DOI:
10.1039/D3TA01059G
(Review Article)
J. Mater. Chem. A, 2023,
11, 9383-9400
Novel palladium-based nanomaterials for multifunctional ORR/OER/HER electrocatalysis
Received
20th February 2023
, Accepted 30th March 2023
First published on 4th April 2023
Abstract
With the growing demand for sustainable energy, research on electrocatalysis is attracting more attention in the fabrication of clean energy devices (e.g., fuel cells, electrolyzers, and metal–air batteries). The oxygen reduction reaction (ORR), oxygen evolution reaction (OER), and hydrogen evolution reaction (HER) are the most significant electrocatalytic reactions requiring highly active and stable catalysts to address the cost, performance, and durability issues. This review summarizes novel palladium-based nanomaterials for multifunctional ORR/OER/HER electrocatalysis in both acidic and alkaline media. The mechanism of electrocatalytic reactions and the challenges of the Pd-based catalysts are demonstrated. To solve the problems, researchers' strategies include but are not limited to alloying design, single-atom catalyst design, hybrid nanomaterial fabrication, interface engineering, etc. Finally, the advantages of Pd-based nanomaterials are summarized and their prospects for high activity and stable electrocatalysis are proposed.
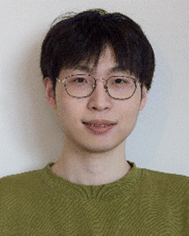 Hangxuan Li | Hangxuan Li received his bachelor's degree in 2021 from Beijing Institute of Technology. He is currently a master's student at the University of Alberta. His current research is focused on the preparation of self-supporting electrocatalysts and their application in water splitting. |
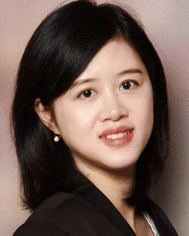 Ge Li | Prof. Ge Li is currently an assistant professor in the Department of Mechanical Engineering at the University of Alberta. Her current research is mainly focused on the rational design and development of advanced nanostructured materials for clean energy conversion and storage technologies, including various types of rechargeable batteries, supercapacitors, and electrocatalysis-based systems. |
1. Introduction
With the growth of the Earth's population worldwide and industrialization in developing countries, the demand for energy by humans has reached an unprecedented level.1 The major source of energy in daily life is fossil fuels. However, the huge consumption of fossil fuels has caused many problems in the past two centuries.2 For example, the rising emissions of greenhouse gases are closely related to the combustion of fossil fuels. Besides, due to the non-renewable nature of fossil fuels, people may face a shortage of fossil fuels in the next few decades. Hence, seeking alternatives to fossil fuels is very important for addressing the energy and environmental problems of the 21st century.3 The oxygen reduction reaction (ORR), oxygen evolution reaction (OER), and hydrogen evolution reaction (HER) are the most important reactions with extensive applications in electrocatalysis.4 In detail, the ORR occurs in the cathode of fuel cells; water splitting involves the OER and HER; the ORR and OER also occur in metal–air batteries. Developing efficient and stable electrocatalysts for these reactions has become more and more important over the years.5 Pt/C is a commonly used commercial electrocatalyst, but its scarcity and inferior stability greatly impede the development of Pt-based electrocatalysts. At the same time, Pt-based materials cannot be applied to OER electrocatalysis due to their poor activity. For the OER, the commercial catalyst is RuO2 or IrO2 supported on carbon, which shows similar problems to commercial Pt/C. Moreover, the development of noble materials for multifunctional electrocatalysis has gradually gained researchers' attention due to the demand for energy conversion devices (e.g., fuel cells, electrolyzers, and metal–air batteries).
In general, some significant parameters need to be considered for electrocatalysis,6 including the rarity of the materials, their electrocatalytic performance (e.g., the overpotential and current density of the reaction), their long-term durability in different working environments,7 their multiple functions in different electrocatalytic reactions, the complexity of the synthesis processes, etc. For these points, researchers have proposed different strategies to solve the related problems and have addressed the issues of the materials. To decrease the cost, researchers have tried to develop non-noble metal-based materials such as transition metal oxides, transition metal sulfides,8 transition metal phosphides and some carbon materials such as metal–graphite-based and MOF-based electrocatalysts.9–11 However, to date, due to the lack of noble metal active sites, the electrocatalytic performances of non-noble catalysts are not comparable to those of noble metal-based catalysts. To achieve high catalytic performance of catalysts, it is of paramount importance to focus on the reaction mechanisms to manipulate the adsorption energy of intermediates and pay attention to several aspects: (1) increasing the number of active sites, (2) enhancing the adsorption of reactants, (3) speeding up the desorption of products, and (4) dealing with the intermediates. Notably, these improvements are related to the materials' geometry, morphology, electronic structure, active sites on the surface, etc. To improve the long-term durability at the working potential, various morphologies have been designed to stabilize the materials' structures and reduce the aggregation of the nanoparticles during the electrochemical reactions.
Platinum-based materials are still state-of-the-art catalysts for the ORR, HER, and other electrocatalytic reactions. However, the high cost, scarcity, and poor impurity tolerance of Pt-based materials have stimulated the development of non-platinum electrocatalysts as alternatives,12 including other noble metals like Pd, non-noble metals, metal-free catalysts, etc. By comparing Pt and Pd, it can be concluded that these two noble metals have both commonality and unique characteristics. Both Pd and Pt are platinum group metals, with similar physical and chemical properties like stability in the air,5 the feasibility of forming alloys, and designability towards nanostructures. The similar electronic properties of Pd and Pt result in optimal adsorption energy with intermediates. Besides, many researchers combined Pd and Pt and used the synergistic effect to enhance the electrocatalytic activity.13–17 In terms of Pt-based catalysts, their availability is low, and stability is inferior due to the poor methanol tolerance and low resistance to other impurities.12 Compared with platinum, Pd is more abundant and historically the price of Pd was lower than that of Pt, although the price of Pd has become higher in recent years. The catalyst fabrication cost is also related to the loading mass of noble metals, and researchers utilized different strategies to address the cost issue like alloying with non-noble metals.13,18–20 These comparable properties of Pd make it attractive for high-performance electrocatalysis. Based on Pd, researchers can design different materials to meet different requirements for electrocatalytic functions. Pd-based materials have been used for not only ORR, HER, or OER single-functional electrocatalysis, but also multifunctional reactions like water splitting and in metal–air batteries. It's hard for Pd metal on its own to have all the advantages mentioned above including in terms of cost, performance, stability, and multifunctional applications, but there are some strategies to improve the overall performance, lower the cost, reduce the overpotential, increase the current density, and enhance the stability. For example, alloying with nonprecious metals, fabricating Pd single-atom catalysts, synthesizing composite materials, and constructing 2D nanomaterials21 are good ways to reduce the loading mass of Pd and improve the performance at the same time. Pd-based electrocatalysts are among the most important candidates in electrocatalysis but have received less attention. Inspired by the fast development of efficient strategies in the catalytic research field, in this review, the mechanism of electrocatalytic reactions, the challenges of Pd-based catalysts, and researchers' strategies and solutions to current problems are demonstrated and summarized.
2. Oxygen reduction reaction (ORR)
2.1 Challenges
The ORR is a sluggish reaction hindering the development of fuel cells. Many issues related to the ORR need to be solved or optimized, including the slow kinetics, inappropriate adsorption strength, corrosive environment for catalysts,22,23etc. On account of the complicated nanostructure of some novel materials, such as a composite consisting of graphene oxide and a Cu-centered MOF assembly,24 the sophisticated design enables excellent ORR performance, but it is usually accompanied by the destruction of the structure, dissolution of the metal elements, agglomeration of the nanoparticles, and leaching of the alloyed nanostructures, and all these effects will lead to performance degradation and shorter catalyst durability.25,26 Besides, adsorption strength also has a great influence on electrocatalysis. However, the weak adsorption strength between the reactants and the active sites will limit the initial mass transfer, thus decreasing the overall catalytic activity. If the adsorption strength between the products and the active sites is too strong, the molecules will poison the active sites and block further reactions on these active sites.27 Therefore, it is important to leverage the adsorption of the reactants and the desorption of the products, as well as the mass transfer of different intermediates at different reaction steps. The ORR has two reaction pathways including four-electron transfer and two-electron transfer. Four-electron transfer is highly desired aiming at a higher efficiency of the fuel cells' half reaction. However, in the practical working environment, the two pathways coexist so that the average electron transfer number is lower than four electrons.28 Therefore, improving the selectivity by the material's design is also a big challenge. Pd nanomaterials' conductivity also attract researchers' attention due to the poor intrinsic electrical conductivity, so how to choose supported carbon materials or other conductive substrates and how to grow Pd-based nanomaterials on them should be well addressed. For the above challenges, the design and synthesis of high-performance catalysts is critical though many strategies to solve these problems have already been proposed.
2.2 Strategies
2.2.1 Alloying design.
Alloying is a commonly used method to improve the catalytic activity and stabilize the materials for Pd-based catalysts.29 Among different alloying strategies, transition metals such as Fe, Ni, Co, etc. are utilized as alloying elements. Bimetallic compounds (e.g., Pd–Fe, Pd–Mo) formed by two transition metals benefit the catalytic activity of both metals, lower the price due to the addition of the cheap elements, and improve the durability of the unstable transition metals.30 Besides, alloying brings about some beneficial effects towards good ORR performance: (1) constructing the controllable morphology and specific geometry like curved bimetallene (Fig. 1c),31 (2) causing strain in the surface, (3) adjusting the electronic structure to fit the optimal oxygen binding energy, etc.32 M. Neergat et al. prepared Pd–Fe bimetallic compounds in a low-temperature synthesis route (Fig. 1b),33 and concluded that the low-temperature treatment maintained the size of PdFe bimetallic crystals without surface segregation, and the performance results showed a good methanol tolerance. This early work indicated the key points of alloy synthesis.
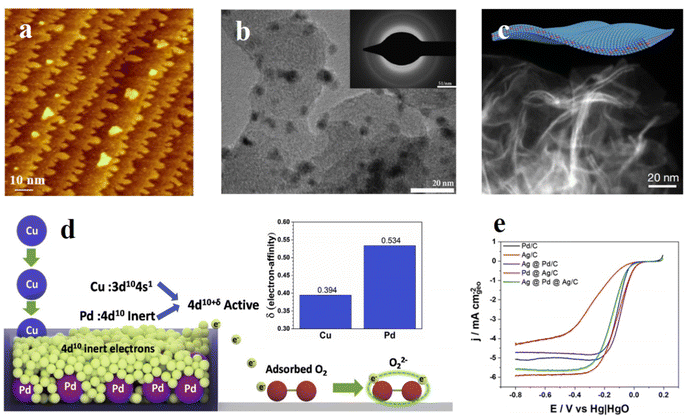 |
| Fig. 1 (a) STM image after deposition of 0.4 mL Au on Pd3Fe(111) at 300 K. Au films grew preferentially at step edges and formed 2-D nanoislands (∼5 nm) and nanogaps (∼2 nm). Image size: (100 × 100) nm2. Reproduced with permission.18 Copyright 2019, John Wiley and Sons. (b) TEM image of 20 wt% Pd3Fe/C. Inset shows the selected area diffraction pattern of Pd3Fe/C. Reproduced with permission.33 Copyright 2011, Elsevier. (c) TEM image of PdMo bimetallene. Reproduced with permission.31 Copyright 2019, Springer Nature. (d) Illustration of the active electron-affinity of Pd3Cu for boosting the alkaline ORR. Inset: Determination of electron-affinity of Pd and Cu. Reproduced with permission.19 Copyright 2019, Elsevier. (e) Electrochemical oxygen reduction reaction (ORR) activity comparison between Ag and Pd alloys and their pure constituents. Reproduced with permission.34 Copyright 2020, American Chemical Society. | |
Recently, Luis E. Betancourt et al. designed the interactions between Pd and Ag to synthesize the Pd–Ag alloy supported on carbon.34 The deposition of Pd tuned the electronic properties of the catalyst and increased the ORR performance to twice that of Pd/C (Fig. 1e). The Pd in the alloy accelerated the adsorption of reactants and the Ag facilitated the desorption step of the products, demonstrating a unique collaboration of the bimetallic alloys for the ORR. In order to investigate the original influences of different Pd-based bimetallic alloys, Georgios Bampos et al.20 researched several kinds of alloys like Pd–Cu, Pd–Ag, Pd–Ca, and Pd–Ni synthesized by the wet impregnation method and compared their intrinsic ORR activities. Based on electrochemical tests and various characterization techniques, Pd–Cu achieved the best performance due to the optimal Pd lattice strain among all the alloys. In another study, Tong Wu et al.19 applied DFT (Density Functional Theory) calculations to study the intrinsic properties of Pd3Cu and tried to explain the excellent balance between activity and durability (Fig. 1d). By constructing a possible mechanism for the reaction occurring on the Pd3Cu surface, the researchers analyzed the adsorption strengths of reaction intermediates and the electron-affinity properties, thus developing the application of d-band center theory for the explanation of ORR activity.
However, problems of catalytic activity and stability still exist in binary alloys. Although the two active metals provide optimal electronic properties, oxygen binding energy, and super good performance, they cannot remain stable under the working environment like an alkaline medium due to the high activity.35 To solve the problems existing in binary alloy catalysts, researchers have started to try to add more components in the alloy compound, like in ternary alloys. Xiaofang Yang et al.18 used inactive bulk gold to cover the surface of the Pd–Fe alloy catalyst and the results showed a large improvement of stability (Fig. 1a). In this compound, the Pd–Fe part exhibited highly active ORR performance and Au served as an electronic modifier and structural stabilizer. This balancing strategy took advantage of the different metals' properties and verified the feasibility of ternary alloy catalysts. Xian Jiang et al.36 grew PdPb bimetallic alloy nanolayers on Au nanowires to fabricate a trimetallic catalyst for highly active ORR. The core–shell structure of Au@PdPb and the anisotropic one-dimensional nanowire morphology greatly increased the utilization efficiency of noble metal atoms, exhibiting enhanced ORR activity. Compared to a zero-dimensional nanostructure, the anisotropic one-dimensional nanowires could prevent aggregation and dissolution, thus improving the ORR stability. Qiuyan Chen et al.13 designed core–shell nanosheets with Pd, Pt, and Ni, and tuned the shell thickness to optimize the electrocatalytic performance. Combining the core–shell morphology and ultra-thin nanosheet structure, this ternary alloy exhibited promising ORR performance compared with commercial Pt/C. However, the material becomes more complex with the additional element and it's hard to predict and control the morphology and structure of ternary alloys. Mashu Torihata et al.14 studied the surface structure and composition of the Pt–Pd–Co ternary alloy; they fabricated several composition ratio compounds and compared several surface planes, and showed that the low index planes of the Pt–Pd–Co ternary alloy had higher activity compared with Pt's corresponding planes. With more elements in the material synthesis, it's hard to control the catalytic properties of the prepared samples. Dinesh Bhalothia et al.15 carefully studied the effect of the third metal Pt in the ternary alloy NiPdPt nanoparticles and showed that the adsorption time and content of Pt had a severe influence on the atomic arrangement and charge density distribution of the final ternary alloy nanoparticles. In the presence of atomic Pt clusters in the surface, the defect active sites were well protected, thus enhancing the overall ORR performance.
The alloying strategy with Pd and non-noble metals indeed provides superior catalytic performance and reduces the cost. However, the reduced atomic percentage of Pd leads to limited durability under harsh operating conditions, especially when the atomic percentage of Pd is lower than 50%.37 To solve this problem, Zhi-Peng Wu et al.37 developed an alloying–realloying strategy to address the issue of Pd-based alloys' durability. Researchers synthesized PtPdM (M = Cu, Ni or Co) ternary alloys and studied their durability issues by in situ high-energy synchrotron XRD. Results showed that the dealloying–realloying cycles played an important role in the overall stability. The realloying process occurred in the compression-strained single-phase alloy state and helped with the “self-healing” of the material. Inside the ternary compositions, Pd could decrease the dealloying degree and facilitate the realloying process, thus improving the durability in the alloying–realloying strategy.
2.2.2 Size effect.
The size effect, changing from nanoparticles to nanoclusters, then to a single/dual-atom scale, plays a critical role in the nanomaterials' physicochemical properties. Pd nanoclusters could provide extra active sites based on the transition metal catalysts. Xiaoqian Wei et al.38 utilized the electronic interaction between Pd nanoclusters and Fe single atoms, triggering the Fe single atom's spin-state to change to an intermediate spin. The change of spin state enhanced the ORR dissociative pathway and improved the catalytic activity. This atomic-scale mechanism study provided researchers with new insights into the spin-regulation strategy. H. Cruz-Martínez et al.39 applied auxiliary density functional theory to study the ORR activity of the Pd-based core–shell clusters, including Pd nanoclusters, M@Pd bimetallic nanoclusters, and M@PdPt trimetallic nanoclusters. Researchers computed the adsorption energies of some intermediates (O, OH) as predictors to evaluate the ORR activity and investigated the catalytic trends of these Pd-based nanoclusters. From the theoretical viewpoint, the conclusion of the activity trends was M6@Pd30Pt8 > M6@Pd38 > Pd44 and the stability of these nanoclusters was further studied in a vacuum and an oxidizing environment.
To maximize the utilization of precious metals, fabricating single-atom catalysts (SACs) is an efficient method to fully make use of the active sites and achieve 100% atom efficiency.40 In traditional bulk Pd materials, waste of inner precious metals greatly increases the cost, while palladium single atoms supported on some carbon materials could maximize the benefits. Hee-Eun Kim et al.41 synthesized Pd single atoms supported on graphitic carbon nitride with carbon black and this catalyst showed high activity and selectivity towards two-electron transfer ORR. Due to the abundant N-anchoring sites in the carbon nitride, researchers used the quite simple impregnation method to immobilize the Pd single atoms into the sites without forming Pd nanoparticles. This work showed the advantages of SACs: low cost, simple synthesis, high activity, and high selectivity, and many studies confirmed these features. Nan Wang et al.40 synthesized a Pd-doped zeolitic imidazolate framework material for H2O2 generation (Fig. 2c). Similar to the Pd single atoms immobilized on the graphitic carbon material, this catalyst is also an N-coordinated single-atom Pd material. Based on DFT calculations, the researcher pointed out that the Pd single-atom sites in the form of Pd–N4 preferred breaking the bonds between oxygen atoms and active sites, resulting in the high selectivity of two-electron transfer during the ORR process. The fabrication of SACs depends on different transition metals as active sites and various carbon materials as the conductive supporting base. Kun Jiang et al.42 fabricated transition metal single atoms immobilized on carbon nanotubes and achieved flexible control towards oxygen reduction pathways. The transition metal SACs in the work like Fe–C–O single atom catalysts tuned the product selectivity and brought inspiration and guidance towards other metals like Pd. Similar to N-coordinated Pd SACs, some researchers focused on carbon-coordinated SACs, like defective graphene. Zengxi Wei et al.43 found a new Pd SAC (C4Pd) and analyzed the mechanism behind the high activity and selectivity for two-electron transfer to produce H2O2 (Fig. 2d). As a reference, they also fabricated Pd clusters immobilized on the defective graphene with the formula C4Pdx (x = 2, 3, 4, 5), which exhibited worse performance than the single atom catalyst (Fig. 2e). Considering their multifunctional nature, SACs are still competitive. In order to find the trifunctional catalysts for the ORR, OER and HER, Zhanzhao Fu et al.44 took advantage of single-layer Ti3C2 and fabricated Pd SACs on Ti3C2O2 (Fig. 2b). The combination of Ti3C2's intrinsic high activity for the HER and traditional ORR and OER active centers of the Pd metal successfully constructed the highly active trifunctional catalyst Pd1@Ti3C2O2. The interaction between these two parts could change the d-band center of Pd atoms to an optimal position and improve the catalytic performance. As for the stability, the study demonstrated that the aggregation of Pd single atoms into small clusters could not influence the activity.
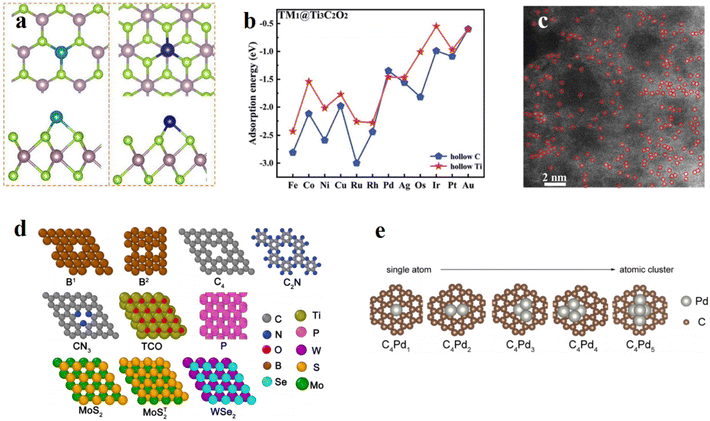 |
| Fig. 2 (a) The obtained lowest-energy configurations for a single TM atom anchored on 2H- and 1T-MoSe2 monolayers. Reproduced with permission.45 Copyright 2022, Elsevier. (b) Adsorption energies of different TM atoms on the hollow Ti (red stars) and hollow C (blue pentagons) sites of Ti3C2O2. Reproduced with permission.44 Copyright 2020, Royal Society of Chemistry. (c) Structural characterization for Pd0.157-NC, showing the configuration of Pd single atoms in Pd-NC. Reproduced with permission.40 Copyright 2022, American Chemical Society. (d) Proposed 2D materials with different binding environments (B, C, N, O, P, S, Se) for one single Pd atom. Reproduced with permission.43 Copyright 2022, Elsevier. (e) Optimized structures of C4Pdx (x = 1, 2, 3, 4, 5). Reproduced with permission.43 Copyright 2022, Elsevier. | |
Besides using one kind of transition metal as the active sites, distributing two or more kinds of elements as single atoms in carbon materials is also a feasible strategy. Matthew T. Darby et al.46 reported single-atom alloys (SAAs) for ORR electrocatalysis. Through DFT calculations, the SAAs exhibited excellent activity and durability like high tolerance to CO poisoning due to the C–C coupling and C–H activation reactions. Among 10 kinds of SAAs, doped metals like Pd, Pt, Rh greatly improved the activity of the host metals like Au and Ag, demonstrating the SAAs enhanced ORR performance over the corresponding monometallic SACs.
Transition metal dichalcogenide (TMD) nanosheets provide new ideas for the choice of supporting 2D nanostructures. TMD nanosheets are promising for anchoring metal single atoms due to their low cost and good stability. Zengming Qin et al.45 stabilized various transition metals on the TMD MoSe2 (Fig. 2a) and compared their overpotentials during the ORR and OER processes (Fig. 6b). Ni@1T-MoSe2 had the lowest OER overpotential while Pd@1T-MoSe2 achieved the best performance comprehensively.
Dual-atom catalysts are also promising for high atom-utilization efficiency.47,48 Compared with single-atom catalysts, they could supply more active sites for reactants and intermediates, especially some necessary bridge adsorption. Xuewei Wang et al.49 engineered both a single-Pd-site catalyst and dual-Pd-site catalyst with different coordination environments, and compared the adsorption characteristics. Specifically, researchers tried C, B, and N as coordination environments, and evaluated their influences on the adsorption of the reactant (O2) and intermediates. The results showed that the removal of *OH should be the potential determining step for the B coordination environment, while the formation of *OOH should be the potential determining step for C and N coordination environments. From the perspective of mechanism, the Pd2B6/C material could facilitate O
O cleavage, thus enhancing both associative and dissociative pathways.
2.2.3 Atomic arrangement design.
Based on the number of electrons transferred in the ORR process, the reaction pathway can be divided into a four-electron transfer pathway and two-electron transfer pathway, with final products of water or hydroxide ions, and hydrogen peroxide molecules or peroxide ions, respectively. The indispensable step of the four-electron transfer pathway is O
O cleavage, preventing the formation of hydrogen peroxide molecules.50 Additionally, hydrogen peroxide molecules can be further reduced by electrons to achieve the four-electron transfer pathway, and also high pH can lead to its decomposition. Therefore, the selectivity of these two pathways is sensitive to the active sites of materials' structures, the chemical composition of the catalysts, and the electrochemical environment. To design highly selective 2e− ORR catalysts, Juyeon Lee et al.50 applied DFT calculations to find suitable materials and fabricated Pd–Se–B nanocorals, which showed excellent 2e− ORR activity and selectivity in near-neutral electrolyte. The synthesis strategy is to control the atomic arrangement by inserting some less catalytically active sites such as Se, Hg, and Au. These kinds of atoms can serve as interatomic spacers to increase the distance between two Pd atoms and eliminate the ensemble sites, thus preventing the cleavage of O
O and generating hydrogen peroxide molecules. Besides, A. Verdaguer-Casadevall et al.51 studied the factors for 2e− ORR selectivity and synthesized Ag–Hg and Pd–Hg electrocatalysts for H2O2 production. Based on mechanism research and DFT calculations, researchers concluded that the highest activity for H2O2 production should be achieved with moderate interaction with intermediates, and also the isolated active sites cannot break the O
O bond, resulting in high selectivity for H2O2 production.
2.2.4 Functionalized composite design.
Functionalized hybrid materials composed of metals and other materials like carbide, nitride, and polymers are promising for electrocatalysis due to the simple synthesis routes and relatively low cost.52,53 Researchers could design the morphology of different materials separately and think about the combination strategy, thus fully utilizing the advantages of the hybrid materials' different parts and their interactions. Recently, Hongjing Wang et al.54 prepared polyaniline-functionalized porous Pd metallene (Fig. 3a) and used it for a long-term ORR test. The ultrathin porous Pd nanosheets could provide extensive catalytic active sites due to the high ratio of surface area to bulk volume, while the polyaniline could provide high conductivity, excellent hydrophilicity and relatively good stability. As mentioned above, the interactions between different parts of hybrid materials could be beneficial to the whole catalytic performance (Fig. 3b). The results revealed that the π-conjugated ligand in the polyaniline would modify the d-band center and electronic structure. In order to investigate the specific function of polyaniline in the Pd-based nanocomposites, Mphoma S. Matseke et al.55 synthesized Pd/PANI/C (carbon black) composite materials for the electrocatalytic ORR. Based on the TEM images, the sample with polyaniline showed a more even distribution of the Pd nanoparticles on the surface than the traditional Pd/carbon black catalysts. The stability test demonstrated that the incorporation of polyaniline could improve the durability due to the alleviation of the Pd nanoparticles' agglomeration. Besides, the properties of polyaniline have been studied comprehensively like the relationship between the catalytic activity and the thickness of the polyaniline shell.56 Researchers have broadened their horizons to extensive carbon material supports. To utilize nitrogen-doped reduced graphene oxide, Ammara Ejaz et al.57 reported an efficient method to fabricate a Pd@nitrogen-doped reduced graphene oxide nanocomposite, with various inner interactions among the nitrogen, oxygenated functionalities of graphene oxide and Pd nanoparticles. Due to the complex coordination environment of the nitrogen, researchers investigated the distinct role of pyrrolic, pyridinic and graphitic nitrogen. The results showed that the type of nitrogen influenced the size and distribution of the Pd nanoparticles.
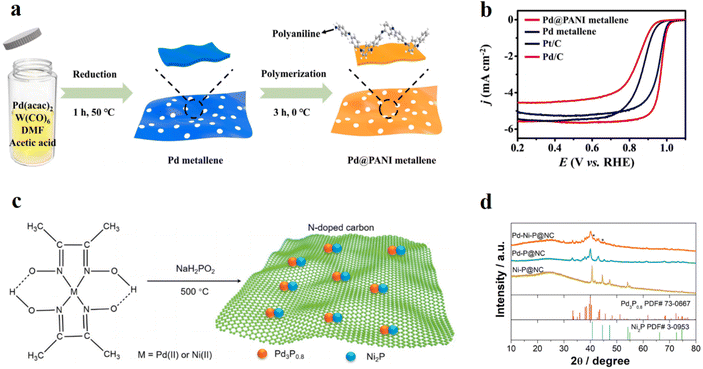 |
| Fig. 3 (a) The synthetic scheme of Pd@PANI metallene. Reproduced with permission.54 Copyright 2022, Elsevier. (b) ORR polarization curves in an O2-saturated 0.1 M KOH solution with a rotation rate of 1600 rpm at a scan speed of 10 mV s−1. Reproduced with permission.54 Copyright 2022, Elsevier. (c) Schematic illustration for the synthesis of Pd–Ni–P@NC by reacting Pd(II)/Ni(II)-dimethylglyoxime with NaH2PO2. Reproduced with permission.58 Copyright 2019, Elsevier. (d) XRD patterns of Ni–P@NC, Pd–P@NC, and Pd–Ni–P@NC. Reproduced with permission.58 Copyright 2019, Elsevier. | |
Metal phosphide is another choice because of the high mass activity and specific activity resulting from enhanced charge transfer and electronic modification.59 Xue Dong et al.58 studied a bimetallic phosphide supported on a nitrogen doped carbon material and investigated the effects of the interfacial coupling interaction of metal phosphides (Fig. 3c and d). The incorporation of the palladium phosphide and the nickel phosphide produced synergistic effects which optimized the electronic structure. By using dimethylglyoxime, the nitrogen doped carbon material was easily synthesized during the pyrolysis while the phosphating process occurred in the same step. Electroless deposition is another effective method to synthesize hybrid materials. Barun Kumar Barman et al.60 coated Pd on a prepared Ru-based nitrogen-doped graphene nanocomposite by galvanic electroless deposition. In detail, some Ru atoms were replaced by Pd atoms during the galvanic replacement, and it constructed more catalytic active sites for good ORR and HER performance. Compared with Pt coated samples, the Pd–Ru@NG showed better HER activity due to the formation of PdHx in the solution and better ORR stability due to the promising alcohol tolerance. Marta Nunes et al.61 tried to investigate the loading effect of noble metals supported on high surface area graphite (HSAG) and the results showed that the addition of Pd into Cu/HSAG could increase the electrocatalytic activity no matter how small the amount of the Pd added.
Pd-based hybrid materials can also be used for an H2O2-generating two-electron pathway. A. P. Reyes-Cruzaley et al.62 designed a novel hybrid material named Pd NP-PTH-CNTs for two-electron transfer ORR. By incorporating polythiophene (PTH) onto the carbon nanotubes (CNTs), researchers greatly increased the conductivity of CNTs, and at the same time, the PTH served as a reducing and stabilizing agent to assist in synthesizing the Pd nanoparticles in a moderate way. Furthermore, the H2O2 selectivity could be enhanced by the size control and the distribution position of Pd nanoparticles.
Synthesizing palladium-MOF/ZIF-based materials provides an idea for fabricating low-cost catalysts. At the same time, the transition metals perform quite well in electrocatalysis. Therefore, combining these two materials to fabricate composite electrocatalysts could be a feasible strategy. The synthesis of composite materials not only decreases the whole cost, but also provides more active sites both in number and variety. Here, “MOF/ZIF” means that they are used as precursors but there will be pyrolysis or carbonization or phosphating or vulcanization later, based on the particular design and requirements. Zelin Cui et al.63 synthesized Fe/Co/N doped carbon to support 40% wt palladium nanoparticles. As aforementioned, the transition metal-based materials already show good performance towards electrocatalysis, and here the FeCo alloy on the carbon material works as active sites for the ORR. Without the addition of palladium, the component ratio of the carbon material from the pyrolysis of bimetallic ZIFs can be adjusted to achieve the best ORR performance. Then, slightly oxidized palladium nanoparticles were introduced into the FeCo-based carbon materials to finish designing the palladium-based catalyst. The transition metals on it promote the oxygen activation process and prevent the agglomeration of nanoparticles.
Pd-based materials are also promising choices for multifunctional electrolysis due to their electronic structures and combining properties with other materials. Noble metals like platinum and palladium are excellent catalysts for the ORR and HER and they could show good intrinsic properties towards these two electrocatalytic processes, while the transition metal oxides possess intrinsic activity towards OER electrocatalysis. In order to fabricate trifunctional electrocatalysts, combining noble metal materials with other OER catalytic materials can be a practical approach. Recently, L. Karuppasamy et al.64 reported a kind of multifunctional electrocatalyst for the ORR, OER and HER which shows competitive electrocatalytic performances (0.90 V half-wave potential for the ORR, 65 mV overpotential at 10 mA cm−2 for the HER and 180 mV overpotential at 10 mA cm−2 for the OER) and good stability. The researchers synthesized a defect-enriched N–MoO2–Mo2C heterointerface supported Pd nanocomposite keeping the following design ideas in mind. The unique icosahedral palladium structure with suitable crystal defects improves the adsorption properties; the presence of MoO2 and Mo2C increases the conductivity of the whole material and reduces the OH formation on active sites resulting in quite good durability. In the accelerated durability test (ADT), the half-wave potential only moves towards a negative potential of 24.9 mV. Taeoh Kang et al.65 reported another multifunctional composite material, Pd/CoOx/d-NC, for the ORR and OER. The core design idea of this composite material is that when combining two parts of materials, where the first part is beneficial for the ORR and the second part is beneficial for the OER, there are some synergistic effects, leading to the result that one plus one is greater than two. For this material, the palladium nanoparticles as active sites are responsible for the main performance of the ORR, while the defect-rich N-doped carbon improves the ORR activity. The CoOx with oxygen vacancies improve the adsorption of oxygen species, with the electron-deficient palladium structure achieving quite good OER performance.
3. Oxygen evolution reaction (OER)
3.1 Challenges
The OER is a half-cell reaction of water splitting. Compared with the other half-cell reaction, HER (a two-electron transfer process), the OER is more sluggish in terms of the kinetics due to the four-electron transfer.66 The sluggish kinetics means that a large overpotential should be applied and more energy should be consumed.67 Currently, RuO2/C and IrO2/C are commercial catalysts for the OER.68 But the high mass ratio of precious metals leads to the high cost of these commercial catalysts, which is not sustainable due to the low natural reserves of Ru and Ir. Ideal electrocatalysts require lower fabrication cost and relatively fast kinetics, so researchers always tried to design and synthesize alternative materials for RuO2/C and IrO2/C. For the OER catalytic performance, both number and activity of the active sites are critical, influenced by the intrinsic properties of the materials, nanostructures and the surface area.69 Therefore, facing the above problems, it's necessary to design and fabricate a less expensive and more efficient catalyst to promote the overall electricity-driven water splitting.
3.2 Hybrid material design strategies
Alloying is an excellent strategy for efficient and durable catalysts of the OER. Zafar Khan Ghouri et al.70 synthesized PdCe alloy nanoparticles supported on carbon nanowires for the OER (Fig. 4a and b). Due to the large number of active sites and low charge transfer resistance, the cooperative effect of bimetallic nanoparticles improved the catalytic activity and selectivity (Fig. 4c).70 In addition, this kind of cooperative effect could enhance the chemisorption efficiency of oxygen-containing species and the 3D structure of the nanowires could improve the electrochemical surface area. The palladium in the catalyst could offer active centers by an electron inductive effect. Tao Zhang et al.71 tuned the nanostructure of an Ir–Pd alloy with three shapes, including hollow spheres, wires, and tetrahedrons, and investigated the OER performance of these nanoparticles (Fig. 4e). Among the three microstructures, the nanowires and nanotetrahedrons exhibited excellent OER performance whose mass activity was five times that of the commercial Ir/C catalyst. Palladium oxide-based nanomaterials also exhibit great potential for the OER. G. M. Salvador et al.72 replaced the Mn3+ of α-MnO2 by Pd4+ due to the similar radius of Mn3+ and Pd4+, and synthesized Pd/α-MnO2 for the OER (Fig. 4d). The PdO in the surface of the catalysts provided active sites and enhanced the overall OER performance compared with pure α-MnO2, indicating the successful Pd-doping strategy.
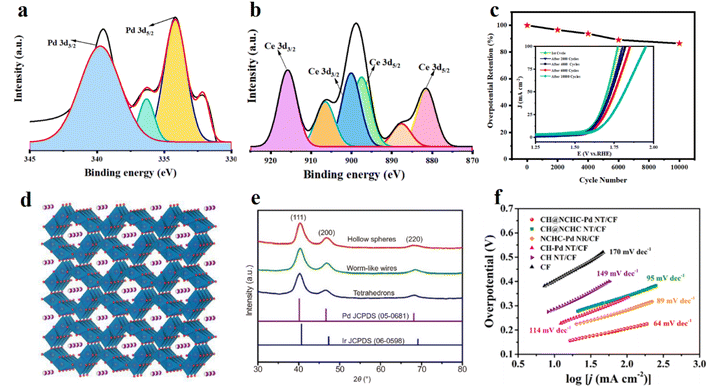 |
| Fig. 4 (a) XPS Pd 3d spectra and (b) XPS Ce 3d spectra for a synthesized PdCe/CNWs nanocomposite. Reproduced with permission.70 Copyright 2022, Elsevier. (c) Overpotential retention as a function of LSV cycle number and (inset) corresponding LSV cycles for synthesized PdCe/CNWs electrodes. Reproduced with permission.70 Copyright 2022, Elsevier. (d) Framework lattice crystal structure of α-MnO2. Reproduced with permission.72 Copyright 2021, Elsevier. (e) XRD patterns of as-synthesized Ir–Pd alloy nanocrystals. Reproduced with permission.71 Copyright 2018, Springer Nature. (f) Tafel plots of developed catalysts in 1 M KOH. Reproduced with permission.77 Copyright 2019, American Chemical Society. | |
Nanocluster engineering is also an effective way to fabricate active and durable OER electrocatalysts. R. Manjunatha et al.73 synthesized a nitrogen-doped carbon nanotube supported Co–Fe nanocomposite and decorated Pd nanoclusters to further improve the electrocatalytic performance. The Pd nanocluster-coated Co–Fe–NCNTs exhibited superior trifunctional electrocatalytic activity for the ORR, OER, and HER. Researchers fabricated a water electrolysis cell and rechargeable zinc–air battery with this catalyst, which displayed comparable performance with commercial catalysts. Fan He et al.74 synthesized Pd nanoclusters immobilized on MoS2 monolayers to explore the size effect of a nanocluster. The defective MoS2 monolayers could provide extensive catalytic active sites and the interaction between Pd nanoclusters and the monolayers led to high stability. The diatomic Pd nanoclusters (Pd2) showed the best performance for the OER while the Pd6 nanoclusters exhibited the best performance for the ORR.
Dual-atom catalysts (DACs) have also attracted extensive attention in recent years and DACs can provide more flexible active sites than single-atom catalysts. Mengjie Huang et al.48 calculated the ORR/OER activity and stability of C2N-supported dual-atom catalysts (DACs), including homonuclear DACs like Pd2–C2N, Pt2–C2N, and heteronuclear DACs like PdPt–C2N, GaPd–C2N, and PdRh–C2N. The results showed that the heteronuclear PdRh–C2N and GaPd–C2N catalysts exhibited better OER and ORR catalytic performance compared with their homonuclear counterparts, respectively. Also, the PdPt–C2N catalyst showed potential for bifunctional OER/ORR catalytic performance.
Composite nanomaterials are promising for enhancing the OER performance.75 The design of nanocomposite materials has been widely developed, including core–shell structure-based nanocomposites, multidimensional nanocomposites consisting of 3D and 2D blocks,76etc. Jiahui Kang et al.77 designed a quite complex nanomaterial named “Pd nanoparticle-interspersed nickel cobalt hydroxide carbonate nanothorn-coated copper hydroxide nanotube arrays grown on a copper foam” and it exhibited quite high OER performance due to the large surface area, the increased number of active sites and the fast electron and mass transfer (Fig. 4f). This Pd nanoparticle and core–shell carbon composite material possessed interfacial interaction resulting in optimized electronic structure, leading to enhanced OER activity.
3.3 Multifunctional OER/ORR catalyst design
The demand for bifunctional electrocatalysts for the OER and ORR greatly increased with the development of rechargeable metal–air batteries like zinc–air batteries.78–80 However, it is hard for them to possess both excellent OER and ORR catalytic activity at the same time. For example, Pt/C is a commercial catalyst applied in the ORR, and RuO2 and IrO2 are recognized as the best catalysts for the OER, but they can only serve as single functional catalysts.81 As substitutes for Pt metal, many Pd-based catalysts are developed for the ORR. Due to the oxide materials' promising activity for the OER, designing Pd metal oxide catalysts or Pd-based transition-metal oxides can be a promising way to develop bifunctional electrocatalysts. Hong-Chao Li et al.9 applied a two-step carbonization strategy based on a Pd-incorporating ZIF material to form structural nanocubes (Fig. 5c). This catalyst showed a better catalytic performance for the OER and ORR than the commercial RuO2 and the commercial Pt/C, respectively. In the OER working environment, CoOOH formed in the surface of the nanocubes, preventing further oxidation of the whole material, and served as OER active sites simultaneously (Fig. 6a). To increase the electronic conductivity, researchers chose to make the conducting carbon materials derived from ZIF the base and also to maintain the porous morphology of the initial framework. Jia-Xing Guo et al.82 studied comprehensively the bifunctional catalysis of single precious metals (including Pd, Pt, and Ir) anchored on Janus MoSSe monolayers (Fig. 5b). Based on the analysis of the reaction mechanism, the intermediate form and the adsorption behaviors, the Pd and Pt single atom samples exhibited better OER and ORR activity compared with the Ir sample. Researchers pointed out that the built-in electric field of the Janus MoSSe monolayer structure could be one of the reasons for the excellent OER performance. Xiaomeng Liu et al.83 prepared heterostructured Pd/Pd4S supported on hollow carbon spheres for efficient bifunctional electrocatalysis (Fig. 5d). The porous morphology provided an extremely large surface area and abundant transportation channels for reactants and intermediates, while the interfacial engineering of Pd/Pd4S provided excellent intrinsic catalytic activity. The synergistic effect of the two parts enhanced the catalytic performance and the application for the lithium oxygen battery showed quite good specific capacities. Mi Young Oh et al.84 aimed to use Pd-based perovskite oxide materials to fabricate a lithium oxygen battery and synthesized a nanostructured La(Sr)Fe(Co)O3−δ@Pd matrix (Fig. 5a). The combination of Pd nanoparticles' ORR activity and perovskite oxides' OER activity enabled the superior charge–discharge capacity and cycling performance of the lithium oxygen battery.
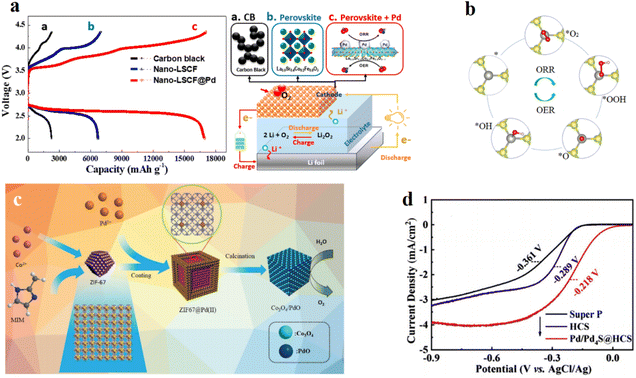 |
| Fig. 5 (a) Electrochemical performances of Li–O2 batteries: the initial discharge profiles of (a) carbon black, (b) Nano-LSCF, and (c) Nano-LSCF@Pd as air cathode materials in the potential range of 2.0–4.35 V vs. Li/Li+ at a current density of 200 mA g−1. Schematic illustration comparing different cathode catalysts: (a) carbon black, (b) Nano-LSCF, and (c) Nano-LSCF@Pd (right). Reproduced with permission.84 Copyright 2019, American Chemical Society. (b) Reaction schemes of the ORR and OER processes on Pd- and Pt-MoSSe. Reproduced with permission.82 Copyright 2022, Elsevier. (c) Schematic illustration of the synthesis of the Pd@PdO–Co3O4 nanocubes. Reproduced with permission.9 Copyright 2018, John Wiley and Sons. (d) LSV curves for the ORR of Super P, HCS, and Pd/Pd4S@HCS. Reproduced with permission.83 Copyright 2021, Elsevier. | |
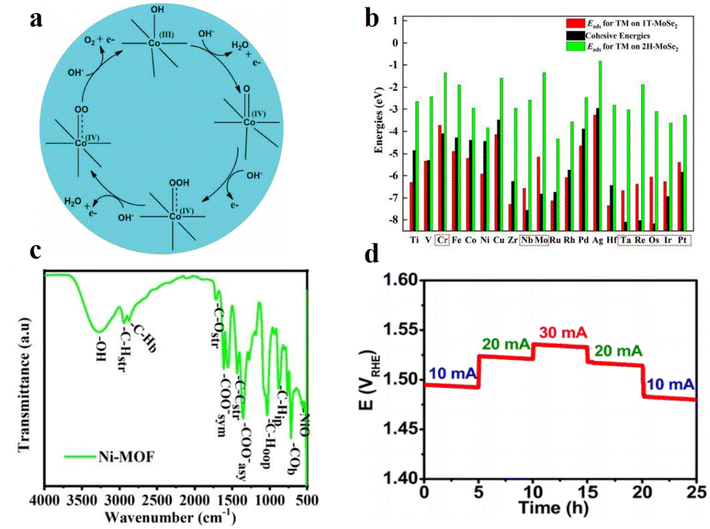 |
| Fig. 6 (a) Catalytic cycle of Co during the water oxidation process. Reproduced with permission.9 Copyright 2018, John Wiley and Sons. (b) Adsorption energies (Eads) and cohesive energies for a single TM atom anchored on 2H- and 1T-MoSe2 monolayers. Reproduced with permission.45 Copyright 2022, Elsevier. (c) FTIR spectrum of Ni-MOF. Reproduced with permission.88 Copyright 2020, John Wiley and Sons. (d) Chronopotentiometric stability measurements of an NComp-3-based electrolyzer for 25 h. Reproduced with permission.89 Copyright 2021, American Chemical Society. | |
3.4 Multifunctional OER/HER catalyst design
Water-splitting bifunctional electrocatalysts have also attracted researchers' attention.85,86 Among numerous catalysts, MOF-based materials are desirable multifunctional electrocatalysts due to the intrinsic properties of well-defined compositions, tunable morphology, porous structures, and high surface area.87 Adewale K. Ipadeola et al.88 developed bimetallic Pd/Ni nanocrystals on MOF-derived carbon materials for water-splitting, including OER and HER electrocatalysis (Fig. 6c). The researchers treated the MOF template with chemical etching and compared the sample with and without the etching process. The results showed that the untreated sample performed better due to the superior conductivity. MOF derived carbon materials have promising properties for achieving high catalytic activity resulting from the tunable porosity and high surface area. The metal oxides have quite good intrinsic OER activity and based on this, Papri Mondal et al.89 studied metal permanganate materials. After the compositing and doping process, researchers fabricated silver permanganate modified by both metallic and oxidized Pd (Pd0 and Pdδ+). Owing to the synergistic effect of the different parts of the nanocomposites (i.e., silver permanganate, metallic Pd, and oxidized Pd), the adsorption ability, charge transport and long-term durability improved remarkably (Fig. 6d). Researchers also investigated the influence of oxidation states of oxidized Pd species on the bifunctional catalytic properties and the mixed-valence Pd showed the best performance. Xiao Zhang et al.90 developed bimetallic nanoparticles doped in carbon nanotubes for trifunctional (OER, HER, and ORR) electrocatalysis. Based on calcination and reducing agent treatment, researchers immobilized the Ni and Pd nanoparticles into CNTs with sizes of 1 nm and 2–5 nm, respectively. This accurate control of size and distribution of the metal nanoparticles successfully enhanced the catalytic activity in the acidic medium.
4. Hydrogen evolution reaction (HER)
4.1 Challenges
Hydrogen fuel, with no carbon and high enthalpy of combustion, is considered to be an alternative clean fuel for the future.91 In order to take advantage of hydrogen fuel, hydrogen production technologies have been widely researched and the electrocatalytic hydrogen evolution reaction is one of the most promising implementations.92 The cost of materials, the electrocatalytic performance and the long-term stability are the most significant parameters used to evaluate catalysts. Pt-based materials are known to be the most efficient HER catalysts but the high cost, poor tolerance towards products and the scarcity of Pt have restricted the development of the HER.93 Besides, the catalytic performance varies in different pH media and adsorption energy analysis of different reactants is needed for acidic or alkaline medium. Therefore, fabricating low-cost electrocatalysts for highly active and stable HER electrocatalysis is urgent for researchers.
4.2 Design strategies
4.2.1 Interface engineering.
Interface engineering is a good method to provide more highly active reaction sites for the hydrogen evolution reaction and enhance the performance.94 Palladium nanoparticles could serve as active sites in the surface of HER electrocatalysts. Yang-Yi Liu et al.94 constructed perfect interfaces between Pd–Ag and carbon/nitrogen (C/N) materials by controlling the fabrication temperature and successfully synthesized a Pd-doped core–shell nanowire structure (Fig. 7a). This interface controlling strategy could provide more active sites in the surface and enhance the adsorption, activation, and conversion of the reactants and intermediates to the products. Following this initial idea, the researchers chose the noble metal alloy–carbon material interface system. Based on ratio adjustment of Pd and Ag, the catalysts exhibited extremely high performance with a low Tafel slope of 64 mV per decade. Besides the 3D nanostructure, the 2D nanosheet-based interface is also a good choice. Jin Li et al.95 atomically dispersed Pd on MoO3 nanosheets to form a Pd@MoO3 heterostructure and interface (Fig. 8a and b). This catalyst performed very well in acidic medium with a quite low overpotential and Tafel slope value. The well-designed interface enhanced ion/electron transfer in the surface and increased the catalysis rate. Based on the self-assembly-directed synthesis method, the researchers achieved the fabrication of an efficient and large-size electrocatalyst.
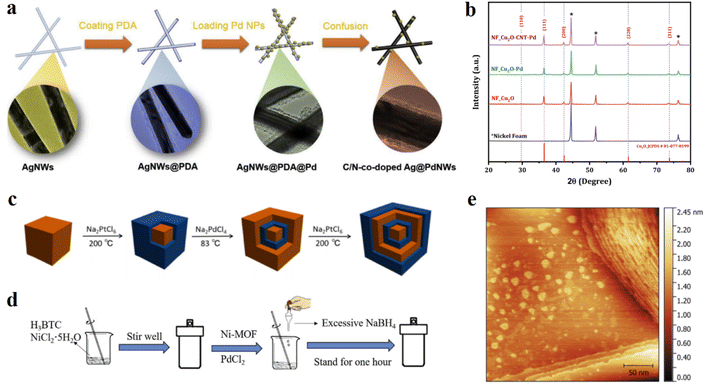 |
| Fig. 7 (a) Schematic illustration of the preparation of C/N-co-doped Ag@Pd NWs. Reproduced with permission.94 Copyright 2018, Elsevier. (b) X-ray diffraction patterns of NF_Cu2O, NF_Cu2O–Pd, and NF_Cu2O-CNT–Pd samples. Reproduced with permission.103 Copyright 2021, Elsevier. (c) Schematic illustration of the transformation from cubic Pd to the final Pd–Pt triple-shelled nanocube via a layer-by-layer epitaxial approach. Blue = Pt, brown = Pd. Reproduced with permission.16 Copyright 2019, Springer Nature. (d) Schematic for the preparation of a Pd/Ni alloy. Reproduced with permission.104 Copyright 2020, Elsevier. (e) Example STM image of a Au(111) surface after Pd displacement of Cu UPD (10 s UPD duration at 0.47 V vs. SHE). Reproduced with permission.98 Copyright 2021, Elsevier. | |
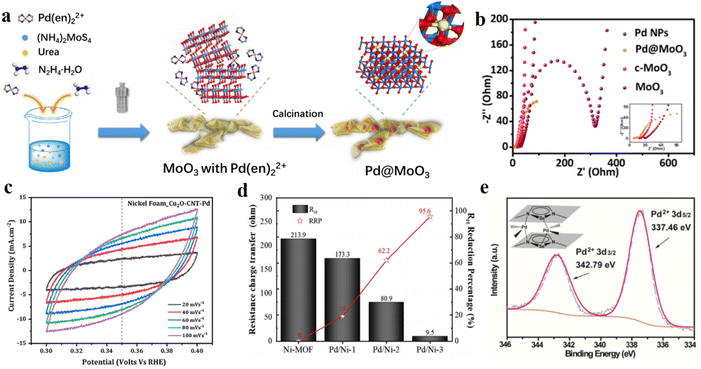 |
| Fig. 8 (a) Synthetic scheme of the Pd@MoO3 heterostructure. Reproduced with permission.95 Copyright 2019, American Chemical Society. (b) Nyquist plots of Pd@MoO3, c-MoO3, MoO3, Pd NPs, and Pt/C. Reproduced with permission.95 Copyright 2019, American Chemical Society. (c) Cyclic voltammetry curves in a non-faradaic region at scan rates of 20, 40, 60, 80, and 100 mV s−1 for NF_Cu2O–CNT–Pd samples. Reproduced with permission.103 Copyright 2021, Elsevier. (d) Comparison of Rct and reduction percentage based on Ni-MOF. Reproduced with permission.104 Copyright 2020, Elsevier. (e) XPS spectrum of Pd 3d in PY–SE–COF–Pd and inset shows the possible Pd2+ coordination modes in PY–SE–COF–Pd. Reproduced with permission.111 Copyright 2022, Elsevier. | |
4.2.2 Sub-nanoscale engineering.
Pd-based nanoclusters also demonstrated excellent HER electrocatalytic performance due to the physicochemical property change resulting from sub-nanoscale engineering. The conductive MOF materials are desirable frameworks to support Pd nanoclusters. Fuqin Zheng et al.96 fabricated Pd nanoclusters immobilized in MOF-74 for efficient bifunctional electrocatalysis of both the ORR and HER. The tiny Pd nanoclusters were dispersed uniformly and confined in the MOFs, successfully reducing the noble metal mass loading and lowering the cost. This work further verified the template function of conductive MOFs for metal nanoclusters' confinement. An Pei et al.97 used nickel hydroxide to support Pd nanoclusters and Ru single atoms, and applied the catalyst for both the HER and OER. After tuning the Pd/Ru ratio of Pd nanoclusters and Ru single atoms, the Pd12Ru3/Ni(OH)2/C displayed excellent performance in HER and OER processes, resulting from the synergistic effect of the adjacent Pd and Ru atoms.
4.2.3 Morphology design.
Morphology design is another promising strategy to design the catalysts. To increase the number of active sites and lower the costs, Daniel Ohm et al.98 used the nano-confining strategy to grow some atomically dispersed Pd/Ir islands on Au(111) and optimized the HER performance by controlling the morphology, density and size of the islands (Fig. 7e). Based on the Cu underpotential deposition and galvanic displacement, the researchers deposited the sublayers on the surface of Au and then successfully fabricated discrete islands of Pd/Ir. By controlling the deposition process of Cu, researchers could shape the islands as the designed morphology. B. T. Jebaslinhepzybai et al.99 also used the galvanic replacement method to synthesize distinct nanostructures. The researchers developed a facile route to fabricate the carbon-supported porous Pd core with a Pt enriched shell surface. The prepared PdPt/C nanocrystals had high crystallinity, thus exhibiting super good stability, and provided a feasible way to synthesize bimetallic catalysts.
Core–shell structures are widely studied with different elements. Yihe Wang et al.16 fabricated a Pd/Pt core–shell nanostructure and adjusted the shell layers by the etching method (Fig. 7c). The hollow nanocages could increase the atom utilization efficiency of both noble metals and the bilayer nanocages performed better than the monolayer ones. It's worth noting that the activity improvement of this bilayer thin wall was more than twice that of the monolayer one. To further reduce the cost of fabrication, constructing a core–shell structure with some less expensive transition metals like Cu could be a feasible strategy. Jing Li et al.100 reduced Cu(acac)2 and Na2PdCl4 to synthesize PdCu alloy nanocubes and used electrochemical reactions to fabricate core@shell PdCu@Pd nanocubes by etching the surface Cu to enhance the overall HER activity through weakening the binding energy of the intermediates and exposing more Pd active sites on the surface. Compared with the original PdCu alloy nanocubes, this core–shell structure brought about Pt-like performance and much greater stability. In order to further decrease the content of noble metals for low-cost electrocatalysts, fabricating bimetallic catalysts with a large amount of non-noble metals is a feasible strategy. However, balancing the stability of this kind of catalyst and the super low loading of noble metals is quite difficult. To find the optimal solution to this problem, Xiaocheng Liu et al.101 synthesized nanoporous NiPd/metallic glass and minimized the Pd loading in the alloys by implementing the dealloying method and galvanic replacement to load Pd on the surface.
To fabricate durable and active HER electrocatalysts, U. Lačnjevac et al.102 deposited Pd nanoparticles on the surface of TiO2 nanotube arrays via a galvanic displacement strategy. The Pd fills the entire tube wall with the matching length. The semiconductor properties of the TiO2 nanotubes and interactive Pd active sites provided optimized atomic hydrogen binding energy, thus boosting the HER activity. The 3D TiO2 nanotubes not only supported a large amount of Pd nanoparticles, but also prevented degradation of the Pd active sites, enhancing the electrocatalytic durability under harsh working environments.
4.2.4 Nanocomposite fabrication.
Designing functionalized nanocomposite materials for the high performance electrocatalysis has caught researchers' attention.105 Didem Balun Kayan et al.106 used two methods to synthesize rGO-Pd nanocomposites, including electrochemical deposition and chemical synthesis followed by electrochemical reduction. Based on a comparison between these two methods, the chemical synthesis route performed better with a lower overpotential. With more active sites for reactant adsorption and Pd binding, it optimized the hydrogen evolution performance. Chandraraj Alex et al.107 embedded Pd nanoparticles into a coordination polymer-rGO composite for highly efficient HER electrocatalysis. The organic ligand inside stabilized the Pd nanoparticles to maintain the intact framework, thus enhancing the durability by preventing the aggregation of Pd nanoparticles and the rGO carbon materials improved the conductivity of the whole electrocatalyst. Taking advantage of the various carbon materials to support Pd nanoparticles could be an effective way to fabricate catalysts.
Designing multifunctional carbon materials is beneficial to the electron transfer and stability of the whole structure. S. Shiva Kumar et al.108 synthesized phosphorus-doped carbon nanoparticles as novel carbon materials to support palladium. The researchers used the spray pyrolysis method to synthesize P-CNPs as an electron carrier material with perfect electrical conductivity. To evaluate the activity of this novel low-cost catalyst, the researchers fabricated membrane electrode assemblies by using this catalyst as an HER electrode. Samane Asadi et al.109 prepared a Ni–Pd(OH)2/C nanocomposite by the electrodeposition method and investigated the effect of Mo ions in alkaline electrolyte on the improvement of the catalyst's activity. Based on analysis of the Tafel curves and EIS, the results showed that the Mo ions could be the activator for the HER of the Ni–Pd(OH)2/C nanocomposite. Besides, excellent durability was observed in this catalytic system. Ali A. Ensafi et al.17 designed a novel nanocomposite, i.e., Pt–Pd alloy nanoparticles combined with a sulfonated graphene-based conductive polymer. Due to the π-network in the graphene, it provided excellent electrical conductivity and mechanical stability. Also, the sulfonated graphene nanosheets provided super large surface area for the HER.
4.2.5 Modification strategy.
Pd-based modification is a cost-efficient strategy for electrocatalysis, and improves the electrocatalytic performance owing to surface modification of Pd-containing molecules.103 Vishal Burungale et al.103 fabricated Pd/CNT modified Cu2O/NF by the spraying method to deal with the sluggish reaction kinetics of the HER (Fig. 7b). Compared with the pure Cu2O/NF, the presence of Pd improved the catalytic activity while the CNT part in the surface could greatly enhance the charge transportation and conductivity (Fig. 8c). The surface modification also provided porous structures and enhanced the dispersivity of Pd nanoparticles. Besides, noble metals like Pd are beneficial for the overall conductivity and activity of the catalysts.
Due to the poor conductivity of MOF-based materials, researchers modified these materials with some metal nanoparticles. Ming Nie et al.110 took advantage of the Pd nanoparticles to enhance the electrical conduction of MOF materials for high performance HER. Based on the direct reduction method, researchers prepared Pd/MOF materials and the synergistic effect of the improved specific surface area and conductivity enlarged the electrocatalytic activity. M. Nie et al.104 reported the fabrication of Pd doped Ni-MOF materials as high conductivity and stable three-dimensional HER catalysts (Fig. 7d). To further improve the performance, researchers investigated the effect of different content ratios of Pd to Ni, and found that this adjustment could reduce the resistance charge transfer (Fig. 8d). The pore structure and adsorption ability derived from MOF structures, combined with the excellent electron transfer ability and highly active sites from the modified Pd nanoparticles, resulted in super good performance.
In addition to MOF materials, other three-dimensional carbon materials could also be functionalized by noble metals to fabricate electrocatalysts. Jie-Yu Yue et al.111 constructed Pd ions supported on COF materials for application in the HER. This work developed the first Pd2+/COF system for HER electrocatalysis and the performance results indicated that the vinylene-linked COF platforms without a pyrolysis-free process could be a new design idea (Fig. 8e). Due to the ordered crystalline porous structure linked by covalent bonds, the COF-based materials could be highly stable. Besides, the carbon–carbon double bonds derived from the vinylene adjusted the electronic structure and increased the surface area. Therefore, the combination of Pd active sites and this COF structure enhanced the whole catalytic performance and durability. Chao Shu et al.112 used the hydrothermal method to synthesize low-loading Pd on a hollow titanium dioxide sphere and the Pd doping effect could provide more active sites, resulting in promoted catalytic performance.
Utilizing the synergistic effect of a Pd-based alloy as a modifier is also a promising strategy to fabricate cost-efficient electrocatalysts. M. Smiljanić et al.113 deposited Pd/Rh nanoislands on a polycrystalline gold electrode and compared it to Pd–polycrystalline gold and Rh–polycrystalline gold to investigate the synergistic effect of the tri-metallic catalyst. The results indicated that the strong interaction between these three metals lowered the adsorption energy of the intermediate during the HER process, thus enhancing the catalytic activity.
5. Conclusion and perspectives
With the development of electrocatalysis, more and more materials have been studied for different electrocatalytic reactions. Besides the studies on commercial catalyst-based materials (like Pt-based catalysts), researchers have proposed and designed lots of novel materials114 such as alloys, transition metal phosphides, sulfides, nanocomposites, single atom catalysts, etc. Due to the higher reserves of Pd compared with Pt,115 researchers have tried to fabricate Pd-based catalysts for efficient and stable ORR, OER and HER. Recent studies demonstrated that the precious metal Pd has the potential to achieve equivalent or even better electrocatalytic performance in comparison with Pt.116 Based on a comprehensive analysis, Pd-containing nanomaterials have the following intrinsic advantages: (a) palladium is more abundant than platinum. (b) Pd could provide active sites for both the ORR and HER, and PdO could provide active sites for the OER. Therefore, the palladium element has intrinsic activities for these reactions, providing a foundation for further electrocatalytic performance improvement. (c) The oxygen binding strength of Pd-based materials is closer to the optimized oxygen binding strength in alkaline medium, so it's easier to balance the oxygen-containing chemicals' adsorption and desorption, which plays an important role in the electrocatalytic ORR and OER.117 (d) Pd-containing materials with different nanostructures and morphologies are studied extensively by researchers and they have developed relatively mature methods for designing and synthesizing novel materials.
Recent studies on Pd-based nanomaterials for the ORR, OER, HER and multifunctional applications are summarized in this review. There are many challenges no matter in ORR, OER or HER, and the key point is to design low-cost electrocatalysts that exhibit high activity, good stability and multifunctionality. Many studies have proposed useful strategies to draw closer to the goal such as alloying, defect engineering, strain engineering, single atom catalyst design, framework-directed nanocomposite design, morphology engineering, surface treatment and so on.118 There is still a long way to go before the successful commercialization of Pd-based nanomaterials. Although some Pd-based nanomaterials exhibit excellent mass activity and specific activity, the mass production of novel materials needs multi-dimensional investigation, and the industry production conditions are quite different from the laboratory conditions. It is believed that Pd-based nanomaterials are promising for high-performance and stable electrocatalysis119 and many novel materials can be explored and we hope that this review could provide useful summaries and motivate researchers to further prompt the development of Pd-based nanomaterials for multifunctional electrocatalysis.
Conflicts of interest
The authors declare no conflicts of interest.
Acknowledgements
This work was financially supported by the Natural Sciences and Engineering Research Council of Canada (NSERC) through the Discovery Grant Program (RGPIN-2020-05184) and the Alliance-Alberta Innovates Program (ALLRP-561137-20). This research was also supported by funding from the Canada First Research Excellence Fund as part of the University of Alberta's Future Energy Systems research initiative.
References
- F. D. Sanij, P. Balakrishnan, P. Leung, A. Shah, H. Su and Q. Xu, Advanced Pd-based nanomaterials for electro-catalytic oxygen reduction in fuel cells: A review, Int. J. Hydrogen Energy, 2021, 46, 14596–14627 CrossRef CAS.
- K. Zhang and R. Zou, Advanced transition metal-based OER electrocatalysts: current status, opportunities, and challenges, Small, 2021, 17, 2100129 CrossRef CAS PubMed.
- Z. F. Huang, J. Wang, Y. Peng, C. Y. Jung, A. Fisher and X. Wang, Design of efficient bifunctional oxygen reduction/evolution electrocatalyst: recent advances and perspectives, Adv. Energy Mater., 2017, 7, 1700544 CrossRef.
- R. Devivaraprasad, N. Nalajala, B. Bera and M. Neergat, Electrocatalysis of oxygen reduction reaction on shape-controlled Pt and Pd nanoparticles—importance of surface cleanliness and reconstruction, Front. Chem., 2019, 7, 648 CrossRef CAS PubMed.
- T. Wang, A. Chutia, D. J. Brett, P. R. Shearing, G. He, G. Chai and I. P. Parkin, Palladium alloys used as electrocatalysts for the oxygen reduction reaction, Energy Environ. Sci., 2021, 14, 2639–2669 RSC.
- Z. Zhao, C. Chen, Z. Liu, J. Huang, M. Wu, H. Liu, Y. Li and Y. Huang, Pt-based nanocrystal for electrocatalytic oxygen reduction, Adv. Mater., 2019, 31, 1808115 CrossRef PubMed.
- D. Bhalothia, L. Krishnia, S.-S. Yang, C. Yan, W.-H. Hsiung, K.-W. Wang and T.-Y. Chen, Recent advancements and future prospects of noble metal-based heterogeneous nanocatalysts for oxygen reduction and hydrogen evolution reactions, Appl. Sci., 2020, 10, 7708 CrossRef CAS.
- H. Zhong, C. A. Campos-Roldán, Y. Zhao, S. Zhang, Y. Feng and N. Alonso-Vante, Recent advances of cobalt-based electrocatalysts for oxygen electrode reactions and hydrogen evolution reaction, Catalysts, 2018, 8, 559 CrossRef.
- H. C. Li, Y. J. Zhang, X. Hu, W. J. Liu, J. J. Chen and H. Q. Yu, Metal–organic framework templated Pd@ PdO–Co3O4 nanocubes as an efficient bifunctional oxygen electrocatalyst, Adv. Energy Mater., 2018, 8, 1702734 CrossRef.
- B. Y. Xia, Y. Yan, N. Li, H. B. Wu, X. W. D. Lou and X. Wang, A metal–organic framework-derived bifunctional oxygen electrocatalyst, Nat. Energy, 2016, 1, 1–8 Search PubMed.
- X. F. Lu, B. Y. Xia, S. Q. Zang and X. W. Lou, Metal–organic frameworks based electrocatalysts for the oxygen reduction reaction, Angew. Chem., 2020, 132, 4662–4678 CrossRef.
- M. Zhou, J. Guo and J. Fang, Nanoscale Design of Pd-Based Electrocatalysts for Oxygen Reduction Reaction Enhancement in Alkaline Media, Small Struct., 2022, 3, 2100188 CrossRef CAS.
- Q. Chen, Z. Chen, A. Ali, Y. Luo, H. Feng, Y. Luo, P. Tsiakaras and P. K. Shen, Shell-thickness-dependent Pd@ PtNi core–shell nanosheets for efficient oxygen reduction reaction, Chem. Eng. J., 2022, 427, 131565 CrossRef CAS.
- M. Torihata, M. Nakamura, N. Todoroki, T. Wadayama and N. Hoshi, Activity for the ORR on Pt-Pd-Co ternary alloy electrodes is markedly affected by surface structure and composition, Electrochem. Commun., 2021, 125, 107007 CrossRef CAS.
- D. Bhalothia, J.-P. Chou, C. Yan, A. Hu, Y.-T. Yang and T.-Y. Chen, Programming ORR Activity of Ni/NiO x@ Pd Electrocatalysts via Controlling Depth of Surface-Decorated Atomic Pt Clusters, ACS Omega, 2018, 3, 8733–8744 CrossRef CAS PubMed.
- Y. Wang, L. Zhang, C. Hu, S. Yu, P. Yang, D. Cheng, Z.-J. Zhao and J. Gong, Fabrication of bilayer Pd-Pt nanocages with sub-nanometer thin shells for enhanced hydrogen evolution reaction, Nano Res., 2019, 12, 2268–2274 CrossRef CAS.
- A. A. Ensafi, N. Zandi-Atashbar, Z. Mohamadi, A. Abdolmaleki and B. Rezaei, Pt-Pd nanoparticles decorated sulfonated graphene-poly(3,4-ethylene dioxythiophene) nanocomposite, An efficient HER electrocatalyst, Energy, 2017, 126, 88–96 CrossRef CAS.
- X. Yang, J. Hu, R. Wu and B. E. Koel, Balancing Activity and Stability in a Ternary Au-Pd/Fe Electrocatalyst for ORR with High Surface Coverages of Au, ChemCatChem, 2019, 11, 693–697 CrossRef CAS.
- T. Wu, M. Sun and B. Huang, Highly active electron-affinity for ultra-low barrier for alkaline ORR in Pd3Cu, Mater. Today Energy, 2019, 12, 426–430 CrossRef.
- G. Bampos, L. Sygellou and S. Bebelis, Oxygen reduction reaction activity of Pd-based bimetallic electrocatalysts in alkaline medium, Catal. Today, 2020, 355, 685–697 CrossRef CAS.
- R. Sun, W. Guo, X. Han and X. Hong, Two-dimensional noble metal nanomaterials for electrocatalysis, Chem. Res. Chin. Univ., 2020, 36, 597–610 CrossRef CAS.
- M. Kannan, Current status, key challenges and its solutions in the design and development of graphene based ORR catalysts for the microbial fuel cell applications, Biosens. Bioelectron., 2016, 77, 1208–1220 CrossRef CAS PubMed.
- S. S. A. Shah, T. Najam, M. K. Aslam, M. Ashfaq, M. M. Rahman, K. Wang, P. Tsiakaras, S. Song and Y. Wang, Recent advances on oxygen reduction electrocatalysis: Correlating the characteristic properties of metal organic frameworks and the derived nanomaterials, Appl. Catal., B, 2020, 268, 118570 CrossRef CAS.
- M. Jahan, Z. Liu and K. P. Loh, A Graphene oxide and copper-centered metal organic framework composite as a tri-functional catalyst for HER, OER, and ORR, Adv. Funct. Mater., 2013, 23, 5363–5372 CrossRef CAS.
- K. Jayasayee, J. R. Van Veen, T. G. Manivasagam, S. Celebi, E. J. Hensen and F. A. De Bruijn, Oxygen reduction reaction (ORR) activity and durability of carbon supported PtM (Co, Ni, Cu) alloys: Influence of particle size and non-noble metals, Appl. Catal., B, 2012, 111, 515–526 CrossRef.
- I. Roche, E. Chaînet, M. Chatenet and J. Vondrák, Durability of carbon-supported manganese oxide nanoparticles for the oxygen reduction reaction (ORR) in alkaline medium, J. Appl. Electrochem., 2008, 38, 1195–1201 CrossRef CAS.
- D.-D. Qin, Y. Tang, G. Ma, L. Qin, C.-L. Tao, X. Zhang and Z. Tang, Molecular metal nanoclusters for ORR, HER and OER: achievements, opportunities and challenges, Int. J. Hydrogen Energy, 2021, 46, 25771–25781 CrossRef CAS.
- J. Hu, W. Liu, C. Xin, J. Guo, X. Cheng, J. Wei, C. Hao, G. Zhang and Y. Shi, Carbon-based Single Atom Catalysts for Tailoring ORR Pathway: A Concise Review, J. Mater. Chem. A, 2021, 9, 24803–24829 RSC.
- C. Kim, F. Dionigi, V. Beermann, X. Wang, T. Möller and P. Strasser, Alloy nanocatalysts for the electrochemical oxygen reduction (ORR) and the direct electrochemical carbon dioxide reduction reaction (CO2RR), Adv. Mater., 2019, 31, 1805617 CrossRef PubMed.
- M. Sankar, N. Dimitratos, P. J. Miedziak, P. P. Wells, C. J. Kiely and G. J. Hutchings, Designing bimetallic catalysts for a green and sustainable future, Chem. Soc. Rev., 2012, 41, 8099–8139 RSC.
- M. Luo, Z. Zhao, Y. Zhang, Y. Sun, Y. Xing, F. Lv, Y. Yang, X. Zhang, S. Hwang and Y. Qin, PdMo bimetallene for oxygen reduction catalysis, Nature, 2019, 574, 81–85 CrossRef CAS PubMed.
- D. Wu, X. Shen, Y. Pan, L. Yao and Z. Peng, Platinum alloy catalysts for oxygen reduction reaction: Advances, challenges and perspectives, ChemNanoMat, 2020, 6, 32–41 CrossRef CAS.
- M. Neergat, V. Gunasekar and R. Rahul, Carbon-supported Pd–Fe electrocatalysts for oxygen reduction reaction (ORR) and their methanol tolerance, J. Electroanal. Chem., 2011, 658, 25–32 CrossRef CAS.
- L. E. Betancourt, A. Rojas-Perez, I. Orozco, A. I. Frenkel, Y. Li, K. Sasaki, S. D. Senanayake and C. R. Cabrera, Enhancing ORR performance of bimetallic PdAg electrocatalysts by designing interactions between Pd and Ag, ACS Appl. Energy Mater., 2020, 3, 2342–2349 CrossRef CAS.
- J. Kim, Y. Hong, K. Lee and J. Y. Kim, Highly stable Pt-based ternary systems for oxygen reduction reaction in acidic electrolytes, Adv. Energy Mater., 2020, 10, 2002049 CrossRef CAS.
- X. Jiang, Y. Xiong, R. Zhao, J. Zhou, J.-M. Lee and Y. Tang, Trimetallic Au@ PdPb nanowires for oxygen reduction reaction, Nano Res., 2020, 13, 2691–2696 CrossRef CAS.
- Z.-P. Wu, D. T. Caracciolo, Y. Maswadeh, J. Wen, Z. Kong, S. Shan, J. A. Vargas, S. Yan, E. Hopkins and K. Park, Alloying–realloying enabled high durability for Pt–Pd-3d-transition metal nanoparticle fuel cell catalysts, Nat. Commun., 2021, 12, 859 CrossRef CAS PubMed.
- X. Wei, S. Song, W. Cai, X. Luo, L. Jiao, Q. Fang, X. Wang, N. Wu, Z. Luo and H. Wang, Tuning the spin-state of Fe single atoms by Pd nanoclusters enables robust oxygen reduction with dissociative pathway, Chem, 2022, 9, 181–197 Search PubMed.
- H. Cruz-Martínez, M. Tellez-Cruz, O. Solorza-Feria, P. Calaminici and D. Medina, Catalytic activity trends from pure Pd nanoclusters to M@ PdPt (M= Co, Ni, and Cu) core-shell nanoclusters for the oxygen reduction reaction: A first-principles analysis, Int. J. Hydrogen Energy, 2020, 45, 13738–13745 CrossRef.
- N. Wang, X. Zhao, R. Zhang, S. Yu, Z. H. Levell, C. Wang, S. Ma, P. Zou, L. Han and J. Qin, Highly Selective Oxygen Reduction to Hydrogen Peroxide on a Carbon-Supported Single-Atom Pd Electrocatalyst, ACS Catal., 2022, 12, 4156–4164 CrossRef CAS.
- H. E. Kim, I. H. Lee, J. Cho, S. Shin, H. C. Ham, J. Y. Kim and H. Lee, Palladium Single-Atom Catalysts Supported on C@ C3N4 for Electrochemical Reactions, ChemElectroChem, 2019, 6, 4757–4764 CrossRef CAS.
- K. Jiang, S. Back, A. J. Akey, C. Xia, Y. Hu, W. Liang, D. Schaak, E. Stavitski, J. K. Nørskov and S. Siahrostami, Highly selective oxygen reduction to hydrogen peroxide on transition metal single atom coordination, Nat. Commun., 2019, 10, 1–11 CrossRef PubMed.
- Z. Wei, B. Deng, P. Chen, T. Zhao and S. Zhao, Palladium-based single atom catalysts for high-performance electrochemical production of hydrogen peroxide, Chem. Eng. J., 2022, 428, 131112 CrossRef CAS.
- Z. Fu, C. Ling and J. Wang, A Ti 3 C 2 O 2 supported single atom, trifunctional catalyst for electrochemical reactions, J. Mater. Chem. A, 2020, 8, 7801–7807 RSC.
- Z. Qin and J. Zhao, 1 T-MoSe2 monolayer supported single Pd atom as a highly-efficient bifunctional catalyst for ORR/OER, J. Colloid Interface Sci., 2022, 605, 155–162 CrossRef CAS PubMed.
- M. T. Darby and M. Stamatakis, Single-Atom Alloys for the Electrochemical Oxygen Reduction Reaction, ChemPhysChem, 2021, 22, 499–508 CrossRef CAS PubMed.
- R. Li and D. Wang, Superiority of dual-atom catalysts in electrocatalysis: one step further than single-atom catalysts, Adv. Energy Mater., 2022, 12, 2103564 CrossRef CAS.
- M. Huang, H. Meng, J. Luo, H. Li, Y. Feng and X.-X. Xue, Understanding the Activity and Design Principle of Dual-Atom Catalysts Supported on C2N for Oxygen Reduction and Evolution Reactions: From Homonuclear to Heteronuclear, J. Phys. Chem. Lett., 2023, 14, 1674–1683 CrossRef CAS PubMed.
- X. Wang, L. Guo, Z. Xie, X. Peng, X. Yu, X. Yang, Z. Lu, X. Zhang and L. Li, Coordination environment engineering of graphene-supported single/dual-Pd-site catalysts improves the electrocatalytic ORR activity, Appl. Surf. Sci., 2022, 606, 154749 CrossRef CAS.
- J. Lee, S. W. Choi, S. Back, H. Jang and Y. J. Sa, Pd17Se15-Pd3B nanocoral electrocatalyst for selective oxygen reduction to hydrogen peroxide in near-neutral electrolyte, Appl. Catal., B, 2022, 309, 121265 CrossRef CAS.
- A. Verdaguer-Casadevall, D. Deiana, M. Karamad, S. Siahrostami, P. Malacrida, T. W. Hansen, J. Rossmeisl, I. Chorkendorff and I. E. Stephens, Trends in the electrochemical synthesis of H2O2: enhancing activity and selectivity by electrocatalytic site engineering, Nano Lett., 2014, 14, 1603–1608 CrossRef CAS PubMed.
- Z. Chen, F. Lin, D. He, H. Jiang, J. Zhang, X. Wang and M. Huang, A hybrid composite catalyst of Fe 3 O 4 nanoparticles-based carbon for electrochemical reduction of oxygen, New J. Chem., 2017, 41, 4959–4965 RSC.
- H. Park, S. Oh, S. Lee, S. Choi and M. Oh, Cobalt-and nitrogen-codoped porous carbon catalyst made from core–shell type hybrid metal–organic framework (ZIF-L@ ZIF-67) and its efficient oxygen reduction reaction (ORR) activity, Appl. Catal., B, 2019, 246, 322–329 CrossRef CAS.
- H. Wang, W. Wang, H. Yu, Q. Mao, Y. Xu, X. Li, Z. Wang and L. Wang, Interface engineering of polyaniline-functionalized porous Pd metallene for alkaline oxygen reduction reaction, Appl. Catal., B, 2022, 307, 121172 CrossRef CAS.
- M. S. Matseke, T. S. Munonde, K. Mallick and H. Zheng, Pd/PANI/C nanocomposites as electrocatalysts for oxygen reduction reaction in alkaline media, Electrocatalysis, 2019, 10, 436–444 CrossRef CAS.
- G. Wu, Z. Chen, K. Artyushkova, F. H. Garzon and P. Zelenay, Polyaniline-derived non-precious catalyst for the polymer electrolyte fuel cell cathode, ECS Trans., 2008, 16, 159 CrossRef CAS.
- A. Ejaz and S. Jeon, The individual role of pyrrolic, pyridinic and graphitic nitrogen in the growth kinetics of Pd NPs on N-rGO followed by a comprehensive study on ORR, Int. J. Hydrogen Energy, 2018, 43, 5690–5702 CrossRef CAS.
- X. Dong, J. Li, D. Wei, R. Li, K. Qu, L. Wang, S. Xu, W. Kang and H. Li, Pd (II)/Ni (II)-dimethylglyoxime derived Pd–Ni–P@ N-doped carbon hybrid nanocatalysts for oxygen reduction reaction, Appl. Surf. Sci., 2019, 479, 273–279 CrossRef CAS.
- Y. Wu, Z. Xiao, Z. Jin, X. Li and Y. Chen, The cobalt carbide/bimetallic CoFe phosphide dispersed on carbon nanospheres as advanced bifunctional electrocatalysts for the ORR, OER, and rechargeable Zn–air batteries, J. Colloid Interface Sci., 2021, 590, 321–329 CrossRef CAS PubMed.
- B. K. Barman, B. Sarkar and K. K. Nanda, Pd-coated Ru nanocrystals supported on N-doped graphene as HER and ORR electrocatalysts, Chem. Commun., 2019, 55, 13928–13931 RSC.
- M. Nunes, D. M. Fernandes, M. Morales, I. Rodríguez-Ramos, A. Guerrero-Ruiz and C. Freire, Cu and Pd nanoparticles supported on a graphitic carbon material as bifunctional HER/ORR electrocatalysts, Catal. Today, 2020, 357, 279–290 CrossRef CAS.
- A. P. Reyes-Cruzaley, R. Félix-Navarro, B. Trujillo-Navarrete, C. Silva-Carrillo, J. Zapata-Fernández, J. Romo-Herrera, O. Contreras and E. Reynoso-Soto, Synthesis of novel Pd NP-PTH-CNTs hybrid material as catalyst for H2O2 generation, Electrochim. Acta, 2019, 296, 575–581 CrossRef CAS.
- Z. Cui and X. Bai, Highly Active and Stable Fe/Co/N Co-doped Carbon-Anchored Pd Nanoparticles for Oxygen Reduction Reaction, ACS Appl. Mater. Interfaces, 2022, 14, 9024–9035 CrossRef CAS PubMed.
- L. Karuppasamy, L. Gurusamy, S. Anandan, C.-H. Liu and J. Wu, Defect-enriched heterointerfaces N–MoO2–Mo2C supported Pd nanocomposite as a novel multifunctional electrocatalyst for oxygen reduction reaction and overall water splitting, Mater. Today Chem., 2022, 24, 100799 CrossRef CAS.
- T. Kang, D. Nam and J. Kim, Activated palladium deposited on defect-rich carbon and cobalt oxide composite materials as bi-functional catalysts for stable rechargeable Zn–air battery, Appl. Surf. Sci., 2022, 582, 152442 CrossRef CAS.
- H.-Y. Qu, X. He, Y. Wang and S. Hou, Electrocatalysis for the oxygen evolution reaction in acidic media: Progress and challenges, Appl. Sci., 2021, 11, 4320 CrossRef CAS.
- X.-K. Gu, J. C. A. Camayang, S. Samira and E. Nikolla, Oxygen evolution electrocatalysis using mixed metal oxides under acidic conditions: Challenges and opportunities, J. Catal., 2020, 388, 130–140 CrossRef CAS.
- Z. Ma, Y. Zhang, S. Liu, W. Xu, L. Wu, Y.-C. Hsieh, P. Liu, Y. Zhu, K. Sasaki and J. N. Renner, Reaction mechanism for oxygen evolution on RuO2, IrO2, and RuO2@ IrO2 core-shell nanocatalysts, J. Electroanal. Chem., 2018, 819, 296–305 CrossRef CAS.
- A. Karmakar, K. Karthick, S. S. Sankar, S. Kumaravel, R. Madhu and S. Kundu, A vast exploration of improvising synthetic strategies for enhancing the OER kinetics of LDH structures: a review, J. Mater. Chem. A, 2021, 9, 1314–1352 RSC.
- Z. K. Ghouri, K. Elsaid, M. M. Nasef, S. Ahmed, A. Badreldin and A. Abdel-Wahab, Cooperative electrocatalytic effect of Pd and Ce alloys nanoparticles in PdCe@ CNWs electrode for oxygen evolution reaction (OER), Mol. Catal., 2022, 522, 112255 CrossRef CAS.
- T. Zhang, S.-A. Liao, L.-X. Dai, J.-W. Yu, W. Zhu and Y.-W. Zhang, Ir-Pd nanoalloys with enhanced surface-microstructure-sensitive catalytic activity for oxygen evolution reaction in acidic and alkaline media, Sci. China Mater., 2018, 61, 926–938 CrossRef CAS.
- G. M. Salvador, A. L. Silva, L. P. Silva, F. B. Passos and N. M. Carvalho, Enhanced activity of Pd/α-MnO2 for electrocatalytic oxygen evolution reaction, Int. J. Hydrogen Energy, 2021, 46, 26976–26988 CrossRef CAS.
- R. Manjunatha, L. Dong, Z. Zhai, J. Wang, Q. Fu, W. Yan and J. Zhang, Pd nanocluster-decorated CoFe composite supported on nitrogen carbon nanotubes as a high-performance trifunctional electrocatalyst, Green Energy Environ., 2022, 7, 933–947 CrossRef CAS.
- F. He, Y. Liu, Q. Cai and J. Zhao, Size-dependent electrocatalytic activity of ORR/OER on palladium nanoclusters anchored on defective MoS 2 monolayers, New J. Chem., 2020, 44, 16135–16143 RSC.
- A. Saad, D. Liu, Y. Wu, Z. Song, Y. Li, T. Najam, K. Zong, P. Tsiakaras and X. Cai, Ag nanoparticles modified crumpled borophene supported Co3O4 catalyst showing superior oxygen evolution reaction (OER) performance, Appl. Catal., B, 2021, 298, 120529 CrossRef CAS.
- Y. Wang, J. Ma, J. Wang, S. Chen, H. Wang and J. Zhang, Interfacial Scaffolding Preparation of Hierarchical PBA-Based Derivative Electrocatalysts for Efficient Water Splitting, Adv. Energy Mater., 2019, 9, 1802939 CrossRef.
- J. Kang, J. Chen, J. Sheng, J. Xie, X.-Z. Fu, R. Sun and C.-P. Wong, Pd nanoparticle-interspersed
hierarchical copper hydroxide@ nickel cobalt hydroxide carbonate tubular arrays as efficient electrocatalysts for oxygen evolution reaction, ACS Sustainable Chem. Eng., 2019, 7, 16459–16466 CrossRef CAS.
- Y. Li and H. Dai, Recent advances in zinc–air batteries, Chem. Soc. Rev., 2014, 43, 5257–5275 RSC.
- D. Chen, J. Zhu, X. Mu, R. Cheng, W. Li, S. Liu, Z. Pu, C. Lin and S. Mu, Nitrogen-Doped carbon coupled FeNi3 intermetallic compound as advanced bifunctional electrocatalyst for OER, ORR and Zn–air batteries, Appl. Catal., B, 2020, 268, 118729 CrossRef CAS.
- S. Ramakrishnan, J. Balamurugan, M. Vinothkannan, A. R. Kim, S. Sengodan and D. J. Yoo, Nitrogen-doped graphene encapsulated FeCoMoS nanoparticles as advanced trifunctional catalyst for water splitting devices and zinc–air batteries, Appl. Catal., B, 2020, 279, 119381 CrossRef CAS.
- H. Over, Fundamental studies of planar single-crystalline oxide model electrodes (RuO2, IrO2) for acidic water splitting, ACS Catal., 2021, 11, 8848–8871 CrossRef CAS.
- J.-X. Guo, S.-Y. Wu, G.-J. Zhang, L. Yan, J.-G. Hu and X.-Y. Li, Single noble metals (Pd, Pt and Ir) anchored Janus MoSSe monolayers: Efficient oxygen reduction/evolution reaction bifunctional electrocatalysts and harmful gas detectors, J. Colloid Interface Sci., 2022, 616, 177–188 CrossRef CAS PubMed.
- X. Liu, Q. Huang, J. Wang, L. Zhao, H. Xu, Q. Xia, D. Li, L. Qian, H. Wang and J. Zhang, In-situ deposition of Pd/Pd4S heterostructure on hollow carbon spheres as efficient electrocatalysts for rechargeable Li-O2 batteries, Chin. Chem. Lett., 2021, 32, 2086–2090 CrossRef CAS.
- M. Y. Oh, J. H. Kim, Y. W. Lee, K. J. Kim, H. R. Shin, H. Park, K. T. Lee, K. Kang and T. H. Shin, Enhancing Bifunctional Catalytic Activity via a Nanostructured La (Sr) Fe (Co) O3− δ@ Pd Matrix as an Efficient Electrocatalyst for Li–O2 Batteries, ACS Appl. Energy Mater., 2019, 2, 8633–8640 CrossRef CAS.
- Y. Liu, S. Jiang, S. Li, L. Zhou, Z. Li, J. Li and M. Shao, Interface engineering of (Ni, Fe) S2@ MoS2 heterostructures for synergetic electrochemical water splitting, Appl. Catal., B, 2019, 247, 107–114 CrossRef CAS.
- M. You, X. Du, X. Hou, Z. Wang, Y. Zhou, H. Ji, L. Zhang, Z. Zhang, S. Yi and D. Chen, In-situ growth of ruthenium-based nanostructure on carbon cloth for superior electrocatalytic activity towards HER and OER, Appl. Catal., B, 2022, 317, 121729 CrossRef CAS.
- Y. Wang, L. Zhao, J. Ma and J. Zhang, Confined interface transformation of metal–organic frameworks for highly efficient oxygen evolution reactions, Energy Environ. Sci., 2022, 15, 3830–3841 RSC.
- A. K. Ipadeola, P. V. Mwonga, S. C. Ray, R. R. Maphanga and K. I. Ozoemena, Bifunctional Behavior of Pd/Ni Nanocatalysts on MOF-Derived Carbons for Alkaline Water-splitting, Electroanalysis, 2020, 32, 3060–3074 CrossRef CAS.
- P. Mondal, J. Satra, D. N. Srivastava, G. R. Bhadu and B. Adhikary, Pdδ+-Mediated Surface Engineering of AgMnO4 Nanorods as Advanced Bifunctional Electrocatalysts for Highly Efficient Water Electrolysis, ACS Catal., 2021, 11, 3687–3703 CrossRef CAS.
- X. Zhang and P. Yang, Pd nanoparticles assembled on Ni-and N-doped carbon nanotubes towards superior electrochemical activity, Int. J. Hydrogen Energy, 2021, 46, 2065–2074 CrossRef CAS.
- N. Mahmood, Y. Yao, J. W. Zhang, L. Pan, X. Zhang and J. J. Zou, Electrocatalysts for hydrogen evolution in alkaline electrolytes: mechanisms, challenges, and prospective solutions, Adv. Sci., 2018, 5, 1700464 CrossRef PubMed.
- J. Verma and S. Goel, Cost-effective electrocatalysts for Hydrogen Evolution Reactions (HER): Challenges and Prospects, Int.
J. Hydrogen Energy, 2022, 47, 38964–38982 CrossRef CAS.
- S. C. Paul, S. C. Dey, M. A. I. Molla, M. S. Islam, S. Debnath, M. Y. Miah, M. Ashaduzzaman and M. Sarker, Nanomaterials as electrocatalyst for hydrogen and oxygen evolution reaction: Exploitation of challenges and current progressions, Polyhedron, 2021, 193, 114871 CrossRef CAS.
- Y.-Y. Liu, H.-P. Zhang, B. Zhu, H.-W. Zhang, L.-D. Fan, X.-Y. Chai, Q.-L. Zhang, J.-H. Liu and C.-X. He, C/N-co-doped Pd coated Ag nanowires as a high-performance electrocatalyst for hydrogen evolution reaction, Electrochim. Acta, 2018, 283, 221–227 CrossRef CAS.
- J. Li, Y. Cheng, J. Zhang, J. Fu, W. Yan and Q. Xu, Confining Pd Nanoparticles and Atomically Dispersed Pd into Defective MoO3 Nanosheet for Enhancing Electro- and Photocatalytic Hydrogen Evolution Performances, ACS Appl. Mater. Interfaces, 2019, 11, 27798–27804 CrossRef CAS PubMed.
- F. Zheng, C. Zhang, X. Gao, C. Du, Z. Zhuang and W. Chen, Immobilizing Pd nanoclusters into electronically conductive metal-organic frameworks as bi-functional electrocatalysts for hydrogen evolution and oxygen reduction reactions, Electrochim. Acta, 2019, 306, 627–634 CrossRef CAS.
- A. Pei, G. Li, L. Zhu, Z. Huang, J. Ye, Y. C. Chang, S. M. Osman, C. W. Pao, Q. Gao and B. H. Chen, Nickel Hydroxide-Supported Ru Single Atoms and Pd Nanoclusters for Enhanced Electrocatalytic Hydrogen Evolution and Ethanol Oxidation, Adv. Funct. Mater., 2022, 2208587 CrossRef CAS.
- D. Ohm and K. F. Domke, Controlled deposition of 2D-confined Pd or Ir nano-islands on Au(1 1 1) following Cu UPD, and their HER activity, J. Electroanal. Chem., 2021, 896, 115285 CrossRef CAS.
- B. T. Jebaslinhepzybai, N. Prabu and M. Sasidharan, Facile galvanic replacement method for porous Pd@Pt nanoparticles as an efficient HER electrocatalyst, Int. J. Hydrogen Energy, 2020, 45, 11127–11137 CrossRef CAS.
- J. Li, F. Li, S. X. Guo, J. Zhang and J. Ma, PdCu@Pd Nanocube with Pt-like Activity for Hydrogen Evolution Reaction, ACS Appl. Mater. Interfaces, 2017, 9, 8151–8160 CrossRef CAS PubMed.
- X. Liu, S. Zhu, Y. Liang, H. Jiang, Z. Li, S. Wu and Z. Cui, Self-standing nanoporous NiPd bimetallic electrocatalysts with ultra-low Pd loading for efficient hydrogen evolution reaction, Electrochim. Acta, 2022, 411, 140077 CrossRef CAS.
- U. Lačnjevac, R. Vasilić, T. Tokarski, G. Cios, P. Żabiński, N. Elezović and N. Krstajić, Deposition of Pd nanoparticles on the walls of cathodically hydrogenated TiO2 nanotube arrays via galvanic displacement: A novel route to produce exceptionally active and durable composite electrocatalysts for cost-effective hydrogen evolution, Nano energy, 2018, 47, 527–538 CrossRef.
- V. Burungale, H. Bae, P. Mane, H. Rho, S.-W. Ryu, S. H. Kang and J.-S. Ha, Improved performance of CNT-Pd modified Cu2O supported on Nickel foam for hydrogen evolution reaction in basic media, J. Mol. Liq., 2021, 343, 117612 CrossRef CAS.
- M. Nie, Z. H. Xue, H. Sun, J. M. Liao, F. J. Xue and X. X. Wang, Pd doped Ni derived from Ni - Metal organic framework for efficient hydrogen evolution reaction in alkaline electrolyte, Int. J. Hydrogen Energy, 2020, 45, 28870–28875 CrossRef CAS.
- W. Li, X.-s. Chu, F. Wang, Y.-y. Dang, X.-y. Liu, X.-c. Wang and C.-y. Wang, Enhanced cocatalyst-support interaction and promoted electron transfer of 3D porous g-C3N4/GO-M (Au, Pd, Pt) composite catalysts for hydrogen evolution, Appl. Catal., B, 2021, 288, 120034 CrossRef CAS.
- D. B. Kayan, T. Baran and A. Menteş, Functionalized rGO-Pd nanocomposites as high-performance catalysts for hydrogen generation via water electrolysis, Electrochim. Acta, 2022, 422, 140513 CrossRef CAS.
- C. Alex, S. A. Bhat, N. S. John and C. V. Yelamaggad, Highly Efficient and Sustained Electrochemical Hydrogen Evolution by Embedded Pd-Nanoparticles on a Coordination Polymer—Reduced Graphene Oxide Composite, ACS Appl. Energy Mater., 2019, 2, 8098–8106 CrossRef CAS.
- S. Shiva Kumar, S. U. B. Ramakrishna, B. Rama Devi and V. Himabindu, Phosphorus-doped carbon nanoparticles supported palladium electrocatalyst for the hydrogen evolution reaction (HER) in PEM water electrolysis, Ionics, 2018, 24, 3113–3121 CrossRef CAS.
- S. Asadi, A. R. Madram, E. Biglari, M. Fekri and F. Fotouhi-Far, Preparation and study of electrocatalytic activity of Ni-Pd(OH)2/C nanocomposite for hydrogen evolution reaction in alkaline solution, Int. J. Hydrogen Energy, 2019, 44, 8223–8232 CrossRef CAS.
- M. Nie, H. Sun, D. Lei, S. Kang, J. Liao, P. Guo, Z. Xue and F. Xue, Novel Pd/MOF electrocatalyst for hydrogen evolution reaction, Mater. Chem. Phys., 2020, 254, 123481 CrossRef CAS.
- J.-Y. Yue, X.-L. Ding, L.-P. Song, Y.-T. Wang, P. Yang, Y. Ma and B. Tang, Pd(II) functionalized vinylene-linked covalent organic frameworks for acidic electrocatalytic hydrogen evolution reaction, Microporous Mesoporous Mater., 2022, 344, 112169 CrossRef CAS.
- C. Shu, H. Du, W. Pu, C. Yang and J. Gong, Trace amounts of palladium-doped hollow TiO2 nanosphere as highly efficient electrocatalyst for hydrogen evolution reaction, Int. J. Hydrogen Energy, 2021, 46, 1923–1933 CrossRef CAS.
- M. Smiljanić, I. Srejić, J. Potočnik, M. Mitrić, Z. Rakočević and S. Štrbac, Synergistic electrocatalytic effect of Pd and Rh nanoislands co-deposited on Au(poly) on HER in alkaline solution, Int. J. Hydrogen Energy, 2018, 43, 19420–19431 CrossRef.
- H. Xu, H. Shang, C. Wang and Y. Du, Recent progress of ultrathin 2D Pd-based nanomaterials for fuel cell electrocatalysis, Small, 2021, 17, 2005092 CrossRef CAS PubMed.
- M. Li, Z. Xia, M. Luo, L. He, L. Tao, W. Yang, Y. Yu and S. Guo, Structural Regulation of Pd-Based Nanoalloys for Advanced Electrocatalysis, Small Sci., 2021, 1, 2100061 CrossRef CAS.
- S. Han, C. He, Q. Yun, M. Li, W. Chen, W. Cao and Q. Lu, Pd-based intermetallic nanocrystals: From precise synthesis to electrocatalytic applications in fuel cells, Coord, Chem. Rev., 2021, 445, 214085 CAS.
- T. Gunji and F. Matsumoto, Electrocatalytic activities towards the electrochemical oxidation of formic acid and oxygen reduction reactions over bimetallic, trimetallic and core–shell-structured Pd-based materials, Inorganics, 2019, 7, 36 CrossRef CAS.
- Q. T. Phan, K. C. Poon and H. Sato, A review on amorphous noble-metal-based electrocatalysts for fuel cells: Synthesis, characterization, performance, and future perspective, Int. J. Hydrogen Energy, 2021, 46, 14190–14211 CrossRef CAS.
- J. Solla-Gullón and J. M. Feliu, State of the art in the electrochemical characterization of the surface structure of shape-controlled Pt, Au, and Pd nanoparticles, Curr. Opin. Electrochem., 2020, 22, 65–71 CrossRef.
|
This journal is © The Royal Society of Chemistry 2023 |