Insights into the mechanism of persulfate activation by hollow MOF-derived carbon: electron transfer-triggered non-radical oxidization for antibiotic removal†
Received
18th September 2023
, Accepted 17th November 2023
First published on 17th November 2023
Abstract
Non-radical oxidation triggered by hollow MOF-derived carbon in persulfate-based AOPs shows potential for antibiotic wastewater remediation. However, the inherent relationship between the hollow structure and catalytic activity and the evolution mechanism involving the transformation from solid structures into hollow frameworks is not clear. Considering this, herein, hollow ZIF-8-derived carbon (HZC) was fabricated via TA etching and carbonization for the activation of PDS. The results indicated that HZC-800 exhibited an excellent antibiotic removal performance through electron-transfer mediated non-radical oxidation. Characterization studies revealed the key role of graphitic N in the catalytic reaction, which was linearly correlated with the kinetic constant (k) and a high graphitic N content enhanced the degradation of antibiotics. Further analysis suggested that the evolution mechanism from an ROS-dominated process in solid ZIF-8-derived carbon (ZC-800)/PDS to electron-transfer oxidation in HZC-800/PDS originated from the transformation into a hollow structure. Compared to solid ZC-800, hollow HZC-800 with a higher graphitic N and lower electron-withdrawing O group content exhibited an enhanced electron conductivity and was more conducive to PDS adsorption and forming activated PDS* for electron-transfer non-radical oxidation, reducing the direct activation of PDS into ROS. HZC-800 with a larger porosity and more defects facilitated the mass diffusion for antibiotic removal with great practicality. This study provides a new insight into the evolution mechanism for the transformation from solid structures into hollow structures and designing carbon catalysts for wastewater treatment.
Environmental significance
Non-radical oxidation in persulfate-based AOPs shows potential for antibiotic wastewater treatment. In this work, hollow ZIF-derived carbon was fabricated via tannic acid etching and carbonization, which showed effective antibiotic removal in PDS activation by triggering a non-radical pathway. The mechanism study revealed the vital role of graphitic N in electron-transfer non-radical oxidization and the evolution from a ROS-driven radical process to an electron-transfer non-radical process from the solid structure to hollow structure transformation. This work provides an insight into the structure–activity relationship for the fabrication of hollow high-performance MOF-derived carbon catalysts for wastewater remediation.
|
1. Introduction
Antibiotics as emerging organic pollutants have attracted significant concern due to their induced environmental pollution. Especially, in water environments, the residue and accumulation of antibiotics cause significant ecological issues and pose potential risks to human health, and antibiotic resistance genes (ARGs) originating from antibiotics bring more challenges to medical security.1 Focusing on antibiotic removal, advanced oxidation processes (AOPs) based on activating persulfate exhibit a high efficiency by generating free radicals with a high redox potential (e.g., SO4˙− (2.5–3.1 V) and ˙OH (1.8–2.7 V)) to oxidize antibiotics.2 However, the non-selectivity of free radicals towards contaminants and the coexistence of anions and dissolved organic matter in complex water matrices inevitably result in the inefficient elimination of pollutants by a free radical process in persulfate activation,3,4 thus hindering its practical application. Compared to the free radical process, the non-radical process exhibits a high selectivity for electron-rich organics and a high efficiency in background water matrices without producing toxic halogen-containing products.5 Accordingly, instead of the free radical pathway, non-radical-dominated pollutant removal in persulfate activation becomes attractive, receiving much interest from researchers.
Typically, towards the non-radical process, there are three mainstream pathways for pollutant removal: singlet oxygen-involving (1O2), high-valent metal-triggered oxidation, and electron-transfer processes.6 Hence, the key to activating PDS via non-radical pathways is designing high-performance catalysts. Presently, carbon-based catalysts, such as graphene oxide, carbon nanotubes, and biochar, are widely adopted in persulfate activation. Among them, MOF-derived carbon with an adjustable morphology and controlled functional sites has been proven to be a potential catalyst for persulfate activation.7 Given the skeleton constitution of MOFs containing metal centers, the metal is partially retained during carbonization and usually combined with O and N, which is further encapsulated in the carbon framework. The retained metal oxides, metal nitrides (Fe–Nx, Co–Nx, etc.), or zero-valent metals are widely reported and recognized as active sites to excite persulfate for pollutant degradation via a non-radical or free radical process in MOF-derived carbon/persulfate systems.8 Nevertheless, during the catalytic reaction, the low mass diffusion and transformation among catalysts, persulfate, and pollutants and limited accessibility of active sites by the microporous-dominated solid structure of MOF-derived carbon hinder a further enhancement in catalytic performance.9
Recently, the fabrication of hollow structures in MOF-derived carbon via morphological control was demonstrated to be effective in tailoring their structure–activity relationships.10,11 In contrast to the solid carbon skeleton, hollow carbon structures shorten mass diffusion, enhance the electron transport efficiency, and improve the accessibility of catalytic sites, achieving a higher performance. For instance, hollow carbon-supported Co3O4 exhibited enhanced degradation of bisphenol A compared with solid carbon-supported Co3O4, given that PMS activation was facilitated in the hollow skeleton with more SO4˙− generated by the transition of Co(II)/Co(III).12 Li et al. found that carbonization of core–shell ZIF-8@ZIF-67 resulted in the formation of abundant Co–Nx sites on hollow N-doped carbon, which was recognized as the key to deriving singlet oxygen and direct electron-transfer-mediated non-radical reactions towards the degradation of BPA.13 Moreover, according to another study, it seems that with the introduction of metal, the persulfate activation pathway in metal-containing hollow carbon was dominated by the metal-bound species, where metal nitrides were more inclined to trigger a non-radical reaction than metal oxides or metal nanoparticles driving the free radical process.14,15 Accordingly, the evolution of the hollow structure on the effect of persulfate activation is not clear, and the contribution of other possible catalytic sites such as pure N configuration and O groups is unknown. Besides, secondary pollution caused by metal etching during catalytic reactions remains an inevitable problem. Considering the above-mentioned facts and the lack of research on metal-free hollow carbon for persulfate activation, the exploration of the relationship between hollow structures and catalytic activity is valuable.
In this study, hollow ZIF-8-derived carbon (HZC) was successfully fabricated via tannic acid (TA) etching of a ZIF-8 precursor to obtain a hollow skeleton and subsequent carbonization, where Zn was removed by volatilization and pickling. The PDS activation performance of HZC and solid ZIF-8-derived carbon (ZC) was assessed with tetracycline (TC). Based on various characterizations, the evolution in morphological and structural characteristics was distinguished. Further, the identification of ROS by quenching and EPR and electrochemical analysis revealed the mechanism evolution of PDS activation in the HZC/PDS and ZC/PDS systems. Based on this, the structure–activity relationship was further quantified and insight into the mechanism transformation from a solid structure to a hollow skeleton was proposed. Besides, the degradation pathways of TC and the factors influencing the HZC/PDS system were evaluated, as well as its reusability and practicality towards actual water. This study revealed the changes in the PDS activation pathways in the evolution from solid carbon to hollow carbon in metal-free MOF-derived carbon catalysts and is expected to provide a reference for the design of high-performance carbon catalysts for wastewater remediation.
2. Experimental
2.1 Chemicals
The chemicals employed in the experiment were used as received without further purification including 2-methylimidazole (MIM, 98%), methanol (99.7%), tannic acid (TA, AR), tetracycline (TC, 99.7%), zinc nitrate hexahydrate (Zn(NO3)2·6H2O, 99%), ethanol (99.7%), sodium persulfate (PDS, Na2S2O8, 97%), sodium carbonate (Na2CO3, 99%), sodium bicarbonate (NaHCO3, 99%), trisodium phosphate dodecahydrate (Na3PO4·12H2O, 98%), sodium chloride (NaCl, 99.5%), furfuryl alcohol (C5H6O2, 98%), potassium iodide (KI, AR), p-benzoquinone (C6H4O2, 98%), tertiary butanol (C4H10O, 98%), and ultrapure water (18.25 MΩ cm−1).
2.2 Synthesis
ZIF-8.
Briefly, 1.19 g Zn(NO3)2·6H2O and 1.232 g MIM were dissolved separately in methanol (30 mL). Then, the two solutions were mixed and stirred for 24 h at 100 rpm. ZIF-8 was obtained via centrifugation, washing with ethanol, and vacuum drying.
H-ZIF-8.
ZIF-8 dispersed in solution (4 mg mL−1) was etched with TA (5 mg L−1) under stirring for 10 min. The produced H-ZIF-8 was washed with ultrapure water until the pH reached neutral and vacuum dried for 6 h.
HZC-X (X = 700/800/900).
In a nitrogen atmosphere, H-ZIF-8 was carbonized in a tube furnace at specific temperatures (700/800/900 °C, 5 °C min−1) for 120 min with a natural cooling process. The black products were further washed with 1 M HCl and ultrapure water and vacuum dried for 6 h, which were denoted as HZC-X (X = 700/800/900) according to the carbonization temperature. For comparison, ZIF-8 carbonized at 800 °C under the same conditions as above was denoted as ZC-800.
2.3 Characterization
The analysis of morphological features and crystal configuration was based on scanning electron microscopy (SEM, Zeiss Sigma 3000), transmission electron microscopy (TEM, JEM-F200), and X-ray diffraction (XRD, Bruker D8 Advance). The chemical composition and degree of graphitization were detected by X-ray photoelectron spectroscopy (XPS, Thermo Scientific K-Alpha) and Raman spectroscopy (Horiba LabRAM HR Evolution). N2 adsorption–desorption tests were conducted using a Brunauer–Emmett–Teller (BET) analyzer (Micromeritics ASAP 2020). Electrochemical measurements such as linear sweep voltammetry (LSV) and chronoamperometry were carried out using an electrochemical workstation (CHI 66e).
2.4 Degradation experiment
To evaluate the performance of HZC-X (X = 700/800/900) and ZC-800, before the degradation reaction, TC solution (50 mL, 30 mg L−1) was mixed with 10 mg catalyst to reach adsorption equilibrium and shaken at 160 rpm and fixed temperatures. Then, PDS (15 mg) was added to the system. The filtered sample was quenched with methanol and analyzed employing high-performance liquid chromatography (HPLC) to detect the residual concentration of TC. More details (quenching experiment, affecting factors, cyclic experiment, etc.) are displayed in the ESI.†
3. Results and discussion
3.1 Characterization
As displayed in Fig. 1a, ZIF-8 prepared via the self-assembly of Zn2+ and MIM appeared as a regular rhombic dodecahedron. To obtain a hollow structure, TA was employed as an in situ etching agent to fabricate hollow ZIF-8. With the addition of TA, driven by the interaction between Zn2+ and plenty of catechol or galloyl in TA, TA was firstly adsorbed and coated on the surface of ZIF-8 as a protective layer.16 Subsequently, the H+ released by TA attacked the Zn–N bonds in the core of ZIF-8, ultimately leading to the formation of hollow ZIF-8.17 As shown in Fig. 1b, with the etching of TA, partially broken hollow structures of H-ZIF-8 were observed, indicating the effectiveness of etching by TA. After carbonizing ZIF-8, there was no obvious change in ZC-800 (Fig. 1c and d). Compared with ZC-800, HZC-800 became less regular (Fig. 1e and g), while the carbonized hollow structure also could be seen in the broken HZC-800 (Fig. 1f). Further, regarding the effect of the carbonization temperature, Fig. S1† exhibits the similar structures of HZC-700 and HZC-900, suggesting the negligible change in morphology. Moreover, TEM provided a clearer observation of the structural evolution. As displayed in Fig. 1i, ZC-800 was a solid carbon structure. Differing from ZC-800, an evident hollow skeleton with a carbon shell could be seen in HZC-800 (Fig. 1h). Hence, by etching with TA and carbonization of ZIF-8, HZC with a hollow carbon structure was successfully constructed.
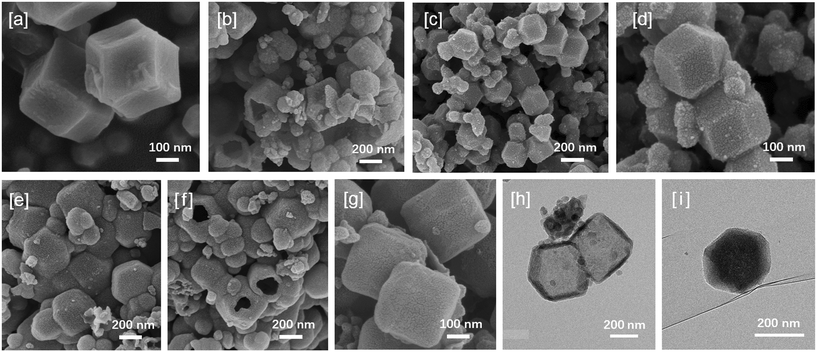 |
| Fig. 1 The SEM images of ZIF-8 (a), H-ZIF-8 (b), ZC-800 (c and d), and HZC-800 (e–g). The TEM images of HZC-800 (h) and ZC-800 (i). | |
XRD and Raman spectroscopy were conducted for the characterization of the material composition and electronic structure. As shown in Fig. S2a,† ZIF-8 with sharp crystalline peaks conformed to the simulated pattern of ZIF-8, indicating its effective synthesis. As displayed in Fig. 2a, the XRD patterns of the carbonized samples possessed two broad diffraction peaks at 2θ values around 24° and 44°, corresponding to the (002) and (101) diffractions of graphitized carbon, respectively.18 Considering the presence of Zn in ZIF-8, no diffractions corresponding to Zn or oxidized Zn appeared in HZC, indicating that the content of Zn was low content and effectively removed via volatilization during pyrolysis and acid washing. The Raman spectra of the different samples further demonstrated their degree of graphitization (Fig. 2b). The distinct characteristic peaks located at 1340 cm−1 (D band) and 1580 cm−1 (G band) match disordered and graphited carbon, respectively, and their intensity ratio (ID/IG) can represent the degree of graphitization.19 According to the calculated result, with an increase in the carbonization temperature, the value of ID/IG decreased from 4.56 (HZC-700) to 4.18 (HZC-800) and 3.49 (HZC-900). This indicates that a higher pyrolysis temperature turned the disordered carbon into ordered carbon, resulting in higher graphitization with fewer defects in the carbon skeleton. Meanwhile, compared with ZC-800 (ID/IG = 3.18), HZC with a higher ID/IG ratio possessed more structural defects, which was caused by the etching of TA and the formation of hollow configuration in the carbon skeleton, as reflected in the SEM and TEM images.
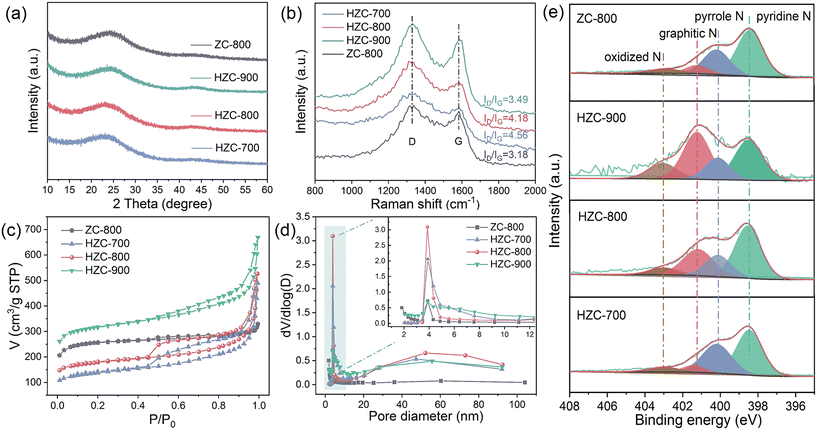 |
| Fig. 2 The XRD spectra (a), Raman spectra (b), N2 adsorption–desorption curves (c), pore diameter distribution (d), and N 1s spectra (e) of HZC-x (x = 700/800/900) and ZC-800. | |
Based on the above analysis of HZC with more defects than ZC-800, a specific surface and porosity analyzer was employed to measure the change in structure performance. The isotherm of the original ZIF-8 (Fig. S2b†) was a representative type I isotherm with a high adsorption amount of N2 at low P/P0 due to its abundant micropores. As depicted in Fig. 2c, after carbonization, the isotherm of ZC-800 still tended to be a type I isotherm, while the isotherm of HZC with increasing N2 adsorption amount at medium P/P0 was recognized as a type IV isotherm coupled with an obvious hysteresis loop (type H4), indicating the existence of mesoporous besides micropores.20 This demonstrates that TA etching significantly altered the pore structure of the samples. The pore size distribution (Fig. 2d) further illustrated the significant increase in the mesoporous region of HZC in contrast to ZC-800. Moreover, as listed in Table 1, the pore size of HZC was larger than ZC-800, and HZC-800 showed the largest pore size of 9.42 nm. Also, the increased carbonized temperature improved the BET surface area, micropore volume, and total pore volume of HZC. However, under the same carbonization condition, the surface area of HZC-800 (587.5 m2 g−1) was less than that of ZC-800 (832.2 m2 g−1) which possessed abundant micropores in the framework, but HZC-800 exhibited a larger pore size and total pore volume. Hence, the result demonstrated an enhancement in pore size and porosity via the fabrication of hollow structures with TA etching and carbonization, which was more conducive to diffusion and mass transfer during the catalytic process.
Table 1 The parameters of structural performance of HZC-X and ZC-800
Sample |
BET surface areaa (m2 g−1) |
Pore sizeb (nm) |
V
t
(cm3 g−1) |
V
m
(cm3 g−1) |
Determined by the Brunauer–Emmett–Teller (BET) method.
Calculated via Barrett–Joyner–Halenda (BJH) method.
Total pore volume measured at P/P0 = 0.99.
t-Plot micropore volume.
|
HZC-700 |
460.9 |
9.18 |
0.7576 |
0.1148 |
HZC-800 |
587.5 |
9.42 |
0.8142 |
0.1827 |
HZC-900 |
1035.2 |
8.74 |
1.0344 |
0.3541 |
ZC-800 |
832.2 |
4.28 |
0.5093 |
0.3015 |
Moreover, the XPS survey exhibited four main peaks in the different samples (Fig. S3a†), corresponding to C, N, O, and Zn. As the carbonized temperature increased, the reduced intensity of the peaks related to Zn and N implied that a higher temperature led to the removal of Zn and loss of N, coupled with a higher C content. Also, as shown in Table S2,†via TA etching and carbonization, the O content in HZC-800 (5.76%) significantly decreased compared with ZC-800 (19.60%), as well as the Zn content. According to the high-resolution spectra (Fig. S3b†), the fitted C 1s contained C–C/C
C (284.8 eV), C–N/C–O (286.2 eV), C
O (287.6 eV), and π–π* shake up peaks (289.3 eV).21,22 The relative content of C–N/C–O and C
O increased from 21.6% (HZC-700) to 28.6% (HZC-800) and reduced to 24.5% (HZC-900), which indicates that an excessive temperature caused the breaking of the C–N or C–O bonds. In the case of N 1s, as shown in Fig. 2e, the four fitting peaks were identified to be pyridine N (398.4 eV), pyrrole N (400.1 eV), graphitized N (401.2 eV), and oxidized N (403.0 eV).23,24 It is clear that an increase in temperature turned more N configurations into graphitized N, which favored the catalytic performance, given that graphitized N could facilitate the electron transfer efficiency by regulating the charge density of the carbon framework.25 Besides, in contrast to ZC-800 with a lower content of graphitized N (0.89%), HZC-800 also showed a much higher content of graphitized N (2.01%) (Table S3†). This result manifests that a hollow structure was easier to induce the formation of graphitized N during pyrolysis,13 thus enhancing the properties of the carbon material with N doping.
3.2 PDS activation performance
The PDS activation performance of the carbonized samples with or without TA etching was assessed using TC, a typical antibiotic. In the absence of the catalyst, PDS without activation hardly degraded TC. As PDS was activated by HZC or ZC-800, TC was effectively degraded (Fig. 3a). Regarding the carbonization temperatures, HZC-800 exhibited a better removal efficiency (99.4%) than HZC-700 (85.0%) and HZC-900 (95.0%), and their corresponding kinetic constants (k) were 0.048 (HZC-700), 0.122 (HZC-800), and 0.090 min−1 (HZC-900), respectively (Fig. 3b), indicating that a lower or higher carbonization condition weakened the performance of HZC. Simultaneously, compared with ZC-800, HZC equipped with a hollow structure showed an enhanced adsorption performance, which can be attributed to its enlarged porosity, pore size, and abundant defects. Also, the TC removal efficiency and corresponding k of HZC-800 were higher than that of ZC-800 (91.4%, k = 0.065 min−1). As evidenced by the analysis of the characterization results, the improved performance was derived from the hollow skeleton associated with more defects, enhanced porosity, and high content of graphitized N, and thus HZC-800 was employed in the following experiments.
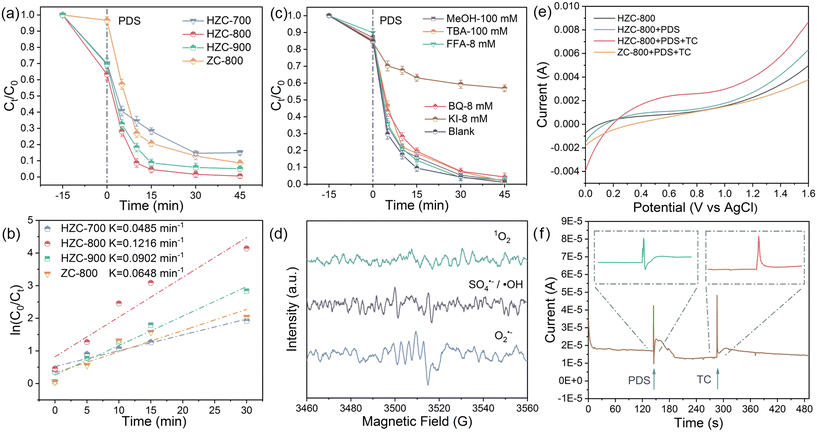 |
| Fig. 3 A comparison of different catalytic systems (a) and the corresponding kinetic fitting (b) and quenching experiment (c). Conditions: V = 50 mL, C0 = 30 mg L−1, PDS = 0.3 g L−1, catalyst = 0.2 g L−1, and T = 298 K. The EPR experiment result (d), LSV curves of different systems, (e) and chronoamperometry curves (f). | |
3.3 Identification of ROS
To uncover the predominant ROS in the HZC-800/PDS system, quenching experiments were conducted. Given the high reaction rates of scavengers with ROS, MeOH and TBA were applied to quench SO4˙− and ˙OH.26 As presented in Fig. 3c, with the addition of MeOH or TBA (100 mM), there was a negligible reduction in TC degradation compared with the blank group, suggesting the small contribution of SO4˙− and ˙OH in the degradation process. Besides, O2˙− is another important free radical in PDS activation, which can be quenched and recognized by BQ.27 The quenching result implies that the introduction of BQ (8 mM) had little effect on the removal of TC. Therefore, O2˙− fractionally contributed to TC oxidization in the HZC-800/PDS system. The above-mentioned analysis indicates that TC degradation did not rely on the free radical pathway, while the non-radical pathway played a key role in the HZC-800/PDS catalytic system. Aiming at the non-radical pathway, 1O2, as a type of vital ROS, was further identified via the quenching effect of FFA.28 Clearly, FFA (8 mM) resulted in a negligible inhibition towards TC removal, demonstrating the minimal contribution of 1O2 to oxidize TC.
To further prove the conclusion obtained from the quenching experiment, EPR (electron paramagnetic resonance) that could in situ verify the existence of ROS in the HZC-800/PDS system was employed. As displayed in Fig. 3d, when DMPO served as the spin-trapping agent towards SO4˙−/˙OH and O2˙−,29 tiny and indistinct characteristics of DMPO–SO4˙− or DMPO–˙OH adducts appeared, which was the same with the signal of the DMPO–O2− adduct. This result also manifests their minimal contribution to the degradation of TC. Meanwhile, when TEMP was added for trapping 1O2, there was no three-line signal for the TEMP-1O2 adduct, suggesting the absence of 1O2 in the HZC-800/PDS oxidation system. Overall, the result of EPR testing is in agreement with the quenching experiments for ROS, both proving that TC removal in the HZC-800/PDS systems was not dependent on ROS-determined pathways (SO4˙−, ˙OH, O2˙−, and 1O2) but other degradative pathways.
3.4 Electron-transfer non-radical pathway
Based on the above-mentioned analysis, it can be concluded that TC degradation in the HZC-800/PDS system did not rely on ROS-dominating oxidation related to the free radical pathway (SO4˙−, ˙OH, etc.) and 1O2-based non-radical pathway. Therefore, the electron-transfer mediated non-radical pathway can participate in the HZC-800/PDS system for TC removal. KI is usually recognized as the scavenger of surface-bound reactive complexes in electron-transfer non-radical oxidization.30 As expected, the presence of KI (8 mM) greatly inhibited the degradation of TC (Fig. 3c), where only 43.1% TC was removed, indicating the formation of reactive surface-bound PDS* that was quenched by KI. This result manifests that the electron-transfer pathway based on activated PDS* in the HZC-800/PDS system was the leading means to oxidize TC. Further, LSV measurement was conducted to evaluate the electron transfer process in the catalytic system among TC, PDS, and HZC-800.31 As displayed in Fig. 3e, compared with the HZC-800 system, as PDS was added, a higher current response was observed, suggesting the formation of metastable reactive complexes between HZC-800 and PDS coupled with electron transfer.32 In the presence of TC, the current response increased significantly in the HZC-800/PDS/TC ternary system. This signifies that rapid electron transfer occurred between activated PDS* and TC, thus driving the oxidization of TC. Moreover, the current response in the HZC-800/PDS/TC system was higher than that in the ZC-800/PDS/TC system, indicating a strengthened catalytic performance. In view of the observed directivity of electron transfer in LSV, chronoamperometry was applied to monitor the direction of current flow in the HZC-800/PDS/TC ternary system via the change in the positive or negative current signal.33 As shown in Fig. 3f, it can be seen that the injection of PDS resulted in a negative current signal, suggesting electron migration from HZC-800 to the adsorbed PDS. On the contrary, adding TC induced a positive current signal, which revealed that TC as an electron donor supplied electrons to the bonded PDS* on HZC-800, thus leading to the oxidation of TC. Besides, via the KI-based spectrophotometric method, the detection of PDS consumption in Fig. S4† depicted a faster consumption rate in HZC-800/PDS/TC than that in the HZC-800/PDS/water system due to the adsorbed and bound PDS on the surface of HZC-800 extracting electrons from the TC, expediting PDS consumption.
Further, in situ Raman and galvanic oxidation processes (GOP) were conducted to verify the electron-transfer non-radical pathway for TC degradation in the HZC-800/PDS system. As shown in Fig. 4a, the two characteristic peaks located at 836 and 1076 cm−1 belong to S2O82− in the PDS solution.34 When HZC-800 was added, besides the peaks of S2O82−, a new peak (812 cm−1) emerged, which corresponds to the activated S2O82−* species produced via the bending vibrations of the prolonged O–O bond in the HZC-800-PDS* complex.35 This result suggests the formation of activated PDS* on HZC-800, which contributed to pollution degradation via electron transfer. GOP was conducted with PDS and TC separately in two half-cells connected by a salt bridge using graphite electrodes coated with HZC-800. As exhibited in Fig. 4b, as TC was added to the TC cell without adding PDS to the PDS cell, there was no obvious current detected. However, when PDS and TC were added to the PDS and TC cells, respectively, a strong current was detected, which increased rapidly to the maximum and gradually declined. This result illustrates that HZC-800 interacted with PDS and formed surface-bound PDS*, which promoted the direct electron transfer oxidization of TC.36 The above-mentioned analysis provides evidence that the degradation of TC in the HZC-800/PDS system is ascribed to the electron transfer process via the formation of activated PDS* to degrade TC.
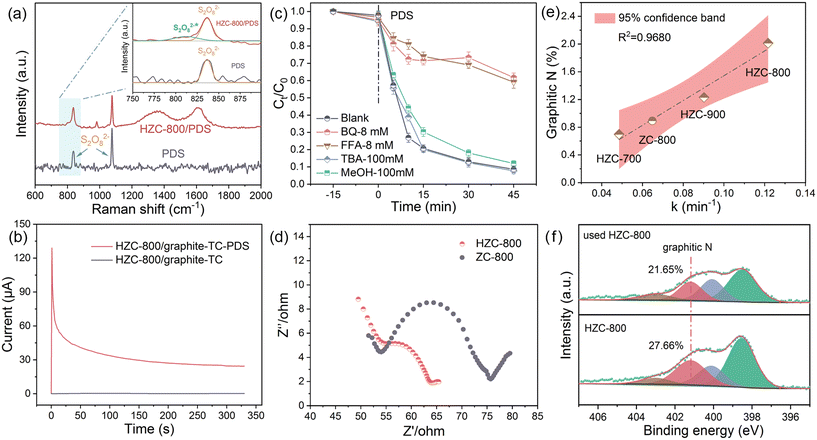 |
| Fig. 4 The in situ Raman spectra of PDS and HZC-800/PDS (a). Current in the galvanic oxidation processes (GOP) (b). The quenching experiments of ZC-800/PDS/TC system (c). Conditions: V = 50 mL, C0 = 30 mg L−1, PDS = 0.3 g L−1, catalyst = 0.2 g L−1, T = 298 K, pH = 4.3; EIS of ZC-800 and HZC-800 (d). The correlation analysis of N configuration with kinetic constants (e) and the N 1s spectra of fresh and used HZC-800 (f). | |
3.5 Insight into the mechanism transformation
In this work, effective etching by TA and carbonization successfully transformed ZIF-8 into HZC-800 with a hollow carbon structure. As revealed by the above-mentioned characterization analysis, HZC-800 exhibited more structural defects, larger pore size and porosity, and higher content of graphitic N in comparison with ZC-800, which was more conducive to the PDS activation and TC degradation according to the better removal efficiency of HZC-800 (Fig. 3a). However, the performance change derived from structural transformation was not clear. Thus, insight into the relationship between structure and performance was explored, focusing on the mechanism transformation resulting in a strengthened capacity in PDS activation for TC oxidation. Firstly, ROS quenching experiments for the ZC-800/PDS/TC system under the same conditions as the HZC-800/PDS/TC system were conducted. The result in Fig. 4c implies that TC degradation was significantly inhibited by the presence of BQ and FFA, while the effect of MeOH and TBA was also negligible, suggesting the pivotal role of O2˙− and 1O2 in the ZC-800/PDS system aimed at TC removal. Meanwhile, in the probe experiments employing nitro-blue tetrazolium (NBT) for O2˙− and 1,3-diphenylisobenzofuran (DPBF) for 1O2 (Fig. S5c and d†), the fast consumption of NBT and DPBF in the ZC-800/PDS system suggested the existence and production of O2˙− and 1O2 to degrade NBT and DPBF, respectively.37,38 Further, the oxygen-limited experiment under N2 and co-existing ions resulted in reduced TC removal, demonstrating the contribution of O2˙− to the degradation of TC (Fig. S5a and b†). Besides, the lower current response in the ZC-800/PDS/TC system in LSV (Fig. 3e) and two positive current signals when adding PDS and TC in the i–t curve (Fig. S6†) corresponded to PDS activation coupled with the production of ROS for the further degradation of TC, both accounting for the ROS-based catalytic reaction in the ZC-800/PDS system. Hence, TC removal in the ZC-800/PDS system was more dependent on an ROS-dominated process, differing from the electron transfer-mediated process by generating reactive PDS* in the HZC-800/PDS system.
Generally, the electron transfer-mediated process in carbonaceous catalysts is related to their higher electron conductivity.39 Accordingly, EIS (electrochemical impedance spectroscopy) was adopted to assess the inherent electron transfer performance of HZC-800 and ZC-800. As displayed in the Nyquist diagram (Fig. 4d), in the high-frequency range, the radius of the semicircle of HZC-800 was smaller than that of ZC-800. A smaller radius corresponds to smaller electrochemical impedance with better conductivity.40 This indicates that HZC-800 possesses higher electron conductivity than ZC-800, which is more favorable for the electron-transfer mediated process in PDS activation for the degradation of TC. This result is also consistent with the LSV analysis, showing an enhanced current response in the HZC-800/PDS/TC system (Fig. 3e).
Previous studies implied that N doping in the carbon skeleton can significantly improve the performance of carbon-based catalysts by tailoring of the local electronic structures surrounding carbon.41 In this case, the XPS analysis suggested that an increasing carbonization temperature resulted in a lower N content with a higher proportion of graphitic N. Accordingly, the total content of graphitic N was 0.70% (HZC-700), 2.01% (HZC-800), 1.23% (HZC-900), and 0.89% (ZC-800). HZC-800 showed a higher graphitic N than HZC, which was 2.26-times that of ZC-800. As revealed in Fig. 4e, graphitic N possessed a high linear correlation (R2 = 0.9680) with the kinetic constant (k). This result implies that the graphitic N in the carbon framework is a vital active site in activating PDS, and a higher content of graphitic N can facilitate the activation of PDS for the removal of TC. Meanwhile, other N configurations (pyridinic N, pyrrolic N, and oxidized N) with a reduction trend had no significant correlation with k (Fig. S7a†). Therefore, a high content of graphitic N in HZC-800 would turn adjacent carbon into a positively charged state, promoting the reaction with the negatively charged O atoms of PDS to form reactive PDS* on HZC-800. The enhanced conductivity derived from more graphitic N induced the electron transfer from TC to activated PDS*, achieving TC oxidation, rather than directly breaking the O–O bond in PDS to generate ROS. Moreover, the XPS analysis of HZC-800 before and after use was compared. The N 1s spectrum (Fig. 4f) indicated a significant decrease in graphitic N from 27.66% to 21.65% after use, coupled with the increase in pyrrole N from 18.95% to 25.11%. This further proved that graphitic N played a crucial role in the formation of reactive PDS* in the surface of HZC-800 and further TC removal via electron transfer, which was partially transferred into pyrrole N during the catalytic reaction. Accordingly, ZC-800 with low graphitic N hindered the direct electron transfer reaction.
In addition to the N configuration, O functional groups such as C
O and C–O are also recognized as catalytic sites for PDS activation. Based on the fitting result of the O configurations described in Fig. S7b,† the poor correlation between oxygen groups and k demonstrated the limited role of O groups in the electron transfer non-radical oxidation for TC removal in the HZC/PDS system. However, in ZC-800, the content of O (19.6%) was 3.40-times that of HZC-800 (5.76%). The high content of O in ZC-800 with more C
O as an electron-withdrawing group was conducive to exciting PDS by extracting electrons from the carbon skeleton and donating electrons to PDS,42 coupled with ROS (1O2, O2˙−, etc.) produced directly for degrading TC. Moreover, overabundant O groups caused insufficient electrons in the carbon skeleton, weakened the conduction in the carbon framework, and led to a steric hindrance effect between the oxygen-occupied reactive edges and PDS,43 thus hindering the electron transfer process.
Therefore, the mechanism transformation from ROS-dominated catalytic process to electron transfer-mediated oxidization was related to the structural changes from a solid carbon structure (ZC-800) to hollow carbon shell skeleton (HZC-800). As revealed in Fig. 5, the hollow structure during carbonization was more favorable to form graphitic N, which was linearly correlated with the degradation kinetic constant, and a high content of graphitic N was key to enhancing the conductivity of HZC-800, as proven by EIS. Also, compared with ZC-800 with a high content of O-containing groups to directly activate PDS into ROS (O2˙− and 1O2), the obvious reduction of O-containing groups in HZC-800 weakened the direct excitation of PDS into ROS and favored the adsorption of PDS to form surface-bound activated PDS* due to the reduced steric hindrance. Besides, the enlarged pore size, higher pore volume, and more defects in the hollow skeleton with better mass transfer also contributed to the adsorption and degradation of TC. Consequently, in the ZC-800/PDS system, PDS was mainly activated into ROS for the removal of TC. Alternatively, in the HZC-800/PDS system, due to its higher graphitic N and lower O-containing group contents, forming regions with sufficient electron density in the hollow carbon shell, PDS adsorption was enhanced to form reactive PDS*, and then the oxidation of TC occurred via the electron transfer from TC to the activated PDS* on HZC-800, coupled with the reduction of PDS.
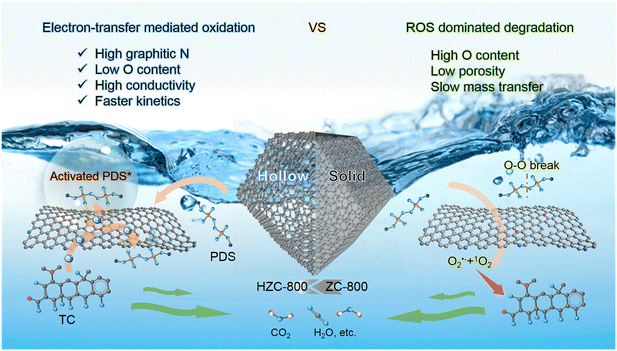 |
| Fig. 5 Insights into the mechanism for the degradation of TC on the HZC-800/PDS and ZC-800/PDS systems. | |
3.6 Possible degradation pathway
In the HZC-800/PDS system, TC degradation mainly depended on the electron transfer regime between TC and activated PDS*. The detection of TOC showed that the mineralization efficiency of TC was 62.95%, indicating the generation of intermediate products in the degradation of TC. Further, based on LC-MS, the intermediates were identified, as listed in Table S4,† and the possible pathway in degrading TC was proposed, as shown in Fig. 6a. The TC solution displayed a distinct MS signal at m/z = 445, corresponding to the TC molecules. As reported, TC degradation mainly involves deamination, hydroxyl addition, demethylation, and ring opening.44 According to the intermediates (P1–P6), hydroxyl addition occurred, coupled with deamination and demethylation, forming P6. Besides, P1 could also transfer to P6 via dihydroxylation and breaking of C–N. Subsequently, through ring-opening reactions, the produced P6 was further oxidized into by-products (P7–P11), eventually being degraded into the small molecules CO2 and H2O. Moreover, 3D EEM (excitation–emission matrix) spectroscopy was employed to monitor the transformation of TC during its oxidization. As shown in Fig. 6b, no obvious fluorescence signal appeared in the initial TC sample. As TC was degraded (Fig. 6c–e), two fluorescent peaks appeared at Ex/Em 280–320/380–440 nm and 350–420/460–540 nm, corresponding to humic acid-like compounds.45 Subsequently, the changed fluorescence signals at 15/30/45 min suggested that the TC molecules were translated into humic acid-like compounds, and then degraded into low molecular organic compounds.
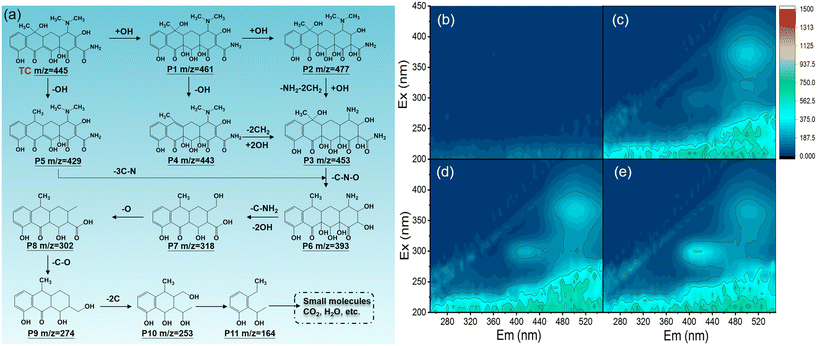 |
| Fig. 6 The TC degradation pathway (a); the 3D EEM spectra of the initial TC solution (b) and TC degradation for 15 min (c), 30 min (d), and 45 min (e). | |
3.7 Influence of operation conditions
Further, several key variables that significantly affected the removal of TC in PDS activation were investigated. The influence of initial pH, which can alter the charged state of pollutants and catalysts, was revealed,46 as described in Fig. 7a. It was observed that TC could be efficiently removed in the HZC-800/PDS system at pH 2–10. When the pH reached 12, a reduction in the removal of TC was observed with slow degradation kinetics. Given that the electron-transfer-mediated pathway via the formation of reactive PDS* was responsible for removing TC in the HZC-800/PDS system, the changed pH had no effect on ROS production to affect the removal of TC, while the changed state of TC and HZC-800 accounted for this. The dissociation of TC at the pH of 3.32, 7.78, and 9.58 made the TC molecules electropositive (pH < 3.32) or electronegative (pH > 9.58). According to Fig. S8,† the zero-potential point of HZC-800 was around pH 11, and HZC-800 was in an electropositive state when the pH was less than 11. This indicates that the strengthened electrostatic repulsion (pH < 3.3 or pH > 9.58) between HZC-800 and TC molecules hindered the electron transfer from TC to the activated PDS*. Additionally, the higher suppression effect at the pH of 12 can be ascribed to the unfavorable adsorption and integration of PDS to activate PDS due to the strong electrostatic resistance under alkaline conditions.
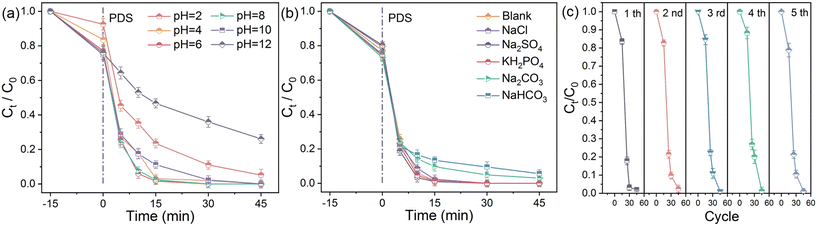 |
| Fig. 7 Factors influencing the removal of TC: pH (a) and anions (b); recycling experiments (c). Conditions: V = 50 mL, C0 = 30 mg L−1, PDS = 0.3 g L−1, catalyst = 0.2 g L−1, T = 298 K. | |
Given the complexity of natural water,47 the effect of anions was explored. As is known, anions (Cl−, HCO3−, etc.) in persulfate-based AOPs compete with target pollution for radicals such as SO4˙− and ˙OH, accompanied by the production of radicals with low oxidation capacity.48 In contrast, it was observed that in the HZC-800/PDS system, the coexistence of anions at a concentration of 5 mM (Cl−, SO42−, H2PO4−, HCO3−, and CO32−) exhibited little influence on the removal of TC with a high removal efficiency (Fig. 7b). The small inhibition by HCO3− and CO32− was most likely due to the enhanced pH caused by their hydrolysis. Thus, the degradation of TC in the HZC-800/PDS system through the electron transfer reaction was less affected by co-existing anions with high anti-interference ability. Besides, in persulfate-based AOPs, an increase in the reaction temperature usually improves the removal of pollutant with expedited degradation kinetics. As expected, when the temperature increased from 288 K to 308 K, the removal of TC was enhanced (Fig. S9a†), given that a higher temperature increased the reactivity of the system by accelerating the adsorption of TC and the interaction between TC and reactive PDS* on HZC-800.
3.8 Reusability and practicality
The assessment of the recycling performance of HZC-800 was conducted. As displayed in Fig. S10,† an obvious reduction in TC removal occurred in the second use without recovery, with only 72.0% TC being removed. According to the XPS, the content of N decreased and O content increased in the used HZC-800. Given the vital contribution of N in PDS activation, N 1s fitting further suggested that graphite N decreased from 27.66% to 21.65% after use (Fig. 4f). Hence, the weakened performance was ascribed to the reduction in graphite N and increase in O group contents, as well as the adsorbed intermediates on the surface of catalyst, resulting in a depressed catalytic performance. To recover the activity of the used HZC-800, the carbonization strategy was employed, which is widely used for carbon catalysts.49 The result in Fig. 7c indicates the effectiveness of the catalytic activity recovery, where the TC removal was at a high level in the fifth use. Also, compared with the used HZC-800, the content of graphite N increased to 24.65% after carbonization, coupled with a reduced O content (Tables S2 and S3†). This result proves that carbonization could recover the activity of HZC-800, aiming at improving the content of graphite N and reducing the content of O groups. Moreover, towards practicality, simulated TC degradation using actual water was carried out. The removal efficiency in Fig. S9b† indicates that TC was degraded efficiently in tap water and river water, while a decreased removal efficiency was observed in medical wastewater, which can be attributed to its complex composition.
4. Conclusion
In this work, to explore the inherent relationship between hollow structure and catalytic activity as well as mechanism evolution from a solid structure to a hollow framework, hollow HZC was successfully constructed by TA etching the core of ZIF-8 and carbonization for activating PDS. As expected, HZC-800 achieved the best TC removal efficiency among the different HZC via PDS activation, which was also better than the solid ZC-800. The quenching experiments, EPR, in situ Raman, GOP, and electrochemical analysis suggested that TC removal in the HZC-800/PDS system was mainly dependent on electron-transfer-mediated oxidation via the formation of activated PDS* on HZC-800, rather than directly exciting PDS into free radicals. It was found that graphitic N, which can improve the conductivity of the carbon framework, played a key role in the electron transfer process and linearly correlated with k (R2 = 0.9680), and XPS further proved that the reduced performance was related to the decreased content of graphitic N. Meanwhile, the hollow structure resulted in the mechanism transformation of PDS activation. In the solid ZC-800/PDS system, ROS oxidization dominated the removal of TC via electron donation by the abundant O groups (C
O) to excite PDS into O2˙− and 1O2. By contrast, a significantly increased graphitic N content and lower content of O groups in HZC-800 enhanced the conductivity of the hollow carbon skeleton with reduced steric-hindrance effect towards PDS, inducing the evolution of PDS activation from ROS-derived degradation (solid ZC-800) to electron-transfer-mediated oxidization (hollow HZC-800). Also, the higher porosity and more defects enhanced the mass transfer in HZC-800, achieving expedited TC removal. Benefitting from this, the HZC-800/PDS system exhibited an excellent performance in a wide pH range (2–10), in the presence of anion interferents (Cl−, H2PO4−, HCO3−, CO32−, etc.), and in simulated degradation using actual water matrices, coupled with good reusability. This study provides insight into the structure–activity relationship for the fabrication of hollow MOF-derived carbon and enriches the research on designing carbon catalysts for wastewater remediation.
Author contributions
Haihao Peng: data curation and writing – original draft; Weiping Xiong: supervision, funding acquisition and writing – reviewing & editing; Zhaohui Yang: supervision and funding acquisition; Jing Tong: writing – review & editing; Yinping Xiang: writing – review & editing; Zhenfeng Zhang: writing – review & editing; Zhengyong Xu: writing – review & editing.
Conflicts of interest
The authors declare that they have no known competing financial interests or personal relationships that could have appeared to influence the work reported in this paper.
Acknowledgements
The study was financially supported by the National Natural Science Foundation of China (52379064), the National Youth Foundation of China (52000064), the Natural Science Foundation of Hunan Province (2023JJ0013), the Special Funding for the Construction of Hunan's Innovative Province (2021SK2040), the Science and Technology Innovation Program of Hunan Province (2021RC3133), and the Hunan Municipal Natural Science Foundation (2023JJ41048).
References
- Z. Yu, H. Rabiee and J. Guo, Synergistic effect of sulfidated nano zerovalent iron and persulfate on inactivating antibiotic resistant bacteria and antibiotic resistance genes, Water Res., 2021, 198, 117141 CrossRef CAS PubMed.
- H. Peng, J. Tong, J. Huang, Z. Yang, W. Xiong, Y. Yao, Y. Xiang and Z. Xu, In-situ immobilization of MIL-100(Fe) on the microchannels in wood aerogel: Efficient persulfate activation toward antibiotic removal, Sep. Purif. Technol., 2023, 124195 CrossRef CAS.
- Y. Jiang, B. Gao, Z. Wang, J. Li, Y. Du, C. He, Y. Liu, G. Yao and B. Lai, Efficient wastewater disinfection by raised 1O2 yield through enhanced electron transfer and intersystem crossing via photocatalysis of peroxymonosulfate with CuS quantum dots modified MIL-101(Fe), Water Res., 2023, 229, 119489 CrossRef CAS.
- B. Sun, Y. Zheng, C. Shang and R. Yin, Concentration-dependent chloride effect on radical distribution and micropollutant degradation in the sulfate radical-based AOPs, J. Hazard. Mater., 2022, 430, 128450 CrossRef CAS PubMed.
- Y. Yao, C. Wang, X. Yan, H. Zhang, C. Xiao, J. Qi, Z. Zhu, Y. Zhou, X. Sun, X. Duan and J. Li, Rational regulation of Co-N-C coordination for high-efficiency generation of 1O2 toward nearly 100% selective degradation of organic pollutants, Environ. Sci. Technol., 2022, 56(12), 8833–8843 CrossRef CAS.
- W. Ren, C. Cheng, P. Shao, X. Luo, H. Zhang, S. Wang and X. Duan, Origins of electron-transfer regime in persulfate-based nonradical oxidation processes, Environ. Sci. Technol., 2022, 56(1), 78–97 CrossRef CAS.
- N. Li, R. Li, X. Duan, B. Yan, W. Liu, Z. Cheng, G. Chen, L. Hou and S. Wang, Correlation of active sites to generated reactive species and degradation routes of organics in peroxymonosulfate activation by Co-loaded carbon, Environ. Sci. Technol., 2021, 55(23), 16163–16174 CrossRef CAS PubMed.
- H. Dai, W. Zhou and W. Wang, Co/N co-doped carbonaceous polyhedron as efficient peroxymonosulfate activator for degradation of organic pollutants: Role of cobalt, Chem. Eng. J., 2021, 417, 127921 CrossRef CAS.
- Z. Wu, Z. Xiong, R. Liu, C. He, Y. Liu, Z. Pan, G. Yao and B. Lai, Pivotal roles of N-doped carbon shell and hollow structure in nanoreactor with spatial confined Co species in peroxymonosulfate activation: Obstructing metal leaching and enhancing catalytic stability, J. Hazard. Mater., 2022, 427, 128204 CrossRef CAS PubMed.
- X. Li, S. Wang, B. Xu, X. Zhang, Y. Xu, P. Yu and Y. Sun, MOF etching-induced Co-doped hollow carbon nitride catalyst for efficient removal of antibiotic contaminants by enhanced perxymonosulfate activation, Chem. Eng. J., 2022, 441, 136074 CrossRef CAS.
- C. Xiao, M. Zhang, C. Wang, X. Yan, H. Zhang, S. Chen, Y. Yao, J. Qi, S. Zhang and J. Li, 2D metal–organic framework derived hollow Co/NC carbon sheets for peroxymonosulfate activation, Chem. Eng. J., 2022, 444, 136385 CrossRef CAS.
- M. Abdul Nasir Khan, P. Kwame Klu, C. Wang, W. Zhang, R. Luo, M. Zhang, J. Qi, X. Sun, L. Wang and J. Li, Metal-organic framework-derived hollow Co3O4/carbon as efficient catalyst for peroxymonosulfate activation, Chem. Eng. J., 2019, 363, 234–246 CrossRef CAS.
- Y. Li, Y. Jing, Y. Zhao, W. Li, J. Li, Y. Song, Y. Yang, T. Feng, G. Peng, Z. Huang, T. Yang and Q. Zhou, Enhanced activation of peroxymonosulfate by abundant Co-Nx sites onto hollow N-doped carbon polyhedron for bisphenol A degradation via a nonradical mechanism, Sep. Purif. Technol., 2023, 306, 122773 CrossRef CAS.
- Y. Gao, Y. Zhu, Z. Chen and C. Hu, Nitrogen-coordinated cobalt embedded in a hollow carbon polyhedron for superior catalytic oxidation of organic contaminants with peroxymonosulfate, ACS ES&T Engg, 2020, 1(1), 76–85 Search PubMed.
- D. D. Tuan, E. Kwon, S. Phattarapattamawong, B. X. Thanh, T. C. Khiem, G. Lisak, H. Wang and K.-Y. A. Lin, Nitrogen-containing carbon hollow nanocube-confined cobalt nanoparticle as a magnetic and efficient catalyst for activating monopersulfate to degrade a UV filter in water, J. Environ. Chem. Eng., 2022, 10(2), 106989 CrossRef CAS.
- Z. Wu, L. Wang, S. Chen, X. Zhu, Q. Deng, J. Wang, Z. Zeng and S. Deng, Facile and low-temperature strategy to prepare hollow ZIF-8/CNT polyhedrons as high-performance lithium-sulfur cathodes, Chem. Eng. J., 2021, 404, 126579 CrossRef CAS.
- W. Zhang, X. Jiang, Y. Zhao, A. Carne-Sanchez, V. Malgras, J. Kim, J. H. Kim, S. Wang, J. Liu, J. S. Jiang, Y. Yamauchi and M. Hu, Hollow carbon nanobubbles: monocrystalline MOF nanobubbles and their pyrolysis, Chem. Sci., 2017, 8(5), 3538–3546 RSC.
- J. Tong, L. Chen, J. Cao, Z. Yang, W. Xiong, M. Jia, Y. Xiang and H. Peng, Biochar supported magnetic MIL-53-Fe derivatives as an efficient catalyst for peroxydisulfate activation towards antibiotics degradation, Sep. Purif. Technol., 2022, 294, 121064 CrossRef CAS.
- H. Peng, J. Cao, W. Xiong, Z. Yang, M. Jia, S. Sun, Z. Xu, Y. Zhang and H. Cai, Two-dimension N-doped nanoporous carbon from KCl thermal exfoliation of Zn-ZIF-L: Efficient adsorption for tetracycline and optimizing of response surface model, J. Hazard. Mater., 2021, 402, 123498 CrossRef CAS.
- C. C. Hou, L. Zou, L. Sun, K. Zhang, Z. Liu, Y. Li, C. Li, R. Zou, J. Yu and Q. Xu, Single-atom iron catalysts on overhang-eave carbon cages for high-performance oxygen reduction reaction, Angew. Chem., Int. Ed., 2020, 59(19), 7384–7389 CrossRef CAS.
- Q. Wu, Y. Zhang, H. Liu, H. Liu, J. Tao, M.-H. Cui, Z. Zheng, D. Wen and X. Zhan, FexN produced in pharmaceutical sludge biochar by endogenous Fe and exogenous N doping to enhance peroxymonosulfate activation for levofloxacin degradation, Water Res., 2022, 224, 119022 CrossRef CAS PubMed.
- J. Huang, Y. Yang, G. Zeng, Y. Gu, Y. Shi, K. Yi, Y. Ouyang, J. Hu and L. Shi, Membrane layers intensifying quorum quenching alginate cores and its potential for membrane biofouling control, Bioresour. Technol., 2019, 279, 195–201 CrossRef CAS PubMed.
- H. Peng, W. Xiong, Z. Yang, J. Cao, M. Jia, Y. Xiang, Q. Hu and Z. Xu, Facile fabrication of three-dimensional hierarchical porous ZIF-L/gelatin aerogel: Highly efficient adsorbent with excellent recyclability towards antibiotics, Chem. Eng. J., 2021, 426, 130798 CrossRef CAS.
- P. Song, H. Xu, S. Sun, W. Xiong and Z. Yang, Remediation of arsenic-spiked soil by biochar-loaded nanoscale zero-valent iron: Performance, mechanism, and microbial response, J. Cleaner Prod., 2022, 380, 134985 CrossRef CAS.
- S. Zhu, X. Huang, F. Ma, L. Wang, X. Duan and S. Wang, Catalytic removal of aqueous contaminants on N-doped graphitic biochars: Inherent roles of adsorption and nonradical mechanisms, Environ. Sci. Technol., 2018, 52(15), 8649–8658 CrossRef CAS PubMed.
- H. Zhang, C. Xie, L. Chen, J. Duan, F. Li and W. Liu, Different reaction mechanisms of SO4•- and •OH with organic compound interpreted at molecular orbital level in Co(II)/peroxymonosulfate catalytic activation system, Water Res., 2023, 229, 119392 CrossRef CAS PubMed.
- F. Sun, T. Chen, Z. Chu, P. Zhai, H. Liu, Q. Wang, X. Zou and D. Chen, The synergistic effect of calcite and Cu2+ on the degradation of sulfadiazine via PDS activation: A role of Cu(III), Water Res., 2022, 219, 118529 CrossRef CAS PubMed.
- E. T. Yun, J. H. Lee, J. Kim, H. D. Park and J. Lee, Identifying the Nonradical Mechanism in the Peroxymonosulfate Activation Process: Singlet Oxygenation Versus Mediated Electron Transfer, Environ. Sci. Technol., 2018, 52(12), 7032–7042 CrossRef CAS.
- C. Yu, Z. Zhao, Y. Zong, L. Xu, B. Zhang and D. Wu, Electric field-enhanced coupled with metal-free peroxymonosulfate activactor: The selective oxidation of nonradical species-dominated system, Water Res., 2022, 227, 119323 CrossRef CAS.
- S. Wang, Y. Liu and J. Wang, Peroxymonosulfate activation by Fe-Co-O-codoped graphite carbon nitride for degradation of sulfamethoxazole, Environ. Sci. Technol., 2020, 54(16), 10361–10369 CrossRef CAS PubMed.
- Y. Zhen, S. Zhu, Z. Sun, Y. Tian, Z. Li, C. Yang and J. Ma, Identifying the persistent free radicals (PFRs) formed as crucial metastable intermediates during peroxymonosulfate (PMS) activation by N-doped carbonaceous materials, Environ. Sci. Technol., 2021, 55(13), 9293–9304 CrossRef CAS.
- H. Wang, W. Guo, B. Liu, Q. Wu, H. Luo, Q. Zhao, Q. Si, F. Sseguya and N. Ren, Edge-nitrogenated biochar for efficient peroxydisulfate activation: An electron transfer mechanism, Water Res., 2019, 160, 405–414 CrossRef CAS PubMed.
- M.-M. Wang, L.-J. Liu, J.-T. Wen, Y. Ding, J.-R. Xi, J.-C. Li, F.-Z. Lu, W.-K. Wang and J. Xu, Multimetallic CuCoNi oxide nanowires in situ grown on a nickel foam substrate catalyze persulfate activation via mediating electron transfer, Environ. Sci. Technol., 2022, 56(17), 12613–12624 CrossRef CAS PubMed.
- J. Yang, X. He, J. Dai, Y. Chen, Y. Li and X. Hu, Electron-transfer-dominated non-radical activation of peroxydisulfate for efficient removal of chlorophenol contaminants by one-pot synthesized nitrogen and sulfur codoped mesoporous carbon, Environ. Res., 2021, 194, 110496 CrossRef CAS PubMed.
- J. Dou, Y. Tang, Z. Lu, G. He, J. Xu and Y. He, Neglected but efficient electron utilization driven by biochar-coactivated phenols and peroxydisulfate: Polyphenol accumulation rather than mineralization, Environ. Sci. Technol., 2023, 57(14), 5703–5713 CrossRef CAS.
- J. Peng, P. Zhou, H. Zhou, B. Huang, M. Sun, C. S. He, H. Zhang, Z. Ao, W. Liu and B. Lai, Removal of phenols by highly active periodate on carbon nanotubes: A mechanistic investigation, Environ. Sci. Technol., 2023, 57(29), 10804–10815 CrossRef CAS.
- S. Wu, H. Liu, C. Yang, X. Li, Y. Lin, K. Yin, J. Sun, Q. Teng, C. Du and Y. Zhong, High-performance porous carbon catalysts doped by iron and nitrogen for degradation of bisphenol F via peroxymonosulfate activation, Chem. Eng. J., 2020, 392, 123683 CrossRef CAS.
- T. Entradas, S. Waldron and M. Volk, The detection sensitivity of commonly used singlet oxygen probes in aqueous environments, J. Photochem. Photobiol., B, 2020, 204, 111787 CrossRef CAS.
- F. Chen, G. X. Huang, F. B. Yao, Q. Yang, Y. M. Zheng, Q. B. Zhao and H. Q. Yu, Catalytic degradation of ciprofloxacin by a visible-light-assisted peroxymonosulfate activation system: Performance and mechanism, Water Res., 2020, 173, 115559 CrossRef CAS PubMed.
- F. Chen, L. L. Liu, J. J. Chen, W. W. Li, Y. P. Chen, Y. J. Zhang, J. H. Wu, S. C. Mei, Q. Yang and H. Q. Yu, Efficient decontamination of organic pollutants under high salinity conditions by a nonradical peroxymonosulfate activation system, Water Res., 2021, 191, 116799 CrossRef CAS.
- W. Ren, G. Nie, P. Zhou, H. Zhang, X. Duan and S. Wang, The Intrinsic Nature of Persulfate Activation and N-Doping in Carbocatalysis, Environ. Sci. Technol., 2020, 54(10), 6438–6447 CrossRef CAS.
- C. Han, X. G. Duan, M. J. Zhang, W. Z. Fu, X. Z. Duan, W. J. Ma, S. M. Liu, S. B. Wang and X. G. Zhou, Role of electronic properties in partition of radical and nonradical processes of carbocatalysis toward peroxymonosulfate activation, Carbon, 2019, 153, 73–80 CrossRef CAS.
- W. Ren, L. Xiong, G. Nie, H. Zhang, X. Duan and S. Wang, Insights into the electron-transfer regime of peroxydisulfate activation on carbon nanotubes: The role of oxygen functional groups, Environ. Sci. Technol., 2020, 54(2), 1267–1275 CrossRef CAS PubMed.
- Q. Hu, J. Cao, Z. Yang, W. Xiong, Z. Xu, P. Song, M. Jia, Y. Zhang, H. Peng and A. Wu, Fabrication of Fe-doped cobalt zeolitic imidazolate framework derived from Co(OH)2 for degradation of tetracycline via peroxymonosulfate activation, Sep. Purif. Technol., 2021, 259, 118059 CrossRef CAS.
- S. Ye, M. Cheng, G. Zeng, X. Tan, H. Wu, J. Liang, M. Shen, B. Song, J. Liu, H. Yang and Y. Zhang, Insights into catalytic removal and separation of attached metals from natural-aged microplastics by magnetic biochar activating oxidation process, Water Res., 2020, 179, 115876 CrossRef CAS PubMed.
- M. Jia, Q. Liu, W. Xiong, Z. Yang, C. Zhang, D. Wang, Y. Xiang, H. Peng, J. Tong, J. Cao and H. Xu, Ti3+ self-doped TiO2 nanotubes photoelectrode decorated with Ar-Fe2O3 derived from MIL-100(Fe): Enhanced photo-electrocatalytic performance for antibiotic degradation, Appl. Catal., B, 2022, 310, 121344 CrossRef CAS.
- L. Shi, J. Huang, G. Zeng, L. Zhu, Y. Gu, Y. Shi, K. Yi and X. Li, Roles of surfactants in pressure-driven membrane separation processes: a review, Environ. Sci. Pollut. Res., 2019, 26(30), 30731–30754 CrossRef.
- Y. Jing, M. Jia, Z. Xu, W. Xiong, Z. Yang, H. Peng, J. Cao, Y. Xiang and C. Zhang, Facile synthesis of recyclable 3D gelatin aerogel decorated with MIL-88B(Fe) for activation peroxydisulfate degradation of norfloxacin, J. Hazard. Mater., 2021, 424(424), 127503 Search PubMed.
- H. Peng, W. Xiong, Z. Yang, J. Tong, M. Jia, Y. Xiang, S. Sun and Z. Xu, Fe3O4-supported N-doped carbon channels in wood carbon form etching and carbonization: Boosting performance for persulfate activating, Chem. Eng. J., 2023, 457, 141317 CrossRef CAS.
|
This journal is © The Royal Society of Chemistry 2024 |