoS2/MS2-HPMS heterostructures. During the synthesis, the selection of solvent is a critical factor. For instance, ZIF-67-HMS were obtained in DES medium, while ZIF-67 polyhedra were obtained in ethanol or methanol medium. Transmission electron microscopy (TEM) and scanning electron microscopy (SEM) techniques were employed to characterize the self-assembled ZIF-67-HMS. The SEM and TEM images showed that the ZIF-67 polyhedra were self-assembled into ZIF-67-HMS in DES medium (Fig. S1a and b†), which is different from the ZIF-67 polyhedra synthesized in methanol (Fig. S1c†) or ethanol medium (Fig. S1d†). This result displays the excellent self-assembly of ZIF-67 polyhedra in DESs. Notably, the self-assembled products of ZIF-67 polyhedra such as rhombic dodecahedra,46 two-dimensional microplates, and ultrathin nanosheets47,48 have been reported so far. Most importantly, the synthesized ZIF-67-HMS possess significant advantages such as good dispersibility and large specific surface areas, making them an ideal material for electrocatalysis research. The ZIF-67-HMS were subjected to sulfurization to increase the chemical/structural stability and electrical conductivity of the prepared sample49 and to produce heterogeneous CoS2/MS2-HPMS. TEM images show the hollow porous microspheres of CoS2/MS2-HPMS (Fig. 1b and c). A comparison of the electron microscopy images of CoS2/MS2-HPMS (Fig. 1b and c) and ZIF-67-HMS (Fig. S1a and b†) reveals good morphology retention after the sulfurization of ZIF-67-HMS. The magnified TEM images of CoS2/MS2-HPMS reveal the crossed lattice fringes of MS2 and CoS2 nanoparticles (Fig. 1d–f), which is different from those of CoS/CoS2-HMS (Fig. S2a–c†). This result suggests the formation of CoS2/MS2-HPMS heterostructures. From the magnified TEM images (Fig. 1g and h), the lattice spacings of 0.195 and 0.275 nm are assigned to the (220) and (200) crystal planes of CoS2, respectively. From the enlarged TEM images (Fig. 1i–k), the lattice spacings for the (101) and (102) crystal planes of FeCoS2 and the (200) crystal plane of FeS2 were found to be 0.254, 0.196, and 0.270 nm, respectively. Although a low crystallinity of C composition was found in CoS2/MS2-HMS, rich active sites and heterostructures were fabricated, which aids in the promotion of OER performance. The high-angle annular dark-field scanning TEM images and the corresponding elemental mapping images of CoS2/MS2-HPMS are shown in Fig. 1l. Similar to CoS/CoS2-HMS (Fig. S2c and d†), hollow structures are seen for CoS2/MS2-HPMS. Most importantly, the signals of Co, Fe, and S elements in CoS2/MS2-HPMS were evenly distributed in the whole selected areas, again indicating the heterostructures of CoS2/MS2-HPMS.
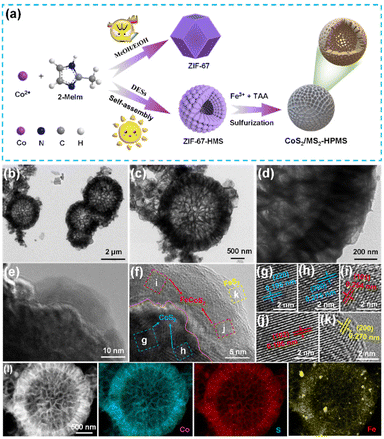 |
| Fig. 1 (a) Schematic synthesis of CoS2/MS2-HPMS. (b–d) TEM images, (e–k) HRTEM images, and (l) HAADF-STEM, and the corresponding mapping images of CoS2/MS2-HPMS. | |
The crystal structures and phase compositions of the synthesized ZIF-67-HMS, CoS/CoS2-HMS, and CoS2/MS2-HPMS were determined by the X-ray powder diffraction (XRD) technique. As shown in Fig. 2a, the XRD pattern verifies the successful fabrication of ZIF-67-HMS, which agrees with the previous report.36 Compared with ZIF-67 polyhedra, ZIF-67-HMS exhibit much sharper diffraction peaks, indicating a much higher crystallinity of ZIF-67-HMS and a more excellent assembled medium of DESs. As shown in Fig. S3,† the XRD pattern of CoS/CoS2-HMS well matches the standard cards of CoS (JCPDS no. 75-0605) and CoS2 (JCPDS no. 89-1492). As for CoS2/MS2-HPMS (Fig. 2b), the diffraction peaks at 32.30, 36.24, 39.83, 46.33, and 54.94° correspond to CoS2 (JCPDS no. 89-1492), and the diffraction peaks at 28.52, 33.04, 37.08, 40.77, 47.43, 56.28, 59.02, 61.69, and 64.29° are ascribed to FeS2 (JCPDS no. 71-1680). In addition, the diffraction peaks at 30.70, 35.17, 46.35, and 54.58° are assigned to FeCoS2 (JCPDS no. 75-0607). The X-ray photoelectron spectroscopy (XPS) spectra of CoS/CoS2-HMS and CoS2/MS2-HPMS (Fig. 2c) exhibited the signals of Co 2p, S 2p, and Fe 2p, reconfirming the presence of Co, S, and Fe elements in CoS2/MS2-HPMS. The N 1s signal originated from the 2-methylimidazole (C4H6N2) precursor and high-temperature decomposition of the urea component in DES. Fig. 2d shows the high-resolution XPS spectra of Co 2p in CoS/CoS2-HMS and CoS2/MS2-HPMS, respectively. The fitted doublets at 777.8 (Co 2p3/2) and 792.88 eV (Co 2p1/2) are indexed to Co2+, while the other doublets located at 779.86 (Co 2p3/2) and 795.67 eV (Co 2p1/2) are matched to Co3+, which originated from the oxidation of Co2+ species exposed to air.50 In addition, the peak of Co2+ in CoS2/MS2-HPMS located at 777.8 eV (Co 2p3/2) shows a significant negative shift of ca. 0.41 eV compared to Co2+ in CoS/CoS2-HMS (778.21 eV for Co 2p3/2). This result demonstrates a strong electronic interaction at the interface of CoS2 microspheres and the MS2 layer, which is very advantageous for the enhancement of electrocatalytic performance towards the OER.51–53 For the high-resolution spectrum of Fe 2p in CoS2/MS2-HPMS (Fig. 2e), two different valence states of Fe2+ (712.24 eV for Fe 2p3/2 and 724.36 eV for Fe 2p1/2) and Fe3+ (716.24 eV for Fe 2p3/2 and 729.38 eV for Fe 2p1/2) were verified in the prepared material. The presence of Fe3+ was ascribed to the easy oxidation of Fe2+ before the XPS characterization.54 The S 2p high-resolution spectra of CoS/CoS2-HMS and CoS2/MS2-HPMS indicate the existence of the S22− (162.85 and 164.16 eV) and S2− (160.92 and 162.03 eV) species in both materials (Fig. 2f).55 Moreover, the peaks at 167.15 and 168.47 eV confirm that S22− has been partly oxidized to SxOy− because of its inevitable contact with air. In addition, the existence of S22−, Co2+, and Fe2+ further confirms the formation of CoS2 and FeS2 phases, and the presence of S2−, Co2+, and Fe2+ also verifies the formation of CoS and FeCoS2 phases.
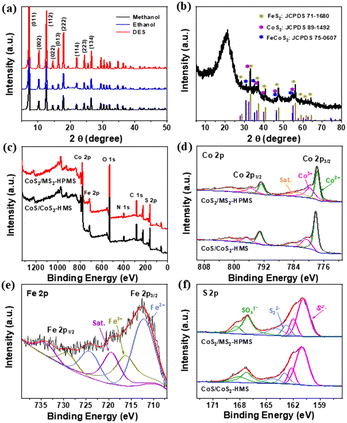 |
| Fig. 2 (a) XRD patterns of ZIF-67-HMS and ZIF-67 polyhedra, (b) XRD patterns of CoS2/MS2-HPMS, (c, d) XPS survey spectra and Co 2p spectra of CoS/CoS2-HMS and CoS2/MS2-HPMS, (e) Fe 2p spectra of CoS2/MS2-HPMS, and (f) S 2p spectra of CoS/CoS2-HMS and CoS2/MS2-HPMS. | |
To benchmark the electrocatalytic performance of the prepared CoS2/MS2-HPMS against ZIF-67-HMS, CoS/CoS2-HMS, and commercial RuO2, we conducted OER experiments in 1 M KOH solution. The LSV measurements with 85% iR compensation are shown in Fig. 3a. Specifically, CoS2/MS2-HPMS showed a small overpotential of 217 mV at a current density of 10 mA cm−2, which is significantly lower than that of CoS/CoS2-HMS (322 mV), ZIF-67-HMS (341 mV), commercial RuO2 (336 mV), and other reported materials (Table S1†). In addition, the overpotential of ZIF-67-HMS is much lower than that of previously reported ZIF-67 polyhedra (530 mV) synthesized in a conventional solvent,56 indicating that the unique hollow structure of ZIF-67-HMS facilitates the enhancement of OER performance. The higher OER activity on CoS2/MS2-HPMS compared to CoS/CoS2-HMS can be due to the following features: first, the hollow porous heterostructure of CoS2/MS2-HPMS, which provides more exposed active centers and facilitates faster charge transfer; second, the synergistic effect of different components and the formation of heterojunctions. Most importantly, CoS2/MS2-HPMS displayed an overpotential of only 333 mV at a higher current density of 831 mA cm−2, which is highly close to that of commercial RuO2 at a current density of 10 mA cm−2. This result implies that CoS2/MS2-HPMS possesses higher O2 production efficiency compared to ZIF-67-HMS, CoS/CoS2-HMS and commercial RuO2. In Fig. 3b, the corresponding Tafel slope of CoS2/MS2-HPMS (65 mV dec−1) is much smaller than that of ZIF-67-HMS (94 mV dec−1), CoS/CoS2-HMS (68 mV dec−1), commercial RuO2 (139 mV dec−1), and most of the electrocatalysts reported earlier (Table S1†). These results indicate that CoS2/MS2-HPMS is more favorable for the promotion of OER kinetics. Notably, the catalytic activity of CoS/CoS2-HMS is higher than that of ZIF-67-HMS, inferring that the presence of metal sulfide on the surface of the catalyst increases the number of unsaturated S atoms and enriches new active sites and, consequently, promotes its electrocatalytic ability towards the OER.57 To further study the reason for the high OER catalytic activity on the synthesized CoS2/MS2-HPMS, cyclic voltammetry (CV) and EIS techniques were used for the estimation of the electrochemical surface areas (ECSAs) and the charge transfer kinetics, respectively. In the calculation formula of ECSA = Cdl/Csp, the value of the electrochemical double-layer capacitance (Cdl) is half of the slope value of Δj versus the scan rate curve, whereas the specific capacitance (Csp) is taken as 0.040 mF cm−2 according to the relevant reports.58,59 From the formula, the value of ECSA is positively correlated with Cdl. Thus, the Cdl obtained from CV at different scan rates from 10 to 60 mV s−1 was used to evaluate the value of ECSAs.
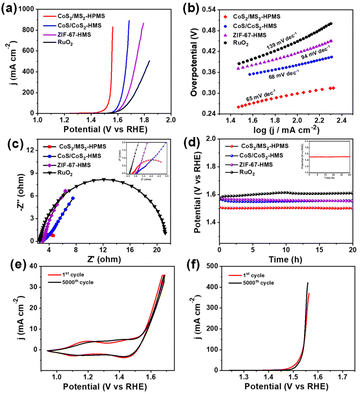 |
| Fig. 3 (a) LSV curves, (b) the corresponding Tafel slopes, (c) Nyquist plots, and (d) chronopotentiometric curves of ZIF-67-HMS, CoS/CoS2-HMS, CoS2/MS2-HPMS, and commercial RuO2. (e) The 1st and 5000th CV curves of CoS2/MS2-HPMS were recorded in 1 M KOH alkaline solution at a scan rate of 100 mV s−1. (f) The 1st and 5000th LSV polarization curves of CoS2/MS2-HPMS were recorded in 1.0 M KOH with iR compensation of 85% at a scan rate of 5 mV s−1. | |
Fig. S4a and b† show the CV curves of CoS2/MS2-HPMS and commercial RuO2 in the potential range of the non-faradaic region (1.19–1.24 V vs. RHE), respectively, and Fig. S4c† displays the linear fitted line of Δj vs. the scan rate (ν) in the corresponding CV curves. As shown in Fig. S4c,† CoS2/MS2-HPMS has a significantly increased Cdl of 18.11 mF cm−2 compared to that of commercial RuO2 (1.73 mF cm−2), which indicates that CoS2/MS2-HPMS possesses larger ECSAs. The ECSA–normalized LSV curves are used to evaluate the intrinsic catalytic activity of CoS2/MS2-HPMS. As shown in Fig. S5,† CoS2/MS2-HPMS exhibits the lowest onset potentials for the OER, indicating a good specific activity of CoS2/MS2-HPMS. The EIS results of the prepared samples disclose a much smaller semicircle diameter of CoS2/MS2-HPMS compared to those of ZIF-67-HMS, CoS/CoS2-HMS, and commercial RuO2 (Fig. 3c). These results indicate that CoS2/MS2-HPMS has the smallest electrochemical impedance and the most favorable charge transfer. To evaluate the actual application of CoS2/MS2-HPMS, durability tests without iR compensation were performed for all the prepared catalysts at a constant current density of 10 mA cm−2. As shown in Fig. 3d, CoS2/MS2-HPMS shows excellent stability compared to ZIF-67-HMS, CoS/CoS2-HMS, and commercial RuO2 catalysts, even after a continuous operation of 20 h. The overpotential of CoS2/MS2-HPMS hardly increased after 40 h of the CA test, further indicating the above conclusion (inset of Fig. 3d). Furthermore, the long-term durability of CoS2/MS2-HPMS was further studied by performing 5000 cycles of CV and LSV in the potential range of 0.94 to 1.69 V vs. RHE. As shown in Fig. 3e and f, the CV and LSV polarization curves almost overlap with their initial cycle after 5000 cycles, respectively, again confirming the good long-term stability of CoS2/MS2-HPMS. Notably, after 5000 cycles of CV tests, the morphology of CoS2/MS2-HPMS was well preserved without any noticeable change (Fig. S6†). The corresponding high-resolution TEM (HRTEM) images of CoS2/MS2-HPMS in Fig. S7† further reveal the appearance of FeOOH and CoOOH.60–62 In addition, the corresponding peak ratios of Fe3+/Fe2+ and Co3+/Co2+ increase after 5000 cycles of CV measurements (Fig. S8†). These results indicate the true electrocatalytic active sites of Co and Fe species in CoS2/MS2-HPMS for the OER.
We used DFT to illustrate the mechanisms of the OER process on CoS2/FeCoS2 together with CoS2 and FeCoS2. Fig. 4a shows the calculated models of CoS2, FeCoS2, and CoS2/MS2-HPMS, where the CoS2(001), FeCoS2(001), and FeCoS2@CoS2 heterogeneous structures were used as the calculated faces, respectively.63,64 As shown in Fig. 4b, the OER includes four elementary reaction steps with *OH, *O, *OOH, and *OO intermediates in the reaction pathway from *H2O to O2.65 The elementary reaction step for the formation of O2 (*OO → O2) on CoS2 is the potential-determining step (PDS) with a ΔG of 1.42 eV. As for FeCoS2, the PDS is the formation of *OOH (*O → OOH*), in which the corresponding ΔG is 0.99 eV. Compared with CoS2 and FeCoS2, the ΔG for each elementary reaction step on CoS2/FeCoS2 is much lower. Our DFT calculations show that the two reactions (*O → OOH* and *OOH → OO*) are endothermic, but the energy barrier for *OOH → OO* (0.84 eV) is much higher, which can be regarded as the RDS of the OER and is accordant with the recent report.66–68 These results indicate the heterojunction in the CoS2/FeCoS2 catalyst is conducive to promoting the OER activity. In addition, the density of states (DOS) of CoS2/FeCoS2 is much greater than that of CoS2 and FeCoS2 near the Fermi level (Fig. 4c). These results demonstrate that CoS2/FeCoS2 possesses a large carrier concentration and high intrinsic electrical conductivity originating from the electronic states near the Fermi level, which is favorable for the electron mobility in CoS2/FeCoS2.
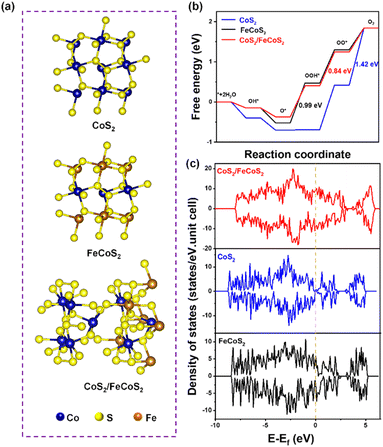 |
| Fig. 4 DFT calculations of CoS2, FeCoS2 and CoS2/MS2-HPMS. (a) Models for DFT calculations, (b) calculated Gibbs free energy, and (c) calculated DOS. | |
4. Conclusions
In summary, we demonstrated a green approach for the fabrication of CoS2/MS2-HPMS. By using DESs as a solvent, we successfully synthesized ZIF-67-HMS via the excellent self-assembly of ZIF-67 polyhedra. The obtained Fe3+-modified ZIF-67-HMS can be sulfurized into CoS2/MS2-HPMS with a unique heterojunction, besides the promoted conductivity, faster charge transfer, and enlarged ECSAs. All the results of electrochemical measurements and DFT calculations indicated that the heterojunction in CoS2/MS2-HPMS is key to the promotion of OER performance. Thus, the prepared CoS2/MS2-HPMS catalyst has a prospective application in overall water splitting. This work opens a novel approach for the self-assembly of MOF-based materials and the subsequent construction of heterostructures as well as their applications in the fields of energy, environment, and other catalytic reactions.
Conflicts of interest
There are no conflicts to declare.
Acknowledgements
This work was supported by the National Natural Science Foundation of China (22162006 and 22102035) and the Natural Science Foundation of Guangxi Province (2019GNSFGA245003, 2021GNSFBA220077, and GUIKE AD23026050).
References
- H. Ding, H. Liu, W. Chu, C. Wu and Y. Xie, Structural Transformation of Heterogeneous Materials for Electrocatalytic Oxygen Evolution Reaction, Chem. Rev., 2021, 121, 13174–13212 CrossRef CAS.
- B. M. Hunter, H. B. Gray and A. M. Muller, Earth-Abundant Heterogeneous Water Oxidation Catalysts, Chem. Rev., 2016, 116, 14120–14136 CrossRef CAS PubMed.
- N. Suen, S. Hung, Q. Quan, N. Zhang, Y. Xu and H. M. Chen, Electrocatalysis for the oxygen evolution reaction: recent development and future perspectives, Chem. Soc. Rev., 2017, 46, 337–365 RSC.
- E. Fabbri, M. Nachtegaal, T. Binninger, X. Cheng, B. J. Kim, J. Durst, F. Bozza, T. Graule, R. Schaublin, L. Wiles, M. Pertoso, N. Danilovic, K. E. Ayers and T. J. Schmidt, Dynamic surface self-reconstruction is the key of highly active perovskite nano-electrocatalysts for water splitting, Nat. Mater., 2017, 16, 925–931 CrossRef CAS.
- Y. Fang, Y. Hou, X. Fu and X. Wang, Semiconducting Polymers for Oxygen Evolution Reaction under Light Illumination, Chem. Rev., 2022, 122, 4204–4256 CrossRef CAS.
- J. Du, F. Li and L. Sun, Metal-organic frameworks and their derivatives as electrocatalysts for the oxygen evolution reaction, Chem. Soc. Rev., 2021, 50, 2663–2695 RSC.
- J. Song, C. Wei, Z.-F. Huang, C. Liu, L. Zeng, X. Wang and Z. J. Xu, A review on fundamentals for designing oxygen evolution electrocatalysts, Chem. Soc. Rev., 2020, 49, 2196–2214 RSC.
- D. Zhou, P. Li, X. Lin, A. McKinley, Y. Kuang, W. Liu, W.-F. Lin, X. Sun and X. Duan, Layered double hydroxide-based electrocatalysts for the oxygen evolution reaction: identification and tailoring of active sites, and superaerophobic nanoarray electrode assembly, Chem. Soc. Rev., 2021, 50, 8790–8817 RSC.
- F. Fan, Q. Huang, R. Devasenathipathy, X. Peng, F. Yang, X. Liu, L. Wang, D. Chen, Y. Fan and W. Chen, Composite-structure-defined nitrogen-doped carbon nanocage embedded Co/CoxP for enhanced oxygen reduction and evolution reactions, Electrochim. Acta, 2023, 437, 141514 CrossRef CAS.
- F. Fan, Q. Huang, R. Devasenathipathy, X. Peng, F. Yang, X. Liu, L. Wang, D. Chen, Y. Fan and W. Chen, Composition-adjustable Mo6Co6C2/Co@carbon nanocage for enhanced oxygen reduction and evolution reactions, J. Colloid Interface Sci., 2023, 636, 450–458 CrossRef CAS.
- Y. Wang, M. Zhang, Y. Liu, Z. Zheng, B. Liu, M. Chen, G. Guan and K. Yan, Recent Advances on Transition-Metal-Based Layered Double Hydroxides Nanosheets for Electrocatalytic Energy Conversion, Adv. Sci., 2023, 10, 2207519 CrossRef CAS.
- Z. Chen, W. Wei, L. Song and B.-J. Ni, Hybrid Water Electrolysis: A New Sustainable Avenue for Energy-Saving Hydrogen Production, Sustainable Horiz., 2022, 1, 100002 CrossRef.
- D. Zhao, J.-L. Shui, L. R. Grabstanowicz, C. Chen, S. M. Commet, T. Xu, J. Lu and D. Liu, Highly Efficient Non-Precious Metal Electrocatalysts Prepared from One-Pot Synthesized Zeolitic Imidazolate Frameworks, Adv. Mater., 2014, 26, 1093–1097 CrossRef CAS PubMed.
- C. Liu, J. Wang, J. Wan, Y. Cheng, R. Huang, C. Zhang, W. Hu, G. Wei and C. Yu, Amorphous Metal-Organic
Framework-Dominated Nanocomposites with Both Compositional and Structural Heterogeneity for Oxygen Evolution, Angew. Chem., Int. Ed., 2020, 59, 3630–3637 CrossRef CAS.
- F. Fan, Q. Huang, K. K. Rani, X. Peng, X. Liu, L. Wang, Z. Yang, D. Huang, R. Devasenathipathy, D.-H. Chen, Y. Fan and W. Chen, Interface and doping engineering of Co-based electrocatalysts for enhanced oxygen reduction and evolution reactions, Chem. Eng. J., 2023, 470, 144380 CrossRef CAS.
- D. Liu, C. Wang, Z. Zhou, C. Ye, R. Yu, C. Wang and Y. Du, Ultra-low Ru doped MOF-derived hollow nanorods for efficient oxygen evolution reaction, Inorg. Chem. Front., 2022, 9, 6158–6166 RSC.
- H. Wang, L. Chen, H. Pang, S. Kaskel and Q. Xu, MOF-derived electrocatalysts for oxygen reduction, oxygen evolution and hydrogen evolution reactions, Chem. Soc. Rev., 2020, 49, 1414–1448 RSC.
- H. Furukawa, K. E. Cordova, M. O'Keeffe and O. M. Yaghi, The Chemistry and Applications of Metal-Organic Frameworks, Science, 2013, 341, 1230444 CrossRef.
- H.-H. Tan, X.-L. Lv, J.-L. Liu, Y.-F. Cheng, Y.-T. Lin and W. Meng, A 2D Layer Copper(II) Coordination Polymer with 3-Nitrophthalic Acid: Synthesis, Crystal Structure and Copper 3-Nitrophthalate Metal-organic Framework-graphene Oxide Nanocomposite, Chin. J. Struct. Chem., 2021, 40, 459–464 CAS.
- M. Abdul Nasir Khan, P. Kwame Klu, C. Wang, W. Zhang, R. Luo, M. Zhang, J. Qi, X. Sun, L. Wang and J. Li, Metal-organic framework-derived hollow Co3O4/carbon as efficient catalyst for peroxymonosulfate activation, Chem. Eng. J., 2019, 363, 234–246 CrossRef CAS.
- W. Sun, X. Zhai and L. Zhao, Synthesis of ZIF-8 and ZIF-67 nanocrystals with well-controllable size distribution through reverse microemulsions, Chem. Eng. J., 2016, 289, 59–64 CrossRef CAS.
- W. Xiong, H. Li, H. You, M. Cao and R. Cao, Encapsulating metal organic framework into hollow mesoporous carbon sphere as efficient oxygen bifunctional electrocatalyst, Natl. Sci. Rev., 2020, 7, 609–619 CrossRef CAS PubMed.
- H. B. Wu, B. Y. Guan, P. He and X. Yu, Synthesis of ZIF-67 nanocubes with complex structures co-mediated by dopamine and polyoxometalate, J. Mater. Chem. A, 2018, 6, 19338–19341 RSC.
- M. Watanabe, M. L. Thomas, S. Zhang, K. Ueno, T. Yasuda and K. Dokko, Application of Ionic Liquids to Energy Storage and Conversion Materials and Devices, Chem. Rev., 2017, 117, 7190–7239 CrossRef CAS PubMed.
- X. Kang, X. Sun and B. Han, Synthesis of Functional Nanomaterials in Ionic Liquids, Adv. Mater., 2016, 28, 1011–1030 CrossRef CAS PubMed.
- R. Wang, X. Ma, D. Ding, B. Huang, Z. Zhu, T. Su, W. Liao, H. Lü and K. Yang, Synthesis of a Pt3Sn catalyst using a solvothermal method assisted by deep eutectic solvents for selective hydrogenation of furfural to furfuryl alcohol, Inorg. Chem. Front., 2023, 10, 5420–5429 RSC.
- J. Jiang, C. Yan, X. Zhao, H. Luo, Z. Xue and T. Mu, A PEGylated deep eutectic solvent for controllable solvothermal synthesis of porous NiCo2S4 for efficient oxygen evolution reaction, Green Chem., 2017, 19, 3023–3031 RSC.
- Y. Wang and H. Zhou, A green and cost-effective rechargeable battery with high energy density based on a deep eutectic catholyte, Energy Environ. Sci., 2016, 9, 2267–2272 RSC.
- M. Wu, H. Ma, Z. Ma, Y. Jin, C. Chen, X. Guo, Y. Qiao, C. M. Pedersen, X. Hou and Y. Wang, Deep Eutectic Solvents: Green Solvents and Catalysts for the Preparation of Pyrazine Derivatives by Self-Condensation of d-Glucosamine, ACS Sustainable Chem. Eng., 2018, 6, 9434–9441 CrossRef CAS.
- X. Zhao, Z. Xue, W. Chen, Y. Wang and T. Mu, Eutectic Synthesis of High-Entropy Metal Phosphides for Electrocatalytic Water Splitting, ChemSusChem, 2020, 13, 2038–2042 CrossRef CAS.
- M. S. Dibyendu Mondal, C.-H. Wang, Y.-C. Lin, H.-C. Huang, A. Saha, S. K. Nataraj and K. Prasad, Deep eutectic solvent promoted one step sustainable conversion of fresh seaweed biomass to functionalized graphene as a potential electrocatalyst, Green Chem., 2016, 18, 2819–2826 RSC.
- S. Zhao, Y. Wang, J. Dong, C. He, H. Yin, P. An, K. Zhao, X. Zhang, C. Gao, L. Zhang, J. Lv, J. Wang, J. Zhang, A. M. Khattak, N. A. Khan, Z. Wei, J. Zhang, S. Liu, H. Zhao and Z. Tang, Ultrathin metal-organic framework nanosheets for electrocatalytic oxygen evolution, Nat. Energy, 2016, 1, 16184 CrossRef CAS.
- G. Jia, W. Zhang, G. Fan, Z. Li, D. Fu, W. Hao, C. Yuan and Z. Zou, Three-Dimensional Hierarchical Architectures Derived from Surface-Mounted Metal-Organic Framework Membranes for Enhanced Electrocatalysis, Angew. Chem., Int. Ed., 2017, 56, 13781–13785 CrossRef CAS PubMed.
- T. C. Narayan, T. Miyakai, S. Seki and M. Dincă, High Charge Mobility in a Tetrathiafulvalene-Based Microporous Metal-Organic Framework, J. Am. Chem. Soc., 2012, 134, 12932–12935 CrossRef CAS PubMed.
- J. Cao, C. Lei, J. Yang, X. Cheng, Z. Li, B. Yang, X. Zhang, L. Lei, Y. Hou and K. Ostrikov, An ultrathin cobalt-based zeolitic imidazolate framework nanosheet array with a strong synergistic effect towards the efficient oxygen evolution reaction, J. Mater. Chem. A, 2018, 6, 18877–18883 RSC.
- Q. Wang, F. Gao, B. Xu, F. Cai, F. Zhan, F. Gao and Q. Wang, ZIF-67 derived amorphous CoNi2S4 nanocages with nanosheet arrays on the shell for a high-performance asymmetric supercapacitor, Chem. Eng. J., 2017, 327, 387–396 CrossRef CAS.
- X. Xiao, C. He, S. Zhao, J. Li, W. Lin, Z. Yuan, Q. Zhang, S. Wang, L. Dai and D. Yu, A general approach to cobalt-based homobimetallic phosphide ultrathin nanosheets for highly efficient oxygen evolution in alkaline media, Energy Environ. Sci., 2017, 10, 893–899 RSC.
- X. Lv, W. Xu, W. Tian, H. Wang and Z. Yuan, Activity Promotion of Core and Shell in Multifunctional Core-Shell Co2P@NC Electrocatalyst by Secondary Metal Doping for Water Electrolysis and Zn–Air Batteries, Small, 2021, 17, 2101856 CrossRef CAS PubMed.
- J. Xu, J. Li, D. Xiong, B. Zhang, Y. Liu, K. Wu, I. Amorim, W. Li and L. Liu, Trends in activity for the oxygen evolution reaction on transition metal (M = Fe, Co, Ni) phosphide pre-catalysts, Chem. Sci., 2018, 9, 3470–3476 RSC.
- J. Jiang, S. Lu, W. Wang, G. Huang, B. Huang, F. Zhang, Y. Zhang and H. Yu, Ultrahigh electrocatalytic oxygen evolution by iron-nickel sulfide nanosheets/reduced graphene oxide nanohybrids with an optimized autoxidation process, Nano Energy, 2018, 43, 300–309 CrossRef CAS.
- D. B. Andrew, P. Abbott, G. Capper, D. L. Davies and R. K. Rasheed, Deep Eutectic Solvents Formed between Choline Chloride and Carboxylic Acids: Versatile Alternatives to Ionic Liquids, J. Am. Chem. Soc., 2004, 126, 9142–9147 CrossRef PubMed.
- A. P. Abbott, K. El Ttaib, G. Frisch, K. J. McKenzie and K. S. Ryder, Electrodeposition of copper composites from deep eutectic solvents based on choline chloride, Phys. Chem. Chem. Phys., 2009, 11, 4269–4277 RSC.
- G. Kresse and J. Furthmüller, Efficiency of ab-initio total energy calculations for metals and semiconductors using a plane-wave basis set, Comput. Mater. Sci., 1996, 6, 15–50 CrossRef CAS.
- G. Kresse and J. Furthmüller, Efficient iterative schemes for ab initio total-energy calculations using a plane-wave basis set, Phys. Rev. B: Condens. Matter Mater. Phys., 1996, 54, 11169–11186 CrossRef CAS.
- J. P. Perdew, K. Burke and M. Ernzerhof, Generalized Gradient Approximation Made Simple, Phys. Rev. Lett., 1996, 77, 3865–3868 CrossRef CAS PubMed.
- K. Sun, J. Sun, G. Lu and C. Cai, Enhanced catalytic activity of cobalt nanoparticles encapsulated with an N-doped porous carbon shell derived from hollow ZIF-8 for efficient synthesis of nitriles from primary alcohols in water, Green Chem., 2019, 21, 4334–4340 RSC.
- D. Sun, D. Yang, P. Wei, B. Liu, Z. Chen, L. Zhang and J. Lu, One-Step Electrodeposition of Silver Nanostructures on 2D/3D Metal-Organic Framework ZIF-67: Comparison and Application in Electrochemical Detection of Hydrogen Peroxide, ACS Appl. Mater. Interfaces, 2020, 12, 41960–41968 CrossRef CAS PubMed.
- G. Wei, Z. Zhou, X. Zhao, W. Zhang and C. An, Ultrathin Metal-Organic Framework Nanosheet-Derived Ultrathin Co3O4 Nanomeshes with Robust Oxygen-Evolving Performance and Asymmetric Supercapacitors, ACS Appl. Mater. Interfaces, 2018, 10, 23721–23730 CrossRef CAS.
- H. S. Jadhav, H. A. Bandal, S. Ramakrishna and H. Kim, Critical Review, Recent Updates on Zeolitic Imidazolate Framework-67 (ZIF-67) and Its Derivatives for Electrochemical Water Splitting, Adv. Mater., 2022, 34, 2107072 CrossRef CAS.
- H. Zhu, J. Zhang, R. Yanzhang, M. Du, Q. Wang, G. Gao, J. Wu, G. Wu, M. Zhang, B. Liu, J. Yao and X. Zhang, When Cubic Cobalt Sulfide Meets Layered Molybdenum Disulfide: A Core-Shell System Toward Synergetic Electrocatalytic Water Splitting, Adv. Mater., 2015, 27, 4752–4759 CrossRef CAS PubMed.
- B. Qiu, Q. Zhu, M. Du, L. Fan, M. Xing and J. Zhang, Efficient Solar Light Harvesting CdS/Co9S8 Hollow Cubes for Z-Scheme Photocatalytic Water Splitting, Angew. Chem., Int. Ed., 2017, 56, 2684–2688 CrossRef CAS.
- Y. Tan, H. Wang, P. Liu, Y. Shen, C. Cheng, A. Hirata, T. Fujita, Z. Tang and M. Chen, Versatile nanoporous bimetallic phosphides towards electrochemical water splitting, Energy Environ. Sci., 2016, 9, 2257–2261 RSC.
- H. Schäfer, D. M. Chevrier, P. Zhang, J. Stangl, K. Müller-Buschbaum, J. D. Hardege, K. Kuepper, J. Wollschläger, U. Krupp, S. Dühnen, M. Steinhart, L. Walder, S. Sadaf and M. Schmidt, Electro-Oxidation of Ni42 Steel: A Highly Active Bifunctional Electrocatalyst, Adv. Funct. Mater., 2016, 26, 6402–6417 CrossRef.
- S. Li, J. Tang, Q. Liu, X. Liu and B. Gao, A novel stabilized carbon-coated nZVI as heterogeneous persulfate catalyst for enhanced degradation of 4-chlorophenol, Environ. Int., 2020, 138, 105639 CrossRef CAS PubMed.
- X. Ma, W. Zhang, Y. Deng, C. Zhong, W. Hu and X. Han, Phase and composition controlled synthesis of cobalt sulfide hollow nanospheres for electrocatalytic water splitting, Nanoscale, 2018, 10, 4816–4824 RSC.
- Y. Wang, Y. Wang, L. Zhang, C. Liu and H. Pang, Core-shell-type ZIF-8@ZIF-67@POM hybrids as efficient electrocatalysts for the oxygen evolution reaction, Inorg. Chem. Front., 2019, 6, 2514–2520 RSC.
- X. Z. Song, N. Zhang, X. F. Wang and Z. Tan, Recent advances of metal-organic frameworks and their composites toward oxygen evolution electrocatalysis, Mater. Today Energy, 2021, 19, 100597 CrossRef CAS.
- C. C. L. McCrory, S. Jung, J. C. Peters and T. F. Jaramillo, Benchmarking Heterogeneous Electrocatalysts for the Oxygen Evolution Reaction, J. Am. Chem. Soc., 2013, 135, 16977–16987 CrossRef CAS PubMed.
- S. Niu, W. Jiang, T. Tang, L. Yuan, H. Luo and J. Hu, Autogenous Growth of Hierarchical NiFe(OH)x/FeS Nanosheet-On-Microsheet Arrays for Synergistically Enhanced High-Output Water Oxidation, Adv. Funct. Mater., 2019, 29, 1902180 CrossRef.
- J. Chen, F. Zheng, S. Zhang, A. Fisher, Y. Zhou, Z. Wang, Y. Li, B. Xu, J. Li and S. Sun, Interfacial Interaction between FeOOH and Ni-Fe LDH to Modulate the Local Electronic Structure for Enhanced OER Electrocatalysis, ACS Catal., 2018, 8, 11342–11351 CrossRef CAS.
- J. Feng, H. Xu, Y. Dong, S. Ye, Y. Tong and G. Li, FeOOH/Co/FeOOH Hybrid Nanotube Arrays as High-Performance Electrocatalysts for the Oxygen Evolution Reaction, Angew. Chem., Int. Ed., 2016, 55, 3694–3698 CrossRef CAS PubMed.
- M. Li and L. Feng, NiSe2-CoSe2 with a Hybrid Nanorods and Nanoparticles Structure for Efficient Oxygen Evolution Reaction, Chin. J. Struct. Chem., 2022, 41, 2201019–2201024 CAS.
- J. Hao, W. Yang, Z. Peng, C. Zhang, Z. Huang and W. Shi, A Nitrogen Doping Method for CoS2 Electrocatalysts with Enhanced Water Oxidation Performance, ACS Catal., 2017, 7, 4214–4220 CrossRef CAS.
- J. Zhang, Y. Liu, C. Sun, P. Xi, S. Peng, D. Gao and D. Xue, Accelerated Hydrogen Evolution Reaction in CoS2 by Transition-Metal Doping, ACS Energy Lett., 2018, 3, 779–786 CrossRef CAS.
- Y. Jiao, Y. Zheng, M. Jaroniec and S. Z. Qiao, Design of electrocatalysts for oxygen- and hydrogen-involving energy conversion reactions, Chem. Soc. Rev., 2015, 44, 2060–2086 RSC.
- Z. He, J. Zhang, Z. Gong, H. Lei, D. Zhou, N. Zhang, W. Mai, S. Zhao and Y. Chen, Activating lattice oxygen in NiFe-based (oxy) hydroxide for water electrolysis, Nat. Commun., 2022, 13, 2191 CrossRef CAS PubMed.
- Y. Wang, Y.-J. Tang and K. Zhou, Self-Adjusting Activity Induced by Intrinsic Reaction Intermediate in Fe-N-C Single-Atom Catalysts, J. Am. Chem. Soc., 2019, 141, 14115–14119 CrossRef CAS PubMed.
- B. Zhang, X. Zheng, V. Oleksandr, C. Riccardo, B. Michal, G.-M. Max, L. Han, J. Xu, M. Liu, L. Zheng, F. P. G. de Arquer, C. T. Dinh, F. Fan, Y. Mingjian, Y. Emre, N. Chen, R. Tom, P. Liu, Y. Li, P. De Luna, J. Alyf, H. L. Xin, H. Yang, V. Aleksandra and E. H. Sargent, Homogeneously dispersed multimetal oxygen-evolving catalysts, Science, 2016, 352, 333–337 CrossRef CAS PubMed.
|
This journal is © the Partner Organisations 2024 |