DOI:
10.1039/D2VA00158F
(Critical Review)
Environ. Sci.: Adv., 2023,
2, 586-611
Potential of microalgae and cyanobacteria to improve soil health and agricultural productivity: a critical view
Received
8th July 2022
, Accepted 13th February 2023
First published on 15th February 2023
Abstract
Microalgae are a source of scientific curiosity and inspiration for their utilization as ‘inoculants’ in agriculture and the commercial production of high-value products. Their diversity and abundance in the soil environment highlight the fact that these integral members of the soil microbial community modify the physical and chemical conditions of soils and interact with other microorganisms and even with higher plants with varying degrees of association. However, to date, the agronomic benefits of the nitrogen fixation trait of cyanobacteria have not been fully realized. Thus, the ecological functions of these organisms in the biological soil crusts should be thoroughly evaluated and widely applied given that climate change events can increase desertification. Currently, the crop yield increments and pest control due to these biostimulants and the reclamation of saline and sodic soils by these bioameliorants are considered economically marginal. Similarly, the carbon capture and storage by eukaryotic microalgae and cyanobacteria in soils are poorly understood. Limitations in their commercial production for agricultural use include inadequate technological innovations and the enormous expectation for yield increments, together with the contemporary monetization of their environmental benefits. Thus, this critical review presents the desirable reappraisal of their agronomic benefits and the invigoration of research and culture collections to utilize these organisms or their metabolites, considering the evolutionary consequences and environmental advantages and finally their commercial production for widespread application in agriculture.
Environmental significance
Microalgae, which include phylogenetically diverse microscopic organisms of eukaryotic algae (green algae and other microscopic algal forms), and prokaryotic cyanobacteria are abundant in agricultural soils. These organisms have beneficial traits such as N2 and CO2 fixation ability, cycling of nutrients, and plant growth promotion. However, their utilization as inoculants and metabolites requires new research and better understanding of environmental advantages, ranging from individual field plots to the planetary scale. Thus, the present review provides the recent developments in their agronomic and ecological assessments and the need for innovations in commercial production and cognizance of their evolutionary significance while promoting them for widespread applications in agriculture.
|
1. Introduction
Photosynthetic organisms are essential for life on Earth, the evolutionary developments ranging from anoxygenic to oxygenic photosynthesis determine the energetics of all carbon (C)-based life forms.1 Almost all the atmospheric oxygen is derived from oxygenic photosynthetic organisms (autotrophic plants, algae, and cyanobacteria), which obtain electrons from light-driven reaction centres (RCs) to fix atmospheric carbon dioxide (CO2). In addition, oxygenic photosynthetic organisms are responsible for fixing more than 99% of organic C annually.2 The evolutionary consequences and environmental impact of microalgae, including cyanobacteria, are significant. Recently, molecular evidence has provided significant new evolutionarily insights into cyanobacteria, which are traditionally considered strictly oxygenic phototrophs. In the new cyanobacterial lineages (4C0d-2 and ML635J-21), i.e., metagenome-assembled genome (MAG) representatives, the absence of genes for photosynthesis and CO2 fixation was demonstrated.3–5 The primary endosymbiosis between a cyanobacterium and a heterotrophic eukaryotic host led to the formation of primary plastids (e.g., chloroplast organelles) in the first photosynthetic eukaryotic lineages including green algae (and land plants as their descendants).6 Qiu et al.7 suggested that algae (endosymbionts) are the vectors of endosymbiotic and horizontal gene transfer in the evolutionary history of many photosynthetic lineages. The ‘red carpet hypothesis,’ proposed by Ponce-Toledo et al.8 suggests that the mixed heritage of green plastids in secondary algae is due to many previous red algal gene acquisitions. These evolutionary events and the diversity and abundance of eukaryotic microalgae and cyanobacteria in soils are significant in achieving success in the present in human agricultural endeavours. Although ‘blue-green algae’ are monerans (prokaryotes), they were included in one of the nine algal phyla, i.e., ‘Cyanobacteria’, in 2008. Presently, this large and morphologically diverse group of photoautotrophic prokaryotes are classified as ‘Cyanobacteria’ in one of the three domains of life, i.e., ‘Bacteria’, to clearly differentiate them from eukaryotic algae that belong to the other domain Eukaryota. Alternatively, microscopic forms of algae (microalgae), which play a major role in nutrient cycling and fixing atmospheric CO2, are essentially eukaryotic forms. In the present review, the terms ‘microalgae’ and ‘cyanobacteria’ are used to separately represent two distinct microbial groups, i.e., ‘eukaryotes’ and ‘prokaryotes’.
The systematic composition (diversity) and abundance of cyanobacteria in subaerial and freshwaters of the tropics, especially from the observations made in Ceylon (Sri Lanka now) and their presence and likely similarities in other parts of the world were reported by Fritsch9 in 1907. Harrison and Aiyer10 reported the beneficial effects of algal growth in aerating the upper layers of submerged rice soils. In contrast to the report by Bouilhac11 on the absence of dinitrogen (N2) fixation in Nostoc species, Molisch12 showed the ability of two strains of Nostoc to grow well in nitrogen (N)-free solution. In 1939, De13 demonstrated N2 fixation by cyanobacteria isolated from dried soil samples collected and brought from rice fields in India. Consequently, the contribution of cyanobacteria to cultivating rice in the same land for long periods was established.13,14 These beginnings have led to the widespread examination and utilization of the potential of cyanobacteria in improving soil fertility and plant growth promotion (see reviews by Renuka et al.,15 Abinandan et al.,16 Chittora et al.,17 and Santini et al.18). However, although the biochemical potential of microalgal resources has been previously considered by most researchers, the need for translational research into commercial production, cognizance of ecological consequences and environmental impact between farming communities and policy makers require reappraisal. In the present critical review, we present the potential contributions of eukaryotic microalgae and cyanobacteria to the soil structure and fertility improvements, plant stimulatory effects related to nutrition and metabolisms, reclamation of saline and sodic soils, pest control, and soil C storage, and perspectives that should be considered for their commercial production and wide-spread applications in agriculture.
2. Microalgae and cyanobacteria in improving soil structure and fertility
Global food production depends on the soil, which acts as a home to several terrestrial species, thus significantly supporting biodiversity. The soil structure, influenced by the distribution of soil aggregates (i.e., basic units of soil structure) and pore spaces, is an indicator of fertility and determines not only the ability of soil to retain water and nutrients for crop growth but also the drainage ability, water-holding capacity, and soil aeration. In this case, although compacted soil can retain moisture and nutrients better, it is poorly drained, and the emergence of seedlings and plants becomes difficult. On the contrary, ‘less compact’ soil cannot retain water and nutrients. Thus, depending on the soil type, the distribution of solids, air, and water determines whether it is an ‘ideal’ agricultural soil. Typically, the ‘ideal’ agricultural soil has about 50% solids (about 45% minerals and 5% organic matter), 25% air, and 25% water and has strong relationships with biodiversity and agricultural productivity. Hence, the soil structure determines and supports the biodiversity of terrestrial species, including plants and microorganisms, which consequently alter the soil structure. In agricultural soils, the inter-relationships between crop plants and microbial communities are complex, where certain species can improve the soil structure and increase crop yields. However, agricultural practices, such as use of heavy machinery and tillage, can damage the soil structure. Thus, management practices such as reducing or no-tillage become critical to improving the soil structure. Furthermore, management practices involving microbial entities can also have long-term benefits. Cyanobacteria are considered ecological engineers because of their ability to modulate the ecological processes in the soil and create habitats for other organisms.19 The experimental addition of cyanobacteria under laboratory and field conditions resulted in a significant improvement in soil structure and aggregate stability20–23 (see Fig. 1).
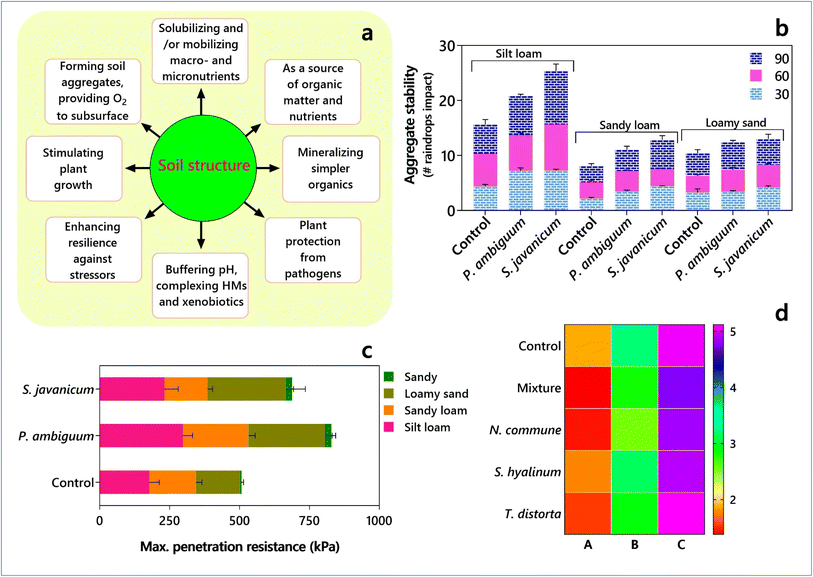 |
| Fig. 1 Implications of cyanobacteria in (a) soil structure (based on data from Nichols24). (b) Aggregate stability by Phormidium ambiguum and Scytonema javanicum. (c) Maximum penetration resistance of P. ambiguum and S. javanicum in different textured soils (based on data from Chamizo et al.25). (d) Albedo properties (400–700 nm range of soil surface reflectance spectra) of three soils (A – Amoladeras, B – El Cautivo, and C – Gádorquarry) treated with different cyanobacterial inoculants (Nostoc commune, Scytonema hyalinum and Tolypothrix distorta) (based on data from Román et al.26). | |
Chamizo et al.25 inoculated two cyanobacterial species (non-N2-fixing Phormidium ambiguum Gomont NIES-2121 of the order Oscillatoriales and the N2-fixing Scytonema javanicum Bornet & Flahault NIES-1956 of the order Nostocales) in the microcosms of silt loam, sandy loam, loamy sand, and sandy soil types. After 90 days, the cyanobacterial inoculation led to the formation of biocrusts, and those by P. ambiguum were characterized by thin filaments enveloping soil particles, while that by S. javanicum exhibited thicker filaments as bunches between soil particles. Interestingly, the total exopolysaccharide content and soil penetration resistance improved with P. ambiguum, while the total organic C and N contents increased with S. javanicum with variable levels in different soil types. The cyanobacterial filaments and extracellular secretions (exopolysaccharides) act as gluing agents for binding soil particles for the enhanced formation of soil aggregates.27 ‘Cyanobacterization’ (soil inoculation with cyanobacteria) is effective for stabilizing burned soils and restoring post-fire ecosystems.28,29 Recently, Shantakumar et al.30 demonstrated that the algalization of acid soils with acid-tolerant microalgae, i.e., Desmodesmus sp. MAS1 and Heterochlorella sp. MAS3, resulted in the initial development of soil algal crust, followed by the release of exopolysaccharides, which facilitated the stability of the soil aggregates and increase in soil pH. Importantly, the most suitable species should be selected for the restoration of poorly structured soils, depending on the soil type, texture, and mineralogy composition, which influence the growth of cyanobacteria and exopolysaccharide production.26 However, although the ecological and agronomic potential of micro algalization or cyanobacterization for restoring degraded lands has been reported, the technological advances for their widespread application are inadequate. Pelletized cyanobacteria facilitated the storage of inoculum for extended periods, which is a promising technological innovation for restoring arid lands.26
Although Metting and Rayburn31 and Barclay and Lewin32 suggested the use of microalgal polysaccharides for the conditioning of agricultural soils, the necessity for frequent irrigation to maintain algal growth or high inoculant rates hinders the direct application of microalgae for soil conditioning. Eukaryotic green algae can enrich and enhance nutrient availability and improve the fertility and quality of soils by the production of polysaccharides, antimicrobial compounds, plant growth hormones such as abscisic acid, cytokinin, gibberellins, and ethylene, and other metabolites that can promote plant growth.33,34 The microalgal polysaccharides are generally heteropolymers with different proportions of galactose, xylose, and glucose linked by glycosidic bonds except for homopolymer polysaccharides of galactose and β-(-1,3)-glucan by Gyrodinium impudicum and Chlorella vulgaris. Together with sugars such as rhamnose, fucose, fructose, and methyl sugars, microalgal polysaccharides can serve as bio-stimulants for improving the nutrient uptake, growth performance and physiological status, and abiotic stress tolerance of crop plants via their mechanistic actions of enhanced root growth and metal chelation in soils and enhanced scavenging of reactive oxygen species, Rubisco synthesis, photosynthesis and higher PSII activity in the above-ground parts of the crop plants.35 In a recent study, Rachidi et al.36 applied the crude polysaccharide extracts from three microalgal strains (Arthrospira platensis MS001, Dunaliella salina MS002 and Porphyridium sp. MS099) on tomato plants and observed an increase in the contents of carotenoid, chlorophyll, and proteins, enhancement in the activities of nitrate reductase (about 102%) and NAD-glutamate dehydrogenase (about 400%) and changes in metabolomics related to the synthesis of lipids, sterols, and alkanes in the leaves. In another study, Mutale-Joan et al.37 found that the application of microalgal liquid extracts led to an increase in the accumulation of pyridine-3-carboxamide (active amide form of vitamin B3) and linolenic acid (C18
:
3), which are precursors involved in the jasmonate pathways in plants. However, it is necessary to standardize and optimize protocols for applying microalgal extracts for individual crop species at different growth and developmental stages.
Microalgal metabolites belonging to the chemical groups of phenols, fatty acids, indoles, terpenes, acetogenins, and volatile halogenated hydrocarbons possess antimicrobial, antiviral, and antiprotozoal activity. Alsenani et al.38 reported that linoleic acid, oleic acid, docosahexaenoic acid (DHA), and eicosapentaenoic acid (EPA) in the extracts of three microalgae (Isochrysis galbana, Scenedesmus sp. NT8c, and Chlorella sp. FN1) are potential candidates for inhibiting the growth of Gram-positive bacteria. However, only a small percentage of microalgal species, about 50 species from the described species of 40
000 to 50
000, has been screened for antimicrobial compounds, while the global estimate of microalgal species is approximately 10 million.39,40 Furthermore, the culture collections of microalgae are limited, even at the global level, and their commercial cultivation is restricted to even less. Hence, the agronomic applications of microalgae-derived antimicrobial compounds for modulating the soil microbiomes and eukaryotes have not received sufficient attention to date.
Ancient microalgal species are considered the origin of modern higher plant phytohormone biosynthesis pathways.33 Accordingly, a better understanding of the evolution, metabolism, and regulatory networks of microalgal phytohormone systems will provide new avenues for using phylogenetically divergent microalgal resources as feedstock for phytohormone production and their agricultural applications. The modulation of phytohormone systems in higher plants by the introduction, enhancement, or engineering of beneficial agronomic traits such as tolerance to high levels of salinity, drought, flooding, and temperature extremes in crop plants is considered significant to successfully realise the ‘Green Revolution’. Interestingly, the application of phytohormones improves the growth and accumulation of lipids and metabolites in microalgae.41 Furthermore, the exogenous application of phytohormones can enhance the biomass of cultivated microalgae, where microalgal species can modulate plant growth and development. However, little has been done beyond the laboratory microcosm- and experiment plot studies to develop microalgal resources for agronomic applications.42 Because of the limitations of the direct applications of microalgae, including cyanobacteria, for soil structure and fertility improvements, such as the need for frequent irrigation and high inoculant rates, only their practical applications as biofertilizers or biostimulants can become popular for the cultivation of crops.
3. Microalgae and cyanobacteria as biofertilizers: biological potential and technological constraints
The application of microorganisms for improved plant growth, especially nodulation in pulse crops, has been known for more than 125 years. The first US patented microbial inoculant was ‘Nitragin’ in 1896. Commercially, this bacterial fertilizer (a species of N2-fixing Rhizobium) was sold as a soil inoculator for legume crops using the Nobbe–Hiltner process. However, the chemical process of ammonia synthesis, a German patent granted to Fritz Haber in 1908, led to the production of synthetic nitrogenous chemical fertilizers worldwide and eclipsed the utilization of bacterial fertilizers. Meanwhile in Russia, ‘Phosphobacterin’, a biofertilizer having the spores of Bacillus megaterium var. Phosphaticum with kaolin rocks for improving the availability of phosphorus (P) to crops, became popular in the 1950s. Cyanobacteria represent a dominant bacterial group, playing significant roles in providing photosynthetically fixed C, N, phosphorus, phytohormones and polysaccharides to promote rice growth and enhance the fertility of soils. Thus, cyanobacteria have been explored as biofertilizers in flooded rice paddies, especially in India, where they form mats on the surface of water and enable an increase in yield to the extent of 10–24%.13,43–46
According to their types and guilds of microorganisms and benefits accrued due to their application, biofertilizers can be classified as N2 fixers, phosphate- and potassium solubilizers, phosphate mobilizers, zinc- and iron-solubilizers and plant growth-promoting rhizobacteria or plant stimulants. In general, the selection of cyanobacteria as biofertilizers is based on their ability to fix atmospheric nitrogen and plant growth-promoting activity. Chemical fertilizers or bioinoculants are often applied to the soil, and their effect on the rhizosphere microbiome is beginning to be understood.47,48 Most soils are deficient in N for supporting higher crop growth and yields, and the application of nitrogenous fertilizers is a critical determinant of the condition of ‘feast or famine’ in many countries. Given that the N use efficiency associated with synthetic chemical fertilizers is often low at about 20–40%, the economic losses and environmental pollution necessitate intensive efforts in developing newer biological methods for nutrient management in agricultural soils. The global biofertilizer market size is estimated to increase from USD 2.6 billion in 2021 to USD 4.5 billion by 2026, with a CAGR of 11.9%49 (see Fig. 2). In the following section, we present a critical analysis on the potential of eukaryotic microalgae and cyanobacteria as CO2 fixers, N2 fixers, phosphate solubilizers, and plant biostimulants. In fact, the potential of several species of microalgae, including cyanobacteria, as biofertilizers/biostimulants in enhancing the yield components and/or yield of certain crop plants has been well realized (Table 1 and Fig. 3).
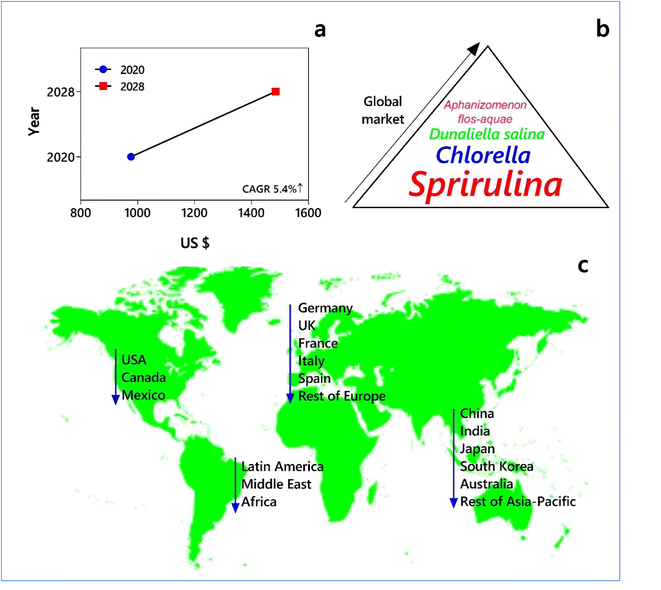 |
| Fig. 2 Global biofertilizers market potential of microalgae and cyanobacteria. (a) Market size of microalgae for the years 2020 and 2028 (CAGR = compound annual growth rate). Key market consumption in terms of (b) microalgal/cyanobacterial type and (c) region globally (decrease in the order of arrow direction). (Source: https://www.alliedmarketresearch.com/microalgae-market-A13419). | |
Table 1 Impact of eukaryotic microalgae and cyanobacteria as biofertilizers or biostimulators on selected crop plants
Crop plant |
Microalgal/cyanobacterial species |
Effect (increase) |
References |
Rice |
Calothrix sp. |
Plant growth |
48, 50 and 51 |
Oscillatoria sp. |
Anabaena sp. |
Phormidium sp. |
Aulosira sp. |
Plectonema sp. |
Nostoc spongiaeforme
|
Yield components such as tillers per hill, panicles per hill, length of panicle, straw, and grain yield |
52
|
Nostoc commune
|
Calothrix marchica
|
Stigonema sp. |
Scytonema hofmanii
|
Salt stress tolerance |
53
|
Chlorella vulgaris
|
Seed germination and growth |
54 and 55 |
C. pyrenoidosa
|
Wheat |
|
|
|
Nostoc sp. |
Plant growth |
56–58
|
Calothrix sp. |
Nutrient content |
Anabaena sp. |
Seed yield |
Chlorella sorokiniana
|
Plant growth (length and dry biomass) |
59 and 60 |
Phosphorus acquisition |
Maize |
C. sorokiniana
|
Plant growth (root development) |
61
|
Chlamydomonas reinhardtii
|
Nutrient content |
Pseudanabaena sp. |
Seed germination |
62
|
Phormidium sp. |
Geitlerinema sp. |
Arthrospira sp. |
Oscillatoria sp. |
Lettuce; capsicum; tomato |
Calothrix anomala
|
Plant growth, and nutrient content |
36, 63 and 64 |
Arthrospira platensis
|
Aphanothece sp. |
Dunaliella salina
|
Salt tolerance |
36 and 65 |
Nutrient uptake |
Metabolomics profile |
Chlorella sp. (in consortium) |
Plant growth |
66
|
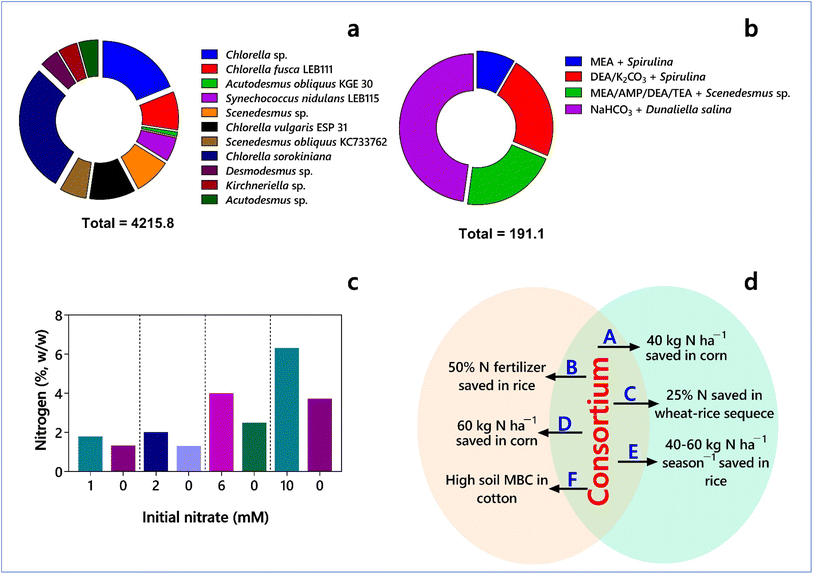 |
| Fig. 3 Metabolic potential of microalgae and cyanobacteria. (a) CO2 bio-fixation rate (mg L−1 day−1) under different cultivation systems (based on data from Basu et al.,67 Duarte et al.,68 Kumar and Das,69 Nayak et al.,70 Swarnalatha et al.,71 Yeh and Chang,72 and Yun et al.73). (b) Carbon fixation efficiency (%) of absorption-microalgae hybrid (based on data from Cardias et al.,74 da Rosa et al.,75 Kim et al.,76 and Kim et al.77) (DEA – diethanolamine; MEA – monoethanolamine; AMP – 2-amino-2-methyl-1-propanol; TEA – triethanolamine). (c) Nitrogen (%, w/w) in Chlorella vulgaris biomass harvested after 10 days (in the presence of different initial nitrate concentrations) and 7 days (in nitrogen-free medium) from algal batch culture system (based on data from Farooq et al.78). (d) Efficiency of nitrogen savings by a microbial consortium containing cyanobacteria during crop cultivation (based on data from Prasanna et al.79–83). (A, B and C = Anabaena spp. + Nostoc spp.; D = Anabaena torulosa + Trichoderma viride/A. torulosa + Azotobacter sp.; E = A. torulosa + T. viride/A. torulosa + Pseudomonas sp.; F = A. torulosa + T. viride). | |
3.1. Role as CO2 fixers
Terrestrial C cycling processes are critical for plant growth and development and higher agricultural productivity. ‘Green carbon’ refers to the C stored in plants and soils of the natural ecosystems. In this case, forests can accumulate and have large stocks of C, whereas agricultural croplands have relatively lower stocks due to their release with the short cycles of plant production and harvest. About 75% of soils have already been degraded, resulting in a negative impact on food security and family farming. Thus, the measures of reforestation and afforestation have become necessary to enhance the stocks of ‘green carbon’. The primary producers, such as plants, algae, and autotrophic prokaryotes, including cyanobacteria (aerobic) and anaerobic photosynthetic organisms, assimilate by transforming CO2. In contrast, animals (consumers), microbial decomposers, and primary producers release CO2 through dissimilatory (chiefly respiratory) processes. The biotic interactions among the primary producers, microbial decomposers, and consumers determine the rates of the assimilatory and dissimilatory processes. Higher plants modulate the activity of microbial decomposition by influencing the microbial availability of oxygen, nutrients, and labile substrates. Given that most of humanity depends on terrestrial soils for food, feed, and fiber, soils without sufficient C will make agricultural cultivation less profitable and environmentally catastrophic. Accordingly, nature-based solutions for conserving and restoring the C content of soils necessitate a shift from chemical fertilizer-based, modern agriculture to sustainable farming that can regenerate C-rich soils and reduce the emissions of greenhouse gas emissions and minimize the use of energy and inputs (http://www.4p1000.org).
In agricultural lands, microbial inoculants can improve C sequestration by capturing CO2, forming carbonates by sedimentation, producing recalcitrant compounds, and enhancing the soil C stability.84 In algae, the CO2-concentrating mechanism (CCM) involves carbonic anhydrase, which provides CO2 for C fixation by Rubisco. The natural ability and efficiency of CO2 sequestration of several microalgal species are poor. However, In-na et al.85 recently used loofah-based microalgal and cyanobacterial biocomposites for enhancing CO2 capture. Also, some attempts have been reported on genetically engineering cyanobacteria for enhanced carbon capture and storage (CCS) in photobioreactor systems.86 Presently, agricultural practices do not include the utilization of eukaryotic microalgae and cyanobacteria as microbial inoculants for biological C sequestration. However, the ‘soil carbon debt’ due to human land use suggests that 1200 years of human agriculture has led to the loss of 133 billion tonnes of C, i.e., about 8% of total global soil C stocks (estimated at 2400 petagrams of C, PgC) or equivalent to 65 ppm of atmospheric CO2, from the top two meters of the soil worldwide.87 The global ‘hotspots’ of C losses include agricultural lands, which are not equally distributed. In addition, the annual loss of topsoil is about 75 billion tons, with an economic loss of approximately US$ 400 billion.88 Moreover, the functions of plant roots include the absorption of CO2 and carbonate with the β-carboxylation of keto acids and movement of dicarboxylic acid to leaves.89 Thus, the continuing soil C loss and soil erosion through the loss of top soil due to agriculture, increasing concentrations of atmospheric CO2 as a greenhouse gas, and the ability of plants to utilize CO2 provide incentives to screen, select and use eukaryotic microalgae and cyanobacterial species and other autotrophic microorganisms for improved CO2 fixation in soils or rhizospheres. Nevertheless, tracking the below-ground processes and differentiating the CO2 fixers from soil-derived and plant-derived C-utilizing microorganisms, and their efficiencies are essential to develop methods for rationally manipulating plant–soil microorganism systems for promoting soil C storage. In a recent report, Chen et al.90 showed that the CO2 fertilization effects due to increased land-use management through croplands were greater in India (82%) than in China (32%), while it was the opposite for greening through forests with 42% and 4.40% in China and India, respectively. Interestingly, the net primary productivity of soil algae in croplands is estimated to be the highest (157 g C m−2 years−1) among the biomes, equalling about 6% of the net primary production of terrestrial vegetation91 (see Table 2). The CO2 fertilization efforts through soil algae can benefit croplands, not only through plant growth stimulation but also through improved terrestrial carbon uptake.
Table 2 Abundance and net primary productivity of soil microalgae and cyanobacteria across different biomesa
Ecosystem |
Abundance (median value × 103 cells g−1 dry soil) |
Net primary productivity (NPP) (g C m−2 years−1) |
Contribution to total terrestrial NPP (%) |
Data from Jassey et al.91
|
Croplands |
161 |
157 |
>30 |
Drylands |
85 |
<15 |
<10 |
Wetlands |
1036 |
<15 |
<10 |
Alpine and polar lands |
20 |
<15 |
<10 |
Grasslands |
410 |
22.6 |
<15 |
Broadleaf and mixed forests |
59 |
28.2 |
<10 |
Broadleaf evergreen forests |
202 |
18 |
>15 |
Average |
550 ± 340 |
30 |
|
3.2. Dinitrogen fixers
Biological N2 fixation by prokaryotic microorganisms is an ancient process that probably originated in anaerobic methanogens about 1.5 to 2.2 Ga (Giga annum or billions of years). The distribution of nitrogenase enzyme among biological organisms is limited to bacteria and archaea. The presence of nif gene clusters is limited to a few terrestrial (i.e., Cyanothece and Synechococcus) and marine (Crocosphaera) cyanobacterial strains. Besides, the genomes of Firmicutes, Chloroflexi, Chlorobi, and Bacteroidetes and the lineages of Actinobacteria and Proteobacteria have nif gene clusters. Cyanobacteria are the only diazotrophic lineage capable of fixing N2, while producing molecular oxygen as their metabolic product. They employ different mechanisms to protect the oxygen-sensitive nitrogenase, as follows: (i) up-regulation of N2 fixation at night on a diurnal cycle with reduced oxygen tensions due to decreased photosynthetic oxygen production, especially in non-filamentous cyanobacteria and (ii) spatial segregation of nitrogenase in heterocysts with the involvement of the Mehler reaction.92 Cyanobacteria, with their ‘introvert’ lifestyle of maintaining C/N homeostasis, tightly interconnect the fixation of CO2 with N-assimilation. The complex network of signal transduction pathways involves 2-oxoglutarate to sense the cellular C/N status. The accumulation of carbon or nitrogen storage polymers in the cells cope with the imbalance in the homeostatic control.93 Recently, Hirose et al.94 sequenced the genomes of 28 cyanobacterial strains that can form heterocysts, which are morphologically distinct cells for fixing N2 from a collection of 827 strains in the National Institute for Environmental Studies (NIES), Japan.
Cyanobacteria can establish symbiotic relationships with bryophytes (hornworts and liverworts), algae, Azolla, cycads, fungi (Geosiphon together with yeasts forming lichens), and Gunnera because of their ability to differentiate heterocysts (specialized N2-fixing cells) and hormogonia.95,96 Thus, the only angiosperm species that can form symbiotic relationships with cyanobacteria is Gunnera. Besides, in higher plants, the symbiotic relationships with mycorrhizal fungi and N2-fixing bacteria (symbiotic nitrogen fixation, SNF) began about 540–420 million years ago (Ma), with the occurrence of the first peak in CO2, and the second peak occurred about 150–100 Ma.97 Hence the selection of N2-fixing cyanobacteria as bacterial inoculants for higher plants is primarily limited to free-living members. Nevertheless, both the free-living N2-fixing cyanobacterial strains are utilized as biofertilizers, and the symbiotic Azolla–Anabaena system is used as ‘green manure’ in many rice-cultivating countries such as India, Nepal, Vietnam, China, Thailand, and the Philippines. Although the application of free-living cyanobacteria can fix about 25–30 kg N ha−1 season−1, the Azolla–Anabaena system can contribute about 20–40 kg N ha−1 to the rice crop in about 25 days.98–100 Chittora et al.17 detailed the steps required to mass-produce cyanobacterial biofertilizers. They introduced affordable (rural) technology to economically poorer farmers, with the soil-based inoculum consisting of Aulosira, Nostoc, Anabaena, and Tolypothrix, is now a commercial enterprise of cyanobacterial biofertilizer technology in India.101 The symbiotic relationships between eukaryotic microalgae and cyanobacteria, such as that between various genera of diatoms with the diazotrophic cyanobacterium Richelia intracellularis still have not been investigated for their potential for N2 fixation and their utilization as inoculants to date. Interestingly, diazotrophic cyanobacteria with an alternative nitrogenase (V-nitrogenase, encoded by vnfH, vnfDGK), in addition to molybdenum nitrogenase have been reported.102 Thus, it is necessary to prepare comprehensive genomic data of cyanobacteria to benefit from their nitrogenases with different metal co-factors.
3.3. Phosphorus cycling by microalgae and cyanobacteria
The P content of most soils is about 0.5% (w/w), while the plant available form is only 0.10% of the total P content. The P use efficiency of phosphatic fertilizers is about 46%, leading to the environmental problems of groundwater contamination and eutrophication.103 The P availability in soil is an essential determinant of global food security. The current global reserves of phosphate rocks, a non-renewable source for chemical P fertilizers, may by depleted in the next 50 to 100 years.104 In this case, soil microorganisms, including eukaryotic microalgae and cyanobacteria, are capable of phosphate solubilization. The solubilization of rock phosphate (i.e., Mussoorie rock phosphate, MRP) by cyanobacteria was demonstrated by Roychoudhury and Kaushik,105 and organic sources by Whitton et al.106 Yandigeri et al.107 showed the differential response of two diazotrophic cyanobacteria, Westiellopsis prolifica and Anabaena variabilis, to solubilize extracellular insoluble tricalcium phosphate (TCP) and MRP. Nevertheless, the mineral phosphate solubilizing (mps) phenotype is not common among cyanobacteria. Microalgae (e.g., Chlorella sp. and Scenedesmus sp.) and cyanobacteria (e.g., Aphanothece sp., Spirulina sp., Arthrospira sp., and Phormidium sp.) are capable of assimilating P as polyphosphates as linear polymers of orthophosphate residues linked by phosphoanhydride bonds to mitigate stress conditions.108 These microalgal and cyanobacterial strains can be used as biofertilizers for the slow and moderate release of P, supplying it within the ‘critical value’ for the crop rhizosphere, thus reducing the probability of excess supply.
Microalgae are acclimated to low-P conditions because of their capabilities related to the ‘luxury uptake’ of P and storage as polyphosphate whenever P is available. The expression patterns of Pi (inorganic orthophosphate) transporters are a function of the P availability of microalgae.109 Mukherjee et al.110 proposed the development of technology to recover P from waste and effluent (e.g., parboiled rice mill effluent) using microalgal and cyanobacterial strains capable of sequestering P as polyphosphate inclusions and recycling these strains as biofertilizers for the slow and moderate release of P. Golzary et al.111 reported the potential of Azolla filiculoides (Azolla–Anabaena system) to remove about 40–44% of P from secondary effluents.
3.4. Plant biostimulants
Because of phototrophic and aerobic growth requirements, cyanobacteria are conventionally considered plant biostimulants, although some strains were isolated from rhizospheres. The N2-fixing trait of cyanobacteria is the primary choice for their screening, selection, and utilization. However, recent reports suggest that diverse bioactive molecules such as osmolytes, phenolics, vitamins, phytohormones, and others act synergistically for plant growth promotion and tolerance to abiotic stresses18 (see Table 1). Singh et al.112 suggested that two biological processes, i.e., biological fixation of CO2 and atmospheric dinitrogen, make them biostimulants, especially in paddy fields. Ördög et al.113 applied freeze-dried biomass of Nostoc piscinale after resuspending it in tap water (1.0 g L−1 DW) as a single foliar treatment (400 L ha−1) to maize (Zea mays SY Zephir hybrid) and found that the number of leaves, the contents of chlorophyll (SPAD value) and grain protein, and grain yield (6.50–11.50%) were significantly higher. Seed priming and soil application of cyanobacterial strains, with or without other bacterial biofertilizers have shown variable plant growth-promoting effects in different crops.79,114 Since the 1940s, extracts of seaweed (macroalgae) have been applied to improve the stress tolerance and crop yields due to their elicitors and phytohormones.115 Similarly, microalgal extracts have diverse biological activities and are potential agents for mitigating stresses and plant growth promotion.116,117 In fact, Colla and Rouphael117 identified the bottlenecks such as high cost of microalgal production and application, poorly characterized products with varying performance, lack of knowledge about the mechanisms, and lack of awareness among farmers in deriving the benefits from microalgal and cyanobacterial biostimulants.
4. Microalgae and cyanobacteria in biological soil crusts: significance in drylands
Arid and semiarid environments occupy 41% of the global land area and support more than 38% of the total human population. Desertification affects about 25% of the global land area due to climatic change, human activities, and other factors, and more than 40% of the Earth's terrestrial area is in danger of desertification.118 The desertification risks are about 13%, 7% and 9% of the global land area at moderate, high, and very high levels, respectively.119 The significant features of these dry lands include the presence of biological soil crusts, which are of two types, as follows: (i) hypoliths, also referred to as biocrusts, consisting of microscopic cyanobacteria, algae, fungi, and bacteria, and (ii) poikilohydric organisms consisting of macroscopic lichens, mosses, and microarthropods.120 Fossil records suggest that biocrusts, one of the earliest and dominant types of community terrestrial life forms, appeared about 2.6 Ga. The biocrusts determine the structure, surface morphology, capture and retention, organic matter and other resources, and fertility of soil by influencing the atmospheric fixation of C and N. In drylands, the critical zone (described as the ‘heterogeneous, near-surface environment in which complex interactions involving rock, soil, water, air, and living organisms regulate the natural habitat and determine the availability of life-sustaining resources’) (US National Research Council, 2001) is chiefly characterized by biocrusts, endoliths, and hypoliths, whereas sporadically by vascular plant roots at, on or in the top few cm of the soil surface or rock. This critical zone mediates: (i) the exchange of all gaseous, nutrients, and water inputs and outputs; (ii) the deposition, transformation, and availability of nutrients; (iii) the structuring of the vascular plant community at the temporal, spatial, and compositional levels; (iv) the direct delivery of C, nutrients, and water by biocrusts to plants; (v) the creation of biodiversity hotspots by biocrusts and (vi) the cover and composition of biocrusts, which can modify the soil stability, fertility, and hydrology and be used as an index of soil health.120
In 1729, Micheli121 described the thallus of Nostoc, which occurs in most biocrust communities worldwide. The oldest fossil record of biological soil crusts of 2.6 Ga presumably had cyanobacteria. The cyanobacteria associated with biocrusts can be grouped as follows: (i) filamentous cyanobacteria (e.g., Microcoleus) stabilizing soils by gluing and forming soil aggregates with extracellular matrix; (ii) either unicellular or filamentous cyanobacteria (e.g., Chroococcidiopsis, Scytonema, and Stigonema) contributing to C and N cycling, and (iii) stochastically occurring cyanobacteria (e.g., Chroococcus, Gleocapsa, Gloeocapsopsis, Cylindrospermum, Phormidium and Tolypothrix). The molecular clock estimates suggest that the formation of biocrusts by eukaryotic algae occurred about 600 million years ago (Ma). The eukaryotic algae are not exclusively found in biocrusts, with the major functional groups as follows: (i) crust-forming algae due to filamentous nature and/or mucilage secretion (e.g., Klebsormidium and Zygogonium), (ii) algae attached to soil particles and crust-forming algae (e.g., Spongiochloris, Neochlorosarcina, and diatoms); (iii) algae that occur within lichens as symbionts, free-living within the biocrusts and those living epiphytically (e.g., Myrmecia and Stichococcus), and (iv) freshwater algae originating from aquatic habitats (e.g., Chlorococcum, Chlamydomonas, Scenedesmus and Mychonastes). Besides cyanobacteria, the members of Proteobacteria (e.g., β-Proteobacteria – Massilia and Comamonas), Actinobacteria (e.g., Streptomyces, Sphaerobacter, Actinomadura, Rubrobacter, Nonomuraea and mycelia genera), Bacteroidetes (Flexibacter, Spirosoma, Flavobacterium, and Sphingobacterium), and those of Armatimonadetes, Gemmatimonadetes, Planctomycetes, and Verrucomicrobia are present in the biological soil crusts. The fungal diversity, which varies with the age and type of biocrusts, includes the lineages of the class Dothideomycetes with the representatives of Pleosporales and the genus Alternaria but with no arbuscular mycorrhizal fungi.122 Applying molecular, culture-independent approaches coupled with physiological and biochemical assessments and their cultured isolates can improve our understanding of the functioning of individual constituents of biological soil crusts.
Human activities, even compressed stress due to footsteps or the use of machinery, and air pollutants from industrial activities have adverse environmental impacts on biological soil crusts. The agronomic importance of biological soil crusts in improving the soil structure and stability, minimizing soil erosion, and plant growth-promoting effect as biofertilizers and biostimulants has received significant attention.123 Kheirfam and Roohi124 examined an inoculation-based technique for forming biocrusts using the soil profile collected from the dried region of Lake Urmia. After 120 days, the microbial inoculation significantly improved the biocrusts indicators such as the contents of total C (225%) and N (3200%), available P (70%) and potassium (19%), pH (−1.20%), soil moisture (15.70%), bulk density (−2.50%), aggregate stability (133%), and surface roughness (34.80%). Thus, inoculation-based techniques can be considered for restoring dried-up and degraded lands. Earlier, Zhou and Zhang125 reported the feasibility of artificially cultivated biological soil crusts for increasing the runoff efficiency with less runoff sediment and recommended them as green materials for harvesting rainwater. In arid or semiarid environments, algae employ diverse survival strategies including the synthesis of intracellular antioxidants (e.g., carotenoids lutein in microalgae) and anti-oxidative enzymes, the strengthening of the cell wall by the secretion of extracellular polymeric substances (EPSs) and cellular membranes (water stress protein and trehalose), and the formation of microbial consortia with fungi and bacteria. These survival strategies have a significant contribution to the ecological functions of biocrusts.
Together with these strategies, the soil fertility improvements by biocrusts make them excellent agents for practical applications to control desertification.126 Recently, De Oliveira and Maciel-Silva127 compared the Brazilian biocrust communities with that from different ecosystems and proposed the utilization of biocrusts as ‘ecosystem engineers’ for colonizing other ecosystems. The meta-analysis of the biocrusts suggested that the soil organic carbon (SOC) concentration increased by 70.9% compared to the uncrusted soil, and the random forest analysis showed that the total N was an essential determinant of the concentration of SOC, followed by climate (p < 0.01).128 Because of their ecological advantages, improvements in soil physical structure and stability, and enhanced concentrations of soil labile C and microbial C and N, biological soil crusts were applied (as broken-skin inoculation, moss) as a nature-based strategy (NbS) in the fields of semiarid rainfed alfalfa (Medicago sativa L.).129 The restoration efforts of degraded lands can significantly benefit from the soil microbiota,130 where biocrusts can be more effective than individual microbial inoculants.
5. Amelioration of saline and sodic soils by microalgae and cyanobacteria
Soil salinization has failed many human civilizations, including the early Mesopotamia from 1200 and 900 BC and now the river basins of the Indo-Ganges in India, the Murray-Darling in Australia, the Yellow River in China, and the San Joaquin Valley in the United States of America. About 23% (0.34 × 109 ha) and 37% (0.56 × 109 ha) of the cultivated lands are saline and sodic soils, respectively, predominantly located in arid and semi-arid regions distributed worldwide. These soil environmental services and agricultural productivities are adversely affected due to natural causes and human activities such as irrigated agriculture, when poorly managed with over-irrigation, seepage, and silting.119 The annual cost of land degradation in irrigated areas due to salinity and sodicity can lead to the lost crop production of about US$ 27.3 billion worldwide. Cyanobacteria colonize the habitats of saline soils because of their salinity sensing-signaling (e.g., activation of K+ transport system, glycosyl glycerol (GG) synthesizing enzyme, histidine protein kinase (HIK), response-regulator protein (RRE) two-component signaling system, and salt overly sensitive (SOS) pathway) and tolerance mechanisms such as active Na+ efflux, K+ transport system, phospholipid modifications, osmotically active molecules and activation and deactivation of enzymes although growth, photosynthesis, plasma membrane composition, cellular homeostasis, reactive oxygen species (ROS) generation, other biochemical pathways, and gene expression and protein synthesis are adversely affected under high salinity stress.131 Thus, the microbial remediation of saline soils has been attempted by employing halophilic and halotolerant strains, consortia, and bacterial enzymes. These approaches are successful to some extent, but many complex factors such as their poor adaptability and application methods limit their widespread acceptance.132
Thomas133 found that the repeated cultivation of Anabaena torulosa in the saline Kharland soils led to a reduction in salinity by 12–35%, which was attributed to an active photosynthesis-linked mechanism for the extrusion of sodium according to the studies using 24Na and 22Na. Kaushik and Subhasini134 reported that algalization decreased the electrical conductivity, soil pH, and exchangeable sodium content in saline soils. On the contrary, Rao and Burns135 found that cyanobacteria were ineffective in alleviating highly degraded alkali soil, with an insignificant increase in the contents of C and N from the soil column studies, even after 17 weeks. Due to their inability to mobilize calcium from native soil calcite for exchanging with sodium in the exchange complex, these organisms were considered ineffective. Pandey et al.136 reported that the sequence of appearance of cyanobacteria, the dominant species in usar soil (alfisol, solonetz, alkaline and widespread in India) was Nostoc calcicola. Its bicarbonate-resistant mutant (HCO3–R) was better in modifying soil pH compared to the wildtype in the laboratory incubation study for two years. Similarly, in another laboratory simulation experiment, Jaiswal et al.137 showed that N. calcicola lowered the pH of usar soil in a 20% soil extract compared to the basal medium. However, the bicarbonate mutant (HCO3–R) could grow at a higher percentage of soil extract (60%). The bicarbonate mutant strain was suggested to be a potential bio-ameliorant after further evaluation at the field level. Srivastava et al.138 found that Aulosira was more prevalent than Nostoc in soil samples collected in Eastern Uttar Pradesh after the denaturing gradient gel electrophoresis profiling of cyanobacterial communities. In this case, the selection of salinity-adapted cyanobacterial species specific to the location to be facilitated is vital for the success of its utilization. Li et al.139 suggested the potential of co-culture of cyanobacteria and bacteria as bio-remediating agents for salt-affected soils. Likewise, Naorem and Udayana140 considered cyanobacteria as add-ons of the beneficial soil microbiota to remediate salt-affected soils under organic farming. In a recent report, Brito et al.141 showed that the soil application of a new terrestrial cyanobacterial species (Oculatella lusitanica LEGE 161147) on lettuce (Lactuca sativa L. cv Marvel of Four Seasons) had a limited priming effect. However, there were beneficial effects by mitigating the deleterious salinity stress by eliciting non-enzymatic antioxidant (i.e., proline, H2O2, and reduced glutathione) responses. However, the cyanobacterial priming effect, including the enhanced activity of N metabolism-related enzymes such as glutamate dehydrogenase, glutamine synthetase, and nitrate reductase, was observed in lettuce plants under non-stress conditions.
The eukaryotic microalgal forms and cyanobacteria are beneficial, renewable resources for agriculture, even in saline and sodic soils.42 Mutale-joan et al.142 examined the potential of microalgae–cyanobacterial extracts as stimulators of salt stress tolerance responses in tomato (Solanum lycopersicum L. JANA F1). The extract formulations (MEF) of microalgae (Dunaliella salina MSD 002 and Chlorella ellipsoidea BEA 0337) and cyanobacterial species (Aphanothece sp. BEA O935B and Arthrospira maxima MSS001) at different levels (MEF1%, MEF5%, and MEF10%) were applied to tomato plants grown under four NaCl concentrations (0, 80, 120, and 150 mM) by soil drenching. The application of MEF5% was found to enhance the vegetative growth, photosynthetic pigments due to improved osmotic adjustment and ion homeostasis, proline accumulation, the activities of CAT and SOD, and absorption of N, P, and K in the plants grown at 80 and 120 mM NaCl. In contrast, leaf lipid peroxidation through ROS oxidative stress was significantly decreased together with a decrease in fatty acid content. The application of MEF led to the enhanced uptake of K+ and reduced Na+/K+ ratio in leaves, suggesting its role in re-establishing ion homeostasis. Thus, the combined microalgal–cyanobacterial formulations can better stimulate the salt tolerance responses, nutrient uptake, and growth of plants. In an earlier study, Grzesik et al.143 observed that the foliar application (i.e., sprayed three times on leaves at three-week intervals during vegetation season) with intact cells of Microcystis aeruginosa MKR 0105, Anabaena sp. PCC 7120 and Chlorella sp. influenced the growth and physiological performance, such as the stability of cytomembranes, chlorophyll content, intensity of net photosynthesis, transpiration, and stomatal conductance of willow plants (Salix viminalis L.), a horticultural plant whose flexible branchlets are used in basketry. Similar investigations on the potential of intact or cellular extracts of microalgae–cyanobacteria in crop plants for improving salinity tolerance are necessary. Up to 2010, the utilization of microalgal and cyanobacterial resources was about 10
000 tons per year, while their commercial production will increase to 27
500 tons per year with a market value of US$ 1.10 billion, especially as food, feed, dietary supplements, cosmetic care products, and biofuels.144,145 Because of their potential to stimulate plant growth and improve stress tolerance, the biomolecules from these organisms can increase their commercial production and agronomic applications.
6. Microalgae and cyanobacteria as biocontrol agents of pathogens and pests
Pathogens and pests are integral to agricultural ecosystems, where pathogens including members of fungi, bacteria, viruses, oomycetes, and Chromista and ‘animal pests’ such as insects, mites, and weeds cause economic losses of crops at the local, regional, national, and global levels. The major food crops such as wheat, rice, maize, potato, and soybean have about 137 pathogens and pests. Their global burden in terms of yield losses ranges from 17% (potato) to 30% (rice), with more losses associated with food-deficit regions and frequently with the emergence or re-emergence of pests and diseases.146 The annual cost of plant diseases is more than US$ 220 billion, which may increase due to global climate change events, a major threat to food security and the environment.147 Evolutionarily, plant-pathogen or pest interactions help develop defense mechanisms to protect plants, pest host breadths and impacts, and maintain the ecological balance in the wild and human-managed systems.148 However, intensive agriculture with pesticides to control pathogens and pests has caused environmental disturbance and non-targeted effects, with long-lasting and far-reaching consequences.149 The theory of ‘trophobiosis’, which was proposed by Chaboussou,150 suggests that the increased availability of soluble nutrients in plant tissues and modification of morphological and chemical defensive traits due to intensive use of chemical fertilizers and pesticides can promote the damage by pathogens and pests in crops. Thus, the vicious cycle of chemical input applications, either repeated or with new compounds, becomes undesirable in agricultural ecologies.
Presently, almost 50% of all crop production compounds available are based on natural products (NP), their semisynthetic derivatives (NPDs), compounds inspired by NPs (NP mimics, NPMs), and especially NP synthetic equivalents, which constitute about 30%.151 Pratt152 described the use of the antibacterial substance, chlorellin, against human pathogenic Gram-positive (Staphylococcus aureus, Streptococcus pyogenes, and Bacillus subtilis) and Gram-negative bacteria (Bacterium coli and Pseudomonas pyocyanea) extracted from Chlorella sp. (C. vulgaris and C. pyrenoidosa), which also could inhibit the further multiplication of their cells. Shaima et al.153 reported the antibacterial activity of microalgae such as C. sorokiniana (UKM2), Chlorella sp. (UKM8), and Scenedesmus sp. (UKM9), which is attributed to the presence of phenol, hexadecenoic acid, phytol, 9,12-octadecadienoic acid, and bicyclo[3.1.1]heptane. Kulik154 reviewed the potential of cyanobacteria and algae to produce antibacterial and antifungal compounds against plant pathogens for biological control. Fungal pathogens (soil-borne, foliar, or fruit pathogens) can cause the most plant diseases with severe economic losses and produce mycotoxins, which are harmful to humans and livestock (see Dean et al.155 for top 10 fungal pathogens). The data presented in Fig. 4 clearly highlights the important role of microalgae and cyanobacteria as potential biocontrol agents against fungal and bacterial pathogens.
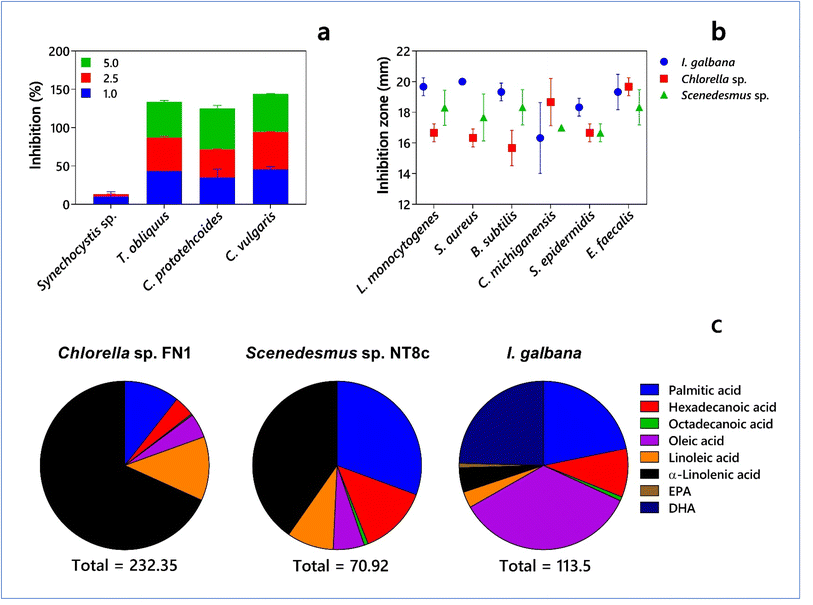 |
| Fig. 4 Biocontrol properties of microalgae and cyanobacteria. (a) Inhibition (%) of Fusarium oxysporum by Synechocystis sp., Tetradesmus obliquus, Chlorella protothecoides, and Chlorella vulgaris at different levels of biomass (g L−1) (based on data from Ferreira et al.156). (b) Antibacterial activity of Isochrysis galbana, Scenedesmus sp. NT8c and Chlorella sp. FN1 against bacterial pathogens, Listeria monocytogenes, Staphylococcus aureus, Bacillus subtilis, Clavibacter michiganensis, Staphylococcus epidermis and Enterococcus faecalis. (c) Fatty acids methyl esters (FAME) in I. galbana, Scenedesmus sp. NT8c and Chlorella sp. FN1 implicated in antimicrobial activity (based on data from Alsenani et al.38) EPA – Eicosapentaenoic acid and DHA – Docosahexaenoic acid. | |
The extracts prepared with methanol, acetone, diethyl ether, ethanol, methyl chloride, and other solvents, using Microcystis, Anabaena, Nostoc, Scytonema, Lyngbya, Oscillatoria, Trichodesmium, and Synechococcus were inhibitory to Aspergillus spp. Fusarium spp. and other pathogens under many in vitro and some in vivo studies (reviewed by Righini et al.157). Roberti et al.158 reported that when a water extract of Anabaena sp. BEA0300B was applied to cotyledonary leaves (two leaves, with other cotyledonary and true leaves untreated) of zucchini (Cucurbita pepo cv. Consul Semencoop, s. r. l, Italy) and challenged with a conidial suspension of Podosphaera xanthii, it resulted in the induction of systemic defense responses (systemic acquired resistance (SAR) and induced systemic resistance (ISR)). On 1, 2, and 3 days of treatment, the total chitinase activity increased (15–38%) and the transient increases of β-1-3-glucanase and peroxidases further increased. The powdery mildew symptoms were reduced by 25%, while the water extract had direct antifungal activity by reducing the pathogen sporulation. Based on the results from this greenhouse experiment, Roberti et al.158 suggested the use of microalgal extracts, after further evaluation, in environmentally friendly disease management.
Zhou et al.159 reported the biocontrol effect of Nostoc piscinale SCAU04 and Anabaena variabilis SCAU26 against the sheath blight pathogen (Rhizoctonia solani Kühn) in rice (cv. Huanghuazhan) under microchamber and micro-area experiments. Diisooctyl adipate, among other cyanobacterial metabolites, had the highest inhibitory effect, and the phytohormone production of salicylic acid, trans-zeatin-riboside, and isopentenyl adenine increased several folds. Thus, the biocontrol mechanisms of the selected cyanobacteria against the sheath blight pathogen include the direct inhibitory effect of biologically active compounds against the fungus, improved production of phytohormones for plant growth and induction of disease resistance, and soil nutrients through N2-fixing ability. In another study, Bao et al.160 demonstrated the inhibitory effect of Anabaena variabilis SCAU30 against not only the rice sheath blight fungal pathogen (R. solani AG-1 IA strain GD-118 by 62–70%) but also bacterial blight pathogen (Xanthomonas oryzae pv. oryzae CCTCC AB 91122 by 45–50%) in microchamber experiments. In the field experiments (12 m × 8 m plots with rice variety Yuexiangzhan and 20 m × 5 m plots with rice variety Gengxiangyou-8), the rice yield increments were 13.90% to 22% due to the combined treatment of A. variabilis SCAU30 and 50% topdressing fertilizer, respectively. A. variabilis SCAU30 is considered a biofertilizer-biopesticide agent for ‘Green Super Rice variety-Huanghuazhan’ production under green agriculture and advocated as ‘a resource-saving and environmentally friendly agriculture system’.161 More importantly, the field-level evaluation of microalgae or their cellular extracts is critical for the widespread acceptance of their application as an agronomic practice in green agriculture.
The insecticidal properties of several macro- and microalgae as well as cyanobacteria have been reported and recently reviewed by Asimakis et al.162 The crude ethanolic extracts of a cyanobacterium (Nostoc carneum), green algae (Parachlorella kessleri, Ulva intestinalis, and Cladophora glomerata), and stonewort (Chara vulgaris) showed insecticidal activity against cotton leafworm (Spodoptera littoralis) when treated against the 2nd and 4th instars in the in vitro assay.163 The ethanolic crude extracts contained an array of saturated and unsaturated fatty acids (particularly polyunsaturated ω-6 linoleic, palmitic, oleic, myristic, α-linolenic, and 7,10-hexadecadienoic acids), essential oils, and phytol and other terpene compounds, and their effects were inhibitory to larval duration, percent pupal formation, pupal duration and weight, moth emergence (%), fecundity and longevity of adults, hatching of eggs, sex ratios, and even larval-pupal-moth malformations. The water- and ethanol-extracts (diluted with distilled water to prepare concentrations of 3%, 5% and 7%) of Spirulina platensis, which contained phenolic compounds such as quercetin, kaempferol, resorcinol, and naphthaline, were found to be toxic to the larvae (newly hatched 2nd and 4th instars) of Spodoptera littoralis.164 Earlier studies indicated that microalgal and cyanobacterial extracts may contain toxic metabolites to higher plants.165,166 Hence, utilizing microalgal and cyanobacterial resources needs scrutiny against individual crop species and at the field levels. However, these organisms can be a source and inspiration for developing synthetic pesticides, especially their applications in organic or green agriculture.
7. Microalgal forms and cyanobacteria in improving grain yield and yield components
The trait of N2 fixation has attracted attention from researchers and agronomic utilization of cyanobacterial strains, especially in rice cultivation. The first report was by Fritsch,9 who examined the abundance and contributions of cyanobacteria to soil fertility through biological N2 fixation in paddy fields. The cyanobacterial symbiosis with Azolla, a heterosporous pteridophyte, was traditionally used in rice cultivation in China as early as 540 AD.167 Shukla and Gupta168 reported that the extract of Phormidium foveolarum (Mont.) Gomont when treated with rice seeds (type 21) by pre-soaking for 24 h in 0.5%, 1% and 5% water led to an increase in the total weight of all ears per plant, the weight of a single ear and weight of 1000 grains. Generally, cyanobacterial strains and microalgae are utilized as biostimulants, which are defined as ‘certain substances, mixtures, and microorganisms, not as inputs of nutrients, that can stimulate the natural nutrition processes in plants. The use of these products is aimed solely at improving the nutrient use efficiency, tolerance to abiotic stress, and quality traits of plants or increasing the availability of confined nutrients in the soil or rhizosphere, which can act in addition to fertilizers to optimize the efficiency of fertilizers and reduce the nutrient application rates.169
During the developmental stages of crop growth, the potential of a yield component is determined by environmental and management conditions and the yield components interact to achieve a given yield. For example, yield components such as the number of plant parts (i.e., tillers per unit area or the number of kernels per head) limit yield more than the size of parts such as kernels. In rice, the grain-yield contributing traits include tillering ability, panicle length, and spikelet fertility, when cultivated under dry-direct seeded conditions and those such as plant height, number of primary branches, secondary branches and filled grains per panicle, dominant effects for yield per hill, yield per tiller and 1000 grain weight are associated with rice under submerged conditions, respectively.170,171 Generally, higher grain yields of rice can be achieved with increasing numbers of tillers and/or panicles per plant and grain setting rates. In intensive agriculture, applying chemical N fertilizer is the most common practice to increase the yields. Generally, biofertilizers and biostimulants are considered as substitutes for chemical fertilizers. Zhang et al.172 reported that the partial substitution of nitrogenous chemical fertilizers with the N2-fixing cyanobacterium, Anabaena azotica FACHB-119, at 30% and 50% significantly increased the number of tillers and panicles per plant compared to higher substitution rates, where the substitution by 30% sustained the rice yields.
Organic agriculture offers a multitude of environmental and soil health benefits. In Brazil, the use of microalgae is allowed for organic vegetable production. Mógor et al.173 examined the potential of lyophilized biomass of Arthrospira platensis (also known as Spirulina platensis) at 1 and 3 g L−1 on the yield gains of organic red beet (Beta vulgaris) and found significant increases in hypocotyl diameters and consequent yield gains. In another study, Geries and Elsadany174 found that the foliar spraying of Spirulina platensis 100 and 120 days after transplanting (DAT) and seedling inoculation with endophytic-, N2-fixing Pseudomonas stutzeri (108 CFU mL−1) in onion (Allium cepa L., var. Giza red), even in the presence of different N doses (100% and 75% of the recommended dose), improved the marketable yield, total bulb yield, bulb weight and diameter, lowest cumulative weight loss percentages of bulbs during storage and reduced the cull weight. On the contrary, Soppelsa et al.175 found that the microalgal extract prepared using Spirulina spp. (4.0 g L−1) when applied to strawberry (Fragaria ananassa cv. Elsanta) as foliar spray from the pre-flowering stage led to lower fruit firmness (−18%).
Faheed et al.176 reported that seeds of lettuce (Lactuca sativa) after germination in culture medium containing Chlorella vulgaris and the addition of 2 and 3 g dry microalga kg−1 soil significantly increased both the fresh and dry weight of the seedlings. The yield potential in maize (Zea mays L.) under the influence of Spirulina platensis and Chlorella vulgaris was shown to be significant by Dineshkumar et al.177 (Fig. 5). Garcia-Gonzalez and Sommerfeld178 applied the green algal cellular extracts and dry biomass of Acutodesmus dimorphus as a seed primer, foliar spray, and biofertilizer to tomato and evaluated the seed germination, plant growth, and fruit production. Although the experiment was terminated early, the higher fruit numbers suggested the likely effectiveness of 100 g biofertilizer 22 days before transplant. Presently, there is renewed interest in microalgae for improving global food security. Vigani et al.179 proposed that the strategies adopted by the European Union entitled ‘Innovating for Sustainable Growth: A Bioeconomy for Europe’180 and ‘Microalgae-based Products for the Food and Feed Sector: An Outlook for Europe’,181 and their scientific and technological capacity can be employed to prepare novel microalgae-based products for foreign markets, leading to the strategic acquisitions of foreign companies. Biotechnological innovations can improve microalgal productivity and utilize waste and cheaper substrates to reduce production costs.
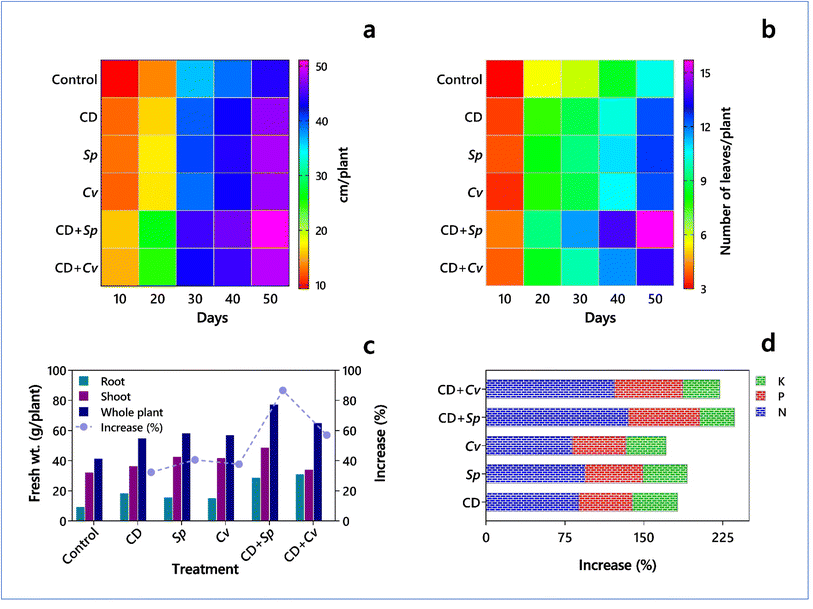 |
| Fig. 5 Impact of microalgae and cyanobacteria on the yield potential of maize (Zea mays L.) yield potential (based on data from Dineshkumar et al.177). Impact of Spirulina platensis and Chlorella vulgaris on (a) shoot length, (b) number of leaves, (c) fresh weight, and (d) nutrient composition in maize plant. CD – Cow dung, Sp – S. platensis, and Cv – C. vulgaris. | |
8. Microalgae and cyanobacteria in biosequestration of soil organic carbon (SOC)
C-containing compounds, also present in the complex organic material of soil, are a critical component of soil organic matter (SOM). The SOC is primarily from the fixation of atmospheric CO2 by photosynthetic higher plants and microorganisms. Globally, the stock of SOC in the top 1 m and 3 m soil profiles is about 1500–2400 Pg C (1 Pg = 1 Gt) and 2300 Pg C, respectively.182 Because of land cultivation (i.e., about 45% of global soils), arable soils contribute more to agricultural greenhouse gas emissions than that under permanent crops and pastures. Given that all living forms are C-based, soil biodiversity is critical to many ecosystem functions, including C cycling, which occurs over long- and short-term time scales, ranging from a few minutes to a few billion years. The SOC, which is captured, sequestered, and mineralized by microorganisms and an indicator of soil health, significantly contributes to food production, mitigation, and adaptation to climate change. Intensive land management and the vast degraded land areas warrant efforts to improve future C storage, the capacity of soil to buffer against climate change events, and the resilience of agricultural food production systems. The most crucial constraint with aggressive targets for soil C sequestration is the requirement of N to maintain the C
:
N stoichiometry in soils. In general, agricultural soils have about C
:
N at the ratio of 10–12. Then, about 100 million tons of N is required to increase 4 billion tons of CO2 annually.183 Any synthetic chemical N fertilizer application will increase nitrous oxide emissions, another highly potent greenhouse gas from soils. Hence, many ‘best management practices’ and ‘frontier technologies’ for which many technological and economic barriers need to be overcome are being evaluated worldwide to enhance the soil C sequestration potential in the long-term. The biological negative emission (BNE) strategies involving management practices that can regulate the biotic processes for improved soil C balance in the long-term have gained significance. In the present context, reducing atmospheric CO2 with almost no enhanced emission of nitrous oxide due to the fertilizer application is desirable.181
Among the ‘best management practices’, reduced tillage or ‘no-till’ can improve the soil C capture, sequestration, and storage, depending on the soil texture, type and the prevailing climate. Navarro-Noya et al.184 reported that tillage practice affected the relative abundance of Actinobacteria, β-Proteobacteria, and α-Proteobacteria more, while that of Bacteroidetes, β-Proteobacteria, Cyanobacteria, and Gemmatimonadetes was affected by residue management. Both edaphic cyanobacteria and microalgae can improve the soil aggregate stability by producing exopolymeric matrices and enmeshing filamentous microbiota over aggregates, improving the soil C sequestration.185 Haiming et al.186 showed that even short-term tillage managements influenced the soil autotrophic bacterial community in the double-cropping rice fields. There have been several surprises regarding cyanobacterial clades from phylogenetic studies although they are the most studied aerobic and phototrophic microorganisms. Recently, two new clades (i.e., 4C0d-2 (Melainabacteria) and ML635J-21 (Sericytochromatia)) with chemoheterotrophic and fermentative metabolisms were proposed.5,187 Cano-Diaz et al.188 showed the environmental preferences of different cyanobacterial clades from a field survey and co-occurrence network analysis. The members of the photosynthetic Oxyphotobacteria-dominated cluster were abundant in arid and semi-arid areas, while that of non-photosynthetic Sericytochromatia- and Melainabacteria-dominated clusters are in hyper-arid oligotrophic and acidic/humid environments, respectively. Recently, Larsson et al.189 demonstrated the involvement of mixotrophic protists capable of both phototrophy and phago-heterotrophy in the production of C-rich ‘mucospheres’ in disproportionally contributing to the vertical flux in the ocean C cycling. In soils, the mixotrophic and fermentative metabolisms of cyanobacteria associated with C cycling have been overlooked to date. Besides the agronomic application of N2-fixing and plant bio-stimulatory cyanobacteria, the utilization of CO2-fixing (photosynthetic) cyanobacteria warrants attention and future research to improve the soil C sequestration.
In arid and semi-arid environments, bacteria, cyanobacteria, or their combination collected from the biological soil crusts and inoculated into the soils were found to have higher potential for C sequestration.124 The sequestered C increased from 0.232 to 0.294 g m−2 days−1, and when scaled up, it could increase from 0.84 to 1.07 ton ha−1 year−1, equivalent to the atmospheric CO2 removal of 3.11 to 3.93 ton ha−1 year−1. The addition of biochar is one of the options among the ‘frontier technologies for soil C sequestration.183 The C capture by photosynthesis in microalgae is more efficient than by higher plants due to the presence of active bicarbonate pumps, which can elevate the internal concentrations of CO2 and inhibit photorespiration. Earlier, Sayre190 suggested three strategies for algal C capture and sequestration, as follows: (i) permanent burial of total fresh biomass, (ii) permanent burial of algal lipids, and (iii) soil amendment with algal biochar, each having some advantages and liabilities as well. Algal biochar can sequester about 50% of C present in biomass. Many microalgal species, including Endornia sp., Chlorella sp., Euglena gracilis, Chlorococcum littorale, Scenedesmus sp., and Cyanidium caldarium can tolerate CO2 up to the level of 20% to 100% (v/v).191 The successful implementation of microalgae-based C capture and sequestration requires many improvements and innovations and the application of biorefinery principles. Earlier, Beal et al.192 evaluated the techno-economic and life-cycle assessment of an integrated 2800 ha production facility of algae with bioenergy carbon capture and storage (ABECCS). They reported the financial break-even achieved for algal biomass, sold as fishmeal replacement for US$ 1400 per ton with a $ 68 per ton carbon credit and as soybean replacement for $ 600 per ton with a $ 278 per ton C credit. Thus, ABECCS can sustainably reduce atmospheric CO2 with economic, energetic, and environmental benefits.192 In a recent report, Jassey et al.91 showed that soil algae capture about 3.60 Pg C annually, corresponding to about 6% of the net primary production of terrestrial vegetation (Table 2). Although certain species of eukaryotic microalgae and cyanobacteria have been identified as potential agents in soil C capture, sequestration, and storage (Fig. 6), the biodiversity and global implications of these organisms demand more research efforts and the development of technological opportunities.
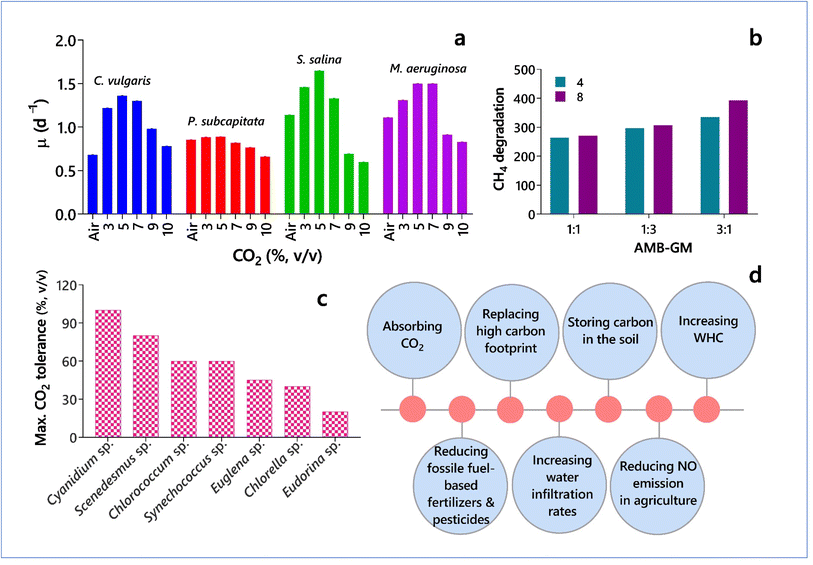 |
| Fig. 6 (a) Specific growth rates of microalgae (Chlorella vulgaris and Pseudokirchneriella subcapitata) and cyanobacteria (Synechocystis salina and Microcystis aeruginosa) at different levels of CO2 (based on data from Gonçalves et al.193). (b) Methane degradation rate (mg CH4 gbiomass−1 day−1) by the coculture of an alkaliphilic methanotrophic bacteria consortium (AMB) and green microalga (GM) Scenedesmus obtusiusculus at different methane concentrations (4% and 8%) (based on data from Ruiz-Ruiz et al.194). (c) Maximum CO2 tolerance by different microalgal species (based on data from Onyeaka et al.191). (d) Climate resilience and mitigation by microalgae and cyanobacteria (based on data from Nichols24). | |
9. Algalization of soils: successes and limitations
‘Algalization’ which was referred earlier to the process of cyanobacterial application as biofertilizer in rice fields can include microalgal species or their products as plant biostimulants.195 The potential of the microalgae- and cyanobacteria-based products has been evaluated at various levels, more often by researchers. Recently, Abinandan et al.196 demonstrated that algalization of two acid soils with Desmodesmus sp. MAS1 and Heterochlorella sp. MAS3 significantly enriched bacteria belonging to rhizobacteria and diazotrophs such as Acetobacter, Azospirillum, Bradyrhizobium, Gluconacetobacter, Nitrobacter, Burkholderia, Comamonas, Herbaspirillum, Enterobacter, Nitrosococcus, Brevibacillus, Enterococcus, Frankia, and Anabaena, which are largely implicated in promoting soil health and plant growth. Successful field-scale algalization has been reported in a few states in India and in other countries such as China, Egypt, Vietnam, and the Philippines.13,101,197 Although the agronomic importance of algae has been known since the 1900s, the commercial production of algal biofertilizers or biostimulants is in its infancy. Interestingly, several microalgal species are cultured and produced as human health food and nutraceuticals, animal/aquaculture feed, and feed additives in the industries. As human food and animal feed, Spirulina (Arthrospira platensis) has been collected as the blue-colored ‘techuitlats’ and as ‘dihe’ and eaten by the Aztecs living in the valley of Mexico and the Kanembu people along the shore of Lake Chad, respectively, for centuries.198,199 The industrial production of this well-known microalgal product only commenced in man-made ponds in 1978.200 Similarly, the first commercial production of Chlorella, marketed as a health food product for immune stimulation, reducing blood lipids, and treating gastric ulcers, wounds, and constipation, was by the Nihon Chlorella in Taiwan during the 1960s.201 In a recent report, Beal et al.192 suggested that the sustainability of global fisheries and aquaculture can be improved by the commercial production of microalgal products as a replacement for fishmeal and fish oil. Their proposed model of establishing and deploying about 100 facilities (based on microalgal production in Hawaii, each of 111 ha with a hybrid system of photobioreactors and raceway ponds for the cultivation of Desmodesmus sp.) can replace all the domestic production of fishmeal in Thailand.
Many algal products are produced from low-tech farming (harvesting from the wild or simple man-made structures of ponds and tanks, i.e., ‘open’ systems) and high-tech bioprocess engineering industries (using fermenters and photobioreactors, i.e., ‘closed’ systems).202,203 The need for light, relatively slow growth rate, the requirements for temperature, C, oxygen, source and supply of nutrients, selection of strains suitable either for out-door cultivation or in bioreactors, the capital-intensive bioreactors, and the contaminants and diseases of both the ‘open’ and ‘closed’ systems continue to be significant challenges in scaling up and realizing the potential of microalgae, especially from laboratory to land applications. Besides understanding the foundations of good science, skilled and experienced personnel are required to produce high value bioproducts or biomass of microalgal species. Most commercially successful companies have chosen high-priced products, either metabolites or whole cells of microalgae.
The agronomic applications of cyanobacteria as biofertilizers for improved N2 fixation and/or microalgae as biostimulants depend largely on their commercial production. In the global market, the popular biofertilizers with N2-fixing ability are Cell-Tech, Nitragin Gold, TagTeam, Mamezo, R-Processing seeds and Hyper Coating seeds from Novozymes and Tokachi Federation of Agricultural Cooperatives, respectively, and the popular genera used for production are Bradyrhizobium, Sinorhizobium, Azorhizobium, Mesorhizobium, and Allorhizobium (http://www.mordorintelligence.com). The global biofertilizers market is predicted to have a CAGR of 12.10% during the period 2022–2028. In fact, the market projection exclusively for microalgae during 2020–2028 is expected to have a CAGR of 5.40%. In addition, the global area under organic farming will increase to more than 75 million ha during this period. In this case, increased awareness, environmental benefits, and government policies can make the commercial production of biofertilizers and biostimulants including microalgae more relevant. To date, the regional and national markets, especially in India, have more rhizobacteria-based products than phototrophs-based products. The increases in crop yields due to biofertilization compared to uninoculated crops remain insufficient to motivate farmers and industrialists to utilize microalgal resources. According to the global meta-analysis, Schütz et al.204 showed that the yield responses due to biofertilization by microbial inoculants ranged from 6.10% to 21.70%, with better responses in the areas under dry climate, followed by that under tropical climate. The soil P concentration, organic matter content, and pH are also important determinants of the yield responses due to microbial inoculation. These limiting factors and inconsistencies due to climate variabilities thwart the efforts of governments, industries, and farming communities to rely on biofertilizers and biostimulants for yield increments. However, the environmental benefits of CO2 sequestration by microalgae can now provide the necessary impetus for their commercial production. More importantly, the collective benefits from the metabolism of C, N, and oxygen of phototrophic inoculants, in addition to their bio-stimulatory effects on crop yields, need better agronomic- and environmental auditing. Furthermore, the benefit-to-harm ratio of microalgal applications needs to be considered, especially during their long-term usage. Compared to microalgae, certain species of cyanobacteria can produce toxic metabolites known as cyanotoxins. These cyanotoxins are hepatotoxins (i.e., microcystins, nodularins and cylindrospermopsins) and neurotoxins such as anatoxin-a, saxitoxins, and beta-N-methylamino-L-alanine. These toxins, of which microcystins have been examined the most, can inhibit germination and plant growth, and accumulate in plants, adversely affecting other micro- and macroorganisms, and eventually entering the food web of soils.205 Future studies should examine the genomic potential for all the traits of microalgae, including those related to the production of toxins and antimicrobial compounds.
10. Future perspectives
There are many challenges related to the technological advances for agricultural applications and environmental benefits from microalgae and cyanobacteria, beginning with taxonomic identification, evaluation of genomic potential and functional capabilities, and availability of authentic cultures. The conventional phenotype-based classification of algae has several limitations, in addition to modern taxonomy, guided by the rRNA relationships inferred from the DNA sequences associated with different subunits. Both Park et al.22 and Parks et al.206 proposed the Genome Taxonomy Database (GTDB; http://gtdb.ecogenomic.org, a normalized genome-based phylogenetic framework) based on the phylogenetic inferences from the concatenation of ubiquitous, single-copy proteins (covering about 254
090 bacterial and 4316 archaeal genomes and metagenome-assembled or single-cell genomes of uncultured members). In addition, there is a proposal of the suffix -ota to denote phyla.207 These considerations have led to a new proposal on cyanobacteria. Presently, the phylum Cyanobacteriota encompasses three classes: (i) Cyanobacteria (previously called Oxyphotobacteria – all oxygenic phototrophs), (ii) Vampirovibrionia (replacing the Candidatus name Melainabacteria) and Candidatus Sericytochromatia.208 Interestingly, Vampirovibrio chlorellavorus, the first cultured representative of Candidatus Melainabacteria is an obligate predator of Chlorella vulgaris.209 Similar to the difficulties associated with the identification of cyanobacteria, the systematic process for identifying eukaryotic microalgal strains requires curated databases on the DNA barcodes and repositories.210 These advances in microalgal taxonomy present a major disadvantage, where all the described cultures need renaming and revision of their classification. However, the chief opportunity of polyphasic, molecular taxonomic methods is the inclusion of uncultured microalgae (such as the representatives of Metagenome-Assembled Genomes, MAGs) in our understanding. The genomic and metagenomic elucidation of culturable and uncultured cyanobacterial and microalgal lineages will help to re-evaluate their diversity, distribution, and ecological functions.
The primary and high-order endosymbiosis and the diversification of many eukaryotic photosynthetic lineages strongly suggest the global ecological importance of microalgae and demand greater appreciation for their conservation and avoiding the disruption of the evolution of eukaryotes. Nevertheless, the benefits of microalgal evolutionary developments are difficult to account for in monetary terms. Recently, Altman and Mesoudi211 showed how plant genes (e.g., domestication) coevolve with human agricultural activities by applying frameworks and concepts such as Gene-Culture Coevolution (GCC), Niche Construction (NC), and Cumulative Cultural Evolution (CCE). Similar investigations are necessary for the soil microbiomes of agro ecosystems to gain insights into the potential and limitations of soil C sequestration, atmospheric N2 fixation, and oxygen cycling in the rhizospheres. Earlier, Thrall et al.212 also proposed the need for applying the evolutionary approaches to the management of pests and pathogens (biotic interactions) in agroecosystems.
Microbial culture collections are critical in understanding the metabolic lifestyles and genome characteristics of individual organisms. Globally, the microbial culture collections of 146 centers situated in 51 countries have about 67
812 and 61
421 plant-associated strains and those of soil origin, respectively (https://gcm.wdcm.org). Hirose et al.94 noted that there are only about major 15 culture collections that maintain about 7000 cyanobacterial strains. The British Phycological Society reported that algal culture collections are necessary to ‘generate solutions to societal challenges by stimulating interaction between academia and bioindustry’ (https://brphycsoc.org/algal-culture-collections-in-the-omics-age/).
The transformation of ‘energy to matter’ is important in living soils, which are central to most agricultural activities.213 Recently, Gunina and Kuzyakov214 provided an experiment-based review on the soil microbial communities using organics as the source of energy, not as a source of C. Hence, the plant litter and rhizodeposition are sources of energy, rather than sources of C, in most soil microbial communities. This hypothesis strengthens the importance of phototrophic microorganisms in the C cycling processes of soils. There is also a strong need to understand the ecological roles of nitrogenases present in N2-fixing prokaryotes. Oehlmann and Rebelein215 reviewed the ‘side reactivities’ of three isozymes of nitrogenases (Mo-, V- and Fe-only) for the reduction of carbon monoxide (highest activity by V nitrogenase) and CO2 (highest activity by Fe-only nitrogenase). The cyanobacterial members also possess genes coding for the structural components of V-nitrogenase.101 Hence, the nitrogenases and alternative nitrogenases (V- and Fe-only) of the N2-fixing cyanobacteria warrant future research on their ecological significance in the soil N and C cycling processes.
Heterotrophic bacteria can promote the growth of cyanobacteria, for example, Pannonibacter sp. and Chryseobacterium sp. had a positive effect on the growth of Microcystis aeruginosa FACHB-905.34 Earlier, Borowitzka and Vonshak203 suggested that the microbiome (bacterial flora associated with microalga) could be the causal agent for the collapse of large-scale heterotrophic algal cultures under stressed and poorly mixed systems. Presently, we know that Vampirovibrio chlorellavorus is a cyanobacterial obligate predator of Chlorella vulgaris.209 Thus, the inadequacies in culture collections, inconsistencies and changing taxonomic assignments, and limited innovations in commercial production pose serious challenges to their utilization in agriculture. More importantly, the commercial production of microalgae for agricultural applications will remain difficult to achieve if profitability is limited to yield increments and contemporary monetary gains.
11. Conclusions
Microalgae and cyanobacteria are effective in improving the nutrient contents, structure, and fertility of soils. Several microalgal species are selected based on traits, such as the fixation of carbon dioxide and dinitrogen and phosphorus cycling, which are beneficial to plants. However, their potential to ameliorate drylands and saline and sodic soils is yet to gain widespread acceptance. Similarly, their biocontrol properties and potential to improve the yield and yield components and the biosequestration of soil organic carbon demand more research efforts and technological innovations. The benefit-to-harm ratio of microalgal applications needs to be considered based on their genomic potential, especially during their long-term application. Nevertheless, the new hope for utilizing these microalgal resources in agriculture is their abundance, which is underestimated by the present-day culture-based methods and improved understanding of their evolutionary benefits and their ecological services. Future work should improve their pure-culture collections, understand their metabolic lifestyles, examine their ecological roles, know their evolutionary significance, advance new knowledge and create awareness among the farming communities and policymakers.
Author contributions
B. R.: writing-original draft, investigation, and data curation. N. R. M.: visualization and writing-original draft. K. V.: conceptualization, writing-review & editing, and supervision. M. M.: conceptualization, data curation, resources, validation, writing review & editing, and supervision.
Conflicts of interest
There are no conflicts to declare.
Acknowledgements
B. R. thanks and acknowledges ICAR-Indian Agricultural Research Institute and the Indian Council of Agricultural Research for support and funding under the extra-mural grant & colleagues and students for experiments, experiences, and insights.
References
- M. F. Hohmann-Marriott and R. E. Blankenship, Evolution of photosynthesis, Annu. Rev. Plant Biol., 2011, 62, 515–548 CrossRef CAS PubMed.
- L. M. Ward and P. M. Shih, The evolution and productivity of carbon fixation pathways in response to changes in oxygen concentration over geological time, Free Radical Biol. Med., 2019, 140, 188–199 CrossRef CAS PubMed.
- D. An, S. M. Caffrey, J. Soh, A. Agrawal, D. Brown, K. Budwill, X. Dong, P. F. Dunfield, J. Foght and L. M. Gieg, Metagenomics of hydrocarbon resource environments indicates aerobic taxa and genes to be unexpectedly common, Environ. Sci. Technol., 2013, 47, 10708–10717 CrossRef CAS PubMed.
- S. C. Di Rienzi, I. Sharon, K. C. Wrighton, O. Koren, L. A. Hug, B. C. Thomas, J. K. Goodrich, J. T. Bell, T. D. Spector and J. F. Banfield, The human gut and groundwater harbor non-photosynthetic bacteria belonging to a new candidate phylum sibling to Cyanobacteria, Elife, 2013, 2, e01102 CrossRef PubMed.
- R. M. Soo, C. T. Skennerton, Y. Sekiguchi, M. Imelfort, S. J. Paech, P. G. Dennis, J. A. Steen, D. H. Parks, G. W. Tyson and P. Hugenholtz, An expanded genomic representation of the phylum Cyanobacteria, Genome Biol. Evol., 2014, 6, 1031–1045 CrossRef PubMed.
- J. F. Strassert, I. Irisarri, T. A. Williams and F. Burki, A molecular timescale for eukaryote evolution with implications for the origin of red algal-derived plastids, Nat. Commun., 2021, 12, 1–13 CrossRef PubMed.
- H. Qiu, H. S. Yoon and D. Bhattacharya, Algal endosymbionts as vectors of horizontal gene transfer in photosynthetic eukaryotes, Front. Plant Sci., 2013, 4, 366 Search PubMed.
- R. I. Ponce-Toledo, P. López-García and D. Moreira, Horizontal and endosymbiotic gene transfer in early plastid evolution, New Phytol., 2019, 224, 618–624 CrossRef PubMed.
- F. E. Fritsch, The subaerial and freshwater algal flora of the tropics. A phytogeographical and ecological study, Ann. Bot., 1907, 21, 235–275 CrossRef.
-
W. H. Harrison and P. A. S. Aiyer, The Gases of Swamp Rice Soils, Their Composition and Relationship to the Crop, Imperial Department of Agriculture, N. D. I. A. R. Institute, India, 1913 Search PubMed.
- R. Bouilhac, Sur la fixation de l'azote atmosphérique par l'association des algues et des bactéries, C. R. Acad., 1896, 123, 828–830 CAS.
-
H. Molisch, Pflanzenbiologie in Japan auf Grund eigener Beobachtungen Jena, 1926 Search PubMed.
- P. K. De, The role of blue-green algae in nitrogen fixation in rice-fields, Proc. R. Soc. B: Biol. Sci., 1939, 127, 121–139 CAS.
-
A. Howard, Crop-production in India: a Critical Survey of its Problems, Oxford University Press, 1924 Search PubMed.
- N. Renuka, A. Guldhe, R. Prasanna, P. Singh and F. Bux, Microalgae as multi-functional options in modern agriculture: current trends, prospects and challenges, Biotechnol. Adv., 2018, 36, 1255–1273 CrossRef CAS PubMed.
- S. Abinandan, S. R. Subashchandrabose, K. Venkateswarlu and M. Megharaj, Soil microalgae and cyanobacteria: the biotechnological potential in the maintenance of soil fertility and health, Crit. Rev. Biotechnol., 2019, 39, 981–998 CrossRef PubMed.
- D. Chittora, M. Meena, T. Barupal, P. Swapnil and K. Sharma, Cyanobacteria as a source of biofertilizers for sustainable agriculture, Biochem. Biophys. Rep., 2020, 22, 100737 Search PubMed.
- G. Santini, N. Biondi, L. Rodolfi and M. R. Tredici, Plant biostimulants from cyanobacteria: an emerging strategy to improve yields and sustainability in agriculture, Plants, 2021, 10, 643 CrossRef CAS PubMed.
- C. Jones, J. Lawton and M. Shachak, Organisms as ecosystem engineers, Oikos, 1994, 69, 373–386 CrossRef.
- M. Maqubela, P. Mnkeni, O. M. Issa, M. Pardo and L. D’acqui, Nostoc cyanobacterial inoculation in South African agricultural soils enhances soil structure, fertility, and maize growth, Plant Soil, 2009, 315, 79–92 CrossRef CAS.
- W. Wang, Y. Liu, D. Li, C. Hu and B. Rao, Feasibility of cyanobacterial inoculation for biological soil crusts formation in desert area, Soil Biol. Biochem., 2009, 41, 926–929 CrossRef CAS.
- C.-H. Park, X. R. Li, Y. Zhao, R. L. Jia and J.-S. Hur, Rapid development of cyanobacterial crust in the field for combating desertification, PLoS One, 2017, 12, e0179903 CrossRef PubMed.
- G. Mugnai, F. Rossi, V. J. M. N. L. Felde, C. Colesie, B. Büdel, S. Peth, A. Kaplan and R. De Philippis, Development of the polysaccharidic matrix in biocrusts induced by a cyanobacterium inoculated in sand microcosms, Biol. Fertil. Soils, 2018, 54, 27–40 CrossRef.
-
K. Nichols, Microalgae as a beneficial soil amendment, https://myland.ag/wp-content/uploads/MyLand-Microalgae-White-Paper-nov2020.pdf, 2020, accessed 22 June 2022 Search PubMed.
- S. Chamizo, G. Mugnai, F. Rossi, G. Certini and R. De Philippis, Cyanobacteria inoculation improves soil stability and fertility on different textured soils: gaining insights for applicability in soil restoration, Front. Environ. Sci., 2018, 6, 49 CrossRef.
- J. R. Román, B. Roncero-Ramos, S. Chamizo, E. Rodríguez-Caballero and Y. Cantón, Restoring soil functions by means of cyanobacteria inoculation: importance of soil conditions and species selection, Land Degrad. Dev., 2018, 29, 3184–3193 CrossRef.
- G. Mazor, G. J. Kidron, A. Vonshak and A. Abeliovich, The role of cyanobacterial exopolysaccharides in structuring desert microbial crusts, FEMS Microbiol. Ecol., 1996, 21, 121–130 CrossRef CAS.
- S. Chamizo, A. Adessi, G. Mugnai, A. Simiani and R. De Philippis, Soil type and cyanobacteria species influence the macromolecular and chemical characteristics of the polysaccharidic matrix in induced biocrusts, Microb. Ecol., 2019, 78, 482–493 CrossRef CAS PubMed.
- M. Muñoz-Rojas, N. M. M. de Lima, S. Chamizo and M. A. Bowker, Restoring post-fire ecosystems with biocrusts: living, photosynthetic soil surfaces, Curr. Opin. Environ. Sci. Health, 2021, 23, 100273 CrossRef.
- S. Shanthakumar, S. Abinandan, K. Venkateswarlu, S. R. Subashchandrabose and M. Megharaj, Algalization of acid soils with acid-tolerant strains: improvement in pH, carbon content, exopolysaccharides, indole acetic acid and dehydrogenase activity, Land Degrad. Dev., 2021, 32, 3157–3166 CrossRef.
- B. Metting and W. R. Rayburn, The influence of a microalgal conditioner on selected Washington soils: an empirical study, Soil Sci. Soc. Am. J., 1983, 47, 682–685 CrossRef.
- W. R. Barclay and R. A. Lewin, Microalgal polysaccharide production for the conditioning of agricultural soils, Plant Soil, 1985, 88, 159–169 CrossRef CAS.
- Y. Lu and J. Xu, Phytohormones in microalgae: a new opportunity for microalgal biotechnology?, Trends Plant Sci., 2015, 20, 273–282 CrossRef CAS PubMed.
- S. Gao, Y. Kong, J. Yu, L. Miao, L. Ji, L. Song and C. Zeng, Isolation of axenic cyanobacterium and the promoting effect of associated bacterium on axenic cyanobacterium, BMC Biotechnol., 2020, 20, 1–11 CrossRef PubMed.
- M.-J. Chanda, N. Merghoub and H. El Arroussi, Microalgae polysaccharides: the new sustainable bioactive products for the development of plant bio-stimulants?, World J. Microbiol. Biotechnol., 2019, 35, 1–10 CrossRef CAS PubMed.
- F. Rachidi, R. Benhima, L. Sbabou and H. El Arroussi, Microalgae polysaccharides bio-stimulating effect on tomato plants: growth and metabolic distribution, Biotechnol. Rep., 2020, 25, e00426 CrossRef PubMed.
- C. Mutale-Joan, B. Redouane, E. Najib, K. Yassine, K. Lyamlouli, S. Laila, Y. Zeroual and E. A. Hicham, Screening of microalgae liquid extracts for their bio stimulant properties on plant growth, nutrient uptake and metabolite profile of Solanum lycopersicum L, Sci. Rep., 2020, 10, 1–12 CrossRef PubMed.
- F. Alsenani, K. R. Tupally, E. T. Chua, E. Eltanahy, H. Alsufyani, H. S. Parekh and P. M. Schenk, Evaluation of microalgae and cyanobacteria as potential sources of antimicrobial compounds, Saudi Pharm. J., 2020, 28, 1834–1841 CrossRef CAS PubMed.
- D. Mc Gee, L. Archer, T. J. Smyth, G. T. Fleming and N. Touzet, Bioprospecting and LED-based spectral enhancement of antimicrobial activity of microalgae isolated from the west of Ireland, Algal Res., 2020, 45, 101704 CrossRef.
- W. A. Stirk and J. van Staden, Bioprospecting for bioactive compounds in microalgae: antimicrobial compounds, Biotechnol. Adv., 2022, 107977 CrossRef CAS PubMed.
- C. Wang, M. Qi, J. Guo, C. Zhou, X. Yan, R. Ruan and P. Cheng, The active phytohormone in microalgae: the characteristics, efficient detection, and their adversity resistance applications, Molecules, 2021, 27, 46 CrossRef PubMed.
- A. L. Alvarez, S. L. Weyers, H. M. Goemann, B. M. Peyton and R. D. Gardner, Microalgae, soil and plants: a critical review of microalgae as renewable resources for agriculture, Algal Res., 2021, 54, 102200 CrossRef.
- P. De and M. Suiaiman, Influence o! algal growth in the rice fields on the yield of crop, Indian J. Agric. Sci., 1950, 20, 327–342 Search PubMed.
-
R. N. Singh, Role of Blue-Green Algae in Nitrogen Economy of Indian Agriculture, Indian Counsil of Agricutlure Research, New Delhi, 1961 Search PubMed.
-
G. Venkataraman, Algal Biofertilizers and Rice Cultivation, Today and Tommorrow’s Printers and Publishers, New Delhi, 1972, p. 71 Search PubMed.
- B. Mandal, P. Vlek and L. Mandal, Beneficial effects of blue-green algae and Azolla, excluding supplying nitrogen, on wetland rice fields: a review, Biol. Fertil. Soils, 1999, 28, 329–342 CrossRef CAS.
- A. Rai, E. Söderbäck and B. Bergman, Cyanobacterium-plant symbioses, New Phytol., 2000, 147, 449–481 CrossRef CAS PubMed.
- H. Priya, R. Prasanna, B. Ramakrishnan, N. Bidyarani, S. Babu, S. Thapa and N. Renuka, Influence of cyanobacterial inoculation on the culturable microbiome and growth of rice, Microbiol. Res., 2015, 171, 78–89 CrossRef CAS PubMed.
-
BiofertilizerMarket, Biofertilizers Market by Form (Liquid, Carrier-Based), Mode of Application (Soil Treatment, Seed Treatment), Type (Nitrogen-fixing, Phosphate solubilizing & Mobilizing, Potash Solubilizing & Mobilizing), Crop Type, and Region – Global Forecast to 2026, May 2021, https://www.marketsandmarkets.com/Market-Reports/compound-biofertilizers-customized-fertilizers-market-856.html?gclid=Cj0KCQiAhP2BBhDdARIsAJEzXlEkF1eSdH2KUL3nwAB2O09fxC5VIXkjEcvHQTyAtnLf1ROFCrquYQcaAsM-EALw_wcB, accessed 05 July 2022 Search PubMed.
- Y. Yanni, Potential of indigenous cyanobacteria to contribute to rice performance under different schedules of nitrogen application, World J. Microbiol. Biotechnol., 1991, 7, 48–52 CrossRef CAS PubMed.
- D. P. Singh, R. Prabha, M. S. Yandigeri and D. K. Arora, Cyanobacteria-mediated phenylpropanoids and phytohormones in rice (Oryza sativa) enhance plant growth and stress tolerance, Antonie Van Leeuwenhoek, 2011, 100, 557–568 CrossRef CAS PubMed.
- Z. N. T. Begum, R. Mandal and S. Islam, Effect of cyanobacterial biofertilizer on the growth and yield components of two HYV of rice, J. Algal Biomass Util., 2011, 2, 1–9 Search PubMed.
- A. Rodríguez, A. Stella, M. Storni, G. Zulpa and M. Zaccaro, Effects of cyanobacterial extracellular products and gibberellic acid on salinity tolerance in Oryza sativa L, Saline Syst., 2006, 2, 1–4 CrossRef PubMed.
- T. Taubaev, N. Galkina and T. Vasigov, Some data on stimulating effect of Chlorella vulgaris (strain 157) on seeds and seedlings of cotton and rice, Uzb. Biol. Zh., 1970, 60–61 Search PubMed.
- A. Elhafiz, A. E. S. S. Gaur, N. H. M. Osman and T. R. Lakshmi,
Chlorella vulgaris and Chlorella pyrenoidosa live cells appear to be promising sustainable biofertilizer to grow rice, lettuce, cucumber and eggplant in the UAE soils, Rec. Res. Sci. Technol., 2015, 7, 14–21 CrossRef.
- Z. Obreht, N. W. Kerby, M. Gantar and P. Rowell, Effects of root-associated N2-fixing cyanobacteria on the growth and nitrogen content of wheat (Triticum vulgare L.) seedlings, Biol. Fertil. Soils, 1993, 15, 68–72 CrossRef CAS.
- N. Karthikeyan, R. Prasanna, L. Nain and B. D. Kaushik, Evaluating the potential of plant growth promoting cyanobacteria as inoculants for wheat, Eur. J. Soil Biol., 2007, 43, 23–30 CrossRef CAS.
- A. Rana, M. Joshi, R. Prasanna, Y. S. Shivay and L. Nain, Biofortification of wheat through inoculation of plant growth promoting rhizobacteria and cyanobacteria, Eur. J. Soil Biol., 2012, 50, 118–126 CrossRef CAS.
- R. Kholssi, E. A. Marks, J. Miñón, O. Montero, A. Debdoubi and C. Rad, Biofertilizing effect of Chlorella sorokiniana suspensions on wheat growth, J. Plant Growth Regul., 2019, 38, 644–649 CrossRef CAS.
- L. Mau, J. Kant, R. Walker, C. M. Kuchendorf, S. D. Schrey, U. Roessner and M. Watt, Wheat can access phosphorus from algal biomass as quickly and continuously as from mineral fertilizer, Front. Plant Sci., 2021, 12, 631314 CrossRef PubMed.
- F. Martini, G. Beghini, L. Zanin, Z. Varanini, A. Zamboni and M. Ballottari, The potential use of Chlamydomonas reinhardtii and Chlorella sorokiniana as biostimulants on maize plants, Algal Res., 2021, 60, 102515 CrossRef PubMed.
- K. M. Sivalingam and K. Delfe, Isolation and identification of cyanobacteria and its impact on seed germination potential of maize (Zea mays L.) using seed germination experiment, Int. J. Eng. Appl. Sci. Technol., 2019, 4, 65–72 Search PubMed.
- K. Dadhich, A. Varma and G. Venkataraman, The effect of Calothrix inoculation on vegetable crops, Plant Soil, 1969, 31, 377–379 CrossRef.
- E. La Bella, A. Baglieri, E. I. Rovetto, P. Stevanato and I. Puglisi, Foliar spray application of Chlorella vulgaris extract: effect on the growth of lettuce seedlings, Agronomy, 2021, 11, 308 CrossRef CAS.
- H. El Arroussi, R. Benhima, A. Elbaouchi, B. Sijilmassi, N. El Mernissi, A. Aafsar, I. Meftah-Kadmiri, N. Bendaou and A. Smouni, Dunaliella salina exopolysaccharides: a promising biostimulant for salt stress tolerance in tomato (Solanum lycopersicum), J. Appl. Phycol., 2018, 30, 2929–2941 CrossRef CAS.
- K. Supraja, B. Behera and P. Balasubramanian, Efficacy of microalgal extracts as biostimulants through seed treatment and foliar spray for tomato cultivation, Ind. Crops Prod., 2020, 151, 112453 CrossRef CAS.
- S. Basu, A. S. Roy, K. Mohanty and A. K. Ghoshal, Enhanced CO2 sequestration by a novel microalga: Scenedesmus obliquus SA1 isolated from bio-diversity hotspot region of Assam, India, Bioresour. Technol., 2013, 143, 369–377 CrossRef CAS PubMed.
- J. H. Duarte, E. G. de Morais, E. M. Radmann and J. A. V. Costa, Biological CO2 mitigation from coal power plant by Chlorella fusca and Spirulina sp, Bioresour. Technol., 2017, 234, 472–475 CrossRef CAS PubMed.
- K. Kumar and D. Das, Growth characteristics of Chlorella sorokiniana in airlift and bubble column photobioreactors, Bioresour. Technol., 2012, 116, 307–313 CrossRef CAS PubMed.
- M. Nayak, A. Karemore and R. Sen, Sustainable valorization of flue gas CO2 and wastewater for the production of microalgal biomass as a biofuel feedstock in closed and open reactor systems, RSC Adv., 2016, 6, 91111–91120 RSC.
- G. Swarnalatha, N. S. Hegde, V. S. Chauhan and R. Sarada, The effect of carbon dioxide rich environment on carbonic anhydrase activity, growth and metabolite production in indigenous freshwater microalgae, Algal Res., 2015, 9, 151–159 CrossRef.
- K. L. Yeh and J. S. Chang, Nitrogen starvation strategies and photobioreactor design for enhancing lipid content and lipid production of a newly isolated microalga Chlorella vulgaris ESP-31: implications for biofuels, Biotechnol. J., 2011, 6, 1358–1366 CrossRef CAS PubMed.
- H. S. Yun, M. K. Ji, Y. T. Park, E. S. Salama and J. Choi, Microalga, Acutodesmus obliquus KGE 30 as a potential candidate for CO2 mitigation and biodiesel production, Environ. Sci. Pollut. Res., 2016, 23, 17831–17839 CrossRef CAS PubMed.
- B. B. Cardias, M. G. de Morais and J. A. V. Costa, CO2 conversion by the integration of biological and chemical methods: Spirulina sp. LEB 18 cultivation with diethanolamine and potassium carbonate addition, Bioresour. Technol., 2018, 267, 77–83 CrossRef CAS PubMed.
- G. M. da Rosa, L. Moraes, B. B. Cardias and J. A. V. Costa, Chemical absorption and CO2 biofixation via the cultivation of Spirulina in semicontinuous mode with nutrient recycle, Bioresour. Technol., 2015, 192, 321–327 CrossRef PubMed.
- G. Kim, W. Choi, C.-H. Lee and K. Lee, Enhancement of dissolved inorganic carbon and carbon fixation by green alga Scenedesmus sp. in the presence of alkanolamine CO2 absorbents, Biochem. Eng. J., 2013, 78, 18–23 CrossRef CAS.
- G.-Y. Kim, J. Heo, H.-S. Kim and J.-I. Han, Bicarbonate-based cultivation of Dunaliella salina for enhancing carbon utilization efficiency, Bioresour. Technol., 2017, 237, 72–77 CrossRef CAS PubMed.
- W. Farooq, S. R. Naqvi, M. Sajid, A. Shrivastav and K. Kumar, Monitoring lipids profile, CO2 fixation, and water recyclability for the economic viability of microalgae Chlorella vulgaris cultivation at different initial nitrogen, J. Biotechnol., 2022, 345, 30–39 CrossRef CAS PubMed.
- R. Prasanna, S. Babu, A. Rana, S. R. Kabi, V. Chaudhary, V. Gupta, A. Kumar, Y. S. Shivay, L. Nain and R. K. Pal, Evaluating the establishment and agronomic proficiency of cyanobacterial consortia as organic options in wheat–rice cropping sequence, Exp. Agric., 2013, 49, 416–434 CrossRef.
- R. Prasanna, A. Adak, S. Verma, N. Bidyarani, S. Babu, M. Pal, Y. S. Shivay and L. Nain, Cyanobacterial inoculation in rice grown under flooded and SRI modes of cultivation elicits differential effects on plant growth and nutrient dynamics, Ecol. Eng., 2015, 84, 532–541 CrossRef.
- R. Prasanna, S. Babu, N. Bidyarani, A. Kumar, S. Triveni, D. Monga, A. K. Mukherjee, S. Kranthi, N. Gokte-Narkhedkar and A. Adak, Prospecting cyanobacteria-fortified composts as plant growth promoting and biocontrol agents in cotton, Exp. Agric., 2015, 51, 42–65 CrossRef.
- R. Prasanna, N. Bidyarani, S. Babu, F. Hossain, Y. S. Shivay and L. Nain, Cyanobacterial inoculation elicits plant defense response and enhanced Zn mobilization in maize hybrids, Cogent Food Agric., 2015, 1, 998507 CrossRef.
- R. Prasanna, F. Hossain, S. Babu, N. Bidyarani, A. Adak, S. Verma, Y. S. Shivay and L. Nain, Prospecting cyanobacterial formulations as plant-growth-promoting agents for maize hybrids, S. Afr. J. Plant Soil, 2015, 32, 199–207 CrossRef.
-
A. A. Q. Ahmed, K. A. Odelade and O. O. Babalola, Microbial inoculants for improving carbon sequestration in agroecosystems to mitigate climate change, Handbook of Climate Change Resilience, Springer International Publishing, Cham, Switzerland, 2019, pp. 1–21 Search PubMed.
- P. In-na, A. A. Umar, A. D. Wallace, M. C. Flickinger, G. S. Caldwell and J. G. Lee, Loofah-based microalgae and cyanobacteria biocomposites for intensifying carbon dioxide capture, J. CO2 Util., 2020, 42, 101348 CrossRef CAS.
- P.-H. Chen, H.-L. Liu, Y.-J. Chen, Y.-H. Cheng, W.-L. Lin, C.-H. Yeh and C.-H. Chang, Enhancing CO2 bio-mitigation by genetic engineering of cyanobacteria, Energy Environ. Sci., 2012, 5, 8318–8327 RSC.
- J. Sanderman, T. Hengl and G. J. Fiske, Soil carbon debt of 12,000 years of human land use, Proc. Natl. Acad. Sci., 2017, 114, 9575–9580 CrossRef CAS PubMed.
-
GSP, Global Soil Partnership Endorses Guidelines on Sustainable Soil Management, 2017 Search PubMed.
- S. Mitsui and K. Kurihara, The intake and utilization of carbon by plant roots from C14-labeled urea, Part I, The determination of radioactive carbon of plant materials and a preliminary seedling experiment utilizing C14-labeled urea, Soil Sci. Plant Nutr., 1957, 3, 59–64 CrossRef CAS.
- C. Chen, T. Park, X. Wang, S. Piao, B. Xu, R. K. Chaturvedi, R. Fuchs, V. Brovkin, P. Ciais and R. Fensholt, China and India lead in greening of the world through land-use management, Nat. Sustainable, 2019, 2, 122–129 CrossRef PubMed.
- V. E. Jassey, R. Walcker, P. Kardol, S. Geisen, T. Heger, M. Lamentowicz, S. Hamard and E. Lara, Contribution of soil algae to the global carbon cycle, New Phytol., 2022, 234, 64–76 CrossRef CAS PubMed.
- E. S. Boyd and J. W. Peters, New insights into the evolutionary history of biological nitrogen fixation, Front. Microbiol., 2013, 4, 201 Search PubMed.
- K. Forchhammer and K. A. Selim, Carbon/nitrogen homeostasis control in cyanobacteria, FEMS Microbiol. Rev., 2020, 44, 33–53 CrossRef CAS PubMed.
- Y. Hirose, Y. Ohtsubo, N. Misawa, C. Yonekawa, N. Nagao, Y. Shimura, T. Fujisawa, Y. Kanesaki, H. Katoh and M. Katayama, Genome sequencing of the NIES Cyanobacteria collection with a focus on the heterocyst-forming clade, DNA Res., 2021, 28, dsab024 CrossRef PubMed.
-
A. N. Rai, B. Bergman and U. Rasmussen, Cyanobacteria in symbiosis, Springer Dordrecht, 2002 Search PubMed.
- F.-W. Li, P. Brouwer, L. Carretero-Paulet, S. Cheng, J. De Vries, P.-M. Delaux, A. Eily, N. Koppers, L.-Y. Kuo and Z. Li, Fern genomes elucidate land plant evolution and cyanobacterial symbioses, Nat. Plants, 2018, 4, 460–472 CrossRef CAS PubMed.
- P. E. Mortimer, J. Hammond, K. D. Hyde, H. Gui and J. Xu, Root symbioses as belowground C pumps: a mitigation strategy against rising CO2 levels, Circular Agric. Syst., 2021, 1, 1–3 Search PubMed.
-
G. S. Venkataraman, Blue-green algae for rice production: a manual for its promotion, Food & Agriculture Org., 1981 Search PubMed.
- P.-A. Roger and J. Ladha, Biological N2 fixation in wetland rice fields: estimation and contribution to nitrogen balance, Plant Soil, 1992, 141, 41–55 CrossRef CAS.
-
M. R. Setiawati, M. Damayani, D. Herdiyantoro, P. Suryatmana, D. Anggraini and F. H. Khumairah, The application dosage of Azolla pinnata in fresh and powder form as organic fertilizer on soil chemical properties, growth and yield of rice plant, in AIP Conference Proceedings, AIP Publishing LLC, 2018, vol. 1927, 1, p. 030017 Search PubMed.
- N. Bhooshan, A. Singh, A. Sharma, C. Verma, A. Kumar and S. Pabbi, Cyanobacterial biofertilizer's successful journey from rural technology to commercial enterprise: an Indian perspective, J. Appl. Phycol., 2020, 32, 3995–4002 CrossRef.
- M. A. Addo and P. C. Dos Santos, Distribution of nitrogen-fixation genes in prokaryotes containing alternative nitrogenases, Chembiochem, 2020, 21, 1749–1759 CrossRef CAS PubMed.
- R. B. Chowdhury and X. Zhang, Phosphorus use efficiency in agricultural systems: a comprehensive assessment through the review of national scale substance flow analyses, Ecol. Indicators, 2021, 121, 107172 CrossRef.
- D. Cordell, J.-O. Drangert and S. White, The story of phosphorus: global food security and food for thought, Global Environ. Change, 2009, 19, 292–305 CrossRef.
- P. Roychoudhury and B. Kaushik, Solubilization of Mussorie rock phosphate by cyanobacteria, Curr. Sci., 1989, 58, 569–570 CAS.
- B. Whitton, S. Grainger, G. Hawley and J. Simon, Cell-bound and extracellular phosphatase activities of cyanobacterial isolates, Microb. Ecol., 1991, 21, 85–98 CrossRef CAS PubMed.
- M. S. Yandigeri, K. K. Meena, R. Srinivasan and S. Pabbi, Effect of mineral phosphate solubilization on biological nitrogen fixation by diazotrophic cyanobacteria, Indian J. Microbiol., 2011, 51, 48–53 CrossRef CAS PubMed.
- L. Achbergerová and J. Nahálka, Polyphosphate-an ancient energy source and active metabolic regulator, Microb. Cell Fact., 2011, 10, 1–14 CrossRef PubMed.
- A. E. Solovchenko, T. T. Ismagulova, A. A. Lukyanov, S. G. Vasilieva, I. V. Konyukhov, S. I. Pogosyan, E. S. Lobakova and O. A. Gorelova, Luxury phosphorus uptake in microalgae, J. Appl. Phycol., 2019, 31, 2755–2770 CrossRef CAS.
- C. Mukherjee, R. Chowdhury and K. Ray, Phosphorus recycling from an unexplored source by polyphosphate accumulating microalgae and cyanobacteria – a step to phosphorus security in agriculture, Front. Microbiol., 2015, 6, 1421 Search PubMed.
- A. Golzary, O. Tavakoli, Y. Rezaei and A. Karbassi, Wastewater treatment by Azolla filiculoides: a study on color, odor, COD, nitrate, and phosphate removal, Pollution, 2018, 4, 69–76 CAS.
-
Cyanobacterial Lifestyle and its Applications in Biotechnology, ed. P. K. Singh, A. Kumar, M. K. Yadav and S. Singh, Academic Press, Cambridge, Massachusetts, United States, 2022, pp. 281–306 Search PubMed.
- V. Ördög, W. Stirk, G. Takács, P. Pőthe, A. Illés, C. Bojtor, A. Széles, B. Tóth, J. van Staden and J. Nagy, Plant biostimulating effects of the cyanobacterium Nostoc piscinale on maize (Zea mays L.) in field experiments, S. Afr. J. Bot., 2021, 140, 153–160 CrossRef.
- V. Sharma, R. Prasanna, F. Hossain, V. Muthusamy, L. Nain, S. Das, Y. S. Shivay and A. Kumar, Priming maize seeds with cyanobacteria enhances seed vigour and plant growth in elite maize inbreds, 3 Biotech, 2020, 10, 1–15 CrossRef CAS PubMed.
- J. S. Craigie, Seaweed extract stimuli in plant science and agriculture, J. Appl. Phycol., 2011, 23, 371–393 CrossRef CAS.
- D. Ronga, E. Biazzi, K. Parati, D. Carminati, E. Carminati and A. Tava, Microalgal biostimulants and biofertilisers in crop productions, Agronomy, 2019, 9, 192 CrossRef CAS.
- G. Colla and Y. Rouphael, Microalgae: new source of plant biostimulants, Agronomy, 2020, 10, 1240 CrossRef.
- I. Perera, S. R. Subashchandrabose, K. Venkateswarlu, R. Naidu and M. Megharaj, Consortia of cyanobacteria/microalgae and bacteria in desert soils: an underexplored microbiota, Appl. Microbiol. Biotechnol., 2018, 102, 7351–7363 CrossRef CAS PubMed.
- J. Huang, G. Zhang, Y. Zhang, X. Guan, Y. Wei and R. Guo, Global desertification vulnerability to climate change and human activities, Land Degrad. Dev., 2020, 31, 1380–1391 CrossRef.
-
Biological Soil Crusts: an Organizing Principle in Drylands, ed. J. Belnap, B. Weber and B. Büdel, Springer, Cham, 2016, pp. 3–13 Search PubMed.
-
P. A. Micheli, Noua plantarum genera iuxta Tournefortii methodum disposita auctore Petro Antonio Michelio Flor, Typis Bernardi Paperini, 1729 Search PubMed.
-
B. Büdel, T. Dulić, T. Darienko, N. Rybalka and T. Friedl, Cyanobacteria and Algae of Biological Soil Crusts, in Biological Soil Crusts: an Organizing Principle in Drylands, ed. J. Belnap, B. Weber and B. Büdel, Springer, Cham, 2016, pp. 55–80 Search PubMed.
-
A. Gholamhosseinian, A. Sepehr, B. Asgari Lajayer, N. Delangiz and T. Astatkie, Biological soil
crusts to keep soil alive, rehabilitate degraded soil, and develop soil habitats, in Microbial Polymers, A. Vaishnav and D. K. Choudhary, Springer, Singapore, 2021, pp. 289–309 Search PubMed.
- H. Kheirfam and M. Roohi, Accelerating the formation of biological soil crusts in the newly dried-up lakebeds using the inoculation-based technique, Sci. Total Environ., 2020, 706, 136036 CrossRef CAS PubMed.
- G. Zhou and W. Zhang, Artificial biological soil crust property and potential for rainwater harvest, Zhongguo Shengtai Nongye Xuebao, 2012, 20, 1329–1333 Search PubMed.
- Q. Lu, Y. Xiao and Y. Lu, Employment of algae-based biological soil crust to control desertification for the sustainable development: a mini-review, Algal Res., 2022, 65, 102747 CrossRef.
- M. F. De Oliveira and A. S. Maciel-Silva, A review of biological soil crusts and how they can colonize other worlds: insights from these Brazilian ecosystem engineers, J. Exp. Bot., 2022, 73, 4362–4379 CrossRef PubMed.
- H. Xu, Y. Zhang, X. Shao and N. Liu, Soil nitrogen and climate drive the positive effect f biological soil crusts on soil organic carbon sequestration in drylands: a meta-analysis, Sci. Total Environ., 2022, 803, 150030 CrossRef CAS PubMed.
- W. Wang, R. Zhou, B.-Z. Wang, L. Zhao, Z.-Y. Zhao, M. S. Sheteiwy, X.-W. Fang, J.-M. Deng, Y.-Z. Su and Y.-C. Xiong, Biocrust as a nature-based strategy (NbS) to restore the functionality of degraded soils in semiarid rainfed alfalfa (Medicago sativa L.) field, J. Cleaner Prod., 2022, 336, 130378 CrossRef CAS.
- O. Coban, G. B. De Deyn and M. van der Ploeg, Soil microbiota as game-changers in restoration of degraded lands, Science, 2022, 375, abe0725 CrossRef PubMed.
-
R. P. Singh, P. Yadav, R. Kujur, K. D. Pandey and R. K. Gupta, Cyanobacteria and Salinity Stress Tolerance, in Cyanobacterial Lifestyle and its Applications in Biotechnology, ed. P. Singh, M. Fillat and A. Kumar, Academic Press, Cambridge, Massachusetts, United States, 2022, pp. 253–280 Search PubMed.
-
S. Arora, Microbial approaches for bio-amelioration and management of salt affected soils, in Soil Science: Fundamentals to Recent Advances, ed. A. Rakshit, S. Singh, P. Abhilash and A. Biswas, Springer, Singapore, 2021, pp. 433–447 Search PubMed.
- J. Thomas, Biological nitrogen fixation, Nuclear India, 1977, 15, 2–8 Search PubMed.
- B. Kaushik and D. Subhashini, Amelioration of salt-affected soils with blue-green algae. II. Improvement in soil properties, Proc. Natl. Acad. Sci., India, Sect. B, 1985, 51, 386–389 Search PubMed.
- D. Rao and R. G. Burns, The influence of blue-green algae on the biological amelioration of alkali soils, Biol. Fertil. Soils, 1991, 11, 306–312 CrossRef.
- K. D. Pandey, P. N. Shukla, D. D. Giri and A. K. Kashyap, Cyanobacteria in alkaline soil and the effect of cyanobacteria inoculation with pyrite amendments on their reclamation, Biol. Fertil. Soils, 2005, 41, 451–457 CrossRef.
- P. Jaiswal, A. K. Kashyap, R. Prasanna and P. K. Singh, Evaluating the potential of N. calcicola and its bicarbonate resistant mutant as bioameleorating agents for ‘usar’soil, Indian J. Microbiol., 2010, 50, 12–18 CrossRef CAS PubMed.
- A. K. Srivastava, P. Bhargava, A. Kumar, L. C. Rai and B. A. Neilan, Molecular characterization and the effect of salinity on cyanobacterial diversity in the rice fields of Eastern Uttar Pradesh, India, Saline Systems, 2009, 5, 1–17 CrossRef PubMed.
- H. Li, Q. Zhao and H. Huang, Current states and challenges of salt-affected soil remediation by cyanobacteria, Sci. Total Environ., 2019, 669, 258–272 CrossRef CAS PubMed.
-
A. Naorem and S. K. Udayana, Remediation of salt affected soils through microbes to promote organic farming, in Advances in Organic Farming, ed. V. Meena, S. Meena, A. Rakshit, J. Stanley and S. Rao, Woodhead Publishing, Sawston, United Kingdom, 2021, pp. 75–92 Search PubMed.
- Â. Brito, M. Rocha, J. Kaštovský, J. Vieira, C. P. Vieira, V. Ramos, M. Correia, M. Santos, R. Mota and J. Roque, A new cyanobacterial species with a protective effect on lettuce grown under salinity stress: envisaging sustainable agriculture practices, J. Appl. Phycol., 2022, 34, 915–928 CrossRef.
- C. Mutale-joan, F. Rachidi, H. A. Mohamed, N. E. Mernissi, A. Aasfar, M. Barakate, D. Mohammed, L. Sbabou and H. E. Arroussi, Microalgae-cyanobacteria–based biostimulant effect on salinity tolerance mechanisms, nutrient uptake, and tomato plant growth under salt stress, J. Appl. Phycol., 2021, 33, 3779–3795 CrossRef CAS.
- M. Grzesik, Z. Romanowska-Duda and H. Kalaji, Effectiveness of cyanobacteria and green algae in enhancing the photosynthetic performance and growth of willow (Salix viminalis L.) plants under limited synthetic fertilizers application, Photosynthetica, 2017, 55, 510–521 CrossRef CAS.
- M. L. Amorim, J. Soares, J. S. D. R. Coimbra, M. D. O. Leite, L. F. T. Albino and M. A. Martins, Microalgae proteins: production, separation, isolation, quantification, and application in food and feed, Crit. Rev. Food Sci. Nutr., 2021, 61, 1976–2002 CrossRef CAS PubMed.
- R. Hachicha, F. Elleuch, H. Ben Hlima, P. Dubessay, H. de Baynast, C. Delattre, G. Pierre, R. Hachicha, S. Abdelkafi and P. Michaud, Biomolecules from microalgae and cyanobacteria: applications and market survey, Appl. Sci., 2022, 12, 1924 CrossRef CAS.
- S. Savary, L. Willocquet, S. J. Pethybridge, P. Esker, N. McRoberts and A. Nelson, The global burden of pathogens and pests on major food crops, Nat. Ecol. Evol., 2019, 3, 430–439 CrossRef PubMed.
-
IPPC, IPPC Secretariat. 2021. Scientific review of the impact of climate change on plant pests – a global challenge to prevent and mitigate plant pest risks in agriculture, forestry and ecosystems, FAO on behalf of the IPPC Secretariat, Rome, 2021, DOI:10.4060/cb4769en, accessed 05 July 2022.
- A. V. Gougherty and T. J. Davies, Towards a phylogenetic ecology of plant pests and pathogens, Philos. Trans. R. Soc. B: Biol. Sci., 2021, 376, 20200359 CrossRef PubMed.
- D. A. Martinez, U. E. Loening, M. C. Graham and A. Gathorne-Hardy, When the medicine feeds the problem; do nitrogen fertilisers and pesticides enhance the nutritional quality of crops for their pests and pathogens?, Front. Sustain. Food Syst., 2021, 5, 234 Search PubMed.
-
F. Chaboussou, Healthy crops: a new agricultural revolution, Jon Carpenter Publishing, Charlbury, UK, 2004 Search PubMed.
- T. C. Sparks and R. J. Bryant, Impact of natural products on discovery of, and innovation in, crop protection compounds, Pest Manage. Sci., 2022, 78, 399–408 CrossRef CAS PubMed.
- R. Pratt, T. Daniels, J. J. Eiler, J. Gunnison, W. Kumler, J. F. Oneto, L. A. Strait, H. Spoehr, G. Hardin and H. Milner, Chlorellin, an antibacterial substance from Chlorella, Science, 1944, 99, 351–352 CrossRef CAS PubMed.
- A. F. Shaima, N. H. M. Yasin, N. Ibrahim, M. S. Takriff, D. Gunasekaran and M. Y. Ismaeel, Unveiling antimicrobial activity of microalgae Chlorella sorokiniana (UKM2), Chlorella sp. (UKM8) and Scenedesmus sp. (UKM9), Saudi J. Biol. Sci., 2022, 29, 1043–1052 CrossRef CAS PubMed.
- M. M. Kulik, The potential for using cyanobacteria (blue-green algae) and algae in the biological control of plant pathogenic bacteria and fungi, Eur. J. Plant Pathol., 1995, 101, 585–599 CrossRef.
- R. Dean, J. A. Van Kan, Z. A. Pretorius, K. E. Hammond-Kosack, A. Di Pietro, P. D. Spanu, J. J. Rudd, M. Dickman, R. Kahmann and J. Ellis, The Top 10 fungal pathogens in molecular plant pathology, Mol. Plant Pathol., 2012, 13, 414–430 CrossRef PubMed.
- A. Ferreira, L. Melkonyan, S. Carapinha, B. Ribeiro, D. Figueiredo, G. Avetisova and L. Gouveia, Biostimulant and biopesticide potential of microalgae growing in piggery wastewater, Environ. Adv., 2021, 4, 100062 CrossRef CAS.
- H. Righini, O. Francioso, A. Martel Quintana and R. Roberti, Cyanobacteria: a natural source for controlling agricultural plant diseases caused by fungi and oomycetes and improving plant growth, Horticulturae, 2022, 8, 58 CrossRef.
- R. Roberti, S. Galletti, P. Burzi, H. Righini, S. Cetrullo and C. Perez, Induction of defence responses in zucchini (Cucurbita pepo) by Anabaena sp. water extract, Biol. Control, 2015, 82, 61–68 CrossRef CAS.
- Y. Zhou, J. Bao, D. Zhang, Y. Li, H. Li and H. He, Effect of heterocystous nitrogen-fixing cyanobacteria against rice sheath blight and the underlying mechanism, Appl. Soil Ecol., 2020, 153, 103580 CrossRef.
- J. Bao, C. Zhuo, D. Zhang, Y. Li, F. Hu, H. Li, Z. Su, Y. Liang and H. He, Potential applicability of a cyanobacterium as a biofertilizer and biopesticide in rice fields, Plant Soil, 2021, 463, 97–112 CrossRef CAS.
-
Q. Zhang, Theory and Practice of Resource-Saving and Environment-Friendly Agricultural Production System, Science Press, Beijing, China, 2015, 1–10 Search PubMed.
- E. Asimakis, A. A. Shehata, W. Eisenreich, F. Acheuk, S. Lasram, S. Basiouni, M. Emekci, S. Ntougias, G. Taner and H. May-Simera, Algae and their metabolites as potential bio-pesticides, Microorganisms, 2022, 10, 307 CrossRef CAS PubMed.
-
A. Saber, S. Hamed, E. Abdel-Rahim and M. Cantonati, Insecticidal prospects of algal and cyanobacterial extracts against the cotton leafworm Spodoptera littoralis, Vie Milieu, 2018, 68, pp. 199–212 Search PubMed.
- R. S. Rashwan and D. M. Hammad, Toxic effect of Spirulina platensis and Sargassum vulgar as natural pesticides on survival and biological characteristics of cotton leaf worm Spodoptera littoralis, Sci. Afr., 2020, 8, e00323 Search PubMed.
- F. K. Gleason and D. E. Case, Activity of the natural algicide, cyanobacterin, on angiosperms, Plant Physiol., 1986, 80, 834–837 CrossRef CAS PubMed.
- C. Rioboo, J. E. O'Connor, R. Prado, C. Herrero and Á. Cid, Cell proliferation alterations in Chlorella cells under stress conditions, Aquat. Toxicol., 2009, 94, 229–237 CrossRef CAS PubMed.
-
S. Kulasooriya, Constraints for the widespread use of Azolla in rice production, in Nitrogen Fixation – Developments in Plant and Soil Sciences, ed. M. Polsinelli, R. Materassi and M. Vincenzini, Springer, Dordrecht, 1991, 48, pp. 473–479 Search PubMed.
- A. Shukla and A. Gupta, Influence of algal growth-promoting substances on growth, yield and protein contents of rice plants, Nature, 1967, 213, 744 CrossRef CAS.
-
EU, EU 2019/1009. Regulation of the European Parliament and of the Council Laying Down Rules on the Making Available on the Market of EU Fertilising Products and Amending Regulations (EC) No 1069/2009 and (EC) No 1107/2009 and Repealing Regulation (EC) No 2003/2003, 2019, https://eur-lex.europa.eu/legal-content/EN/TXT/PDF/?uri=OJ:%20L:2019:170:FULL%26from=EN, accessed 12 June 2022 Search PubMed.
- N. Sandhu, S. R. Subedi, V. K. Singh, P. Sinha, S. Kumar, S. Singh, S. K. Ghimire, M. Pandey, R. B. Yadaw and R. K. Varshney, Deciphering the genetic basis of root morphology, nutrient uptake, yield, and yield-related traits in rice under dry direct-seeded cultivation systems, Sci. Rep., 2019, 9, 1–16 CrossRef CAS PubMed.
- R. K. Ganapati, M. G. Rasul, U. Sarker, A. Singha and M. Faruquee, Gene action of yield and yield contributing traits of submergence tolerant rice (Oryza sativa L.) in Bangladesh, Bull. Natl. Res. Cent., 2020, 44, 1–7 CrossRef.
- J. Zhang, X. Song, H. Wei, W. Zhou, C. Peng and D. Li, Effect of substituting nitrogen fertilizer with nitrogen-fixing cyanobacteria on yield in a double-rice cropping system in southern China, J. Appl. Phycol., 2021, 33, 2221–2232 CrossRef CAS.
- Á. F. Mógor, J. de Oliveira Amatussi, G. Mógor and G. B. de Lara, Bioactivity of cyanobacterial biomass related to amino acids induces growth and metabolic changes on seedlings and yield gains of organic red beet, Am. J. Plant Sci., 2018, 9, 966 CrossRef.
- L. Geries and A. Y. Elsadany, Maximizing growth and productivity of onion (Allium cepa L.) by Spirulina platensis extract and nitrogen-fixing endophyte Pseudomonas stutzeri, Arch. Microbiol., 2021, 203, 169–181 CrossRef CAS PubMed.
- S. Soppelsa, M. Kelderer, C. Casera, M. Bassi, P. Robatscher, A. Matteazzi and C. Andreotti, Foliar applications of biostimulants promote growth, yield and fruit quality of strawberry plants grown under nutrient limitation, Agronomy, 2019, 9, 483 CrossRef CAS.
- F. A. Faheed and Z. A. Fattah, Effect of Chlorella vulgaris as bio-fertilizer on growth parameters and metabolic aspects of lettuce plant, J. Agric. Soc. Sci., 2008, 4, 165–169 Search PubMed.
- R. Dineshkumar, J. Subramanian, J. Gopalsamy, P. Jayasingam, A. Arumugam, S. Kannadasan and P. Sampathkumar, The impact of using microalgae as biofertilizer in maize (Zea mays L.), Waste Biomass Valorization, 2019, 10, 1101–1110 CrossRef CAS.
- J. Garcia-Gonzalez and M. Sommerfeld, Biofertilizer and biostimulant properties of the microalga Acutodesmus dimorphus, J. Appl. Phycol., 2016, 28, 1051–1061 CrossRef PubMed.
- M. Vigani, C. Parisi, E. Rodríguez-Cerezo, M. J. Barbosa, L. Sijtsma, M. Ploeg and C. Enzing, Food and feed products from micro-algae: market opportunities and challenges for the EU, Trends Food Sci. Technol., 2015, 42, 81–92 CrossRef CAS.
-
EC, Communication from the Commission to the European Parliament, the Council, the European Economic and Social Committee and the Committee of the Regions. Innovating for Sustainable Growth: a Bioeconomy for Europe, https://eur-lex.europa.eu/legal-content/EN/TXT/?uri=CELEX%3A52012DC0060#document1, 2012, accessed 05 July 2022 Search PubMed.
- C. Enzing, M. Ploeg, M. Barbosa and L. Sijtsma, Microalgae-based products for the food and feed sector: an outlook for Europe, JRC Sci. Policy Rep., 2014, 19–37 Search PubMed.
-
G. Peralta, L. Di Paolo, I. Luotto, C. Omuto, M. Mainka, K. Viatkin and Y. Yigini, Global soil organic carbon sequestration potential map (GSOCseq v1. 1) – Technical manual, FAO, Rome, 2022, pp. 255, ISBN: 978-92-5-133796-7, DOI:10.4060/cb2642en.
- K. Paustian, E. Larson, J. Kent, E. Marx and A. Swan, Soil C sequestration as a biological negative emission strategy, Front. Climate, 2019, 8 CrossRef.
- Y. E. Navarro-Noya, S. Gómez-Acata, N. Montoya-Ciriaco, A. Rojas-Valdez, M. C. Suárez-Arriaga, C. Valenzuela-Encinas, N. Jiménez-Bueno, N. Verhulst, B. Govaerts and L. Dendooven, Relative impacts of tillage, residue management and crop-rotation on soil bacterial communities in a semi-arid agroecosystem, Soil Biol. Biochem., 2013, 65, 86–95 CrossRef CAS.
- O. Crouzet, L. Consentino, J.-P. Pétraud, C. Marrauld, J.-P. Aguer, S. Bureau, C. Le Bourvellec, L. Touloumet and A. Berard, Soil photosynthetic microbial communities mediate aggregate stability: influence of cropping systems and herbicide use in an agricultural soil, Front. Microbiol., 2019, 10, 1319 CrossRef PubMed.
- T. Haiming, L. Chao, S. Lihong, C. Kaikai, W. Li, L. Weiyan and X. Xiaoping, Effects of different short-term tillage managements on rhizosphere soil autotrophic CO2-fixing bacteria in a double-cropping rice paddy field, Environ. Microbiol. Rep., 2022, 14, 245–253 CrossRef PubMed.
- R. M. Soo, J. Hemp, D. H. Parks, W. W. Fischer and P. Hugenholtz, On the origins of oxygenic photosynthesis and aerobic respiration in cyanobacteria, Science, 2017, 355, 1436–1440 CrossRef CAS PubMed.
- C. Cano-Díaz, F. T. Maestre, D. J. Eldridge, B. K. Singh, R. D. Bardgett, N. Fierer and M. Delgado-Baquerizo, Contrasting environmental preferences of photosynthetic and non-photosynthetic soil cyanobacteria across the globe, Global Ecol. Biogeogr., 2020, 29, 2025–2038 CrossRef.
- M. E. Larsson, A. R. Bramucci, S. Collins, G. Hallegraeff, T. Kahlke, J.-B. Raina, J. R. Seymour and M. A. Doblin, Mucospheres produced by a mixotrophic protist impact ocean carbon cycling, Nat. Commun., 2022, 13, 1–15 Search PubMed.
- R. Sayre, Microalgae: the potential for carbon capture, Bioscience, 2010, 60, 722–727 CrossRef.
- H. Onyeaka, T. Miri, K. Obileke, A. Hart, C. Anumudu and Z. T. Al-Sharify, Minimizing carbon footprint via microalgae as a biological capture, Carbon Capture Sci. Technol., 2021, 1, 100007 CrossRef CAS.
- C. M. Beal, L. N. Gerber, S. Thongrod, W. Phromkunthong, V. Kiron, J. Granados, I. Archibald, C. H. Greene and M. E. Huntley, Marine microalgae commercial production improves sustainability of global fisheries and aquaculture, Sci. Rep., 2018, 8, 1–8 CAS.
- A. L. Gonçalves, C. M. Rodrigues, J. C. Pires and M. Simões, The effect of increasing CO2 concentrations on its capture, biomass production and wastewater bioremediation by microalgae and cyanobacteria, Algal Res., 2016, 14, 127–136 CrossRef.
- P. Ruiz-Ruiz, T. L. Gómez-Borraz, S. Revah and M. Morales, Methanotroph–microalgae co-culture for greenhouse gas mitigation: effect of initial biomass ratio and methane concentration, Chemosphere, 2020, 259, 127418 CrossRef CAS PubMed.
- G. Venkataraman, Algalization, Phykoa, 1966, 5, 748–749 Search PubMed.
- S. Abinandan, S. Shanthakumar, L. Panneerselvan, K. Venkateswarlu and M. Megharaj, Algalization of acid soils with Desmodesmus sp. MAS1 and Heterochlorella sp. MAS3 enriches bacteria of ecological importance, ACS Agric. Sci. Technol., 2022, 2, 512–520 CrossRef CAS.
-
A. Chatterjee, S. Singh, C. Agrawal, S. Yadav, R. Rai and L. Rai, Role of algae as a biofertilizer, in Algal Green Chemistry, ed. R. Rastogi, D. Madamwar and A. Pandey, Elsevier, Netherlands, 2017, pp. 189–200 Search PubMed.
-
A. Sasson, Micro Biotechnologies: Recent Developments and Prospects for Developing Countries. BIOTEC Publication 1/2542. Place de Fontenoy, Paris. France. United Nations Educational, Scientific and Cultural Organization, https://agris.fao.org/agris-search/search.do?recordID=TH2002002100, 1997, p. 16, accessed 25 June 2022 Search PubMed.
- G. Abdulqader, L. Barsanti and M. R. Tredici, Harvest of Arthrospira platensis from Lake Kossorom (Chad) and its household usage among the Kanembu, J. Appl. Phycol., 2000, 12, 493–498 CrossRef.
-
A. Belay, Biology and industrial production of Arthrospira (Spirulina), in Handbook of Microalgal Culture: Applied Phycology and Biotechnology, ed. A. Richmond and Q. Hu, Willey Blackwell, Chichester, West Sussex, UK, 2nd edn, 2013, pp. 339–358 Search PubMed.
- P. Spolaore, C. Joannis-Cassan and E. Duran, Commercial applications of microalgae, J. Biosci. Bioeng., 2006, 101, 87–96 CrossRef CAS PubMed.
- M. A. Borowitzka, Commercial production of microalgae: ponds, tanks, tubes and fermenters, J. Biotechnol., 1999, 70, 313–321 CrossRef CAS.
- M. A. Borowitzka and A. Vonshak, Scaling up microalgal cultures to commercial scale, Eur. J. Phycol., 2017, 52, 407–418 CrossRef CAS.
- L. Schütz, A. Gattinger, M. Meier, A. Müller, T. Boller, P. Mäder and N. Mathimaran, Improving crop yield and nutrient use efficiency via biofertilization – a global meta-analysis, Front. Plant Sci., 2018, 8, 2204 CrossRef PubMed.
- B. Nowruzi, N. Bouaïcha, J. S. Metcalf, S. J. Porzani and O. Konur, Plant–cyanobacteria interactions: beneficial and harmful effects of cyanobacterial bioactive compounds on soil-plant systems and subsequent risk to animal and human health, Phytochemistry, 2021, 192, 112959 CrossRef PubMed.
- D. H. Parks, M. Chuvochina, C. Rinke, A. J. Mussig, P.-A. Chaumeil and P. Hugenholtz, GTDB: an ongoing census of bacterial and archaeal diversity through a phylogenetically consistent, rank normalized and complete genome-based taxonomy, Nucleic Acids Res., 2022, 50, D785–D794 CrossRef CAS PubMed.
- W. B. Whitman, A. Oren, M. Chuvochina, M. S. da Costa, G. M. Garrity, F. A. Rainey, R. Rossello-Mora, B. Schink, I. Sutcliffe and M. E. Trujillo, Proposal of the suffix–ota to denote phyla. Addendum to ‘Proposal to include the rank of phylum in the International Code of Nomenclature of Prokaryotes’, Int. J. Syst. Evol. Microbiol., 2018, 68, 967–969 CrossRef PubMed.
- R. M. Soo, J. Hemp and P. Hugenholtz, Evolution of photosynthesis and aerobic respiration in the cyanobacteria, Free Radical Biol. Med., 2019, 140, 200–205 CrossRef CAS PubMed.
- P. Alexander and A. Svetlana, New life for old discovery: amazing story about how bacterial predation on Chlorella resolved a paradox of dark cyanobacteria and gave the key to early history of oxygenic photosynthesis and aerobic respiration, Protistology, 2021, 15, 107–126 Search PubMed.
- M. W. Fawley and K. P. Fawley, Identification of eukaryotic microalgal strains, J. Appl. Phycol., 2020, 32, 2699–2709 CrossRef PubMed.
- A. Altman and A. Mesoudi, Understanding agriculture within the frameworks of cumulative cultural evolution, gene-culture co-evolution, and cultural niche construction, Hum. Ecol., 2019, 47, 483–497 CrossRef.
- P. H. Thrall, J. G. Oakeshott, G. Fitt, S. Southerton, J. J. Burdon, A. Sheppard, R. J. Russell, M. Zalucki, M. Heino and R. Ford Denison, Evolution in agriculture: the application of evolutionary approaches to the management of biotic interactions in agro-ecosystems, Evol. Appl., 2011, 4, 200–215 CrossRef PubMed.
-
J.-M. Gobat, M. Aragno and W. Matthey, The Living Soil: Fundamentals of Soil Science and Soil Biology, Science Publishers, 2004 Search PubMed.
- A. Gunina and Y. Kuzyakov, From energy to (soil organic) matter, Global Change Biol., 2022, 28, 2169–2182 CrossRef CAS PubMed.
- N. N. Oehlmann and J. G. Rebelein, The conversion of carbon monoxide and carbon dioxide by nitrogenases, Chembiochem, 2022, 23, e202100453 CAS.
|
This journal is © The Royal Society of Chemistry 2023 |