Economically viable N-doped graphene aerogel for the photodegradation of structurally different dyes and a plant-model-based environmental assessment†
Received
20th October 2023
, Accepted 19th January 2024
First published on 20th January 2024
Abstract
Photocatalysis has become a feasible process to tackle the increasingly severe water pollution. Despite the extensive research on photocatalytic degradation, very little progress has been made on the solar light induced degradation of complex mixtures of dyes. The mixture of harmful pollutant dyes is a critical concern of water pollution. Existing photocatalysts might be effective but not practical because of difficulty in separation from aqueous medium and requirement of a complex synthesis process and expensive raw materials. In order to enable easy separation of photocatalysts from aqueous medium without any further contamination, self-standing N-doped graphene aerogel (N-GA) was synthesized in an environment-friendly and cost-effective approach. The N-GA synthesized through a one-step process from biomass demonstrates remarkable photocatalytic efficiency in degrading a mixture of dyes with varying structures under sunlight-induced conditions. A photocatalytic degradation efficiency of ∼98% for CV, ∼98% for MB, and ∼99% for CR was achieved in 300 min from the mixture. Moreover, the N-GA has excellent reusability, sustainability, high stability and easy recyclability due to its structural integrity and distinctive inherent properties. Insights into the photocatalytic mechanism were gained from radical trapping experiments, and results showed the substantial influence of superoxide radicals. Furthermore, another novelty of this work is environmental assessment of treated water by checking the germination and growth of green gram beans in treated water. The treated wastewater showed enhanced germination and healthy growth of green gram beans compared to dye-contaminated water. These results reveal the environmental safety and practical compliance of N-GA for broad applications.
Environmental significance
Mixed dyes in natural water reservoirs pose a significant threat to the environment. These dyes can induce mutations and bioaccumulation in plants and animals, disrupting the balance of natural habitats. Moreover, carcinogenic and mutagenic dyes endanger aquatic life and pose a risk to humans depending entirely on water sources. In order to address this pressing issue, our study focuses on the synthesis of environment-friendly and easily separable photocatalysts derived from biomass-based precursor materials contributing to sustainability efforts. Herein, we have developed N-doped graphene aerogel (N-GA) produced by utilizing clinically expired glucose powder. N-GA exhibits exceptional proficiency in the photodegradation of a mixture of dyes in wastewater and treated water and was further tested for plant growth assessment, ensuring a valuable environmental impact.
|
1. Introduction
The advancement in industrialization has brought severe concerns worldwide in the form of water pollution such as discharge of toxic organic dyes.1 Residual dyes and waste in industrial effluent discharge are a major contributor to water pollution due to their highly toxic and hazardous nature even at low concentrations.2,3 More seriously, most organic dyes are resistant to aerobic digestion, recalcitrant,4 and stable toward oxidizing agents,5 which create serious threats to human health, including carcinogenic6 and mutagenic effects.7 From an environmental view, they can potentially damage aquatic biota by blocking natural sunlight and can dissolve oxygen in water bodies due to their complex chemical nature.8,9 The removal of pollutants from wastewater is necessary for living life.10 Hence, efficient removal of dyes from industrial effluent in an environmentally friendly and economically viable manner is crucial to solve the biological, ecological, and industrial problems.
Several water remediation methods including advanced oxidation,11 membrane filtration,12 chemical precipitation,13 ion exchange,14 catalytic degradation,15 photocatalysis,16 and adsorption17 were used for the abolition of harmful dyes from the wastewater system. However, most of the available removal processes are sometimes constrained by issues like energy intensiveness, lack of practical applicability, low efficiency and high operational cost.18,19 Among the diverse physical and chemical methods for water decontamination, photocatalytic degradation is one of the robust processes for water remediation because of its advantageous nature such as high efficiency,20 easy handling, economic feasibility,21 wide suitability for diverse contaminants, insensitivity towards toxic substances and negligible generation of toxic by-products.22 For this purpose, different kinds of photocatalysts,23 including activated carbons,24 metal–organic frameworks,25 mesoporous silica,26 polymers,27 carbon nanomaterials,28 natural fibers,29 and inorganic materials,30 have been developed for the sequestration of organic dyes. However, most of the photocatalysts face regeneration difficulties and low loading capacity or are restricted due to higher production cost.31 Therefore, sustainable32 and cost-effective materials with high photocatalytic activity and regeneration capabilities are needed for the removal of pollutant dye from wastewater to maintain the ecological balance.
3D graphene aerogel (GA) as one of the fascinating allotropes of carbon is emerging as a highly efficient material for water purification because of its structural integrity, which remains unchanged throughout the water purification process. GA is often considered as one of the lightest solid materials in existence, making its separation from water systems very easy, which is vital in practical application.33 GA has a three-dimensional network of interconnected graphene sheets with a very high surface area.34 The inherent unique properties of GA such as a hierarchical microstructure with multiscale pores and continuous pore network can make it one of the most appealing substances for a varied range of applications such as energy storage, microwave adsorption, sensors, catalysis, water remediation, thermal insulators, and gas adsorption.35–38 Tremendous efforts have been dedicated to the fabrication of GA using biomass as the precursor.39 The conversion of biomass to GA utilizing freeze drying process is hindered due to the long synthesis time and high cost in large scale production.40 Therefore, the development of a facile synthesis process of GA from biomass has more advantages than the traditional synthesis route.
The majority of synthesized GA consist of a graphene framework within organized 3D nanostructures that incorporate both macropores and mesopores.41 This 3D structured nanoscale arrangement of GA offers an innovative method for suitable water remediation.42 However, GA is not so photoactive towards solar light adsorption because of their high band gap, but heteroatom (nitrogen, sulphur, phosphorus) doping in GA is proven to tune the band gap.43,44 Heteroatoms can be doped in GA either by surface modification with heteroatoms containing functional groups or by incorporation of hetero elements in the graphitic lattice.45,46 Doping of heteroatoms in nano-carbons can boost the photocatalytic efficiency due to the lowering of the diffusion limitation barrier and enhanced exposure of active sites for chemical reactions.47–49 The versatility of doping can enable the effective treatment of complex pollutants encountered in textile and pharmaceutical industries effluent.50 Heteroatom-doped GA can be easily combined with standing wastewater remediation technologies due to their versatile characteristics. However, addressing the scalability and cost-effectiveness of doped GA is crucial for their practical real-world application.51 To overcome this matter, biomass-based production approaches may reduce costs associated with doping practices and separation issues.
The current approach employs a sustainable, economically feasible, and streamlined method for the synthesis of nitrogen-doped graphene aerogel (N-GA) in just one-step using clinically expired commercially available glucose powder as a carbon source and ammonium chloride as a nitrogen source. The N-GA demonstrated photoactivity in degrading dyes with varying chemical structures, such as crystal violet (CV), methylene blue (MB) and Congo red (CR) and their complex mixtures, under direct sunlight. After complete degradation of the dyes the N-GA remained floating on water, which favored easy removal from the system without the need of any centrifugation or filtration process, and can be reused multiple times. Overall, the process mitigates the risk of secondary contamination of nanomaterials. Further, the ecological assessment of treated water was done using a plant-based model to check the overall sustainability of the process and the reusability of the treated water. Surprisingly, the germination and growth of green gram beans in the treated water are significantly high compared to that in dye-containing water. The overall approach is cost-effective, environment-friendly, sustainable and practically valuable not only for wastewater treatment but also for other photocatalytic applications.
2. Experimental section
2.1 Materials
Commercially available glucose powder known as an energy drink (Glucose D) which already meets the expiry date was obtained free of cost from a local market. Ammonium chloride was used for nitrogen doping and purchased from Emplura, Merck. Crystal violet, methylene blue, and Congo red as pollutant dyes and other reagents used in the trap study such as para-benzoquinone (p-BZQ), Na2EDTA and isopropyl alcohol (i-PA) were purchased from Sigma-Aldrich. Green gram seeds used in the plant-based model study were purchased from the local market. No further purification of reagents was required, and used as received. Millipore water was used throughout the experiments unless otherwise stated.
2.2 Synthesis of nitrogen-doped graphene aerogel
N-GA was synthesized by using a simple pyrolytic approach to avoid the necessity of a freeze-drying process. A mixture of expired glucose powder and ammonium chloride was taken at a ratio of 1
:
1.52 First, glucose was mixed adequately with ammonium chloride. Then, the glucose and ammonium chloride mixture was kept in a 50 mL silica boat and pyrolyzed at 1000 °C under a constant flow of argon gas at a ramp rate of 5 °C min−1, further kept for 2 h for graphitization, then allowed to cool naturally. Finally, a black, highly porous N-GA was collected without any further processing. The generation of NH3 and HCl during the heat treatment of ammonium chloride is vital for the formation of a porous structure and N-doping in N-GA.53 The evolution of NH3 and HCl during the polymerization of glucose gives a compact porous sponge, which after subsequent polymerization resulted in 3D N-GA.
2.3 Instrumentation
To ascertain the successful synthesis of N-GA, diverse characterization techniques were used. Field-emission scanning electron microscopy (FE-SEM) using a JSM-7500F instrument operated at 20 kV was used for the morphological characterization of the N-GA. Internal morphological and microstructural analysis was conducted using transmission electron microscopy (TEM), with further detailed examination of the internal structure accomplished through high-resolution transmission electron microscopy (HR-TEM) utilizing an FEI Tecnai G2 F30 instrument operating at 200 kV. Determination of the number and thickness of graphene layers was done by atomic force microscopy (AFM) via a Dimension ICON AFM instrument (Bruker, Singapore). X-ray diffraction (XRD) patterns of N-GA were obtained using a D8-Advance (Bruker) diffractometer with Cu-Kα radiation. Surficial functional groups were investigated via Fourier transform infrared (FTIR) spectroscopy employing a Spectrum 2 model (Perkin Elmer) spectrophotometer. UV-vis absorption was measured under the diffuse reflection mode using a UV-vis spectrometer (UV-2550, Shimadzu). Raman studies were conducted using a Raman spectrometer with laser excitation at 532 nm (Alpha 300 model, Oxford, Germany). X-ray photoelectron spectroscopy (XPS) analysis of the N-GA was performed using an ESCA+ instrument (Omicron Nanotechnology, Oxford).
2.4 Photodegradation experiment
The photocatalytic activity of as-synthesized N-GA under natural sunlight was evaluated by the use of three pollutant dyes: CV, MB, and CR. All the photocatalytic experiments were done under direct sunlight. For this approach, stock solutions of individual dyes CV, MB, and CR were made in DI water at a concentration of 250 mg L−1 for each dye. The mixed solution of harmful pollutant dyes (comprising CV, MB and CR) maintains a 12.5, 62.5, and 50 mg L−1 individual concentration for CV, MB, and CR, respectively, intended for the monitoring of the photocatalytic degradation efficiency of the synthesized N-GA. The dose of 1.5 mg mL−1 of N-GA was made constant in the solutions of individual dyes throughout the experiment. First the dyes and their mixed solutions were maintained under stirring for 25–30 min in the absence of light (dark) to achieve the adsorption and desorption equilibrium between the catalyst and the dyes. During the photocatalytic degradation experiments, 3 mL of dye solution was withdrawn at regular 10 min intervals of time. Before the measurement of absorbance of the individual dyes and their mixture, the solution was centrifuged every time. Afterwards a UV-visible spectrophotometer was used to measure the absorbance at wavelengths of 498, 663, and 585 nm for the CR, MB, and CV dye solution, respectively. The same experimentation as to individual dyes was done for the mixed dyes, comprising the three dyes CV, MB, and CR.
2.5 Practical wastewater remediation and recycle study
For the exploration of real-world applications, a mixture of three structurally different dyes (CV + MB + CR) was spiked in groundwater and seawater at the same specific individual concentrations of 12.5, 50, and 62.5 mg L−1 as above for CV, CR, and MB, respectively. Then 15 mg of N-GA was added to 10 mL of real water samples spiked with organic dyes. Later the above-mentioned procedure was followed. For the practical analysis, the degradation of complex mixtures of structurally different dyes was also assessed in seawater and tap water. For the recycling study of N-GA, after each photo degradation experiment of a mixture of dyes, N-GA was collected and washed several times with Millipore water. Finally, N-GA was kept in a hot air oven for drying at 60 °C and again the same N-GA was used for more solar light induced degradation of mixed dyes (CV + MB + CR) 10 times.
2.6 Plant-model-based ecological assessment
For the analysis of treated water to determine whether it is applicable for further utilization, a plant-model-based ecological assessment was done. First, good quality and same size green gram seeds were soaked in three different sets of water, DI water, mixture of dyes and treated water, for germination for a day. Then, after germination the seeds were distributed among three new Petri dishes, each containing an equal number of seeds (10 each), and tissue paper sheets that contained the control (DI water), mixture of dye (CV + MB + CR), and treated water. After 5 days of growth, the analysis of root and shoot development of plants was performed.
3. Results and discussion
The synthesis of 3D interconnected hierarchical N-GA was achieved in a single step by the pyrolysis of glucose powder in the presence of ammonium chloride. The addition of ammonium chloride allowed the simultaneous doping of nitrogen in the carbon framework.52 Utilization of expired glucose powder as a carbon source added the advantage of cost reduction. The volatile nature of ammonium chloride is beneficial for the evolution of gases at the start of the pyrolysis process, which evolves in the form of bubbles and is responsible for the generation of porosity in N-GA.53 Glucose and ammonium chloride at a weight ratio of 1
:
1 were pyrolyzed at 1000 °C for 2 h under inert conditions (argon gas atmosphere). Glucose was gradually polymerized to carbon clusters and during this process pores were created by the bubbling of NH3 and HCl gases. With the increase in temperature, subsequent graphitization of carbon clusters resulted in highly porous N-GA and there was no need for any additional freeze-drying or supercritical drying process. The whole synthesis process is sustainable; there were no chemicals used other than ammonium chloride. No further washing or solvent exchange was needed to obtain a self-standing N-GA. Fig. 1a represents the simple synthesis process of N-GA. The steady stabilization of N-GA on a delicate wildflower without any damage as shown in Fig. 1a indicates its ultralightweight nature.
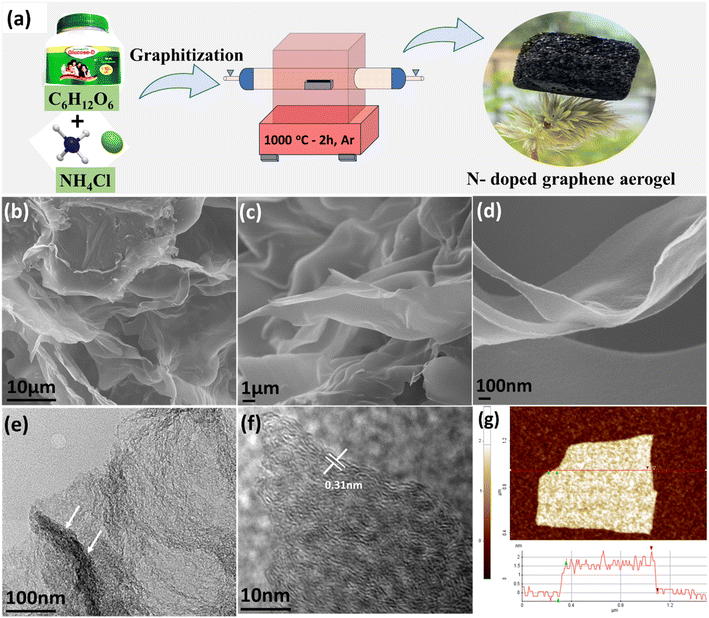 |
| Fig. 1 (a) Synthesis of N-GA from expired glucose powder and ammonium chloride using a simple pyrolytic approach, (b and c) FE-SEM images of N-GA showing the microporous architecture, (d) high-resolution FE-SEM image of a randomly oriented graphene sheet, (e) low-magnification TEM image of N-GA (white arrows indicate a multilayer of graphene), (f) HRTEM image of N-GA (white line shows the inter layer distance), (g) AFM image of N-GA (the inset shows the line profile). | |
3.1 Characterization of N-GA
The microporous 3D nano-architecture of N-GA is uneven and very porous (Fig. 1b).54 The 3D linked hierarchical framework comprises interconnected wrinkled graphene nanosheets with a greater number of pores (Fig. 1c). This structure provided reduced reflection and enhanced absorption of light during solar light induced photocatalysis.55 A randomly oriented graphene sheet is easily visible in a high-resolution SEM micrograph as displayed in Fig. 1d. The structural characteristics of N-GA are important for enhanced active site accessibility for photocatalysis.56Fig. 1e depicts the TEM characterization of N-GA. N-GA has a sheet-like morphology with different levels of transparency. The numerous graphene layers that make up the N-GA structure are depicted in dense black and indicated by white arrows.57 The HR-TEM image confirm the crystalline nature of the graphitic layers (Fig. 1f). In N-GA, the average inter layer distance between two graphitic planes is 0.31 nm. Fig. 1g displays an AFM micrograph of N-GA. There are a few layers of graphene with a lateral height of 1.5 to 2.2 nm.58,59
The XRD pattern of N-GA in Fig. 2a shows a broad and high intensity peak at 2θ = 23.89° ascribed to the reflections of disordered graphitic carbon and another peak with lower intensity at 2θ = 43.79° ascribed to the graphene pattern and interlayer condensation formation.41 A very small peak was observed at 2θ = 80.20° due to high graphitization. The FTIR spectrum of N-GA displays various characteristic bands located at ∼3440 cm−1 indicating O–H bonding, ∼2925 cm−1 signifying N–H bonds, ∼2855 cm−1 for C–H stretching, ∼1744 cm−1 corresponding to the presence of –C
O functional groups, ∼1635 cm−1 associated with C
C or C
N bonds, ∼1384 cm−1 denoting the presence of C–N bonds, ∼1119 cm−1 indicating the existence of –C–O groups, and ∼671 cm−1 indicating the presence of
C–H bonds, as illustrated in Fig. 2b.58,60 UV-vis DRS was employed to analyze the bandgap energy of the synthesized N-GA. Tauc's equation determined the bandgap of N-GA to be 1.7 eV as shown in Fig. 2c.61 According to the observed Raman spectrum, N-GA has two distinct D and G bands characteristic of nano-carbons centered at ∼1355 and ∼1598 cm−1, respectively (Fig. 2d).62 The disordered structure of randomly oriented graphene in N-GA can be confirmed by the slightly higher intensity of the D band at 1355 cm−1 attributed to the high density of sp3 carbon atoms. The G band shows the in-plane stretching of sp2 carbon atoms in N-GA. The degree of disorder was measured by the ID/IG ratio, which is 0.998. The high ID/IG ratio is attributed to the presence of a higher degree of defects. XPS was used to investigate the valence states of atoms and elemental configurations of N-GA. The full scan XPS spectrum (Fig. 2e) of N-GA illustrates the co-presence of three elements C, O, and N with an elemental composition of 88.77%, 7.27%, 3.96%, respectively. A pie chart showing the percentage elemental composition is displayed in Fig. 2f. Five peaks are seen in the deconvoluted C 1s spectrum (Fig. 2g) of N-GA at binding energies of 284.29, 284.98, 285.88, 287.08, and 288.88 eV, which correspond to the C
C sp2, C–C sp3, C–O/C
N, C
O/C–N, and O
C–O− bonds of carbon, respectively.63Fig. 2h depicts the deconvoluted O 1s spectrum. Three peaks in the O 1s spectra of N-GA are associated with the C
O, C–O, and O
C–O−, with binding energies of 530.78, 532.25, and 533.72 eV, respectively.64 The doping of nitrogen into the carbon framework was thought to be the cause of the existence of C–N. Fig. 2i shows the deconvoluted high-resolution XPS spectra of N 1s, with three peaks at 397.99, 400.09, and 401.08 eV, corresponding to pyridinic-N, pyrrolic-N, and graphitic-N, respectively.65,66
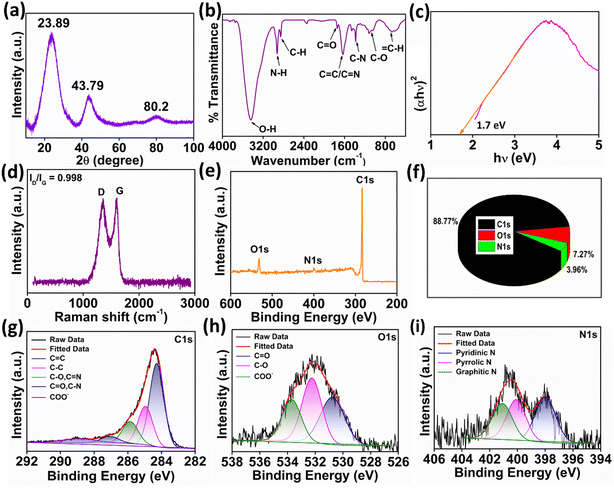 |
| Fig. 2 Characterization of N-GA. (a) XRD pattern, (b) FTIR spectrum, (c) Tauc plot of N-GA showing band gap, (d) Raman spectrum of N-GA with characteristic D and G bands, (e) XPS survey scan spectrum, (f) 3D pie chart showing the corresponding percentage elemental composition of N-GA, (g) deconvoluted short scan spectrum of C 1s, (h) deconvoluted short scan spectrum of O 1s, (i) deconvoluted short scan spectrum of N 1s. | |
3.2 Optimum concentration of dyes and N-GA
Three structurally different model pollutant dyes, which include anionic (CR) and cationic (CV and MB) dyes, were chosen for the photodegradation study using N-GA as a photocatalyst under the effect of direct solar light. To optimize the concentration of mixed dyes, we initially tried taking all three dyes using the same concentrations as for individual dyes (5 mg L−1 for CV, 20 mg L−1 for CR and 10 mg L−1 for MB). Here, two of the dyes exhibited significantly higher absorbance, while the other one showed lower absorbance (Fig. S1a†). However, at a concentration of 12.5, 50, and 62.5 mg L−1 for CV, CR and MB, respectively, a clear and distinct absorbance peak appears in the UV-visible spectrum as shown in Fig. S1b,† and this concentration is used throughout the experiments for a complex mixture of CV, MB, and CR dyes. For optimization of the dose, the photodegradation rate of a fixed concentration of dye (12.5, 62.5, and 50 mg L−1 for CV, MB, and CR, respectively) was investigated with a variable dosage of N-GA from 0.5 to 2.0 mg mL−1. It was found that with increasing dose of N-GA up to 1.5 mg mL−1 the photodegradation of dyes increased, and no significant changes were observed when the loading was increased to 2.0 mg mL−1 (Fig. S2†). Consequently, all experiments conducted in this study utilized a mixture of dye concentrations set at 12.5, 62.5, and 50 mg L−1 for CV, MB, and CR, respectively, alongside a 1.5 mg mL−1 dose of N-GA under the influence of natural sunlight.
3.3 Photodegradation of dyes and complex mixture of dyes
The potential of N-GA as a photocatalyst for water remediation under natural sunlight has been investigated using three different dyes with concentrations of 5, 10, and 20 mg L−1 for CV, MB, and CR, respectively, as individual and mixed dyes (12.5, 62.5, and 50 mg L−1 for CV, MB, and CR, respectively). An equilibrium between adsorption and desorption in N-GA was established within the initial 30 min as the individual dyes CV, MB, and CR and the mixed dye solution (CV + MB + CR) were stirred under dark conditions while interacting with N-GA. The observation was that ∼16.5% CV, ∼25.4% MB and ∼9% CR dyes were adsorbed on N-GA in the first 30 min.67 Afterwards, under natural sunlight, the absorbance peak of the dye solution decreases with time, which shows the solar light induced degradation of dyes and their complex mixture.68 The reduction in absorbance of specific peaks was recorded for CV, MB, and CR at 585, 663, and 498 nm, respectively, using UV-visible absorption spectroscopy. One control set of experiments was also done to check the photo-stability of the dyes and their mixture, where dyes and their mixed solutions were kept in direct natural sunlight in the absence of N-GA and in the dark with N-GA.69 In contrast to dark conditions, N-GA acts as a photocatalyst in the occurrence of natural sunlight, according to the plot of C/C0vs. time for individual dyes CV (Fig. 3a), MB (Fig. 3b), and CR (Fig. 3c) and in mixtures of dyes (Fig. 3d). The control solutions of the individual dyes CV, MB, and CR as well as the mixture of dye (CV + MB + CR) were considerably less degraded in the presence of only sunlight and had a significantly lower adsorption rate than N-GA with natural sunlight. Fig. 3d–f shows the individual dye degradation from a mixed dye (comprising CV, MB and CR). In comparison to the control, it was observed that in the presence of natural solar light, the percentage of photodegradation rate of the individual dyes and mixed dyes was quite noteworthy.
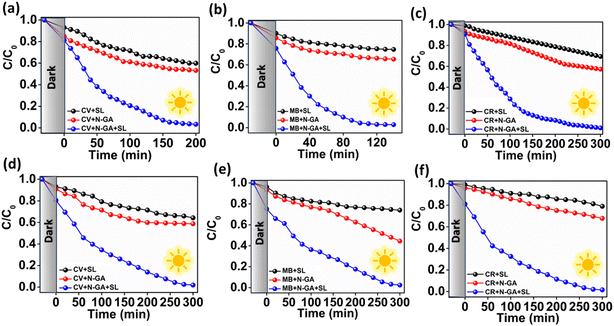 |
| Fig. 3 The plot of C/C0vs. time for the degradation of dyes under different conditions (in the presence of sunlight (SL) without N-GA, in the dark with N-GA, and in the presence of both sunlight and N-GA). (a) CV, (b) MB, (c) CR. (d) CV, (e) MB, and (f) CR in the mixture of dyes. | |
MB was photodegraded very fast, as ∼98% MB degradation was achieved in 140 min. CV showed a lower degradation rate which took 200 min for ∼98% degradation, while CR took a much longer time than the others, where ∼99% degradation was achieved in ∼280 min. The UV-visible absorbance spectra of individual dyes (CV, MB, and CR) are displayed in Fig. S3a–c.† The UV-visible absorbance spectrum of the mixed dyes (CV + MB + CR) exhibits clear peaks at 663 nm for MB, 585 nm for CV, and 498 nm for CR, as shown in Fig. S3d.† The distinct and well-separated peaks for each dye facilitate precise monitoring of concentration variations through UV-vis absorbance spectra. The effectiveness of photodegradation of the harmful dyes from the mixed solution (CV + MB + CR) was determined by assessing the alteration in the respective absorbance intensity.
To assess the rate of dye degradation, we applied the C/C0 ratio to fit in a pseudo-first-order (PFO) kinetic model for CV (Fig. 4a), MB (Fig. 4b), CR (Fig. 4c), and their combined mixture (Fig. 4d). All the three individual dyes and their mixture exhibit photodegradation in accordance with pseudo-first-order kinetics. This is validated by the linearly fitted data and a respectable regression coefficient value (R2 is nearly equals to 1). The photodegradation rate was determined using the following pseudo-first-order reaction (eqn (1)).
where
C0 is the initial concentration and
C is the concentration at time
t.
k is the PFO kinetic rate constant. The ln(
C0/
C)
vs. time plot displays that the degradation rate follows the Langmuir–Hinshelwood model and PFO kinetics.
70 In the context of a PFO process, the slope (
m) observed in the linearly fitted curve represents the rate constant (
k) for the photocatalytic degradation of dyes. The rate constant values for individual dyes CV, MB, and CR are 0.016, 0.019, and 0.013 min
−1, respectively. The photodegradation rate constants for a mixture of dyes (CV + MB + CR) are 0.011, 0.010, and 0.012 min
−1 for CV, MB and CR, respectively.
71
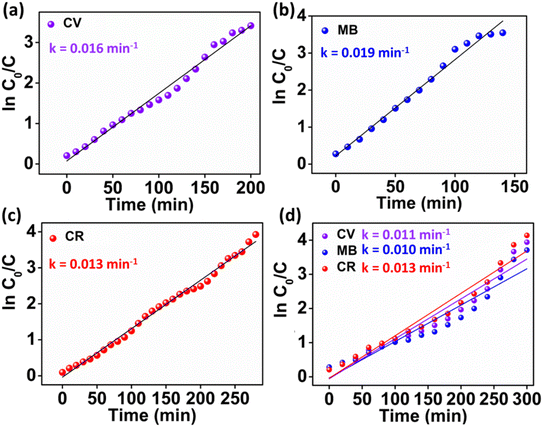 |
| Fig. 4 Linearly fitted ln C0/C plots of different dyes and their mixture with time for the pseudo-first-order model: (a) CV, (b) MB, (c) CR and (d) mixed CV, MB, and CR dyes. | |
3.4 Trap study for photocatalytic mechanism
A study was carried out to identify the active moieties responsible for the solar light induced degradation of dyes, both individually and as a mixture (comprising CV, MB, and CR). In this investigation, various scavengers were employed to capture different reactive radicals: superoxide radicals (O2˙−) were intercepted by p-BZQ, hydroxyl radicals (OH˙) were trapped using i-PA, and surface holes (h+) were captured with Na2-EDTA.72 The concentration of the scavenger was kept constant throughout the trapping experiment and the effects of the scavenger can be seen in the form of change in C/C0 of CV (Fig. 5a), MB (Fig. 5b), and CR (Fig. 5c). In the control experiment, which was similar to the previous one herein, DI water was employed with the individual dyes and their mixture instead of the scavenger. With the addition of p-BZQ, the photodegradation rate for CV reduced from 98% to 30%, for MB from 98% to 31%, and for CR from 99% to 27%, thus restricting the photocatalytic degradation process. When Na2EDTA was added, the photodegradation rate for CV dropped from 98% to 86%; for MB from 98% to 73%; and for CR from 99% to 69%. With the addition of i-PA, the CV degradation efficiency dropped from 98% to 71%; for MB from 98% to 64%; while for CR from 99% to 55%. Overall, it was shown that when i-PA and Na2EDTA are used, the photodegradation efficiency of pollutant dyes is significantly reduced. However, the photodegradation efficiency is drastically reduced when p-BZQ was used. Similarly, in mixed dyes (comprising CV, MB and CR), a similar trend was seen. Here, with the addition of p-BZQ, the photodegradation efficiency for CV drops from 98% to 34%; for MB from 98% to 37%; and for CR from 99% to 29%. With the addition of Na2EDTA, the photodegradation rate for CV drops from 98% to 87%; for MB from 98% to 74%; and for CR from 99% to 72%. When i-PA was added to the mixture of dyes, the photodegradation rate of CV was lowered from 98% to 72%, for MB from 98% to 66%, and for CR from 99% to 58%. The findings of the scavenger's addition on the dye mixtures are shown in Fig. 5d. Similar to individual dyes, using i-PA and Na2EDTA results in a considerably smaller reduction in photodegradation efficiency in mixtures of dyes; however, p-BZQ significantly slows down the photodegradation rates. Thus, in this instance, the superoxide radical (O2˙−) has key responsibilities for the photocatalytic process.
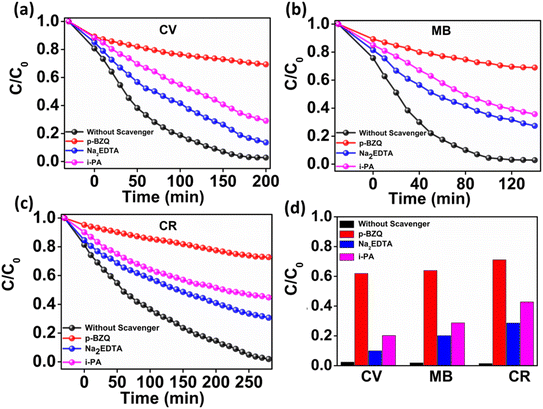 |
| Fig. 5 Effect of trapping agents: p-BZQ for superoxide, Na2-EDTA for holes, and i-PA for hydroxyl radicals, on the photocatalytic degradation activity of dyes. (a) CV, (b) MB, (c) CR, and (d) mixed dyes (CV + MB + CR). | |
3.5 Mechanism for photocatalytic degradation of dyes
Plausible mechanistic insights of the solar light induced degradation of dyes and their mixture in the presence of N-GA were gained with radical-trapping experiments. A schematic representation for the mechanistic insights regarding the photocatalytic activity of N-GA towards dye degradation is shown in Fig. 6a. N-GA with a suitable bandgap, when irradiated with natural sunlight, absorbs photons and is excited to produce electron–hole (e–h) pairs (Fig. 6b, eqn (1)). The photogenerated electrons in the valence band go to the conduction band and transfer to the surface of N-GA.73 Some of these electrons are trapped by surface defects, slowing down the recombination of e–h pairs; meanwhile photosensitized positive holes (h+) can react with water molecules adsorbed on the surface, forming (OH˙) (Fig. 6b, eqn (2)), which are subsequently involved in the degradation of dyes. Some electrons are captured by dissolved oxygen (O2), leading to the production of (O2˙−) (Fig. 6b, eqn (3)).74 Further, photosensitized h+ react with the dyes and are converted into radicals (Fig. 6b, eqn (5)), and simultaneously dye molecules react with either OH˙ or O2˙−, which finally break down their aromatic structure and are converted into non-toxic CO2 and H2O molecules (Fig. 6b, eqn (5) and (6)).75
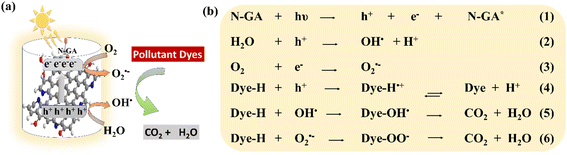 |
| Fig. 6 (a) A schematic mechanism of the photocatalytic degradation of dyes in natural sunlight and (b) overall steps involved in the sunlight-promoted degradation of dyes with N-GA. | |
4. Practical analysis in groundwater, seawater and recycle study
To explore the efficiency of N-GA as a photocatalyst for practical applications, the photodegradation of three dyes (CV + MB + CR) was evaluated in groundwater and seawater under similar conditions in the presence of natural sunlight. UV-vis absorbance peaks at 585, 663, and 498 nm for CV, MB, and CR dye, respectively, were observed, and the percentage degradation was calculated. The bar graph of percentage degradation of individual dyes in a mixture of dyes clearly demonstrates the exceptional efficiency of N-GA as a photocatalyst. In DI water, ∼98% photodegradation of dyes was achieved, while in groundwater, it remained at a noteworthy ∼90% (Fig. 7a). Even under the challenging conditions of seawater, a substantial ∼78% photodegradation was achieved (Fig. 7a). This highlights the remarkable potential of N-GA as a photocatalyst. Further, the real-world applicability of N-GA was evaluated through a recycling and reusability study. N-GA can be used several times as a photocatalyst. N-GA, after the photodegradation experiments, was recollected and further used for the same experiment after just simple washing with water and drying in a hot air oven. The dye degradation efficiency of N-GA remains constant up to 4 cycles and only a slight decrease (∼8–9%) was observed until ten cycles (Fig. 7b). Moreover, no change in the monolithic shape of N-GA was observed and it remained floating on the water (Fig. 7c), which makes separation of N-GA very easy without any extra efforts of centrifugation and filtration. In contrast to traditional photocatalysts, the structural integrity of N-GA reduces the risk of secondary contamination.
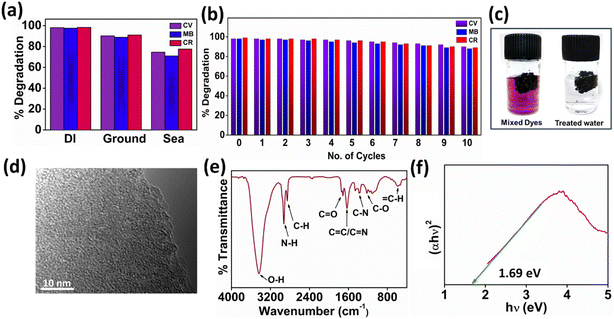 |
| Fig. 7 (a) Bar graph showing the percentage degradation of individual dyes from the mixture of dyes in spiked water, (b) recyclability of N-GA in a mixture of structurally different dyes for 10 cycles, (c) digital image showing easily separable N-GA. Characterization of N-GA after 10 successive photocatalytic cycles. (d) TEM image of N-GA showing the integrity of the microstructure, (e) FTIR spectrum of N-GA after degradation, (f) Tauc plot of N-GA showing an almost similar bandgap after the photocatalytic degradation. | |
The stability and structural integrity of N-GA after 10 sequential photodegradation cycles was confirmed with the help of TEM, FTIR, and UV-vis DRS analysis. The TEM image of N-GA in Fig. 7d, after 10 cycles of solar light induced degradation of mixed dyes comprising CV, MB and CR, confirms that no noteworthy changes in the internal morphology and structure of N-GA occurred. The FTIR spectrum of N-GA in Fig. 7e shows the persistence of chemical bonding of N-GA even after 10 successive cycles. The bandgap analysis obtained with the Tauc plot in Fig. 7f confirms the consistent photocatalytic stability of N-GA even after 10 cycles. The direct bandgap (Eg) of N-GA after the degradation experiment was measured to be 1.69 eV, remarkably similar to the bandgap of N-GA prior to photodegradation. These findings imply that N-GA could retain its outstanding photodegradation stability and efficiency even after repeated uses in the degradation of dye mixtures, which exhibits significant potential for practical adaptability.
5. Plant-model-based ecological assessment of treated water
To assess the sustainability and feasibility of the entire photocatalytic process in relation to the potential reuse of the treated water, a simpler ecotoxicological bioassay was carried out.22 The germination and growth of plants in treated water was used to investigate the ecotoxicological bioassay.76 One of the most delicate and quickly developing plants, green gram (Vigna radiata) plants, were chosen under three different conditions, such as (DI water) control, mixture of dyes, and photodegraded N-GA-treated water, for 5 days of growth. The mixture of dyes greatly inhibited the germination and development of the green gram plants (Fig. 8a). However, the growth of green gram plants in N-GA-treated water was higher than that in the control (Fig. 8b and c). Remarkably, a slight increase in the germination rate (∼89%) was observed for treated water compared with that of DI water (∼78%). The enhanced germination and growth of green gram plants significantly support the non-toxicity of N-GA.77 In mixed dyes comprising CV, MB and CR the germination rate was found to be only ∼32%, which was very low as shown in Fig. 8d. Similar results for the shoot lengths and root lengths were also observed after the 5th day of the growth period in DI water. The shoot and root lengths were lesser in DI water than in treated water, while the green gram beans germinated in water containing a mixture of dyes (CV + MB + CR) exhibited very short shoot and root lengths as shown in Fig. 8e. The observed results support the safe applications of the treated wastewater and the sustainability of the overall process. Nevertheless, further in-depth research that is more precise is needed for edible plants. Hence, this strategy will help to reduce the overuse of water resources, then encourage recycling of remediated water best for watering non-edible plants and grasslands.
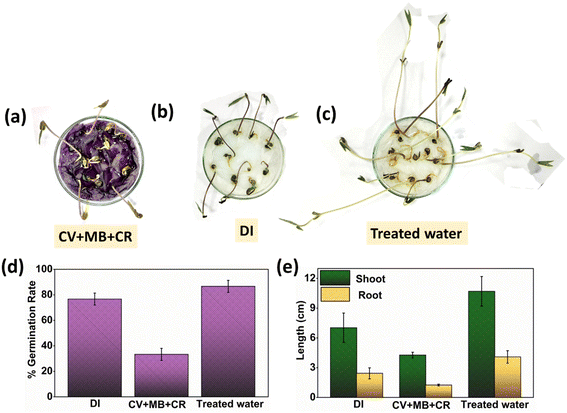 |
| Fig. 8 The growth of green gram plants after 5 days under different conditions: (a) in a mixture of dyes (MB + CV + CR), (b) in DI water (control), (c) in N-GA-treated water. (d) Germination assessment of green gram beans, (e) shoot and root length of green gram plant. | |
Conclusion
A N-GA has been synthesized from commercially available glucose powder which already meets the expiry date by an easy pyrolytic approach in a single step. The as-synthesized N-GA exhibits a 3D structured nanoscale arrangement and high density of active sites. N-GA acts as a photocatalyst for the degradation of a mixture of three structurally different dyes under natural solar light. In 300 minutes, N-GA successfully degraded approximately 99% of the mixture of dyes. The rate of photocatalysis is elucidated by PFO. To gain further understanding of the photocatalytic mechanism, radical trapping agents were employed to probe insights into the process. The practical adaptability of photodegradation was checked in groundwater and seawater. No remarkable changes towards photodegradation of dyes were observed in groundwater and seawater compared to DI water. Moreover, N-GA exhibited high recyclability and ease in recovery without any additional step. The sustainability and ecological adaptability of N-GA was tested for the germination and growth of green gram beans. The treated wastewater showed enhanced germination (∼89%) and healthy growth of green gram plants compared to mixed dye water.
Conflicts of interest
The authors declare no conflict of interest.
Acknowledgements
H. A. thanks IIPE Visakhapatnam for a fellowship. K. M. T. acknowledges the financial support from DST (SP/YO/2021/2102). Authors would like to thanks SBIF XRD analytical lab, IIPE for XRD analysis.
References
- P. Samanta, A. V. Desai, S. Let and S. K. Ghosh, Advanced Porous Materials for Sensing, Capture and Detoxification of Organic Pollutants toward Water Remediation, ACS Sustainable Chem. Eng., 2019, 7, 7456–7478 CrossRef CAS.
- S. Kolesnikov, T. Minnikova, K. Kazeev, Y. Akimenko and N. Evstegneeva, Assessment of the Ecotoxicity of Pollution by Potentially Toxic Elements by Biological Indicators of Haplic Chernozem of Southern Russia (Rostov region), Water, Air, Soil Pollut., 2022, 233, 18 CrossRef CAS PubMed.
- S. Akash, B. Sivaprakash, V. C. V. Raja, N. Rajamohan and G. Muthusamy, Remediation techniques for uranium removal from polluted environment – Review on methods, mechanism and toxicology, Environ. Pollut., 2022, 302, 119068 CrossRef CAS PubMed.
- L. Wu, Z. Shen, Y. Zhou and J. Zuo, Stimulating anaerobic digestion to degrade recalcitrant organic pollutants: Potential role of conductive materials-led direct interspecies electron transfer, J. Environ. Manage., 2023, 344, 118337 CrossRef CAS PubMed.
- A. Kausar, S. T. Zohra, S. Ijaz, M. Iqbal, J. Iqbal, I. Bibi, S. Nouren, N. El Messaoudi and A. Nazir, Cellulose-based materials and their adsorptive removal efficiency for dyes: A review, Int. J. Biol. Macromol., 2023, 224, 1337–1355 CrossRef CAS PubMed.
- M. Zulfajri, S. Sudewi, R. Damayanti and G. G. Huang, Rambutan seed waste-derived nitrogen-doped carbon dots with l-aspartic acid for the sensing of Congo red dye, RSC Adv., 2023, 13, 6422–6432 RSC.
- M. D. Lord, G. Neve, M. Keating and J. Budhathoki-Uprety, Polycarbodiimide for Textile Dye Removal from Contaminated Water, ACS Appl. Polym. Mater., 2022, 4, 6192–6201 CrossRef.
- A. Saravanan, P. Senthil Kumar, S. Jeevanantham, S. Karishma, B. Tajsabreen, P. R. Yaashikaa and B. Reshma, Effective water/wastewater treatment methodologies for toxic pollutants removal: Processes and applications towards sustainable development, Chemosphere, 2021, 280, 130595 CrossRef CAS PubMed.
- S. Varjani, P. Rakholiya, T. Shindhal, A. V. Shah and H. H. Ngo, Trends in dye industry effluent treatment and recovery of value added products, J. Water Process. Eng., 2021, 39, 101734 CrossRef.
- Y. Asakura, Y. Inaguma, K. Ueda, Y. Masubuchi and S. Yin, Synthesis of gallium oxynitride nanoparticles through hydrothermal reaction in the presence of acetylene black and their photocatalytic NOx decomposition, Nanoscale, 2018, 10, 1837–1844 RSC.
- J. Zhang, X. Jin, H. Zhao and C. Yang, Synergistic advanced oxidation process for enhanced degradation of organic pollutants in spent sulfuric acid over recoverable apricot shell-derived biochar catalyst, RSC Adv., 2022, 12, 1904–1913 RSC.
- S. Ahmadipouya, S. A. Mousavi, A. Shokrgozar and D. V. Mousavi, Improving dye removal and antifouling performance of polysulfone nanofiltration membranes by incorporation of UiO-66 metal-organic framework, J. Environ. Chem. Eng., 2022, 10, 107535 CrossRef CAS.
- A. Singh, D. B. Pal, A. Mohammad, A. Alhazmi, S. Haque, T. Yoon, N. Srivastava and V. K. Gupta, Biological remediation technologies for dyes and heavy metals in wastewater treatment: New insight, Bioresour. Technol., 2022, 343, 126154 CrossRef CAS PubMed.
- F. N. Türk, H. Çiftçi and H. Arslanoğlu, Removal of Basic Yellow 51 Dye by Using Ion Exchange Resin Obtained by Modification of Byproduct Sugar Beet Pulp, Sugar Tech, 2023, 25, 569–579 CrossRef.
- A. B. A. Abdellatif, H. M. El-Bery, H. N. Abdelhamid and S. A. El-Gyar, ZIF-67 and Cobalt-based@heteroatom–doped carbon nanomaterials for hydrogen production and dyes removal via adsorption and catalytic degradation, J. Environ. Chem. Eng., 2022, 10, 108848 CrossRef CAS.
- L. Liu, D. Wang, J. Huang, Z. Huang, Y. Zhang and L. Li, Multicomponent Composite Membrane with Three-Phase Interface Heterostructure as Photocatalyst for Organic Dye Removal, ACS Omega, 2022, 7, 17128–17143 CrossRef CAS PubMed.
- Y. Y. Tan, A. A. Abdul Raman, M. I. I. Zainal Abidin and A. Buthiyappan, Sustainable Dye Adsorption Using Novel Activated Carbon Prepared from Passion Fruit (Passiflora edulis) Leaf: Mechanism and Cost Analysis, Ind. Eng. Chem. Res., 2023, 62(36), 14507–14521 CrossRef CAS.
- R. Tanveer, A. Yasar, A.-U.-B. Tabinda, A. Ikhlaq, H. Nissar and A.-S. Nizami, Comparison of ozonation, Fenton, and photo-Fenton processes for the treatment of textile dye-bath effluents integrated with electrocoagulation, J. Water Process. Eng., 2022, 46, 102547 CrossRef.
- G. A. Ismail and H. Sakai, Review on effect of different type of dyes on advanced oxidation processes (AOPs) for textile color removal, Chemosphere, 2022, 291, 132906 CrossRef CAS PubMed.
- M. Shabir, M. Yasin, M. Hussain, I. Shafiq, P. Akhter, A.-S. Nizami, B.-H. Jeon and Y.-K. Park, A review on recent advances in the treatment of dye-polluted wastewater, J. Ind. Eng. Chem., 2022, 112, 1–19 CrossRef CAS.
- A. Galushchinskiy, R. González-Gómez, K. McCarthy, P. Farràs and A. Savateev, Progress in Development of Photocatalytic Processes for Synthesis of Fuels and Organic Compounds under Outdoor Solar Light, Energy Fuels, 2022, 36, 4625–4639 CrossRef CAS PubMed.
- S. J. Park, G. S. Das, F. Schütt, R. Adelung, Y. K. Mishra, K. M. Tripathi and T. Kim, Visible-light photocatalysis by carbon-nano-onion-functionalized ZnO tetrapods: degradation of 2,4-dinitrophenol and a plant-model-based ecological assessment, NPG Asia Mater., 2019, 11, 8 CrossRef CAS.
- P. M. Rajaitha, S. Hajra, M. Sahu, K. Mistewicz, B. Toroń, R. Abolhassani, S. Panda, Y. K. Mishra and H. J. Kim, Unraveling highly efficient nanomaterial photocatalyst for pollutant removal: a comprehensive review and future progress, Mater. Today Chem., 2022, 23, 100692 CrossRef CAS.
- R. Zhang, J. Jiang and K. Zeng, Synthesis of Bi2WO6/g-C3N4 heterojunction on activated carbon fiber membrane as a thin-film photocatalyst for treating antibiotic wastewater, Inorg. Chem. Commun., 2022, 140, 109418 CrossRef CAS.
- B. Fu, H. Sun, J. Liu, T. Zhou, M. Chen, Z. Cai, D. Hao and X. Zhu, Construction of MIL-125-NH2@BiVO4 Composites for Efficient Photocatalytic Dye Degradation, ACS Omega, 2022, 7, 26201–26210 CrossRef CAS PubMed.
- M. El Hajam, N. I. Kandri, A. Zerouale, X. Wang, J. Gustafsson, L. Wang, E. Mäkilä, L. Hupa and C. Xu, Lignocellulosic Nanocrystals from Sawmill Waste as Biotemplates for Free-Surfactant Synthesis of Photocatalytically Active Porous Silica, ACS Appl. Mater. Interfaces, 2022, 14, 19547–19560 CrossRef CAS PubMed.
- H. Bagheri, M. A. Pasha, M. M. Lakouraj, V. Hasantabar and M. Mohseni, Highly efficient, bioactive, and bifunctional sorbent p–n–p visible light heterogeneous photocatalyst utilizing ultra-fine ZnS nanoparticles embedded in a polymeric nanocomposite, RSC Adv., 2022, 12, 15950–15972 RSC.
- G. Gallareta-Olivares, A. Rivas-Sanchez, A. Cruz-Cruz, S. M. Hussain, R. B. González-González, M. F. Cárdenas-Alcaide, H. M. N. Iqbal and R. Parra-Saldívar, Metal-doped carbon dots as robust nanomaterials for the monitoring and degradation of water pollutants, Chemosphere, 2023, 312, 137190 CrossRef CAS PubMed.
- A. A. El-Samak, D. Ponnamma, M. K. Hassan and M. A. A. Al-Maadeed, A stable porous vessel for photocatalytic degradation of Azocarmine G dye, Microporous Mesoporous Mater., 2022, 341, 111994 CrossRef CAS.
- U. Chakraborty, G. Kaur, H.-G. Rubahn, A. Kaushik, G. R. Chaudhary and Y. K. Mishra, Advanced metal oxides nanostructures to recognize and eradicate water pollutants, Prog. Mater. Sci., 2023, 139, 101169 CrossRef CAS.
- S. Zhou, Y. Cai, J. Zhang, Y. Liu, L. Zhou and J. Lei, Au-Loaded Resorcinol–Formaldehyde Resin Photocatalysts: Hollow Sphere Structure Design and Localized Surface Plasmon Resonance Effect Synergistically Promote Efficient Nicotinamide Adenine Dinucleotide (NADH) Regeneration, ACS Sustainable Chem. Eng., 2022, 10, 14464–14473 CrossRef CAS.
- A. K. Rana, Y. K. Mishra, V. K. Gupta and V. K. Thakur, Sustainable materials in the removal of pesticides from contaminated water: Perspective on macro to nanoscale cellulose, Sci. Total Environ., 2021, 797, 149129 CrossRef CAS PubMed.
- M. He, D. Xie, L. Yin, K. Gong and K. Zhou, Influences of reduction temperature on energy storage performance of paraffin wax/graphene aerogel composite phase change materials, Mater. Today Commun., 2023, 34, 105288 CrossRef CAS.
- Z. Xue, Y. Ru, Z. Wang, Q. Li, M. Yu, J. Li and H. Sun, A 3D-printed freestanding graphene aerogel composite photocathode for high-capacity and long-life photo-assisted Li–O2 batteries, Nanoscale, 2023, 15(36), 14877–14885 RSC.
- Y. Mu, L. Wang, R. Zhang, R. A. Pashameah, E. Alzahrani, Z. Li, A. K. Alanazi, H. Algadi, M. Huang, Z. Guo, T. Wan and H. Wei, Rapid and facile fabrication of hierarchically porous graphene aerogel for oil-water separation and piezoresistive sensing applications, Appl. Surf. Sci., 2023, 613, 155982 CrossRef CAS.
- W. Wu, M. Du, H. Shi, Q. Zheng and Z. Bai, Application of graphene aerogels in oil spill recovery: A review, Sci. Total Environ., 2023, 856, 159107 CrossRef CAS PubMed.
- Z. Cheng, R. Wang, Y. Wang, Y. Cao, Y. Shen, Y. Huang and Y. Chen, Recent advances in graphene aerogels as absorption-dominated electromagnetic interference shielding materials, Carbon, 2023, 205, 112–137 CrossRef CAS.
- N. Li, J. Wu, R. Su, N. Zhang, J. Zhao and Z. Wang, Bioinspired green tea waste/graphene aerogel for solar-enhanced uranium extraction from seawater, Desalination, 2023, 545, 116153 CrossRef CAS.
- J. Kaushik, V. Kumar, A. K. Garg, P. Dubey, K. M. Tripathi and S. K. Sonkar, Bio-mass derived functionalized graphene aerogel: a sustainable approach for the removal of multiple organic dyes and their mixtures, New J. Chem., 2021, 45, 9073–9083 RSC.
- Y. Fu, G. Wang, T. Mei, J. Li, J. Wang and X. Wang, Accessible Graphene Aerogel for Efficiently Harvesting Solar Energy, ACS Sustainable Chem. Eng., 2017, 5, 4665–4671 CrossRef CAS.
- Y. Myung, S. Jung, T. T. Tung, K. M. Tripathi and T. Kim, Graphene-Based Aerogels Derived from Biomass for Energy Storage and Environmental Remediation, ACS Sustainable Chem. Eng., 2019, 7, 3772–3782 CrossRef CAS.
- S. Dong, L. Xia, T. Guo, F. Zhang, L. Cui, X. Su, D. Wang, W. Guo and J. Sun, Controlled synthesis of flexible graphene aerogels macroscopic monolith as versatile agents for wastewater treatment, Appl. Surf. Sci., 2018, 445, 30–38 CrossRef CAS.
- T. Selvakumar, M. Rajaram, A. Natarajan, L. Harikrishnan, K. Alwar and A. Rajaram, Highly Efficient Sulfur and Nitrogen Codoped Graphene Quantum Dots as a Metal-Free Green Photocatalyst for Photocatalysis and Fluorescent Ink Applications, ACS Omega, 2022, 7, 12825–12834 CrossRef CAS PubMed.
- M. Tahir, A. Sherryna, A. A. Khan, M. Madi, A. Y. Zerga and B. Tahir, Defect Engineering in Graphitic Carbon Nitride Nanotextures for Energy Efficient Solar Fuels Production: A Review, Energy Fuels, 2022, 36, 8948–8977 CrossRef CAS.
- K. P. Gopinath, N. V. Madhav, A. Krishnan, R. Malolan and G. Rangarajan, Present applications of titanium dioxide for the photocatalytic removal of pollutants from water: A review, J. Environ. Manage., 2020, 270, 110906 CrossRef CAS PubMed.
- S. J. Lee, J. Theerthagiri, P. Nithyadharseni, P. Arunachalam, D. Balaji, A. Madan Kumar, J. Madhavan, V. Mittal and M. Y. Choi, Heteroatom-doped graphene-based materials for sustainable energy applications: A review, Renewable Sustainable Energy Rev., 2021, 143, 110849 CrossRef CAS.
- A. Cai, H. He, Q. Zhang, Y. Xu, X. Li, F. Zhang, X. Fan, W. Peng and Y. Li, Synergistic Effect of N-Doped sp2 Carbon and Porous Structure in Graphene Gels toward Selective Oxidation of C–H Bond, ACS Appl. Mater. Interfaces, 2021, 13, 13087–13096 CrossRef CAS PubMed.
- J. Wang, Y. Asakura, T. Hasegawa and S. Yin, High-concentration N-doped La2Ti2O7 nanocrystals: Effects of nano-structuration and doping sites on enhancing the photocatalytic activity, J. Chem. Eng., 2021, 423, 130220 CrossRef CAS.
- R. Yuksel, O. Buyukcakir, P. K. Panda, S. H. Lee, Y. Jiang, D. Singh, S. Hansen, R. Adelung, Y. K. Mishra, R. Ahuja and R. S. Ruoff, Necklace-like Nitrogen-Doped Tubular Carbon 3D Frameworks for Electrochemical Energy Storage, Adv. Funct. Mater., 2020, 30, 1909725 CrossRef CAS.
- S. Akash, B. Sivaprakash, N. Rajamohan, M. Govarthanan and B. T. Elakiya, Remediation of pharmaceutical pollutants using graphene-based materials – A review on operating conditions, mechanism and toxicology, Chemosphere, 2022, 306, 135520 CrossRef CAS PubMed.
- Y. Wei, Y. Kong, Q. Yue, H. Dan, X. Cheng, L. Feng, W. Yin, B. Gao and Y. Gao, Magnetic field assisted synthesis of JANUS Fe3C@ Enteromorpha doped graphene aerogels for simultaneous recovery of fresh water and salt in high salinity wastewater, Sep. Purif. Technol., 2023, 308, 122845 CrossRef CAS.
- S. Jung, Y. Myung, B. N. Kim, I. G. Kim, I.-K. You and T. Kim, Activated Biomass-derived Graphene-based Carbons for Supercapacitors with High Energy and Power Density, Sci. Rep., 2018, 8, 1915 CrossRef PubMed.
- F. Han, O. Qian, B. Chen, H. Tang and M. Wang, Sugar blowing-assisted reduction and interconnection of graphene oxide into three-dimensional porous graphene, J. Alloys Compd., 2018, 730, 386–391 CrossRef CAS.
- S. Shukla, I. Khan, V. K. Bajpai, H. Lee, T. Kim, A. Upadhyay, Y. S. Huh, Y.-K. Han and K. M. Tripathi, Sustainable Graphene Aerogel as an Ecofriendly Cell Growth Promoter and Highly Efficient Adsorbent for Histamine from Red Wine, ACS Appl. Mater. Interfaces, 2019, 11, 18165–18177 CrossRef CAS PubMed.
- Y. Kong, H. Dan, W. Kong, Y. Gao, Y. Shang, K. Ji, Q. Yue and B. Gao, Self-floating maize straw/graphene aerogel synthesis based on microbubble and ice crystal templates for efficient solar-driven interfacial water evaporation, J. Mater. Chem. A, 2020, 8, 24734–24742 RSC.
- I. Lee, S.-M. Kang, S.-C. Jang, G.-W. Lee, H. E. Shim, M. Rethinasabapathy, C. Roh and Y. S. Huh, One-pot gamma ray-induced green synthesis of a Prussian blue-laden polyvinylpyrrolidone/reduced graphene oxide aerogel for the removal of hazardous pollutants, J. Mater. Chem. A, 2019, 7, 1737–1748 RSC.
- J. Kaushik, Gunture, K. M. Tripathi, R. Singh and S. K. Sonkar, Thiourea-functionalized graphene aerogel for the aqueous phase sensing of toxic Pb(II) metal ions and H2O2, Chemosphere, 2022, 287, 132105 CrossRef CAS PubMed.
- G. S. Das, A. Bhatnagar, P. Yli-Pirilä, K. M. Tripathi and T. Kim, Sustainable nitrogen-doped functionalized graphene nanosheets for visible-light-induced photocatalytic water splitting, Chem. Commun., 2020, 56, 6953–6956 RSC.
- V. Kumar, A. Kumar, D.-J. Lee and S.-S. Park, Estimation of Number of Graphene Layers Using Different Methods: A Focused Review, Materials, 2021, 14, 4590 CrossRef CAS PubMed.
- R. Aggarwal, S. K. Sonkar and K. M. Tripathi, Visible-light promoted hydrogen production by diesel soot derived onion like carbon nanoparticles, Carbon, 2023, 208, 436–442 CrossRef CAS.
- M. Karaca, Z. Eroğlu, Ö. Açışlı, Ö. Metin and S. Karaca, Boosting Tetracycline Degradation with an S-Scheme Heterojunction of N-Doped Carbon Quantum Dots-Decorated TiO2, ACS Omega, 2023, 8, 26597–26609 CrossRef CAS PubMed.
- C. Das, T. Shafi, S. Pan, M. Naushad, B. K. Dubey and S. Chowdhury, MoS2 Nanoflowers Decorated on Graphene Aerogels for Visible-Light-Driven Photocatalytic Degradation of Tetracycline, ACS Appl. Nano Mater., 2023, 6, 12991–13000 CrossRef.
- J. Kaushik, C. Sharma, N. K. Lamba, P. Sharma, G. S. Das, K. M. Tripathi, R. K. Joshi and S. K. Sonkar, 3D Porous MoS2-Decorated Reduced Graphene Oxide Aerogel as a Heterogeneous Catalyst for Reductive Transformation Reactions, Langmuir, 2023, 39, 12865–12877 CrossRef CAS PubMed.
- X.-T. Jing, Z. Zhu, L.-W. Chen, D. Liu, H.-Z. Huang, W.-J. Tian and A.-X. Yin, Boosting CO2 Electroreduction on Bismuth Nanoplates with a Three-Dimensional Nitrogen-Doped Graphene Aerogel Matrix, ACS Appl. Mater. Interfaces, 2023, 15, 20317–20324 CrossRef CAS PubMed.
- R. Zhang and Q. Meng, Ultralight, superhydrophobic, low-cost nitrogen-doped graphene aerogels with a “honeycomb” porous structure for efficient oil removal, New J. Chem., 2023, 47, 2706–2710 RSC.
- M. Park, A. Sharma, C. Kang, J. Han, K. M. Tripathi and H.-J. Lee, N-Doped Carbon Nanorods from Biomass as a Potential Antidiabetic Nanomedicine, ACS Biomater. Sci. Eng., 2022, 8, 2131–2141 CrossRef CAS PubMed.
- D. Dodoo-Arhin, F. P. Buabeng, J. M. Mwabora, P. N. Amaniampong, H. Agbe, E. Nyankson, D. O. Obada and N. Y. Asiedu, The effect of titanium dioxide synthesis technique and its photocatalytic degradation of organic dye pollutants, Heliyon, 2018, 4, e00681 CrossRef PubMed.
- Y. Yang, N. Ali, A. Khan, S. Khan, S. Khan, H. Khan, S. Xiaoqi, W. Ahmad, S. Uddin, N. Ali and M. Bilal, Chitosan-capped ternary metal selenide nanocatalysts for efficient degradation of Congo red dye in sunlight irradiation, Int. J. Biol. Macromol., 2021, 167, 169–181 CrossRef CAS PubMed.
- A. Bhati, S. R. Anand, Gunture, A. K. Garg, P. Khare and S. K. Sonkar, Sunlight-Induced Photocatalytic Degradation of Pollutant Dye by Highly Fluorescent Red-Emitting Mg-N-Embedded Carbon Dots, ACS Sustainable Chem. Eng., 2018, 6, 9246–9256 CrossRef CAS.
- S. Mosleh, M. R. Rahimi, M. Ghaedi, K. Dashtian and S. Hajati, Photocatalytic degradation of binary mixture of toxic dyes by HKUST-1 MOF and HKUST-1-SBA-15 in a rotating packed bed reactor under blue LED illumination: central composite design optimization, RSC Adv., 2016, 6, 17204–17214 RSC.
- M.-L. Xu, X.-J. Jiang, J.-R. Li, F.-J. Wang, K. Li and X. Cheng, Self-Assembly of a 3D Hollow BiOBr@Bi-MOF Heterostructure with Enhanced Photocatalytic Degradation of Dyes, ACS Appl. Mater. Interfaces, 2021, 13, 56171–56180 CrossRef CAS PubMed.
- Y. Xiao, J. Liu, J. Mai, C. Pan, X. Cai and Y. Fang, High-performance silver nanoparticles coupled with monolayer hydrated tungsten oxide nanosheets: The structural effects in photocatalytic oxidation of cyclohexane, J. Colloid Interface Sci., 2018, 516, 172–181 CrossRef CAS PubMed.
- A. G. Olabi, M. A. Abdelkareem, T. Wilberforce and E. T. Sayed, Application of graphene in energy storage device – A review, Renewable Sustainable Energy Rev., 2021, 135, 110026 CrossRef CAS.
- J. Wang, Y. Asakura and S. Yin, Preparation of (Zn1+xGe)(N2Ox) nanoparticles with enhanced NOx decomposition activity under visible light irradiation by nitridation of Zn2GeO4 nanoparticles designed precisely, Nanoscale, 2019, 11, 20151–20160 RSC.
- K. S. Raju, G. S. Das and K. M. Tripathi, Nitrogen-doped carbon quantum dots from biomass as a FRET-based sensing platform for the selective detection of H2O2 and aspartic acid, RSC Sustainability, 2024, 2, 223–232 RSC.
- Gunture, J. Kaushik, A. K. Garg, D. Saini, P. Khare and S. K. Sonkar, Pollutant Diesel Soot Derived Onion-like Nanocarbons for the Adsorption of Organic Dyes and Environmental Assessment of Treated Wastewater, Ind. Eng. Chem. Res., 2020, 59, 12065–12074 CrossRef CAS.
- Gunture, A. Singh, A. Bhati, P. Khare, K. M. Tripathi and S. K. Sonkar, Soluble Graphene Nanosheets for the Sunlight-Induced Photodegradation of the Mixture of Dyes and its Environmental Assessment, Sci. Rep., 2019, 9, 2522 CrossRef CAS PubMed.
|
This journal is © The Royal Society of Chemistry 2024 |