DOI:
10.1039/D3MA01126G
(Review Article)
Mater. Adv., 2024,
5, 3602-3628
Plant-derived selenium nanoparticles: investigating unique morphologies, enhancing therapeutic uses, and leading the way in tailored medical treatments
Received
15th December 2023
, Accepted 28th March 2024
First published on 3rd April 2024
Abstract
Selenium (Se) is a paramount micronutrient, indispensable for the holistic health of humans, animals, and microorganisms. At its core, Se is instrumental for the genesis of selenocysteine, an exclusive amino acid vital for the assembly of selenoproteins, critical factors in human physiology. While the health dividends of Se are profound, its therapeutic potential is intricately tethered to a precise dosage window, underlining the imperativeness of meticulous calibration. Emerging from this backdrop, selenium nanoparticles (SeNPs) have become the gold standard in nanomaterials, distinguished by their unparalleled immunomodulatory prowess, superior biocompatibility, and enhanced bioavailability. Their reduced toxicity further consolidates their preeminence in biomedical applications. Synthesis methodologies for SeNPs span a spectrum from physical and chemical to biological approaches. Yet, it is the phyto-synthesized SeNPs that reign supreme, characterized by their singular morphological and biochemical attributes, which impart an unmatched compatibility with human tissues. Strikingly, despite their evident superiority, plant-derived SeNPs remain conspicuously underrepresented in the pharmaceutical arena. Contemporary research not only extols the therapeutic potency of SeNPs but also underscores their formidable efficacy against formidable adversaries like cancer cells, microbial pathogens, and viral threats, as well as their robust antioxidant capabilities. This review embarks on a rigorous exposition of the vanguard in plant-mediated SeNPs synthesis, traversing the diverse botanical landscape. It further ventures into the frontier of SeNPs functionalization, illuminating prospects for precision-targeted drug delivery. Conclusively, the review furnishes a comprehensive elucidation of the intricate therapeutic mechanisms harnessed by SeNPs, charting a blueprint for the future of personalized medical interventions.
Introduction
As the 21st century unfolds, nanotechnology emerges not merely as an innovative domain, but as a transformative force, laying the foundation for intricate nanoscale constructs imbued with unparalleled functional capabilities. Distinctively characterized by their size-dependent properties, these nano-architectures transcend the limitations of their macroscopic counterparts, forging path-breaking avenues across sectors – from catalysis and avant-garde electronics to the frontiers of biomedicine, advanced therapeutics, and precision biosensing. Central to this technological renaissance is the emergence of nanoparticles, which, propelled by rapid innovations, have established a formidable footprint in therapeutics and the broader spectrum of medicine. In this context, the intersection of nanotechnology with medical applications birthed ‘nanomedicine’,1 a novel paradigm poised to overhaul conventional diagnostic, detection, and treatment modalities, pushing the boundaries of medical science. Navigating this vast ocean of possibilities, green nanotechnology emerges as a beacon of sustainability and ecological consonance. It represents an overarching commitment to scientific pursuits hallmarked by safety, judicious energy consumption, minimalistic waste generation, and substantive reductions in greenhouse gas footprints.2 Anchored in the bedrock principles of green chemistry and engineering, this approach, symbolized by the epithet “green”, draws inspiration and raw materials from nature's vast repertoire, especially plant-based matrices. Green nanotechnology's core ethos revolves around the synthesis of benign, eco-affable nanomaterials, orchestrated under benign conditions, capitalizing on minimal energy and ideally, renewable resources. A distinctive hallmark of this eco-centric paradigm is its unparalleled precision in nanoparticle morphogenesis, courtesy of the plants' inherent capabilities as stabilizers and reducing agents. Notably, green nanoparticles, as empirical evidence suggests, demonstrate a superior therapeutic profile compared to their chemically derived counterparts.3
Facilitating this transformative synthesis are a plethora of water-soluble phytochemicals, encompassing flavones, tannins, quinones, and a spectrum of organic acids. Phytoconstituents, encapsulating nature's timeless wisdom, have graced therapeutic traditions since time immemorial. These plant-derived metabolites, ubiquitously distributed across the botanical landscape, serve not just as functional biomolecules but as powerful pharmacological agents. Many medicinal flora house metabolites endowed with potent anticancer and cytotoxic proclivities.4 Exemplifying this are bioactives like triterpenoids, polyphenolic flavonoids, and specific alkaloids, which, through a myriad of molecular mechanisms, exert antineoplastic effects, modulate cellular signaling pathways, reinforce DNA repair cascades, and invoke potent antioxidant responses.5 However, harnessing the full potential of these phytomedicines remains an intricate challenge. While many display profound in vitro efficacies, their inherent solubility patterns, absorption kinetics, and inability to effectively navigate lipidic barriers often impede their in vivo therapeutic prowess. Recognizing this, contemporary research has shifted its gaze toward integrating these extracts within nanostructured systems. The underlying postulate is compelling: encapsulating these bioactives within nano-constructs could amplify their therapeutic footprint, minimize untoward effects, and enhance overall efficacy.6 This review, in its essence, seeks to chart this confluence of traditional phytomedicine with modern nanotechnological innovations. What sets our discourse apart is the meticulous exploration of the novelty in green nanotechnology applications, especially in the context of therapeutic interventions, thereby presenting an enriched, holistic perspective for both researchers and practitioners.
Selenium nanoparticles (SeNPs)
The name selenium” (Se) comes from the Greek word “Selene”, which means “moon”. With an atomic number of 34, this element can be found in the periodic table. Swedish scientist Berzelius initially discovered selenium. Historically, Se was thought to be poisonous to humans. Scientists didn't learn that Se was an essential trace element until much later in the 1950s. Dietary Se is obtained from plants that utilize methionine as a protein precursor to transform inorganic selenium from the soil into selenomethionine.7 Se is a semisolid metal that resembles sulphur and tellurium chemically and looks as a red powder, a black vitreous material, and a metallic grey crystalline solid. The oxidation states of Se include 2+, 4+, 6+, 0+, and -2+. Selenium is a transition metal that appears in three forms: selenate SeO42−, selenide Se2, and selenite SeO32. Humans store selenium in the form of selenoproteins, which can be synthesised from either organic or inorganic selenium, as illustrated in Fig. 1. Absorption of elemental selenium is difficult unless it is nanosized; however, the digestive tract has no trouble absorbing organic selenium (mainly selenomethionine) or ionic selenium (e.g., selenite and selenate).8 Humans can safely consume organic food sources of selenium however, inorganic selenium supplied with chemicals has a limited therapeutic-to-toxic impact spectrum.9 To exert its physiological effects after being absorbed into the bloodstream, selenium forms a complex in vivo with several proteins (selenoprotein).10
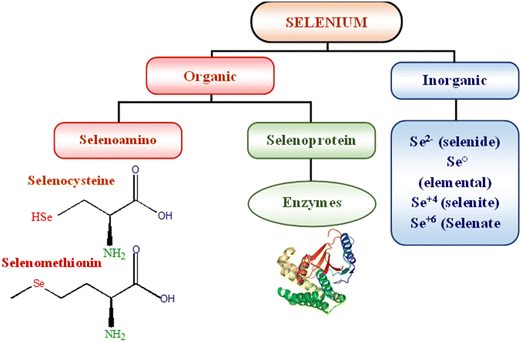 |
| Fig. 1 Various forms of selenium. | |
Physiological roles of SeNPs
SeNPs possess the unique qualities of being biocompatible and biodegradable, setting them apart from precious metals like platinum, gold, and silver. The toxicity of Se in its nanoforms is lower, and its anti-oxidant and anti-tumor potential is higher than those of its organic and inorganic analogs.11,12 Incorporating selenium into various organoselenium compounds (synthetic and natural) often results in beneficial pharmacological activity. The type of selenium linkage in an organic molecule significantly influences the biological activity of these compounds, i.e., whether they exhibit a beneficial effect (reactive oxygen species (ROS) uptake, oxidative capacity) or a toxic effect (ROS induction or release of elemental Se). Se-containing compounds can affect gene expression, cell signal pathways, DNA repair/damage, as well as angiogenesis and metastasis through the formation of ROS and the oxidation of protein thiol groups. Evidence revealed that these compounds induce antioxidant effects at low levels when selenium is incorporated into SePs, while pro-oxidant and anticancer effects occur at high doses. Some compounds have also been found endowed with chemosensitising properties, potentiating the efficacy of anticancer drugs.13,14
Both selenite (SeO32 SeL) and its sodium salt, sodium selenite (disodium selenite, Na2SeO3, SS) belong to a group of inorganic compounds, which were tested as the first Se-containing compounds, and the scope of research on their anticancer properties was very extensive. During the metabolism of SS in vivo, the formation of hydrogen selenide (H2Se) occurs which is then methylated forming methylselenol.25,26 The in vivo study concluded that SeL (3 mg per kg body weight (bw)) was well tolerated but was genotoxic, while at a concentration of about 127 μM Se, it was toxic and genotoxic to primary human keratinocytes (NHK).28 This is due to the fact that medium (3–5 ppm; 0.3–0.5 mg Se per kg bw) or dietary (0.1 ppm; 0.01 mg Se per kg bw) doses of SeL are metabolized to selenide (Se2−), which is then incorporated into selenoproteins. In turn, higher concentrations (>5 ppm; >0.5 mg Se per kg bw) of this compound lead to its reaction with oxygen and the formation of ROS, which causes oxidative stress, and this ultimately results in the cytotoxic and genotoxic effects.
Diselenides are a class of organic selenium compounds that contain the Se–Se bond in their structure and have the general chemical formula R2Se2. Dimethyl diselenide is a compound found in nature that exhibits antioxidant properties and strongly induces NADPH quinone oxidoreductase, whereas dipropyl diselenide and dibutyl diselenide are known for their prooxidant effects, even at low concentrations. In vivo studies revealed that diphenyl diselenide can be toxic, depending on its route of administration and dosage. Mainly, this compound was studied for its antioxidant activity, while there is less research on its cytotoxic effects on cancer cells. Another class of selenoorganic compounds is selenides (also called selenoethers), with the general formula R–Se–R. Among them, there are derivatives with chemopreventive properties, involving the binding of heavy metals or Gpx-like (glutathione peroxidase-like) activity. Meanwhile, the anticancer activity of the compounds was tested on various cancer cell lines, including colon, uterine, lung, liver, and breast cancer.15 In herbs and living systems, selenium plays a substantial role in biosynthesis; however, its toxicity surges above trace levels. Selenium, like selenoenzymes, can be easily incorporated into proteins (glutathione peroxidase). It has been hypothesized that it functions as an anti-oxidant enzyme, mitigating free radical damage to cells.16 Numerous inorganic Se, organic Se compounds and Se nanoparticles have shown promise as possible anti-cancer agents. It is thought that Se compounds, by virtue of their direct or indirect anti-oxidant qualities, play a significant role in the prevention of cancer by shielding healthy cells from the oxidative damage induced by reactive oxygen species (ROS) and thereby maintaining the cellular redox balance.17 Diseases including heart disease, cancer, rheumatoid arthritis, and cystic fibrosis benefit from selenium treatment. The toxicity of selenium oxyanions is reduced when it is present in its elemental form, but at higher amounts, it can be harmful to humans.18 Selenium is well known for its anti-oxidant properties; it is primarily used in metabolic disorders associated with oxidative stress, such as atherosclerosis, diabetes mellitus, and fatty liver. Selenoproteins are responsible for selenium's anti-oxidative stress action.19 Selenocysteine (SeC) and selenomethionine (SeM) are amino acids in proteins known collectively as selenoproteins. Somewhere around 25 unique selenoproteins have been identified in cells and tissues. Among the functionally classed selenoproteins, there is only one SeC residue in the structures of five glutathione peroxidases (GPx) and three thioredoxin reductases (TrxR). The most abundant selenoprotein identified in plasma is selenoprotein P (SEPP), which includes ten SeC residues. Many health problems linked with low selenium consumption are thought to be attributable to the absence of one or more specific selenoproteins, which is why selenium insufficiency inhibits the cell's capacity to synthesize selenoproteins.20,21 There is mounting evidence that selenium helps the immune and host defense systems do their jobs. The immune system and the host's defense mechanisms protect the body from harmful bacteria and viruses. The immune system may be significantly influenced by selenium.
Anti-oxidants like glutathione peroxidase (GPx) may protect neutrophils from damage caused by oxygen radicals produced during digestion. In order to prevent peroxidative damage to lipid-containing organelles and cell membranes, GPx relies on the presence of the selenium molecule. Together with vitamin E, it helps maintain healthy cell membranes by participating in redox processes that generate hydrogen peroxide with glutathione (GSH).22 The already harmful effects of redox by-products and oxidative stress on cell membranes are compounded by selenium deficiency. Acceptors of electrons are the SeO32− ions, whereas donors are enzymes like NADH dehydrogenase or other parts of electron transport chains. Selenium nanoparticles stand out for their intended use as ligands due to their high surface area per unit volume and high permeability.23,24 Nano-selenium is a low-toxicity antioxidant that boosts the activities of glutathione peroxidase (GSH-Px) and thioredoxin reductase. Since the nanoparticle's size affects its biological activity, it may be useful.25,26 SeNPs between 5 and 200 nm in size bind free radicals in a size-dependent manner. The majority of selenium nanoparticles are obtained through chemical reduction.27 A precursor containing an appropriate reducing agent can reduce it to an atomic structure. A typical green approach is selenate/selenite reduction in the presence of phytoextracts rich in polyphenols, flavonoids, alcohols, aldehydes, and amines to manufacture Se nanoparticles.28,29 As shown in, researchers are captivated by selenium nanoparticles (Nano-Se) because to its remarkable biopotential, enhanced biocompatibility, and reduced toxicity Fig. 2.30
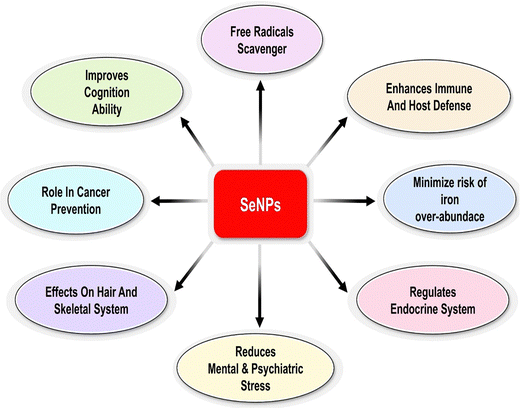 |
| Fig. 2 Biopotential of selenium nanoparticles. | |
Biocompatibility of SeNPs
Surface embellishments improve the biocompatibility of selenium nanoparticles. SeNPs derived from plants are considered superior to elemental selenium due to their biocompatibility.31 Physicochemical reaction parameters representing different surface Plasmon resonance bands and the ideal concentration of the plant extract used determine SeNPs' biocompatibility (SPR).32 The biocompatibility of Emblica officinalis fruit extract phytofabricated selenium nanoparticles (PF-SeNPs) was studied. Compared to sodium selenite, PF-SeNPs exhibited a much higher IC50 in N2a cells. Compared to sodium selenite, PF-SeNPs had a substantially smaller effect on mitochondrial membrane potential (MMP) and caspase-3. In definitive cytotoxic studies, PF-SeNPs were significantly less harmful and safer than sodium selenite.33 Thermosensitive nanocomposite hydrogels based on CS chitosan glycerol phosphate and their complex selenium nanoparticles were effectively fabricated, and the antioxidant characteristics and biocompatibility of hydrogels were examined in vitro (Wu et al. 2021). The CS/GP/SeNPs system, having optimal doses of SeNPs, exhibited potent antioxidative activity and excellent biocompatibility with GES-1 cells.34 Fang et al. (2017) coated SeNPs with folic acid and baicalin and was used to treat hepatocellular cancer (B-SeNPs-FA). Increased cellular uptake of folic acid is facilitated by the nano-incorporation systems of folic acid, which in turn increases the expression level of folic acid receptors (FARs) on the cell membranes of cancer cell types. Baicalin flavonoid is highly recommended for treating viral illnesses such as hepatitis B or cancer; nevertheless, its applicability is restricted due to low biocompatibility and hydrophobicity. To improve the properties of baicalin, selenium nanoparticles with exceptional biocompatibility, high stability, and minimal toxicity to healthy cells were included.35
Biodistribution and metabolism of SeNPs
The biodistribution of nanoparticles in normal mice is primarily determined by particle size and capping agent.36 Selenium is mostly absorbed in the small intestine's lower portion through various methods dependent on the selenium type. Se's biological activities mostly depend on this metalloid's incorporation into selenoproteins as the amino acid selenocysteine.20 Selenium nanoparticles (SeNPs) have drawn much interest as potential drug delivery systems. For starters, selenium (Se), a trace element, plays a significant role in many human physiological processes. Liver and kidney Se accumulations were observed in in vivo experiments using animal models treated with Se or SeNPs. Mechanisms and pathways governing the transformation of nano selenium within the body are shown in Fig. 3. According to Zhang et al.,37 selenium content in mice's blood, liver, and kidneys was not significantly different after 7 days of repeated administration of SeNPs or methyl selenocysteine at dietary levels (0.035 and 0.070 mg kg−1 bw per day). Se concentration increased in methyl selenocysteine-exposed rats when a supra-nutritional dose (1 mg kg−1 bw per day) was given. Loeschner et al.,38 quantifiable findings for the proportion of total Se concentration found in the liver, kidneys, and feces were displayed in their experiment. Results showed that Se was detectable in biological samples collected after administering SeNPs or Se(IV) at both dose levels. Se intake from feed was 0.03 mg kg−1 body weight per day, leading to a detectable Se level in the range of 5–10% in the control samples. Se was also found in the kidney and liver samples of rats given 0.05 mg kg−1 bw per day, but this was not different from the corresponding controls and was not related to the dosage form. This result demonstrated that the Se concentration was well within the capability of elimination by natural mechanisms, therefore minimizing the risk of organ toxicity. In contrast, rats given a tenfold higher dose, i.e. 0.5 mg kg−1 bw per day, had between 17 and 40% of their total Se concentration concentrated in their livers and kidneys, up from 2 to 7% in control and low-dose rats. Whether Se is given as Se-NPs or Se(IV), the varied physicochemical and microbiological conditions in the rat intestine's compartments may be affected differently after gavage delivery of the dose. The similar fraction of Se present and the identical concentration of Se discovered in the blood, and the organs after oral administration of Se as Se-NPs or Se(IV) suggest that the two chemical forms were metabolized along the same pathways. The reduction of Se(IV) to Se and the oxidation of Se-NPs to inorganic Se oxo-anions are conceivable in a microbial environment.39 A different study showed that Se-NPs have a nephroprotective role against the progression of acute kidney injury by ameliorating the histological alterations brought on by glycerol injection and restoring normal kidney biochemical characteristics. Se therapy decreased the alterations in kidney function measures and conserved the histological structure of renal tissue in a mouse model of acute kidney injury.40
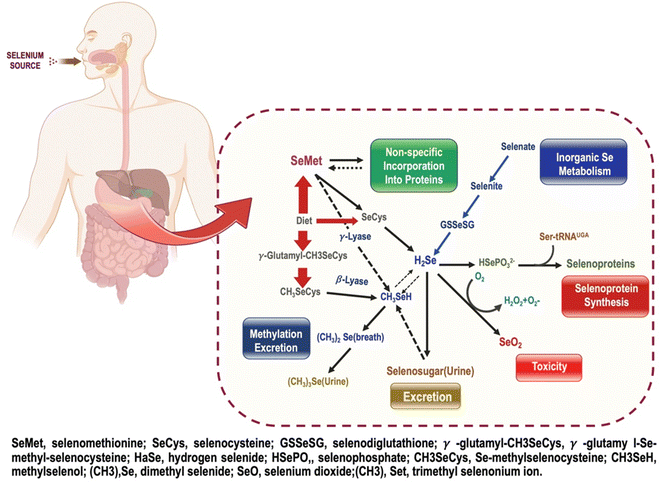 |
| Fig. 3 Mechanisms and pathways governing the transformation of nano selenium within the body. | |
Toxicity of Se-NPs
Se-NPs have a greater effect on organisms than inorganic Se forms. In addition, each individual's need for antioxidant defense determines how Se affects health status. Se becomes toxic if it is present in excess. Se toxicity in general and Se-NP toxicity have been assumed to be related. Both Se and Se-NPs possess pro-oxidative properties that produce reactive oxygen species (ROS) at higher concentrations. The bioaccumulation phenomenon may amplify this effect in various tissues, of which the liver is most susceptible.41 To yet, only antioxidant system function, body weight, and bioaccumulation in the liver, kidney, and heart have been given much attention in the toxicological examination of SeNPs. Very little is known about how SeNPs interact with the immune system, the gastrointestinal tract, bioaccumulation in muscles, and other indirect targets of Se. SeNPs and other nanoparticles seem more reactive and display better biodistribution in organisms than other forms of Se, likely because of their huge surface area and tiny size.42 Compared to inorganic Se, SeNPs are less harmful in most tests. Sublethal dosages of 20 nm SeNPs at 0.05, 0.5, or 4 mg Se per kg body weight (bw) per d showed no difference in brain neurotransmitters or hematological markers between the control and sodium selenite-treated groups (0.5 mg Se per kg bw per d) during a 28-day trial.43 A detailed toxicological evaluation revealed that SeNPs with a size range of 20–50 nm increased Se accumulation in whole blood, liver, and kidneys in a dose-dependent manner when compared to the control. It was found that SeNPs did not bioaccumulate more efficiently in blood and tissues at the dietary amount of Se (10 mg Se per kg bw). Plasma, liver, and kidney GPx activity did not differ between Se-methionine and SeNPs. SeNPs displayed less immediate liver injury and abridged toxicity compared to Se-Met. A reduction in dietary Se stockpile and a surge in the lethal dosage in SeNPs fed mice demonstrates the efficacy of employing SeNPs to avoid Se toxicity. The hypothesized mechanism operates through the cell's unique Se uptake and phase 2 response. Despite the varying toxicological effects of SeNPs, it has been stated that biologically or ecologically fabricated and altered NPs have enhanced the impact on the health of animals and abridged their toxicity. The technique of biogenic fabrication of SeNPs with bioactive chemicals appears to be their principal benefit with unique features.44,45
Drug delivery by selenium nanoparticles
Targeted medication delivery and regulated drug release are two advantages of functional nano-systems that can inspire novel therapeutic methods to treat disease. Due to their low toxicity, high bioavailability, and biocompatibility, Se nanoparticles can be conjugated with various agents for precise drug administration.10 The anticancer effects of SeNPs can be greatly enhanced since medications can be put into them at quantities that exceed their inherent solubility.46 Drug efficacy and safety were improved when selenium nanoparticles were combined with other treatments because of their enhanced selectivity for cancer cells, high bioactivity, increased drug solubility, and targeting effects.47 The selenium nanoparticles' surface chemistry must be modified so that they can be conjugated with ligands that precisely target cancer cells. Because of its ability to attach to a receptor on the surface of cancer cells, this complex could improve the selective uptake and accumulation of nanomaterial in tumor-containing organs while reducing toxicity to healthy cells. Conjugating various drugs to the nanoparticle's capping group results in a highly specific drug delivery mechanism.48 Consequently, the nanoparticles can function as drug-delivery vehicles. To a large extent, surface decorators aid cancer cell's uptake of nanoparticles by the cells themselves. Many therapeutic drugs can be successfully delivered to tumour sites that overexpress Tf receptors with the help of transferrin (Tf), a targeting ligand. However, cancers at either the primary or secondary stage could be targeted with a nanoparticle with transferrin conjugated.49 Using selenium nanoparticles coated with ATP improves their uptake and stability within cells and their selectivity for cancer cells over healthy ones. Nanomaterials are widely used as “nanocarriers” for chemotherapeutics due to their propensity to concentrate in cancer cells via a process known as passive targeting. Since the chemotherapeutics are being administered randomly, this technique has drawbacks. Targeted medication administration can take advantage of the fact that cancer cells have a significantly lower pH than healthy cells to limit the chance of off-target effects.50 The multivalent surface of SeNPs allows for efficient binding to chemical medicines or biomacromolecules via covalent or non-covalent linkages. In the field of medication delivery, SeNPs stand out as highly-tunable, adaptable nanocarriers. Naked stabilizers, or unreacted ions, are found on the surface of the SeNPs. These species can form conjugates with the amino, carbonyl, carboxy, and hydroxyl groups in pharmaceuticals and can be positively or negatively charged (API). Because of this, SeNPs have a greater adsorption capacity, making them better suited for drug loading, stabilisation, and delivery.51
Se-based nanoparticles formulation can be manufactured using two methods:
(I) Reduction-based in situ formation and (II) nanoparticle-based fabrication; in the first approach, selenium nitride nanoparticles (Se-Ni-NPs) are produced by a chemical reaction that uses various precursor materials, and reducing and stabilizing agents. Various selenium compounds, including sodium selenite, sodium selenate, and selenic acid, are present in the precursors. Reducing agents include ascorbic acid, mercaptoethanol, and glutathione sulphate, just a few examples. Inorganic nanoparticles can be employed for drug delivery thanks to stabilizers that facilitate the creation of physically stable colloidal SeNPs and bestow functionality on SeNPs. Due to the functionality stabilizers impart to SeNPs, inorganic nanoparticles can be employed for drug administration. In several steps of the manufacturing process, polysaccharides, proteins, and polymers are used to create selenium nanoparticles (SeNPs). These factors affect the physicochemical characteristics and bioavailability of selenium nanoparticles. Second, selenium-plated nanoparticles can be created by plating the surface of premade nanoparticles with selenium, which involves attaching selenium to the surface of the nanoparticles. Both methods have been shown in Fig. 4.
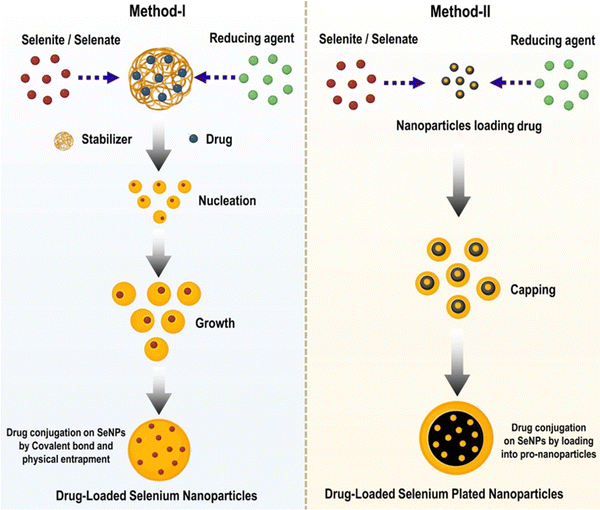 |
| Fig. 4 Techniques for the preparation of drug-delivering SeNPs and other Se-functionalized nanoparticles. | |
For the first tactic to succeed, the size of the SeNPs must be managed, and a suitable functional modifier must be chosen if the drug loading and pharmacokinetic characteristics are to be optimized. This can be done by controlling the amount of space between the SeNPs. The second strategy allows for flexible drug loading. The first strategy encapsulates the payload in nanoparticles. Many researchers are interested in SeNPs' potential as a nano-vehicle for transporting medicinal compounds for a synergistic impact. Because of their versatility and ease of application, selenium nanoparticles are gaining much attention in engineering. When ligands are added to SeNPs, they can be directed to certain cells or organelles. This technique can target cancerous cells and cell mitochondria. Nanomedicines, inspired by nanotechnology and used to treat mitochondrial dysfunction, is a major focus of precision medicine. Mitochondrial dysfunction is linked to cancer, CVD, diabetes, and other diseases.52–54
Synthesis of selenium nanoparticles (SeNPs)
SeNPs have a more complex chemical structure than standard organic or inorganic selenium compounds. Size, dispersion, content, shape, and surface property are just a few factors that must be considered while designing and synthesizing SeNPs for biomedical purposes. Functional agents, morphological features, and surface properties are important in designing novel SeNPs with variable size distribution.55 The traditional Se material in dietary supplements or additives presented significant absorption and bioavailability problems. SeNPs-containing nutritional supplements are advantageous because they increase the bioavailability and controlled release of selenium in an organism's body, thereby enhancing the efficacy and effectiveness of supplements. Sadly, a high selenium intake can cause a variety of fatal diseases. Controlling the therapeutic dose is crucial because selenium's toxicity and activity are typically altered at high doses. In addition, despite their excellent therapeutic potential, high amounts of methyl selenium, sodium selenite, and selenocysteine pose significant toxicity concerns. The aforementioned issues are linked to selenium levels that are too high. There may also be therapeutic uses for nanotechnology outside of the medical area. Nanoscale selenium has attracted much attention worldwide because of its potential as both a powerful therapeutic agent with low side effects in medicine and a food additive, particularly for selenium-deficient patients.56 To begin with, SeNPs, like other NPs, were manufactured by several chemical procedures. However, due to the high production cost and harmful by-products, new procedures for the synthesis of NPs have been developed.
Factors affecting synthesis of SeNPs
Principal factors in the production of SeNPs are reducing and stabilizing agents. Phenolics, tannins, saponins, flavonoids, sugars, amino acids, and proteins are the biomolecules in plant extracts known for medicinal value, which can also act as potential selenium-reducing agents. A stabilizer is often used to avoid the aggregation of nanoparticles by coating the particles with a thin layer of polymer or surfactant that reduces their interactions.57 The primary objective of selenium nanoparticle synthesis is the creation of particles with the smallest possible size and the highest possible stability.
Temperature, extract concentration, pH, time, agitation, and reactant concentration are a few variables that influence nanoparticle synthesis, as depicted in Fig. 5.32,58,59
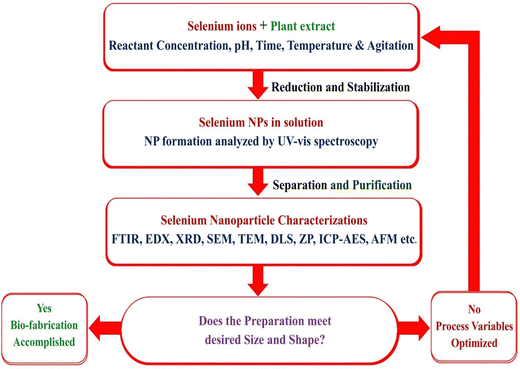 |
| Fig. 5 Flow chart for optimization of selenium nanoparticle fabrication process. | |
Effect of pH on the fabrication of SeNPs
A pH value aids in the measurement of a solution's acidity and basicity. The solution pH has a crucial role in plant-mediated nanoparticle biogenesis. The effect of pH on synthesizing SeNPs and other metallic oxide nanoparticles has been reported in the literature. Researchers have shown that the pH of the solution medium influences the crystal's size, shape, and crystallinity.60 This process is caused by creating nucleation centers, which increase as pH rises. The reduction of metal ions into metal nanoparticles occurs at higher nucleation centers. Simultaneously, the pH of a solution affects the activity of the functional groups in a plant extract or biomass and the rate of reduction of a metal salt. Changes in UV-vis spectra and solution color, surface plasmon resonance (SPR) peak intensity, and solution color depth were influenced by pH. With either low or high pH, the solution displayed a pale hue and weak SPR peaks.61
Some nanoparticles biosynthesized from plant extracts have been shown to have a size and texture highly sensitive to the pH at which they are created. In addition, pH variations were used to regulate the form and size of the nanoparticles.62 Alipour et al.63 reported that the medium's pH impacts the particle size and polydispersity index (PDI) of produced SeNPs. The particle sizes ranged from 136 to 190 nm, indicating that the nanoparticle sizes were comparable at pH 6, 7, and 8 but were much larger at pH 5. The PDI ranged from 4.2–16.6, with pH 6 and 7 exhibiting significantly lower values than pH 5 and 8.
Effect of plant extracts and concentrations on the fabrication of SeNPs
The volume and concentration of the plant extract and the kinds and numbers of biomolecules it contains have significant effects on the quality of the SeNP made from the extract. The concentration of plant extracts can affect the size of synthesized nanoparticles. Researchers have shown that increasing the concentration of an extract accelerates the bio-reduction and stability of nanoparticles, as more chemical ingredients are present in the solution to bond with the precursor. The ratio of plant extract volume to selenium precursor concentration needs to be optimized to produce SeNPs under ideal conditions.64 Alam et al.32 described the effect of leaf extract concentration on the synthesis of SeNPs by recording UV-Vis spectra. The spectra revealed that absorption intensity increases as the concentration increases and reaches its maximum. The increase in concentration causes a decrease in absorption intensity and an increase in absorbance max. The change in maximum sodium selenite concentration for the SeNPs was due to forming nanoparticles with a larger diameter. Maximum synthesis is achievable with a sodium selenite concentration of 25 mM. In order to determine the time of SeNPs synthesis, 5% leaf extract and 25 mM sodium selenite were incubated at a ratio of 1
:
9. In the UV-Vis spectra, a distinctive peak of selenium nanoparticles was seen at 381 nm as a function of time, showing that biosynthesis was complete in 3 hours. The two controls, sodium selenite in distilled water and leaf extract, lacked a 381 nm peak. Therefore, metal ion concentrations, reducing agent concentrations, and incubation times are crucial for synthesizing SeNPs.65 The quantity of extract used in the synthesis of SeNP has a significant impact on the yield. The research has stated that the amount and type of extract used in the synthesis of nanoparticles have a significant impact on their morphological characteristics and biological activities.66 When reducing agents are present in smaller amounts, nanoparticles grow in size.
Because the nucleation and growth processes are modified by the relative concentrations of the reducing agent and the precursor, this behavior is similar to that seen in several nano-system chemical reduction procedures. We hypothesize that the nucleation process is faster than particle development at greater concentrations, leading to smaller particles. To amplify this action, apply a strong reducing agent.67
Effect of time on the fabrication of SeNPs
An incubation time influences the morphological properties and qualities of the fabricated nanoparticles. A lengthy incubation period causes particle aggregation and contraction. Sani-e-Zahra et al.62 assessed the stability of SeNPs by utilizing UV spectrometry analysis. Their findings recorded the absorbance peak at 350 nm for SeNPs. Immediately after combining the plant extract and salt solution, the reaction mixture was incubated for three hours at a temperature of 75 °C before being cooled to 37 °C and incubated for 24, 48, 72, and 96 hours. Here stability of fabricated Solanum lycopersicum SeNPs was robust after 96 hours of incubation SeNPs yielded the highest peak at 350 nm. According to Alipour et al.63 the size measurements performed under various light exposure conditions, the development of SeNPs with a size of roughly 150 nm occurred under a 12 h dark/12 h light cycle, which is on par with the size formed under continuous light exposure. The formation of nanoparticles smaller than 200 nm took place during the other illumination cycles, the other hand. It demonstrated the importance of light exposure time in the fabrication of nanoparticles.
Effect of temperature on the fabrication of SeNPs
SeNPs and other metallic oxide nanoparticles are best created utilizing plant extracts at temperatures between 25 °C and 100 °C, as the temperature is one of the most significant variables in synthesizing metallic oxide nanoparticles. Since some of the secondary metabolites included in plant extracts are volatile, SeNP production often occurs at room temperature. The shape of nanoparticles can be altered by changing the reaction solution temperature. Plant-based, environmentally friendly synthesis of metal oxides at elevated temperatures has been established. However, the yield of nanoparticles is poor at high temperatures due to the potential inactivation of the biomolecules responsible for reducing the iron precursor. Akçay et al.68 reported that the influence of reaction temperature on the production of SeNPs was investigated by adjusting the pH of a supernatant containing 6.4 mM SeO2 to 9 and incubating the mixture between 30 °C and 45 °C. There was no change in the reaction solution colour at 45 °C, but a reddish-orange hue formed at temperatures of 30 °C, 33 °C, and 40 °C. In order to achieve maximal absorbance, the ideal temperature was determined to be 33 °C. According to Mollania et al.,69 the reduction of selenium ions may have been hindered by interactions between temperature (45 °C) and pH (9). In light of these findings, it is possible to conclude that an increase in temperature inhibits the formation of SeNPs.
Various methods for the fabrication of SeNPs
A variety of methods have been developed for the synthesis of SeNPs. The two most common types of these techniques are chemical reduction and biological reduction. When living things like bacteria or plant extracts are used to break down selenium compounds, both organic and inorganic, the resulting SeNPs are safe and useful. Chemical substances are used as reducing agents. The energy source or equipment used during the reaction can further categorize this method, as shown in Fig. 6. In this field, scientists have mostly documented using sonochemical, hydrothermal, and microwave techniques.70
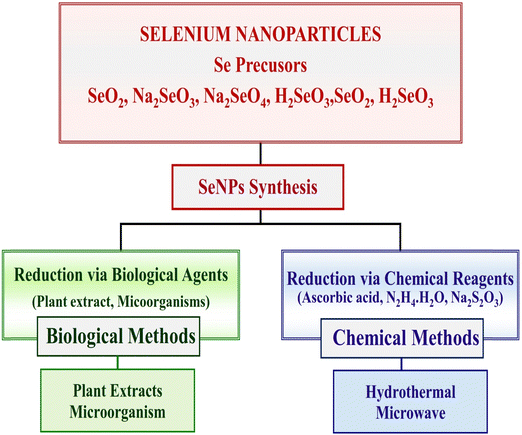 |
| Fig. 6 Various methods of selenium nanoparticles synthesis. | |
Chemical method of reduction for synthesis of SeNP
Reducing Se salts is the most widely used and straightforward approach for generating SeNPs. Sources that can lower the oxidation state include ascorbic acid and other reagents/chemicals. In contrast, it has been found that NPs manufactured from natural components are safer than those prepared with chemicals. To generate a stable colloidal solution of SeNPs for diverse applications, a chemical reduction is used to reduce the element, its salt, or compounds, and the size is regulated by applying surfactants or growth-terminating reagents.
Hydrothermal method of SeNP synthesis
The lack of the literature indicates that the hydrothermal method is not widely used to synthesize biologically compatible SeNPs. Shar et al.71 reported a simple hydrothermal method that uses ascorbic acid as a reducer and stabilizer and sodium selenite (Na2SeO3) as a precursor for creating SeNPs. The size range of the SeNPs that were obtained was 169.11 nm. The synthesis described is yet another illustration of an environmentally friendly synthesis. Hatami et al.72 a colloidal solution was hydrothermally treated in a laboratory autoclave at 121 °C and 1.5 atm for 15 minutes. The zeta potential of the synthesized SeNPs was 44.9 mV, and their size range was 110 nm. The polydispersity index was 0.226. The use of coffee bean extract to reduce Na2SeO3 to Se has been reported by Abbasian et al.73 the reaction that was completed in fifteen minutes at a moderate temperature. The Na2SeO3 was reduced using N2H6Cl2, and nanoparticles with a mean size of 15 nm were produced.
Microwave method of SeNP synthesis
The microwave approach is now widely employed in the chemical process of material production. The process is frequently referred to as a “green synthesis route” because it is quick, simple, affordable, clean, and produces a fair amount of the desired product. Microwave heating is more uniform and effective than traditional heating techniques that rely on conduction because the radiation interacts directly with the molecules. Because of these merits, scientists have begun employing this technique to synthesize SeNPs. However, microwave radiation for the preparation of SeNPs has received surprisingly little attention. As a reducing and stabilizing agent for generating SeNPs, the bean shells of Theobroma cacao L. (CBS) have been reported for the first time by Mellina et al.58 In order to find the best possible conditions for a reaction, the authors have developed a model utilizing a central composite design approach. Parameters such as time, power, and precursor quantity, i.e., Na2SeO3, Z-potential, and crystallite size, were entered for 23 samples. Using this model, we found that the best reaction conditions for producing particles with a diameter of less than 42 nm were 15.6 minutes in duration, 788.6 watts of power, 0.14 g of Na2SeO3, and 50 mL of a CBS extract solution. After two months of stability, the TEM images showed that the particles were consistently spherical, with a diameter of 1–3 nm. This work presents a new strategy for the microwave-assisted synthesis of SeNPs and highlights their industrial implications in the food and medical industries. Sheikhlou et al.74 has reported that the extract of the walnut tree leaves (Juglans regia L.) contains bioactive chemicals that can be used as a reductant and stabilizing agent for the manufacture of SeNPs. Two synthetic variables, the volume of selenium salt solution (15–25 mL) and the volume of walnut leaf extract (1–5 mL), were tested for their effects using the response surface methodology. Colloidal solutions containing SeNPs were measured for their broad emission peak (max) and absorbance. Using microwave radiation (800 W for 4 minutes) and 5 mL of walnut leaf extract, and 15 mL of selenium salt solution, SeNPs were successfully synthesized, as evidenced by their absorbance of 375 nm, the particle size of 208 nm, polydispersity index of 0.206, and zeta potential values 24.7 mV.
Plant mediated green synthesis of SeNPs
Researchers have paid great attention to developing environmental friendly methods for synthesizing metal NPs over the past 20 years. Researchers have used biological materials to their advantage to develop a cheap and eco-friendly process for producing metallic nanoparticles. In biological (green) synthesis, biological mass or extract is used to reduce metal ions within or outside the cell. Catalytic reactions in an aqueous medium at standard temperature and pressure, as well as the process's flexibility, as it may be done in almost any setting and on any scale, are two further advantages of the biological approach over conventional physical and chemical approaches. The main disadvantage of inorganic selenium compounds over SeNPs is that they are poorly taken up by cells. SeNPs need to be manufactured in a biological setting to maximize their biocompatibility and stability. The chemical-reducing and stabilizing agents needed for the above-mentioned techniques can be hazardous, ruling them out for usage in living systems. It is commonly accepted that biological agents derived from plant extracts are preferable to chemical techniques to address the rising demand for inexpensive, nonhazardous preparation methods. It is believed that bioactive compounds can operate as bio-reducers and nanoparticle stabilizers. Biogenic synthesis is simple because it requires no special instruments or a controlled environment. In this category of reagents, organisms include bacteria, fungi, algae, proteins, and plant extracts. An emphasis of the study was placed on their potential green NP synthesis. The following plant extracts were used in the production of SeNPs as an example of green synthesis: T. arjuna leaf extract, C. dentata plant leaf extract, A. sativum aqueous extract, and U. pinnatifida polysaccharide solutions. When compared to other biological approaches, the biosynthesis of nanomaterials from plant extracts offers greater benefits due to its low cost and lack unusual environmental requirements.75 To synthesize SeNPs, multiple physical and chemical techniques have been employed, requiring various chemical compounds and physical methods. Unfortunately, these technologies are expensive and result in the incorporation of harmful chemical residues limiting the therapeutic application potential of SeNPs. Compared to microbial enzymes, synthesizing SeNPs using plant extracts is safer, easier, and cheaper. As cited in Fig. 7. Table 1, a plethora of plant species has been successfully investigated for the synthesis of SeNPs.
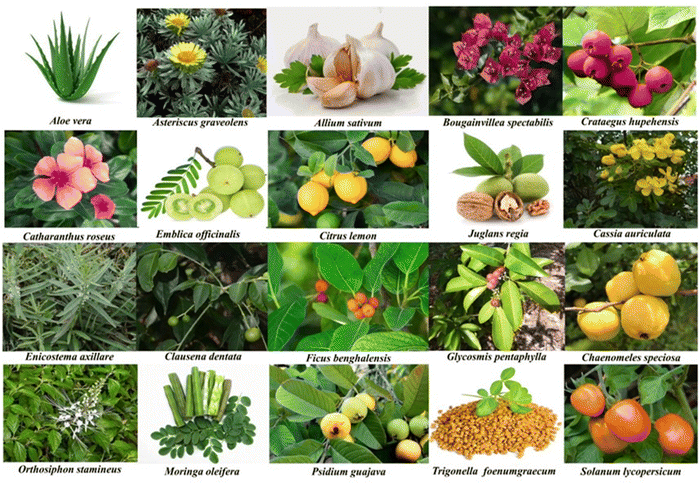 |
| Fig. 7 Plants parts utilized in green synthesis of SeNPs. | |
Table 1 List of plants utilized in green synthesis of SeNPs
Plant |
Common name |
Plant part |
Shape |
Size in nm |
Biological activity |
Ref. |
Azolla pinnata
|
Mosquito fern |
Entire plant |
Spherical |
30–40 |
Antioxidant |
76
|
Aloe vera
|
Aloe |
Fresh leaves |
Spherical |
7–48 |
Anticancer |
77
|
Aloe vera
|
Aloe |
Dried leaves |
Spherical |
121 |
Antimicrobial |
59
|
Asteriscus graveolens
|
— |
Aerial |
Spherical |
216 |
Anticancer |
13
|
Allium sativum
|
Garlic |
Buds, clove |
Spherical |
8–100 |
Antioxidant |
78
|
Bougainvillea spectabilis
|
Bougainvillea |
Flowers |
Spherical |
18–35 |
Antioxidant |
79
|
Crataegus hupehensis
|
Hawthorn |
Fruits |
Spherical |
113 |
Antitumor |
80
|
Catharanthus roseus
|
Vinca |
Flowers |
Spherical |
32 |
Anticancer |
81
|
Citrus reticulata
|
Orange peel |
Peels |
Spherical |
70 |
Antihelminthics |
82
|
Clausena dentata
|
Kadu karabevu |
Leaves |
Spherical |
46–79 |
Insecticidal |
83
|
Citrus limon
|
Lemon plant |
Leaves |
Spherical |
60–80 |
Protective |
84
|
Chaenomeles speciosa
|
Chinese quince |
Fruit |
Spherical |
80.5 |
Antitumor |
85
|
Cassia auriculata
|
Avaram |
Leaves |
Spherical |
10–20 |
Antileukemic |
57
|
Cassia auriculata
|
Avaram |
Flower |
Globular |
263 |
Antimicrobial |
86
|
Diospyros montana
|
Persimmon |
Leaves |
Spherical |
4–16 |
Antimicrobial |
87
|
Enicostema axillare
|
Whitehead |
Leaves |
Spherical |
98.18 |
Anticancer |
88
|
Emblica officinalis
|
Amla |
Fruit |
Spherical |
20–60 |
Antimicrobial |
33
|
Ficus benghalensis
|
Banyan |
Leaves |
Spherical |
45–95 |
Photocatalytic |
89
|
Glycosmis pentaphylla
|
Orangeberry |
Leaves |
Spherical |
56.21 |
Antibacterial |
90
|
Juglans regia
|
Walnut |
Leaves |
Spherical |
150 |
Antibacterial |
74
|
Leucs lavandulifolia
|
Lavender |
Plant |
Oval |
55–75 |
Antifungal |
91
|
Moringa oleifera
|
Drumstick |
Leaves |
Spherical |
25–35 |
Photocatalytic |
50
|
Orthosiphon stamineus
|
Java tea |
Leaves |
Spherical |
80–144 |
Cytotoxic |
92
|
Pelargonium zonale
|
Geranium |
Leaves |
Spherical |
50 |
Antibacterial |
31
|
Psidium guajava
|
Guava |
Leaves |
Spherical |
8–20 |
Antibacterial |
32
|
Solanum lycopersicum
|
Tomato |
Fruit seeds |
Spherical |
1155 |
Antimicrobial |
62
|
Tinospora cordifolia
|
Giloy |
Stems |
Spherical |
100–200 |
Anticancer |
93
|
Trigonella foenumgraecum
|
Fenugreek |
Seed |
Oval |
50–150 |
Anticancer |
94
|
Terminalia arjuna
|
Arjuna |
Leaves |
Spherical |
10–80 |
Antitoxin |
95
|
Theobroma cacao
|
Coca |
Bean shell |
Spherical |
1–3 |
Antioxidant |
58
|
Vitis vinifera
|
Resins |
Raisin |
Nanoballs |
3–18 |
Antioxidant |
96
|
Withania somnifera
|
Ashwagandha |
Leaves |
Spherical |
40–90 |
Antibacterial |
97
|
Biomimetic attributes of selenium nanoparticles
SeNPs are being evaluated for treating numerous diseases due to their superior qualities over Se. SeNPs enhance bioavailability while also reducing toxicity. Both prooxidant and anti-oxidant effects simultaneously open new lines of investigation in several clinical disorders. SeNPs exhibit antioxidant, antibacterial, antimalarial, anti-inflammatory, antidengue, antileishmanial, hepatoprotective, anticancer, antistress, immunomodulator, antiviral, and antidiabetic activity. The progress of targeted tactics and sophisticated applications of SeNPs is summarized below in Fig. 8.
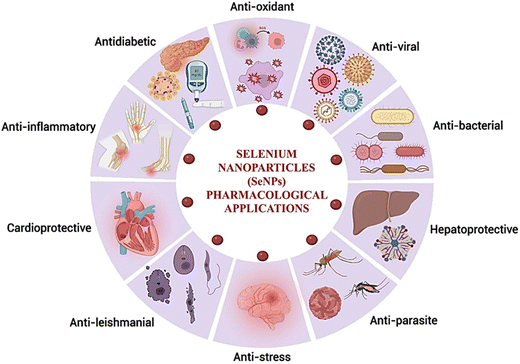 |
| Fig. 8 Pharmacological applications of selenium nanoparticles. | |
Selenium nanoparticles as a potential anti-cancer agent
Doctors and scientists today worry a great deal about cancer because it is one of the most devastating diseases of this century. Unfortunately, the situation has been made worse by the growing issue of drug-induced toxicity and resistance. Numerous therapy options exist in the fight against cancer, and various approaches are applied.98 The nanotechnology approach enables the design & synthesis of tailored medicine to enhance target-specific drug delivery, which obviates the toxicity of normal cells. Several inorganic nanoparticles, including SeNPs, have been shown to induce cytotoxicity in cancer cells.99 SeNP-based strategies have shown promise in overcoming drug resistance and reducing the side effects of chemotherapeutics. SeNPs as a vehicle for delivering chemotherapeutics to a specific site has the great potential.100 SeNPs have a disparity of effects on malignant and normal cells as depicted in Fig. 9.
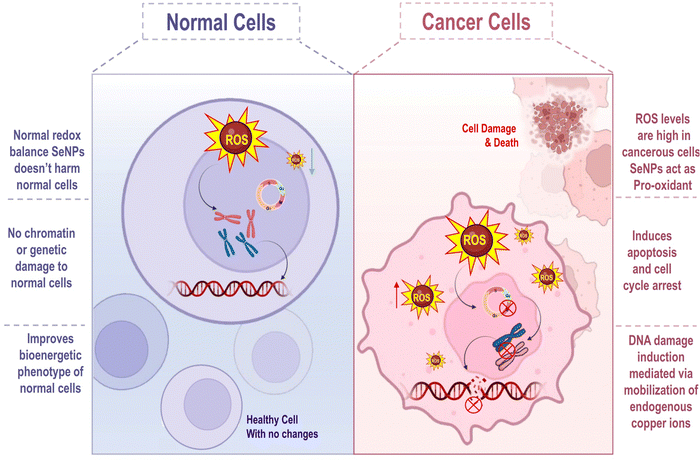 |
| Fig. 9 Mechanism for the effect of SeNPs on normal and cancer cells. | |
SeNPs have pro-oxidant activity within malignant cells, owing to the diseased cell's unique osmotic and redox states. Selenium has a regulated release profile with an increased cellular bioavailability in its nanoparticulate form. SeNPs exhibit less toxicity than their inorganic counterparts, even if the underlying mechanism of cellular death stays the same due to the significant distinction between malignant and ordinary cells. SeNPs' putative mechanism of action against numerous cancer types is depicted in Fig. 10.
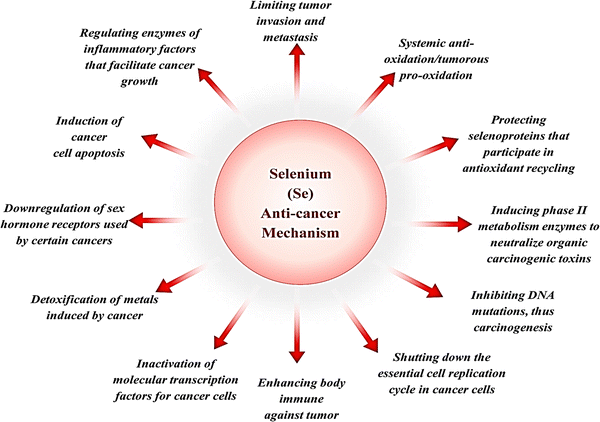 |
| Fig. 10 Putative mechanism of actions of SeNPs' against numerous types of cancers. | |
Selenium's anti-cancer processes and the modality of biological activities
The prooxidant conversion of SeNPs in a cancer cell microenvironment result in the production of additional free radicals, the disruption of the mitochondrial membrane, and the release of mitochondrial (Mt) proteins. Contrarily, this causes endoplasmic reticulum stress (ER). Several proteins are released when the mitochondrial outer membrane (Mt) is broken, and the cell death pathway caspase is activated. Multiple biochemical pathways, such as NFB, PI3K/Akt/mTOR, Wnt/β-catenin, MAPK/Erk, and apoptotic, become activated in response to this cellular stress state. Cells' Homeostasis is disrupted by inflammation signals and oxidative stress induced by the NF-B pathway. Because oncogenic signaling depends on the PI3K/Akt/mTOR, MAPK/Erk, VEGF, and Wnt/β-catenin signaling pathways, SeNPs can block these pathways and hence cellular proliferation, reducing growth-promoting signals in the tumour microenvironment. To add insult to injury, Fig. 11 shows that SeNPs can suppress angiogenic signaling in cancer cells, effectively stopping cell growth and proliferation. DNA damage occurs when these disruptive biological activities converge, causing a cell to stop dividing and die.101,102
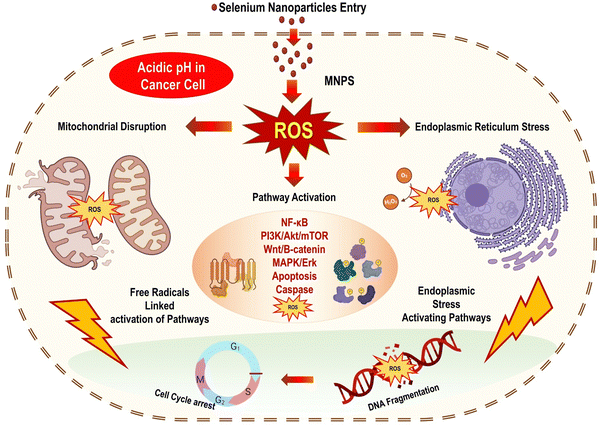 |
| Fig. 11 Putative mechanism of anti-cancer activity of SeNPs reproduced from Ikram et al.103 with permission from Dove Medical Press Limited 2021. | |
Cell apoptosis induced by selenium nanoparticles
Apoptosis, an active form of cell death, is a genetically regulated suicide mechanism that aids in the growth and protection of multicellular organisms. Apoptosis is another name for the progression known as automated cell death.104 Several studies have linked ROS production to the mitochondrial electron respiratory chain, both of which play important roles in the apoptosis process. In most cases, it has been discovered that SeNPs are capable of producing excessive ROS. This, in turn, can inhibit the electron transport pathway, leading to cell death. Since SeNPs cause an increase in intracellular ROS generation, these molecules can directly kill cancer cells by inducing apoptosis and stopping cell cycle progression, as shown in Fig. 12.105 Apoptosis can also kill cancer cells in two different ways: extrinsic and intrinsic. One way to initiate the extrinsic pathway is when a pro-apoptotic ligand binds to a death receptor and activates caspase 8.106 On the other hand, the intracellular caspase 9-mediated intrinsic mechanism can be triggered by cellular DNA damage or oxidative stress.
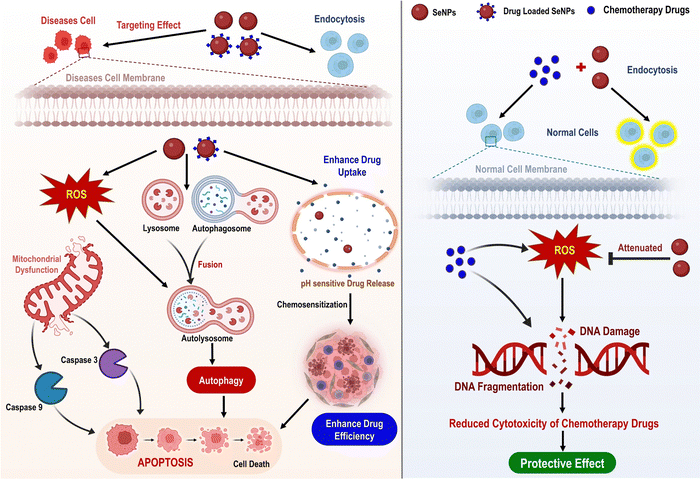 |
| Fig. 12 Fundamental biological functions of selenium nanoparticles, such as induction of apoptosis and autophagy, drug delivery, chemosensitization, and protective effects in chemotherapy reproduced from Lin et al.55 with permission from Frontiers 2021. | |
Excessive ROS production is responsible for Se-NPs' cytotoxicity, damaging DNA and triggering apoptosis.107 Most often, apoptosis triggered by selenium nanoparticles (Se NPs) is used to eliminate cancer cells.49 The production of reactive oxygen species (ROS), the down-regulation of anti-apoptotic genes, the up-regulation of pro-apoptotic genes, and the activation of caspases are all crucial signaling events in this process. Apoptosis is the most common form of natural cell death, and SeNPs, if internalized, can seemingly rapidly begin extrinsic signaling pathways, such as DISC/caspase-8/caspase-3 signaling. Meanwhile, the cell cycle analysis results demonstrate that Se-NPs can induce apoptosis dose-dependently, with the participation of G2/M phase arrest. The activation of the mitochondrial apoptosis pathway may also be facilitated by mitochondrial malfunction brought on by ROS overproduction, such as the disturbance of the mitochondrial membrane potential (MMP). An increase in cytochrome c, which triggers the activation of caspase-9, may result from an excess of reactive oxygen species (ROS). Caspases-9 acts as a crucial pro-apoptotic protein by stimulating the activation of caspase-3 in the cell's downstream pathway, leading to intrinsic apoptosis. SeNPs' high cytotoxicity toward cancer cells and low cytotoxicity toward normal cells could help suppress tumour growth in vivo.55
Selenium nanoparticles in cancer treatments
Al-Otaibi et al. (2022) established that MCF-7 cells were induced to commit suicide by SeNPs-apigenin, demonstrating that the cells would rather die than continue to grow in the lab. In order to detect apoptosis, flow cytometry and PCR were utilized. In order to determine the effects that SeNPs had on the cells, assays for cell migration and invasion were performed. Apigenin-SeNPs reduced the growth and viability of MCF-7 cells in cytotoxicity tests. Flow cytometry and PCR both demonstrated that SeNPs-apigenin brought on the death of MCF-7 cells. When SeNPs-apigenin are added to MCF-7 cells, they quickly begin to target Bcl-2, Bax, and caspase-3. This results in the DNA being damaged and the cells being killed. The cell death process began when cytochrome c migrated from the mitochondria to the cell's cytoplasm. The oxidative stress and ROS levels in MCF-7 cells increased when SeNPs-apigenin was added to the culture. According to the study's findings, SeNPs-apigenin can potentially be a cytotoxic agent for breast cancer.108 Chen et al. (2018) studied a new radiosensitizer, selenium nanoparticles (Nano-Se). This research examined how well nano-Se and irradiation work against MCF-7 breast cancer cells and what processes are at play. Radiation doesn't break down selenium nanoparticles, but it can increase intracellular Nano-Se toxicity by increasing selenium ion concentration. MCF-7 cells were irradiated and given Nano-Se. More cells were killed, autophagy was activated, the cell cycle was arrested in the G2/M phase, and more reactive oxygen species were produced in the combo therapy compared to irradiation alone. Autophagy was upregulated metabolically in stressful tumour mass regions early and late in tumour progression. To that end, the researchers hypothesized that the method used to suppress autophagy in this study might reduce tumour cells' ability to adapt to radiation treatment, raise tumour cells' susceptibility to metabolic stress and, ultimately, cause cell death and the suppression of tumour cell proliferation. These results indicate that combining Nano-Se with radiotherapy could enhance breast cancer treatment.109 Yang et al. (2021) Overproduction of free radicals in the body is associated with diabetes, which can cause tissue and cell damage, decreased autoimmunity, and increased tumour risk. Diabetes patients produce more free radicals due to high blood sugar and inflammation. SeNPs are a form of nanoparticle with antioxidant and anti-tumor activities. Also, metformin is commonly used to treat type 2 diabetes. So, this study used functionalized SeNPs and metformin to investigate a cancer treatment. Metformin and TW80-SeNPs synergize in MCF-7 cells. As illustrated in Fig. 13, this synergistic effect was achieved by inducing cell cycle arrest, upregulating p21 expression, downregulating cyclin-dependent kinases, and upregulating DNA-damage-related proteins such p-ATM, p-ATR, and p38. This is all due to synergy. Modifications to AMPK expression influenced mitochondrial membrane potential for a synergistic impact.110
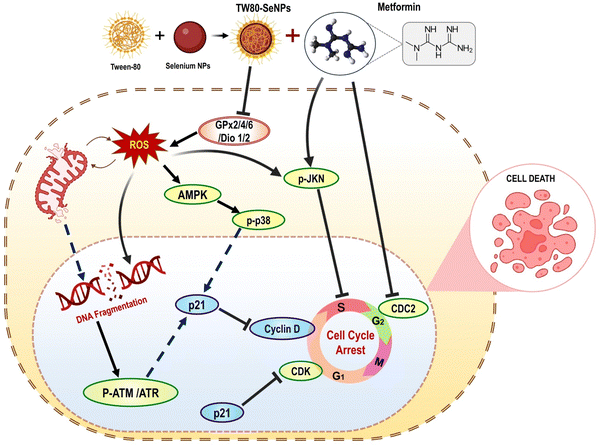 |
| Fig. 13 Mechanism for inhibition of breast cancer cell proliferation using SeNPs combined with metformin reproduced from Yang et al.110 with permission from Frontiers 2021. | |
Mary et al. (2016) elucidated thoroughly on the synthesis methodology and usage of PEG–SeNPs as a cancer-selective transport mechanism to heighten the anticancer potential. PEG–SeNPs were conjugated with Crocin extracted from dried Crocus sativus stigmas to optimize its therapeutic potential. PEG–SeNPs released Crocin, a promising cancer treatment, in a pH-triggered fashion. The in vivo anticancer efficacy of crocin conjugated PEG–SeNPs was assessed by treating nude mice implanted with A549 xenografts with different doses of the treatment. Upon the conclusion of 486 experiments, the mice were terminated, and the researchers obtained measurements of the tumors' mass and size. The outcomes indicate that the anti-proliferative effects of crocin conjugated PEG–SeNPs are dependent on the dose, as evidenced by the reduction in tumor volume and weight. Furthermore, the surface embellishment of crocin did not appear to influence the mineral side effect of PEG–SeNPs, as indicated by the lack of body mass reduction in nude mice. The outcomes demonstrate that PEG–SeNPs modified with crocin demonstrate a potent capability to stifle tumors in vivo. The PEG–SeNPs' cytotoxicity towards lung cancer cells significantly increased following the conjugation with Crocin. SeNPs' design presents a unique means of treating cancer by precisely targeting cancer cells resulting in diminished side effects and heightened effectiveness.111
Purohit et al. (2017) demonstrated SeNPs targeting thioredoxin reductase (TrxR), a selenoenzyme, could be a promising therapy for impeding tumor growth in cancer treatment. Target-specific DOX delivery was achieved hyaluronic acid (HA)-functionalized selenopolymeric nanocarriers Se@CMHA. The Se@CMHA-DOX NPs showed better cytotoxicity towards cancer cells than free DOX at the same concentration, selectively targeting cancer cells depicted in Fig. 14. One of the primary ways chemotherapy works is by inducing apoptosis, which involves regulating various cellular factors. To determine the necessary number of anti-apoptotic and pro-apoptotic proteins required for cancer cell death, strict regulation and conservation of Bcl-2 family proteins is crucial. The western blot analysis revealed that the Se@CMHA-DOX NPs induced apoptosis, leading to cell death, as evidenced by the expressions of tumor suppressor protein p53 and Bcl-2 family proteins. DOX's capacity to intercalate with DNA and augment ROS generation, leading to the destruction of cancer cells, is a widely acknowledged mechanism of its chemotherapeutic potency. The expression of Bcl-2 was suppressed, whereas pro-apoptotic proteins PUMA and Bax were upregulated in response to Se@CMHA-DOX NPs, as seen in the western blot results. This was attributed to the activation of the tumor suppressor transcription factor p53. As a result of these effects, the outer membrane of mitochondria was permeabilized, leading to the induction of Cyt c. Through translocation, Cyt c was transferred from the mitochondria to the cytoplasm. Western blot analysis showed Se@CMHA-DOX NPs induced p53 and increased PUMA and Bax. The NPs suppressed anti-apoptotic protein Bcl-2. Outer mitochondrial membrane permeabilization, induced by these effects, ultimately leads to Cyt c The cytoplasm relies on mitochondria to effectively translocate Cyt c. The activation of caspase-9 is initiated by releasing Cyt c from the mitochondria into the cytoplasm, which interacts with apoptotic protease activating factors. The classic apoptotic cascade involves procaspase-3 activation by caspase-9 cleavage, leading to nuclear changes and DNA fragmentation, ultimately resulting in cell death. The absence of caspase does not hinder the process of apoptosis, as AIF and Endo-G can activate this pathway. Chromatin condensation and DNA fragmentation occur when mitochondrial membrane permeabilization happens, which releases AIF and Endo-G from the inter-membrane space of mitochondria and Cyt c to the nucleus. MCF7 cells undergo caspase-independent apoptosis when exposed to Selenocysteine, an organic selenium compound that acts through AIF. The combination of DOX and Se NPs in the polymeric carriers led to a synergistic effect, with Se NPs progressively inhibiting TrxR activity and enhancing the anticancer efficacy of DOX. The molecular studies conducted on MCF7 cells showed that Se@CMHA-DOX NPs exposure induced p53 mediated caspase independent apoptosis and G2/M cell cycle arrest. A 3D tumor sphere model was used to evaluate the effectiveness of the developed nanosystem in reducing tumor activity in vivo settings. The results showed that our nanocarriers were highly effective in this regard. Se@CMHA-DOX NPs have shown promising results, indicating their potential as an effective cancer treatment modality.112
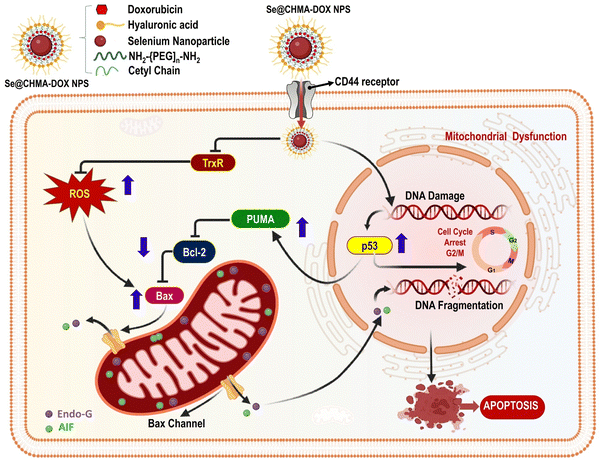 |
| Fig. 14 Se@CMHA-DOX NPs triggered apoptosis by internalization in MCF7 cells via the CD44 receptor reproduced from Purohit et al.112 with permission from American Chemical Society 2017. | |
Yazdi et al. (2012) reported Se-NPs stimulating an immunological response to 4 T1 breast cancer tumors in mice. To begin, 15 female BALB/c mice were split into two groups between the ages of 6 and 8 weeks. Before mice formed tumors, they were given selenium nanoparticles orally for two weeks. Each rat received (1 × 106) 4T1 cells subcutaneously. Nanoparticles were given orally once a day for three weeks to develop cancer artificially. Tumor development and survival were assessed in treated and untreated rats, along with immunological markers like cytokine level and DTH reaction. Selenium nanoparticles increased Th1 cytokine production in spleen cells. Cytokines include IFN- and IL-12. Statistically, test mice had higher DTH levels than control mice. Selenium nanoparticles increased the survival rate of mice compared to the placebo. This research reveals that selenium nanoparticles may enhance the prognosis of cancer-ridden mice by activating the Th1 platform of the immune response, which increases IFN- and IL-12.113 Shahverdi et al. (2018) synthesised folic acid derived selenium nanoparticles FA-SeNPs and SeNPs, tested it in vitro and in vivo against breast cancer. Both nanomaterials' cytotoxic effects were examined using 4T1 cells as the test subject. Another experiment examined the effects of weekly intravenous injections of these nanomaterials (at a concentration of 300 μg) on survival and tumor formation in mice genetically modified to develop cancer. Even though SeNPs had an antiproliferative impact on the cell line, FA-SeNPs were far more cytotoxic. When FA-SeNPs were present at a concentration equal to 8.75 μg mL−1 of elemental SeNPs (25 μg mL−1), almost 68% of the cells were killed. An in vivo trial showed that the nanomaterials were effective at slowing tumors' progression in cancerous mice compared to a control group. Significantly higher levels of efficacy were shown by FA-SeNPs when compared to SeNPs. The antiproliferative effects of SeNPs and FA on 4T1 cells are amplified when employed together, cell viability is greatly increased, and tumor development is avoided.114 Vekariya et al. (2012) created stable selenium nanoparticles (SeNPs) and determined how they inhibit breast cancer development. Sodium alginate was utilized with selenium acid and reduced glutathione to create SeNPs. Apoptotic markers (pp38, Bax, and cytochrome c) and cell survival were measured in MCF-7 and MDA-MB-231 breast cancer cells to determine the impact of SeNPs. Mammary tumor volumes were measured in dimethylbenz[a]anthracene-treated and untreated rats. The synthesis yielded SeNPs with diameters between 40 and 90 nm and storage stability of up to three months. It was discovered that ER-positive MCF-7 cells were more sensitive to SeNP-induced cell death and pp38, Bax, and cytochrome c expression than ER-negative MCF-7 cells (MDA-MB-231). The animals whose tumor volumes decreased the most had the lowest ER values (large tumor). For all they know, this may be the first hard evidence linking ER expression in breast cancer cells in vivo and in vitro with the anticancer effect of SeNPs.115
Selenium nanoparticles as an anti-oxidant
Anti-oxidants are crucial because they bind to free radicals and stabilize them, stopping their chain reactions and transforming them into innocuous byproducts.116 Anti-oxidants are important in treating free radical-induced degenerative illnesses because they can reduce oxidative stress.117 Nevertheless, plant-based nanoparticles have the potential as antioxidant functional groups, and these nanoparticles are more stable, have a more regulated release, and are more biocompatible. The increased nano selenium content of plant-based SeNPs is largely responsible for their effective antioxidant properties; this is because selenoenzymes, such as glutathione peroxidase, are stimulated, which in turn protects cells and tissues in vivo from free radical damage. SeNPs exhibit powerful anti-oxidant properties, ideal for shielding cells from the worst effects of reactive oxygen species (ROS), as shown in Fig. 15.118 Selenium, an essential ingredient of seleno-proteins, including thioredoxin reductases and glutathione peroxidases, protect cells from oxidative damage and may be responsible for the antioxidant regulating potential of plant-based SeNPs. Nanoparticles derived from secondary metabolites, like those found in plants, may have antioxidant properties due to functional groups on their surfaces. Plant-based SeNPs are excellent biocompatible replacements for synthetic anti-oxidants used as embedding agents in packaged food. To treat diseases including heart disease, cancer, and osteoporosis, where oxidative stress from reactive oxygen species (ROS) plays a major role in disease development, plant-based SeNPs show promise as therapeutic agents.119
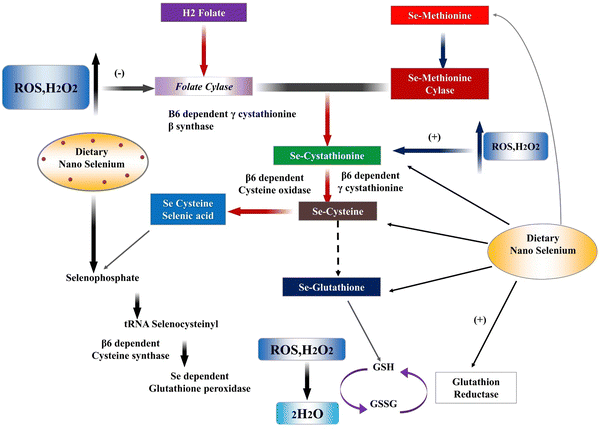 |
| Fig. 15 Schematic illustration of the probable anti-oxidant mechanism of SeNPs. | |
Selenium nanoparticles as anti-microbial agent
The development of antibiotics in the early twentieth century made it possible to be used as the first line of defense against bacterial infections of many types, both Gram-negative and Gram-positive. Most antibiotics work by interfering with the ribosome's ability to translate RNA into proteins, effectively slowing or stopping the growth of bacteria.120 The side effects of antibiotics are diverse and can occur from taking one to taking them all. The endosymbiotic theory states that the mitochondrion is a bacterial organelle with almost comparable structural and molecular components of the protein expression system.121 Different antibiotics trigger myopathies in the mitochondria because of the structural similarity between mitochondrial and bacterial ribosomes. Mutations and metabolic changes in the host cell and the production of harmful reactive radical species can be triggered by the bactericidal doses of antibiotics.122 Derivatives of selenium, such as selenium sulphide, are commonly used in the medical industry to treat microbial infections since selenium (Se) is a powerful antibacterial agent. Since its toxicity is a major concern, scientists have been working hard to find ways to improve selenium's biofunctional qualities while simultaneously decreasing its toxicity. In this case, nanotechnology has supplied the most secure technique for reducing selenium's toxicity and increasing its bio functionality via green production.123 Phyto-SeNPs successfully overcome elemental selenium due to their superior antibacterial activity and biocompatibility. Plant-based SeNPs are known to be effective against both Gram-positive (Staphylococcus aureus) and Gram-negative (Escherichia coli, and Klebsiella pneumonia) bacteria; however, it appears that SeNPs are more effective against Gram-negative bacteria due to their thinner peptidoglycan cell wall.124 SeNPs display antibacterial activity attributed to two mechanisms: oxygen radical production and membrane permeability impairment, as depicted in Fig. 16.125
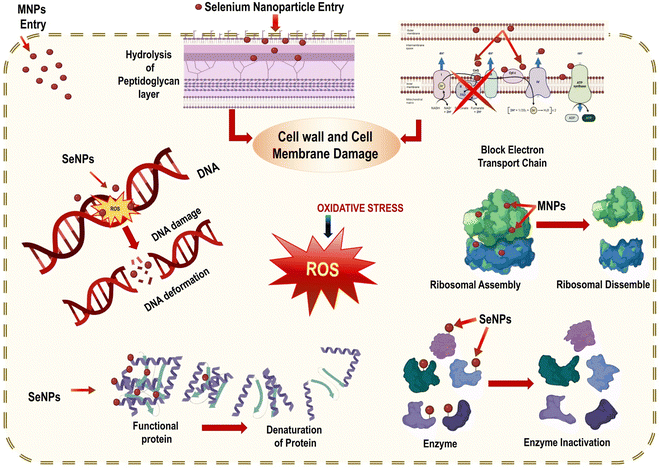 |
| Fig. 16 Mechanism of anti-microbial activity of SeNPs reproduced from Ikram et al.56 with permission from Dove Medical Press Limited 2021. | |
Additionally, plant-mediated SeNPs impede microbial growth by adhering to the cell membrane, which disrupts DNA replication, the metabolic cycle, and the protein synthesis cycle. After reaching the membrane, SeNPs bind to the thiol or sulfhydryl groups in membrane proteins, leading to denaturation and, eventually, cell death. However, the mechanism by which SeNPs generated from plants exert their antibacterial effects is still unclear.126 The nanosize of SeNPs and biological properties confer the ability to be hazardous to infections via functional groups on nanoparticles that aid in crossing the plasma cell membrane of bacteria. The total charge on nanoparticles and their electrostatic interactions with cells dictates their toxicity and biocompatibility, as demonstrated in Fig. 17.127 Previous research demonstrated that nanoparticles are biocompatible at low doses. On the other hand, an increased dose of nanomaterials poses grave dangers to the host cells.128
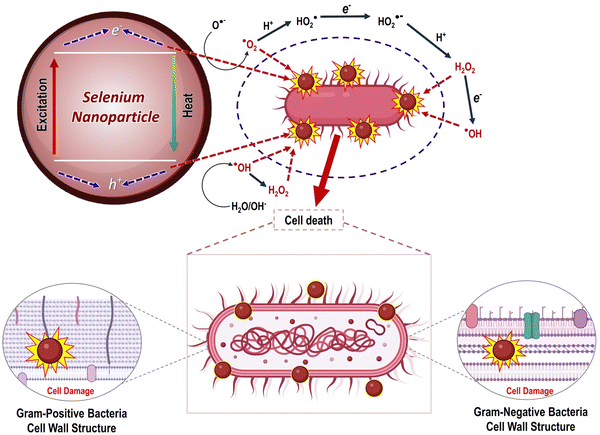 |
| Fig. 17 Mechanism of antibacterial activity of SeNPs reproduced from Cittrarasu et al.129 with permission from Springer Nature 2021. | |
Nanomaterials' distinctive physicochemical features have expanded their applications in cancer research, particularly in radiotherapy, chemotherapy, and integrated cancer detection and treatment. The trace element selenium has recently received much attention due to its beneficial health effects, especially concerning the immune response and cancer prevention. Liver, breast, prostate, colon, and lung cancers are a few of the many diseases linked to low selenium levels, and the results of epidemiological, pre-clinical, and clinical investigations have all shown that selenium can lower cancer risk.130 Scientific investigations confirmed that cancer patients' plasma selenium levels dropped significantly during radiation.131 The anti-cancer effects of molecular selenium compounds such as sodium selenite, methyl-selenocysteine, and seleno-methionine are enhanced at large doses.132 Although selenium has anti-tumor and necessary microelement properties, its clinical utility is severely constrained because its effective dose is so close to the hazardous range. Selenium nanoparticles may be a suitable replacement for selenite (Se2+ or Se4+) ions within the realm of nutritional supplements and medicinal dosage forms since there is less proof of their toxicity at nano-size compared to elemental selenium (Se).133
Selenium nanoparticles as anti-diabetic agent
Diabetes, a metabolic disease that has reached epidemic proportions, can have a debilitating effect on the quality of life of those affected. According to the World Health Organization, around 1.5 million people die every year due to diabetes worldwide, and the number of cases is expected to reach 366 million by 2030. The development of diabetes is influenced by multiple factors including unhealthy food habits, stress, low physical activity, obesity, inflammation, genetics, and age. To control diabetes and its serious complications, it's crucial to monitor blood cholesterol, glucose levels, and blood pressure. A balanced diet and regular exercise are also essential. People with diabetes rely on insulin, a protein hormone, which is usually administered through subcutaneous injections. While the daily insulin injections are effective, they can cause discomfort, local infections, and pain. Furthermore, they can lead to the deposition of fat at the injection site, hypertrophy, and trypanophobia.56 Plant-based SeNPs are widely accepted as a non-toxic alternative for managing diabetes, making it one of the deadliest diseases to control. The STZ-induced diabetic rats were observed to have reduced oxidative stress when treated with SeNPs synthesized from Hibiscus sabdariffa leaf extract. The bacterium, Streptomyces achromogenes, is the source of streptozotocin, an antibiotic drug that works against a wide range of bacteria. Streptozotocin is also known to be a pancreatic beta-cell specific cytotoxin so that's why it is used to induce diabetes in the rodent models during the experiments. In rats, exposure to STZ results in increased levels of malondialdehyde (MDA) and nitric oxide (NO), while also decreasing the antioxidant potential of CAT, SOD, GR, and GPx. Acting on the specialized GLUT 2 receptors, Streptozotocin competes with glucose molecules, ultimately leading to Akt phosphorylation. Moreover, STZ prompts programmed cell death and cellular damage by augmenting the production of ROS and NOS. By lowering the antioxidant potential of CAT, SOD, and other substances, STZ can cause oxidative stress. This, in turn, leads to a decrease in testosterone levels, mitochondrial cleavage, and DNA fragmentation. The process ultimately results in the apoptosis of the cell. After being created through phyto-fabrication, SeNPs were internalized through receptors mediated endocytosis. This process helped to increase the antioxidant potential of CAT, POD, and serum testosterone, while reducing the production of ROS and NOS, ultimately leading to an increase in lipid levels in STZ induced rats (Fig. 18).
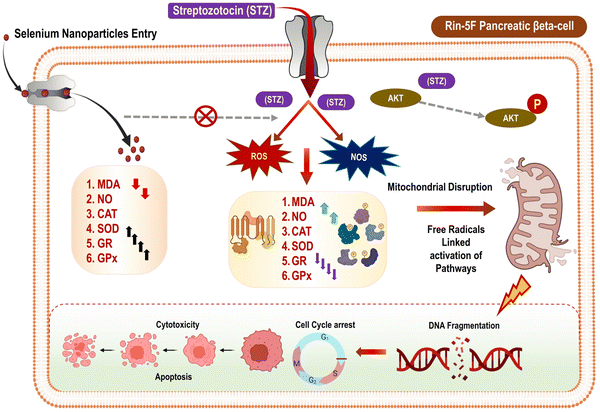 |
| Fig. 18 Mechanism of antidiabetic activity of SeNPs reproduced from Ikram et al.56 with permission from Dove Medical Press Limited 2021. | |
Clinical significance of selenium nanoparticles
Vundela et al. (2022) tested C. papaya extract as a starting material for selenium nanoparticle (SeNP) production and analyzed the resulting SeNPs' biological effects. Here, the components of a fruit extract from C. papaya acted competitively to decrease nanoparticle size and stabilize them. As measured by the DPPH and ABTS assays, the synthesized SeNPs displayed strong antioxidant activity, with EC50 values of 45 ± 2 and 43 ± 4 μg mL−1, respectively. It was discovered that SeNPs had a broad-spectrum antibacterial action, inhibiting microorganisms in the following order: fungi > Gram-positive bacteria > Gram-negative bacteria. These SeNPs inhibited the development and OTA production of the mycotoxigenic fungi Aspergillus ochraceus and Penicillium verrucosum. When tested on multiple cell lines, the SeNPs were found to be biocompatible and slow the growth of cancer cells without harming healthy cells. SeNPs were found to be less toxic to Danio rerio (zebrafish) at a concentration of 50 μg mL−1, and they did not result in the death of embryos. Thus, it may be useful for biological determinations to infer a lower dose of SeNPs.134 Al-Otaibi et al. (2022) attempted to create apigenin SeNPs (SeNPs-apigenin) using green synthesis and evaluated their anti-breast cancer activity. Multiple modified assays confirmed that SeNPs can inhibit MCF-7 cell viability and induce apoptosis in vitro. Flow cytometry, polymerase chain reaction, and cell migration and invasion tests were employed to assess the possible effect of SeNPs-apigenin treatment on these activities. In a concentration-dependent manner, the results suggested that treatment with SeNPs-apigenin could effectively suppress MCF-7 cell proliferation and survival. The treatment of MCF-7 cells with SeNPs-apigenin induced apoptosis, as shown by flow cytometric and polymerase chain reaction analyses. Targeting Bcl-2, Bax, and caspase-3 with SeNPs-apigenin could lead to the release of cytochrome c from the mitochondria into the cytosol and the initiation of cell death in MCF-7 cells. Damage to DNA and cell death are the inevitable outcomes. In addition, MCF-7 cells treated with SeNPs-apigenin showed increased levels of reactive oxygen species production and oxidative stress.108 Perumal et al. (2021) fabricated of SeNPs utilizing the E. axillare leaf extract. Possible dangers of nanotoxicity were investigated by determining the median lethal concentration (LC50) and Na+/K+-ATPase activity in zebrafish. With rising SeNPs concentration, the LC50 and Na+/K+-ATPase activity appear to drop considerably. It was also determined whether or not SeNPs had any bactericidal effect on several microorganisms. SeNPs may inhibit cell proliferation dose-dependent, as demonstrated by their cytotoxicity against human lung cancer cells (A549). The first study reported green fabricated selenium nanoparticles with antibacterial and anticancer activities utilizing E. axillare.88 Anu et al. (2020) demonstrated a green method for synthesizing selenium nanoparticles and functionalizing them as an anti-leukemia nanomaterial using Cassia auriculata. Selenium nanoparticles and their anticancer potential have been investigated in vitro via anti-leukemia activity. The study demonstrates nuclear morphological labeling in leukemia cells as a consequence of Se-NPs therapy, with the effects being mostly dose-dependent. Se-NPs triggered apoptosis in leukaemia cells, with early apoptotic cells appearing a vivid green and condensed, fragmented nuclei appearing a deep red. These results demonstrate unequivocally that the newly discovered selenium nanoparticles exhibit anti-cancer activity. Newly synthesized selenium nanoparticles from C. auriculata leaf extract were tested for their effect on HL60 leukemic cancer cells and vero cells using the MTT assay. The technique used to measure cell viability took 120 hours to complete. Leukemia cells were subjected to dose-dependent cytotoxicity when exposed to selenium nanoparticles produced from C. auricula leaf extract.57 Alagesan et al. (2019) fabricated SeNP by combining selenious acid and W. somnifera leaves extract. Green synthesized biogenic Se-NPs possess notable antioxidant activity and substantial antibacterial activity. SeNPs' antiproliferative efficacy was particularly strong against A549 cells (IC50 at 25 μg mL−1).97 Menon et al. (2019) attempted bio-fabrication of Se-NPs using Z. officinale, and investigated their antibacterial and antioxidant activity. The fabrication of SeNPs was validated by the colour shift from light yellow to red. To investigate its antimicrobial activity, pathogens Klebsiella sp., Pseudomonas sp., Escherichia coli, Staphylococcus aureus, and Proteus species were compared. Because selenium nanoparticles (SeNPs) were effective against Proteus sp., the growth curve of a Proteus sp. bacterial isolate treated with SeNPs was also assessed. To kill Proteus sp. bacteria, a minimum concentration of about 250 μg mL−1 of SeNP was found using the MIC method. The DPPH test was also used to evaluate antioxidant activity.135 Gunti et al. (2019) utilized an aqueous fruit extract of Emblica officinalis to fabricate selenium nanoparticles (PF-SeNPs). PF-SeNPs have many interesting bio-applications, such as antioxidant, antibacterial, and high biocompatibility. In addition, they showed free radical scavenging activity that increased with dosage in the DPPH and ABTS tests. The PF-SeNPs showed potent antibacterial activity against a wide variety of food-borne pathogens, particularly fungus, then Gram-positive and Gram-negative bacteria. The biocompatibility of PF-SeNPs was evaluated using N2a cells; their IC50 value was much greater than that of sodium selenite. Both mitochondrial membrane potential (MMP) and caspase-3 were less affected by PF-SeNPs therapy than sodium selenite administration. The cytotoxic experiments show that PF-SeNPs are safer and less harmful than sodium selenite. Experiments on cell viability showed that PF-SeNPs were significantly less toxic and safer than sodium selenite.33 D. Cui et al. (2018) used aqueous hawthorn fruit extract (HE) to synthesize SeNPs, which were then tested for anticancer efficacy and potential mechanisms of action in several cell-based assays. After treating HepG2 cells for 24 hours with HE-SeNPs at 0, 5, 10, and 20 μg mL−1, cytotoxicity was measured. Annexin V-FITC/PI labeling was used to detect apoptosis in HepG2 cells. In addition, intracellular reactive oxygen species (ROS) levels were measured, and the mitochondrial membrane potential (MMP) was calculated. The expression levels of caspase-9 and Bcl-2 proteins were then assessed through western blotting. The IC50 and ED50 values for HE-SeNPs showed considerable anticancer activity against HepG2 cells (19.22 and 5.3 μg mL−1, respectively). HE-SeNPs caused mitochondrial dysfunction and intracellular oxidative stress, leading to the death of HepG2 cells. Therefore, HE-SeNPs might be worth exploring further as a possible chemotherapeutic treatment for human liver cancer.80 Sivakumar et al. (2018) synthesized Se Nano balls using an aqueous extract of Java tea leaves (Orthosiphon stamineus). L6 rat skeletal muscle cell lines were used to test the cytotoxic potential of SeNPs. The L6 rat skeletal muscle cell line was shown to be an effective target for the cell-killing properties of selenium nanoparticles.92 Alam et al. (2018) reported the synthesis of SeNPs utilizing aqueous sodium selenite (Na2SeO3) in combination with an alcoholic extract of guava (Psidium guajava) leaves. To kill bacteria, the SeNPs were effective against both Gram-positive and Gram-negative organisms. Changes in the membrane of bacterial cells by SeNPs were seen using SEM. Fluorescence microscopy was used to investigate the bactericidal impact. The MTT test against HepG2 and CHO cell lines determined the cytotoxicity. They were reported to be effective against hepatocellular carcinoma cell lines and are speculated to be employed as efficient antibiotics against a wide range of bacterial strains.32 Ezhuthupurakkal et al. (2017) synthesized SeNPs from an aqueous fraction of Allium sativum (AqEAS) spherical-shaped crystals of SeNPs. The impact of SeNPs with a CT-DNA stacked base pair on intercalation and groove binding was evaluated in this study. This study's findings on the low cost and long half-life of SeNPs and the complex mechanism of DNA binding have implications for the development of tailored chemotherapies.136 Kokila et al. (2017) attempted to synthesize Se-NPs using Diospyros montana bark extract by applying a simple precipitation technique. Se-NPs' cytotoxicity was evaluated by an assay using human breast carcinoma cells (MCF-7). In cases where the anticancer properties of Se-NPs might dose-dependently impede cell growth.87 Nie et al. (2016) presented uniform SeNPs (Glu-SeNPs) synthesized using glucose as a reductant and
surface decorator, which were shown to be effective in causing cancer cell death. At a high temperature (115 °C), glucose was used to decrease sodium selenite (Na2SeO3), and it also worked as a surface decorator for SeNPs, keeping them from aggregating in an aqueous solution and so boosting their stability under physiological conditions. The functionalized NPs showed excellent hemocompatibility and cytotoxicity against several human cancer cells but not normal cells. This was accomplished by inducing cell death through both intrinsic and extrinsic pathways. Internalized Glu-SeNPs rapidly and substantially upregulated intracellular ROS production and mitochondrial malfunction, hence regulating cell fate, as shown by the mechanism of action. This work demonstrates a novel and efficient approach to easily synthesizing SeNPs with high anticancer activity. Where it can be easily speculated as the cancer cells continually differentiate and require a high amount of glucose, Glu-SeNPs can promise tumor-specific accumulation compared to normal cells.137 Prasad et al. (2014) synthesized Se-NPs from T. arjuna leaf extract. The subsequent impact of Se-NPs on arsenite-treated mammalian lymphocytes was investigated.84 Experiments on cell viability (MTT assay) and DNA damage (XTT method) showed that Se-NP mitigated As(III)-induced cytotoxicity and DNA damage.95 Sharma et al. (2014) revealed an environmentally friendly method for producing biogenic Se-NPs from Vitis vinifera (raisin) extracts.30 The Se-NPs characterization confirmed the existence of a steady lignin biopolymer on the exterior of selenium nano balls, indicating the potential role of phytomolecules as a capping agent.96 Ramamurthy et al. (2013) reported Trigonella foenum-graecum seed extract mediated SeNPs by a simple natural procedure. This study synthesized SeNPs of about 50–150 nm under a controlled environment. SeNPs' cytotoxicity was tested in vitro and in vivo on human breast cancer cells (MCF-7). Based on the results of this study, combining SeNPs with doxorubicin improves their anticancer effects and their ability to inhibit cell growth in a dose-dependent manner.94 Huang et al. (2013) reported that SeNPs, combined with 5-fluorouracil, enhance anti-tumour outcomes on various cell lines like A375 human melanoma cells.138 Prasad et al. (2013) reported lemon plant leaf extract mediated synthesis of SeNPs. In this case, SeNPs increased cell viability and decreased DNA damage in human lymphocytes exposed to UV light.84 H. Wu et al. (2007) fabricated SeNPs using mushroom polysaccharides protein complexes (PSP) as a capping agent, and their production greatly slowed the expansion of the MCF-7 cell line.139
Challenges in the optimization and translational application of selenium nanoparticles (SeNPs)
The burgeoning realm of Selenium nanoparticles (SeNPs) has profoundly highlighted the transformative potential of nanomedicine in reshaping the healthcare landscape. As we stand at the nexus of innovation and translational applications for SeNPs, a spectrum of multifaceted challenges presents itself, demanding rigorous attention, comprehensive assessment, and strategic innovation.140
Biodistribution, clearance, and toxicity.
One of the foremost challenges encapsulates the critical need to meticulously fine-tune SeNPs' biodistribution, clearance, and toxicity profiles. The established phenomenon of the enhanced permeability and retention (EPR) effect, as observed with SeNPs, amplifies the urgency of refining tumor targeting mechanisms, striving to harness this effect for enhanced therapeutic efficacy.
Delivery efficiency and clearance mechanisms.
A pivotal concern that necessitates redressal pertains to SeNPs' sub-optimal delivery efficiency, which, in its current state, impedes their full translational potential. The predominant mode of SeNP clearance, steered via the hepatic and splenic pathways owing to their inherent size, raises pertinent questions about potential long-term toxicities associated with such clearance trajectories.
Synthesis and standardization.
Embarking on the path of SeNP synthesis, achieving scalability without compromising particle uniformity and maintaining rigorously controlled surface properties, remains a formidable challenge. Any variability in parameters like size, surface coating, and overarching physicochemical properties can wield a disproportionate influence on SeNPs' in vivo behavior, thereby impacting their therapeutic index. Consequently, championing a standardized, reproducible synthesis methodology becomes imperative for ensuring consistent translational outcomes.
EPR effect and clinical trials.
As we navigate the clinical trial landscape, the EPR effect, despite its potential, remains a conundrum. While various trials have endeavoured to evaluate SeNP-based drugs leveraging this effect, empirical data on its efficacy, especially concerning smaller tumor lesions or metastatic sites, remains inconclusive, necessitating further exploration.
Surface modifications and molecular targeting.
Diving deeper into the molecular intricacies of SeNPs, it becomes evident that their surface topography and molecular architecture are not mere structural attributes but pivotal determinants of their pharmacokinetics and biodistribution. To optimize this in vivo performance, integrating molecular targeting ligands emerges as a strategic imperative, facilitating enhanced tumor specificity and precision in therapeutic delivery, especially when validated using human-derived tumor model
Metabolism and renal clearance.
The current generation of SeNPs, characterized by larger sizes and specific charge configurations, poses inherent challenges for effective renal clearance. Crafting SeNPs that are not only efficacious but also biodegradable, metamorphosing into benign byproducts, is quintessential to assuage potential toxicities and ensure unobstructed radiological assessments. Biodegradable metals are metals expected to corrode gradually in vivo, with an appropriate host response elicited by released corrosion products, then dissolve completely upon fulfilling the mission to assist with tissue healing with no implant residues. Therefore, the major component of BM should be essential metallic elements that can be metabolized by the human body, and demonstrate appropriate degradation rates and modes in the human body.
Biodegradable metal matrix composites.
When metals react with body fluid, they give away electrons and form positive ions. In electrochemistry, the values of standard electrode potential provide a way to compare the relative ease of different metal elements to lose electrons to form ions in solutions. Some metals have a much greater tendency to form their ions than hydrogen does; an element is more ready to lose electrons and form ions, the more negative its standard electrode potential value is. In other words, the more negative the standard electrode potential value is, then the more readily the metal degrades in an aqueous solution.141
Tumor biology and SeNP delivery dynamics.
The heterogeneity and complexity of tumor biology, encompassing a myriad of parameters like blood flow, perfusion, and stromal composition, inherently modulate the drug delivery potential of SeNPs. The need for personalized therapeutic considerations, taking into account nuances like a patient's age, gender, tumor morphology, and location, further amplifies this challenge.
SeNPs and biological system integration.
The eventual success of SeNPs hinges on their harmonious integration with the designated biological systems they target. Crafting SeNPs necessitates not just a robust understanding of nanoparticle science but an intricate appreciation of the interaction dynamics they would engage in within their biological microenvironments.
In essence, as we envision the future trajectory of SeNPs, addressing these challenges is not a mere academic prerogative but a strategic necessity, pivotal for their seamless and efficacious integration into the therapeutic milieu of tomorrow.
Conclusion and future prospectives
Selenium, beyond its fundamental role as a micronutrient, plays an indispensable role in ensuring the optimal functionality of intricate biological and metabolic pathways within the human physiology. The intricate integration of selenocysteine into selenoproteins underscores the profound relevance of selenium, as these proteins pivotally mediate antioxidant, immunomodulatory, and regulatory mechanisms. The expansive influence of selenium, which spans vital domains like thyroid regulation, hepatic metabolism, neural integrity, reproductive health, coupled with its antitumoral and antimicrobial attributes, elucidates its crucial significance in the broader spectrum of human health. Yet, the therapeutic application of selenium remains delicately poised, given its narrow therapeutic window, necessitating meticulous dosing considerations. Within the burgeoning therapeutic arena, plant-derived selenium nanoparticles (SeNPs) are progressively being acknowledged as potent agents, particularly in the face of challenges like antimicrobial and antifungal resistance. Their green synthesis, embodying biocompatibility and ecological congruence, positions them favorably for potential integration into commercial therapeutic formulations. Concurrently, the cosmetic sector is on the precipice of a paradigm shift, with SeNPs primed to redefine contemporary anti-ageing and dermatological solutions. Furthermore, as global health narratives increasingly intersect with viral pandemics, the immunomodulatory attributes of SeNPs present tantalizing prospects for innovative antiviral prophylactics and interventions.
A salient focal point in SeNP research converges on its anticancer potential, predicated on its unique ability to induce oxidative stress selectively in malignant cells, while preserving the integrity of healthy counterparts. This distinctive property, however, is accompanied by challenges intrinsic to Se's therapeutic bandwidth, necessitating calibrated therapeutic strategies. Cutting-edge innovations are steering towards the conjugation of SeNPs with ligands exhibiting high selectivity for oncogenic receptors, further supplemented with established chemotherapeutic agents and pertinent genetic modulators. This envisaged synergy promises enhanced oncological outcomes, concomitant with minimized systemic adversities. As we gaze into the future, a dual imperative crystallizes: a clarion call for comprehensive clinical evaluations to decipher the precise therapeutic efficacy and potential of SeNPs, and a sustained commitment to innovative research to fine-tune their synthesis, ensuring superior efficacy coupled with enhanced safety profiles. Such endeavors should encompass the development of cost-efficient, environmentally benign synthesis methodologies, and optimizing Se's therapeutic potential in pivotal areas like oncology, chemotherapy, and radiological interventions. Advancements in nanotechnology present tantalizing possibilities, particularly in devising novel SeNP delivery architectures, facilitating targeted Se delivery to designated organ systems. Such pioneering endeavors hold the promise of not only reshaping clinical therapeutics but also revolutionizing dietary and nutritional paradigms, positioning selenium as an integral component in the future lexicon of health and wellness.
Conflicts of interest
Authors declare no conflict of interest exists.
Acknowledgements
N. D. T. acknowledges funding under Science Foundation Ireland and Irish Research Council (SFI-IRC) pathway program (21/PATH-S/9634). The authors would like to acknowledge their respective departments for the conduct of the study.
References
- I. Khan, K. Saeed and I. Khan, Arabian J. Chem., 2019, 12, 908–931 CrossRef CAS.
-
A. Singh, N. Kaur, A. Parmar and H. K. Chopra, Handbook of Greener Synthesis of Nanomaterials and Compounds, Elsevier, 2021, pp. 3–36 Search PubMed.
- A. Verma, S. P. Gautam, K. K. Bansal, N. Prabhakar and J. M. Rosenholm, Medicines, 2019, 6, 39 CrossRef CAS PubMed.
- A. Sofowora, E. Ogunbodede and A. Onayade, Afr. J. Tradit., Complementary Altern. Med., 2013, 10, 210–229 Search PubMed.
- J. Iqbal, B. A. Abbasi, T. Mahmood, S. Kanwal, B. Ali, S. A. Shah and A. T. Khalil, Asian Pac. J. Trop. Biomed., 2017, 7, 1129–1150 CrossRef.
- J. Dai and R. J. Mumper, Molecules, 2010, 15, 7313–7352 CrossRef CAS PubMed.
- V. R. Ranjitha and V. R. Rai, J. Pharm. Invest., 2021, 51, 117–135 CrossRef CAS.
- C. Thiry, Y.-J. Schneider, L. Pussemier, L. De Temmerman and A. Ruttens, Biol. Trace Elem. Res., 2013, 152, 152–160 CrossRef CAS PubMed.
- M. Vinceti, G. Dennert, C. M. Crespi, M. Zwahlen, M. Brinkman, M. P. A. Zeegers, M. Horneber, R. D'Amico and C. Del Giovane, Cochrane Database Syst. Rev., 2014, 2014, CD005195–CD005195 Search PubMed.
- B. Guan, R. Yan, R. Li and X. Zhang, Int. J. Nanomed., 2018, 13, 7473–7490 CrossRef CAS PubMed.
- T. M. Sakr, M. Korany and K. V. Katti, J. Drug Delivery Sci. Technol., 2018, 46, 223–233 CrossRef CAS.
- S. Hariharan and S. Dharmaraj, Inflammopharmacology, 2020, 28, 667–695 CrossRef CAS PubMed.
- S. Y. S. Zeebaree, A. Y. S. Zeebaree and O. I. H. Zebari, Sustainable Chem. Pharm., 2020, 15, 100210 CrossRef.
- S. Garbo, S. Di Giacomo, D. Łażewska, E. Honkisz-Orzechowska, A. Di Sotto, R. Fioravanti, C. Zwergel and C. Battistelli, Pharmaceutics, 2023, 15, 104 CrossRef CAS PubMed.
- D. Radomska, R. Czarnomysy, D. Radomski and K. Bielawski, Int. J. Mol. Sci., 2021, 22, 1009 CrossRef CAS PubMed.
- B. Yu, P. You, M. Song, Y. Zhou, F. Yu and W. Zheng, New J. Chem., 2016, 40, 1118–1123 RSC.
- H. W. Tan, H.-Y. Mo, A. T. Y. Lau and Y.-M. Xu, Int. J. Mol. Sci., 2018, 20, 75 CrossRef PubMed.
- V. Nayak, K. R. B. Singh, A. K. Singh and R. P. Singh, New J. Chem., 2021, 45, 2849–2878 RSC.
- M. L. Ojeda, O. Carreras and F. Nogales, Antioxidants, 2022, 11, 1402 CrossRef CAS PubMed.
- V. M. Labunskyy, D. L. Hatfield and V. N. Gladyshev, Physiol. Rev., 2014, 94, 739–777 CrossRef CAS PubMed.
- R. L. Schmidt and M. Simonović, Croat. Med. J., 2012, 53, 535–550 CrossRef CAS PubMed.
- L. Johansson, G. Gafvelin and E. S. J. Arnér, Biochim. Biophys. Acta, Gen. Subj., 2005, 1726, 1–13 CrossRef CAS PubMed.
- R. Jain, D. Dominic, N. Jordan, E. R. Rene, S. Weiss, E. D. van Hullebusch, R. Hübner and P. N. L. Lens, Environ. Chem. Lett., 2016, 14, 381–386 CrossRef CAS.
- N. Ahmad, S. Sharma, M. K. Alam, V. N. Singh, S. F. Shamsi, B. R. Mehta and A. Fatma, Colloids Surf., B, 2010, 81, 81–86 CrossRef CAS PubMed.
- S. Ansar, S. M. Alshehri, M. Abudawood, S. S. Hamed and T. Ahamad, Int. J. Nanomed., 2017, 12, 7789–7797 CrossRef CAS PubMed.
- K. Bai, B. Hong, W. Huang and J. He, Pharmaceutics, 2020, 12, 43 CrossRef CAS PubMed.
- S. Shoeibi, P. Mozdziak and A. Golkar-Narenji, Top. Curr. Chem., 2017, 375, 88 CrossRef PubMed.
- A. Husen and K. S. Siddiqi, J. Nanobiotechnol., 2014, 12, 28 CrossRef PubMed.
- R. Ceylan, A. Demirbas, I. Ocsoy and A. Aktumsek, Sustainable Chem. Pharm., 2021, 21, 100426 CrossRef CAS.
- G. Sharma, A. R. Sharma, R. Bhavesh, J. Park, B. Ganbold, J. S. Nam and S. S. Lee, Molecules, 2014, 19, 2761–2770 CrossRef PubMed.
- B. Fardsadegh, H. Vaghari, R. Mohammad-Jafari, Y. Najian and H. Jafarizadeh-Malmiri, Green Process Synth., 2019, 8, 191–198 CAS.
- H. Alam, N. Khatoon, M. Raza, P. C. Ghosh and M. Sardar, Bionanoscience, 2019, 9, 96–104 CrossRef.
- L. Gunti, R. S. Dass and N. K. Kalagatur, Front. Microbiol., 2019, 10, 931 CrossRef PubMed.
- L. Wu, Y. Wu, X. Che, D. Luo, J. Lu, R. Zhao, M. Zubair Iqbal, Q. Zhang, X. Wang and X. Kong, J. Biomater. Sci., Polym. Ed., 2021, 32, 1370–1385 CrossRef CAS PubMed.
- X. Fang, X. Wu, C. E. Li, B. Zhou, X. Chen, T. Chen and F. Yang, RSC Adv., 2017, 7, 8178–8185 RSC.
- J. P. M. Almeida, A. L. Chen, A. Foster and R. Drezek, Nanomedicine, 2011, 6, 815–835 CrossRef CAS PubMed.
- J. Zhang, X. Wang and T. Xu, Toxicol. Sci, 2008, 101, 22–31 CrossRef CAS PubMed.
- K. Loeschner, N. Hadrup, M. Hansen, S. A. Pereira, B. Gammelgaard, L. H. Møller, A. Mortensen, H. R. Lam and E. H. Larsen, Metallomics, 2013, 6, 330–337 CrossRef PubMed.
- R. S. Oremland, M. J. Herbel, J. S. Blum, S. Langley, T. J. Beveridge, P. M. Ajayan, T. Sutto, A. V. Ellis and S. Curran, Appl. Environ. Microbiol., 2004, 70, 52–60 CrossRef CAS PubMed.
- G. AlBasher, S. Alfarraj, S. Alarifi, S. Alkhtani, R. Almeer, N. Alsultan, M. Alharthi, N. Alotibi, A. Al-dbass and A. E. Abdel Moneim, Biol. Trace Elem. Res., 2020, 194, 444–454 CrossRef CAS PubMed.
- A. P. Fernandes and V. Gandin, Biochim. Biophys. Acta, Gen. Subj., 2015, 1850, 1642–1660 CrossRef CAS PubMed.
- U. Tinggi, Environ. Health Prev. Med., 2008, 13, 102–108 CrossRef CAS PubMed.
- N. Hadrup, K. Loeschner, K. Mandrup, G. Ravn-Haren, H. L. Frandsen, E. H. Larsen, H. R. Lam and A. Mortensen, Drug Chem. Toxicol., 2019, 42, 76–83 CrossRef CAS PubMed.
- I. Bano, S. Skalickova, S. Arbab, L. Urbankova and P. Horky, J. Anim. Sci. Biotechnol., 2022, 13, 72 CrossRef CAS PubMed.
- P. Kondaparthi, M. Deore, S. Naqvi and S. J. S. Flora, Environ. Sci. Pollut. Res. Int., 2021, 28, 53034–53044 CrossRef CAS PubMed.
- W. Liu, X. Li, Y.-S. Wong, W. Zheng, Y. Zhang, W. Cao and T. Chen, ACS Nano, 2012, 6, 6578–6591 CrossRef CAS PubMed.
- Z. Cheng, X. Zhi, G. Sun, W. Guo, Y. Huang, W. Sun, X. Tian, F. Zhao and K. Hu, J. Med. Virol., 2016, 88, 653–663 CrossRef CAS PubMed.
- S. Kumar, M. S. Tomar and A. Acharya, Colloids Surf., B, 2015, 126, 546–552 CrossRef CAS PubMed.
- Y. Huang, L. He, W. Liu, C. Fan, W. Zheng, Y.-S. Wong and T. Chen, Biomaterials, 2013, 34, 7106–7116 CrossRef CAS PubMed.
- R. Hassanien, A. A. I. Abed-Elmageed and D. Z. Husein, ChemistrySelect, 2019, 4, 9018–9026 CrossRef CAS.
- F. Maiyo and M. Singh, Nanomedicine, 2017, 12, 1075–1089 CrossRef CAS PubMed.
- C. Dwivedi, C. P. Shah, K. Singh, M. Kumar and P. N. Bajaj, J. Nanotechnol., 2011, 2011, 651971 Search PubMed.
- J. Yin, Y. Hou, Y. Yin and X. Song, Int. J. Nanomed., 2017, 12, 8671–8680 CrossRef CAS PubMed.
- R. Wen, B. Banik, R. K. Pathak, A. Kumar, N. Kolishetti and S. Dhar, Adv. Drug Delivery Rev., 2016, 99, 52–69 CrossRef CAS PubMed.
- W. Lin, J. Zhang, J.-F. Xu and J. Pi, Front. Pharmacol., 2021, 12, 682284 CrossRef CAS PubMed.
- M. Ikram, B. Javed, N. I. Raja and Z.-U.-R. Mashwani, Int. J. Nanomed., 2021, 16, 249–268 CrossRef PubMed.
- K. Anu, S. Devanesan, R. Prasanth, M. S. AlSalhi, S. Ajithkumar and G. Singaravelu, J. King Saud Univ., Sci., 2020, 32, 2520–2526 CrossRef.
- C. Mellinas, A. Jiménez and M. D. C. Garrigós, Molecules, 2019, 24, 4048 CrossRef CAS PubMed.
- B. Fardsadegh and H. Jafarizadeh-Malmiri, Green Process Synth., 2019, 8, 399–407 CAS.
- J. K. Patra and K.-H. Baek, J. Nanomater., 2014, 2014, 417305 Search PubMed.
- S. Lian, C. S. Diko, Y. Yan, Z. Li, H. Zhang, Q. Ma and Y. Qu, 3 Biotech., 2019, 9, 221 CrossRef PubMed.
- Z. Sanie, M. S. Iqbal, K. Abbas and M. I. Qadir, Arabian J. Chem., 2022, 15, 103901 CrossRef.
- S. Alipour, S. Kalari, M. H. Morowvat, Z. Sabahi and A. Dehshahri, BioMed Res. Int., 2021, 2021, 6635297 Search PubMed.
- S. Kiprotich, F. B. Dejene and M. O. Onani, Appl. Phys. A: Mater. Sci. Process., 2018, 125, 4 CrossRef.
- M. Madani, S. Hosny, D. M. Alshangiti, N. Nady, S. A. Alkhursani, H. Alkhaldi, S. A. Al-Gahtany, M. M. Ghobashy and G. A. Gaber, Nanotechnol. Rev., 2022, 11, 731–759 CrossRef CAS.
- D. Sharma, S. Kanchi and K. Bisetty, Arabian J. Chem., 2019, 12, 3576–3600 CrossRef CAS.
- Z.-H. Lin and C. R. Chris Wang, Mater. Chem. Phys., 2005, 92, 591–594 CrossRef CAS.
- F. A. Akçay and A. Avcı, Arch. Microbiol., 2020, 202, 2233–2243 CrossRef PubMed.
- N. Mollania, R. Tayebee and F. Narenji-Sani, Res. Chem. Intermed., 2016, 42, 4253–4271 CrossRef CAS.
- N. Bisht, P. Phalswal and P. K. Khanna, Mater. Adv., 2022, 3, 1415–1431 RSC.
- A. Shar, M. Lakhan, J. Wang, M. Ahmed, K. Alali, R. Naich, I. Soomro and A. Q. Dayo, Dig. J. Nanomater. Bios., 2019, 14, 867–872 Search PubMed.
- R. Hatami, A. Javadi and H. Jafarizadeh-Malmiri, Green Process Synth., 2020, 9, 685–692 Search PubMed.
- R. Abbasian and H. Jafarizadeh-Malmiri, Open Agric., 2020, 5, 761–767 CrossRef.
- K. Sheikhlou, S. Allahyari, S. Sabouri, Y. Najian and H. Jafarizadeh-Malmiri, Open Agric., 2020, 5, 227–235 CrossRef.
- B. Hosnedlova, M. Kepinska, S. Skalickova, C. Fernandez, B. Ruttkay-Nedecky, Q. Peng, M. Baron, M. Melcova, R. Opatrilova, J. Zidkova, G. Bjørklund, J. Sochor and R. Kizek, Int. J. Nanomed., 2018, 13, 2107–2128 CrossRef CAS PubMed.
- G. Rajagopal, A. Nivetha, S. Ilango, G. P. Muthudevi, I. Prabha and R. Arthimanju, J. Environ. Chem. Eng., 2021, 9, 105483 CrossRef CAS.
- J. Vyas and S. Rana, Int. J. Curr. Pharm. Res., 2017, 9, 147–152 CrossRef CAS.
- J. Vyas and S. Rana, Int. J. Phytomed., 2017, 9, 634–641 CrossRef CAS.
- V. Ganesan, Int. J. Sci. Res., 2015, 4, 690–695 Search PubMed.
- D. Cui, T. Liang, L. Sun, L. Meng, C. Yang, L. Wang, T. Liang and Q. Li, Pharm. Biol., 2018, 56, 528–534 CrossRef CAS PubMed.
- B. Deepa and V. Ganesan, Int. J. Chem. Tech. Res., 2015, 7, 725–733 Search PubMed.
- S. Santanu, R. Sowmiya and R. Balakrishnaraja, World J. Pharm. Res., 2015, 4, 1322–1330 Search PubMed.
- P. Sowndarya, G. Ramkumar and M. S. Shivakumar, Artif. Cells, Nanomed., Biotechnol., 2017, 45, 1490–1495 CrossRef CAS PubMed.
- K. S. Prasad, H. Patel, T. Patel, K. Patel and K. Selvaraj, Colloids Surf., B, 2013, 103, 261–266 CrossRef CAS PubMed.
- L. Zhou, Y. Li, X. Gong, Z. Li, H. Wang, L. Ma, M. Tuerhong, M. Abudukeremu, Y. Ohizumi, J. Xu and Y. Guo, Arabian J. Chem., 2022, 15, 103943 CrossRef CAS.
- T. Deepa, S. Mohan and P. Manimaran, Results Chem., 2022, 4, 100367 CrossRef CAS.
- K. Kokila, N. Elavarasan and V. Sujatha, New J. Chem., 2017, 41, 7481–7490 RSC.
- S. Perumal, M. V. Gopal Samy and D. Subramanian, Bioprocess Biosyst. Eng., 2021, 9, 634–641 Search PubMed.
- R. M. Tripathi, P. Hameed, R. P. Rao, N. Shrivastava, J. Mittal and S. Mohapatra, Bionanoscience, 2020, 10, 389–396 CrossRef.
- R. D. Sarkar, P. Lahkar and M. C. Kalita, Bioresour. Technol. Rep., 2022, 17, 100894 CrossRef CAS.
- R. Kirupagaran, A. Saritha and S. Bhuvaneswari, J. Nanosci. Nanotechnol., 2016, 224–226 Search PubMed.
- C. Sivakumar and K. Jeganathan, J. Drug Delivery Ther., 2018, 8, 195–200 CrossRef CAS.
- A. Puri and S. Patil, Pharmacogn. Res., 2022, 14, 289–296 CrossRef CAS.
- C. Ramamurthy, K. S. Sampath, P. Arunkumar, M. S. Kumar, V. Sujatha, K. Premkumar and C. Thirunavukkarasu, Bioprocess Biosyst. Eng., 2013, 36, 1131–1139 CrossRef CAS PubMed.
- K. S. Prasad and K. Selvaraj, Biol. Trace Elem. Res., 2014, 157, 275–283 CrossRef CAS PubMed.
- G. Sharma, A. R. Sharma, R. Bhavesh, J. Park, B. Ganbold, J.-S. Nam and S.-S. Lee, Molecules, 2014, 19, 2761–2770 CrossRef PubMed.
- V. Alagesan and S. Venugopal, Bionanoscience, 2019, 9, 105–116 CrossRef.
- P. Sarotra and B. Medhi, Recent Results Cancer Res., 2016, 209, 111–121 CAS.
- H. Vahidi, H. Barabadi and M. Saravanan, J. Cluster Sci., 2020, 31, 301–309 CrossRef CAS.
- O. Bilek, Z. Fohlerova and J. Hubalek, PLoS One, 2019, 14, e0214066 CrossRef CAS PubMed.
- E. G. Varlamova, E. A. Turovsky and E. V. Blinova, Int. J. Mol. Sci., 2021, 22, 10808 CrossRef CAS PubMed.
- A. Khurana, S. Tekula, M. A. Saifi, P. Venkatesh and C. Godugu, Biomed. Pharmacother., 2019, 111, 802–812 CrossRef CAS PubMed.
- M. Ikram, B. Javed, N. I. Raja and Z. U. Mashwani, Int. J. Nanomed., 2021, 16, 249–268 CrossRef PubMed.
- M. Hassan, H. Watari, A. AbuAlmaaty, Y. Ohba and N. Sakuragi, BioMed Res. Int., 2014, 2014, 150845 Search PubMed.
- G. Zhao, X. Wu, P. Chen, L. Zhang, C. S. Yang and J. Zhang, Free Radicals Biol. Med., 2018, 126, 55–66 CrossRef CAS PubMed.
-
A. Yanumula and J. K. Cusick, Biochemistry, Extrinsic Pathway of Apoptosis, StatPearls, StatPearls Publishing, Treasure Island (FL), 2023 Search PubMed.
- Z. Yu, Q. Li, J. Wang, Y. Yu, Y. Wang, Q. Zhou and P. Li, Nanoscale Res. Lett., 2020, 15, 115 CrossRef CAS PubMed.
- A. M. Al-Otaibi, A. S. Al-Gebaly, R. Almeer, G. Albasher, W. S. Al-Qahtani and A. E. Abdel Moneim, Environ. Sci. Pollut. Res., 2022, 29, 47539–47548 CrossRef CAS PubMed.
- F. Chen, X. H. Zhang, X. D. Hu, P. D. Liu and H. Q. Zhang, Artif. Cells, Nanomed.,
Biotechnol., 2018, 46, 937–948 CrossRef CAS PubMed.
- Y. Yang, Z. Zhang, Q. Chen, Y. You, X. Li and T. Chen, Front. Bioeng. Biotechnol., 2021, 9, 758482 CrossRef PubMed.
- T. A. Mary, K. Shanthi, K. Vimala and K. Soundarapandian, RSC Adv., 2016, 6, 22936–22949 RSC.
- M. P. Purohit, N. K. Verma, A. K. Kar, A. Singh, D. Ghosh and S. Patnaik, ACS Appl. Mater. Interfaces, 2017, 9, 36493–36512 CrossRef CAS PubMed.
- M. H. Yazdi, M. Mahdavi, B. Varastehmoradi, M. A. Faramarzi and A. R. Shahverdi, Biol. Trace Elem. Res., 2012, 149, 22–28 CrossRef CAS PubMed.
- A. R. Shahverdi, F. Shahverdi, E. Faghfuri, M. Rezakhoshayand, F. Mavandadnejad, M. H. Yazdi and M. Amini, Arch. Med. Res., 2018, 49, 10–17 CrossRef CAS PubMed.
- K. K. Vekariya, J. Kaur and K. Tikoo, Nanomedicine, 2012, 8, 1125–1132 CrossRef CAS PubMed.
- V. Lobo, A. Patil, A. Phatak and N. Chandra, Pharmacognosy reviews, 2010, 4, 118–126 CrossRef CAS PubMed.
- F. A. Matough, S. B. Budin, Z. A. Hamid, N. Alwahaibi and J. Mohamed, Sultan Qaboos Univ. Med. J., 2012, 12, 5–18 CrossRef PubMed.
- K. Pyrzynska and A. Sentkowska, J. Nanostruct. Chem., 2021, 12, 467–480 CrossRef.
- M. Ikram, N. I. Raja, Z.-U.-R. Mashwani, A. A. Omar, A. H. Mohamed, S. H. Satti and E. Zohra, Nanomaterials, 2022, 12, 356 CrossRef CAS PubMed.
- K.-F. Kong, L. Schneper and K. Mathee, APMIS, 2010, 118, 1–36 CrossRef CAS PubMed.
- D. Pacheu-Grau, A. Gómez-Durán, M. J. López-Pérez, J. Montoya and E. Ruiz-Pesini, Drug Discovery Today, 2010, 15, 33–39 CrossRef CAS PubMed.
- R. Singh, L. Sripada and R. Singh, Mitochondrion, 2014, 16, 50–54 CrossRef CAS PubMed.
- E. O. Ogunsona, R. Muthuraj, E. Ojogbo, O. Valerio and T. H. Mekonnen, Appl. Mater. Today, 2020, 18, 100473 CrossRef.
- M. C. Escobar-Ramírez, A. Castañeda-Ovando, E. Pérez-Escalante, G. M. Rodríguez-Serrano, E. Ramírez-Moreno, A. Quintero-Lira, E. Contreras-López, J. Añorve-Morga, J. Jaimez-Ordaz and L. G. González-Olivares, Fermentation, 2021, 7, 130 CrossRef.
- V. Cittrarasu, D. Kaliannan, K. Dharman, V. Maluventhen, M. Easwaran, W. C. Liu, B. Balasubramanian and M. Arumugam, Sci. Rep., 2021, 11, 1032 CrossRef CAS PubMed.
- G. M. Khiralla and B. A. El-Deeb, LWT–Food Sci. Technol., 2015, 63, 1001–1007 CrossRef CAS.
- C. Egbuna, V. K. Parmar, J. Jeevanandam, S. M. Ezzat, K. C. Patrick-Iwuanyanwu, C. O. Adetunji, J. Khan, E. N. Onyeike, C. Z. Uche, M. Akram, M. S. Ibrahim, N. M. El Mahdy, C. G. Awuchi, K. Saravanan, H. Tijjani, U. E. Odoh, M. Messaoudi, J. C. Ifemeje, M. C. Olisah, N. J. Ezeofor, C. J. Chikwendu and C. G. Ibeabuchi, J. Toxicol., 2021, 2021, 9954443 Search PubMed.
- Y.-W. Huang, M. Cambre and H.-J. Lee, Int. J. Mol. Sci., 2017, 18, 2702 CrossRef PubMed.
- V. Cittrarasu, D. Kaliannan, K. Dharman, V. Maluventhen, M. Easwaran, W. C. Liu, B. Balasubramanian and M. Arumugam, Sci. Rep., 2021, 11, 1032 CrossRef CAS PubMed.
- Y. C. Chen, K. S. Prabhu and A. M. Mastro, Nutrients, 2013, 5, 1149–1168 CrossRef CAS PubMed.
- R. J. Lobb, G. M. Jacobson, R. T. Cursons and M. B. Jameson, Int. J. Mol. Sci., 2018, 19, 3167 CrossRef PubMed.
- S. J. Kim, M. C. Choi, J. M. Park and A. S. Chung, Int. J. Mol. Sci., 2021, 22, 11844 CrossRef CAS PubMed.
- B. Toubhans, S. A. Gazze, C. Bissardon, S. Bohic, A. T. Gourlan, D. Gonzalez, L. Charlet, R. S. Conlan and L. W. Francis, Nanomedicine, 2020, 29, 102258 CrossRef CAS PubMed.
- S. R. Vundela, N. K. Kalagatur, A. Nagaraj, K. Kadirvelu, S. Chandranayaka, K. Kondapalli, A. Hashem, E. F. Abd_Allah and S. Poda, Front. Microbiol., 2022, 12, 769891 CrossRef PubMed.
- S. Menon, S. Devi K.S., H. Agarwal and V. K. Shanmugam, Colloid Interface Sci. Commun., 2019, 29, 1–8 CrossRef CAS.
- P. B. Ezhuthupurakkal, L. R. Polaki, A. Suyavaran, A. Subastri, V. Sujatha and C. Thirunavukkarasu, Mater. Sci. Eng., C, 2017, 74, 597–608 CrossRef CAS PubMed.
- T. Nie, H. Wu, K.-H. Wong and T. Chen, J. Mater. Chem. B, 2016, 4, 2351–2358 RSC.
- Y. Huang, L. He, W. Liu, C. Fan, W. Zheng, Y. S. Wong and T. Chen, Biomaterials, 2013, 34, 7106–7116 CrossRef CAS PubMed.
- H. Wu, X. Li, W. Liu, T. Chen, Y. Li, W. Zheng, C. W.-Y. Man, M.-K. Wong and K.-H. Wong, J. Mater. Chem., 2012, 22, 9602–9610 RSC.
- M. C. Zambonino, E. M. Quizhpe, L. Mouheb, A. Rahman, S. N. Agathos and S. A. Dahoumane, Nanomaterials, 2023, 13, 424 CrossRef CAS PubMed.
- Y. F. Zheng, X. N. Gu and F. Witte, Mater. Sci. Eng., R, 2014, 77, 1–34 CrossRef.
Footnote |
† Equally contributing first authors. |
|
This journal is © The Royal Society of Chemistry 2024 |