DOI:
10.1039/D3QM00477E
(Review Article)
Mater. Chem. Front., 2023,
7, 4635-4657
Recent progress in metal halide perovskite photocatalysts for hydrogen evolution
Received
28th April 2023
, Accepted 24th August 2023
First published on 25th August 2023
Abstract
Photocatalytic hydrogen production, which directly converts solar energy into green chemical fuel, has received widespread attention. However, despite significant efforts, the efficiency of conventional photocatalytic materials remains below industrial requirements, owing to the intrinsic limitations such as insufficient light absorption and poor carrier transport capability. Metal halide perovskite (MHP) materials feature superior optoelectronic properties and structural flexibility, rendering them highly attractive candidates for photocatalysis. This review provides a concise introduction to the structural characteristics of MHPs and summarizes their recent progress in the field of photocatalytic hydrogen evolution, including single-component MHPs and MHP-based composites. The review also discuss the current challenges and prospects of MHP photocatalysts, which hold promise for advancing photocatalytic solar-to-hydrogen technology.
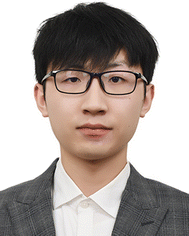
Xing Wang
| Xing Wang received his BS degree from East China University of Science and Technology (ECUST) in 2022. He is currently a PhD candidate at the ECUST under the supervision of Prof. Yu Hou. His research focuses on the design and fabrication of stable perovskite for effective photocatalytic hydrogen evolution. |
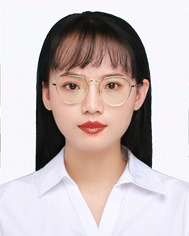
Yu Peng
| Yu Peng completed her PhD from Fujian Institute of Research on the Structure of Matter, Chinese Academy of Sciences in 2021. She is currently a postdoctoral research fellow at ECUST. Her research focuses on the rational design of photoactive materials for photocatalysis. |
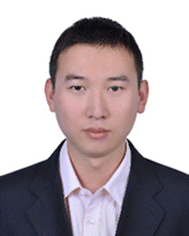
Shuang Yang
| Shuang Yang obtained his BS degree from Tsingtao University of Science and Technology in 2011 and completed his PhD in 2016 from ECUST. He then conducted postdoctoral research at Nanyang Technological University and University of Nebraska-Lincoln. Currently, he is a professor at ECUST. His research focuses on the chemistry and physics of semiconducting materials and optoelectrical devices. |
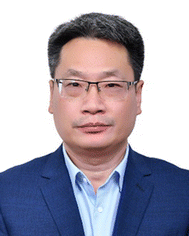
Hua Gui Yang
| Hua Gui Yang obtained his PhD degree from the National University of Singapore in 2005. He joined the University of Queensland in 2007 as a postdoctoral research fellow. After finishing his postdoctoral training, he came back to China and took up a professor position at ECUST at the end of 2008. His research focuses on the rational design and fabrication of functional materials for solar energy conversion. |
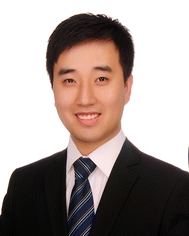
Yu Hou
| Yu Hou completed his BS and PhD from ECUST in 2010 and 2015, respectively. From 2015 to 2017, he worked as a postdoctoral research fellow at ECUST and then joined the Griffith University as a visiting scholar. Currently, he is a professor at ECUST. His research focuses on novel functional materials of thin film-based solar devices and aims at developing new semiconductor absorbers and cell structures towards next-generation photovoltaic technology. |
1. Introduction
Solar energy is a clean and renewable energy source that is abundant, almost infinite, and widely distributed on earth. However, only 1% of the solar energy received by earth has been effectively used,1,2 highlighting the importance of finding a suitable solar energy carrier to use this valuable resource more efficiently.3–5 Hydrogen (H2) is a clean, non-toxic, colorless, and environmentally friendly fuel without carbon dioxide emissions. Additionally, H2 offers a broad range of energy applications, including H2 fuel cells,6–8 combustible fuels,9–11 and synthetic chemical materials.12–14 Thus, H2 serves as an ideal carrier for solar energy. Among the various solar-to-H2 conversion methods (e.g. photoelectrochemical H2 evolution, photothermochemical H2 evolution, and photocatalytic H2 evolution), photocatalytic H2 evolution technology, which converts solar energy to H2 resembling natural photosynthesis, has garnered significant research interests.15–18
Photocatalytic H2 evolution reaction (HER) involves three main steps: (1) absorption of light by a photocatalyst to generate excitons; (2) separation and migration of carriers from the bulk to the surface of the photocatalyst; and (3) reduction and oxidation reactions on the photocatalyst surface. For achieving high-efficiency photocatalytic H2 evolution, photocatalysts need to meet several requirements. First, appropriate energy levels are thermodynamically necessary for suitable photocatalysts. As exemplified by photocatalytic water splitting, the conduction band minimum (CBM) of photocatalysts should locate at a more negative potential than the redox potential of H+/H2 (0 V at pH = 0 with respect to the normal hydrogen electrode (NHE)), the valence band maximum (VBM) of photocatalysts should locate at a more positive potential than the oxide potential of O2/H2O (1.23 V versus NHE), and a minimum bandgap of 1.23 eV is required. In addition, the bandgap of photocatalysts should be narrow enough to make full use of sunlight while satisfying the sufficient overpotential, together with sufficient carrier separation and transport ability. Since the original research of Fujishima and Honda in 1972, a rich variety of semiconductors have been exploited in the field of photocatalytic H2 evolution, such as TiO2,19–21 CdS,22–24 SrTiO3,25–27 and g-C3N4.28–30 However, state-of-the-art solar-to-hydrogen (STH) efficiencies of most reported photocatalysts are still around 1%, far below the commercialization requirements, owing to the intrinsic limitations such as insufficient light absorption, poor carrier transport capability, and prevailing electron–hole recombination. In this context, it is highly urgent to develop a new class of photocatalysts with suitable optical and electronic properties for efficient photocatalytic H2 evolution.
In the past few years, metal halide perovskites (MHPs) have achieved noteworthy breakthroughs in the field of optoelectronics,31–33 including solar cells,34–37 light emitting diodes,38–40 and photodetectors.41–43 Particularly, the light conversion efficiency (PCE) of MHP-based solar cells reached a record of 25.73%,44 suggesting the highly efficient light absorption and charge transport abilities of MHP materials. Emphatically, MHP materials have plentiful superiorities for photocatalysts, such as high light absorption coefficient,45,46 long carrier diffusion lengths, low binding energy,47,48 tunable bandgaps,49,50 and low-cost fabrication, rendering MHPs as viable candidates for photocatalytic H2 evolution.
To date, despite several reviews on MHPs in photocatalysis,51–53 comprehensive assessments focusing on elucidating the crucial relationship between the structure of MHPs (including the intrinsic structure of MHPs and the composites based on MHPs) and their performance in photocatalytic hydrogen evolution have been scarce. In this review, a thorough overview of recent advancements in the field of photocatalytic H2 evolution is provided, including component engineering, energy band adjustment (e.g., extra halogen element doping), and composite structure (e.g., MHPs/conductor, MHPs/semiconductor). A specific emphasis is placed on elucidating the correlation between the structure of MHP-based photocatalysts and their performance in hydrogen evolution (Fig. 1). Additionally, the review highlights the current challenges and provides valuable perspectives for the further development of halide perovskites in photocatalytic H2 evolution. This comprehensive review serves as a valuable reference for the design and development of highly efficient and stable MHP-based photocatalysts for H2 evolution.
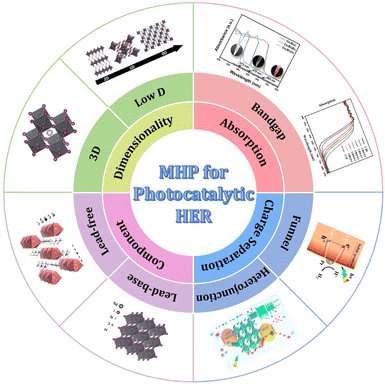 |
| Fig. 1 Schematic overview of metal halide perovskite for photocatalytic hydrogen evolution. | |
2. Structure and properties of metal halide perovskites
MHPs have a general formula ABX3, where A is a univalent organic or inorganic cation, such as methylamine cation (MA+), formamidine cation (FA+), and Cs+; B site is a divalent metal ion, such as Pb2+ and Sn2+; and X is a halogen ion, such as Cl−, Br−, and I−.54,55 As shown in Fig. 2a, the B site and X site form a [BX6]4− octahedron, with B at the center and X at the vertices of the octahedron. Then, [BX6]4− octahedra are connected in three-dimensional (3D) space by sharing vertices to form a 3D network structure. The formability and structural stability of these perovskite-type metal halide materials can be evaluated by tolerance factor t, which is calculated as follows:
where RA, RB, and RX are the effective ionic radii of ion A, ion B, and ion X in perovskite respectively. In general, to form a stable perovskite structure at room temperature, the t values need to be 0.81–1.11. An ideal cubic perovskite will be formed when t is 0.9 to 1.0, while the orthorhombic or tetragonal (Fig. 2b and c) may be formed when t is larger or smaller.56
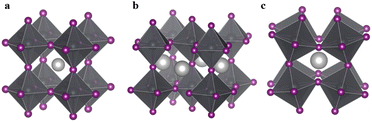 |
| Fig. 2 The different crystal structures of MHPs: (a) cubic structure, (b) tetragonal structure, and (c) orthorhombic structure. | |
The introduction of large-sized organic cations at A site can break down the 3D framework, giving rise to low-dimensional perovskites including two-dimensional (2D), one-dimensional (1D), and zero-dimensional (0D) perovskites (Fig. 3).57 2D perovskites consist of alternating arrangements of inorganic [BX6]4− slabs and the interlayer organic molecules. Notably, the formation of 2D perovskites is not affected by the Goldschmidt tolerance factor, allowing for the utilization of an extensive library of organic cations for their preparation. Based on their crystal structure, 2D perovskites can be divided into Ruddlesden–Popper (RP) phase, Dion–Jacobson (DJ) phase, and the alternating cations in the interlayer space (ACI) phase. The structural formula of RP phase 2D perovskite is A12An−1BnX3n+1, where A1 is a long-chain univalent organic cation such as butylamine cation (BA+) or phenethylamine cation (PEA+), and A is a short-chain organic cation such as MA+ or FA+. n represents the number of inorganic skeleton layers wrapped by long-chain organic cations. When n = 1, it represents a single-layer perovskite at the molecular scale, and when n ≥ 2, it is a multilayer quasi-2D perovskite structure. The structural formula of the DJ phase 2D perovskite is A2An−1BnX3n+1, where A2 represents a long-chain divalent organic cation, such as ethylenediamine cation (EDA2+) or 1,3-propanediamine cation (PDA2+). The structural formula of the ACI phase 2D perovskite is A3AnBnX3n+1, where A3 is limited to some specific univalent organic cations, such as guanidine cation (GA+) and methylphenethylammonium cation (MPA+).58,59
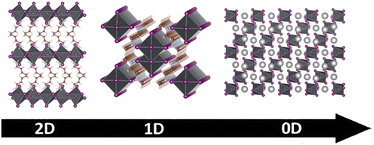 |
| Fig. 3 The crystal structures of 2D, 1D, and 0D perovskite. | |
Further increase in the size of A-site organic cations will lead to a reduction in the structural dimension of MHPs to 1D and 0D. The structure of 1D perovskites consists of [BX6]4- octahedra chains with shells of organic cations, such as [DMEDA]PbBr4 (DMEDA = N,N′-dimethylethylenediamine),60 and [TDMP]PbBr4 (TDMP = trans-2,5-dimethylpiperazine).61 0D perovskites are bulk assemblies of individual [BX6]4− octahedra at the molecular level, where the [BX6]4− octahedra are separated by organic molecules, such as (C9NH20)2SnBr4,62 (C4N2H14Br)4SnBr6.63
On the one hand, the electronic dimension of MHPs decreases as the structural dimension decreases from 3D to 0D.64 Thus, the bandgaps and quantum confinement effect of MHPs increase with decreasing structural dimension, leading to narrower absorption ranges and larger excitation binding energies for low-dimensional MHPs.31,65 On the other hand, in contrast to 3D MHPs, where the A-site cation is limited to a few cations (including MA+, FA+, and Cs+), low-dimensional MHPs are less limited by the Goldschmidt tolerance factor and compatible with an abundance of functional organic cations such as chiral cations,66,67 π-conjugated cations,68,69 and flexible asymmetric cations.70,71 This facilitates the separation and transportation of photogenerated carriers by reducing the quantum confinement effect and forming a polarization-induced electric field. Furthermore, owing to the hydrophobicity of bulky organic cations, low-dimensional MHPs exhibit much better stability than 3D MHPs, which has been confirmed in a lot of previously reported works.72–74
3. Single-component MHP photocatalysts
Driven by their outstanding performance in optoelectronic devices, MHPs have captured significant attention in the field of photocatalysis. However, their susceptibility to moisture poses challenges for their application in photocatalytic water-splitting. Recently, Park et al. have found that MHPs can be stabilized in saturated HI solutions, leading to a surge of interest in photocatalytic HI splitting for H2 production (Fig. 4).75,76 In comparison to water splitting, which involves four electrons, photocatalytic HI splitting utilizes only two electrons,77 resulting in an extremely low overpotential.78,79 Additionally, this reaction presents a feasible alternative to the thermodynamic unfavourable HI thermal decomposition and holds promise for facilitating the industrial iodine-sulphur cycle for H2 generation.80 Consequently, there is considerable potential for designing efficient and stable MHPs for photocatalytic HER. In this section, we discuss the progress of single-component MHPs, classified into Pb-based and lead-free MHPs, for photocatalytic H2 production (Table 1).
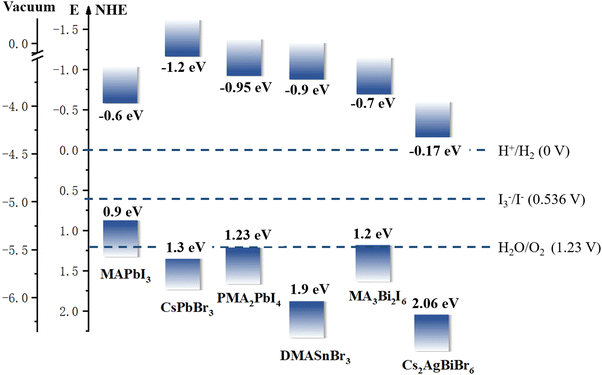 |
| Fig. 4 The schematic diagram of HI splitting with different MHP photocatalysts.81,83,84 | |
Table 1 Summary of works on photocatalytic application of MHPs. R = H2 evolution reaction rate, S = STH efficiency, A = AQE
MHP catalysts |
Test conditions |
Cocatalysts |
MHP usage (mg) |
Performance |
YearRef. |
MAPbI3 |
Xe lamp (λ ≥ 475 nm) |
Pt |
200 |
R = 11.4 μmol h−1 |
201675 |
100 mW cm−2 |
HI solution |
S = 0.81% |
MAPbBr3−xIx |
Xe lamp (300 W, λ ≥ 420 nm) |
Pt |
250 |
R = 651.2 μmol h−1 |
201885 |
100 mW cm−2 |
HI solution |
S = 0.81% |
DMA3BiI6 |
LED (λ = 465 nm, 9 mW) |
Pt |
500 |
R = 47 μmol h−1 |
201886 |
HI solution |
A = 82.8% at 465 nm |
DMASnI3 |
Xe lamp (300 W) |
Pt |
200 |
R = 0.64 μmol h−1 |
201887 |
DI water |
MAPb(I0.9Br0.1)3 |
Xe lamp (300 W, λ ≥ 420 nm) |
Pt |
20 |
R = 66.69 μmol h−1 |
201988 |
100 mW cm−2 |
The mixed HI/HBr solution |
S = 1.42% |
CsPbBr3−xIx |
Xe lamp (λ ≥ 420 nm) |
Pt |
200 |
R = 44.8 μmol h−1 |
201989 |
120 mW cm−2 |
HI solution |
A = 2.15% at 450 nm |
MAPbI3 |
Xe lamp |
Pt |
50 |
R = 11.2 μmol h−1 |
201990 |
120 mW cm−2 |
HI solution |
Ultrasonication (70 W) |
2-AMPSbI5 (Sb-precursor is SbI3) |
ABET 103 solar simulator (150 W, λ = 250-1900 nm) |
|
10 |
R = 1.86 μmol h−1 |
201991 |
100 mW cm−2 |
Methanol solution (10% vol) |
MA3Bi2I9 |
Xe lamp (300 W, λ ≥ 400 nm) |
Pt |
40 |
R = 6.77 μmol h−1 |
201992 |
HI solution |
S = 0.48% |
Cs3Bi0.6Sb1.4I9 |
Simulated sunlight (AM 1.5 G) |
Pt |
100 |
R = 92.6 μmol h−1 |
202093 |
100 mW cm−2 |
HI solution |
MAPbI3 with MA+ film |
Xe lamp (300 W) |
|
50 |
R = 15.65 μmol h−1 |
202194 |
HI solution |
(C6H5CH2NH3)2PbI4 |
Xe lamp (300 W, λ ≥ 420 nm) |
Pt |
150 |
R = 333 μmol h−1 |
202181 |
HI solution |
S = 1.57% |
Cs2Pt0.09Sn0.95Cl6 |
Xe lamp (300 W) |
Pt |
|
R = 16.11 μmol h−1 |
202195 |
TEOA solution (10% vol) |
DMA3BiI6 |
LED lamp (100 W) |
Pt |
50 |
R = 5.7 μmol h−1 |
202196 |
76.4 mW cm−2 |
HI solution |
A = 0.83% at 535 nm |
Defect-rich Cs2AgBiBr6 |
Xe lamp (300 W, λ ≥ 420 nm) |
|
500 |
R = 0.406 μmol h−1 |
202197 |
332.5 mW cm−2 |
HBr solution |
Cs2SnI6 |
Xe lamp (300 W, λ ≥ 420 nm) |
PtSA |
10 |
R = 4.30 μmol h−1 |
202198 |
100 mW cm−2 |
HI solution |
FAPbBr3−xIx |
Xe lamp with an AM1.5G filter (300 W, λ ≥ 420 nm) |
PtSA |
100 |
R = 682.6 μmol h−1 |
202299 |
100 mW cm−2 |
S = 4.50% |
The mixed HI/HBr solution |
A = 33.4% at 530 nm |
OA0.15MA0.85PbI3 |
PL-MW2000 light source in the full visible range |
Pt |
5 |
R = 2.68 μmol h−1 |
202282 |
HI solution |
A = 1.67% at 420 nm |
MA3Bi2Cl9−xIx |
Xe lamp (300 W, λ ≥ 420 nm) |
Pt |
500 |
R = 341 ± 61.7 μmol h−1 |
2022100 |
100 mW cm−2 |
Saturated HCl (or HCl/HI) solution |
A = 1.25% at 435 nm |
Cs3Bi2I9 PNs |
Xe lamp (300 W, λ = 350–780 nm, 790 mW cm−2) |
|
50 |
R = 10.79 μmol h−1 |
2022101 |
Ethanol solution |
3.1 Pb-based perovskites for photocatalytic hydrogen evolution
In 2016, Park et al. successfully demonstrated the first photocatalytic HER of MHP by stabilizing MAPbI3 in its saturated HI solution using the dynamic equilibrium between dissolution and precipitation (as shown in Fig. 5a and b). Under visible light excitation, the photogenerated electrons in MAPbI3 reduced H+ to H2 and the photogenerated holes oxidized I− to I3−. The oxidized I3− ions were subsequently reduced back to I− by H3PO2, achieving sustainable photocatalytic H2 production. With Pt as co-catalyst, the HER rate and STH efficiency of MAPbI3 (200 mg) were measured to be 11.4 μmol h−1 and 0.81%, respectively. This result provided a new solution for the photocatalytic application of MHPs.
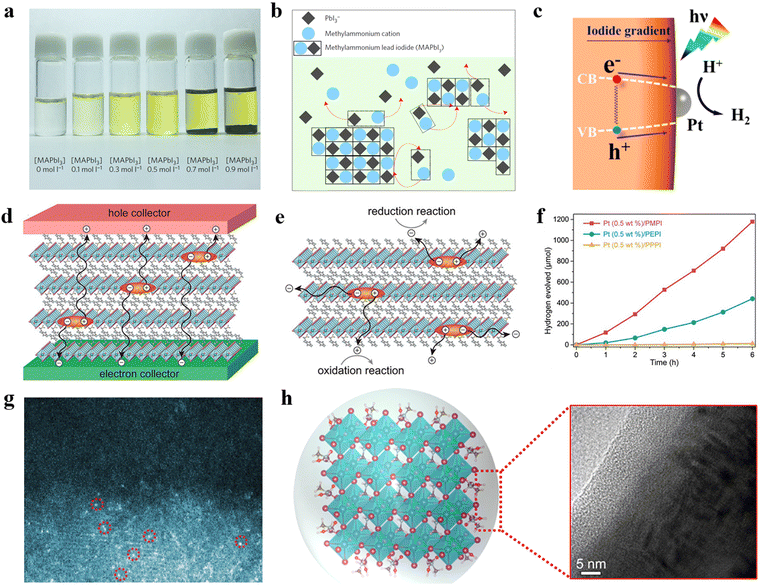 |
| Fig. 5 (a) Photographs of MAPbI3 in different concentrations of HI solution. (b) Diagram of equilibrium dissolution of MAPbI3 powder in saturated HI solution.75 Copyright 2016 Springer Nature. (c) The funnel bandgap of MAPbBr3−xIx. Copyright 2018 American Chemical Society. The schematic diagram of the migration of carriers for 2D perovskite in (d) solar cell and (e) photocatalyst. (f) The photocatalytic HER rates of (C6H5CH2NH3)2PbI4 (PMPI), (C6H5(CH2)2NH3)2PbI4 (PEPI), and (C6H5(CH2)3NH3)2PbI4 (PPPI).81 Copyright 2021 Wiley-VCH GmbH. (g) The scanning transmission election microscope-high angle annular dark field (STEM-HAADF) images of PtSA/FAPbBr3−xIx. Copyright 2022 Wiley-VCH GmbH. (h) Schematic diagram of the growth of organic passivation membrane on the surface of MAPbI3.94 Copyright 2021 American Chemical Society. | |
Following this approach, halide perovskites with different structures have been explored for photocatalytic HER. For example, Wang et al. synthesized three 2D RP perovskites with different lengths of phenylalkylammonium ions (C6H5(CH2)nNH3+; n = 1, 2, and 3).81 In 2D MHP-based solar cells, the 2D MHP layer is consistently oriented parallel to the charge extraction layer, necessitating the passage of carriers through the poorly conductive organic layer for utilization, ultimately resulting in decreased overall charge transport capacity. (Fig. 5d). However, in 2D MHP-based powder photocatalytic systems, carriers traveling along the more conductive inorganic layer can be effectively utilized (Fig. 5e), thus rendering 2D MHPs as promising candidates for photocatalytic HER. Moreover, the excellent moisture resistance of 2D RP MHPs allows the photocatalytic measurement to be carried out in aqueous salt solutions, reducing reliance on strongly acidic HI-saturated solutions. This improvement significantly reduced the acidity of the solution, making the experimental conditions milder, and improved the reproducibility of the measurement due to the lower solubility of 2D perovskite in the aqueous salt solution. Further analysis demonstrated that the length of organic cations could significantly affect the dissolution behaviour, morphology, and photoelectric properties, such as bandgap and band edge position of 2D MHP crystals. Among the investigated 2D MHPs, (C6H5CH2NH3)2PbI4 with the shortest length of organic cations showed the best photocatalytic performance with an HER rate of 333 μmol h−1 (150 mg) and an STH efficiency of 1.57% when using Pt as cocatalyst (Fig. 5f).
In addition, Li et al. combined the 3D perovskite MAPbI3 with the 2D octadecylammonium lead iodide perovskite (OA2PbI4) to create multidimensional perovskites, denoted as OAxMA1−xPbI3.82 These materials exhibited superior charge transfer abilities compared to traditional 3D perovskites and better photocatalytic performance than 2D perovskites. Consequently, OAxMA1−xPbI3 samples showed an optimal photocatalytic HER rate of 2.68 μmol h−1 (5 mg), outperforming bare MAPbI3 (1.38 μmol h−1, 5 mg). This excellent performance was ascribed to their superior absorption properties throughout the visible region compared to other OAxMA1−xPbI3 samples.
Notably, the structural flexibility of MHPs imparts them great potential to tune their energy levels by adjusting halide composition. Huang et al. fabricated a mixed halide perovskite MAPbBr3−xIx (I-doped MAPbBr3) with I gradient distribution in a mixed HI/HBr solution, via a light-assisted halide exchange method. In this material, the concentration of I− increases gradually from the interior to the surface, forming a suitable bandgap funnel structure (Fig. 5c). As a result, MAPbBr3−xIx exhibited an HER rate of 652.1 μmol h−1 (250 mg) and an STH efficiency of 1.05% with the use of Pt as co-catalyst, owing to the bandgap funnel facilitated transfer of photogenerated carriers. Furthermore, Zhao et al. tunned the energy levels of MAPbI3 by one-pot crystallization.88 It should be noticed that there was no bandgap funnel in obtained MAPb(I1−xBrx)3 (Br-doped MAPbI3) by this method. The synthesized mixed halide perovskite showed a championing HER rate of 66.96 μmol h−1 (20 mg) and an outstanding STH efficiency of 1.42% without the use of co-catalysts when x = 0.1. This material exhibited great stability without significant decrease in photocatalytic activity over 252 h (63 runs, 4 h per run).
Similarly, Guan et al. synthesized an all-inorganic perovskite CsPbBr3−xIx with a funnel bandgap for photocatalytic HER.89 The CsPbBr3−xIx/Pt system showed an optimized HER rate of 224 μmol h−1. Moreover, the CsPbBr3−xIx/Pt system also displayed an apparent quantum efficiency (AQE) of 2.15% at 450 nm without obvious decrease in 50 h continuous HER. Such all-inorganic MHPs exhibit better stability compared with organic–inorganic hybrid MHPs, according to the previously reported works in MHPs-based solar cells.102,103
Halide perovskites with FA+ ion as the A cation exhibit significantly longer carrier lifetimes and diffusion lengths compared to those based on MA+ ion,104 making them promising candidates for photocatalytic HER. Wu et al. investigated the photocatalytic HER performance of Pt single atoms (PtSA) loaded FAPbBr3−xIx in mixed HBr/HI solution, whose crystal structure and morphology are depicted in Fig. 5g.99 The PtSAs, which were uniformly distributed on the surface of FAPbBr3−xIx by coordinating with Br/I ions, decreased the free energy of H* adsorption for Pt/FAPbBr3−xIx to almost 0 (around 0.04 eV), leading to an enhanced photocatalytic HER rate of 682.6 μmol h−1 (100 mg) and an impressive STH efficiency of 4.50%.
In addition, Wang et al. demonstrated that ultrasonication could improve the photocatalytic HER performance of MAPbI3, resulting in a 7 times higher photocatalytic HER rate under ultrasonic conditions (23.30 μmol h−1, ultrasonic power = 70 W) than under visible-light conditions alone (3.42 μmol h−1).90 This enhancement in the HER rate can be attributed to the establishment of a build-in polarized electric field triggered by dipole moments under ultrasonication, which can efficiently separate photogenerated carriers and thus enhance catalytic efficiency.
Though great progress has been made, the water-stability of MHPs remains a critical issue that needs to be addressed. To improve their moisture stability, Liu et al. created an organic membrane that effectively passivates MAPbI3, using a light- and solution-based treatment (Fig. 5h).94 The HIO3 molecule on the surface of MAPbI3 formed a hydrogen bond with another HIO3 molecule on the neighbouring MAPbI3 crystal, enhancing the crystallinity of MAPbI3. Moreover, MA+ was anchored to the surface of the HIO3 layer by electrostatic interaction with IO3−, further enhancing the stability of MAPbI3. In consequence, the photocatalytic HER rate was 2.24-fold enhancement than the bare MAPbI3 without a passivating layer.
3.2 Lead-free perovskites for photocatalytic hydrogen evolution
Despite the impressive achievements of Pb-based perovskites in photocatalytic H2 production, the toxicity of Pb limits their further application. To address this issue, lead-free perovskites have been explored, involving the replacement of Pb2+ with Sn2+, Bi3+, Sb3+, or the substitution of two Pb2+ with one univalent metal cation (e.g. K+, Ag+, and Cu+) and one trivalent metal cation (e.g. Sb3+, Bi3+).105–107 In this section, the recent advances in lead-free perovskites for photocatalytic HER are discussed.
3.2.1 Sn-based metal halide perovskites.
Sn, a non-toxic element possessing a similar electron configuration to Pb, is regarded as a promising substitute for Pb. Ju et al. reported the fabrication of a Sn-based halide perovskite, DMASnI3 (DMA = dimethylammonium cation, CH3NH2CH3+) (Fig. 6a), which displayed excellent water stability with no decomposition observed after 16 h of immersion in deionized (DI) water (Fig. 6b).87 They observed that DMASnI3 crystals exhibited invertible bandgap narrowing behaviour and long carrier lifetimes, indicating enormous potential for optoelectronic applications. However, the HER rate of DMASnI3 crystals was measured to be only 0.64 μmol h−1 (Fig. 6c), which could be ascribed to the 1D structure-induced broad bandgap, large effective masses of electrons/holes, and Sn-vacancies induced deep defect states.
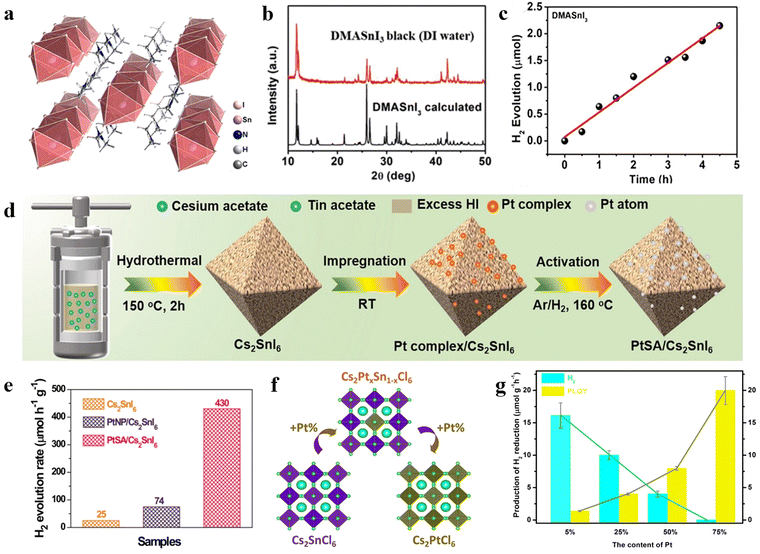 |
| Fig. 6 (a) The crystal structure of DMASnI3. (b) The XRD pattern of black DMASnI3 after treatment of DI water. (c) The photocatalytic HER rate of DMASnI3 in DI water.87 Copyright 2021 Wiley-VCH GmbH. (d) The schematic diagram of PtSA/Cs2SnI6 preparation process. (e) The photocatalytic HER rates of Cs2SnI6, PtNP/Cs2SnI6, and PtSA/Cs2SnI6.98 Copyright 2021 Springer Nature. (f) The crystal structure of Cs2SnCl6 with different Pt doping concentrations. (g) The photocatalytic HER rates and average photoluminescence quantum yields of Cs2PtxSn1−xCl6 (0 < x < 1).95 Copyright 2021 Wiley-VCH GmbH. | |
Furthermore, Zhuo et al. synthesized Cs2SnI6 perovskite and loaded PtSA on its surface using an impregnation-heat activation method (Fig. 6d).98 The unique coordination structure and electronic properties of Pt–I3 sites led to a strong metal–support interaction effect and promoted the photogenerated electron transfer from Cs2SnI6 to the PtSA, reducing the Gibbs free energy and accelerating the H2 generation kinetics. The 0.12 wt% PtSA/Cs2SnI6 demonstrated the highest activity for photocatalytic H2 production, with a rate of 4.30 μmol h−1 (10 mg), which was 17.2- and 5.8-fold enhancement than that of pure Cs2SnI6 and optimized 3.88 wt% Pt nanoparticle/Cs2SnI6, respectively (Fig. 6e).
Yin et al. employed a hydrothermal method to partially replace Sn4+ in Cs2SnI6 with Pt4+ to obtain a range of highly stable Cs2PtxSn1−xCl6 perovskites, where Cs+, Cl−, Sn4+, and Pt4+ ions were uniformly distributed (Fig. 6f).95 Among them, Cs2Pt0.05Sn0.95Cl6 demonstrated remarkable phase stability in water for 25 days and exhibited an excellent photocatalytic HER rate (16.11 μmol h−1). Interestingly, the photocatalytic function of Cs2PtxSn1−xCl6 could be fine-tuned by controlling the amount of Pt4+ (Fig. 6g). As the amount of Pt4+ increased, the photocatalytic HER performance of Cs2PtxSn1−xCl6 decreased.
3.2.2 Bi- and Sb-based metal halide perovskites.
Bi3+ with lone-pair 6s2 exhibits a similar outer electronic configuration as Pb2+. Owing to their shallow carrier traps and excellent transport properties, Bi-based MHPs have emerged as promising candidates for lead-free halide perovskite photocatalysts. In this context, Zhao et al. synthesized a crystalline photocatalyst DMA3BiI6via a solvothermal method (Fig. 7a).86 Photocatalytic measurements were carried out in its well-dispersed HI-H3PO2 system containing separated Pt ions and [BiI6]3− ions. When irradiated at 465 nm, the Pt/DMA3BiI6 photocatalytic system exhibited a superior AQE of ∼82.8% for photocatalytic H2 production, achieving an impressive photocatalytic HER rate of 47 μmol h−1 (500 mg) and showing stable activity without obvious decrease over 100 h. This high activity could be attributed to the efficient separation of carriers at the interface between [BiI6]3− and Pt ions.108 In a subsequent study, they found that DMA3BiI6 could be stabilized in the aqueous DMAI solution for at least two weeks without additional acids or coatings, through a dissolution–recrystallization process of DMA3BiI6 surface molecules.96 However, the photocatalytic performance of DMA3BiI6 in the DMAI solution was unsatisfactory, showing an HER rate of 4.55 μmol h−1 (50 mg) and an AQE of 0.83% at 535 nm with Pt as the co-catalyst.
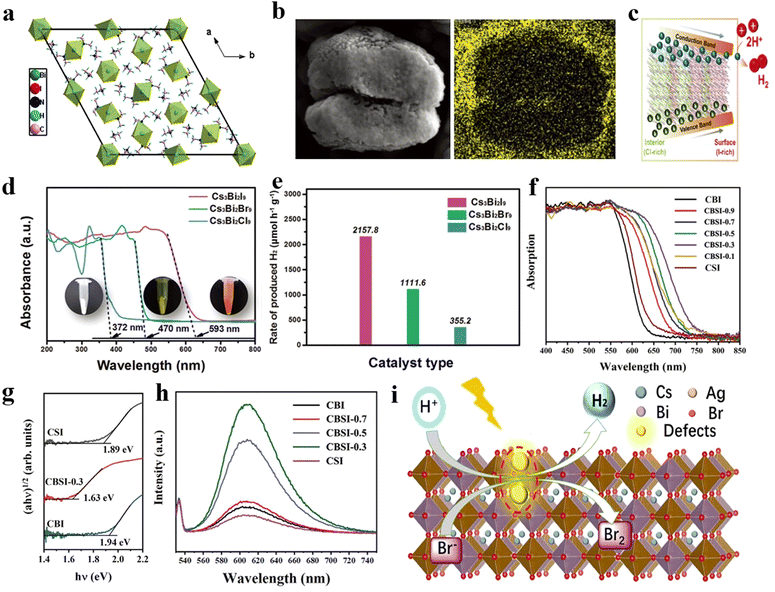 |
| Fig. 7 (a) The crystal structure of DMA3BiI6.86 Copyright 2018 Elsevier. (b)The SEM image (left) and EDS mapping (right) for I of MA3Bi2Cl9−xIx. (c) The funnel bandgap of MA3Bi2Cl9−xIx.100 Copyright 2022 Wiley-VCH GmbH. (d) ultraviolet-visible diffuse reflection spectra (UV-vis DRS) of Cs3Bi2X9 (X = Cl, Br, I) (the inset are physical photographs of them). (e) The photocatalytic HER rates of Cs3Bi2X9 (X = Cl, Br, I).101 Copyright 2022 Wiley-VCH GmbH. (f) The UV-vis DRS of Cs3Bi2xSb2−2xI9 with different x. (g) Optical absorptions of Cs3Bi2I9, Cs3Sb2I9, and Cs3Bi0.6Sb1.4I9. (h) The steady-state photoluminescence spectra of Cs3Bi2I9, Cs3Sb2I9, and Cs3Bi0.6Sb1.4I9.93 Copyright 2020 Wiley-VCH GmbH. (i) The schematic diagram of photocatalytic HER on defect-rich Cs2AgBiBr6.97 Copyright 2021 American Chemical Society. | |
Furthermore, Guo et al. synthesized a Bi-based perovskite MA3Bi2I9 using a facile hydrothermal method, which displayed a photocatalytic HER rate of 6.77 μmol h−1 (40 mg) and an STH efficiency of 0.48%.92 Additionally, it displayed no obvious decrease in HER rate after a 70 h test. In the later study, Tang et al. developed a mixed halide perovskite MA3Bi2Cl9−xIx in which the iodine element exhibited a gradient distribution (Fig. 7b). The MA3Bi2Cl9−xIx possessed a funnel bandgap (Fig. 7c), which could efficiently promote the migration of photogenerated carriers from the bulk to the surface to participate in the photocatalytic redox reaction.100 Consequently, the HER rate of MA3Bi2Cl9−xIx (500 mg) was significantly improved to 341 ± 61.7 μmol h−1 with Pt as the co-catalyst.
Ji et al. fabricated a number of Cs3Bi2X9 perovskites, where X represents Cl, Br, or I.101 They observed that the absorption range of Cs3Bi2X9 increases with the atomic number of halogens (Fig. 7d). Additionally, the Bi–Bi distance in the octahedral units gradually decreases from Cs3Bi2Cl9 to Cs3Bi2I9, alleviating the strong confinement effect of electron–hole pairs and accelerating the separation and transfer of photogenerated charges. Consequently, Cs3Bi2I9 with the higher light absorption exhibited the most outstanding photocatalytic activity, with an HER rate of 107.89 μmol h−1 (50 mg) in ethanol solution (Fig. 7e).
In contrast, under I-rich and Bi-poor conditions, Bi3+ vacancies readily appear in Bi-based perovskites with the formation energy of 1.34 eV.93 These vacancies form deep energy levels within the band gap, impeding the separation of photogenerated carriers and serving as sites for recombination. To confront this challenge, Chen et al. introduced Sb into Cs3Bi2I9 and obtained Cs3Bi2xSb2−2xI9.93 The theoretical calculation confirmed that the addition of Sb resulted in a lower participation of Bi3+ in the conduction band, which weakened the impact of Bi vacancies on the band structure. This effect was reflected in the narrower band gap and fewer intermediate band of Cs3Bi2xSb2−2xI9 than pure Cs3Bi2I9 and Cs3Sb2I9 (Fig. 7f–h), which significantly improved its performance of H2 evolution reaction, with an optimal HER rate of 92.6 μmol h−1 (100 mg) among all reported lead-free perovskites.
Additionally, He et al. prepared a defect-rich Cs2AgBiBr6 by inducing surface defects by visible light, which exhibited better photocatalytic efficiency than Cs2AgBiBr6.97 The defect-rich Cs2AgBiBr6 exhibited an HER rate of 0.406 μmol h−1 (500 mg), surpassing that of pure Cs2AgBiBr6 (0.04 μmol h−1, 500 mg), and exhibited no performance degradation after an 80 h photocatalytic HER.
Similarly, the Sb element, which is adjacent to Pb, also contains ns2 lone pair electrons and exhibits stable positive trivalent state, making it a promising alternative to Pb.109 Rokesh et al. synthesized a new organic–inorganic hybrid perovskite, named 2-(aminomethyl pyridine)SbI5 (2-AMPSbI5), via sol–gel method.91 The 2-AMPSbI5, using Sb-iodide precursors, displayed a promising HER rate (1.86 μmol h−1, 10 mg) in ethanol solution.
4. MHP-based composite photocatalysts
4.1 Band structure of composite photocatalysts
To enhance the separation of photogenerated carriers, composite photocatalysts with heterogeneous structures have been investigated. These composite photocatalysts often consist of a combination of photocatalysts with other materials, including conductors, semiconductors, and insulators. Among the various composites, heterojunctions formed between semiconductors have received significant attention, primarily categorized into I-type, II-type, Z-type, and S-type heterojunctions.
The band arrangement of two semiconductors in a I-type heterojunction is straddling. When exposed to light, photogenerated carriers are transferred from the wide-bandgap semiconductor to the semiconductor with lower conduction and higher valence bands to participate in the photocatalytic reaction (Fig. 8a).110 In contrast, the band arrangement of two semiconductors in a II-type heterojunction is staggered. Under illumination, electrons transfer to the lower conduction band, while holes transfer to the higher valence band, both actively participating in the photocatalytic reaction (Fig. 8b).111 Although the II-type heterojunction enhances charge carrier separation, it comes at the expense of the oxidation–reduction capacity of the two semiconductors. Z-type heterojunctions, named for their photogenerated carrier transfer process resembling the letter “Z”, share a similar structure with II-type heterojunctions.112 However, oxidation and reduction reactions occur in semiconductors with higher conduction band and lower valence band, respectively. The remaining electrons and holes are recombined indirectly or interactively (Fig. 8c). The S-type heterojunction originates from the band bending that occurs at the interface of two semiconductors, which resembles the shape of the letter “S”, and its internal charge transfer is akin to the Z-type heterojunction (Fig. 8d).139 Both S-type and Z-type heterostructures effectively promote charge transfer without compromising the respective oxidation and reduction capabilities of the two semiconductors, garnering considerable research interest in recent years.140–143 Meanwhile, the redox reaction is separated in space, and it is therefore expected to achieve an efficient overall water splitting through the appropriate medium and test system.77
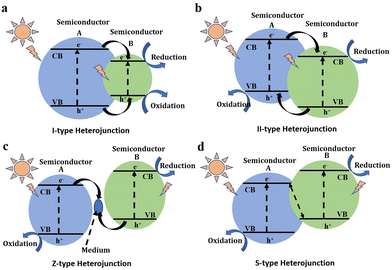 |
| Fig. 8 Schematic diagrams of semiconductor heterojunctions. (a) I-type heterojunction. (b) II-type heterojunction. (c) Z-type heterojunction. (d) S-type heterojunction. | |
In order to improve the hydrogen production performance of MHP-based photocatalysts, various composite structures have been constructed, such as black phosphorus/MAPbI3 I heterojunction,116 MAPbI3/MoS2 II heterojunction,131etc. (Table 2). In the following section, we mainly introduce the applications of MHP/conductor or MHP/semiconductor composites in the photocatalytic HER.
Table 2 Summary of works on photocatalytic application of MHP-based composite catalysts. R = H2 evolution reaction rate, S = STH efficiency, A = AQE
Composite catalysts |
Test conditions |
Total catalyst usage (mg) |
Performance |
YearRef. |
Pt(0.75 wt%)@TiO2/MAPbI3 |
Xe lamp (300 W, λ ≥ 420 nm) |
15 |
R = 79.4 μmol h−1 |
2018113 |
200 mW cm−2 |
S = 0.86% |
HI solution |
A = 70% at 420 nm |
CsPbBr3/Ru@TiO2 |
Continuous wave (CW) laser (445 nm, 50 mW) |
3.2 |
R = 30.72 μmol h−1 |
2018114 |
Trifluoroacetic acid/triethylamine |
A = 0.4 ± 0.1% at 445 nm |
5% rGO/MAPbI3 |
Xe lamp (300 W, λ ≥ 420 nm) |
100 |
R = 93.9 μmol h−1 |
2018115 |
120 mW cm−2 |
A = 1.5% at 450 nm |
HI solution |
1.2% BP/MAPbI3 |
Xe lamp (300 mW cm−2, λ ≥ 420 nm) |
30 |
R = 112.26 μmol h−1 |
2019116 |
25.3 mW cm−2 |
S = 0.93% |
HI solution |
A = 1.5% at 450 nm |
15% Ni3C/MAPbI3 |
Xe lamp (300 mW cm−2, λ ≥ 420 nm) |
50 |
R = 118.1 μmol h−1 |
2019117 |
100 mW cm−2 |
S = 0.91% |
HI solution |
A = 16.6% at 420 nm |
MAPbBr3@Pt/Ta2O5/PEDOT:PSS |
Xe lamp (300 W, λ ≥ 420 nm) |
157.5 |
R = 105 μmol h−1 |
2019118 |
150 mW cm−2 |
A = 16.4% at 420 nm |
HBr solution |
2.5% rGO/Cs2AgBiBr6 |
Xe lamp (300 W, λ ≥ 420 nm) |
200 |
R = 9.78 μmol h−1 |
2020119 |
HBr solution |
A = 0.16% at 450 nm |
g-C3N4/Cs3Bi2I9 (10 : 0.1 wt%) |
Xe lamp (450 W) equipped with a water-filter and a WG320-filter |
6 |
R = 5.52 μmol h−1 |
2020120 |
Methanol solution (10% vol) |
Pt(1.25 wt%)@TiO2/EtbtBi2I10/rGO |
Xe lamp (300 W) |
61 |
R = 16.76 μmol h−1 |
2020121 |
HF solution |
CPD(1 wt%)/MAPbI3/Pt(2 wt%) |
Xe lamp (300 W, λ ≥ 420 nm, 300 mW cm−2) |
103 |
R = 1184.2 μmol h−1 |
2020122 |
100 mW cm−2 |
S = 2.15% |
HI solution |
A = 53.6% at 420 nm |
MoS2 NSs/MAPbI3 |
White-light LED lamps (10 W × 9, 380 nm ≤ λ ≤ 780 nm, 450 mW cm−2) |
105 |
R = 206.1 μmol h−1 |
2020123 |
HI solution |
MA3Bi2I9/DMA3BiI6 |
Xe lamp (300 W, λ ≥ 420 nm) |
200 |
R = 39.64 μmol h−1 |
2020124 |
HI solution |
MAPbI3/CoP |
Xe lamp (150 W, λ ≥ 420 nm) |
ca. 3.12 |
R = 6.5 μmol h−1 |
2020125 |
HF solution |
MAPbI3-MC/ML-MoS2 |
Xe lamp (300 W, λ ≥ 420 nm) |
100 |
R = 1360 μmol h−1 |
2020126 |
100 mW cm−2 |
S = 1.09% |
HI solution |
MoS2(20 wt%)/MA0.6FA0.4PbI3 |
Xe lamp (λ ≥ 420 nm, 300 mW cm−2) |
50 |
R = 66.4 μmol h−1 |
2020127 |
35 mW cm−2 |
S = 0.87% |
HI solution |
A = 14.5% at 550 nm |
DMASnBr3(33 wt%)/g-C3N4@Pt(3 wt%) |
Xe lamp (1500 W, 300–800 nm, 500 W m−2) equipped with UV outdoor filter |
21 |
R = 36.33 μmol h−1 |
2021128 |
TEOA solution (10% vol) |
NiCoB/MAPbI3 |
Xe lamp (300 W, λ ≥ 420 nm) |
65 |
R = 170.7 μmol h−1 |
2021129 |
HI solution |
A = 1.96% at 450 nm |
Cs3Bi2Br9/g-C3N4 |
Xe lamp (1500 W, 300-800 nm, 500 W m−2) equipped with UV outdoor filter |
21 |
R = 22.05 μmol h−1 |
2021130 |
TEOA solution (10% vol) |
MAPbI3/MoS2 nanoflowers |
Xe lamp (280 W, λ ≥ 420 nm, 410 mW cm−2) |
50 |
R = 1469.45 μmol h−1 |
2021131 |
100 mW cm−2 |
S = 7.35% |
HI solution |
A = 22.1% at 500 nm |
Cs2AgBiBr6/N-C |
Xe lamp (300 W, λ ≥ 420 nm) |
10 |
R = 3.8 μmol h−1 |
2021132 |
100 mW cm−2 |
A = 0.59% at 420 nm |
HBr solution |
MAPbI3/PANI NWs |
White-light LED lamps (10 W, 380 nm ≤ λ ≤ 780 nm) |
107 |
R = 38.8 μmol h−1 |
2021133 |
HI solution |
A = 0.3% at 420 nm |
Cs2AgBiBr6/MoS2(20 wt%) |
Xe lamp (300 W, λ ≥ 420 nm, 300 mW cm−2) |
50 |
R = 4.375 μmol h−1 |
2022134 |
41 mW cm−2 |
A = 0.2% at 450 nm |
HBr solution |
CsPbBr3(30 wt%)/ZIF-8 |
Xe lamp (300 W) |
300 |
R = 2.36 μmol h−1 |
2022135 |
Na2SO3 DI water solution |
g-C3N4/Cs2AgBiBr6 (1 : 10 wt%) |
Xe lamp (300 W, λ ≥ 420 nm) |
50 |
R = 3 μmol h−1 |
2022136 |
HBr solution |
NiCoP(12.5% wt%)/Cs2AgBiBr6 |
Xe lamp (300 W, λ ≥ 420 nm) |
100 |
R = 37.32 μmol h−1 |
2022137 |
HBr solution |
CsPbBr3/GO-Pt |
Xe lamp (300 W, λ ≥ 400 nm) |
10 |
R = 10.6 μmol h−1 |
2022138 |
Aromatic alcohol |
4.2 Metal halide perovskite/0D nanoparticles composites
4.2.1 Metal oxide nanoparticles.
TiO2 is often used as an electron transport layer in perovskite solar cells due to its good energy level alignment with MHP materials,144,145 making MHPs/TiO2 composite systems highly promising for photocatalytic HER. Pavliuk et al. first prepared a Pt@TiO2/CsPbBr3 composite photocatalyst in which CsPbBr3 and Pt@TiO2 acted as solar-light harvesters and photocatalysts (Fig. 9a), respectively. Triethylamine and trifluoroacetic acid were employed as a sacrificial agent and an electron donor, respectively, to stabilize CsPbBr3. As a result, the Pt@TiO2/CsPbBr3 system demonstrated an HER rate of approximately 30.72 μmol h−1 (3.2 mg) and an STH efficiency of about 0.4%. Similarly, Wang et al. established a TiO2 electron-transporting channel between Pt and MAPbI3.113 This combination resulted in a significant elevation in the photocatalytic HER rate of 79.4 μmol h−1 (15 mg), an approximately 89-fold increase compared to the HER rate of Pt/MAPbI3. Additionally, the AQE of HER was about 70% at 420 nm, and the STH efficiency was approximately 0.86%. Furthermore, Ta2O5 nanoparticles were loaded with Pt and then combined with MAPbI3 as Ta2O5/MAPbI3/Pt composite photocatalyst, which exhibited an HER rate of 39.9 μmol h−1 (15 mg).113
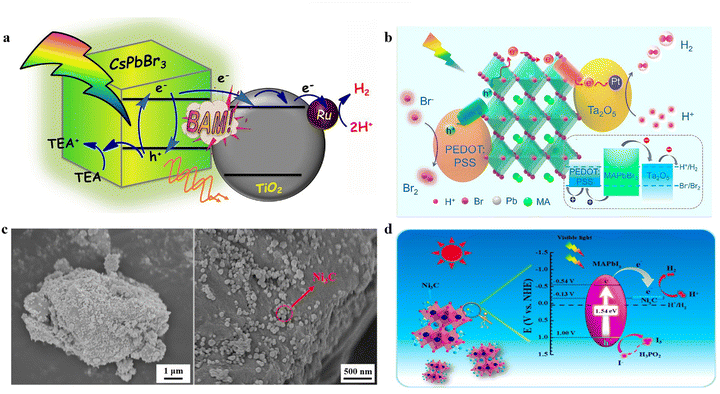 |
| Fig. 9 (a) The schematic diagram of photocatalytic HER on Pt@TiO2/CsPbBr3 in TEOA solution.114 Copyright 2018 Elsevier. (b) The schematic diagram of photocatalytic HER on Pt@Ta2O5/MAPbBr3/PEDOT:PSS in HBr solution.118 Copyright 2019 American Chemical Society. (c) SEM images of Ni3C/MAPbI3. (d) The mechanism of photocatalytic HER of Ni3C/MAPbI3.117 Copyright 2019 American Chemical Society. | |
In a subsequent study, Wang et al. prepared a MAPbBr3@Pt/Ta2O5/PEDOT:PSS composite photocatalyst by simultaneously introducing a photogenerated electron extractor (Ta2O5) and a photogenerated hole extractor (PEDOT:PSS) (Fig. 9b).118 By combining MAPbBr3 with Pt/Ta2O5 and PEDOT:PSS, electron and hole transport channels were established, which greatly improved the charge transport efficiency and realized efficient catalytic reactions. Consequently, the HER rate of the MAPbBr3@Pt/Ta2O5/PEDOT:PSS composite photocatalyst (105 μmol h−1, 157.5 mg) was 52-fold higher than that of pristine MAPbBr3, emphasizing the outstanding advantages of establishing a dual charge transport highway for improving the photocatalytic performance of MHP materials.
4.2.2 Transition metal carbide nanoparticles.
Ni3C, as an earth-abundant and fascinating iron group transition metal carbide, has demonstrated exceptional photocatalytic activity for H2 production in acidic conditions.146–148 Through a simple surface charge-promoted self-assembly technique, Zhao et al. synthesized a MAPbI3/Ni3C composite photocatalyst.117 Scanning electron microscope (SEM) images showed that Ni3C was loaded on the surface of MAPbI3 (Fig. 9c). The photocatalytic process of this composite is shown in the Fig. 9d. The HER rate of 15% Ni3C/MAPbI3 reached 118.1 μmol h−1 (50 mg), which was about 55-fold enhancement than that of MAPbI3 (2.15 μmol h−1, 50 mg) and far higher than that of Pt/MAPbI3 (26.7 μmol h−1, 50 mg). Notably, the photocatalytic HER rate of Ni3C/MAPbI3 showed no obvious decrease after a designed photoreaction process involving 10 cycles, one-month storage, and an additional 10 cycles, highlighting the superb stability of this composite photocatalyst. The excellent photocatalytic HER activity and stability of Ni3C/MAPbI3 were believed to originate from the improved carrier separation and transfer ability, the increased number of reaction sites on the surface of MAPbI3, and the intrinsic stability of the composite photocatalyst.
4.2.3 Transition metal phosphide nanoparticles.
Transition metal phosphides are hopeful substitutes for noble metal cocatalysts to enhance the outstanding carriers’ separation and migration capacity and enable low-cost production.149–151 Using a simple in situ photosynthesis method, Cai et al. first synthesized a MAPbI3/cobalt phosphide (CoP) composite structure.125 CoP nanoparticles could be used as cocatalysts as they can not only effectively extract photoinduced electrons from MAPbI3 and enhance the separation of carriers but also promote interfacial photocatalytic reactions. Based on these advantages, the MAPbI3/CoP composite photocatalyst exhibited an excellent HER rate of ca. 2.45 μmol h−1 (ca. 3.12 mg) in HI solution within 3 h, which was about 8-fold enhancement than that of bare MAPbI3. Moreover, when the photocatalytic reaction time increased to 27 h, its HER rate reached ca. 6.5 μmol h−1 (ca. 3.12 mg), which could be attributed to the ameliorative crystallinity of MAPbI3 and the increased reaction active sites of CoP co-catalyst during the incessant photocatalytic reaction. Furthermore, Huang et al. developed a NiCoP/Cs2AgBiBr6 composite photocatalyst via the electrostatic coupling method.137 With visible-light irradiation, NiCoP acted as an electron extractor to improve the charge separation efficiency of Cs2AgBiBr6, thereby boosting the photocatalytic H2 production ability. Specifically, the composite photocatalyst displayed a maximum HER rate of 37.32 μmol h−1 (100 mg) at a loading amount of 12.5% NiCoP, with approximately 88-fold enhancement over that of pristine Cs2AgBiBr6. In addition, transition metal phosphides are economical and environmentally friendly, favouring their further development.
4.2.4 Carbon-based nanomaterials.
Being part of the carbon-based nanomaterial family, carbonized polymer dots (CPDs) exhibit exceptional potential in enhancing the photocatalytic HER activity of MHP owing to their high photochemical stability, outstanding photophysical, and chemical properties.152,153 Zhao et al. developed a hybrid photocatalytic system by combining Pt/MAPbI3 with CPDs synthesized from citric acid (CA) and p-aminosalicylic acid (PASA), in which the CPDs acted as efficient photogenerated hole extractors. Benefiting from the well-matched energy levels, the optimized Pt/MAPbI3/CA-PASA CPD hybrid photocatalytic system exhibited an outstanding HER rate of 1184.2 μmol h−1 (103 mg), an STH efficiency of 2.15%, and an AQE of 53.6% at 420 nm.
4.3 Metal halide perovskite/2D vdW material composites
In recent years, 2D van der Waals (vdW) materials have demonstrated promising potential in the field of photocatalysis. The advantages of using 2D vdW materials can be summarized as follows: (1) the 2D nature of these materials leads to unique electronic properties that can enhance electron transport and improve charge transfer capacity; (2) the atomic-scale thickness can effectively shorten the carrier diffusion distance and improve carrier utilization; (3) the inherently large specific surface area is beneficial for the adsorption and activation of reactive molecules. Thus, combining MHPs with 2D vdW materials provides great potential for photocatalytic HER.
4.3.1 Graphene derivatives.
Graphene derivatives, such as graphene oxide (GO) and reduced graphene oxide (rGO), which hold good electron transfer properties and acid stability,154–156 have been utilized in MHP-based composites to improve the HER performance of MHP. For instance, Wu et al. synthesized a MAPbI3/rGO composite using a photoreduction method.115 SEM images showed that rGO was loaded on the surface of MAPbI3. During photocatalytic HER, photoinduced electrons in MAPbI3 were transferred to rGO via the Pb–O–C bond to participate in the reduction of H+ at the rGO position, and photogenerated holes oxidized I− to I3− (Fig. 10a). Consequently, the MAPbI3/rGO composite displayed an enhanced HER rate of 93.9 μmol h−1 (100 mg), which was 67-fold enhancement than that of bare MAPbI3. In another study, Chen et al. first demonstrated the selective oxidation of aromatic alcohol to the aromatic aldehyde using CsPbBr3/GO-Pt composite, accompanied by HER.138 GO with abundant functional groups induced the nucleation process of CsPbBr3, leading to the smaller size perovskite nanocrystals. Additionally, GO-Pt acted as photogenerated electron extractors, inhibiting the recombination of electron–hole pairs. Consequently, the optimized CsPbBr3/GO-Pt exhibited an HER rate of 10.6 μmol h−1 (10 mg) with high selectivity (>99%) for benzyl aldehyde generation (10.5 μmol h−1, 10 mg) under visible light, which was about 5-fold enhancement than that of the CsPbBr3-Pt sample.
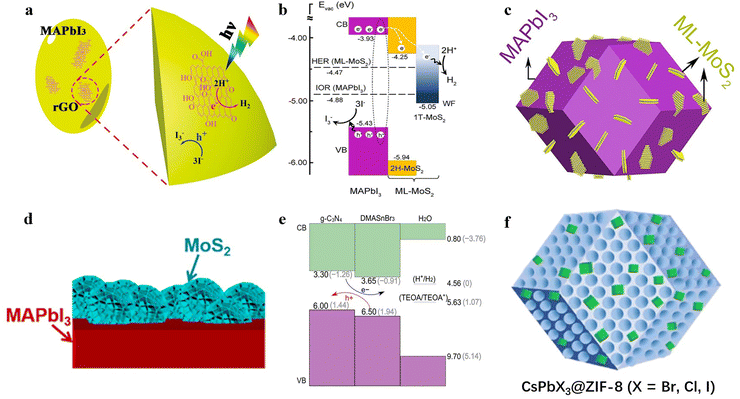 |
| Fig. 10 (a) The schematic diagram of photocatalytic HER on MAPbI3/rGO.115 Copyright 2018 Wiley-VCH GmbH. (b) The schematic diagram of photocatalytic HER on ML-MoS2/MAPbI3-MCs. (c) The schematic illustration of structure of ML-MoS2/MAPbI3-MCs.126 Copyright 2020 Elsevier. (d) The schematic illustration of structure of MoS2 nanoflowers/MAPbI3 microcrystals.131 Copyright 2021 American Chemical Society. (e) The schematic mechanism of photocatalytic HER on g-C3N4@DMASnBr3/Pt in triethanolamine solution.128 Copyright 2018 Wiley-VCH GmbH. (f) The schematic illustration of structure of CsPbX3@ZIF-8 (X = Br, Cl, I).135 Copyright 2022 American Chemical Society. | |
Liu et al. reported a 0D lead-free perovskite (Etbt)4Bi2I10 (Etbt = 3-ethylbenzo[d]thiazol-3-ium) and constructed a (Etbt)4Bi2I10-based composite photocatalyst by introducing Pt/TiO2 and rGO as an electron extractor and a hole extractor, respectively.121 Unlike conventional protonated cations, Etbt does not contain conventional hydrogen-bond donors, which helps prevent hydrogen-bonding interactions from eroding hybrid perovskites. Consequently, (Etbt)4Bi2I10 showed outstanding stability in DI water, acid solution, and ultraviolet light, achiving a potential HER rate of 0.736 μmol h−1 (130 mg) with 0.5 wt% Pt nanoparticles loading. After incorporating Pt/TiO2 and rGO to achieve a dual-charge transport-modulated composite photocatalyst, the photocatalytic system showed a significantly improved HER rate of 16.76 μmol h−1 (61 mg). In a later study, Wang et al. combined Cs2AgBiBr6 with rGO by a photo-reduction process and applied it for HER in a saturated HBr aqueous solution.119 The Cs2AgBiBr6/rGO with optimal rGO loading displayed improved photocatalytic performance compared to that of pure Cs2AgBiBr6, which could be attributed to the promoted separation and migration of carriers by rGO. The HER rate reached 9.78 μmol h−1 (200 mg) under visible light irradiation, without significant decrease for 120 h continuous photocatalytic HER.
4.3.2 Molybdenum disulphide.
MoS2 is a layered material that contains unsaturated sulphur, providing plentiful active sites for H2 at the edges.157–160 Owing to its specific structure and properties, MoS2 has been combined with various semiconductors to improve their catalytic performance in HER.161,162 Wang et al. demonstrated the in situ coupling of MAPbI3 with MoS2 nanosheets (MoS2 NSs), resulting in a hybrid photocatalyst with high efficiency for visible-light-driven photocatalytic HER.123 The composite exhibited excellent HER performance with an HER rate of 206.1 μmol h−1 (105 mg), which was a 121-fold enhancement compared to that of bare MAPbI3. In addition, Zhang et al. fabricated a series of MA1−xFAxPbI3 by partially replacing MA+ with FA+, which was then coupled with MoS2 cocatalyst for photocatalytic HER. The optimized MoS2/MA0.6FA0.4PbI3 composite (i.e., 20 wt% MoS2/MA0.6FA0.4PbI3) exhibited a superior 66.4 μmol h−1 (50 mg) visible HER rate, which was approximately 88-fold enhancement compared to that of pristine MAPbI3 (1.2 μmol h−1, 50 mg). The outstanding photocatalytic HER performances of MoS2/MA0.6FA0.4PbI3 could be attributed to the prolonged lifetime of photoinduced carriers inside MA0.6FA0.4PbI3 due to the partial replacement of MA+ with FA+, and the enhanced carrier transfer and separation capabilities by MoS2 decoration.
Subsequently, Zhao et al. developed a novel II-type heterojunction composite photocatalyst (Fig. 10b), consisting of monolayer MoS2 nanosheets (ML-MoS2) and MAPbI3 microcrystals (MAPbI3-MCs) (Fig. 10c), which could effectively inhibit carriers recombination for high-efficiency photocatalytic HER.126 This discovery developed new possibilities for enhancing the HER performance of perovskite-based photocatalysts, achieving a remarkable STH efficiency of 1.09% and an HER rate of 1360 μmol h−1 (100 mg) under visible light. Furthermore, when other MHP microcrystals such as δ-FAPbI3-MCs, MASnI3-MCs, and MA3Bi2I9-MCs were combined with ML-MoS2 nanosheets, their HER rates increased by 8.4, 14.4, and 26 times, respectively, compared to their respective bare perovskite microcrystals. This indicates a hopeful approach for promoting the photocatalytic HER performance of halide perovskites. To further increase the active area of MoS2, Guan et al. fabricated a MoS2 nanoflowers/MAPbI3 microcrystals heterostructure (Fig. 10d),131 The MoS2 edges offered large amounts of unsaturated S22− that could be considered as active sites for HER.163,164 Specifically, MAPbI3/MoS2 (3
:
7) exhibited a drastic enhancement in the HER rate, reaching up to 1469.45 μmol h−1 (50 mg) and a champion STH efficiency of 7.35%. Based on available research, this is one of the highest STHs in photocatalytic HER. Furthermore, Zhang et al. fabricated an I-type heterostructured MoS2/Cs2AgBiBr6 for efficient and enduring photocatalytic HER via loading MoS2 on Cs2AgBiBr6 using a dissolution–recrystallization method.134 The optimized MoS2/Cs2AgBiBr6 composite achieved an HER rate of 4.375 μmol h−1 (50 mg) in aqueous HBr solution, approximately 20-fold enhancement compared to that of bare Cs2AgBiBr6 (0.215 μmol h−1, 50 mg), and presented an intermittent 500 h photocatalytic HER stability without obvious decrease. The enhanced activity of MoS2/Cs2AgBiBr6 could be attributed to the kinetics-facilitated heterostructure consisting of stable Cs2AgBiBr6 and MoS2.
4.3.3 Graphitic carbon nitride.
g-C3N4 is a promising photocatalyst due to its high surface area, excellent interfacial charge separation and transport capability, and easy preparation, which is expected to integrate with MHPs with high light absorption ability.165–168 Bresolin et al. first fabricated a Cs3Bi2I9/g-C3N4 composite photocatalyst via anchoring Cs3Bi2I9 perovskite on g-C3N4 nanosheets.120 This composite was tested in a methanol solution (10 vol% in water) with an HER rate of 5.52 μmol h−1 (6 mg), which was approximately 46% higher than that of bare g-C3N4. The improved photocatalytic performance was attributed to the enhanced light absorption and electrochemical properties of the newly designed composite. Romani et al. synthesized water-insoluble DMASnBr3/g-C3N4 heterostructures for photocatalytic HER in a 10 vol% triethanolamine (TEOA) solution (Fig. 10e).128 The HER rate of the optimized g-C3N4@DMASnBr3/Pt heterostructure (36.33 μmol h−1, 21 mg) was more than 288-fold higher than that of Pt/DMASnBr3 and 100-fold better than that of bare g-C3N4. This excellent photocatalytic HER performance could be attributed to the good band alignment of g-C3N4/DMASnBr3, which effectively enhance the separation of photogenerated carriers. The researchers also developed a heterostructured photocatalyst by combining Cs3Bi2Br9 with g-C3N4.130 Photocatalytic HER was performed in a 10 vol% aqueous TEOA with Pt as the cocatalyst. The optimal photocatalytic HER rate was achieved when the loading of Cs3Bi2Br9 was 2.5%, reaching approximately 22.05 μmol h−1 (21 mg), which was about an order of magnitude better than that of bare g-C3N4 (1.701 μmol h−1, 21 mg). However, when the Cs3Bi2Br9 loading amount exceeded 5%, the HER capacity of the composite photocatalyst decreased, probably due to the self-trapping of the photogenerated electrons.
Additionally, Song et al. synthesized mesoporous g-C3N4 and then loaded it onto the surface of Cs2AgBiBr6.136 High-resolution transmission electron microscope (HRTEM) images clearly showed the secure contact between Cs2AgBiBr6 and g-C3N4. Spectroscopy analysis indicated the establishment of a II-type heterojunction structure between Cs2AgBiBr6 and g-C3N4. The optimized g-C3N4/Cs2AgBiBr6-10 (with a mass ratio of g-C3N4 and Cs2AgBiBr6 being 1
:
10) exhibited an excellent photocatalytic HER rate of 3 μmol h−1 (50 mg) without using any noble metal cocatalyst, achieving a 2.5-fold enhancement compared to that of the original Cs2AgBiBr6. Moreover, its photocatalytic HER efficiency showed no obvious decrease after storage for 40 days in air condition, indicating excellent stability and photocatalytic recyclability. Jiang et al. utilized a nitrogen-doped carbon (N-C) material based on g-C3N4 and coupled it with Cs2AgBiBr6via a facile hydrothermal method.132,169 The photocatalytic HER performance test found that N-C itself had no photocatalytic HER ability. After combining with Cs2AgBiBr6, the composite material exhibited good photocatalytic HER activity. Particularly, when tested a hydrothermal temperature of 140 °C, Cs2AgBiBr6/N-C-140 exhibited the best HER rate of 3.8 μmol h−1 (10 mg), about 19 times that of the bare Cs2AgBiBr6. This improvement was ascribed to the large surface area of N-C and the presence of nitrogen atoms in N-C, which enhanced electron conductivity and promoted interfacial charge separation between Cs2AgBiBr6 and N-C.
4.3.4 Black phosphorous.
Black phosphorus (BP) with high charge carrier mobility and abundant active sites has also been introduced to improve light absorption and photoinduced carriers separation of MHPs.170,171 For example, Li et al. reported a BP/MAPbI3 heterostructure.116 Under solar-light irradiation, the photogenerated electrons could transfer from perovskite MAPbI3 to BP to participate in the HER. Interestingly, the HER rate of the BP/MAPbI3 heterostructure (112.26 μmol h−1, 30 mg) was more than 100-fold enhancement compared to that of bare MAPbI3. This outstanding photocatalytic HER activity could be ascribed to the construction of a type I heterojunction, which could broaden light harvesting and enhance the separation and migration of carriers at the interface of BP/MAPbI3.
4.3.5 Lamellar transition metal borides.
Jiang et al. developed a NiCoB/MAPbI3 composite photocatalyst by combining amorphous NiCoB with a nanoscale lamellar structure, and MAPbI3 using electrostatic self-assembly.129 The photogenerated electrons from MAPbI3 were able to transfer to NiCoB and participate in the reduction of H+, thereby promoting the separation of photoinduced carriers. At a NiCoB loading of 30%, the photocatalytic HER rate of MAPbI3 reached a maximum of 170.7 μmol h−1 (65 mg) under visible light irradiation. Additionally, the composite showed good stability in a 24-hour cycling photocatalytic HER.
4.4 Metal halide perovskite/1D semiconductor composites
Conductive 1D polyaniline nanowires (PANI NWs) with high electrical conductivity and excellent chemical stability have been coupled with MHP to create high-performance composite photocatalysts. For example, Li et al. fabricated a high-performance 3D/1D heterostructured composite photocatalyst by combining conductive 1D polyaniline nanowires (PANI NWs) with MAPbI3 using an in situ doping-induced assembly method.133 SEM images obtained by Li et al. showed that PANI NWs were firmly embedded on the surface of MAPbI3. The MAPbI3/PANI NWs composite photocatalyst showed a remarkable 29-fold increase in the photocatalytic HER rate (38.8 μmol h−1, 107 mg) compared to that of pristine MAPbI3 microcrystals. Additionally, this composite photocatalyst demonstrated good stability during 30 h of irradiation, with each cycle lasting 6 h.
4.5 Metal halide perovskite/other material composites
In addition to the aforementioned materials, other semiconductors have also been used to couple with MHPs to create efficient photocatalytic systems. One such material is metal–organic frameworks (MOFs), which possess a large specific surface area, adjustable morphology, and diverse structure.172,173 By coupling MOFs with MHPs, the photocatalytic HER performance of MHPs can be enhanced. For instance, Feng et al. fabricated a CsPbBr3/zeolitic imidazolate framework (ZIF-8) composite using the mechanical milling method. This fabrication method was expected to enable large-scale synthesis of ZIF-8 composites (Fig. 10f).135 SEM images showed that ZIF-8 was loaded on the surface of CsPbBr3. The HER rate of this composite was measured to be ∼11 times higher (2.36 μmol·h−1, 300 mg, 2.5 h) than that of ZIF-8 alone (0.205 μmol·h−1, 300 mg, 2.5 h), benefiting from the ZIF-8 promoted photogenerated carrier separation. Moreover, ZIF-8 was discerned to enhance the stability of CsPbBr3 in aqueous solution, with only ∼8% decrease in H2 productivity after 4 weeks.
In addition to the above-mentioned strategies, the formation of MHP-MHPs heterojunctions has also been demonstrated as an effective approach to obtaining high-performance photocatalytic systems. For example, Tang et al. developed a lead-free Bi-based hybrid perovskite heterojunction, MA3Bi2I9/DMA3BiI6, through an in situ growth method.124 The VB and CB positions of MA3Bi2I9 and DMA3BiI6 showed a well-matched II-type heterostructure, which facilitates the formation of an interfacial carriers transfer pathway at the interface of MA3Bi2I9 and DMA3BiI6. This resulted in an HER rate of 39.64 μmol h−1 (200 mg) without the addition of Pt co-catalysts.
5. Summary and prospects
MHPs have garnered significant interest in the field of photocatalytic H2 evolution due to their outstanding photoelectric properties and tunable crystal structure. This review comprehensively discusses the structure and photocatalytic HER performance of different kinds of MHPs, including both lead-based perovskites and lead-free perovskites. Additionally, we summarize the developed strategies used to improve the photocatalytic performance of MHPs, including energy band structure adjustment (e.g., extra halogen element doping), and composite structures (e.g., MHPs/conductor, MHPs/semiconductor). However, despite their potential, the current photocatalytic performance of MHPs remains unsatisfactory for practical application, due to energy loss during charge generation, separation, and transfer, as well as concerns about the instability and toxicity of MHPs. In light of current MHP research, we propose several possible methods to further enhance the photocatalytic performance of MHP-based photocatalysts.
(1) Designing MHPs with optimal intrinsic crystalline structures at the molecular level. Photocatalysts play a crucial role in photocatalytic reactions, encompassing light absorption, photogenerated carriers generation, separation, transport, and surface redox reactions. To achieve efficient H2 evolution, photocatalysts require appropriate electronic structures, wide light absorption range, large absorption coefficients, and excellent carrier transport performance.174 The strong structural diversity and designability of MHPs make them highly advantageous for the design of photocatalysts. Emphatically, incorporating bulky organic cations at the A site can improve the intrinsic moisture resistance of MHPs, owing to the hydrophobic nature of the organic cations. However, introducing bulky organic cations may cause the breakdown of the 3D perovskite framework, leading to the formation of low-dimensional perovskites such as 2D, 1D, and 0D perovskites. These low-dimensional perovskites, especially 1D and 0D perovskites, usually exhibit stronger quantum confinement effects and wider bandgaps compared to their 3D analogues, which may impair their optoelectronic properties.65 Thus, striking a balance between optoelectronic performance and stability in MHPs design is of utmost importance. For instance, quasi-2D MHPs with a general formula of A2An−1′PbnI3n+1, (where A′ is a bulky organic cation and A is MA or FA) have demonstrated higher environmental stability compared with MAPbI3, due to the protection of the hydrophobic organic spacers. In particular, solar cells based on quasi-2D MHPs have recently achieved a remarkable power conversion efficiency (PCE) of 22.26%, indicating the preserved excellent semiconducting properties of these materials.175 Further theoretical calculations and molecular engineering hold promise in promoting the development of MHPs for efficient and stable photocatalytic HER.
(2) Constructing band-matched heterostructured MHP composites. Although some strategies have achieved improved carrier separation efficiency, they often come at the expense of redox ability, ultimately leading to unsatisfactory photocatalytic HER performance. Additionally, the existence of photoinduced electron–hole pairs in the pristine photocatalyst may hinder the efficient interfacial transfer of electron–hole pairs in other catalysts. To overcome these limitations and further improve the photocatalytic HER performance of MHPs, novel forms of heterostructures, such as Z- and S-scheme heterojunctions, are expected.139,176 By selectively consuming or recombining a certain portion of photogenerated electrons and holes, electrons and holes with stronger redox ability can be retained, thus enhancing the photocatalytic activity of these heterojunctions. Moreover, it is important to consider the condition under which most MHPs-based photocatalytic HERs are realized, often using a HI solution. Thus, selecting a suitable acid-resistant photocatalyst for oxygen evolution based on the I−/I3− redox pair is expected to improve the efficiency of the photocatalytic HER process and enable overall water splitting.
(3) Developing encapsulation layers. Encapsulation is a crucial step in improving the stability of photocatalytic plate devices, as the water-instability of MHPs presents a significant challenge in the context of photocatalytic H2 production. An ideal encapsulation material should possess excellent stability, good electrical conductivity, and light transmission. There are generally two types of encapsulations: full coverage and edge encapsulation. Full coverage encapsulation involves preparing the encapsulation layer on top of the module, while edge encapsulation involves placing a sealant around the module.177–179 Edge encapsulation offers advantages such as reduced impact on the contact layer, lowered light transmission requirements, and minimized potential side reactions between the encapsulating materials and the MHPs. However, the encapsulation effect may be slightly reduced compared to full coverage encapsulation. To further increase the barrier effect, desiccant can be introduced into the edge encapsulation process. Fully covered encapsulation provides better protection, but it has a greater impact on the MHP layer and has higher light transmission requirements, as it directly contacts the MHP functional layer. An alternative approach involves multilayer encapsulation, which combines the benefits of both full coverage and edge encapsulation methods to improve the water stability of MHPs while maintaining their photocatalytic activity.180 However, it is important to note that this method is may be challenging to fabricate and could incur higher expensive.
(4) Developing lead-free MHP photocatalysts. Among the candidates, Sn-based perovskites have shown promise due to the similarity of Sn2+ to Pb2+ in terms of electron configuration and ionic radius. However, the rapid oxidation of Sn2+ to Sn4+ remains a challenge. Various stabilization methods have been explored, such as adding reducing agents (e.g. H3PO2),181 but their stability remains inferior to Pb-based perovskites. A deeper understanding of Sn2+ bonding interactions in Sn-based perovskites could offer insights into addressing this issue. Similarly, Ge-based perovskites also face the challenge of Ge2+ oxidation. Bi-, Sb-based perovskites and double perovskites have demonstrated good stability under various conditions. However, their photocatalytic performance is generally limited by poor optoelectronic properties arising from their low electronic dimensional.182 Therefore, a promising direction for MHP-based photocatalytic H2 evolution is the rational design of Bi-, Sb-based perovskites and double perovskites with high electronic dimensional using theoretical calculations and molecular engineering.
Conflicts of interest
There are no conflicts to declare.
Acknowledgements
This work was financially supported by National Ten Thousand Talent Program for Young Top-notch Talent, National Natural Science Fund for Excellent Young Scholars (52022030), National Natural Science Foundation of China (51972111, 52203330), the Science and Technology Innovation Plan of Shanghai Science and Technology Commission (22YF1410000), Shanghai Pilot Program for Basic Research (22TQ1400100-5), “Dawn” Program of Shanghai Education Commission (22SG28), Shanghai Municipal Natural Science Foundation (22ZR1418000), Postdoctoral Research Foundation of China (2021M701190) and Shanghai Engineering Research Center of Hierarchical Nanomaterials (18DZ2252400).
Notes and references
- J. Sun, D. K. Zhong and D. R. Gamelin, Composite photoanodes for photoelectrochemical solar water splitting, Energy Environ. Sci., 2010, 3, 1252–1261 RSC.
- J. Song, T. L. Kim, J. Lee, S. Y. Cho, J. Cha, S. Y. Jeong, H. An, W. S. Kim, Y.-S. Jung, J. Park, G. Y. Jung, D.-Y. Kim, J. Y. Jo, S. D. Bu, H. W. Jang and S. Lee, Domain-engineered BiFeO3 thin-film photoanodes for highly enhanced ferroelectric solar water splitting, Nano Res., 2018, 11, 642–655 CrossRef CAS.
- J. Liu, Y. Liu, N. Y. Liu, Y. Z. Han, X. Zhang, H. Huang, Y. Lifshitz, S. T. Lee, J. Zhong and Z. H. Kang, Metal-free efficient photocatalyst for stable visible water splitting via a two-electron pathway, Science, 2015, 347, 970–974 CrossRef CAS PubMed.
- Y. O. Wang, A. Vogel, M. Sachs, R. S. Sprick, L. Wilbraham, S. J. A. Moniz, R. Godin, M. A. Zwijnenburg, J. R. Durrant, A. I. Cooper and J. W. Tang, Current understanding and challenges of solar-driven hydrogen generation using polymeric photocatalysts, Nat. Energy, 2020, 5, 633 CrossRef.
- W. Bi, X. Li, L. Zhang, T. Jin, L. Zhang, Q. Zhang, Y. Luo, C. Wu and Y. Xie, Molecular co-catalyst accelerating hole transfer for enhanced photocatalytic H2 evolution, Nat. Commun., 2015, 6, 8647 CrossRef CAS PubMed.
- M. Z. Jacobson, W. G. Colella and D. M. Golden, Cleaning the air and improving health with hydrogen fuel-cell vehicles, Science, 2005, 308, 1901–1905 CrossRef CAS PubMed.
- J. Pan, Y. Sun, W. Li, J. Knight and A. Manthiram, A green lead hydrometallurgical process based on a hydrogen-lead oxide fuel cell, Nat. Commun., 2013, 4, 2178 CrossRef PubMed.
- Y. Yang, X. He, P. Zhang, Y. H. Andaloussi, H. Zhang, Z. Jiang, Y. Chen, S. Ma, P. Cheng and Z. Zhang, Combined intrinsic and extrinsic proton conduction in robust covalent organic frameworks for hydrogen fuel cell applications, Angew. Chem., Int. Ed., 2020, 59, 3678–3684 CrossRef CAS PubMed.
- S. Manigandan, R. Sarweswaran, P. B. Devi, Y. Sohret, A. Kondratiev, S. Venkatesh, M. R. Vimal and J. J. Joshua, Comparative study of nanoadditives TiO2, CNT, Al2O3, CuO and CeO2 on reduction of diesel engine emission operating on hydrogen fuel blends, Fuel, 2020, 262, 116336 CrossRef CAS.
- C. Gong, Z. Li, J. Sun and F. Liu, Evaluation on combustion and lean-burn limit of a medium compression ratio hydrogen/methanol dual-injection spark-ignition engine under methanol late-injection, Appl. Energy, 2020, 277, 115622 CrossRef CAS.
- C. Acar and I. Dincer, The potential role of hydrogen as a sustainable transportation fuel to combat global warming, Int. J. Hydrogen Energy, 2020, 45, 3396–3406 CrossRef CAS.
- J. Zhong, X. Yang, Z. Wu, B. Liang, Y. Huang and T. Zhang, State of the art and perspectives in heterogeneous catalysis of CO2 hydrogenation to methanol, Chem. Soc. Rev., 2020, 49, 1385–1413 RSC.
- C. Mao, J. Wang, Y. Zou, G. Qi, J. Y. Yang Loh, T. Zhang, M. Xia, J. Xu, F. Deng, M. Ghoussoub, N. P. Kherani, L. Wang, H. Shang, M. Li, J. Li, X. Liu, Z. Ai, G. A. Ozin, J. Zhao and L. Zhang, Hydrogen spillover to oxygen vacancy of TiO2–xHy/Fe: Breaking the scaling relationship of ammonia synthesis, J. Am. Chem. Soc., 2020, 142, 17403–17412 CrossRef CAS PubMed.
- D. Wu, K. Deng, B. Hu, Q. Lu, G. Liu and X. Hong, Plasmon-assisted photothermal catalysis of low-pressure CO2 hydrogenation to methanol over Pd/ZnO catalyst, ChemCatChem, 2019, 11, 1598–1601 CrossRef CAS.
- Q. Xiang, J. Yu and M. Jaroniec, Synergetic effect of MoS2 and graphene as cocatalysts for enhanced photocatalytic H2 production activity of TiO2 nanoparticles, J. Am. Chem. Soc., 2012, 134, 6575–6578 CrossRef CAS PubMed.
- J. Ran, J. Zhang, J. Yu, M. Jaroniec and S. Z. Qiao, Earth-abundant cocatalysts for semiconductor-based photocatalytic water splitting, Chem. Soc. Rev., 2014, 43, 7787–7812 RSC.
- Q. Lu, Y. Yu, Q. Ma, B. Chen and H. Zhang, 2D transition-metal-dichalcogenide-nanosheet-based composites for photocatalytic and electrocatalytic hydrogen evolution reactions, Adv. Mater., 2016, 28, 1917–1933 CrossRef CAS PubMed.
- M. Zhu, Z. Sun, M. Fujitsuka and T. Majima, Z-scheme photocatalytic water splitting on a 2D heterostructure of black phosphorus/bismuth vanadate using visible light, Angew. Chem., Int. Ed., 2018, 57, 2160–2164 CrossRef CAS PubMed.
- Q. Guo, Z. Ma, C. Zhou, Z. Ren and X. Yang, Single molecule photocatalysis on TiO2 surfaces, Chem. Rev., 2019, 119, 11020–11041 CrossRef CAS PubMed.
- S. Jayachitra, D. Mahendiran, P. Ravi, P. Murugan and M. Sathish, Highly conductive NiSe2 nanoparticle as a co-catalyst over TiO2 for enhanced photocatalytic hydrogen production, Appl. Catal., B, 2022, 307, 121159 CrossRef CAS.
- H. G. Yang, C. H. Sun, S. Z. Qiao, J. Zou, G. Liu, S. C. Smith, H. M. Cheng and G. Q. Lu, Anatase TiO2 single crystals with a large percentage of reactive facets, Nature, 2008, 453, 638–641 CrossRef CAS PubMed.
- Q. Sun, N. Wang, J. Yu and J. C. Yu, A hollow porous CdS photocatalyst, Adv. Mater., 2018, 30, 1804368 CrossRef PubMed.
- H. Ren, J.-L. Yang, W.-M. Yang, H.-L. Zhong, J.-S. Lin, P. M. Radjenovic, L. Sun, H. Zhang, J. Xu, Z.-Q. Tian and J.-F. Li, Core–shell–satellite plasmonic photocatalyst for broad-spectrum photocatalytic water splitting, ACS Mater. Lett., 2021, 3, 69–76 CrossRef CAS.
- C.-Q. Li, X. Du, S. Jiang, Y. Liu, Z.-L. Niu, Z.-Y. Liu, S.-S. Yi and X.-Z. Yue, Constructing direct Z-scheme heterostructure by enwrapping ZnIn2S4 on CdS hollow cube for efficient photocatalytic H2 generation, Adv. Sci., 2022, 9, 2201773 CrossRef CAS PubMed.
- L. Mu, Y. Zhao, A. Li, S. Wang, Z. Wang, J. Yang, Y. Wang, T. Liu, R. Chen, J. Zhu, F. Fan, R. Li and C. Li, Enhancing charge separation on high symmetry SrTiO3 exposed with anisotropic facets for photocatalytic water splitting, Energy Environ. Sci., 2016, 9, 2463–2469 RSC.
- C. M. Pelicano, M. Saruyama, R. Takahata, R. Sato, Y. Kitahama, H. Matsuzaki, T. Yamada, T. Hisatomi, K. Domen and T. Teranishi, Bimetallic synergy in ultrafine cocatalyst alloy nanoparticles for efficient photocatalytic water splitting, Adv. Funct. Mater., 2022, 32, 2202987 CrossRef CAS.
- J. Pan, Z. Chen, P. Wang, P. Wang, Q. Yu, W. Zhao, J. Wang, M. Zhu, Y. Zheng and C. Li, The overall water splitting of CdS/Ti3+-SrTiO3 core–shell heterojunction via OER enhancement of MnOx nanoparticles, Chem. Eng. J., 2021, 424, 130357 CrossRef CAS.
- Q. Liu, J. Shen, X. Yu, X. Yang, W. Liu, J. Yang, H. Tang, H. Xu, H. Li, Y. Li and J. Xu, Unveiling the origin of boosted photocatalytic hydrogen evolution in simultaneously (S, P, O)-codoped and exfoliated ultrathin g-C3N4 nanosheets, Appl. Catal., B, 2019, 248, 84–94 CrossRef CAS.
- C. Hu, F. Chen, Y. Wang, N. Tian, T. Ma, Y. Zhang and H. Huang, Exceptional cocatalyst-free photo-enhanced piezocatalytic hydrogen evolution of carbon nitride nanosheets from strong in-plane polarization, Adv. Mater., 2021, 33, 2101751 CrossRef CAS PubMed.
- Z. Wang, Z. Wang, X. Zhu, C. Ai, Y. Zeng, W. Shi, X. Zhang, H. Zhang, H. Si, J. Li, C.-Z. Wang and S. Lin, Photodepositing CdS on the active cyano groups decorated g-C3N4 in Z-scheme manner promotes visible-light-driven hydrogen evolution, Small, 2021, 17, 2102699 CrossRef CAS PubMed.
- Y. Liu, B. Dong, A. Hagfeldt, J. Luo and M. Graetzel, Chemically tailored molecular surface modifiers for efficient and stable perovskite photovoltaics, SmartMat, 2021, 2, 33–37 CrossRef CAS.
- Y. Peng, X. Wang, L. Li, H. Ye, S. Yang, H. G. Yang, J. Luo and Y. Hou, Moisture-resistant chiral perovskites for white-light circularly polarized photoluminescence, Adv. Opt. Mater., 2023, 11, 2201888 CrossRef CAS.
- C. Zou, Z. Zhou, X. Liu, F. Zhang, J. Xie, Y. Su, S. Yang and Y. Hou, Multication tin-lead perovskite photodiodes with engineered lattice strain for ultrasensitive broadband photodetection, Adv. Opt. Mater., 2022, 10, 2201769 CrossRef CAS.
- Y. Yin, Z. Guo, G. Chen, H. Zhang and W.-J. Yin, Recent progress in defect tolerance and defect passivation in halide perovskite solar cells, Acta Phys. Chim. Sin., 2021, 4, 2008048 Search PubMed.
- Y.-H. Zhang and Y. Li, Interface materials for perovskite solar cells, Rare Met., 2021, 40, 2993–3018 CrossRef CAS.
- Q. Li, Y. Zheng, Z. Wei, J. Xie, C. Zou, X. Liu, D. Liu, Z. Zhou, H. G. Yang, S. Yang and Y. Hou, Halide diffusion equilibrium and its impact on efficiency evolution of perovskite solar cells, Adv. Energy Mater., 2022, 12, 2202982 CrossRef CAS.
- Y. Shi, J. He, H. Lian, X. Liu, H. Yuan, Y. Hou, S. Yang and H. G. Yang, Cooperative adsorption of metal-organic complexes on CsPbI2Br perovskite surface for photovoltaic efficiency exceeding 17%, ChemSusChem, 2022, 15, e202201394 CrossRef CAS PubMed.
- Z. Yuan, Z. Hu, I. Persson, C. Wang, X. Liu, C. Kuang, W. Xu, S. Bai and F. Gao, Interface-assisted cation exchange enables high-performance perovskiteLEDs with tunable near-infrared emissions, Joule, 2022, 6, 2423–2436 CrossRef CAS.
- C. Zhang, S. Wang, X. Li, M. Yuan, L. Turyanska and X. Yang, Core/shell perovskite nanocrystals: Synthesis of highly efficient and environmentally stable FAPbBr3/CsPbBr3 for LED applications, Adv. Funct. Mater., 2020, 30, 1910582 CrossRef CAS.
- E.-P. Yao, Z. Yang, L. Meng, P. Sun, S. Dong, Y. Yang and Y. Yang, High-brightness blue and white LEDs based on inorganic perovskite nanocrystals and their composites, Adv. Mater., 2017, 29, 1606859 CrossRef PubMed.
- D. H. Chun, Y. J. Choi, Y. In, J. K. Nam, Y. J. Choi, S. Yun, W. Kim, D. Choi, D. Kim, H. Shin, J. H. Cho and J. H. Park, Halide perovskite nanopillar photodetector, ACS Nano, 2018, 12, 8564–8571 CrossRef CAS PubMed.
- L. Martínez-Goyeneche, L. Gil-Escrig, I. Susic, D. Tordera, H. J. Bolink and M. Sessolo, Narrowband monolithic perovskite–perovskite tandem photodetectors, Adv. Opt. Mater., 2022, 10, 2201047 CrossRef.
- W. Tian, H. Zhou and L. Li, Hybrid organic–inorganic perovskite photodetectors, Small, 2017, 13, 1702107 CrossRef PubMed.
- J. Park, J. Kim, H.-S. Yun, M. J. Paik, E. Noh, H. J. Mun, M. G. Kim, T. J. Shin and S. I. Seok, Controlled growth of perovskite layers with volatile alkylammonium chlorides, Nature, 2023, 616, 724–730 CrossRef CAS PubMed.
- S. Sun, T. Salim, N. Mathews, M. Duchamp, C. Boothroyd, G. Xing, T. C. Sum and Y. M. Lam, The origin of high efficiency in low-temperature solution-processable bilayer organometal halide hybrid solar cells, Energy Environ. Sci., 2014, 7, 399–407 RSC.
- N.-G. Park, Perovskite solar cells: an emerging photovoltaic technology, Mater. Today, 2015, 18, 65–72 CrossRef CAS.
- W. Zhang, M. Saliba, S. D. Stranks, Y. Sun, X. Shi, U. Wiesner and H. J. Snaith, Enhancement of perovskite-based solar cells employing core–shell metal nanoparticles, Nano Lett., 2013, 13, 4505–4510 CrossRef CAS PubMed.
- V. D’Innocenzo, G. Grancini, M. J. P. Alcocer, A. R. S. Kandada, S. D. Stranks, M. M. Lee, G. Lanzani, H. J. Snaith and A. Petrozza, Excitons versus free charges in organo-lead tri-halide perovskites, Nat. Commun., 2014, 5, 3586 CrossRef PubMed.
- C. Zhou, Q. Ou, W. Chen, Z. Gan, J. Wang, Q. Bao, X. Wen and B. Jia, Illumination-induced halide segregation in gradient bandgap mixed-halide perovskite nanoplatelets, Adv. Opt. Mater., 2018, 6, 1801107 CrossRef.
- W. Zhou, P. Han, X. Zhang, D. Zheng, S. Yang, Y. Yang, C. Luo, B. Yang, F. Hong, D. Wei, R. Lu and K. Han, Lead-free small-bandgap Cs2CuSbCl6 double perovskite nanocrystals, J. Phys. Chem. Lett., 2020, 11, 6463–6467 CrossRef CAS PubMed.
- S. Purohit, K. L. Yadav and S. Satapathi, Metal halide perovskite heterojunction for photocatalytic hydrogen generation: Progress and future opportunities, Adv. Mater. Interfaces, 2022, 9, 2200058 CrossRef CAS.
- K. Ren, S. Yue, C. Li, Z. Fang, K. A. M. Gasem, J. Leszczynski, S. Qu, Z. Wang and M. Fan, Metal halide perovskites for photocatalysis applications, J. Mater. Chem. A, 2022, 10, 407–429 RSC.
- B.-M. Bresolin, Y. Park and D. W. Bahnemann, Recent progresses on metal halide perovskite-based material as potential photocatalyst, Catalysts, 2020, 10, 709 CrossRef CAS.
- G. E. Eperon, G. M. Paternò, R. J. Sutton, A. Zampetti, A. A. Haghighirad, F. Cacialli and H. J. Snaith, Inorganic caesium lead iodide perovskite solar cells, J. Mater. Chem. A, 2015, 3, 19688–19695 RSC.
- Y. Zhao and K. Zhu, Organic–inorganic hybrid lead halide perovskites for optoelectronic and electronic applications, Chem. Soc. Rev., 2016, 45, 655–689 RSC.
- Y. Liu, Z. Yang and S. Liu, Recent progress in single-crystalline perovskite research including crystal preparation, property evaluation, and applications, Adv. Sci., 2018, 5, 1700471 CrossRef PubMed.
- C. Zhou, H. Lin, S. Lee, M. Chaaban and B. Ma, Organic–inorganic metal halide hybrids beyond perovskites, Mater. Res. Lett., 2018, 6, 552–569 CrossRef CAS.
- W. Wu, X. Shang, Z. Xu, H. Ye, Y. Yao, X. Chen, M. Hong, J. Luo and L. Li, Toward efficient two-photon circularly polarized light detection through cooperative strategies in chiral quasi-2D perovskites, Adv. Sci., 2023, 10, 2206070 CrossRef CAS PubMed.
- C. M. M. Soe, C. C. Stoumpos, M. Kepenekian, B. Traoré, H. Tsai, W. Nie, B. Wang, C. Katan, R. Seshadri, A. D. Mohite, J. Even, T. J. Marks and M. G. Kanatzidis, New type of 2D perovskites with alternating cations in the interlayer space, (C(NH2)3)(CH3NH3)nPbnI3n+1: Structure, properties, and photovoltaic performance, J. Am. Chem. Soc., 2017, 139, 16297–16309 CrossRef CAS PubMed.
- Z. Yuan, C. Zhou, Y. Tian, Y. Shu, J. Messier, J. C. Wang, L. J. van de Burgt, K. Kountouriotis, Y. Xin, E. Holt, K. Schanze, R. Clark, T. Siegrist and B. Ma, One-dimensional organic lead halide perovskites with efficient bluish white-light emission, Nat. Commun., 2017, 8, 14051 CrossRef CAS PubMed.
- L.-J. Feng, Y.-Y. Zhao, R.-Y. Song and X.-W. Lei, Three homologous 1D lead halide perovskites with broadband white-light emissions, Inorg. Chem. Commun., 2022, 136, 109146 CrossRef CAS.
- C. Zhou, H. Lin, H. Shi, Y. Tian, C. Pak, M. Shatruk, Y. Zhou, P. Djurovich, M.-H. Du and B. Ma, A Zero-dimensional organic seesaw-shaped tin bromide with highly efficient strongly Stokes-shifted deep-red emission, Angew. Chem., Int. Ed., 2018, 57, 1021–1024 CrossRef CAS PubMed.
- C. Zhou, Y. Tian, M. Wang, A. Rose, T. Besara, N. K. Doyle, Z. Yuan, J. C. Wang, R. Clark, Y. Hu, T. Siegrist, S. Lin and B. Ma, Low-dimensional organic tin bromide perovskites and their photoinduced structural transformation, Angew. Chem., Int. Ed., 2017, 56, 9018–9022 CrossRef CAS PubMed.
- Z. Xiao, W. Meng, J. Wang, D. B. Mitzi and Y. Yan, Searching for promising new perovskite-based photovoltaic absorbers: the importance of electronic dimensionality, Mater. Horizons, 2017, 4, 206–216 RSC.
- S. A. Kulkarni, N. Yantara, K. S. Tan, N. Mathews and S. G. Mhaisalkar, Perovskite nanostructures: Leveraging quantum effects to challenge optoelectronic limits, Mater. Today, 2020, 33, 122–140 CrossRef CAS.
- Y. Peng, X. Liu, L. Li, Y. Yao, H. Ye, X. Shang, X. Chen and J. Luo, Realization of vis–NIR dual-modal circularly polarized light detection in chiral perovskite bulk crystals, J. Am. Chem. Soc., 2021, 143, 14077–14082 CrossRef CAS PubMed.
- Y. Ai, X.-G. Chen, P.-P. Shi, Y.-Y. Tang, P.-F. Li, W.-Q. Liao and R.-G. Xiong, Fluorine substitution induced high Tc of enantiomeric perovskite ferroelectrics: (R)- and (S)-3-(Fluoropyrrolidinium)MnCl3, J. Am. Chem. Soc., 2019, 141, 4474–4479 CrossRef CAS PubMed.
- Y. Gao, E. Shi, S. Deng, S. B. Shiring, J. M. Snaider, C. Liang, B. Yuan, R. Song, S. M. Janke, A. Liebman-Peláez, P. Yoo, M. Zeller, B. W. Boudouris, P. Liao, C. Zhu, V. Blum, Y. Yu, B. M. Savoie, L. Huang and L. Dou, Molecular engineering of organic–inorganic hybrid perovskites quantum wells, Nat. Chem., 2019, 11, 1151–1157 CrossRef CAS PubMed.
- Y. Gao, Z. Wei, S.-N. Hsu, B. W. Boudouris and L. Dou, Two-dimensional halide perovskites featuring semiconducting organic building blocks, Mater. Chem. Front., 2020, 4, 3400–3418 RSC.
- Y. Peng, J. Bie, X. Liu, L. Li, S. Chen, W. Fa, S. Wang, Z. Sun and J. Luo, Acquiring high-TC layered metal halide ferroelectrics via cage-confined ethylamine rotators, Angew. Chem., Int. Ed., 2021, 60, 2839–2843 CrossRef CAS PubMed.
- H.-Y. Liu, H.-Y. Zhang, X.-G. Chen and R.-G. Xiong, Molecular design principles for ferroelectrics: Ferroelectrochemistry, J. Am. Chem. Soc., 2020, 142, 15205–15218 CrossRef CAS PubMed.
- M. Shao, T. Bie, L. Yang, Y. Gao, X. Jin, F. He, N. Zheng, Y. Yu and X. Zhang, Over 21% efficiency stable 2D perovskite solar cells, Adv. Mater., 2022, 34, 2107211 CrossRef CAS PubMed.
- B. R. Wygant, A. Z. Ye, A. Dolocan, Q. Vu, D. M. Abbot and C. B. Mullins, Probing the degradation chemistry and enhanced stability of 2D organolead halide perovskites, J. Am. Chem. Soc., 2019, 141, 18170–18181 CrossRef CAS PubMed.
- R. K. Ulaganathan, R. C. Murugesan, C.-Y. Lin, A. Subramanian, W.-L. Chen, Y.-M. Chang, A. Rozhin and R. Sankar, Stable formamidinium-based centimeter long two-dimensional lead halide perovskite single-crystal for long-life optoelectronic applications, Adv. Funct. Mater., 2022, 32, 2112277 CrossRef CAS.
- S. Park, W. J. Chang, C. W. Lee, S. Park, H.-Y. Ahn and K. T. Nam, Photocatalytic hydrogen generation from hydriodic acid using methylammonium lead iodide in dynamic equilibrium with aqueous solution, Nat. Energy, 2016, 2, 16185 CrossRef.
- P. C. K. Vesborg, Photocatalysis: HI-time for perovskites, Nat. Energy, 2017, 2, 16205 CrossRef.
- C. Bie, L. Wang and J. Yu, Challenges for photocatalytic overall water splitting, Chem, 2022, 8, 1567–1574 CAS.
- J. Schneidewind, M. A. Argüello Cordero, H. Junge, S. Lochbrunner and M. Beller, Two-photon, visible light water splitting at a molecular ruthenium complex, Energy Environ. Sci., 2021, 14, 4427–4436 RSC.
- C. Levy-Clement, A. Heller, W. A. Bonner and B. A. Parkinson, Spontaneous photoelectrolysis of HBr and
HI, J. Electrochem. Soc., 1982, 129, 1701 CrossRef CAS.
- J. H. Norman, K. J. Mysels, R. Sharp and D. Williamson, Studies of the sulfur-iodine thermochemical water-splitting cycle, Int. J. Hydrogen Energy, 1982, 7, 545–556 CrossRef CAS.
- H. Wang, H. Zhang, J. Wang, Y. Gao, F. Fan, K. Wu, X. Zong and C. Li, Mechanistic understanding of efficient photocatalytic H2 evolution on two-dimensional layered lead iodide hybrid perovskites, Angew. Chem., Int. Ed., 2021, 60, 7376–7381 CrossRef CAS PubMed.
- Y. Li, L. Li, S. She, S. Chen, Y. Liu, B. Xu, F.-W. Lee and X. Zhu, Multidimensional perovskite for visible light driven hydrogen production in aqueous HI solution, ACS Appl. Energy Mater., 2022, 5, 207–213 CrossRef CAS.
- H. Huang, D. Verhaeghe, B. Weng, B. Ghosh, H. Zhang, J. Hofkens, J. A. Steele and M. B. J. Roeffaers, Metal halide perovskite based heterojunction photocatalysts, Angew. Chem., Int. Ed., 2022, 61, e202203261 CrossRef CAS PubMed.
- V. Armenise, S. Colella, F. Fracassi and A. Listorti, Lead-free metal halide perovskites for hydrogen evolution from aqueous solutions, Nanomaterials, 2021, 11, 433 CrossRef CAS PubMed.
- Y. Wu, P. Wang, Z. Guan, J. Liu, Z. Wang, Z. Zheng, S. Jin, Y. Dai, M.-H. Whangbo and B. Huang, Enhancing the photocatalytic hydrogen evolution activity of mixed-halide perovskite CH3NH3PbBr3–xIx achieved by bandgap funneling of charge carriers, ACS Catal., 2018, 8, 10349–10357 CrossRef CAS.
- H. Zhao, Y. Li, B. Zhang, T. Xu and C. Wang, PtIx/[(CH3)2NH2]3[BiI6] as a well-dispersed photocatalyst for hydrogen production in hydroiodic acid, Nano Energy, 2018, 50, 665–674 CrossRef CAS.
- D. Ju, X. Zheng, J. Liu, Y. Chen, J. Zhang, B. Cao, H. Xiao, O. F. Mohammed, O. M. Bakr and X. Tao, Reversible band gap narrowing of Sn-based hybrid perovskite single crystal with excellent phase stability, Angew. Chem., Int. Ed., 2018, 57, 14868–14872 CrossRef CAS PubMed.
- Z. Zhao, J. Wu, Y.-Z. Zheng, N. Li, X. Li, Z. Ye, S. Lu, X. Tao and C. Chen, Stable hybrid perovskite MAPb(I1−xBrx)3 for photocatalytic hydrogen evolution, Appl. Catal., B, 2019, 253, 41–48 CrossRef CAS.
- Z. Guan, Y. Wu, P. Wang, Q. Zhang, Z. Wang, Z. Zheng, Y. Liu, Y. Dai, M.-H. Whangbo and B. Huang, Perovskite photocatalyst CsPbBr3-xIx with a bandgap funnel structure for H2 evolution under visible light, Appl. Catal., B, 2019, 245, 522–527 CrossRef CAS.
- M. Wang, Y. Zuo, J. Wang, Y. Wang, X. Shen, B. Qiu, L. Cai, F. Zhou, S. P. Lau and Y. Chai, Remarkably enhanced hydrogen generation of organolead halide perovskites via piezocatalysis and photocatalysis, Adv. Energy Mater., 2019, 9, 1901801 CrossRef CAS.
- K. Rokesh, M. Sakar and T.-O. Do, 2-(Aminomethyl pyridine)SbI5: An emerging visible-light driven organic–inorganic hybrid perovskite for photoelectrochemical and photocatalytic applications, Mater. Lett., 2019, 242, 99–102 CrossRef CAS.
- Y. Guo, G. Liu, Z. Li, Y. Lou, J. Chen and Y. Zhao, Stable lead-free (CH3NH3)3Bi2I9 perovskite for photocatalytic hydrogen generation, ACS Sustain. Chem. Eng., 2019, 7, 15080–15085 CrossRef CAS.
- G. Q. Chen, P. Wang, Y. Q. Wu, Q. Q. Zhang, Q. Wu, Z. Y. Wang, Z. K. Zheng, Y. Y. Liu, Y. Dai and B. B. Huang, Lead-free halide perovskite Cs3Bi2xSb2-2xI9(x ≈ 0.3) possessing the photocatalytic activity for hydrogen evolution comparable to that of (CH3NH3)PbI3, Adv. Mater., 2020, 32, 2001344 CrossRef CAS PubMed.
- F. Liu, M. Wang, X. Liu, B. Wang, C. Li, C. Liu, Z. Lin and F. Huang, A rapid and robust light-and-solution-triggered in situ crafting of organic passivating membrane over metal halide perovskites for markedly improved stability and photocatalysis, Nano Lett., 2021, 21, 1643–1650 CrossRef CAS PubMed.
- H. Yin, J. Chen, P. Guan, D. Zheng, Q. Kong, S. Yang, P. Zhou, B. Yang, T. Pullerits and K. Han, Controlling photoluminescence and photocatalysis activities in lead-free Cs2PtxSn1-xCl6 perovskites via ion substitution, Angew. Chem., Int. Ed., 2021, 60, 22693–22699 CrossRef CAS PubMed.
- H. Zhao, K. Chordiya, P. Leukkunen, A. Popov, M. Upadhyay Kahaly, K. Kordas and S. Ojala, Dimethylammonium iodide stabilized bismuth halide perovskite photocatalyst for hydrogen evolution, Nano Res., 2021, 14, 1116–1125 CrossRef CAS.
- Z. He, Q. Tang, X. Liu, X. Yan, K. Li and D. Yue, Lead-free Cs2AgBiBr6 perovskite with enriched surface defects for efficient photocatalytic hydrogen evolution, Energy Fuels, 2021, 35, 15005–15009 CrossRef CAS.
- P. Zhou, H. Chen, Y. G. Chao, Q. H. Zhang, W. Y. Zhang, F. Lv, L. Gu, Q. Zhao, N. Wang, J. S. Wang and S. J. Guo, Single-atom Pt-I3 sites on all-inorganic Cs2SnI6 perovskite for efficient photocatalytic hydrogen production, Nat. Commun., 2021, 12, 4412 CrossRef CAS PubMed.
- Y. Q. Wu, Q. Wu, Q. Q. Zhang, Z. Z. Lou, K. F. Liu, Y. D. Ma, Z. Y. Wang, Z. K. Zheng, H. F. Cheng, Y. Y. Liu, Y. Dai, B. B. A. Huang and P. Wang, An organometal halide perovskite supported Pt single-atom photocatalyst for H2 evolution, Energy Environ. Sci., 2022, 15, 1271–1281 RSC.
- Y. Q. Tang, C. H. Mak, C. Wang, Y. Fu, F. F. Li, G. H. Jia, C. W. Hsieh, H. H. Shen, J. C. Colmenares, H. S. Song, M. J. Yuan, Y. Chen and H. Y. Hsu, Bandgap funneling in bismuth-based hybrid perovskite photocatalyst with efficient visible-light-driven hydrogen evolution, Small Methods, 2022, 6, 2200326 CrossRef CAS PubMed.
- Y. L. Ji, M. Y. She, X. Bai, E. Z. Liu, W. H. Xue, Z. Zhang, K. R. Wan, P. Liu, S. Y. Zhang and J. L. Li, In-depth understanding of the effect of halogen-induced stable 2D bismuth-based perovskites for photocatalytic hydrogen evolution activity, Adv. Funct. Mater., 2022, 32, 2201721 CrossRef CAS.
- C. X. Bao, J. Yang, S. Bai, W. D. Xu, Z. B. Yan, Q. Y. Xu, J. M. Liu, W. J. Zhang and F. Gao, High performance and stable all-inorganic metal halide perovskite-based photodetectors for optical communication applications, Adv. Mater., 2018, 30, 1803422 CrossRef PubMed.
- C. Liu, Y. Yang, O. A. Syzgantseva, Y. Ding, M. A. Syzgantseva, X. F. Zhang, A. M. Asiri, S. Y. Dai and M. K. Nazeeruddin, α-CsPbI3 bilayers via one-step deposition for efficient and stable all-inorganic perovskite solar cells, Adv. Mater., 2020, 32, 2002632 CrossRef CAS PubMed.
- A. A. Zhumekenov, M. I. Saidaminov, M. A. Haque, E. Alarousu, S. P. Sarmah, B. Murali, I. Dursun, X.-H. Miao, A. L. Abdelhady, T. Wu, O. F. Mohammed and O. M. Bakr, Formamidinium lead halide perovskite crystals with unprecedented long carrier dynamics and diffusion length, ACS Energy Lett., 2016, 1, 32–37 CrossRef CAS.
- B.-W. Park, B. Philippe, X. Zhang, H. Rensmo, G. Boschloo and E. M. J. Johansson, Bismuth based hybrid perovskites A3Bi2I9 (A: Methylammonium or cesium) for solar cell application, Adv. Mater., 2015, 27, 6806–6813 CrossRef CAS PubMed.
- W. Zhang, M. Hong and J. Luo, Halide double perovskite ferroelectrics, Angew. Chem., Int. Ed., 2020, 59, 9305–9308 CrossRef CAS PubMed.
- F. Jiang, D. Yang, Y. Jiang, T. Liu, X. Zhao, Y. Ming, B. Luo, F. Qin, J. Fan, H. Han, L. Zhang and Y. Zhou, Chlorine-incorporation-induced formation of the layered phase for antimony-based lead-Free perovskite solar cells, J. Am. Chem. Soc., 2018, 140, 1019–1027 CrossRef CAS PubMed.
- M. Liu, Y. Chen, J. Su, J. Shi, X. Wang and L. Guo, Photocatalytic hydrogen production using twinned nanocrystals and an unanchored NiSx co-catalyst, Nat. Energy, 2016, 1, 16151 CrossRef CAS.
- Y. Yang, C. Liu, M. L. Cai, Y. J. Liao, Y. Ding, S. Ma, X. P. Liu, M. Guli, S. Y. Dai and M. K. Nazeeruddin, Dimension-controlled growth of antimony-based perovskite-like halides for lead-free and semitransparent photovoltaics, ACS Appl. Mater. Interfaces, 2020, 12, 17062–17069 CrossRef CAS PubMed.
- X. Meng, S. Wang, C. Zhang, C. Dong, R. Li, B. Li, Q. Wang and Y. Ding, Boosting hydrogen evolution performance of a CdS-based photocatalyst: In situ transition from type I to type II heterojunction during photocatalysis, ACS Catal., 2022, 12, 10115–10126 CrossRef CAS.
- H. Cai, B. Wang, L. Xiong, J. Bi, L. Yuan, G. Yang and S. Yang, Orienting the charge transfer path of type-II heterojunction for photocatalytic hydrogen evolution, Appl. Catal., B, 2019, 256, 117853 CrossRef CAS.
- B.-J. Ng, L. K. Putri, X. Y. Kong, Y. W. Teh, P. Pasbakhsh and S.-P. Chai, Z-Scheme photocatalytic systems for solar water splitting, Adv. Sci., 2020, 7, 1903171 CrossRef CAS PubMed.
- X. Wang, H. Wang, H. Zhang, W. Yu, X. Wang, Y. Zhao, X. Zong and C. Li, Dynamic interaction between methylammonium lead iodide and TiO2 nanocrystals leads to enhanced photocatalytic H2 evolution from HI splitting, ACS Energy Lett., 2018, 3, 1159–1164 CrossRef CAS.
- M. V. Pavliuk, M. Abdellah and J. Sá, Hydrogen evolution with CsPbBr3 perovskite nanocrystals under visible light in solution, Mater. Today Commun., 2018, 16, 90–96 CrossRef CAS.
- Y. Wu, P. Wang, X. Zhu, Q. Zhang, Z. Wang, Y. Liu, G. Zou, Y. Dai, M.-H. Whangbo and B. Huang, Composite of CH3NH3PbI3 with reduced graphene oxide as a highly efficient and stable visible-light photocatalyst for hydrogen evolution in aqueous HI solution, Adv. Mater., 2018, 30, 1704342 CrossRef PubMed.
- R. Li, X. Li, J. Wu, X. Lv, Y.-Z. Zheng, Z. Zhao, X. Ding, X. Tao and J.-F. Chen, Few-layer black phosphorus-on-MAPbI3 for superb visible-light photocatalytic hydrogen evolution from HI splitting, Appl. Catal., B, 2019, 259, 118075 CrossRef CAS.
- Z. Zhao, J. Wu, Y.-Z. Zheng, N. Li, X. Li and X. Tao, Ni3C-decorated MAPbI3 as visible-light photocatalyst for H2 evolution from HI splitting, ACS Catal., 2019, 9, 8144–8152 CrossRef CAS.
- H. Wang, X. Wang, R. Chen, H. Zhang, X. Wang, J. Wang, J. Zhang, L. Mu, K. Wu, F. Fan, X. Zong and C. Li, Promoting photocatalytic H2 evolution on organic–inorganic hybrid perovskite nanocrystals by simultaneous dual-charge transportation modulation, ACS Energy Lett., 2019, 4, 40–47 CrossRef CAS.
- T. Wang, D. Yue, X. Li and Y. Zhao, Lead-free double perovskite Cs2AgBiBr6/RGO composite for efficient visible light photocatalytic H2 evolution, Appl. Catal., B, 2020, 268, 118399 CrossRef CAS.
- B. M. Bresolin, P. Sgarbossa, D. W. Bahnemann and M. Sillanpaa, Cs3Bi2I9/g-C3N4 as a new binary photocatalyst for efficient visible-light photocatalytic processes, Sep. Purif. Technol., 2020, 251, 117320 CrossRef CAS.
- G. N. Liu, R. Y. Zhao, B. Xu, Y. Sun, X. M. Jiang, X. Hu and C. Li, Design, synthesis, and photocatalytic application of moisture-stable hybrid lead-free perovskite, ACS Appl. Mater. Interfaces, 2020, 12, 54694–54702 CrossRef CAS PubMed.
- Y. Zhao, Q. Zeng, Y. Yu, T. Feng, Y. Zhao, Z. Wang, Y. Li, C. Liu, J. Liu, H. Wei, S. Zhu, Z. Kang, H. Zhang and B. Yang, Enhanced charge separation and photocatalytic hydrogen evolution in carbonized-polymer-dot-coupled lead halide perovskites, Mater. Horizons, 2020, 7, 2719–2725 RSC.
- F. Wang, X. Liu, Z. Zhang and S. Min, A noble-metal-free MoS2 nanosheet-coupled MAPbI3 photocatalyst for efficient and stable visible-light-driven hydrogen evolution, Chem. Commun., 2020, 56, 3281–3284 RSC.
- Y. Tang, C. H. Mak, R. Liu, Z. Wang, L. Ji, H. Song, C. Tan, F. Barrière and H. Y. Hsu, In situ formation of bismuth-based perovskite heterostructures for high-performance cocatalyst-free photocatalytic hydrogen evolution, Adv. Funct. Mater., 2020, 30, 2006919 CrossRef CAS.
- C. Cai, Y. Teng, J. H. Wu, J. Y. Li, H. Y. Chen, J. H. Chen and D. B. Kuang, In situ photosynthesis of an MAPbI3/CoP hybrid heterojunction for efficient photocatalytic hydrogen evolution, Adv. Funct. Mater., 2020, 30, 2001478 CrossRef CAS.
- X. Zhao, S. Chen, H. Yin, S. Jiang, K. Zhao, J. Kang, P. F. Liu, L. Jiang, Z. Zhu, D. Cui, P. Liu, X. Han, H. G. Yang and H. Zhao, Perovskite microcrystals with intercalated monolayer MoS2 nanosheets as advanced photocatalyst for solar-powered hydrogen generation, Matter, 2020, 3, 935–949 CrossRef.
- Y. Zhang, J. Shi, X. Ding, J. Wu, Y.-Z. Zheng and X. Tao, Stable mixed-organic-cation perovskite MA1–xFAxPbI3 integrated with MoS2 for enhanced visible-light photocatalytic H2 evolution, Ind. Eng. Chem. Res., 2020, 59, 20667–20675 CrossRef CAS.
- L. Romani, A. Speltini, F. Ambrosio, E. Mosconi, A. Profumo, M. Marelli, S. Margadonna, A. Milella, F. Fracassi, A. Listorti, F. De Angelis and L. Malavasi, Water-stable DMASnBr3 lead-free perovskite for effective solar-driven photocatalysis, Angew. Chem., Int. Ed., 2021, 60, 3611–3618 CrossRef CAS PubMed.
- L. Jiang, Y. Guo, S. Qi, K. Zhang, J. Chen, Y. Lou and Y. Zhao, Amorphous NiCoB-coupled MAPbI3 for efficient photocatalytic hydrogen evolution, Dalton Trans., 2021, 50, 17960–17966 RSC.
- L. Romani, A. Speltini, C. N. Dibenedetto, A. Listorti, F. Ambrosio, E. Mosconi, A. Simbula, M. Saba, A. Profumo, P. Quadrelli, F. De Angelis and L. Malavasi, Experimental strategy and mechanistic view to boost the photocatalytic activity of Cs3Bi2Br9 lead-free perovskite derivative by g-C3N4 composite engineering, Adv. Funct. Mater., 2021, 31, 2104428 CrossRef CAS.
- W. Guan, Y. Li, Q. Zhong, H. Liu, J. Chen, H. Hu, K. Lv, J. Gong, Y. Xu, Z. Kang, M. Cao and Q. Zhang, Fabricating MAPbI3/MoS2 composites for improved photocatalytic performance, Nano Lett., 2021, 21, 597–604 CrossRef CAS PubMed.
- Y. Jiang, K. Li, X. Wu, M. Zhu, H. Zhang, K. Zhang, Y. Wang, K. P. Loh, Y. Shi and Q. H. Xu, In situ synthesis of lead-free halide perovskite Cs2AgBiBr6 supported on nitrogen-doped carbon for efficient hydrogen evolution in aqueous HBr solution, ACS Appl. Mater. Interfaces, 2021, 13, 10037–10046 CrossRef CAS PubMed.
- W. B. Li, F. Wang, Z. G. Zhang, X. H. Ma and S. X. Min, Coupling of MAPbI3 microcrystals with conductive polyaniline for efficient visible-light-driven H2 evolution, Sustain, Energy Fuels, 2021, 6, 76–80 Search PubMed.
- Y. Zhang, Z. Sun, Z. Wang, Y. Zang and X. Tao, Efficient and long-term photocatalytic H2 evolution stability enabled by Cs2AgBiBr6/MoS2 in aqueous HBr solution, Int. J. Hydrogen Energy, 2022, 47, 8829–8840 CrossRef CAS.
- S. Feng, S. Ning, L. Wang, J. Zhao, J. Ou, Z. Wu, S. Luo, Z. Lin, K. Yan, C. Wu and Y. Xu, Modifying CsPbX3 (X = Cl, Br, I) with a zeolitic imidazolate framework through mechanical milling for aqueous photocatalytic H2 evolution, ACS Appl. Energy Mater., 2022, 5, 6248–6255 CrossRef CAS.
- K. Song, J. Gou, L. Yang and C. Zeng, Environmentally stable mesoporous g-C3N4 modified lead-free double perovskite Cs2AgBiBr6 for highly efficient photocatalytic hydrogen evolution, Catal. Lett., 2023, 153, 534–543 CrossRef CAS.
- Q. Huang, Y. M. Guo, J. X. Chen, Y. B. Lou and Y. X. Zhao, NiCoP modified lead-free double perovskite Cs2AgBiBr6 for efficient photocatalytic hydrogen generation, New J. Chem., 2022, 46, 7395–7402 RSC.
- T. Chen, M. Li, L. Shen, M. B. J. Roeffaers, B. Weng, H. Zhu, Z. Chen, D. Yu, X. Pan, M. Q. Yang and Q. Qian, Photocatalytic anaerobic oxidation of aromatic alcohols coupled with H2 production over CsPbBr3/GO-Pt catalysts, Front. Chem., 2022, 10, 833784 CrossRef CAS PubMed.
- Q. Xu, L. Zhang, B. Cheng, J. Fan and J. Yu, S-scheme heterojunction photocatalyst, Chem, 2020, 6, 1543–1559 CAS.
- H. Li, S. Tao, S. Wan, G. Qiu, Q. Long, J. Yu and S. Cao, S-scheme heterojunction of ZnCdS nanospheres and dibenzothiophene modified graphite carbon nitride for enhanced H2 production, Chin. J. Catal., 2023, 46, 167–176 CrossRef CAS.
- C. Cheng, J. Zhang, B. Zhu, G. Liang, L. Zhang and J. Yu, Verifying the charge-transfer mechanism in S-scheme heterojunctions using femtosecond transient absorption spectroscopy, Angew. Chem., Int. Ed., 2023, 62, e202218688 CrossRef CAS PubMed.
- F. Zhao, Y. L. Law, N. Zhang, X. Wang, W. Wu, Z. Luo and Y. Wang, Constructing spatially separated cage-like Z-scheme heterojunction photocatalyst for enhancing photocatalytic H2 evolution, Small, 2023, 19, 2208266 CrossRef CAS PubMed.
- Z. Long, X. Yang, X. Huo, X. Li, Q. Qi, X. Bian, Q. Wang, F. Yang, W. Yu and L. Jiang, Bioinspired Z-scheme In2O3/C3N4 heterojunctions with tunable nanorod lengths for enhanced photocatalytic
hydrogen evolution, Chem. Eng. J., 2023, 461, 141893 CrossRef CAS.
- H. Zhou, Q. Chen, G. Li, S. Luo, T.-B. Song, H.-S. Duan, Z. Hong, J. You, Y. Liu and Y. Yang, Interface engineering of highly efficient perovskite solar cells, Science, 2014, 345, 542–546 CrossRef CAS PubMed.
- N. J. Jeon, H. G. Lee, Y. C. Kim, J. Seo, J. H. Noh, J. Lee and S. I. Seok,
o-Methoxy substituents in spiro-OMeTAD for efficient inorganic–organic hybrid perovskite solar cells, J. Am. Chem. Soc., 2014, 136, 7837–7840 CrossRef CAS PubMed.
- M. B. Zakaria, C. Li, Q. Ji, B. Jiang, S. Tominaka, Y. Ide, J. P. Hill, K. Ariga and Y. Yamauchi, Self-construction from 2D to 3D: One-pot layer-by-layer assembly of graphene oxide sheets held together by coordination polymers, Angew. Chem., Int. Ed., 2016, 55, 8426–8430 CrossRef CAS PubMed.
- H. Zhang, Z. Luo, Y. Liu and Y. Jiang, Noble-metal-free Ni3C as co-catalyst on LaNiO3 with enhanced photocatalytic activity, Appl. Catal., B, 2020, 277, 119166 CrossRef CAS.
- R. C. Shen, K. L. He, A. P. Zhang, N. Li, Y. H. Ng, P. Zhang, J. Hu and X. Li, In-situ construction of metallic Ni3C@Ni core-shell cocatalysts over g-C3N4 nanosheets for shell-thickness-dependent photocatalytic H2 production, Appl. Catal., B, 2021, 291, 120104 CrossRef CAS.
- M. Sun, H. Liu, J. Qu and J. Li, Earth-Rich transition metal phosphide for energy conversion and storage, Adv. Energy Mater., 2016, 6, 1600087 CrossRef.
- S. Cao, C.-J. Wang, W.-F. Fu and Y. Chen, Metal phosphides as co-catalysts for photocatalytic and photoelectrocatalytic water splitting, ChemSusChem, 2017, 10, 4306–4323 CrossRef CAS PubMed.
- F. Zhang, J. Zhang, J. Li, X. Jin, Y. Li, M. Wu, X. Kang, T. Hu, X. Wang, W. Ren and G. Zhang, Modulating charge transfer dynamics for g-C3N4 through a dimension and interface engineered transition metal phosphide co-catalyst for efficient visible-light photocatalytic hydrogen generation, J. Mater. Chem. A, 2019, 7, 6939–6945 RSC.
- Y. Liu, X. Li, Q. Zhang, W. Li, Y. Xie, H. Liu, L. Shang, Z. Liu, Z. Chen, L. Gu, Z. Tang, T. Zhang and S. Lu, A general route to prepare low-ruthenium-content bimetallic electrocatalysts for pH-universal hydrogen evolution reaction by using carbon quantum dots, Angew. Chem., Int. Ed., 2020, 59, 1718–1726 CrossRef CAS PubMed.
- M. Han, C. Y. Kang, Z. X. Qu, S. J. Zhu and B. Yang, Surface molecule induced effective light absorption and charge transfer for H2 production photocatalysis in a carbonized polymer dots-carbon nitride system, Appl. Catal., B, 2022, 305, 121064 CrossRef CAS.
- Z. H. Pan, T. Hisatomi, Q. Wang, S. S. Chen, A. Iwase, M. Nakabayashi, N. Shibata, T. Takata, M. Katayama, T. Minegishi, A. Kudo and K. Domen, Photoreduced graphene oxide as a conductive binder to improve the water splitting activity of photocatalyst sheets, Adv. Funct. Mater., 2016, 26, 7011–7019 CrossRef CAS.
- H. L. Tan, H. A. Tahini, X. Wen, R. J. Wong, X. Tan, A. Iwase, A. Kudo, R. Amal, S. C. Smith and Y. H. Ng, Interfacing BiVO4 with reduced graphene oxide for enhanced photoactivity: A tale of facet dependence of electron shuttling, Small, 2016, 12, 5295–5302 CrossRef CAS PubMed.
- D. Mateo, I. Esteve-Adell, J. Albero, J. F. S. Royo, A. Primo and H. Garcia, 111 oriented gold nanoplatelets on multilayer graphene as visible light photocatalyst for overall water splitting, Nat. Commun., 2016, 7, 11819 CrossRef PubMed.
- Y. Li, Z. Yin, G. Ji, Z. Liang, Y. Xue, Y. Guo, J. Tian, X. Wang and H. Cui, 2D/2D/2D heterojunction of Ti3C2 MXene/MoS2 nanosheets/TiO2 nanosheets with exposed (001) facets toward enhanced photocatalytic hydrogen production activity, Appl. Catal., B, 2019, 246, 12–20 CrossRef CAS.
- B. J. Sun, W. Zhou, H. Z. Li, L. P. Ren, P. Z. Qiao, W. Li and H. G. Fu, Synthesis of particulate hierarchical tandem heterojunctions toward optimized photocatalytic hydrogen production, Adv. Mater., 2018, 30, 1804282 CrossRef PubMed.
- C. B. Bie, B. C. Zhu, L. X. Wang, H. G. Yu, C. H. Jiang, T. Chen and J. G. Yu, A bifunctional CdS/MoO2/MoS2 catalyst enhances photocatalytic H2 evolution and pyruvic acid synthesis, Angew. Chem., Int. Ed., 2022, 61, e2022120 CrossRef PubMed.
- Y. Lei, M. Yang, J. Hou, F. Wang, E. Cui, C. Kong and S. Min, Thiomolybdate [Mo3S13]2− nanocluster: a molecular mimic of MoS2 active sites for highly efficient photocatalytic hydrogen evolution, Chem. Commun., 2018, 54, 603–606 RSC.
- J. Shi, R. Tong, X. Zhou, Y. Gong, Z. Zhang, Q. Ji, Y. Zhang, Q. Fang, L. Gu, X. Wang, Z. Liu and Y. Zhang, Temperature-mediated selective growth of MoS2/WS2 and WS2/MoS2 vertical stacks on Au foils for direct photocatalytic applications, Adv. Mater., 2016, 28, 10664–10672 CrossRef CAS PubMed.
- H. He, J. Lin, W. Fu, X. Wang, H. Wang, Q. Zeng, Q. Gu, Y. Li, C. Yan, B. K. Tay, C. Xue, X. Hu, S. T. Pantelides, W. Zhou and Z. Liu, MoS2/TiO2 edge-on heterostructure for efficient photocatalytic hydrogen evolution, Adv. Energy Mater., 2016, 6, 1600464 CrossRef.
- T. F. Jaramillo, K. P. Jørgensen, J. Bonde, J. H. Nielsen, S. Horch and I. Chorkendorff, Identification of active edge sites for electrochemical H2 evolution from MoS2 nanocatalysts, Science, 2007, 317, 100–102 CrossRef CAS PubMed.
- H. I. Karunadasa, E. Montalvo, Y. Sun, M. Majda, J. R. Long and C. J. Chang, A molecular MoS2 edge site mimic for catalytic hydrogen generation, Science, 2012, 335, 698–702 CrossRef CAS PubMed.
- J. S. Cai, J. Y. Huang, S. C. Wang, J. Iocozzia, Z. T. Sun, J. Y. Sun, Y. K. Yang, Y. K. Lai and Z. Q. Lin, Crafting mussel-inspired metal nanoparticle-decorated ultrathin graphitic carbon nitride for the degradation of chemical pollutants and production of chemical resources, Adv. Mater., 2019, 31, 1806314 CrossRef PubMed.
- F. Xue, Y. T. Si, C. Cheng, W. L. Fu, X. Y. Chen, S. H. Shen, L. Z. Wang and M. C. Liu, Electron transfer via homogeneous phosphorus bridges enabling boosted photocatalytic generation of H2 and H2O2 from pure water with stoichiometric ratio, Nano Energy, 2022, 103, 107799 CrossRef CAS.
- F. Lin, S. Zhou, G. H. Wang, J. Wang, T. Y. Gao, Y. R. Su and C. P. Wong, Electrostatic self-assembly combined with microwave hydrothermal strategy: construction of 1D/1D carbon nanofibers/crystalline g-C3N4 heterojunction for boosting photocatalytic hydrogen production, Nano Energy, 2022, 99, 107432 CrossRef CAS.
- Z. P. Wang, Z. L. Wang, X. D. Zhu, C. Z. Ai, Y. M. Zeng, W. Y. Shi, X. D. Zhang, H. R. Zhang, H. W. Si, J. Li, C. Z. Wang and S. W. Lin, Photodepositing CdS on the active cyano groups decorated g-C3N4 in Z-scheme manner promotes visible-light-driven hydrogen evolution, Small, 2021, 17, 2102699 CrossRef CAS PubMed.
- H. Yu, L. Shang, T. Bian, R. Shi, G. I. N. Waterhouse, Y. Zhao, C. Zhou, L.-Z. Wu, C.-H. Tung and T. Zhang, Nitrogen-doped porous carbon nanosheets templated from g-C3N4 as metal-free electrocatalysts for efficient oxygen reduction reaction, Adv. Mater., 2016, 28, 5080–5086 CrossRef CAS PubMed.
- Q. Zhang, S. Huang, J. Deng, D. T. Gangadharan, F. Yang, Z. Xu, G. Giorgi, M. Palummo, M. Chaker and D. Ma, Ice-assisted synthesis of black phosphorus nanosheets as a metal-free photocatalyst: 2D/2D heterostructure for broadband H2 evolution, Adv. Funct. Mater., 2019, 29, 1902486 CrossRef.
- M. Z. Rahman, C. W. Kwong, K. Davey and S. Z. Qiao, 2D phosphorene as a water splitting photocatalyst: fundamentals to applications, Energy Environ. Sci., 2016, 9, 709–728 RSC.
- R. Yan, T. Ma, M. Cheng, X. Tao, Z. Yang, F. Ran, S. Li, B. Yin, C. Cheng and W. Yang, Metal–organic-framework-derived nanostructures as multifaceted electrodes in metal–sulfur batteries, Adv. Mater., 2021, 33, 2008784 CrossRef CAS PubMed.
- A. Dhakshinamoorthy, A. M. Asiri and H. Garcia, 2D Metal–organic frameworks as multifunctional materials in heterogeneous catalysis and electro/photocatalysis, Adv. Mater., 2019, 31, 1900617 CrossRef CAS PubMed.
- X. Tao, Y. Zhao, S. Wang, C. Li and R. Li, Recent advances and perspectives for solar-driven water splitting using particulate photocatalysts, Chem. Soc. Rev., 2022, 51, 3561–3608 RSC.
- Y. Zhang and N.-G. Park, Quasi-two-dimensional perovskite solar cells with efficiency exceeding 22%, ACS Energy Lett., 2022, 7, 757–765 CrossRef CAS.
- G. Liao, C. Li, S.-Y. Liu, B. Fang and H. Yang, Z-scheme systems: From fundamental principles to characterization, synthesis, and photocatalytic fuel-conversion applications, Phys. Rep., 2022, 983, 1–41 CrossRef CAS.
- F. Bella, G. Griffini, J.-P. Correa-Baena, G. Saracco, M. Grätzel, A. Hagfeldt, S. Turri and C. Gerbaldi, Improving efficiency and stability of perovskite solar cells with photocurable fluoropolymers, Science, 2016, 354, 203–206 CrossRef CAS PubMed.
- Y. Kim, H. Kim, S. Graham, A. Dyer and J. R. Reynolds, Durable polyisobutylene edge sealants for organic electronics and electrochemical devices, Sol. Energy Mater. Sol. Cells, 2012, 100, 120–125 CrossRef CAS.
- J. Li, R. Xia, W. Qi, X. Zhou, J. Cheng, Y. Chen, G. Hou, Y. Ding, Y. Li, Y. Zhao and X. Zhang, Encapsulation of perovskite solar cells for enhanced stability: Structures, materials and characterization, J. Power Sources, 2021, 485, 229313 CrossRef CAS.
- Z. Huang, J. Long, R. Dai, X. Hu, L. Le, X. Meng, L. Tan and Y. Chen, Ultra-flexible and waterproof perovskite photovoltaics for washable power source applications, Chem. Commun., 2021, 57, 6320–6323 RSC.
- Q. Zhang, S. Liu, M. He, W. Zheng, Q. Wan, M. Liu, X. Liao, W. Zhan, C. Yuan, J. Liu, H. Xie, X. Guo, L. Kong and L. Li, Stable lead-free tin halide perovskite with operational stability > 1200
h by suppressing Tin(II) oxidation, Angew. Chem., Int. Ed., 2022, 61, e202205463 CrossRef CAS PubMed.
- Z. Xiao, Z. Song and Y. Yan, From lead halide perovskites to lead-free metal halide perovskites and perovskite derivatives, Adv. Mater., 2019, 31, 1803792 CrossRef CAS PubMed.
|
This journal is © the Partner Organisations 2023 |