DOI:
10.1039/D3TB00192J
(Review Article)
J. Mater. Chem. B, 2023,
11, 4153-4170
Ferritin nanocages: a versatile platform for nanozyme design
Received
31st January 2023
, Accepted 11th April 2023
First published on 13th April 2023
Abstract
Nanozymes are a class of nanomaterials with enzyme-like activities and have attracted increasing attention due to their potential applications in biomedicine. However, nanozyme design incorporating the desired properties remains challenging. Natural or genetically engineered protein scaffolds, such as ferritin nanocages, have emerged as a promising platform for nanozyme design due to their unique protein structure, natural biomineralization capacity, self-assembly properties, and high biocompatibility. In this review, we highlight the intrinsic properties of ferritin nanocages, especially for nanozyme design. We also discuss the advantages of genetically engineered ferritin in the versatile design of nanozymes over natural ferritin. Additionally, we summarize the bioapplications of ferritin-based nanozymes based on their enzyme-mimicking activities. In this perspective, we mainly provide potential insights into the utilization of ferritin nanocages for nanozyme design.
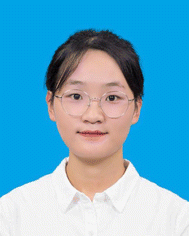
Chunyu Wang
| Chunyu Wang received her bachelor's degree in 2017 from Southwest University, China. She is now pursuing her master's degree under the supervision of Professor Xinglu Huang. Her main research interests are to construct multifunctional nanoplatforms based on ferritin nanocages and investigate their functions in neurodegenerative diseases. |
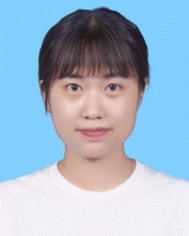
Qiqi Liu
| Qiqi Liu received her PhD degree in 2022 from Nankai University, China. She is currently undertaking her postdoctoral research under the guidance of Professor Xinglu Huang. Her scientific interests are focused on the biological barrier penetration and biological effects of functional nanomaterials based on protein engineering principles. |
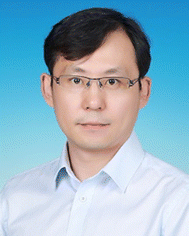
Xinglu Huang
| Xinglu Huang obtained his PhD degree in 2010 from the Technical Institute of Physics and Chemistry at the Chinese Academy of Science. He then worked as a Postdoctoral Fellow at the National Institute of Health (NIH) and Johns Hopkins University (JHU). He is currently a Professor at Nankai University, China. His research focuses on the development of genetically engineered nanomedicines and AI-assisted nanotechnologies for exploring the interactions between nanomaterials and biological barriers, with aims to solve the problems facing oncology and regenerative medicine. |
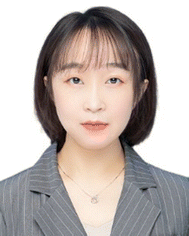
Jie Zhuang
| Jie Zhuang obtained her PhD in 2010 from the Institute of Biophysics at the Chinese Academy of Sciences. After two years of postdoctoral training, she was promoted to Research Fellow at the National Institute of Health (NIH). She is currently an Associate Professor at the School of Medicine, Nankai University, China. Her research focuses on the development of protein-based nanozymes and their applications in the treatment of various diseases. |
1. Introduction
The catalytic functions of natural enzymes are critical in living organisms. However, natural enzymes have poor environmental stability and are easily denatured at extreme pH and temperature, which makes them difficult to prepare and preserve. To solve this problem, researchers have developed artificial enzymes that can mimic natural enzymes more effectively. Rapid development of nanotechnology and emergence of numerous nanomaterials provide new insight for artificial enzymes. Nanozymes are nanomaterials with intrinsic enzyme-like activities that can catalyze substrates and chemical reactions like natural enzymes, with similar reaction dynamics and catalytic mechanisms.1 Since the discovery of peroxidase-like activity of Fe3O4 nanoparticles (NPs) in 2007,2 nanozymes with various enzyme-like activities such as peroxidase (POD), oxidase (OXD), catalase (CAT) and superoxide dismutase (SOD) have been reported and widely used in biosensing,3–5 antibacterial,6 antioxidant,7,8 and theranostics applications.9–11 Despite the remarkable progress of nanozymes, their applications still face many issues. For example, the catalytic activities of nanozymes are size-dependent. Nanozymes with a smaller size typically show higher enzyme-like activities due to their larger surface area.2 However, synthesizing size-controlled nanozymes is still challenging. Additionally, some specific targeting capabilities of nanozymes are also necessary for disease theranostics, but this is not practical because of the complex steps involved. Furthermore, the protein corona that forms on the nanozyme surface affects their in vivo fates, which can alter their distribution and delivery efficiency. In recent years, some biomacromolecules such as heat shock protein (HSP),12 encapsulating proteins,13 virus-like particles (VLPs), phage P2214 and lumazine synthase15 have been used as pre-organized scaffolds or templates for nanomaterial construction. However, their biomedical applications are hampered due to their limited controllability and functionality. To tackle these problems, designable ferritin nanocages have been introduced as a promising display platform of artificial enzymes. As a natural iron storage protein, the biomineralization ability of ferritin enables the synthesis of metal clusters and metal oxides with restricted size and ferritin efficiently reduces the protein corona formation. Human H-ferritin (FTn) can also naturally target tumors by binding with its receptor, transferrin receptor 1 (TfR1), which is highly expressed among various types of tumors. In addition, ferritin can be customized on demand via genetic or chemical techniques to give more functions to nanozymes. These properties allow broad applications of ferritin based nanozymes in tumor diagnosis and treatment. Collectively, ferritin nanocages combine the designable advantages of protein scaffolds with the catalytic activities of nanozymes, thus creating more possibilities for the design of multifunctional nanozymes.
A well-summarized review of ferritin nanozymes was previously reported by Jiang et al.16 In this review, we will systemically provide in-depth insights and detailed mechanisms of ferritin nanocages. Based on the properties, we will highlight the benefits of genetically engineered ferritin nanocages and discuss their potential in nanozyme design. We will also provide an update on the relevant design and biomedical applications of ferritin nanozymes from the past three years. Through this overview (Fig. 1), we hope to clarify the potential of genetically engineered ferritin in serving as a nanozyme generator and provide insights for better nanozyme design.
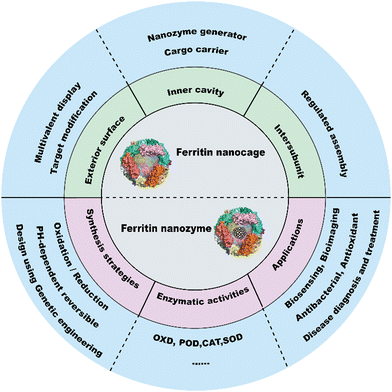 |
| Fig. 1 Three designable interfaces of ferritin nanocages and ferritin as a nanozyme generator. | |
2. Ferritin nanocages as a multifunctional nanoplatform
2.1 Structure and function of ferritin
Ferritin was first discovered in horse spleen in 1937.17 As a natural iron storage protein, ferritin is almost ubiquitous in biological terms. To date, various species of ferritin have been identified, including bacteria, plants, animals, microorganisms, etc. Typically, ferritins are classified into three subfamilies, namely, classical ferritin (Ferritin), bacterial ferritin (Bfr), and DNA-binding proteins (Dps) from starved cells. Ferritin and Bfr proteins are classified as maxi-ferritin, consisting of 24 subunits that self-assemble to form a hollow cage-like structure with an inner and outer diameter of approximately 8 nm and 12 nm, respectively (Fig. 2a). Dps are classified as micro-ferritin with only 12 subunits, which are smaller than ferritin and Bfr.
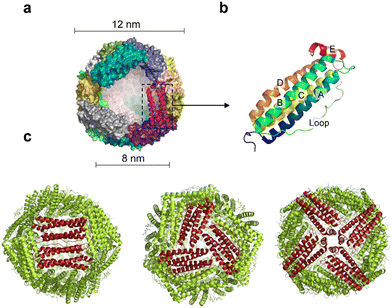 |
| Fig. 2 Structure of ferritin nanocages. (a) A spherical cage-like structure composed of an iron core inside and a protein shell, with inner and outer diameters of 8 nm and 12 nm, respectively. (b) Four-helix bundle structure of a single subunit of ferritin. A, B, C, D: α-helix; E: short helix. (c) The tertiary structure of the human ferritin heavy chain presents 4.3.2 symmetry. | |
Naturally, ferritin consists of a protein shell and an inner cavity. The inner cavity has a non-uniform ferric core composed of numerous iron hydroxide and phosphate molecules. Natural ferritin of different origins differs significantly in mineral core composition and structure. Bacterial and plant ferritin show higher phosphate ratios than mammalian ferritin, and the variation in phosphate causes different rates of iron mineralization for various types of ferritin.18 The internal cavity of maxi-ferritin could accumulate up to 4500 iron atoms without affecting the normal function, which is crucial for maintaining iron homeostasis.19,20 The properties of maxi-ferritin make it an ideal platform for nanomedicine, which will be mainly discussed in this review. Dps exhibit a lower iron storage capacity and a unique ferrous oxidase center.21 It could also utilize H2O2 to rapidly oxidize Fe2+ to Fe3+ and store it in its inner cavity. Dps is a natural nanomaterial that can inhibit Fenton reactions and ˙OH formation. Based on this, Min et al. introduced an appropriate amount of His into the N-terminal of the Dps to give it the function of cell membrane penetration. The modified Dps entered the cells via reticulin-mediated endocytosis and showed antioxidant effects on mouse dermatitis.22
The amino acid sequences of ferritin from different species vary widely, but their spatial structure is highly conserved.23,24 Specifically, each subunit of maxi-ferritin has four long α-helixes (A, B, C, D) from the N terminus, arranged in an antiparallel manner to form a helix bundle, and a short helix (E) forms the C terminus.24 There are short non-helical regions at both N and C terminals as well as at the bends of AB and DE, while a long loop L is between B and C helixes (Fig. 2b). The 24 subunits form 12 pairs that are parallel and opposite to each other, creating a protein nanocage with 432 points of symmetry. There are 12 duplex, 8 triplex and 6 quadruplex axes/channels between ferritin subunits (Fig. 2c). Regarding the roles of these channels, ferritin with various electrostatic properties exhibit different functions. The C3 channel of mammalian ferritin is hydrophilic and contains six acidic amino acids, which allows metal ions and H2O molecules to enter the ferritin cavity. However, based on the electrostatic properties of the bacterial ferritin (BfrA) of Mycobacterium tuberculosis (Mtb), it was demonstrated that the C4 channel and B-pore may be potential pathways for Fe2+ entry and play a role in Fe2+ uptake/oxidation in Mtb BfrA.25 These channels of ferritin nanocages are critical for drug loading and nanozyme synthesis.
Mammalian ferritin, such as human ferritin, is composed of a H-subunit (21 kDa) and L-subunit (19 kDa). The H-subunit contains a ferroxidase site and the L-subunit has a nucleation site, both of which are essential for iron storage and distribution.26 The ferroxidase site of the human H-subunit is mainly formed by three amino acid residues (Glu 27, Glu 62, His 65), which is important for maintaining ferroxidase activity.27 Adjacent to the ferroxidase site are Glu residues on the inner surface of ferritin that facilitate iron core formation.28 Mammalian ferritin occurs in different molecular forms called iso-ferritin with varying ratios of H and L-subunits. The H/L ratio depends on the proliferation, differentiation, and developmental stages of animal cells, which is consistent with the functions of ferritin. For example, ferritin in the heart and brain has a high H/L ratio with high ferroxidase activity for antioxidant function, which can efficiently oxidize and sequester iron. In contrast, ferritin in the liver and spleen has a low H/L ratio with high iron storage capacity.20 Learning about the different H/L ratios of ferritin is important for understanding its structure and functions.
Besides the unique structure, ferritin also exhibits several natural functions. For example, ferritin nanocages could sequester excess cellular iron as hydrated iron oxide in their inner lumen, thus preventing oxidative damage caused by iron overload. It can also release iron to cells for synthesizing iron-containing proteins. The ferroxidase activity of ferritin is able to block the production of free radicals through the Fenton reaction. Furthermore, ferritin from different species exhibits distinct receptor binding properties, which are not fully understood. For instance, FTn could target tumors by recognizing the highly expressed TfR1 on the surface of tumor cells,29,30 which makes it an ideal platform for active tumor targeting. However, the mouse H-ferritin is endocytosed by the TIM-2 (T-cell immunoglobulin and mucin structural domain protein 2) receptor.31 Regarding mouse L-ferritin, scavenger receptor class A member 5 (Scara5) has been identified as its receptor in the mouse kidney.32 But human L-ferritin does not show a similar binding capacity to human Scara5. These natural receptor binding properties of various ferritins provide a reference for ferritin selection and practical applications.
2.2 Properties of natural ferritin
2.2.1 Biomineralization.
In contrast to conventionally synthesized nanomaterials in vitro, ferritin is a natural biological nanomaterial generated by living organisms. Ferritin has the ability for iron biomineralization due to the high ferroxidase-like activity of its H-subunit, which also enables the encapsulation of metal NPs. For eukaryotic ferritin, the C3 pore plays an important role in Fe2+ sequestration.33 The mechanisms of iron incorporation into ferritin in vitro can be described by four main steps: iron entry, iron oxidation, iron oxide nucleation, and iron oxide particle growth. This multi-step mineralization process is mediated by the protein nanocage. Firstly, iron enters the cage through the C3 channel formed at the interface between subunits. Then the oxidation of Fe2+ is carried out through an enzymatic reaction at the ferroxidase center, resulting in the formation of Fe3+. Next, after a series of complex hydrolytic reactions, the inner cavity of ferritin nanocages facilitates the nucleation of iron oxide particles with a confined size. During the Fe2+ sequestration process, the carboxylate side chains of Asp and Glu residues along the C3 pore (D127 and E130) are essential for iron uptake, while E136 and E57 (also known as translocation sites) play an important role in the transfer of Fe2+ from the C3 pore to the ferroxidase center.34–37
Besides selective Fe2+ sequestration, other metal ions could also be incorporated into ferritin nanocages under in vitro conditions.34 For example, the X-ray crystal structure indicated that divalent metal ions, such as Cu2+, Co2+, Zn2+, and Mn2+, could bind to the C3 pore or ferroxidase center, thus competing with Fe2+ and reducing ferroxidase activity.37–40 It was also revealed that His residues near the C4 pore could bind with Zn2+, Cu2+, Co2+, Mn2+, and Mg2+ to form octahedral geometry during Fe2+ sequestration in FTn and bullfrog M H-ferritin.41 In horse spleen L-ferritin, Pd2+ could interact with H114, C126 and E130 and enter the ferritin nanocage through the C3 pore.42 Moreover, the synthesis of Au NPs in ferritin has also been investigated in FTn, and Au3+ could interact with H65 and bind four His residues to form a square planar structure at the C4 pore.43 Thus, different metal ions show different sequestration forms with various ferritin, which provides a reference for understanding and selecting ferritin nanoreactors for nanozyme synthesis. In fact, natural ferritin nanocages such as horse spleen ferritin (HosFt), human ferritin (HuFt), Archaebacterial ferritin (AfFt), and Pyrococcus furiosus ferritin (PfFn) have been widely used as a template for nanozyme synthesis. Under specific conditions in vitro, some metal NPs have been successfully assembled into ferritin to exert multiple functions, such as FeS, CdS, Mn3O4, and Fe3O4, etc.44,45
For the rational design and synthesis of ferritin nanozymes, it is important to understand how metal NPs grow in the ferritin cavity. The incorporation mechanism of NPs in ferritin is dependent on metal types and ferritin species, and it is not fully understood yet. Recently, several studies have shed light on this process. For example, the growth details of cobalt oxide NPs in ferritin nanocages were investigated by transmission electron microscopy (TEM) and the initial nucleation of small metals at the inner surface of ferritin was discovered.46 The structural details of Pd ion accumulation in a ferritin nanocage were also revealed, which indicated that many metal ions could accumulate in the C3 channels of ferritin.47,48 Therefore, the synthesis of metal NPs in ferritin may involve two steps: first, metal ions are pre-organized in the narrow channels of ferritin; second, they are converted into single sub-nanoclusters by oxidation or reduction reactions.49,50 Moreover, the intermediate structures with flexible amino acid residues could be stabilized due to the unique coordination environment of the ferritin cavity,51 thus resulting in the synthesis of metal NPs in a controlled manner.
2.2.2 Self-assembly of ferritin.
Natural ferritin nanocages are self-assembled by 24 monomers to form a highly ordered structure, which allows the self-assembly forms to be modulated on demand. Specifically, various heterogeneous forms of ferritin nanocages could be obtained via modification of their subunits, which have new structures and functions compared to natural ferritin. For example, by altering the key subunit interface, Zhang et al. made progress in regulating the oligomerization state and structure of recombinant FTn. They inserted the peptide (∇139LNEQVKA) into the D-helix through a genetic strategy, which resulted in the transformation of the natural 24-polymeric ferritin nanocage into a lenticular 16-polymeric unnatural analogue.52 Moreover, they constructed a 48-oligomeric ferritin nanocage by selectively deleting the amino acid residues at the key subunit interface (Δ139NEQVKA).53 These novel designed ferritin nanocages help us better understand the self-assembly mechanisms of ferritin.
Natural ferritin maintains a stable cage-like structure over a wide pH range from 3.4 to 10.0, but when the pH of the solution is drastically and repeatedly changed, the structure undergoes the conversion between disassembly and reassembly. Under extremely acidic or alkaline conditions, the subunits depolymerize, and when the pH returns to neutral, the subunits reassemble into the nanocage.54 However, the pH-induced disassembly and reassembly process is not fully reversible. When ferritin nanocages depolymerize at pH = 1.96, they could not recover to the original hollow spherical structure even after returning to neutral pH (Fig. 3).55 Similarly, ferritin can disassemble under high concentrations of guanidine hydrochloride (6 M) and urea (8 M), and return to cage-like structures when these denaturing agents are withdrawn. Appropriate understanding of the in vitro self-assembly characteristics of natural ferritin is crucial for its applications in cargo loading, nanozyme synthesis and other biomedical fields. Several studies have reported the mechanisms of this process. Specifically, the self-assembly of ferritin is affected by multiple factors, such as ferritin concentration, surface modifications, pH and ionic strength. For example, high concentrations of ferritin may promote unnecessary aggregation at lower pH and its surface modifications may affect subunit interactions.56 Abhinav et al. used a laser scattering technique to study the self-assembly process of bullfrog M ferritin (BfMF, a structural and functional analogue of FTn).57 It was found that the self-assembly kinetics of BfMF exhibited a unique manner. When the pH was increased from 1.5 to 7.0, the kinetic profile showed three phases: a rapid increase, a slow-down and a plateau. How different physical parameters affected the self-assembly process of BfMF was also investigated. For each individual factor, the rate of ferritin assembly increased with the concentration of protein, which was probably due to more opportunities for interactions caused by an increasing number of subunits. The rate of assembly decreased with the final pH of the solution. This was because the net negative charge strength of the ferritin subunits increased with pH, resulting in electrostatic repulsion between them, which explained the slow-down phase in the assembly kinetic profile. It was also found that the rate of assembly increased with the ionic strength (from 0.55 to 0.75 M), which was possibly due to the increased medium ionic strength that could shield the electrostatic repulsion between ferritin subunits. Also, as a multimeric protein cage, subunit–subunit interactions may be crucial for assembly.58,59 A set of important His residues was shown to be involved in BfMF self-assembly, possibly by affecting the subunit–subunit interactions at the pore/interface due to its pH-dependent protonated/deprotonated state. It was reported that His also played an important role in regulating the reversible self-assembly of FTn.60 Thus, understanding the self-assembly mechanisms of ferritin can help optimize the relevant parameters for incorporating nanomaterials into ferritin nanocages and provide insights into nanozyme synthesis.
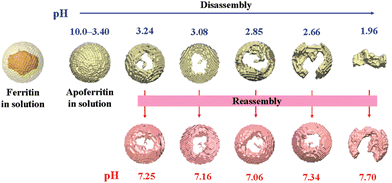 |
| Fig. 3 The pH-induced apoferritin disassembly and reassembly processes.55 Reprinted with permission from ref. 55, © American Chemical Society 2011. | |
2.3 Genetically engineered ferritin nanocages
The biomineralization and self-assembly properties of natural ferritin render it a powerful tool for nanomedicine design. However, natural ferritin nanocages need to be modified for more flexible and diverse functional applications. Recently, genetically recombinant ferritin nanocages have been developed to facilitate the diversification of ferritin design. In general, there are three functional interfaces of ferritin nanocages including the inner cavity, the outer surface, and the interface between subunits.61 In particular, the 8 nm cavity provides a location for drug/biomacromolecules loading and nanozyme synthesis. For example, Zhang et al. have modified the electrostatics of ferritin to improve the loading efficiency of nucleic acid drugs. They replaced the negatively charged amino acids (Glu and Asp) on the internal surface of H-ferritin with positively charged ones (Lys and Arg) through genetic engineering.62 Subsequently, these ferritin mutants with various electrostatics were screened and applied for efficient nucleic acid drug delivery.
Ferritin nanocage is an excellent display and delivery platform due to its easily modified outer surface. The N-terminus of ferritin is exposed on the outer surface of the nanocage, and various targeting peptides, antigens, and nanobody could be displayed onto ferritin via genetic or chemical methods. For example, the GRP78 targeting peptide SP94 was displayed on the outer surface of PfFn (named HccFn) by genetic engineering, and then Adriamycin (Dox) was encapsulated into the cavity of HccFn.63 This nanomedicine (i.e., HccFn–Dox) can be applied for targeted therapy of hepatocellular carcinoma. In detail, HccFn–Dox was bound to the glucose receptor binding protein (i.e., GRP78) on the surface of hepatocellular carcinoma cells and was endocytosed. Then it localized in the lysosome, where the ferritin cleaved and released Dox in an acidic environment, thus leading to cell death.
Genetically recombinant FTn has attracted more attention in nanomedicine research in recent years. FTn preserves the optimal physicochemical properties and biocompatibility of natural ferritin, and it can specifically target tumor cells without further modification. These properties make FTn an ideal platform for cancer theranostics. For example, Liang et al. encapsulated large amounts of Dox into FTn to achieve effective tumor killing.64 Fan et al. used FTn to synthesize Fe3O4 nanozymes for tumor targeting diagnosis.65 More recently, our group synthesized multiple nanozymes in FTn for cancer treatment.66–68
2.4 Cargo carrier
The hollow spherical core of ferritin allows various cargo loading. To date, a variety of cargo molecules have been encapsulated into the cavities of ferritin nanocages, including drugs, imaging/contrast agents, nucleic acids, and metal NPs, and their applications cover the fields of bioassay analysis and various disease theranostics.69Table 1 summarizes the examples of drug or imaging/contrast molecules encapsulated in ferritin and their applications. Currently, the most common method for cargo loading is pH or urea dependent reassembly and disassembly. However, this method has some drawbacks. For example, extreme pH may damage the structure of protein cages and cargo molecules, thus reducing the encapsulation efficiency. It is desirable to explore more efficient ways for cargo loading in ferritin. Recently, Lin et al. reported that FTn had a natural hydrophilic drug entry channel and Dox could be encapsulated into its cavity by simple incubation at 60 °C for 4 h.70 The encapsulation efficiency was up to 100 Dox molecules per protein, and the protein recovery reached more than 90%. Moreover, the FTn–Dox exhibited high stability, good safety and an excellent anti-tumor effect. In addition to Dox, Cisplatin (CDDP) and Oxaliplatin were also successfully loaded into the FTn via the channel. This strategy provided insights into the cargo loading in ferritin via a more convenient approach. Besides drug molecules, imaging signal molecules can also be incorporated into ferritin to construct a ferritin-based multifunctional nanoplatform. For example, Huang et al. loaded the NIR dye IR820 into ferritin to develop a novel therapeutic diagnostic probe for fluorescence imaging-guided photothermal therapy with high imaging contrast and enhanced photothermal conversion efficiency.71
Table 1 Examples of ferritin nanocages as cargo carriers
|
Ferritin type |
Molecules |
Strategies |
Applications |
Ref. |
Natural ferritin |
HosFt |
Desferrioxamine B |
pH-dependent reversible assembly |
None. |
72
|
HosFt |
Cisplatin (CDDP) and carboplatin (CBDCA) |
pH-dependent reversible assembly |
MTT Test for drugs |
73
|
Genetically recombinant FTn |
Curcumin |
pH-dependent reversible assembly |
Enhance the therapeutic effect of triple negative breast cancer |
74
|
Genetically recombinant FTn |
Dox |
Temperature control of drug channels |
Prove the natural drug channel of ferritin heavy chain subunit |
64,70
|
Genetically recombinant FTn |
CDDP |
pH-dependent reversible assembly |
Tumor targeted drug delivery and treatment |
73
|
Genetically recombinant FTn |
Artesunate |
Urea disassembly |
Cooperate with internal ferric oxide to activate the anti-tumor effect |
75
|
Genetically recombinant FTn |
MnPc |
pH-dependent reversible assembly |
Facilitate PDT in cancer |
76
|
Genetically engineered ferritin |
Genetically recombinant FTn |
Metformin |
pH-dependent reversible assembly |
Targeted therapy for osteoarthritis |
77
|
Genetically recombinant FTn |
Genz-644282 |
pH-dependent reversible assembly |
Colorectal cancer targeted therapy |
78
|
Genetically recombinant FTn (point mutation) |
TLR activating nucleic acid ligands |
Construction of ferritin mutants with various electrostatics |
Improved efficiency of TLR nucleic acid ligands delivery |
79
|
Genetically recombinant FTn |
Paclitaxel (PTX) |
Urea (20 mM) could expand the 4-fold channel size |
Exhibited higher in vivo tumor therapeutic efficacy |
80 and 81
|
RGD4C-modified FTn |
64Cu, Cy5.5 |
pH-dependent reversible assembly |
Multimodal imaging |
82
|
RGD4C-modified FTn |
ZnF 16 Pc |
Passive diffusion |
Photosensitizer delivery, imaging, PDT |
83
|
2.5 Multivalent display platform
Ferritin nanocages are ideal carriers for antigen and peptide display due to their highly uniform size, symmetry, self-assembled repetitive structure and easily modified outer surface. Based on this, Zhang et al. designed a ferritin-based detection probe to improve the sensitivity of autoimmune disease detection.84 Taking advantage of the multimeric display ability of ferritin, the antigen M3 and α-fodrin was arranged on the surface of ferritin, which greatly enhanced their ability to capture autoantibodies. In a similar manner, they developed a multimeric secondary antibody by displaying a human IgG binding peptide on the surface of ferritin and labeling it with Horseradish Peroxidase (HRP). This ferritin nanocage-based probe exhibited a higher affinity for human IgG, and its sensitivity was 10 times higher than conventional enzyme-labeled secondary antibodies. Combining the ferritin-based multimeric antigen with the secondary antibody probe, the sensitivity of human IgG detection was enhanced by 100–1000 fold. This ferritin-based capture system was an effective detection platform and created a new method for the diagnosis of autoimmune diseases. It also opened up more opportunities for ferritin as a multivalent display nanoplatform.
Ferritin nanocages also hold potential for vaccine design. In contrast to the weak binding of monovalent antigen, multivalent antigens displayed on a ferritin scaffold improve the recruitment of antigen presenting cells (APCs). The repeated arrangement of antigens also enables the effective activation of B cells. Ferritin vaccines have been a hot topic in new vaccine research due to the immunological and physicochemical properties, as well as the advantages of mass production through an Escherichia coli or eukaryotic system. Recently, Ma et al. developed a novel nanovaccine against SARS-Cov-2 by displaying the receptor binding domain (RBD) and heptapeptide repeat (HR) domain of spike protein onto ferritin via the Spy-Tag/Spy-Catcher system.85 Compared to monomeric vaccine, this ferritin vaccine induced more potent neutralizing antibodies and cellular immune responses, which prompted novel vaccine design. Table 2 lists some examples of peptides or antigens displayed on the surface of ferritin.
Table 2 Examples of ferritin nanocages for antigen/peptide display
|
Ferritin type |
Antigen/peptide |
Display strategies |
Applications |
Ref. |
Natural ferritin |
PfFn |
M3, α-fodrin Fc binding peptide |
N-Terminal fusion |
Improve the sensitivity of ELISA for the diagnosis of autoimmune disease |
84
|
PfFn |
SP94 |
N-Terminal fusion |
Targeted therapy for hepatocellular carcinoma in combination with Dox |
63 and 86
|
PfFn |
FcBP |
D loop and E helix |
Ability to bind the Fc region of antibodies |
87
|
PfFn |
PreS1 |
N terminal |
Hepatitis B virus vaccine |
88
|
Helicobacter pylori ferritin |
GP140 |
N terminal |
HIV-1 virus vaccine |
89
|
Helicobacter pylori ferritin |
S protein |
N Terminal |
COVID-19 vaccine |
85, 90 and 91 |
Genetically recombinant FTn |
EGF |
N-Terminal fusion |
Target breast cancer cells with high EGFR expression |
92
|
Genetically recombinant FTn |
PAS and RGDK |
N-Terminal fusion |
PAS prolongs blood circulation time and RGDK increases tumor targeting |
93
|
Genetically recombinant FTn |
BCP1 |
N-Terminal fusion |
Enhance tumor therapeutic effect |
94
|
Genetically recombinant FTn |
COL2 |
N-Terminal fusion |
Targeted treatment of osteoarthritis with internal metformin |
77
|
Genetically engineered ferritin |
Genetically recombinant FTn |
TNF Trimer |
N-Terminal fusion |
Mediate tumor cell apoptosis |
95
|
Genetically recombinant FTn |
RGERPPR |
C terminal |
Combined with antitumor drug PTX, enhance therapeutic efficacy |
80 and 96
|
Human light chain ferritin |
AP1 and RGD |
157, N-terminal fusion |
Super affinity and targeting to tumor cells |
97 and 98
|
Rat heavy chain ferritin |
EV71 VP1 peptide |
146/N terminal/C terminal |
EV71 virus vaccine |
99
|
Helicobacter pylori ferritin (N19Q) |
HA trimer protein |
N terminal |
Influenza virus vaccine |
100–102
|
3. Ferritin as a generator for nanozymes
3.1 Natural ferritin and genetically engineered ferritin for nanozyme synthesis
The biomineralization capacity and the size-constrained inner cavity of natural ferritin make it an ideal template for nanozyme synthesis. In 1991, Meldrum et al. used natural HosFt as a template to synthesize magnetic iron NPs in its cavity,44 which started a new field of NP biosynthesis. Since then, multiple metal NPs such as Ag,103 Au,104 Pd,105 Cu,106 Co,107 and Ni108 have been incorporated into the cavity of HosFt. In addition, bacterial ferritin with high tolerance to harsh environments has also been discovered. For example, PfFn is an extremely thermally stable protein nanocage in which Pd–Ag NPs109 and Co–Pt NPs110 can be incorporated into its cavity. AfFt is another commonly used bacterial ferritin with a negatively charged inner surface and Au NPs can be loaded inside it.111 Compared to other methods, the biomimetic synthesis of these ferritin nanozymes are characterized by the following features: (i) homogeneous particle size. The 8 nm cavity of ferritin provides a size-constrained template for nanozyme synthesis. (ii) Good biocompatibility and monodispersity. Ferritin is endogenous to an organism with low toxicity, immunogenicity and safe metabolites. (iii) Strong stability. Ferritin usually maintains structural stability over a wide range of temperature and pH. For some unstable nanozymes such as metallic NP materials, the overall stability can be improved by encapsulation of a ferritin scaffold. (iv) Simple synthesis. The NPs are easily synthesized inside ferritin nanocages at near room temperature and mild conditions. (v) Prolonged half-life in vivo. Rationally designed ferritin can exhibit prolonged blood circulation. For example, using a urea disassembly–reassembly approach, we prepared ferritin heterodimers in vitro,112 which consist of H-chains and PEGylated H-chains of ferritin. Specifically, the H-chains provided TfR1 targeting to tumor cells and the PEGylated H-chains prolonged the circulation time in vivo. Furthermore, we constructed modularly assembled multimeric FTn-Ner nanostructures through PEG modification with various sizes, different morphologies and increasing binding valency to TfR1. These changes also led to differences in blood clearance of various ferritin oligomeric NPs. FTn-4er exhibited the best blood circulation half-life in mice, which facilitated the aggregation of ferritin nanozyme at the target site.66
Among various types of ferritin, it is necessary to choose the appropriate ferritin according to the biomedical applications of nanozymes. For example, for different species of ferritin, HosFt, AfFt and PfFt could better tolerate extreme environments, which might be suitable for in vitro assays and diagnostics. HuFt are ideal nanocarriers for disease treatments owing to their low immune responses induced by the body. Secondly, regarding natural and various modified ferritin, although natural ferritin can be used as simple templates for nanozyme synthesis, their applications are limited when certain targeting functions are required. Genetically and chemically modified ferritin nanocages could give nanozymes specific functions on demand. For example, recombinant FTn could recognize a TfR1 receptor more specifically with an enhanced tumor targeting ability. Moreover, the ferritin nanozyme was endowed with mitochondria-targeting abilities via tri-phenyl-phosphonium (TPP)-based modification.113 Through gene fusion expression, SP94, a targeting peptide that specifically targeted hepatocellular carcinoma tissue, was incorporated into ferritin and the synthesized ferritin nanozyme could be applied for targeting diagnosis of hepatocellular carcinoma samples.86 It has been demonstrated that modification of the ferritin cavity via genetic engineering could improve the efficiency of metal NP synthesis. In detail, site-specific mutation of the inner lumen is beneficial for metal binding and growth, and the introduction of some specific components could facilitate the synthesis of metal NPs. For example, the synthesis of Ag NPs was achieved by doping silver-binding small peptides inside recombinant L-ferritin.114 Likewise, the addition of Cys and His residues inside ferritin increased metal loading by sulfhydryl/imidazole chelation and enabled the synthesis of Au–Ag NPs.43 The designable strengths of ferritin scaffolds combined with the catalytic activities of the nanozymes will fulfill more valuable applications in the future. Table 3 shows examples of ferritin-based nanozymes with various enzyme-like activities and their applications.
Table 3 Examples of ferritin nanocages as nanozyme generators
Enzyme like activity |
|
Ferritin type |
Nanozyme core |
Synthesis means |
Applications |
Ref. |
POD |
Natural ferritin |
Horse spleen apoferritin |
Au |
Reduction |
Glucose detection |
115 and 116
|
Horse spleen apoferritin |
Prussian Blue NPs |
Oxidation |
Glucose detection |
117 and 118
|
PfFn |
Co3O4 |
Oxidation |
Hepatocellular carcinoma diagnosis |
86
|
Genetically recombinant FTn |
Fe3O4 |
Oxidation |
Tumor diagnosis |
119
|
Genetically recombinant FTn |
Fe3O4 |
Oxidation |
Pathological identification of ruptured atherosclerotic plaques |
11
|
Genetically engineered ferritin |
Genetically recombinant FTn |
Fe3O4 |
Oxidation |
Modulate intracellular oxidative stress |
120
|
Genetically recombinant FTn |
Pd |
Reduction |
Biorthogonal catalysis for targeted drug delivery |
121
|
Modular assembly FTn |
Pt |
Reduction |
Prolong blood circulation and tumor accumulation |
66
|
Genetically recombinant FTn |
Hemin |
pH-dependent disassembly-reassembly |
Tumor cell detection |
122
|
CAT |
Genetically engineered ferritin |
Genetically recombinant FTn |
Ru |
Reduction |
Enhanced tumor therapy |
67
|
Genetically recombinant FTn |
MnO2@Ce6 |
Oxidation |
Enhanced effectiveness of tumor PDT |
123
|
OXD |
Natural ferritin |
PfFn |
Pd |
Reduction |
Catalyze the aerobic oxidation of alcohols |
42
|
SOD |
Natural ferritin |
Horse spleen apoferritin |
CeO2 |
pH-dependent disassembly-reassembly |
ROS scavenger |
124
|
Natural ferritin |
Horse spleen apoferritin |
Pt |
Reduction |
ROS scavenger |
125 and 126
|
POD, CAT |
Genetically engineered ferritin |
Genetically recombinant FTn (apo) |
MnO2@Dox |
Oxidation |
TME-responsive tumor therapy |
127
|
|
Genetically recombinant FTn |
Fe3O4 |
Oxidation |
Treatment of cerebral malaria |
128
|
Natural ferritin |
Horse spleen apoferritin |
Pt |
Reduction |
Reduce cellular oxidative stress |
129
|
SOD, CAT |
Genetically engineered ferritin |
Horse spleen apoferritin |
Au–Pt alloy NPs |
Reduction |
Eliminate intracellular ROS |
130
|
TPP-labeled FTn |
MnO2 |
Oxidation |
Mitochondria targeting alleviated oxidative damage |
113
|
SOD, CAT, POD |
Natural ferritin |
Horse spleen apoferritin |
Au–Ag alloy NPs |
Reduction |
Cytoprotecting |
131
|
SOD, CAT, POD, OXD |
Genetically engineered ferritin |
Genetically recombinant FTn |
Nitrogen-doped Porous Carbon Nanospheres (N-PCNSs) |
Chemical modification |
Tumor treatment |
10
|
Natural ferritin |
Apoferritin |
Cu |
pH-dependent disassembly-reassembly |
Trigger cell autophagy dependent apoptosis |
132
|
Others |
Genetically engineered ferritin |
Horse spleen apoferritin |
Au |
Reduction |
Imaging of breast cancer cells |
133
|
Genetically recombinant FTn |
Cu |
Reduction |
Catalyze NO release; biomimetic design for artificial hybrid nanocells |
134
|
Genetically recombinant FTn |
Pd |
Reduction |
Biorthogonal catalysis targeted drug delivery |
121
|
3.2 Strategies for the synthesis of ferritin nanozymes
The biomineralization process of iron in ferritin provides the theoretical basis for the synthesis of metal-based ferritin nanozymes. Due to the unique inner cavity and hydrophilic channels, metal ions could enter the ferritin easily and form a metal oxide core inside. Using ferritin as a protein scaffold, it is possible to synthesize size-homogeneous nanozymes in a controllable manner. At present, the most commonly used method for ferritin nanozyme synthesis is oxidation or reduction of metal ions in the ferritin cavity. To be specific, for the preparation of reductive nanozymes, different metal ions, such as Ir2+, Pt2+, Pd2+, Cu2+, Ru3+, and Au2+ are incubated with ferritin under alkaline conditions (pH = 8.0), and these metal ions enter ferritin and form metal clusters in situ in the presence of the reducing agent NaBH4. For oxidative ferritin nanozymes, such as Fe2+, Mn2+, and Co2+ are incubated with additional NaOH and oxidizing agent H2O2, which facilitate the formation of metal oxide NPs. In addition to in situ synthesis, metal NPs could also be loaded into ferritin nanocages via pH-induced disassembly and reassembly and the specific details have been discussed above. To sum up, these methods of nanozyme synthesis and the modification strategies of ferritin protein shells give rise to a ferritin nanozyme with diverse catalytic activities (POD, OXD, CAT, SOD, etc.) and biological functions.
Most relevant studies have focused on the formation of metal cores in the ferritin cavity. Indeed, structural analysis is also important to understand the coordination mechanisms and details of metal NPs in ferritin. X-ray crystal structure analysis has been used to observe how metal ions interact chemically inside protein crystals at different phases.135,136 For example, Maity et al. used this method to show that Au ions moved toward the center of the C3 channels and formed sub-nanoclusters on ferritin. The amino acid residues that bound to Au ions changed the conformation of these sub-nanoclusters significantly.137 Therefore, besides the metal–core form of ferritin nanozymes, metal moieties may also bind to other sites of ferritin such as C3/C4 channels, residues at key subunits and the outer surface, etc. More specific mechanisms and diverse designs of ferritin nanozymes will be explored in the future.
3.3 Biomedical applications of ferritin nanozymes with diverse catalytic activities
3.3.1 Ferritin nanozymes with POD-like activities.
The POD-like activities of ferritin were first discovered in 1997 in HosFt.138 Naturally, the intrinsic POD-like activities of ferritin could catalyze the decomposition of H2O2 to produce toxic ˙OH, which help regulate redox metabolism in vivo.138–140 In 2011, Tang et al. demonstrated that HosFt could catalyze the oxidation of 3,3′,5′,5-tetramethylbenzidine (TMB), ortho-phenylenediamine (OPD), etc. at 50 °C, in the presence of H2O2. Moreover, even at high temperature (above 80 °C) or an extreme acidic environment (pH 2.0), it showed high POD-like activity, and its thermal stability and pH tolerance were comparable to that of HRP.141 Recently, ferritin nanozymes with POD-like activities have been applied to kill tumor cell, virus and bacteria, etc. In addition, the generated ˙OH can oxidize various dyes for biomolecular detection/sensing.
For disease-targeted catalytic diagnosis.
The ferritin protein shell can interact with TfR1 and provide a natural binding scaffold, while the nanozyme core can provide a catalytic effect. In 2012, Fan et al. synthesized Fe3O4 NPs inside FTn with POD-like activities and tumor targeting abilities (M-FTn), which could catalyze the oxidation of substrate in the presence of H2O2 and result in a color reaction that visualized tumor tissue.119 This study showed the potential of ferritin nanozymes for targeted diagnostic applications without any targeting ligands. Recently, they also found that the POD-like activities of M-FTn based nanozymes could identify and visualize macrophages in plaque samples from patients with a symptomatic carotid artery,11 which expanded the application scopes of ferritin nanozymes.
Interestingly, except for metal NPs, hemin is the active site of POD and also has potential for biomimetic nanozyme design. However, hemin is hydrophobic and tends to aggregate, which makes it hard to control its structure and functions. Various materials such as polymers,142 carbon nanotubes143 and metal organic frameworks144,145 have been used for hemin incorporation. Liu et al. used ferritin to encapsulate hemin and created a POD-like nanozyme (hemin@FTn),122 which could target tumors and tolerate to harsh environments. Hemin@FTn could serve as a colorimetric probe for effective tumor cell detection. Although ferritin nanocages can target tumors by binding to TfR1, more diverse and accurate targeting modifications are urgent for a wider range of bioapplications. For instance, Jiang et al. designed a ferritin nanozyme with high POD-like activity and hepatocellular carcinoma targeting ability (HccFn–Co3O4).86 The targeting peptide SP94 was modified on the outer surface of a ferritin nanocage via genetic fusion and Co3O4 was biomimetically synthesized in the inner cavity. HccFn–Co3O4 could specially target and visualize liver cancer tissues (Fig. 4), and its detection sensitivity was higher than that of the clinical marker α-fetoprotein (AFP). Taken together, the specific targeting ligands integrated into ferritin by genetic methods facilitate more precise and effective disease diagnosis.
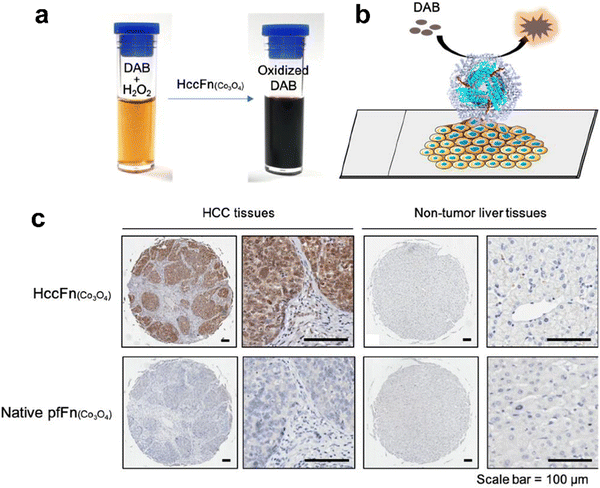 |
| Fig. 4 HccFn (Co3O4) for the diagnosis of clinical HCC tissues.86 (a) HccFn (Co3O4) nanozymes showed POD-like activity and catalyzed the oxidation of DAB to produce a colorimetric reaction. (b) Schematic diagram of the HccFn (Co3O4)-based IHC approach. (c) HccFn (Co3O4)-based IHC staining (top row) and native PfFn (Co3O4)-based IHC staining (bottom row) of HCC tissues and nontumor liver tissues. Reprinted with permission from ref. 86, © American Chemical Society 2019. | |
For cancer-targeted catalytic therapy.
FTn with tumor targeting abilities could make nanozymes more effective for catalytic therapy. Recently, our group introduced a fully synthetic cellular system based on POD-like ferritin nanozyme. Specifically, Fe3O4 NPs were in situ synthesized inside FTn and then they were integrated into membrane-mimicking vesicles, which could simulate the cellular oxidative stress process via a Fenton reaction120 (Fig. 5). Moreover, this system could be delivered to tumor cells in vivo and catalyze H2O2 into toxic ˙OH in the tumor microenvironment (TME), thus triggering tumor cell apoptosis and achieving tumor catalytic therapy.
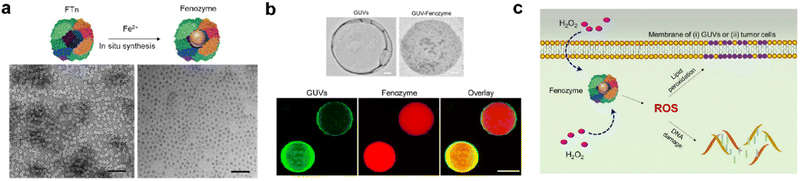 |
| Fig. 5 Fenozyme-powered GUVs mimic oxidative stress in tumor cells.120 (a) Fe3O4 NPs cores were in situ synthesized into FTn protein shells (Fenozyme). TEM image of Fenozyme loaded into GUVs. (b) Schematic illustration of Fenozyme packaged into GUVs using electroformation technology. (c) DNA damage mimicry and lipid peroxidation mimicry induced by GUV Fenozyme in tumor cells. Reprinted with permission from ref. 120, © American Chemical Society 2019. | |
In addition to the tumor targeting capability, our group also used ferritin nanocages to investigate the interactions between biomaterials and organisms on a nanoscale, which was also crucial for the biomimetic synthesis of nanozyme. Based on the concept of synthetic biology, we developed a modular assembly strategy for precise and quantitative customization of highly ordered nanostructures.66 Specifically, we used FTn as a protein building block, after recombination and purification in Escherichia coli, it was reassembled with PEG arms (Fig. 6a). Using the “bottom-up” hierarchical modular assembly idea, a series of nano multimers with ferritin nanocages were created (Fig. 6b). Then the Pt nanoclusters were incorporated into their cavities, which exhibited POD-like activity. As a result, these multivalent assembled ferritin nanozymes (FTn-Ner-Pt) with tunable protein structures and various binding domains were applied in tumor treatment. It was found that they could contribute to prolonged blood circulation time, effective accumulation in tumor cells via natural targeting abilities, and deep tumor issue penetration via a transcytosis mechanism, thus enhancing the anti-tumor efficiency (Fig. 6c and d). By integrating synthetic biology with nanotechnology, we offered a novel assembly strategy of ferritin nanocages, which opened up more possibilities for the de novo design of nanozymes.
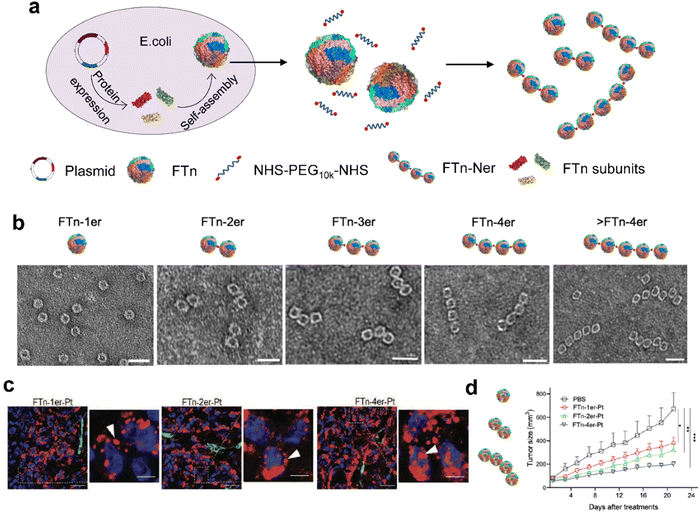 |
| Fig. 6 Modular-assembly ferritin nanozymes.66 (a) Schematic illustration for the preparation of FTn-Ner via a two-step self-assembly/post-assembly approach. The uniform FTn as motifs were obtained by the self-assembly of 24 FTn subunits expressed in E. coli. Subsequently, purified FTn motifs were further assembled to form different FTn-Ner by using two-armed PEG. (b) Representative TEM images of various assembled nanostructures. Scale bar: 20 nm (c) Representative confocal images of intracellular distribution of various Cy5-labeled FTn-Ner-Pt in HT29 tumor tissues 6 h following systemic administration. (d) Tumor growth curves of different groups of HT29 tumor-bearing mice after various indicated treatments. Reprinted with permission from ref. 66, © Wiley-VCH GmbH 2021. | |
3.3.2 Ferritin nanozymes with antioxidant enzyme-like activities.
Ferritin nanozymes with SOD-like activities.
Ferritin nanozymes with SOD-like activity can catalyze O2− into O2 and H2O2, which can serve as a ROS scavenger and antioxidant therapy. In 2012, nanoceria particles with a size of 4.5 nm were successfully encapsulated into the apoferritin through pH-induced disassembly and reassembly.124 As a protein shell, the ferritin nanocage not only reduced the cytotoxicity of nano-CeO2, but also modulated the electron localization at its surface, thereby enhancing the SOD-like activities of the nanozyme.
Ferritin nanozymes with CAT-like activities.
Generally, the hypoxic microenvironment of malignant solid tumors hinders effective treatment, such as chemotherapy, radiation therapy, photodynamic therapy and acoustic dynamic therapy. Ferritin nanozymes with CAT-like activities could specifically target tumor tissue and catalyze the conversion of H2O2 into O2 in a TME, which can act as a H2O2 scavenger for reducing oxidative stress and O2 supplier for alleviating tumor hypoxia. Capitalizing on this, we synthesized MnO2 NPs inside FTn to prepare a hypoxia-tropic nanozyme with CAT-like activity as an O2 generator (OGzyme). Aggregation-induced emission (AIE) was also used as a photosensitizer for photodynamic therapy (PDT). The AIE molecules and OGzymes were encapsulated into phospholipid bilayers and the inner lumen of liposome, respectively, to develop a reaction cascade theranostic nanoplatform. Under light irradiation, the AIE molecules generated cytotoxic ROS, which also enabled imaging-guided PDT. The OGzymes supplied enough O2 to relieve tumor hypoxia, and they were effectively enriched and penetrated in tumor tissues consequently. Thus, this cascade catalytic nanoplatform based on ferritin nanozyme enhanced PDT efficacy.68 Veroniaina et al. designed a TME responsive nanozyme by encapsulating MnO2 into FTn through oxidation. As a cargo carrier, Dox was also loaded inside FTn for combination therapy.127 The hybrid MnO2-Dox@FTn showed both POD and CAT activities which could produce abundant O2 and Mn2+, respectively, thus relieving tumor hypoxia and enabling MRI guided therapy. Zhu et al.123 also synthesized MnO2 in FTn and subsequently loaded Ce6, one of the most commonly used photosensitizers, to obtain an innovative therapeutic MnO2-Ce6@FTn nanoplatform. This nanoplatform could accumulate in tumor tissues and catalyze the continuous production of O2 from endogenous H2O2. Combining the advantages of ferritin nanozyme with the photosensitizers Ce6, tumor PDT achieved great outcomes.
Moreover, nanozymes with different enzyme-like activities could be integrated in one platform to induce a cascade reaction for enhanced tumor therapy. Recently, our group utilized FTn as a nanozyme reactor for achieving enzymatic cascade reactions. We proposed a cascade catalytic nanoplatform using FTn as unique pre-coated protein corona and nanozymes as steady O2 suppliers.67 Specifically, ultra-small Au NPs were in situ synthesized into mesoporous silica nanoparticles (MSNs) which exhibited glucose oxidase-like activity. Then the Ru NP nanoclusters were encapsulated into the FTn inner cavity and showed CAT-like activity. The Au NPs catalyzed the oxidation of endogenous glucose to generate gluconic acid and H2O2, then FTn-Ru decomposed H2O2 into O2 for alleviating hypoxia in a TME. Therefore, the cascade nanozymes achieved enhanced TME-responsive synergistic therapeutic outcomes. Taken together, the rational design of O2-dependent therapies based on ferritin nanozymes has potential in tumor treatment.
3.3.3 Ferritin nanozymes with multienzyme-like activities.
In recent years, an increasing number of nanozymes have been discovered to possess multiple enzymatic activities, which is also one of the most important features of nanozymes distinguishing them from natural enzymes. Multienzyme-like activities include: (i) nanozymes display different enzyme-like activities separately under different conditions; (ii) nanozymes exhibit two or more enzyme-like activities simultaneously under the same conditions. Due to the unique constrained inner cavity and other properties, ferritin nanocages have been demonstrated as an effective strategy for constructing a multienzyme cascade reaction system.
For cancer therapy.
Due to the complex physiological environment in vivo, accurate and effective regulation of multienzyme activities of nanozymes remains challenging. In 2018, Fan et al.10 reported a nitrogen-doped porous carbon nanozyme with four kinds of enzymatic activities (OXD, POD, CAT and SOD) to regulate ROS. Specifically, ferritin was used as a protein shell mediator to deliver nitrogen-doped porous carbon nanosphere nanozymes (N-PCNS) into lysosomes of tumor cells, which allowed the nanozymes to perform the required activities (Fig. 7). Under acidic conditions in lysosomes, the N-PCNS generated ROS to kill tumor cells through OXD and POD activities, while under a neutral environment, they exhibited CAT and SOD activities to scavenge free radicals, thus protecting normal cells from damage. Therefore, ferritin not only endowed N-PCNSs with the ability to target tumor cells, but facilitated the delivery of N-PCNSs to lysosomes where they initiated ROS production. This ferritin guiding N-PCNS showed multiple and controllable advantages for enhanced tumor therapy.
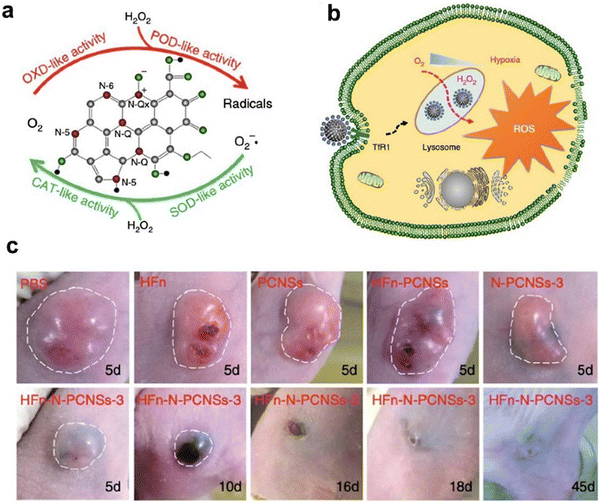 |
| Fig. 7 Ferritin guiding N-PCNSs for cancer therapy.10 (a) Schematic presentation of enzyme-like activities of N-PCNSs. (b) Schematic for N-PCNS induced tumor cell destruction via ferritin-mediated specific delivery. (c) Tumor morphology and progress with HFn-N-PCNSs treatment (n = 5). Reprinted with permission from ref. 10, © The Author(s) 2018. | |
For cardiac ischemia-reperfusion injury alleviation.
Based on de novo design of synthetic biology, we utilized FTn as the protein scaffold and synthesized MnO2 in its inner cavity, then tri-phenyl-phosphonium (TPP) was modified onto the surface of ferritin nanocages via chemical methods for mitochondria targeting.113 This novel ferritin Mn-SOD nanozyme (Mito-Fenozyme) was a promising joint cascade-catalytic platform, which exhibited high SOD-like and CAT-like activities. Moreover, this cascade nanozyme showed limited POD-like activities, thus protecting cells from toxic ˙OH. It was further confirmed that the Mito-Fenozyme could specifically target mitochondria and scavenge ˙O2− to prevent mitochondrial oxidative injury through SOD-CAT cascade reactions (Fig. 8). We previously found that the receptors of ferritin were highly expressed under tissue hypoxia,68,112,146 which enabled the Mito-Fenozyme to be delivered and enriched in the ischemic region. Taking advantage of our tailored ferritin nanozymes, we illustrated a combined therapeutic strategy for cardiac ischemia-reperfusion injury (IR) that integrated the targeting and catalytic capabilities of ferritin nanozymes, which could inspire further functional nanozyme design for other cardiovascular diseases.
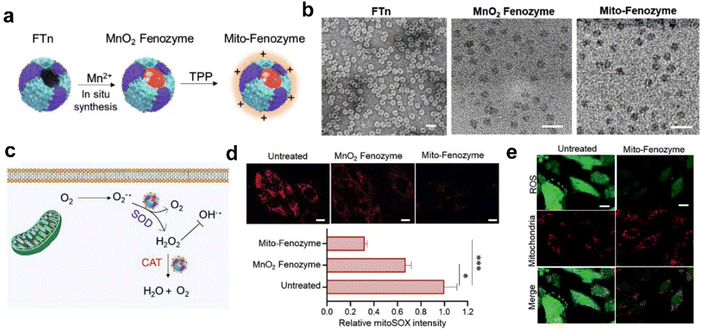 |
| Fig. 8 Hybrid ferritin nanozyme (Mito-Fenozyme) protects mitochondrial functions.113 (a) Schematic diagram for the preparation of Mito-Fenozyme. (b) TEM images of the FTn protein shell (negative staining with 1% uranyl acetate), MnO2 NPs inside the FTn shell and Mito-Fenozyme. Scale bar: 20 nm. (c) Schematic diagram for the intracellular conversion of free radicals to non-cytotoxic molecules under the catalysis of Mito-Fenozyme. (d) Confocal images (top) and quantitative analysis (bottom) for the effect of Mito-Fenozyme on mitochondrial oxidative damage. (e) Mito-Fenozyme reduced intracellular free radical levels in oxidatively damaged cells. Reprinted with permission from ref. 113, © Wiley-VCH GmbH 2021. | |
For reconstruction of artificial cells.
In addition to the significant effects and advantages in the treatment of various diseases, ferritin nanozymes have potential applications for mimicking artificial synthetic cells.147 We have investigated the enzyme-mediated molecular mechanisms in artificial cells based on a ferritin nanozyme system. In particular, we synthesized eight metal nanozymes in FTn and screened them for various catalytic activities. Meanwhile, the corresponding inducers or inhibitors were also screened to modulate their enzyme activities. We constructed conceptual cell-mimicking catalytic vesicles based on ferritin nanozyme to simulate redox homeostasis events in living cells. We aimed to provide an example for the applications of ferritin nanozyme in the top-down construction of an artificial synthetic cellular system, which could expand the application fields of nanozymes.
3.3.4 Ferritin nanozymes with other enzymatic activities.
Ferritin nanozymes also have other enzymatic activities for specific biological reactions besides the oxidoreductase activities. As we mentioned above, ferritin shows intrinsic ferroxidase-like activity, which is important for iron sequestration, antioxidant and detoxification metabolisms. Moreover, we constructed a FTn-based nanozyme library to better investigate their catalytic functions. It was unexpectedly found that FTn-Pd could mimic the activity of mutant P450BM3 which cleaved the propargyl ether moiety specially. Also, FTn-Pd with intrinsic POD-like activity produced free radicals under acidic conditions, thus causing leakage of uncoated molecules from the lysosomal membrane to the cytoplasm. Therefore, we used FTn-Pd to achieve in situ bio-orthogonal catalysis and nanozyme-mediated lysosomal membrane leakage, which was successfully applied to model pro-drug design for anticancer therapy.121
Recently, we designed cell-like catalytic NPs based on FTn nanozyme and applied them to vascular regeneration in ischemic tissue (Fig. 9).134 We found that copper-containing FTn nanozyme (FTn-Cu) enhanced the accumulation of NPs in ischemic tissues and promoted NO production from endogenous NO donor, such as S-nitrosothiol (R’SNO). Moreover, by mimicking biological processes, we previously demonstrated that bioactive factors secreted by hypoxic stem cells could promote angiogenesis effectively.134 Based on this cellular mimicry, we constructed artificial hybrid nano-cells (Hynocell) by integrating hypoxic stem cell secretome into NPs with a cell membrane surface coating fused to FTn-Cu. Hynocell fused with different cell-derived components provided synergistic effects of targeting ischemic tissues and promoting vascular regeneration in acute hindlimb ischemia (HI) as well as acute myocardial infarction models (MI). This was an innovation of building biomimetic nano-cells with ferritin nanozymes, which boosted the development of cell-free therapy.
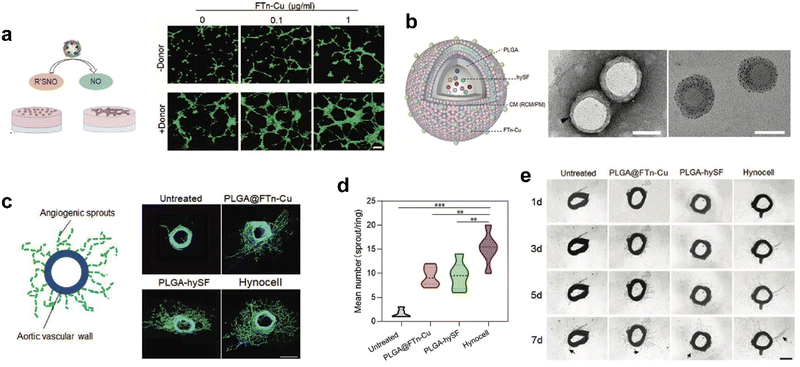 |
| Fig. 9 Artificial Hynocell with FTn-Cu nanozyme for boosted vascular regeneration in ischemic tissues.134 (a) Tube formation capacity of HUVECs with or without FTn-Cu treatments in the absence and presence of NO-donors. Scale bar: 100 μm. (b) Schematic illustration of the conceptual design of Hynocell. The Hynocell was prepared by loading hySF into PLGA, followed by coating with FTn-Cu fused CM (RCM and PM); TEM images of PLGA-CM-FTn after negative staining (left) and PLGA@FTn-Cu without negative staining (right). Scale bar: 100 nm. (c and d) Representative images and quantification analysis of induced sprouts of endothelial microvessels after different treatments based on an aortic ring assay at day 7. The formed capillary-like structures were stained with FITC-labeled phalloidin (green). Scale bar: 500 μm. (e) Representative images of microvessel sprouts (arrow) from ex vivo culture of mouse aortic rings in fibrin gel. Reprinted with permission from ref. 134, © Wiley-VCH GmbH 2022. | |
4. Perspective
Ferritin has emerged as an excellent and promising platform for nanozyme design due to its unique protein structure and intrinsic properties. Natural ferritin can be used as a template for nanozyme synthesis because of its mineralization ability. Genetically engineered ferritin as a protein scaffold provides more possibilities for nanozyme design than natural ferritin. For example, their inner surface can be genetically modified to improve the catalytic efficiency and substrate selectivity of nanozymes. The entry channels of ferritin can be regulated by mutation or deletion of amino acids at specific sites to achieve the pass-through of small molecules and metal ions.25,34,35 Furthermore, genetic engineering enables the display of targeting peptides/ligands on the surface of ferritin, making the obtained nanozymes with targeting capability in a label-free manner. Many essential issues are pending for nanozyme research, such as low enzyme-mimicking activities and poor substrate selectivity. Despite the fascinating properties of ferritin nanozymes, their catalytic activities are much lower than that of natural enzymes, which is also the main challenge of other nanozymes. Learning from engineered metalloenzymes would be a recommended direction for the rational design of nanozymes. The metalloenzymes account for 40% of all enzymes. Typically, they contain a catalytic active site and a flexible binding pocket for the substrate, providing a design principle for developing nanozymes with high enzyme-mimicking activity and specific substrate recognition. Undoubtedly, genetically modified ferritin shows great potential in these aspects. For example, by using a genetic engineering approach, ferritin-based nanozymes containing at least 24 active sites and 24 substrate binding pockets might be obtained by rational design since a ferritin nanocage is composed of 24 assembled protein subunits.
Enzymes are essential for maintaining cell functions by catalyzing a series of reactions to mediate cell metabolism, which is the basis for designing nanozymes for disease therapy. In this regard, most studies focus on how to achieve high enzyme-mimicking activities at a comparable or superior level. In our opinion, the delivery efficiency and the activity-maintaining of nanozymes are of equal importance. Several aspects are discussed as follows: (i) delivery efficacy in disease sites. Consistent with other nanomedicines, targeted delivery of nanozymes in disease sites is essential for treatment. However, the readily formed protein corona greatly limits the delivery efficacy of nanozymes due to their nonspecific absorption with protein in plasma. (ii) Intracellular locations. After cell uptake, the nanozymes tend to be trapped into lysosomes (∼pH 5.0). However, pH determines the enzyme-mimicking activities of nanozymes. For example, the same nanozyme possesses POD-like activity under acid conditions but exhibits CAT-like activity at neutral pH. (iii) Activity-maintaining in disease sites. The enzyme-mimicking activities of nanozymes are detected and screened in solution in vitro, which is not consistent with the complex microenvironment in vivo. For example, the formed protein corona greatly reduces enzyme-mimicking activities by hindering the access of substrate to catalytic active sites. The trapped nanozymes in lysosomes also render their unexpected enzyme-like activities. As protein-based nanozymes, ferritin nanozymes undoubtedly have advantages in the delivery efficiency and the activity-maintaining over other conventional nanozymes. These properties were demonstrated in our recent reports.66–68,112,146 For example, FTn nanocages actively bind with TfR1, which is highly expressed in hypoxic cells, such as tumor cells and the cells involved in ischemic tissues. The intrinsic properties render their direct targeted delivery without extra modification. Furthermore, ferritin-based nanozymes do not easily form protein corona due to the protection of their protein shell. Ferritin-based nanozymes capable of reducing lysosomal trapping can also be designed using a genetic engineering strategy. Future studies should continue to improve the properties of ferritin nanozymes (e.g. substrate binding, enzyme-mimicking activity) by combining with the design of ferritin structure in order to maximize their therapeutic potential.
Conflicts of interest
The authors declare no conflicts of interests.
Acknowledgements
We acknowledge the support from the National Natural Science Foundation of China (NSFC) (82072054, 32271448), the National Key Research and Development Program of China (2022YFA1105100), the Tianjin Synthetic Biotechnology Innovation Capacity Improvement Project (TSBICIP-KJGG-014-03), and the Nankai University Hundred Young Academic Leaders Program.
References
- M. Liang and X. Yan, Acc. Chem. Res., 2019, 52, 2190–2200 CrossRef CAS PubMed.
- L. Gao, J. Zhuang, L. Nie, J. Zhang, Y. Zhang, N. Gu, T. Wang, J. Feng, D. Yang, S. Perrett and X. Yan, Nat. Nanotechnol., 2007, 2, 577–583 CrossRef CAS PubMed.
- X. Y. Wang, Y. H. Hu and H. Wei, Inorg. Chem. Front., 2016, 3, 41–60 RSC.
- A. Pratsinis, G. A. Kelesidis, S. Zuercher, F. Krumeich, S. Bolisetty, R. Mezzenga, J. C. Leroux and G. A. Sotiriou, ACS Nano, 2017, 11, 12210–12218 CrossRef CAS PubMed.
- H. Wei and E. Wang, Anal. Chem., 2008, 80, 2250–2254 CrossRef CAS PubMed.
- L. Q. Mei, S. Zhu, Y. P. Liu, W. Y. Yin, Z. J. Gu and Y. L. Zhao, Chem. Eng. J., 2021, 418, 129431 CrossRef CAS.
- Y. Chen, H. Zou, B. Yan, X. J. Wu, W. W. Cao, Y. H. Qian, L. Zheng and G. W. Yang, Adv. Sci., 2022, 9, 2103977 CrossRef CAS PubMed.
- H. Zhao, R. Zhang, X. Yan and K. Fan, J. Mater. Chem. B, 2021, 9, 6939–6957 RSC.
- D. M. Duan, K. L. Fan, D. X. Zhang, S. G. Tan, M. F. Liang, Y. Liu, J. L. Zhang, P. H. Zhang, W. Liu, X. G. Qiu, G. P. Kobinger, G. F. Gao and X. Y. Yan, Biosens. Bioelectron., 2015, 74, 134–141 CrossRef CAS PubMed.
- K. L. Fan, J. Q. Xi, L. Fan, P. X. Wang, C. H. Zhu, Y. Tang, X. D. Xu, M. M. Liang, B. Jiang, X. Y. Yan and L. Z. Gao, Nat. Commun., 2018, 9, 1440 CrossRef PubMed.
- T. Wang, J. Y. He, D. M. Duan, B. Jiang, P. X. Wang, K. L. Fan, M. M. Liang and X. Y. Yan, Nano Res., 2019, 12, 863–868 CrossRef CAS.
- S. H. Choi, I. C. Kwon, K. Y. Hwang, I. S. Kim and H. J. Ahn, Biomacromolecules, 2011, 12, 3099–3106 CrossRef CAS PubMed.
- H. Moon, J. Lee, J. Min and S. Kang, Biomacromolecules, 2014, 15, 3794–3801 CrossRef CAS PubMed.
- E. J. Lee, N. K. Lee and I. S. Kim, Adv. Drug Delivery Rev., 2016, 106, 157–171 CrossRef CAS PubMed.
- J. Min, S. Kim, J. Lee and S. Kang, RSC Adv., 2014, 4, 48596–48600 RSC.
- B. Jiang, L. Fang, K. Wu, X. Yan and K. Fan, Theranostics, 2020, 10, 687–706 CrossRef CAS PubMed.
- V. Laufberger, Bull. Soc. Chim. Biol., 1937, 19, 1575–1582 CAS.
- A. Parida, A. Mohanty, B. T. Kansara and R. K. Behera, Inorg. Chem., 2020, 59, 629–641 CrossRef CAS PubMed.
- E. C. Theil, Adv. Enzymol. Relat. Areas Mol. Biol., 1990, 63, 421–449 CAS.
- P. M. Harrison and P. Arosio, Biochim. Biophys. Acta, 1996, 1275, 161–203 CrossRef PubMed.
- Y. Zhang and B. P. Orner, Int. J. Mol. Sci., 2011, 12, 5406–5421 CrossRef CAS PubMed.
- X. H. Min, T. Fang, L. L. Li, C. Q. Li, Z. P. Zhang, X. E. Zhang and F. Li, Nanoscale, 2020, 12, 2340–2344 RSC.
- E. C. Theil, R. K. Behera and T. Tosha, Coord. Chem. Rev., 2013, 257, 579–586 CrossRef CAS PubMed.
- E. C. Theil, Annu. Rev. Biochem., 1987, 56, 289–315 CrossRef CAS PubMed.
- A. Parida, A. Mohanty, R. K. Raut, I. Padhy and R. K. Behera, Inorg. Chem., 2023, 62, 178–191 CrossRef CAS PubMed.
- P. D. Hempstead, S. J. Yewdall, A. R. Fernie, D. M. Lawson, P. J. Artymiuk, D. W. Rice, G. C. Ford and P. M. Harrison, J. Mol. Biol., 1997, 268, 424–448 CrossRef CAS PubMed.
- D. M. Lawson, A. Treffry, P. J. Artymiuk, P. M. Harrison, S. J. Yewdall, A. Luzzago, G. Cesareni, S. Levi and P. Arosio, FEBS Lett., 1989, 254, 207–210 CrossRef CAS PubMed.
- V. J. Wade, S. Levi, P. Arosio, A. Treffry, P. M. Harrison and S. Mann, J. Mol. Biol., 1991, 221, 1443–1452 CrossRef CAS PubMed.
- T. R. Daniels, T. Delgado, J. A. Rodriguez, G. Helguera and M. L. Penichet, Clin. Immunol., 2006, 121, 144–158 CrossRef CAS PubMed.
- T. R. Daniels, T. Delgado, G. Helguera and M. L. Penichet, Clin. Immunol., 2006, 121, 159–176 CrossRef CAS PubMed.
- T. T. Chen, L. Li, D. H. Chung, C. D. C. Allen, S. V. Torti, F. M. Torti, J. G. Cyster, C. Y. Chen, F. M. Brodsky, E. C. Niemi, M. C. Nakamura, W. E. Seaman and M. R. Daws, J. Exp. Med., 2005, 202, 955–965 CrossRef CAS PubMed.
- J. Y. Li, N. Paragas, R. M. Ned, A. D. Qiu, M. Viltard, T. Leete, I. R. Drexler, X. Chen, S. Sanna-Cherchi, F. Mohammed, D. Williams, C. S. Lin, K. M. Schmidt-Ott, N. C. Andrews and J. Barasch, Dev. Cell, 2009, 16, 35–46 CrossRef CAS PubMed.
- A. Mohanty, A. Parida, R. K. Raut and R. K. Behera, ACS Bio Med Chem Au, 2022, 2, 258–281 CrossRef CAS PubMed.
- R. K. Behera and E. C. Theil, Proc. Natl. Acad. Sci. U. S. A., 2014, 111, 7925–7930 CrossRef CAS PubMed.
- R. K. Behera, R. Torres, T. Tosha, J. M. Bradley, C. W. Goulding and E. C. Theil, JBIC, J. Biol. Inorg. Chem., 2015, 20, 957–969 CrossRef CAS PubMed.
- A. Treffry, E. R. Bauminger, D. Hechel, N. W. Hodson, I. Nowik, S. J. Yewdall and P. M. Harrison, Biochem. J., 1993, 296, 721–728 CrossRef CAS PubMed.
- T. Tosha, H.-L. Ng, O. Bhattasali, T. Alber and E. C. Theil, J. Am. Chem. Soc., 2010, 132, 14562–14569 CrossRef CAS PubMed.
- K. Honarmand Ebrahimi, P.-L. Hagedoorn and W. R. Hagen, Chem. Rev., 2015, 115, 295–326 CrossRef CAS PubMed.
- L. Toussaint, L. Bertrand, L. Hue, R. R. Crichton and J. P. Declercq, J. Mol. Biol., 2007, 365, 440–452 CrossRef CAS PubMed.
- R. R. Crichton and J. P. Declercq, Biochim. Biophys. Acta, 2010, 1800, 706–718 CrossRef CAS PubMed.
- O. Kasyutich, A. Ilari, A. Fiorillo, D. Tatchev, A. Hoell and P. Ceci, J. Am. Chem. Soc., 2010, 132, 3621–3627 CrossRef CAS PubMed.
- S. Kanbak-Aksu, M. Nahid Hasan, W. R. Hagen, F. Hollmann, D. Sordi, R. A. Sheldon and I. W. Arends, Chem. Commun., 2012, 48, 5745–5747 RSC.
- C. A. Butts, J. Swift, S. G. Kang, L. Di Costanzo, D. W. Christianson, J. G. Saven and I. J. Dmochowski, Biochemistry, 2008, 47, 12729–12739 CrossRef CAS PubMed.
- F. C. Meldrum, V. J. Wade, D. L. Nimmo, B. R. Heywood and S. Mann, Nature, 1991, 349, 684–687 CrossRef CAS.
- J. F. Hainfeld, Proc. Natl. Acad. Sci. U. S. A., 1992, 89, 11064–11068 CrossRef CAS PubMed.
- J. W. Kim, S. H. Choi, P. T. Lillehei, S. H. Chu, G. C. King and G. D. Watt, Chem. Commun., 2005, 4101–4103, 10.1039/b505097a.
- T. Ueno, M. Abe, K. Hirata, S. Abe, M. Suzuki, N. Shimizu, M. Yamamoto, M. Takata and Y. Watanabe, J. Am. Chem. Soc., 2009, 131, 5094–5100 CrossRef CAS PubMed.
- S. Abe, T. Hikage, Y. Watanabe, S. Kitagawa and T. Ueno, Inorg. Chem., 2010, 49, 6967–6973 CrossRef CAS PubMed.
- S. Abe, J. Niemeyer, M. Abe, Y. Takezawa, T. Ueno, T. Hikage, G. Erker and Y. Watanabe, J. Am. Chem. Soc., 2008, 130, 10512–10514 CrossRef CAS PubMed.
- P. A. Sontz, J. B. Bailey, S. Aln and F. A. Tezcan, J. Am. Chem. Soc., 2015, 137, 11598–11601 CrossRef CAS PubMed.
- K. Zeth, S. Offermann, L. O. Essen and D. Oesterhelt, Proc. Natl. Acad. Sci. U. S. A., 2004, 101, 13780–13785 CrossRef CAS PubMed.
- S. L. Zhang, J. C. Zang, W. M. Wang, H. Chen, X. R. Zhang, F. D. Wang, H. F. Wang and G. H. Zhao, Angew. Chem., Int. Ed., 2016, 55, 16064–16070 CrossRef CAS PubMed.
- S. L. Zhang, J. C. Zang, X. R. Zhang, H. Chen, B. Mikami and G. H. Zhao, ACS Nano, 2016, 10, 10382–10388 CrossRef CAS PubMed.
- S. Kang, L. M. Oltrogge, C. C. Broomell, L. O. Liepold, P. E. Prevelige, M. Young and T. Douglas, J. Am. Chem. Soc., 2008, 130, 16527–16529 CrossRef CAS PubMed.
- M. Kim, Y. Rho, K. S. Jin, B. Ahn, S. Jung, H. Kim and M. Ree, Biomacromolecules, 2011, 12, 1629–1640 CrossRef CAS PubMed.
- D. Sato, H. Ohtomo, Y. Yamada, T. Hikima, A. Kurobe, K. Fujiwara and M. Ikeguchi, Biochemistry, 2016, 55, 287–293 CrossRef CAS PubMed.
- A. Mohanty, K. Mithra, S. S. Jena and R. K. Behera, Biomacromolecules, 2021, 22, 1389–1398 CrossRef CAS PubMed.
- R. R. Crichton and C. F. A. Bryce, Biochem. J., 1973, 133, 289–299 CrossRef CAS PubMed.
- D. J. E. Huard, K. M. Kane and F. A. Tezcan, Nat. Chem. Biol., 2013, 9, 169–176 CrossRef CAS.
- C. Gu, T. Zhang, C. Lv, Y. Liu, Y. Wang and G. Zhao, ACS Nano, 2020, 14, 17080–17090 CrossRef CAS PubMed.
- M. Uchida, S. Kang, C. Reichhardt, K. Harlen and T. Douglas, Biochim. Biophys. Acta, 2010, 1800, 834–845 CrossRef CAS.
- B. Zhang, X. Chen, G. Tang, R. Zhang, J. Li, G. Sun, X. Yan and K. Fan, Nano Today, 2022, 46, 101564 CrossRef CAS.
- B. Jiang, R. Zhang, J. Zhang, Y. Hou, X. Chen, M. Zhou, X. Tian, C. Hao, K. Fan and X. Yan, Theranostics, 2019, 9, 2167–2182 CrossRef CAS PubMed.
- M. Liang, K. Fan, M. Zhou, D. Duan, J. Zheng, D. Yang, J. Feng and X. Yan, Proc. Natl. Acad. Sci. U. S. A., 2014, 111, 14900–14905 CrossRef CAS.
- K. Fan, L. Gao and X. Yan, Wiley Interdiscip. Rev.: Nanomed. Nanobiotechnol., 2013, 5, 287–298 CAS.
- Q. Liu, J. Tian, J. Liu, M. Zhu, Z. Gao, X. Hu, A. C. Midgley, J. Wu, X. Wang, D. Kong, J. Zhuang, J. Liu, X. Yan and X. Huang, Adv. Mater., 2021, 33, 2103128 CrossRef CAS PubMed.
- Z. J. Liu, Q. Q. Liu, H. Q. Zhang, X. Y. Zhang, J. Wu, Z. Y. Sun, M. S. Zhu, X. Y. Hu, T. Y. Qi, H. L. Kang, R. Chen, X. L. Huang and J. Zhuang, Adv. Funct. Mater., 2022, 32, 2208513 CrossRef CAS.
- F. Gao, J. Wu, H. Gao, X. Hu, L. Liu, A. C. Midgley, Q. Liu, Z. Sun, Y. Liu, D. Ding, Y. Wang, D. Kong and X. Huang, Biomaterials, 2020, 230, 119635 CrossRef CAS PubMed.
- J. Zhang, D. Cheng, J. He, J. Hong, C. Yuan and M. Liang, Nat. Protoc., 2021, 16, 4878–4896 CrossRef CAS.
- B. Jiang, X. H. Chen, G. M. Sun, X. R. Chen, Y. F. Yin, Y. L. Jin, Q. Mi, L. Ma, Y. L. Yang, X. Y. Yan and K. L. Fan, Nano Today, 2020, 35, 100948 CrossRef CAS.
- P. Huang, P. F. Rong, A. Jin, X. F. Yan, M. G. Zhang, J. Lin, H. Hu, Z. Wang, X. Y. Yue, W. W. Li, G. Niu, W. B. Zeng, W. Wang, K. C. Zhou and X. Y. Chen, Adv. Mater., 2014, 26, 6401–6408 CrossRef CAS PubMed.
- J. M. Dominguez-Vera, J. Inorg. Biochem., 2004, 98, 469–472 CrossRef CAS PubMed.
- Z. Yang, X. Wang, H. Diao, J. Zhang, H. Li, H. Sun and Z. Guo, Chem. Commun., 2007, 3453–3455, 10.1039/b705326f.
- P. Ji, X. Wang, J. Yin, Y. Mou, H. Huang and Z. Ren, Drug Delivery, 2022, 29, 986–996 CrossRef CAS.
- P. Ji, H. Huang, S. Yuan, L. Wang, S. Wang, Y. Chen, N. Feng, H. Veroniaina, Z. Wu, Z. Wu and X. Qi, Adv. Healthcare Mater., 2019, 8, e1900911 CrossRef.
- X. Lin, Q. Wang, C. Gu, M. Li, K. Chen, P. Chen, Z. Tang, X. Liu, H. Pan, Z. Liu, R. Tang and S. Fan, J. Am. Chem. Soc., 2020, 142, 17543–17556 CrossRef CAS.
- Y. He, E. Ren, Z. Lu, H. Chen, Z. Qin, J. Wang, M. He, G. Liu, L. Zheng and J. Zhao, Nanomedicine, 2020, 28, 102210 CrossRef CAS PubMed.
- E. Falvo, A. Arcovito, G. Conti, G. Cipolla, M. Pitea, V. Morea, V. Damiani, G. Sala, G. Fracasso and P. Ceci, Pharmaceutics, 2020, 12, 992 CrossRef CAS PubMed.
- Z. L. Zhu, H. Y. Luo, T. Wang, C. H. Zhang, M. M. Liang, D. Q. Yang, M. H. Liu, W. W. Yu, Q. Bai, L. N. Wang and N. Sui, Chem. Mater., 2022, 34, 1356–1368 CrossRef CAS.
- R. Li, Y. Ma, Y. Dong, Z. Zhao, C. You, S. Huang, X. Li, F. Wang and Y. Zhang, ACS Biomater. Sci. Eng., 2019, 5, 6645–6654 CrossRef CAS.
- R. Yang, Y. Liu, D. Meng, Z. Chen, C. L. Blanchard and Z. Zhou, J. Agric. Food Chem., 2017, 65, 1410–1419 CrossRef CAS PubMed.
- X. Lin, J. Xie, G. Niu, F. Zhang, H. K. Gao, M. Yang, Q. M. Quan, M. A. Aronova, G. F. Zhang, S. Lee, R. Leaprnan and X. Y. Chen, Nano Lett., 2011, 11, 814–819 CrossRef CAS.
- Z. P. Zhen, W. Tang, C. L. Guo, H. M. Chen, X. Lin, G. Liu, B. W. Fei, X. Y. Chen, B. Q. Xu and J. Xie, ACS Nano, 2013, 7, 6988–6996 CrossRef CAS.
- Y. Zhang, Y. Li, J. Zhang, X. Chen, R. Zhang, G. Sun, B. Jiang, K. Fan, Z. Li and X. Yan, Small, 2021, 17, e2101655 CrossRef PubMed.
- X. Ma, F. Zou, F. Yu, R. Li, Y. Yuan, Y. Zhang, X. Zhang, J. Deng, T. Chen, Z. Song, Y. Qiao, Y. Zhan, J. Liu, J. Zhang, X. Zhang, Z. Peng, Y. Li, Y. Lin, L. Liang, G. Wang, Y. Chen, Q. Chen, T. Pan, X. He and H. Zhang, Immunity, 2020, 53, 1315–1330e1319 CrossRef CAS.
- B. Jiang, L. Yan, J. Zhang, M. Zhou, G. Shi, X. Tian, K. Fan, C. Hao and X. Yan, ACS Appl. Mater. Interfaces, 2019, 11, 9747–9755 CrossRef CAS PubMed.
- H. J. Kang, Y. J. Kang, Y. M. Lee, H. H. Shin, S. J. Chung and S. Kang, Biomaterials, 2012, 33, 5423–5430 CrossRef CAS.
- W. Wang, X. Zhou, Y. Bian, S. Wang, Q. Chai, Z. Guo, Z. Wang, P. Zhu, H. Peng, X. Yan, W. Li, Y. X. Fu and M. Zhu, Nat. Nanotechnol., 2020, 15, 406–416 CrossRef CAS PubMed.
- K. Sliepen, G. Ozorowski, J. Burger, T. van Montfort, M. Stunnenberg, I. Bontjer, C. LaBranche, D. Montefiori, J. Moore, A. Ward and R. Sanders, JAIDS, J. Acquired Immune Defic. Syndr., 2016, 71, 52 CrossRef.
- U. Kalathiya, M. Padariya, R. Fahraeus, S. Chakraborti and T. R. Hupp, Biomolecules, 2021, 11, 297 CrossRef CAS PubMed.
- A. E. Powell, K. M. Zhang, M. Sanyal, S. G. Tang, P. A. Weidenbacher, S. S. Li, T. D. Pham, J. E. Pak, W. Chiu and P. S. Kim, ACS Cent. Sci., 2021, 7, 183–199 CrossRef CAS PubMed.
- X. Li, L. H. Qiu, P. Zhu, X. Y. Tao, T. Imanaka, J. Zhao, Y. G. Huang, Y. P. Tu and X. N. Cao, Small, 2012, 8, 2505–2514 CrossRef CAS PubMed.
- S. Yin, Y. Wang, B. Y. Zhang, Y. R. Qu, Y. D. Liu, S. Dai, Y. Zhang, Y. L. Wang and J. X. Bi, Pharmaceutics, 2021, 13, 521 CrossRef CAS PubMed.
- P. P. Jin, R. Sha, Y. J. Zhang, L. Liu, Y. P. Bian, J. Qian, J. Y. Qian, J. Lin, N. Ishimwe, Y. Hu, W. B. Zhang, Y. C. Liu, S. H. Yin, L. Ren and L. P. Wen, Nano Lett., 2019, 19, 1467–1478 CrossRef CAS PubMed.
- M. Kih, E. J. Lee, N. K. Lee, Y. K. Kim, K. E. Lee, C. Jeong, Y. Yang, D. H. Kim and I. S. Kim, Biomaterials, 2018, 180, 67–77 CrossRef CAS PubMed.
- Y. Ma, Y. Dong, X. Li, F. Wang and Y. Zhang, ACS Appl. Bio Mater., 2021, 4, 2654–2663 CrossRef CAS PubMed.
- S. Kim, J. O. Jeon, E. Jun, J. Jee, H. K. Jung, B. H. Lee, I. S. Kim and S. Kim, Biomacromolecules, 2016, 17, 1150–1159 CrossRef CAS PubMed.
- J. O. Jeon, S. Kim, E. Choi, K. Shin, K. Cha, I. S. So, S. J. Kim, E. Jun, D. Kim, H. J. Ahn, B. H. Lee, S. H. Lee and I. S. Kim, ACS Nano, 2013, 7, 7462–7471 CrossRef CAS PubMed.
- Z. T. Wang, L. F. Xu, H. Yu, P. Lv, Z. Lei, Y. Zeng, G. Liu and T. Cheng, Biomater. Sci., 2019, 7, 1794–1800 RSC.
- M. Kanekiyo, C. J. Wei, H. M. Yassine, P. M. McTamney, J. C. Boyington, J. R. R. Whittle, S. S. Rao, W. P. Kong, L. S. Wang and G. J. Nabel, Nature, 2013, 499, 102–106 CrossRef CAS PubMed.
- N. Darricarrere, S. Pougatcheva, X. C. Duan, R. S. Rudicell, T. H. Chou, J. DiNapoli, T. M. Ross, T. Alefantis, T. U. Vogel, H. Kleanthous, C. J. Wei and G. J. Nabel, J. Virol., 2018, 92, e01349-18 CrossRef PubMed.
- H. G. Kelly, H. X. Tan, J. A. Juno, R. Esterbauer, Y. Ju, W. B. Jiang, V. C. Wimmer, B. C. Duckworth, J. R. Groom, F. Caruso, M. Kanekiyo, S. J. Kent and A. K. Wheatley, Jci Insight, 2020, 5, e136653 CrossRef PubMed.
- J. M. Dominguez-Vera, N. Galvez, P. Sanchez, A. J. Mota, S. Trasobares, J. C. Hernandez and J. J. Calvino, Eur. J. Inorg. Chem., 2007, 4823–4826, DOI:10.1002/ejic.200700606.
- L. Zhang, J. Swift, C. A. Butts, V. Yerubandi and I. J. Dmochowski, J. Inorg. Biochem., 2007, 101, 1719–1729 CrossRef CAS PubMed.
- T. Ueno, M. Suzuki, T. Goto, T. Matsumoto, K. Nagayama and Y. Watanabe, Angew. Chem., Int. Ed., 2004, 43, 2527–2530 CrossRef CAS PubMed.
- D. Ensign, M. Young and T. Douglas, Inorg. Chem., 2004, 43, 3441–3446 CrossRef CAS PubMed.
- T. Douglas and V. T. Stark, Inorg. Chem., 2000, 39, 1828–1830 CrossRef CAS PubMed.
- M. Okuda, K. Iwahori, I. Yamashita and H. Yoshimura, Biotechnol. Bioeng., 2003, 84, 187–194 CrossRef CAS PubMed.
- M. Peskova, L. Ilkovics, D. Hynek, S. Dostalova, E. M. Sanchez-Carnerero, M. Remes, Z. Heger and V. Pekarik, J. Colloid Interface Sci., 2019, 537, 20–27 CrossRef CAS PubMed.
- C. C. Jolley, M. Uchida, C. Reichhardt, R. Harrington, S. Kang, M. T. Klem, J. B. Parise and T. Douglas, Chem. Mater., 2010, 22, 4612–4618 CrossRef CAS.
- J. Swift, C. A. Butts, J. Cheung-Lau, V. Yerubandi and I. J. Dmochowski, Langmuir, 2009, 25, 5219–5225 CrossRef CAS PubMed.
- X. Huang, J. Zhuang, S. W. Chung, B. Huang, G. Halpert, K. Negron, X. Sun, J. Yang, Y. Oh, P. M. Hwang, J. Hanes and J. S. Suk, ACS Nano, 2019, 13, 236–247 CrossRef CAS PubMed.
- Y. Zhang, A. Khalique, X. Du, Z. Gao, J. Wu, X. Zhang, R. Zhang, Z. Sun, Q. Liu, Z. Xu, A. C. Midgley, L. Wang, X. Yan, J. Zhuang, D. Kong and X. Huang, Adv. Mater., 2021, 33, e2006570 CrossRef PubMed.
- R. M. Kramer, C. Li, D. C. Carter, M. O. Stone and R. R. Naik, J. Am. Chem. Soc., 2004, 126, 13282–13286 CrossRef CAS PubMed.
- R. Fan, S. W. Chew, V. V. Cheong and B. P. Orner, Small, 2010, 6, 1483–1487 CrossRef CAS PubMed.
- X. Jiang, C. Sun, Y. Guo, G. Nie and L. Xu, Biosens. Bioelectron., 2015, 64, 165–170 CrossRef CAS PubMed.
- N. Gálvez, P. Sánchez and J. M. Domínguez-Vera, Dalton Trans., 2005, 2492–2494, 10.1039/B506290J.
- W. Zhang, Y. Zhang, Y. Chen, S. Li, N. Gu, S. Hu, Y. Sun, X. Chen and Q. Li, J. Nanosci. Nanotechnol., 2013, 13, 60–67 CrossRef CAS PubMed.
- K. Fan, C. Cao, Y. Pan, D. Lu, D. Yang, J. Feng, L. Song, M. Liang and X. Yan, Nat. Nanotechnol., 2012, 7, 459–464 CrossRef CAS PubMed.
- X. Hu, X. Wang, Q. Liu, J. Wu, H. Zhang, A. Khalique, Z. Sun, R. Chen, J. Wei, H. Li, D. Kong, J. Zhuang, X. Yan and X. Huang, ACS Appl. Mater. Interfaces, 2021, 13, 21087–21096 CrossRef CAS PubMed.
- Z. Sun, Q. Liu, X. Wang, J. Wu, X. Hu, M. Liu, X. Zhang, Y. Wei, Z. Liu, H. Liu, R. Chen, F. Wang, A. C. Midgley, A. Li, X. Yan, Y. Wang, J. Zhuang and X. Huang, Theranostics, 2022, 12, 1132–1147 CrossRef CAS PubMed.
- M. Liu, Y. Zhu, D. Jin, L. Li, J. Cheng and Y. Liu, Inorg. Chem., 2021, 60, 14515–14519 CrossRef CAS PubMed.
- Y. Zhu, D. Jin, M. Liu, Y. Dai, L. Li, X. Zheng, L. Wang, A. Shen, J. Yu, S. Wu, Y. Wu, K. Zhong, J. Cheng and Y. Liu, Small, 2022, 18, e2200116 CrossRef PubMed.
- X. Liu, W. Wei, Q. Yuan, X. Zhang, N. Li, Y. Du, G. Ma, C. Yan and D. Ma, Chem. Commun., 2012, 48, 3155–3157 RSC.
- J. Fan, J. J. Yin, B. Ning, X. Wu, Y. Hu, M. Ferrari, G. J. Anderson, J. Wei, Y. Zhao and G. Nie, Biomaterials, 2011, 32, 1611–1618 CrossRef CAS PubMed.
- A. Sennuga-Arowolo, J. Marwijk and C. Whiteley, Nanotechnology, 2011, 23, 035102 CrossRef PubMed.
- H. Veroniaina, Z. Wu and X. Qi, J. Adv. Res., 2021, 33, 201–213 CrossRef CAS PubMed.
- S. Zhao, H. Duan, Y. Yang, X. Yan and K. Fan, Nano Lett., 2019, 19, 8887–8895 CrossRef CAS PubMed.
- L. Zhang, L. Laug, W. Munchgesang, E. Pippel, U. Gosele, M. Brandsch and M. Knez, Nano Lett., 2010, 10, 219–223 CrossRef PubMed.
- L. Zhang, W. Fischer, E. Pippel, G. Hause, M. Brandsch and M. Knez, Small, 2011, 7, 1538–1541 CrossRef CAS PubMed.
- F. Dashtestani, H. Ghourchian and A. Najafi, Mater. Sci. Eng., C, 2019, 94, 831–840 CrossRef CAS PubMed.
- K. Xiong, Y. Zhou, J. Karges, K. Du, J. Shen, M. Lin, F. Wei, J. Kou, Y. Chen, L. Ji and H. Chao, ACS Appl. Mater. Interfaces, 2021, 13, 38959–38968 CrossRef CAS PubMed.
- T. N. Aslan, E. Asik, N. T. Guray and M. Volkan, J. Biol. Inorg. Chem., 2020, 25, 1139–1152 CrossRef CAS PubMed.
- X. Zhang, Y. Zhang, R. Zhang, X. Jiang, A. C. Midgley, Q. Liu, H. Kang, J. Wu, A. Khalique, M. Qian, D. An, J. Huang, L. Ou, Q. Zhao, J. Zhuang, X. Yan, D. Kong and X. Huang, Adv. Mater., 2022, 34, 2110352 CrossRef CAS PubMed.
- C. Cavazza, C. Bochot, P. Rousselot-Pailley, P. Carpentier, M. V. Cherrier, L. Martin, C. Marchi-Delapierre, J. C. Fontecilla-Camps and S. Menage, Nat. Chem., 2010, 2, 1069–1076 CrossRef CAS PubMed.
- R. Kubota, S. Tashiro, M. Shiro and M. Shionoya, Nat. Chem., 2014, 6, 913–918 CrossRef CAS PubMed.
- B. Maity, S. Abe and T. Ueno, Nat. Commun., 2017, 8, 14820 CrossRef CAS PubMed.
- G. S. Arapova, A. N. Eryomin and D. I. Metelitza, Biochemistry, 1997, 62, 1415–1423 CAS.
- G. S. Arapova, A. N. Eryomin and D. I. Metelitza, Biochemistry, 1998, 63, 1191–1199 CAS.
- V. Borelli, E. Trevisan, F. Vita, C. Bottin, M. Melato, C. Rizzardi and G. Zabucchi, J. Toxicol. Environ. Health, Part A, 2012, 75, 603–623 CrossRef CAS PubMed.
- Z. Tang, H. Wu, Y. Zhang, Z. Li and Y. Lin, Anal. Chem., 2011, 83, 8611–8616 CrossRef CAS PubMed.
- J. Y. Guo, Y. T. Liu, J. Q. Zha, H. H. Han, Y. T. Chen and Z. F. Jia, Polym. Chem., 2021, 12, 858–866 RSC.
- W. W. Wu, Q. Q. Wang, J. X. Chen, L. Huang, H. Zhang, K. Rong and S. J. Dong, Nanoscale, 2019, 11, 12603–12609 RSC.
- A. M. Alsharabasy, A. Pandit and P. Farras, Adv. Mater., 2021, 33, 2003883 CrossRef CAS PubMed.
- Y. Shu, J. Y. Chen, Z. Xu, D. Q. Jin, Q. Xu and X. Y. Hu, J. Electroanal. Chem., 2019, 845, 137–143 CrossRef CAS.
- X. Huang, J. Chisholm, J. Zhuang, Y. Xiao, G. Duncan, X. Chen, J. S. Suk and J. Hanes, Proc. Natl. Acad. Sci. U. S. A., 2017, 114, E6595–E6602 CAS.
- J. Wu, Y. Wei, J. Lan, X. Hu, F. Gao, X. Zhang, Z. Gao, Q. Liu, Z. Sun, R. Chen, H. Zhao, K. Fan, X. Yan, J. Zhuang and X. Huang, Small, 2022, e2202145, DOI:10.1002/smll.202202145.
Footnote |
† C. Wang and Q. Liu contributed equally to this work. |
|
This journal is © The Royal Society of Chemistry 2023 |